- 1Department of Computer and Information Sciences, Northumbria University, Newcastle upon Tyne, United Kingdom
- 2Cumbria, Northumberland Tyne and Wear NHS Foundation Trust, Wolfson Research Centre, Campus for Ageing and Vitality, Newcastle upon Tyne, United Kingdom
- 3Department of Nursing, Midwifery and Health, Northumbria University, Newcastle upon Tyne, United Kingdom
- 4Northumbria Healthcare NHS Foundation Trust, Newcastle upon Tyne, United Kingdom
- 5Department of Sport, Exercise and Rehabilitation, Northumbria University, Newcastle upon Tyne, United Kingdom
1 Introduction
The advancement of wearable technologies (wearables) and their use in neurosciences signifies a new period filled with many possibilities and great potential (Byrom et al., 2018). Contemporary wearables incorporate a range of sensing modalities including but not limited to inertial measurement units (IMUs i.e., accelerometer and gyroscope), electroencephalogram (EEG), and functional near-infrared spectroscopy (fNIRS) which can provide high-resolution multimodal data to monitor significant (digital) clinical-based biomarkers or functional/performance outcomes pertaining to domains of interests within neuroscience. Notable examples include walking (i.e., gait performance) while simultaneously examining electrical brain activity or concentration changes of oxygenated and deoxygenated hemoglobin following neuronal activation (Stuart et al., 2018). Digital biomarkers or performance outcomes arising from wearables could aid a better understanding of neurological conditions like Parkinson's Disease (PD), epilepsy, or stroke during routine functional tasks (like walking) to get a better insight into real-world challenges and incidents arising from home and/or community-based activities. Moreover, integrating multi-modal wearable data (e.g., environmental) could enhance the understanding of real-world challenges for those with a neurological disorder (Johnson and Picard, 2020) e.g., unstable gait leading to near falls or falls during free-living walking (Warmerdam et al., 2020; Moore et al., 2023).
1.1 Going beyond the clinic
Traditionally, the evaluation of motor impairments in PD is often performed through visual observation by a clinician. However, that process is significantly dependent on the experience and subjective opinion of the clinician. To curb subjectivity, the use of specialized equipment, such as instrumented walkways has been adopted to obtain objective high-resolution data and greater insights. Yet, regardless of the effectiveness of the objective measures that process necessitates patients to travel to bespoke facilities, which can be inconvenient, costly, and physically challenging for those with mobility impairments (Syed et al., 2013). For a more decentralized approach, home motion systems (e.g., Microsoft Kinect) that include cameras, infrared, and radar-based devices have been utilized (Wang et al., 2014) but are costly and have limited widespread use (Demiris et al., 2009; Stone and Skubic, 2013). Accordingly, the need for low-cost/cost-effective technology became apparent. Achieving an economically decentralized approach would have added benefits of empowering patients to use these devices at home which in turn could ease the strain on healthcare systems to foster individualized care and independent living (Chen et al., 2023). Equally, the onus is no longer completely on the restrictive operational hours of clinics or the availability of medical professionals.
Wearables can provide a cost-effective and empowering approach for monitoring and rehabilitation beyond the clinic. They enable real-time assessment, potentially enabling healthcare providers to e.g., remotely monitor daily or weekly progress during rehabilitation exercises. Not only could wearables make rehabilitation more accessible by better accessing remote geographical areas, but they could also enable the personalization of treatment plans based on precise data tracking. However, the global push for technological integration and societal acceptance is an essential precondition for its widespread acceptance. Here, we broadly examine the technology-based challenges facing the use of wearables by care delivery teams or those working in clinical trials within neuroscience. It is worth noting that real-world deployment of wearables in care delivery and clinical trial settings face different regulatory pathways and the reader is directed elsewhere for those topics (Vasudevan et al., 2022; Izmailova et al., 2023; Leyens et al., 2024).
2 Clinical challenges
2.1 Validation
For wearables to be used consistently and reliably they must have a clearly defined contribution to improving clinical decisions while not dramatically increasing workload. For consistency and reliability, academic-based literature with robust evidence to show effectiveness in quantifying a physiological response is required. For example, quantifying gait in PD has emerged as a potential outcome to inform diagnosis and disease progression (Zhu et al., 2022). Validation of gait outcomes to known gold standard references is a fundamental must and/or investigation to determine any absolute differences due to any technology-based discrepancies between device comparison (Del Din et al., 2016). Recently, guidance on a flexible framework to inform clinical validation has been presented (Goldsack et al., 2020). In short, those creating, and using new wearables must follow proposed frameworks to show clinical effectiveness in their cohort of interest to convince associated healthcare professionals that adopting the technology has a real-world positive effect for their patients. Moreover, adoption of any wearable can only be truly achieved if it does not add extra burden to the healthcare professional to undertake patient assessments in what might be very time-limited meetings.
2.2 Perceptions and the future: education and upskilling
The successful trajectory of wearables within neurosciences is intertwined with perceptions and understanding (Karahanoğlu and Erbuğ, 2011). As with any technology, its widespread acceptance is contingent upon trust and comprehension of its functionalities. It's not solely about the technological proficiency of these devices but also about how their usability is determined by the public (Lee and Lee, 2020). For example, older adults often grapple with digital illiteracy and present a layer of complexity in this landscape (Wu et al., 2015). A well-informed public can discern the transformative capabilities of wearables, recognizing their potential to revolutionize healthcare, enhance daily life, and potentially unlock deeper health insights. Conversely, misconceptions or unwarranted fears, often stemming from a lack of understanding or misinformation, could hinder adoption (Cheung et al., 2019). Apprehensions may include privacy concerns, potential health implications, or even societal implications of widespread patient monitoring (Awotunde et al., 2021). To effectively engage older adults resistant to technology, a more personalized approach is required. For example, Xie (2011) proposed an initial assessment of technological skills to identify specific knowledge gaps and areas of discomfort. Following this assessment, training programs are tailored to the individual's needs and interests, focusing on practical technology applications they find engaging and relevant. To foster a conducive environment for the growth of wearable neurotech, proactive steps must be taken to educate where educational campaigns could play an important role. Leveraging various media platforms, from traditional outlets like television and newspapers to digital platforms such as social media and podcasts, could reach a diverse audience. Interactive workshops could offer hands-on experiences, allowing individuals to familiarize themselves with the technology (Yang et al., 2022). Furthermore, open dialogues in the form of talks at local community centers and societies or webinars can provide a platform for experts to address questions, dispel myths, and engage in constructive discussions about the future of wearables in healthcare (Shegog et al., 2020). However, public education is just one facet of the equation. As wearables become more integrated into healthcare and daily living, professionals interfacing with them may often require specialized training, so they can adequately inform patients how to use them in their homes (Bruno et al., 2018). Healthcare providers must be adept at interpreting arising data and understanding the application for altering patient care (Hilty et al., 2021). Caregivers could also need training, such as through video tutorials, to ensure optimal benefits for those in their care (Bruno et al., 2018; Li et al., 2019).
Upskilling should also extend to future healthcare professionals. Higher education institutions are beginning to employ the use of digital learning tools such as games, podcasts, e-compendiums, massive open online courses (MOOCs), and simulations. Those tools aim to produce better-skilled healthcare professionals, supplementing conventional teaching approaches with indispensable training in utilizing contemporary technologies that enhance patient care (Foss and Haraldseid, 2014). However, more adoption and integration into curricula is required to ensure the next generation of healthcare professionals is adept at dealing with a wearable revolution (Grimwood and Snell, 2020). It is essential that the future workforce is accustomed to the technology and what it is providing. That includes learning intricate functionalities and implications of wearables and how they can effectively utilize data (Brice and Almond, 2020). It is imperative they also have an awareness of how to integrate this information into personalized care plans, ensuring a balance between technological efficiency and human-centered care to ultimately improve patient care (Dyb et al., 2021). These unique challenges highlight the need for advanced but intuitive devices, where design prioritizes simplicity and intuitive interactions over mere technological feats (Noble and Blandford, 2013).
2.3 Data integrity: security, privacy, and user autonomy
The increasing connectivity of devices brings a concern: the secure handling of data. Wearables collect vast personal data, from activity patterns to sleep cycles. In malicious hands, those data could reveal an individual's habits and health, leading to threats like tailored phishing attacks (Peppet, 2014). Additionally, as wearables integrate with payment platforms and smart homes, breaches could expose financial data or allow unauthorized device control (Bezovski, 2016). Therefore, manufacturers have a responsibility to ensure comprehensive and evolutionary security measures (Safavi and Shukur, 2014). Central to this is the principle of clear data management, where users should be clearly informed about the handling of their data e.g., storage location, accessibility, and use (Troiano, 2016).
On a broader level, the healthcare industry faces its own set of challenges, highlighted by a report from the HIPAA Journal which noted 505 healthcare data breaches in 2019 alone, underscoring vulnerability to cyber-attacks (Alder, 2020). These breaches not only risk patients' personal information but also expose healthcare providers to legal actions from affected individuals seeking compensation for the invasion of privacy and potential harm. That emphasizes the necessity for robust data security measures, such as adopting advanced encryption, two-factor authentication, and conducting regular security audits. Those approaches should instill strong customer trust but may only be maintained through transparent communication and continuous technical support (Seh et al., 2020). However, the variability of data security and privacy regulations across jurisdictions poses a significant challenge for healthcare. Dimitropoulos and Rizk (2009) highlight the difficulty in accommodating varying policy requirements for health information exchange, stressing the need for a consensus on common policies to ensure adequate data protection and security.
Manufacturers should provide users with easily accessible and understandable privacy policies, ensuring that even those without technical expertise can grasp how their data are used (Gluck et al., 2016). Moreover, the principle of data minimization should be adopted. This means wearables should only collect data that is necessary for their functionality, thereby reducing potential harm if a breach occurs. For instance, if a fitness tracker's primary function is to measure steps, it shouldn't unnecessarily collect location data unless it's essential for a specific feature (Wolf et al., 2015). Finally, another crucial aspect is the implementation of user-controlled data permissions, where users should have the power to decide what data they are comfortable sharing and with whom. That empowers individuals but also fosters trust between users and manufacturers (Waldman, 2018).
2.4 Joined-up thinking: interoperability and IoT
The real value of this innovation emerges when wearables and peripheral technologies can communicate and work together. For example, heart failure events in patients with implanted devices were accurately predicted using a multi-modal approach with the integration of physical activity, heart rate, respiration rate, and other physiological variables (Boehmer et al., 2017). Another example could involve evaluating the relationship between sleep quality, heart rate variability, and stress levels during the day (Khanna and Jones, 2023). That scenario would require data fusion from various sensors, including actigraphy, respiratory sensors (e.g., nasal airflow sensors), galvanic skin response (GSR), body temperature, and heart rate sensors (Celik and Godfrey, 2023).
Currently, many proprietary systems exist, and each has its bespoke protocols and interfaces, resulting in compatibility issues. That fragmented environment poses challenges for users who wish to integrate wearables from different manufacturers in a seamless manner (Zeadally et al., 2019). Consequently, the need for standardization becomes essential, with Ravizza et al. (2019) providing a review of the current and upcoming regulatory requirements for wearable sensors in preclinical and clinical testing, highlighting the need for adherence to international standards set by bodies such as the International Electrotechnical Commission (IEC) and the International Organization for Standardization (ISO). Although some guidelines exist, e.g., Surface Electromyography for the Non-Invasive Assessment of Muscles (SENIAM) other physiological outcomes are lacking e.g., guidelines for inertial-based wearable placement (Celik et al., 2021). Key stakeholders should often prioritize collaboration over competition for a unified approach. By supporting standard protocols, wearables of any origin could communicate effectively, improving interoperability. Another potential approach to overcoming interoperability challenges involves standardizing the data outputs from various wearable technologies. This could be implemented similar to the model of the WHO's cytokine standards, which have facilitated uniform comparisons by setting a universal metric system (Mire-Sluis et al., 1998). That could also help facilitate, seamless integration and technological advancement by benchmarking against established standards to (i) understand data variations and normalize outputs and, (ii) enhance the precision and reliability of wearable healthcare technologies (Baker et al., 2017). Additionally, embracing open-source platforms could be a key driver for innovation beyond borders. Such collaborative efforts not only improve user experience but also enable a more inclusive and dynamic future for wearable neurotechnology (Bhat et al., 2019).
3 Developmental challenges
Some wearables can be expensive, making them elusive to a large proportion of society, where such financial constraints potentially deprive many of benefiting from the technologies ability to support a healthy life (He et al., 2023). For democratization of wearables, it's pivotal for manufacturers to innovate. That encompasses cost-effective production, scalable designs, and creating partnerships. Collaborations, especially with entities like governmental health bodies, can be instrumental in offsetting costs, ensuring wearables are universally accessible, and of benefit to the wider public (Kang and Exworthy, 2022). Manufacturers must also prioritize applications that offer the most substantial benefits and value. That concept, much like the evaluation of potential technology platforms in pharmaceutical pipeline management, would involve assessing wearables for their cost-effectiveness, patient outcome improvements, and efficiency in healthcare processes (Bode-Greuel and Greuel, 2005).
3.1 User-centric design: ergonomics, accessibility, and support
Continuing technological advancements can improve daily lives, but even advanced wearables can lose appeal if they don't align with user needs/preferences (Lidwell et al., 2010). That sentiment is accentuated in those with a neurological disorder, where people might be intricately navigating the challenges of physical or cognitive impediments (Wilson et al., 2022). Therefore, ergonomics is key. A device, irrespective of its capabilities, must be crafted to blend seamlessly into the user's daily routine. For instance, those with tremors might benefit from stabilization features or larger tactile buttons, while those with sensory sensitivities might prefer adjustable feedback settings or hypoallergenic materials (Imbesi and Scataglini, 2021; Kang and Exworthy, 2022). It is also essential that the wearables be comfortable for extended wear, ensuring they neither exert undue pressure nor evolve into a source of discomfort (Lewis and Neider, 2017). The user interface (tactile buttons, interactive touchscreens, or voice-activated commands), should be conceptualized with user ease at the forefront and should resonate as a harmonious extension of the user's intent, rather than an unwieldy appendage (Kumari et al., 2017). Furthermore, individuals grappling with functional limitations may experience difficulties in adeptly utilizing wearables that lack user-friendly fixation to their body, e.g., devices designed for lower back attachment. Consequently, these challenges serve to curtail their engagement with, and adoption of, such technological innovations (Guerra et al., 2023). Beyond the wearable itself, a robust support infrastructure can also play a pivotal role in amplifying the user experience. This includes available customer helplines, comprehensive online tutorials, and active community forums, where the primary goal is to ensure that users not only have access to a modern tool but are adequately supported in using it to its full potential (Vassli and Farshchian, 2018).
3.2 Evolution and sustainability: meeting the needs of tomorrow
In a time of rapid technological progress, there's a genuine concern about devices becoming outdated quickly (Spender et al., 2019). This poses a distinct challenge for manufacturers where they must now think ahead, creating devices that cater to current demands while also being prepared for future needs. This can be realized through regular software updates that bring new features and security, ensuring these devices remain current and reliable (Schukat et al., 2016). Another concern is battery life where a wearable's utility is significantly diminished if it cannot last a day without recharging (Godfrey et al., 2018). Manufacturers face the challenge of innovating while balancing power consumption with performance, especially since battery technology, despite its advancements, often lags the demands of modern devices, resulting in user frustrations. Solutions must encompass energy-efficient designs, the exploration of alternative power sources, and software optimizations (Rault et al., 2017). The emerging use of energy harvesting systems in wearable technology is spearheading this ethos, utilizing sophisticated methods to transform the body's kinetic energy, such as movement and gait, and thermal energy, like body heat differential, into electrical power to enhance the autonomy and longevity of wearables (Ali et al., 2023). For wearables to thrive, they must be forward-thinking and power-sustainable.
4 Conclusion
The use of wearables has the potential to reshape the understanding of neurological conditions and revolutionizing patient care. Wearables, with their potential to monitor intricate biomarkers, could provide a plausible decentralized approach to healthcare. However, the journey to pragmatically integrate wearables into routine practice has clinical and developmental challenges: validation, perceptions, data integrity, lack of joined up thinking, weak user-centric designs, and evolutionary needs. Realizing wearable neurotech potential requires a concerted effort from manufacturers, healthcare professionals and the public (Figure 1). By addressing these challenges and fostering a collaborative environment, wearables can find their rightful place to unlock new possibilities in better understanding neurological conditions from beyond the clinic.
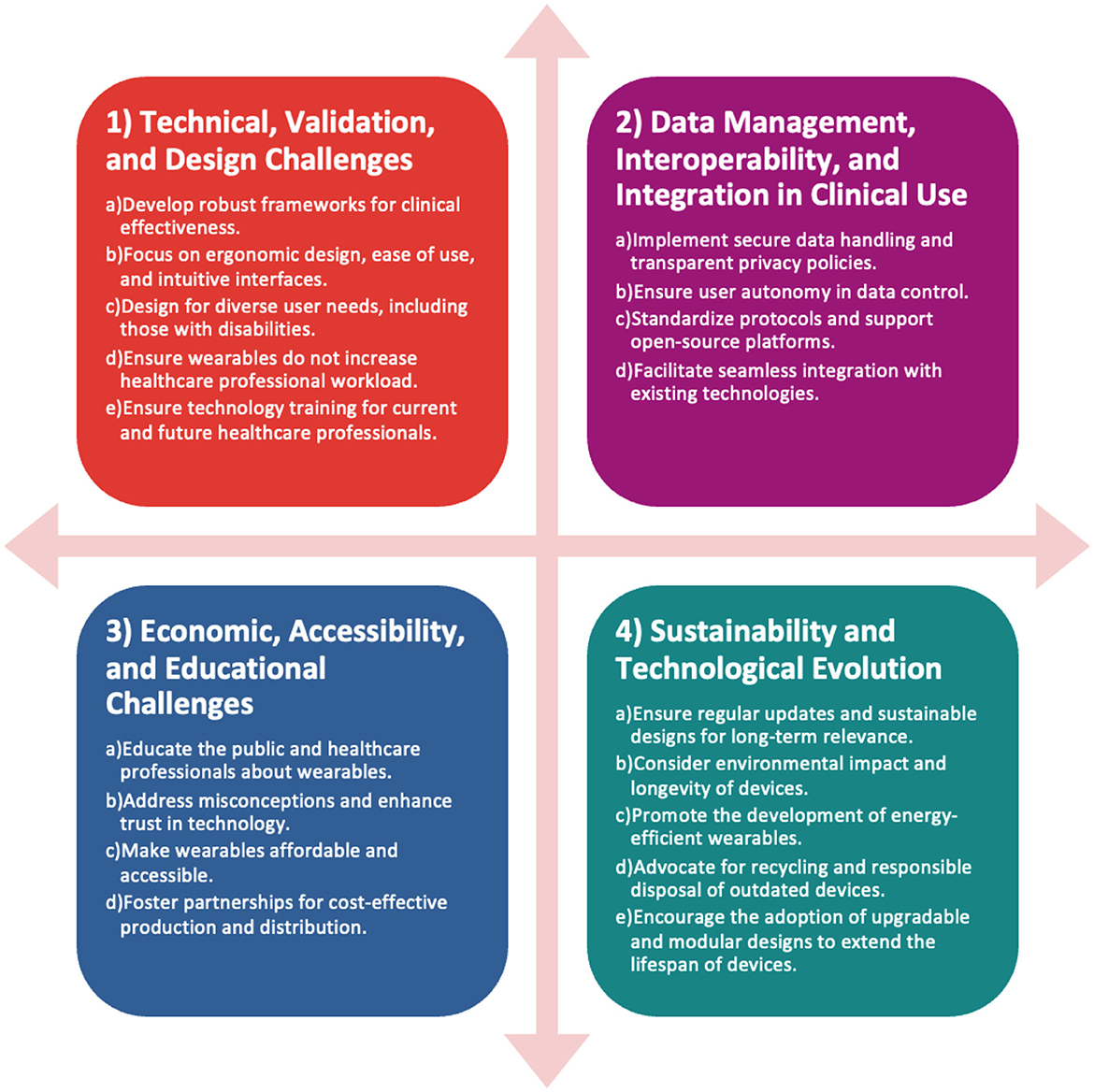
Figure 1. Key challenges and strategies for wearable healthcare technologies. This diagram classifies the challenges into four main categories: technical and design, data management, economic and accessibility, and sustainability, with a focus on ensuring effectiveness, secure integration, affordability, and long-term viability of wearables in healthcare.
Author contributions
CW: Writing – original draft, Writing – review & editing. YC: Writing – original draft, Writing – review & editing. VH: Writing – original draft, Writing – review & editing. PM: Writing – original draft, Writing – review & editing. RW: Writing – original draft, Writing – review & editing. LG: Writing – original draft, Writing – review & editing. RV: Writing – original draft, Writing – review & editing. AG: Writing – original draft, Writing – review & editing.
Funding
The author(s) declare that financial support was received for the research, authorship, and/or publication of this article. This research was co-funded by a grant from the National Institute of Health Research (NIHR) Applied Research Collaboration (ARC) North East and North Cumbria (NENC). This research was also co-funded by the Faculty of Environment and Engineering at Northumbria University.
Conflict of interest
The authors declare that the research was conducted in the absence of any commercial or financial relationships that could be construed as a potential conflict of interest.
Publisher's note
All claims expressed in this article are solely those of the authors and do not necessarily represent those of their affiliated organizations, or those of the publisher, the editors and the reviewers. Any product that may be evaluated in this article, or claim that may be made by its manufacturer, is not guaranteed or endorsed by the publisher.
References
Alder, S. (2020). 2019 healthcare data breach report. HIPAA J. Available online at: https://www.hipaajournal.com/may-2019-healthcare-data-breach-report/
Ali, A., Shaukat, H., Bibi, S., Altabey, W. A., Noori, M., and Kouritem, S. A. (2023). Recent progress in energy harvesting systems for wearable technology. Energy Strat. Rev. 49:101124. doi: 10.1016/j.esr.2023.101124
Awotunde, J. B., Jimoh, R. G., Folorunso, S. O., Adeniyi, E. A., Abiodun, K. M., and Banjo, O. O. (2021). “Privacy and security concerns in IoT-based healthcare systems,” in The Fusion of Internet of Things, Artificial Intelligence, and Cloud Computing in Health Care Internet of Things, eds P. Siarry, M. A. Jabbar, R. Aluvalu, A. Abraham, and A. Madureira (Cham: Springer International Publishing), 105–134.
Baker, S. B., Xiang, W., and Atkinson, I. (2017). Internet of things for smart healthcare: technologies, challenges, and opportunities. IEEE Access 5, 26521–26544. doi: 10.1109/ACCESS.2017.2775180
Bezovski, Z. (2016). The future of the mobile payment as electronic payment system. Eur. J. Bus. Manag. 8, 127–132. Available online at: https://iiste.org/Journals/index.php/EJBM/article/view/29473/30263
Bhat, G., Deb, R., and Ogras, U. Y. (2019). OpenHealth: open-source platform for wearable health monitoring. IEEE Design Test 36, 27–34. doi: 10.1109/MDAT.2019.2906110
Bode-Greuel, K. M., and Greuel, J. M. (2005). Determining the value of drug development candidates and technology platforms. J. Commer. Biotechnol. 11, 155–170. doi: 10.1057/palgrave.jcb.3040113
Boehmer, J. P., Hariharan, R., Devecchi, F. G., Smith, A. L., Molon, G., Capucci, A., et al. (2017). A multisensor algorithm predicts heart failure events in patients with implanted devices: results from the MultiSENSE study. JACC: Heart Fail. 5, 216–225. doi: 10.1016/j.jchf.2016.12.011
Brice, S., and Almond, H. (2020). Health professional digital capabilities frameworks: a scoping review. J. Multidiscip. Healthc. 13, 1375–1390. doi: 10.2147/JMDH.S269412
Bruno, E., Simblett, S., Lang, A., Biondi, A., Odoi, C., Schulze-Bonhage, A., et al. (2018). Wearable technology in epilepsy: the views of patients, caregivers, and healthcare professionals. Epilepsy Behav. 85, 141–149. doi: 10.1016/j.yebeh.2018.05.044
Byrom, B., McCarthy, M., Schueler, P., and Muehlhausen, W. (2018). Brain monitoring devices in neuroscience clinical research: the potential of remote monitoring using sensors, wearables, and mobile devices. Clin. Pharmacol. Ther. 104, 59–71. doi: 10.1002/cpt.1077
Celik, Y., and Godfrey, A. (2023). Bringing it all together: wearable data fusion. npj Digit. Med. 6, 1–3. doi: 10.1038/s41746-023-00897-6
Celik, Y., Stuart, S., Woo, W. L., and Godfrey, A. (2021). Gait analysis in neurological populations: progression in the use of wearables. Med. Eng. Phys. 87, 9–29. doi: 10.1016/j.medengphy.2020.11.005
Chen, C., Ding, S., and Wang, J. (2023). Digital health for aging populations. Nat. Med. 29, 1623–1630. doi: 10.1038/s41591-023-02391-8
Cheung, M. L., Chau, K. Y., Lam, M. H. S., Tse, G., Ho, K. Y., Flint, S. W., et al. (2019). Examining consumers' adoption of wearable healthcare technology: the role of health attributes. Int. J. Environ. Res. Public Health 16:2257. doi: 10.3390/ijerph16132257
Del Din, S., Godfrey, A., and Rochester, L. (2016). Validation of an accelerometer to quantify a comprehensive battery of gait characteristics in healthy older adults and Parkinson's disease: toward clinical and at home use. IEEE J. Biomed. Health Inform. 20, 838–847. doi: 10.1109/JBHI.2015.2419317
Demiris, G., Oliver, D. P., Giger, J., Skubic, M., and Rantz, M. (2009). Older adults' privacy considerations for vision based recognition methods of eldercare applications. Technol. Health Care 17, 41–48. doi: 10.3233/THC-2009-0530
Dimitropoulos, L., and Rizk, S. (2009). A state-based approach to privacy and security for interoperable health information exchange. Health Aff. 28, 428–434. doi: 10.1377/hlthaff.28.2.428
Dyb, K., Berntsen, G., and Kvam, L. (2021). Adopt, adapt, or abandon technology-supported person-centred care initiatives: healthcare providers' beliefs matter. BMC Health Serv. Res. 21:240. doi: 10.1186/s12913-021-06262-1
Foss, B., and Haraldseid, C. (2014). Improvement of health education: the opportunities of technology and digital learning tools. J. Biosafety Health Educ. 2:e114. doi: 10.4172/2332-0893.1000e114
Gluck, J., Schaub, F., Friedman, A., Habib, H., Sadeh, N., Cranor, L. F., et al. (2016). How Short Is Too Short? Implications of Length and Framing on the Effectiveness of Privacy Notices, 321–340. Available online at: https://www.usenix.org/conference/soups2016/technical-sessions/presentation/gluck (accessed September 18, 2023).
Godfrey, A., Hetherington, V., Shum, H., Bonato, P., Lovell, N. H., and Stuart, S. (2018). From A to Z: wearable technology explained. Maturitas 113, 40–47. doi: 10.1016/j.maturitas.2018.04.012
Goldsack, J. C., Coravos, A., Bakker, J. P., Bent, B., Dowling, A. V., Fitzer-Attas, C., et al. (2020). Verification, analytical validation, and clinical validation (V3): the foundation of determining fit-for-purpose for Biometric Monitoring Technologies (BioMeTs). npj Digit. Med. 3:55. doi: 10.1038/s41746-020-0260-4
Grimwood, T., and Snell, L. (2020). The use of technology in healthcare education: a literature review. MedEdPublish 9:137. doi: 10.15694/mep.2020.000137.1
Guerra, A., D'Onofrio, V., Ferreri, F., Bologna, M., and Antonini, A. (2023). Objective measurement versus clinician-based assessment for Parkinson's disease. Exp. Rev. Neurother. 23, 689–702. doi: 10.1080/14737175.2023.2229954
He, C., Chen, Y.-Y., Phang, C.-R., Stevenson, C., Chen, I.-P., Jung, T.-P., et al. (2023). Diversity and suitability of the state-of-the-art wearable and wireless EEG systems review. IEEE J. Biomed. Health Inf. 27, 3830–3843. doi: 10.1109/JBHI.2023.3239053
Hilty, D. M., Armstrong, C. M., Edwards-Stewart, A., Gentry, M. T., Luxton, D. D., and Krupinski, E. A. (2021). Sensor, wearable, and remote patient monitoring competencies for clinical care and training: scoping review. J. Technol. Behav. Sci. 6, 252–277. doi: 10.1007/s41347-020-00190-3
Imbesi, S., and Scataglini, S. (2021). A user centered methodology for the design of smart apparel for older users. Sensors 21:2804. doi: 10.3390/s21082804
Izmailova, E. S., Demanuele, C., and McCarthy, M. (2023). Digital health technology derived measures: biomarkers or clinical outcome assessments? Clin. Transl. Sci. 16, 1113–1120. doi: 10.1111/cts.13529
Johnson, K. T., and Picard, R. W. (2020). Advancing neuroscience through wearable devices. Neuron 108, 8–12. doi: 10.1016/j.neuron.2020.09.030
Kang, H. S., and Exworthy, M. (2022). Wearing the future—wearables to empower users to take greater responsibility for their health and care: scoping review. JMIR Mhealth Uhealth 10:e35684. doi: 10.2196/35684
Karahanoğlu, A., and Erbuğ, Ç. (2011). “Perceived qualities of smart wearables: determinants of user acceptance,” in Proceedings of the 2011 Conference on Designing Pleasurable Products and Interfaces DPPI '11 (New York, NY: Association for Computing Machinery), 1–8.
Khanna, A., and Jones, G. (2023). Toward personalized medicine approaches for Parkinson disease using digital technologies. JMIR Form. Res. 7:e47486. doi: 10.2196/47486
Kumari, P., Mathew, L., and Syal, P. (2017). Increasing trend of wearables and multimodal interface for human activity monitoring: a review. Biosens. Bioelectron. 90, 298–307. doi: 10.1016/j.bios.2016.12.001
Lee, S. M., and Lee, D. (2020). Healthcare wearable devices: an analysis of key factors for continuous use intention. Serv. Bus. 14, 503–531. doi: 10.1007/s11628-020-00428-3
Lewis, J. E., and Neider, M. B. (2017). Designing wearable technology for an aging population. Ergon. Design 25, 4–10. doi: 10.1177/1064804616645488
Leyens, L., Northcott, C. A., Maloney, L., McCarthy, M., Dokuzova, N., Pfister, T., et al. (2024). Why language matters in digital endpoint development: harmonized terminology as a key prerequisite for evidence generation. Digit. Biomark. 8, 1–12. doi: 10.1159/000534954
Li, J., Ma, Q., Chan, A., and Man, S. S. (2019). Health monitoring through wearable technologies for older adults: smart wearables acceptance model. Appl. Ergon. 75, 162–169. doi: 10.1016/j.apergo.2018.10.006
Lidwell, W., Holden, K., and Butler, J. (2010). Universal Principles of Design, Revised and Updated: 125 Ways to Enhance Usability, Influence Perception, Increase Appeal, Make Better Design Decisions, and Teach Through Design. Rockport Publishers.
Mire-Sluis, A. R., Das, R. G., and Padilla, A. (1998). WHO cytokine standardization: facilitating the development of cytokines in research, diagnosis and as therapeutic agents. J. Immunol. Methods 216, 103–116. doi: 10.1016/S0022-1759(98)00073-8
Moore, J., Stuart, S., McMeekin, P., Walker, R., Celik, Y., Pointon, M., et al. (2023). Enhancing free-living fall risk assessment: contextualizing mobility based IMU data. Sensors 23:891. doi: 10.3390/s23020891
Noble, P., and Blandford, A. (2013). “You Can't Touch This: potential perils of patient interaction with clinical medical devices,” in Human-Computer Interaction-INTERACT 2013:14th IFIP TC 13 International Conference (Cape Town: Springer Berlin Heidelberg), 395–402.
Peppet, S. R. (2014). Regulating the internet of things: first steps toward managing discrimination, privacy, security and consent. Tex. L. Rev. 93, 85–178. Available online at: https://heinonline.org/HOL/P?h=hein.journals/tlr93&i=95
Rault, T., Bouabdallah, A., Challal, Y., and Marin, F. (2017). A survey of energy-efficient context recognition systems using wearable sensors for healthcare applications. Pervasive Mob. Comput. 37, 23–44. doi: 10.1016/j.pmcj.2016.08.003
Ravizza, A., Pietro, L. D., Sternini, F., Maria, C. D., Bignardi, C., and Audenino, A. (2019). Comprehensive review on current and future regulatory requirements on wearable sensors in preclinical and clinical testing. Front. Bioeng. Biotechnol. 7:313. doi: 10.3389/fbioe.2019.00313
Safavi, S., and Shukur, Z. (2014). Conceptual privacy framework for health information on wearable device. PLoS ONE 9:e114306. doi: 10.1371/journal.pone.0114306
Schukat, M., McCaldin, D., Wang, K., Schreier, G., Lovell, N. H., Marschollek, M., et al. (2016). Unintended consequences of wearable sensor use in healthcare. Yearbook Med. Informatics 25, 73–86. doi: 10.15265/IY-2016-025
Seh, A. H., Zarour, M., Alenezi, M., Sarkar, A. K., Agrawal, A., Kumar, R., et al. (2020). Healthcare data breaches: insights and implications. Healthcare 8:133. doi: 10.3390/healthcare8020133
Shegog, R., Braverman, L., and Hixson, J. D. (2020). Digital and technological opportunities in epilepsy: toward a digital ecosystem for enhanced epilepsy management. Epilepsy Behav. 102:106663. doi: 10.1016/j.yebeh.2019.106663
Spender, A., Bullen, C., Altmann-Richer, L., Cripps, J., Duffy, R., Falkous, C., et al. (2019). Wearables and the internet of things: considerations for the life and health insurance industry. Br. Act. J. 24:e22. doi: 10.1017/S1357321719000072
Stone, E. E., and Skubic, M. (2013). Unobtrusive, continuous, in-home gait measurement using the Microsoft Kinect. IEEE Trans. Biomed. Eng. 60, 2925–2932. doi: 10.1109/TBME.2013.2266341
Stuart, S., Vitorio, R., Morris, R., Martini, D. N., Fino, P. C., and Mancini, M. (2018). Cortical activity during walking and balance tasks in older adults and in people with Parkinson's disease: a structured review. Maturitas 113, 53–72. doi: 10.1016/j.maturitas.2018.04.011
Syed, S. T., Gerber, B. S., and Sharp, L. K. (2013). Traveling towards disease: transportation barriers to health care access. J. Community Health 38, 976–993. doi: 10.1007/s10900-013-9681-1
Troiano, A. (2016). Wearables and personal health data: putting a premium on your privacy notes. Brook. L. Rev. 82, 1715–1754. Available online at: https://brooklynworks.brooklaw.edu/blr/vol82/iss4/6
Vassli, L. T., and Farshchian, B. A. (2018). Acceptance of health-related ICT among elderly people living in the community: a systematic review of qualitative evidence. Int. J. Hum. Comp. Interact. 34, 99–116. doi: 10.1080/10447318.2017.1328024
Vasudevan, S., Saha, A., Tarver, M. E., and Patel, B. (2022). Digital biomarkers: convergence of digital health technologies and biomarkers. NPJ Digit. Med. 5:36. doi: 10.1038/s41746-022-00583-z
Waldman, A. E. (2018). Privacy as Trust: Information Privacy for an Information Age. Cambridge: Cambridge University Press.
Wang, F., Skubic, M., Rantz, M., and Cuddihy, P. E. (2014). Quantitative gait measurement with pulse-Doppler radar for passive in-home gait assessment. IEEE Trans. Biomed. Eng. 61, 2434–2443. doi: 10.1109/TBME.2014.2319333
Warmerdam, E., Hausdorff, J. M., Atrsaei, A., Zhou, Y., Mirelman, A., Aminian, K., et al. (2020). Long-term unsupervised mobility assessment in movement disorders. Lancet Neurol. 19, 462–470. doi: 10.1016/S1474-4422(19)30397-7
Wilson, S., Ardle, R. M., Tolley, C., and Slight, S. (2022). Usability and acceptability of wearable technology in the early detection of dementia. Alzheimers Dement. 18(Suppl. 2):e059820. doi: 10.1002/alz.062148
Wolf, C., Polonetsky, J., and Finch, K. (2015). A Practical Privacy Paradigm for Wearables. Future of Privacy Forum. Available online at: https://fpf.org/blog/new-from-fpf-a-practical-privacy-paradigm-for-wearables/ (accessed January 8, 2015).
Wu, Y.-H., Damnée, S., Kerhervé, H., Ware, C., and Rigaud, A.-S. (2015). Bridging the digital divide in older adults: a study from an initiative to inform older adults about new technologies. Clin. Interv. Aging 10, 193–201. doi: 10.2147/CIA.S72399
Xie, B. (2011). Older adults, e-health literacy, and collaborative learning: an experimental study. J. Am. Soc. Inf. Sci. Technol. 62, 933–946. doi: 10.1002/asi.21507
Yang, Q., Mamun, A. A., Hayat, N., Salleh, M. F. M., Jingzu, G., and Zainol, N. R. (2022). Modelling the mass adoption potential of wearable medical devices. PLoS ONE 17:e0269256. doi: 10.1371/journal.pone.0269256
Zeadally, S., Siddiqui, F., Baig, Z., and Ibrahim, A. (2019). Smart healthcare: Challenges and potential solutions using internet of things (IoT) and big data analytics. PSU Res. Rev. 4, 149–168. doi: 10.1108/PRR-08-2019-0027
Keywords: wearables, validation, remote, internet of things, sustainability
Citation: Wall C, Celik Y, Hetherington V, McMeekin P, Walker R, Graham L, Vitorio R and Godfrey A (2024) Considering and understanding developmental and deployment barriers for wearable technologies in neurosciences. Front. Neurosci. 18:1379619. doi: 10.3389/fnins.2024.1379619
Received: 31 January 2024; Accepted: 27 February 2024;
Published: 13 March 2024.
Edited by:
Xiaying Wang, ETH Zürich, SwitzerlandReviewed by:
Arni Ariani, Indonesian Biomedical Engineering Society (IBES), IndonesiaElena Izmailova, Koneksa Health, United States
Copyright © 2024 Wall, Celik, Hetherington, McMeekin, Walker, Graham, Vitorio and Godfrey. This is an open-access article distributed under the terms of the Creative Commons Attribution License (CC BY). The use, distribution or reproduction in other forums is permitted, provided the original author(s) and the copyright owner(s) are credited and that the original publication in this journal is cited, in accordance with accepted academic practice. No use, distribution or reproduction is permitted which does not comply with these terms.
*Correspondence: Alan Godfrey, YWxhbi5nb2RmcmV5QG5vcnRodW1icmlhLmFjLnVr