- 1Department of Functioning and Disability, Institute for Developmental Research, Aichi Developmental Disability Center, Kasugai, Japan
- 2Section of Brain Function Information, National Institute for Physiological Sciences, Okazaki, Japan
- 3Department of Psychiatry, Okazaki City Hospital, Okazaki, Japan
- 4Department of Psychiatry, Gifu University Graduate School of Medicine, Gifu, Japan
- 5Department of Anesthesiology, Nagoya University Graduate School of Medicine, Nagoya, Japan
- 6Multidisciplinary Pain Center, Aichi Medical University, Nagakute, Japan
- 7Department of Clinical Laboratory, Mie University Hospital, Tsu, Japan
- 8Department of Neuropsychiatry, Mie University Graduate School of Medicine, Tsu, Japan
Responses to a sensory stimulus are inhibited by a preceding stimulus; if the two stimuli are identical, paired-pulse suppression (PPS) occurs; if the preceding stimulus is too weak to reliably elicit the target response, prepulse inhibition (PPI) occurs. PPS and PPI represent excitability changes in neural circuits induced by the first stimulus, but involve different mechanisms and are impaired in different diseases, e.g., impaired PPS in schizophrenia and Alzheimer’s disease and impaired PPI in schizophrenia and movement disorders. Therefore, these measures provide information on several inhibitory mechanisms that may have roles in clinical conditions. In the present study, PPS and PPI of the auditory change-related cortical response were examined to establish normative data on healthy subjects (35 females and 32 males, aged 19–70 years). We also investigated the effects of age and sex on PPS and PPI to clarify whether these variables need to be considered as biases. The test response was elicited by an abrupt increase in sound pressure in a continuous sound and was recorded by electroencephalography. In the PPS experiment, the two change stimuli to elicit the cortical response were a 15-dB increase from the background of 65 dB separated by 600 ms. In the PPI experiment, the prepulse and test stimuli were 2- and 10-dB increases, respectively, with an interval of 50 ms. The results obtained showed that sex exerted similar effects on the two measures, with females having stronger test responses and weaker inhibition. On the other hand, age exerted different effects: aging correlated with stronger test responses and weaker inhibition in the PPS experiment, but had no effects in the PPI experiment. The present results suggest age and sex biases in addition to normative data on PPS and PPI of auditory change-related potentials. PPS and PPI, as well as other similar paradigms, such as P50 gating, may have different and common mechanisms. Collectively, they may provide insights into the pathophysiologies of diseases with impaired inhibitory function.
1 Introduction
Paired-pulse suppression (PPS) describes short-term plasticity in neural circuits in which the response to a stimulus is inhibited by the preceding same stimulus. The activity-dependent modulation of a circuit is a primary form of synaptic modulation and is important for generating appropriate outputs with various functional roles (O’Donovan and Rinzel, 1997). In humans, PPS is typically evaluated using the P50 gating paradigm, in which two identical sound stimuli are presented 500 ms apart and the degree of inhibition is assessed using the P50 component of auditory evoked potentials (AEPs) elicited by the two stimuli (Adler et al., 1982); the PPS value indicates how strongly the first stimulus filters out the response to the second stimulus. The PPS of P50 gating is impaired in several diseases, including schizophrenia (Adler et al., 1982), bipolar disorder (Lijffijt et al., 2009b), and Alzheimer’s disease (Jessen et al., 2001). In another paradigm for assessing sensory inhibition, the first stimulus is weak enough to elicit reliable responses, but inhibits subsequent responses to a strong sensory stimulus. This is called prepulse inhibition (PPI) (Graham, 1975). Startle reflexes are generally elicited by a loud sound and the blink reflex in humans is recorded from the orbitalis muscle using electromyography with an electrode placed on the lower eyelid. When a weak sound, or prepulse, precedes the reflex-evoking test stimulus, the magnitude of the startle reflex is reduced. PPI deficits are widely known in neuropsychiatric diseases, such as schizophrenia (Braff, 2010), movement disorders (Swerdlow, 2013), and developmental disorders (McAlonan et al., 2002). Previous animal studies revealed some neural circuits of the acoustic startle reflex in the brainstem, which did not involve cortical regions (Lee et al., 1996). Similar to PPS, PPI is considered to represent a gating mechanism; however, previous studies showed that these two measures reflected different mechanisms (Light and Braff, 2001; Brenner et al., 2004; Braff et al., 2007).
Several neural mechanisms were shown to be involved in PPS in animal studies, e.g., a release-independent presynaptic mechanism and release-dependent postsynaptic mechanism (Kirischuk et al., 2002). Therefore, impairments in electrophysiological measures of inhibition in some diseases may reflect abnormalities in different mechanisms. For example, reduced P50 gating in patients with schizophrenia and Alzheimer’s disease may reflect different aspects of the measure. On the other hand, impairments in different diseases may also represent common mechanisms (Swerdlow, 2013). PPI of different methods may involve distinct mechanisms. Since the mechanisms underlying sensory inhibition remain unclear, information from multiple sources needs to be accumulated.
We previously examined a similar phenomenon using auditory change-related cortical responses. A change-related cortical response is elicited by abrupt changes in sensory inputs and recorded using electroencephalography and magnetoencephalography (Inui et al., 2010a,b). It represents automatic brain processes to detect salient sensory events and is common across auditory (Yamashiro et al., 2009a, 2011; Nishihara et al., 2011), somatosensory (Yamashiro et al., 2009b; Otsuru et al., 2011), and visual (Tanaka et al., 2009a,b; Urakawa et al., 2010a,b) systems. When the same change stimulus is presented twice 600 ms apart, a similar change-related response is elicited twice, with the second response being smaller, i.e., PPS (Takeuchi et al., 2017, 2018). If a weak change stimulus is presented 10–800 ms before the test change stimulus, the magnitude of the test response is smaller than when the test stimulus is presented alone, i.e., PPI (Inui et al., 2012, 2016). Although PPI is generally used for the startle reflex, this phenomenon indicates that a weak preceding input affects excitability somewhere in its own pathway. Therefore, similar mechanisms may exist in other neural systems. In the present study, a reduction in the test response by a weak leading stimulus, irrespective of the neural circuit, is referred to as PPI. In addition to the startle reflex and cortical responses, we showed that the R1 component of the trigeminal blink reflex with an oligosynaptic pathway showed PPI over a similar time course to conventional PPI (Inui et al., 2023). In the present study, the auditory change-related response was used as the test response. Previous studies on PPI and PPS reported high test–retest reliability when the change-related cortical response was used (Takeuchi et al., 2021a,b).
One of the issues associated with interpreting PPI and PPS data is that they are affected by age and sex, similar to many other indexes. Previous studies using P50 gating and PPI of the startle reflex revealed significant sex and age effects (Swerdlow et al., 1993; Kumari et al., 2003; Fuerst et al., 2007; de Oliveira et al., 2023). Accumulating evidence has shown that age and sex widely affect brain function, and these variables may have an impact on the findings or even conclusion of a study (Cahill, 2012). Since these effects on PPI or PPS of the change-related response have not been investigated, the present study was undertaken. These effects need to be confirmed before current methods are applied in clinical studies. Therefore, the present study was conducted to obtain normative data on PPI and PPS as indexed by the change-related cortical response and to establish whether age and sex need to be considered as bias factors.
2 Methods
The present study was approved in advance by the Ethics Committee of the Aichi Developmental Disability Center, Kasugai, Japan (approval number: RC0502) and conducted in accordance with the Declaration of Helsinki. Written consent was obtained from all subjects. The experiment was performed on 67 healthy non-smokers (35 females and 32 males) aged 36.8 ± 12.3 (range, 19–70) years. None of the subjects were treated for neurological or mental diseases or substance abuse in the last 2 years. They had normal hearing as assessed by an audiometer (AA-71, Rion, Tokyo, Japan). Two experiments were conducted on all subjects, Experiment 1 (Exp1) and Experiment 2 (Exp2), in that order. The former was the PPS experiment, while the latter was the PPI experiment. Although females are always tested in the luteal phase of the menstrual cycle in standard studies, information on menstruation was not obtained in the present study.
2.1 Auditory stimuli
Repeats of 25-ms pure tones (800 Hz, rise and fall times of 5 ms) were used for auditory stimuli because abrupt changes in any sound feature are possible without any undesired effect (Inui et al., 2010a). Although the carrier may be any audible frequency, we have used 800 Hz because it has the advantage of varying the sound location by manipulating the interaural time difference (Inui et al., 2010a; Akiyama et al., 2011; Ohoyama et al., 2012). The standard sound stimulus was 80 repeats of the 25-ms tone at 65 dB SPL (Figure 1A) in Exp1 and 24 repeats at 70 dB in Exp2. The change stimulus to evoke the change-related cortical response was two consecutive 25-ms tones of 80 dB (Takeuchi et al., 2017). In Exp1, two identical change stimuli were inserted at 1,100 and 1,700 ms of the 2000-ms standard stimulus. Therefore, sound pressure was abruptly increased twice with a 600-ms interval. In Exp2, there were four types of sound stimuli (Figure 1B): standard sound without any changes, a test alone stimulus (Test alone) with a sound pressure increase to 80 dB at 350 ms, a prepulse alone stimulus with a slight increase in sound pressure of 2 dB at 300 ms, and a test + prepulse stimulus with the prepulse and test. Therefore, the prepulse–test interval was 50 ms or the inter-stimulus interval was 25 ms. Sound stimuli were created using a PC (Windows XP, 32 bit) and delivered through a headphone.
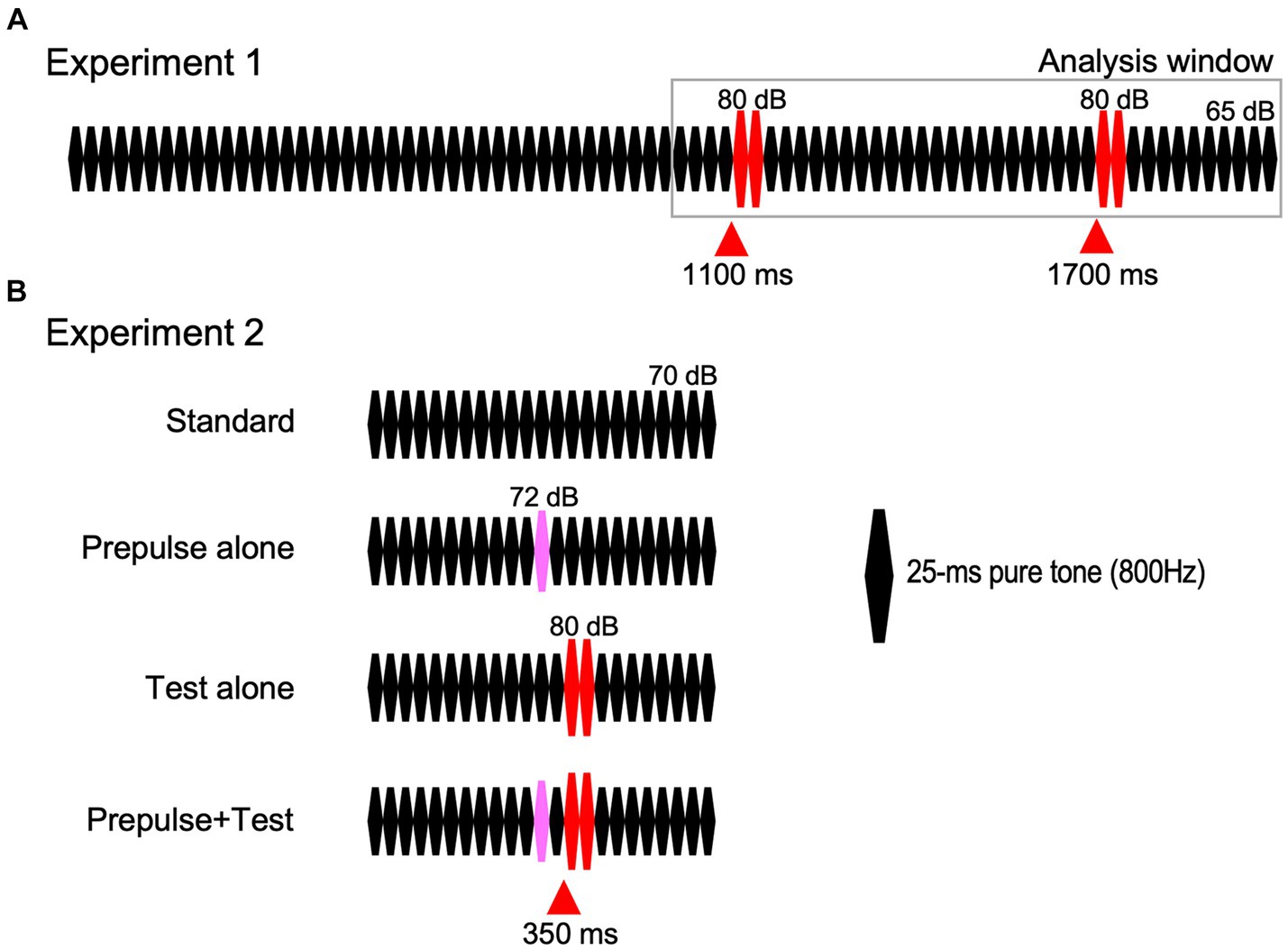
Figure 1. Sound stimuli. Red-filled triangles indicate the timing of the abrupt sound pressure increase for eliciting the test response.
2.2 Recordings
Auditory evoked potentials were recorded using an EMG/EP measuring system (MEB-2300, Nihon Kohden, Tokyo) at a sampling rate of 1,000 Hz. An exploring electrode was placed at FzCz referenced to the linked P9-P10 (Inui et al., 2010a). The activity at FzCz was the sum of both hemispheres. To reject trials with eye blinks, a pair of electrodes were placed on the supra- and infra-orbit of the right eye. Impedance for all the electrodes was <5 kΩ. The analysis window was 1,000 to 2,000 ms from the onset of the auditory stimulus in Exp1 (Figure 1A) and from 100 ms before to 600 ms after the stimulus onset in Exp2. The analog filter was 0.5–100 Hz. Trials with activity >100 μV were automatically rejected from averaging.
2.3 Procedures
Subjects were seated on a chair and watched a silent movie on a screen 1.5 m in front of them throughout the recording. They were instructed to ignore stimuli and concentrate on the movie (Inui et al., 2010a). Exp1 was conducted before Exp2. In Exp1, the sound stimulus was presented with a trial-trial interval of 2,200 ms or inter-trial interval of 200 ms. After completing an average of 120 trials, Exp2 was immediately initiated. Four sound stimuli were presented randomly at a trial-trial interval of 800 ms or inter-trial interval of 200 ms. A total of 120 artifact-free epochs were averaged for each sound stimulus. Order effects were unlikely because the change-related cortical response is resistant to repetition (Inui et al., 2010a).
2.4 Analysis
Recorded signals were subjected to band-pass filtering of 0.9–35 Hz (Takeuchi et al., 2023). Any abrupt changes in a continuous sound elicit triphasic change-related cortical responses with peaks at approximately at 60, 120, and 200 ms (P50/N100/P200, Figure 2) (Inui et al., 2010a). In the present study, the peak-to-peak-to-peak amplitude was used to evaluate the magnitude of the response. This procedure minimizes issues associated with a baseline shift (Inui et al., 2010b). The P50-N100-P200 amplitude was calculated using the following formula: (P50-N100 peak-to-peak amplitude + N100-P200 peak-to-peak amplitude)/2.
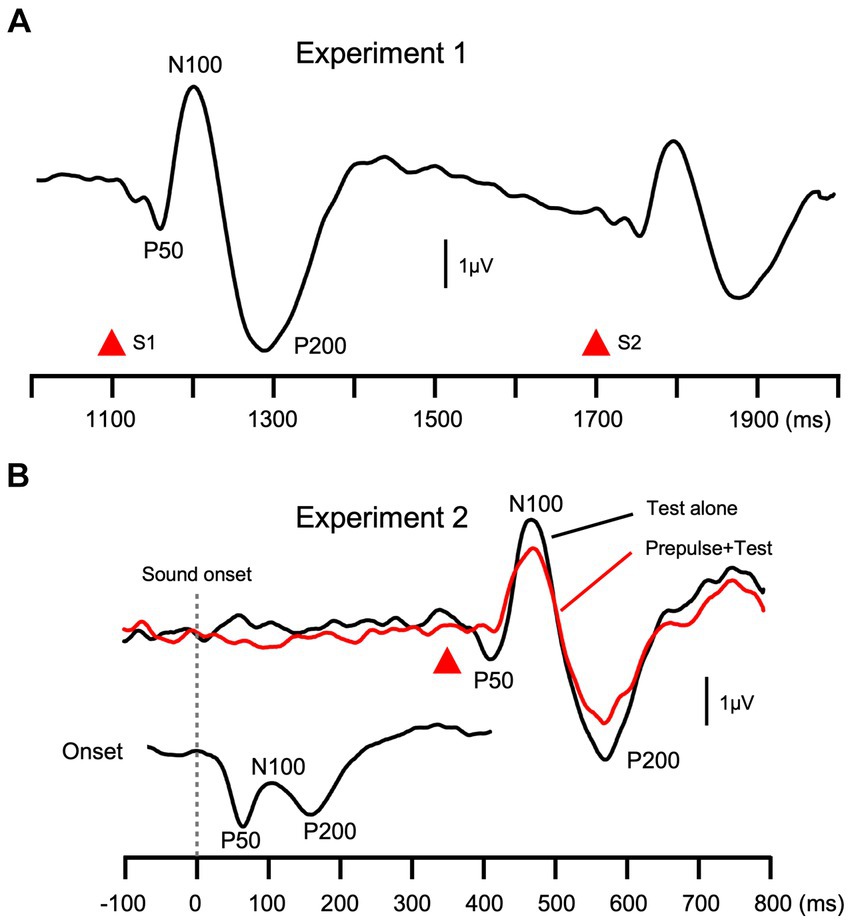
Figure 2. Grand-averaged waveforms. Grand-averaged waveforms across all subjects in Experiment 1 (A) and 2 (B) are shown. The peak-to-peak-to-peak amplitude (P50-N100-P200) was used as the magnitude of the test response.
In Exp1, there was an abrupt change in sound pressure twice, S1 and S2, which elicited S1-evoked change-related responses and smaller S2-related responses. The degree of inhibition of the S2 response (PPS) was calculated using the following formula: (S1 amplitude – S2 amplitude)/S1 amplitude × 100. In Exp2, subtraction procedures were necessary to calculate the response amplitude (Inui et al., 2010a). To obtain the test alone response, responses to the standard were subtracted from those to the test alone stimulus. Prepulse + Test responses were obtained by subtracting responses to the prepulse alone stimulus from those to the test + prepulse stimulus. The amplitude of the response was measured using difference waveforms. The degree of PPI was calculated as follows: (Test alone amplitude – Prepulse + Test amplitude)/Test alone amplitude × 100.
In addition to the test response and its inhibition, the amplitude of the onset response was analyzed in Exp2. The onset response was obtained by averaging all trials. Similar to the change-related response, the peak-to-peak-to-peak amplitude was measured (Figure 2B). Therefore, five electrophysiological variables were used in the present study: the amplitude of the response to the first stimulus (S1) and the degree of inhibition of the second stimulus (PPS) in Exp1 and the amplitude of the Test alone response (Test Alone), PPI, and the amplitude of the onset response (Onset) in Exp2.
The effects of sex on these five variables were assessed using a discriminant analysis. To assess the contributions of each variable to separate sexes, the standardized canonical discriminant function coefficient was calculated. The relationships between age and variables were evaluated using a partial correlation analysis controlling for sex in addition to Pearson’s correlation coefficients. The significance of differences was set at 0.05. All statistical analyses were performed using SPSS ver. 24.
3 Results
3.1 Effects of sex and age
Females (39.1 ± 12.6 y.o.) were slightly older than males (34.0 ± 11.4) (p = 0.09). In both experiments, the abrupt change in sound pressure (Figure 1) elicited triphasic change-related cortical responses with peaks at approximately 60, 120, and 200 ms (P50/N100/P200), consistent with previous findings (Inui et al., 2010a). Grand-averaged waveforms across subjects are shown in Figure 2. Conditioning stimuli significantly reduced the amplitude of subsequent test responses in both Exp1 (paired t-test, p = 1.3 × 10−20) and Exp2 (2.0 × 10−11). The effects of sex were examined using a discriminant analysis with five electrophysiological measures as independent variables. Results showed that the function was significant (λ = 0.70, p = 3.9 × 10−4) with larger contributions of Onset, S1, Test Alone, and PPS in that order as judged by standardized canonical discriminant coefficients (Table 1): females had stronger Test Alone, Onset, and S1 and weaker PPS than males. The results of t-tests obtained using each evoked potential component (P50, N100, and P200) and peak-to-peak amplitudes are shown in Supplementary Table S1.
The effects of age were assessed using a partial correlation analysis controlling for sex. As shown in Table 2, aging significantly affected S1 (p = 2.4 × 10−4) and Onset (p = 0.022). PPS showed a weak negative correlation with age (r = −0.21, p = 0.087). Older subjects showed stronger S1 and weaker PPS in Exp1 and stronger Onset in Exp2. On the other hand, the amplitude of the test alone response and its PPI in Exp2 were not affected by age. Figure 3 shows the relationships between age and five variables with regression lines obtained from the simple linear regression analysis. Results based on each evoked potential component are presented in Supplementary Table S2.
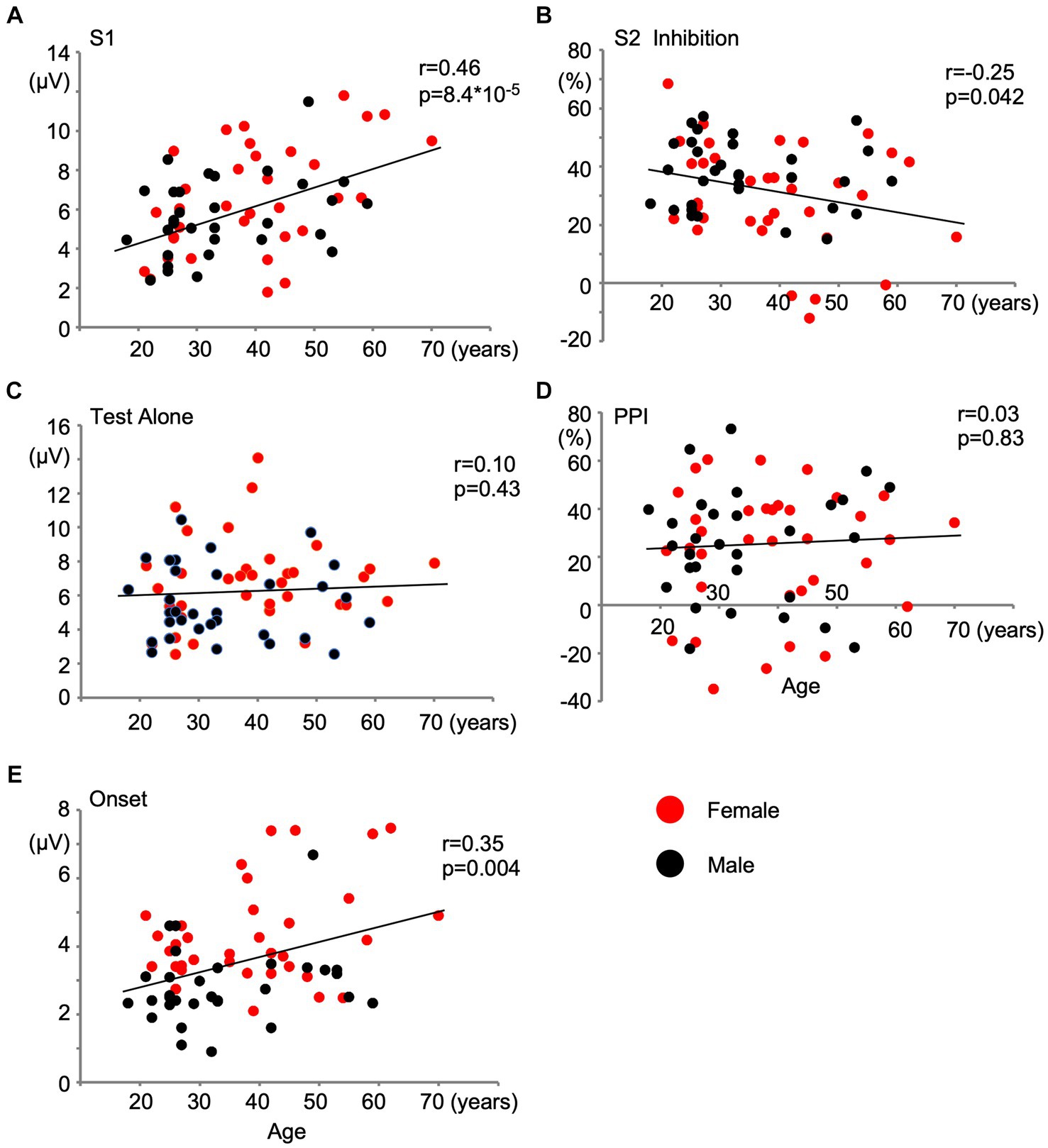
Figure 3. Effects of age on change-related cortical response and inhibition. Scatter plots show the relationship of age with the amplitude of the first response (A) and inhibition of the second response (B) in Experiment 1, and with the amplitude of the test alone response (C), prepulse inhibition (D), and the amplitude of the onset response (E) in Experiment 2. Correlation coefficients and t-values obtained by the simple linear regression analysis are shown.
3.2 Relationships among variables
Some additional comparisons were performed among variables. Table 3 shows the results of partial correlation analyses with sex and age as covariates. Since the change stimulus was the same, the amplitudes of the test responses, S1 and Test Alone, correlated between the two experiments (r = 0.64). However, the degree of inhibition did not correlate between the two experiments (r = 0.08), which suggested that these two inhibitions were controlled by different mechanisms. As previously reported (Inui et al., 2012; Kodaira et al., 2013), a correlation was observed between Test Alone and PPI in Exp2: subjects with stronger Test alone responses showed stronger PPI (r = 0.44). However, this was not the case in Exp1: the S1 amplitude and PPS did not correlate (r = 0.09), which was investigated for the first time in the present study. A positive correlation was noted among S1, Test alone, and Onset.
4 Discussion
The present study showed the significant effects of sex and age on electrophysiological measures. The amplitude of the test response in both experiments and that of the onset response were greater for females, while inhibition in both experiments was slightly weaker (Table 1). Aging correlated with the greater S1 amplitude and weaker PPS in Exp1 and the stronger Onset response in Exp2, but did not affect the amplitude of the Test alone response or its PPI (Table 2). In the brain, sex differences have been reported in cognitive functions (Delgado and Prieto, 1996), structures (de Courten-Myers, 1999), connectivity (Ingalhalikar et al., 2014), gene expression (Gegenhuber and Tollkuhn, 2020), neurotransmission (Pandya et al., 2019), and neurodegeneration (Cuenca-Bermejo et al., 2023). These differences may lead to sex differences in vulnerability to some diseases, such as autism spectrum disorder and mood disorder, clinical presentation, the severity of symptoms, the course of illness, and treatment outcomes (Vega et al., 2011; Fernández-Guasti et al., 2012; Fernando et al., 2020; Ziemka-Nalecz et al., 2023). Aging affects some cognitive functions, but not others (Rey-Mermet and Gade, 2018). Since the mechanisms underlying these effects in clinical aspects have not yet been elucidated in detail, the accumulation of information from multiple sources is needed. Therefore, the present study was performed to establish normative data and identify possible biases with a view to their use in clinical research.
4.1 Effects of sex
The results obtained herein revealed clear sex effects on some variables. Females showed weaker inhibition and stronger test responses than males. Although PPI of the cortical response differed from previously reported PPI in neural circuits, similarities and dissimilarities were examined between previous studies and the present investigation. Weaker inhibition in females was consistent with the findings of previous studies using PPI of the acoustic startle reflex (Swerdlow et al., 1993; Kumari et al., 2003; Aasen et al., 2005; Naysmith et al., 2022) and PPI of the trigeminal blink reflex (Kofler et al., 2013). Since the target responses and, hence, neural circuits examined in these studies differed from that of the present study, the similar results and findings reported are important because they suggest common mechanisms. One explanation for the mechanisms underlying sex differences is ovarian hormones. PPI of the startle reflex fluctuates with the menstrual cycle, with weaker PPI in the luteal phase (Swerdlow et al., 1997; Jovanovic et al., 2004). More specifically, a larger increase in progesterone levels in the luteal phase is associated with a smaller decrease in PPI from the follicular to luteal phase (Kumari et al., 2010). As a possible mechanism, the female hormone cycle affects the dopaminergic system (Zachry et al., 2021), which, in turn, has an impact on PPI (Zhang et al., 2000) such that an increase in the release of dopamine decreases PPI. Therefore, weaker inhibition in females in the present study may be attributed to young female subjects being in the luteal phase at the time of testing.
Another interesting effect of sex is that prepulse facilitation (PPF) was shown to be stronger in females in studies with the auditory startle reflex (Aasen et al., 2005). Weaker PPI and stronger PPF in females indicated that this bias is based on a general shift in facilitation/inhibition in the direction of facilitation in women relative to that in men (Kumari et al., 2003). In the present study, augmented S2 responses were only observed in females in Exp1 (Figure 3B). PPF was also slightly stronger in females in Exp2 (Figure 3D): PPF was present in 7 females with a mean value of −18.7% of PPI and 6 males with −9.3% (p = 0.09, t-test), which is consistent with the above proposal. In this regard, Kumari et al. (2010) reported that progesterone plays a role in balancing facilitation/inhibition. In the present study, the majority of female subjects with PPF were younger than 50 years of age (Figure 3D), suggesting that this mechanism contributed to weaker PPI in females.
Sex differences have been reported in the brain that are independent of gonadal hormone levels (Ingalhalikar et al., 2014; Pandya et al., 2019; Gegenhuber and Tollkuhn, 2020; Williams et al., 2021). Among them, differences in inhibitory synaptic transmission appear to be important (Uhl et al., 2022). The excitability of a neural circuit is controlled by excitatory pyramidal neurons and inhibitory interneurons. Since gamma-amino butyric acid (GABA) is the main inhibitory neurotransmitter in the brain, GABAergic transmission is expected to be involved in PPS and PPI (Kodsi and Swerdlow, 1995; Fendt et al., 2001; Inui et al., 2018). In a study using post-mortem brain tissues and Western Blotting, Pandya et al. (2019) found that in the superior temporal gyrus (STG), the neural source of the present auditory change-related response (Inui et al., 2010b, 2012), GABA-A receptor expression was markedly higher in males than in females; the α1 subunit in both the young and older age groups and the α2, α5, and β3 subunits in the older age groups, which is consistent with the present results showing stronger inhibition in males (Table 1). This sex bias and stronger test- or onset-evoked responses in females in the present study is consistent given that excitation and inhibition always coexist in the neural circuit of the change-related response (Inui et al., 2016).
The findings of previous studies using AEPs are inconsistent. The amplitude of AEPs was greater in females for N100 (Xin et al., 2021), but greater in males for P50 (Fuerst et al., 2007) and N100 (Fuerst et al., 2007; Golob et al., 2007), while no differences were observed for P50 (Hetrick et al., 1996; Rasco et al., 2000; Clementz and Blumenfeld, 2001; Brinkman and Stauder, 2007; Golob et al., 2007; Lijffijt et al., 2009a; Xin et al., 2021), N100 (Hetrick et al., 1996; Clementz and Blumenfeld, 2001; Lijffijt et al., 2009a), or P200 (Hetrick et al., 1996; Lijffijt et al., 2009a; Xin et al., 2021). Sex differences in PPS are also inconsistent. While some studies reported the stronger inhibition of P50 or N100 for males (Hetrick et al., 1996; Fuerst et al., 2007), others demonstrated the opposite (Xin et al., 2021) or no differences (Rasco et al., 2000; Clementz and Blumenfeld, 2001; Brinkman and Stauder, 2007; Lijffijt et al., 2009a). In the present study, the amplitudes of both the change-related test response (S1 and Test alone) and onset response were greater in females than in males. Since the onset response is a type of change-related response against the silent background (Nishihara et al., 2011), these results suggest that the cortical response to auditory changes is stronger in females. The change-related cortical response is a subtype of defense reactions (Inui et al., 2012) similar to startle reflexes (Turpin, 1986); therefore, stronger change-related responses in females are consistent with the flexion reflex (Sandrini et al., 2005), auditory startle reflex (Kofler et al., 2001), and PPF of the startle reflex (Aasen et al., 2005) being stronger in females. This information is important for understanding clinical conditions that show sex differences. For example, females are vulnerable to stress-induced anxiety, with the prevalence of panic disorder being two- to three-fold higher than in males (Donner and Lowry, 2013). In the present study, females responded more strongly to subtle sensory changes than males, but were less able to inhibit the process, which may lead to excessive automatic responses to the ever-changing environment in females. A previous study demonstrated that the amplitude of the change-related cortical response correlated with trait anxiety (Tanahashi et al., 2016).
4.2 Effects of age
The present results showed that aging had a significant effect on the S1 amplitude, onset amplitude, and PPS. The increase in S1 and decrease in PPS indicate a decrease in inhibitory ability with age and a consequential increase in the S1 amplitude, which is consistent with previous AEP studies showing greater amplitudes in older than in younger subjects (Judd et al., 1992; Amenedo and Díaz, 1998; Bennett et al., 2004; Golob et al., 2007; Sörös et al., 2009) and less somatosensory gating in older subjects (Lenz et al., 2012; Terrasa et al., 2018). Although the mechanisms underlying reduced inhibition in the elderly remain unclear, a reduction in GABAergic neurons in the thalamus has been proposed (Woods and Clayworth, 1986; Amenedo and Díaz, 1998). Since inhibitory interneurons are ubiquitous components of all neural circuits (Isaacson and Scanziani, 2011), the auditory cortex is also a candidate site for age-related modulation. In this regard, a study using post-mortem brain tissues showed that glutamic acid decarboxylase expression, an enzyme that synthesizes GABA, and α3 subunit expression in STG were higher in younger than in older subjects (Pandya et al., 2019). Although the GABA receptor subtype involved in PPS and PPI in the present study was unclear, the results of Exp1 are consistent with these findings. Therefore, these findings support the inhibition deficit hypothesis of cognitive aging (Hasher and Zacks, 1988), in which decreased inhibition in the elderly results in impairments in various cognitive aspects, such as concentration and working memory. In a recent study, Alain et al. (2022) claimed that enhanced neural activity in the elderly was due to the deterioration of supra-modal brain areas. They recorded sensory evoked potentials in the somatosensory, visual, and auditory systems and found that older subjects showed significantly stronger responses in all modalities. In addition, older subjects who showed enhanced neural activity in one sensory system also exhibited enhanced activity in other systems, leading to the conclusion of a common cause of age-related decline in sensory systems. Therefore, in addition to the sensory cortex, brain areas outside of it, e.g., the prefrontal cortex, are a potential site of modulation.
An important result in the present study was that aging was resistant to PPI in Exp2, which is inconsistent with the generalized decline in inhibitory function with aging. Some cognitive processes are considered to be less susceptible to aging, e.g., automatic processes (Hasher and Zacks, 1979), and the inhibition of PPI noted in this study may be involved in these functions. The change-related cortical response used in the present study as the test response is automatic and elicited without subjects’ attention (Inui et al., 2010a) and is considered to be a fundamental function for survival (Inui et al., 2012). Although there are some paradigms for observing PPI, each PPI appears to reflect a similar automatic process (Ellwanger et al., 2003). A recent study using the trigeminal blink reflex showed that PPI of the early component of the blink reflex occurred in the first stage of brain processing in the principal nucleus of the trigeminal nerve and did not involve higher complex processes (Inui et al., 2023). In studies with PPI of the acoustic startle reflex, one reported decreased inhibition in older subjects (de Oliveira et al., 2023), while others reported no effect of age (Filion and Poje, 2003; Scholes and Martin-Iverson, 2009) or an inverted U-shaped relationship between age and PPI, with the strongest inhibition in middle age (Ellwanger et al., 2003). PPI of the trigeminal blink reflex by somatosensory inputs showed no age effect (Kofler et al., 2013). These findings are generally in line with the present results and support the view that whatever the paradigm, PPI reflects age-resistant inhibitory function. In this regard, the finding reported by Ueki et al. (2006) is important: PPI of the acoustic startle reflex was reduced in patients with Alzheimer’s disease, but enhanced in patients with mild cognitive impairments, which suggests that the measure differentiates abnormal cognitive impairment from normal aging and pathophysiological changes in the early stage from advanced stages.
4.3 Methodological consideration
The PPI of the acoustic startle reflex and the current PPI have similar age and sex biases, but different age and sex effects on the test response: the elderly showed a weaker acoustic startle reflex in previous studies (Ellwanger et al., 2003; de Oliveira et al., 2023), but not in the present study (Figure 3C), and females showed stronger test alone responses in this study, but not for the acoustic startle reflex (Kumari et al., 2004). The most important methodological difference may be that the acoustic startle reflex was the peripheral response in previous studies and the brain response in the present study. These findings indicate that muscle activity, but not the change-related response is affected by aging, while sex has an impact on the change-related response, but not muscle activity. These variables need to be considered when designing a study.
Regarding the effect of age on the magnitude of the test response, the weaker acoustic startle reflex and its decreased inhibition by the prepulse is problematic because both may be attributed to a reduction in peripheral activity. On the other hand, in Exp1 in the present study, age correlated with a stronger test response and decreased PPS, suggesting that a decline in the periphery was not the cause of decreased inhibition. However, the underlying mechanisms appear to be complex: in older individuals, reduced afferent inputs to the brain result in the loss of GABAergic inhibition, which, in turn, enhances brain activity (Harris et al., 2022). Therefore, the results obtained in Exp1 are in agreement with the central gain hypothesis (Gutiérrez et al., 1994; Turrigiano and Nelson, 2004). The weaker acoustic startle reflex in older subjects may be due to an age-related decline in the trigeminal blink system. This needs to be confirmed in further studies with older subjects and larger sample sizes than those in the present study.
5 Limitations
A limitation of the present study is that although the results obtained clearly showed sex effects in both measures, its significance has yet to be clarified due to the lack of information on menstruation or the contraceptive status in the present study. Another limitation is the component of evoked potentials tested. Previous studies reported differences in age and sex effects among components. In the present study, the peak-to-peak amplitude was employed to simplify the results, leaving the possibility that distinct effects for each component were not examined. As shown in Supplementary Tables S1, S2, there were some minor differences among components; however, it is difficult to establish whether these differences are meaningful. Large variations in P50 are problematic, with some data showing negative values. This is largely due to the filter setting in the present study; higher low-cut filters are necessary for P50, but are not appropriate for later components. We consider the peak-to-peak amplitude to be a good method due to the lack of baseline shift issues and the high signal-to-noise ratio.
6 Conclusion
The effects of age and sex on PPS and PPI of auditory change-related cortical responses were investigated. Sex showed a similar bias in both tests with stronger test responses and weaker inhibition in females. Age affected the two measures differently, with the test response being stronger and inhibition being weaker in older subjects in the PPS paradigm, while no age effects were observed in the test alone response amplitude or the degree of inhibition in the PPI paradigm. Although the neural mechanisms of PPS and PPI in humans currently remain unclear, some of the mechanisms proposed for PPS, such as the depletion of transmitters, are unlikely for PPI. In addition, the most effective interval of the two successive stimuli differs between PPS and PPI; 600 ms for PPS (Takeuchi et al., 2017) and 20–60 ms for PPI (Inui et al., 2016). The inhibition threshold also differs (Inui et al., 2016). Therefore, the two methods represent distinct inhibitory mechanisms. The present results showing the different impact of age on PPS and PPI and the lack of a correlation between the degree of PPS and PPI support this notion. In comparisons with studies using different paradigms, some of the findings obtained were congruent with the present results, whereas others were not. Since these measures are widely impaired in neuropsychiatric diseases (Adler et al., 1982; Jessen et al., 2001; Swerdlow et al., 2008; Santos-Carrasco and De la Casa, 2023), they are not of high diagnostic value. However, each method has a common and unique neural basis, which may be useful for insights into pathophysiology when considered in combination.
Data availability statement
The original contributions presented in the study are included in the article/Supplementary material, further inquiries can be directed to the corresponding author.
Ethics statement
The studies involving humans were approved by Ethics Committee of the Aichi Developmental Disability Center, Kasugai, Japan. The studies were conducted in accordance with the local legislation and institutional requirements. The participants provided their written informed consent to participate in this study.
Author contributions
KI: Conceptualization, Data curation, Formal analysis, Funding acquisition, Writing – original draft. NT: Data curation, Formal analysis, Methodology, Writing – review & editing. BB: Data curation, Formal analysis, Writing – review & editing. MS: Data curation, Formal analysis, Writing – review & editing. SS: Data curation, Formal analysis, Supervision, Writing – review & editing. TT: Data curation, Formal analysis, Writing – review & editing. MN: Data curation, Formal analysis, Supervision, Writing – review & editing. TW: Data curation, Formal analysis, Writing – review & editing. DS: Data curation, Formal analysis, Writing – review & editing. EM: Conceptualization, Data curation, Formal analysis, Supervision, Writing – review & editing. TK: Data curation, Formal analysis, Software, Supervision, Writing – review & editing.
Funding
The author(s) declare financial support was received for the research, authorship, and/or publication of this article. This study was supported by the Japan Society for the Promotion of Science KAKENHI Grants 18K07619 and 23K07026 to KI and 21K07480 to EM.
Conflict of interest
The authors declare that the research was conducted in the absence of any commercial or financial relationships that could be construed as a potential conflict of interest.
Publisher’s note
All claims expressed in this article are solely those of the authors and do not necessarily represent those of their affiliated organizations, or those of the publisher, the editors and the reviewers. Any product that may be evaluated in this article, or claim that may be made by its manufacturer, is not guaranteed or endorsed by the publisher.
Supplementary material
The Supplementary material for this article can be found online at: https://www.frontiersin.org/articles/10.3389/fnins.2024.1378619/full#supplementary-material
References
Aasen, I., Kolli, L., and Kumari, V. (2005). Sex effects in prepulse inhibition and facilitation of the acoustic startle response: implications for pharmacological and treatment studies. J. Psychopharmacol. 19, 39–45. doi: 10.1177/0269881105048890
Adler, L. E., Pachtman, E., Franks, R. D., Pecevich, M., Waldo, M. C., and Freedman, R. (1982). Neurophysiological evidence for a defect in neuronal mechanisms involved in sensory gating in schizophrenia. Biol. Psychiatry 17, 639–654.
Akiyama, L. F., Yamashiro, K., Inui, K., and Kakigi, R. (2011). Automatic cortical responses to sound movement: a magnetoencephalography study. Neurosci. Lett. 488, 183–187. doi: 10.1016/j.neulet.2010.11.025
Alain, C., Chow, R., Lu, J., Rabi, R., Sharma, V. V., Shen, D., et al. (2022). Aging enhances neural activity in auditory, visual, and somatosensory cortices: the common cause revisited. J. Neurosci. 42, 264–275. doi: 10.1523/JNEUROSCI.0864-21.2021
Amenedo, E., and Díaz, F. (1998). Effects of aging on middle-latency auditory evoked potentials: a cross-sectional study. Biol. Psychiatry 43, 210–219. doi: 10.1016/S0006-3223(97)00255-2
Bennett, I. J., Golob, E. J., and Starr, A. (2004). Age-related differences in auditory event-related potentials during a cued attention task. Clin. Neurophysiol. 115, 2602–2615. doi: 10.1016/j.clinph.2004.06.011
Braff, D. L. (2010). Prepulse inhibition of the startle reflex: a window on the brain in schizophrenia. Curr. Top. Behav. Neurosci. 4, 349–371. doi: 10.1007/7854_2010_61
Braff, D. L., Light, G. A., and Swerdlow, N. R. (2007). Prepulse inhibition and P50 suppression are both deficient but not correlated in schizophrenia patients. Biol. Psychiatry 61, 1204–1207. doi: 10.1016/j.biopsych.2006.08.015
Brenner, C. A., Edwards, C. R., Carroll, C. A., Kieffaber, P. D., and Hetrick, W. P. (2004). P50 and acoustic startle gating are not related in healthy participants. Psychophysiology 41, 702–708. doi: 10.1111/j.1469-8986.2004.00206.x
Brinkman, M. J., and Stauder, J. E. (2007). Development and gender in the P50 paradigm. Clin. Neurophysiol. 118, 1517–1524. doi: 10.1016/j.clinph.2007.04.002
Cahill, L. (2012). A half-truth is a whole lie: on the necessity of investigating sex influences on the brain. Endocrinology 153, 2541–2543. doi: 10.1210/en.2011-2167
Clementz, B. A., and Blumenfeld, L. D. (2001). Multichannel electroencephalographic assessment of auditory evoked response suppression in schizophrenia. Exp. Brain Res. 139, 377–390. doi: 10.1007/s002210100744
Cuenca-Bermejo, L., Prinetti, A., Kublickiene, K., Raparelli, V., Kautzky-Willer, A., Norris, C. M., et al. (2023). Fundamental neurochemistry review: old brain stories - influence of age and sex on the neurodegeneration-associated lipid changes. J. Neurochem. 166, 427–452. doi: 10.1111/jnc.15834
de Courten-Myers, G. M. (1999). The human cerebral cortex: gender differences in structure and function. J. Neuropathol. Exp. Neurol. 58, 217–226. doi: 10.1097/00005072-199903000-00001
de Oliveira, Y. G., Poltronieri, B. C., Woodruff, E., da Costa, B. F., and Panizzutti, R. A. (2023). Age-related changes in prepulse inhibition of the startle response. Front. Psych. 14:1145783. doi: 10.3389/fpsyt.2023.1145783
Delgado, A. R., and Prieto, G. (1996). Sex differences in visuospatial ability: do performance factors play such an important role? Mem. Cogn. 24, 504–510. doi: 10.3758/bf03200938
Donner, N. C., and Lowry, C. A. (2013). Sex differences in anxiety and emotional behavior. Pflugers Arch. 465, 601–626. doi: 10.1007/s00424-013-1271-7
Ellwanger, J., Geyer, M. A., and Braff, D. L. (2003). The relationship of age to prepulse inhibition and habituation of the acoustic startle response. Biol. Psychol. 62, 175–195. doi: 10.1016/s0301-0511(02)00126-6
Fendt, M., Li, L., and Yeomans, J. S. (2001). Brain stem circuits mediating prepulse inhibition of the startle reflex. Psychopharmacology 156, 216–224. doi: 10.1007/s002130100794
Fernández-Guasti, A., Fiedler, J. L., Herrera, L., and Handa, R. J. (2012). Sex, stress, and mood disorders: at the intersection of adrenal and gonadal hormones. Horm. Metab. Res. 44, 607–618. doi: 10.1055/s-0032-1312592
Fernando, P., Sommer, I. E. C., and Hasan, A. (2020). Do we need sex-oriented clinical practice guidelines for the treatment of schizophrenia? Curr. Opin. Psychiatry 33, 192–199. doi: 10.1097/YCO.0000000000000597
Filion, D. L., and Poje, A. B. (2003). Selective and non-selective attention effects on prepulse inhibition of startle: a comparison of task and no-task protocols. Biol. Psychol. 64, 283–296. doi: 10.1016/s0301-0511(03)00077-2
Fuerst, D. R., Gallinat, J., and Boutros, N. N. (2007). Range of sensory gating values and test-retest reliability in normal subjects. Psychophysiology 44, 620–626. doi: 10.1111/j.1469-8986.2007.00524.x
Gegenhuber, B., and Tollkuhn, J. (2020). Signatures of sex: sex differences in gene expression in the vertebrate brain. Wiley Interdiscip. Rev. Dev. Biol. 9:e348. doi: 10.1002/wdev.348
Golob, E. J., Irimajiri, R., and Starr, A. (2007). Auditory cortical activity in amnestic mild cognitive impairment: relationship to subtype and conversion to dementia. Brain 130, 740–752. doi: 10.1093/brain/awl375
Graham, F. K. (1975). The more or less startling effects of weak prestimulation. Psychophysiology 12, 238–248. doi: 10.1111/j.1469-8986.1975.tb01284.x
Gutiérrez, A., Khan, Z. U., Morris, S. J., and De Blas, A. L. (1994). Age-related decrease of GABAA receptor subunits and glutamic acid decarboxylase in the rat inferior colliculus. J. Neurosci. 14, 7469–7477. doi: 10.1523/JNEUROSCI.14-12-07469.1994
Harris, K. C., Dias, J. W., McClaskey, C. M., Rumschlag, J., Prisciandaro, J., and Dubno, J. R. (2022). Afferent loss, GABA, and central gain in older adults: associations with speech recognition in noise. J. Neurosci. 42, 7201–7212. doi: 10.1523/JNEUROSCI.0242-22.2022
Hasher, L., and Zacks, R. T. (1979). Automatic and effortful processes in memory. J. Exp. Psychol. Gen. 108, 356–388. doi: 10.1037/0096-3445.108.3.356
Hasher, L., and Zacks, R. T. (1988). Working memory, comprehension, and aging: a review and a new view. Psychol. Learn. Motiv. 22, 193–225. doi: 10.1016/S0079-7421(08)60041-9
Hetrick, W. P., Sandman, C. A., Bunney, W. E. Jr., Jin, Y., Potkin, S. G., and White, M. H. (1996). Gender differences in gating of the auditory evoked potential in normal subjects. Biol. Psychiatry 39, 51–58. doi: 10.1016/0006-3223(95)00067-4
Ingalhalikar, M., Smith, A., Parker, D., Satterthwaite, T. D., Elliott, M. A., Ruparel, K., et al. (2014). Sex differences in the structural connectome of the human brain. Proc. Natl. Acad. Sci. USA 111, 823–828. doi: 10.1073/pnas.1316909110
Inui, K., Itoh, Y., Bayasgalan, B., Shingaki, M., Taniguchi, T., Motomura, E., et al. (2023). Target site of prepulse inhibition of the trigeminal blink reflex in humans. J. Neurosci. 43, 261–269. doi: 10.1523/JNEUROSCI.1468-22.2022
Inui, K., Nakagawa, K., Nishihara, M., Motomura, E., and Kakigi, R. (2016). Inhibition in the human auditory cortex. PLoS One 11:e0155972. doi: 10.1371/journal.pone.0155972
Inui, K., Takeuchi, N., Sugiyama, S., Motomura, E., and Nishihara, M. (2018). GABAergic mechanisms involved in the prepulse inhibition of auditory evoked cortical responses in humans. PLoS One 13:e0190481. doi: 10.1371/journal.pone.0190481
Inui, K., Tsuruhara, A., Kodaira, M., Motomura, E., Tanii, H., Nishihara, M., et al. (2012). Prepulse inhibition of auditory change-related cortical responses. BMC Neurosci. 13:135. doi: 10.1186/1471-2202-13-135
Inui, K., Urakawa, T., Yamashiro, K., Otsuru, N., Nishihara, M., Takeshima, Y., et al. (2010a). Non-linear laws of echoic memory and auditory change detection in humans. BMC Neurosci. 11:80. doi: 10.1186/1471-2202-11-80
Inui, K., Urakawa, T., Yamashiro, K., Otsuru, N., Takeshima, Y., Nishihara, M., et al. (2010b). Echoic memory of a single pure tone indexed by change-related brain activity. BMC Neurosci. 11:135. doi: 10.1186/1471-2202-11-135
Isaacson, J. S., and Scanziani, M. (2011). How inhibition shapes cortical activity. Neuron 72, 231–243. doi: 10.1016/j.neuron.2011.09.027
Jessen, F., Kucharski, C., Fries, T., Papassotiropoulos, A., Hoenig, K., Maier, W., et al. (2001). Sensory gating deficit expressed by a disturbed suppression of the P50 event-related potential in patients with Alzheimer's disease. Am. J. Psychiatry 158, 1319–1321. doi: 10.1176/appi.ajp.158.8.1319
Jovanovic, T., Szilagyi, S., Chakravorty, S., Fiallos, A. M., Lewison, B. J., Parwani, A., et al. (2004). Menstrual cycle phase effects on prepulse inhibition of acoustic startle. Psychophysiology 41, 401–406. doi: 10.1111/1469-8986.2004.00166.x
Judd, L. L., McAdams, L., Budnick, B., and Braff, D. L. (1992). Sensory gating deficits in schizophrenia: new results. Am. J. Psychiatry 149, 488–493. doi: 10.1176/ajp.149.4.488
Kirischuk, S., Clements, J. D., and Grantyn, R. (2002). Presynaptic and postsynaptic mechanisms underlie paired pulse depression at single GABAergic boutons in rat collicular cultures. J. Physiol. 543, 99–116. doi: 10.1113/jphysiol.2002.021576
Kodaira, M., Tsuruhara, A., Motomura, E., Tanii, H., Inui, K., and Kakigi, R. (2013). Effects of acute nicotine on prepulse inhibition of auditory change-related cortical responses. Behav. Brain Res. 256, 27–35. doi: 10.1016/j.bbr.2013.07.045
Kodsi, M. H., and Swerdlow, N. R. (1995). Ventral pallidal GABA-A receptors regulate prepulse inhibition of acoustic startle. Brain Res. 684, 26–35. doi: 10.1016/0006-8993(95)00372-w
Kofler, M., Kumru, H., Schaller, J., and Saltuari, L. (2013). Blink reflex prepulse inhibition and excitability recovery: influence of age and sex. Clin. Neurophysiol. 124, 126–135. doi: 10.1016/j.clinph.2012.07.001
Kofler, M., Müller, J., Reggiani, L., and Valls-Solé, J. (2001). Influence of gender on auditory startle responses. Brain Res. 921, 206–210. doi: 10.1016/s0006-8993(01)03120-1
Kumari, V., Aasen, I., and Sharma, T. (2004). Sex differences in prepulse inhibition deficits in chronic schizophrenia. Schizophr. Res. 69, 219–235. doi: 10.1016/j.schres.2003.09.010
Kumari, V., Jeffrey, A., Gray, J. A., Gupta, P., Luscher, S., and Sharma, T. (2003). Sex differences in prepulse inhibition of the acoustic startle response. Personal. Individ. Differ. 35, 733–742. doi: 10.1016/S0191-8869(02)00266-0
Kumari, V., Konstantinou, J., Papadopoulos, A., Aasen, I., Poon, L., Halari, R., et al. (2010). Evidence for a role of progesterone in menstrual cycle-related variability in prepulse inhibition in healthy young women. Neuropsychopharmacology 35, 929–937. doi: 10.1038/npp.2009.195
Lee, Y., López, D. E., Meloni, E. G., and Davis, M. (1996). A primary acoustic startle pathway: obligatory role of cochlear root neurons and the nucleus reticularis pontis caudalis. J. Neurosci. 16, 3775–3789. doi: 10.1523/JNEUROSCI.16-11-03775.1996
Lenz, M., Tegenthoff, M., Kohlhaas, K., Stude, P., Höffken, O., Gatica Tossi, M. A., et al. (2012). Increased excitability of somatosensory cortex in aged humans is associated with impaired tactile acuity. J. Neurosci. 32, 1811–1816. doi: 10.1523/JNEUROSCI.2722-11.2012
Light, G. A., and Braff, D. L. (2001). Measuring P50 suppression and prepulse inhibition in a single recording session. Am. J. Psychiatry 158, 2066–2068. doi: 10.1176/appi.ajp.158.12.2066
Lijffijt, M., Moeller, F. G., Boutros, N. N., Burroughs, S., Lane, S. D., Steinberg, J. L., et al. (2009a). The role of age, gender, education, and intelligence in P50, N100, and P200 auditory sensory gating. J. Psychophysiol. 23, 52–62. doi: 10.1027/0269-8803.23.2.52
Lijffijt, M., Moeller, F. G., Boutros, N. N., Steinberg, J. L., Meier, S. L., Lane, S. D., et al. (2009b). Diminished P50, N100 and P200 auditory sensory gating in bipolar I disorder. Psychiatry Res. 167, 191–201. doi: 10.1016/j.psychres.2008.04.001
McAlonan, G. M., Daly, E., Kumari, V., Critchley, H. D., van Amelsvoort, T., Suckling, J., et al. (2002). Brain anatomy and sensorimotor gating in Asperger's syndrome. Brain 125, 1594–1606. doi: 10.1093/brain/awf150
Naysmith, L. F., Williams, S. C. R., and Kumari, V. (2022). The influence of stimulus onset asynchrony, task order, sex and hormonal contraception on prepulse inhibition and prepulse facilitation: methodological considerations for drug and imaging research. J. Psychopharmacol. 36, 1234–1242. doi: 10.1177/02698811221133469
Nishihara, M., Inui, K., Motomura, E., Otsuru, N., Ushida, T., and Kakigi, R. (2011). Auditory N1 as a change-related automatic response. Neurosci. Res. 71, 145–148. doi: 10.1016/j.neures.2011.07.004
O’Donovan, M. J., and Rinzel, J. (1997). Synaptic depression: a dynamic regulator of synaptic communication with varied functional roles. Trends Neurosci. 20, 431–433. doi: 10.1016/s0166-2236(97)01124-7
Ohoyama, K., Motomura, E., Inui, K., Nishihara, M., Otsuru, N., Oi, M., et al. (2012). Memory-based pre-attentive auditory N1 elicited by sound movement. Neurosci. Res. 73, 248–251. doi: 10.1016/j.neures.2012.04.003
Otsuru, N., Inui, K., Yamashiro, K., Urakawa, T., Keceli, S., and Kakigi, R. (2011). Effects of prior sustained tactile stimulation on the somatosensory response to the sudden change of intensity in humans: an magnetoencephalography study. Neuroscience 182, 115–124. doi: 10.1016/j.neuroscience.2011.03.019
Pandya, M., Palpagama, T. H., Turner, C., Waldvogel, H. J., Faull, R. L., and Kwakowsky, A. (2019). Sex- and age-related changes in GABA signaling components in the human cortex. Biol. Sex Differ. 10:5. doi: 10.1186/s13293-018-0214-6
Rasco, L., Skinner, R. D., and Garcia-Rill, E. (2000). Effect of age on sensory gating of the sleep state-dependent P1/P50 midlatency auditory evoked potential. Sleep Res. Online 3, 97–105.
Rey-Mermet, A., and Gade, M. (2018). Inhibition in aging: what is preserved? What declines? A meta-analysis. Psychon. Bull. Rev. 25, 1695–1716. doi: 10.3758/s13423-017-1384-7
Sandrini, G., Serrao, M., Rossi, P., Romaniello, A., Cruccu, G., and Willer, J. C. (2005). The lower limb flexion reflex in humans. Prog. Neurobiol. 77, 353–395. doi: 10.1016/j.pneurobio.2005.11.003
Santos-Carrasco, D., and De la Casa, L. G. (2023). Prepulse inhibition deficit as a transdiagnostic process in neuropsychiatric disorders: a systematic review. BMC Psychol. 11:226. doi: 10.1186/s40359-023-01253-9
Scholes, K. E., and Martin-Iverson, M. T. (2009). Relationships between prepulse inhibition and cognition are mediated by attentional processes. Behav. Brain Res. 205, 456–467. doi: 10.1016/j.bbr.2009.07.031
Sörös, P., Teismann, I. K., Manemann, E., and Lütkenhöner, B. (2009). Auditory temporal processing in healthy aging: a magnetoencephalographic study. BMC Neurosci. 10:34. doi: 10.1186/1471-2202-10-34
Swerdlow, N. R. (2013). Update: studies of prepulse inhibition of startle, with particular relevance to the pathophysiology or treatment of Tourette syndrome. Neurosci. Biobehav. Rev. 37, 1150–1156. doi: 10.1016/j.neubiorev.2012.09.002
Swerdlow, N. R., Auerbach, P., Monroe, S. M., Hartston, H., Geyer, M. A., and Braff, D. L. (1993). Men are more inhibited than women by weak prepulses. Biol. Psychiatry 34, 253–260. doi: 10.1016/0006-3223(93)90079-s
Swerdlow, N. R., Hartman, P. L., and Auerbach, P. P. (1997). Changes in sensorimotor inhibition across the menstrual cycle: implications for neuropsychiatric disorders. Biol. Psychiatry 41, 452–460. doi: 10.1016/S0006-3223(96)00065-0
Swerdlow, N. R., Weber, M., Qu, Y., Light, G. A., and Braff, D. L. (2008). Realistic expectations of prepulse inhibition in translational models for schizophrenia research. Psychopharmacology 199, 331–388. doi: 10.1007/s00213-008-1072-4
Takeuchi, N., Fujita, K., Kinukawa, T., Sugiyama, S., Kanemoto, K., Nishihara, M., et al. (2021a). Test-retest reliability of paired pulse suppression paradigm using auditory change-related response. J. Neurosci. Methods 352:109087. doi: 10.1016/j.jneumeth.2021.109087
Takeuchi, N., Fujita, K., Taniguchi, T., Kinukawa, T., Sugiyama, S., Kanemoto, K., et al. (2023). Auditory sensory suppression and personality traits using bear-Fedio inventory. Curr. Psychol. 43, 9598–9601. doi: 10.1007/s12144-023-05082-2
Takeuchi, N., Kinukawa, T., Sugiyama, S., Inui, K., and Nishihara, M. (2021b). Test-retest reliability of prepulse inhibition paradigm using auditory evoked potentials. Neurosci. Res. 170, 187–194. doi: 10.1016/j.neures.2020.08.011
Takeuchi, N., Sugiyama, S., Inui, K., Kanemoto, K., and Nishihara, M. (2017). New paradigm for auditory paired pulse suppression. PLoS One 12:e0177747. doi: 10.1371/journal.pone.0177747
Takeuchi, N., Sugiyama, S., Inui, K., Kanemoto, K., and Nishihara, M. (2018, 2018). Long-latency suppression of auditory and somatosensory change-related cortical responses. PLoS One 13:e0199614. doi: 10.1371/journal.pone.0199614
Tanahashi, M., Motomura, E., Inui, K., Ohoyama, K., Tanii, H., Konishi, Y., et al. (2016). Auditory change-related cerebral responses and personality traits. Neurosci. Res. 103, 34–39. doi: 10.1016/j.neures.2015.08.005
Tanaka, E., Inui, K., Kida, T., and Kakigi, R. (2009a). Common cortical responses evoked by appearance, disappearance and change of the human face. BMC Neurosci. 10:38. doi: 10.1186/1471-2202-10-38
Tanaka, E., Kida, T., Inui, K., and Kakigi, R. (2009b). Change-driven cortical activation in multisensory environments: an MEG study. NeuroImage 48, 464–474. doi: 10.1016/j.neuroimage.2009.06.037
Terrasa, J. L., Montoya, P., González-Roldán, A. M., and Sitges, C. (2018). Inhibitory control impairment on somatosensory gating due to aging: an event-related potential study. Front. Hum. Neurosci. 12:280. doi: 10.3389/fnhum.2018.00280
Turpin, G. (1986). Effects of stimulus intensity on autonomic responding: the problem of differentiating orienting and defense reflexes. Psychophysiology 23, 1–14. doi: 10.1111/j.1469-8986.1986.tb00583.x
Turrigiano, G. G., and Nelson, S. B. (2004). Homeostatic plasticity in the developing nervous system. Nat. Rev. Neurosci. 5, 97–107. doi: 10.1038/nrn1327
Ueki, A., Goto, K., Sato, N., Iso, H., and Morita, Y. (2006). Prepulse inhibition of acoustic startle response in mild cognitive impairment and mild dementia of Alzheimer type. Psychiatry Clin. Neurosci. 60, 55–62. doi: 10.1111/j.1440-1819.2006.01460.x
Uhl, M., Schmeisser, M. J., and Schumann, S. (2022). The sexual dimorphic synapse: from spine density to molecular composition. Front. Mol. Neurosci. 15:818390. doi: 10.3389/fnmol.2022.818390
Urakawa, T., Inui, K., Yamashiro, K., and Kakigi, R. (2010a). Cortical dynamics of the visual change detection process. Psychophysiology 47, 905–912. doi: 10.1111/j.1469-8986.2010.00987.x
Urakawa, T., Inui, K., Yamashiro, K., Tanaka, E., and Kakigi, R. (2010b). Cortical dynamics of visual change detection based on sensory memory. NeuroImage 52, 302–308. doi: 10.1016/j.neuroimage.2010.03.071
Vega, P., Barbeito, S., Ruiz de Azúa, S., Martínez-Cengotitabengoa, M., González-Ortega, I., Saenz, M., et al. (2011). Bipolar disorder differences between genders: special considerations for women. Womens Health 7, 663–674. doi: 10.2217/whe.11.71
Williams, C. M., Peyre, H., Toro, R., and Ramus, F. (2021). Neuroanatomical norms in the UK biobank: the impact of allometric scaling, sex, and age. Hum. Brain Mapp. 42, 4623–4642. doi: 10.1002/hbm.25572
Woods, D. L., and Clayworth, C. C. (1986). Age-related changes in human middle latency auditory evoked potentials. Electroencephalogr. Clin. Neurophysiol. 65, 297–303. doi: 10.1016/0168-5597(86)90008-0
Xin, Z., Gu, S., Wang, W., Lei, Y., and Li, H. (2021). Acute stress and gender effects in sensory gating of the auditory evoked potential in healthy subjects. Neural Plast. 2021:8529613. doi: 10.1155/2021/8529613
Yamashiro, K., Inui, K., Otsuru, N., and Kakigi, R. (2011). Change-related responses in the human auditory cortex: an MEG study. Psychophysiology 48, 23–30. doi: 10.1111/j.1469-8986.2010.01038.x
Yamashiro, K., Inui, K., Otsuru, N., Kida, T., and Kakigi, R. (2009a). Automatic auditory off-response in humans: an MEG study. Eur. J. Neurosci. 30, 125–131. doi: 10.1111/j.1460-9568.2009.06790.x
Yamashiro, K., Inui, K., Otsuru, N., Kida, T., and Kakigi, R. (2009b). Somatosensory off-response in humans: an MEG study. NeuroImage 44, 1363–1368. doi: 10.1016/j.neuroimage.2008.11.003
Zachry, J. E., Nolan, S. O., Brady, L. J., Kelly, S. J., Siciliano, C. A., and Calipari, E. S. (2021). Sex differences in dopamine release regulation in the striatum. Neuropsychopharmacology 46, 491–499. doi: 10.1038/s41386-020-00915-1
Zhang, J., Forkstam, C., Engel, J. A., and Svensson, L. (2000). Role of dopamine in prepulse inhibition of acoustic startle. Psychopharmacology 149, 181–188. doi: 10.1007/s002130000369
Keywords: aging, change-related potential, P50 gating, sensory suppression, sensory gating
Citation: Inui K, Takeuchi N, Borgil B, Shingaki M, Sugiyama S, Taniguchi T, Nishihara M, Watanabe T, Suzuki D, Motomura E and Kida T (2024) Age and sex effects on paired-pulse suppression and prepulse inhibition of auditory evoked potentials. Front. Neurosci. 18:1378619. doi: 10.3389/fnins.2024.1378619
Edited by:
Claude Alain, Rotman Research Institute (RRI), CanadaReviewed by:
Dolores E. López García, University of Salamanca, SpainVictoria M. Bajo Lorenzana, University of Oxford, United Kingdom
Copyright © 2024 Inui, Takeuchi, Borgil, Shingaki, Sugiyama, Taniguchi, Nishihara, Watanabe, Suzuki, Motomura and Kida. This is an open-access article distributed under the terms of the Creative Commons Attribution License (CC BY). The use, distribution or reproduction in other forums is permitted, provided the original author(s) and the copyright owner(s) are credited and that the original publication in this journal is cited, in accordance with accepted academic practice. No use, distribution or reproduction is permitted which does not comply with these terms.
*Correspondence: Koji Inui, aW51aUBpbnN0LWhzYy5qcA==