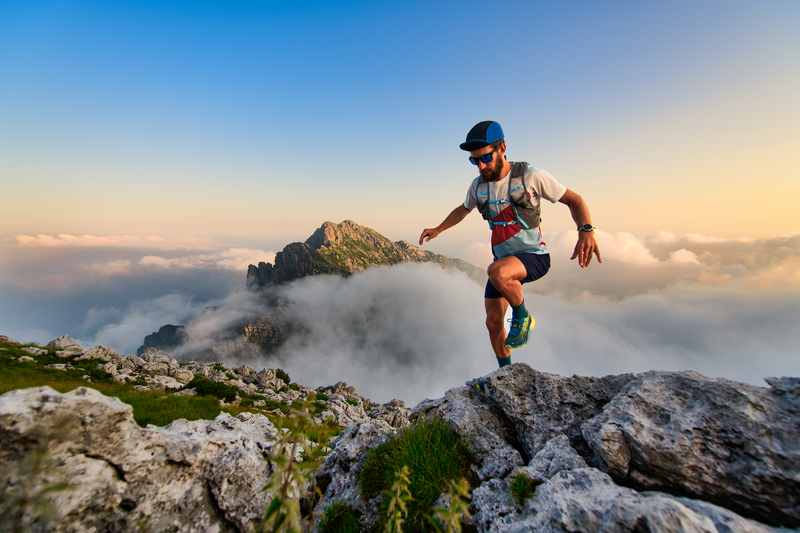
95% of researchers rate our articles as excellent or good
Learn more about the work of our research integrity team to safeguard the quality of each article we publish.
Find out more
SYSTEMATIC REVIEW article
Front. Neurosci. , 14 February 2024
Sec. Neural Technology
Volume 18 - 2024 | https://doi.org/10.3389/fnins.2024.1345128
This article is part of the Research Topic Innovative approaches to promote stroke recovery View all 18 articles
Introduction: Although rare, central post-stroke pain remains one of the most refractory forms of neuropathic pain. It has been reported that repetitive transcranial magnetic stimulation (rTMS) may be effective in these cases of pain.
Aim: The aim of this study was to investigate the efficacy of rTMS in patients with central post-stroke pain (CPSP).
Methods: We included randomized controlled trials or Controlled Trials published until October 3rd, 2022, which studied the effect of rTMS compared to placebo in CPSP. We included studies of adult patients (>18 years) with a clinical diagnosis of stroke, in which the intervention consisted of the application of rTMS to treat CSP.
Results: Nine studies were included in the qualitative analysis; 6 studies (4 RCT and 2 non-RCT), with 180 participants, were included in the quantitative analysis. A significant reduction in CPSP was found in favor of rTMS compared with sham, with a large effect size (SMD: −1.45; 95% CI: −1.87; −1.03; p < 0.001; I2: 58%).
Conclusion: The findings of the present systematic review with meta-analysis suggest that there is low quality evidence for the effectiveness of rTMS in reducing CPSP.
Systematic review registration: Identifier (CRD42022365655).
According to the International Association for the Study of Pain (IASP), neuropathic pain is any pain caused by a lesion or disease of the somatosensory system (Jensen et al., 2011; Treede et al., 2015). Central post-stroke pain (CPSP) is defined by the IASP as ‘pain initiated or caused by a primary lesion or dysfunction of the central nervous system (IASP, 2011) and occurs in the absence of other nociceptive, peripheral and psychogenic pain (Şahin-Onat et al., 2016).
A recent meta-analysis involving a total of 69 studies by Liampas et al. (2020) estimated that approximately 1 in 10 of all stroke patients will experience neuropathic pain. Other studies (Bowsher et al., 1993; Stitik et al., 2005) indicate that in the USA, the prevalence of CPSP reported in one study ranged from 2 to 8% in 250,000 people who suffered a cerebrovascular accident in the course of 1 year. Other authors widen the range even further, establishing a prevalence between 1 and 35% (Oh and Seo, 2015). This broad estimate is possibly due to variabilities in the definition of this pain category, the inclusion criteria, and the length of patients’ evaluation post-stroke (Kumar et al., 2009).
Once stroke patients overcome the acute phase of the event, they need early neurorehabilitation treatment to alleviate the consequences of the injury, such as spasticity or CSPS. Currently, there is no globally accepted and approved pharmacological therapy to accelerate the recovery of these patients (Figueroa et al., 2015). Therefore, new therapies, such as transcranial magnetic stimulation (TMS), have emerged. TMS consist of a high voltage and high intensity discharge system attached to a transducing coil. This system generates short lasting (<1 ms) magnetic fields of 1–2.5 Tesla, which penetrates the skull and induces secondary electric currents in the cerebral cortex that depolarizes neurons (Groppa et al., 2012). This phenomenon could be used as evaluation tool to assess corticospinal pathway integrity, applying a single pulse at cortical level and registering electric activity at the motor end-plate (Spampinato et al., 2023). Also, it could be used to evaluate intra-cortical excitability changes applying paired pulses with different time intervals (Wagle-Shukla et al., 2009).
For CPSP treatment one of the most used TMS techniques is repetitive transcranial magnetic stimulation (rTMS). rTMS is a noninvasive brain stimulation technique that generates brief, rapidly changing magnetic fields capable of inducing electric currents in the brain (Young et al., 2014). It is safe, well tolerated, and has a very favorable side effect profile, provided that safety recommendations are followed (Burke et al., 2019). Depending on stimulation parameters, rTMS can have an excitatory or inhibitory effect on the underlying neural networks (Young et al., 2014). At frequencies ≥5 Hz (high-frequency rTMS), rTMS has been shown to produce an increment in cortical excitability in healthy humans (Fitzgerald et al., 2006) and stroke patients (Belardinelli et al., 2021). This improvement in cortical excitability is the result of a modulation of the GABAergic and glutamatergic systems (Esser et al., 2006; Belardinelli et al., 2021) producing a long-term potentiation phenomenon in the stimulated neural networks (Esser et al., 2006). Moreover, ≤1 Hz frequencies (low-frequency rTMS) produce the opposite effect via long-term depression (Chen et al., 1997). These neuroplastic changes could induce reorganization of neural networks in the motor cortex, supplementary motor area, premotor area, cerebellum, thalamus and corpus callosum (Tosun et al., 2017; Guo et al., 2021; Wanni Arachchige et al., 2023). As well as reversal of functional connectivity changes (Grefkes et al., 2010; Guo et al., 2021; Juan et al., 2022) that occur after the stroke (Li et al., 2017; Vecchio et al., 2019).
According to the scientific literature, rTMS has numerous applications as analgesic tool in different neuropathic pain conditions. A long lasting analgesic effect has been reported when applying 5 sessions of high-frequency rTMS in CPSP or trigeminal neuralgia patients, compared to sham stimulation (Khedr et al., 2005). This reduction in pain intensity is also observed in other neuropathic pain conditions after receiving a rTMS treatment (Ahmed et al., 2011; Attal et al., 2021). In the only one systematic review conducted on the effect of non-invasive brain stimulation on CPSP, Ramger et al. (2019) concluded that noninvasive brain stimulation can have a therapeutic effect on the pain level of people with CPSP, as evidenced by significant decreases in clinical and experimental pain scores. Although no more systematic reviews or meta-analysis have been conducted on the analgesic effect of rTMS on CPSP, we can observe the same effect across other chronic neuropathic pain conditions (Gatzinsky et al., 2021).
To date no quantitative synthesis of the effect of rTMS on CPSP has been performed. Therefore, the aim of this study was to perform a meta-analysis of the randomized clinical trials (RCTs) or non-randomized clinical trials (CTs) that investigated the efficacy of rTMS in patients with CPSP.
Guidelines from the Preferred Reporting Items for Systematic Review and Meta-analysis (PRISMA) statement were consulted to develop this systematic review (Moher et al., 2010). The computerized databases Medline (Pubmed), SCOPUS, Cochrane Library, Embase, and Web of Science were used to search for relevant studies. Keywords referring to the intervention were used, combined with Boolean operators (complete search strategy is shown in Supplementary Appendix S1).
Searches were performed between September 3rd 2022, and October 3rd 2022, (from the date of inception of each database) using a combination of controlled vocabulary (i.e., medical subject headings) and free-text terms. Search strategies were modified to meet the specific requirements of each database. Searches of the reference lists of included studies and previously published systematic reviews were also conducted.
This meta-analysis was registered in the International Prospective Register of Systematic Reviews (PROSPERO registration no: CRD42022365655).
We used the Population, Intervention, Comparison, Outcomes, Time, and Study design (PICOTS) as a framework to formulate eligibility criteria (Lira and Rocha, 2019).
Individuals diagnosed with CPSP secondary to an ischemic or hemorrhagic stroke in the central nervous system.
Treatment must consist of the application of at least one session of rTMS in the motor cortex.
Comparison groups could be another type of intervention or non-intervention.
The measurement used to assess the outcomes and effects of the exercise was pain intensity. Measurements were to be recorded by objective methods, using validated and reliable scales or questionnaires (e.g., pain intensity by visual analog scale or numerical rating scale). Variables were to be assessed before and after the intervention.
No temporal restrictions were applied to the duration of the intervention or outcome measures.
Only RCTs or CTs were included.
At first, two blinded investigators (JLS-G and FG-A) examined the studies obtained from the databases by screening by title and abstract according to the established inclusion criteria. In the case of discrepancies, a third investigator (SV-R) intervened. After this first screening, the selected articles were read in full to see if they definitely met the criteria and could be included in the analysis. The authors of the included studies were contacted by e-mail, with the aim of accessing possible unclear data. If no response was received, the data were excluded from the analysis.
Two reviewers independently assessed the risk of bias in the studies (FGA and JLSG).
The risk of bias in non-randomized studies of interventions (NRSI) was assessed through the Risk of Bias In Non-randomized Studies of interventions (ROBINS-I) (Sterne et al., 2016). This tool focuses on assessing the risk of bias (RoB) in the results of NRSIs. The types of NRSIs that can be assessed with this tool are quantitative studies estimating the efficacy (harm or benefit) of an intervention, which did not use randomization to assign units (individuals or groups of individuals) to comparison groups. ROBINS-I takes into account 6 domains: Randomization process (D1), Bias arising from period and carryover effects (DS), Deviations from the intended interventions (D3), missing outcome data (D4), Selection of the reported result (D5).
On the other hand, a revised tool to assess the risk of bias in randomized clinical trials (RoB2) (Higgins et al., 2011) was used to assess the risk of bias in randomized trials. The tool is structured into five domains through which bias could be introduced into the outcome. These were identified based on empirical evidence and theoretical considerations. Because the domains cover all types of bias that may affect the results of randomized trials, each domain is mandatory, and no additional domains should be added. The five domains for individually randomized trials (including crossover trials) are: bias arising from the randomization process (D1); bias due to deviations from intended interventions (D2); bias due to missing outcome data (D3); bias in the measurement of the outcome (D4); bias in the selection of the reported result (D5).
In addition, methodological quality was evaluated using the PEDro list (de Morton, 2009), which assesses the internal and external validity of a study and consists of 11 criteria: (1) specified study eligibility criteria; (2) random allocation of subjects; (3) concealed allocation; (4) measure of similarity between groups at baseline; (5) subject blinding; (6) therapist blinding; (7) assessor blinding; (8) fewer than 15% dropouts; (9) intention-to-treat analysis; (10) between group statistical comparisons; and (11) point measures and variability data. The methodological criteria were scored as follows: yes (one point), no (zero points), or unknown (zero points). The PEDro score of each selected study provided an indicator of the methodological quality (9–10 = excellent; 6–8 = good; 4–5 = fair; 3–0 = poor) (Higgins et al., 2011).
The overall quality of evidence was based on the classification of the results into levels of evidence according to the Grading Of Recommendations Assessments, Development, and Evaluation (GRADE), which is based on 5 domains: (1) Study design; (2) Imprecision; (3) Indirectness; (4) Inconsistency; (5) Publication bias.
Evidence was categorized into the following 4 levels accordingly: (a) High quality: further research is very unlikely to change our confidence in the estimate of effect, all 5 domains are also met; (b) Moderate quality: further research is likely to have an important impact on our confidence and might change the estimate of effect, one of the 5 domains is not met; (c) Low quality: further research is very likely to have an important impact on our confidence and is likely to change the estimate of effect, two of the 5 domains are not met; and (d) Very low quality: any estimate of effect is very uncertain, 3 of the 5 domains are not met (Balshem et al., 2011; Andrews et al., 2013).
Meta-analysis was performed using ReviewManager statistical software (version 5.4; Cochrane, London, UK). Effects were investigated by calculating standardized mean differences (SMDs) for change scores from baseline to intervention. For this, the sample size, mean difference, and standard deviations (SDs) were extracted. When the study only reported median and first and third quartile values, they were converted to means and SDs (Luo et al., 2018).
When the authors presented only standard errors, these were converted to SDs. If the study did not present the results, the authors were contacted to request them. If results were not available in this way, means and SDs were estimated from graphs (Image J program; National Institute of Health in Bethesda, Maryland, United States). If none of this was possible, the study was excluded from the quantitative analysis and the information was presented narratively.
If the study did not report the preintervention postintervention mean difference in each group, the mean difference was obtained using the pre-postintervention values. In the absence of SD of the difference, we imputed from other data reported in the study: (1) using other measures reported in the study (e.g., confidence intervals and p values, following the principles described in Chapter 6.5.2.2 of the Cochrane Handbook) (Higgins et al., 2023); or, if that was not possible, (2) using the correlation coefficient of the most similar study included (following the principles described in Chapter 6.5.2.8 of the Cochrane Handbook) (Higgins et al., 2023); or if that was not possible, (3) using a conservative correlation coefficient of 0.5 (Deeks et al., 2022). This methodology has been performed in other meta-analyses (Gurdiel-Álvarez et al., 2023).
Meta-analysis was performed using the inverse variance method and a random effects model with 95% confidence intervals, as it provides more conservative results in case of heterogeneity between studies, which is expected. p values < 0.05 were considered statistically significant. An effect size (SMD) of 0.8 or greater was considered large, an effect size between 0.5 and 0.8 was considered moderate, and an effect size between 0.2 and 0.5 was considered small.
A sensitivity analysis was performed to evaluate the results. For this purpose, the meta-analysis was performed only with studies with low RoB, and then with the correlation coefficient of 0.5, instead of being estimated from the other studies. Sensitivity analysis was performed when the analysis could be performed in at least 5 studies. Study heterogeneity was assessed by the degree of between study inconsistency (I2). The Cochrane Group has established the following interpretation of the I2 statistic: 0–40% may not be relevant/important heterogeneity, 30–60% suggests moderate heterogeneity, 50–90% represents substantial heterogeneity, and 75–100% represents considerable heterogeneity (Balk et al., 2012). Skewness was assessed using funnel plots according to application method (cathodic, anodic), and stimulation site. These analyses were performed only if the subgroups had at least three studies.
Inter-rater reliability for screening, risk of bias assessment, and quality of the evidence rating were assessed using percentage agreement and Cohen’s kappa coefficient (Cohen, 1968; McHugh, 2012). There was strong agreement between reviewers for the screening records and full texts (98.51% agreement rate and k = 0.91), the risk of bias assessment (92.86% agreement rate and k = 0.83), and the quality and strength of the evidence assessment (97.73% rate and k = 0.95).
The search found 851 records, of which 384 were duplicates and 467 were screened by title and abstract. Twenty five studies were potentially relevant and full reports were obtained and screened. Seventeen studies were justifiably excluded. Nine studies met the eligibility criteria and were included for review (Figure 1).
Nine studies (180 participants; 78 women) were included for review (Table 1). Six were RCTs and three were CTs. The mean age of participants was 56.73 ± 9.78 years. Mean pain duration was 39.08 ± 23.42 months. Mean pain intensity was 66.57 ± 12.20 in a 0 to 100 scale.
Methodological quality scores ranged from 3 to 10 out of a maximum of 10 points. Three studies (33%) were of high methodological quality (greater than or equal to 6 points). Table 2 show the details of the PEDro scale.
Table 2. Methodological score of randomized clinical trials using the Physiotherapy Evidence Database (PEDro) scale.
We assessed the quality of the included studies using the RoB 2 tool for the RCTs and the ROBINS-E tool for the non-randomized clinical trials. We only judged 1 study to be at low risk of bias. The majority of the RCTs presented limitations in the randomization process or the report of the outcomes. While in the CTs, there was a risk of selection bias. Assessment of the risk of bias in the included studies is shown in Figures 2, 3.
Figure 2. Assessment of the risk of bias according to the revised Cochrane risk-of-bias tool for randomized trials (ROB-2).
Meta-analysis showed that significantly (p < 0.001), rTMS-based intervention produces a reduction in pain compared to sham based interventions with a large effect size (SMD: −1.45; 95% CI: −1.87; − 1.03; Z: 6.79; p < 0.001), and a moderate- substantial heterogeneity (I2: 58%; p = 0.001) (Figure 4). Sensitivity analysis by RoB could not be performed since only one study showed a low risk of bias. In the sensitivity analysis, a conservative correlation coefficient of 0.5 was applied, instead of being estimated from the other studies, the effect size was reduced from large to medium, but the significance remained in favor of the rTMS (SMD: −1.45; 95% CI: −1.87; −1.03; Z: 6.79; p < 0.001 to SMD: −1.81; 95% CI: −1.07; −0.54; Z: 6.06; p < 0.001). Heterogeneity was reduced from I2: 58%; p = 0.001 to I2 = 13%; p = 0.3 (Figure 5). The funnel plot presents asymmetry, indicating the risk of publication bias (Figure 6).
Figure 4. rTMS versus sham forest plot. Forest plot of the results of a random-effects meta-analysis shown as standardized mean differences (SMD) with 95% confidence interval (CI) for the effects of rTMS compared with sham in post-stroke central pain. The shaded square represents the point estimate for each individual study and the study weight in the meta-analysis. The diamond represents the overall mean difference of the studies.
Figure 5. Sensitivity analysis of rTMS versus sham forest plot. Forest plot of the results of a random-effects meta-analysis shown as standardized mean differences (SMD) with 95% confidence interval (CI) for the effects of rTMS compared with sham in post-stroke central pain. The shaded square represents the point estimate for each individual study and the study weight in the meta-analysis. The diamond rep-resents the overall mean difference of the studies.
Two non-randomized clinical trials did not mention adverse effects when reporting their results (Khedr et al., 2005; Matsumura et al., 2013). In one RCT, two patients reported transient, slight scalp discomfort after real rTMS (Kobayashi et al., 2015). Another RCT reported mild and transient adverse effects, such as headaches, tiredness, paresthesia, transient increase of pain, collapse, increased spasticity, or dizziness (Ojala et al., 2022). Lastly, other RCT reported short periods of numbness in the scalp or twitching of the fascial muscle during the stimulation in three participants (Zhao et al., 2021). The rest of the studies reported no adverse effects experienced during the duration of the intervention or follow-up (Lefaucheur et al., 2001a,b; André-Obadia et al., 2006; Hosomi et al., 2013).
Table 3 collects the details of the GRADE assessment. Three levels of evidence were downgraded due to the serious inconsistency of the results, publication bias, and overall risk of bias, which suggests a very small level of evidence regarding the effects of rTMS in patients with CPSP.
This systematic review included nine studies, of which six were RCTs, the largest cohort of clinical studies on the effects of rTMS on CPSP. This meta-analysis of data from six trials provides very small level of evidence of a large effect size on pain reduction when active rTMS is delivered on affected M1 in CPSP compared to a sham intervention. However, after carrying a sensitivity analysis, the effect size of the intervention is determined to be moderate with a low heterogeneity.
To date, this is the first meta-analysis evaluating the analgesic effect of rTMS on CPSP. Other studies have reviewed the antalgic effect of non-invasive physical modalities on CPSP, including rTMS. In other systematic review (Chen et al., 2016), a reduction of 10.8–32.6% in pain intensity was found in four non-randomized controlled trials (Hirayama et al., 2006; Goto et al., 2008; Ohn et al., 2012; Hosomi et al., 2013) and 1 case study (Lefaucheur et al., 2004) but no effect was seen in 2 RCTs (Lefaucheur et al., 2001b; de Oliveira et al., 2014). As a result, a Level B of evidence was given to rTMS as an analgesic tool for the treatment of CPSP (Chen et al., 2016). Another systematic review evaluated the effect of non-invasive brain stimulation on CPSP (Ramger et al., 2019). It found that of the 5 studies about rTMS (Ohn et al., 2012; Matsumura et al., 2013; de Oliveira et al., 2014; Hasan et al., 2014), 2 RCTs (Matsumura et al., 2013; Kobayashi et al., 2015) and 2 non-randomized clinical trials (Ohn et al., 2012; Hasan et al., 2014) reported a decrease in pain intensity after the treatment with high-frequency rTMS on the affected hemisphere (Ramger et al., 2019).
After the stroke, there is a remapping of the motor cortex that has been observe by functional magnetic resonance imaging (fMRI) or TMS (Cicinelli et al., 1997, 2003; Traversa et al., 1997). In animal stroke models, we can observe a significant reduction of the affected area in the motor cortex (Nudo, 2007). In addition, neuronal connections on the side contralateral to the lesion appear to be altered resulting in a lateralization of the neural activity (Bütefisch et al., 2008). In consequence, a decrease in short interval intracortical inhibition in both hemispheres, and an increase in intracortical facilitation in the non lesioned hemisphere can be observed (Liepert et al., 2000; Swayne et al., 2008). This results in an imbalance in the interhemispheric inhibition that could result in an obstacle for recovery (Vallone et al., 2016).
With regard to CPSP treatment, motor cortex stimulation has been researched since 1991 (Tsubokawa et al., 1991) as an invasive procedure to treat drug-resistant central pain. In motor cortex stimulation, higher frequencies are used, and the electrode is implanted in the affected hemisphere. High-frequency rTMS of the affected hemisphere tends to be the most common type of stimulation seen in CPSP trials, whereas low-frequency rTMS of the affected hemisphere is less common and tends to not have an effect (Lefaucheur et al., 2001a). Several mechanisms for high-frequency rTMS modulation of CPSP have been proposed (Pan et al., 2023; Radiansyah and Hadi, 2023). Electrical stimulation of the motor cortex increases blood flow to the lateral thalamus, the anterior cingulate cortex, the anterior insula, and the brainstem of CPSP patients (García-Larrea et al., 1999). Similar patterns of activity had been reported on fMRI after rTMS of M1 (Bestmann et al., 2004), implying common mechanisms of action. This analgesic effect of motor cortex stimulation in CPSP patients seems to be determined by the availability of opioid receptors in the anterior cingulate cortex, the insula, the thalamus, and the periaqueductal gray matter (Maarrawi et al., 2013). Meaning that rTMS of affected M1 in CPSP patients could modulate these structures of the medial system of pain (Xie et al., 2009), which has been shown to mediate the affective processing of the pain experience (Vogt and Sikes, 2000). Regarding this, animal CPSP models exhibit a reduction in the number of fibers in the thalamocortical pathway between the ventral posterolateral nucleus of the thalamus and the somatosensory cortices (Kadono et al., 2021) and increased functional connectivity between the medial thalamus and the amygdala (Mitchell and Chakraborty, 2013). The analgesic effect of rTMS in these models is associated with a reduction in the strength of the functional connectivity between medial thalamus and amygdala, normalizing during the rTMS treatment (Kadono et al., 2021).
Another proposed mechanism is the increase in excitability of the affected M1, that seems to be reduced in CPSP patients as a result of an asymmetric interhemispheric inhibition (Pan et al., 2023; Radiansyah and Hadi, 2023). The lesion of one M1 reduces its inhibitory activity in the contralateral M1. This results in an increase on the excitability of the contralateral M1 and a higher inhibitory output from the contralateral M1 to the injured M1 (Boddington and Reynolds, 2017). The application of high frequency rTMS to the injured M1 produce an increment on the excitability of the affected cortex, and an inhibition of the augmented excitability of the contralateral M1 (Bai et al., 2022). Finally, the activation of the descending inhibitory system is another mechanism that could explain the analgesic effect of non-invasive brain stimulation (DosSantos et al., 2018). However, in CPSP patients, heterotopic noxious conditioning stimulation, which activates the descending inhibitory system, has failed to reduce ongoing pain and dynamic mechanical allodynia (Tuveson et al., 2009).
Pharmacological treatment of the CPSP tend to use some drugs that could interact with the mechanism of action of TMS, and therefore alter its effects (Ziemann et al., 2015). Amitriptyline is used as first line treatment for CPSP (Ziemann et al., 2015), but its interaction with TMS is not known. It acts inhibiting voltage gated ion channels (Yan et al., 2010; Wu et al., 2012) and could act as agonist of TrkA and TrkB receptors, which mediate neural plasticity (Jang et al., 2009). Also, it seems to decrease GABAergic transmission (Bang et al., 2021). These mechanisms could potentially result in the increase of the facilitatory effect of the high frequency rTMS, and in the decrease of the inhibitory effect of the low frequency rTMS. Anticonvulsants like gabapentin or pregabalin, are other type of drugs that have been implemented in the management of CPSP (Hesami et al., 2015). Gabapentin and pregabalin have been shown to block voltage-gated ion channels, increase the synthesis and brain concentrations of GABA (Löscher et al., 1991) and reduce the synaptic release of glutamate (Taylor et al., 2007). These effects could produce an increase in the motor threshold measured by TMS (Menzler et al., 2014), a more sustained intracortical inhibition (Rizzo et al., 2001; Sommer et al., 2012) and a diminished intracortical facilitation (Rizzo et al., 2001). Accordingly, to this, stroke patients receiving anticonvulsant treatment could benefit less from high frequency rTMS treatment.
Considering the results of the present systematic review with meta-analysis, rTMS could be considered useful tool in the clinical context for management CPSP. Not only it has several possible mechanisms of action on the pathophysiological processes underlying CPSP as previously presented (Bestmann et al., 2004; Bai et al., 2022; Pan et al., 2023; Radiansyah and Hadi, 2023), but it is also a less invasive treatment that motor cortex stimulation (Tsubokawa et al., 1991). Also, high frequency rTMS protocols last only about 10 min and its adverse effects tend to be rare and mild in nature. Due to its suitability for the clinical practice, future studies should consider evaluating rTMS effectiveness compared to other treatments recommended for the management of patients with CPSP (e.g., adrenergic antidepressants or anticonvulsants) or its interaction with them.
Several limitations should be kept in mind when interpreting the results of the meta-analysis. Two of the studies included in the meta-analysis were not RCTs, so there exists some risk of selection bias. Regarding the duration of pain, some studies did not report it, while others ranged between acute (<3 months) to chronic presentation (>3 months). Mixing patients with acute and chronic CPSP in the study sample could account to an increased variability in the results, due to differences in the underlying pathophysiological processes. So future studies should consider these differences when stablishing their inclusion criteria. Also, the dosage of the rTMS varied between studies, with the frequency of stimulation ranging between 5 and 20 Hz, the intensity of stimulation ranging between 80 and 100% resting motor threshold, and the total number of sessions ranging between 1 and 18 sessions. Analyzing together studies with different rTMS protocols could in fact account to differences in the measured effects, accounting to increased heterogeneity in the results. Due to scarcity in studies applying same rTMS protocols in CPSP, future studies should take into account replicating the methodology of stimulation of previous studies to reduce this problem. Lastly, there seems to be a common risk of bias between the included studies regarding the randomization process or the clarity in the report of the outcomes. Researchers must consider reporting clearly the randomization processes to reduce possible biases and facilitate replicability, as well as expressing measures of centralization and dispersion to improve transparency and better understanding of the results.
The mains strengths of this study, is that this is the first systematic review with meta-analysis that has investigated the efficacy of rTMS on patients with CPSP. An analysis methodology has been applied in which pre-post mean differences were compared, which provides robustness to the results. The analysis has been developed based on the most recommended guidelines so that the study is replicable. The sensitivity analysis allowed to reduce the heterogeneity of the analyzed data sample, increasing the robustness of the results. Future studies should aim to improve the randomization and blinding processes to reduce the risk of bias, and define better the characteristics of the included subjects to provide homogeneous samples.
The findings of the current systematic review with meta-analysis suggest that there is low quality evidence for the effectiveness of rTMS in reducing CPSP intensity with a large effect size. Future studies should consider improving methodology by blinding the therapist and taking into account patients’ characteristics and rTMS parameters to reduce heterogeneity.
The original contributions presented in the study are included in the article/Supplementary material, further inquiries can be directed to the corresponding author.
FG-Á: Conceptualization, Methodology, Supervision, Writing – original draft. VN-L: Data curation, Formal analysis, Investigation, Software, Writing – original draft. SV-R: Investigation, Validation, Visualization, Writing – review & editing. RJ-V: Data curation, Project administration, Writing – review & editing. AC-R: Data curation, Formal analysis, Resources, Validation, Writing – review & editing. JS-G: Conceptualization, Investigation, Methodology, Supervision, Writing – original draft.
The author(s) declare that no financial support was received for the research, authorship, and/or publication of this article.
The authors declare that the research was conducted in the absence of any commercial or financial relationships that could be construed as a potential conflict of interest.
The reviewer ER-A declared a past co-authorship with the author RJ-V to the handling editor.
All claims expressed in this article are solely those of the authors and do not necessarily represent those of their affiliated organizations, or those of the publisher, the editors and the reviewers. Any product that may be evaluated in this article, or claim that may be made by its manufacturer, is not guaranteed or endorsed by the publisher.
The Supplementary material for this article can be found online at: https://www.frontiersin.org/articles/10.3389/fnins.2024.1345128/full#supplementary-material
Ahmed, M. A., Mohamed, S. A., and Sayed, D. (2011). Long-term antalgic effects of repetitive transcranial magnetic stimulation of motor cortex and serum beta-endorphin in patients with phantom pain. Neurol. Res. 33, 953–958. doi: 10.1179/1743132811Y.0000000045
André-Obadia, N., Peyron, R., Mertens, P., Mauguière, F., Laurent, B., and Garcia-Larrea, L. (2006). Transcranial magnetic stimulation for pain control. Double-blind study of different frequencies against placebo, and correlation with motor cortex stimulation efficacy. Clin. Neurophysiol. 117, 1536–1544. doi: 10.1016/j.clinph.2006.03.025
Andrews, J., Guyatt, G., Oxman, A. D., Alderson, P., Dahm, P., Falck-Ytter, Y., et al. (2013). GRADE guidelines: 14. Going from evidence to recommendations: the significance and presentation of recommendations. J. Clin. Epidemiol. 66, 719–725. doi: 10.1016/j.jclinepi.2012.03.013
Attal, N., Poindessous-Jazat, F., De Chauvigny, E., Quesada, C., Mhalla, A., Ayache, S. S., et al. (2021). Repetitive transcranial magnetic stimulation for neuropathic pain: a randomized multicentre sham-controlled trial. Brain 144, 3328–3339. doi: 10.1093/brain/awab208
Bai, Z., Zhang, J., and Fong, K. N. K. (2022). Effects of transcranial magnetic stimulation in modulating cortical excitability in patients with stroke: a systematic review and meta-analysis. J. Neuroeng. Rehabil. 19:24. doi: 10.1186/s12984-022-00999-4
Balk, E. M., Earley, A., Patel, K., Trikalinos, T. A., and Dahabreh, I. J. Empirical assessment of within-arm correlation imputation in trials of continuous outcomes. 12. 13 Rockville, MD: Agency for Healthcare Research and Quality (US); (2012).
Balshem, H., Helfand, M., Schünemann, H. J., Oxman, A. D., Kunz, R., Brozek, J., et al. (2011). GRADE guidelines: 3. Rating the quality of evidence. J. Clin. Epidemiol. 64, 401–406. doi: 10.1016/j.jclinepi.2010.07.015
Bang, E., Tobery, A., Montgomery, K. S., Fincher, A. S., Earnest, D. J., Murchison, D. A., et al. (2021). Amitriptyline decreases GABAergic transmission in basal forebrain neurons using an Optogenetic model of aging. Front. Aging Neurosci. 13:13. doi: 10.3389/fnagi.2021.673155
Belardinelli, P., König, F., Liang, C., Premoli, I., Desideri, D., Müller-Dahlhaus, F., et al. (2021). TMS-EEG signatures of glutamatergic neurotransmission in human cortex. Sci. Rep. 11:8159. doi: 10.1038/s41598-021-87533-z
Bestmann, S., Baudewig, J., Siebner, H. R., Rothwell, J. C., and Frahm, J. (2004). Functional MRI of the immediate impact of transcranial magnetic stimulation on cortical and subcortical motor circuits. Eur. J. Neurosci. 19, 1950–1962. doi: 10.1111/j.1460-9568.2004.03277.x
Boddington, L. J., and Reynolds, J. N. J. (2017). Targeting interhemispheric inhibition with neuromodulation to enhance stroke rehabilitation. Brain Stimul. 10, 214–222. doi: 10.1016/j.brs.2017.01.006
Bowsher, D., Vantrappen, G., Mabey, D., Treasure, T., Woods Kent, L., Treasure, T., et al. (1993). Cerebrovascular disease. Lancet 341:156. doi: 10.1016/0140-6736(93)90015-9
Burke, M. J., Fried, P. J., and Pascual-Leone, A. (2019). Transcranial magnetic stimulation: Neurophysiological and clinical applications. Handb. Clin. Neurol. 163, 73–92. doi: 10.1016/B978-0-12-804281-6.00005-7
Bütefisch, C. M., Weβling, M., Netz, J., Seitz, R. J., and Hömberg, V. (2008). Relationship between interhemispheric inhibition and motor cortex excitability in subacute stroke patients. Neurorehabil. Neural Repair 22, 4–21. doi: 10.1177/1545968307301769
Chen, C.-C., Chuang, Y.-F., Huang, A. C.-W., Chen, C.-K., and Chang, Y.-J. (2016). The antalgic effects of non-invasive physical modalities on central post-stroke pain: a systematic review. J. Phys. Ther. Sci. 28, 1368–1373. doi: 10.1589/jpts.28.1368
Chen, R., Classen, J., Gerloff, C., Celnik, P., Wassermann, E. M., Hallett, M., et al. (1997). Depression of motor cortex excitability by low-frequency transcranial magnetic stimulation. Neurology 48, 1398–1403. doi: 10.1212/WNL.48.5.1398
Cicinelli, P., Pasqualetti, P., Zaccagnini, M., Traversa, R., Oliveri, M., and Rossini, P. M. (2003). Interhemispheric asymmetries of motor cortex excitability in the Postacute stroke stage. Stroke 34, 2653–2658. doi: 10.1161/01.STR.0000092122.96722.72
Cicinelli, P., Traversa, R., and Rossini, P. M. (1997). Post-stroke reorganization of brain motor output to the hand: a 2–4 month follow-up with focal magnetic transcranial stimulation. Electroencephalography and clinical neurophysiology/electromyography and motor. Control 105, 438–450. doi: 10.1016/S0924-980X(97)00052-0
Cohen, J. (1968). Weighted kappa: nominal scale agreement provision for scaled disagreement or partial credit. Psychol. Bull. 70, 213–220. doi: 10.1037/h0026256
de Morton, N. A. (2009). The PEDro scale is a valid measure of the methodological quality of clinical trials: a demographic study. Aust. J. Physiother. 55, 129–133. doi: 10.1016/S0004-9514(09)70043-1
de Oliveira, R. A. A., de Andrade, D. C., Mendonça, M., Barros, R., Luvisoto, T., Myczkowski, M. L., et al. (2014). Repetitive transcranial magnetic stimulation of the left premotor/dorsolateral prefrontal cortex does not have analgesic effect on central Poststroke pain. J. Pain 15, 1271–1281. doi: 10.1016/j.jpain.2014.09.009
Deeks, J. J., Higgins, P. T., and Altman, D. G. Analysing data and undertaking meta-analyses. In: J. Higgins and J. Thomas, editors. Cochrane handbook for systematic reviews of interventions, 3; 6. Bristol; (2022).
DosSantos, M. F., Oliveira, A. T., Ferreira, N. R., Carvalho, A. C. P., and Rosado de Castro, P. H. (2018). The contribution of endogenous modulatory systems to TMS-and tDCS-induced analgesia: evidence from PET studies. Pain Res. Manag. 2018:2368386. doi: 10.1155/2018/2368386
Esser, S. K., Huber, R., Massimini, M., Peterson, M. J., Ferrarelli, F., and Tononi, G. (2006). A direct demonstration of cortical LTP in humans: a combined TMS/EEG study. Brain Res. Bull. 69, 86–94. doi: 10.1016/j.brainresbull.2005.11.003
Figueroa, J., Villamayor, B., and Antelo, A. (2015). Reeducación funcional tras un ictus. Spain: Elsevier.
Fitzgerald, P., Fountain, S., and Daskalakis, Z. (2006). A comprehensive review of the effects of rTMS on motor cortical excitability and inhibition. Clin. Neurophysiol. 117, 2584–2596. doi: 10.1016/j.clinph.2006.06.712
García-Larrea, L., Peyron, R., Mertens, P., Gregoire, C. M., Lavenne, F., Le Bars, D., et al. (1999). Electrical stimulation of motor cortex for pain control: a combined PET-scan and electrophysiological study. Pain 83, 259–273. doi: 10.1016/S0304-3959(99)00114-1
Gatzinsky, K., Bergh, C., Liljegren, A., Silander, H., Samuelsson, J., Svanberg, T., et al. (2021). Repetitive transcranial magnetic stimulation of the primary motor cortex in management of chronic neuropathic pain: a systematic review. Scand J Pain 21, 8–21. doi: 10.1515/sjpain-2020-0054
Goto, T., Saitoh, Y., Hashimoto, N., Hirata, M., Kishima, H., Oshino, S., et al. (2008). Diffusion tensor fiber tracking in patients with central post-stroke pain; correlation with efficacy of repetitive transcranial magnetic stimulation. Pain 140, 509–518. doi: 10.1016/j.pain.2008.10.009
Grefkes, C., Nowak, D. A., Wang, L. E., Dafotakis, M., Eickhoff, S. B., and Fink, G. R. (2010). Modulating cortical connectivity in stroke patients by rTMS assessed with fMRI and dynamic causal modeling. NeuroImage 50, 233–242. doi: 10.1016/j.neuroimage.2009.12.029
Groppa, S., Oliviero, A., Eisen, A., Quartarone, A., Cohen, L. G., Mall, V., et al. (2012). A practical guide to diagnostic transcranial magnetic stimulation: report of an IFCN committee. Clin. Neurophysiol. 123, 858–882. doi: 10.1016/j.clinph.2012.01.010
Guo, Z., Jin, Y., Bai, X., Jiang, B., He, L., McClure, M. A., et al. (2021). Distinction of high-and low-frequency repetitive transcranial magnetic stimulation on the functional reorganization of the motor network in stroke patients. Neural Plast. 2021, 1–11. doi: 10.1155/2021/8873221
Gurdiel-Álvarez, F., González-Zamorano, Y., Lerma-Lara, S., Gómez-Soriano, J., Sánchez-González, J. L., Fernández-Carnero, J., et al. (2023). Transcranial direct current stimulation (tDCS) effects on quantitative sensory testing (QST) and nociceptive processing in healthy subjects: a systematic review and Meta-analysis. Brain Sci. 14:9. doi: 10.3390/brainsci14010009
Hasan, M., Whiteley, J., Bresnahan, R., MacIver, K., Sacco, P., Das, K., et al. (2014). Somatosensory change and pain relief induced by repetitive transcranial magnetic stimulation in patients with central Poststroke pain. Neuromodulation: Technology at the Neural. Interface 17, 731–736. doi: 10.1111/ner.12198
Hesami, O., Gharagozli, K., Beladimoghadam, N., Assarzadegan, F., Mansouri, B., and Sistanizad, M. (2015). The efficacy of gabapentin in patients with central Post-stroke pain. Iran. J. Pharm. Res. 14, 95–101.
Higgins, J. P., Altman, D. G., Gøtzsche, P. C., Jüni, P., Moher, D., Oxman, A. D., et al. (2011). The Cochrane Collaboration’s tool for assessing risk of bias in randomised trialss. BMJ. 18,343: d5928. doi: 10.1136/bmj.d5928
Higgins, P. T., Li, T., and Deeks, J. J. (2023). “Chapter 6: Choosing effect measures and computing estimates of effect” in Cochrane Handbook for Systematic Reviews of Interventions
Hirayama, A., Saitoh, Y., Kishima, H., Shimokawa, T., Oshino, S., Hirata, M., et al. (2006). Reduction of intractable deafferentation pain by navigation-guided repetitive transcranial magnetic stimulation of the primary motor cortex. Pain 122, 22–27. doi: 10.1016/j.pain.2005.12.001
Hosomi, K., Kishima, H., Oshino, S., Hirata, M., Tani, N., Maruo, T., et al. (2013). Cortical excitability changes after high-frequency repetitive transcranial magnetic stimulation for central poststroke pain. Pain 154, 1352–1357. doi: 10.1016/j.pain.2013.04.017
Hosomi, K., Shimokawa, T., Ikoma, K., Nakamura, Y., Sugiyama, K., Ugawa, Y., et al. (2013). Daily repetitive transcranial magnetic stimulation of primary motor cortex for neuropathic pain: a randomized, multicenter, double-blind, crossover, sham-controlled trial. Pain 154, 1065–1072. doi: 10.1016/j.pain.2013.03.016
IASP (2011). “Classification of chronic pain” in Descriptions of Chronic Pain Syndromes and Definitions of Pain Terms. 2 (Revised) ed
Jang, S.-W., Liu, X., Chan, C.-B., Weinshenker, D., Hall, R. A., Xiao, G., et al. (2009). Amitriptyline is a TrkA and TrkB receptor agonist that promotes TrkA/TrkB Heterodimerization and has potent neurotrophic activity. Chem. Biol. 16, 644–656. doi: 10.1016/j.chembiol.2009.05.010
Jensen, T. S., Baron, R., Haanpää, M., Kalso, E., Loeser, J. D., Rice, A. S. C., et al. (2011). A new definition of neuropathic pain. Pain 152, 2204–2205. doi: 10.1016/j.pain.2011.06.017
Juan, D., Yao, W., Li, J., Yang, F., Hu, J., Xu, Q., et al. (2022). Motor network reorganization after repetitive transcranial magnetic stimulation in early stroke patients: a resting state fMRI study. Neurorehabil. Neural Repair 36, 61–68. doi: 10.1177/15459683211054184
Kadono, Y., Koguchi, K., Okada, K., Hosomi, K., Hiraishi, M., Ueguchi, T., et al. (2021). Repetitive transcranial magnetic stimulation restores altered functional connectivity of central poststroke pain model monkeys. Sci. Rep. 11:6126. doi: 10.1038/s41598-021-85409-w
Khedr, E. M., Kotb, H., Kamel, N. F., Ahmed, M. A., Sadek, R., and Rothwell, J. C. (2005). Longlasting antalgic effects of daily sessions of repetitive transcranial magnetic stimulation in central and peripheral neuropathic pain. J. Neurol. Neurosurg. Psychiatry 76, 833–838. doi: 10.1136/jnnp.2004.055806
Kobayashi, M., Fujimaki, T., Mihara, B., and Ohira, T. (2015). Repetitive transcranial magnetic stimulation once a week induces sustainable long-term relief of central Poststroke pain. Neuromodulation 18, 249–254. doi: 10.1111/ner.12301
Kumar, B., Kalita, J., Kumar, G., and Misra, U. K. (2009). Central Poststroke pain: a review of pathophysiology and treatment. Anesth. Analg. 108, 1645–1657. doi: 10.1213/ane.0b013e31819d644c
Lefaucheur, J. P., Drouot, X., Keravel, Y., and Nguyen, J.-P. (2001a). Pain relief induced by repetitive transcranial magnetic stimulation of precentral cortex. Neuroreport 12, 2963–2965. doi: 10.1097/00001756-200109170-00041
Lefaucheur, J.-P., Drouot, X., Ménard-Lefaucheur, I., and Nguyen, J. P. (2004). Neuropathic pain controlled for more than a year by monthly sessions of repetitive transcranial magnetic stimulation of the motor cortex. Clin. Neurophysiol. 34, 91–95. doi: 10.1016/j.neucli.2004.02.001
Lefaucheur, J. P., Drouot, X., and Nguyen, J. P. (2001b). Interventional neurophysiology for pain control: Duration of pain relief following repetitive transcranial magnetic stimulation of the motor cortex. Neurophysiol. Clin. 31, 247–252. doi: 10.1016/S0987-7053(01)00260-X
Li, Y., Wang, Y., Liao, C., Huang, W., and Wu, P. (2017). Longitudinal brain functional connectivity changes of the cortical motor-related network in subcortical stroke patients with acupuncture treatment. Neural Plast. 2017, 1–9. doi: 10.1155/2017/5816263
Liampas, A., Velidakis, N., Georgiou, T., Vadalouca, A., Varrassi, G., Hadjigeorgiou, G. M., et al. (2020). Prevalence and management challenges in central Post-stroke neuropathic pain: a systematic review and Meta-analysis. Adv. Ther. 37, 3278–3291. doi: 10.1007/s12325-020-01388-w
Liepert, J., Hamzei, F., and Weiller, C. (2000). Motor cortex disinhibition of the unaffected hemisphere after acute stroke. Muscle Nerve 23, 1761–1763. doi: 10.1002/1097-4598(200011)23:11<1761::AID-MUS14>3.0.CO;2-M
Lira, R. P. C., and Rocha, E. M. (2019). PICOT: imprescriptible items in a clinical research question. Arq. Bras. Oftalmol. 82:1. doi: 10.5935/0004-2749.20190028
Löscher, W., Hönack, D., and Taylor, C. P. (1991). Gabapentin increases aminooxyacetic acid-induced GABA accumulation in several regions of rat brain. Neurosci. Lett. 128, 150–154. doi: 10.1016/0304-3940(91)90249-S
Luo, D., Wan, X., Liu, J., and Tong, T. (2018). Optimally estimating the sample mean from the sample size, median, mid-range, and/or mid-quartile range. Stat. Methods Med. Res. 27, 1785–1805. doi: 10.1177/0962280216669183
Maarrawi, J., Peyron, R., Mertens, P., Costes, N., Magnin, M., Sindou, M., et al. (2013). Brain opioid receptor density predicts motor cortex stimulation efficacy for chronic pain. Pain 154, 2563–2568. doi: 10.1016/j.pain.2013.07.042
Matsumura, Y., Hirayama, T., and Yamamoto, T. (2013). Comparison between pharmacologic evaluation and repetitive transcranial magnetic stimulation-induced analgesia in poststroke pain patients. Neuromodulation 16, 349–354. doi: 10.1111/ner.12019
McHugh, M. L. (2012). Interrater reliability: the kappa statistic. Biochem. Med. (Zagreb) 22, 276–282. doi: 10.11613/BM.2012.031
Menzler, K., Hermsen, A., Balkenhol, K., Duddek, C., Bugiel, H., Bauer, S., et al. (2014). A common splice-site polymorphism modifies the effect of carbamazepine on cortical excitability—a pharmacogenetic transcranial magnetic stimulation study. Epilepsia 55, 362–369. doi: 10.1111/epi.12515
Mitchell, A. S., and Chakraborty, S. (2013). What does the mediodorsal thalamus do? Front. Syst. Neurosci. 7:37. doi: 10.3389/fnsys.2013.00037
Moher, D., Hopewell, S., Schulz, K. F., Montori, V., Gotzsche, P. C., Devereaux, P. J., et al. (2010). CONSORT 2010 explanation and elaboration: updated guidelines for reporting parallel group randomised trials. BMJ 340:c869. doi: 10.1136/bmj.c869
Nudo, R. J. (2007). Postinfarct cortical plasticity and behavioral recovery. Stroke 38, 840–845. doi: 10.1161/01.STR.0000247943.12887.d2
Oh, H., and Seo, W. (2015). A comprehensive review of central Post-stroke pain. Pain Manag. Nurs. 16, 804–818. doi: 10.1016/j.pmn.2015.03.002
Ohn, S. H., Chang, W. H., Park, C., Kim, S. T., Il, L. J., Pascual-Leone, A., et al. (2012). Neural correlates of the Antinociceptive effects of repetitive transcranial magnetic stimulation on central pain after stroke. Neurorehabil. Neural Repair 26, 344–352. doi: 10.1177/1545968311423110
Ojala, J., Vanhanen, J., Harno, H., Lioumis, P., Vaalto, S., Kaunisto, M. A., et al. (2022). A randomized, sham-controlled trial of repetitive transcranial magnetic stimulation targeting M1 and S2 in central Poststroke pain: a pilot trial. Neuromodulation 25, 538–548. doi: 10.1111/ner.13496
Pan, L.-J., Zhu, H.-Q., Zhang, X.-A., and Wang, X.-Q. (2023). The mechanism and effect of repetitive transcranial magnetic stimulation for post-stroke pain. Front. Mol. Neurosci. 15:15. doi: 10.3389/fnmol.2022.1091402
Radiansyah, R. S., and Hadi, D. W. (2023). Repetitive transcranial magnetic stimulation in central post-stroke pain: current status and future perspective. Korean J. Pain 36, 408–424. doi: 10.3344/kjp.23220
Ramger, B. C., Bader, K. A., Davies, S. P., Stewart, D. A., Ledbetter, L. S., Simon, C. B., et al. (2019). Effects of non-invasive brain stimulation on clinical pain intensity and experimental pain sensitivity among individuals with central Post-stroke pain: a systematic review. J. Pain Res. 12, 3319–3329. doi: 10.2147/JPR.S216081
Rizzo, V., Quartarone, A., Bagnato, S., Battaglia, F., Majorana, G., and Girlanda, P. (2001). Modification of cortical excitability induced by gabapentin: a study by transcranial magnetic stimulation. Neurol. Sci. 22, 229–232. doi: 10.1007/s100720100002
Şahin-Onat, Ş., Ünsal-Delialioğlu, S., Kulaklı, F., and Özel, S. (2016). The effects of central post-stroke pain on quality of life and depression in patients with stroke. J. Phys. Ther. Sci. 28, 96–101. doi: 10.1589/jpts.28.96
Sommer, M., Gileles, E., Knappmeyer, K., Rothkegel, H., Polania, R., and Paulus, W. (2012). Carbamazepine reduces short-interval interhemispheric inhibition in healthy humans. Clin. Neurophysiol. 123, 351–357. doi: 10.1016/j.clinph.2011.07.027
Spampinato, D. A., Ibanez, J., Rocchi, L., and Rothwell, J. (2023). Motor potentials evoked by transcranial magnetic stimulation: interpreting a simple measure of a complex system. J. Physiol. 601, 2827–2851. doi: 10.1113/JP281885
Sterne, J. A., Hernán, M. A., Reeves, B. C., Savović, J., Berkman, N. D., Viswanathan, M., et al. (2016). ROBINS-I: a tool for assessing risk of bias in non-randomised studies of interventions. BMJ 355:i4919. doi: 10.1136/bmj.i4919
Stitik, T., Klecz, R., and Grenwald, B. Pharmacotherapy of disability. In: J. LisaDe, editor. Physical medicine and rehabilitation. 4, Philadelphia: Lippincott Williams Wilkins; (2005), p. 1207–1247.
Swayne, O. B. C., Rothwell, J. C., Ward, N. S., and Greenwood, R. J. (2008). Stages of motor output reorganization after hemispheric stroke suggested by longitudinal studies of cortical physiology. Cereb. Cortex 18, 1909–1922. doi: 10.1093/cercor/bhm218
Taylor, C. P., Angelotti, T., and Fauman, E. (2007). Pharmacology and mechanism of action of pregabalin: the calcium channel α2–δ (alpha2–delta) subunit as a target for antiepileptic drug discovery. Epilepsy Res. 73, 137–150. doi: 10.1016/j.eplepsyres.2006.09.008
Tosun, A., Türe, S., Askin, A., Yardimci, E. U., Demirdal, S. U., Kurt Incesu, T., et al. (2017). Effects of low-frequency repetitive transcranial magnetic stimulation and neuromuscular electrical stimulation on upper extremity motor recovery in the early period after stroke: a preliminary study. Top. Stroke Rehabil. 24, 361–367. doi: 10.1080/10749357.2017.1305644
Traversa, R., Cicinelli, P., Bassi, A., Rossini, P. M., and Bernardi, G. (1997). Mapping of motor cortical reorganization after stroke. Stroke 28, 110–117. doi: 10.1161/01.STR.28.1.110
Treede, R.-D., Rief, W., Barke, A., Aziz, Q., Bennett, M. I., Benoliel, R., et al. (2015). A classification of chronic pain for ICD-11. Pain 156, 1003–1007. doi: 10.1097/j.pain.0000000000000160
Tsubokawa, T., Katayama, Y., Yamamoto, T., Hirayama, T., and Koyama, S. (1991). Chronic motor cortex stimulation for the treatment of central. Pain 52, 137–139. doi: 10.1007/978-3-7091-9160-6_37
Tuveson, B., Leffler, A.-S., and Hansson, P. (2009). Influence of heterotopic noxious conditioning stimulation on spontaneous pain and dynamic mechanical allodynia in central post-stroke pain patients. Pain 143, 84–91. doi: 10.1016/j.pain.2009.02.002
Vallone, F., Lai, S., Spalletti, C., Panarese, A., Alia, C., Micera, S., et al. (2016). Post-stroke longitudinal alterations of inter-hemispheric correlation and hemispheric dominance in mouse pre-motor cortex. PLoS One 11:e0146858. doi: 10.1371/journal.pone.0146858
Vecchio, F., Tomino, C., Miraglia, F., Iodice, F., Erra, C., Di Iorio, R., et al. (2019). Cortical connectivity from EEG data in acute stroke: a study via graph theory as a potential biomarker for functional recovery. Int. J. Psychophysiol. 146, 133–138. doi: 10.1016/j.ijpsycho.2019.09.012
Vogt, B. A., and Sikes, R. W. (2000). The medial pain system, cingulate cortex, and parallel processing of nociceptive information. Prog. Brain Res. 122, 223–235. doi: 10.1016/S0079-6123(08)62141-X
Wagle-Shukla, A., Ni, Z., Gunraj, C. A., Bahl, N., and Chen, R. (2009). Effects of short interval intracortical inhibition and intracortical facilitation on short interval intracortical facilitation in human primary motor cortex. J. Physiol. 587, 5665–5678. doi: 10.1113/jphysiol.2009.181446
Wanni Arachchige, P. R., Ryo, U., Karunarathna, S., and Senoo, A. (2023). Evaluation of fMRI activation in hemiparetic stroke patients after rehabilitation with low-frequency repetitive transcranial magnetic stimulation and intensive occupational therapy. Int. J. Neurosci. 133, 705–713. doi: 10.1080/00207454.2021.1968858
Wu, W., Ye, Q., Wang, W., Yan, L., Wang, Q., Xiao, H., et al. (2012). Amitriptyline modulates calcium currents and intracellular calcium concentration in mouse trigeminal ganglion neurons. Neurosci. Lett. 506, 307–311. doi: 10.1016/j.neulet.2011.11.031
Xie, Y., Huo, F., and Tang, J. (2009). Cerebral cortex modulation of pain. Acta Pharmacol. Sin. 30, 31–41. doi: 10.1038/aps.2008.14
Yan, L., Wang, Q., Fu, Q., Ye, Q., Xiao, H., and Wan, Q. (2010). Amitriptyline inhibits currents and decreases the mRNA expression of voltage-gated sodium channels in cultured rat cortical neurons. Brain Res. 1336, 1–9. doi: 10.1016/j.brainres.2010.04.016
Young, N. A., Sharma, M., and Deogaonkar, M. (2014). Transcranial magnetic stimulation for chronic pain. Neurosurg. Clin. N. Am. 25, 819–832. doi: 10.1016/j.nec.2014.07.007
Zhao, C. G., Sun, W., Ju, F., Jiang, S., Wang, H., Sun, X. L., et al. (2021). Analgesic effects of navigated repetitive transcranial magnetic stimulation in patients with acute central Poststroke pain. Pain Ther. 10, 1085–1100. doi: 10.1007/s40122-021-00261-0
Keywords: meta-analysis, stroke, transcranial magnetic stimulation, pain management, pain
Citation: Gurdiel-Álvarez F, Navarro-López V, Varela-Rodríguez S, Juárez-Vela R, Cobos-Rincón A and Sánchez-González JL (2024) Transcranial magnetic stimulation therapy for central post-stroke pain: systematic review and meta-analysis. Front. Neurosci. 18:1345128. doi: 10.3389/fnins.2024.1345128
Received: 27 November 2023; Accepted: 01 February 2024;
Published: 14 February 2024.
Edited by:
Xingang Zhao, Chinese Academy of Sciences (CAS), ChinaReviewed by:
Raúl Ferrer-Peña, CSEU La Salle, SpainCopyright © 2024 Gurdiel-Álvarez, Navarro-López, Varela-Rodríguez, Juárez-Vela, Cobos-Rincón and Sánchez-González. This is an open-access article distributed under the terms of the Creative Commons Attribution License (CC BY). The use, distribution or reproduction in other forums is permitted, provided the original author(s) and the copyright owner(s) are credited and that the original publication in this journal is cited, in accordance with accepted academic practice. No use, distribution or reproduction is permitted which does not comply with these terms.
*Correspondence: Ana Cobos-Rincón, YW5hLmNvYm9zQHVuaXJpb2phLmVz
Disclaimer: All claims expressed in this article are solely those of the authors and do not necessarily represent those of their affiliated organizations, or those of the publisher, the editors and the reviewers. Any product that may be evaluated in this article or claim that may be made by its manufacturer is not guaranteed or endorsed by the publisher.
Research integrity at Frontiers
Learn more about the work of our research integrity team to safeguard the quality of each article we publish.