- 1Child Neurology, Emma Children’s Hospital, Amsterdam Leukodystrophy Center, Amsterdam University Medical Centers, Vrije Universiteit and Amsterdam Neuroscience, Amsterdam, Netherlands
- 2Department of Integrative Neurophysiology, Center for Neurogenomics and Cognitive Research, VU University, Amsterdam, Netherlands
- 3Department of Pediatrics, University of Utah, Salt Lake City, UT, United States
Vanishing white matter (VWM) is a devastating autosomal recessive leukodystrophy, resulting in neurological deterioration and premature death, and without curative treatment. Pathogenic hypomorphic variants in subunits of the eukaryotic initiation factor 2B (eIF2B) cause VWM. eIF2B is required for regulating the integrated stress response (ISR), a physiological response to cellular stress. In patients’ central nervous system, reduced eIF2B activity causes deregulation of the ISR. In VWM mouse models, the extent of ISR deregulation correlates with disease severity. One approach to restoring eIF2B activity is by inhibition of GSK3β, a kinase that phosphorylates eIF2B and reduces its activity. Lithium, an inhibitor of GSK3β, is thus expected to stimulate eIF2B activity and ameliorate VWM symptoms. The effects of lithium were tested in zebrafish and mouse VWM models. Lithium improved motor behavior in homozygous eif2b5 mutant zebrafish. In lithium-treated 2b4he2b5ho mutant mice, a paradoxical increase in some ISR transcripts was found. Furthermore, at the dosage tested, lithium induced significant polydipsia in both healthy controls and 2b4he2b5ho mutant mice and did not increase the expression of other markers of lithium efficacy. In conclusion, lithium is not a drug of choice for further development in VWM based on the limited or lack of efficacy and significant side-effect profile.
Introduction
Vanishing white matter (VWM) is leukodystrophy with an autosomal recessive mode of inheritance, which most often presents in young children (Hamilton et al., 2018). This disease causes neurological deterioration characterized by motor decline, cerebellar ataxia, cognitive decline, and premature death; curative treatment is currently not available. White matter pathology is characterized by rarefaction and cystic degeneration with loss of all white matter components. Numbers of immature astrocytes and oligodendrocytes are increased, which fail in their respective mature functions of forming astroglial scar tissue and producing and maintaining sufficient myelin (Bugiani et al., 2011). Astrocyte dysfunction is central to VWM pathogenesis (Dooves et al., 2016).
VWM is caused by bi-allelic pathogenic variants in any of the five genes encoding the subunits (α-ε) of eukaryotic initiation factor 2B (eIF2B) (van der Knaap et al., 2002). The eIF2B protein complex is essential for mRNA translation initiation in all eukaryotic cells due to its function as a guanine nucleotide exchange factor (GEF) for the eIF2 complex (Konieczny and Safer, 1983; Wortham et al., 2014). In addition, eIF2B is central in the integrated stress response (ISR), an adaptive cell response to different types of cellular stress that requires translation attenuation and selective expression of stress-ameliorating proteins (Proud, 2001). eIF2B activity is inhibited via direct phosphorylation of serine 540 in the ε subunit by the glycogen synthase kinase 3β (GSK3β) (Stambolic et al., 1996; Welsh et al., 1998; Beurel et al., 2015) or upon phosphorylation of the conserved serine 51 in the α subunit of eIF2 (eIF2α) (Pakos-Zebrucka et al., 2016). Decreased eIF2B activity directly attenuates bulk protein translation and increases the production of transcription factors ATF4, CHOP, and phosphatase co-factor GADD34 (Lu et al., 2004; Han et al., 2013). ATF4 and CHOP increase the expression of stress-ameliorating proteins (Han et al., 2013), and GADD34 constitutes an important ISR negative feedback loop imperative in eIF2α dephosphorylation and transcription attenuation (Pakos-Zebrucka et al., 2016).
Pathogenic variants in any eIF2B subunit reduce its activity (van Kollenburg et al., 2006; Horzinski et al., 2010; Liu et al., 2011; Wisse et al., 2017; Abbink et al., 2019; Wong et al., 2019). In the brains of VWM patients, reduced eIF2B activity leads to increased ATF4 and CHOP activities and reduced levels of phosphorylated eIF2α (p-eIF2α) (Abbink et al., 2019). This increased ATF4 activity is detected selectively in patients’ astrocytes. eIF2B activators ameliorate neurological disease in VWM mouse models; their degree of disease amelioration correlates strongly with the degree of attenuated expression of ATF4 and its transcriptome (Abbink et al., 2019; Wong et al., 2019). These studies highlight two important findings: (1) eIF2B is a promising treatment target, and (2) the expression levels of ATF4-regulated transcripts are suitable markers for eIF2B activity and VWM pathogenesis.
Inhibiting GSK3β to enhance eIF2B activity has been suggested as a potential treatment target for VWM (Halliday et al., 2017; Pavitt, 2018). GSK3β inhibition is expected to enhance eIF2B activity, attenuate the expression of ATF4-regulated transcripts, and ameliorate VWM (Stambolic et al., 1996; Pap and Cooper, 2002; Pavitt, 2005; Pasquali et al., 2010). The transcription-regulating factor β-catenin is another target of GSK3β. GSK3β inhibition increases β-catenin stability, allowing its relocation into the nucleus (Doble and Woodgett, 2003; Valvezan et al., 2012; Stamos and Weis, 2013). Inside the nucleus, β-catenin regulates the expression of specific markers and is part of the Wnt signaling pathway (Figure 1) (Stambolic et al., 1996; Niehrs, 2012). Therefore, GSK3β inhibition is also expected to increase the level of non-phosphorylated β-catenin with consequential increases of β-catenin-regulated transcripts.
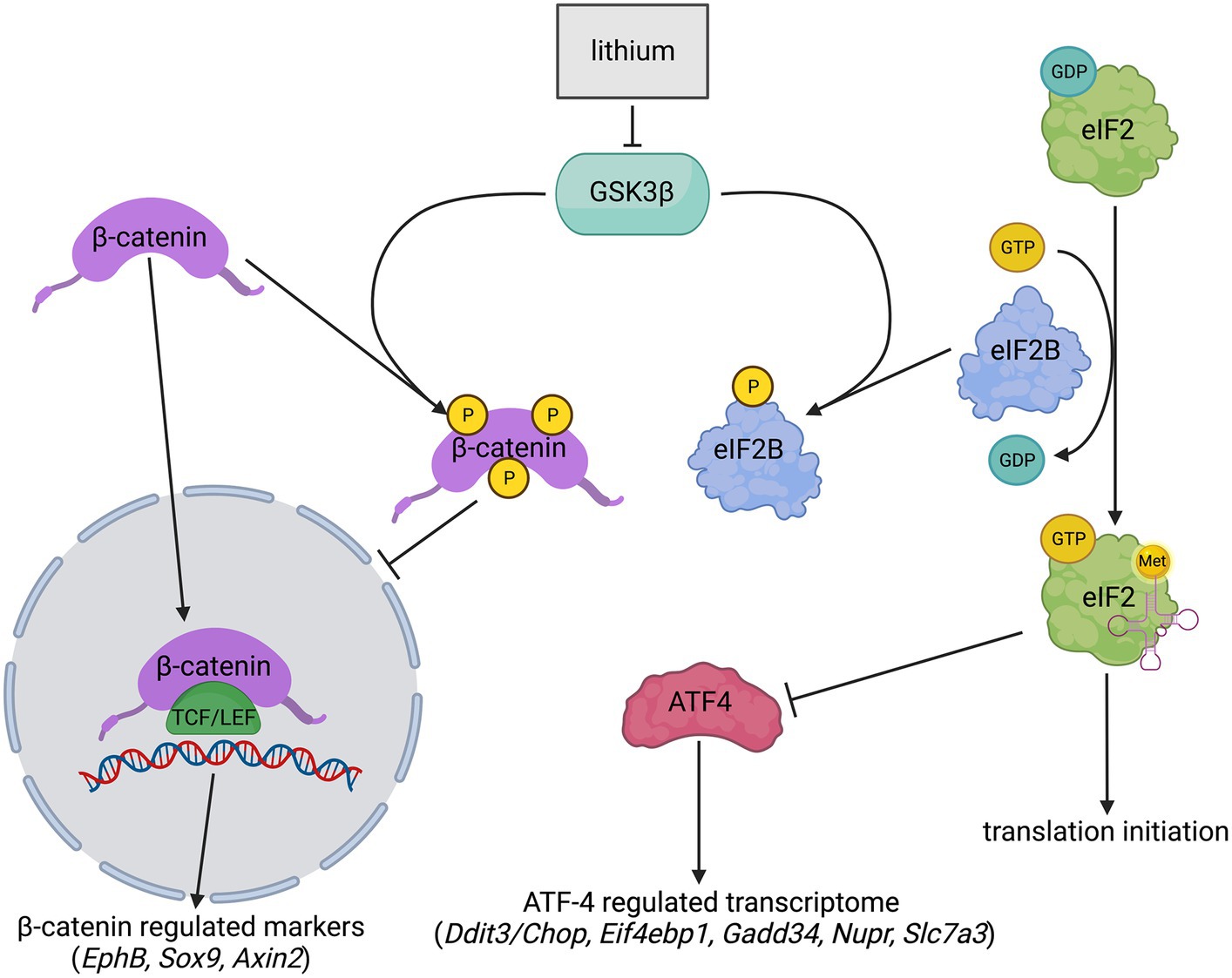
Figure 1. Simplified schematic overview of lithium effects on GSK3β, eIF2B, and β-catenin. Lithium inhibits GSK3β activity. GSK3β inhibition lowers eIF2B phosphorylation (yellow) levels resulting in increased eIF2B activity and presumably downregulated expression of ATF4 and the ATF4-regulated transcriptome. GSK3β inhibition also stabilizes β-catenin by impeding its phosphorylation (yellow) at Ser33, Ser37, and Thr41. Phosphorylated β-catenin is highly unstable and was therefore not quantified. Non-phosphorylated β-catenin moves to the nucleus and joins with a member of the T-cell factor/lymphoid enhancer factor (TCF/LEF) family of transcription factors. This joining upregulates the expression of several genes, including EphB, Sox9, and Axin2. ➔ indicates stimulation; --| indicates inhibition. The image was created with BioRender.com.
Lithium is a GSK3β-inhibiting drug (Stambolic et al., 1996; Beurel et al., 2015), which is used clinically as a treatment for bipolar disorder (Shorter, 2009). In the current project, the potential effect of lithium was assessed in two VWM disease models. Initial studies with lithium were performed in eif2b5 mutant zebrafish, which is homozygous for an internal deletion in exon 1 and displays reduced locomotor activity, decreased thickness of the myelin sheath, and ISR activation (Keefe et al., 2020). Treatment of these zebrafish resulted in improved motor behavior. Subsequent tests were performed in the 2b4he2b5ho mouse model, an established preclinical model for human disease (Abbink et al., 2019; Witkamp et al., 2022). This mouse model replicates clinical and neuropathological signs of human VWM, including the deregulated ISR in the central nervous system (Dooves et al., 2016; Abbink et al., 2019). The levels of ISR-regulated mRNAs in their brain correlate well with disease state and respond quickly to therapeutic targeting of the ISR [e.g., Figure 1 in Witkamp et al. (2022)]. Human eIF2Bε is more conserved in mice than in zebrafish. Due to greater phylogenetic differences between zebrafish and humans than between mice and humans, we decided to validate lithium effects observed in zebrafish in 2b4he2b5ho mutant VWM mice before considering application in VWM patients. Lithium-induced side-effects polydipsia and polyuria (Bendz and Aurell, 1999) were monitored. β-catenin and ATF4 activity were investigated by the quantification of the expression of their regulated transcripts.
Methods
Animals
Zebrafish
Healthy controls (wild-type; WT) and eif2B5zc103 zebrafish were used in this study (Keefe et al., 2020). Heterozygous in-crosses were used for experiments. Genotyping was performed as previously described (Keefe et al., 2020) but consisted of survival genotyping at 72 hpf. In brief, embryos were loaded individually onto genotyping wells of a ZEG chip (wFluidx, Inc.). Once embryos were loaded, the loaded ZEG chip was vibrated. The corresponding embryo was removed from the chip well with a transfer pipette and a small amount of E3 into a 96 square well plate (650 μL/well) until after genotyping. For genotyping PCR, 5 μL of E3 was removed from each ZEG chip well and was used directly in PCR.
All methods have been reported in accordance with recommendations in the ARRIVE guidelines. Adult fish were bred according to standard methods. Embryos were raised at 28.5°C in E3 embryo medium and staged by time and morphology. Zebrafish were randomly allocated to control or experimental groups, and investigators were blinded to genotype and/or experimental status during experiments and analysis of the data. Zebrafish experiments were performed in accordance with the guidelines from the University of Utah Institutional Animal Care and Use Committee (IACUC), regulated under federal law (the Animal Welfare Act and Public Health Services Regulation Act) by the U.S. Department of Agriculture (USDA) and the Office of Laboratory Animal Welfare at the NIH, and accredited by the Association for Assessment and Accreditation of Laboratory Care International (AAALAC). For zebrafish welfare, ethical considerations, and steps to minimize suffering, we followed and used the specific guidelines and regulations at our institution (the University of Utah IACUC, the USDA, and the NIH Office of Laboratory Animal Welfare). Less than 1% of embryos died during the experiment, with no difference between the control and treatment (lithium) groups.
Mice
Mouse breeding was done inside the VU-VUmc animal facility. VWM mice heterozygous for eIF2Bδ Arg484Trp and homozygous for eIF2Bε Arg191His mutation (2b4he2b5ho) were used in this study. WT (C57BL/6 J) mice were included as healthy controls. Genotyping of the mutant mouse line was done as before (Dooves et al., 2016), before weaning. The 2b4he2b5ho genotype was phenotypically confirmed during weaning on the basis of size. Mice were weaned at P28 and kept with a 12-h light/dark cycle with food and water provided ad libitum. Mice were kept in individually ventilated cages and provided with enrichment including a house, nesting material, and gnawing sticks. The humane endpoint (HEP) for the mutant mice was defined by weight loss of more than 15% of body weight for 2 consecutive days or weight loss of more than 20%, although in the current study, HEP was not applied. All mouse experiments were carried out in compliance with the Dutch and European law and with the approval of the local animal care and use committee of the VU University (license CCD AVD1140020172804, work protocol 2,804-NEU19-11). All methods have been reported in accordance with recommendations in the ARRIVE guidelines.
Lithium treatment in WT and eif2b5 mutant zebrafish
Collected zebrafish embryos were manually dechorionated at 1 day post-fertilization (dpf) and transferred into the pre-equilibrated solution (E3) containing 1 mM LiCl in water and 0.1% DMSO. The 1 mM concentration is a standard dose and causes inhibition of GSK3β signaling (e.g., Liu et al., 2018). 0.1% DMSO in solution (E3) was used as a placebo control in 50 mL Petri dishes. Embryos were incubated until 7 dpf. Larval behavior analysis consisting of recordings of total movement time, total distance moved, and average velocity was performed on 7 dpf larvae in 96-well square bottom plates (Krackeler Scientific) using automated video analysis software (Noldus EthoVision). Animals were transferred at 6 dpf to the 96-well plate and kept at 28.5°C overnight. At 7 dpf, the plate was placed on the video imaging system, and the animals adapted in the dark for 10 min and recorded for 5 min (1-min dark and 4-min light). Data consisted of 48 WT and 38 eif2b5zc103/zc103 zebrafish treated with placebo and 12 WT and 26 eif2b5zc103/zc103 zebrafish with lithium. In the zebrafish, experiments were performed prior to the biological determination of sex, which happens when zebrafish are older than 2 months.
Lithium treatment in WT and 2b4he2b5ho mutant mice
Lithium chloride (Sigma-Aldrich) was dissolved in water for injection (WFI) (pH ~7.7). Three-month-old WT and 2b4he2b5ho mutant VWM female mice were weighed before the start of the experiment and evenly assigned to treatment groups based on this initial body weight to prevent a body weight bias. Other than body weight, the mice were randomly assigned to a treatment group in the presence of two researchers to prevent human error. Two animals of the same genotype and treatment group were housed per cage. The mice were injected daily in the intraperitoneal (i.p.) cavity with 200 mg/kg lithium chloride or placebo (WFI) (n = 8 per test condition, n = 32 in total). To guarantee constant dosing, we chose i.p. injection over oral gavage, which was considered more burdensome in the very small mice. Can et al. (2011) showed that i.p. injection with this dose resulted in brain lithium concentrations comparable to the human therapeutic range (Can et al., 2011). The duration of the treatment was based on a study from Makoukji et al. (2012) showing beneficial effects on remyelination after nerve-crush injury after 7 days of lithium treatment. The general wellbeing of the animals was monitored throughout the experiment. Researchers performing the injections were not blinded as mouse body weight and lithium-associated side effects would make both genotypes and treatment obvious. Injections were therefore performed in the presence of two researchers to prevent human error. Body weight was measured daily. Lithium-induced polydipsia was monitored by daily weighing of the water bottles. Two empty cages with a water bottle were included as drip control. Water intake was calculated per cage by dividing water intake per 24 h, corrected for general dripping, by the total body weight of the two mice. After 1 week of injections, the mice were euthanized by cervical dislocation 7–8 h after their final injection. This time frame was selected because lithium concentrations in mouse brains were highest between 5 and 8 h after i.p. injection and comparable to the human therapeutic range (Can et al., 2011). Brains were taken out, snap-frozen in liquid nitrogen, and stored at −80°C for molecular examination.
Quantification of ISR deregulation in mouse brain
The cerebella were prepared for the qPCR and Western blot tests, as these parts of the brain showed amelioration of ISR components in previous studies and have relatively high ratios of white to gray matter (Abbink et al., 2019; Wong et al., 2019; Witkamp et al., 2022). Samples were prepared in the presence of two researchers to minimize human error. qPCR was performed as described (Wisse et al., 2017; Abbink et al., 2018; Witkamp et al., 2022). Sorcs1 and Nrxn mRNA were used as reference genes because the expression of previously used reference genes varied depending on lithium treatment. Oligonucleotide primers for mRNA quantification can be found in Supplementary Data Sheet S2. The researcher performing the qPCR was blinded to treatment and genotype. The SDS-PAGE with 2,2,2-trichloroethanol (TCE) (Ladner et al., 2004) and the Western blotting were done as described (Wisse et al., 2017; Witkamp et al., 2022). Protein yield in one lysate from the lithium-treated VWM group was insufficient for use in the Western blot and was excluded from the study. As primary antibodies, anti-eIF2α SC-11386/SantaCruz (1:1000), anti-phospho-eIF2α (Ser51) 119A11/Cell Signaling (1:1000), and anti-non-phospho (Active) β-Catenin (Ser33/37/Thr41) 8814S/Cell Signaling (1,1,000) were used. HRP-labeled anti-IgG rabbit (1,10,000, Dako, P0448) was used as the secondary antibody. Quantification was done as described (Wisse et al., 2017). Researchers were not blinded to genotype or treatment for the Western blot experiments because the order of samples was set to prevent confounding effects caused by the location of the sample on the blot.
Statistical analysis
Statistical analyses on zebrafish and mice data were performed with GraphPad Prism 9.3.1 software. Broadly, the statistical analyses follow the statistical analyses that we and others have applied (Kim, 2015; Keefe et al., 2020; Witkamp et al., 2022; Le et al., 2023; Tran et al., 2023; McKnight and Najab, n.d.). Importantly, in certain experiments, WT animals were included to ensure that the disease phenotype in mutant animals was as expected (“the experiment worked”—controls). Once this difference was verified, these control data were removed from the dataset, which was used in subsequent statistical analyses to address the treatment effects in VWM mice. For these subsequent analyses, correction for multiple testing was omitted to minimize the chance of a type 2 error. Differences of p <0.05 were considered significant. Statistically significant differences between WT and eif2b5zc103/zc103 placebo-treated zebrafish were examined using an unpaired t-test (Kim, 2015) or Mann–Whitney test (McKnight and Najab, n.d.) (distance moved and velocity; Supplementary Data Sheet S1). Lithium treatment effects were examined separately in WT and eif2b5zc103/zc103 zebrafish using an unpaired t-test or Mann–Whitney test (VWM, distance moved; Supplementary Data Sheet S1). Examination of treatment differences in water intake within and between WT and 2b4he2b5ho mutant genotypes was performed with a mixed effects model followed by post-Tukey’s multiple comparison test. For results obtained using qPCR and the Western blot, session variation was corrected with the software program factor (Ruijter et al., 2006). Variation in treatments or conditions was not corrected in this program. For qPCR, the mean Cp values of the reference genes Sorcs1 and Nrxn were calculated per sample using the LightCycler 480-II software as described in Wisse et al. (2017). Relative expression of the target gene was obtained by dividing the Cp value of the target gene with the mean Cp value of the two reference genes (Livak and Schmittgen, 2001; Pfaffl, 2001; Schmittgen and Livak, 2008; Riedel et al., 2014). For the Western blots, protein signal values were corrected over TCE values (loading control) per lane. Relative levels of phosphorylated eIF2α were determined by dividing their signals with those of total eIF2α (Harding et al., 2000b). Statistically significant differences between WT and 2b4he2b5ho mutant placebo-treated mice were examined using an unpaired t-test or Mann–Whitney test (relative EphB, Sox9, Axin2, eIF2α phosphorylation, Ddit3/Chop, Eif4ebp1, Gadd34, Nupr, Trib3, and Slc3a2 levels) (Supplementary Data Sheet S1). If a statistically significant difference was observed, lithium treatment effects were examined separately in WT and 2b4he2b5ho mutant mice using an unpaired t-test or Mann–Whitney test (VWM: relative Ddit3/Chop, Gadd34, Nupr, Psat1 levels) (Supplementary Data Sheet S1). When there was no significant difference between the WT and VWM phenotypes, data were analyzed using a two-way ANOVA (Gelman, 2005) (Supplementary Data Sheet S1).
Results
Lithium ameliorates velocity in eif2B5zc103 mutant zebrafish
To test the effects of lithium on VWM, a previously published zebrafish (Danio rerio) small vertebrate model of VWM (Keefe et al., 2020) was used. Zebrafish eif2B5zc103 homozygous mutants show impaired swimming behavior: their distance moved, time, and swimming velocity were, respectively, −66, −73%, and − 66% lower than in wild-type (WT) fish (all p < 0.0001; Figure 2). Lithium or placebo was administered to WT (placebo: n = 48, lithium: n = 12) or eif2B5zc103 mutant zebrafish (placebo: n = 38, lithium: n = 26), and their swimming velocity was determined by assessing distance and time. Lithium did not affect distance moved, time, or swimming velocity in WT zebrafish but significantly improved all these parameters in mutant zebrafish (+60%, p = 0.0202; +70%, p = 0.0079; +60%, p = 0.0120, respectively; Figure 2; Supplementary Data Sheet S1).
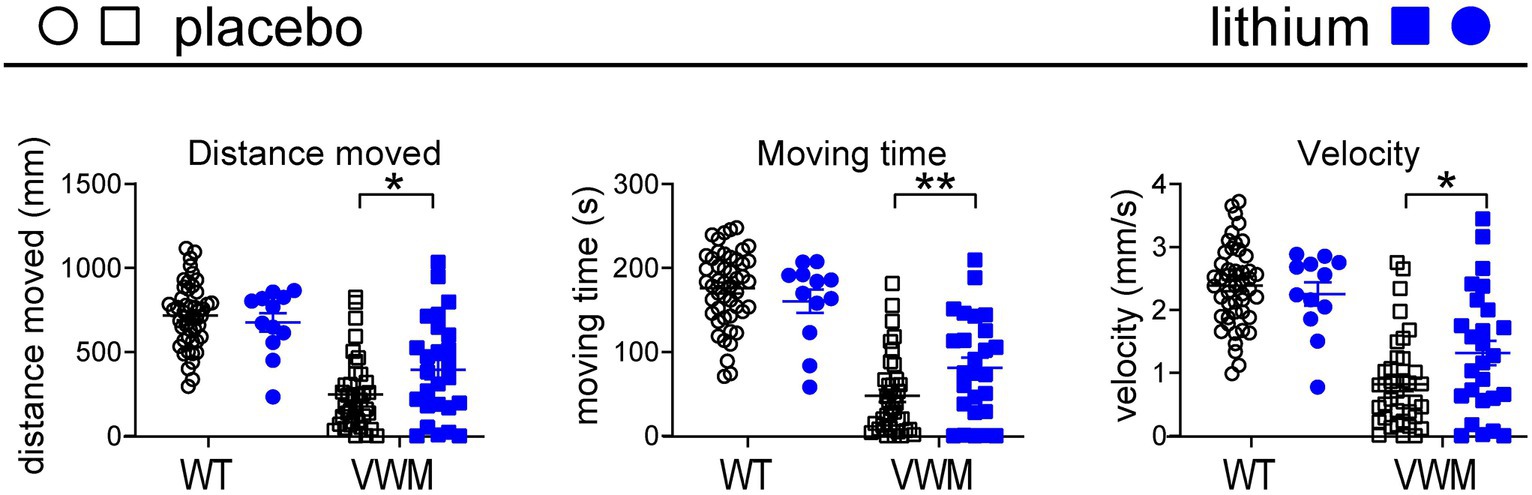
Figure 2. Lithium improves motor deficits in mutant eif2b5zc103/zc103 zebrafish. WT (circles) and eif2b5zc103/zc103 (VWM; squares) zebrafish were treated with placebo (black; WT: n = 48, VWM: n = 38) or 1 mM lithium (blue; WT: n = 12, VWM: n = 26) and assessed on distance moved, moving time, and velocity at 7 dpf. The graphs show individual and mean values, +/− SEM. The shown motor behavior parameters differ significantly in placebo-treated WT versus placebo-treated eif2b5zc103/zc103 zebrafish (p < 0.0001; not indicated, Supplementary Data Sheet S1). Treatment effects were statistically analyzed using an unpaired t-test or Mann–Whitney test (VWM, distance moved; Supplementary Data Sheet S1). *p < 0.05 and **p < 0.01.
Lithium increases water intake in WT and in 2b4he2b5ho mutant mice
Lithium increased water intake in WT and 2b4he2b5ho mutant mice and was accompanied by markedly increased urination, as observed by the wetness of the cage bedding. The start and extent of the response to lithium injections differed per genotype (n = 8 per treatment, per genotype) (interaction effect time x treatment x genotype: F(6, 70) = 12,68, p < 0.0001). The lithium-induced increased water intake was significantly higher in WT mice than in 2b4he2b5ho mutant mice (p < 0.0001). The water intake increase was also earlier in WT mice: Lithium-injected WT mice started to drink more from experimental day 4 onward (+260%, p = 0.0006) but in 2b4he2b5ho mutant mice not until experimental day 6 (+185%, p = 0.0298) as compared to their placebo-treated genotype controls. Lithium injections increased water intake most on the final day of the experiment in both WT (day 7: +651%, p < 0.0001) and 2b4he2b5ho mutant mice (day 7: +342%, p < 0.0001) as compared to placebo-treated mice (Figure 3; Supplementary Data Sheet S1).
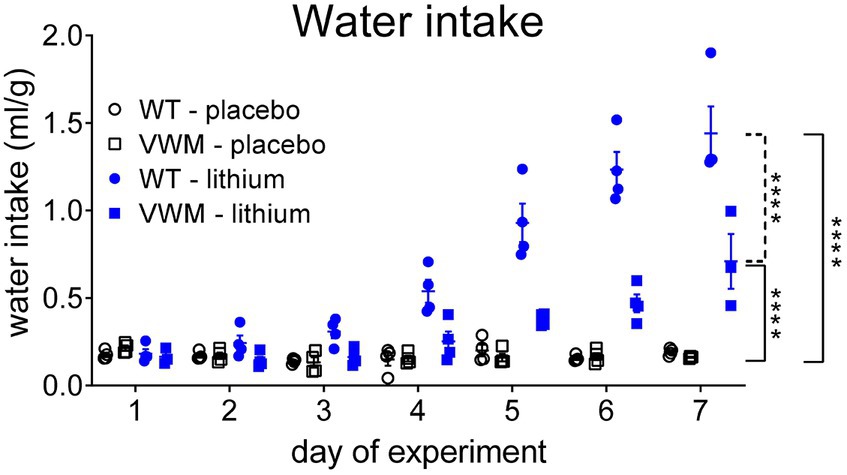
Figure 3. Lithium induces more pronounced polydipsia in WT than in 2b4he2b5ho mutant mice. WT (circles) and 2b4he2b5ho mutant (VWM; squares) mice were i.p. injected with placebo (black) or 200 mg/kg lithium (blue) (n = 8 per treatment, per genotype). Water intake (ml/g) is shown per cage housing two mice (individual and mean values, +/− SEM). Statistical analysis was performed with a mixed-effects model with post-hoc Tukey’s multiple comparison test (Supplementary Data Sheet S1). ****p < 0.0001; placebo-lithium differences: ____, WT-VWM difference: ----. Raw data are in Supplementary Data Sheet S2.
Lithium does not increase the levels of β-catenin or its regulated mRNAs in mouse cerebellum
Lithium is reported to increase the stability and transcription-regulating activity of β-catenin (O’brien et al., 2004), which increases transcription of β-catenin-regulated mRNAs EphB (Batlle et al., 2002; Sansom et al., 2004), Sox9 (Blache et al., 2004; Panza et al., 2013), and Axin2 (Jho et al., 2002; Clevers, 2006; Fancy et al., 2011) in mouse brain. Baseline expression of β-catenin and its downstream targets was similar in the brains of placebo-treated WT and 2b4he2b5ho mutant mice (Figure 4). Here, we find that lithium did not substantially or consistently affect the accumulation of non-phosphorylated β-catenin (WT: −5%, p = 0.9312, 2b4he2b5ho mutant: +18%, p = 0.4446) and its regulated mRNAs in the brain (Figure 4; Supplementary Data Sheet S1; Supplementary Figure S2).
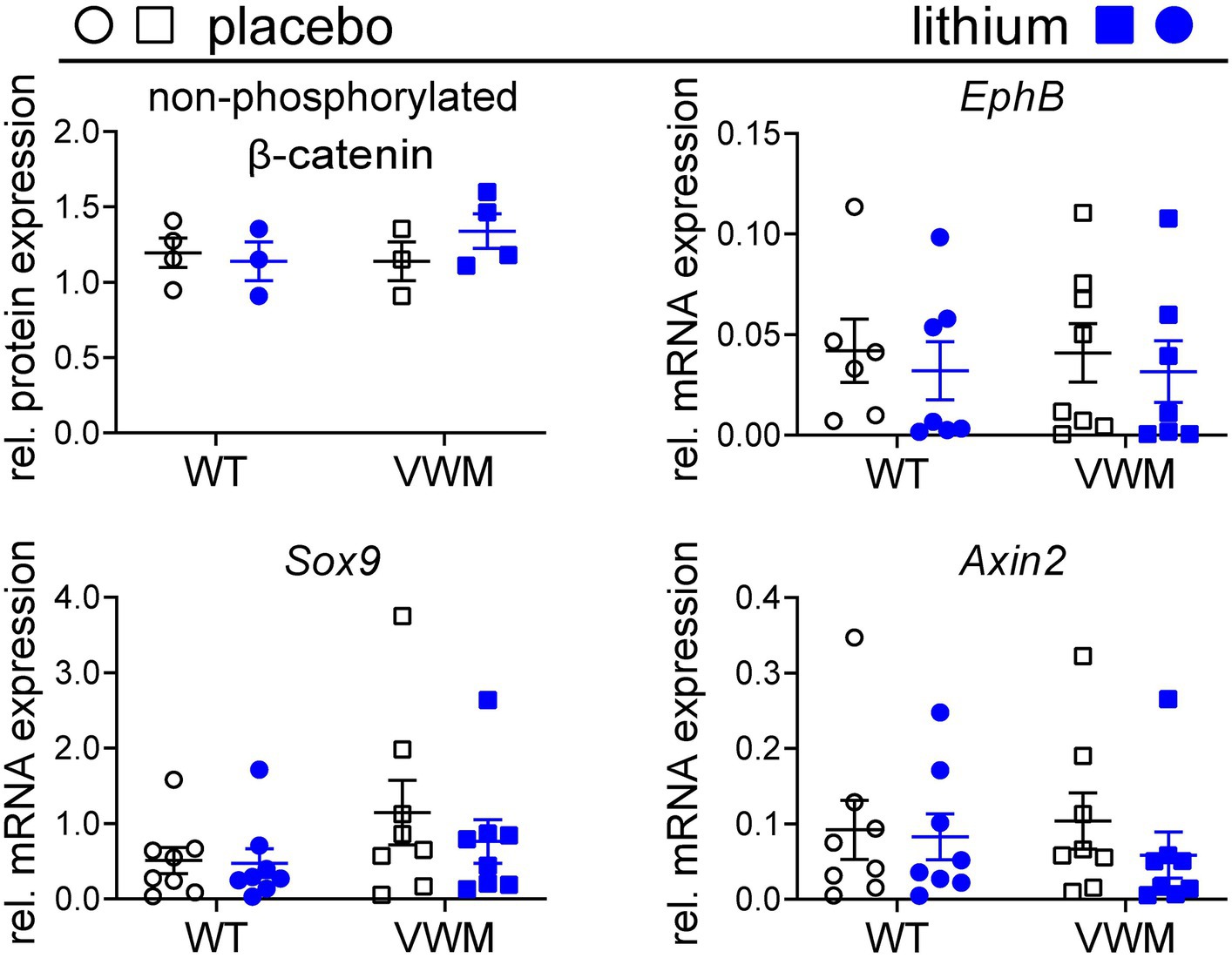
Figure 4. Lithium does not increase the expression and activity of non-phosphorylated β-catenin. WT (circles) and 2b4he2b5ho mutant (VWM; squares) mice (n = 8 per treatment, per genotype) were i.p. injected daily with placebo (black) or 200 mg/kg lithium (blue) for 1 week. Mice were terminated approximately 8 h after the final injection. Cerebellar RNA and protein samples were derived from the same lysate. Levels of non-phosphorylated β-catenin were determined using the Western blot (n = 3–4). Lithium treatment effects on indicated β-catenin-regulated markers were determined with qPCR (n = 6–8) (Sorcs1 and Nrxn as reference). Lithium differences were analyzed with two-way ANOVAs (Supplementary Data Sheet S1). The graphs show individual and mean values, +/− SEM. Raw data are available in Supplementary Data Sheet S2.
Lithium enhances the deregulated expression of some ATF4-regulated targets
Lithium-dependent effects on the ISR were investigated as a measure of ATF4 activity. eIF2α phosphorylation levels were lower, and ATF4-regulated mRNA levels were higher in the 2b4he2b5ho mutant than in the WT mouse brain (Abbink et al., 2019). Remarkably, lithium treatment somewhat increased eIF2α phosphorylation that was not statistically significant in the WT (+8%, p = 0.3193) or 2b4he2b5ho mutant mice (+21%, p = 0.1087) relative to the placebo treatment. Unexpectedly, the expression of ATF4-regulated mRNAs was higher in the lithium-treated than in placebo-treated 2b4he2b5ho mutant mice, although statistical significance was reached only for some (Figure 5; Supplementary Figures S1, S3; Supplementary Data Sheet S1). mRNA expression levels of Gadd34, Nupr, and Slc7a3 were notable for being increased by lithium treatment (+81%, p = 0.0035; +84%, p = 0.0524; +76%, p = 0.0041, respectively). A similar effect was observed in lithium-injected WT mice, in which statistical significance was reached for the lithium-induced Ddit3/Chop and Slc7a3 mRNA expression (+101%, p = 0.0071 and + 17%, p = 0.0403, respectively), and a statistical trend was observed for Gadd34 mRNA expression (+74%, p = 0.061).
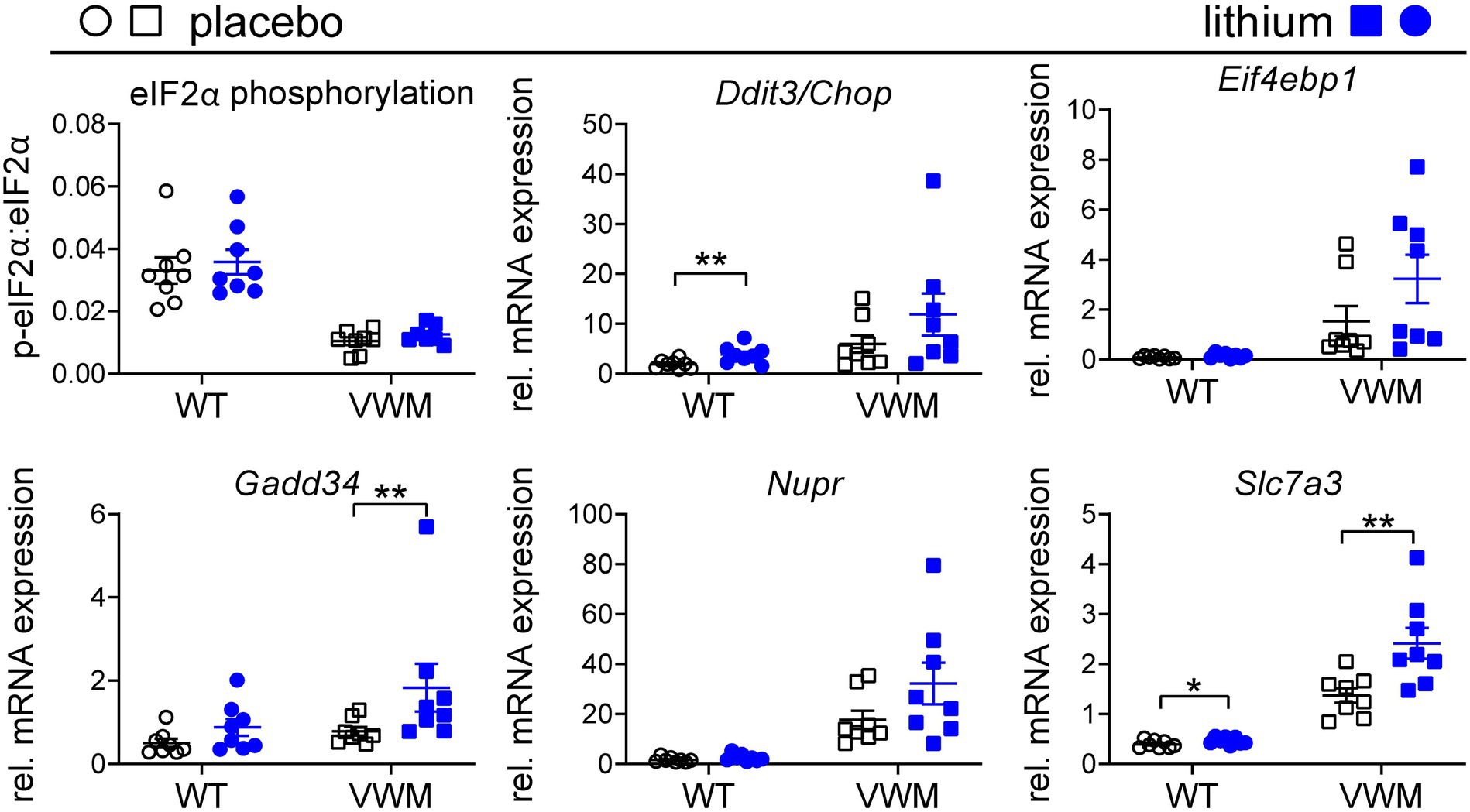
Figure 5. Lithium subtly increases the expression of the ATF4-regulated transcriptome in the mouse brain. WT (circles) and 2b4he2b5ho mutant (VWM; squares) mice were i.p. injected daily with 200 mg/kg lithium (blue) or placebo (black) for 1 week (n = 8 per treatment, per genotype). Mice were terminated approximately 8 h after injection. Cerebellar RNA and protein samples were derived from the same lysate. Lithium effects on indicated ISR markers were determined using the Western blot for eIF2α phosphorylation (n = 7–8) and qPCR for mRNA expression (Sorcs1 and Nrxn as reference, n = 8). The graphs show individual and mean values, +/− SEM. The shown ISR markers differ significantly in placebo-treated WT versus placebo-treated 2b4he2b5ho mice (p < 0.05; not indicated, Supplementary Data Sheet S1). Treatment effects were statistically analyzed using an unpaired t-test or Mann–Whitney test (VWM: Ddit3/Chop, Gadd34, Nupr; Supplementary Data Sheet S1). Raw data are available in Supplementary Data Sheet S2. *p < 0.05 and **p < 0.01.
Discussion
Mutant eif2b5 zebrafish and 2b4he2b5ho mice were subjected to lithium treatments, which were expected to inhibit GSK3β and consequently enhance eIF2B activity, resulting in improved disease signs. Mutant eif2b5 zebrafish displayed improved swimming behavior in response to lithium treatment. Lithium treatment induced the known side effects of polydipsia and polyuria in the WT and 2b4he2b5ho mutant mice indicating drug-induced nephrogenic diabetes insipidus (Bendz and Aurell, 1999). The lithium treatment regimen did, however, not show any clinical beneficial effects in 2b4he2b5ho mutant mice. The side effects limited our ability to increase the dose of lithium and prevented us from fully testing the efficacy of lithium in the VWM mice.
Curiously and contrary to our initial hypothesis and an earlier study (Sun et al., 2014), the brain tissue from the lithium-treated mice showed a somewhat enhanced expression of several ATF4-regulated transcripts. These transcript levels were affected by lithium in both WT and 2b4he2b5ho mutant mouse brains, although the effects differed for individual transcripts between genotypes. As ATF4 translation increases in response to reduced eIF2B activity (Harding et al., 2000a; Young and Wek, 2016), upregulation of ATF4-regulated targets suggests that lithium increased ATF4 translation and reduced eIF2B activity even further. Statistical analyses were hampered by the high degree of variation, especially in the lithium-treated VWM mice, obscuring the clear effects of lithium. Still, in the 2b4he2b5ho mutant mouse brains, ATF4-regulated targets were consistently upregulated by lithium. It is unlikely that the effect of lithium on the ATF4-regulated transcripts of astrocytes is masked by the effects on other cell types present in the brain tissue/cerebellum, such as oligodendrocytes, because the ATF4 and ATF4-regulated mRNAs are only detected in VWM astrocytes (Abbink et al., 2019). Lithium was not effective in increasing non-phosphorylated β-catenin protein levels in either WT or 2b4he2b5ho mutant mouse brain, which is a main effect downstream of GSK3β inhibition (Wu and Pan, 2010). In line with this negative finding and similar to other studies (Leroy et al., 2010; Del Grosso et al., 2022), increased lithium-induced β-catenin activity in the brain was not observed, and the expression of β-catenin-regulated mRNAs in the WT and 2b4he2b5ho mutant mouse brain was unaltered by lithium. The unchanged β-catenin activity in the brain suggests that GSK3β inhibition by lithium was negligible or temporary. Therefore, it remains an open question if the altered ATF4 expression in the 2b4he2b5ho mutant mouse brain was induced via lithium-dependent GSK3β inhibition or via an alternative route.
Limitations of this study include that lithium impacts multiple biochemical pathways, making the determination of the precise mechanism of action less certain. In addition, we were limited in assessing higher dosages of lithium in mice because of the observed toxicities. Although we did not measure lithium concentration in the brain or blood, the observed toxicities and increase in ATF4 activity show that lithium was active in the animals. Our experimental approach was to use zebrafish as a rapid and inexpensive screening platform, with subsequent validation and characterization in an established mouse model, representative of VWM.
The effects of lithium in the mutant eif2b5 zebrafish and the 2b4he2b5homouse model perhaps differ. In both animal models, the catalytic subunit eIF2Bε, the target of GSK3β, is mutant, although the exact mutation differs between the animal models. Further tests are needed to investigate whether the mutant eIF2B complex in the mutant eif2b5 zebrafish and 2b5he2b5ho mice is similarly under normal control of GSK3β activity. In addition, lithium effects were studied in female mice as a homogenous experimental group to reduce the number of animals needed for the experiment. To the best of our knowledge, sex-related differences in lithium brain concentrations or therapeutic effects have not been reported, suggesting that they are similar in VWM female and male mice (Pizzasegola et al., 2009; Can et al., 2011).
Lithium is a drug with a multitude of effects on many proteins and pathways (Hart, 1979; Roux and Dosseto, 2017). Possibly, lithium’s inhibitory effects on eIF2B activity occur via a GSK3β-independent mechanism. Lithium may have caused dehydration (Bendz and Aurell, 1999), which has been described to activate ATF4 (Greenwood et al., 2015). WT mice experienced more lithium-induced polydipsia than 2b4he2b5ho mutant mice. However, the levels of ATF4-regulated mRNAs increased more in the 2b4he2b5ho mutant than in the WT brain, and lithium had no impact on weight, arguing against dehydration. Another possible explanation for the negative results in mice is that the given dosage of 200 mg/kg lithium was not high enough to inhibit GSK3β in the mouse brains. In this study, higher lithium dosages were not pursued for three reasons. First, higher dosages may not be more effective as a lithium dosage of 200 mg/kg has been shown to be equally effective in antidepressant-like responses as compared to higher dosages in C57BL/6 J mice. Second, the current dosage already induced severe polydipsia. If a higher lithium dosage would be necessary for beneficial ISR effects, then translation to human VWM patients would most likely be difficult. VWM mainly presents in young children (Hamilton et al., 2018), and lithium has reported additional side effects in pediatric patients with bipolar disorders (Rosen, 2017). Third, lithium treatment actually increased the disease-associated ISR deregulation in the 2b4he2b5ho mutant mice, suggesting a potential risk for increasing the disease severity (Abbink et al., 2019; Wong et al., 2019). A recent study confirmed the idea that treatment with a high lithium dosage has deteriorating effects on motor skills (Li et al., 2020). Reducing the dosage would limit the observed toxicities but would not translate to the human therapeutic range and would be less likely to inhibit GSK3β than the current dosage of 200 mg/kg (Can et al., 2011). Considering lithium’s already well described and sometimes serious side effects in bipolar patients (Volkmann et al., 2020), the apparent lack of efficacy, and the evidence of potential ISR-activating action in 2b4he2b5ho mutant mice, it is not a drug of choice for further development in VWM patients.
Further research is needed to establish if GSK3β inhibition is a suitable strategy for attenuating the deregulated ISR in VWM. Highly specific and potent GSK3β inhibitors that cross the blood–brain barrier may warrant more studies and are expected to have less side effects than lithium (Meares et al., 2011). For example, the antidepressant trazodone has ISR-modulating properties, possibly by inhibiting GSK3β (Halliday et al., 2017; Pavitt, 2018). Other strategies for future investigations could include the use of antisense oligonucleotides to downregulate GSK3β expression including with specific CNS targeting by using intrathecal injections. In conclusion, the data show that lithium may not be a good therapeutic option but that GSK3β remains a target of interest to be investigated for VWM.
Data availability statement
The original contributions presented in the study are included in the article/Supplementary material, further inquiries can be directed to the corresponding author.
Ethics statement
Zebrafish experiments were performed in accordance of guidelines from the University of Utah Institutional Animal Care and Use Committee (IACUC), regulated under federal law (the Animal Welfare Act and Public Health Services Regulation Act) by the U.S. Department of Agriculture (USDA) and the Office of Laboratory Animal Welfare at the NIH, and accredited by the Association for Assessment and Accreditation of Laboratory Care International (AAALAC). All mouse experiments were carried out in compliance with the Dutch and European law and with approval of the local animal care and use committee of the VU University (license CCD AVD1140020172804, work protocol 2,804-NEU19-11). The study was conducted in accordance with the local legislation and institutional requirements.
Author contributions
DW: Formal analysis, Investigation, Methodology, Project administration, Visualization, Writing – original draft, Writing – review & editing. EO: Investigation, Methodology, Writing – review & editing. LH: Investigation, Methodology, Writing – review & editing. GH-A-N: Investigation, Methodology, Writing – review & editing. KG: Investigation, Methodology, Writing – review & editing. TS: Investigation, Methodology, Writing – review & editing. MH: Investigation, Methodology, Writing – review & editing. TA: Investigation, Methodology, Project administration, Supervision, Writing – original draft, Writing – review & editing. MK: Conceptualization, Funding acquisition, Supervision, Writing – review & editing. JB: Conceptualization, Funding acquisition, Investigation, Methodology, Supervision, Visualization, Writing – original draft, Writing – review & editing.
Funding
The author(s) declare financial support was received for the research, authorship, and/or publication of this article. This work was supported by the National Institutes of Health (grant number R33NS109441); the Bray Chair in Child Neurology Research at the University of Utah; and the Brain and Spine Center of Primary Children's Hospital. This study was supported by ZonMW TOP grant 91217006.
Acknowledgments
The authors thank Wouter Smit and Ruben de Boer (Tytgat Institute for Liver and Intestinal Research, Amsterdam UMC) for fruitful discussions during the preparation of the manuscript. The authors thank Joyce Bruijnsvoort for advice on the lithium solutions. The authors thank the animal caretakers of the VU-VUmc animal facility for mouse breeding and advice.
Conflict of interest
JB is on the board of wFluidx, Inc., owns stock in Orchard Therapeutics, and has consulted for Bluebird bio, Calico Life Sciences, Denali Therapeutics, Enzyvant, and Neurogene. MK and TEMA have a patent PCT/NL2018/050293 on guanabenz in VWM pending to VUmc.
The remaining authors declare that the research was conducted in the absence of any commercial or financial relationships that could be construed as a potential conflict of interest.
Publisher’s note
All claims expressed in this article are solely those of the authors and do not necessarily represent those of their affiliated organizations, or those of the publisher, the editors and the reviewers. Any product that may be evaluated in this article, or claim that may be made by its manufacturer, is not guaranteed or endorsed by the publisher.
Supplementary material
The Supplementary material for this article can be found online at: https://www.frontiersin.org/articles/10.3389/fnins.2024.1275744/full#supplementary-material
SUPPLEMENTARY DATA SHEET 1 | Statisitcal analysis.
SUPPLEMENTARY DATA SHEET 2 | qPCR primers.
SUPPLEMENTARY TABLE 1 | Raw data.
SUPPLEMENTARY IMAGE 1 | Subtle increase of the ATF4-regulated transcriptome in the mouse cerebellum by lithium.
SUPPLEMENTARY IMAGE 2 | Overview of gels and immunoblots used for the quantification of non-phosphorylated beta-catenin shown in figure 4.
SUPPLEMENTARY IMAGE 3 | Overview of gels and immunoblots used for the quantification of eIF2α phosphorylation shown in figure 5.
References
Abbink, T. E. M., Wisse, L. E., Jaku, E., Thiecke, M. J., Voltolini-González, D., Fritsen, H., et al. (2018). Integrated stress response deregulation underlies vanishing white matter and is a target for therapy. bioRxiv :460840. doi: 10.1101/460840
Abbink, T. E. M., Wisse, L. E., Jaku, E., Thiecke, M. J., Voltolini-Gonzalez, D., Fritsen, H., et al. (2019). Vanishing white matter: deregulated integrated stress response as therapy target. Ann. Clin. Transl. Neurol. 6, 1407–1422. doi: 10.1002/acn3.50826
Batlle, E., Henderson, J. T., Beghtel, H., Van Den Born, M. M., Sancho, E., Huls, G., et al. (2002). β-Catenin and Tcf mediate cell positioning in the intestinal epithelium by controlling the expression of EphB/ephrinB. Cell 111, 251–263. doi: 10.1016/S0092-8674(02)01015-2
Bendz, H., and Aurell, M. (1999). Drug-induced diabetes insipidus. Drug Saf. 21, 449–456. doi: 10.2165/00002018-199921060-00002
Beurel, E., Grieco, S. F., and Jope, R. S. (2015). Glycogen synthase kinase-3 (Gsk3): regulation, actions, and diseases. Pharmacol. Ther. 148, 114–131. doi: 10.1016/j.pharmthera.2014.11.016
Blache, P., Van De Wetering, M., Duluc, I., Domon, C., Berta, P., Freund, J.-N., et al. (2004). Sox9 is an intestine crypt transcription factor, is regulated by the Wnt pathway, and represses the Cdx2 and Muc2 genes. J. Cell Biol. 166, 37–47. doi: 10.1083/jcb.200311021
Bugiani, M., Boor, I., Van Kollenburg, B., Postma, N., Polder, E., Van Berkel, C., et al. (2011). Defective glial maturation in vanishing white matter disease. J. Neuropathol. Exp. Neurol. 70, 69–82. doi: 10.1097/NEN.0b013e318203ae74
Can, A., Blackwell, R. A., Piantadosi, S. C., Dao, D. T., O'donnell, K. C., and Gould, T. D. (2011). Antidepressant-like responses to lithium in genetically diverse mouse strains. Genes Brain Behav. 10, 434–443. doi: 10.1111/j.1601-183X.2011.00682.x
Clevers, H. (2006). Wnt/β-catenin signaling in development and disease. Cell 127, 469–480. doi: 10.1016/j.cell.2006.10.018
Del Grosso, A., Parlanti, G., Angella, L., Giordano, N., Tonazzini, I., Ottalagana, E., et al. (2022). Chronic lithium administration in a mouse model for Krabbe disease. JIMD Rep. 63, 50–65. doi: 10.1002/jmd2.12258
Doble, B. W., and Woodgett, J. R. (2003). Gsk-3: tricks of the trade for a multi-tasking kinase. J. Cell Sci. 116, 1175–1186. doi: 10.1242/jcs.00384
Dooves, S., Bugiani, M., Postma, N. L., Polder, E., Land, N., Horan, S. T., et al. (2016). Astrocytes are central in the pathomechanisms of vanishing white matter. J. Clin. Invest. 126, 1512–1524. doi: 10.1172/JCI83908
Fancy, S. P., Harrington, E. P., Yuen, T. J., Silbereis, J. C., Zhao, C., Baranzini, S. E., et al. (2011). Axin2 as regulatory and therapeutic target in newborn brain injury and remyelination. Nat. Neurosci. 14, 1009–1016. doi: 10.1038/nn.2855
Greenwood, M., Greenwood, M. P., Paton, J. F. R., and Murphy, D. (2015). Transcription factor Creb3L1 regulates endoplasmic reticulum stress response genes in the osmotically challenged rat hypothalamus. PLoS One 10:e0124956. doi: 10.1371/journal.pone.0124956
Halliday, M., Radford, H., Zents, K. A. M., Molloy, C., Moreno, J. A., Verity, N. C., et al. (2017). Repurposed drugs targeting eif2α-P-mediated translational repression prevent neurodegeneration in mice. Brain 140, 1768–1783. doi: 10.1093/brain/awx074
Hamilton, E. M. C., Van Der Lei, H. D. W., Vermeulen, G., Gerver, J. A. M., Lourenco, C. M., Naidu, S., et al. (2018). The natural history of vanishing white matter. Ann. Neurol. 84, 274–288. doi: 10.1002/ana.25287
Han, J., Back, S. H., Hur, J., Lin, Y.-H., Gildersleeve, R., Shan, J., et al. (2013). Er-stress-induced transcriptional regulation increases protein synthesis leading to cell death. Nat. Cell Biol. 15, 481–490. doi: 10.1038/ncb2738
Harding, H. P., Novoa, I., Zhang, Y., Zeng, H., Wek, R., Schapira, M., et al. (2000a). Regulated translation initiation controls stress-induced gene expression in mammalian cells. Mol. Cell 6, 1099–1108. doi: 10.1016/S1097-2765(00)00108-8
Harding, H. P., Zhang, Y., Bertolotti, A., Zeng, H., and Ron, D. (2000b). Perk is essential for translational regulation and cell survival during the unfolded protein response. Mol. Cell 5, 897–904. doi: 10.1016/S1097-2765(00)80330-5
Hart, D. (1979). Augmentation of zinc ion stimulation of lymphoid cells by calcium and lithium. Exp. Cell Res. 121, 419–425. doi: 10.1016/0014-4827(79)90024-7
Horzinski, L., Kantor, L., Huyghe, A., Schiffmann, R., Elroy-Stein, O., Boespflug-Tanguy, O., et al. (2010). Evaluation of the endoplasmic reticulum-stress response in eif2B-mutated lymphocytes and lymphoblasts from Cach/Vwm patients. BMC Neurol. 10:94. doi: 10.1186/1471-2377-10-94
Jho, E. H., Zhang, T., Domon, C., Joo, C. K., Freund, J. N., and Costantini, F. (2002). Wnt/beta-catenin/Tcf signaling induces the transcription of Axin2, a negative regulator of the signaling pathway. Mol. Cell. Biol. 22, 1172–1183. doi: 10.1128/MCB.22.4.1172-1183.2002
Keefe, M. D., Soderholm, H. E., Shih, H.-Y., Stevenson, T. J., Glaittli, K. A., Bowles, D. M., et al. (2020). Vanishing white matter disease expression of truncated Eif2B5 activates induced stress response. Elife 9:e56319. doi: 10.7554/eLife.56319
Kim, T. K. (2015). T test as a parametric statistic. Korean J. Anesthesiol. 68, 540–546. doi: 10.4097/kjae.2015.68.6.540
Konieczny, A., and Safer, B. (1983). Purification of the eukaryotic initiation factor 2-eukaryotic initiation factor 2B complex and characterization of its guanine nucleotide exchange activity during protein synthesis initiation. J. Biol. Chem. 258, 3402–3408. doi: 10.1016/S0021-9258(18)32875-8
Ladner, C. L., Yang, J., Turner, R. J., and Edwards, R. A. (2004). Visible fluorescent detection of proteins in polyacrylamide gels without staining. Anal. Biochem. 326, 13–20. doi: 10.1016/j.ab.2003.10.047
Le, V. H., Minh, T. N. T., Kha, Q. H., and Le, N. Q. K. (2023). A transfer learning approach on Mri-based radiomics signature for overall survival prediction of low-grade and high-grade gliomas. Med. Biol. Eng. Comput. 61, 2699–2712. doi: 10.1007/s11517-023-02875-2
Leroy, K., Ando, K., Héraud, C., Yilmaz, Z., Authelet, M., Boeynaems, J.-M., et al. (2010). Lithium treatment arrests the development of neurofibrillary tangles in mutant tau transgenic mice with advanced neurofibrillary pathology. J. Alzheimers Dis. 19, 705–719. doi: 10.3233/JAD-2010-1276
Li, M., Xia, M., Chen, W., Wang, J., Yin, Y., Guo, C., et al. (2020). Lithium treatment mitigates white matter injury after intracerebral hemorrhage through brain-derived neurotrophic factor signaling in mice. Transl. Res. 217, 61–74. doi: 10.1016/j.trsl.2019.12.006
Liu, D., Gao, L., Zhang, Z., Tao, S., Pang, Q., Li, A., et al. (2018). Lithium promotes the production of reactive oxygen species via Gsk-3β/Tsc2/Tor signaling in the gill of zebrafish (Danio rerio). Chemosphere 195, 854–863. doi: 10.1016/j.chemosphere.2017.12.130
Liu, R., Van Der Lei, H. D., Wang, X., Wortham, N. C., Tang, H., Van Berkel, C. G., et al. (2011). Severity of vanishing white matter disease does not correlate with deficits in eif2B activity or the integrity of eif2B complexes. Hum. Mutat. 32, 1036–1045. doi: 10.1002/humu.21535
Livak, K. J., and Schmittgen, T. D. (2001). Analysis of relative gene expression data using real-time quantitative Pcr and the 2−ΔΔct method. Methods 25, 402–408. doi: 10.1006/meth.2001.1262
Lu, P. D., Harding, H. P., and Ron, D. (2004). Translation reinitiation at alternative open reading frames regulates gene expression in an integrated stress response. J. Cell Biol. 167, 27–33. doi: 10.1083/jcb.200408003
Makoukji, J., Belle, M., Meffre, D., Stassart, R., Grenier, J., Shackleford, G., et al. (2012). Lithium enhances remyelination of peripheral nerves. Proc. Natl. Acad. Sci. U. S. A. 109, 3973–3978. doi: 10.1073/pnas.1121367109
Mcknight, P. E., and Najab, J. (2010) “Mann-Whitney U test” in The Corsini Encyclopedia of Psychology. (eds I.B. Weiner and W.E. Craighead).
Meares, G. P., Mines, M. A., Beurel, E., Eom, T.-Y., Song, L., Zmijewska, A. A., et al. (2011). Glycogen synthase kinase-3 regulates endoplasmic reticulum (Er) stress-induced Chop expression in neuronal cells. Exp. Cell Res. 317, 1621–1628. doi: 10.1016/j.yexcr.2011.02.012
Niehrs, C. (2012). The complex world of Wnt receptor signalling. Nat. Rev. Mol. Cell Biol. 13, 767–779. doi: 10.1038/nrm3470
O’brien, W. T., Harper, A. D., Jové, F., Woodgett, J. R., Maretto, S., Piccolo, S., et al. (2004). Glycogen synthase kinase-3β haploinsufficiency mimics the behavioral and molecular effects of lithium. J. Neurosci. 24, 6791–6798. doi: 10.1523/JNEUROSCI.4753-03.2004
Pakos-Zebrucka, K., Koryga, I., Mnich, K., Ljujic, M., Samali, A., and Gorman, A. M. (2016). The integrated stress response. EMBO Rep. 17, 1374–1395. doi: 10.15252/embr.201642195
Panza, A., Pazienza, V., Ripoli, M., Benegiamo, G., Gentile, A., Valvano, M. R., et al. (2013). Interplay between Sox9, β-catenin and Pparγ activation in colorectal cancer. Biochim Biophys Acta 1833, 1853–1865. doi: 10.1016/j.bbamcr.2013.04.004
Pap, M., and Cooper, G. M. (2002). Role of translation initiation factor 2B in control of cell survival by the phosphatidylinositol 3-kinase/Akt/glycogen synthase kinase 3β signaling pathway. Mol. Cell. Biol. 22, 578–586. doi: 10.1128/MCB.22.2.578-586.2002
Pasquali, L., Busceti, C. L., Fulceri, F., Paparelli, A., and Fornai, F. (2010). Intracellular pathways underlying the effects of lithium. Behav. Pharmacol. 21, 473–492. doi: 10.1097/FBP.0b013e32833da5da
Pavitt, G. D. (2005). eif2B, a mediator of general and gene-specific translational control. Biochem. Soc. Trans. 33, 1487–1492. doi: 10.1042/BST0331487
Pavitt, G. D. (2018). Regulation of translation initiation factor eif2B at the hub of the integrated stress response. Wiley Interdiscip Rev RNA 9:e1491. doi: 10.1002/wrna.1491
Pfaffl, M. W. (2001). A new mathematical model for relative quantification in real-time Rt-Pcr. Nucleic Acids Res. 29:e45, 45e–445e. doi: 10.1093/nar/29.9.e45
Pizzasegola, C., Caron, I., Daleno, C., Ronchi, A., Minoia, C., Carri, M. T., et al. (2009). Treatment with lithium carbonate does not improve disease progression in two different strains of Sod1 mutant mice. Amyotroph. Lateral Scler. 10, 221–228. doi: 10.1080/17482960902803440
Proud, C. G. (2001). Regulation of eukaryotic initiation factor eif2B. Prog. Mol. Subcell. Biol. 26, 95–114. doi: 10.1007/978-3-642-56688-2_4
Riedel, G., Rüdrich, U., Fekete-Drimusz, N., Manns, M. P., Vondran, F. W., and Bock, M. (2014). An extended Δct-method facilitating normalisation with multiple reference genes suited for quantitative Rt-Pcr analyses of human hepatocyte-like cells. PLoS One 9:e93031. doi: 10.1371/journal.pone.0093031
Rosen, M. S. (2017). Lithium in child and adolescent bipolar disorder. Am. J. Psych. Resid. J. 12, 3–5. doi: 10.1176/appi.ajp-rj.2017.120202
Roux, M., and Dosseto, A. (2017). From direct to indirect lithium targets: a comprehensive review of omics data. Metallomics 9, 1326–1351. doi: 10.1039/C7MT00203C
Ruijter, J. M., Thygesen, H. H., Schoneveld, O. J., Das, A. T., Berkhout, B., and Lamers, W. H. (2006). Factor correction as a tool to eliminate between-session variation in replicate experiments: application to molecular biology and retrovirology. Retrovirology 3:2. doi: 10.1186/1742-4690-3-2
Sansom, O. J., Reed, K. R., Hayes, A. J., Ireland, H., Brinkmann, H., Newton, I. P., et al. (2004). Loss of Apc in vivo immediately perturbs Wnt signaling, differentiation, and migration. Genes Dev. 18, 1385–1390. doi: 10.1101/gad.287404
Schmittgen, T. D., and Livak, K. J. (2008). Analyzing real-time Pcr data by the comparative Ct method. Nat. Protoc. 3, 1101–1108. doi: 10.1038/nprot.2008.73
Shorter, E. (2009). The history of lithium therapy. Bipolar Disord. 11, 4–9. doi: 10.1111/j.1399-5618.2009.00706.x
Stambolic, V., Ruel, L., and Woodgett, J. R. (1996). Lithium inhibits glycogen synthase kinase-3 activity and mimics wingless signalling in intact cells. Curr. Biol. 6, 1664–1669. doi: 10.1016/S0960-9822(02)70790-2
Stamos, J. L., and Weis, W. I. (2013). The β-catenin destruction complex. Cold Spring Harb. Perspect. Biol. 5:a007898. doi: 10.1101/cshperspect.a007898
Sun, X.-B., Lu, H.-E., Chen, Y., Fan, X.-H., and Tong, B. (2014). Effect of lithium chloride on endoplasmic reticulum stress-related perk/rock signaling in a rat model of glaucoma. Pharmazie 69, 889–893. doi: 10.1691/ph.2014.4672
Tran, T. N., Thi Dang, T. H., Thai, T. T., Le, H. T., Nguyen, T. T. T., Nguyen, H. T., et al. (2023). Development and validation of the Vietnamese smell identification test. Parkinsonism Relat. Disord. 113:105494. doi: 10.1016/j.parkreldis.2023.105494
Valvezan, A. J., Zhang, F., Diehl, J. A., and Klein, P. S. (2012). Adenomatous polyposis coli (Apc) regulates multiple signaling pathways by enhancing glycogen synthase kinase-3 (Gsk-3) activity. J. Biol. Chem. 287, 3823–3832. doi: 10.1074/jbc.M111.323337
Van Der Knaap, M. S., Leegwater, P. A., Konst, A. A., Visser, A., Naidu, S., Oudejans, C. B., et al. (2002). Mutations in each of the five subunits of translation initiation factor eif2B can cause leukoencephalopathy with vanishing white matter. Ann. Neurol. 51, 264–270. doi: 10.1002/ana.10112
Van Kollenburg, B., Thomas, A. A., Vermeulen, G., Bertrand, G. A., Van Berkel, C. G., Pronk, J. C., et al. (2006). Regulation of protein synthesis in lymphoblasts from vanishing white matter patients. Neurobiol. Dis. 21, 496–504. doi: 10.1016/j.nbd.2005.08.009
Volkmann, C., Bschor, T., and Köhler, S. (2020). Lithium treatment over the lifespan in bipolar disorders. Front. Psych. 11:377.
Welsh, G. I., Miller, C. M., Loughlin, A. J., Price, N. T., and Proud, C. G. (1998). Regulation of eukaryotic initiation factor eif2B: glycogen synthase kinase-3 phosphorylates a conserved serine which undergoes dephosphorylation in response to insulin. FEBS Lett. 421, 125–130. doi: 10.1016/S0014-5793(97)01548-2
Wisse, L. E., Penning, R., Zaal, E. A., Van Berkel, C. G. M., Ter Braak, T. J., Polder, E., et al. (2017). Proteomic and metabolomic analyses of vanishing white matter mouse astrocytes reveal deregulation of Er functions. Front. Cell. Neurosci. 11:411. doi: 10.3389/fncel.2017.00411
Witkamp, D., Oudejans, E., Hu-A-Ng, G. V., Hoogterp, L., Krzywanska, A. M., Znidarsic, M., et al. (2022). Guanabenz ameliorates disease in vanishing white matter mice in contrast to sephin1. Ann. Clin. Transl. Neurol. 9, 1147–1162. doi: 10.1002/acn3.51611
Wong, Y. L., Lebon, L., Basso, A. M., Kohlhaas, K. L., Nikkel, A. L., Robb, H. M., et al. (2019). eif2B activator prevents neurological defects caused by a chronic integrated stress response. Elife 8:e42940. doi: 10.7554/eLife.42940
Wortham, N. C., Martinez, M., Gordiyenko, Y., Robinson, C. V., and Proud, C. G. (2014). Analysis of the subunit organization of the eif2B complex reveals new insights into its structure and regulation. FASEB J. 28, 2225–2237. doi: 10.1096/fj.13-243329
Wu, D., and Pan, W. (2010). Gsk3: a multifaceted kinase in Wnt signaling. Trends Biochem. Sci. 35, 161–168. doi: 10.1016/j.tibs.2009.10.002
Keywords: lithium, vanishing white matter, integrated stress response, GSK3β, ATF4
Citation: Witkamp D, Oudejans E, Hoogterp L, Hu-A-Ng GV, Glaittli KA, Stevenson TJ, Huijsmans M, Abbink TEM, van der Knaap MS and Bonkowsky JL (2024) Lithium: effects in animal models of vanishing white matter are not promising. Front. Neurosci. 18:1275744. doi: 10.3389/fnins.2024.1275744
Edited by:
Rachita Yadav, Massachusetts General Hospital and Harvard Medical School, United StatesReviewed by:
Celine De Esch, Massachusetts General Hospital and Harvard Medical School, United StatesGuohua Wang, Nantong University, China
Nguyen Quoc Khanh Le, Taipei Medical University, Taiwan
Copyright © 2024 Witkamp, Oudejans, Hoogterp, Hu-A-Ng, Glaittli, Stevenson, Huijsmans, Abbink, van der Knaap and Bonkowsky. This is an open-access article distributed under the terms of the Creative Commons Attribution License (CC BY). The use, distribution or reproduction in other forums is permitted, provided the original author(s) and the copyright owner(s) are credited and that the original publication in this journal is cited, in accordance with accepted academic practice. No use, distribution or reproduction is permitted which does not comply with these terms.
*Correspondence: Marjo S. van der Knaap, bXMudmFuZGVya25hYXBAYW1zdGVyZGFtdW1jLm5s
†These authors share senior authorship