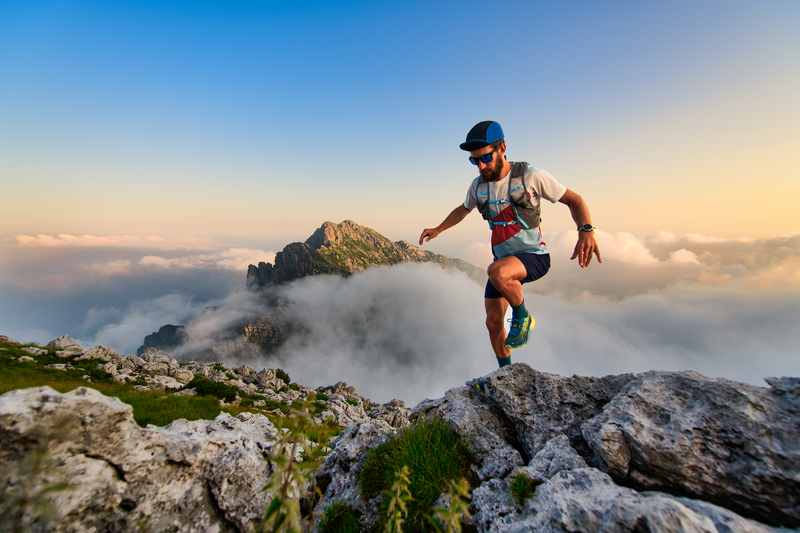
95% of researchers rate our articles as excellent or good
Learn more about the work of our research integrity team to safeguard the quality of each article we publish.
Find out more
ORIGINAL RESEARCH article
Front. Neurosci. , 28 February 2023
Sec. Sleep and Circadian Rhythms
Volume 17 - 2023 | https://doi.org/10.3389/fnins.2023.989512
This article is part of the Research Topic Assessing Sleep Neuroplasticity in Pathological Conditions and in Extreme Environments Through Neurophysiological and Multi-faceted Daily Lifestyle Patterns View all 8 articles
Introduction: Many studies have provided evidence of a damage effect triggered by total sleep deprivation (TSD). However, it remains unclear whether the motor preparation processing is affected by TSD.
Methods: In the current study, 23 volunteers performed a stimulus-response compatibility visual search task before and after TSD while undergoing spontaneous electroencephalography (EEG).
Results: Repeated-measures analysis of variance revealed that: Compared with that at baseline, the visual search task’s accuracy decreased after TSD, while the response time variance increased significantly. The peak amplitude of the stimulus-locked lateralized readiness potential (LRP) induced by a compatible stimulus was significantly more negative than that induced by an incompatible stimulus before TSD, whereas this difference was not significant after TSD. However, when taking sleep status into consideration, there were no significant main or interaction effects on response-locked LRPs.
Discussion: Our findings suggest that TSD damages visual search behavior, selectively impairs the earlier sub-stages of motor preparation (sensory integration). These findings will provide a new perspective for understanding the effects of sleep loss.
There is increasing evidence that sleep influences the risk of cardiovascular diseases (St-Onge and Zuraikat, 2019). The development of smart devices has altered leisure-time activities and work schedules (Chang et al., 2015), and this has contributed to the growth of the sleep-restricted population. Therefore, exploring how sleep deprivation influences human cognitive functions is critical for preventing its deleterious effects. In numerous studies, shortened sleep is operationalized as total sleep deprivation (TSD), which is a useful metric that comprehensively examines how shortened sleep jeopardizes cognition (Lowe et al., 2017). Previous studies have suggested that TSD impairs different levels of cognitive function; for example, attention performance (measured using vigilant attention and psychomotor vigilance tasks) is significantly weakened by TSD (Lim and Dinges, 2008; McMahon et al., 2018; Gibbings et al., 2020; Stepan et al., 2020). TSD also impairs higher-order cognitive processes such as executive functions (Aidman et al., 2018; Honn and Hinson, 2019), long-term memory (Ratcliff et al., 2018), risky decision-making (Acheson et al., 2007), emotion processing (Kahn et al., 2013; Ben et al., 2020), even social cognitive abilities (Boardman et al., 2017; Deliens and Bukowski, 2018). In addition, studies from animal models have demonstrated that TSD affects various cognitive functions (e.g., Kumar and Jha, 2012; Qureshi and Jha, 2017).
The mechanisms underlying TSD-induced dysfunctions have been explored to a certain degree using cognitive neuroscience technologies. Numerous studies have shown that the reduced activity in the dorsolateral prefrontal and parietal cortexes is correlated with detrimental effects on working memory (Chee and Choo, 2004; Habeck et al., 2004; Choo et al., 2005; Chee and Chuah, 2007; Lythe et al., 2012). Using a stop-signal task with simultaneous electroencephalography (EEG), Kusztor et al. (2019) identified three subcomponents of cognitive control (sustained attention, automatic bottom-up processing, and strategic top-down control) and found that TSD triggered a decline in sustained attention and reduced P3 and P-e amplitudes, suggesting a progressive impairment in top-down control rather than in the other two subcomponents. Trujillo et al. (2009) examined TSD-induced deficits in exogenous and endogenous attention using an adapted attention network test. They demonstrated that TSD affected the early stage, which was indexed through the N1 component, of endogenous attention, whereas the early stage of exogenous attention processes showed a less significant effect (Trujillo et al., 2009). Previous studies had also made an exploration of the relationship between sleep-associated oscillating waves and cognitive function, including both human studies (e.g., Gibbings et al., 2020; Zhang et al., 2023) and animal models (e.g., Tripathi et al., 2018).
While a large amount of research has focused on the neural correlates of the alterations brought about by TSD, there have been almost no investigations of the neural processes involved in motor preparation to date, despite these processes being highly relevant to human reactions. The lateralized readiness potential (LRP) is an event-related potential (ERP) that reflects the activation of the contralateral motor cortex (M1) during voluntary movement (Shibasaki and Hallett, 2006; Smulders and Miller, 2012). A large amount of evidence from physiological studies unambiguously indicates that M1 is the principal generator of LRPs (De Jong et al., 1988; Coles, 1989). LRPs are widely used in many fields of research to assess the processes of motor preparation and execution (Smulders and Miller, 2012; Debnath and Franz, 2016; Jost et al., 2017; Dayan-Riva et al., 2021; Morand-Beaulieu et al., 2021). The LRPs can be divided into two categories: stimulus-locked LRP (s-LRP) reflects sensory integration, while response-locked LRP (r-LRP) is thought to be related to the subsequent processes involved in response execution (Mordkoff and Gianaros, 2000; Rinkenauer et al., 2004; Smulders and Miller, 2012). The sub-stages of motor preparation that are affected by other variables can be accurately identified (e.g., Cheval et al., 2018; Van Voorhis et al., 2019; Qian and Gao, 2021).
Although a large number of studies in the field of cognitive neuroscience of shortened sleep used the ERP technique, there has been almost no research on motor preparation and LRPs. Only Stojanoski et al. (2019) investigated the impact of reduced vigilance using the psychomotor vigilance task following at-home mild sleep restriction. They found that mild sleep restriction reduced the amplitude of LRPs, which indicates that it can negatively affect motor preparation and execution. However, the most serious flaw of their study was that all responses were made with the right hand; in other words, the handedness variable rendered their findings ambiguous. Additionally, the electrodes used to extract the characteristics of LRPs were not as precise as standard paradigms, and the two sub-stages of LRPs were not classified. In this study, we addressed these shortcomings. In the current study, we employed the LRP index to determine whether TSD influences motor preparation processes and to further investigate which sub-stage of motor preparation is influenced by TSD. When responses are made with both hands, the LRPs are easily observed in a stimulus-response compatibility task (Gorman Bozorgpour et al., 2013; Van Voorhis et al., 2019; Nayak et al., 2020; Dayan-Riva et al., 2021; Morand-Beaulieu et al., 2021). Given that a systematic study by Clark et al. (2015) in which various ERP components were measured found that the “visual search task” was suitable for analyzing the two types of LRPs, we employed this method in the current study to analyze these potentials. In the current study, we designed the present study to quantify the LRPs evoked by a stimulus-response compatibility visual search task and explore whether TSD damages the motor preparation process. We hypothesized that: (i) TSD would affect motor preparation function; (ii) TSD impaired two sub-stages of motor preparation (sensory integration and response execution).
Twenty-four young male volunteers (22.91 ± 2.26 years) who were healthy university students in Beijing participated in this study. All were right-handed, had normal or corrected vision, and had no history of neurological or mental disorders. The experimental protocol was approved by the Ethics Committee of Beihang University (approval number BM20180040). All participants maintained healthy sleep habits (Pittsburgh Sleep Quality Index score < 5) (Buysse et al., 1989). The participants were instructed to ensure adequate sleep (7–9 h every day) for half a month before the beginning of the TSD experiment. All participants declared that they did not have a habit of smoking cigarettes, that they did not drink alcohol or coffee, and that they had not taken any medications within 48 h before the experiment. Each participant signed an informed consent form before the beginning of the experiment.
The stimulus sequence for the visual search task is shown in Figure 1. A circular search array consisted of 12 items (1.7°× 1.7°, 50% contrast, 13.5 cd/m2), 11 diamonds, and one circle (target) positioned on a black background. The target was randomly located at either 2/4 o’clock (right) or 8/10 o’clock (left) with equal probability. All subjects were instructed to maintain their gaze at the center of the screen, respond to the position of the target (upper or lower), and ignore other extraneous items. Participants assigned odd numbers pressed the F key with their left hands for the upper stimulus and the J key with their right hands for the lower stimulus; those assigned even numbers followed these rules in reverse. The combination of the position of the target (right or left) and the responding hand produced stimuli that were either compatible (right-right, left-left) or incompatible (left-right, right-left). Each trial started with a fixation cross displayed for 900–1,100 ms randomly, followed by the stimulus array for 200 ms, and a blank screen for 2,300 ms; participants responded during the blank screen interval. The experiment consisted of two 120-trial blocks and lasted approximately 10 min. The design of the visual search task was based on previous studies by Sun et al. (2018) and Wang et al. (2016). All the participants completed the task using the same computer.
The experimental procedure is illustrated in Figure 2. Two participants conducted the experiment simultaneously. The participants arrived at the laboratory the day before the experiment and slept there that night to ensure adequate sleep (i.e., more than 8 h). Before commencing the experiment, all participants performed 20 trial runs of the visual search task to ensure that they had mastered the task’s requirements. The following day, the participants completed the visual search task with simultaneous EEG recordings (baseline readings) at 8:00 am. After these baseline recordings were obtained, the participants stayed in the laboratory for the entire duration of their sleep deprivation; they were offered refreshments and were permitted to play games and watch movies. After 36 h of TSD, they completed the same task while again subject to simultaneous EEG recordings (TSD readings). During each EEG recording, the two participants completed the visual search task separately in randomized order. The participants were prevented from taking any drugs/stimulating agents during the TSD period. At least two paramedics accompanied and observed the participants and reminded them to remain awake throughout the entire experiment.
Three behavioral performance measures during the visual search task were recorded for analysis under both baseline and TSD conditions. Trials with error responses and those with response times (RT) less than 200 ms or more than 2,000 ms were excluded from the behavioral and subsequent LRP data analyses. The accuracy was calculated as the number of correct trials meeting the RT range divided by the total number of trials. The RT was the average reaction time of all correct trials meeting the RT range. The RTSD was calculated as the variance of RT. One participant’s behavioral performance was negative (accuracy = 14.58%), and his data were therefore excluded from the analysis as well. Descriptive statistics are presented as means ± standard deviations. Repeated-measures analysis of variance (ANOVA) was used to evaluate accuracy and RT. The main effects and interactions between sleep conditions (baseline and TSD) and stimulus types (compatible and incompatible) were also analyzed. The estimates of effect size were reported as partial η2 or Cohen’s d, and the statistical power was estimated as power. These analyses were performed using SPSS Statistics for Windows, version 22.0 (IBM Corp., Armonk, NY, USA).
Continuous EEG was recorded from an elastic cap with 30 electrodes that were mounted according to the 10–20 system standard positions using a SynAmps2 amplifier (Compumedics Neuroscan, Victoria, Australia). The online sampling rate was 1,000 Hz, and the impedance of each electrode was reduced and maintained below 5 kΩ. The vertical eye movements were monitored with two electrodes placed 10 mm above and below the left eye, whereas the horizontal eye movements were monitored with two electrodes placed on the left and right temples. The reference electrodes were placed bilaterally on the mastoids.
Raw EEG data were preprocessed offline using MATLAB R2017a (The MathWorks, Inc., Natick, MA, United States)1 that incorporated the EEGLAB2020_0 toolbox2 (Delorme and Makeig, 2004). The offline sampling rate was reduced to 250 Hz, and the average reference was used for re-referencing. A band-pass filter of 0.1–30 Hz was used by a 6th order Butterworth filter with a frequency slope of 36 dB/oct via the ERPLAB plugin3 (Lopez-Calderon and Luck, 2014). After independent component analysis, elements symbolizing eye movement and inordinate muscle activity were identified using two auxiliary plugins for artifact recognition, ICLabel4 (Pion-Tonachini et al., 2019) and Adjust5 (Mognon et al., 2011), and were removed manually. Epochs with a length of 600 ms ranging from −200 to 400 ms with respect to the onset of the stimuli as well as those with a length of 600 ms ranging from −500 to 100 ms with respect to the onset of the motor response were then extracted from the continuous EEG data to determine the s-LRP and r-LRP, respectively. The s-LRP was baseline-corrected in the range of −200 ms to 0 ms before stimulus onset, while the r-LRP was baseline-corrected in the range of −500 to −300 ms before response onset. The baseline correction was performed by subtracting the mean activity during the corresponding baseline period from the segmented data. The trials with voltages exceeding ± 75 μV in any channel were detected using a sliding time window with a length of 200 ms, and were excluded from the ERP grand average automatically via the ERPLAB plugin (see text footnote 3; Lopez-Calderon and Luck, 2014). One participant’s ERPs data were excluded from the analysis because of the tiny ratio of valid to invalid epochs (i.e., less than one). According to Luck (2005), the numbers of included trials under the two sleep conditions were sufficient to produce stable results and draw robust conclusions.
The characteristics of LRPs were extracted using the ERPLAB plugin (see text footnote 3; Lopez-Calderon and Luck, 2014) after averaging and calculating data from only the corrected responses post-screening. The LRPs are calculated as the difference in amplitude between the contralateral and ipsilateral electrodes located near M1 to the responding hand (Slobounov, 2010). The C3 and C4 electrodes were targeted to calculate LRPs using the averaging method LRP = (mean [C4-C3]left hand + mean [C3-C4]right hand)/2 (Coles, 1989). We chose the time windows for the two types of LRP in this study based on the definition of LRPs (Mordkoff and Gianaros, 2000; Rinkenauer et al., 2004; Smulders and Miller, 2012) and the waveforms we obtained. The peak amplitude and onset latency of s-LRP were measured from 200 to 350 ms as governed by the onset of the stimuli, while the peak amplitude and onset latency of r-LRP were measured from −200 to 0 ms as governed by the onset of the motor response. A 2 (sleep conditions: baseline, TSD) × 2 (stimulus types: compatible, incompatible) repeated-measures ANOVA was used to analyze the peak amplitudes of the LRP components. The main effects and interaction effects between sleep conditions and stimulus types were analyzed separately. The estimates of effect size are reported as partial η2 or Cohen’s d. A jackknife-based method was applied to extract the onset latencies of the LRPs (Miller et al., 1998). Twenty-one subsamples of grand average LRPs were calculated by excluding the LRP data of a different participant under each sleep condition. The onset latencies of the LRPs were defined as 50% of the peak amplitude. Statistical analyses were conducted using the corrected F-values approach (Fc = F/[n−1]2) or corrected t-values approach (tc = t/[n−1]), where Fc and tc denote the corrected values and n denotes the number of participants (Kiesel et al., 2008). These analyses were performed using the SPSS software.
The means and standard deviations of the behavioral performance measures (accuracy, RT, and RTSD) are shown in Table 1.
Table 1. Means and standard deviations of various measures of behavioral performance and lateralized readiness potentials.
As shown in Figure 3A, repeated-measures ANOVA revealed that the interaction effect between sleep status and stimulus types was significant [F(1,22) = 4.37, p = 0.048, partial η2 = 0.17, power = 0.52], suggesting that the accuracy of different stimulus types differed across sleep conditions. The simple effect analysis showed that the accuracies of both compatible (t22 = 2.62, p = 0.008, Cohen’s d = 0.58, power = 0.90) and incompatible (t22 = 3.29, p = 0.004, Cohen’s d = 0.69, power = 0.99) stimuli were higher at baseline than those after TSD. The main effect of sleep status was significant [F(1,22) = 12.06, p = 0.002, partial η2 = 0.36, power = 0.91], which indicated that TSD affected accuracy, that is, the accuracy was higher at baseline (0.94 ± 0.07) than after TSD (0.89 ± 0.09). The stimulus types also played a significant role [F(1,22) = 40.51, p < 0.001, partial η2 = 0.65, power = 1.00], suggesting that the accuracy of the compatible stimulus (0.96 ± 0.05) was higher than that of the incompatible stimulus (0.87 ± 0.09).
Figure 3. Behavioral results of ACC (A), RT (B), RTSD (C) in the stimulus-response compatibility visual search task after a full night’s sleep (at baseline) versus after total sleep deprivation (TSD). ACC, accuracy; RT, response time; RTSD, response time variance; BS, at baseline; SD, after total sleep deprivation; *p < 0.05; **p < 0.01; ***p < 0.001; n.s., not significant.
As shown in Figure 3B, repeated-measures ANOVA of RT revealed a significant effect of stimulus types [F(1,22) = 85.01, p < 0.001, partial η2 = 0.79, power = 1.00]; that is, the RT of the compatible stimulus (485.05 ± 82.89 ms) was faster than that of the incompatible stimulus (541.30 ± 78.30 ms). In terms of sleep conditions, the main effect was not significant [F(1,22) = 0.22, p = 0.642, partial η2 = 0.01, power = 0.07]. The interaction effect between sleep status and stimulus types was not significant [F(1,22) = 0.02, p = 0.886, partial η2 = 0, power = 0.05] as well.
As shown in Figure 3C, repeated-measures ANOVA revealed that sleep status significantly affected RTSD [F(1,22) = 4.66, p = 0.042, partial η2 = 0.18, power = 0.54]; participants exhibited more variability after TSD (126.45 ± 48.52 ms) than at baseline (107.25 ± 32.52 ms). The type of stimulus also had a significant effect [F(1,22) = 6.74, p = 0.017, partial η2 = 0.23, power = 0.70], as the RTSD of the incompatible stimulus (122.14 ± 46.21 ms) was greater than that of the compatible stimulus (111.58 ± 37.48 ms). The interaction effect between sleep status and stimulus types did not meet the threshold for significance [F(1,22) = 3.64, p = 0.070, partial η2 = 0.14, power = 0.45].
The means and standard deviations of LRP characteristics (peak amplitude and onset latency) are shown in Table 1.
The polarity of the s-LRP induced by the incompatible stimulus was the inverse of that induced by the compatible stimulus; moreover, the polarity of the s-LRP induced by the incompatible stimulus the inverse of that of the r-LRP. These results were consistent with the notion that lateralized sensory potentials are one of the sources of LRP when stimuli were presented to the left or the right of the participant’s midline (Smulders and Miller, 2012). This in turn indicated that the s-LRP reflected sensory integration (Mordkoff and Gianaros, 2000; Rinkenauer et al., 2004). To compare the peak amplitudes and onset latency of the s-LRP between the two sleep conditions, we inverted the polarity; that is, we calculated the s-LRP using the contralateral electrodes of the visual field, where incompatible stimuli appear, rather than the responding hands.
The grand-average waves of s-LRP waves were present in Figure 4A.
Figure 4. Grand average waves of the s-LRP (A) and r-LRP (B) induced by the target stimulus of the stimulus-response compatibility visual search task after a full night’s sleep (at baseline) versus after total sleep deprivation (TSD). The polarity of the s-LRP induced by the incompatible stimulus was the inverse of that induced by the compatible stimulus; therefore, we inverted the polarity to compare the peak amplitudes and onset latencies of the s-LRPs between the two sleep conditions. s-LRP, stimulus-locked lateralized readiness potential; r-LRP, response-locked lateralized readiness potential; BS, at baseline; SD, after total sleep deprivation.
Repeated-measures ANOVA of peak amplitudes of the s-LRP components revealed that the interaction effect between sleep status and stimulus types was significant [F(1,21) = 4.60, p = 0.044, partial η2 = 0.18, power = 0.54], suggesting that the peak amplitudes of the s-LRP triggered by the two types of stimuli differed across sleep conditions. The simple effect analysis showed that the peak amplitude of the s-LRP induced by a compatible stimulus was significantly more negative than that induced by an incompatible stimulus before TSD (t21 = 2.24, p = 0.036, Cohen’s d = 0.48, power = 0.69), whereas this difference was not significant after TSD (t21 = 0.77, p = 0.451, Cohen’s d = 0.16, power = 0.11). The main effects of stimulus types [F(1,21) = 1.24, p = 0.278, partial η2 = 0.06, power = 0.19] and sleep status [F(1,21) = 2.55, p = 0.125, partial η2 = 0.11, power = 0.33] were not significant.
Repeated-measures ANOVA of onset latency in the s-LRP components found no significant main effects of sleep status [Fc(1,21) = 0.18, p = 0.673], stimulus types [Fc(1,21) = 0.21, p = 0.653], or the interaction effect between them [Fc(1,21) = 0.40, p = 0.534].
The grand-average waves of r-LRP were presented in Figure 4B.
Repeated-measures ANOVA of peak amplitudes of the r-LRP components revealed that the main effects of stimulus types [F(1,21) = 7.87, p = 0.011, partial η2 = 0.27, power = 0.76] was significant, suggesting that the peak amplitudes of the r-LRP components triggered by a compatible stimulus (−3.27 ± 2.84 μV) was more negative than that triggered by an incompatible stimulus (−1.46 ± 1.73 μV). Otherwise, there was no significant effect of sleep status [F(1,21) < 0.01, p = 0.966, partial η2 = 0, power = 0.05] or interaction between sleep status and stimulus types [F(1,21) = 1.66, p = 0.212, partial η2 = 0.07, power = 0.23].
Repeated-measures ANOVA of the onset latency of the r-LRP components revealed that the main effects of stimulus types [Fc(1,21) = 14.48, p = 0.001] was significant, suggesting that the onset latency of the r-LRP induced by a compatible stimulus (−181.55 ± 5.8 ms) was earlier than that induced by an incompatible stimulus (−106.91 ± 5.21 ms) when combining the two sleep conditions. The main effects of sleep status [Fc(1,21) = 0.01, p = 0.939] and the interaction effect between sleep status and stimulus types [Fc(1,21) = 0.13, p = 0.719] were not significant.
We applied a visual search task that included stimulus-response compatibility to observe the processing of motor preparation by LRPs. Our most important finding was the selective impairments on the two types of LRP, which fully supported hypothesis (i) and partially supported hypothesis (ii). The s-LRP, which is related to the earlier sub-stage of motor preparation (sensory integration), was sensitive to sleep deprivation, while the r-LRP, which is related to the later sub-stage of motor preparation (response execution), was not.
In the current study, TSD decreased the accuracy of the visual search task while increasing the RT variance; this suggested that participants manipulated the visual search task less accurately and with less stability after TSD. These behavioral findings are consistent with those of previous studies, many of which have shown that TSD weakens attention performance (Lim and Dinges, 2008; McMahon et al., 2018; Gibbings et al., 2020; Stepan et al., 2020) and impairs higher-order cognitive processes that are based on attention (such as long-term memory) (Ratcliff et al., 2018). Many studies have suggested that executive function is impaired by TSD (Aidman et al., 2018; Honn and Hinson, 2019). It is more difficult for participants to respond to incompatible stimuli than to compatible stimuli; the cognitive processing of incompatible stimuli include additional cognitive functions such as conflict monitoring and inhibitory control, which are important for central executive functions.
The amplitudes of the LRPs reflect the intensity of neural activity in the M1 area. In terms of the peak amplitudes of the s-LRP components, the significant interaction effect between sleep status and stimulus types indicated that TSD damages the processing of sensory integration. Before TSD, the s-LRP induced by a compatible stimulus was more negative than that induced by an incompatible stimulus. The incompatible stimulus was more complicated than the compatible stimulus. For incompatible stimulus, the sensory integration processes included additional cognitive functions such as conflict monitoring and inhibitory control, which required both contralateral and ipsilateral brain activity. This was not the case with a compatible stimulus. When extracting the amplitude of s-LRP using a subtractive formula, the difference between s-LRPs induced by the two types of stimuli before TSD was pronounced. After TSD, however, the attention was thoroughly weakened to the extent that this difference disappeared. For peak r-LRP component amplitudes, the lack of a significant effect of sleep status as well as the interaction effect between sleep status and stimulus types suggested that TSD seldom affected the later sub-stage of motor preparation (response execution).
The onset latency of LRPs reflects the relative onset time of neural activity in the M1 area. There was no significant difference between the onset latency of s-LRP evoked by two types of stimuli at baseline or after TSD, suggesting that there were no obvious TSD-induced setbacks that would delay the relative onset time of the earlier sub-stage of motor preparation. In the later sub-stage of motor preparation, the onset latency of r-LRP induced by an incompatible stimulus was slower than that induced by a compatible stimulus when combining the two sleep conditions, which suggested that the relative onset time of the response execution is delayed during more complicated cognition processing. This delay is consistent with the postulate that the processing of incompatible stimuli included additional cognitive functions; extra processing such as conflict monitoring between sensory integration and response execution deferred the onset time of the response execution.
An additional observation in the current study was that the polarity of the s-LRP induced by the incompatible stimulus was opposite to that induced by the compatible stimulus. The different polarities of s-LRP and r-LRP evoked by incompatible stimuli provided new evidence supporting the two-stage classification of LRPs (Mordkoff and Gianaros, 2000; Rinkenauer et al., 2004). That is, the difference between the two subcomponents of LRP is not only reflected in the onset latency, but also in the relationship between the position of the stimulus presentation and the brain regions. The contralateral brain hemisphere of the visual field and that of the response hand are the same for compatible stimuli but different for incompatible stimuli. The formula “LRP = (mean [C4-C3]left hand + mean [C3-C4]right hand)/2” involved response hands rather than visual fields; therefore, the polarity of the s-LRP induced by the incompatible stimulus was opposite to that induced by compatible stimulus. Hence, when we calculated the s-LRP using the contralateral electrodes of the visual field, the waves of the s-LRP induced by the incompatible stimulus were similar to those induced by compatible stimulus (Figure 4A). The corresponding relationship between the dominant brain hemisphere and the visual fields rather than the responding hands showed that the s-LRP was an underlying mechanism of early sensory integration. Taken together, our data show that stimuli are first processed in the contralateral hemisphere corresponding to the visual field during the former sub-stage of motor preparation (sensory integration), then in the contralateral hemisphere corresponding to the response hand during the later sub-stage of motor preparation (response execution). The interesting question of whether the polarity of the s-LRP is inverted ought to be investigated in future studies.
As mentioned in the section “1. Introduction”, LRPs are widely used in many fields of research to assess the processes of motor preparation. The s-LRP reflects sensory integration, while the r-LRP is thought to be related to the subsequent processes involved in response execution. This study contributed to our knowledge regarding how sleep loss damages motor preparation. Previous study found that mild sleep restriction reduced the amplitude of LRPs in the psychomotor vigilance task, indicating that sleep loss can negatively affect motor preparation processes (Stojanoski et al., 2019). Our study revealed that TSD impaired sensory integration (the earlier sub-stage of motor preparation) rather than response execution (the later sub-stage of motor preparation). In other words, sensory integration (s-LRP) is more susceptible to TSD than response execution (r-LRP).
There were some limitations that deserve consideration in our current study. First, the sample size was not very large, which influenced the generalizability of the findings. Second, other sample-specific features limited the ecological validity; for example, all the participants were young adult males, and future studies ought to broaden characteristics such as sex and age. Third, the number of electrodes used was insufficient; high-density EEG would be more effective for acquiring the signal of M1 activation. Finally, given that the M1 activation in the time window between s-LRP and r-LRP involves a combination of ERP components (e.g., N2 and P3), we did not trace the intermediate processes of s-LRP and r-LRP. More exploratory analyses may be necessary to clarify these intermediate processes in future studies.
In summary, our study revealed that TSD selectively attenuates motor preparation processing; that is, TSD impairs the earlier sensory integration sub-stage rather than the later response execution. These findings provide new evidence regarding the weakened cognitive functions induced by sleep loss.
The raw data supporting the conclusions of this article will be made available by the authors, without undue reservation.
The studies involving human participants were reviewed and approved by the Ethics Committee of Beihang University. The patients/participants provided their written informed consent to participate in this study.
YS, XW, and SL designed the experiment. LX, ZP, LW, CD, MX, and YZ collected the experiment data. TS and FD analyzed the data, interpreted the results, and wrote the manuscript. All authors listed have read and approved the manuscript.
This study was supported by the National Natural Science Foundation of China (No. 82073833) and National Key R&D plan (No. 2022YFC3600500).
The authors declare that the research was conducted in the absence of any commercial or financial relationships that could be construed as a potential conflict of interest.
All claims expressed in this article are solely those of the authors and do not necessarily represent those of their affiliated organizations, or those of the publisher, the editors and the reviewers. Any product that may be evaluated in this article, or claim that may be made by its manufacturer, is not guaranteed or endorsed by the publisher.
Acheson, A., Richards, J. B., and de Wit, H. (2007). Effects of sleep deprivation on impulsive behaviors in men and women. Physiol. Behav. 91, 579–587. doi: 10.1016/j.physbeh.2007.03.020
Aidman, E., Jackson, S. A., and Kleitman, S. (2018). Effects of sleep deprivation on executive functioning, cognitive abilities, metacognitive confidence, and decision making. Appl. Cogn. Psychol. 33, 188–200. doi: 10.1002/acp.3463
Ben, S. E., Vallat, R., Barnes, C. M., and Walker, M. P. (2020). Sleep loss and the socio-emotional brain. Trends Cogn. Sci. 24, 435–450. doi: 10.1016/j.tics.2020.02.003
Boardman, J. M., Bei, B., Mellor, A., Anderson, C., Sletten, T. L., and Drummond, S. P. A. (2017). The ability to self-monitor cognitive performance during 60h total sleep deprivation and following 2nights recovery sleep. J. Sleep Res. 27:e12633. doi: 10.1111/jsr.12633
Buysse, D. J., Reynolds, C. F. III, Monk, T. H., Berman, S. R., and Kupfer, D. J. (1989). The Pittsburgh Sleep Quality Index: a new instrument for psychiatric practice and research. Psychiatry Res. 28, 193–213. doi: 10.1016/0165-1781(89)90047-4
Chang, A. M., Aeschbach, D., Duffy, J. F., and Czeisler, C. A. (2015). Evening use of light-emitting eReaders negatively affects sleep, circadian timing, and next-morning alertness. Proc. Natl. Acad. Sci. 112, 1232–1237. doi: 10.1073/pnas.1418490112
Chee, M. W., and Choo, W. C. (2004). Functional imaging of working memory after 24 hr of total sleep deprivation. J. Neurosci. 24, 4560–4567. doi: 10.1523/JNEUROSCI.0007-04.2004
Chee, M. W., and Chuah, Y. M. (2007). Functional neuroimaging and behavioral correlates of capacity decline in visual short-term memory after sleep deprivation. Proc. Natl. Acad. Sci. U.S.A. 104, 9487–9492. doi: 10.1073/pnas.0610712104
Cheval, B., Tipura, E., Burra, N., Frossard, J., Chanal, J., Orsholits, D., et al. (2018). Avoiding sedentary behaviors requires more cortical resources than avoiding physical activity: An EEG study. Neuropsychologia 119, 68–80. doi: 10.1016/j.neuropsychologia.2018.07.029
Choo, W. C., Lee, W. W., Venkatraman, V., Sheu, F. S., and Chee, M. W. (2005). Dissociation of cortical regions modulated by both working memory load and sleep deprivation and by sleep deprivation alone. Neuroimage 25, 579–587. doi: 10.1016/j.neuroimage.2004.11.029
Clark, K., Appelbaum, L. G., van den Berg, B., Mitroff, S. R., and Woldorff, M. G. (2015). Improvement in visual search with practice: mapping learning-related changes in neurocognitive stages of processing. J. Neurosci. 35, 5351–5359. doi: 10.1523/jneurosci.1152-14.2015
Coles, M. G. H. (1989). Modern mind-brain reading: psychophysiology, physiology, and cognition. Psychophysiology 26, 251–269. doi: 10.1111/j.1469-8986.1989.tb01916.x
Dayan-Riva, A., Berger, A., and Anholt, G. (2021). Affordances, response conflict, and enhanced-action tendencies in obsessive-compulsive disorder: An ERP study. Psychol. Med. 51, 948–963. doi: 10.1017/S0033291719003866
De Jong, R., Wierda, M., Mulder, G., and Mulder, L. J. (1988). Use of partial stimulus information in response processing. J. Exp. Psychol. 14, 682–692. doi: 10.1037/0096-1523.14.4.682
Debnath, R., and Franz, E. A. (2016). Perception of hand movement by mirror reflection evokes brain activation in the motor cortex contralateral to a non-moving hand. Cortex 81, 118–125. doi: 10.1016/j.cortex.2016.04.015
Deliens, G., and Bukowski, H. (2018). The impact of sleep deprivation on visual perspective taking. J. Sleep Res. 27, 175–183. doi: 10.1111/jsr.12595
Delorme, A., and Makeig, S. (2004). EEGLAB: an open source toolbox for analysis of single-trial EEG dynamics including independent component analysis. J. Neurosci. Methods 134, 9–21. doi: 10.1016/j.jneumeth.2003.10.009
Gibbings, A., Ray, L. B., Berberian, N., Nguyen, T., Zandi Shahidi, A., Owen, A. M., et al. (2020). EEG and behavioural correlates of mild sleep deprivation and vigilance. Clin. Neurophysiol. 132, 45–55. doi: 10.1016/j.clinph.2020.10.010
Gorman Bozorgpour, E. B., Klorman, R., and Gift, T. E. (2013). Effects of subtype of attention-deficit/hyperactivity disorder in adults on lateralized readiness potentials during a go/no-go choice reaction time task. J. Abnorm. Psychol. 122, 868–878. doi: 10.1037/a0033992
Habeck, C., Rakitin, B. C., Moeller, J., Scarmeas, N., Zarahn, E., Brown, T., et al. (2004). An event-related fMRI study of the neurobehavioral impact of sleep deprivation on performance of a delayed-match-to-sample task. Cogn. Brain Res. 18, 306–321. doi: 10.1016/j.cogbrainres.2003.10.019
Honn, K. A., and Hinson, J. M. (2019). Cognitive flexibility: a distinct element of performance impairment due to sleep deprivation. Accident Anal. Prev. 126, 191–197. doi: 10.1016/j.aap.2018.02.013
Jost, K., Wendt, M., Luna-Rodriguez, A., Löw, A., and Jacobsen, T. (2017). Strategic control over extent and timing of distractor-based response activation. J. Exp. Psychol. 43, 326–333. doi: 10.1037/xlm0000326
Kahn, M., Sheppes, G., and Sadeh, A. (2013). Sleep and emotions: bidirectional links and underlying mechanisms. Int. J. Psychophysiol. 89, 218–228. doi: 10.1016/j.ijpsycho.2013.05.010
Kiesel, A., Miller, J., Jolicceur, P., and Brisson, B. (2008). Measurement of ERP latency differences, comparison of single-participant and jackknife-based scoring methods. Psychophysiology 45, 250–274. doi: 10.1111/j.1469-8986.2007.00618.x
Kumar, T., and Jha, S. K. (2012). Sleep deprivation impairs consolidation of cued fear memory in rats. PLoS One 7:e47042. doi: 10.1371/journal.pone.0047042
Kusztor, A., Raud, L., Juel, B. E., Nilsen, A. S., Storm, J. F., and Huster, R. J. (2019). Sleep deprivation differentially affects subcomponents of cognitive control. Sleep 42:zsz016. doi: 10.1093/sleep/zsz016
Lim, J., and Dinges, D. F. (2008). Sleep deprivation and vigilant attention. Ann. NY Acad. Sci. 1129, 305–322. doi: 10.1196/annals.1417.002
Lopez-Calderon, J., and Luck, S. J. (2014). ERPLAB: An open-source toolbox for the analysis of event-related potentials. Front. Hum. Neurosci. 8:213. doi: 10.3389/fnhum.2014.00213
Lowe, C. J., Safati, A., and Hall, P. A. (2017). The neurocognitive consequences of sleep restriction: a meta-analytic review. Neurosci. Biobehav. Rev. 80, 586–604. doi: 10.1016/j.neubiorev.2017.07.010
Luck, S. J. (2005). “Ten simple rules for designing ERP experiments,” in Event-related potentials: A methods handbook, ed. T. C. Handy (Cambridge, MA: MIT Press), 17–32.
Lythe, K. E., Williams, S. C., Anderson, C., Libri, V., and Mehta, M. A. (2012). Frontal and parietal activity after sleep deprivation is dependent on task difficulty and can be predicted by the fMRI response after normal sleep. Behav. Brain Res. 233, 62–70. doi: 10.1016/j.bbr.2012.04.050
McMahon, W. R., Ftouni, S., Drummond, S. P. A., Maruff, P., Lockley, S. W., Rajaratnam, S. M. W., et al. (2018). The wake maintenance zone shows task dependent changes in cognitive function following one night without sleep. Sleep 41: zsy148. doi: 10.1093/sleep/zsy148
Miller, J., Patterson, T., and Ulrich, R. (1998). Jackknife-based method for measuring LRP onset latency differences. Psychophysiology 35, 99–115. doi: 10.1111/1469-8986.3510099
Mognon, A., Jovicich, J., Bruzzone, L., and Buiatti, M. (2011). ADJUST: An automatic EEG artifact detector based on the joint use of spatial and temporal features. Psychophysiology 48, 229–240. doi: 10.1111/j.1469-8986.2010.01061.x
Morand-Beaulieu, S., Aardema, F., O’Connor, K. P., and Lavoie, M. E. (2021). Lateralized readiness potentials and sensorimotor activity in adults with obsessive-compulsive disorder. Progress Neuro-Psychopharmacol. Biol. Psychiatry 104:110061. doi: 10.1016/j.pnpbp.2020.110061
Mordkoff, J. T., and Gianaros, P. J. (2000). Detecting the onset of the lateralized readiness potential: A comparison of available methods and procedures. Psychophysiology 37, 347–360. doi: 10.1111/1469-8986.3730347
Nayak, S., Salem, H. Z., and Tarullo, A. R. (2020). Neural mechanisms of response-preparation and inhibition in bilingual and monolingual children: Lateralized Readiness Potentials (LRPs) during a nonverbal Stroop task Author links open overlay panel. Dev. Cogn. Neurosci. 41:100740. doi: 10.1016/j.dcn.2019.100740
Pion-Tonachini, L., Kreutz-Delgado, K., and Makeig, S. (2019). ICLabel: An automated electroencephalographic independent component classifier, dataset, and website. NeuroImage 198, 181–197. doi: 10.1016/j.neuroimage.2019.05.026
Qian, H., and Gao, X. (2021). Representing strangers in personal space triggers coding of defensive hand movement. Neuropsychologia 158:107909. doi: 10.1016/j.neuropsychologia.2021.107909
Qureshi, M. F., and Jha, S. K. (2017). Short-term total sleep-deprivation impairs contextual fear memory, and contextual fear-conditioning reduces rem sleep in moderately anxious swiss mice. Front. Behav. Neurosci. 11:239. doi: 10.3389/fnbeh.2017.00239
Ratcliff, R., Van, D., and Hans, P. (2018). The effects of sleep deprivation on item and associative recognition memory. J. Exp. Psychol. 44, 193–208. doi: 10.1037/xlm0000452
Rinkenauer, G., Osman, A., Ulrich, R., Müller-Gethmann, H., and Mattes, S. (2004). On the locus of speed-accuracy trade-off in reaction time: inferences from the lateralized readiness potential. J. Exp. Psychol. 133, 261–282. doi: 10.1037/0096-3445.133.2.261
Shibasaki, H., and Hallett, M. (2006). What is the Bereitschaftspotential? Clin. Neurophysiol. 117, 2341–2356. doi: 10.1016/j.clinph.2006.04.025
Slobounov, S. M. (2010). “Event-related potentials: Slow Potentials,” in Encyclopedia of movement disorders, Ed. K. Kompoliti (Cambridge, MA: Elsevier Academic Press), 456–458. doi: 10.1016/b978-0-12-374105-9.00458-5
Smulders, F. T., and Miller, J. (2012). “The lateralized readiness potential,” in The Oxford handbook of event-related potential components, eds S. J. Luck and E. S. Kappenman (Oxford: Oxford University Press), 209–229. doi: 10.1093/oxfordhb/9780195374148.013.0115
Stepan, M. E., Altmann, E. M., and Fenn, K. M. (2020). Effects of total sleep deprivation on procedural place keeping: more than just lapses of attention. J. Exp. Psychol. 149, 800–806. doi: 10.1037/xge0000717
Stojanoski, B., Benoit, A., Van Den Berg, N., Ray, L. B., Owen, A. M., Shahidi Zandi, A., et al. (2019). Sustained vigilance is negatively impacted by mild and acute sleep loss reflected by reduced capacity for decision making, motor preparation and execution. Sleep 42, 1–9. doi: 10.1093/sleep/zsy200
St-Onge, M. P., and Zuraikat, F. M. (2019). Reciprocal roles of sleep and diet in cardiovascular health: a review of recent evidence and a potential mechanism. Curr. Atheroscler. Rep. 21:11. doi: 10.1007/s11883-019-0772-z
Sun, M., Wang, E., Huang, J., Zhao, C., Guo, J., Li, D., et al. (2018). Attentional selection and suppression in children and adults. Dev. Sci. 21:e12684. doi: 10.1111/desc.12684
Tripathi, S., Taneja, P., and Jha, S. K. (2018). Training on an appetitive (delay)-conditioning task enhances oscillatory waves during sleep in the cortical and amygdalar network. Front. Behav. Neurosci. 12:260. doi: 10.3389/fnbeh.2018.00260
Trujillo, L. T., Kornguth, S., and Schnyer, D. M. (2009). An ERP examination of the different effects of sleep deprivation on exogenously cued and endogenously cued attention. Sleep 32, 1285–1297. doi: 10.1093/sleep/32.10.1285
Van Voorhis, A. C., Kent, J. S., Kang, S. S., Goghari, V. M., MacDonald, A. W., and Sponheim, S. R. (2019). Abnormal neural functions associated with motor inhibition deficits in schizophrenia and bipolar disorder. Hum. Brain Mapp. 40, 5397–5411. doi: 10.1002/hbm.24780
Wang, E., Sun, L., Sun, M., Huang, J., Tao, Y., Zhao, X., et al. (2016). Attentional selection and suppression in children with attention-deficit/hyperactivity disorder. Biol. Psychiatry 1, 372–380. doi: 10.1016/j.bpsc.2016.01.004
Keywords: sleep deprivation, lateralized readiness potentials, event-related potentials, electroencephalography, motor preparation, visual search
Citation: Song T, Du F, Xu L, Peng Z, Wang L, Dai C, Xu M, Zhang Y, Shao Y, Weng X and Li S (2023) Total sleep deprivation selectively impairs motor preparation sub-stages in visual search task: Evidence from lateralized readiness potentials. Front. Neurosci. 17:989512. doi: 10.3389/fnins.2023.989512
Received: 08 July 2022; Accepted: 09 February 2023;
Published: 28 February 2023.
Edited by:
Chrysoula Kourtidou-Papadeli, Independent Researcher, Thessaloniki, GreeceReviewed by:
Panteleimon Chriskos, Aristotle University of Thessaloniki, GreeceCopyright © 2023 Song, Du, Xu, Peng, Wang, Dai, Xu, Zhang, Shao, Weng and Li. This is an open-access article distributed under the terms of the Creative Commons Attribution License (CC BY). The use, distribution or reproduction in other forums is permitted, provided the original author(s) and the copyright owner(s) are credited and that the original publication in this journal is cited, in accordance with accepted academic practice. No use, distribution or reproduction is permitted which does not comply with these terms.
*Correspondence: Yongcong Shao, YnVkZXNoYW9AYnN1LmVkdS5jbg==; Xiechuan Weng,
d2VuZ3hjMjAwMEAxNjMuY29t; Shijun Li,
c2hpanVubGkwN0B5ZWFoLm5ldA==
†These authors have contributed equally to this work
Disclaimer: All claims expressed in this article are solely those of the authors and do not necessarily represent those of their affiliated organizations, or those of the publisher, the editors and the reviewers. Any product that may be evaluated in this article or claim that may be made by its manufacturer is not guaranteed or endorsed by the publisher.
Research integrity at Frontiers
Learn more about the work of our research integrity team to safeguard the quality of each article we publish.