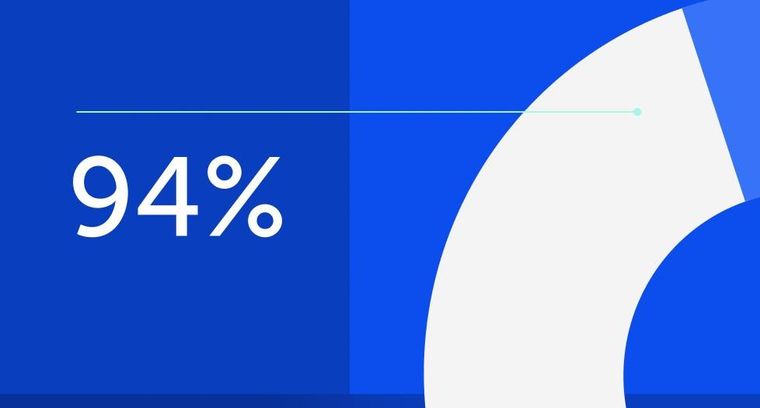
94% of researchers rate our articles as excellent or good
Learn more about the work of our research integrity team to safeguard the quality of each article we publish.
Find out more
REVIEW article
Front. Neurosci., 08 January 2024
Sec. Neurodevelopment
Volume 17 - 2023 | https://doi.org/10.3389/fnins.2023.1320319
This article is part of the Research TopicThe Immunological Effects of Respiratory Viruses during Pregnancy and BreastfeedingView all 4 articles
Brain development is a complex process that begins during pregnancy, and the events occurring during this sensitive period can affect the offspring’s neurodevelopmental outcomes. Respiratory viral infections are frequently reported in pregnant women, and, in the last few decades, they have been related to numerous neuropsychiatric sequelae. Respiratory viruses can disrupt brain development by directly invading the fetal circulation through vertical transmission or inducing neuroinflammation through the maternal immune activation and production of inflammatory cytokines. Influenza virus gestational infection has been consistently associated with psychotic disorders, such as schizophrenia and autism spectrum disorder, while the recent pandemic raised some concerns regarding the effects of severe acute respiratory syndrome coronavirus 2 on neurodevelopmental outcomes of children born to affected mothers. In addition, emerging evidence supports the possible role of respiratory syncytial virus infection as a risk factor for adverse neuropsychiatric consequences. Understanding the mechanisms underlying developmental dysfunction allows for improving preventive strategies, early diagnosis, and prompt interventions.
The human brain, constituted of 85 billion neurons and 85 billion non-neuronal cells, is a complex structure responsible for higher cognitive and behavioral abilities (i.e., perception, memories, thoughts, and language; Bystron et al., 2008; Azevedo et al., 2009).
The central nervous system (CNS) development mainly occurs during the prenatal period, and most cerebral neurogenesis happens within the 28th gestational week (Davis et al., 2011; Hadders-Algra, 2021); therefore, pregnancy is considered a vulnerable epoch for the neuropsychiatric outcome of the offspring since genetic, epigenetic, and environmental factors may disrupt the typical developmental trajectory (Hadders-Algra, 2021; San Martín-González et al., 2023).
The intrauterine environment represents the first influence on the fetus’s development, and maternal health has long been considered an important factor impacting the child’s well-being (Barker, 1990).
According to the Developmental Origins of Health and Disease hypothesis, events occurring during this sensitive period might have short- and long-term consequences on the fetus epigenetic program, depending on the timing and duration of exposure to the noxa (Davis et al., 2011; Palma-Gudiel et al., 2019).
Numerous studies proved that neurotropic viral agents may cross the placental barrier and that in utero exposure may severely affect fetal brain development (Zimmer et al., 2021). Depending on the specific virus, the timing of infection during gestation, and the efficiency of viral neutralization and clearance by the host immune system, neuropsychiatric sequelae can range from mild to severe, and even compromise fetal viability (Gordon-Lipkin et al., 2021; Manti et al., 2022). Additionally, the maternal immune response, including the synthesis of pro-inflammatory cytokines, as well as the fetal pro-inflammatory mediators, can sustain the neuroinflammation affecting the CNS development in the fetus (Han et al., 2021; Elgueta et al., 2022; Kwon et al., 2022).
Consistent literature data reported the association between prenatal risk factors, such as in utero infections, and diseases in childhood and adulthood, including neuropsychiatric disorders (Zimmer et al., 2021; San Martín-González et al., 2023). In line with this theory, Torrey and Peterson proposed the “Viral Hypothesis of Schizophrenia,” according to which a viral infection acquired during prenatal life could be involved in schizophrenia development (Torrey and Peterson, 1976). Subsequently, several reports confirmed an increased risk for schizophrenia onset in patients born by women affected by viral infection during pregnancy, especially in subjects born during late winter and spring, seasons during which Influenza infection is primarily detected (Hare et al., 1972; Machón et al., 1987; Mednick et al., 1988; O’Callaghan et al., 1991; Adams et al., 1993; Mednick et al., 1994; Kunugi et al., 1995; Izumoto et al., 1999; Limosin et al., 2003; Brown, 2006; Fatemi et al., 2012). In addition, further research highlighted the occurrence of neurodevelopmental disorders, such as Autism Spectrum Disorder (ASD), Attention-Deficit/Hyperactivity Disorder (ADHD), microcephaly, epilepsy, cognitive impairment, and bipolar disorder in children with a history of prenatal maternal infections (Jiang et al., 2016; Tioleco et al., 2021; Elgueta et al., 2022; Walle et al., 2022; Zhu et al., 2022).
In the past, respiratory viruses were not considered highly neurotropic agents, and most authors did not heed the possible vertical transmission to the fetus (Zimmer et al., 2021; San Martín-González et al., 2023). However, respiratory viral infections are the most frequently reported during pregnancy, and the recent pandemics raised some concerns regarding the neurodevelopmental consequences for the offspring of women affected by respiratory infections (San Martín-González et al., 2023; Sturrock et al., 2023).
This review aims to investigate the impact of prenatal exposure to maternal respiratory infections on neuropsychiatric outcomes throughout the epochs of life. In order to achieve this goal, we summarized what is known about the early stages of brain development and the systemic changes, including immune activation, occurring in pregnant women affected by respiratory viral infections, such as Influenza virus, severe acute respiratory syndrome coronavirus 2 (SARS-CoV-2), Respiratory Syncytial virus (RSV), and other respiratory viruses (Figure 1). Finally, we reviewed literature data focusing on neuropsychiatric disorders associated with common respiratory viral infections, including Influenza virus, SARS-CoV-2, RSV, and other respiratory viruses.
Figure 1. Mechanisms affecting the brain development during maternal respiratory viral infections. During pregnancy, physiological changes in the maternal immune system increase the susceptibility to respiratory viral infections. (A) The reduction in the Th1/Th2 phenotype ratio and natural killer cell activation impair the immune response, while the intravascular inflammation enhances the virulence, increasing the risk of vertical transmission. (B) Cytokines and inflammatory mediators produced by the mother during infections can cross the placental barrier and interfere with the fetal brain development. (C) Both the vertical transmission and maternal immune activation induce neuroinflammation, further sustained by fetal production of inflammatory products. All these mechanisms disrupt the development of the fetal central nervous system.
Brain development is a process that starts during the early weeks of the pregnancy and requires about 40 years to reach a mature configuration (Hadders-Algra, 2021). However, gestation is an epoch of profound changes in the CNS, and it is widely accepted that prenatal experiences can influence the developing motor and sensory-perceptual systems, affecting the neurobehavioral and cognitive outcomes of the offspring (Kostović et al., 1995; Han et al., 2021). During pregnancy, brain development consists of four main processes, including neuronal proliferation and migration, mainly occurring during the embryonic and early fetal periods, synaptogenesis and myelination, which start during the intrauterine life and continue through the postnatal age (Kostović et al., 1995; Borsani et al., 2019).
By the 2–3th gestational week, the ectoderm initiates a process of folding and fusion that culminates in the neural tube formation at embryonic day 30. The rostral portion of the neural tube gives rise to the prosencephalic, mesencephalic and romboencephalic vesicles, namely the precursors of the forebrain, midbrain, and hindbrain. The prosencephalon will originate the diencephalon (subcortical structures) and the telencephalon (cerebral cortices; Bystron et al., 2008; Borsani et al., 2019). Neurogenesis begins after the complete closure of the neural tube, approximately at embryonic day 33, in the ventricular zone (VZ). Neuronal and glial progenitors, named neural stem/precursor cells (NPCs), arise from the VZ and their proliferation results in a new layer, called subventricular zone or germinal matrix (Bystron et al., 2008; Elgueta et al., 2022). Most cortical neurons emerge from this last region and form the preplate, that together with the marginal zone (near the pial surface) and the subplate zone (near the ventricle), constitutes the primordial cerebral cortex (Kostović et al., 1995; Borsani et al., 2019). Notably, the subplate is a transient layer rich in neuronal fibers and represents the primary site of neuronal differentiation and synaptogenesis (Hadders-Algra, 2021).
The cerebellum, which contains 80% of the brain neurons, also originates between the end of the first and the beginning of the second trimester of pregnancy. At 11 weeks of gestational age, the VZ will give rise to neurons constituting the future deep cerebellar nuclei and the Purkinje cells. Moreover, the cerebellar anlage has its transient area, known as external granular layer. Since the 15th week of gestational age, it will generate the granule cells and disappear after the first post-term year (Amore et al., 2021).
Neuronal migration and myelination begin around the 20th week of gestational age and occur with a spatio-temporal gradient: the former takes place slightly earlier in the occipital areas than in the frontal ones, the latter starts in the subcortical regions before the cortical ones (Trivedi et al., 2009; Tau and Peterson, 2010). Moreover, neuronal migration usually ends within 26–29 weeks of gestational age; on the contrary, myelination of the corticospinal tracts lasts until 2–3 years of postnatal life (Borsani et al., 2019).
During the third trimester of pregnancy, neuronal proliferation gradually declines while glial cell production continues, inducing an increase in the volume of the white matter. In this period, the brain reaches a full-blown adult configuration, presenting an immature cytoarchitecture. In particular, neurotransmitter systems have developed, and neuronal differentiation, axonal ingrowth, and synaptogenesis processes become more intense (Hadders-Algra, 2021).
In addition, depending on the cortical region, area differentiation begins between the 24th and the 34th gestational weeks, persisting until the term age (Kostović et al., 1995; Borsani et al., 2019).
Pregnancy induces significant physiological changes in the mother, involving the immune, respiratory, cardiovascular, and hormonal systems (Manti et al., 2022). Since there is no vascular continuity between the mother and the fetus, the placenta plays a fundamental role in the maternal-fetal exchanges (Veenstra van Nieuwenhoven et al., 2003). It allows the transport of nutrients and antibodies from the maternal circulation through membrane transporters and endocytosis, production of hormones, and waste removal from the fetal blood. In addition, it represents a barrier from pathogens, toxicants, and teratogens (Gordon-Lipkin et al., 2021).
Recent proof of trans-syncytial nanopores in the human placenta demonstrated that potentially harmful molecules can cross the placenta through paracellular diffusion and adversely affect fetal development (Lewis et al., 2022).
The fetus and placenta have been historically considered an allograft since they express “non-self” paternal antigens. Moreover, the placenta expresses tissue-specific antigens that might potentially trigger maternal immune activation, and data from the literature confirm systemic immune responses to numerous pathogens in animal models and pregnant women (Erlebacher, 2013). At the same time, mechanisms involved in fetomaternal tolerance also prevent pregnancy-associated complications, such as prematurity, preeclampsia, fetal growth restriction, and miscarriages (Kwon et al., 2022). All this evidence proves that pregnancy must not be considered a classical transplantation; rather, it represents a unique immunological condition in which immune activation is locally regulated and limited to the maternal-fetal interface (Saito, 2022).
Nevertheless, the maternal immune system incurs numerous adjustments during gestation that may increase the risk of infections. CD4+ T cell population shows a reduction in the Th1/Th2 phenotype ratio, which allows the pregnancy to progress but can affect the clearance of infected cells (Parkin and Cohen, 2001; Veenstra van Nieuwenhoven et al., 2003). Some authors suggested that this disproportion could be caused by a suppression of maternal T cells by progesterone and fetal regulatory T cells (Saito, 2022). Dendritic cells (DCs), the most potent antigen-presenting cells, have also been implicated in regulating of the Th1/Th2 phenotype ratio (Veenstra van Nieuwenhoven et al., 2003). Moreover, it has been proposed that paternal/fetal antigens are not directly presented by fetal antigen-presenting cells but by maternal DCs, to reduce the probability of acute transplant rejection. Furthermore, maternal DCs are suggested to be trapped in the uterus, minimizing fetal antigen presentation to the lymph nodes (Saito, 2022). This hypothesis is supported by studies on mice models that proved the inability of DCs to migrate from the decidua to lymphatic vessels of the uterus, even after immune stimulation (Erlebacher, 2013).
The fetal regulatory T cells may also reduce the activation of natural killer (NK) cells, which are embryotoxic in pregnant women (Veenstra van Nieuwenhoven et al., 2003; Saito, 2022). Both DCs and NK cells are fundamental mediators of the immune response to viral infections and their reduction can expose the mother to an increased risk of infection (Parkin and Cohen, 2001; Veenstra van Nieuwenhoven et al., 2003).
Besides the immunological effects, pregnancy may induce other systemic modifications that increase susceptibility to viral respiratory infections. Pulmonary compliance is reduced due to diaphragm elevation and shape alterations of the chest, affecting the airway clearance of pathogens. Furthermore, increased intravascular inflammation, with coagulation and fibrinolytic factors circulation, can enhance virulence (Manti et al., 2022).
All these collective mechanisms concur in the onset and evolution of maternal infections, particularly respiratory viral ones, and their spreading to the fetal circulation (Veenstra van Nieuwenhoven et al., 2003; Gordon-Lipkin et al., 2021; San Martín-González et al., 2023). Vertical transmission from the mother to the fetus has been described for neurotropic and non-neurotropic viruses, which can cause the invasion of neural cells and microglia in the developing brain (Zimmer et al., 2021; Manti et al., 2022). Moreover, viral agents can affect the newly formed CNS by interfering with NPCs generation, progenitors of neurons, astrocytes, and oligodendrocytes (Elgueta et al., 2022).
Alternatively to vertical transmission, accumulating evidence in the literature supports the hypothesis that maternal immune activation (MIA) constitutes one of the crucial mechanisms through which prenatal infections exert their effects on neurodevelopment (Han et al., 2021; Kwon et al., 2022; Woods et al., 2023; Figure 2). Maternal systemic immune response against infections leads to the production of cytokines and inflammatory mediators, such as interleukin (IL)-6, IL-17a, IL-1β, tumor necrosis factor (TNF)-α, and interferon (IFN)-γ, which may cross the placental barrier depending on the gestational age by diffusion through paracellular trans-trophoblastic water-filled channels (Kwon et al., 2022; Woods et al., 2023). In a rat model, Dahlgren and colleagues proved that the transfer of IL-6 to the fetal circulation was significantly higher during mid-gestation than in late pregnancy (Dahlgren et al., 2006). On the contrary, in a different rat model of MIA, traced IL-1β showed minimal fetal passage during the late gestational period (Girard and Sebire, 2016). These inflammatory factors can affect NPCs development, resulting in cortical defects and neurodevelopmental disorders. Particularly, it has been proved that IL-1β influences neuronal migration, and IL-6 affects the temporal regulation of neurogenesis and reduces the neuronal pool within the cortical layers, promoting an early switch to gliogenic cells and inducing an alteration in the ratio of the neuronal/glial cells (Sloan and Barres, 2014; Woods et al., 2023). Additionally, IL-6 induces differentiation of naïve T cells to a Th17 phenotype, producing IL-17a, a cytokine that disorganises the cortical cytoarchitecture. These cortical alterations are associated with ASD-like behaviors in the offspring, including abnormal ultrasonic vocalization, a deficit in social interaction, and repetitive behavior (Elgueta et al., 2022). Proinflammatory cytokines can also affect myelination by inhibiting the proliferation and survival of oligodendrocyte progenitors, a cellular type that usually persists later in life and can differentiate even in adulthood (Taylor et al., 2010; Favrais et al., 2011).
Figure 2. Mechanisms and effects of Maternal Immune Activation on fetal brain. The maternal immune response to respiratory infections induces the release of pro-inflammatory cytokines such as IL-6, IL-1β, TNF-α, and IFN-γ. In turn, IL-6 leads to the activation of Th17 cells and the production of IL-17a. In the placenta, TNF-α contributes to the activation of the immune resident cells, resulting in further production of pro-inflammatory cytokines that cross the placental-fetal barrier and participate in the neuroinflammatory processes in the fetal brain. Neuroinflammation may alter normal brain development, resulting in adverse neuropsychiatric outcomes during childhood and adulthood, such as developmental delay/intellectual disability (DD/ID), autism spectrum disorder (ASD), cerebral palsy (CP), attention-deficit/hyperactivity disorder (ADHD), schizophrenia, depression, anxiety, and bipolar disorder.
The pool of NPCs is further controlled by the microglia, which are also responsible for antigen presentation, neuronal remodelling, and synaptic plasticity (Elgueta et al., 2022). In addition, fetal microglia activation may sustain neuroinflammation and impair neurodevelopment by secreting inflammatory products (i.e., TNF-α) and reducing the production of neurotrophins, such as brain-derived neurotrophic factor (BDNF) (Harry et al., 2006; Gibney et al., 2013). Neurotrophins, especially BDNF, are critical mediators of neuronal survival, and their altered levels have been linked to several neurodevelopmental disorders (Manti et al., 2023). Cytokines and active microglia also have a role in astrogliosis: proliferating astrocytes produce pro-inflammatory factors that may impact synaptogenesis, particularly inducing a preferential loss of inhibitory γ-aminobutyric acid (GABA)ergic synapses. This excitatory/inhibitory imbalance has been previously reported in neuropsychiatric disorders, such as ASD and epilepsy (Henstridge et al., 2019; Woods et al., 2023).
Additionally, MIA has been shown to alter the transplacental transportation of essential amino acids (i.e., leucine) (Kowash et al., 2022). In the human placenta, leucine and tryptophan are transferred from the mother to the fetus by the same transporter (Cleal and Lewis, 2008). Essential amino acids are precursors of neurotransmitters that are fundamental in neurodevelopment. Particularly, through the placental tryptophan hydroxylase activity, the placenta represents an important source of serotonin during the first trimester of pregnancy, influencing cortical neurogenesis, migration and initial axon targeting (Bonnin et al., 2011).
A recent study conducted by Perez-Palomar and colleagues in a rodent model proved that MIA disrupts dopaminergic and noradrenergic pathways in the prefrontal cortex, affecting cognitive behavior in offspring. These circuitries are notably impaired in schizophrenia (Perez-Palomar et al., 2023).
Several animal models and epidemiological studies have investigated the association between MIA and neuropsychiatric disorders, including schizophrenia, bipolar disorder, ADHD, cerebral palsy, developmental delay, cognitive dysfunction, anxiety/depression, and ASD (Garbett et al., 2012; Gibney et al., 2013; Canetta et al., 2014; Knuesel et al., 2014; Jiang et al., 2016; Han et al., 2021). It has been hypothesized that inflammatory responses to insults, such as respiratory infections and autoimmunity, might cause alterations in neural activity and brain connectivity (Kwon et al., 2022). Indeed, high amniotic interleukin concentration has been associated with preterm delivery, which is a risk factor for adverse neurodevelopmental outcomes (Hillier et al., 1995; Saigal and Doyle, 2008; Dicanio et al., 2022). Recent research proved that MIA can affect the neuropsychiatric outcomes of the offspring, even in asymptomatic mothers with increased intra-amniotic inflammatory cytokines levels (Gervasi et al., 2022). Noteworthy, neuropsychiatric disorders associated with MIA have heterogeneous clinical phenotypes. Therefore, some authors suggested that neurodevelopment is influenced by multiple risk factors and that MIA, by regulating gene expression and disrupting the neuronal differentiation and arborization, represents only one of the numerous “hits” that intervene in the onset of these conditions (Garbett et al., 2012; Woods et al., 2023).
Influenza virus is an enveloped single-stranded RNA virus belonging to the Orthomyxoviridae family, which is classified into three genera (A, B, and C types) based on its antigenic profile. A further subdivision in serotypes takes into account the surface glycoproteins, such as hemagglutinin (HA) and neuraminidase (NA) (Beigi, 2014). All Influenza viruses have been implicated in human disease, and especially Influenza A and B, thanks to the antigenic drift (variation) of HA and NA, are responsible for annual epidemics with seasonal peaks in fall and winter (Manti et al., 2022). Conversely, the antigenic shift occurs when two or more different Influenza strains combine to produce a novel antigenic structure. This process happens approximately every 20–30 years and has been reported in Influenza A virus, the only type associated with Influenza pandemics until now (Beigi, 2014; Zimmer et al., 2021).
Influenza virus can affect the feto-placental unit both directly and indirectly. The virus can induce the apoptosis of chorion by a direct cytopathic effect, and it can reach the placenta and the amniotic fluid through the maternal bloodstream, causing chorioamnionitis with degeneration of vascular endothelial cells, placental trophoblasts, decidual and amniotic cells (Manti et al., 2022). Moreover, the maternal immune response to the viral infection has been implicated in the association between Influenza and neuropsychiatric disorders. Indeed, it may lead to vascular inflammation and the production of inflammatory mediators such as IL-6, IL-1β, TNF-α, and IFN-γ, with detrimental consequences on brain development (Kwon et al., 2022) (Figure 3).
Figure 3. Immunopathological mechanisms of maternal respiratory infections. Maternal respiratory infections caused by Influenza virus, SARS-CoV-2, and RSV trigger the host’s immune response, leading to the production of several pro-inflammatory cytokines. This Maternal Immune Activation (MIA) is deemed responsible for adverse neuropsychiatric outcomes in offspring. Passing the viruses in the maternal blood may result in placental infection, potentially leading to vertical transmission to the fetus. In turn, the invasion of placental cells can provoke further production of pro-inflammatory cytokines, contributing to MIA.
The association between the Influenza virus and neuropsychiatric disorders has long been studied in the literature, since Menninger - 1926 - reported an increase in influenza psychoses that looked like schizophrenia after the 1918 Influenza epidemic (Torrey and Peterson, 1976). Afterwards, epidemiologic studies confirmed a 3-fold increased risk of developing schizophrenia following Influenza exposure during the first half of gestation; the risk increased to 7-fold whether the infection occurred during the first trimester of pregnancy (Brown and Derkits, 2010). Moreover, research on the seasonality of birth in patients affected by schizophrenia and autism suggested a possible correlation with Influenza infection during the prenatal age (Zerbo et al., 2011; Fatemi et al., 2012; Zimmer et al., 2021).
Numerous animal models have been developed to explore better the correlation between the Influenza virus and the onset of neuropsychiatric disorders. Shi and colleagues administered intranasal Influenza virus on embryonic day 9.5 to pregnant mice. The authors demonstrated that the offspring showed behavioral abnormalities reminiscent of autism and schizophrenia (i.e., decreased sensitivity to auditory stimulation, increased anxiety, social deficits, and repetitive behaviors), some of which improved after antipsychotic drug treatment. Authors also suggested that Influenza infection contributed to the thinning of the neocortex and hippocampus, pyramidal cells atrophy, and reduction of reelin, a molecule responsible for proper laminar organization of the brain (Shi et al., 2003). In subsequent work, Fatemi and colleagues confirmed this evidence and hypothesized that prenatal Influenza infection might disrupt structure and gene expression in the placenta, as well as in the hippocampus and the prefrontal cortex of the offspring (Fatemi et al., 2012). Moreover, in a rodent model of Influenza infection, Garbett and colleagues highlighted alterations in gene expression involved in neurogenesis and neuroprotection in the acute phase of infection (Garbett et al., 2012). In the study conducted by Short and colleagues on Rhesus monkey, mothers’ infection during pregnancy also reduced early social interaction between the offspring and the mother, and alterations in neuroimaging were comparable to those shown in schizophrenic and autistic patients. Specifically, a significant reduction in brain volume has been described in the grey (prefrontal, frontal, cingulate, insula, parietal, and temporal-auditory regions) and in the white matter (parietal lobe) (Short et al., 2010).
The association between the Influenza virus and increased risk of neuropsychiatric conditions different from schizophrenia has also been investigated. Particularly, Parboosing and colleagues showed a 6-fold increased risk of developing bipolar disorder after exposure to maternal Influenza during pregnancy in a population-based cohort study (Parboosing et al., 2013). In a successive study on the same cohort, the authors assessed the difference between patients with bipolar disorder and patients with bipolar disorder and psychotic symptoms. They found a 5-fold increased risk of bipolar disorder with, but not without, psychotic features. Therefore, they proposed that maternal Influenza exposure was a risk factor for psychosis, rather than for a specific psychotic disorder diagnosis, influencing the same neuronal circuitries of schizophrenia (Canetta et al., 2014). These data were confirmed by a recent systematic review conducted by Fung and colleagues, which confirmed an increased risk for schizophrenia and other primary psychotic disorders in children exposed to maternal Influenza infection during pregnancy, especially during the first and second trimesters. Moreover, the authors reported an increased risk of developing ASD in offspring exposed to maternal Influenza infection throughout the whole gestation (Fung et al., 2022).
However, the Influenza virus has already been proven to impact neurodevelopment in the early post-partum period. In a population-based cohort study, Borren and colleagues reported slightly poorer psychomotor development scores in 6-month-old children whose mothers had Influenza symptoms during pregnancy. The difference became significant when considering the fine-motor alterations in children with early exposure to the Influenza virus (within the 8th week of gestation) and the communication subdomain in infants with later exposure (9–40th weeks of gestation). This evidence confirmed the impact of maternal Influenza infection on the cognitive and social abilities of the offspring (Borren et al., 2018).
SARS-CoV-2 belongs to the Coronaviridae family, comprising six genera, three of which include viruses potentially infecting humans (Zimmer et al., 2021). It is an enveloped RNA virus primarily transmitted via respiratory droplets. After infecting the nasal mucosa, it can be transmitted to lower airway cells that co-express angiotensin-converting enzyme 2 (ACE2) and transmembrane serine protease 2 (TMPRSS2). Moreover, SARS-CoV-2 infection induces an abnormal systemic inflammatory response (cytokine storm) that may lead to acute respiratory distress syndrome by producing IL-6, C-X-C motif chemokine 10 (CXCL10), and INF-1 (Tay et al., 2020).
Placental expression of ACE2 mRNA is gestational-age dependent, with the highest levels in early pregnancy, and viral proteins and/or viral RNA have been detected in syncytiotrophoblasts, cytotrophoblasts, villous stroma, and possibly Hofbauer cells (Li et al., 2020; Fahmi et al., 2021; Lye et al., 2021). Therefore, vertical transmission has been hypothesized, and it has been estimated as 3.67% in newborns of SARS-CoV-2-positive mothers (Dube and Kar, 2020; Fenizia et al., 2020). Several studies showed that neurons, microglia, and NPCs might express ACE2 and TMPRSS2, allowing the infection of the developing brain (Cui et al., 2019; Baig et al., 2020; Yang et al., 2020; Tiwari et al., 2021). Remarkably, in a recent study, expression of ACE2 mRNA was detected in circulating granulocytes, monocytes, and lymphocytes from pregnant women with chorioamnionitis, proving an increased risk of SARS-CoV-2 vertical transmission (Lye et al., 2021). However, it has been proposed that the damage to the fetal brain due to SARS-CoV-2 infection is mainly caused by vascular injury, leading to placental ischemia and inflammation (Sharps et al., 2020; Lye et al., 2021; Liu et al., 2022). In fact, MIA response to SARS-CoV-2 infection is considered the main mediator of the neurodevelopmental disruption in the fetus (Woods et al., 2023). Particularly, increased levels of IL-1β, IL-6, IL-17, and TNF-α have been detected in the peripheral blood of pregnant women compared to controls (Fenizia et al., 2020; Figure 3).
Given the recent onset of the COVID-19 pandemic, no studies address the long-term consequences of maternal infection on the offspring’s neuropsychiatric outcome. Nevertheless, abundant research has been conducted to explore the effects of SARS-CoV-2 on neurodevelopment (San Martín-González et al., 2023). Particularly, Aldrete-Cortez and colleagues examined the general movements of 28 3-to5-months-old children exposed to SARS-CoV-2 infection during the third month of gestation compared to the same number of controls. The exposed children showed significantly poorer motor performances and higher rates of absent or abnormal fidgety movements, proving an increased risk of adverse neurodevelopmental outcomes (Aldrete-Cortez et al., 2022). General movements assessment, particularly fidgety movements, has been extensively used in the literature to predict cerebral palsy since it showed a sensitivity of 98% and a specificity of 91%. However, atypical fidgety movements have also been associated with cognitive impairment, ADHD, and ASD (Hadders-Algra, 2021).
Early fine motor abnormalities have been described by Liu and colleagues at the age of 1–2 months in the offspring of a mother to SARS-CoV-2 during pregnancy. However, these abnormalities were not reported at 12–13 months, after a parent-guided postnatal training. This data shows that a precocious intervention could correct neurodevelopmental delay (Liu et al., 2022). In the study conducted by Cheng and colleagues, the fine motor abnormalities were significantly higher in 9-month-old children exposed to prenatal maternal infection compared to non-exposed children. The authors also found lower scores in communication, gross movement, problem-solving, and personal-social domains, yet not statistically significant (Cheng et al., 2021). Similarly, Ayed and colleagues conducted a prospective cohort study on 298 children born to mothers with SARS-CoV-2 infection during pregnancy and reported a 10% of developmental delay at the age of 10–12 months, with a prevalent deficit in fine motor abilities. Noteworthy, the developmental delay was more common when infection occurred during the first and second trimesters of pregnancy (Ayed et al., 2022). Additionally, Mulkey and colleagues reported an increased risk of developmental delay related to maternal symptomatology. Children born to mothers with symptomatic SARS-CoV-2 infection were found to be at a higher risk of having lower developmental scores, especially in fine motor and personal-social domains. These infants also showed mild truncal hypotonia, which can be associated with lower motor skills (Mulkey et al., 2022).
On the contrary, Shuffrey and colleagues did not find significant differences among their cohort of infants exposed to maternal SARS-CoV-2 infection during pregnancy and children born before the pandemic. However, the authors observed that infants born during the pandemic obtained significantly lower scores on the gross motor, fine motor, and personal-social subdomains, irrespective of maternal infection. Therefore, they suggested that COVID-19-related stress should be considered a potential mechanism underlying the neurodevelopmental abnormalities experienced by these children (Shuffrey et al., 2022). Similarly, Wu and colleagues reported significantly lower scores in fine motor, problem-solving and personal-social subdomains in a cohort 3-month-old infants with prenatal exposure to SARS-CoV-2; however, the social–emotional and overall developmental delay did not correlate to the maternal infection. Interestingly, the authors found a negative correlation between lower gross motor scores and the length of mother-infant separation after birth (Wu et al., 2021). It is worthy of note that the last two studies had few cases of infection during the first trimester, which is considered by some authors the most critical period for fetal infection (Li et al., 2020; Lye et al., 2021; Wu et al., 2021; Shuffrey et al., 2022). Nevertheless, children exposed to third-trimester maternal infection have also reported an increased risk for neurodevelopmental disorders. Edlow and colleagues, in a retrospective cohort study performed on 222 newborns of mothers infected with SARS-CoV-2, observed higher rates of neurodevelopmental disorders compared to children delivered in the same period. The majority of these diagnoses reflected developmental disorders of motor function or speech and language (Edlow et al., 2022).
Besides the neurodevelopmental impairment, sparse cases of children with neonatal ischemic brain lesions correlated with SARS-CoV-2 maternal infection have been reported in the literature. The patients mainly showed thalamic infarction, though alterations of the white matter have also been described (Vivanti et al., 2020; Beslow et al., 2021; Engert et al., 2021; Brogna et al., 2022; Campi et al., 2022). Placental inflammation, vascular endothelial damage, and consequent activation of the clotting cascade that induces thrombotic events have been proposed as pathogenic mechanisms involved in neurodevelopmental impairment (Sharps et al., 2020; Lye et al., 2021; Brogna et al., 2022; Liu et al., 2022).
Respiratory syncytial virus (RSV) is an enveloped single-stranded RNA virus of the Paramyxoviridae family (Keyserling, 1997). The surface glycoproteins G (Ga and Gb) identify two subtypes, namely A and B; together with the surface glycoprotein F (fusion). These proteins facilitate the interaction with the host airway epithelial cells, merge the viral envelope to the membranes of multiple adjacent cells, and form the “syncytia.” Thus, they appear crucial for the virulence of RSV and the principal antigens presented to the host immune system (Piedimonte, 2015; Andeweg et al., 2021).
RSV causes annual epidemics lasting 2–5 months and it is the primary cause of hospitalization in the pediatric population within 6 months of age; by the age of 2 years, most infants have been infected at least once by RSV and reinfected in 40% of the cases (Keyserling, 1997; Kazakova et al., 2019; Andeweg et al., 2021; Manti et al., 2023). However, it is among the most common pathogens causing acute respiratory tract infections, even in adulthood. Its disease burden has been estimated to be similar to that of Influenza A in high-risk populations (Falsey et al., 2005; Nam and Ison, 2019). Pregnant women are more susceptible to respiratory infection, and RSV is responsible for up to 10–11% of this population’s acute respiratory tract infections (Beigi, 2014; Wheeler et al., 2015; Hause et al., 2019).
Several animal models have proven vertical transmission of RSV (Piedimonte et al., 2013; Piedimonte and Perez, 2014; Brown et al., 2017; Manti et al., 2018; Piedimonte and Harford, 2020). Moreover, RSV has been identified at birth in an infant presenting with respiratory distress symptoms, born to a woman with an elevated titer of antibodies against RSV (Manti et al., 2017). Accordingly, in a clinical study conducted at birth on 22 newborns from mothers who contracted RSV during the third trimester of pregnancy, anti-RSV IgA, IgM, and IgG titers were found in the cord blood samples (Manti et al., 2020). All these data together enforce the assumption that RSV may reach the fetus via the placenta and subsequently affect brain development (Andrade et al., 2021; Figure 3).
In addition to respiratory symptoms, RSV showed a neurotropism as assessed by several studies showing that RSV infection is associated with neurological manifestations, such as seizures, status epilepticus, encephalitis, neonatal encephalopathy with SIADH, hemiconvulsion–hemiplegia syndrome, persistent lethargy, feeding or swallowing difficulties, abnormalities of tone, and strabismus (Hirayama et al., 1999; Ng et al., 2001; Kho et al., 2004; Otake et al., 2007; Millichap and Wainwright, 2009; Picone et al., 2019). Additionally, RSV RNA was detected in the cerebrospinal fluid of patients presenting with RSV infection and seizures (Zlateva and Van Ranst, 2004; Kawashima et al., 2009). Moreover, CSF analysis of patients with RSV-associated neurological abnormalities showed increased levels of inflammatory cytokines, particularly IL-6 (Otake et al., 2007; Kawashima et al., 2009).
Following these reports, emerging research focused on the mechanisms through which RSV infection might disrupt brain development. In an in vivo model, Espinoza and colleagues proved that RSV infection can reach the CNS via the hematogenous pathway associated with leukocytes. The authors suggested RSV may cause behavioral and cognitive impairment through reduced synaptic plasticity in the hippocampal regions (Espinoza et al., 2013). Similarly, Bohmwald and colleagues reported a significant impairment of exploratory behavior in RSV-infected mice compared to controls. They also found increased levels of inflammatory cytokines (i.e., IL-4 and IL-10) and glial fibrillary acidic protein (GFAP) mRNA levels in the infected mice. Therefore, the authors suggested these alterations may contribute to cognitive impairment, as observed in other preclinical studies regarding Influenza virus and SARS-CoV-2 (Bohmwald et al., 2021).
Although no study has been conducted on the relationship between RSV and MIA, alterations in proinflammatory cytokine levels and their effects on brain development have been investigated in other respiratory viruses (Andrade et al., 2021). Nevertheless, RSV showed a differential tropism for distinct human placental cells, particularly the Hofbauer cells, which in turn produced increased levels of proinflammatory cytokines (i.e., IL-6, TNF-α, and IFN-γ; Bokun et al., 2019). All this evidence supports the potential role of the maternal immune response against RSV as an additional mechanism in disrupting brain development.
Other respiratory viral infections during pregnancy have been related to adverse neuropsychiatric outcomes in the offspring.
SARS-CoV-1 and Middle East respiratory syndrome (MERS) showed a weak neurotropism, and some reports of related neuropsychiatric sequelae had been described in infected adults, though infection complications in the offspring of affected mothers have not been investigated (Cheng et al., 2004; Arabi et al., 2015; Zimmer et al., 2021).
On the contrary, rubella’s effects on neurodevelopment have been widely investigated. Rubivirus is an enveloped single-stranded RNA virus belonging to the Matonaviridae family, and it is known to infect the fetal brain directly, causing neuronal death, gliosis, and a disruption of mitosis (Brown et al., 2000; Ejaz et al., 2022). Congenital rubella syndrome includes systemic symptoms, such as cataracts or congenital glaucoma, congenital heart disease, hearing impairment, and pigmentary retinopathy (Gordon-Lipkin et al., 2021). However, maternal infection may also cause neuropsychiatric conditions in the offspring. Brown and colleagues observed an increased rate of nonaffective psychosis in patients exposed to rubella during pregnancy (Brown et al., 2000). Following the 1964 pandemic, Chess found an increased prevalence of ASD in a retrospective observational study including 243 children who had been affected by rubella in utero (Chess, 1971). Moreover, a 10-to-15-fold increased risk of developing schizophrenia spectrum disorders and a decline in intelligence quotient between childhood and adolescence have been reported (Zimmer et al., 2021).
Finally, some reports in the literature examined the adverse outcome of respiratory viral infections without specifying the etiologic agents. Freedman and colleagues evaluated children born to mothers affected by upper respiratory infections during pregnancy. The authors reported a worse ability to maintain attention and bond to their parents when maternal choline levels were lower than 7.5 μM, suggesting a protective role of choline in fetal behavioral development, even if the viral infection occurred in early gestation (Freedman et al., 2020). Bear and colleagues performed a retrospective cohort study to analyze the association between gestational infections and cerebral palsy. They found a 2-fold increased risk of developing cerebral palsy in children whose mothers suffered from respiratory illness during pregnancy; the risk raised to almost 3-fold if the infection was diagnosed in the third trimester (Bear and Wu, 2016).
The physiological modifications occurring in pregnant women allow the survival of the fetus but increase susceptibility to infections and adverse offspring outcomes (Bear and Wu, 2016; Zimmer et al., 2021; Manti et al., 2022). The gestational age is a period featuring significant growth and changes in the CNS, making the brain vulnerable to intrauterine environmental insults throughout a protracted developmental window and with potentially long-lasting effects (Davis et al., 2011). A possible role of infection in the aetiology of psychotic diseases has been hypothesized for over a century (Torrey and Peterson, 1976; Khandaker et al., 2013). To date, a strong association between neuropsychiatric disorders and prenatal viral infections, especially respiratory viruses, has been outlined (Zimmer et al., 2021; San Martín-González et al., 2023).
Respiratory viruses can interfere with CNS development both directly, through vertical transmission, and indirectly, by inducing placental hypoxia and affecting its physiologic functioning (Lye et al., 2021; Liu et al., 2022; Manti et al., 2022). Additionally, numerous studies investigated the possible role of MIA and cytokines effects on the developing brain, resulting in lifelong neurodevelopmental disorders. The disproportionate maternal immune response can interfere with the placental capacity to provide nutrients and crucial precursors for normal fetal development. Furthermore, cytokines contribute to placental regulation, and their excessive production has been implicated in the dysfunction of developmental processes and derangement of physiological fetal homeostasis (Knuesel et al., 2014; Woods et al., 2023). Respiratory viral infections have been frequently reported in pregnant women, and the recent pandemics raised some concerns regarding the neurodevelopmental outcomes of the children born to these mothers (San Martín-González et al., 2023; Sturrock et al., 2023). However, they offered a remarkable opportunity to understand better the effects of prenatal infections on the offspring’s neurodevelopmental outcomes.
Particularly, the association between the Influenza virus and neuropsychiatric disorders has been extensively studied throughout the last century, proving an increased risk for conditions involving cognitive and social abilities in children and adults (i.e., schizophrenia, autism, and bipolar disorder with psychotic symptoms). Data from recent systematic reviews indicate that exposure to Influenza may be more harmful during the early stages of gestation (Torrey and Peterson, 1976; Brown and Derkits, 2010; Zerbo et al., 2011; Fatemi et al., 2012; Khandaker et al., 2013; Canetta et al., 2014; Zimmer et al., 2021; Fung et al., 2022).
On the contrary, given the recent onset of the COVID-19 pandemic, the literature addressing neuropsychiatric consequences of the SARS-CoV-2 infection during pregnancy was mainly focused on early infancy. Overall, an impairment of the fine motor abilities, associated or not with global developmental delay, is the most reported outcome in children born to mothers affected by SARS-CoV-2 during gestation (Cheng et al., 2021; Aldrete-Cortez et al., 2022; Ayed et al., 2022; Liu et al., 2022; Mulkey et al., 2022). Moreover, though not statistically significant, lower scores in the personal-social domains have been reported in several researches (Cheng et al., 2021; Wu et al., 2021; Mulkey et al., 2022; Shuffrey et al., 2022).
Despite the scarcity of literature regarding the neuropsychiatric disorders that could affect offspring born to mothers with prenatal RSV infection, consistent data reported neurological sequelae in children with RSV infection in the perinatal epoch or during the early months of life (Ng et al., 2001; Kho et al., 2004; Millichap and Wainwright, 2009; Picone et al., 2019). Emerging findings support the capacity of RSV to cross the placental-fetal interface, inducing respiratory conditions overlapping the symptoms of a postnatal RSV infection (Manti et al., 2017, 2020). Therefore, it is reasonable to hypothesize that neurological complications could also manifest after RSV infection during pregnancy, and the possible consequences should also be considered.
Early identification of brain developmental trajectories in children linked to maternal respiratory infections is crucial to conceiving preventive strategies for clinical outcomes. In light of the above data, vaccinations, antiviral therapy, and immune modulators are urgently required to minimize maternal infection, fetal transmission, and an “inappropriate” excessive immune response. It is worth considering that potent adjuvants could affect the cytokine balance at the fetal–maternal interface (Gerdts et al., 2016); therefore, inactivated vaccines against the most common viral agents before and during the pregnancy have been considered safe and strongly recommended (Knuesel et al., 2014). Very recently, the US Food and Drug Administration approved the administration of an RSV vaccine to pregnant people as a single injected dose during the third trimester (Harris, 2023). Especially during the early gestation stages, when the offspring CNS is more vulnerable to infectious triggers, vaccinations and monoclonal antibodies could provide adequate protection for the mother and the fetus, leading to better short- and long-term outcomes (Manti et al., 2022).
In parallel, recognizing altered developmental trajectories allows a prompt intervention that can improve the neurodevelopmental outcome of children (Liu et al., 2022). Accordingly, neuropsychiatric follow-up is advisable in newborns of mothers affected by respiratory infections during pregnancy. Finally, more research is necessary to assess the long-term consequences of SARS-CoV-2 infection during pregnancy on the offspring’s neuropsychiatric outcome and to detect adverse neurological manifestations possibly related to RSV maternal infection.
SM: Data curation, Methodology, Resources, Visualization, Writing – review & editing. GS: Data curation, Methodology, Software, Visualization, Writing – original draft, Writing – review & editing. AN: Data curation, Software, Validation, Visualization, Writing – review & editing. GR: Validation, Visualization, Writing – review & editing. GP: Conceptualization, Funding acquisition, Resources, Supervision, Validation, Visualization, Writing – review & editing.
The author(s) declare financial support was received for the research, authorship, and/or publication of this article. This research was supported in part by grant NIH/NHLBI HL-061007 to Dr. Giovanni Piedimonte.
The authors declare that the research was conducted in the absence of any commercial or financial relationships that could be construed as a potential conflict of interest.
The author(s) declared that they were an editorial board member of Frontiers, at the time of submission. This had no impact on the peer review process and the final decision.
All claims expressed in this article are solely those of the authors and do not necessarily represent those of their affiliated organizations, or those of the publisher, the editors and the reviewers. Any product that may be evaluated in this article, or claim that may be made by its manufacturer, is not guaranteed or endorsed by the publisher.
Adams, W., Kendell, R. E., Hare, E. H., and Munk-Jørgensen, P. (1993). Epidemiological evidence that maternal influenza contributes to the aetiology of schizophrenia. An analysis of Scottish, English, and Danish data. Br. J. Psychiatry J. Ment. Sci. 163, 522–534. doi: 10.1192/bjp.163.4.522
Aldrete-Cortez, V., Bobadilla, L., Tafoya, S. A., Gonzalez-Carpinteiro, A., Nava, F., Viñals, C., et al. (2022). Infants prenatally exposed to SARS-CoV-2 show the absence of fidgety movements and are at higher risk for neurological disorders: a comparative study. PLoS One 17:e0267575. doi: 10.1371/journal.pone.0267575
Amore, G., Spoto, G., Ieni, A., Vetri, L., Quatrosi, G., Di Rosa, G., et al. (2021). A focus on the cerebellum: from embryogenesis to an age-related clinical perspective. Front. Syst. Neurosci. 15:646052. doi: 10.3389/fnsys.2021.646052
Andeweg, S. P., Schepp, R. M., van de Kassteele, J., Mollema, L., Berbers, G. A. M., and van Boven, M. (2021). Population-based serology reveals risk factors for RSV infection in children younger than 5 years. Sci. Rep. 11:8953. doi: 10.1038/s41598-021-88524-w
Andrade, C. A., Kalergis, A. M., and Bohmwald, K. (2021). Potential neurocognitive symptoms due to respiratory syncytial virus infection. Pathogens 11:47. doi: 10.3390/pathogens11010047
Arabi, Y. M., Harthi, A., Hussein, J., Bouchama, A., Johani, S., Hajeer, A. H., et al. (2015). Severe neurologic syndrome associated with Middle East respiratory syndrome corona virus (MERS-CoV). Infection 43, 495–501. doi: 10.1007/s15010-015-0720-y
Ayed, M., Embaireeg, A., Kartam, M., More, K., Alqallaf, M., AlNafisi, A., et al. (2022). Neurodevelopmental outcomes of infants born to mothers with SARS-CoV-2 infections during pregnancy: a national prospective study in Kuwait. BMC Pediatr. 22:319. doi: 10.1186/s12887-022-03359-2
Azevedo, F. A., Carvalho, L. R., Grinberg, L. T., Farfel, J. M., Ferretti, R. E., Leite, R. E., et al. (2009). Equal numbers of neuronal and nonneuronal cells make the human brain an isometrically scaled-up primate brain. J. Comp. Neurol. 513, 532–541. doi: 10.1002/cne.21974
Baig, A. M., Khaleeq, A., Ali, U., and Syeda, H. (2020). Evidence of the COVID-19 virus targeting the CNS: tissue distribution, host-virus interaction, and proposed neurotropic mechanisms. ACS Chem. Neurosci. 11, 995–998. doi: 10.1021/acschemneuro.0c00122
Barker, D. J. (1990). The fetal and infant origins of adult disease. BMJ (Clinical research ed.) 301:1111. doi: 10.1136/bmj.301.6761.1111
Bear, J. J., and Wu, Y. W. (2016). Maternal infections during pregnancy and cerebral palsy in the child. Pediatr. Neurol. 57, 74–79. doi: 10.1016/j.pediatrneurol.2015.12.018
Beigi, R. H. (2014). Prevention and management of influenza in pregnancy. Obstet. Gynecol. Clin. N. Am. 41, 535–546. doi: 10.1016/j.ogc.2014.08.002
Beslow, L. A., Linds, A. B., Fox, C. K., Kossorotoff, M., Zuñiga Zambrano, Y. C., Hernández-Chávez, M., et al. (2021). Pediatric ischemic stroke: an infrequent complication of SARS-CoV-2. Ann. Neurol. 89, 657–665. doi: 10.1002/ana.25991
Bohmwald, K., Soto, J. A., Andrade-Parra, C., Fernández-Fierro, A., Espinoza, J. A., Ríos, M., et al. (2021). Lung pathology due to hRSV infection impairs blood-brain barrier permeability enabling astrocyte infection and a long-lasting inflammation in the CNS. Brain Behav. Immun. 91, 159–171. doi: 10.1016/j.bbi.2020.09.021
Bokun, V., Moore, J. J., Moore, R., Smallcombe, C. C., Harford, T. J., Rezaee, F., et al. (2019). Respiratory syncytial virus exhibits differential tropism for distinct human placental cell types with Hofbauer cells acting as a permissive reservoir for infection. PLoS One 14:e0225767. doi: 10.1371/journal.pone.0225767
Bonnin, A., Goeden, N., Chen, K., Wilson, M. L., King, J., Shih, J. C., et al. (2011). A transient placental source of serotonin for the fetal forebrain. Nature 472, 347–350. doi: 10.1038/nature09972
Borren, I., Tambs, K., Gustavson, K., Schjølberg, S., Eriksen, W., Håberg, S. E., et al. (2018). Early prenatal exposure to pandemic influenza a (H1N1) infection and child psychomotor development at 6 months – a population-based cohort study. Early Hum. Dev. 122, 1–7. doi: 10.1016/j.earlhumdev.2018.05.005
Borsani, E., Della Vedova, A. M., Rezzani, R., Rodella, L. F., and Cristini, C. (2019). Correlation between human nervous system development and acquisition of fetal skills: an overview. Brain Dev. 41, 225–233. doi: 10.1016/j.braindev.2018.10.009
Brogna, C., Brogna, B., De Biase, M., Sini, F., Mirra, F., Moro, M., et al. (2022). Perinatal cerebral ischemic lesion and SARS-CoV-2 infection during pregnancy: a case report and a review of the literature. J. Clin. Med. 11:6827. doi: 10.3390/jcm11226827
Brown, A. S. (2006). Prenatal infection as a risk factor for schizophrenia. Schizophr. Bull. 32, 200–202. doi: 10.1093/schbul/sbj052
Brown, A. S., Cohen, P., Greenwald, S., and Susser, E. (2000). Nonaffective psychosis after prenatal exposure to rubella. Am. J. Psychiatry 157, 438–443. doi: 10.1176/appi.ajp.157.3.438
Brown, A. S., and Derkits, E. J. (2010). Prenatal infection and schizophrenia: a review of epidemiologic and translational studies. Am. J. Psychiatry 167, 261–280. doi: 10.1176/appi.ajp.2009.09030361
Brown, P. M., Harford, T. J., Agrawal, V., Yen-Lieberman, B., Rezaee, F., and Piedimonte, G. (2017). Prenatal exposure to respiratory syncytial virus alters postnatal immunity and airway smooth muscle contractility during early-life reinfections. PLoS One 12:e0168786. doi: 10.1371/journal.pone.0168786
Bystron, I., Blakemore, C., and Rakic, P. (2008). Development of the human cerebral cortex: Boulder committee revisited. Nat. Rev. Neurosci. 9, 110–122. doi: 10.1038/nrn2252
Campi, F., Longo, D., Bersani, I., Savarese, I., Lucignani, G., Haass, C., et al. (2022). Neonatal cerebral venous thrombosis following maternal SARS-CoV-2 infection in pregnancy. Neonatology 119, 268–272. doi: 10.1159/000520537
Canetta, S. E., Bao, Y., Co, M. D., Ennis, F. A., Cruz, J., Terajima, M., et al. (2014). Serological documentation of maternal influenza exposure and bipolar disorder in adult offspring. Am. J. Psychiatry 171, 557–563. doi: 10.1176/appi.ajp.2013.13070943
Cheng, Y., Teng, H., Xiao, Y., Yao, M., Yin, J., and Sun, G. (2021). Impact of SARS-CoV-2 infection during pregnancy on infant neurobehavioral development: a case-control study. Front. Pediatr. 9:762684. doi: 10.3389/fped.2021.762684
Cheng, S. K., Tsang, J. S., Ku, K. H., Wong, C. W., and Ng, Y. K. (2004). Psychiatric complications in patients with severe acute respiratory syndrome (SARS) during the acute treatment phase: a series of 10 cases. Br. J. Psychiatry J. Ment. Sci. 184, 359–360. doi: 10.1192/bjp.184.4.359
Chess, S. (1971). Autism in children with congenital rubella. J. Autism Child. Schizophr. 1, 33–47. doi: 10.1007/BF01537741
Cleal, J. K., and Lewis, R. M. (2008). The mechanisms and regulation of placental amino acid transport to the human foetus. J. Neuroendocrinol. 20, 419–426. doi: 10.1111/j.1365-2826.2008.01662.x
Cui, C., Xu, P., Li, G., Qiao, Y., Han, W., Geng, C., et al. (2019). Vitamin D receptor activation regulates microglia polarization and oxidative stress in spontaneously hypertensive rats and angiotensin II-exposed microglial cells: role of renin-angiotensin system. Redox Biol. 26:101295. doi: 10.1016/j.redox.2019.101295
Dahlgren, J., Samuelsson, A. M., Jansson, T., and Holmäng, A. (2006). Interleukin-6 in the maternal circulation reaches the rat fetus in mid-gestation. Pediatr. Res. 60, 147–151. doi: 10.1203/01.pdr.0000230026.74139.18
Davis, E. P., Glynn, L. M., Waffarn, F., and Sandman, C. A. (2011). Prenatal maternal stress programs infant stress regulation. J. Child Psychol. Psychiatry 52, 119–129. doi: 10.1111/j.1469-7610.2010.02314.x
Dicanio, D., Spoto, G., Alibrandi, A., Minutoli, R., Nicotera, A. G., and Di Rosa, G. (2022). Long-term predictivity of early neurological assessment and developmental trajectories in low-risk preterm infants. Front. Neurol. 13:958682. doi: 10.3389/fneur.2022.958682
Dube, R., and Kar, S. S. (2020). COVID-19 in pregnancy: the foetal perspective-a systematic review. BMJ Paediatr. Open 4:e000859. doi: 10.1136/bmjpo-2020-000859
Edlow, A. G., Castro, V. M., Shook, L. L., Kaimal, A. J., and Perlis, R. H. (2022). Neurodevelopmental outcomes at 1 year in infants of mothers who tested positive for SARS-CoV-2 during pregnancy. JAMA Netw. Open 5:e2215787. doi: 10.1001/jamanetworkopen.2022.15787
Ejaz, H., Zeeshan, H. M., Iqbal, A., Ahmad, S., Ahmad, F., Abdalla, A. E., et al. (2022). Rubella virus infections: a bibliometric analysis of the scientific literature from 2000 to 2021. Healthcare 10:2562. doi: 10.3390/healthcare10122562
Elgueta, D., Murgas, P., Riquelme, E., Yang, G., and Cancino, G. I. (2022). Consequences of viral infection and cytokine production during pregnancy on brain development in offspring. Front. Immunol. 13:816619. doi: 10.3389/fimmu.2022.816619
Engert, V., Siauw, C., Stock, A., Rehn, M., Wöckel, A., Härtel, C., et al. (2021). Severe brain damage in a moderate preterm infant as complication of post-COVID-19 response during pregnancy. Neonatology 118, 505–508. doi: 10.1159/000516846
Erlebacher, A. (2013). Mechanisms of T cell tolerance towards the allogeneic fetus. Nat. Rev. Immunol. 13, 23–33. doi: 10.1038/nri3361
Espinoza, J. A., Bohmwald, K., Céspedes, P. F., Gómez, R. S., Riquelme, S. A., Cortés, C. M., et al. (2013). Impaired learning resulting from respiratory syncytial virus infection. Proc. Natl. Acad. Sci. U. S. A. 110, 9112–9117. doi: 10.1073/pnas.1217508110
Fahmi, A., Brügger, M., Démoulins, T., Zumkehr, B., Oliveira Esteves, B. I., Bracher, L., et al. (2021). SARS-CoV-2 can infect and propagate in human placenta explants. Cell reports. Medicine 2:100456. doi: 10.1016/j.xcrm.2021.100456
Falsey, A. R., Hennessey, P. A., Formica, M. A., Cox, C., and Walsh, E. E. (2005). Respiratory syncytial virus infection in elderly and high-risk adults. N. Engl. J. Med. 352, 1749–1759. doi: 10.1056/NEJMoa043951
Fatemi, S. H., Folsom, T. D., Rooney, R. J., Mori, S., Kornfield, T. E., Reutiman, T. J., et al. (2012). The viral theory of schizophrenia revisited: abnormal placental gene expression and structural changes with lack of evidence for H1N1 viral presence in placentae of infected mice or brains of exposed offspring. Neuropharmacology 62, 1290–1298. doi: 10.1016/j.neuropharm.2011.01.011
Favrais, G., van de Looij, Y., Fleiss, B., Ramanantsoa, N., Bonnin, P., Stoltenburg-Didinger, G., et al. (2011). Systemic inflammation disrupts the developmental program of white matter. Ann. Neurol. 70, 550–565. doi: 10.1002/ana.22489
Fenizia, C., Biasin, M., Cetin, I., Vergani, P., Mileto, D., Spinillo, A., et al. (2020). Analysis of SARS-CoV-2 vertical transmission during pregnancy. Nat. Commun. 11:5128. doi: 10.1038/s41467-020-18933-4
Freedman, R., Hunter, S. K., Law, A. J., D’Alessandro, A., Noonan, K., Wyrwa, A., et al. (2020). Maternal choline and respiratory coronavirus effects on fetal brain development. J. Psychiatr. Res. 128, 1–4. doi: 10.1016/j.jpsychires.2020.05.019
Fung, S. G., Fakhraei, R., Condran, G., Regan, A. K., Dimanlig-Cruz, S., Ricci, C., et al. (2022). Neuropsychiatric outcomes in offspring after fetal exposure to maternal influenza infection during pregnancy: a systematic review. Reprod. Toxicol. 113, 155–169. doi: 10.1016/j.reprotox.2022.09.002
Garbett, K. A., Hsiao, E. Y., Kálmán, S., Patterson, P. H., and Mirnics, K. (2012). Effects of maternal immune activation on gene expression patterns in the fetal brain. Transl. Psychiatry 2:e98. doi: 10.1038/tp.2012.24
Gerdts, V., Van Drunen Littel-van den Hurk, S., and Potter, A. (2016). Protection of neonates and infants by maternal immunization. Expert Rev. Vaccines 15, 1347–1349. doi: 10.1080/14760584.2016.1195266
Gervasi, M. T., Romero, R., Cainelli, E., Veronese, P., Tran, M. R., Jung, E., et al. (2022). Intra-amniotic inflammation in the mid-trimester of pregnancy is a risk factor for neuropsychological disorders in childhood. J. Perinat. Med. 51, 363–378. doi: 10.1515/jpm-2022-0255
Gibney, S. M., McGuinness, B., Prendergast, C., Harkin, A., and Connor, T. J. (2013). Poly I:C-induced activation of the immune response is accompanied by depression and anxiety-like behaviours, kynurenine pathway activation and reduced BDNF expression. Brain Behav. Immun. 28, 170–181. doi: 10.1016/j.bbi.2012.11.010
Girard, S., and Sebire, G. (2016). Transplacental transfer of Interleukin-1 receptor agonist and antagonist following maternal immune activation. Am. J. Reproduct. Immunol. 75, 8–12. doi: 10.1111/aji.12444
Gordon-Lipkin, E., Hoon, A., and Pardo, C. A. (2021). Prenatal cytomegalovirus, rubella, and Zika virus infections associated with developmental disabilities: past, present, and future. Dev. Med. Child Neurol. 63, 135–143. doi: 10.1111/dmcn.14682
Hadders-Algra, M. (2021). Early diagnostics and early intervention in neurodevelopmental disorders-age-dependent challenges and opportunities. J. Clin. Med. 10:861. doi: 10.3390/jcm10040861
Han, V. X., Patel, S., Jones, H. F., Nielsen, T. C., Mohammad, S. S., Hofer, M. J., et al. (2021). Maternal acute and chronic inflammation in pregnancy is associated with common neurodevelopmental disorders: a systematic review. Transl. Psychiatry 11:71. doi: 10.1038/s41398-021-01198-w
Hare, E. H., Price, J. S., and Slater, E. (1972). Schizophrenia and season of birth. Br. J. Psychiatry J. Ment. Sci. 120, 124–125. doi: 10.1192/bjp.120.554.124-a
Harry, G. J., Lawler, C., and Brunssen, S. H. (2006). Maternal infection and white matter toxicity. Neurotoxicology 27, 658–670. doi: 10.1016/j.neuro.2006.05.004
Hause, A. M., Avadhanula, V., Maccato, M. L., Pinell, P. M., Bond, N., Santarcangelo, P., et al. (2019). Clinical characteristics and outcomes of respiratory syncytial virus infection in pregnant women. Vaccine 37, 3464–3471. doi: 10.1016/j.vaccine.2019.04.098
Henstridge, C. M., Tzioras, M., and Paolicelli, R. C. (2019). Glial contribution to excitatory and inhibitory synapse loss in neurodegeneration. Front. Cell. Neurosci. 13:63. doi: 10.3389/fncel.2019.00063
Hillier, S. L., Nugent, R. P., Eschenbach, D. A., Krohn, M. A., Gibbs, R. S., Martin, D. H., et al. (1995). Association between bacterial vaginosis and preterm delivery of a low-birth-weight infant. The vaginal infections and prematurity study group. N. Engl. J. Med. 333, 1737–1742. doi: 10.1056/NEJM199512283332604
Hirayama, K., Sakazaki, H., Murakami, S., Yonezawa, S., Fujimoto, K., Seto, T., et al. (1999). Sequential MRI, SPECT and PET in respiratory syncytial virus encephalitis. Pediatr. Radiol. 29, 282–286. doi: 10.1007/s002470050589
Izumoto, Y., Inoue, S., and Yasuda, N. (1999). Schizophrenia and the influenza epidemics of 1957 in Japan. Biol. Psychiatry 46, 119–124. doi: 10.1016/s0006-3223(98)00359-x
Jiang, H. Y., Xu, L. L., Shao, L., Xia, R. M., Yu, Z. H., Ling, Z. X., et al. (2016). Maternal infection during pregnancy and risk of autism spectrum disorders: a systematic review and meta-analysis. Brain Behav. Immun. 58, 165–172. doi: 10.1016/j.bbi.2016.06.005
Kawashima, H., Ioi, H., Ushio, M., Yamanaka, G., Matsumoto, S., and Nakayama, T. (2009). Cerebrospinal fluid analysis in children with seizures from respiratory syncytial virus infection. Scand. J. Infect. Dis. 41, 228–231. doi: 10.1080/00365540802669543
Kazakova, A., Kakkola, L., Päkkilä, H., Teros-Jaakkola, T., Soukka, T., Peltola, V., et al. (2019). Serological Array-in-well multiplex assay reveals a high rate of respiratory virus infections and reinfections in young children. mSphere 4, e00447–e00419. doi: 10.1128/mSphere.00447-19
Keyserling, H. L. (1997). Other viral agents of perinatal importance. Varicella, parvovirus, respiratory syncytial virus, and enterovirus. Clin. Perinatol. 24, 193–211. doi: 10.1016/S0095-5108(18)30192-1
Khandaker, G. M., Zimbron, J., Lewis, G., and Jones, P. B. (2013). Prenatal maternal infection, neurodevelopment and adult schizophrenia: a systematic review of population-based studies. Psychol. Med. 43, 239–257. doi: 10.1017/S0033291712000736
Kho, N., Kerrigan, J. F., Tong, T., Browne, R., and Knilans, J. (2004). Respiratory syncytial virus infection and neurologic abnormalities: retrospective cohort study. J. Child Neurol. 19, 859–864. doi: 10.1177/08830738040190110301
Knuesel, I., Chicha, L., Britschgi, M., Schobel, S. A., Bodmer, M., Hellings, J. A., et al. (2014). Maternal immune activation and abnormal brain development across CNS disorders. Nat. Rev. Neurol. 10, 643–660. doi: 10.1038/nrneurol.2014.187
Kostović, I., Judas, M., Petanjek, Z., and Simić, G. (1995). Ontogenesis of goal-directed behavior: anatomo-functional considerations. Int. J. Psychophysiol. 19, 85–102. doi: 10.1016/0167-8760(94)00081-o
Kowash, H. M., Potter, H. G., Woods, R. M., Ashton, N., Hager, R., Neill, J. C., et al. (2022). Maternal immune activation in rats induces dysfunction of placental leucine transport and alters fetal brain growth. Clin. Sci. 136, 1117–1137. doi: 10.1042/CS20220245
Kunugi, H., Nanko, S., Takei, N., Saito, K., Hayashi, N., and Kazamatsuri, H. (1995). Schizophrenia following in utero exposure to the 1957 influenza epidemics in Japan. Am. J. Psychiatry 152, 450–452. doi: 10.1176/ajp.152.3.450
Kwon, H. K., Choi, G. B., and Huh, J. R. (2022). Maternal inflammation and its ramifications on fetal neurodevelopment. Trends Immunol. 43, 230–244. doi: 10.1016/j.it.2022.01.007
Lewis, R. M., Baskaran, H., Green, J., Tashev, S., Palaiologou, E., Lofthouse, E. M., et al. (2022). 3D visualization of trans-syncytial nanopores provides a pathway for paracellular diffusion across the human placental syncytiotrophoblast. iScience 25:105453. doi: 10.1016/j.isci.2022.105453
Li, M., Chen, L., Zhang, J., Xiong, C., and Li, X. (2020). The SARS-CoV-2 receptor ACE2 expression of maternal-fetal interface and fetal organs by single-cell transcriptome study. PLoS One 15:e0230295. doi: 10.1371/journal.pone.0230295
Limosin, F., Rouillon, F., Payan, C., Cohen, J. M., and Strub, N. (2003). Prenatal exposure to influenza as a risk factor for adult schizophrenia. Acta Psychiatr. Scand. 107, 331–335. doi: 10.1034/j.1600-0447.2003.00052.x
Liu, H. Y., Guo, J., Zeng, C., Cao, Y., Ran, R., Wu, T., et al. (2022). Transient early fine motor abnormalities in infants born to COVID-19 mothers are associated with placental hypoxia and ischemia. Front. Pediatr. 9:793561. doi: 10.3389/fped.2021.793561
Lye, P., Dunk, C. E., Zhang, J., Wei, Y., Nakpu, J., Hamada, H., et al. (2021). ACE2 is expressed in immune cells that infiltrate the placenta in infection-associated preterm birth. Cells 10:1724. doi: 10.3390/cells10071724
Machón, R. A., Mednick, S. A., and Schulsinger, F. (1987). Seasonality, birth complications and schizophrenia in a high risk sample. Br. J. Psychiatry J. Ment. Sci. 151, 122–124. doi: 10.1192/bjp.151.1.122
Manti, S., Cuppari, C., Lanzafame, A., Salpietro, C., Betta, P., Leonardi, S., et al. (2017). Detection of respiratory syncytial virus (RSV) at birth in a newborn with respiratory distress. Pediatr. Pulmonol. 52, E81–E84. doi: 10.1002/ppul.23775
Manti, S., Esper, F., Alejandro-Rodriguez, M., Leonardi, S., Betta, P., Cuppari, C., et al. (2020). Respiratory syncytial virus seropositivity at birth is associated with adverse neonatal respiratory outcomes. Pediatr. Pulmonol. 55, 3074–3079. doi: 10.1002/ppul.25001
Manti, S., Harford, T. J., Salpietro, C., Rezaee, F., and Piedimonte, G. (2018). Induction of high-mobility group Box-1 in vitro and in vivo by respiratory syncytial virus. Pediatr. Res. 83, 1049–1056. doi: 10.1038/pr.2018.6
Manti, S., Leonardi, S., Rezaee, F., Harford, T. J., Perez, M. K., and Piedimonte, G. (2022). Effects of vertical transmission of respiratory viruses to the offspring. Front. Immunol. 13:853009. doi: 10.3389/fimmu.2022.853009
Manti, S., Staiano, A., Orfeo, L., Midulla, F., Marseglia, G. L., Ghizzi, C., et al. (2023). UPDATE - 2022 Italian guidelines on the management of bronchiolitis in infants. Ital. J. Pediatr. 49:19. doi: 10.1186/s13052-022-01392-6
Manti, S., Xerra, F., Spoto, G., Butera, A., Gitto, E., Di Rosa, G., et al. (2023). Neurotrophins: expression of brain-lung Axis development. Int. J. Mol. Sci. 24:7089. doi: 10.3390/ijms24087089
Mednick, S. A., Huttunen, M. O., and Machón, R. A. (1994). Prenatal influenza infections and adult schizophrenia. Schizophr. Bull. 20, 263–267. doi: 10.1093/schbul/20.2.263
Mednick, S. A., Machon, R. A., Huttunen, M. O., and Bonett, D. (1988). Adult schizophrenia following prenatal exposure to an influenza epidemic. Arch. Gen. Psychiatry 45, 189–192. doi: 10.1001/archpsyc.1988.01800260109013
Millichap, J. J., and Wainwright, M. S. (2009). Neurological complications of respiratory syncytial virus infection: case series and review of literature. J. Child Neurol. 24, 1499–1503. doi: 10.1177/0883073808331362
Mulkey, S. B., Williams, M. E., Jadeed, N., Zhang, A., Israel, S., and DeBiasi, R. L. (2022). Neurodevelopment in infants with antenatal or early neonatal exposure to SARS-CoV-2. Early Hum. Dev. 175:105694. doi: 10.1016/j.earlhumdev.2022.105694
Nam, H. H., and Ison, M. G. (2019). Respiratory syncytial virus infection in adults. BMJ 366:l5021. doi: 10.1136/bmj.l5021
Ng, Y. T., Cox, C., Atkins, J., and Butler, I. J. (2001). Encephalopathy associated with respiratory syncytial virus bronchiolitis. J. Child Neurol. 16, 105–108. doi: 10.1177/088307380101600207
O’Callaghan, E., Sham, P., Takei, N., Glover, G., and Murray, R. M. (1991). Schizophrenia after prenatal exposure to 1957 A2 influenza epidemic. Lancet (London, England) 337, 1248–1250. doi: 10.1016/0140-6736(91)92919-s
Otake, Y., Yamagata, T., Morimoto, Y., Imi, M., Mori, M., Aihara, T., et al. (2007). Elevated CSF IL-6 in a patient with respiratory syncytial virus encephalopathy. Brain Dev. 29, 117–120. doi: 10.1016/j.braindev.2006.06.008
Palma-Gudiel, H., Eixarch, E., Crispi, F., Morán, S., Zannas, A. S., and Fañanás, L. (2019). Prenatal adverse environment is associated with epigenetic age deceleration at birth and hypomethylation at the hypoxia-responsive EP300 gene. Clin. Epigenetics 11:73. doi: 10.1186/s13148-019-0674-5
Parboosing, R., Bao, Y., Shen, L., Schaefer, C. A., and Brown, A. S. (2013). Gestational influenza and bipolar disorder in adult offspring. JAMA Psychiatry 70, 677–685. doi: 10.1001/jamapsychiatry.2013.896
Parkin, J., and Cohen, B. (2001). An overview of the immune system. Lancet 357, 1777–1789. doi: 10.1016/S0140-6736(00)04904-7
Perez-Palomar, B., Erdozain, A. M., Erkizia-Santamaría, I., Ortega, J. E., and Meana, J. J. (2023). Maternal immune activation induces cortical Catecholaminergic hypofunction and cognitive impairments in offspring. J. Neuroimmune Pharmacol. 18, 348–365. doi: 10.1007/s11481-023-10070-1
Picone, S., Mondì, V., Di Palma, F., Martini, L., and Paolillo, P. (2019). Neonatal encephalopathy and SIADH during RSV infection. Am. J. Perinatol. 36, S106–S109. doi: 10.1055/s-0039-1692132
Piedimonte, G. (2015). RSV infections: state of the art. Cleve. Clin. J. Med. 82, S13–S18. doi: 10.3949/ccjm.82.s1.03
Piedimonte, G., and Harford, T. J. (2020). Effects of maternal-fetal transmission of viruses and other environmental agents on lung development. Pediatr. Res. 87, 420–426. doi: 10.1038/s41390-019-0657-4
Piedimonte, G., and Perez, M. K. (2014). Alternative mechanisms for respiratory syncytial virus (RSV) infection and persistence: could RSV be transmitted through the placenta and persist into developing fetal lungs? Curr. Opin. Pharmacol. 16, 82–88. doi: 10.1016/j.coph.2014.03.008
Piedimonte, G., Walton, C., and Samsell, L. (2013). Vertical transmission of respiratory syncytial virus modulates pre- and postnatal innervation and reactivity of rat airways. PLoS One 8:e61309. doi: 10.1371/journal.pone.0061309
Saigal, S., and Doyle, L. W. (2008). An overview of mortality and sequelae of preterm birth from infancy to adulthood. Lancet 371, 261–269. doi: 10.1016/S0140-6736(08)60136-1
Saito, S. (2022). Reconsideration of the role of regulatory T cells during pregnancy: differential characteristics of regulatory T cells between the maternal-fetal Interface and peripheral sites and between early and late pregnancy. Med. Princ. Pract. 31, 403–414. doi: 10.1159/000527336
San Martín-González, N., Castro-Quintas, Á., Marques-Feixa, L., Ayesa-Arriola, R., López, M., and Fañanás, L. (2023). Maternal respiratory viral infections during pregnancy and offspring’s neurodevelopmental outcomes: a systematic review. Neurosci. Biobehav. Rev. 149:105178. doi: 10.1016/j.neubiorev.2023.105178
Sharps, M. C., Hayes, D. J. L., Lee, S., Zou, Z., Brady, C. A., Almoghrabi, Y., et al. (2020). A structured review of placental morphology and histopathological lesions associated with SARS-CoV-2 infection. Placenta 101, 13–29. doi: 10.1016/j.placenta.2020.08.018
Shi, L., Fatemi, S. H., Sidwell, R. W., and Patterson, P. H. (2003). Maternal influenza infection causes marked behavioral and pharmacological changes in the offspring. J. Neurosci. Off. J. Soc. Neurosci. 23, 297–302. doi: 10.1523/JNEUROSCI.23-01-00297.2003
Short, S. J., Lubach, G. R., Karasin, A. I., Olsen, C. W., Styner, M., Knickmeyer, R. C., et al. (2010). Maternal influenza infection during pregnancy impacts postnatal brain development in the rhesus monkey. Biol. Psychiatry 67, 965–973. doi: 10.1016/j.biopsych.2009.11.026
Shuffrey, L. C., Firestein, M. R., Kyle, M. H., Fields, A., Alcántara, C., Amso, D., et al. (2022). Association of birth during the COVID-19 pandemic with neurodevelopmental status at 6 months in infants with and without in utero exposure to maternal SARS-CoV-2 infection. JAMA Pediatr. 176:e215563. doi: 10.1001/jamapediatrics.2021.5563
Sloan, S. A., and Barres, B. A. (2014). Mechanisms of astrocyte development and their contributions to neurodevelopmental disorders. Curr. Opin. Neurobiol. 27, 75–81. doi: 10.1016/j.conb.2014.03.005
Sturrock, S., Ali, S., Gale, C., Battersby, C., and Le Doare, K. (2023). Neonatal outcomes and indirect consequences following maternal SARS-CoV-2 infection in pregnancy: a systematic review. BMJ Open 13:e063052. doi: 10.1136/bmjopen-2022-063052
Tau, G. Z., and Peterson, B. S. (2010). Normal development of brain circuits. Neuropsychopharmacology: official publication of the American college of. Neuropsychopharmacology 35, 147–168. doi: 10.1038/npp.2009.115
Tay, M. Z., Poh, C. M., Rénia, L., MacAry, P. A., and Ng, L. F. P. (2020). The trinity of COVID-19: immunity, inflammation and intervention. Nat. Rev. Immunol. 20, 363–374. doi: 10.1038/s41577-020-0311-8
Taylor, D. L., Pirianov, G., Holland, S., McGinnity, C. J., Norman, A. L., Reali, C., et al. (2010). Attenuation of proliferation in oligodendrocyte precursor cells by activated microglia. J. Neurosci. Res. 88, 1632–1644. doi: 10.1002/jnr.22335
Tioleco, N., Silberman, A. E., Stratigos, K., Banerjee-Basu, S., Spann, M. N., Whitaker, A. H., et al. (2021). Prenatal maternal infection and risk for autism in offspring: a meta-analysis. Autism Res 14, 1296–1316. doi: 10.1002/aur.2499
Tiwari, S. K., Wang, S., Smith, D., Carlin, A. F., and Rana, T. M. (2021). Revealing tissue-specific SARS-CoV-2 infection and host responses using human stem cell-derived lung and cerebral organoids. Stem Cell Rep. 16, 437–445. doi: 10.1016/j.stemcr.2021.02.005
Torrey, E. F., and Peterson, M. R. (1976). The viral hypothesis of schizophrenia. Schizophr. Bull. 2, 136–146. doi: 10.1093/schbul/2.1.136
Trivedi, R., Gupta, R. K., Husain, N., Rathore, R. K., Saksena, S., Srivastava, S., et al. (2009). Region-specific maturation of cerebral cortex in human fetal brain: diffusion tensor imaging and histology. Neuroradiology 51, 567–576. doi: 10.1007/s00234-009-0533-8
Veenstra van Nieuwenhoven, A. L., Heineman, M. J., and Faas, M. M. (2003). The immunology of successful pregnancy. Hum. Reprod. Update 9, 347–357. doi: 10.1093/humupd/dmg026
Vivanti, A. J., Vauloup-Fellous, C., Prevot, S., Zupan, V., Suffee, C., Do Cao, J., et al. (2020). Transplacental transmission of SARS-CoV-2 infection. Nat. Commun. 11:3572. doi: 10.1038/s41467-020-17436-6
Walle, K. M., Askeland, R. B., Gustavson, K., Mjaaland, S., Ystrom, E., Lipkin, W. I., et al. (2022). Risk of attention-deficit hyperactivity disorder in offspring of mothers with infections during pregnancy. JCPP Adv. 2:e12070. doi: 10.1002/jcv2.12070
Wheeler, S. M., Dotters-Katz, S., Heine, R. P., Grotegut, C. A., and Swamy, G. K. (2015). Maternal effects of respiratory syncytial virus infection during pregnancy. Emerg. Infect. Dis. 21, 1951–1955. doi: 10.3201/eid2111.150497
Woods, R. M., Lorusso, J. M., Fletcher, J., ElTaher, H., McEwan, F., Harris, I., et al. (2023). Maternal immune activation and role of placenta in the prenatal programming of neurodevelopmental disorders. Neuronal Signal. 7:NS20220064. doi: 10.1042/NS20220064
Wu, T., Chen, L., Wang, Y., Shi, H., Niu, J., Yin, X., et al. (2021). Effects of SARS-CoV-2 infection during late pregnancy on early childhood development: a prospective cohort study. Front. Pediatr. 9:750012. doi: 10.3389/fped.2021.750012
Yang, L., Han, Y., Nilsson-Payant, B. E., Gupta, V., Wang, P., Duan, X., et al. (2020). A human pluripotent stem cell-based platform to study SARS-CoV-2 tropism and model virus infection in human cells and organoids. Cell Stem Cell 27, 125–136.e7. doi: 10.1016/j.stem.2020.06.015
Zerbo, O., Iosif, A. M., Delwiche, L., Walker, C., and Hertz-Picciotto, I. (2011). Month of conception and risk of autism. Epidemiology 22, 469–475. doi: 10.1097/EDE.0b013e31821d0b53
Zhu, C. Y., Jiang, H. Y., and Sun, J. J. (2022). Maternal infection during pregnancy and the risk of attention-deficit/hyperactivity disorder in the offspring: a systematic review and meta-analysis. Asian J. Psychiatr. 68:102972. doi: 10.1016/j.ajp.2021.102972
Zimmer, A., Youngblood, A., Adnane, A., Miller, B. J., and Goldsmith, D. R. (2021). Prenatal exposure to viral infection and neuropsychiatric disorders in offspring: a review of the literature and recommendations for the COVID-19 pandemic. Brain Behav. Immun. 91, 756–770. doi: 10.1016/j.bbi.2020.10.024
Keywords: influenza virus, maternal infection, neurodevelopmental outcome, respiratory syncytial virus, respiratory viral infections, severe acute respiratory syndrome coronavirus 2
Citation: Manti S, Spoto G, Nicotera AG, Di Rosa G and Piedimonte G (2024) Impact of respiratory viral infections during pregnancy on the neurological outcomes of the newborn: current knowledge. Front. Neurosci. 17:1320319. doi: 10.3389/fnins.2023.1320319
Received: 13 October 2023; Accepted: 14 December 2023;
Published: 08 January 2024.
Edited by:
Stavros Selemidis, RMIT University, AustraliaReviewed by:
Gisela Canedo-Marroquín, University of the Andes, ChileCopyright © 2024 Manti, Spoto, Nicotera, Di Rosa and Piedimonte. This is an open-access article distributed under the terms of the Creative Commons Attribution License (CC BY). The use, distribution or reproduction in other forums is permitted, provided the original author(s) and the copyright owner(s) are credited and that the original publication in this journal is cited, in accordance with accepted academic practice. No use, distribution or reproduction is permitted which does not comply with these terms.
*Correspondence: Giovanni Piedimonte, Z3BpZWRpbW9udGVAdHVsYW5lLmVkdQ==
†These authors have contributed equally to this work
Disclaimer: All claims expressed in this article are solely those of the authors and do not necessarily represent those of their affiliated organizations, or those of the publisher, the editors and the reviewers. Any product that may be evaluated in this article or claim that may be made by its manufacturer is not guaranteed or endorsed by the publisher.
Research integrity at Frontiers
Learn more about the work of our research integrity team to safeguard the quality of each article we publish.