- 1Department of Biology, Texas A&M University, College Station, TX, United States
- 2Department of Neurosciences, University of California, San Diego, La Jolla, CA, United States
- 3Veterans Affairs Medical Center, San Diego, CA, United States
- 4Texas A&M Institute for Neuroscience, Texas A&M University, College Station, TX, United States
Spinal cord injury (SCI) substantially reduces the quality of life of affected individuals. Recovery of function is therefore a primary concern of the patient population and a primary goal for therapeutic interventions. Currently, even with growing numbers of clinical trials, there are still no effective treatments that can improve neurological outcomes after SCI. A large body of work has demonstrated that transplantation of neural stem/progenitor cells (NSPCs) can promote regeneration of the injured spinal cord by providing new neurons that can integrate into injured host neural circuitry. Despite these promising findings, the degree of functional recovery observed after NSPC transplantation remains modest. It is evident that treatment of such a complex injury cannot be addressed with a single therapeutic approach. In this mini-review, we discuss combinatorial strategies that can be used along with NSPC transplantation to promote spinal cord regeneration. We begin by introducing bioengineering and neuromodulatory approaches, and highlight promising work using these strategies in integration with NSPCs transplantation. The future of NSPC transplantation will likely include a multi-factorial approach, combining stem cells with biomaterials and/or neuromodulation as a promising treatment for SCI.
Introduction
Spinal cord injury (SCI) has devastating consequences for the physical and social wellbeing of individuals. It affects more than 250,000 people each year worldwide, and has a significant impact on quality of life, life expectancy and financial wellbeing of people living with SCI (National Spinal Cord Injury Statistical Center, 2022). Traumatic SCI occurs by an external physical impact such as through a motor vehicle accident, fall, or sports-related injury. SCI typically results in permanent neurological deficits including impaired sensory, motor, and autonomic function. The primary mechanical injury is followed by a secondary injury cascade resulting in progressive cell death, inflammation, and scar formation, and the resulting cystic cavitation and reactive scar tissue creates a physical and chemical barrier for axonal regrowth (Thuret et al., 2006; Gomes et al., 2019). To date, despite significant progress in preclinical SCI research and a rapidly increasing number of clinical trials (Dietz et al., 2022), there are no proven-effective treatments that can improve neurological function after SCI.
“Neural stem/progenitor cells” is a catch-all term that refers to either neural stem cells, neural progenitor cells, or fetal-derived tissue that contains a mixture of both cell types. For the purposes of this review article, we will use neural stem/progenitor cells (NSPCs) as a general terminology, but will refer to the specific cell types used on a case-by-case basis as we discuss individual studies. In past decades, transplantation of NSPCs has shown great potential to reconstruct injured spinal cord tissue and promote functional recovery after SCI (Lane et al., 2017; Fischer et al., 2020). NSPCs are undifferentiated precursor cells of the central nervous system that have potential to differentiate into neurons, oligodendrocytes and astrocytes (McDonald et al., 1999; Cao et al., 2002; Han et al., 2002). NSPCs can be derived from human or rodent sources, either isolated from primary fetal or adult tissue or differentiated from pluripotent stem cells (Fischer et al., 2020). Transplanted NSPCs can also be differentiated to glial cells, providing scaffolds for axon regeneration as well as promoting remyelination and plasticity (Cummings et al., 2005, 2006; Wilcox et al., 2014; Nori et al., 2018). For the purposes of this review, we will focus on studies transplanting undifferentiated NSPCs in combination with different therapeutic strategies. These transplants possess beneficial properties capable of promoting repair and enhancing functional outcomes. The NSPC transplantation strategy is focused on promoting remyelination, modulating the immune response, providing neuroprotective benefits, and promoting neural relay formation (Fischer et al., 2020). NSPC grafts have been shown to promote axonal sprouting and regeneration by attenuating the growth inhibitory environment (Richardson et al., 1980; Bonner et al., 2011). Transplant-derived neurons can also anatomically and synaptically integrate into the host CNS, and even establish neuronal relays across the site of injury (White et al., 2010; Bonner et al., 2011; Lee et al., 2014; Spruance et al., 2018; Zholudeva et al., 2018). Additionally, multiple preclinical studies have reported improvement in voluntary motor function, respiratory function, sensory function, or bladder function following transplantation of NSPCs (Cummings et al., 2005; Lynskey et al., 2006; White et al., 2010; Lu et al., 2012; Lee et al., 2014; Dougherty et al., 2016; Fandel et al., 2016; Kadoya et al., 2016; Tashiro et al., 2016; Brock et al., 2018; Kumamaru et al., 2018; Rosenzweig et al., 2018; Zholudeva et al., 2018; Dugan et al., 2020; Kawai et al., 2021; Kitagawa et al., 2022). Together, these properties of neural transplants demonstrate their high potential to treat human SCI. Multiple clinical trials to evaluate safety and efficacy of NSPCs for SCI have been conducted (reviewed in Fischer et al., 2020). Recently, a first-in-human study evaluating safety of human induced pluripotent stem cell (iPSC)-derived NSPCs for human SCI has begun (Tsuji et al., 2019; Sugai et al., 2021).
Despite the promise of NSPC transplantation, there is a general consensus that there will be no single “magic bullet” therapy for SCI. Rather, combinatorial therapies may be more effective at achieving robust and reproducible functional improvements (Griffin and Bradke, 2020; Morse et al., 2021). In the context of NSPC transplantation, Pieczonka and Fehlings (2023) recently published a review of combinatorial techniques that can be used to direct transplanted NSPCs toward integration into targeted neural circuits, including molecular approaches to promote chemotaxis, task-specific rehabilitation, and galvanotaxis or magnet-based tools to promote graft migration. Here, we review previous and ongoing work to combine NSPC transplantation with two promising therapeutic approaches: biomaterials and neuromodulation.
Combinatorial strategies integrating NSPCs with biomaterials
Biomaterials-based strategies are considered promising treatments for SCI that can stimulate axon regeneration, release growth-promoting factors, and promote functional recovery. Biomaterials are classified as degradable or non-degradable natural or synthetic polymers that can be implanted into the site of injury and used as bridges and/or delivery agents for cells, growth factors, or exosomes (Jeong et al., 2021). In recent years, there have been several published studies combining NSPCs with biomaterials. The main goal of this strategy is to deposit living cells on extracellular matrix that can provide a physical structure for cell growth and differentiation, guide growth and/or migration of grafted cells, and promote regeneration of host axons (Liu et al., 2019). Biomaterials can be used to make 3D scaffolds that promote cell survival, cellular interactions, proliferation, physical protection, and regeneration of cells while avoiding any adverse reactions to the organism (Marin et al., 2020; Feng et al., 2023). The idea of developing scaffolds is to mimic the extracellular environment of the spinal cord and reconstruct a favorable niche for SCI repair (Liu et al., 2019). At present, natural, synthetic and combined materials can be used to fabricate regenerative biomaterial scaffolds for SCI repair based on their different characteristics (Figure 1).
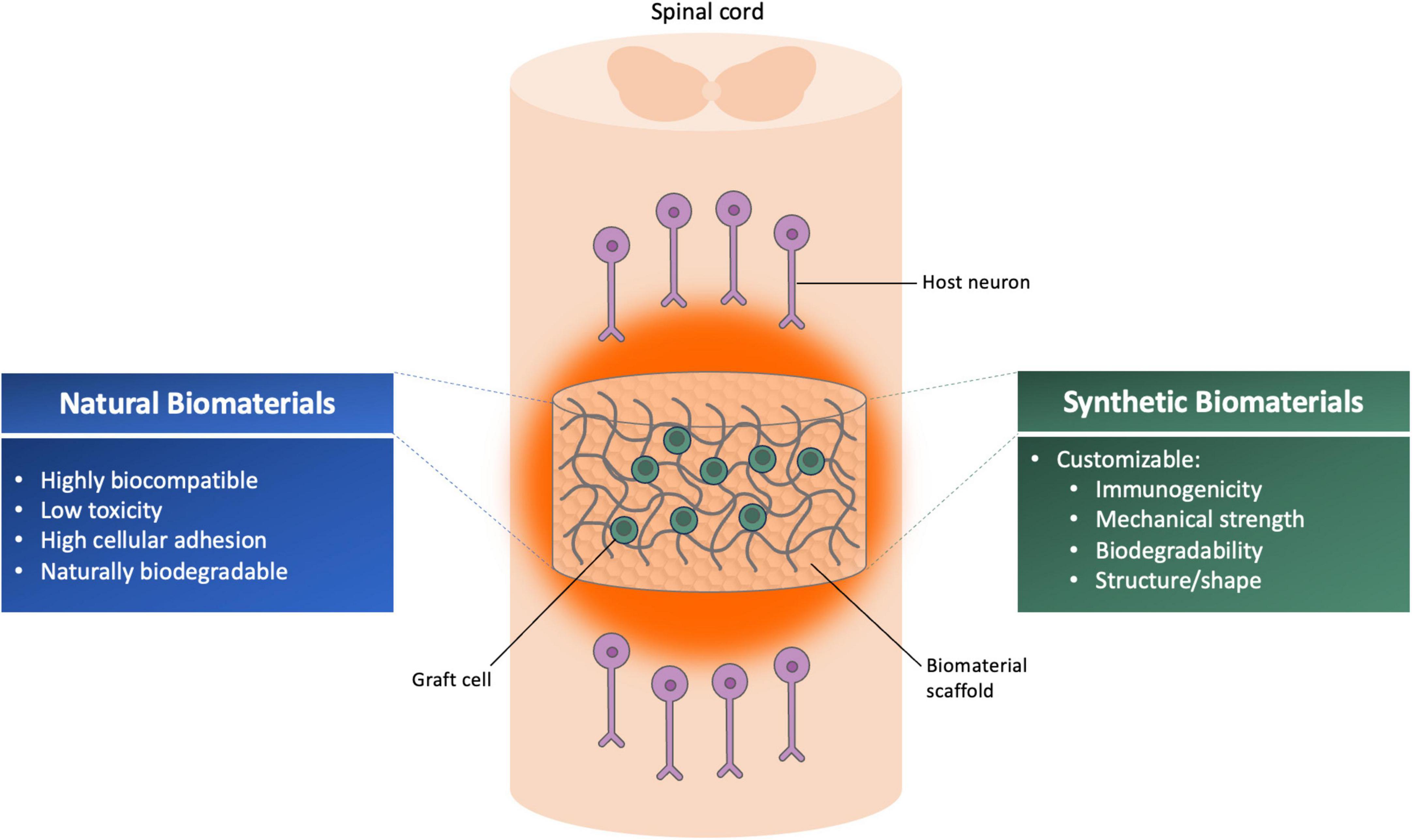
Figure 1. Illustration summarizing the advantages of different classes of biomaterial scaffolds for use with neural progenitor/cell transplantation therapy for spinal cord injury.
Natural materials have been widely used to develop various forms of scaffolds due to their advantageous properties such as excellent biocompatibility, biodegradability, and low toxicity (Libro et al., 2017). Natural polymers include nucleic acids, polysaccharides, proteins, lipids, and complex macromolecules such as proteoglycans. These natural polymers can form non-cytotoxic scaffolds either through self-assembly or using cross-linking techniques to encapsulate natural tissue properties (Joyce et al., 2021). Natural biomaterials mainly includes collagen, chitosan, hyaluronic acid, alginate, gelatin, agarose, and fibrin (Kim et al., 2000; Liu et al., 2019). Natural materials possess variety of advantages for use as biomaterial scaffolds for SCI repair but they also have inevitable disadvantages due to their intrinsic properties. For example, the mechanical properties of natural biomaterials cannot be easily manipulated as compared to synthetic biomaterials (Feng et al., 2023). For example, hyaluronic acid (HA) is a natural ECM component that is highly prevalent in the CNS, is known to promote regeneration, and is widely used in biomedical research (Jensen et al., 2020). However, HA may not necessarily be advantageous for severe SCI models; for instance, Koffler et al. (2019) demonstrated that a scaffold made of HA failed after transplantation of fetal rat spinal cord cells into an in vivo model of complete T3 spinal cord transection. The structure was not mechanically stable and collapsed by 4 weeks post-transplantation (Koffler et al., 2019).
Synthetic biomaterials are attractive to use due to their customizability. Their properties are often easier to tune than those of natural biomaterials; for example, porosity, stiffness, and degradation rates can be altered to match specific type of tissues (Feng et al., 2023). Some synthetic biomaterials possess strong mechanical properties, well-controlled biodegradability, low toxicity, low inflammatory response, customizable structure, and low immunogenicity (Subramanian et al., 2009). Widely used synthetic biomaterials include polyethylene glycol (PEG), polylactic acid, polylactic acid-hydroxyacetic acid copolymer, polyacrylamide, polyvinyl alcohol, polymethyl methacrylate, polyglycolic acid, and polycaprolactone. Among these polylactic acid, polyglycolic acid, and polycaprolactone are biodegradable synthetic polymers and are well known for their high mechanical strength, flexibility, and nontoxic degradability (Gunatillake and Adhikari, 2003; Shalumon et al., 2011). To improve efficacy of SCI repair, biomaterials can also be combined to form hybrid scaffolds that allow to incorporate the advantages of different single scaffolds and to overcome some disadvantages (Turnbull et al., 2018).
The elastic modulus as well as 3D architecture of biomaterial scaffolds are important considerations for experimental SCI studies. In one prominent study, Koffler et al. (2019) developed a method for 3D printing of biomimetic hydrogel scaffolds that mimic the architecture of a rodent spinal cord. Scaffolds were loaded with cells obtained from fetal (E14) rat spinal cords to support regeneration and form new neural relays across the site of injury. They observed axon outgrowth, graft survival, regeneration of host axons into scaffolds, and formation of synaptic connections between host axons and graft-derived neurons. In addition, the animals that received cell-loaded scaffolds recovered significantly greater locomotor function compared to the graft alone or scaffold alone groups (Koffler et al., 2019) (details of this and all subsequent studies highlighted in this article are described in detail in Table 1). Electrophysiological recordings before and after re-transection was performed suggest that establishment of de novo neural relays through the scaffolds are the underlying mechanism for this functional recovery (Koffler et al., 2019). Hence, this study demonstrated the importance of biomimetic materials to support directional growth of axons along conduits that resemble the architecture of the intact spinal cord. In another study, it was shown that a multichannel biodegradable polymer scaffold seeded with brain-derived fetal rat neural stem cells supported axonal regeneration after spinal cord transection in rats, with significantly greater host axon regeneration into neural stem cell (NSC)-containing scaffolds compared to scaffolds without cells. This study shows an effective use of multichannel biodegradable polymer scaffolds for quantitative analysis of axonal regeneration (Olson et al., 2009).
In recent years, decellularized tissue matrix (DTM) has garnered interest as a promising natural biomaterial for soft tissue repair or replacement therapeutics (Hussey et al., 2018). DTMs are prepared by either chemical or physical decellularization of mammalian tissues, removal of cellular antigens makes DTMs less immunogenic for host environment after implantation (Badylak, 2014). In one study, fetal rat hippocampus-derived NSPCs were embedded in DTM hydrogels (derived from either spinal cord or peripheral nerve) with low seeding density, and a large amount of cell proliferation was observed after 3 days of culture in DTM-gel (Xu et al., 2021). It was observed that NSPCs embedded in the hydrogel started spreading out and migrated farther into the DTM-gel within a 200-μm radius. The authors concluded that DTM-gel seeded with NSPCs facilitated stem cell proliferation and migration which might be because of its high porosity and tissue-specific components that provide a growth permissive environment. The authors also performed an in vivo study and reported that animals complete spinal cord transection had slightly improved hindlimb locomotor function if implanted with spinal cord-derived DTM compared to other hydrogels, but did not evaluate combined transplantation of NSPCs and hydrogels. This functional recovery was attributed by the authors to recruitment of endogenous NSPCs and enhancement of neurite sprouting toward the lesion gap (Xu et al., 2021). This study highlights the benefits of using DTMs; as these are decellularized form of mammalian tissue they are able to provide the native microenvironment and structure of the tissue. The DTM microenvironment may provide an ideal environment for cell transplantation and could reduce immunogenicity following transplantation into the injured spinal cord (Lee et al., 2018; Xu et al., 2021).
In another study, a porous collagen-based scaffold (PCS) was used to examine the proliferation, viability and neuronal differentiation of fetal mouse neural stem cells, as well as the therapeutic effect of NSCs on locomotor recovery following SCI (Kourgiantaki et al., 2020). The authors found that PCS-enabled delivery of NSCs at the SCI lesion site supported survival, axon ingrowth, and significantly reduced astrogliosis. These scaffolds seeded with NSCs supported significant locomotor functional recovery compared to injury-only controls, which the authors attribute to the ability of PCS to promote retention of seeded cells within the lesion site and graft integration with the host spinal cord (Kourgiantaki et al., 2020). Although some studies have used biomaterials for SCI treatment, clinical translation remains challenging as most of the biomaterials are not clinically approved. This study is remarkable in its demonstration of survival, differentiation of NSCs and functional recovery using a biomaterial that the authors indicate is similar to FDA-approved materials already utilized in the clinic for promoting regeneration skin and peripheral nerve wounds (Yannas et al., 1982, 1989; Soller et al., 2012).
Biomaterials may also provide a reliable and inexpensive way to control mechanical or chemical properties of the cellular environment, which is critical to direct cell behavior (Discher et al., 2009). Recent research has provided insight into the necessary chemical (Kang et al., 2007; Johnson et al., 2010; Fuhrmann et al., 2016) and mechanical properties (Brannvall et al., 2007; Rosenberg et al., 2008; Aurand et al., 2012) to direct differentiation of NSPCs toward neuron and astrocyte cell fate. For example, it was shown that a collagen, HA, and laminin-based hydrogel system could direct the differentiation of fetal rat NPCs into oligodendrocytes, potentially by mimicking ECM characteristics (Geissler et al., 2018). This study also reported significantly improved recovery of forelimb-dependent sensorimotor tasks in rats that received NPCs embedded in these hydrogels, versus controls. Although the authors did not explore the mechanism underlying functional recovery, they observed that animals receiving combined treatment exhibited significantly greater spinal cord tissue sparing and significantly decreased astroglial immunoreactivity compared to controls, suggesting a neuroprotective effect of treatment. This study shows that multiple-component biomaterials have potential to direct fates and therapeutic effects of co-transplanted cells (Geissler et al., 2018).
Recently, Liu W. et al. (2023) demonstrated that covalent conjugation between cells and biomaterials can be utilized to promote neural regeneration in a rat SCI model. Using a click chemistry approach, covalent conjugation of primary human spinal cord NPCs with collagen fibers promoted cell adhesion, prolonged cell retention on the scaffolds, and oriented axon outgrowth along the direction of fibers. Notably, differentiated cell types including astrocytes and Schwann cells were also utilized in this approach, and exhibited different effects on axon regeneration and vascularization. In an in vivo SCI experiment, the authors observed slight but significant improvements in locomotor function with combined treatment of NPC-seeded collagen fibers plus systemically administered liposomes, compared to other treatment groups, but mechanisms of recovery were not explored (Liu W. et al., 2023). These findings illustrate that chemical modification is a novel way to modulate interactions between transplanted cells and the biomaterial scaffold to promote cell retention and other desirable outcomes after SCI.
Natural and synthetic biomaterials have been widely used for clinical and preclinical investigations in biomedical research (Marin et al., 2020). Tissue engineering technology is a promising potential strategy to treat SCI through promoting regeneration of cells and tissue (Masaeli et al., 2019). However, there is still much work to be done toward optimizing biomaterials to promote robust and reproducible functional recovery following SCI. More research evidence are needed to optimize NSPC survival, regeneration and functional integration with host cells using biomaterials, especially in severe SCI models that do not support robust engraftment.
Enhancing the therapeutic efficacy of NSPCs with neuromodulation
Neuromodulation therapy for SCI has developed rapidly in recent years. In fact, over 27% of SCI clinical trials initiated in 2021 employ some form of neuromodulation; more than any other intervention (Dietz et al., 2022). Neuromodulation involves alteration of nerve activity through targeted delivery of electrical or magnetic stimulation or ultrasound in order to alter neuronal activity (De Ridder et al., 2017; Vasanthan et al., 2019). There are invasive and noninvasive strategies for modulating neuronal activity. Invasive techniques include methods to stimulate the spinal cord, deep brain structures, or peripheral nerves using electrodes. Non-invasive techniques such as transcranial magnetic stimulation, transcranial direct current stimulation, epidural stimulation, and focused ultrasound utilize neuromodulatory devices that induce neuronal activity without penetrating the CNS. Neuromodulation is considered as one of the most promising treatment strategies for SCI because of the potential for electrically activating isolated neuronal circuits below the injury site which are intact but can no longer efficiently receives or transmit sensory information for processing (Squair et al., 2016). In recent years, several remarkable studies have reported significant neurological functional recovery in individuals with complete and chronic SCI and other neurodegenerative conditions (Harkema et al., 2011; Angeli et al., 2014; Rejc et al., 2015; Grahn et al., 2017; Gill et al., 2018; Darrow et al., 2019; Rowald et al., 2022; Lorach et al., 2023; Milekovic et al., 2023).
Various studies have also reported modest success in alleviating pain with epidural stimulation (Linderoth and Meyerson, 2010; Deer et al., 2014). The relatively noninvasive implantation of epidural electrodes led researchers to explore its other applications beyond pain relief (Kapural et al., 2016; Al-Kaisy et al., 2018; Deer et al., 2018). A case study including participants with chronic motor-complete SCI shows that epidural stimulation of lumbosacral spinal cord could enable them to make small leg movements with only stimulation, but when the stimulation was combined with physical training, participants was able to sustain contractions and generate force during leg flexion exercise (Angeli et al., 2014). In a follow up study, further improvements were reported with continued activity-based interventions without continuing stimulation (Rejc et al., 2017). These studies highlight the ability of electrical modulation to improve functional outcomes after spinal cord injury. Additionally, progressive improvements in the follow up study even after discontinuing stimulation might be because of neural adaptation during combined treatment with rehabilitation. However, it is important to keep in mind that enrollment in these clinical studies are limited to small numbers of patients; to get robust evidence of functional improvement, further studies including more SCI patients are necessary.
Combining NSPC transplantation with neuromodulatory approaches is a promising strategy for enhancing graft/host neural relay formation and neurological recovery following SCI (Courtine and Sofroniew, 2019; Zheng et al., 2020). To date, however, there have only been a handful of published studies combining these two approaches. Some potential clues about the potential effects of neuromodulation on transplanted NSPCs can be gleaned from studies of how endogenous NSPC behavior is altered by neuromodulation. TMS is used to noninvasively stimulate the nervous system via electromagnetic induction (Blackmore et al., 2019). In a recent study, it was found that low frequency repetitive TMS is effective in promoting endogenous brain NSC proliferation and neuronal differentiation (Abbasnia et al., 2015). Others have observed upregulation of neural stem cell growth promoting factors and neurotransmitters upon repetitive TMS (Feng et al., 2012). In support of that, Piacentini et al. (2008) showed that extremely low frequency electromagnetic fields exposure promotes NSC differentiation by upregulating Ca(v)1-channel expression and function. Together, these studies suggest that neuromodulation using electromagnetic fields could be a useful strategy to improve the injured microenvironment after SCI, through positively regulating cell proliferation and differentiation, release of neural stem cell growth promoting factors, and neurotransmitters.
Becker et al. (2010) showed that functional electrical stimulation (FES) from devices implanted adjacent to the peroneal nerve promoted progenitor proliferation following complete thoracic spinal cord transection. Rats received FES implants 3 weeks after T8/9 transection, and bromodeoxyuridine (BrdU) was injected 10 days later. The authors found that FES led to a near doubling in the birth and survival of BrdU+ cells that expressed nestin, a marker of multipotent neural progenitors, in the lumbar spinal cord (Becker et al., 2010). Another study examined the effects of electrical stimulation on spinal cord neurogenesis in rats after SCI (Bang et al., 2023). In this study, electrodes were used to deliver direct electrical stimulation to the spinal cord lesion site for 4 h/day from 2 to 6 weeks after T10 contusion SCI. Spinal cord neuronal differentiation was quantified at the study endpoint. Compared to animals that received SCI without stimulation, SCI + stimulation animals exhibited significantly greater numbers of neurons and nestin+ cells, suggesting increased neuronal differentiation; improved tissue sparing and locomotor recovery was also observed (Bang et al., 2023). Together, these studies indicate that either direct stimulation of the injured spinal cord or stimulation of the peripheral nerves can promote increased cell survival and neuronal differentiation following SCI, suggesting potential benefits for survival and/or proliferation of engrafted neural stem and progenitor cells.
To date, only a handful of studies have examined the effects of neuromodulation on NSPC engraftment and associated functional recovery after transplantation into sites of SCI. Recently, Lai et al. (2023) performed electrical stimulation of the tail nerve as a neuromodulatory approach to promote conduction of signals in neural stem cell-derived “spinal cord-like tissue” transplants in sites of complete spinal cord transection. Adult hippocampal rat NSCs expressing the neurotrophic factor NT-3 and receptor TrkC were cultured together with oligodendrocyte precursor cells expressing CNTF in collagen scaffolds to form the spinal cord-like tissue transplants, which were placed into sites of T10 transection SCI immediately following injury. Nerve stimulation was performed five times per week beginning at day 8 post-SCI and continuing once daily for 8 weeks. The authors found that the combination of nerve stimulation and transplantation resulted in the greatest locomotor functional recovery compared to SCI only, transplant only, and stimulation only groups, with animals in the combined treatment group achieving weight-supported stepping. These subjects also exhibited increased regeneration of neurofilament+ axons and myelination of regenerating axons, compared to other groups; additionally, transsynaptic tracing results also demonstrated a greater degree of neural connectivity across the lesion site in animals with combined treatment (Lai et al., 2023).
Liu W. et al. (2023) applied physiological electric field stimulation to NSCs prior to transplantation, in order to mimic the electrical potential that is present in the developing neural tube as a method to promote survival and differentiation. NSCs obtained from embryonic mouse brain were subjected to stimulation with a direct current electrical field, then transplanted into sites of spinal cord contusion injury. Following transplantation, the authors reported a significantly higher survival rate in stimulated NSCs versus unstimulated NSCs (40% ± 6.6% versus 21.9% ± 9.1%, respectively), as well as increased neuronal differentiation and neurite outgrowth from stimulated transplants. The PI3K/Akt/GSK-3β/β-catenin signaling cascade was shown to be required for the effects of electrical field stimulation on boosting neuronal differentiation. Mice receiving transplants of stimulated NSCs performed better on locomotor functional assessments versus mice that received unstimulated transplants, presumably due to enhanced graft survival following stimulation (Liu W. et al., 2023).
In another study, a neuroregenerative scaffold in combination with epidural electrical stimulation (EES) was found to promote functional recovery in rats with complete spinal cord transection (Siddiqui et al., 2021). Siddiqui et al. (2021) used hydrogel scaffolds composed of positively charged oligo-[poly(ethylene glycol)fumarate] loaded with neurotrophic factor (GDNF)-secreting rat Schwann cells and rapamycin microspheres. Scaffolds were seeded with GDNF-expressing Schwann cells and rapamycin microspheres, and transplanted into sites of complete T9 transection. Animals received EES via implanted electrodes over the sacral (S1) spinal cord to enable motor training on a treadmill, such that stimulation enabled stepping (EES-facilitated training). Animals received manual bipedal step training rehabilitation 3 days/week for 6 weeks after SCI under the influence of EES. The authors found that combined treatment with scaffolds and EES-enabled stepping led to significantly greater locomotor functional improvement as compared to individual treatment or control groups by 6 weeks post-injury (Siddiqui et al., 2021). This recovery could be due to effects of treatment on plasticity, as animals with combined therapy contained greater densities of synaptic boutons colocalized with motor neurons and interneurons in the lumbosacral spinal cord versus animals with either treatment alone. This study shows the use combinational approach of cell-containing scaffold with electrical stimulation to demonstrate that regenerated axons through the length of the scaffold can reorganize the neural circuitry around SCI and improve motor performance. More studies like this are needed to understand the mechanism behind the recovery and therapeutic effects of these combined interventions and to maximize functional restoration after SCI.
Finally, electrically conductive biomaterials can be used in conjunction with NSPCs as a method to promote neuromodulation of grafted cells. Serafin et al. (2023) demonstrated recently a combinatorial approach using natural biomaterials (hyaluronic acid and gelatin) in combination with polypyrrole (PPy) nanoparticles to create conductive scaffolds. PPy is the most commonly studied conductive biomaterial (George et al., 2017; Oh et al., 2018). The authors found that mesenchymal stem cells seeded on these scaffolds attached well and proliferated in vitro (Serafin et al., 2023). The same group used novel poly(3,4-ethylenedioxythiophene) nanoparticles to increase the conductivity of hydrogel scaffolds as a therapeutic approach for spinal cord injury (Serafin et al., 2022). In this in vivo study, using a model of T3 complete spinal cord transection in rats, the authors showed high conductivity, and reduced gliosis and inflammatory response in the animals receiving scaffolds. In another study, conductive polymer made of PPy allowed for electrical stimulation of human neural progenitor cells (hNPCs) implanted within the biomaterial. Song et al. (2021) applied electrical stimulation in a rat sciatic nerve transection model and found that electrical stimulation of the nerve combined with human neural progenitor cell-laden scaffolds significantly promoted nerve regeneration, nerve conduction, myelination, and recovery of nerve function in rats after sciatic nerve transection. The dramatic improvement in functional recovery observed in this study is attributed by the authors to increased myelination and a greater degree of neurotrophic factor release. These results highlights the positive effect of combinational approaches using conductive materials and electrical stimulation of transplanted stem/progenitor cells to enhance therapeutic potential of stem cell therapy.
Neuromodulation is a rapidly growing field itself with great potential for treatment of SCI, evident by a growing number of clinical trials and a great deal of experimental evidence of functional recovery (Harkema et al., 2011; Ho et al., 2014; Ajiboye et al., 2017). Hence, combinatorial strategies incorporating regenerative therapies such as NSPC transplantation with neuromodulation are likely to lead to the next generation of SCI therapies. As discussed in this review, there is emerging evidence to suggest that neuromodulation intervention can augment the effects of regenerative therapies after SCI. However, much more work is needed to evaluate the therapeutic efficacy of combined cell transplantation and electrical or magnetic stimulation strategies, and to determine the mechanisms by which these therapies alone or in combination can promote functional recovery.
Discussion
Neural stem/progenitor cell transplantation therapies, use of biomaterial scaffolds, and neuromodulation techniques has been extensively used in efforts of improving functional outcomes after SCI. Modest gain in functional outcomes has been observed following treatment with these therapies, but not complete recovery, as per the complex nature of SCI the pathology cannot be fully addressed by a single therapeutic approach. The lack of success may be addressed by a combinatorial or multi-disciplinary approach, where multiple interventions can be used as an integrated approach for SCI. Thus, a critical point for future research is to optimize the degree of functional recovery that can be achieved after SCI.
In this review we highlight and promote the use of NSPC transplantation therapy combined with biomaterial scaffold and neuromodulation techniques to improve anatomical and functional recovery after SCI. Nevertheless, work needs to be done to find out whether any of these therapies when combined with NSPC can produce cumulative improvements after human SCI. To validate the efficacy of combined therapies, preclinical studies should be reproduced by multiple laboratories to increase confidence in positive results.
Author contributions
VJ: Writing – original draft, Writing – review & editing. JK: Funding acquisition, Writing – original draft, Writing – review & editing. JD: Funding acquisition, Writing – original draft, Writing – review & editing.
Funding
The author(s) declare financial support was received for the research, authorship, and/or publication of this article. This work was supported by the National Institutes of Health (R01NS116404, to JD), Mission Connect, a program of TIRR Foundation (JD), and Veterans Affairs (1I01RX003405 to JK).
Conflict of interest
The authors declare that the research was conducted in the absence of any commercial or financial relationships that could be construed as a potential conflict of interest.
Publisher’s note
All claims expressed in this article are solely those of the authors and do not necessarily represent those of their affiliated organizations, or those of the publisher, the editors and the reviewers. Any product that may be evaluated in this article, or claim that may be made by its manufacturer, is not guaranteed or endorsed by the publisher.
Abbreviations
BrdU, bromodeoxyuridine; DTM, decellularized tissue matrix; EES, epidural electrical stimulation; FES, functional electrical stimulation; GDNF, glial-derived neurotrophic factor; HA, hyaluronic acid; hNPCs, human neural progenitor cells; iPSC, induced pluripotent stem cell; NSC, neural stem cell; NSPC, neural stem/progenitor cells; PCS, porous collagen-based scaffold; PEG, polyethylene glycol; PPy, polypyrrole; SCI, spinal cord injury.
References
Abbasnia, K., Ghanbari, A., Abedian, M., Ghanbari, A., Sharififar, S., and Azari, H. (2015). The effects of repetitive transcranial magnetic stimulation on proliferation and differentiation of neural stem cells. Anat. Cell Biol. 48, 104–113. doi: 10.5115/acb.2015.48.2.104
Ajiboye, A. B., Willett, F., Young, D. R., Memberg, W. D., Murphy, B. A., Miller, J., et al. (2017). Restoration of reaching and grasping movements through brain-controlled muscle stimulation in a person with tetraplegia: A proof-of-concept demonstration. Lancet 389, 1821–1830. doi: 10.1016/S0140-6736(17)30601-3
Al-Kaisy, A., Palmisani, S., Pang, D., Sanderson, K., Wesley, S., Tan, Y., et al. (2018). Prospective, randomized, sham-control, double blind, crossover trial of subthreshold spinal cord stimulation at various kilohertz frequencies in subjects suffering from failed back surgery syndrome (SCS frequency study). Neuromodulation 21, 457–465. doi: 10.1111/ner.12771
Angeli, C. A., Edgerton, V., Gerasimenko, Y., and Harkema, S. (2014). Altering spinal cord excitability enables voluntary movements after chronic complete paralysis in humans. Brain 137, 1394–1409. doi: 10.1093/brain/awu038
Aurand, E. R., Lampe, K. J., and Bjugstad, K. B. (2012). Defining and designing polymers and hydrogels for neural tissue engineering. Neurosci. Res. 72, 199–213. doi: 10.1016/j.neures.2011.12.005
Badylak, S. F. (2014). Decellularized allogeneic and xenogeneic tissue as a bioscaffold for regenerative medicine: Factors that influence the host response. Ann. Biomed. Eng. 42, 1517–1527. doi: 10.1007/s10439-013-0963-7
Bang, W. S., Han, I., Mun, S., Hwang, J., Noh, S., Son, W., et al. (2023). Electrical stimulation promotes functional recovery after spinal cord injury by activating endogenous spinal cord-derived neural stem/progenitor cell: An in vitro and in vivo study. Spine J. 24, 534–553. doi: 10.1016/j.spinee.2023.10.004
Becker, D., Gary, D., Rosenzweig, E., Grill, W., and McDonald, J. (2010). Functional electrical stimulation helps replenish progenitor cells in the injured spinal cord of adult rats. Exp. Neurol. 222, 211–218. doi: 10.1016/j.expneurol.2009.12.029
Blackmore, J., Shrivastava, S., Sallet, J., Butler, C., and Cleveland, R. (2019). Ultrasound neuromodulation: A review of results, mechanisms and safety. Ultrasound Med. Biol. 45, 1509–1536. doi: 10.1016/j.ultrasmedbio.2018.12.015
Bonner, J. F., Connors, T., Silverman, W., Kowalski, D., Lemay, M., and Fischer, I. (2011). Grafted neural progenitors integrate and restore synaptic connectivity across the injured spinal cord. J. Neurosci. 31, 4675–4686. doi: 10.1523/JNEUROSCI.4130-10.2011
Brannvall, K., Bergman, K., Wallenquist, U., Svahn, S., Bowden, T., Hilborn, J., et al. (2007). Enhanced neuronal differentiation in a three-dimensional collagen-hyaluronan matrix. J. Neurosci. Res. 85, 2138–2146. doi: 10.1002/jnr.21358
Brock, J. H., Graham, L., Staufenberg, E., Im, S., and Tuszynski, M. (2018). Rodent neural progenitor cells support functional recovery after cervical spinal cord contusion. J. Neurotrauma 35, 1069–1078. doi: 10.1089/neu.2017.5244
Cao, Q., Benton, R. L., and Whittemore, S. R. (2002). Stem cell repair of central nervous system injury. J. Neurosci. Res. 68, 501–510. doi: 10.1002/jnr.10240
Courtine, G., and Sofroniew, M. V. (2019). Spinal cord repair: Advances in biology and technology. Nat. Med. 25, 898–908. doi: 10.1038/s41591-019-0475-6
Cummings, B. J., Uchida, N., Tamaki, S., and Anderson, A. (2006). Human neural stem cell differentiation following transplantation into spinal cord injured mice: Association with recovery of locomotor function. Neurol. Res. 28, 474–481. doi: 10.1179/016164106X115116
Cummings, B. J., Uchida, N., Tamaki, S., Salazar, D., Hooshmand, M., Summers, R., et al. (2005). Human neural stem cells differentiate and promote locomotor recovery in spinal cord-injured mice. Proc. Natl. Acad. Sci. U.S.A. 102, 14069–14074. doi: 10.1073/pnas.0507063102
Darrow, D., Balser, D., Netoff, T., Krassioukov, A., Phillips, A., Parr, A., et al. (2019). Epidural spinal cord stimulation facilitates immediate restoration of dormant motor and autonomic supraspinal pathways after chronic neurologically complete spinal cord injury. J. Neurotrauma 36, 2325–2336. doi: 10.1089/neu.2018.6006
De Ridder, D., Perera, S., and Vanneste, S. (2017). State of the art: Novel applications for cortical stimulation. Neuromodulation 20, 206–214. doi: 10.1111/ner.12593
Deer, T. R., Mekhail, N., Provenzano, D., Pope, J., Krames, E., Leong, M., et al. (2014). The appropriate use of neurostimulation of the spinal cord and peripheral nervous system for the treatment of chronic pain and ischemic diseases: The neuromodulation appropriateness consensus committee. Neuromodulation 17, 515–50; discussion550. doi: 10.1111/ner.12208
Deer, T., Slavin, K., Amirdelfan, K., North, R., Burton, A., Yearwood, T., et al. (2018). Success using neuromodulation with BURST (SUNBURST) study: Results from a prospective, randomized controlled trial using a novel burst waveform. Neuromodulation 21, 56–66. doi: 10.1111/ner.12698
Dietz, V. A., Roberts, N., Knox, K., Moore, S., Pitonak, M., Barr, C., et al. (2022). Fighting for recovery on multiple fronts: The past, present, and future of clinical trials for spinal cord injury. Front. Cell. Neurosci. 16:977679. doi: 10.3389/fncel.2022.977679
Discher, D. E., Mooney, D. J., and Zandstra, P. W. (2009). Growth factors, matrices, and forces combine and control stem cells. Science 324, 1673–1677. doi: 10.1126/science.1171643
Dougherty, B. J., Gonzalez-Rothi, E., Lee, K., Ross, H., Reier, P., and Fuller, D. (2016). Respiratory outcomes after mid-cervical transplantation of embryonic medullary cells in rats with cervical spinal cord injury. Exp. Neurol. 278, 22–26. doi: 10.1016/j.expneurol.2016.01.017
Dugan, E. A., Jergova, S., and Sagen, J. (2020). Mutually beneficial effects of intensive exercise and GABAergic neural progenitor cell transplants in reducing neuropathic pain and spinal pathology in rats with spinal cord injury. Exp. Neurol. 327:113208. doi: 10.1016/j.expneurol.2020.113208
Fandel, T. M., Trivedi, A., Nicholas, C., Zhang, H., Chen, J., Martinez, A., et al. (2016). Transplanted human stem cell-derived interneuron precursors mitigate mouse bladder dysfunction and central neuropathic pain after spinal cord injury. Cell Stem Cell 19, 544–557. doi: 10.1016/j.stem.2016.08.020
Feng, C., Deng, L., Yong, Y., Wu, J., Qin, D., Yu, L., et al. (2023). The application of biomaterials in spinal cord injury. Int. J. Mol. Sci. 24:816. doi: 10.3390/ijms24010816
Feng, S. F., Shi, T., Fan-Yang, Wang, W., Chen, Y., and Tan, Q. (2012). Long-lasting effects of chronic rTMS to treat chronic rodent model of depression. Behav. Brain Res. 232, 245–251. doi: 10.1016/j.bbr.2012.04.019
Fischer, I., Dulin, J. N., and Lane, M. A. (2020). Transplanting neural progenitor cells to restore connectivity after spinal cord injury. Nat. Rev. Neurosci. 21, 366–383. doi: 10.1038/s41583-020-0314-2
Fuhrmann, T., Tam, R., Ballarin, B., Coles, B., Elliott Donaghue, I., van der Kooy, D., et al. (2016). Injectable hydrogel promotes early survival of induced pluripotent stem cell-derived oligodendrocytes and attenuates longterm teratoma formation in a spinal cord injury model. Biomaterials 83, 23–36. doi: 10.1016/j.biomaterials.2015.12.032
Geissler, S. A., Sabin, A., Besser, R., Gooden, O., Shirk, B., Nguyen, Q., et al. (2018). Biomimetic hydrogels direct spinal progenitor cell differentiation and promote functional recovery after spinal cord injury. J. Neural Eng. 15:025004. doi: 10.1088/1741-2552/aaa55c
George, P. M., Bliss, T., Hua, T., Lee, A., Oh, B., Levinson, A., et al. (2017). Electrical preconditioning of stem cells with a conductive polymer scaffold enhances stroke recovery. Biomaterials 142, 31–40. doi: 10.1016/j.biomaterials.2017.07.020
Gill, M. L., Grahn, P., Calvert, J., Linde, M., Lavrov, I., Strommen, J., et al. (2018). Neuromodulation of lumbosacral spinal networks enables independent stepping after complete paraplegia. Nat. Med. 24, 1677–1682. doi: 10.1038/s41591-018-0175-7
Gomes, E. D., Silva, N. A., and Salgado, A. J. (2019). Combinatorial therapies for spinal cord injury: Strategies to induce regeneration. Neural Regen. Res. 14, 69–71. doi: 10.4103/1673-5374.243705
Grahn, P. J., Lavrov, I., Sayenko, D., Van Straaten, M., Gill, M., Strommen, J., et al. (2017). Enabling task-specific volitional motor functions via spinal cord neuromodulation in a human with paraplegia. Mayo Clin. Proc. 92, 544–554. doi: 10.1016/j.mayocp.2017.02.014
Griffin, J. M., and Bradke, F. (2020). Therapeutic repair for spinal cord injury: Combinatory approaches to address a multifaceted problem. EMBO Mol. Med. 12:e11505. doi: 10.15252/emmm.201911505
Gunatillake, P. A., and Adhikari, R. (2003). Biodegradable synthetic polymers for tissue engineering. Eur. Cell Mater. 5, 1–16; discussion16. doi: 10.22203/eCM.v005a01
Han, S. S., Kang, D., Mujtaba, T., Rao, M., and Fischer, I. (2002). Grafted lineage-restricted precursors differentiate exclusively into neurons in the adult spinal cord. Exp. Neurol. 177, 360–375. doi: 10.1006/exnr.2002.7995
Harkema, S., Gerasimenko, Y., Hodes, J., Burdick, J., Angeli, C., Chen, Y., et al. (2011). Effect of epidural stimulation of the lumbosacral spinal cord on voluntary movement, standing, and assisted stepping after motor complete paraplegia: A case study. Lancet 377, 1938–1947. doi: 10.1016/S0140-6736(11)60547-3
Ho, C. H., Triolo, R., Elias, A., Kilgore, K., DiMarco, A., Bogie, K., et al. (2014). Functional electrical stimulation and spinal cord injury. Phys. Med. Rehabil. Clin. N. Am. 25, 631–54, ix. doi: 10.1016/j.pmr.2014.05.001
Hussey, G. S., Dziki, J. L., and Badylak, S. F. (2018). Extracellular matrix-based materials for regenerative medicine. Nat. Rev. Mater. 3, 159–173. doi: 10.1038/s41578-018-0023-x
Jensen, G., Holloway, J. L., and Stabenfeldt, S. E. (2020). Hyaluronic acid biomaterials for central nervous system regenerative medicine. Cells 9:2113. doi: 10.3390/cells9092113
Jeong, H. J., Yun, Y., Lee, S., Ha, Y., and Gwak, S. (2021). Biomaterials and strategies for repairing spinal cord lesions. Neurochem. Int. 144:104973. doi: 10.1016/j.neuint.2021.104973
Johnson, P. J., Tatara, A., Shiu, A., and Sakiyama-Elbert, S. E. (2010). Controlled release of neurotrophin-3 and platelet-derived growth factor from fibrin scaffolds containing neural progenitor cells enhances survival and differentiation into neurons in a subacute model of SCI. Cell Transplant. 19, 89–101. doi: 10.3727/096368909X477273
Joyce, K., Fabra, G., Bozkurt, Y., and Pandit, A. (2021). Bioactive potential of natural biomaterials: Identification, retention and assessment of biological properties. Signal. Transduct. Target. Ther. 6:122. doi: 10.1038/s41392-021-00512-8
Kadoya, K., Lu, P., Nguyen, K., Lee-Kubli, C., Kumamaru, H., Yao, L., et al. (2016). Spinal cord reconstitution with homologous neural grafts enables robust corticospinal regeneration. Nat. Med. 22, 479–487. doi: 10.1038/nm.4066
Kang, S. M., Cho, M., Seo, H., Yoon, C., Oh, S., Choi, Y., et al. (2007). Efficient induction of oligodendrocytes from human embryonic stem cells. Stem Cells 25, 419–424. doi: 10.1634/stemcells.2005-0482
Kapural, L., Yu, C., Doust, M., Gliner, B., Vallejo, R., Sitzman, B., et al. (2016). Comparison of 10-kHz high-frequency and traditional low-frequency spinal cord stimulation for the treatment of chronic back and leg pain: 24-month results from a multicenter, randomized, controlled pivotal trial. Neurosurgery 79, 667–677. doi: 10.1227/NEU.0000000000001418
Kawai, M., Imaizumi, K., Ishikawa, M., Shibata, S., Shinozaki, M., Shibata, T., et al. (2021). Long-term selective stimulation of transplanted neural stem/progenitor cells for spinal cord injury improves locomotor function. Cell Rep. 37:110019. doi: 10.1016/j.celrep.2021.110019
Kim, B. S., Baez, C. E., and Atala, A. (2000). Biomaterials for tissue engineering. World J. Urol. 18, 2–9. doi: 10.1007/s003450050002
Kitagawa, T., Nagoshi, N., Kamata, Y., Kawai, M., Ago, K., Kajikawa, K., et al. (2022). Modulation by DREADD reveals the therapeutic effect of human iPSC-derived neuronal activity on functional recovery after spinal cord injury. Stem Cell Rep. 17, 127–142. doi: 10.1016/j.stemcr.2021.12.005
Koffler, J., Zhu, W., Qu, X., Platoshyn, O., Dulin, J. N., Brock, J., et al. (2019). Biomimetic 3D-printed scaffolds for spinal cord injury repair. Nat. Med. 25, 263–269. doi: 10.1038/s41591-018-0296-z
Kourgiantaki, A., Tzeranis, D., Karali, K., Georgelou, K., Bampoula, E., Psilodimitrakopoulos, S., et al. (2020). Neural stem cell delivery via porous collagen scaffolds promotes neuronal differentiation and locomotion recovery in spinal cord injury. NPJ Regen. Med. 5:12. doi: 10.1038/s41536-020-0097-0
Kumamaru, H., Kadoya, K., Adler, A., Takashima, Y., Graham, L., Coppola, G., et al. (2018). Generation and post-injury integration of human spinal cord neural stem cells. Nat. Methods 15, 723–731. doi: 10.1038/s41592-018-0074-3
Lai, B. Q., Wu, R., Han, W., Bai, Y., Liu, J., Yu, H., et al. (2023). Tail nerve electrical stimulation promoted the efficiency of transplanted spinal cord-like tissue as a neuronal relay to repair the motor function of rats with transected spinal cord injury. Biomaterials 297:122103. doi: 10.1016/j.biomaterials.2023.122103
Lane, M. A., Lepore, A. C., and Fischer, I. (2017). Improving the therapeutic efficacy of neural progenitor cell transplantation following spinal cord injury. Expert Rev. Neurother. 17, 433–440. doi: 10.1080/14737175.2017.1270206
Lee, J. S., Choi, Y. S., and Cho, S. W. (2018). Decellularized Tissue Matrix for Stem Cell and Tissue Engineering. Adv. Exp. Med. Biol. 1064, 161–180. doi: 10.1007/978-981-13-0445-3_10
Lee, K. Z., Lane, M., Dougherty, B., Mercier, L., Sandhu, M., Sanchez, J., et al. (2014). Intraspinal transplantation and modulation of donor neuron electrophysiological activity. Exp. Neurol. 251, 47–57. doi: 10.1016/j.expneurol.2013.10.016
Libro, R., Bramanti, P., and Mazzon, E. (2017). The combined strategy of mesenchymal stem cells and tissue-engineered scaffolds for spinal cord injury regeneration. Exp. Ther. Med. 14, 3355–3368. doi: 10.3892/etm.2017.4939
Linderoth, B., and Meyerson, B. A. (2010). Spinal cord stimulation: Exploration of the physiological basis of a widely used therapy. Anesthesiology 113, 1265–1267. doi: 10.1097/ALN.0b013e3181fcf590
Liu, W., Xu, B., Zhao, S., Han, S., Quan, R., Liu, W., et al. (2023). Spinal cord tissue engineering via covalent interaction between biomaterials and cells. Sci. Adv. 9:eade8829. doi: 10.1126/sciadv.ade8829
Liu, Q., Telezhkin, V., Jiang, W., Gu, Y., Wang, Y., Hong, W., et al. (2023). Electric field stimulation boosts neuronal differentiation of neural stem cells for spinal cord injury treatment via PI3K/Akt/GSK-3beta/beta-catenin activation. Cell Biosci. 13:4. doi: 10.1186/s13578-023-00954-3
Liu, S., Xie, Y. Y., and Wang, B. (2019). Role and prospects of regenerative biomaterials in the repair of spinal cord injury. Neural Regen. Res. 14, 1352–1363. doi: 10.4103/1673-5374.253512
Lorach, H., Galvez, A., Spagnolo, V., Martel, F., Karakas, S., Intering, N., et al. (2023). Walking naturally after spinal cord injury using a brain-spine interface. Nature 618, 126–133. doi: 10.1038/s41586-023-06094-5
Lu, P., Wang, Y., Graham, L., McHale, K., Gao, M., Wu, D., et al. (2012). Long-distance growth and connectivity of neural stem cells after severe spinal cord injury. Cell 150, 1264–1273. doi: 10.1016/j.cell.2012.08.020
Lynskey, J. V., Sandhu, F., Dai, H., McAtee, M., Slotkin, J., MacArthur, L., et al. (2006). Delayed intervention with transplants and neurotrophic factors supports recovery of forelimb function after cervical spinal cord injury in adult rats. J. Neurotrauma 23, 617–634. doi: 10.1089/neu.2006.23.617
Marin, E., Boschetto, F., and Pezzotti, G. (2020). Biomaterials and biocompatibility: An historical overview. J. Biomed. Mater. Res. A 108, 1617–1633. doi: 10.1002/jbm.a.36930
Masaeli, R., Zandsalimi, K., and Tayebi, L. (2019). Biomaterials evaluation: Conceptual refinements and practical reforms. Ther. Innov. Regul. Sci. 53, 120–127. doi: 10.1177/2168479018774320
McDonald, J. W., Liu, X., Qu, Y., Liu, S., Mickey, S., Turetsky, D., et al. (1999). Transplanted embryonic stem cells survive, differentiate and promote recovery in injured rat spinal cord. Nat. Med. 5, 1410–1412. doi: 10.1038/70986
Milekovic, T., Moraud, E., Macellari, N., Moerman, C., Raschellà, F., Sun, S., et al. (2023). A spinal cord neuroprosthesis for locomotor deficits due to Parkinson’s disease. Nat. Med. 29, 2854–2865. doi: 10.1038/s41591-023-02584-1
Morse, L. R., Field-Fote, E., Contreras-Vidal, J., Noble-Haeusslein, L., Rodreick, M., Shields, R., et al. (2021). Meeting proceedings for SCI 2020: Launching a decade of disruption in spinal cord injury research. J. Neurotrauma 38, 1251–1266. doi: 10.1089/neu.2020.7174
National Spinal Cord Injury Statistical Center (2022). Traumatic spinal cord injury facts and figures at a glance. Birmingham: National Spinal Cord Injury Statistical Center.
Nori, S., Khazaei, M., Ahuja, C., Yokota, K., Ahlfors, J., Liu, Y., et al. (2018). Human oligodendrogenic neural progenitor cells delivered with chondroitinase ABC facilitate functional repair of chronic spinal cord injury. Stem Cell Rep. 11, 1433–1448. doi: 10.1016/j.stemcr.2018.10.017
Oh, B., Levinson, A., Lam, V., Song, S., and George, P. (2018). Electrically conductive scaffold to modulate and deliver stem cells. J. Vis. Exp. 134:57367. doi: 10.3791/57367-v
Olson, H. E., Rooney, G., Gross, L., Nesbitt, J., Galvin, K., Knight, A., et al. (2009). Neural stem cell- and Schwann cell-loaded biodegradable polymer scaffolds support axonal regeneration in the transected spinal cord. Tissue Eng. Part A 15, 1797–1805. doi: 10.1089/ten.tea.2008.0364
Piacentini, R., Ripoli, C., Mezzogori, D., Azzena, G., and Grassi, C. (2008). Extremely low-frequency electromagnetic fields promote in vitro neurogenesis via upregulation of Ca(v)1-channel activity. J. Cell Physiol. 215, 129–139. doi: 10.1002/jcp.21293
Pieczonka, K., and Fehlings, M. G. (2023). Incorporating combinatorial approaches to encourage targeted neural stem/progenitor cell integration following transplantation in spinal cord injury. Stem Cells Transl. Med. 12, 207–214. doi: 10.1093/stcltm/szad008
Rejc, E., Angeli, C., and Harkema, S. (2015). Effects of lumbosacral spinal cord epidural stimulation for standing after chronic complete paralysis in humans. PLoS One 10:e0133998. doi: 10.1371/journal.pone.0133998
Rejc, E., Angeli, C., Atkinson, D., and Harkema, S. (2017). Motor recovery after activity-based training with spinal cord epidural stimulation in a chronic motor complete paraplegic. Sci. Rep. 7:13476. doi: 10.1038/s41598-017-14003-w
Richardson, P. M., McGuinness, U. M., and Aguayo, A. J. (1980). Axons from CNS neurons regenerate into PNS grafts. Nature 284, 264–265. doi: 10.1038/284264a0
Rosenberg, S. S., Kelland, E., Tokar, E., De la Torre, A., and Chan, J. (2008). The geometric and spatial constraints of the microenvironment induce oligodendrocyte differentiation. Proc. Natl. Acad. Sci. U.S.A. 105, 14662–14667. doi: 10.1073/pnas.0805640105
Rosenzweig, E. S., Brock, J., Lu, P., Kumamaru, H., Salegio, E., Kadoya, K., et al. (2018). Restorative effects of human neural stem cell grafts on the primate spinal cord. Nat. Med. 24, 484–490. doi: 10.1038/nm.4502
Rowald, A., Komi, S., Demesmaeker, R., Baaklini, E., Hernandez-Charpak, S., Paoles, E., et al. (2022). Activity-dependent spinal cord neuromodulation rapidly restores trunk and leg motor functions after complete paralysis. Nat. Med. 28, 260–271. doi: 10.1038/s41591-021-01663-5
Serafin, A., Culebras, M., Oliveira, J., Koffler, J., and Collins, M. N. (2023). 3D printable electroconductive gelatin-hyaluronic acid materials containing polypyrrole nanoparticles for electroactive tissue engineering. Adv. Comp. Hybrid Mater. 6:109. doi: 10.1007/s42114-023-00665-w
Serafin, A., Rubio, M., Carsi, M., Ortiz-Serna, P., Sanchis, M., Garg, A., et al. (2022). Electroconductive PEDOT nanoparticle integrated scaffolds for spinal cord tissue repair. Biomater. Res. 26:63. doi: 10.1186/s40824-022-00310-5
Shalumon, K. T., Anulekha, K., Nair, S., Nair, S., Chennazhi, K., Jayakumar, R., et al. (2011). Sodium alginate/poly(vinyl alcohol)/nano ZnO composite nanofibers for antibacterial wound dressings. Int. J. Biol. Macromol. 49, 247–254. doi: 10.1016/j.ijbiomac.2011.04.005
Siddiqui, A. M., Islam, R., Cuellar, C., Silvernail, J., Knudsen, B., Curley, D., et al. (2021). Newly regenerated axons via scaffolds promote sub-lesional reorganization and motor recovery with epidural electrical stimulation. NPJ Regen. Med. 6:66. doi: 10.1038/s41536-021-00176-6
Soller, E. C., Tzeranis, D., Miu, K., So, P., and Yannas, I. (2012). Common features of optimal collagen scaffolds that disrupt wound contraction and enhance regeneration both in peripheral nerves and in skin. Biomaterials 33, 4783–4791. doi: 10.1016/j.biomaterials.2012.03.068
Song, S., McConnell, K., Amores, D., Levinson, A., Vogel, H., Quarta, M., et al. (2021). Electrical stimulation of human neural stem cells via conductive polymer nerve guides enhances peripheral nerve recovery. Biomaterials 275:120982. doi: 10.1016/j.biomaterials.2021.120982
Spruance, V. M., Zholudeva, L., Hormigo, K., Randelman, M., Bezdudnaya, T., Marchenko, V., et al. (2018). Integration of transplanted neural precursors with the injured cervical spinal cord. J. Neurotrauma 35, 1781–1799. doi: 10.1089/neu.2017.5451
Squair, J. W., Bjerkefors, A., Inglis, J., Lam, T., and Carpenter, M. (2016). Cortical and vestibular stimulation reveal preserved descending motor pathways in individuals with motor-complete spinal cord injury. J. Rehabil. Med. 48, 589–596. doi: 10.2340/16501977-2101
Subramanian, A., Krishnan, U. M., and Sethuraman, S. (2009). Development of biomaterial scaffold for nerve tissue engineering: Biomaterial mediated neural regeneration. J. Biomed. Sci. 16:108. doi: 10.1186/1423-0127-16-108
Sugai, K., Sumida, M., Shofuda, T., Yamaguchi, R., Tamura, T., Kohzuki, T., et al. (2021). First-in-human clinical trial of transplantation of iPSC-derived NS/PCs in subacute complete spinal cord injury: Study protocol. Regen. Ther. 18, 321–333. doi: 10.1016/j.reth.2021.08.005
Tashiro, S., Nishimura, S., Iwai, H., Sugai, K., Zhang, L., Shinozaki, M., et al. (2016). Functional recovery from neural stem/progenitor cell transplantation combined with treadmill training in mice with chronic spinal cord injury. Sci. Rep. 6:30898. doi: 10.1038/srep30898
Thuret, S., Moon, L. D., and Gage, F. H. (2006). Therapeutic interventions after spinal cord injury. Nat. Rev. Neurosci. 7, 628–643. doi: 10.1038/nrn1955
Tsuji, O., Sugai, K., Yamaguchi, R., Tashiro, S., Nagoshi, N., Kohyama, J., et al. (2019). Concise review: Laying the groundwork for a first-in-human study of an induced pluripotent stem cell-based intervention for spinal cord injury. Stem Cells 37, 6–13. doi: 10.1002/stem.2926
Turnbull, G., Clarke, J., Picard, F., Riches, P., Jia, L., Han, F., et al. (2018). 3D bioactive composite scaffolds for bone tissue engineering. Bioact. Mater. 3, 278–314. doi: 10.1016/j.bioactmat.2017.10.001
Vasanthan, L. T., Nehrujee, A., Solomon, J., and Tilak, M. (2019). Electrical stimulation for people with spinal cord injury. Cochrane Database Syst. Rev. 9:2113. doi: 10.1002/14651858.CD013481
White, T. E., Lane, M., Sandhu, M., O’Steen, B., Fuller, D., and Reier, P. (2010). Neuronal progenitor transplantation and respiratory outcomes following upper cervical spinal cord injury in adult rats. Exp. Neurol. 225, 231–236. doi: 10.1016/j.expneurol.2010.06.006
Wilcox, J. T., Satkunendrarajah, K., Zuccato, J., Nassiri, F., and Fehlings, M. (2014). Neural precursor cell transplantation enhances functional recovery and reduces astrogliosis in bilateral compressive/contusive cervical spinal cord injury. Stem Cells Transl. Med. 3, 1148–1159. doi: 10.5966/sctm.2014-0029
Xu, Y., Zhou, J., Liu, C., Zhang, S., Gao, F., Guo, W., et al. (2021). Understanding the role of tissue-specific decellularized spinal cord matrix hydrogel for neural stem/progenitor cell microenvironment reconstruction and spinal cord injury. Biomaterials 268:120596. doi: 10.1016/j.biomaterials.2020.120596
Yannas, I. V., Burke, J., Orgill, D., and Skrabut, E. (1982). Wound tissue can utilize a polymeric template to synthesize a functional extension of skin. Science 215, 174–176. doi: 10.1126/science.7031899
Yannas, I. V., Lee, E., Orgill, D., Skrabut, E., and Murphy, G. (1989). Synthesis and characterization of a model extracellular matrix that induces partial regeneration of adult mammalian skin. Proc. Natl. Acad. Sci. U.S.A. 86, 933–937. doi: 10.1073/pnas.86.3.933
Zheng, Y., Mao, Y., Yuan, T., Xu, D., and Cheng, L. (2020). Multimodal treatment for spinal cord injury: A sword of neuroregeneration upon neuromodulation. Neural Regen. Res. 15, 1437–1450. doi: 10.4103/1673-5374.274332
Keywords: spinal cord injury, neural stem/progenitor cells, combinatorial therapies, neuromodulation, biomaterials
Citation: Jagrit V, Koffler J and Dulin JN (2024) Combinatorial strategies for cell transplantation in traumatic spinal cord injury. Front. Neurosci. 18:1349446. doi: 10.3389/fnins.2024.1349446
Received: 04 December 2023; Accepted: 20 February 2024;
Published: 06 March 2024.
Edited by:
Biao Wang, Xi’an Honghui Hospital, ChinaReviewed by:
Norbert Weidner, Heidelberg University, GermanyCopyright © 2024 Jagrit, Koffler and Dulin. This is an open-access article distributed under the terms of the Creative Commons Attribution License (CC BY). The use, distribution or reproduction in other forums is permitted, provided the original author(s) and the copyright owner(s) are credited and that the original publication in this journal is cited, in accordance with accepted academic practice. No use, distribution or reproduction is permitted which does not comply with these terms.
*Correspondence: Jennifer N. Dulin, amR1bGluQGJpby50YW11LmVkdQ==