- 1Guangdong Key Laboratory for Genome Stability and Disease Prevention and Marshall Laboratory of Biomedical Engineering, Shenzhen University Medical School, Shenzhen, Guangdong, China
- 2Shenzhen University-Friedrich Schiller Universität Jena Joint PhD Program in Biomedical Sciences, Shenzhen University School of Medicine, Shenzhen, Guangdong, China
- 3Laboratory of Genome Stability, Leibniz Institute on Aging-Fritz Lipmann Institute, Jena, Germany
Primary microcephaly (MCPH), is a neurological disorder characterized by small brain size that results in numerous developmental problems, including intellectual disability, motor and speech delays, and seizures. Hitherto, over 30 MCPH causing genes (MCPHs) have been identified. Among these MCPHs, MCPH5, which encodes abnormal spindle-like microcephaly-associated protein (ASPM), is the most frequently mutated gene. ASPM regulates mitotic events, cell proliferation, replication stress response, DNA repair, and tumorigenesis. Moreover, using a data mining approach, we have confirmed that high levels of expression of ASPM correlate with poor prognosis in several types of tumors. Here, we summarize the neurological and non-neurological functions of ASPM and provide insight into its implications for the diagnosis and treatment of MCPH and cancer.
1. Introduction
Primary microcephaly (MCPH) is a neurodevelopmental disorder characterized by small brain size primarily due to the reduced cerebral cortex, varying degrees of intellectual disability (Woods et al., 2005; Mahmood et al., 2011; Phan and Holland, 2021; Zaqout and Kaindl, 2021; Gupta, 2023), and several additional neurological problems, such as seizures and epilepsy (Shen et al., 2005), with a prevalence ranging from 1/30,000 to 1/250,000. The development of brain relies on neurogenesis, the process by which neural stem cells proliferate, migrate, and differentiate to form neurons, is fundamental to normal brain development (Stiles and Jernigan, 2010; Isaev et al., 2019; Zhou et al., 2020). Neuron formation begins during embryogenesis and continues throughout life. In mammals, the size of the cerebral cortex is determined by the number of neurons it contains (Borrell and Calegari, 2014). In general, the human adult comprises about 86 billion neurons (Herculano-Houzel, 2012) and brain size range from 975 to 1,499 cm3. Studies have shown that a reduced number of neurons results in primary microcephaly, which is diagnosed when the occipital frontal circumference is smaller than two standard deviations below the mean at birth and/or smaller than three standard deviations below the mean after 1 year of age (Duerinckx et al., 2020).
At least 30 MCPHs (MCPH1–MCPH30) have been mapped to date. Mutations in MCPH5, which encodes the ASPM protein, are the most common cause of MCPH, accounting for around 40% of the patient population (Nicholas et al., 2009). To date, functions in cell division (Fish et al., 2006; Capecchi and Pozner, 2015), neurogenesis (Fujimori et al., 2014; Passemard et al., 2016), genome stability (Fujimori et al., 2008; Xu et al., 2021; Wu et al., 2022), and disease development (Fujimori et al., 2014; Liu et al., 2018) have all been annotated for ASPM. Here, we summarize the neurological and non-neurological functions of ASPM and provide insight into its implications for the diagnosis and treatment of MCPH and cancer.
2. Molecular and cellular characteristics of ASPM
To better understand ASPM functions in health and disease, it is important to delineate the structure and cellular roles of ASPM. As a member of the ASH (ASPM, SPD-2, and Hydin) domain-containing protein family, ASPM is the human homolog of the Drosophila melanogaster abnormal spindle protein (asp). ASPM is encoded by MCPH5 on chromosome 1q31.3, a gene that was originally identified in studies of consanguineous Northern Pakistani families (Jamieson et al., 2000; Pattison et al., 2000; Bond et al., 2002). MCPH5 has 28 exons and at least two alternative splicing isoforms: isoform 1 (full-length, amino acids 1–3,477) and isoform 2 (lacking the largest exon, exon 18, which encodes amino acids 1,356–2,940). Human ASPM protein contains four domains: a microtubule-binding domain (MTBD) at the N-terminal (NT), two calponin homology domains (CH), an isoleucine and glutamine domain (IQ motif), and a species-conserved C-terminal (CT; Figure 1).
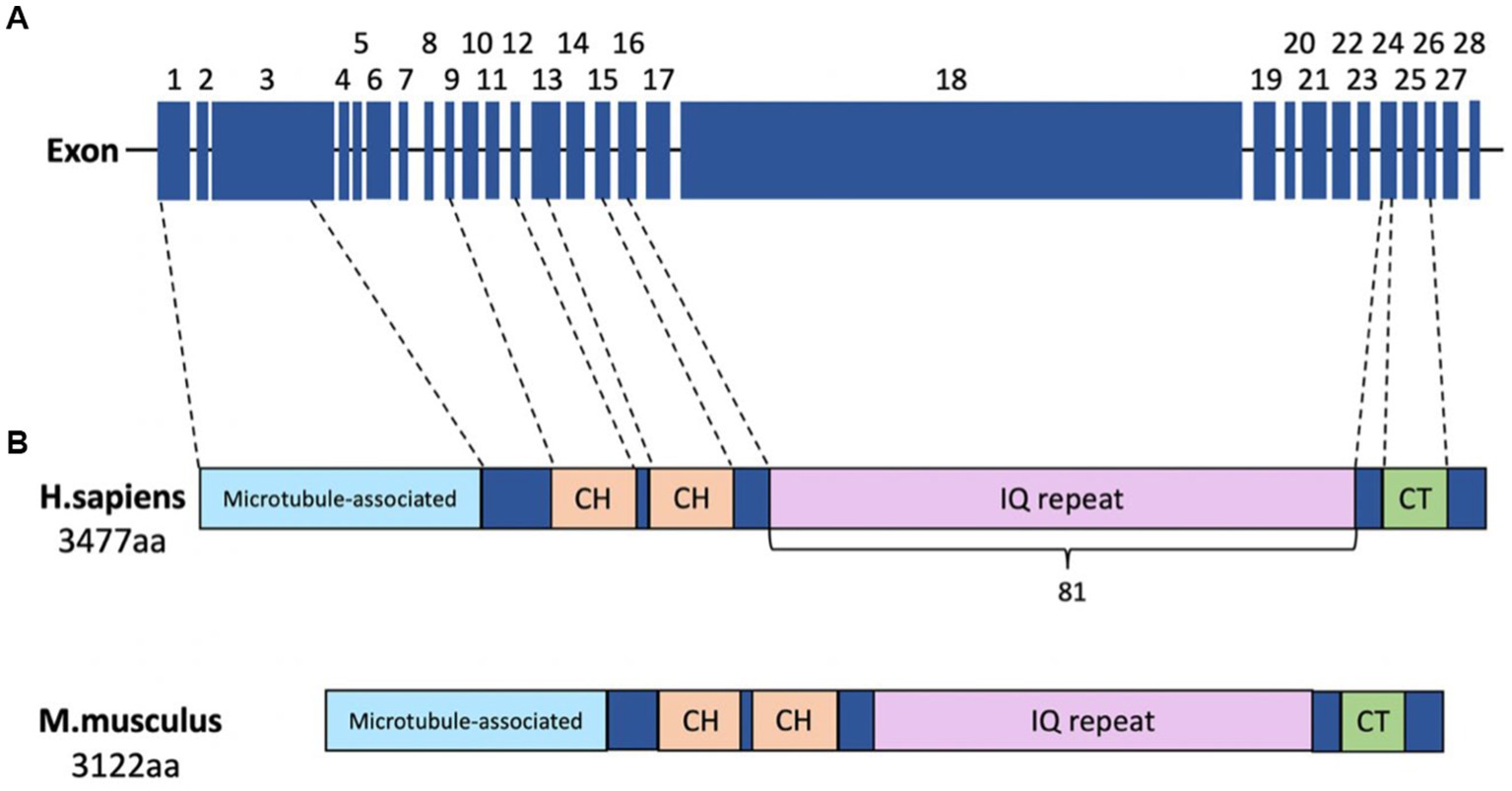
Figure 1. Schematic of human ASPM. (A) The 28 exons of the human ASPM gene, including the largest exon, exon 18 (4.7 kb). (B) Schematic showing the known domains of the human ASPM protein: N-terminal microtubule-binding domain (blue); two calponin homology (CH) domains (orange); 81 isoleucine and glutamine (IQ) repeats (pink); and the C-terminal (CT) domain (green).
The MTBD facilitates the localization of ASPM to the spindle pole and mediates an interaction between ASPM and microtubules that is responsible for the dynamic regulation of microtubules during cell division and neurogenesis (Jiang et al., 2017). The CH domains, commonly found in actin-binding proteins, are also thought to be involved in the interactions between ASPM and the actin cytoskeleton or microtubules (van der Voet et al., 2009). The 81 IQ repeats, many of which are organized into a higher-order repeat structure (Kouprina et al., 2005), are implicated in calmodulin binding (van der Voet et al., 2009).
ASPM primarily localizes at the centrosome and the spindle poles during cell division (Tungadi et al., 2017; Sepulveda et al., 2018). It is predicted to be associated with cilia (Schou et al., 2014; Verdier et al., 2016). Studies in U2OS cells have shown expression of ASPM in the nucleus of interphase cells prior to nuclear envelope breakdown (Higgins et al., 2010). During mitosis, ASPM is recruited to the pericentriolar matrix surrounding γ-tubulin at the spindle pole in a microtubule-dependent manner (Kouprina et al., 2005; Higgins et al., 2010; Figure 2). Further studies have shown ASPM localization at the mitotic spindle poles (Kouprina et al., 2005; Bond and Woods, 2006; Fish et al., 2006) and the midbody ring (Paramasivam et al., 2007) in mammals (Bond and Woods, 2006) and rat neuronal progenitors in the embryonic neocortex (Paramasivam et al., 2007).
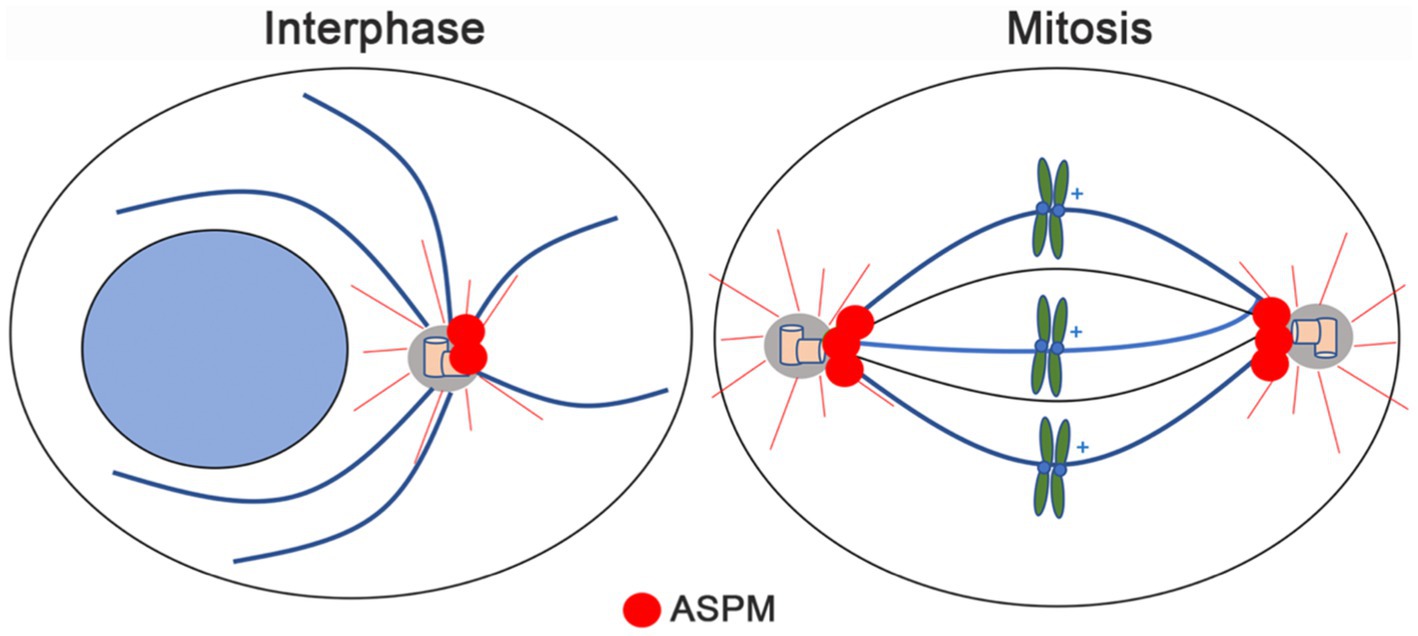
Figure 2. Schematic showing the cellular localization of ASPM during interphase and mitosis. ASPM signal (red dots) is primarily detected at the centrosome in interphase, while during cell division, ASPM signal is enriched at the spindle poles.
ASPM localization at the centrosome and spindle poles, is primarily known for regulating symmetric cell division, during which a mother cell divides into two identical daughter cells (Kouprina et al., 2005; Neumuller and Knoblich, 2009). The correct orientation of the spindle apparatus is a key determinant of symmetric cell division that ensures the accurate segregation of chromosomes (Fish et al., 2006; Higgins et al., 2010; Gai et al., 2016). In neural stem cells and progenitor cells, symmetric division is essential for the expansion of the progenitor cell pool and the generation of an adequate number of neurons during brain development (Fish et al., 2008; Knoblich, 2008; Neumuller and Knoblich, 2009; Jayaraman et al., 2018). Meanwhile, maintaining a balance of symmetric and asymmetric cell division is critical for normal brain development and tissue homeostasis (Gomez-Lopez et al., 2014; Taverna et al., 2014).
ASPM interacts with the minus ends of microtubules and plays a vital role in spindle assembly and orientation, microtubule-based transport, and cytokinesis (Higgins et al., 2010; Tungadi et al., 2017). In mouse embryonic neuroepithelial cells, loss of ASPM altered neuroepithelial cleavage plane orientation, resulting in deviation of the spindle position and an increase in asymmetric division rather than symmetric division (Fish et al., 2006). In the developing cerebellum, knockout of ASPM in cerebellar granule neuron progenitor cells impaired mitotic progression and altered division pattern orientation (Williams et al., 2015). Interestingly, two truncated forms of ASPM (missing exons 1–7 or 1717 C-terminal amino acids) in mice caused microcephaly, while only the mice lacking the C-terminal domain showed spindle misorientation (Pulvers et al., 2010; Capecchi and Pozner, 2015).
Mechanisms underlying ASPM’s regulation of spindle orientation have been investigated in several studies. For instance, Gai et al. identified an interaction between the cytokinesis regulator citron kinase (CITK), also known as MCPH17, and ASPM. CITK served as a downstream factor of ASPM, modulating spindle orientation in a kinase-dependent manner (Gai et al., 2016), and its localization at the spindle poles was ASPM-dependent. Moreover, overexpression of CITK in ASPM-depleted HeLa cells rescued the misorientation phenotype, demonstrating that these two microcephaly proteins function together to regulate spindle orientation (Gai et al., 2016). In another study, Jiang et al. used X-ray crystallography to identify a complex (ASPM-p60/p80) comprising of ASPM and the p60/p80 subunits of katanin, a microtubule-severing ATPase (Jiang et al., 2017). In Drosophila, ASPM-dependent recruitment of katanin to the microtubules enhanced the minus-end blocking activity of ASPM, which could suppress microtubule minus-end growth, while disruption of the interaction between ASPM and katanin caused impaired spindle orientation and poleward flux (Schoborg et al., 2015). In addition, T Schoborg et al. denmostrated that Asp-CaM (Drosophila melanogaster calmodulin) complex is required for centrosome-pole cohesion and centrosome inheritance in neural stem cells (Schoborg et al., 2015). These studies revealed the role of ASPM, together with its binding partners, in regulating symmetric cell division by modulating microtubule dynamics and spindle orientation.
3. ASPM in neurogenesis
Neurogenesis is a highly intricate and precise process involving the generation of functional neurons from neural progenitor cells (NPCs; Ming and Song, 2005, 2011). In mammals, two major brain regions are responsible for neurogenesis: the ventricular zone (VZ) and the subventricular zone (SVZ). During the early stage of neocortex development, the embryonic telencephalon wall is formed, consisting of neuroepithelial cells with apicobasal polarity (Woodworth et al., 2012; Jayaraman et al., 2018). These neuroepithelial cells undergo dynamic nuclear migration along the apical-basal axis in coordination with the cell cycle (Fish et al., 2008). Subsequently, they differentiate into multipotent NPCs capable of generating various cell types, including neurons and glial cells. Radial glial cells, which are located in the VZ, generate neurons and maintain self-renewal through multiple rounds of asymmetric divisions (Misson et al., 1988; Noctor et al., 2001). Additionally, radial glial cells can generate intermediate progenitors, which translocate to the SVZ and undergo symmetric proliferation or neurogenic divisions (Noctor et al., 2001; Jayaraman et al., 2018; de Almeida et al., 2023).
3.1. ASPM expression during neurogenesis
In mice, ASPM exhibits high expression levels in the cerebral cortical VZ at embryonic day (E) 14.5, when there are many progenitor cells. Its levels begin to decrease at E16.5 and are greatly reduced by postnatal day (P) 0, when the cortical VZ is fully formed (Bond et al., 2002). After birth, ASPM is also continuously expressed in zones of postnatal neurogenesis and adult tissues such as the dentate gyrus, cerebellar granule neurons, and the SVZ of the rostral migratory stream fated to become olfactory bulb neurons (Bond et al., 2002; Kouprina et al., 2005; Marinaro et al., 2011; Fujimori et al., 2014; Williams et al., 2015). These observations suggest that ASPM is preferentially expressed during cerebral cortical neurogenesis both before and after birth (Bond et al., 2002). Furthermore, the centrosomal localization of ASPM during interphase and mitotic spindle localization during mitosis has been demonstrated in mouse neuroepithelial cells at E12.5 (Kouprina et al., 2005).
The determinats of the cerebral cortex size include neurons number and neuronal migration during neurogenesis. In general, the final number of cortical neurons have a fundamental impact on the size of the mature cerebral cortex (Fernandez et al., 2016). Besides, B Nadarajah. et al. showed the importance of early-generated neurons in the layer formation and cortical connection establishment and elucidatied migration of neurons occurs during the whole period of corticogenesis and along multiple tangential routes to their destinations in the developing cortex, newly generated neurons must migrate to their appropriate locations within the developing brain to integrate into the cortical layers. Indicating the role of neuronal migration in maintaining cortical surface area (Nadarajah and Parnavelas, 2002; Nadarajah et al., 2003). Despite ASPM’s high expression during neurogenesis, its specific function in this context remains poorly understood. Several studies have shown that ASPM expression is required to balance symmetric proliferative division and differentiation in NPCs (Kouprina et al., 2005; Zhong et al., 2005; Fish et al., 2006; Horvath et al., 2006; Fujimori et al., 2014), as well as for neuronal migration (Buchman et al., 2011; Fujimori et al., 2014) and neural stem cell self-renewal (Horvath et al., 2006; Paik et al., 2009). To monitor the long-term fate of ASPM-expressing cells in vivo during neurogenesis, Marinaro et al. generated Aspm-CreERT2/Nestin-GFPflox-TK mice using the Cre-LoxP system. Tamoxifen was injected into mice at E12.7 and E13.2 to activate the thymidine kinase (TK) gene, and ganciclovir was administered from E14.5 until E18.5 to selectively kill ASPM-positive/TK-positive cells. The mice exhibited severe impairments in forebrain development, SVZ cell proliferation, and the laminar organization of the cortex (Marinaro et al., 2011). ASPM is also required for the orientation of dividing progenitors and neuronal migration in mouse neocortex. Knockdown of Aspm in the telencephalic hemisphere of E10.5 or E12.5 mice via endoribonuclease-prepared, short interfering RNAs altered the orientation of the neuroepithelial cleavage plane, causing it to become less perpendicular to the ventricular surface (Fish et al., 2006). In addition, using Aspm−/− mice generated by Cre-loxP-mediated deletion of exons 2 and 3, Fujimori et al. found disruption of cortical layer-specific transcription factor expression (Satb2, Ctip2, Tbr1) in E16.5 embryos and a thinner cortical layer VI in the adult neocortex, suggesting that loss of ASPM impaired neuronal differentiation (Fujimori et al., 2014). The effects of ASPM depletion, namely altered differentiation, premature cell cycle exit, and apoptosis ultimately reduce cerebellar growth (Williams et al., 2015).
In addition to its role in embryogenesis, ASPM is also involved in neurogenesis in adult mouse tissues. For example, in P30 mouse SVZ, descendants of Aspm-positive cells were shown to promote the generation of neurons, astrocytes, and cells of oligodendrocyte lineage (Marinaro et al., 2011). Moreover, cerebral organoid culture in vitro to generate human brain-like organs has advanced research into human brain disease, particularly with regard to neurogenesis in the developing neocortex (Li et al., 2017). For example, using patient-specific induced pluripotent stem cells with a dysfunctional ASPM gene to generate cerebral organoids, Li et al. found loss of luminal structures and neural precursors, consistent with the Aspm−/− mouse phenotype (Li et al., 2017).
Bond et al. firstly reported human ASPM as a determinant of cerebral cortical size, suggesting that brain size is partially modulated by its mitotic spindle activity (Bond et al., 2002). The correlation between ASPM and brain size has been confirmed in multiple species, including humans, mice, zebrafish, and ferret (Bond et al., 2003; Kim et al., 2011; Johnson et al., 2018; Ogi et al., 2018). Individuals with ASPM mutations may have variable levels of delayed development in various areas, such as motor, speech, and language skills, and cognitive abilities (Naseer et al., 2020; Liaci et al., 2021). Therefore, understanding the functions of ASPM in neurogenesis is crucial. Notably, the clinical manifestations of ASPM-related microcephaly can vary among individuals and may be influenced by the specific mutation present. Numerous mutations in the ASPM gene have been identified in MCPH patients (Table 1), some of which have been incorporated into different animal models seeking to investigate the pathological mechanisms of ASPM mutation in microcephaly. Below, we summarize the detailed functions of ASPM in several animal models.
3.2. Animal models for ASPM
To better understand the function of ASPM in the development of the cerebral cortex and other organs, several studies have used different genetic approaches in various species (Drosophila, mice, ferrets, and zebrafish) to edit ASPM, based on human mutations. These studies have uncovered the molecular mechanisms underlying the microcephaly phenotype (Gonzalez et al., 1988, 1990; Bond et al., 2003; Pulvers et al., 2010; Kim et al., 2011; Fujimori et al., 2014; Johnson et al., 2018; Ogi et al., 2018; Mori et al., 2022).
3.2.1. Drosophila
Drosophila, which has a similar neurodevelopment with human, acts as a desirable animal model to study human neurodevelopmental disorders such as microcephaly (Robinson et al., 2020). From centrosome studies in Drosophila, many human microcephaly genes were originally identified including Asp, merry-go-round (mgr) and polo (Ripoll et al., 1985; Gonzalez et al., 1988; Sunkel and Glover, 1988; Singh et al., 2014; Jana et al., 2016; Ramdas Nair et al., 2016). Drosophila syncytial embryos and larvae with Asp mutants exhibited high mitotic index (MI) and notable presence of hyperploid and polyploid cells (Ripoll et al., 1985; Gonzalez et al., 1990). Lately, Asp was been found as a microtubule-binding protein that localizes to the mitotic spindle polar and maintain the spindle stability in Drosophila (Saunders et al., 1997; Gonzalez et al., 1998; do Carmo Avides and Glover, 1999). Downregulation of Asp by siRNA in S2 cells caused increased mitotic index, loss of spindle pole focus and detached centrosomes (Morales-Mulia and Scholey, 2005). Furthermore, Schoborg et al. and Goshima et al. found the activity of Asp is regulated by calmodulin (CaM), interaction of both is required for focused spindle pole and centrosome detachment (Goshima et al., 2007; Schoborg et al., 2015). To analyze the Asp function in neural development, Rujano et al. characterized a Asp mutant (2,396–2,402 bp missing) with a premature stop codon at amino acid 721 and found defects in the brain size and neuroepithelium morphogenesis (Rujano et al., 2013).
3.2.2. Mice
In mouse embryonic stem (ES) cells, gene trapping is an efficient method of genome mutagenesis that can help to elucidate the roles of genes in specific biological pathways (Friedel and Soriano, 2010). Utilizing this technique, scientists generated mice with various ASPM truncations from gene trap ES cells: AJ0069 (AspmGt(AJ0069)Wtsi), in which ASPM was truncated between exons 25 and 26 of ASPM; AA0137 (AspmGt(AA0137)Wtsi), in which ASPM was truncated between exons 7 and 8; and ASPM SA/SA mice, in which ASPM was truncated between exons 6 and 7 (Pulvers et al., 2010; Williams et al., 2015). Among the mutated mice, both AspmGt(AJ0069)Wtsi and AspmGt(AA0137)Wtsi-hom (homozygotes) showed reduced brain weight in P0.5 day neonates and 8- to 12-week-old adults, while ASPM SA/SA mice showed reduced brain and cerebellar weight at P30W. The microcephaly phenotype in AspmGt(AA0137)Wtsi-hom mice was rescued by expression of human ASPM, indicating the specific role of ASPM mutation in microcephaly.
Two studies using similar strategies to generate CAG-driven Cre-loxP conditional Aspm knockout mice showed decreased fractional anisotropy (FA) values which is mostly used to quantify white matter integrity in the cortex and the changes of FA were closely correlated with neuropathology, including abnormal neurite outgrowth and differentiation, white matter at P5W, reduced brain size in the neocortex, thinner cortical layer VI, and significantly reduced testis weight at P12W, compared with Aspm+/+ mice (Fujimori et al., 2014; Ogi et al., 2018). In addition, in mouse embryos, Martínez et al. recently used CRISPR-Cas9 technology to insert a stop codon into exon 3 of Aspm, which partially reduced ASPM levels at the centrosome and caused mild microcephaly, with decreased brain weight and volume at P30 (González-Martínez et al., 2021).
Notably, in addition to microcephaly, mice carrying Aspm mutations showed decreased fertility, with reductions in testicular size, oocyte number, ovarian weight, pregnancy rate, and offspring number. In addition, in female conditional Aspm knockouts, ovary size was reduced, and there were lower numbers of developing follicles during postnatal maturation and aging, suggesting the crucial role of ASPM in ovarian development (Pulvers et al., 2010; Mori et al., 2022).
It is worth noting, however, that some studies have shown a milder form of microcephaly in mutated mice, compared with that observed in human microcephaly patients. This reduced severity may be due to differences in brain size, gyrification, and progenitor divisions in mice and humans (Pulvers et al., 2010; Johnson et al., 2018).
3.2.3. Ferret
Due to the limited effect of Aspm mutations in mice and the considerable differences between mouse and human brains, some studies have used the ferret as an alternative model animal. Ferrets have a larger, gyrified cortex and greater NPC diversity than mice, and ferret ASPM shares a greater level of homology with the human ASPM (Fietz et al., 2010). Johnson et al. generated Aspm knockout ferrets by carrying out genome editing to target exon 15 with a mutation identified in a previous study (Bond et al., 2003; Johnson et al., 2018). Aspm knockout ferrets showed robust microcephaly, with a reduction in brain weight of around 25–40%. This reduction reflected the loss of cortical units caused by the premature translocation of ventricular radial glial cells to the outer SVZ (Johnson et al., 2018). These findings suggested that ASPM controls the progress of cortical expansion, thus ensuring normal brain development.
3.2.4. Zebrafish
In zebrafish, Kim et al. knocked down aspm by using morpholino antisense oligonucleotides (MO) to target the exon 11 splice donor site, thereby blocking translation (Kim et al., 2011). In the mutated zebrafish, brain size was reduced at 35 h post-fertilization, and the cells showed mitotic arrest followed by apoptotic cell death (Kim et al., 2011). These findings underscore the importance of ASPM in regulating brain development across different species and highlight the correlation between mitotic function and early brain development.
Overall, these findings support the notion that ASPM plays a significant role in neurogenesis by maintaining the pool of NPCs and regulating their differentiation. It has been suggested that positive selection of ASPM may have contributed to the evolutionary expansion of the human brain (Gonzalez et al., 1988, 1990; Mori et al., 2022).
4. ASPM regulates genome stability
While the growth inhibition in cerebellar and medulloblastoma has been confirmed in ASPM knockout animal models, an increase of DNA damage and apoptosis was also noted (Williams et al., 2015). This suggested that ASPM may have functions in maintaining genome stability and cell survival.
4.1. ASPM in DNA replication
DNA replication is an essential cellular event that favors cell growth and proliferation. Increasing numbers of studies have shown that defects in DNA replication initiation and replication stress cause cortical malformations such as microcephaly (Bicknell et al., 2011; de Munnik et al., 2012; Jackson et al., 2014; Reynolds et al., 2017; Jayaraman et al., 2018). Some microcephaly genes are also involved in DNA replication. For example, the microcephaly gene DONSON encodes a replication fork protein that maintains genome stability by stabilizing stalled forks and activating replication checkpoints (Reynolds et al., 2017). Several bioinformatic methods have indicated that ASPM, along with its upstream regulator trophinin-associated protein (TROAP) and downstream factor cell division cycle 20 (CDC20), may regulate cell replication during the S and G2 cell cycle phases; however, details of the mechanism involved remain unknown (Liu et al., 2022). Recently, a study carried out by our group in HeLa cells uncovered the function of ASPM in maintaining genome stability in response to replication stress (Wu et al., 2022). We identified potential ASPM interaction partners from mass spectrum data, including several DNA replication factors, such as mini-chromosome maintenance protein 5 (MCM5), replication factor complex (RFC1-5), and replication protein A (RPA1, RPA2). Nonetheless, in ASPM knockout cells, we did not observe any differences in replication speed or percentages of cells in S phase, suggesting that ASPM is dispensable for normal replication. By contrast, following replication stress induced by hydroxyurea or aphidicolin, ASPM stabilized replication forks and antagonized the degradation of nascent DNA strands mediated by meiotic recombination 11 (MRE11) nuclease. Loss of ASPM also resulted in reduced activation of the ATR-CHK1 signaling pathway (Wu et al., 2022). This work provides new insights to enhance our understanding of the pathogenesis of ASPM loss in diseases such as microcephaly and cancer.
4.2. ASPM in DNA damage response
Diseases such as neurodevelopmental disorders, neurodegenerative disorders, aging-related conditions, and cancer are commonly caused by gene alterations arising from DNA damage, replication errors, chromosomal segregation defects, and other factors. Genomic DNA is constantly threatened by endogenous or exogenous factors, and efficient DNA damage repair is crucial for maintaining genome stability. Impairment of DNA damage repair leads to genetic alterations and can also cause microcephaly if it occurs during neural development (O'Driscoll and Jeggo, 2008; Zhou et al., 2013; Shiwaku and Okazawa, 2015; Wei et al., 2016; Martins et al., 2022). In Drosophila, DNA double-strand breaks caused by ionizing radiation (IR) treatment reportedly induced microcephaly by promoting the premature differentiation of neural stem cells and neuroblasts, without affecting apoptotic cell death (Barazzuol et al., 2017; Wagle and Song, 2020). Loss of ASPM also increased DNA damage in cerebellar granule neuron progenitors in mice (Williams et al., 2015), while ASPM expression was downregulated in IR-treated human cells (embryonic lung fibroblasts, HeLa, and MCF7), mouse embryonic brain cells, and neurospheres (Fujimori et al., 2008). The latter finding could explain the mechanism of microcephaly formation caused by IR (Fujimori et al., 2008). Moreover, ASPM loss sensitized glioblastoma cells (U87MG), cervical cancer cells (HeLa), and normal human fibroblasts (AG1521) to X-irradiation, H2O2, camptothecin, and increased chromosomal aberrations arising from impaired DNA repair (Kato et al., 2011). This sensitization may result from reduced levels of breast cancer type 1 susceptibility protein (BRCA1), a key factor involved in homologous recombination repair, but the precise mechanism is yet to be determined (Zhong et al., 2005). Recently, we demonstrated that ASPM is recruited to DNA damage sites, where it protects BRCA1 from degradation by antagonizing ubiquitination mediated by HECT domain and RCC1-Like domain-containing protein 2 (HERC2, an E3 ligase; Xu et al., 2021). By promoting efficient homologous recombination, ASPM maintained chromosome stability following X-ray-induced damage. However, further research is needed to enhance our understanding of the role of ASPM-related DNA repair in neurological disorders such as microcephaly.
4.3. ASPM in cancer
Cancer is a severe disease characterized by high rates of cell proliferation and continuous cell division. As an essential gene involved in regulating cell division, ASPM also contributes to cancer development. Wnt/β-catenin signaling, which is important for cell proliferation, organogenesis, tissue homeostasis, and embryonic development, is frequently activated in cancer cells (Schunk et al., 2021; Yu et al., 2021). Recently, studies demonstrated that ASPM interacts with and stabilizes disheveled-3 (Dvl-3), a cardinal upstream regulator of the Wnt signaling pathway. This interaction increases Wnt-induced β-catenin transcriptional activity, promoting proliferation, stemness properties, and tumorigenicity in prostate cancer cells, anaplastic thyroid cancer cells, and glioblastoma cells (Pai et al., 2019; Chen et al., 2020; Jiang et al., 2022). In addition, in a rapidly tumorigenic medulloblastoma mouse model, Aspm knockout significantly slowed medulloblastoma growth and increased DNA damage, suggesting that ASPM promotes tumorigenesis (Williams et al., 2015).
Based on The Cancer Genome Atlas database,1 comparison of tumor and normal tissues shows the upregulation of ASPM expression in many tumors (Figure 3A). Genomic analysis has also identified multiple ASPM mutations (Table 2) in tumors.2 Meanwhile, high levels of ASPM expression correlate with poor prognosis in various types of cancer (Figure 3B), including bladder cancer (Chen et al., 2019, 2021; Gao et al., 2020; Liu et al., 2023), prostate cancer (Xie et al., 2017; Pai et al., 2019; Xu et al., 2019), breast cancer (Shubbar et al., 2013; Tang et al., 2019; Wei et al., 2021; Alam et al., 2022; Wang et al., 2022), triple-negative breast cancer (Alam et al., 2022), esophageal cancer (ESCA; Xu et al., 2021), hepatocellular carcinoma (Lin et al., 2008; Li and Xu, 2020; Yang et al., 2021; Hu et al., 2022; Li et al., 2022; Qiao et al., 2022; Tan et al., 2022; Hasan et al., 2023; Hossen et al., 2023), glioblastoma (Visnyei et al., 2011; Qin et al., 2023), epithelial ovarian cancer (Brüning-Richardson et al., 2011; Alsiary et al., 2014; Wu et al., 2022), osteosarcoma (Liu et al., 2021), endometrial carcinoma (Liu et al., 2020; Zhang et al., 2022), malignant pleural mesothelioma (Zhang et al., 2020), cervical squamous cell carcinoma (Wen et al., 2020), lung adenocarcinoma (Feng et al., 2021; Hou et al., 2022; Tang et al., 2022; Yin et al., 2022; Zhang et al., 2022), anaplastic thyroid carcinoma (Fang et al., 2023), cutaneous squamous cell carcinoma (Su et al., 2022), human sarcomas (Tu et al., 2022), pancreatic ductal adenocarcinoma (Shi et al., 2022), anaplastic thyroid cancer (Jiang et al., 2022), and diffuse large B-cell lymphoma (Wu et al., 2021). Furthermore, analysis of The Comparative Toxicogenomics Database revealed ASPM as a hub gene in adenoid cystic carcinoma (Liu et al., 2023) and mucinous gastric carcinoma (Li et al., 2023). Thus, a huge amount of evidence points to a positive correlation between ASPM and cancer. Nonetheless, despite the function of ASPM in cell division, mechanistic details relating to its role in tumorigenesis require further investigation. A deeper understanding of the connection between ASPM and cancers will be critical to aid diagnosis and facilitate the development of therapeutic targets for tumorigenesis.
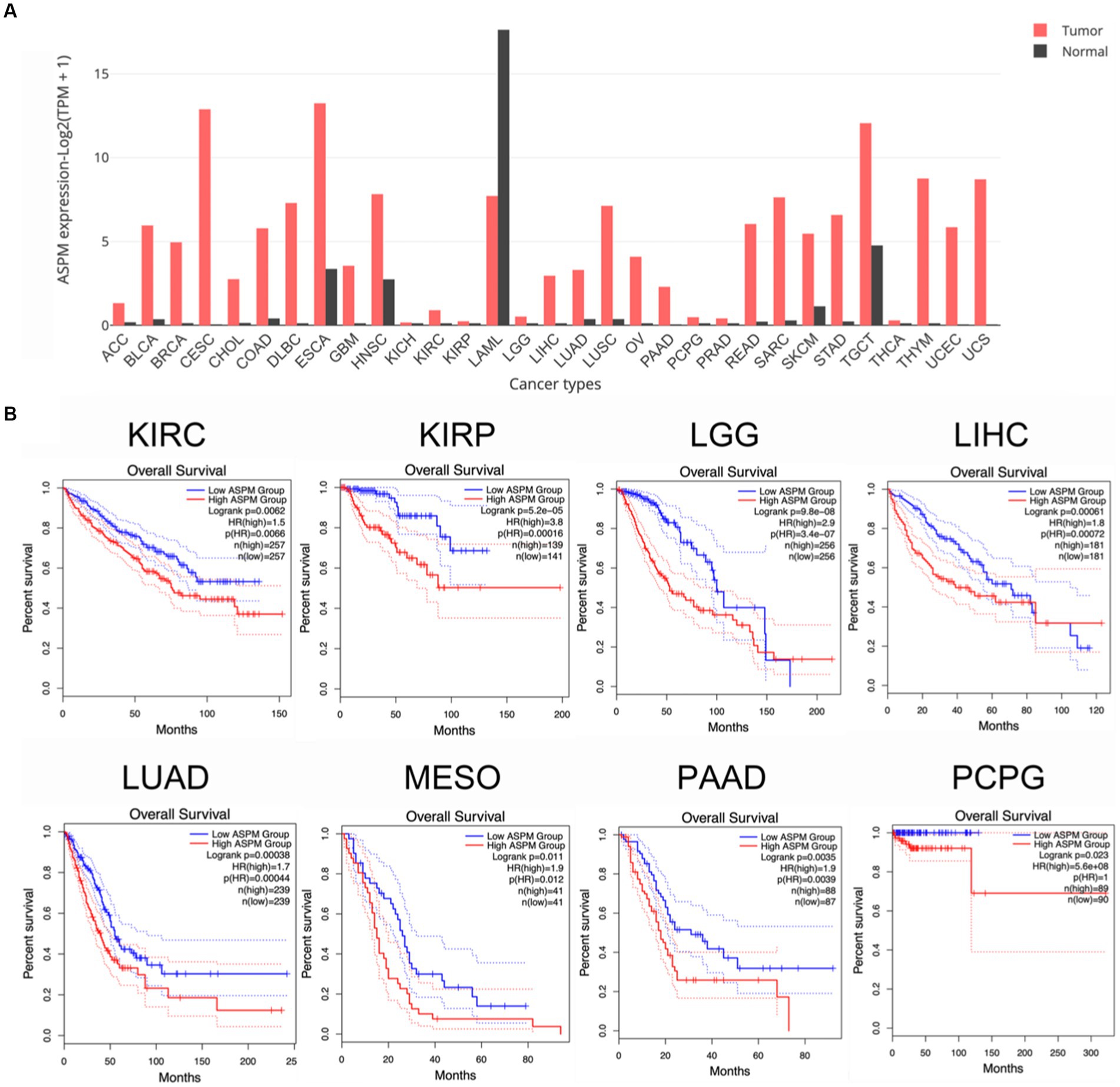
Figure 3. High levels of ASPM expression are associated with poor prognosis in various cancers based on The Cancer Genome Atlas and Gene Expression Profiling Interactive Analysis databases. (A) Bar plot of ASPM gene expression profiles for tumor samples and normal tissues. ACC, adrenocortical carcinoma; BLCA, bladder urothelial Carcinoma; BRCA, breast invasive carcinoma; CESC, cervical squamous cell carcinoma and endocervical adenocarcinoma; CHOL, cholangio carcinoma; COAD, colon adenocarcinoma; DLBC, diffuse large B-cell lymphoma; ESCA, esophageal carcinoma; GBM, glioblastoma multiforme; HNSC, head and neck squamous cell carcinoma; KICH, kidney chromophobe; KIRC, kidney renal clear cell carcinoma; KIRP, kidney renal papillary cell carcinoma; LAML, acute myeloid leukemia; LGG, brain lower grade glioma; LIHC, liver hepatocellular carcinoma; LUAD, lung adenocarcinoma; LUSC, lung squamous cell carcinoma; OV, ovarian serous cystadenocarcinoma; PAAD, pancreatic adenocarcinoma; PCPG, pheochromocytoma and paraganglioma; PRAD, prostate adenocarcinoma; READ, rectum adenocarcinoma; SARC, sarcoma; SKCM, skin cutaneous melanoma; STAD, stomach adenocarcinoma; TGCT, testicular germ cell tumor; THCA, thyroid carcinoma; THYM, thymoma; UCEC, uterine corpus endometrial carcinoma; UCS, uterine carcinosarcoma. (B) High levels of ASPM expression in different types of cancers are correlated with poor prognosis. Survival analysis was performed online (http://gepia.cancer-pku.cn) to analyze correlations between ASPM levels and overall survival in cancers.
5. Conclusion
In this review, we provided a comprehensive overview of the pathogenic mechanisms underlying microcephaly and cancer caused by ASPM mutations. We highlighted the functional aspects of ASPM mutations in relation to the symmetric cell division, proliferation, differentiation, and self-renewal of neural stem/progenitor cells, as well as in genomic stability and disease pathogenesis. Loss or mutation of ASPM leads to abnormal mitotic events in Drosophila, mouse, ferret, and human cultured cells. This is likely due to the abnormal activity of the spindle assembly checkpoint or mitotic slippage. ASPM, together with several interacting partners, including MCPH proteins (CITK, MCPH2; Paramasivam et al., 2007; Gai et al., 2016; Jayaraman et al., 2016), katanin (Jiang et al., 2017), calmodulin (van der Voet et al., 2009), cyclin E (Capecchi and Pozner, 2015), FOXO (Paik et al., 2009), and UBE3A (Singhmar and Kumar, 2011), contributes to normal mitotic progression and neurogenesis. In addition to these functionally confirmed partners, numerous potential interactors have been identified in mass spectrometry data from NCBI database.3 These include proteins involved in DNA repair [TP53 (Liu et al., 2020), MTOR (Hein et al., 2015)], microtubule formation [Aurora A (Adhikari et al., 2020)], cell cycle regulation [CDC16 (Huttlin et al., 2017, 2021), CEP78 (Hein et al., 2015), MYC (Heidelberger et al., 2018)], transcription [CREB3 (Huttlin et al., 2021), FOXJ1 (Huttlin et al., 2017, 2021), T53INP1 (Huttlin et al., 2017)], protein degradation [CUL3 (Bennett et al., 2010; Kouranti et al., 2022), HERC2 (Galligan et al., 2015)], protein chaperoning [DNAJB7 (Huttlin et al., 2021), DNAJB8 (Huttlin et al., 2021)], apoptosis [MYC (Heidelberger et al., 2018)], cell proliferation [NPM1 (Fasci et al., 2018)], kinetochore organization [Ndc80 (Hutchins et al., 2010)], and ciliary motility [ODAD1 (Huttlin et al., 2021)]. This array of binding partners offers new insight into the potential functions of ASPM. However, elucidating the mechanisms underlying the cooperation of ASPM with these factors in microcephaly and other diseases will require further investigation.
Mouse models have been widely used to study the function of ASPM, and research has revealed that ASPM mutations are found not only in microcephaly but also in other diseases and disorders. Indeed, it has been discovered that mice with Aspm mutations also exhibit reductions in sperm count and motility, as well as major defects in the male and female germlines (Pulvers et al., 2010). These findings highlight the complexity of ASPM function. In addition to the mechanisms mentioned above, our group has uncovered potential mechanisms involving ASPM in DNA repair and the DNA replication response, thus advancing our understanding of ASPM from alternative perspectives (Xu et al., 2021; Wu et al., 2022).
Overall, the pathogenic mechanisms of microcephaly are complex, with more than 30 known disease-causing genes identified (Xu et al., 2020; Phan and Holland, 2021; Zaqout and Kaindl, 2021; Razuvaeva et al., 2023) and, as a result, an increasingly broad range of research directions. The analysis of such heterogeneous disorders will facilitate a better understanding of human brain development and evolution. Moreover, refining and revising our understanding of the significant contributions of ASPM to brain development and other diseases, including cancer, will provide guidance for the diagnosis and treatment of this rare heterogeneous disease.
Author contributions
XX are responsible for the conception of this review and finalized the content of the manuscript. XW and ZL wrote the manuscript draft. XW, ZL, Z-QW, and XX scientifically edited the manuscript. All authors contributed to the article and approved the submitted version.
Funding
This work was supported by the National Natural Science Foundation of China (NSFC); grants (32090031, 31761133012, and 31530016) and Shenzhen Science and Technology Innovation Commission projects grants (JCYJ20220818095616035 and JCYJ201805073000163).
Acknowledgments
The authors would like to thank all members of the Xu laboratory for their help and useful discussions.
Conflict of interest
The authors declare that the research was conducted in the absence of any commercial or financial relationships that could be construed as a potential conflict of interest.
Publisher’s note
All claims expressed in this article are solely those of the authors and do not necessarily represent those of their affiliated organizations, or those of the publisher, the editors and the reviewers. Any product that may be evaluated in this article, or claim that may be made by its manufacturer, is not guaranteed or endorsed by the publisher.
Footnotes
References
Abaan, O. D., Polley, E. C., Davis, S. R., Zhu, Y. J., Bilke, S., Walker, R. L., et al. (2013). The exomes of the NCI-60 panel: a genomic resource for cancer biology and systems pharmacology. Cancer Res. 73, 4372–4382. doi: 10.1158/0008-5472.CAN-12-3342
Abdel-Hamid, M. S., Ismail, M. F., Darwish, H. A., Effat, L. K., Zaki, M. S., and Abdel-Salam, G. M. H. (2016). Molecular and phenotypic spectrum of ASPM-related primary microcephaly: identification of eight novel mutations. Am. J. Med. Genet. A 170, 2133–2140. doi: 10.1002/ajmg.a.37724
Adhikari, B., Bozilovic, J., Diebold, M., Schwarz, J. D., Hofstetter, J., Schröder, M., et al. (2020). PROTAC-mediated degradation reveals a non-catalytic function of AURORA-A kinase. Nat. Chem. Biol. 16, 1179–1188. doi: 10.1038/s41589-020-00652-y
Ahmad, I., Baig, S. M., Abdulkareem, A. R., Hussain, M. S., Sur, I., Toliat, M. R., et al. (2017). Genetic heterogeneity in Pakistani microcephaly families revisited. Clin. Genet. 92, 62–68. doi: 10.1111/cge.12955
Ahmed, J., Windpassinger, C., Salim, M., Wiener, M., Petek, E., Schaflinger, E., et al. (2019). Genetic study of Khyber-Pukhtunkhwa resident Pakistani families presenting primary microcephaly with intellectual disability. J. Pak. Med. Assoc. 69, 1–1816. doi: 10.5455/JPMA.300681
Alam, M. S., Rahaman, M. M., Sultana, A., Wang, G., and Mollah, M. N. H. (2022). Statistics and network-based approaches to identify molecular mechanisms that drive the progression of breast cancer. Comput. Biol. Med. 145:105508. doi: 10.1016/j.compbiomed.2022.105508
Alam, M. S., Sultana, A., Wang, G., and Haque Mollah, M. N. (2022). Gene expression profile analysis to discover molecular signatures for early diagnosis and therapies of triple-negative breast cancer. Front. Mol. Biosci. 9:1049741. doi: 10.3389/fmolb.2022.1049741
Alsiary, R., Brüning-Richardson, A., Bond, J., Morrison, E. E., Wilkinson, N., and Bell, S. M. (2014). Deregulation of Microcephalin and ASPM Expression Are Correlated with Epithelial Ovarian Cancer Progression. PLoS One 9:e97059. doi: 10.1371/journal.pone.0097059
Ariani, F., Mari, F., Amitrano, S., di Marco, C., Artuso, R., Scala, E., et al. (2013). Exome sequencing overrides formal genetics: ASPM mutations in a case study of apparent X-linked microcephalic intellectual deficit. Clin. Genet. 83, 288–290. doi: 10.1111/j.1399-0004.2012.01901.x
Barazzuol, L., Ju, L., and Jeggo, P. A. (2017). A coordinated DNA damage response promotes adult quiescent neural stem cell activation. PLoS Biol. 15:e2001264. doi: 10.1371/journal.pbio.2001264
Batool, T., Irshad, S., and Mahmood, K. (2023). Novel pathogenic mutation mapping of ASPM gene in consanguineous Pakistani families with primary microcephaly. Braz. J. Biol. 83:e246040. doi: 10.1590/1519-6984.246040
Bazgir, A., Agha Gholizadeh, M., Sarvar, F., and Pakzad, Z. (2019). A novel frameshift mutation in abnormal spindle-like microcephaly (ASPM) gene in an Iranian patient with primary microcephaly: a case report. Iran. J. Public Health 48, 2074–2078. doi: 10.18502/ijph.v48i11.3528
Bennett, E. J., Rush, J., Gygi, S. P., and Harper, J. W. (2010). Dynamics of cullin-RING ubiquitin ligase network revealed by systematic quantitative proteomics. Cells 143, 951–965. doi: 10.1016/j.cell.2010.11.017
Bhargav, D. S., Sreedevi, N., Swapna, N., Vivek, S., and Kovvali, S. (2017). Whole exome sequencing identifies a novel homozygous frameshift mutation in the ASPM gene, which causes microcephaly 5, primary, autosomal recessive. F1000Res. 6:2163. doi: 10.12688/f1000research.12102.1
Bicknell, L. S., Bongers, E. M. H. F., Leitch, A., Brown, S., Schoots, J., Harley, M. E., et al. (2011). Mutations in the pre-replication complex cause Meier-Gorlin syndrome. Nat. Genet. 43, 356–359. doi: 10.1038/ng.775
Bolat, H., Sağer, S. G., Türkyılmaz, A., Çebi, A. H., Akın, Y., Onay, H., et al. (2022). Autosomal recessive primary microcephaly (MCPH) and novel pathogenic variants in ASPM and WDR62 genes. Mol. Syndromol. 13, 363–369. doi: 10.1159/000524391
Bond, J., Roberts, E., Mochida, G. H., Hampshire, D. J., Scott, S., Askham, J. M., et al. (2002). ASPM is a major determinant of cerebral cortical size. Nat. Genet. 32, 316–320. doi: 10.1038/ng995
Bond, J., Scott, S., Hampshire, D. J., Springell, K., Corry, P., Abramowicz, M. J., et al. (2003). Protein-truncating mutations in ASPM cause variable reduction in brain size. Am. J. Hum. Genet. 73, 1170–1177. doi: 10.1086/379085
Bond, J., and Woods, C. G. (2006). Cytoskeletal genes regulating brain size. Curr. Opin. Cell Biol. 18, 95–101. doi: 10.1016/j.ceb.2005.11.004
Bonilla, X., Parmentier, L., King, B., Bezrukov, F., Kaya, G., Zoete, V., et al. (2016). Genomic analysis identifies new drivers and progression pathways in skin basal cell carcinoma. Nat. Genet. 48, 398–406. doi: 10.1038/ng.3525
Borrell, V., and Calegari, F. (2014). Mechanisms of brain evolution: regulation of neural progenitor cell diversity and cell cycle length. Neurosci. Res. 86, 14–24. doi: 10.1016/j.neures.2014.04.004
Brüning-Richardson, A., Bond, J., Alsiary, R., Richardson, J., Cairns, D. A., McCormack, L., et al. (2011). ASPM and microcephalin expression in epithelial ovarian cancer correlates with tumour grade and survival. Br. J. Cancer 104, 1602–1610. doi: 10.1038/bjc.2011.117
Buchman, J. J., Durak, O., and Tsai, L. H. (2011). ASPM regulates Wnt signaling pathway activity in the developing brain. Genes Dev. 25, 1909–1914. doi: 10.1101/gad.16830211
Cancer Genome Atlas, N. (2012). Comprehensive molecular characterization of human colon and rectal cancer. Nature 487, 330–337. doi: 10.1038/nature11252
Capecchi, M. R., and Pozner, A. (2015). ASPM regulates symmetric stem cell division by tuning cyclin E ubiquitination. Nat. Commun. 6:8763. doi: 10.1038/ncomms9763
Chen, Q., Hu, J., Deng, J., Fu, B., and Guo, J. (2019). Bioinformatics analysis identified key molecular changes in bladder Cancer development and recurrence. Biomed. Res. Int. 2019:3917982. doi: 10.1155/2019/3917982
Chen, X., Huang, L., Yang, Y., Chen, S., Sun, J., Ma, C., et al. (2020). ASPM promotes glioblastoma growth by regulating G1 restriction point progression and Wnt-beta-catenin signaling. Aging (Albany NY) 12, 224–241. doi: 10.18632/aging.102612
Chen, K., Xing, J., Yu, W., Xia, Y., Zhang, Y., Cheng, F., et al. (2021). Identification and validation of hub genes associated with bladder Cancer by integrated bioinformatics and experimental assays. Front. Oncol. 11:782981. doi: 10.3389/fonc.2021.782981
Chen, K., Yang, D., Li, X., Sun, B., Song, F., Cao, W., et al. (2015). Mutational landscape of gastric adenocarcinoma in Chinese: implications for prognosis and therapy. Proc. Natl. Acad. Sci. U. S. A. 112, 1107–1112. doi: 10.1073/pnas.1422640112
Cheng, C., Cui, H., Zhang, L., Jia, Z., Song, B., Wang, F., et al. (2016). Genomic analyses reveal FAM84B and the NOTCH pathway are associated with the progression of esophageal squamous cell carcinoma. Gigascience 5:1. doi: 10.1186/s13742-015-0107-0
Choi, E. J., Kim, M. S., Yoo, N. J., and Lee, S. H. (2016). Frameshift mutation of ASPM gene in colorectal cancers with regional heterogeneity. Pathol. Oncol. Res. 22, 877–879. doi: 10.1007/s12253-016-0108-z
Correia-Costa, G. R., dos Santos, A. M., de Leeuw, N., Rigatto, S. Z. P., Belangero, V. M. S., Steiner, C. E., et al. (2022). Dual molecular diagnoses of recessive disorders in a child from consanguineous parents. Genes 13, 2377. doi: 10.3390/genes13122377
Darvish, H., Esmaeeli-Nieh, S., Monajemi, G. B., Mohseni, M., Ghasemi-Firouzabadi, S., Abedini, S. S., et al. (2010). A clinical and molecular genetic study of 112 Iranian families with primary microcephaly. J. Med. Genet. 47, 823–828. doi: 10.1136/jmg.2009.076398
de Almeida, M. M. A., Goodkey, K., and Voronova, A. (2023). Regulation of microglia function by neural stem cells. Front. Cell. Neurosci. 17:1130205. doi: 10.3389/fncel.2023.1130205
de Munnik, S. A., Bicknell, L. S., Aftimos, S., al-Aama, J. Y., van Bever, Y., Bober, M. B., et al. (2012). Meier-Gorlin syndrome genotype-phenotype studies: 35 individuals with pre-replication complex gene mutations and 10 without molecular diagnosis. Eur. J. Hum. Genet. 20, 598–606. doi: 10.1038/ejhg.2011.269
do Carmo Avides, M., and Glover, D. M. (1999). Abnormal spindle protein, asp, and the integrity of mitotic centrosomal microtubule organizing centers. Science 283, 1733–1735. doi: 10.1126/science.283.5408.1733
Dong, L. Q., Shi, Y., Ma, L. J., Yang, L. X., Wang, X. Y., Zhang, S., et al. (2018). Spatial and temporal clonal evolution of intrahepatic cholangiocarcinoma. J. Hepatol. 69, 89–98. doi: 10.1016/j.jhep.2018.02.029
Duerinckx, S., Jacquemin, V., Drunat, S., Vial, Y., Passemard, S., Perazzolo, C., et al. (2020). Digenic inheritance of human primary microcephaly delineates centrosomal and non-centrosomal pathways. Hum. Mutat. 41, 512–524. doi: 10.1002/humu.23948
Fang, Q., Li, Q., Qi, Y., Pan, Z., Feng, T., and Xin, W. (2023). ASPM promotes migration and invasion of anaplastic thyroid carcinoma by stabilizing KIF11. Cell Biol. Int. 47, 1209–1221. doi: 10.1002/cbin.12012
Fasci, D., van Ingen, H., Scheltema, R. A., and Heck, A. J. R. (2018). Histone interaction landscapes visualized by crosslinking mass spectrometry in intact cell nuclei. Mol. Cell. Proteomics 17, 2018–2033. doi: 10.1074/mcp.RA118.000924
Feng, Z., Zhang, J., Zheng, Y., Liu, J., Duan, T., and Tian, T. (2021). Overexpression of abnormal spindle-like microcephaly-associated (ASPM) increases tumor aggressiveness and predicts poor outcome in patients with lung adenocarcinoma. Transl. Cancer Res. 10, 983–997. doi: 10.21037/tcr-20-2570
Fernandez, V., Llinares-Benadero, C., and Borrell, V. (2016). Cerebral cortex expansion and folding: what have we learned? EMBO J. 35, 1021–1044. doi: 10.15252/embj.201593701
Fietz, S. A., Kelava, I., Vogt, J., Wilsch-Bräuninger, M., Stenzel, D., Fish, J. L., et al. (2010). OSVZ progenitors of human and ferret neocortex are epithelial-like and expand by integrin signaling. Nat. Neurosci. 13, 690–699. doi: 10.1038/nn.2553
Fish, J. L., Dehay, C., Kennedy, H., and Huttner, W. B. (2008). Making bigger brains-the evolution of neural-progenitor-cell division. J. Cell Sci. 121, 2783–2793. doi: 10.1242/jcs.023465
Fish, J. L., Kosodo, Y., Enard, W., Pääbo, S., and Huttner, W. B. (2006). Aspm specifically maintains symmetric proliferative divisions of neuroepithelial cells. Proc. Natl. Acad. Sci. U. S. A. 103, 10438–10443. doi: 10.1073/pnas.0604066103
Friedel, R. H., and Soriano, P. (2010). Gene trap mutagenesis in the mouse. Methods Enzymol. 477, 243–269. doi: 10.1016/S0076-6879(10)77013-0
Fujimori, A., Itoh, K., Goto, S., Hirakawa, H., Wang, B., Kokubo, T., et al. (2014). Disruption of Aspm causes microcephaly with abnormal neuronal differentiation. Brain Dev. 36, 661–669. doi: 10.1016/j.braindev.2013.10.006
Fujimori, A., Yaoi, T., Ogi, H., Wang, B., Suetomi, K., Sekine, E., et al. (2008). Ionizing radiation downregulates ASPM, a gene responsible for microcephaly in humans. Biochem. Biophys. Res. Commun. 369, 953–957. doi: 10.1016/j.bbrc.2008.02.149
Gai, M., Bianchi, F. T., Vagnoni, C., Vernì, F., Bonaccorsi, S., Pasquero, S., et al. (2016). ASPM and CITK regulate spindle orientation by affecting the dynamics of astral microtubules. EMBO Rep. 17, 1396–1409. doi: 10.15252/embr.201541823
Galligan, J. T., Martinez-Noël, G., Arndt, V., Hayes, S., Chittenden, T. W., Harper, J. W., et al. (2015). Proteomic analysis and identification of cellular interactors of the giant ubiquitin ligase HERC2. J. Proteome Res. 14, 953–966. doi: 10.1021/pr501005v
Gao, Y. B., Chen, Z. L., Li, J. G., Hu, X. D., Shi, X. J., Sun, Z. M., et al. (2014). Genetic landscape of esophageal squamous cell carcinoma. Nat. Genet. 46, 1097–1102. doi: 10.1038/ng.3076
Gao, Z. Y., Yu, F., Jia, H. X., Ye, Z., and Yao, S. J. (2020). ASPM predicts poor prognosis and regulates cell proliferation in bladder cancer. Kaohsiung J. Med. Sci. 36, 1021–1029. doi: 10.1002/kjm2.12284
George, J., Lim, J. S., Jang, S. J., Cun, Y., Ozretić, L., Kong, G., et al. (2015). Comprehensive genomic profiles of small cell lung cancer. Nature 524, 47–53. doi: 10.1038/nature14664
Giannakis, M., Hodis, E., Jasmine Mu, X., Yamauchi, M., Rosenbluh, J., Cibulskis, K., et al. (2014). RNF43 is frequently mutated in colorectal and endometrial cancers. Nat. Genet. 46, 1264–1266. doi: 10.1038/ng.3127
Giannakis, M., Mu, X. J., Shukla, S. A., Qian, Z. R., Cohen, O., Nishihara, R., et al. (2016). Genomic correlates of immune-cell infiltrates in colorectal carcinoma. Cell Rep. 15, 857–865. doi: 10.1016/j.celrep.2016.03.075
Gingras, M. C., Covington, K. R., Chang, D. K., Donehower, L. A., Gill, A. J., Ittmann, M. M., et al. (2016). Ampullary Cancers Harbor ELF3 tumor suppressor gene mutations and exhibit frequent WNT dysregulation. Cell Rep. 14, 907–919. doi: 10.1016/j.celrep.2015.12.005
Gomez-Lopez, S., Lerner, R. G., and Petritsch, C. (2014). Asymmetric cell division of stem and progenitor cells during homeostasis and cancer. Cell. Mol. Life Sci. 71, 575–597. doi: 10.1007/s00018-013-1386-1
Gonzalez, C., Casal, J., and Ripoll, P. (1988). Functional monopolar spindles caused by mutation in mgr, a cell division gene of Drosophila melanogaster. J. Cell Sci. 89, 39–47. doi: 10.1242/jcs.89.1.39
Gonzalez, C., Saunders, R. D. C., Casal, J., Molina, I., Carmena, M., Ripoll, P., et al. (1990). Mutations at the asp locus of Drosophila Lead to multiple free centrosomes in syncytial embryos, but restrict centrosome duplication in larval neuroblasts. J. Cell Sci. 96, 605–616. doi: 10.1242/jcs.96.4.605
Gonzalez, C., Sunkel, C. E., and Glover, D. M. (1998). Interactions between mgr, asp, and polo: asp function modulated by polo and needed to maintain the poles of monopolar and bipolar spindles. Chromosoma 107, 452–460. doi: 10.1007/s004120050329
González-Martínez, J., Cwetsch, A. W., Martínez-Alonso, D., López-Sainz, L. R., Almagro, J., Melati, A., et al. (2021). Deficient adaptation to centrosome duplication defects in neural progenitors causes microcephaly and subcortical heterotopias. JCI. Insight 6, e146364. doi: 10.1172/jci.insight.146364
Goshima, G., Wollman, R., Goodwin, S. S., Zhang, N., Scholey, J. M., Vale, R. D., et al. (2007). Genes required for mitotic spindle assembly in Drosophila S2 cells. Science 316, 417–421. doi: 10.1126/science.1141314
Gul, A., Hassan, M. J., Mahmood, S., Chen, W., Rahmani, S., Naseer, M. I., et al. (2006). Genetic studies of autosomal recessive primary microcephaly in 33 Pakistani families: novel sequence variants in ASPM gene. Neurogenetics 7, 105–110. doi: 10.1007/s10048-006-0042-4
Gul, A., Tariq, M., Khan, M. N., Hassan, M. J., Ali, G., and Ahmad, W. (2007). Novel protein-truncating mutations in the ASPM gene in families with autosomal recessive primary microcephaly. J. Neurogenet. 21, 153–163. doi: 10.1080/01677060701508594
Gupta, N. (2023). Deciphering intellectual disability. Indian J. Pediatr. 90, 160–167. doi: 10.1007/s12098-022-04345-3
Halsall, S., Nicholas, A. K., Thornton, G., Martin, H., and Geoffrey Woods, C. (2010). Critical consequences of finding three pathogenic mutations in an individual with recessive disease. J. Med. Genet. 47, 769–770. doi: 10.1136/jmg.2010.079277
Hasan, M. A. M., Maniruzzaman, M., and Shin, J. (2023). Differentially expressed discriminative genes and significant meta-hub genes based key genes identification for hepatocellular carcinoma using statistical machine learning. Sci. Rep. 13:3771. doi: 10.1038/s41598-023-30851-1
Hashmi, J. A., al-Harbi, K. M., Ramzan, K., Albalawi, A. M., Mehmood, A., Samman, M. I., et al. (2016). A novel splice-site mutation in the ASPM gene underlies autosomal recessive primary microcephaly. Ann. Saudi Med. 36, 391–396. doi: 10.5144/0256-4947.2016.391
Hayward, N. K., Wilmott, J. S., Waddell, N., Johansson, P. A., Field, M. A., Nones, K., et al. (2017). Whole-genome landscapes of major melanoma subtypes. Nature 545, 175–180. doi: 10.1038/nature22071
Hedberg, M. L., Goh, G., Chiosea, S. I., Bauman, J. E., Freilino, M. L., Zeng, Y., et al. (2016). Genetic landscape of metastatic and recurrent head and neck squamous cell carcinoma. J. Clin. Invest. 126:1606. doi: 10.1172/JCI86862
Heidelberger, J. B., Voigt, A., Borisova, M. E., Petrosino, G., Ruf, S., Wagner, S. A., et al. (2018). Proteomic profiling of VCP substrates links VCP to K6-linked ubiquitylation and c-Myc function. EMBO Rep. 19, e44754. doi: 10.15252/embr.201744754
Hein, M. Y., Hubner, N. C., Poser, I., Cox, J., Nagaraj, N., Toyoda, Y., et al. (2015). A human interactome in three quantitative dimensions organized by stoichiometries and abundances. Cells 163, 712–723. doi: 10.1016/j.cell.2015.09.053
Herculano-Houzel, S. (2012). The remarkable, yet not extraordinary, human brain as a scaled-up primate brain and its associated cost. Proc. Natl. Acad. Sci. U. S. A. 109, 10661–10668. doi: 10.1073/pnas.1201895109
Higgins, J., Midgley, C., Bergh, A. M., Bell, S. M., Askham, J. M., Roberts, E., et al. (2010). Human ASPM participates in spindle organisation, spindle orientation and cytokinesis. BMC Cell Biol. 11:85. doi: 10.1186/1471-2121-11-85
Hintzsche, J. D., Gorden, N. T., Amato, C. M., Kim, J., Wuensch, K. E., Robinson, S. E., et al. (2017). Whole-exome sequencing identifies recurrent SF3B1 R625 mutation and comutation of NF1 and KIT in mucosal melanoma. Melanoma Res. 27, 189–199. doi: 10.1097/CMR.0000000000000345
Horvath, S., Zhang, B., Carlson, M., Lu, K. V., Zhu, S., Felciano, R. M., et al. (2006). Analysis of oncogenic signaling networks in glioblastoma identifies ASPM as a molecular target. Proc. Natl. Acad. Sci. U. S. A. 103, 17402–17407. doi: 10.1073/pnas.0608396103
Hossen, M. A., Reza, M. S., Harun-Or-Roshid, M., Islam, M. A., Siddika, M. A., and MNH, M. (2023). Identification of drug targets and agents associated with hepatocellular carcinoma through integrated bioinformatics analysis. Curr. Cancer Drug Targets 23, 547–563. doi: 10.2174/1568009623666230214100159
Hou, S., Xu, H., Liu, S., Yang, B., Li, L., Zhao, H., et al. (2022). Integrated bioinformatics analysis identifies a new Stemness index-related survival model for prognostic prediction in lung adenocarcinoma. Front. Genet. 13:860268. doi: 10.3389/fgene.2022.860268
Hu, H., Suckow, V., Musante, L., Roggenkamp, V., Kraemer, N., Ropers, H. H., et al. (2014). Previously reported new type of autosomal recessive primary microcephaly is caused by compound heterozygous ASPM gene mutations. Cell Cycle 13, 1650–1651. doi: 10.4161/cc.28706
Hu, X., Zhou, J., Zhang, Y., Zeng, Y., Jie, G., Wang, S., et al. (2022). Identifying potential prognosis markers in hepatocellular carcinoma via integrated bioinformatics analysis and biological experiments. Front. Genet. 13:942454. doi: 10.3389/fgene.2022.942454
Hussain, S., Nawaz, A., Hamid, M., Ullah, W., Khan, I. N., Afshan, M., et al. (2022). Mutation screening of multiple Pakistani MCPH families revealed novel and recurrent protein-truncating mutations of ASPM. Biotechnol. Appl. Biochem. 69, 2296–2303. doi: 10.1002/bab.2286
Hutchins, J. R., Toyoda, Y., Hegemann, B., Poser, I., Hériché, J. K., Sykora, M. M., et al. (2010). Systematic analysis of human protein complexes identifies chromosome segregation proteins. Science 328, 593–599. doi: 10.1126/science.1181348
Huttlin, E. L., Bruckner, R. J., Navarrete-Perea, J., Cannon, J. R., Baltier, K., Gebreab, F., et al. (2021). Dual proteome-scale networks reveal cell-specific remodeling of the human interactome. Cells 184, 3022–3040.e28. doi: 10.1016/j.cell.2021.04.011
Huttlin, E. L., Bruckner, R. J., Paulo, J. A., Cannon, J. R., Ting, L., Baltier, K., et al. (2017). Architecture of the human interactome defines protein communities and disease networks. Nature 545, 505–509. doi: 10.1038/nature22366
Isaev, N. K., Stelmashook, E. V., and Genrikhs, E. E. (2019). Neurogenesis and brain aging. Rev. Neurosci. 30, 573–580. doi: 10.1515/revneuro-2018-0084
Jackson, A. P., Laskey, R. A., and Coleman, N. (2014). Replication proteins and human disease. Cold Spring Harb. Perspect. Biol. 6:a013060. doi: 10.1101/cshperspect.a013060
Jamieson, C. R., Fryns, J. P., Jacobs, J., Matthijs, G., and Abramowicz, M. J. (2000). Primary autosomal recessive microcephaly: MCPH5 maps to 1q25-q32. Am. J. Hum. Genet. 67, 1575–1577. doi: 10.1086/316909
Jana, S. C., Bettencourt-Dias, M., Durand, B., and Megraw, T. L. (2016). Drosophila melanogaster as a model for basal body research. Cilia 5:22. doi: 10.1186/s13630-016-0041-5
Jayaraman, D., Bae, B. I., and Walsh, C. A. (2018). The genetics of primary microcephaly. Annu. Rev. Genomics Hum. Genet. 19, 177–200. doi: 10.1146/annurev-genom-083117-021441
Jayaraman, D., Kodani, A., Gonzalez, D. M., Mancias, J. D., Mochida, G. H., Vagnoni, C., et al. (2016). Microcephaly proteins Wdr62 and Aspm define a mother centriole complex regulating centriole biogenesis, apical complex, and cell fate. Neuron 92, 813–828. doi: 10.1016/j.neuron.2016.09.056
Jiang, K., Rezabkova, L., Hua, S., Liu, Q., Capitani, G., Altelaar, A. F. M., et al. (2017). Microtubule minus-end regulation at spindle poles by an ASPM-katanin complex. Nat. Cell Biol. 19, 480–492. doi: 10.1038/ncb3511
Jiang, L., Zhang, S., An, N., Chai, G., and Ye, C. (2022). ASPM promotes the progression of anaplastic thyroid carcinomas by regulating the Wnt/beta-catenin signaling pathway. Int. J. Endocrinol. 2022:5316102. doi: 10.1155/2022/5316102
Johnson, M. B., Sun, X., Kodani, A., Borges-Monroy, R., Girskis, K. M., Ryu, S. C., et al. (2018). Aspm knockout ferret reveals an evolutionary mechanism governing cerebral cortical size. Nature 556, 370–375. doi: 10.1038/s41586-018-0035-0
Jones, S., Wang, T. L., Kurman, R. J., Nakayama, K., Velculescu, V. E., Vogelstein, B., et al. (2012). Low-grade serous carcinomas of the ovary contain very few point mutations. J. Pathol. 226, 413–420. doi: 10.1002/path.3967
Kato, T. A., Okayasu, R., Jeggo, P. A., and Fujimori, A. (2011). ASPM influences DNA double-strand break repair and represents a potential target for radiotherapy. Int. J. Radiat. Biol. 87, 1189–1195. doi: 10.3109/09553002.2011.624152
Khan, A., Wang, R., Han, S., Ahmad, W., and Zhang, X. (2018). Identification of a novel nonsense ASPM mutation in a large consanguineous Pakistani family using targeted next-generation sequencing. Genet. Test. Mol. Biomark. 22, 159–164. doi: 10.1089/gtmb.2017.0229
Khan, M. A., Windpassinger, C., Ali, M. Z., Zubair, M., Gul, H., Abbas, S., et al. (2017). Molecular genetic analysis of consanguineous families with primary microcephaly identified pathogenic variants in the ASPM gene. J. Genet. 96, 383–387. doi: 10.1007/s12041-017-0759-x
Kim, H. T., Lee, M. S., Choi, J. H., Jung, J. Y., Ahn, D. G., Yeo, S. Y., et al. (2011). The microcephaly gene aspm is involved in brain development in zebrafish. Biochem. Biophys. Res. Commun. 409, 640–644. doi: 10.1016/j.bbrc.2011.05.056
Knoblich, J. A. (2008). Mechanisms of asymmetric stem cell division. Cells 132, 583–597. doi: 10.1016/j.cell.2008.02.007
Kouprina, N., Pavlicek, A., Collins, N. K., Nakano, M., Noskov, V. N., Ohzeki, J. I., et al. (2005). The microcephaly ASPM gene is expressed in proliferating tissues and encodes for a mitotic spindle protein. Hum. Mol. Genet. 14, 2155–2165. doi: 10.1093/hmg/ddi220
Kouranti, I., Abdel Khalek, W., Mazurkiewicz, S., Loisel-Ferreira, I., Gautreau, A. M., Pintard, L., et al. (2022). Cullin 3 exon 9 deletion in familial hyperkalemic hypertension impairs Cullin3-ring-E3 ligase (CRL3) dynamic regulation and cycling. Int. J. Mol. Sci. 23:5151. doi: 10.3390/ijms23095151
Kousar, R., Nawaz, H., Khurshid, M., Ali, G., Khan, S. U., Mir, H., et al. (2010). Mutation analysis of the ASPM gene in 18 Pakistani families with autosomal recessive primary microcephaly. J. Child Neurol. 25, 715–720. doi: 10.1177/0883073809346850
Kraemer, N., Picker-Minh, S., Abbasi, A. A., Fröhler, S., Ninnemann, O., Khan, M. N., et al. (2016). Genetic causes of MCPH in consanguineous Pakistani families. Clin. Genet. 89, 744–745. doi: 10.1111/cge.12685
Krauthammer, M., Kong, Y., Bacchiocchi, A., Evans, P., Pornputtapong, N., Wu, C., et al. (2015). Exome sequencing identifies recurrent mutations in NF1 and RASopathy genes in sun-exposed melanomas. Nat. Genet. 47, 996–1002. doi: 10.1038/ng.3361
Krauthammer, M., Kong, Y., Ha, B. H., Evans, P., Bacchiocchi, A., McCusker, J. P., et al. (2012). Exome sequencing identifies recurrent somatic RAC1 mutations in melanoma. Nat. Genet. 44, 1006–1014. doi: 10.1038/ng.2359
Kumar, A., Blanton, S. H., Babu, M., Markandaya, M., and Girimaji, S. C. (2004). Genetic analysis of primary microcephaly in Indian families: novel ASPM mutations. Clin. Genet. 66, 341–348. doi: 10.1111/j.1399-0004.2004.00304.x
Kumar, A., Coleman, I., Morrissey, C., Zhang, X., True, L. D., Gulati, R., et al. (2016). Substantial interindividual and limited intraindividual genomic diversity among tumors from men with metastatic prostate cancer. Nat. Med. 22, 369–378. doi: 10.1038/nm.4053
Lau, W. M., Teng, E., Huang, K. K., Tan, J. W., das, K., Zang, Z., et al. (2018). Acquired resistance to FGFR inhibitor in diffuse-type gastric Cancer through an AKT-independent PKC-mediated phosphorylation of GSK3beta. Mol. Cancer Ther. 17, 232–242. doi: 10.1158/1535-7163.MCT-17-0367
Létard, P., Drunat, S., Vial, Y., Duerinckx, S., Ernault, A., Amram, D., et al. (2018). Autosomal recessive primary microcephaly due to ASPM mutations: an update. Hum. Mutat. 39, 319–332. doi: 10.1002/humu.23381
Li, B., Brady, S. W., Ma, X., Shen, S., Zhang, Y., Li, Y., et al. (2020). Therapy-induced mutations drive the genomic landscape of relapsed acute lymphoblastic leukemia. Blood 135, 41–55. doi: 10.1182/blood.2019002220
Li, Y. Y., Chung, G. T. Y., Lui, V. W. Y., To, K. F., Ma, B. B. Y., Chow, C., et al. (2017). Exome and genome sequencing of nasopharynx cancer identifies NF-kappaB pathway activating mutations. Nat. Commun. 8:14121. doi: 10.1038/ncomms14121
Li, Y., Li, J., He, T., Song, Y., Wu, J., and Wang, B. (2022). Significance of identifying key genes involved in HBV-related hepatocellular carcinoma for primary care surveillance of patients with cirrhosis. Genes (Basel) 13:2331. doi: 10.3390/genes13122331
Li, D., Li, X., Li, S., Qi, M., Sun, X., and Hu, G. (2023). Relationship between the deep features of the full-scan pathological map of mucinous gastric carcinoma and related genes based on deep learning. Heliyon 9:e14374. doi: 10.1016/j.heliyon.2023.e14374
Li, M., Luo, J., Yang, Q., Chen, F., Chen, J., Qin, J., et al. (2022). Novel and recurrent ASPM mutations of founder effect in Chinese population. Brain Dev. 44, 540–545. doi: 10.1016/j.braindev.2022.04.007
Li, R., Sun, L., Fang, A., Li, P., Wu, Q., and Wang, X. (2017). Recapitulating cortical development with organoid culture in vitro and modeling abnormal spindle-like (ASPM related primary) microcephaly disease. Protein Cell 8, 823–833. doi: 10.1007/s13238-017-0479-2
Li, C., and Xu, J. (2020). Identification of potentially therapeutic target genes of hepatocellular carcinoma. Int. J. Environ. Res. Public Health 17:1053. doi: 10.3390/ijerph17031053
Liaci, C., Camera, M., Caslini, G., Rando, S., Contino, S., Romano, V., et al. (2021). Neuronal cytoskeleton in intellectual disability: from systems biology and modeling to therapeutic opportunities. Int. J. Mol. Sci. 22, 6167. doi: 10.3390/ijms22116167
Lin, S. Y., Pan, H. W., Liu, S. H., Jeng, Y. M., Hu, F. C., Peng, S. Y., et al. (2008). ASPM is a novel marker for vascular invasion, early recurrence, and poor prognosis of hepatocellular carcinoma. Clin. Cancer Res. 14, 4814–4820. doi: 10.1158/1078-0432.CCR-07-5262
Liu, J., Feng, M., Li, S. Y., Nie, S., Wang, H., Wu, S., et al. (2020). Identification of molecular markers associated with the progression and prognosis of endometrial cancer: a bioinformatic study. Cancer Cell Int. 20:59. doi: 10.1186/s12935-020-1140-3
Liu, J., Guan, D., Dong, M., Yang, J., Wei, H., Liang, Q., et al. (2020). UFMylation maintains tumour suppressor p53 stability by antagonizing its ubiquitination. Nat. Cell Biol. 22, 1056–1063. doi: 10.1038/s41556-020-0559-z
Liu, J., McCleland, M., Stawiski, E. W., Gnad, F., Mayba, O., Haverty, P. M., et al. (2014). Integrated exome and transcriptome sequencing reveals ZAK isoform usage in gastric cancer. Nat. Commun. 5:3830. doi: 10.1038/ncomms4830
Liu, B., Su, J., Fan, B., Ni, X., and Jin, T. (2023). High expression of KIF20A in bladder cancer as a potential prognostic target for poor survival of renal cell carcinoma. Medicine (Baltimore) 102:e32667. doi: 10.1097/MD.0000000000032667
Liu, Z., Yang, C., Li, X., Luo, W., Roy, B., Xiong, T., et al. (2018). The landscape of somatic mutation in sporadic Chinese colorectal cancer. Oncotarget 9, 27412–27422. doi: 10.18632/oncotarget.25287
Liu, H., Zhou, Q., Xu, X., du, Y., and Wu, J. (2022). ASPM and TROAP gene expression as potential malignant tumor markers. Ann. Transl. Med. 10:586. doi: 10.21037/atm-22-1112
Liu, D., Zhou, R., and Zhou, A. (2021). Identification of key biomarkers and functional pathways in osteosarcomas with lung metastasis: evidence from bioinformatics analysis. Medicine (Baltimore) 100:e24471. doi: 10.1097/MD.0000000000024471
Mahmood, S., Ahmad, W., and Hassan, M. J. (2011). Autosomal recessive primary microcephaly (MCPH): clinical manifestations, genetic heterogeneity and mutation continuum. Orphanet J. Rare Dis. 6:39. doi: 10.1186/1750-1172-6-39
Makhdoom, E. U. H., Anwar, H., Baig, S. M., and Hussain, G. (2022). Whole exome sequencing identifies a novel mutation in ASPM and ultra-rare mutation in CDK5RAP2 causing primary microcephaly in consanguineous Pakistani families. Pak. J. Med. Sci. 38, 84–89. doi: 10.12669/pjms.38.1.4464
Makhdoom, E. U. H., Waseem, S. S., Iqbal, M., Abdullah, U., Hussain, G., Asif, M., et al. (2021). Modifier genes in microcephaly: a report on WDR62, CEP63, RAD50 and PCNT variants exacerbating disease caused by Biallelic mutations of ASPM and CENPJ. Genes (Basel) 12, 731. doi: 10.3390/genes12050731
Marakhonov, A. V., Konovalov, F. A., Makaov, A. K., Vasilyeva, T. A., Kadyshev, V. V., Galkina, V. A., et al. (2018). Primary microcephaly case from the Karachay-Cherkess Republic poses an additional support for microcephaly and Seckel syndrome spectrum disorders. BMC Med. Genet. 11:8. doi: 10.1186/s12920-018-0326-1
Marinaro, C., Butti, E., Bergamaschi, A., Papale, A., Furlan, R., Comi, G., et al. (2011). In vivo fate analysis reveals the multipotent and self-renewal features of embryonic AspM expressing cells. PLoS One 6:e19419. doi: 10.1371/journal.pone.0019419
Martins, S., Erichsen, L., Datsi, A., Wruck, W., Goering, W., Chatzantonaki, E., et al. (2022). Impaired p53-mediated DNA damage response contributes to microcephaly in Nijmegen breakage syndrome patient-derived cerebral organoids. Cells 11:802. doi: 10.3390/cells11050802
McMillan, E. A., Ryu, M. J., Diep, C. H., Mendiratta, S., Clemenceau, J. R., Vaden, R. M., et al. (2018). Chemistry-first approach for nomination of personalized treatment in lung Cancer. Cells 173, 864–878.e29. doi: 10.1016/j.cell.2018.03.028
McSherry, M., Masih, K. E., Elcioglu, N. H., Celik, P., Balci, O., Cengiz, F. B., et al. (2018). Identification of candidate gene FAM183A and novel pathogenic variants in known genes: high genetic heterogeneity for autosomal recessive intellectual disability. PLoS One 13:e0208324. doi: 10.1371/journal.pone.0208324
Ming, G. L., and Song, H. (2005). Adult neurogenesis in the mammalian central nervous system. Annu. Rev. Neurosci. 28, 223–250. doi: 10.1146/annurev.neuro.28.051804.101459
Ming, G. L., and Song, H. (2011). Adult neurogenesis in the mammalian brain: significant answers and significant questions. Neuron 70, 687–702. doi: 10.1016/j.neuron.2011.05.001
Misson, J. P., Edwards, M. A., Yamamoto, M., and Caviness, V. S. Jr. (1988). Mitotic cycling of radial glial cells of the fetal murine cerebral wall: a combined autoradiographic and immunohistochemical study. Brain Res. 38, 183–190. doi: 10.1016/0165-3806(88)90043-0
Morales-Mulia, S., and Scholey, J. M. (2005). Spindle pole organization in Drosophila S2 cells by dynein, abnormal spindle protein (Asp), and KLP10A. Mol. Biol. Cell 16, 3176–3186. doi: 10.1091/mbc.e04-12-1110
Mori, M., Tando, S., Ogi, H., Tonosaki, M., Yaoi, T., Fujimori, A., et al. (2022). Loss of abnormal spindle-like, microcephaly-associated (Aspm) disrupts female folliculogenesis in mice during maturation and aging. Reprod. Biol. 22:100673. doi: 10.1016/j.repbio.2022.100673
Morin, R. D., Assouline, S., Alcaide, M., Mohajeri, A., Johnston, R. L., Chong, L., et al. (2016). Genetic landscapes of relapsed and refractory diffuse large B-cell lymphomas. Clin. Cancer Res. 22, 2290–2300. doi: 10.1158/1078-0432.CCR-15-2123
Mouradov, D., Sloggett, C., Jorissen, R. N., Love, C. G., Li, S., Burgess, A. W., et al. (2014). Colorectal cancer cell lines are representative models of the main molecular subtypes of primary cancer. Cancer Res. 74, 3238–3247. doi: 10.1158/0008-5472.CAN-14-0013
Muhammad, F., Mahmood Baig, S., Hansen, L., Sajid Hussain, M., Anjum Inayat, I., Aslam, M., et al. (2009). Compound heterozygous ASPM mutations in Pakistani MCPH families. Am. J. Med. Genet. A 149A, 926–930. doi: 10.1002/ajmg.a.32749
Nadarajah, B., Alifragis, P., Wong, R. O., and Parnavelas, J. G. (2003). Neuronal migration in the developing cerebral cortex: observations based on real-time imaging. Cereb. Cortex 13, 607–611. doi: 10.1093/cercor/13.6.607
Nadarajah, B., and Parnavelas, J. G. (2002). Modes of neuronal migration in the developing cerebral cortex. Nat. Rev. Neurosci. 3, 423–432. doi: 10.1038/nrn845
Nakamura, K., Inui, T., Miya, F., Kanemura, Y., Okamoto, N., Saitoh, S., et al. (2015). Primary microcephaly with anterior predominant pachygyria caused by novel compound heterozygous mutations in ASPM. Pediatr. Neurol. 52, e7–e8. doi: 10.1016/j.pediatrneurol.2015.01.019
Naqvi, S. F., Shabbir, R. M. K., Tolun, A., Basit, S., and Malik, S. (2022). A Two-Base pair deletion in IQ repeats in ASPM underlies microcephaly in a Pakistani family. Genet. Test. Mol. Biomark. 26, 37–42. doi: 10.1089/gtmb.2021.0231
Naseer, M. I., Abdulkareem, A. A., Muthaffar, O. Y., Sogaty, S., Alkhatabi, H., Almaghrabi, S., et al. (2020). Whole exome sequencing identifies three novel mutations in the ASPM gene from Saudi families leading to primary microcephaly. Front. Pediatr. 8:627122. doi: 10.3389/fped.2020.627122
Neumuller, R. A., and Knoblich, J. A. (2009). Dividing cellular asymmetry: asymmetric cell division and its implications for stem cells and cancer. Genes Dev. 23, 2675–2699. doi: 10.1101/gad.1850809
Newell, F., Kong, Y., Wilmott, J. S., Johansson, P. A., Ferguson, P. M., Cui, C., et al. (2019). Whole-genome landscape of mucosal melanoma reveals diverse drivers and therapeutic targets. Nat. Commun. 10:3163. doi: 10.1038/s41467-019-11107-x
Nicholas, A. K., Swanson, E. A., Cox, J. J., Karbani, G., Malik, S., Springell, K., et al. (2009). The molecular landscape of ASPM mutations in primary microcephaly. J. Med. Genet. 46, 249–253. doi: 10.1136/jmg.2008.062380
Noctor, S. C., Flint, A. C., Weissman, T. A., Dammerman, R. S., and Kriegstein, A. R. (2001). Neurons derived from radial glial cells establish radial units in neocortex. Nature 409, 714–720. doi: 10.1038/35055553
Nomura, M., Mukasa, A., Nagae, G., Yamamoto, S., Tatsuno, K., Ueda, H., et al. (2017). Distinct molecular profile of diffuse cerebellar gliomas. Acta Neuropathol. 134, 941–956. doi: 10.1007/s00401-017-1771-1
O'Driscoll, M., and Jeggo, P. A. (2008). The role of the DNA damage response pathways in brain development and microcephaly: insight from human disorders. DNA Repair (Amst) 7, 1039–1050. doi: 10.1016/j.dnarep.2008.03.018
Ogi, H., Nitta, N., Tando, S., Fujimori, A., Aoki, I., Fushiki, S., et al. (2018). Longitudinal diffusion tensor imaging revealed nerve Fiber alterations in Aspm mutated microcephaly model mice. Neuroscience 371, 325–336. doi: 10.1016/j.neuroscience.2017.12.012
Okamoto, N., Kohmoto, T., Naruto, T., Masuda, K., and Imoto, I. (2018). Primary microcephaly caused by novel compound heterozygous mutations in ASPM. Hum. Genome Var. 5:18015. doi: 10.1038/hgv.2018.15
Pai, V. C., Hsu, C. C., Chan, T. S., Liao, W. Y., Chuu, C. P., Chen, W. Y., et al. (2019). ASPM promotes prostate cancer stemness and progression by augmenting Wnt-Dvl-3-beta-catenin signaling. Oncogene 38, 1340–1353. doi: 10.1038/s41388-018-0497-4
Paik, J. H., Ding, Z., Narurkar, R., Ramkissoon, S., Muller, F., Kamoun, W. S., et al. (2009). FoxOs cooperatively regulate diverse pathways governing neural stem cell homeostasis. Cell Stem Cell 5, 540–553. doi: 10.1016/j.stem.2009.09.013
Papari, E., Bastami, M., Farhadi, A., Abedini, S. S., Hosseini, M., Bahman, I., et al. (2013). Investigation of primary microcephaly in Bushehr province of Iran: novel STIL and ASPM mutations. Clin. Genet. 83, 488–490. doi: 10.1111/j.1399-0004.2012.01949.x
Paramasivam, M., Chang, Y. J., and LoTurco, J. J. (2007). ASPM and citron kinase co-localize to the midbody ring during cytokinesis. Cell Cycle 6, 1605–1612. doi: 10.4161/cc.6.13.4356
Passemard, S., Titomanlio, L., Elmaleh, M., Afenjar, A., Alessandri, J. L., Andria, G., et al. (2009). Expanding the clinical and neuroradiologic phenotype of primary microcephaly due to ASPM mutations. Neurology 73, 962–969. doi: 10.1212/WNL.0b013e3181b8799a
Passemard, S., Verloes, A., Billette de Villemeur, T., Boespflug-Tanguy, O., Hernandez, K., Laurent, M., et al. (2016). Abnormal spindle-like microcephaly-associated (ASPM) mutations strongly disrupt neocortical structure but spare the hippocampus and long-term memory. Cortex 74, 158–176. doi: 10.1016/j.cortex.2015.10.010
Pattison, L., Crow, Y. J., Deeble, V. J., Jackson, A. P., Jafri, H., Rashid, Y., et al. (2000). A fifth locus for primary autosomal recessive microcephaly maps to chromosome 1q31. Am. J. Hum. Genet. 67, 1578–1580. doi: 10.1086/316910
Peifer, M., Fernández-Cuesta, L., Sos, M. L., George, J., Seidel, D., Kasper, L. H., et al. (2012). Integrative genome analyses identify key somatic driver mutations of small-cell lung cancer. Nat. Genet. 44, 1104–1110. doi: 10.1038/ng.2396
Phan, T. P., and Holland, A. J. (2021). Time is of the essence: the molecular mechanisms of primary microcephaly. Genes Dev. 35, 1551–1578. doi: 10.1101/gad.348866.121
Pulvers, J. N., Bryk, J., Fish, J. L., Wilsch-Bräuninger, M., Arai, Y., Schreier, D., et al. (2010). Mutations in mouse Aspm (abnormal spindle-like microcephaly associated) cause not only microcephaly but also major defects in the germline. Proc. Natl. Acad. Sci. U. S. A. 107, 16595–16600. doi: 10.1073/pnas.1010494107
Qiao, Y., Yuan, F., Wang, X., Hu, J., Mao, Y., and Zhao, Z. (2022). Identification and validation of real hub genes in hepatocellular carcinoma based on weighted gene co-expression network analysis. Cancer Biomark. 35, 227–243. doi: 10.3233/CBM-220151
Qin, S., Yuan, Y., Liu, H., Pu, Y., Chen, K., Wu, Y., et al. (2023). Identification and characterization of sex-dependent gene expression profile in glioblastoma. Neuropathology 43, 72–83. doi: 10.1111/neup.12845
Rabbie, R., Ferguson, P., Wong, K., Couturier, D. L., Moran, U., Turner, C., et al. (2021). The mutational landscape of melanoma brain metastases presenting as the first visceral site of recurrence. Br. J. Cancer 124, 156–160. doi: 10.1038/s41416-020-01090-2
Ramdas Nair, A., Singh, P., Salvador Garcia, D., Rodriguez-Crespo, D., Egger, B., and Cabernard, C. (2016). The microcephaly-associated protein Wdr62/CG7337 is required to maintain centrosome asymmetry in Drosophila neuroblasts. Cell Rep. 14, 1100–1113. doi: 10.1016/j.celrep.2015.12.097
Rasool, S., Baig, J. M., Moawia, A., Ahmad, I., Iqbal, M., Waseem, S. S., et al. (2020). An update of pathogenic variants in ASPM, WDR62, CDK5RAP2, STIL, CENPJ, and CEP135 underlying autosomal recessive primary microcephaly in 32 consanguineous families from Pakistan. Mol. Genet. Genomic Med. 8:e1408. doi: 10.1002/mgg3.1408
Razuvaeva, A. V., Graziadio, L., Palumbo, V., Pavlova, G. A., Popova, J. V., Pindyurin, A. V., et al. (2023). The multiple mitotic roles of the ASPM orthologous proteins: insight into the etiology of ASPM-dependent microcephaly. Cells 12:922. doi: 10.3390/cells12060922
Reynolds, J. J., Bicknell, L. S., Carroll, P., Higgs, M. R., Shaheen, R., Murray, J. E., et al. (2017). Mutations in DONSON disrupt replication fork stability and cause microcephalic dwarfism. Nat. Genet. 49, 537–549. doi: 10.1038/ng.3790
Ripoll, P., Pimpinelli, S., Valdivia, M. M., and Avila, J. (1985). A cell division mutant of Drosophila with a functionally abnormal spindle. Cells 41, 907–912. doi: 10.1016/S0092-8674(85)80071-4
Robinson, B. V., Faundez, V., and Lerit, D. A. (2020). Understanding microcephaly through the study of centrosome regulation in Drosophila neural stem cells. Biochem. Soc. Trans. 48, 2101–2115. doi: 10.1042/BST20200261
Rujano, M. A., Sanchez-Pulido, L., Pennetier, C., le Dez, G., and Basto, R. (2013). The microcephaly protein Asp regulates neuroepithelium morphogenesis by controlling the spatial distribution of myosin II. Nat. Cell Biol. 15, 1294–1306. doi: 10.1038/ncb2858
Saadi, A., Borck, G., Boddaert, N., Chekkour, M. C., Imessaoudene, B., Munnich, A., et al. (2009). Compound heterozygous ASPM mutations associated with microcephaly and simplified cortical gyration in a consanguineous Algerian family. Eur. J. Med. Genet. 52, 180–184. doi: 10.1016/j.ejmg.2009.03.013
Saito, T., Niida, A., Uchi, R., Hirata, H., Komatsu, H., Sakimura, S., et al. (2018). A temporal shift of the evolutionary principle shaping intratumor heterogeneity in colorectal cancer. Nat. Commun. 9:2884. doi: 10.1038/s41467-018-05226-0
Sajid Hussain, M., Marriam Bakhtiar, S., Farooq, M., Anjum, I., Janzen, E., Reza Toliat, M., et al. (2013). Genetic heterogeneity in Pakistani microcephaly families. Clin. Genet. 83, 446–451. doi: 10.1111/j.1399-0004.2012.01932.x
Sanborn, J. Z., Chung, J., Purdom, E., Wang, N. J., Kakavand, H., Wilmott, J. S., et al. (2015). Phylogenetic analyses of melanoma reveal complex patterns of metastatic dissemination. Proc. Natl. Acad. Sci. U. S. A. 112, 10995–11000. doi: 10.1073/pnas.1508074112
Saunders, R. D., Avides, M. C., Howard, T., Gonzalez, C., and Glover, D. M. (1997). The Drosophila gene abnormal spindle encodes a novel microtubule-associated protein that associates with the polar regions of the mitotic spindle. J. Cell Biol. 137, 881–890. doi: 10.1083/jcb.137.4.881
Schoborg, T., Zajac, A. L., Fagerstrom, C. J., Guillen, R. X., and Rusan, N. M. (2015). An asp-CaM complex is required for centrosome-pole cohesion and centrosome inheritance in neural stem cells. J. Cell Biol. 211, 987–998. doi: 10.1083/jcb.201509054
Schou, K. B., Morthorst, S. K., Christensen, S. T., and Pedersen, L. B. (2014). Identification of conserved, centrosome-targeting ASH domains in TRAPPII complex subunits and TRAPPC8. Cilia 3:6. doi: 10.1186/2046-2530-3-6
Schunk, S. J., Floege, J., Fliser, D., and Speer, T. (2021). WNT-beta-catenin signalling - a versatile player in kidney injury and repair. Nat. Rev. Nephrol. 17, 172–184. doi: 10.1038/s41581-020-00343-w
Sepulveda, G., Antkowiak, M., Brust-Mascher, I., Mahe, K., Ou, T., Castro, N. M., et al. (2018). Co-translational protein targeting facilitates centrosomal recruitment of PCNT during centrosome maturation in vertebrates. elife 7:7. doi: 10.7554/eLife.34959
Seshagiri, S., Stawiski, E. W., Durinck, S., Modrusan, Z., Storm, E. E., Conboy, C. B., et al. (2012). Recurrent R-spondin fusions in colon cancer. Nature 488, 660–664. doi: 10.1038/nature11282
Sharpe, H. J., Pau, G., Dijkgraaf, G. J., Basset-Seguin, N., Modrusan, Z., Januario, T., et al. (2015). Genomic analysis of smoothened inhibitor resistance in basal cell carcinoma. Cancer Cell 27, 327–341. doi: 10.1016/j.ccell.2015.02.001
Shen, J., et al. (2005). ASPM mutations identified in patients with primary microcephaly and seizures. J. Med. Genet. 42, 725–729. doi: 10.1136/jmg.2004.027706
Shi, H., Xu, H., Chai, C., Qin, Z., and Zhou, W. (2022). Integrated bioinformatics analysis of potential biomarkers for pancreatic cancer. J. Clin. Lab. Anal. 36:e24381. doi: 10.1002/jcla.24381
Shiwaku, H., and Okazawa, H. (2015). Impaired DNA damage repair as a common feature of neurodegenerative diseases and psychiatric disorders. Curr. Mol. Med. 15, 119–128. doi: 10.2174/1566524015666150303002556
Shubbar, E., Kovács, A., Hajizadeh, S., Parris, T. Z., Nemes, S., Gunnarsdóttir, K., et al. (2013). Elevated cyclin B2 expression in invasive breast carcinoma is associated with unfavorable clinical outcome. BMC Cancer 13:1. doi: 10.1186/1471-2407-13-1
Singh, P., Ramdas Nair, A., and Cabernard, C. (2014). The centriolar protein Bld10/Cep135 is required to establish centrosome asymmetry in Drosophila neuroblasts. Curr. Biol. 24, 1548–1555. doi: 10.1016/j.cub.2014.05.050
Singhmar, P., and Kumar, A. (2011). Angelman syndrome protein UBE3A interacts with primary microcephaly protein ASPM, localizes to centrosomes and regulates chromosome segregation. PLoS One 6:e20397. doi: 10.1371/journal.pone.0020397
Stiles, J., and Jernigan, T. L. (2010). The basics of brain development. Neuropsychol. Rev. 20, 327–348. doi: 10.1007/s11065-010-9148-4
Su, W., Huang, B., Zhang, Q., Han, W., An, L., Guan, Y., et al. (2022). Exploring potential biomarkers, Ferroptosis mechanisms, and therapeutic targets associated with cutaneous squamous cell carcinoma via integrated transcriptomic analysis. J. Healthc. Eng. 2022:3524022. doi: 10.1155/2022/3524022
Sunkel, C. E., and Glover, D. M. (1988). Polo, a mitotic mutant of Drosophila displaying abnormal spindle poles. J. Cell Sci. 89, 25–38. doi: 10.1242/jcs.89.1.25
Tan, S., Chen, W., Kong, G., and Wei, L. (2022). ASPM may be related to the malignant progression of hepatitis B and is associated with a poor prognosis of hepatocellular carcinoma. Front. Bioinform. 2:871027. doi: 10.3389/fbinf.2022.871027
Tan, C. A., del Gaudio, D., Dempsey, M. A., Arndt, K., Botes, S., Reeder, A., et al. (2014). Analysis of ASPM in an ethnically diverse cohort of 400 patient samples: perspectives of the molecular diagnostic laboratory. Clin. Genet. 85, 353–358. doi: 10.1111/cge.12172
Tang, Y., Li, Q., Zhang, D., Ma, Z., Yang, J., Cui, Y., et al. (2022). Cuproptosis-related gene signature correlates with the tumor immune features and predicts the prognosis of early-stage lung adenocarcinoma patients. Front. Genet. 13:977156. doi: 10.3389/fgene.2022.977156
Tang, J., Lu, M., Cui, Q., Zhang, D., Kong, D., Liao, X., et al. (2019). Overexpression of ASPM, CDC20, and TTK confer a poorer prognosis in breast Cancer identified by gene co-expression network analysis. Front. Oncol. 9:310. doi: 10.3389/fonc.2019.00310
Taverna, E., Gotz, M., and Huttner, W. B. (2014). The cell biology of neurogenesis: toward an understanding of the development and evolution of the neocortex. Annu. Rev. Cell Dev. Biol. 30, 465–502. doi: 10.1146/annurev-cellbio-101011-155801
Tessoulin, B., Moreau-Aubry, A., Descamps, G., Gomez-Bougie, P., Maïga, S., Gaignard, A., et al. (2018). Whole-exon sequencing of human myeloma cell lines shows mutations related to myeloma patients at relapse with major hits in the DNA regulation and repair pathways. J. Hematol. Oncol. 11:137. doi: 10.1186/s13045-018-0679-0
Tran, T. H., Diep, Q. M., Cao, M. H., Luong, L. H., Pham, V. A., Lan Dinh, O. T., et al. (2021). Microcephaly primary hereditary (MCPH): report of novel ASPM variants and prenatal diagnosis in a Vietnamese family. Taiwan. J. Obstet. Gynecol. 60, 907–910. doi: 10.1016/j.tjog.2021.07.022
Trimborn, M., Bell, S. M., Felix, C., Rashid, Y., Jafri, H., Griffiths, P. D., et al. (2004). Mutations in microcephalin cause aberrant regulation of chromosome condensation. Am. J. Hum. Genet. 75, 261–266. doi: 10.1086/422855
Tu, B., Jia, Y., and Qian, J. (2022). Bioinformatics analysis identified five widely expressed genes associated with prognosis in sarcoma. Int. J. Gen. Med. 15, 3711–3725. doi: 10.2147/IJGM.S352048
Tungadi, E. A., Ito, A., Kiyomitsu, T., and Goshima, G. (2017). Human microcephaly ASPM protein is a spindle pole-focusing factor that functions redundantly with CDK5RAP2. J. Cell Sci. 130, 3676–3684. doi: 10.1242/jcs.203703
Turkyilmaz, A., and Sager, S. G. (2022). Two new cases of primary microcephaly with neuronal migration defect caused by truncating mutations in the ASPM gene. Mol. Syndromol. 13, 56–63. doi: 10.1159/000516201
van der Voet, M., Berends, C. W. H., Perreault, A., Nguyen-Ngoc, T., Gönczy, P., Vidal, M., et al. (2009). NuMA-related LIN-5, ASPM-1, calmodulin and dynein promote meiotic spindle rotation independently of cortical LIN-5/GPR/Galpha. Nat. Cell Biol. 11, 269–277. doi: 10.1038/ncb1834
Verdier, P., Morthorst, S. K., and Pedersen, L. B. (2016). Targeting of ASH domain-containing proteins to the centrosome. Methods Mol. Biol. 1454, 15–33. doi: 10.1007/978-1-4939-3789-9_2
Visnyei, K., Onodera, H., Damoiseaux, R., Saigusa, K., Petrosyan, S., de Vries, D., et al. (2011). A molecular screening approach to identify and characterize inhibitors of glioblastoma stem cells. Mol. Cancer Ther. 10, 1818–1828. doi: 10.1158/1535-7163.MCT-11-0268
von Wrede, R., Schidlowski, M., Huppertz, H. J., Rüber, T., Ivo, A., Baumgartner, T., et al. (2022). Large phenotypic variation of individuals from a family with a novel ASPM mutation associated with microcephaly, epilepsy, and behavioral and cognitive deficits. Genes (Basel) 13, 429. doi: 10.3390/genes13030429
Wagle, R., and Song, Y. H. (2020). Ionizing radiation reduces larval brain size by inducing premature differentiation of Drosophila neural stem cells. Biochem. Biophys. Res. Commun. 523, 555–560. doi: 10.1016/j.bbrc.2019.12.047
Wang, Y., Cheng, H., Zeng, T., Chen, S., Xing, Q., and Zhu, B. (2022). A novel 17 apoptosis-related genes signature could predict overall survival for bladder cancer and its associations with immune infiltration. Heliyon 8:e11343. doi: 10.1016/j.heliyon.2022.e11343
Wang, R., Khan, A., Han, S., and Zhang, X. (2017). Molecular analysis of 23 Pakistani families with autosomal recessive primary microcephaly using targeted next-generation sequencing. J. Hum. Genet. 62, 299–304. doi: 10.1038/jhg.2016.128
Wei, L., Wang, Y., Zhou, D., Li, X., Wang, Z., Yao, G., et al. (2021). Bioinformatics analysis on enrichment analysis of potential hub genes in breast cancer. Transl. Cancer Res. 10, 2399–2408. doi: 10.21037/tcr-21-749
Wei, P. C., et al. (2016). Long neural Genes Harbor recurrent DNA break clusters in neural stem/progenitor cells. Cells 164, 644–655. doi: 10.1016/j.cell.2015.12.039
Wen, X., Liu, S., and Cui, M. (2020). Effect of BRCA1 on the concurrent Chemoradiotherapy resistance of cervical squamous cell carcinoma based on transcriptome sequencing analysis. Biomed. Res. Int. 2020:3598417. doi: 10.1155/2020/3598417
Williams, S. E., Garcia, I., Crowther, A. J., Li, S., Stewart, A., Liu, H., et al. (2015). Aspm sustains postnatal cerebellar neurogenesis and medulloblastoma growth in mice. Development 142, 3921–3932. doi: 10.1242/dev.124271
Woods, C. G., Bond, J., and Enard, W. (2005). Autosomal recessive primary microcephaly (MCPH): a review of clinical, molecular, and evolutionary findings. Am. J. Hum. Genet. 76, 717–728. doi: 10.1086/429930
Woodworth, M. B., Greig, L. C., Kriegstein, A. R., and Macklis, J. D. (2012). SnapShot: cortical development. Cells 151, 918–918.e1. doi: 10.1016/j.cell.2012.10.004
Wu, J., He, Z., Zhu, Y., Jiang, C., Deng, Y., and Wei, B. (2021). ASPM predicts poor clinical outcome and promotes tumorigenesis for diffuse large B-cell lymphoma. Curr. Cancer Drug Targets 21, 80–89. doi: 10.2174/1568009620666200915090703
Wu, X., Xu, S., Wang, P., Wang, Z. Q., Chen, H., Xu, X., et al. (2022). ASPM promotes ATR-CHK1 activation and stabilizes stalled replication forks in response to replication stress. Proc. Natl. Acad. Sci. 119:e2203783119. doi: 10.1073/pnas.2203783119
Wu, Y., You, Y., Chen, L., Liu, Y., Liu, Y., Lou, W., et al. (2022). Abnormal spindle-like microcephaly-associated protein promotes proliferation by regulating cell cycle in epithelial ovarian cancer. Gland Surg. 11, 687–701. doi: 10.21037/gs-22-29
Xicola, R. M., Manojlovic, Z., Augustus, G. J., Kupfer, S. S., Emmadi, R., Alagiozian-Angelova, V., et al. (2018). Lack of APC somatic mutation is associated with early-onset colorectal cancer in African Americans. Carcinogenesis 39, 1331–1341. doi: 10.1093/carcin/bgy122
Xie, J. J., Zhuo, Y. J., Zheng, Y., Mo, R. J., Liu, Z. Z., Li, B. W., et al. (2017). High expression of ASPM correlates with tumor progression and predicts poor outcome in patients with prostate cancer. Int. Urol. Nephrol. 49, 817–823. doi: 10.1007/s11255-017-1545-7
Xu, S., Wu, X., Peng, B., Cao, S. L., and Xu, X. (2020). Primary microcephaly with an unstable genome. Genome Instab. Dis. 1, 235–264. doi: 10.1007/s42764-020-00020-z
Xu, S., Wu, X., Wang, P., Cao, S. L., Peng, B., and Xu, X. (2021). ASPM promotes homologous recombination-mediated DNA repair by safeguarding BRCA1 stability. iScience 24:102534. doi: 10.1016/j.isci.2021.102534
Xu, W., Xu, J., Wang, Z., and Jiang, Y. (2021). Weighted gene correlation network analysis identifies specific functional modules and genes in esophageal cancer. J. Oncol. 2021:8223263. doi: 10.1155/2021/8223263
Xu, Z., Zhang, Q., Luh, F., Jin, B., and Liu, X. (2019). Overexpression of the ASPM gene is associated with aggressiveness and poor outcome in bladder cancer. Oncol. Lett. 17, 1865–1876. doi: 10.3892/ol.2018.9762
Xu, S., Zhang, W., Zhou, R., Huang, H., Chen, W., Xiang, W., et al. (2022). Two novel truncating variants of the ASPM gene identified in a nonconsanguineous Chinese family associated with primary microcephaly. Clin. Dysmorphol. 31, 1–5. doi: 10.1097/MCD.0000000000000395
Yang, Z., Wu, X., Li, J., Zheng, Q., Niu, J., and Li, S. (2021). CCNB2, CDC20, AURKA, TOP2A, MELK, NCAPG, KIF20A, UBE2C, PRC1, and ASPM may be potential therapeutic targets for hepatocellular carcinoma using integrated bioinformatic analysis. Int. J. Gen. Med. 14, 10185–10194. doi: 10.2147/IJGM.S341379
Yin, Q., Chen, W., Zhang, C., and Wei, Z. (2022). A convolutional neural network model for survival prediction based on prognosis-related cascaded Wx feature selection. Lab. Investig. 102, 1064–1074. doi: 10.1038/s41374-022-00801-y
Yu, F., Yu, C., Li, F., Zuo, Y., Wang, Y., Yao, L., et al. (2021). Wnt/beta-catenin signaling in cancers and targeted therapies. Signal Transduct. Target. Ther. 6:307. doi: 10.1038/s41392-021-00701-5
Zaqout, S., and Kaindl, A. M. (2021). Autosomal recessive primary microcephaly: not just a small brain. Front. Cell Dev. Biol. 9:784700. doi: 10.3389/fcell.2021.784700
Zhang, S., Pang, K., Feng, X., and Zeng, Y. (2022). Transcriptomic data exploration of consensus genes and molecular mechanisms between chronic obstructive pulmonary disease and lung adenocarcinoma. Sci. Rep. 12:13214. doi: 10.1038/s41598-022-17552-x
Zhang, Q., Wang, Y., and Xue, F. (2022). ASPM, CDC20, DLGAP5, BUB1B, CDCA8, and NCAPG may serve as diagnostic and prognostic biomarkers in endometrial carcinoma. Genet. Res. 2022:3217248. doi: 10.1155/2022/3217248
Zhang, X., Yang, L., Chen, W., and Kong, M. (2020). Identification of potential hub genes and therapeutic drugs in malignant pleural mesothelioma by integrated bioinformatics analysis. Oncol. Res. Treat. 43, 656–671. doi: 10.1159/000510534
Zhang, Y., Zeng, L., and Lin, L. (2022). Diagnosis and counseling for a Chinese pedigree affected with autosomal recessive primary microcephaly 5 due to variants of ASPM gene. Zhonghua Yi Xue Yi Chuan Xue Za Zhi 39, 405–408. doi: 10.3760/cma.j.cn511374-20200820-00615
Zhang, L., Zhou, Y., Cheng, C., Cui, H., Cheng, L., Kong, P., et al. (2015). Genomic analyses reveal mutational signatures and frequently altered genes in esophageal squamous cell carcinoma. Am. J. Hum. Genet. 96, 597–611. doi: 10.1016/j.ajhg.2015.02.017
Zhong, X., Liu, L., Zhao, A., Pfeifer, G. P., and Xu, X. (2005). The abnormal spindle-like, microcephaly-associated (ASPM) gene encodes a centrosomal protein. Cell Cycle 4, 1227–1229. doi: 10.4161/cc.4.9.2029
Zhou, Z. W., Tapias, A., Bruhn, C., Gruber, R., Sukchev, M., and Wang, Z. Q. (2013). DNA damage response in microcephaly development of MCPH1 mouse model. DNA Repair (Amst) 12, 645–655. doi: 10.1016/j.dnarep.2013.04.017
Keywords: microcephaly, small brain, ASPM, MCPH5, neurogenesis, cancer
Citation: Wu X, Li Z, Wang Z-Q and Xu X (2023) The neurological and non-neurological roles of the primary microcephaly-associated protein ASPM. Front. Neurosci. 17:1242448. doi: 10.3389/fnins.2023.1242448
Edited by:
Zhong-Wei Zhou, Sun Yat-sen University, ChinaReviewed by:
Ling Yuan, Central South University, ChinaDorothy Lerit, Emory University, United States
Copyright © 2023 Wu, Li, Wang and Xu. This is an open-access article distributed under the terms of the Creative Commons Attribution License (CC BY). The use, distribution or reproduction in other forums is permitted, provided the original author(s) and the copyright owner(s) are credited and that the original publication in this journal is cited, in accordance with accepted academic practice. No use, distribution or reproduction is permitted which does not comply with these terms.
*Correspondence: Xingzhi Xu, WGluZ3poaS5YdUBzenUuZWR1LmNu