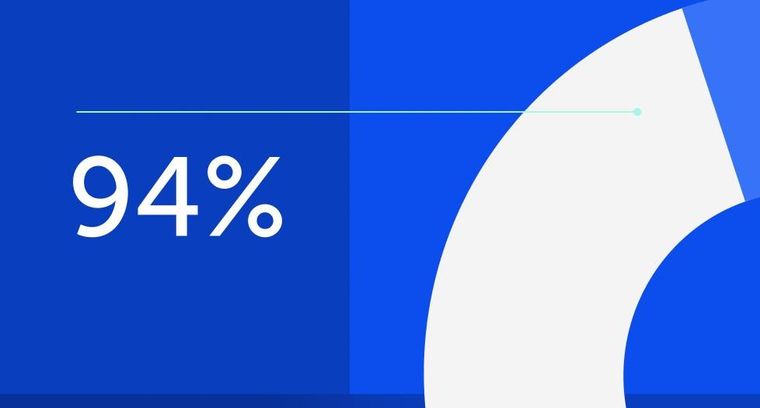
94% of researchers rate our articles as excellent or good
Learn more about the work of our research integrity team to safeguard the quality of each article we publish.
Find out more
SYSTEMATIC REVIEW article
Front. Neurosci., 15 February 2024
Sec. Neuroprosthetics
Volume 18 - 2024 | https://doi.org/10.3389/fnins.2024.1352212
This article is part of the Research TopicInnovative approaches to promote stroke recoveryView all 18 articles
Background: Lower extremity motor dysfunction is one of the most severe consequences after stroke, restricting functional mobility and impairing daily activities. Growing evidence suggests that repetitive transcranial magnetic stimulation (rTMS) can improve stroke patients’ lower extremity motor function. However, there is still controversy about the optimal rTMS protocol. Therefore, we compared and analyzed the effects of different rTMS protocols on lower extremity motor function in stroke patients using network meta-analysis (NMA).
Methods: We systematically searched CNKI, WanFang, VIP, CBM, PubMed, Embase, Web of Science, and Cochrane Library databases (from origin to 31 December 2023). Randomized controlled trials (RCTs) or crossover RCTs on rTMS improving lower extremity motor function in stroke patients were included. Two authors independently completed article screening, data extraction, and quality assessment. RevMan (version 5.4) and Stata (version 17.0) were used to analyze the data.
Results: A total of 38 studies with 2,022 patients were eligible for the NMA. The interventions included HFrTMS-M1, LFrTMS-M1, iTBS-Cerebellum, iTBS-M1, dTMS-M1, and Placebo. The results of NMA showed that LFrTMS-M1 ranked first in FMA-LE and speed, and HFrTMS-M1 ranked first in BBS, TUGT, and MEP amplitude. The subgroup analysis of FMA-LE showed that HFrTMS-M1 was the best stimulation protocol for post-stroke time > 1 month, and LFrTMS-M1 was the best stimulation protocol for post-stroke time ≤ 1 month.
Conclusion: Considering the impact of the stroke phase on the lower extremity motor function, the current research evidence shows that HFrTMS-M1 may be the preferred stimulation protocol to improve the lower extremity motor function of patients for post-stroke time > 1 month, and LFrTMS-M1 for post-stroke time ≤ 1 month. However, the above conclusion needs further analysis and validation by more high-quality RCTs.
Systematic Review Registration:www.crd.york.ac.uk/prospero/, identifier (CRD42023474215).
Stroke is a local brain dysfunction caused by acute cerebrovascular disease (Park et al., 2011). By 2019, the proportion of stroke patients had increased to 2.58% among residents aged ≥40 years in China (Wang et al., 2022). Stroke is the leading cause of adult death and disability in China, with the five characteristics of high incidence, high disability rate, high mortality rate, high recurrence rate, and high economic burden, which has seriously endangered the health of Chinese people. With the development of medical technology, the mortality rate of stroke has decreased year by year, but 72% of the survivors still have lower extremity dysfunction (Ng and Hui-Chan, 2010), which affects the walking function of patients. Walking dysfunction is one of the most severe consequences of stroke. Nearly 30% of stroke patients are unable to walk even in the chronic recovery stage (Park et al., 2011), which significantly affects the patients’ social interactions and can lead to lifelong disability in severe cases (Park and An, 2016). Therefore, improving the motor function of the lower extremity, restoring independent walking as soon as possible, and improving activities of daily living (ADL) are the problems that many stroke patients are eager to solve urgently. However, pharmacological therapy (Mead et al., 2013) and traditional rehabilitation therapies [e.g., neurodevelopmental therapy (Langhammer and Stanghelle, 2011), proprioceptive neuromuscular facilitation (Eng and Tang, 2007), and electromyography biofeedback (Woodford and Price, 2007)] seem to have limited effects on improving motor function of the lower extremity after stroke. Therefore, a more effective treatment is needed.
Repetitive transcranial magnetic stimulation (rTMS), one of the brain stimulation techniques without any trauma, can induce neuroplastic changes and promote brain function restoration (Nathou et al., 2018). At present, the interhemispheric competition (IHC) model is the primary theoretical basis for applying rTMS in stroke rehabilitation. This model suggests that stroke destroys the balance of mutual inhibition of the bilateral cerebral hemispheres through the corpus callosum, resulting in the decreased inhibition of the ipsilateral hemisphere to the contralateral hemisphere and the increased inhibition of the contralateral hemisphere to the ipsilateral hemisphere (Di Pino et al., 2014). Therefore, in clinical practice, there are two main ways to use rTMS to promote functional recovery after stroke. One is to reduce the excitability of the contralateral hemisphere through low-frequency (≤1 Hz) rTMS to reduce the inhibitory effect of the contralateral hemisphere on the ipsilateral hemisphere. The other is to restore the balance of competitive inhibition between the bilateral cerebral hemispheres by stimulating the ipsilateral hemisphere with high-frequency (≥5 Hz) rTMS to increase its excitability (George and Aston-Jones, 2010). Both stimulation modes have been used to treat motor/non-motor dysfunction after stroke.
Theta burst stimulation (TBS), a novel mode of rTMS, saves time in the rehabilitation of motor function after stroke (Huang et al., 2005). There are two types of TBS: intermittent TBS (iTBS) and continuous TBS (cTBS), which generate excitatory and inhibitory effects, respectively (Larson et al., 1986; Huang et al., 2011). Compared with conventional rTMS protocols, TBS provides significant advantages due to its reduced stimulation time (Chung et al., 2015) and long-lasting effects with lower-intensity stimulation (Cárdenas-Morales et al., 2010). Deep transcranial magnetic stimulation (dTMS) is a new non-invasive neuromodulation technique based on rTMS technology, which uses a different coil type (Hesed coil). dTMS has the advantages of deeper and wider stimulation, more precise localization, and less damage to the superficial cortex than conventional TMS (Roth et al., 2014). The primary motor cortex (M1) is typically the target of rTMS. However, some studies have shown that the cerebellum is one of the alternative targets of M1, and rTMS targeting the cerebellum can also improve motor function in stroke patients (Wessel and Hummel, 2018). In conclusion, rTMS can regulate the asymmetry of excitability between the bilateral cerebral hemispheres by changing the stimulation mode, stimulation target, stimulation frequency, and coil type to promote the recovery of lower extremity motor dysfunction after stroke (Kesikburun, 2022).
The effect of rTMS on lower extremity motor function in stroke patients has been demonstrated in previous meta-analysis (Li et al., 2018; Tung et al., 2019). However, an important drawback is that conventional meta-analysis can only compare two interventions simultaneously. At the same time, in these studies, the intervention protocols of the experimental group were roughly classified, and the effects of different stimulation frequencies, stimulation targets, stimulation modes, and post-stroke times on treatment effects were not comprehensively considered. Although Fan et al. (2021) conducted a detailed systematic review of rTMS to improve lower extremity motor function in stroke patients, this study did not consider the new stimulation mode-iTBS, the new stimulation target-cerebellum, and the effect of stroke phase on the efficacy of rTMS.
Network meta-analysis (NMA) is developed from conventional meta-analysis, and its primary function is to comprehensively evaluate and rank multiple interventions simultaneously (Rouse et al., 2017). Therefore, we took the stimulation mode, stimulation frequency, and stimulation target of rTMS into account and summarized the following five different rTMS protocols: high-frequency rTMS-M1 (HFrTMS-M1), low-frequency rTMS-M1 (LFrTMS-M1), iTBS-Cerebellum, iTBS-M1, and dTMS-M1. Then, we compared and analyzed the effects of different protocols on lower extremity motor dysfunction in stroke patients by NMA. In addition, considering the effects of the stroke phase on the efficacy of rTMS at different protocols, we also carried out a subgroup analysis for FMA-LE according to the phase of the stroke to provide sufficient evidence for future clinical practice.
Our NMA was conducted using the Cochrane Handbook for Systematic Reviews of Interventions, and the findings were reported according to the Preferred Reporting Items for Systematic Reviews and Meta-Analyzes (PRISMA) statement (Page et al., 2021). This NMA was prospectively registered in PROSPERO (registration ID: CRD42023474215).
Two authors separately searched for randomized controlled trials (RCTs) and crossover RCTs about rTMS improving lower extremity motor function in stroke patients from China National Knowledge Infrastructure (CNKI), Wanfang database, VIP database, China Biomedical Literature Database (CBM), PubMed, Embase, Web of Science, and Cochrane Library. The search time limit was from the establishment of the database to 31 December 2023. By combining medical subject headings (MeSH) with free words using Boolean logic operators, we integrated the following terms for a comprehensive search: “Stroke,” “cerebrovascular accident,” “CVA,” “Brain Vascular Accident,” “hemiplegia,” “apoplexy,” “hemiparesis,” “repetitive transcranial magnetic stimulation,” “Transcranial Magnetic Stimulation,” “TMS,” “rTMS,” “Theta burst stimulation,” “θ-brust stimulation,” “random,” “randomized controlled trial,” “RCT.” In addition, we also reviewed meta-analysis, reviews, and references of the included studies to supplement the search. PubMed was used as an example, and the specific search strategy was provided in Supplementary Table 1.
Eligibility criteria were defined in accordance with the PICOS framework (Hutton et al., 2015).
1. Populations: Stroke patients with lower extremity dysfunction who were diagnosed according to the stroke diagnostic criteria formulated by The Fourth National Cerebrovascular Disease Conference in 1995.
2. Interventions: HFrTMS-M1, LFrTMS-M1, iTBS-Cerebellum, iTBS-M1, and dTMS-M1.
3. Comparators: The placebo included conventional rehabilitation and sham rTMS (or conventional rehabilitation alone). Sham rTMS refers to the analog sound without any effective magnetic stimulation. Conventional rehabilitation, such as physiotherapy, occupational therapy, physical therapy, treadmill training, motor imagery practice, task-oriented training, and mirror therapy, was acceptable as cointervention.
4. Outcomes: The primary outcome indicator was the Fugl-Meyer Assessment of Lower Extremity (FMA-LE). The secondary outcome indicators included the Berg Balance Scale (BBS), Timed Up and Go Test (TUGT), Motor Evoked Potential amplitude (MEP amplitude), and speed.
5. Study designs: RCTs or crossover RCTs.
1. Patients with lower extremity motor dysfunction were not caused by stroke but by traumatic brain injury, cerebral palsy, Parkinson’s disease, and other diseases.
2. Conference abstracts, researcher protocols, reviews, meta-analysis, dissertations, and non-RCTs (e.g., case reports, observational studies, cross-sectional studies, and studies without a control group).
3. Lack of outcome indicators related to the lower extremity motor function.
4. Studies with more patients withdrawing midway.
5. Studies that could not be downloaded.
6. Studies with incomplete outcome data and contacting the authors three times without response.
7. Repeatedly published studies.
First, two authors (CSW and QZ) used EndnoteX9 software to eliminate duplicate articles. Then, they screened out articles that did not meet the criteria by reading their titles and abstracts. Finally, they browsed the full text to select articles that met the criteria. In case of any disagreement during the review process, the decision was made by consultation between the two authors or by joint decision with the third author (LLZ).
Two authors (DYZ and YNX) independently reviewed all articles and extracted data. The extracted data included basic published information: first author’s name, year of publication, country of origin, participant characteristics (age and sample size), intervention characteristics (intervention protocol, coil type, rTMS target, rTMS frequency, rTMS intensity, No. of pulses, and duration of intervention), and outcome indicators (FMA-LE, BBS, TUGT, MEP amplitude, and speed) at baseline and at last observation to obtain their change scores. The collected data were put into an Excel spreadsheet and cross-checked by two authors (DYZ and YNX). In case of disagreement during data extraction, the third author (ZJL) participated in discussion and decision-making.
Two authors (CLW and SZW) independently assessed the risk of bias for the included articles through the Cochrane Risk of Bias Tool (Savović et al., 2014), which mainly included seven indicators: (I) Random sequence generation; (II) Allocation concealment; (III) Blinding of participants and personnel; (IV) Blinding of outcome assessment; (V) Incomplete outcome data; (VI) Selective reporting; (VII) Other bias. Assessment indicators were rated “low risk,” “unclear,” or “high risk” based on the available information. If there was any dispute during the evaluation, the third author (ZJL) would participate in the discussion and make decisions together.
Odds ratio (OR) for binary variables and mean difference (MD) for continuous variables were used as the effect indicators, and the 95% confidence interval (CI) was provided for each effect size. If a particular study used different methods or scales to measure the same outcome, standardized MD (SMD) was calculated instead of MD. We calculated the difference before and after treatment for continuous variable indicators and the standard deviation (SD) according to the method provided in 16.1.3.2 of Cochrane Handbook 5.0.2 and then performed the statistical analysis. We used RevMan (version 5.4) for pairwise meta-analysis. The p-value of the chi-square test and the I2 index from the heterogeneity test were used to express the level of statistical heterogeneity. Different effect models were selected according to the test data’s heterogeneity level. When the level of heterogeneity was low (p ≥ 0.1, I2 ≤ 50%), we selected the fixed effect model for analysis. Otherwise, a random effect model (p < 0.1, I2 > 50%) was used (Higgins et al., 2003; Tufanaru et al., 2015).
We used Stata (version 17.0) to perform the NMA and produce various charts, such as network meta-analysis diagrams of eligible comparisons, surface under the cumulative ranking area (SUCRA), funnel plot of publication bias, etc (Shim et al., 2017). When there were closed loops between interventions, we first needed to assess global inconsistency. If p > 0.05, the inconsistent model was not significant, and the consistent model was selected (White et al., 2012). We used a node-splitting approach to assess local inconsistency (Dias et al., 2010). At the same time, it was also necessary to evaluate the loop inconsistency and calculate the inconsistency factors (IF) and 95% CI for each closed loop. If the lower limit of 95% CI included or was close to 0, the consistency between the direct and indirect comparison results was good; otherwise, the closed loop was considered to have apparent inconsistency. If no closed loops existed between interventions, the consistency model was used directly for analysis. We used the SUCRA to rank interventions. The closer SUCRA was to 100%, the better the effect of the intervention. Finally, the publication bias of the included articles was evaluated using the funnel plot of publication bias and Egger’s test. Asymmetry in the funnel plot of publication bias and p < 0.05 in Egger’s test indicated publication bias in the included articles (Fleiss, 1993).
We strictly searched the above 8 databases according to the inclusion and exclusion criteria and preliminarily obtained 12,814 articles. After the duplicate articles were removed, 10,166 articles remained in the database. By reading the titles and abstracts of the articles, we excluded articles that did not meet the inclusion criteria, leaving 176 remaining in the database. By reading the full text, we again excluded 138 articles. Ultimately, 38 articles met our study requirements. Figure 1 shows the article search process and results.
Figure 1. Flow diagram of the eligible studies selection process. CNKI, China National Knowledge Infrastructure; WanFang, WanFang Knowledge Service Platform; VIP, Chinese Scientific Journals Database; CBM, Chinese Biomedical Literature Service System; n, number of publications.
Characteristics of studies adopted are shown in Table 1, published between 2012 and 2023. We finally included 38 studies with a total of 2,022 patients. 4 studies were crossover RCTs, and 34 studies were RCTs. Among the included studies, most of them were carried out in China (28/38), and the others were conducted in Korea (5/38), Italy (2/38), Egypt (1/38), India (1/38), and Japan (1/38). Outcome indicators included FMA-LE (29 studies), BBS (11 studies), TUGT (8 studies), MEP amplitude (8 studies), and speed (13 studies). Interventions included HFrTMS-M1 (16 studies), LFrTMS-M1 (18 studies), iTBS-Cerebellum (5 studies), iTBS-M1 (1 study), and dTMS-M1 (2 studies).
25 studies (65.8%) had a low risk of bias concerning random sequence generation. 9 studies (23.7%) had a low risk of bias concerning allocation concealment. 24 studies (63.2%) had a low risk of bias concerning blinding of participants and personnel. 27 studies (71.1%) had a low risk of bias concerning blinding of outcome assessment. 37 studies (97.4%) had a low risk of bias concerning incomplete outcome data. 38 studies (100.0%) had a low risk of bias concerning selective reporting. Other biases were not known. Details of the evaluation of bias results for the included articles are shown in Figure 2.
Figure 2. Quality assessment of selected studies by the Cochrane Risk Of Bias Tool. (A) Risk of bias graph: review authors’ judgments about each risk of bias item presents as percentages across all included studies. (B) Risk of bias summary: review authors’ judgments about each risk of bias item for each included study.
A pairwise meta-analysis was used to compare the two interventions comprehensively. We carried out 6 pairwise meta-analysis to compare FMA-LE, 6 to compare FMA-LE (post-stroke time > 1 month), 3 to compare FMA-LE (post-stroke time ≤ 1 month), 5 to compare BBS, 4 to compare TUGT, 4 to compare MEP amplitude, and 2 to compare speed, respectively, which can be summarily seen in Table 2. The detailed results of pairwise meta-analysis are shown in Supplementary Figures 1–7.
Figure 3 shows the network meta-analysis diagrams of eligible comparisons, where the blue circles represent the different interventions. The circle size represents the sample size. The straight line between the two circles represents a direct comparison between the two different interventions. The thicker the solid line, the greater the number of studies in that pairwise comparison.
Figure 3. Network meta-analysis diagrams of eligible comparisons. The width of the lines is proportional to the number of trials. The size of every circle is proportional to the number of randomly assigned participants (sample size). (A) FMA-LE; (B) FMA-LE (post-stroke time > 1 month); (C) FMA-LE (post-stroke time ≤ 1 month); (D) BBS; (E) TUGT; (F) MEP amplitude; (G) Speed. HFrTMS, high-frequency repetitive transcranial magnetic stimulation; LFrTMS, low-frequency repetitive transcranial magnetic stimulation; iTBS, intermittent theta-burst stimulation; dTMS, deep transcranial magnetic stimulation; M1, primary motor cortex.
A total of 29 included studies evaluated FMA-LE, involving 6 intervention protocols: HFrTMS-M1, LFrTMS-M1, iTBS-Cerebellum, iTBS-M1, dTMS-M1, and Placebo. A total of 1,685 patients were included. The inconsistency model evaluated global inconsistency, which showed p = 0.0772 (>0.05; Supplementary Figure 8A). The inconsistency test was not significant, so we used the consistency model. The node-splitting approach was used to evaluate local inconsistency. The test of local inconsistency from the node-splitting model showed a small percentage of inconsistency (1 of 6 comparisons), as detailed in Supplementary Table 2. 1 closed loop was formed for the 3 interventions, so we assessed the inconsistency of the closed loop. The results showed that the 95% CI included 0, and IF was close to 0, indicating that our NMA was highly credible (Supplementary Figure 9A).
The NMA results showed that FMA-LE generated a total of 15 pairwise comparisons. Compared with Placebo, LFrTMS-M1 (MD = 2.83, 95% CI: 1.96 to 3.70) and HFrTMS-M1 (MD = 2.74, 95% CI: 1.60 to 3.87) significantly improved FMA-LE in stroke patients. There was no statistically significant difference between the other two interventions (p > 0.05; Figure 4). Figure 5A and Table 3 show the SUCRA rankings for all interventions. According to the analysis, LFrTMS-M1 (SUCRA, 84.6%) may be the most effective intervention to improve FMA-LE in stroke patients.
Figure 4. Network meta-analysis of head-to-head comparisons. Red and bold numbers are statistically significant. HFrTMS, high-frequency repetitive transcranial magnetic stimulation; LFrTMS, low-frequency repetitive transcranial magnetic stimulation; iTBS, intermittent theta-burst stimulation; dTMS, deep transcranial magnetic stimulation; M1, primary motor cortex.
Figure 5. Cumulative probability ranking curve of different interventions. The vertical axis represents cumulative probabilities, while the horizontal axis represents ranks. (A) FMA-LE; (B) FMA-LE (post-stroke time > 1 month); (C) FMA-LE (post-stroke time ≤ 1 month); (D) BBS; (E) TUGT; (F) MEP amplitude; (G) Speed. HFrTMS, high-frequency repetitive transcranial magnetic stimulation; LFrTMS, low-frequency repetitive transcranial magnetic stimulation; iTBS, intermittent theta-burst stimulation; dTMS, deep transcranial magnetic stimulation; M1, primary motor cortex.
Different phases of stroke may lead to different therapeutic effects of rTMS. Therefore, we performed a subgroup analysis for FMA-LE according to the phase of stroke, including post-stroke time > 1 month and post-stroke time ≤ 1 month (Brunelli et al., 2019). The global consistency model of FMA-LE (post-stroke time > 1 month) and FMA-LE (post-stroke time ≤ 1 month) showed that the inconsistency test was not significant (Supplementary Figures 8B,C). There was a small percentage of inconsistency (1 of 3 comparisons) only for the local inconsistency test of FMA-LE (post-stroke time ≤ 1 month), details of which are provided in Supplementary Tables 3, 4.
For post-stroke time > 1 month, Figure 4 demonstrated that HFrTMS-M1 significantly improved FMA-LE (post-stroke time > 1 month) compared to iTBS-M1 (MD = 3.84, 95% CI: 0.52 to 7.16) and Placebo (MD = 3.94, 95% CI: 2.50 to 5.38). Figure 5B and Table 3 showed that the HFrTMS-M1 (SUCRA, 95.8%) was the best protocol. For post-stroke time ≤ 1 month, Figure 4 demonstrated that compared with the placebo, the LFrTMS-M1 (MD = 1.49, 95% CI: 0.05 to 2.93) and HFrTMS-M1 (MD = 2.47, 95% CI: 1.41 to 3.53) had better curative effects, with a statistically significant difference (p < 0.05). Figure 5C and Table 3 showed that the LFrTMS-M1 (SUCRA, 98.9%) was the best protocol. We found no publication bias by the funnel plot of publication bias and Egger’s test (Supplementary Figures 10B,C).
A total of 11 included studies evaluated BBS, involving 5 intervention protocols: HFrTMS-M1, LFrTMS-M1, iTBS-Cerebellum, iTBS-M1, and Placebo. A total of 820 patients were included. The inconsistency model evaluated global inconsistency, which showed p = 0.2800 (>0.05; Supplementary Figure 8D). The inconsistency test was not significant, so we used the consistency model. The node-splitting approach was used to evaluate local inconsistency. The measured p-values were all greater than 0.05, indicating good local consistency (Supplementary Table 5). 1 closed loop was formed for the 3 interventions, so we assessed the inconsistency of the closed loop. The results showed that the 95% CI included 0, and IF was close to 0, indicating that our NMA was highly credible (Supplementary Figure 9D).
The NMA results showed that BBS generated a total of 10 pairwise comparisons. Compared with Placebo, HFrTMS-M1 (MD = 6.97, 95% CI: 3.95 to 9.98), LFrTMS-M1 (MD = 4.36, 95% CI: 2.00 to 6.72), and iTBS-Cerebellum (MD = 3.29, 95% CI: 0.63 to 5.95) significantly improved BBS in stroke patients. In addition, HFrTMS-M1 (MD = 6.36, 95% CI: 0.65 to 12.07) was significantly better than iTBS-M1 in improving BBS. Other pairwise comparisons showed no statistically significant differences (p > 0.05; Figure 4). Figure 5D and Table 3 show the SUCRA rankings for all interventions. According to the results of SUCRA analysis, HFrTMS-M1 (SUCRA, 96.8%) may be the most effective intervention to improve BBS in stroke patients.
A total of 8 included studies evaluated TUGT, involving 5 intervention protocols: HFrTMS-M1, LFrTMS-M1, iTBS-Cerebellum, iTBS-M1, and Placebo. A total of 382 patients were included. There was no closed loop, so we did not need to perform a consistency check. The NMA results showed that TUGT generated a total of 10 pairwise comparisons.
Compared with Placebo, HFrTMS-M1 (MD = −3.25, 95% CI: −5.19 to −1.30) and LFrTMS-M1 (MD = −2.72, 95% CI: −3.95 to −1.49) significantly improved TUGT in stroke patients. There was no statistically significant difference between the other two interventions (p > 0.05; Figure 4). Figure 5E and Table 3 show the SUCRA rankings for all interventions. According to the analysis, HFrTMS-M1 (SUCRA, 80.3%) may be the most effective intervention to improve TUGT in stroke patients.
A total of 8 included studies evaluated MEP amplitude, involving 4 intervention protocols: HFrTMS-M1, LFrTMS-M1, iTBS-Cerebellum, and Placebo. A total of 246 patients were included. The inconsistency model evaluated global inconsistency, which showed p = 0.5656 (>0.05; Supplementary Figure 8E). The inconsistency test was not significant, so we used the consistency model. The node-splitting approach was used to evaluate local inconsistency. The measured p-values were all greater than 0.05, indicating good local consistency (Supplementary Table 3). 1 closed loop was formed for the 3 interventions, so we assessed the inconsistency of the closed loop. The results showed that the 95% CI included 0, and IF was close to 0, indicating that our NMA was highly credible (Supplementary Figure 9E).
The NMA results showed that MEP amplitude generated a total of 6 pairwise comparisons. Compared with Placebo, HFrTMS-M1 (SMD = 0.99, 95% CI: 0.17 to 1.82) significantly improved MEP amplitude in stroke patients. There was no statistically significant difference between the other two interventions (p > 0.05; Figure 4). Figure 5F and Table 3 show the SUCRA rankings for all interventions. According to the analysis, HFrTMS-M1 (SUCRA, 93.7%) may be the most effective intervention to improve MEP amplitude in stroke patients.
A total of 13 included studies evaluated speed, involving 3 intervention protocols: HFrTMS-M1, LFrTMS-M1, and Placebo. A total of 667 patients were included. There was no closed loop, so we did not need to perform a consistency check. The NMA results showed that speed generated a total of 3 pairwise comparisons.
Compared with Placebo, LFrTMS-M1 (SMD = 1.01, 95% CI: 0.64 to 1.38) and HFrTMS-M1 (SMD = 0.82, 95% CI: 0.49 to 1.15) significantly improved speed in stroke patients. There was no statistically significant difference between the other two interventions (p > 0.05; Figure 4). Figure 5G and Table 3 show the SUCRA rankings for all interventions. According to the analysis, LFrTMS-M1 (SUCRA, 88.7%) may be the most effective intervention to improve speed in stroke patients.
This study evaluated publication bias for FMA-LE, FMA-LE (post-stroke time > 1 month), FMA-LE (post-stroke time ≤ 1 month), BBS, TUGT, MEP amplitude, and speed using the funnel plot of publication bias (Figure 6) and Egger’s test. The findings revealed that most points were evenly distributed along both sides of the midline and were primarily focused there, indicating that our results were robust and there was no significant publication bias. In addition, we used Egger’s test for secondary validation of publication bias. The results showed FMA-LE (Egger’s test p = 0.273; Supplementary Figure 10A), FMA-LE (post-stroke time > 1 month; Egger’s test p = 0.807; Supplementary Figure 10B), FMA-LE (post-stroke time ≤ 1 month; Egger’s test p = 0.601; Supplementary Figure 10C), BBS (Egger’s test p = 0.843; Supplementary Figure 10D), TUGT (Egger’s test p = 0.123; Supplementary Figure 10E), MEP amplitude (Egger’s test p = 0.089; Supplementary Figure 10F), and speed (Egger’s test p = 0.556; Supplementary Figure 10G), indicating that there was no publication bias in this study.
Figure 6. Funnel plot of publication bias. (A) FMA-LE; (B) FMA-LE (post-stroke time > 1 month); (C) FMA-LE (post-stroke time ≤ 1 month); (D) BBS; (E) TUGT; (F) MEP amplitude; (G) Speed. HFrTMS, high-frequency repetitive transcranial magnetic stimulation; LFrTMS, low-frequency repetitive transcranial magnetic stimulation; iTBS, intermittent theta-burst stimulation; dTMS, deep transcranial magnetic stimulation; M1, primary motor cortex.
Among the 38 included studies, 16 had no adverse events during the course of the experiment, 7 reported adverse events in detail, and the remaining studies did not describe adverse events (Supplementary Table 7). The adverse events reported were mild, such as headache, dizziness, nausea, and vomiting.
Stroke is a common disease worldwide and causes severe disabilities for patients. More than two-thirds of stroke survivors have post-stroke sequelae, including impairment in motor function, balance, gait, and ADL (Paul et al., 2007). Improving lower extremity motor function and balance ability can significantly impact gait function, ADL, and quality of life in stroke patients (Smith et al., 2017). Although the use of rTMS for stroke has attracted considerable attention, there is still a lack of consensus on the optimal protocol for rTMS to improve lower extremity motor function in stroke patients. To the best of our knowledge, this is the first NMA to compare the effects of different rTMS protocols on lower extremity motor function in stroke patients by taking stimulation frequency, stimulation target, and stimulation mode of rTMS and post-stroke time into account simultaneously.
The FMA-LE can predict lower extremity motor recovery in individuals with stroke (Balasubramanian et al., 2016). This assessment exhibits good internal consistency and reliability, discriminative validity, and responsiveness to interventions (Hsieh et al., 2009). This study found that compared with Placebo, LFrTMS-M1 and HFrTMS-M1 significantly improved FMA-LE in stroke patients, and LFrTMS in the contralateral hemisphere was more effective than HFrTMS in the ipsilateral hemisphere. Xie et al. (2021a) suggested that LFrTMS in the contralateral hemisphere was more effective than HFrTMS in the ipsilateral hemisphere, which was consistent with our findings. However, a novel finding from this NMA is that the subgroup analyses of FMA-LE showed that at ≤1 month after stroke, HFrTMS-M1 was the optimal stimulation protocol for improving stroke patients’ lower extremity motor function. At ≤1 month after stroke, LFrTMS-M1 was the best protocol, which was superior to HFrTMS-M1. The difference between these two studies was that when grouping rTMS protocols, we considered that the same rTMS mode applied to different stimulation targets would produce different therapeutic effects. Therefore, we divided stimulation targets into M1 and Cerebellum rather than simply grouping very different stimulation targets together, which allowed the intervention protocol to be more refined. Second, we included more articles that met our research objectives to expand the sample size. Finally, we also carried out a subgroup analysis of the patients on the efficacy of rTMS at different protocols to improve the accuracy of the outcome evidence. In addition, we also found that 10 Hz and 1 Hz were the most commonly used stimulation frequencies for HFrTM and LFrTMS, respectively, regarding rTMS prescription settings, which was the same conclusion as Fan et al. (2021). Meanwhile, rTMS sessions of 15 or 20 min each and lasting 3 or 4 weeks were the most common. In clinical practice, clinicians or rehabilitation therapists can flexibly formulate the best stimulation prescription according to the specific situation of patients and the recommended protocols mentioned above.
In fact, early hyperexcitability and increased interhemispheric inhibition of the contralesional motor cortex have been demonstrated using TMS after unilateral stroke. Therefore, LFrTMS can effectively improve the motor function of stroke patients by reducing the excitability of the motor cortex of the contralateral hemisphere to restore the balance of competitive inhibition between the two hemispheres in the acute phase of stroke. However, in the post-stroke convalescent phase, the interhemispheric competition is less pronounced than in the acute phase, as it is commonly observed that the transcallosal asymmetry decreases with time (Swayne et al., 2008). LFrTMS may reduce the compensatory effect of the contralateral hemisphere by inhibiting its excitability, thereby hindering functional recovery after stroke. Therefore, HFrTMS-M1 may be more effective than LFrTMS-M1 in the convalescent phase of stroke. Xia et al. (2022) also recommended the application of HFrTMS in patients with stroke patients during the convalescent phase.
The BBS is the most widely used clinical scale for assessing balance performance in individuals with neurological conditions, including static and dynamic balance (Neuls et al., 2011). The sum of the scores for the 14 items (each item was rated from 0 to 4) yielded a balance score ranging from 0 to 56 (Neuls et al., 2011). TUGT is a rapid and quantitative assessment of dynamic balance and functional walking ability and is closely related to other measures of gait and balance in stroke patients (Flansbjer et al., 2005). TUGT assesses the time taken to complete a series of actions, including standing up from a chair, walking forward three meters, turning, and returning to the chair. According to the ranking probability of our NMA, HFrTMS-M1 was more advantageous in improving BBS and TUGT. Therefore, we recommend HFrTMS-M1 as a complementary rehabilitation therapy to improve balance function in stroke patients in clinical practice.
Walking speed can reflect the recovery of lower extremity function and walking quality in stroke patients (Patterson et al., 2008). Our NMA results showed that compared with Placebo, LFrTMS-M1 and HFrTMS-M1 significantly improved the speed in stroke patients. LFrTMS-M1 had more advantages in improving the speed of stroke patients. However, Tung et al. (2019) found that HFrTMS was superior to LFrTMS in improving speed in stroke patients. Further understanding of the relationship between different rTMS protocols and walking speed is needed in the future. Transcranial magnetic stimulation (TMS) is a non-invasive neuromodulation technique that produces pulsed magnetic fields that form induced currents in the motor cortex of the brain. After the induced current stimulates one side of the motor cortex, the conduction nerve impulses are transmitted downward, which will cause the target muscle on the opposite side of the subject to produce action potentials, called motor-evoked potentials (MEPs). MEPs is a quantitative evaluation index of central motor conduction function, which can objectively reflect the excitability of the motor cortex. In this study, we used MEP amplitude to assess the functional status of motor conduction pathways, and the results showed that HFrTMS-M1 was the most effective in improving MEP amplitude. However, MEP amplitude included only a few studies, meaning this ranking result should be treated critically. In addition, to ensure the objectivity of the study results, more high-quality RCTs with large sample sizes are needed for further verification.
Like TMS, functional magnetic resonance imaging (fMRI), electroencephalography (EEG), and other neuroimaging techniques are also of great significance in the assessment of motor function in stroke. fMRI can evaluate the neurovascular response induced by rTMS, and the activity changes of brain nerves can be observed through intuitive and visual images (Bergmann et al., 2016). In addition, fMRI can also be used to study the excitability and functional connectivity of the cerebral cortex and subcortex in stroke patients under rTMS intervention. Guo et al. (2021) used fMRI to find that both LFrTMS stimulation in the unaffected hemisphere and HFrTMS stimulation in the affected hemisphere could promote the reorganization of the motor network, and the changes in functional connectivity between the contralateral PMA and the ipsilateral M1, and between the bilateral M1 induced by rTMS were related to motor recovery. Transcranial magnetic stimulation combined with electroencephalography (TMS-EEG) is a new evaluation method in recent years. TMS-EEG can reflect the direct relationship between brain regions and motor function in stroke patients and predict the recovery of motor function after stroke. Koch et al. (2019) performed motor assessment on 34 stroke subjects who received iTBS-Cerebellum or sham iTBS treatment by Fugl-Meyer scale, gait analysis, and so on, and recorded cerebral cortical activity through TMS-EEG to achieve the combination of treatment and assessment feedback. At present, there are relatively few studies using fMRI and EEG to explore the treatment of rTMS to promote the recovery of dysfunction after stroke, and most of the trials are small in sample size and scale. In the future, large-sample, multi-center, and high-quality RCTs should be carried out, and the results of imaging and electrophysiology of stroke patients should be comprehensively analyzed to improve the accuracy and scientificity of rehabilitation efficacy evaluation.
The M1 is the most essential part of the motor cortex in the human cerebral cortex, located in the precentral gyrus. M1 is the most frequently stimulated target in noninvasive brain stimulation studies for post-stroke gait and balance recovery (Parikh et al., 2023). Among the 38 studies included in this article, 33 studies selected M1 as the stimulation target. Recent electrophysiological and imaging evidence underlined that a large motor network includes other key brain areas during the process of post-stroke functional recovery (Koch and Hummel, 2017). The cerebellum is a crucial structure involved in balance and motor control, and it is essential in motor adaptation and learning processes. Therefore, the cerebellum has been proposed as one of the alternative targets of M1. A total of 5 studies in our NMA used the cerebellum as the stimulation target and selected the iTBS mode for intervention. iTBS is a new rTMS mode that lasts only about 5 min, with the characteristics of short time-consuming, low intensity, and strong effect. iTBS can induce long-term potentiation, which helps promote neural plasticity and produce a safer and lasting intervention effect for stroke patients. Our findings showed that iTBS-Cerebellum can significantly improve balance function in stroke patients. Liao et al. (2024) compared the efficacy and safety of iTBS to the cerebellum or M1 on balance and motor recovery in stroke patients. They found that both iTBS-M1 and iTBS-Cerebellum could improve balance function and that iTBS-Cerebellum, but not iTBS-M1, had a more significant effect on motor recovery. Like our findings, Manto et al. (2012) suggested that iTBS-Cerebellum could be a potential therapeutic approach to improve balance and gait function in stroke patients. Thus, iTBS-Cerebellum may be a valuable new therapeutic option in stroke rehabilitation programs. At the same time, our NMA also included a study on the iTBS-M1 protocol, but the limited number of studies and participants may have led to inaccurate results.
It is worth noting that among the included studies, Chieffo et al. (2014) and Chieffo et al. (2021) used H-coil. The H-coil differs from conventional figure-of-8 coil and circular coil in that it can stimulate deeper cortical areas and neural networks (Roth et al., 2014). Roth et al. (2007) found that H-coil, figure-of-8 coil, and double-cone coil could generate the maximum induced electric field in the surface region of the saline head model at 0.9% concentration. With the increased distance from the simulated skull to the brain tissue, the induced electric field generated by the figure-of-8 coil and the double-cone coil decreased to less than 10% of the maximum induced electric field at 6 cm. In comparison, the electric field intensity of the H-coil was more than 63% of the maximum induced electric field. H-coil can theoretically stimulate deeper leg-related cortical motor areas within the intercerebral fissure approximately 3 to 4 cm below the skull. However, the results of Chieffo et al. (2014) and Chieffo et al. (2021) did not show a favorable advantage of dTMS. We suspect this may be due to the small number of current studies. In the future, more RCTs are needed to confirm the applicability and safety of dTMS in stroke patients.
The study also has some limitations, including: (1) Coil type, stimulation intensity, total number of pulses, and duration of intervention were not exactly the same among the included studies, resulting in potential heterogeneity. (2) Despite including the full stimulation protocol in this analysis, the iTBS-M1 and dTMS-M1 groups accounted for 2.6 and 5.3% of the total data, respectively. This affects, to some extent, the quality of the conclusions of this study. (3) The age and disease severity of the included patients were slightly different, and some data indicators will be affected. Further subgroup analysis according to age and disease severity is needed in the future. (4) Adverse events may not be strictly reported in the included studies, so the safety of each intervention protocol needs to be further studied.
In this NMA, we found differences in the therapeutic effects between different rTMS protocols. Considering the impact of the stroke phase on the lower extremity motor function, the current research evidence shows that HFrTMS-M1 may be the preferred stimulation protocol to improve the lower extremity motor function of patients for post-stroke time > 1 month, and LFrTMS-M1 for post-stroke time ≤ 1 month. In the future, high-quality, large-sample, multi-center, and long-term follow-up RCTs are needed to verify the conclusions of this study.
The original contributions presented in the study are included in the article/Supplementary material, further inquiries can be directed to the corresponding authors.
CWa: Software, Writing – original draft. QZ: Software, Writing – original draft. LZ: Data curation, Methodology, Supervision, Writing – review & editing. DZ: Data curation, Methodology, Supervision, Writing – review & editing. YX: Data curation, Methodology, Supervision, Writing – review & editing. ZL: Data curation, Methodology, Supervision, Writing – review & editing. CWu: Supervision, Writing – review & editing, Data curation, Methodology. SW: Supervision, Writing – review & editing, Data curation, Methodology. MY: Supervision, Writing – review & editing. LW: Supervision, Writing – review & editing.
The author(s) declare financial support was received for the research, authorship, and/or publication of this article. This work was supported by the Beijing Municipal Administration of Hospitals Incubating Program (No.PX2024074) and the Capital Health Research and Development of Special (No.2020-2-2201).
The authors declare that the research was conducted in the absence of any commercial or financial relationships that could be construed as a potential conflict of interest.
All claims expressed in this article are solely those of the authors and do not necessarily represent those of their affiliated organizations, or those of the publisher, the editors and the reviewers. Any product that may be evaluated in this article, or claim that may be made by its manufacturer, is not guaranteed or endorsed by the publisher.
The Supplementary material for this article can be found online at: https://www.frontiersin.org/articles/10.3389/fnins.2024.1352212/full#supplementary-material
Balasubramanian, C. K., Li, C.-Y., Bowden, M. G., Duncan, P. W., Kautz, S. A., and Velozo, C. A. (2016). Dimensionality and item-difficulty hierarchy of the lower extremity Fugl-Meyer assessment in individuals with subacute and chronic stroke. Arch. Phys. Med. Rehabil. 97, 582–589.e2. doi: 10.1016/j.apmr.2015.12.012
Bergmann, T. O., Karabanov, A., Hartwigsen, G., Thielscher, A., and Siebner, H. R. (2016). Combining non-invasive transcranial brain stimulation with neuroimaging and electrophysiology: current approaches and future perspectives. Neuroimage 140, 4–19. doi: 10.1016/j.neuroimage.2016.02.012
Brunelli, S., Iosa, M., Fusco, F. R., Pirri, C., Di Giunta, C., Foti, C., et al. (2019). Early body weight-supported overground walking training in patients with stroke in subacute phase compared to conventional physiotherapy: a randomized controlled pilot study. Int. J. Rehabil. Res. 42, 309–315. doi: 10.1097/MRR.0000000000000363
Cárdenas-Morales, L., Nowak, D. A., Kammer, T., Wolf, R. C., and Schönfeldt-Lecuona, C. (2010). Mechanisms and applications of theta-burst rTMS on the human motor cortex. Brain Topogr. 22, 294–306. doi: 10.1007/s10548-009-0084-7
Ceng, B., Fan, S., Li, Y., and Wang, P. (2022). Transcranial magnetic stimulation combined with the dual task training on stroke patients with hemiplegia lower limb motor function. Chin J Integr Med Cardio Cererovasc Dis 20, 4585–4588. doi: 10.12102/j.issn.1672-1349.2022.24.034
Cha, H. G., and Kim, M. K. (2017). Effects of strengthening exercise integrated repetitive transcranial magnetic stimulation on motor function recovery in subacute stroke patients: a randomized controlled trial. Technol. Health Care 25, 521–529. doi: 10.3233/THC-171294
Cha, H. G., Kim, M. K., Nam, H. C., and Ji, S. G. (2014). Effects of high frequency repetitive transcranial magnetic stimulation on function in subacute stroke patients. J Magn 19, 192–196. doi: 10.4283/JMAG.2014.19.2.192
Chen, Y. (2018). Effects of repetitive transcranial magnetic stimulation on spasm and motor function of lower limbs in patients with stroke. Chongqing Med J 47, 3292–3295+3298. doi: 10.3969/j.issn.1671-8348.2018.25.012
Chen, J., Shi, M., Chen, J., Zhang, J., Chen, R., Tu, J., et al. (2023). The effects of supplementing theta burst stimulation of the cerebellum with physical therapy on balance and gait recovery after a stroke: a randomized clinical trial. Chin J Phys Med Rehabil 45, 402–407. doi: 10.3760/cma.j.issn.0254-1424.2023.05.004
Chieffo, R., De Prezzo, S., Houdayer, E., Nuara, A., Di Maggio, G., Coppi, E., et al. (2014). Deep repetitive transcranial magnetic stimulation with H-coil on lower limb motor function in chronic stroke: a pilot study. Arch. Phys. Med. Rehabil. 95, 1141–1147. doi: 10.1016/j.apmr.2014.02.019
Chieffo, R., Giatsidis, F., Santangelo, R., Alyagon, U., Comola, M., Zangen, A., et al. (2021). Repetitive transcranial magnetic stimulation with H-coil coupled with cycling for improving lower limb motor function after stroke: An exploratory study. Neuromodulation 24, 916–922. doi: 10.1111/ner.13228
Chung, S. W., Hoy, K. E., and Fitzgerald, P. B. (2015). Theta-burst stimulation: a new form of TMS treatment for depression? Depress. Anxiety 32, 182–192. doi: 10.1002/da.22335
Dias, S., Welton, N. J., Caldwell, D. M., and Ades, A. E. (2010). Checking consistency in mixed treatment comparison meta-analysis. Stat. Med. 29, 932–944. doi: 10.1002/sim.3767
Di Pino, G., Pellegrino, G., Assenza, G., Capone, F., Ferreri, F., Formica, D., et al. (2014). Modulation of brain plasticity in stroke: a novel model for neurorehabilitation. Nat Rev Neurol. 10, 597–608. doi: 10.1038/nrneurol.2014.162
Du, J., Yang, F., Hu, J., Hu, J., Xu, Q., Cong, N., et al. (2019). Effects of high- and low-frequency repetitive transcranial magnetic stimulation on motor recovery in early stroke patients: evidence from a randomized controlled trial with clinical, neurophysiological and functional imaging assessments. Neuroimage Clin 21:101620. doi: 10.1016/j.nicl.2018.101620
Elkholy, S. H., Atteya, A. A., Hassan, W. A., Sharaf, M., and Gohary, A. M. E. (2014). Low rate repetitive transcranial magnetic stimulation (rTMS) and gait rehabilitation after stroke. Int J Neurorehabil 1:109. doi: 10.4172/2376-0281.1000109
Eng, J. J., and Tang, P.-F. (2007). Gait training strategies to optimize walking ability in people with stroke: a synthesis of the evidence. Expert Rev. Neurother. 7, 1417–1436. doi: 10.1586/14737175.7.10.1417
Fan, H., Song, Y., Cen, X., Yu, P., Bíró, I., and Gu, Y. (2021). The effect of repetitive transcranial magnetic stimulation on lower-limb motor ability in stroke patients: a systematic review. Front. Hum. Neurosci. 15:620573. doi: 10.3389/fnhum.2021.620573
Flansbjer, U.-B., Holmbäck, A. M., Downham, D., Patten, C., and Lexell, J. (2005). Reliability of gait performance tests in men and women with hemiparesis after stroke. J. Rehabil. Med. 37, 75–82. doi: 10.1080/16501970410017215
Fleiss, J. L. (1993). The statistical basis of meta-analysis. Stat. Methods Med. Res. 2, 121–145. doi: 10.1177/096228029300200202
George, M. S., and Aston-Jones, G. (2010). Noninvasive techniques for probing neurocircuitry and treating illness: vagus nerve stimulation (VNS), transcranial magnetic stimulation (TMS) and transcranial direct current stimulation (tDCS). Neuropsychopharmacology. 35, 301–316. doi: 10.1038/npp.2009.87
Gong, Y., Long, X.-M., Xu, Y., Cai, X.-Y., and Ye, M. (2021). Effects of repetitive transcranial magnetic stimulation combined with transcranial direct current stimulation on motor function and cortex excitability in subacute stroke patients: a randomized controlled trial. Clin. Rehabil. 35, 718–727. doi: 10.1177/0269215520972940
Guan, Y.-Z., Li, J., Zhang, X.-W., Wu, S., Du, H., Cui, L.-Y., et al. (2017). Effectiveness of repetitive transcranial magnetic stimulation (rTMS) after acute stroke: a one-year longitudinal randomized trial. CNS Neurosci. Ther. 23, 940–946. doi: 10.1111/cns.12762
Guo, Z., Jin, Y., Bai, X., Jiang, B., He, L., McClure, M. A., et al. (2021). Distinction of high- and low-frequency repetitive transcranial magnetic stimulation on the functional reorganization of the motor network in stroke patients. Neural Plast. 2021, 8873221–8873211. doi: 10.1155/2021/8873221
Higgins, J. P. T., Thompson, S. G., Deeks, J. J., and Altman, D. G. (2003). Measuring inconsistency in meta-analyses. BMJ 327, 557–560. doi: 10.1136/bmj.327.7414.557
Hsieh, Y., Wu, C., Lin, K., Chang, Y., Chen, C., and Liu, J. (2009). Responsiveness and validity of three outcome measures of motor function after stroke rehabilitation. Stroke 40, 1386–1391. doi: 10.1161/STROKEAHA.108.530584
Hua, Y., Li, B., Wang, K., Guo, H., and Gou, L. (2019). Different frequency repetitive transcranial magnetic stimulation treatment of ischemic cerebral apoplexy patients curative effect observation. Chin J Phys Med Rehabil 41, 745–748. doi: 10.3760/cma.j.issn.0254-1424.2019.10.006
Huang, Y.-Z., Edwards, M. J., Rounis, E., Bhatia, K. P., and Rothwell, J. C. (2005). Theta burst stimulation of the human motor cortex. Neuron 45, 201–206. doi: 10.1016/j.neuron.2004.12.033
Huang, Y.-Z., Lin, L.-F., Chang, K.-H., Hu, C.-J., Liou, T.-H., and Lin, Y.-N. (2018). Priming with 1-Hz repetitive transcranial magnetic stimulation over Contralesional leg motor cortex does not increase the rate of regaining ambulation within 3 months of stroke: a randomized controlled trial. Am. J. Phys. Med. Rehabil. 97, 339–345. doi: 10.1097/PHM.0000000000000850
Huang, Y.-Z., Rothwell, J. C., Chen, R.-S., Lu, C.-S., and Chuang, W.-L. (2011). The theoretical model of theta burst form of repetitive transcranial magnetic stimulation. Clin. Neurophysiol. 122, 1011–1018. doi: 10.1016/j.clinph.2010.08.016
Hutton, B., Salanti, G., Caldwell, D. M., Chaimani, A., Schmid, C. H., Cameron, C., et al. (2015). The PRISMA extension statement for reporting of systematic reviews incorporating network meta-analyses of health care interventions: checklist and explanations. Ann. Intern. Med. 162, 777–784. doi: 10.7326/M14-2385
Ji, S.-G., Cha, H.-G., Kim, K.-J., and Kim, M.-K. (2014). Effects of motor imagery practice in conjunction with repetitive transcranial magnetic stimulation on stroke patients. J Magn 19, 181–184. doi: 10.4283/JMAG.2014.19.2.181
Ji, S. G., and Kim, M. K. (2015). The effects of repetitive transcranial magnetic stimulation on the gait of acute stroke patients. J Magn 20, 129–132. doi: 10.4283/JMAG.2015.20.2.129
Kakuda, W., Abo, M., Nakayama, Y., Kiyama, A., and Yoshida, H. (2013). High-frequency rTMS using a double cone coil for gait disturbance. Acta Neurol. Scand. 128, 100–106. doi: 10.1111/ane.12085
Kesikburun, S. (2022). Non-invasive brain stimulation in rehabilitation. Turk J Phys Med Rehabil 68, 1–8. doi: 10.5606/tftrd.2022.10608
Koch, G., Bonnì, S., Casula, E. P., Iosa, M., Paolucci, S., Pellicciari, M. C., et al. (2019). Effect of cerebellar stimulation on gait and balance recovery in patients with hemiparetic stroke: a randomized clinical trial. JAMA Neurol. 76, 170–178. doi: 10.1001/jamaneurol.2018.3639
Koch, P. J., and Hummel, F. C. (2017). Toward precision medicine: tailoring interventional strategies based on noninvasive brain stimulation for motor recovery after stroke. Curr. Opin. Neurol. 30, 388–397. doi: 10.1097/WCO.0000000000000462
Langhammer, B., and Stanghelle, J. K. (2011). Can physiotherapy after stroke based on the Bobath concept result in improved quality of movement compared to the motor relearning programme. Physiother Res Int. 16, 69–80. doi: 10.1002/pri.474
Larson, J., Wong, D., and Lynch, G. (1986). Patterned stimulation at the theta frequency is optimal for the induction of hippocampal long-term potentiation. Brain Res. 368, 347–350. doi: 10.1016/0006-8993(86)90579-2
Lee, S. A., and Cha, H. G. (2020). The effect of high frequency repetitive transcranial magnetic stimulation combined with treadmill training on the recovery of lower limb function in chronic stroke patients: a randomized controlled trial. J Magn 25, 402–408. doi: 10.4283/JMAG.2020.25.3.402
Li, Y., Fan, J., Yang, J., He, C., and Li, S. (2018). Effects of repetitive transcranial magnetic stimulation on walking and balance function after stroke: a systematic review and meta-analysis. Am. J. Phys. Med. Rehabil. 97, 773–781. doi: 10.1097/PHM.0000000000000948
Li, Y., Huang, L., Zhang, J., Tian, J., and Yu, X. (2016). Effects of repetitive transcranial magnetic stimulation on the lower limbmotor functionof cerebral infarctior patients. Chin J Phys Med Rehabil 38, 839–842. doi: 10.3760/cma.j.issn.0254-1424.2016.11.010
Liao, L.-Y., Xie, Y.-J., Chen, Y., and Gao, Q. (2021). Cerebellar theta-burst stimulation combined with physiotherapy in subacute and chronic stroke patients: a pilot randomized controlled trial. Neurorehabil. Neural Repair 35, 23–32. doi: 10.1177/1545968320971735
Liao, L.-Y., Zhu, Y., Peng, Q.-Y., Gao, Q., Liu, L., Wang, Q.-H., et al. (2024). Intermittent theta-burst stimulation for stroke: primary motor cortex versus cerebellar stimulation: a randomized sham-controlled trial. Stroke 55, 156–165. doi: 10.1161/STROKEAHA.123.044892
Lin, L.-F., Chang, K.-H., Huang, Y.-Z., Lai, C.-H., Liou, T.-H., and Lin, Y.-N. (2019). Simultaneous stimulation in bilateral leg motor areas with intermittent theta burst stimulation to improve functional performance after stroke: a feasibility pilot study. Eur. J. Phys. Rehabil. Med. 55, 162–168. doi: 10.23736/S1973-9087.18.05245-0
Lin, Y.-N., Hu, C.-J., Chi, J., Lin, L.-F., Yen, T.-H., Lin, Y.-K., et al. (2015). Effects of repetitive transcranial magnetic stimulation of the unaffected hemisphere leg motor area in patients with subacute stroke and substantial leg impairment: a pilot study. J. Rehabil. Med. 47, 305–310. doi: 10.2340/16501977-1943
Liu, M., Meng, Y., Li, H., and Zhang, G. (2019). Effects of repetitive transcranial magnetic stimulation on motor function and serum levels of MMP-9/hs-CRP of ischemic stroke patients. Tianjin Med J 47, 184–188. doi: 10.11958/20181534
Ma, Q., Li, Z., Fu, W., and Lin, Q. (2023). Effect of repetitive transcranial magnetic stimulation on walking of stroke patients at recovery stage. Chin J Rehabil Theory Pract 29, 167–173. doi: 10.3969/j.issn.1006⁃9771.2023.02.005
Manto, M., Bower, J. M., Conforto, A. B., Delgado-García, J. M., da Guarda, S. N. F., Gerwig, M., et al. (2012). Consensus paper: roles of the cerebellum in motor control--the diversity of ideas on cerebellar involvement in movement. Cerebellum 11, 457–487. doi: 10.1007/s12311-011-0331-9
Mead, G. E., Hsieh, C.-F., Lee, R., Kutlubaev, M., Claxton, A., Hankey, G. J., et al. (2013). Selective serotonin reuptake inhibitors for stroke recovery: a systematic review and meta-analysis. Stroke 44, 844–850. doi: 10.1161/STROKEAHA.112.673947
Mo, L., and Liu, A. (2020). Effects of transcranial Maanetic Stimuation combined with Behabilitation training on gait, balance and FMA score in patients with post stroke hemiplegia. Chin J Integr Med Cardio-Cererovasc Dis 18, 4065–4068. doi: 10.12102/j.issn.1672-1349.2020.23.038
Mou, G., Liu, Y., Ruan, Z., Dai, Y., Xie, L., Ai, Q., et al. (2021). Effects of low frequency repetitive transcranial magnetic stimulation on motor function of lower extremity in early re-covery stroke patients. Chin J Rehabil 36, 465–468. doi: 10.3870/zgkf.2021.08.004
Nathou, C., Duprey, E., Simon, G., Razafimandimby, A., Leroux, E., Dollfus, S., et al. (2018). Effects of low- and high-frequency repetitive transcranial magnetic stimulation on long-latency auditory evoked potentials. Neurosci. Lett. 686, 198–204. doi: 10.1016/j.neulet.2018.09.002
Neuls, P. D., Clark, T. L., Van Heuklon, N. C., Proctor, J. E., Kilker, B. J., Bieber, M. E., et al. (2011). Usefulness of the berg balance scale to predict falls in the elderly. J. Geriatr. Phys. Ther. 34, 3–10. doi: 10.1097/JPT.0b013e3181ff2b0e
Ng, S. S. M., and Hui-Chan, C. W. Y. (2010). Transcutaneous electrical stimulation on acupoints combined with task-related training to improve motor function and walking performance in an individual 7 years poststroke: a case study. J. Neurol. Phys. Ther. 34, 208–213. doi: 10.1097/NPT.0b013e3181fe0ab0
Page, M. J., McKenzie, J. E., Bossuyt, P. M., Boutron, I., Hoffmann, T. C., Mulrow, C. D., et al. (2021). The PRISMA 2020 statement: an updated guideline for reporting systematic reviews. BMJ 372:n71. doi: 10.1136/bmj.n71
Parikh, V., Medley, A., Chung, Y.-C., and Goh, H.-T. (2023). Optimal timing and neural loci: a scoping review on the effect of non-invasive brain stimulation on post-stroke gait and balance recovery. Top. Stroke Rehabil. 30, 84–100. doi: 10.1080/10749357.2021.1990467
Park, C. S., and An, S. H. (2016). Reliability and validity of the modified functional ambulation category scale in patients with hemiparalysis. J. Phys. Ther. Sci. 28, 2264–2267. doi: 10.1589/jpts.28.2264
Park, H.-J., Oh, D.-W., Kim, S.-Y., and Choi, J.-D. (2011). Effectiveness of community-based ambulation training for walking function of post-stroke hemiparesis: a randomized controlled pilot trial. Clin. Rehabil. 25, 451–459. doi: 10.1177/0269215510389200
Patterson, K. K., Parafianowicz, I., Danells, C. J., Closson, V., Verrier, M. C., Staines, W. R., et al. (2008). Gait asymmetry in community-ambulating stroke survivors. Arch. Phys. Med. Rehabil. 89, 304–310. doi: 10.1016/j.apmr.2007.08.142
Paul, S. L., Srikanth, V. K., and Thrift, A. G. (2007). The large and growing burden of stroke. Curr. Drug Targets 8, 786–793. doi: 10.2174/138945007781077418
Roth, Y., Levkovitz, Y., Pell, G. S., Ankry, M., and Zangen, A. (2014). Safety and characterization of a novel multi-channel TMS stimulator. Brain Stimul. 7, 194–205. doi: 10.1016/j.brs.2013.09.004
Roth, Y., Padberg, F., and Zangen, A. (2007). Transcranial magnetic stimulation of deep brain regions: principles and methods. Adv Biol Psychiatr. 23, 204–225. doi: 10.1159/000101039
Rouse, B., Chaimani, A., and Li, T. (2017). Network meta-analysis: an introduction for clinicians. Intern. Emerg. Med. 12, 103–111. doi: 10.1007/s11739-016-1583-7
Savović, J., Weeks, L., Sterne, J. A. C., Turner, L., Altman, D. G., Moher, D., et al. (2014). Evaluation of the Cochrane Collaboration’s tool for assessing the risk of bias in randomized trials: focus groups, online survey, proposed recommendations and their implementation. Syst. Rev. 3:37. doi: 10.1186/2046-4053-3-37
Sharma, H., Vishnu, V. Y., Kumar, N., Sreenivas, V., Rajeswari, M. R., Bhatia, R., et al. (2020). Efficacy of low-frequency repetitive transcranial magnetic stimulation in ischemic stroke: a double-blind randomized controlled trial. Arch Rehabil Res Clin Transl 2:100039. doi: 10.1016/j.arrct.2020.100039
Shim, S., Yoon, B.-H., Shin, I.-S., and Bae, J.-M. (2017). Network meta-analysis: application and practice using Stata. Epidemiol Health 39:e2017047. doi: 10.4178/epih.e2017047
Smith, M.-C., Byblow, W. D., Barber, P. A., and Stinear, C. M. (2017). Proportional recovery from lower limb motor impairment after stroke. Stroke 48, 1400–1403. doi: 10.1161/STROKEAHA.116.016478
Swayne, O. B. C., Rothwell, J. C., Ward, N. S., and Greenwood, R. J. (2008). Stages of motor output reorganization after hemispheric stroke suggested by longitudinal studies of cortical physiology. Cereb. Cortex 18, 1909–1922. doi: 10.1093/cercor/bhm218
Tao, F., Wang, C., Chen, B., Qiu, M., and Jiang, L. (2022). Effects of low frequency repetitive transcranial magnetic stimulation combined with mirror therapy on lower limb motor function and balance in stroke patients with hemiplegia. Chin. J. Rehabil. Med. 37, 611–615+622. doi: 10.3969/j.issn.1001-1242.2022.05.007
Tufanaru, C., Munn, Z., Stephenson, M., and Aromataris, E. (2015). Fixed or random effects meta-analysis? Common methodological issues in systematic reviews of effectiveness. Int. J. Evid. Based Healthc. 13, 196–207. doi: 10.1097/XEB.0000000000000065
Tung, Y.-C., Lai, C.-H., Liao, C.-D., Huang, S.-W., Liou, T.-H., and Chen, H.-C. (2019). Repetitive transcranial magnetic stimulation of lower limb motor function in patients with stroke: a systematic review and meta-analysis of randomized controlled trials. Clin. Rehabil. 33, 1102–1112. doi: 10.1177/0269215519835889
Wang, S., and Li, L. (2022). Effects of cerebellar theta-burst stimulation on lower extremity motor function in stroke patients. Chin J Rehabil Theory Pract 28, 1205–1210. doi: 10.3969/j.issn.1006-9771.2022.10.011
Wang, D., Peng, B., Zhang, H., Wang, Y., Liu, N., Shan, C., et al. (2022). Brief report on stroke prevention and treatment in China, 2020. Chin J Cerebrovasc Dis 19, 136–144. doi: 10.3969/j.issn.1672-5921.2022.02.011
Wang, R.-Y., Tseng, H.-Y., Liao, K.-K., Wang, C.-J., Lai, K.-L., and Yang, Y.-R. (2012). rTMS combined with task-oriented training to improve symmetry of interhemispheric corticomotor excitability and gait performance after stroke: a randomized trial. Neurorehabil. Neural Repair 26, 222–230. doi: 10.1177/1545968311423265
Wang, R.-Y., Wang, F.-Y., Huang, S.-F., and Yang, Y.-R. (2019). High-frequency repetitive transcranial magnetic stimulation enhanced treadmill training effects on gait performance in individuals with chronic stroke: a double-blinded randomized controlled pilot trial. Gait Posture 68, 382–387. doi: 10.1016/j.gaitpost.2018.12.023
Wang, C., Zeng, Q., Yuan, Z., Wang, W., and Shen, M. (2023). Effects of low-frequency (0.5 Hz) and high-frequency (10 Hz) repetitive transcranial magnetic stimulation on neurological function, motor function, and excitability of cortex in ischemic stroke patients. Neurologist 28, 11–18. doi: 10.1097/NRL.0000000000000435
Wessel, M. J., and Hummel, F. C. (2018). Non-invasive cerebellar stimulation: a promising approach for stroke recovery? Cerebellum 17, 359–371. doi: 10.1007/s12311-017-0906-1
White, I. R., Barrett, J. K., Jackson, D., and Higgins, J. P. T. (2012). Consistency and inconsistency in network meta-analysis: model estimation using multivariate meta-regression. Res. Synth. Methods 3, 111–125. doi: 10.1002/jrsm.1045
Woodford, H., and Price, C. (2007). EMG biofeedback for the recovery of motor function after stroke. Cochrane Database Syst. Rev. 2010:CD004585. doi: 10.1002/14651858.CD004585.pub2
Xia, Y., Xu, Y., Li, Y., Lu, Y., and Wang, Z. (2022). Comparative efficacy of different repetitive transcranial magnetic stimulation protocols for stroke: a network meta-analysis. Front. Neurol. 13:918786. doi: 10.3389/fneur.2022.918786
Xie, Y.-J., Chen, Y., Tan, H.-X., Guo, Q.-F., Lau, B. W.-M., and Gao, Q. (2021a). Repetitive transcranial magnetic stimulation for lower extremity motor function in patients with stroke: a systematic review and network meta-analysis. Neural Regen. Res. 16, 1168–1176. doi: 10.4103/1673-5374.300341
Xie, Y.-J., Wei, Q.-C., Chen, Y., Liao, L.-Y., Li, B.-J., Tan, H.-X., et al. (2021b). Cerebellar theta burst stimulation on walking function in stroke patients: a randomized clinical trial. Front. Neurosci. 15:688569. doi: 10.3389/fnins.2021.688569
Yan, Y., Yu, L., and Shao, Y. (2023). Effect of low frequency repetitive transcranial magnetic stimulation on lower limb spasticity and balance gait in patients with hemiplegia after stroke. China Med Her 20, 81–85. doi: 10.20047/j.issn1673-7210.2023.14.17
Yang, L., Sheng, Y., Xie, L., Wei, H., and Peng, T. (2016). The effect of repetitive transcranial magnetic stimulation combined with rehabilitation training on the walking function of stroke patients. Chin J Phys Med Rehabil 38, 907–909. doi: 10.3760/cma.j.issn.0254-1424.2016.12.007
Zhao, X., Liu, T., Zhou, Y., and Zhang, L. (2018). The effect of repetitive transcranial magnetic stimulation on dyskinesia in stroke patients. Chin J Rehabil Med 33, 800–805. doi: 10.3969/j.issn.1001-1242.2018.07.009
Zhou, J., Zhang, L., Li, R., Xu, K., and Wu, C. (2020). Effect of repetitive transcranial magnetic stimulation combined with exercise therapy on the motor function in stroke patients with hemiplegia. Rehabil Med 30, 235–239. doi: 10.3724/SP.J.1329.2020.03013
Keywords: stroke, lower extremity, motor function, repetitive transcranial magnetic stimulation, network meta-analysis
Citation: Wang C, Zhang Q, Zhang L, Zhao D, Xu Y, Liu Z, Wu C, Wu S, Yong M and Wu L (2024) Comparative efficacy of different repetitive transcranial magnetic stimulation protocols for lower extremity motor function in stroke patients: a network meta-analysis. Front. Neurosci. 18:1352212. doi: 10.3389/fnins.2024.1352212
Received: 07 December 2023; Accepted: 25 January 2024;
Published: 15 February 2024.
Edited by:
Rupert Ortner, g.tec medical engineering Spain S.L., SpainReviewed by:
Yuan Peng, Guangzhou First People's Hospital, ChinaCopyright © 2024 Wang, Zhang, Zhang, Zhao, Xu, Liu, Wu, Wu, Yong and Wu. This is an open-access article distributed under the terms of the Creative Commons Attribution License (CC BY). The use, distribution or reproduction in other forums is permitted, provided the original author(s) and the copyright owner(s) are credited and that the original publication in this journal is cited, in accordance with accepted academic practice. No use, distribution or reproduction is permitted which does not comply with these terms.
*Correspondence: Mingjin Yong, cHJvZl9tanlvbmdAMTYzLmNvbQ==; Liang Wu, MTk3Mnd1bGlhbmdAc2luYS5jb20=
†These authors have contributed equally to this work
Disclaimer: All claims expressed in this article are solely those of the authors and do not necessarily represent those of their affiliated organizations, or those of the publisher, the editors and the reviewers. Any product that may be evaluated in this article or claim that may be made by its manufacturer is not guaranteed or endorsed by the publisher.
Research integrity at Frontiers
Learn more about the work of our research integrity team to safeguard the quality of each article we publish.