- 1Department of Otorhinolaryngology, Seoul National University College of Medicine, Seoul, Republic of Korea
- 2Department of Otorhinolaryngology, Boramae Medical Center, Seoul Metropolitan Government-Seoul National University, Seoul, Republic of Korea
Introduction: Several studies have reported a significant correlation between noise-induced hearing loss and cognitive decline. However, comprehensive analyses of this relationship are rare. This study aimed to assess the influence of hearing impairment on cognitive functions by analyzing organ samples in the afferent auditory pathway of deafened mice using mRNA sequencing.
Methods: We prepared 10 female 12-week-old C57BL/6N mice as the experimental and control groups in equal numbers. Mice in the experimental group were deafened with 120 dB sound pressure level (SPL) wideband noise for 2 h. Cochlea, auditory cortex, and hippocampus were obtained from all mice. After constructing cDNA libraries for the extracted RNA from the samples, we performed next-generation sequencing. Subsequently, we analyzed the results using gene ontologies (GOs) and Kyoto Encyclopedia of Genes and Genomes (KEGG) pathway databases for differentially expressed genes (DEGs) of each organ.
Results: Our results revealed 102, 89, and 176 DEGs for cochlea, auditory cortex, and hippocampus, respectively. We identified 294, 203, and 211 GOs; 10, 7, and 17 KEGG pathways in the cochlea, auditory cortex, and hippocampus, respectively. In the long term (12 weeks) from noise-induced hearing loss, GOs and KEGG pathways related to apoptosis or inflammation persisted more actively in the order of hippocampus, auditory cortex, and cochlea.
Discussion: This implies that the neurodegenerative effects of noise exposure persist more longer time in the central regions.
Introduction
Dementia is one of the biggest global healthcare problems, affecting 55 million people worldwide (Gauthier et al., 2022). Since there is no effective disease-modifying treatment option for dementia progression (Tisher and Salardini, 2019), the prevention of early-stage cognitive decline seems more important. Notably, it has been reported that hearing loss is associated with dementia (Lin et al., 2011; Gurgel et al., 2014). As relevant studies progressed, hearing loss has been accepted as a major modifiable risk factor for dementia (Livingston et al., 2017). It is thought that hearing loss can lead to cognitive decline, which may then serve as a “second hit,” exacerbating dementia in the presence of organic brain pathology (Lin and Albert, 2014).
Several independent studies have suggested underlying mechanisms by which hearing impairment leads to cognitive decline. Some studies suggest that the increased cognitive demands resulting from hearing loss can evoke cognitive decline (Lin and Albert, 2014; Cardin, 2016). Other researchers suggest that the oxidative stress triggered by hearing loss may adversely affect the hippocampus by increasing neuroinflammation, accelerating apoptosis, and reducing neurogenesis (Gonzalez-Perez et al., 2011; Paciello et al., 2023). Abnormalities in the neurotransmitter system, such as the N-methyl-d-aspartic acid receptor 2B, have been reported to be related to cognitive decline (Cui et al., 2013). Disorders of Schaffer collateral-1 long-term potentiation through depressed levels of brain-derived neurotrophic factors due to high-intensity sound are known to cause cognitive decline (de Deus et al., 2021). Additionally, it has been proposed that stress hormones and corticosteroids produced in response to hearing loss-induced stress can impact neuronal function in the hippocampus, ultimately leading to cognitive decline (Jin et al., 2017; Kurioka et al., 2021).
These studies provide an overview of how hearing impairment affects cognitive function. However, it is difficult to draw a comprehensive explanation because each study reveals only discrete aspects of the phenomenon from the researcher's viewpoint. In this case, omics studies can provide a broader understanding. An omics study quantitatively analyzes the whole set of specific biomolecules, such as the genome, transcriptome, proteome, or metabolome, in a given time (Vailati-Riboni et al., 2017; Subedi et al., 2022). This enables us to obtain a balanced insight into complex genetic mechanisms. Gene expression patterns can be primarily understood by mRNA sequencing.
Recent studies using omics technologies have uncovered the hidden mechanisms of hearing loss progresses at the molecular level. A previous study showed that noise exposure immediately evokes global cochlear protein ubiquitylation and upregulates ribosomal proteins in the cochlea (Jongkamonwiwat et al., 2020). Another study found that acoustic trauma modulates the expression of inflammation- and immunity-related genes (Maeda et al., 2021; Miao et al., 2021). However, few omics studies have investigated the relationship between hearing loss and cognitive decline. A transcriptome-wide association study has shown that age-related hearing loss impairs the glutamatergic synapse pathway in the hippocampus of BXD-recombinant inbred mice (Deng et al., 2021). Except this, we could not find out any omics study on the relationship between hearing loss and cognitive decline.
From this perspective, the current study was designed to evaluate the impact of auditory impairment on cognitive functioning. This was achieved by analyzing tissue samples from key components of the afferent auditory pathway—specifically the cochlea, the auditory cortex, and the hippocampus—through mRNA sequencing techniques. The auditory cortex was selected for its critical role in the processing and interpretation of auditory signals. The hippocampus was included due to its integral function in spatial and episodic memory; noteworthily, hippocampal impairment has been identified as a hallmark feature in a range of cognitive disorders, including dementia (Nadhimi and Llano, 2021; Billig et al., 2022). Furthermore, we used gene ontologies (GOs) and the Kyoto Encyclopedia of Genes and Genomes (KEGG) pathway databases to examine the types of events that occur after noise-induced hearing loss.
Materials and methods
Animal management
Ten female C57BL/6N mice were used. To prevent sex act as a confounding factor, we used only female mice. Mice were placed in cage with freely access to distilled water and chow under pathogen free condition (12 h light/dark cycle, 23°C, 50% humidity). They were divided into experimental (n = 5) and control (n = 5) groups. Mice in the experimental group were deafened with a single exposure of 120 dB sound pressure level (SPL) wideband noise for 2 h in an audiometric booth at the age of 12 weeks. To confirm the audiometric state, we checked the hearing thresholds of both ears just before noise exposure, 2 weeks post-exposure, and at the age of 24 weeks just before sacrifice.
All experimental procedures were approved by the Institutional Animal Care and Use Committee (IACUC) of Boramae Hospital (IACUC number 2022-0133).
Tissue preparation
All the mice were sacrificed at 24 weeks of age. They were fully anesthetized by isoflurane inhalation in an airtight cage. Cardiac perfusion was performed using phosphate-buffered saline (PBS) to remove red blood cells from the tissues. After decapitation, we harvested the bilateral cochleae, auditory cortical areas, and hippocampus of each mouse by referencing an anatomy atlas (Franklin and Paxinos, 2013). All right and left specimens were combined into one sample for each mouse organ.
mRNA sequencing
The samples were lysed using the RNeasy Mini Kit (Qiagen, Germany) following the manufacturer's instructions for total RNA extraction. Total RNA quantity and quality were determined by UV/Vis spectrophotometer (NanoDrop 2000, ThermoFisher Scientific, USA). All RNA samples showed suitable A260/A280 and 28s/18s ratios. A cDNA library was subsequently constructed, and next-generation sequencing was performed. The entire NGS procedure was performed using an Illumina NovaSeq 6000 system (Macrogen, South Korea).
Statistical analysis on expressed genes
To reduce bias in the results, we conducted quality control analysis of the raw sequencing reads. This involved removing low-quality data, adaptor sequences, contaminant DNA, PCR duplicates, and other artifacts. After confirming all samples satisfied quality control criteria, we selected three sets out of five sets in analyzing samples of cochlea, auditory cortex, and hippocampus for experimental and control groups.
The preprocessed reads were then mapped to the reference genome using HISAT2 version 2.1.0 (https://ccb.jhu.edu/software/hisat2/index.shtml/) to generate aligned reads. Subsequently, transcript assembly was performed using StringTie version 2.1.3b (https://ccb.jhu.edu/software/stringtie/). Expression profiles were extracted through transcript quantification for each sample, and fragments per kilobase of transcript per million mapped reads (FPKM), reads per kilobase of transcript per million mapped reads (RPKM), and transcripts per kilobase million (TPM) values were calculated. Differentially expressed genes (DEGs) were selected using this process, and functional annotation and gene-set enrichment analyses were performed using the GOs and KEGG databases. We set |fold change| of ≥2.0 and p-value of < 0.05 as statistically significant. For the selection of differentially expressed genes (DEGs), we utilized the raw p-values obtained from the direct gene comparisons between control and experimental groups. In contrast, for the identification of GOs and KEGG pathways, we employed adjusted p-values derived from hypergeometric testing and multiple testing corrections to control the false discovery rate. The sample size was determined to ensure sufficient quantity, taking into account cell type, tissue specificity, and library preparation methods. Since all samples met the quality control standards, we required only three samples per group.
We used GOnet (https://tools.dice-database.org/GOnet/) and REVIGO (http://revigo.irb.hr/) to visualize the relationships among genes and their ontologies. In the GOnet, we performed “GO term annotation” analysis using “generic GO slim” subset. In the REVIGO, we set resulting list size as “medium,” and chose “SimRel” semantic similarity measure.
Results
Audiometric tests
Prior to noise exposure, all the mice exhibited normal hearing thresholds in both ears during the auditory brainstem response (ABR) tests using click, 8 and 16 kHz tone-burst sound. Two weeks after noise exposure, it was found that none of the mice in the experimental group had an ABR response to sound stimulations of 90 dB SPL in either ear. In contrast, all the mice in the control group maintained normal thresholds in both ears. The hearing status of all mice was assessed prior to sacrifice, and it was confirmed that there had been no changes since the last hearing test.
Data quality control
We excluded genes that were not detected in at least one of the 18 samples. Of the 45,777 genes detected, 27,122 were not detected in at least one sample and were excluded. Thus, 18,655 genes were analyzed in this study.
To reduce systemic bias in sample comparisons, we performed relative log-expression normalization prior to statistical analysis. The necessary size factor was estimated using the read count data. Additionally, we performed multidimensional scaling and hierarchical clustering analyses to check for the presence of outlier samples and similarity in expression patterns among biological replicates and confirmed that there were no discrepancies.
Differentially expressed genes
To compare the experimental and control groups, we used the up-regulated and down-regulated genes that were differentially expressed in each organ. In the cochlea, there were 57 up-regulated and 45 down-regulated DEGs, while in the auditory cortex, there were 62 up-regulated and 27 down-regulated DEGs. In the hippocampus, 141 DEGS were up-regulated, and 35 were down-regulated (Figure 1).
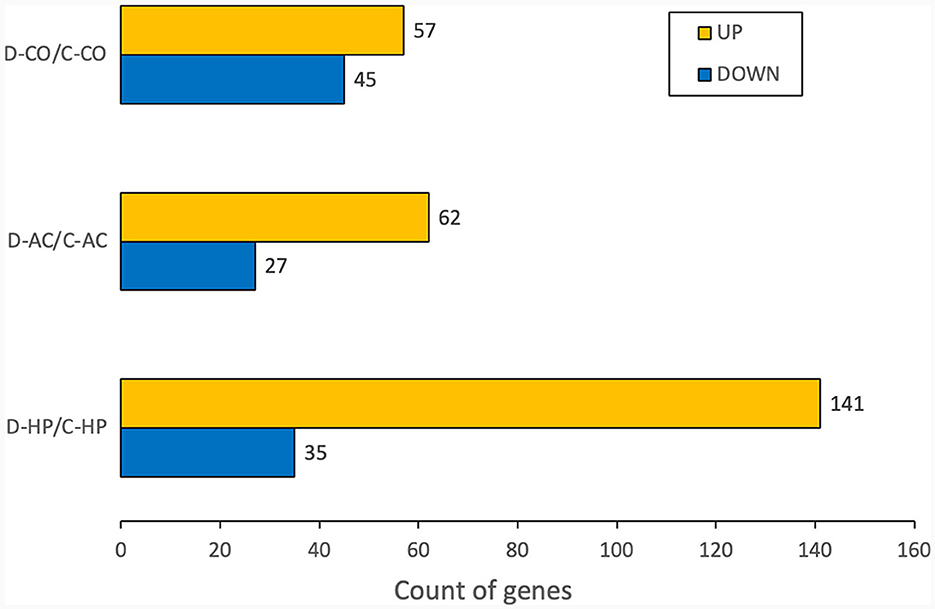
Figure 1. Number of DEGs for each organ. The numbers are calculated for the genes whose fold changes are >2 and raw p-values are within 0.05.
However, these results included not only protein-coding genes but also pseudogenes, lncRNAs, snoRNAs, and miRNAs. We excluded the non-protein-coding genes to determine the functions of the RNAs. When only the protein-coding genes were considered, the number of DEGs reduced. In the cochlea, there were 16 up-regulated and 32 down-regulated protein-coding DEGs (Table 1), while in the auditory cortex, there were 31 up-regulated and 21 down-regulated protein-coding DEGs (Table 2). In the hippocampus, 99 up-regulated and 15 down-regulated protein-coding DEGs were expressed (Table 3). In total, there are 146 up-regulated protein-coding DEGs and 68 down-regulated protein-coding DEGs (Figure 2). This transcriptomic data can be accessed in SRA database of NCBI site (reference number: PRJNA1061000).
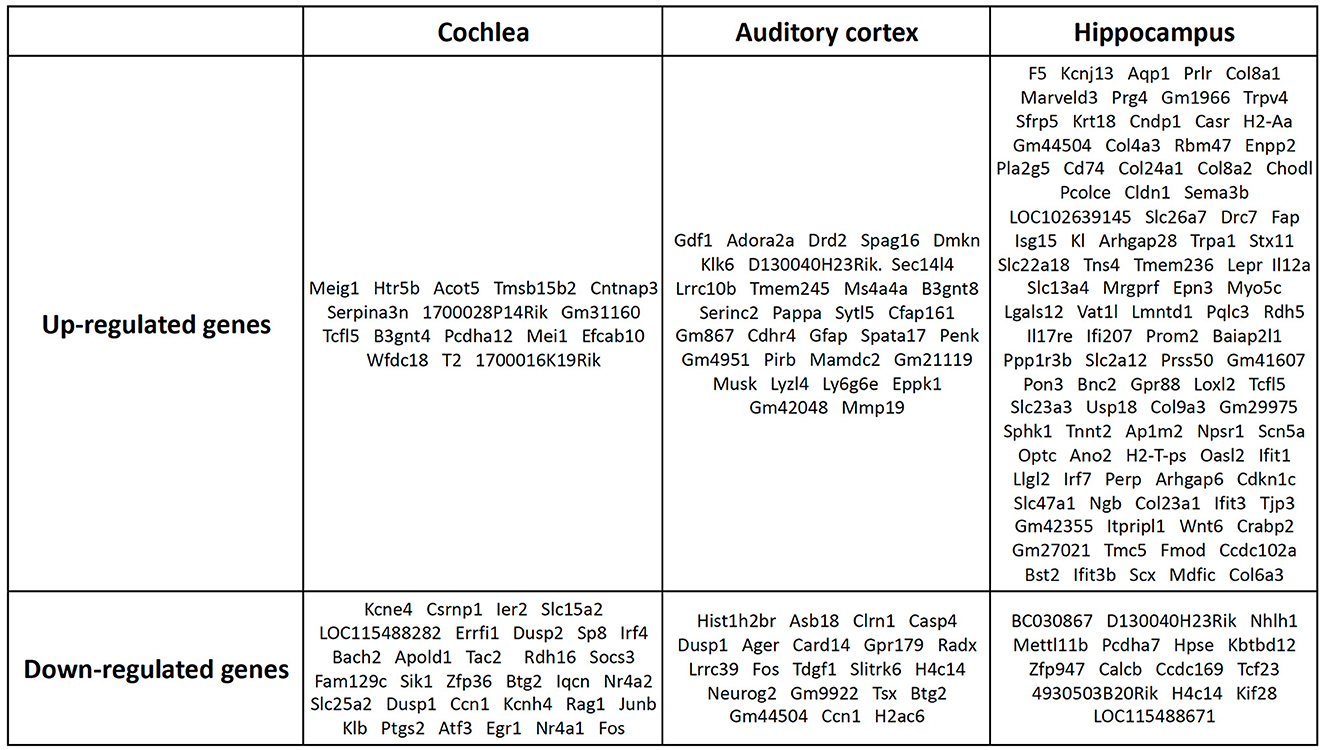
Figure 2. Protein-coding DEGs of the cochlea, audigory cortex, and hippocampus. In the cochlea, there were 16 up-regulated and 32 down-regulated protein-coding DEGs, while in the auditory cortex, there were 31 up-regulated and 21 down-regulated protein-coding DEGs. In the hippocampus, 99 up-regulated and 15 down-regulated protein-coding DEGs were expressed.
Results of GO analysis
Cochlea
When we analyzed the cochlea, 294 GOs were identified as statistically significant (biological process, 259; molecular function, 34; cellular component, 1). The highly enriched GOs in the biological processes of the cochlea were mostly networked with down-regulated genes (Figure 3A). When the GOs of the biological process were classified into upper categories by the treemap technique, many categories were related to cell proliferation (regulation of lipid biosynthetic process, skeletal muscle cell differentiation, transcription by RNA polymerase II, response to fibroblast growth factor, and regulation of keratinocyte differentiation). There were other categories related to apoptosis [positive regulation of the apoptotic process, negative regulation of the mitogen-activated protein kinase (MAPK) cascade, regulation of cell death, and cell death; Figure 3B, Supplementary Table 1].
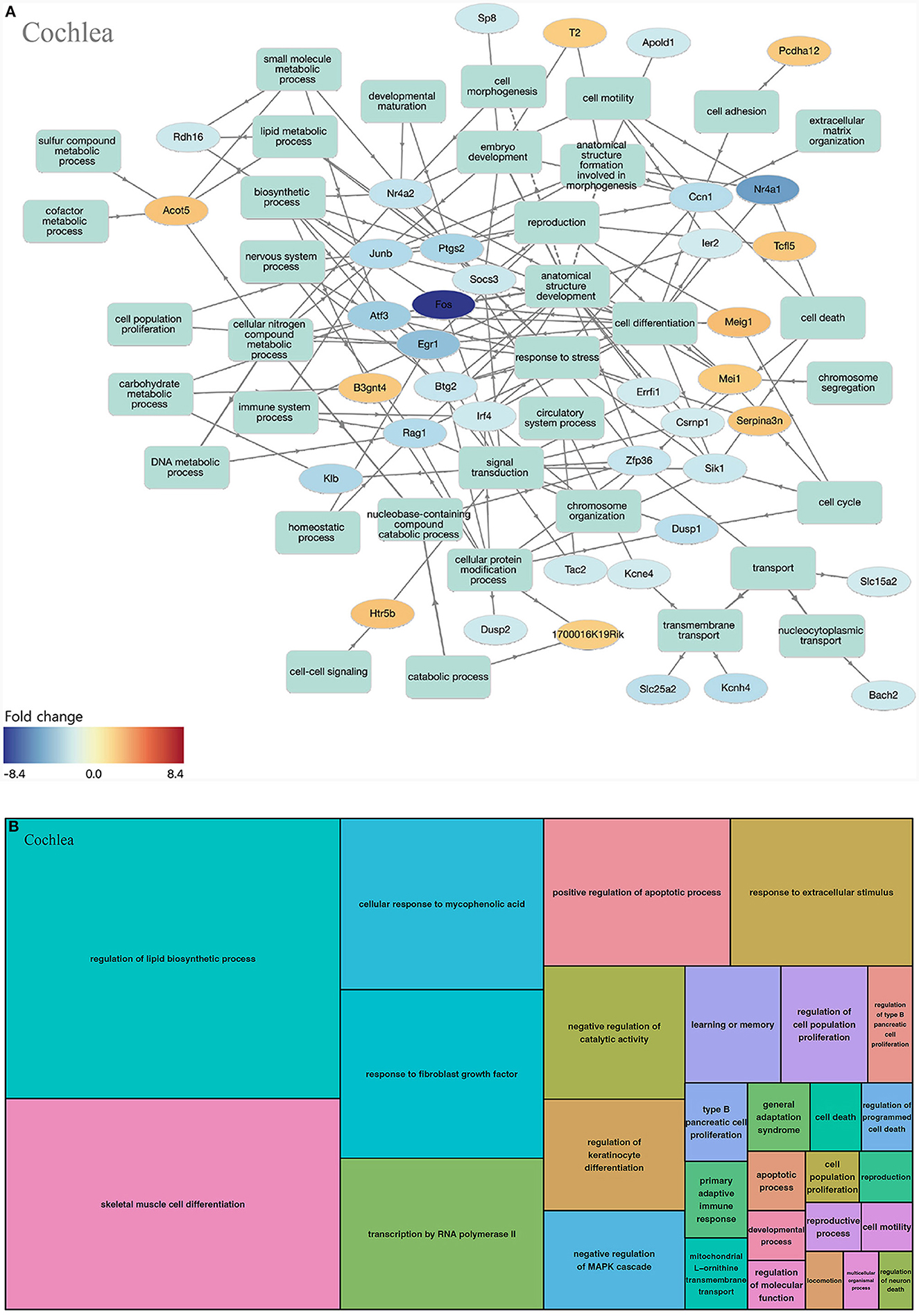
Figure 3. GO analysis on biological process of the cochlea. (A) Term annotation analysis from GOnet. Many genes are turned out down-regulated. (B) Treemap analysis from REVIGO. Many GOs can be categorized into cell proliferation and apoptosis.
Auditory cortex
In the auditory cortex, 211 GOs were identified (biological processes, 193; molecular functions, 1; and cell components, 17). In the biological processes of the auditory cortex, the up-regulated and down-regulated genes play similar roles (Figure 4A). GOs of the biological process can be classified as behavior-concerning (behavior and regulation of behavior), synapse-related (regulation of long-term synaptic potentiation and synaptic signaling), cell-signaling (response to purine-containing compound and regulation of kinase activity), cell metabolism (response to purine-containing compound, regulation of kinase activity, and protein dephosphorylation), and apoptosis (regulation of neuron death) categories, among others (Figure 4B, Supplementary Table 2).
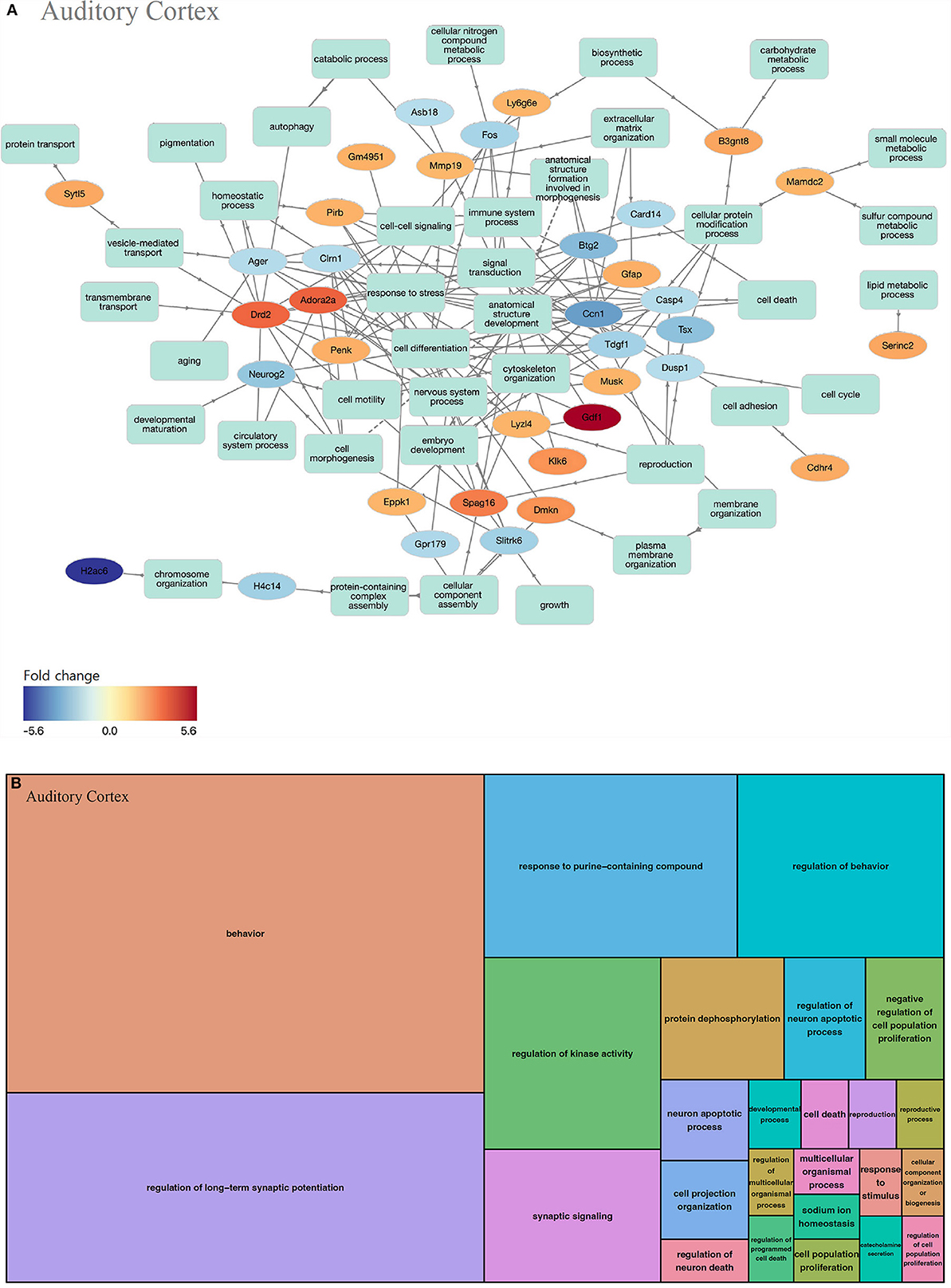
Figure 4. GO analysis on biological process of the auditory cortex. (A) Term annotation analysis from GOnet. Up-regulated genes and down-regulated genes are mixed to a similar degree. (B) Treemap analysis from REVIGO. GOs can be classified into behavior-related, synapse-related, cell signaling, cell metabolism, and apoptosis categories.
Hippocampus
In the hippocampus, 203 GOs were identified (biological processes, 137; molecular functions, 40; and cell components, 26). In the biological process of the hippocampus, most key genes were upregulated (Figure 5A). GOs of the biological process can be classified as inflammation-related (response to cytokine, regulation of cytokine-mediated signaling pathway, and positive regulation of fibroblast proliferation) and viral infection-related categories (negative regulation of the viral process, regulation of viral process, extracellular matrix organization, secretion, and regulation of hydrolase activity, among others; Figure 5B, Supplementary Table 3).
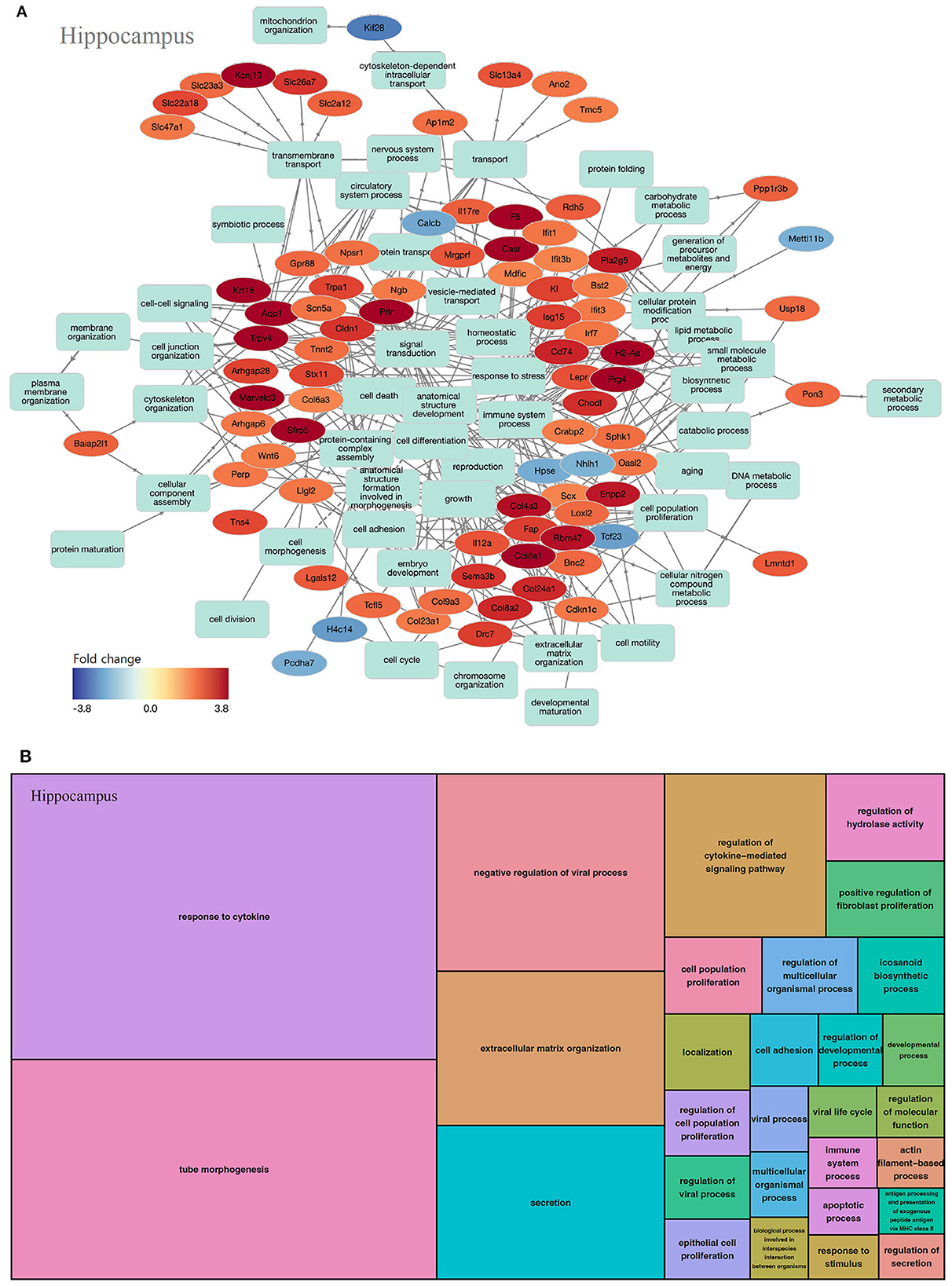
Figure 5. GO analysis on biological process of the hippocampus. (A) Term annotation analysis from GOnet. Most genes are up-regulated. (B) Treemap analysis from REVIGO. GOs can be classified into inflammation-related and viral infection-related categories.
KEGG pathway results
Cochlea
Ten KEGG pathways were statistically significant. These included pathways related to immune responses, such as the tumor necrosis factor (TNF) signaling pathway, and those related to apoptosis, such as the MAPK signaling pathway. Twelve genes were included in these pathways, most of which were down-regulated (Table 4).
Auditory cortex
Seven KEGG pathways were statistically significant. Pathways related to immune reactions (neutrophil extracellular trap formation and B cell receptor signaling pathway), cell death [cyclic adenosine monophosphate (cAMP) signaling pathway], and neuronal synapses (neuroactive ligand-receptor interaction) were expressed. Eleven genes were found in these pathways, and the up-regulated and downregulated genes were combined (Table 5).
Hippocampus
Seventeen KEGG pathways were statistically significant. These include inflammatory pathways [cytokine-cytokine receptor interaction and the Janus kinases-signal transducer and activator of transcription proteins (JAK-STAT) signaling pathway] and viral infection-related pathways. Twenty-seven genes were involved in these pathways, most of which were up-regulated (Table 6).
Discussion
We conducted an omics study to determine how hearing loss affects the central auditory pathway, including cognitive organs. We examined the effects of hearing loss on the cochlea, auditory cortex, and hippocampus using mRNA sequencing. First, we compared the DEGs in these organs. The number of up-regulated genes was the lowest in the cochlea, and it increased toward the central nervous system. In contrast, the number of down-regulated genes increased as we moved toward the periphery (Tables 1–3, Figures 3A, 4A, 5A). Most DEGs in the cochlea exhibited an inhibitory pattern, whereas those in the hippocampus were excitatory.
In the cochlea, genes related to activator protein 1 (AP-1) transcription (Fos and Junb) and inflammation (Ptgs2 and Socs3) were down-regulated. AP-1 transcription factors control cell differentiation, proliferation, and apoptosis during stress and infections (Ameyar et al., 2003; Hess et al., 2004). This suggests that the peak time of inflammatory response and cell death has passed. In the auditory cortex, genes related to the guanine nucleotide-binding protein (G protein)-coupled receptor superfamily (Drd2 and Adora2a) were up-regulated. They are known to have relation with regulation of cognitive function and mood by promoting dopamine binding and dopamine neurotransmitter receptor activity (Komatsu et al., 2014; Khlghatyan et al., 2019). In the hippocampus, genes related to the major histocompatibility complex (H2-T-ps, H2-Aa, Cd74), helper T cell type 1 (Il12a), and the extracellular matrix (Col4a3, Col6a3, Col9a3) were up-regulated, implying an actively processed adaptive immune reactions and cellular changes.
When examining the GOs and KEGG pathways, we observed that the expression patterns differed for each organ. Genes related to cell proliferation and death were simultaneously expressed in the cochlea (Figure 3B, Supplementary Table 1). The TNF signaling pathway, associated with the immune response, and the MAPK signaling pathway, related to apoptosis, were also activated (Table 4). However, most gene activities were down-regulated. Considering cell proliferation, inflammation, and apoptosis occur simultaneously after hearing loss in the cochlea (Shu et al., 2019; Milon et al., 2021; Warnecke et al., 2021; Chen et al., 2022; Paciello et al., 2023), all of these processes seem to be in the finishing stage.
In the auditory cortex, the GO patterns were quite different. Genes related to behavior and neuronal synapse function were highly expressed, and those associated with cellular metabolism, cell signaling, and apoptosis were also observed (Figure 4B, Supplementary Table 2). The cAMP signaling pathway and neuroactive ligand-receptor interactions seem to be related to neuronal cell death and synaptic activity (Table 5). Therefore, it can be inferred that brain plasticity mechanisms occur through synaptic changes along with neuronal cell death, affecting cognitive function in the auditory cortex. Notably, alcoholism and Parkinson's disease appeared in the KEGG pathway, suggesting that changes in the auditory cortex following hearing loss progress in a similar pattern to these disorders.
The hippocampus differs from the other two organs in that most genes exhibited excitatory activity (Figure 5A). Inflammation-related pathways, such as cytokine-cytokine receptor interactions, were commonly activated, indicating ongoing inflammation (Figure 5B, Table 6, Supplementary Table 3). Interestingly, there are many pathways related to viral and fungal infections that seem unrelated, suggesting that when the hippocampus is challenged, it exhibits a response similar to infection. Thus, neuroinflammation persists in the hippocampus even after 3 months of hearing loss.
In conclusion, apoptosis and inflammation persisted more actively in the order of hippocampus, auditory cortex, cochlea in the long term (12 weeks) after noise-induced hearing loss. This implies that the neurodegenerative effects of noise exposure do not resolve quickly in the central regions but persist for a considerable period. Therefore, cognitive decline following noise-induced hearing loss is likely to be a progressive process rather than an instant deterioration (Figure 6). Some studies see the cognitive decline not just as elongated degeneration, but as accelerated aging (Zhuang et al., 2020; Paciello et al., 2021). In the case of normal C57BL/6J mice, no histological changes are observed in the auditory cortex or hippocampus up to 6 months of age (Dong et al., 2018). However, it appears that there are gradual changes in proteins that play significant roles in plasticity and cognitive functions, such as MMP-9 (Dong et al., 2018). Hence, proactive treatment to prevent the progression of cognitive decline remain of substantial importance even if there are little chance of auditory restoration. For example, auditory rehabilitation strategies, including the utilization of hearing aids or cochlear implants, as well as medical interventions such as antioxidant therapy, may prove beneficial for patients with hearing impairment in mitigating the risk of cognitive decline.
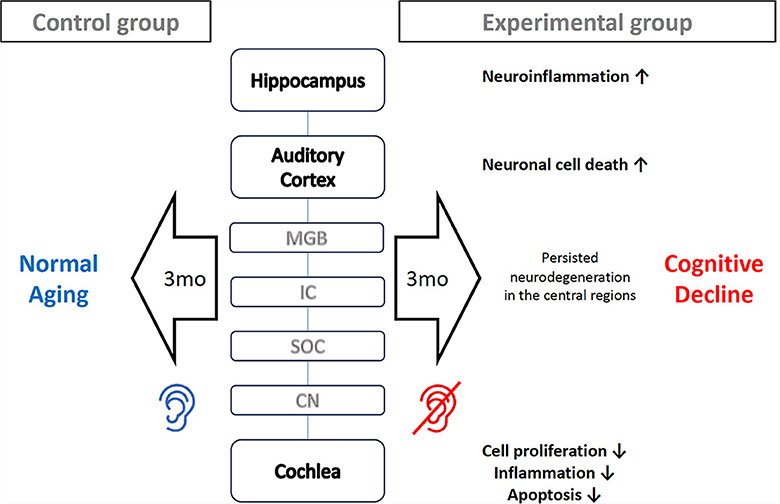
Figure 6. Proposed mechanism how noise-induced hearing loss cause mice cognitive decline. Three months after the onset of noise-induced hearing loss, processes such as cell proliferation, inflammation, and apoptosis come to finishing stage in the cochlea. However, neuronal cell death is still progressing in the auditory cortex, and neuroinflammation persists in the hippocampus. These prolonged neurodegeneration processes in the central region accelerates cognitive decline.
This study has a few limitations. First, this study was conducted only in the aspect of mRNA expression, so it may appear differently at the level of protein. Secondly, this was a cross-sectional study that included 24-week-old mice, and we analyzed its results by considering the general progression of neuroinflammation. However, a time-series study of each organ is required to determine the dynamic mechanisms of neuroinflammation accurately. Finally, the noise used in this study was large enough to evoked permanent hearing loss in a single exposure. Loud noise can affect the central auditory pathway and the hippocampus within a few days (Groschel et al., 2018; Chen et al., 2022). And long-term exposure to noise could affect central neural system differently. In particular, the effects on cognitive function may differ significantly in cases of noise stress or gradual hearing deterioration due to sustained high-intensity noise. Further studies are required to generalize these findings to the overall context of noise-induced hearing loss.
Data availability statement
The datasets presented in this study can be found in online repositories. The names of the repository/repositories and accession number(s) can be found at: https://www.ncbi.nlm.nih.gov/bioproject/PRJNA1061000/.
Ethics statement
The animal study was approved by Institutional Animal Care and Use Committee (IACUC) of Boramae Hospital (IACUC number 2022-0133). The study was conducted in accordance with the local legislation and institutional requirements.
Author contributions
S-YL: Data curation, Formal analysis, Investigation, Software, Visualization, Writing—original draft. HL: Data curation, Investigation, Methodology, Validation, Writing—review & editing. M-HP: Conceptualization, Funding acquisition, Investigation, Methodology, Project administration, Supervision, Writing—review & editing.
Funding
The author(s) declare financial support was received for the research, authorship, and/or publication of this article. This study was supported by the Korea National Research Fund (NRF-2020R1A2C1006254; PI: M-HP). The funding body played no role in the study design, data collection and analysis, decision to publish, or preparation of the manuscript.
Conflict of interest
The authors declare that the research was conducted in the absence of any commercial or financial relationships that could be construed as a potential conflict of interest.
Publisher's note
All claims expressed in this article are solely those of the authors and do not necessarily represent those of their affiliated organizations, or those of the publisher, the editors and the reviewers. Any product that may be evaluated in this article, or claim that may be made by its manufacturer, is not guaranteed or endorsed by the publisher.
Supplementary material
The Supplementary Material for this article can be found online at: https://www.frontiersin.org/articles/10.3389/fnins.2024.1340854/full#supplementary-material
References
Ameyar, M., Wisniewska, M., and Weitzman, J. B. (2003). A role for AP-1 in apoptosis: the case for and against. Biochimie 85, 747–752. doi: 10.1016/j.biochi.2003.09.006
Billig, A. J., Lad, M., Sedley, W., and Griffiths, T. D. (2022). The hearing hippocampus. Prog. Neurobiol. 218:102326. doi: 10.1016/j.pneurobio.2022.102326
Cardin, V. (2016). Effects of aging and adult-onset hearing loss on cortical auditory regions. Front. Neurosci. 10:199. doi: 10.3389/fnins.2016.00199
Chen, D., Jia, G., Zhang, Y., Mao, H., Zhao, L., Li, W., et al. (2022). Sox2 overexpression alleviates noise-induced hearing loss by inhibiting inflammation-related hair cell apoptosis. J. Neuroinflammation 19:59. doi: 10.1186/s12974-022-02414-0
Cui, B., Wu, M. Q., Zhu, L. X., She, X. J., Ma, Q., Liu, H. T., et al. (2013). Effect of chronic noise exposure on expression of N-methyl-D-aspartic acid receptor 2B and Tau phosphorylation in hippocampus of rats. Biomed. Environ. Sci. 26, 163–168. doi: 10.3967/0895-3988.2013.03.002
de Deus, J. L., Amorim, M. R., Ribeiro, A. B., Barcellos-Filho, P. C. G., Ceballos, C. C., Branco, R. M., et al. (2021). Loss of brain-derived neurotrophic factor mediates inhibition of hippocampal long-term potentiation by high-intensity sound. Cell. Mol. Neurobiol. 41, 751–763. doi: 10.1007/s10571-020-00881-8
Deng, T., Li, J., Liu, J., Xu, F., Liu, X., Mi, J., et al. (2021). Hippocampal transcriptome-wide association study reveals correlations between impaired glutamatergic synapse pathway and age-related hearing loss in BXD-recombinant inbred mice. Front. Neurosci. 15:745668. doi: 10.3389/fnins.2021.745668
Dong, Y., Guo, C. R., Chen, D., Chen, S. M., Peng, Y., Song, H., et al. (2018). Association between age-related hearing loss and cognitive decline in C57BL/6J mice. Mol. Med. Rep. 18, 1726–1732. doi: 10.3892/mmr.2018.9118
Franklin, K. B. J., and Paxinos, G. (2013). Paxinos and Franklin's The Mouse Brain in Stereotaxic Coordinates, 4th ed.). Cambridge, MA: Academic Press, an imprint of Elsevier.
Gauthier, S. W., Servaes, C., Morais, S., and Rosa-Neto, J. A. P. (2022). World Alzheimer Report 2022: Life after diagnosis: Navigating reatment, Care and Support. London: Alzheimer's Disease International.
Gonzalez-Perez, O., Chavez-Casillas, O., Jauregui-Huerta, F., Lopez-Virgen, V., Guzman-Muniz, J., Moy-Lopez, N., et al. (2011). Stress by noise produces differential effects on the proliferation rate of radial astrocytes and survival of neuroblasts in the adult subgranular zone. Neurosci. Res. 70, 243–250. doi: 10.1016/j.neures.2011.03.013
Groschel, M., Basta, D., Ernst, A., Mazurek, B., and Szczepek, A. J. (2018). Acute noise exposure is associated with intrinsic apoptosis in murine central auditory pathway. Front. Neurosci. 12:312. doi: 10.3389/fnins.2018.00312
Gurgel, R. K., Ward, P. D., Schwartz, S., Norton, M. C., Foster, N. L., Tschanz, J. T., et al. (2014). Relationship of hearing loss and dementia: a prospective, population-based study. Otol. Neurotol. 35, 775–781. doi: 10.1097/MAO.0000000000000313
Hess, J., Angel, P., and Schorpp-Kistner, M. (2004). AP-1 subunits: quarrel and harmony among siblings. J. Cell Sci. 117(Pt 25), 5965–5973. doi: 10.1242/jcs.01589
Jin, S. G., Kim, M. J., Park, S. Y., and Park, S. N. (2017). Stress hormonal changes in the brain and plasma after acute noise exposure in mice. Auris Nasus Larynx 44, 272–276. doi: 10.1016/j.anl.2016.07.013
Jongkamonwiwat, N., Ramirez, M. A., Edassery, S., Wong, A. C. Y., Yu, J., Abbott, T., et al. (2020). Noise exposures causing hearing loss generate proteotoxic stress and activate the proteostasis network. Cell Rep. 33:108431. doi: 10.1016/j.celrep.2020.108431
Khlghatyan, J., Quintana, C., Parent, M., and Beaulieu, J. M. (2019). High sensitivity mapping of cortical dopamine D2 receptor expressing neurons. Cereb. Cortex 29, 3813–3827. doi: 10.1093/cercor/bhy261
Komatsu, H., Maruyama, M., Yao, S., Shinohara, T., Sakuma, K., Imaichi, S., et al. (2014). Anatomical transcriptome of G protein-coupled receptors leads to the identification of a novel therapeutic candidate GPR52 for psychiatric disorders. PLoS ONE 9:e90134. doi: 10.1371/journal.pone.0090134
Kurioka, T., Mogi, S., and Yamashita, T. (2021). Decreasing auditory input induces neurogenesis impairment in the hippocampus. Sci. Rep. 11:423. doi: 10.1038/s41598-020-80218-z
Lin, F. R., and Albert, M. (2014). Hearing loss and dementia - who is listening? Aging Ment. Health 18, 671–673. doi: 10.1080/13607863.2014.915924
Lin, F. R., Ferrucci, L., Metter, E. J., An, Y., Zonderman, A. B., Resnick, S. M., et al. (2011). Hearing loss and cognition in the baltimore longitudinal study of aging. Neuropsychology 25, 763–770. doi: 10.1037/a0024238
Livingston, G., Sommerlad, A., Orgeta, V., Costafreda, S. G., Huntley, J., Ames, D., et al. (2017). Dementia prevention, intervention, and care. Lancet 390, 2673–2734. doi: 10.1016/S0140-6736(17)31363-6
Maeda, Y., Kariya, S., Uraguchi, K., Takahara, J., Fujimoto, S., Sugaya, A., et al. (2021). Immediate changes in transcription factors and synaptic transmission in the cochlea following acoustic trauma: a gene transcriptome study. Neurosci. Res. 165, 6–13. doi: 10.1016/j.neures.2020.05.001
Miao, L., Zhang, J., Yin, L., and Pu, Y. (2021). TMT-based quantitative proteomics reveals cochlear protein profile alterations in mice with noise-induced hearing loss. Int. J. Environ. Res. Public Health 19:382. doi: 10.3390/ijerph19010382
Milon, B., Shulman, E. D., So, K. S., Cederroth, C. R., Lipford, E. L., Sperber, M., et al. (2021). A cell-type-specific atlas of the inner ear transcriptional response to acoustic trauma. Cell Rep. 36:109758. doi: 10.1016/j.celrep.2021.109758
Nadhimi, Y., and Llano, D. A. (2021). Does hearing loss lead to dementia? A review of the literature. Hear. Res. 402:108038. doi: 10.1016/j.heares.2020.108038
Paciello, F., Rinaudo, M., Longo, V., Cocco, S., Conforto, G., Pisani, A., et al. (2021). Auditory sensory deprivation induced by noise exposure exacerbates cognitive decline in a mouse model of Alzheimer's disease. Elife 10:e70908. doi: 10.7554/eLife.70908.sa2
Paciello, F., Ripoli, C., Fetoni, A. R., and Grassi, C. (2023). Redox imbalance as a common pathogenic factor linking hearing loss and cognitive decline. Antioxidants 12:332. doi: 10.3390/antiox12020332
Shu, Y., Li, W., Huang, M., Quan, Y. Z., Scheffer, D., Tian, C., et al. (2019). Renewed proliferation in adult mouse cochlea and regeneration of hair cells. Nat. Commun. 10:5530. doi: 10.1038/s41467-019-13157-7
Subedi, P., Moertl, S., and Azimzadeh, O. (2022). Omics in radiation biology: surprised but not disappointed. Radiation 2, 124–129. doi: 10.3390/radiation2010009
Tisher, A., and Salardini, A. (2019). A comprehensive update on treatment of dementia. Semin. Neurol. 39, 167–178. doi: 10.1055/s-0039-1683408
Vailati-Riboni, M., Palombo, V., and Loor, J. J. (2017). “What are omics sciences?” in Periparturient Diseases of Dairy Cows: A Systems Biology Approach, ed. B. N. Ametaj (Cham: Springer International Publishing), 1–7. doi: 10.1007/978-3-319-43033-1_1
Warnecke, A., Harre, J., Shew, M., Mellott, A. J., Majewski, I., Durisin, M., et al. (2021). Successful treatment of noise-induced hearing loss by mesenchymal stromal cells: an RNAseq analysis of protective/repair pathways. Front. Cell. Neurosci. 15:656930. doi: 10.3389/fncel.2021.656930
Keywords: noise-induced hearing loss, transcriptomics, neurodegeneration, cochlea, auditory cortex, hippocampus
Citation: Lee S-Y, Lee HS and Park MH (2024) Transcriptomic analysis reveals prolonged neurodegeneration in the hippocampus of adult C57BL/6N mouse deafened by noise. Front. Neurosci. 18:1340854. doi: 10.3389/fnins.2024.1340854
Received: 19 November 2023; Accepted: 25 January 2024;
Published: 12 February 2024.
Edited by:
Gi Jung Im, Korea University, Republic of KoreaReviewed by:
Athanasia Warnecke, Hannover Medical School, GermanyJiwon Chang, Hallym University Medical Center, Republic of Korea
Joong Ho Ahn, University of Ulsan, Republic of Korea
Copyright © 2024 Lee, Lee and Park. This is an open-access article distributed under the terms of the Creative Commons Attribution License (CC BY). The use, distribution or reproduction in other forums is permitted, provided the original author(s) and the copyright owner(s) are credited and that the original publication in this journal is cited, in accordance with accepted academic practice. No use, distribution or reproduction is permitted which does not comply with these terms.
*Correspondence: Min-Hyun Park, ZHJwYXJrQHNudS5hYy5rcg==