- 1Department of Psychology and Neuroscience, Dalhousie University, Halifax, NS, Canada
- 2Picower Institute for Learning and Memory, Department of Brain and Cognitive Sciences, Massachusetts Institute of Technology, Cambridge, MA, United States
- 3College of Optometry, University of Houston, Houston, TX, United States
- 4Department of Ophthalmology and Visual Sciences, Washington University School of Medicine, St. Louis, MO, United States
Amblyopia is a common visual impairment that develops during the early years of postnatal life. It emerges as a sequela to eye misalignment, an imbalanced refractive state, or obstruction to form vision. All of these conditions prevent normal vision and derail the typical development of neural connections within the visual system. Among the subtypes of amblyopia, the most debilitating and recalcitrant to treatment is deprivation amblyopia. Nevertheless, human studies focused on advancing the standard of care for amblyopia have largely avoided recruitment of patients with this rare but severe impairment subtype. In this review, we delineate characteristics of deprivation amblyopia and underscore the critical need for new and more effective therapy. Animal models offer a unique opportunity to address this unmet need by enabling the development of unconventional and potent amblyopia therapies that cannot be pioneered in humans. Insights derived from studies using animal models are discussed as potential therapeutic innovations for the remediation of deprivation amblyopia. Retinal inactivation is highlighted as an emerging therapy that exhibits efficacy against the effects of monocular deprivation at ages when conventional therapy is ineffective, and recovery occurs without apparent detriment to the treated eye.
Amblyopia and its subtypes
Normal development of the mammalian visual system begins with a prenatal sequence of patterned gene expression that interacts with spontaneous electrical activity to produce rudimentary neural circuits (Kuljis and Rakic, 1990). Although the primate visual system exhibits some mature physiological and anatomical properties at birth (Wiesel and Hubel, 1974; Horton and Hocking, 1996), its development at this stage is insufficient to support adult visual perception (Teller and Boothe, 1962). Human infants have limited visual acuity (Brown and Yamamoto, 1986) and are unable to assemble visual details into a whole percept (Cohen and Younger, 1984). Visual experience early in postnatal life directs the maturation of neural circuitry to optimize function of the visual system and produce clear binocular vision. The important synergy between visual experience and neural development is facilitated by a high capacity for neural plasticity that occurs naturally only early in postnatal life, during the so-called critical period (LeVay et al., 1980; Olson and Freeman, 1980; Hensch, 2005; Mitchell and Maurer, 2022). Although this is a formative stage in normal neural development, it also represents a time of vulnerability wherein impressionable neural circuits can be misguided by conditions that interfere with the piloting influence of normal concordant vision. Amblyopia is a visual impairment caused by aberrant neural development in the primary visual pathway resulting from early abnormal visual experience.
Amblyopia is the leading cause of monocular vision loss in the U.S., affecting approximately 2.2 million children (Friedman et al., 2009), and is the most common cause of monocular visual impairment in adults (Grant and Moseley, 2011). It develops as a sequela to misaligned eyes (strabismus), an imbalanced refractive state (anisometropia), or an obstruction to form vision (deprivation). Each of these conditions blocks binocular concordance and derails the typical development of neural connections, seeding persistent abnormalities within brain regions that assemble visual perception. Dysfunctions that result from this cascade of events are many, but foremost among them is a pronounced reduction in spatial acuity in the affected eye (Ellemberg et al., 2000), impairments to fine motor skill and coordination (Kelly et al., 2019), and a reduced or absent capacity for stereoscopic vision (Wallace et al., 2011).
Strabismic and anisometropic amblyopia are the most common subtypes of the disorder (Pediatric Eye Disease Investigator Group, 2002), with deprivation amblyopia being comparatively rare and representing only about 3–4% of amblyopia cases (Flynn and Cassady, 1978; Attebo et al., 1998). Although less common, deprivation amblyopia is by far the most severe subtype, and is the least responsive to conventional treatment. It derives from early onset monocular deprivation (MD) or binocular deprivation. While our focus will be on characteristics of MD, a comparison of the effects of monocular and binocular deprivation has been reviewed elsewhere (Maurer, 2017). The majority of published cases of deprivation amblyopia are due to cataract, which causes the disorder by obstructing the focus of images on the retina. Whereas anisometropic amblyopia started in infancy can take months to develop (Smith et al., 1985), 2 weeks of MD imposed at the same age is sufficient to produce a near complete loss of spatial vision in monkeys (Harwerth et al., 1981). Suppression of the amblyopic eye is stronger in children with deprivation amblyopia compared to those with anisometropic or strabismic amblyopia (Hamm et al., 2017). Treatment outcomes for amblyopia in infants with congenital unilateral cataract before the 1980’s were dismal. Recovery attempts were considered pointless (von Noorden and Maumenee, 1967), and some early published reports even recommended against therapy (Costenbader and Albert, 1957; Ryan and Maumenee, 1977).
Insights that emerged from the discovery of a critical period in animal studies measuring the effects of MD (Wiesel and Hubel, 1963a,b; Hubel et al., 1970) motivated new attempts to examine recovery potential in humans treated for unilateral congenital cataract very early in life. These studies revealed that recovery was possible under strict conditions that included removal of the cataract shortly after birth, provision of corrective contact lenses, and compliance with occlusion therapy (Beller et al., 1981; Birch et al., 1986; Birch and Stager, 1988). Under these ideal circumstances, treated infants under 4 months of age were able to achieve visual acuity that was better than previously thought possible, but was sometimes still within the range of low vision and well short of normal acuity (Figure 1). Infants treated beyond 4 months of age suffered far worse outcomes that range from about 20/160 visual acuity to perception of hand motion (Birch et al., 1986; Birch and Stager, 1988). Even after an excellent standard of care delivered in the prospective National Eye Institute Infant Aphakia Treatment Study (NCT00212134), the average visual acuity achieved in children with MD was 20/160. Therefore, the common understanding that amblyopia can be successfully treated up to about 7 years of age (Holmes et al., 2011; Holmes and Levi, 2018) does not apply to amblyopia caused by MD. Instead, the disorder appears to express an early and ephemeral response to treatment and can improve only under strict therapeutic conditions. For these reasons it has been recommended that surgery, optical correction, and occlusion therapy be implemented before 6 weeks of age to avoid debilitating impairment (Birch and Stager, 1996). Although better outcomes are achieved as the amount of patching increases, good outcomes occur only when there has been both early treatment and extensive patching (Birch et al., 1993; Lewis et al., 1995; Drews-Botsch et al., 2012). These characteristics underscore the severity of MD and distinguish it from the other amblyopia subtypes.
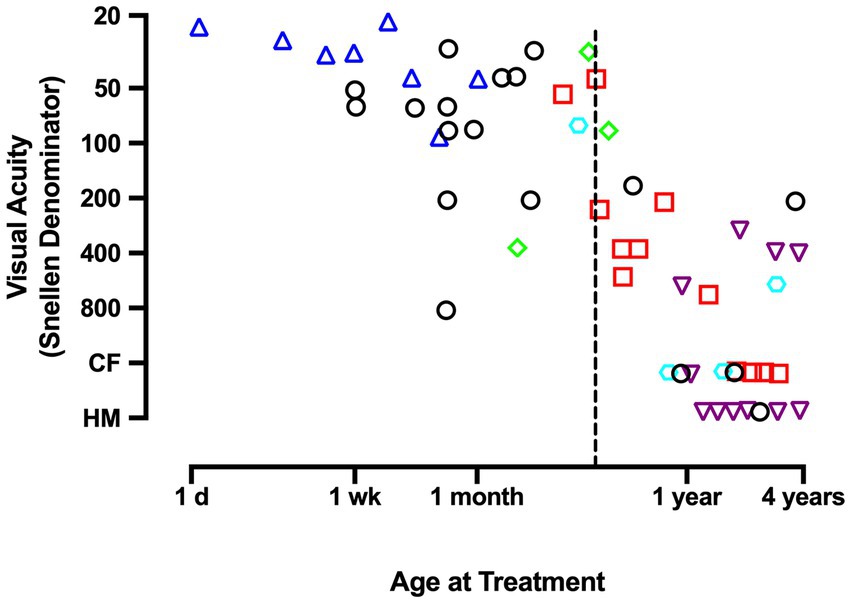
Figure 1. This graph plots the Snellen acuity achieved by the amblyopic eye as a function of the age at which a unilateral congenital cataract was removed and occlusion treatment began. Data demonstrate that, unlike other forms of amblyopia, effective treatment of deprivation amblyopia adheres to a short critical period in which, to promote optimal recovery from congenital MD, therapy must begin before the age of about 4 months (dashed vertical line). Therapy initiated beyond 4 months of age is associated with poor recovery outcomes. Graph displays results that were compiled by Birch and Stager (1988). Data originate from Beller et al., 1981 (triangles); Lewis et al., 1986 (squares); Pratt-Johnson and Tillson, 1981 (diamonds); Helveston et al., 1980 (hexagon); Awaya et al., 1979 (inverted triangles); Birch and Stager, 1988 (circles). CF indicates ability to count fingers; HM indicates the perception of hand movement.
Traditional monocular therapies have been employed for centuries and remain the gold-standard treatment for all types of amblyopia (Levi, 2020), but they are plagued by adherence issues (Stewart et al., 2003; Holmes and Levi, 2018), recurrence of amblyopia after treatment (Levartovsky et al., 1995; Jia et al., 2022), and negligible efficacy at older ages (Birch and Stager, 1988; Wallace et al., 2018). The social stigma associated with patching can raise stress and anxiety, and adversely impact the child–parent relationship (Awan et al., 2005; Loudon et al., 2009; Birch et al., 2019a). Moreover, monocular approaches like patching do not restore the loss of stereoscopic vision (Wallace et al., 2011), which is the most common binocular deficit of amblyopia (Webber and Wood, 2005). Therefore, in recent years, investigation into more effective treatments for human amblyopia has shifted focus away from traditional patching or penalizing the dominant eye to stimulate recovery (Koo et al., 2017). Emerging therapies investigated in humans aim to overcome the issues with monocular therapy through engaging and binocular-based approaches (reviewed in Levi, 2020; Bui Quoc et al., 2023). Some of the additional motivation to pursue binocular treatments has been recognition that the fellow eye is not “normal” and that there are significant binocular deficits that cannot be explained by monocular loss in acuity alone (Murphy et al., 2015; Meier and Giaschi, 2017; Birch et al., 2019c). Binocular treatments are designed to not only restore spatial acuity for the impaired eye but also to promote recovery of binocularity and stereopsis. Recovery of stereovision is an achievement that typically eludes traditional monocular therapies. Notwithstanding the potential benefits that these innovative amblyopia treatments may offer to patients, investigation of their efficacy has focused almost entirely on strabismic and anisometropic amblyopia (Hamm et al., 2017). The exclusion of patients with MD from these studies, as well as from National Eye Institute-sponsored Amblyopia Treatment Studies of the Pediatric Eye Disease Investigator Group (Chen and Cotter, 2016), derives from the obstinance and poor prognosis for recovery associated with this subtype of amblyopia.
Given the severity and intractable characteristics of deprivation amblyopia, and the paucity of human studies focused on its remediation, development of a novel treatment that provides superior recovery, and at older ages, would be clinically transformative. Broadening the age limits of successful treatment would alone represent a major discovery (Levi, 2020). A treatment innovation capable of promoting recovery from the most recalcitrant form of amblyopia may also have superior efficacy for the remediation of the less severe amblyopia subtypes: anisometropic and strabismic.
Animal models: strengths and limitations
Decades of research into the rootedness of amblyopia using animal models has overwhelmingly employed closing the lids of one eye to produce form MD. The ease of its production alongside the large, rapid, and consistent effects that it yields have made lid closure the most prolific method to produce amblyopia in animal models. This has occurred despite the fact that MD is the rarest and most recalcitrant form of amblyopia in humans (Flynn and Cassady, 1978; Attebo et al., 1998). Anisometropic and strabismic amblyopia are both effectively modeled in cats and monkeys (Kiorpes et al., 1998), and can occur naturally in these species (Berman and Payne, 1983; von Grünau and Rauschecker, 1983; Horton et al., 1997; Tychsen et al., 2004). Nevertheless, there are a dwindling number of animal studies that induce these more common forms of amblyopia to investigate etiology and recovery. Increasing use of anisometropia and strabismus to model amblyopia would enable better correspondence with the diversity of amblyopia subtypes in the human population and would also produce better alignment between animal work and the majority of human studies that examine only these conditions.
Although the preponderance of MD studies in animal models may present challenges for expeditious clinical translation across amblyopia subtypes, insights gained from studies using MD continue to be at the vanguard of our knowledge about the regulators of neural plasticity as well as the organization and development of the primary visual pathway. The robust effects of MD in animals facilitates exploration of unconventional approaches to therapy that cannot be developed in human subjects. Further, effective treatments developed and tested in animal models using MD are likely to provide relief from all types of amblyopia for at least two reasons. First, strabismic, anisometropic and MD amblyopia all respond to the same treatments in the clinic (albeit with limitations indicated above). Second, therapy effective against the deepest and most obstinate form of amblyopia should also be efficacious for the more treatable subtypes. Using MD to model amblyopia sets the bar high; indeed, in monkeys the induced impairment can be more severe than that observed in humans (Kiorpes, 2019).
In recent years, the prolific use of rodents (particularly mice) in visual neuroscience has made them a standard model for investigating mechanisms of neural plasticity that underlie the emergence of amblyopia and that enable its recovery (Heynen et al., 2003; Morishita et al., 2010; Kaneko and Stryker, 2023). The mouse has a poorly differentiated visual system. Although the power of mouse genetics is undeniable, the primitive organization of the mouse visual system gives rise to limitations on what can be deduced in humans. Many characteristics that are the exclusive domain of cortex in higher mammals appear to be residual in rodents. This may offer interesting insight into the evolution of the visual system, but it does complicate direct translation of knowledge gained from rodent models to other species with more highly differentiated visual systems. The exorbitant expense, long gestational times, protracted postnatal development, and small litter sizes make mechanistic studies difficult to perform on cats and monkeys. Rodent studies have been paramount in delineating important characteristics of visual system plasticity including the discovery that the mature mammalian brain retains considerable capacity for neural plasticity beyond what was previously thought (Pizzorusso et al., 2002; Sawtell et al., 2003; He et al., 2007; Bavelier et al., 2010; Morishita et al., 2010; Fong et al., 2021). These findings have motivated investigation of the limits of plasticity and recovery from anisometropic and strabismic amblyopia in humans. Although some promising results have been observed (Sharif et al., 2019; Wu et al., 2023), translating these treatment innovations has not been straightforward (Repka et al., 2015; Sofi et al., 2016; Chung et al., 2017; Lagas et al., 2019).
Differences between the visual systems of rodents and humans may represent a formidable obstacle to the smooth transition from bench to bedside. Among the common animal models for amblyopia, visual spatial acuity is highest in monkeys (30 cycles / degree; Kiorpes, 1992), followed by cats (8–10 cycles / degree; Giffin and Mitchell, 1978; Murphy et al., 2015), then rats (1 cycle / degree; Prusky et al., 2000) and finally mice (0.5 cycles / degree; Prusky and Douglas, 2003). Monkeys and cats have forward-facing eyes and a large binocular zone in visual space. Rats and mice have lateral facing eyes and a small binocular zone with poor stereopsis (Baroncelli et al., 2013; Boone et al., 2021). The structure and function of the primary visual pathway in rats and mice is likewise substantially different. These burrowing rodents do not have a laminated lateral geniculate nucleus (LGN) with eye-specific layers, and unlike primates and carnivores, sensory receptive fields can exhibit both orientation selectivity and binocular responses (Suresh et al., 2016). Moreover, the responses of binocular neurons in murine LGN are modified by MD. This plasticity is not a passive reflection of feedback from V1 and appears to result from changes in the retinogeniculate synapses (Jaepel et al., 2017; Sommeijer et al., 2017; Huh et al., 2020). In primary visual cortex (V1), rodents do not have a human-like ocular dominance organization. Instead of stripes or patches of ocular dominance as is observed in human, monkey and cat, eye-specific geniculocortical inputs to mouse V1 are mixed within a single binocular zone dominated by input from the contralateral eye (Coleman et al., 2009). In rats, inputs to V1 exhibit regions of aggregated eye-specific input within the binocular zone, but these domains appear distinct from those observed in higher species (Laing et al., 2015). In comparison to humans, the overall amount of cortical territory taken up by V1 is markedly smaller for all commonly studied model species, including monkeys (Figure 2). The surface area of macaque monkey V1 is about 40% of human V1, and for cats this is even smaller at 15%. However, these size differences are dwarfed by those observed for rat and mouse V1, which are about 0.5% the area of human. The difference in potential computation capacity of V1, alongside their very poor visual ability, has fueled the view that rodents are suboptimal models for studies of human vision (Baker, 2013). Regarding studies of amblyopia, a notable conspicuous limitation to using rodents is that only deprivation amblyopia has been successfully modeled. The apparent inability for rodents to express the two most common subtypes of human amblyopia, namely anisometropic and strabismic amblyopia, presumably derives from their dissimilarities compared to humans, which include those already mentioned as well as others reviewed elsewhere (Espinosa and Stryker, 2012; Baker, 2013; Seabrook et al., 2017; Mitchell and Sengpiel, 2018).
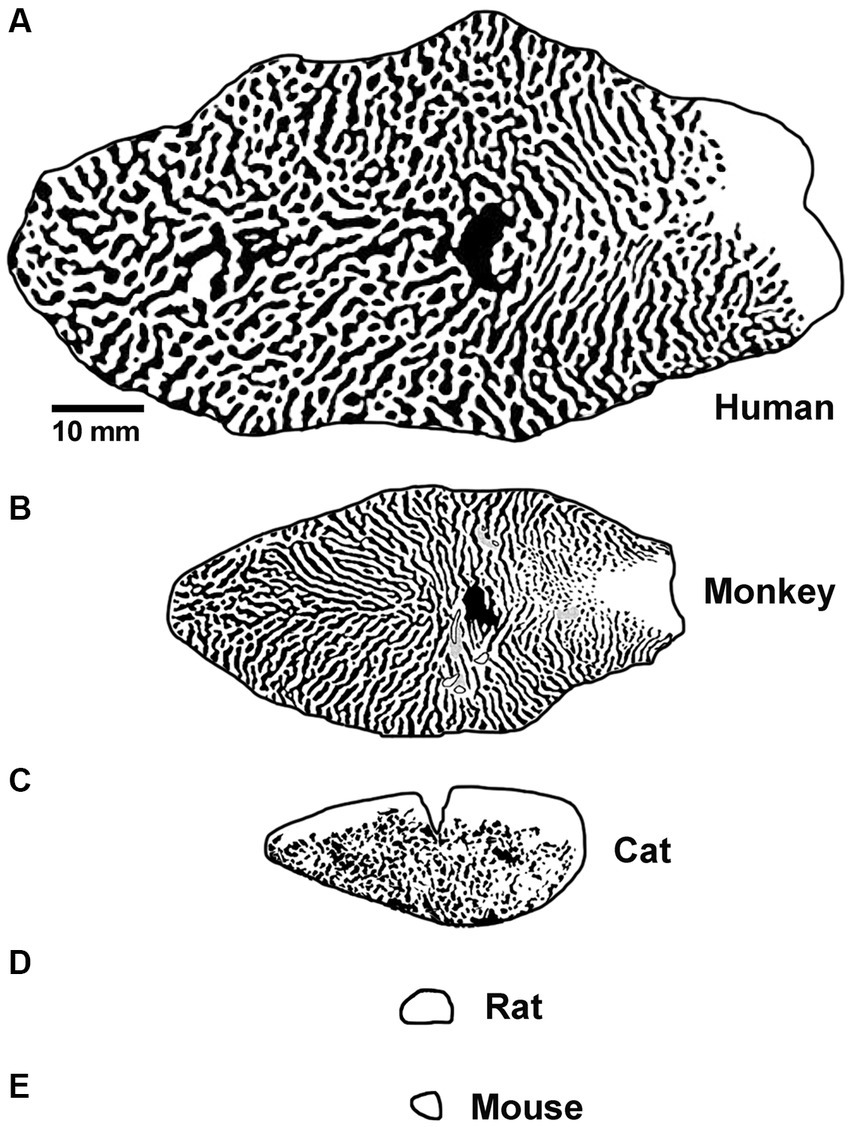
Figure 2. Graphical representation of the surface area and tangential ocular dominance organization of V1 for human (A), macaque monkey (B), cat (C), rat (D), and mouse (E). Note the progressive decrease in overall surface area of V1 moving from human to mouse, and also the difference in organization observed across species. Scale bar is 10 mm. Images and organizational details of V1 originate from Adams et al. (2007) (human), Horton and Hocking (1996) (macaque monkey), Anderson et al. (1988) (cat), Duffy et al. (1998), Laing et al. (2015) (rat), and Airey et al. (2006) (mouse).
A suggested approach to mitigate failures in extrapolating from animals to humans is a “two-species rule”: a result in one species should be confirmed in at least one other, ideally a non-human primate (Mitchell and Sengpiel, 2018; Levi, 2020). As a model of human amblyopia, non-human primates offer significant advantages. Monkeys are similar to humans in possessing a fovea, have excellent spatial vision, stereopsis, analogous visual cortical pathways, and a response to amblyogenic rearing that closely mimics the human condition (see reviews from Kiorpes, 2019; Tychsen, 2020). Parlaying the strengths of rodent and cat studies with the translation power offered by non-human primates is a potential strategy for advancing knowledge gained from animal models.
Insights on neuropathology
We are more than half a century removed from the seminal discoveries of Hubel and Wiesel (Wiesel and Hubel, 1963a,b) and much progress has been made. Their work spawned a vast collection of studies delineating innumerable effects of early visual deprivation. Yet still, the underlying neural pathology that gives rise to the functional consequences of MD is not fully understood. In cats, monkeys and mice, early MD precipitates a weakening and loss of excitatory synaptic connections serving the deprived eye (Thorpe and Blakemore, 1975; Hubel et al., 1977; Shatz and Stryker, 1978; Antonini and Stryker, 1993; Antonini et al., 1999; Coleman et al., 2010). This is a presumed consequence of binocular competition, in part because comparable changes are not observed in the region of V1 receiving input only from the contralateral eye (the monocular segment). The MD-induced redistribution of excitatory terminals produces a shift in cortical ocular dominance that leaves the deprived eye with fewer and weaker synaptic connections (Tieman, 1984). This alteration in connectivity results in a loss of cortical responsiveness to stimulation of the deprived eye (Wiesel and Hubel, 1963a; LeVay et al., 1980; Frenkel and Bear, 2004; Figure 3). Therefore, reduction in the number and strength of excitatory connections is considered the basis of MD-induced amblyopia (Dews and Wiesel, 1970; Giffin and Mitchell, 1978). A similar but less severe shift in cortical ocular dominance is observed in monkeys made anisometropic during the critical period (Kozma and Kiorpes, 2003; Rittenhouse et al., 2006). That these two types of amblyogenic rearing, form deprivation and anisometropia, result in a marked shift in ocular dominance away from the affected eye (Figure 3) suggests a proportionate loss or weakening of excitatory synapses as the primary cause of visual impairment (Khibnik et al., 2010). The loss of input decreases neuronal spatial sampling density, the so-called spatial undersampling hypothesis (Levi and Klein, 1986; Wang et al., 1998).
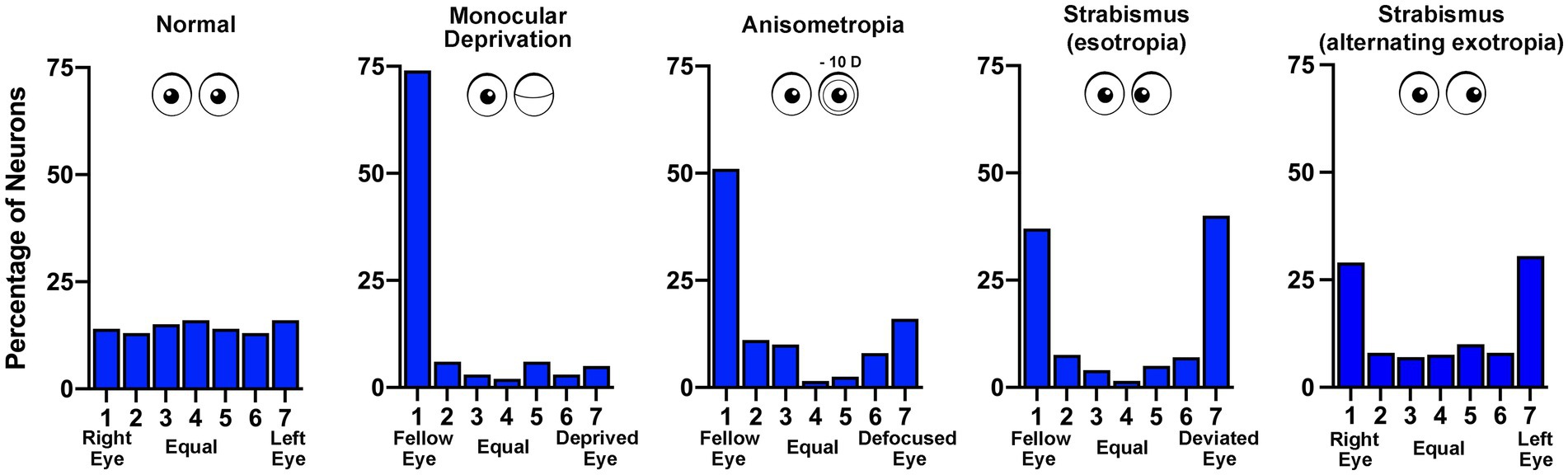
Figure 3. Ocular dominance histograms compare the effects of different rearing conditions that produce amblyopia. The ocular dominance of neurons sampled from the visual cortex of monkeys was measured by assessing the responsivity to stimulation of either the left or right eye. In normal animals there is a similar number of neurons connected to the right (group 1) and left (group 7) eye, with neurons connected to both eyes either equally (group 4) or somewhere in between (groups 2, 3, 5, 6; data from Kiorpes et al., 1998). Following monocular deprivation there is a strong shift in ocular dominance so that most neurons respond only to the non-deprived (fellow) eye (data from Hubel et al., 1977). Anisometropia produced by rearing with a contact lens placed in one eye also causes a shift in ocular dominance away from the affected eye, and like other amblyopia subtypes, reduces cortical binocularity (data from Kiorpes et al., 1998). Strabismus (esotropia or exotropia) does not result in disconnection of the affected eye but rather reduces the number of neurons responsive to both eyes – binocular cells (esotropia data from Kiorpes et al., 1998; exotropia data from Economides et al., 2021).
In contrast to the effect of MD, strabismic monkeys (esotropic or exotropic) do not exhibit a loss of synaptic connections serving the affected eye in V1. Both eyes are about equally connected to the visual cortex, although there is a precipitous decrease in the percentage of neurons receiving input from both eyes (Kiper and Kiorpes, 1994; Economides et al., 2021). This loss of binocularity in V1 is a feature shared across all amblyopia subtypes (Figure 3) and can be explained by a uniform set of assumptions for how excitatory synapses modify after MD and strabismus (see, e.g., Clothiaux et al., 1991). However, observations in V1 leave unexplained how strabismus degrades visual perception in only one eye to cause unilateral amblyopia. The prevailing view is that inputs serving the deviated eye are submerged by intracortical inhibition, an adaption to avoid double vision (Sireteanu, 1982; reviewed in Sengpiel and Blakemore, 1996). Chronic interocular suppression may play an active role in the development and progression of amblyopia in all subtypes. Binocular suppression of (and by) the impaired eye is not specific to strabismic amblyopia. There is evidence for persistence of interocular suppression within the visual cortex of monkeys made amblyopic by experimentally induced anisometropia (Hallum et al., 2017), as well as in humans with deprivation amblyopia (Hamm et al., 2017). Although the origin of neural suppression is not currently known, evidence from strabismic and anisometropic monkeys indicates that the capacity for suppression from both the amblyopic and fellow eyes is intact (Hallum et al., 2017; Economides et al., 2021). In other words, while the obstruction of normal binocular vision stimulates a redistribution of thalamocortical and binocular horizontal excitatory connections (Antonini and Stryker, 1993; Tychsen and Burkhalter, 1995; Trachtenberg and Stryker, 2001), inhibitory connections appear to be preserved and are not appreciably different from normal (Smith et al., 1997; Hallum et al., 2017; Economides et al., 2021). It will be important to determine if this result is observed after MD.
Therapeutic innovations
The many factors that frustrate traditional treatments for amblyopia underscore the need to advance the standard of care. The emergence of binocular experience-based therapies is an attempt to address the shortcomings of traditional treatment. Dichoptic display therapy, for instance, aims to reduce suppression by the fellow eye and promote binocular vision by displaying a stronger stimulus to the weaker eye. Movies or video game stimuli have been used to promote patient engagement and treatment compliance. These and similar therapies have produced a promising degree of visual recovery in children and adults with anisometropic and/or strabismic amblyopia (Hess et al., 2010; Li et al., 2010; Vedamurthy et al., 2015; Holmes et al., 2016; Žiak et al., 2017; Birch et al., 2019b; Xiao et al., 2022). However, an efficacy review by The American Academy of Ophthalmology concluded that there was no evidence to support the use of binocular treatment as a substitute for standard patching or penalization for the common forms of amblyopia (Pineles et al., 2020). Further, binocular treatment has had mixed results when administered to children with deprivation amblyopia: children with MD from unilateral cataract showed modest or no improvement in visual acuity or contrast sensitivity following contrast-balanced binocular treatment (Hamm et al., 2018; Birch et al., 2020). Children with dense congenital cataracts consistently showed no improvement even when cataracts were removed at 4–6 weeks after birth (Birch et al., 2020). Additional emerging treatments for amblyopia include short-term patching of the amblyopic eye (inverse occlusion) that can promote durable recovery (Lunghi et al., 2018; Zhou et al., 2019), and also behavioral training therapy that can improve visual acuity in children and adults (Vedamurthy et al., 2015; Pineles et al., 2020; Kadhum et al., 2023). To our knowledge, the efficacy of these therapies has yet to be tested in patients with deprivation amblyopia.
Over the past decade, a bevy of novel approaches have been used to correct the effects of MD in animals. Several of these have elicited recovery in rodents by selective targeting of specific brain molecules or processes that regulate neural plasticity (Maya Vetencourt et al., 2008; Morishita et al., 2010; Silingardi et al., 2010; Grieco et al., 2020; Venturino et al., 2021). Other manipulations have produced recovery or have elevated plasticity potential through experiential manipulations such as environmental enrichment or exposure to 60 Hz light flicker, which appear to work by modulating GABAergic inhibition (Sale et al., 2007; Greifzu et al., 2014; Venturino et al., 2021). Complete elimination of visually-driven activity through brief dark exposure can also enhance plasticity and promote recovery from MD following reintroduction of the animals to a lighted environment (He et al., 2007). The motivation for using dark exposure derives from studies, both theoretical and experimental, that have shown the threshold for Hebbian synaptic strengthening is changed by periods of reduced activity in the visual system (Bienenstock et al., 1982; Kirkwood et al., 1996; Cooper and Bear, 2012). Once removed from darkness, visually-driven impulses promote strengthening of weak synapses serving the amblyopic eye (reviewed in Leet et al., 2022). The mechanism for this effect appears to include the modification of NMDA receptor structure and function (Quinlan et al., 1999; Philpot et al., 2001), as well as reconfiguration of the extracellular matrix surrounding thalamocortical synapses and inhibitory neurons (Murase et al., 2017). The beneficial effect of dark exposure on plasticity and recovery has now been demonstrated in three species across multiple labs: mice (Erchova et al., 2017); rats (He et al., 2007; Montey and Quinlan, 2011); and cats (Duffy and Mitchell, 2013; Gotou et al., 2021). However, dark treatment for human amblyopia is impeded by the logistical demands required to implement its clinical application. Dark therapy has also failed to promote recovery at older ages in cats (Duffy et al., 2018; Holman et al., 2018).
An alternative approach to reduce cortical activity is silencing retinal ganglion cells by intraocular injection of tetrodotoxin (TTX). A single intravitreal microinjection of TTX, a potent voltage-gated sodium channel blocker, can eliminate retinal output activity for approximately 48 h (Stryker and Harris, 1986; Linden et al., 2009; Fong et al., 2016). In comparison to occlusion therapy that eliminates only visually-driven activity, inactivation of the retina eliminates both visually-driven and spontaneous activity. In the case of MD, only dominant eye inactivation is required to markedly attenuate visual cortex activity because the amblyopic eye is incapable of driving normal cortical activity due to its weak connections. Freed from suppression by the dominant eye during the period of inactivation, the deprived eye’s excitatory synapses can recover via long-term synaptic potentiation (Clothiaux et al., 1991; Fong et al., 2021; Leet et al., 2022). Empirical evidence supporting this theoretical framework comes from cat studies (Kratz et al., 1976; Smith, 1981) as well as human case reports (Klaeger-Manzanell et al., 1994; El Mallah et al., 2000; Vagge et al., 2020; Resnick et al., 2023) that demonstrate post-critical period recovery from MD after loss or damage to the fellow eye. Recent studies have leveraged this knowledge to investigate retinal inactivation as a treatment for amblyopia caused by MD. Inactivation of the dominant eye in MD mouse or cat produces recovery of visually-evoked potentials (VEPs) when applied after the classical critical period (Fong et al., 2021; Hogan et al., 2023). Anatomical recovery also occurs. Neurons post-synaptic to the MD eye grow to normal size (Duffy et al., 2018). To be considered as a treatment for human MD, it will be of paramount importance to demonstrate full recovery of the inactivated eye in a primate model. Assessments to date in cats and monkeys have revealed no ocular pathology after inactivation for up to 10 days (Foeller and Tychsen, 2019; DiCostanzo et al., 2020; Duffy et al., 2023; Hogan et al., 2023). Figure 4 demonstrates restoration of VEPs following brief monocular inactivation in a cat (A) and macaque monkey (B). In both species, VEPs measured after ~10 days of inactivation were restored to pre-inactivation levels about 1 week after the final TTX injection. Assessment of the inactivated monkey eye using optical coherence tomography (OCT) revealed no retinal nerve fiber or ganglion cell layer abnormalities after inactivation (Figures 4C,D). Future studies are aimed at determining if retinal inactivation can enable recovery from deprivation amblyopia in monkeys, as well as assess the influence of age. Success in the primate model could pave the way to human studies.
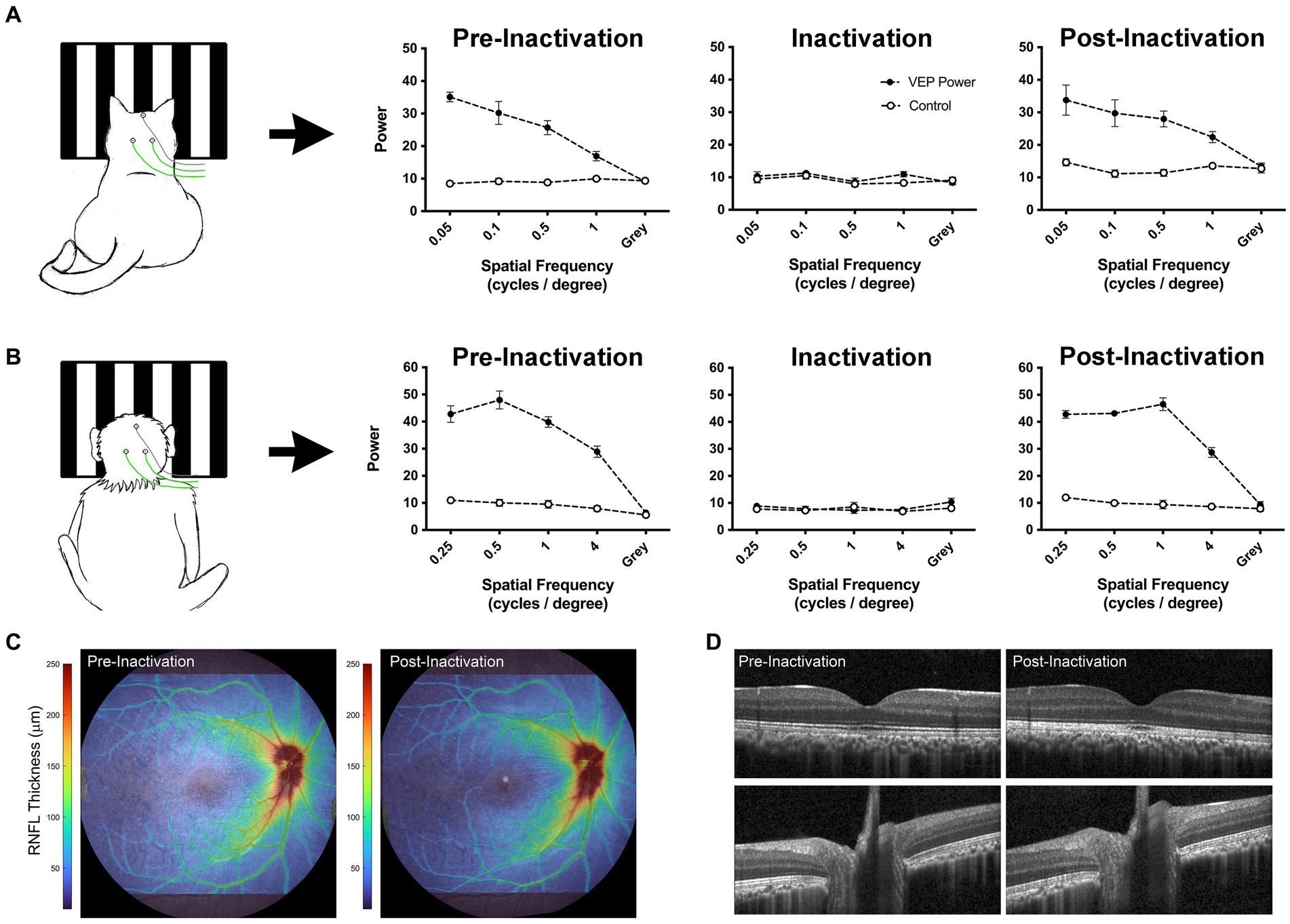
Figure 4. Data from cat and monkey revealing that the effect of intravitreal injection of TTX is reversible. VEPs (solid circles) measured from V1 in a cat (A; Duffy et al., 2023) and monkey (B; preliminary data) using scalp electrodes show a reduction to non-visual baseline levels (open circles) after TTX injection. Measurement of VEPs post-inactivation reveal a full recovery back to pre-inactivation levels for both species, indicating that the effect of inactivation on VEPs is temporary. OCT scans acquired from the monkey displayed in panel (B) demonstrate comparable retinal nerve fiber layer (RNFL) thickness between pre- and post-inactivation measurements (C). Similarly, individual registered b-scans suggest no change in retinal or optic nerve anatomy following injection (D). Monkey VEPs were collected for an experiment in which 4 TTX injections were delivered into the right eye over 4 weeks (one injection per week). Pre-inactivation VEPs were measured from right V1 at 8 months of age. Inactivation VEPs were measured 24 h after the first injection. Post-inactivation VEPs were taken 1 week after the final of four injections. OCT scans were acquired using the Spectralis OCT system (Heidelberg Engineering, Heidelberg, Germany), after pupils were dilated with 1% tropicamide. Scans acquired included a high resolution 55×45 degree raster scan, and 20×20 degree high speed raster scans centered on the optic nerve head and macula. Images were processed using neural network-based segmentation algorithms previously described (Srinivasan et al., 2022).
Conclusion
Amblyopia caused by MD is a rare and debilitating visual impairment that responds poorly to conventional therapy. Novel treatments being developed in human patients largely exclude those with MD because it is so resistant to therapy. Animal models offer a unique opportunity to address this unmet need. Investigation and development of unconventional amblyopia therapies cannot easily be pioneered in humans. With regard to inactivation therapy, the next logical step is to investigate its efficacy in primates. If successful, this innovation in MD treatment could be extended to target all types of amblyopia.
Data availability statement
The original contributions presented in the study are included in the article/supplementary materials, further inquiries can be directed to the corresponding author.
Ethics statement
The animal study was approved by University Committee on Laboratory Animals at Dalhousie University, and the Institutional Animal Care and Use Committee at the University of Houston. The study was conducted in accordance with the local legislation and institutional requirements.
Author contributions
All authors listed have made a substantial, direct, and intellectual contribution to the work and approved it for publication.
Funding
This research was funded by grants from the Canadian Institutes of Health Research (468904) to KD; the National Eye Institute (R01-EY029245) and Research to Prevent Blindness (42894) to MB; and the National Eye Institute (R01-EY026568) to VD.
Conflict of interest
The authors declare that the research was conducted in the absence of any commercial or financial relationships that could be construed as a potential conflict of interest.
Publisher’s note
All claims expressed in this article are solely those of the authors and do not necessarily represent those of their affiliated organizations, or those of the publisher, the editors and the reviewers. Any product that may be evaluated in this article, or claim that may be made by its manufacturer, is not guaranteed or endorsed by the publisher.
References
Adams, D. L., Sincich, L. C., and Horton, J. C. (2007). Complete pattern of ocular dominance columns in human primary visual cortex. J. Neurosci. 27, 10391–10403. doi: 10.1523/JNEUROSCI.2923-07.2007
Airey, D. C., Wu, F., Guan, M., and Collins, C. E. (2006). Geometric morphometrics defines shape differences in the cortical area map of C57BL/6J and DBA/2J inbred mice. BMC Neurosci. 7:63. doi: 10.1186/1471-2202-7-63
Anderson, P. A., Olavarria, J., and Van Sluyters, R. C. (1988). The overall pattern of ocular dominance bands in cat visual cortex. J. Neurosci. 8, 2183–2200. doi: 10.1523/JNEUROSCI.08-06-02183.1988
Antonini, A., Fagiolini, M., and Stryker, M. P. (1999). Anatomical correlates of functional plasticity in mouse visual cortex. J. Neurosci. 19, 4388–4406. doi: 10.1523/JNEUROSCI.19-11-04388.1999
Antonini, A., and Stryker, M. P. (1993). Rapid remodeling of axonal arbors in the visual cortex. Science 260, 1819–1821. doi: 10.1126/science.8511592
Attebo, K., Mitchell, P., Cumming, R., Smith, W., Jolly, N., and Sparkes, R. (1998). Prevalence and causes of amblyopia in an adult population. Ophthalmology 105, 154–159. doi: 10.1016/s0161-6420(98)91862-0
Awan, M., Proudlock, F. A., and Gottlob, I. (2005). A randomized controlled trail of unilateral strabismic and mixed amblyopia using occlusion does monitors to record compliance. Invest. Ophthalmol. Vis. Sci. 46, 1435–1439. doi: 10.1167/iovs.04-0971
Awaya, S., Sugawara, M., and Miyake, S. (1979). Observations in patients with occlusion amblyopia: results of treatment. Trans. Ophthalmol. Soc. U. K. 99, 447–454.
Baker, M. (2013). Neuroscience: through the eyes of a mouse. Nature 502, 156–158. doi: 10.1038/502156a
Baroncelli, L., Braschi, C., and Maffei, L. (2013). Visual depth perception in normal and deprived rats: effects of environmental enrichment. Neuroscience 236, 313–319. doi: 10.1016/j.neuroscience.2013.01.036
Bavelier, D., Levi, D. M., Li, R. W., Dan, Y., and Hensch, T. K. (2010). Removing brakes on adult plasticity: from molecules to behavioral intereventions. J. Neurosci. 30, 14964–14971. doi: 10.1523/JNEUROSCI.4812-10.2010
Beller, R., Hoyt, C., Marg, E., and Odom, V. (1981). Good visual function after neonatal surgery for congenital unilateral cataracts. Am J. Ophthalmol. 91, 559–565. doi: 10.1016/0002-9394(81)90053-2
Berman, N. E., and Payne, B. R. (1983). Alterations in connections of the corpus collasum following convergent and divergent strabismus. Brain Res. 274, 201–212. doi: 10.1016/0006-8993(83)90697-2
Bienenstock, E. L., Cooper, L. N., and Munro, P. W. (1982). Theroy for the development of neuron selectivity: orientation specificity and binocular interaction in visual cortex. J. Neurosci. 2, 32–48. doi: 10.1523/JNEUROSCI.02-01-00032.1982
Birch, E. E., Castañeda, Y. S., Cheng-Patel, C. S., Morale, S. E., Kelly, K. R., Beauchamp, C. L., et al. (2019a). Self-perception of school-aged children with amblyopia and its association with reading speed and motor skills. JAMA Ophthalmol. 137, 167–174. doi: 10.1001/jamaophthalmol.2018.5527
Birch, E. E., Jost, R. M., De La Cruz, A., Kelly, K. R., Beauchamp, C. L., Dao, L., et al. (2019b). Binocular amblyopia treatment with contrast-rebalanced movies. J AAPOS 23, 160.e1–160.e5. doi: 10.1016/j.jaapos.2019.02.007
Birch, E. E., Jost, R. M., Wang, S. X., and Kelly, K. R. (2020). A pilot randomized trial of contrast-rebalanced binocular treatment for deprivation amblyopia. J. AAPOS 24, 344.e1–344.e5. doi: 10.1016/j.jaapos.2020.07.009
Birch, E. E., Kelly, K. R., and Giaschi, D. (2019c). Fellow eye deficits in amblyopia. J. Binocul. Vis. Ocul. Motil. 69, 116–125. doi: 10.1080/2576117X.2019.1624440
Birch, E. E., and Stager, D. R. (1988). Prevalence of good visual acuity following surgery for congenital unilateral cataract. Arch. Ophthalmol. 106, 40–43. doi: 10.1001/archopht.1988.01060130046025
Birch, E. E., and Stager, D. R. (1996). The critical period for surgical treatment of dense congenital unilateral cataract. Invest. Ophthalmol. Vis. Sci. 37, 1532–1538.
Birch, E. E., Stager, D. R., and Wright, W. W. (1986). Grating acuity development after early surgery for congenital unilateral cataract. Arch. Ophthalmol. 104, 1783–1787. doi: 10.1001/archopht.1986.01050240057040
Birch, E. E., Swanson, W. H., Stager, D. R., Woody, M., and Everett, M. (1993). Outcome after early treatment of dense congenital unilateral cataract. Invest. Ophthalmol. Vis. Sci. 34, 3687–3699.
Boone, H. C., Samonds, J. M., Crouse, E. C., Barr, C., Priebe, N. J., and McGee, A. W. (2021). Natural binocular depth discrimination behavior in mice explained by visual cortical activity. Curr. Biol. 31, 2191–2198.e3. doi: 10.1016/j.cub.2021.02.031
Brown, A. M., and Yamamoto, M. (1986). Visual acuity in newborn and preterm infants measured with grating acuity cards. Am J. Ophthalmol. 102, 245–253. doi: 10.1016/0002-9394(86)90153-4
Bui Quoc, E. B., Kulp, M. T., Burns, J. G., and Thompson, B. (2023). Amblyopia: a review of unmet needs, current treatment options, and emerging therapies. Surv. Ophthalmol. 68, 507–525. doi: 10.1016/j.survophthal.2023.01.001
Chen, A. M., and Cotter, S. A. (2016). The amblyopia treatment studies: implications for clinical practice. Adv. Ophthalmol. Optom. 1, 287–305. doi: 10.1016/j.yaoo.2016.03.007
Chung, S. T. L., Li, R. W., Silver, M. A., and Levi, D. M. (2017). Donepezil does not enhance perceptual learning in adults with amblyopia: a pilot study. Front. Neurosci. 11:448. doi: 10.3389/fnins.2017.00448
Clothiaux, E. E., Bear, M. F., and Cooper, L. N. (1991). Synaptic plasticity in visual cortex: comparison of theory with experiment. J. Neurophysiol. 66, 1785–1804. doi: 10.1152/jn.1991.66.5.1785
Cohen, L., and Younger, B. (1984). Infant perception of angular relations. Infant Behav. Dev. 7, 37–47. doi: 10.1016/S0163-6383(84)80021-1
Coleman, J. E., Law, K., and Bear, M. F. (2009). Anatomical origins of ocular dominance in mouse primary visual cortex. Neuroscience 161, 561–571. doi: 10.1016/j.neuroscience.2009.03.045
Coleman, J. E., Nahmani, M., Gavornik, J. P., Haslinger, R., Heynen, A. J., Erisir, A., et al. (2010). Rapid structural remodeling of thalamocortical synapses parallels experience-dependent functional plasticity in mouse primary visual cortex. J. Neurosci. 30, 9670–9682. doi: 10.1523/JNEUROSCI.1248-10.2010
Cooper, L. N., and Bear, M. F. (2012). The BCM theory of synapse modification at 30: interaction of theory with experiment. Nat. Rev. Neurosci. 13, 798–810. doi: 10.1038/nrn3353
Costenbader, F. D., and Albert, D. G. (1957). Conservatism in the management of congential cataract. AMA Arch. Ophthalmol. 58, 426–430. doi: 10.1001/archopht.1957.00940010438018
Dews, P. B., and Wiesel, T. N. (1970). Consequences of monocular deprivation on visual behaviour in kittens. J. Physiol. 206, 437–455. doi: 10.1113/jphysiol.1970.sp009023
DiCostanzo, N. R., Crowder, N. A., Kamermans, B. A., and Duffy, K. R. (2020). Retinal and optic nerve integrity following monocular inactivation for the treatment of amblyopia. Front. Syst. Neurosci. 14:32. doi: 10.3389/fnsys.2020.00032
Drews-Botsch, C. D., Celano, M., Kruger, S., and Hartmann, E. E., Infant Aphakia Treatment Study Group (2012). Adherence to occlusion therapy in the first six months of follow-up and visual acuity among participants in the infant Aphakia treatment study (IATS). Invest. Ophthalmol. Vis. Sci. 53, 3368–3375. doi: 10.1167/iovs.11-8457
Duffy, K. R., Crowder, N. A., Heynen, A. J., and Bear, M. F. (2023). Comparative analysis of structural modifications induced by monocular retinal inactivation and monocular deprivation in the developing cat lateral geniculate nucleus. J. Comp. Neurol. 531, 1244–1260. doi: 10.1002/cne.25493
Duffy, K. R., Fong, M. F., Mitchell, D. E., and Bear, M. F. (2018). Recovery from the anatomical effects of long-term monocular deprivation in cat lateral geniculate nucleus. J. Comp. Neurol. 526, 310–323. doi: 10.1002/cne.24336
Duffy, K. R., and Mitchell, D. E. (2013). Darkness alters maturation of visual cortex and promotes fast recovery from monocular deprivation. Curr. Biol. 23, 382–386. doi: 10.1016/j.cub.2013.01.017
Duffy, K. R., Murphy, K. M., and Jones, D. G. (1998). Analysis of the postnatal growth of visual cortex. Vis. Neurosci. 15, 831–839. doi: 10.1017/S0952523898155049
Economides, J. R., Adams, D. L., and Horton, J. C. (2021). Interocular suppression in primary visual cortex in strabismus. J. Neurosci. 41, 5522–5533. doi: 10.1523/JNEUROSCI.0044-21.2021
El Mallah, M. K., Chakravarthy, U., and Hart, P. M. (2000). Amblyopia: is visual loss permanent? Br. J. Ophthalmol. 84, 952–956. doi: 10.1136/bjo.84.9.952
Ellemberg, D., Lewis, T. L., Maurer, D., and Brent, H. P. (2000). Influence of monocular deprivation during infancy on the later development of spatial and temporal vision. Vis. Res. 40, 3283–3295. doi: 10.1016/S0042-6989(00)00165-6
Erchova, I., Vasalauskaite, A., Longo, V., and Sengpiel, F. (2017). Enhancement of visual cortex plasticity by dark exposure. Philos. Trans. R. Soc. Lond. Ser. B Biol. Sci. 372:20160159. doi: 10.1098/rstb.2016.0159
Espinosa, J. S., and Stryker, M. P. (2012). Development and plasticity of the primary visual cortex. Neuron 75, 230–249. doi: 10.1016/j.neuron.2012.06.009
Flynn, J. T., and Cassady, J. C. (1978). Current trends in amblyopia therapy. Ophthalmology 85, 428–450. doi: 10.1016/s0161-6420(78)35651-7
Foeller, P., and Tychsen, L. (2019). Monocular retinal blockade pharmacological occlusion therapy in macaque monkey: spatial-sweep visually-evoked potential visual acuity, relative afferent pupillary defect, and optokinetic tracking. Invest. Ophthalmol. Vis. Sci. 60:225.
Fong, M. F., Duffy, K. R., Leet, M. P., Candler, C. T., and Bear, M. F. (2021). Correction of amblyopia in cats and mice after the critical period. elife 10:e70023. doi: 10.7554/eLife.70023
Fong, M. F., Mitchell, D. E., Duffy, K. R., and Bear, M. F. (2016). Rapid recovery from the effects of early monocular deprivation is enabled by temporary inactivation of the retinas. Proc. Natl. Acad. Sci. U. S. A. 113, 14139–14144. doi: 10.1073/pnas.1613279113
Frenkel, M. Y., and Bear, M. F. (2004). How monocular deprivation shifts ocular dominance in visual cortex of young mice. Neuron 44, 917–923. doi: 10.1016/j.neuron.2004.12.003
Friedman, D. S., Repka, M. X., Katz, J., Giordano, L., Ibironke, J., Hawse, P., et al. (2009). Prevalence of amblyopia and strabismus in white and African American children aged 6 through 71 months. Ophthalmology 116, 2128–2134.e2. doi: 10.1016/j.ophtha.2009.04.034
Giffin, F., and Mitchell, D. E. (1978). The rate of recovery of vision after early monocular deprivation in kittens. J. Physiol. 274, 511–537. doi: 10.1113/jphysiol.1978.sp012164
Gotou, T., Kameyama, K., Kobayashi, A., Okamura, K., Ando, T., Terata, K., et al. (2021). Dark rearing promotes the recovery of visual cortical responses but not the morphology of geniculocortical axons in amblyopia cat. Front. Neural Circuits 15:637638. doi: 10.3389/fncir.2021.637638
Grant, S., and Moseley, M. J. (2011). Amblyopia and real-world visuomotor task. Strabismus 19, 119–128. doi: 10.3109/09273972.2011.600423
Greifzu, F., Pielecka-Fortuna, J., Kalogeraki, E., Krempler, K., Favaro, P. D., Schluter, O. M., et al. (2014). Environmental enrichment extends ocular dominance plasticity into adulthood and protects from stroke-induced impairments of plasticity. Proc. Natl. Acad. Sci. U. S. A. 111, 1150–1155. doi: 10.1073/pnas.1313385111
Grieco, S. F., Qiao, X., Zheng, X., Liu, Y., Chen, L., Zhang, H., et al. (2020). Subanesthetic ketamine reactivates adult cortical plasticity to restore vision from amblyopia. Curr. Biol. 30, 3591–3603.e8. doi: 10.1016/j.cub.2020.07.008
Hallum, L. E., Shooner, C., Kumbhani, R. D., Kelly, J. G., García-Marín, M. N. J., Movshon, J. A., et al. (2017). Altered balance of receptive field excitation and suppression in visual cortex of amblyopic macaque monkeys. J. Neurosci. 37, 8216–8226. doi: 10.1523/JNEUROSCI.0449-17.2017
Hamm, L. M., Chen, Z., Li, J., Black, J., Dai, S., Yuan, J., et al. (2017). Interocular suppression in children with deprivation amblyopia. Vis. Res. 133, 112–120. doi: 10.1016/j.visres.2017.01.004
Hamm, L. M., Chen, Z., Li, J., Dai Franzco, S., Black, J., Yuan, J., et al. (2018). Contrast-balanced binocular treatment in children with deprivation amblyopia. Clin. Exp. Optom. 101, 541–552. doi: 10.1111/cxo.12630
Harwerth, R. S., Crawford, M. L., Smith, E. L. 3rd, and Boltz, R. L. (1981). Behavioral studies of stimulus deprivation amblyopia in monkeys. Vis. Res. 21, 779–789. doi: 10.1016/0042-6989(81)90175-9
He, H. Y., Ray, B., Dennis, K., and Quinlan, E. M. (2007). Experience-dependent recovery of vision following chronic deprivation amblyopia. Nat. Neurosci. 10, 1134–1136. doi: 10.1038/nn1965
Helveston, E., Saunders, R., and Ellis, F. (1980). Unilateral cataracts in children. Ophthalmic Surg. 11, 102–108.
Hensch, T. K. (2005). Critical period plasticity in local cortical circuits. Nat. Rev. Neurosci. 6, 877–888. doi: 10.1038/nrn1787
Hess, R. F., Mansouri, B., and Thompson, B. (2010). A new binocular approach to the treatment of amblyopia in adults well beyond the critical period of visual development. Restor. Neurol. Neurosci. 28, 793–802. doi: 10.3233/RNN-2010-0550
Heynen, A. J., Yoon, B.-J., Liu, C.-H., Chung, H. J., Huganir, R. L., and Bear, M. F. (2003). Molecular mechanism for loss of visual cortical responsiveness following brief monocular deprivation. Nat. Neurosci. 6, 854–862. doi: 10.1038/nn1100
Hogan, M., DiCostanzo, N. R., Crowder, N. A., Fong, M., and Duffy, K. R. (2023). Investigation of the efficacy and safety of retinal inactivation as a treatment for amblyopia in cats. Front. Neurosci. 17:1167007. doi: 10.3389/fnins.2023.1167007
Holman, K. D., Duffy, K. R., and Mitchell, D. E. (2018). Short periods of darkness fail to restore visual or neural plasticity in adult cats. Vis. Neurosci. 35:E002. doi: 10.1017/S0952523817000335
Holmes, J. M., Lazar, E. L., Melia, B. M., Astle, W. F., Dagi, L. R., Donahue, S. P., et al. (2011). Effect of age on response to amblyopia treatment in children. Arch. Ophthalmol. 129, 1451–1457. doi: 10.1001/archophthalmol.2011.179
Holmes, J. M., and Levi, D. M. (2018). Treatment of amblyopia as a function of age. Vis. Neurosci. 35:E015. doi: 10.1017/S0952523817000220
Holmes, J. M., Manh, V. M., Lazar, E. L., Beck, R. W., Birch, E. E., Kraker, R. T., et al. (2016). Effect of a binocular iPad game vs part-time patching in children aged 5 to 12 years with amblyopia: a randomized clinical trial. JAMA Ophthalmol. 134, 1391–1400. doi: 10.1001/jamaophthalmol.2016.4262
Horton, J. H., and Hocking, D. R. (1996). An adult-like pattern of ocular dominance columns in striate cortex of newborn monkeys prior to visual experience. J. Neurosci. 16, 1791–1807. doi: 10.1523/JNEUROSCI.16-05-01791.1996
Horton, J. H., Hocking, D. R., and Kiorpes, L. (1997). Pattern of ocular dominance columns and cytochrome oxidase activity in a macaque monkey with naturally occurring anisometropic amblyopia. Vis. Neurosci. 14, 681–689. doi: 10.1017/S0952523800012645
Hubel, D. H., Wiesel, T. N., and LeVay, S. (1970). The period of susceptibility to the physiological effects of unilateral eye closure in kittens. J. Physiol. 206, 419–436. doi: 10.1113/jphysiol.1970.sp009022
Hubel, D. H., Wiesel, T. N., and LeVay, S. (1977). Plasticity of ocular dominance columns in monkey striate cortex. Philos. Trans. R. Soc. Lond. B Biol. Sci. 278, 377–409.
Huh, C. Y. L., Abdelaal, K., Salinas, K. J., Gu, D., Zeitoun, J., Velez, D. X. F., et al. (2020). Long-term monocular deprivation during juvenile critical period period disrupts binocular integration in mouse visual thalamus. J. Neurosci. 40, 585–604. doi: 10.1523/JNEUROSCI.1626-19.2019
Jaepel, J., Hübener, M., Bonhoeffer, T., and Rose, T. (2017). Lateral geniculate neurons projecting to primary visual cortex show ocular dominance plasticity in adult mice. Nat. Neurosci. 20, 1708–1714. doi: 10.1038/s41593-017-0021-0
Jia, Y., Liu, J., Ye, Q., Zhang, S., Feng, L., Xu, Z., et al. (2022). Factors predicting regression of visual acuity following successful treatment of anisometropic amblyopia. Front. Med. 9:10131136. doi: 10.3389/fmed.2022.1013136
Kadhum, A., Tan, E. T. C., Fronius, M., Baart, S. J., Levi, D. M., Joosse, M. V., et al. (2023). Supervised dichoptic gaming versus monitored occlusion therapy for childhood amblyopia: effectiveness and efficiency. Acta Ophthalmol., Online ahead of print. doi: 10.1111/aos.15674
Kaneko, M., and Stryker, M. P. (2023). Production of brain-derived neurotrophic factor gates plasticity in developing visual cortex. Proc. Natl. Acad. Sci. U. S. A. 120:e2214833120. doi: 10.1073/pnas.2214833120
Kelly, K. R., Morale, S. E., Wang, S. X., Stager, D. R. Jr., and Birch, E. E. (2019). Impaired fine motor skills in children following extraction of a dense congenital or infantile cataract. J. AAPOS 23, 330.e1–330.e6. doi: 10.1016/j.jaapos.2019.08.278
Khibnik, L. A., Cho, K. K. A., and Bear, M. F. (2010). Relative contribution of feedforward excitatory connections to expression of ocular dominance plasticity in layer 4 of visual cortex. Neuron 66, 493–500. doi: 10.1016/j.neuron.2010.04.012
Kiorpes, L. (1992). Development of vernier acuity and grating acuity in normally reared monkeys. Vis. Neurosci. 9, 243–251. doi: 10.1017/S0952523800010658
Kiorpes, L. (2019). Understanding the development of amblyopia using macaque monkey models. Proc. Natl. Acad. Sci. U. S. A. 116, 26217–26223. doi: 10.1073/pnas.1902285116
Kiorpes, L., Kiper, D. C., O’Keefe, L. P., Cavanaugh, J. R., and Movshon, J. A. (1998). Neuronal correlates of amblyopia in the visual cortex of macaque monkeys with experimental strabismus and anisometropia. J. Neurosci. 18, 6411–6424. doi: 10.1523/JNEUROSCI.18-16-06411.1998
Kiper, D. C., and Kiorpes, L. (1994). Suprathreshold contrast sensitivity in experimentally strabismic monkeys. Vis. Res. 34, 1575–1583. doi: 10.1016/0042-6989(94)90114-7
Kirkwood, A., Rioult, M. C., and Bear, M. F. (1996). Experience-dependent modification of synaptic plasticity in visual cortex. Nature 381, 526–528. doi: 10.1038/381526a0
Klaeger-Manzanell, C., Hoyt, C. S., and Good, W. V. (1994). Two step recovery of vision in the amblyopic eye after visual loss and enucleation of the fixing eye. Br. J. Ophthalmol. 78, 506–507. doi: 10.1136/bjo.78.6.506
Koo, E. B., Gilbert, A. L., and VanderVeen, D. K. (2017). Treatment of amblyopia and amblyopia risk factors based on current evidence. Semin. Ophthalmol. 32, 1–7. doi: 10.1080/08820538.2016.1228408
Kozma, P., and Kiorpes, L. (2003). Contour integration in amblyopic monkeys. Vis. Neurosci. 20, 577–588. doi: 10.1017/S0952523803205113
Kratz, K. E., Spear, P. D., and Smith, D. C. (1976). Postcritical-period reversal of effects of monocular deprivation on striate cortex cells in the cat. J. Neurophysiol. 39, 501–511. doi: 10.1152/jn.1976.39.3.501
Kuljis, R. O., and Rakic, P. (1990). Hypercolumns in primate visual cortex can develop in the absence of cues from photoreceptors. Proc. Natl. Acad. Sci. U. S. A. 87, 5303–5306. doi: 10.1073/pnas.87.14.5303
Lagas, A. K., Black, J. M., Russell, B. R., Kydd, R. R., and Thompson, B. (2019). The effect of combined patching and citalopram on visual acuity in adults with amblyopia: a randomized, crossover, placebo-controlled trial. Neural Plast. 2019, 1–10. doi: 10.1155/2019/5857243
Laing, R. J., Turecek, J., Takahata, T., and Olavarria, J. F. (2015). Identification of eye-specific domains and their relation to callosal connections in primary visual cortex of long evans rats. Cereb. Cortex 25, 3314–3329. doi: 10.1093/cercor/bhu128
Leet, M. P., Bear, M. F., and Gaier, E. D. (2022). Metaplasticity: a key to visual recovery from amblyopia in adulthood? Curr. Opin. Ophthalmol. 33, 512–518. doi: 10.1097/ICU.0000000000000901
Levartovsky, S., Oliver, M., Gottesman, N., and Shimshoni, M. (1995). Factors affecting long term results of successfully treated amblyopia: initial visual acuity and type of amblyopia. Br. J. Ophthalmol. 79, 225–228. doi: 10.1136/bjo.79.3.225
LeVay, S., Wiesel, T. N., and Hubel, D. H. (1980). The development of ocular dominance columns in normal and visually deprived monkeys. J. Comp. Neurol. 191, 1–51. doi: 10.1002/cne.901910102
Levi, D. M., and Klein, S. A. (1986). Sampling in spatial vision. Nature 320, 360–362. doi: 10.1038/320360a0
Lewis, T., Maurer, D., and Brent, H. (1986). Effects on perceptual development of visual deprivation during infancy. Br. J. Ophthalmol. 70, 214–220. doi: 10.1136/bjo.70.3.214
Lewis, T. L., Maurer, D., and Brent, H. P. (1995). Development of grating acuity in children treated for unilateral or bilateral congenital cataract. Invest. Ophthalmol. Vis. Sci. 36, 2080–2095.
Li, J., Thompson, B., Deng, D., Chan, L. Y. L., Yu, M., and Hess, R. F. (2010). Dichoptic training enables the adult amblyopic brain to learn. Curr. Biol. 23, R308–R309. doi: 10.1016/j.cub.2013.01.059
Linden, M. L., Heynen, A. J., Haslinger, R. H., and Bear, M. F. (2009). Thalamic activity that drives visual cortical plasticity. Nat. Neurosci. 12, 390–392. doi: 10.1038/nn.2284
Loudon, S. E., Passchier, J., Chaker, L., de Vos, S., Fornius, M., Harrad, R. A., et al. (2009). Psychological causes of non-compliance with electronically monitored occlusion therapy for amblyopia. Br. J. Ophthalmol. 93, 1499–1503. doi: 10.1136/bjo.2008.149815
Lunghi, C., Sframeli, A. T., Lepri, A., Lepri, M., Lisi, D., Sale, A., et al. (2018). A new counterintuitive training for adult amblyopia. Ann. Clin. Transl. Neurol. 6, 274–284.
Maurer, D. (2017). Critical periods re-examined: evidence from children treated for dense cataracts. Cogn. Dev. 42, 27–36. doi: 10.1016/j.cogdev.2017.02.006
Maya Vetencourt, J. F., Sale, A., Viegi, A., Baroncelli, L., De Pasquale, R., O’Leary, OF, et al. (2008). The antidepressant fluoxetine restores plasticity in the adult visual cortex. Science 320, 385–388. doi: 10.1126/science.1150516
Meier, K., and Giaschi, D. (2017). Unilateral amblyopia affects two eyes: fellow eye deficits in amblyopia. Invest. Ophthalmol. Vis. Sci. 58, 1779–1800. doi: 10.1167/iovs.16-20964
Mitchell, D. E., and Maurer, D. (2022). Critical periods in vision revisited. Annu. Rev. Vis. Sci. 8, 291–321. doi: 10.1146/annurev-vision-090721-110411
Mitchell, D. E., and Sengpiel, F. (2018). Animal models of amblyopia. Vis. Neurosci. 35:E017. doi: 10.1017/S0952523817000244
Montey, K. L., and Quinlan, E. M. (2011). Recovery from chronic monocular deprivation following reactivation of thalamocortical plasticity by dark exposure. Nat. Commun. 2:317. doi: 10.1038/ncomms1312
Morishita, H., Miwa, J. M., Heintz, N., and Hensch, T. K. (2010). Lynx1, a cholinergic brake, limits plasticity in adult visual cortex. Science 330, 1238–1240. doi: 10.1126/science.1195320
Murase, S., Lantz, C. L., and Quinlan, E. M. (2017). Light reintroduction after dark exposure reactivates plasticity in adults via perisynaptic activation of MMP-9. elife 6:e27345. doi: 10.7554/eLife.27345
Murphy, K. M., Roumeliotis, G., Williams, K., Beston, W. K., and Jones, D. G. (2015). Binocular visual training to promote recovery from monocular deprivation. J. Vis. 15:15.1.2. doi: 10.1167/15.1.2
Olson, C. R., and Freeman, R. D. (1980). Profile of the sensitive period for monocular deprivation in kittens. Exp. Brain Res. 39, 17–21.
Pediatric Eye Disease Investigator Group (2002). The clinical profile of moderate amblyopia in children younger than 7 years. Arch. Ophthalmol. 120, 281–287. doi: 10.1001/archopht.120.3.281
Philpot, B. D., Sekhar, A. K., Shouval, H. Z., and Bear, M. F. (2001). Visual experience and deprivation bidirectionally modify the composition and function of NMDA receptors in visual cortex. Neuron 29, 157–169. doi: 10.1016/S0896-6273(01)00187-8
Pineles, S. L., Aakalu, V. K., Hutchinson, A. K., Galvin, J. A., Heidary, G., Binenbaum, G., et al. (2020). Binocular treatment of amblyopia: a report by the American Academy of Ophthalmology. Ophthalmology 127, 261–272. doi: 10.1016/j.ophtha.2019.08.024
Pizzorusso, T., Medini, P., Berardi, N., Chierzi, S., Fawcett, J. W., and Maffei, L. (2002). Reactivation of ocular dominance plasticity in adult visual cortex. Science 298, 1248–1251. doi: 10.1126/science.1072699
Pratt-Johnson, J., and Tillson, J. (1981). Visual results in congenital cataract surgery performed under the age of 1 year. Can. J. Ophthalmol. 16, 19–21.
Prusky, G. T., and Douglas, R. M. (2003). Developmental plasticity of mouse visual acuity. Eur. J. Neurosci. 17, 167–173. doi: 10.1046/j.1460-9568.2003.02420.x
Prusky, G. T., West, P. W., and Douglas, R. M. (2000). Experience-dependent plasticity of visual acuity in rats. Eur. J. Neurosci. 12, 3781–3786. doi: 10.1046/j.1460-9568.2000.00236.x
Quinlan, E. M., Philpot, B. D., Huganir, R. L., and Bear, M. F. (1999). Rapid, experience-dependent expression of synaptic NMDA receptors in visual cortex in vivo. Nat. Neurosci. 2, 352–357. doi: 10.1038/7263
Repka, M. X., Kraker, R. T., Dean, T. W., Beck, R. W., Siatkowski, R. M., Holmes, J. M., et al. (2015). A randomized trial of levodopa as treatment for residual amblyopia in older children. Ophthalmology 122, 874–881. doi: 10.1016/j.ophtha.2015.01.002
Resnick, H. H., Bear, M. F., and Gaier, E. D. (2023). Partial recovery of amblyopia after fellow eye ischemic optic neuropathy. J. Neurophthalmol. 43, 76–81. doi: 10.1097/WNO.0000000000001646
Rittenhouse, C. D., Siegler, B. A., Voelker, C. C., Shouval, H. Z., Paradiso, M. A., and Bear, M. F. (2006). Stimulus for rapid ocular dominance plasticity in visual cortex. J. Neurophysiol. 95, 2947–2950. doi: 10.1152/jn.01328.2005
Ryan, S. J., and Maumenee, A. E. (1977). Unilateral congenital cataracts and their management. Ophthalmic Surg. 8, 35–39.
Sale, A., Maya Vetencourt, J. F., Medini, P., Cenni, M. C., Baroncelli, L., De Pasquale, R., et al. (2007). Environmental enrichment in adulthood promotes amblyopia recovery through reduction of intracortical inhibition. Nat. Neurosci. 10, 679–681. doi: 10.1038/nn1899
Sawtell, N. B., Frenkel, M. Y., Philpot, B. D., Nakazawa, K., Tonegawa, S., and Bear, M. F. (2003). NMDA receptor-dependent ocular dominance plasticity in adult visual cortex. Neuron 38, 977–985. doi: 10.1016/S0896-6273(03)00323-4
Seabrook, T. A., Burbridge, T. J., Crair, M. C., and Huberman, A. D. (2017). Architecture, function, and assembly of the mouse visual system. Annu. Rev. Neurosci. 40, 499–538. doi: 10.1146/annurev-neuro-071714-033842
Sengpiel, F., and Blakemore, C. (1996). The neural basis of suppression and amblyopia in strabismus. Eye 10, 250–258. doi: 10.1038/eye.1996.54
Sharif, M. H., Talebnejad, M. R., Rastegar, K., Khalili, M. R., and Nowroozzadeh, M. H. (2019). Oral fluoxetine in the management of amblyopic patients aged between 10 and 40 years old: a randomized clinical trial. Eye 33, 1060–1067. doi: 10.1038/s41433-019-0360-z
Shatz, C. J., and Stryker, M. P. (1978). Ocular dominance in layer IV of the cat’s visual cortex and the effects of monocular deprivation. J. Physiol. 281, 267–283. doi: 10.1113/jphysiol.1978.sp012421
Silingardi, D., Scali, M., Belluomini, G., and Pizzorusso, T. (2010). Epigenetic treatments of adult rats promote recovery from visual acuity deficits induced by long-term monocular deprivation. Eur. J. Neurosci. 31, 2185–2192. doi: 10.1111/j.1460-9568.2010.07261.x
Sireteanu, R. (1982). Human amblyopia: consequence of chronic interocular suppression. Hum. Neurobiol. 1, 31–33.
Smith, D. C. (1981). Functional restoration of vision in the cat after long-term monocular deprivation. Science 213, 1137–1139. doi: 10.1126/science.7268422
Smith, E. L., Chino, Y. M., Ni, J., Cheng, H., Crawford, M. L., and Harwerth, R. S. (1997). Residual binocular interactions in the striate cortex of monkeys reared with abnormal binocular vision. J. Neurophysiol. 78, 1352–1362.
Smith, E. L., Harwerth, R. S., and Crawford, M. L. (1985). Spatial contrast sensitivity deficits in monkeys produced by optically induced anisometropia. Invest. Ophthalmol. Vis. Sci. 26, 330–342.
Sofi, I. A., Gupta, S. K., Bharti, A., and Tantry, T. G. (2016). Efficiency of the occlusion therapy with and without levodopa-carbidopa in amblyopic children – a tertiary care centre experience. Int. J. Health Sci. 10, 249–257. doi: 10.12816/0048817
Sommeijer, J.-P., Ahmadlou, M., Saiepour, M. H., Seignette, K., Min, R., Heimel, J. A., et al. (2017). Thalamic inhibition regulates critical-period plasticity in visual cortex and thalamus. Nat. Neurosci. 20, 1715–1721. doi: 10.1038/s41593-017-0002-3
Srinivasan, V. V., Das, S., and Patel, N. (2022). Widefield OCT imaging for quantifying inner retinal thickness in the nonhuman primate. Transl. Vis. Sci. Technol. 11:12. doi: 10.1167/tvst.11.8.12
Stewart, C. E., Moseley, M. J., and Fielder, A. R. (2003). Defining and measuring treatment outcome in unilateral amblyopia. Br. J. Ophthalmol. 87, 1229–1231. doi: 10.1136/bjo.87.10.1229
Stryker, M. P., and Harris, W. A. (1986). Binocular impulse blockade prevents the formation of ocular dominance columns in cat visual cortex. J. Neurosci. 6, 2117–2133. doi: 10.1523/JNEUROSCI.06-08-02117.1986
Suresh, V., Çiftçioğlu, U. M., Wang, X., Lala, B. M., Ding, K. R., Smith, W. A., et al. (2016). Synaptic contributions to receptive field structure and response properties in the rodent lateral geniculate nucleus of the thalamus. J. Neurosci. 36, 10949–10963. doi: 10.1523/JNEUROSCI.1045-16.2016
Teller, D. Y., and Boothe, R. (1962). Development of vision in infant primates. Trans. Ophthalmol. Soc. U. K. 99, 333–337.
Thorpe, P. A., and Blakemore, C. (1975). Evidence for a loss of afferent axons in the visual cortex of monocularly deprived cats. Neurosci. Lett. 1, 271–276. doi: 10.1016/0304-3940(75)90042-7
Tieman, S. B. (1984). Effects of monocular deprivation on geniculocortical synapses in the cat. J. Comp. Neurol. 222, 166–176. doi: 10.1002/cne.902220203
Trachtenberg, J. T., and Stryker, M. P. (2001). Rapid anatomical plasticity of horizontal connections in the developing visual cortex. J. Neurosci. 21, 3476–3482. doi: 10.1523/JNEUROSCI.21-10-03476.2001
Tychsen, L. (2020). Animal wrongs and animal rights: why nonhuman primate research is essential for children’s eye health. Am J. Ophthalmol. 216, A14–A17. doi: 10.1016/j.ajo.2020.04.042
Tychsen, L., and Burkhalter, A. (1995). Neuroanatomic abnormalities of primary visual cortex in macaque monkeys with infantile esotropia: preliminary results. J. Pediatr. Ophthalmol. Strabismus 32, 323–328. doi: 10.3928/0191-3913-19950901-13
Tychsen, L., Wong, A. M.-F., and Burkhalter, A. (2004). Paucity of horizontal connections for binocular vision in V1 of naturally strabismic macaques: cytochrome oxidase compartment specificity. J. Comp. Neurol. 474, 261–275. doi: 10.1002/cne.20113
Vagge, A., Shields, C. L., Shields, J. A., Pointdujour-Lim, R., and Schnall, B. (2020). Visual improvement in amblyopic eye following treatment-induced vision loss in the dominant eye with uveal melanoma. Br. J. Ophthalmol. 104, 202–207. doi: 10.1136/bjophthalmol-2018-313505
Vedamurthy, I., Nahum, M., Huang, S. J., Zheng, F., Bayliss, J., Bavelier, D., et al. (2015). A dichoptic custom-made action video game as a treatment for adult amblyopia. Vis. Res. 114, 173–187. doi: 10.1016/j.visres.2015.04.008
Venturino, A., Schulz, R., De Jesús-Cortés, H., Maes, M. E., Nagy, B., Reilly-Andújar, F., et al. (2021). Microglia enable mature perineuronal nets disassembly upon anesthetic ketamine exposure or 60-HZ light entrainment in healthy brain. Cell Rep. 36:109313. doi: 10.1016/j.celrep.2021.109313
von Grünau, M. W., and Rauschecker, J. P. (1983). Natural strabismus in non-Siamese cats: lack of binocularity in the striate cortex. Exp. Brain Res. 52, 307–310. doi: 10.1007/BF00236640
von Noorden, G. K., and Maumenee, A. E. (1967). Clinical observations on stimulus-deprivation amblyopia (amblyopia ex anopsia). Trans. Am. Ophthalmol. Soc. 65, 244–255. doi: 10.1016/0002-9394(68)93590-3
Wallace, D. K., Lazar, E. L., Melia, M., Birch, E. E., Holmes, J. M., Hopkins, K. B., et al. (2011). Stereoacuity in children with anisometropia. J. AAPOS 15, 455–461. doi: 10.1016/j.jaapos.2011.06.007
Wallace, D. K., Repka, M. X., Lee, K. A., Melia, M., Christiansen, S. P., Morse, C. L., et al. (2018). Amblyopia preferred practice pattern. Ophthalmology 125, P105–P142. doi: 10.1016/j.ophtha.2017.10.008
Wang, H., Levi, D. M., and Klein, S. A. (1998). Spatial uncertainty and sampling efficiency in amblyopic position acuity. Vis. Res. 38, 1239–1251. doi: 10.1016/S0042-6989(97)00278-2
Webber, A. L., and Wood, J. (2005). Prevalence, natural history, functional effects and treatment. Clin. Exp. Optom. 88, 365–375. doi: 10.1111/j.1444-0938.2005.tb05102.x
Wiesel, T. N., and Hubel, D. H. (1963a). Single-cell responses in striate cortex of kittens deprived of vision in one eye. J. Neurophysiol. 26, 1003–1017. doi: 10.1152/jn.1963.26.6.1003
Wiesel, T. N., and Hubel, D. H. (1963b). Effects of visual deprivation on morphology and physiology of cells in the cats lateral geniculate nucleus. J. Neurophysiol. 26, 978–993. doi: 10.1152/jn.1963.26.6.978
Wiesel, T. N., and Hubel, D. H. (1974). Ordered arrangement of orientation columns in monkeys lacking visual experience. J. Comp. Neurol. 158, 307–318. doi: 10.1002/cne.901580306
Wu, C., Gaier, E. D., Nihalani, B. R., Whitecross, S., Hensch, T. K., and Hunter, D. G. (2023). Durable recovery from amblyopia with donepezil. Sci. Rep. 13:10161. doi: 10.1038/s41598-023-34891-5
Xiao, S., Angjeli, E., Wu, H. C., Gaier, E. D., Gomez, S., Travers, D. A., et al. (2022). Randomized controlled trial of a dicoptic digital therapeutic for amblyopia. Ophthalmology 129, 77–85. doi: 10.1016/j.ophtha.2021.09.001
Zhou, J., He, Z., Wu, Y., Chen, X., Liang, Y., Mao, Y., et al. (2019). Inverse occlusion: a binocularly motivated treatment for amblyopia. Neural. Plast. 2019:5157628.
Keywords: amblyopia, monocular deprivation, neural plasticity (NP), animal models, neuropathology, amblyopia therapies, recovery
Citation: Duffy KR, Bear MF, Patel NB, Das VE and Tychsen L (2023) Human deprivation amblyopia: treatment insights from animal models. Front. Neurosci. 17:1249466. doi: 10.3389/fnins.2023.1249466
Edited by:
Ewa Niechwiej-Szwedo, University of Waterloo, CanadaReviewed by:
Krista Rose Kelly, Retina Foundation of the Southwest, United StatesDaphne Maurer, McMaster University, Canada
Katsuro Kameyama, Tottori University, Japan
Copyright © 2023 Duffy, Bear, Patel, Das and Tychsen. This is an open-access article distributed under the terms of the Creative Commons Attribution License (CC BY). The use, distribution or reproduction in other forums is permitted, provided the original author(s) and the copyright owner(s) are credited and that the original publication in this journal is cited, in accordance with accepted academic practice. No use, distribution or reproduction is permitted which does not comply with these terms.
*Correspondence: Kevin R. Duffy, a2V2aW4uZHVmZnlAZGFsLmNh