- 1School of Biomedical Sciences, Institute of Clinical Sciences, University of Birmingham, Birmingham, United Kingdom
- 2Department of Neurology, University Hospitals Birmingham NHS Foundation Trust, Birmingham, United Kingdom
- 3Institute of Inflammation and Ageing, University of Birmingham, Birmingham, United Kingdom
MicroRNAs (miRNAs) are small non-coding RNAs involved in gene regulation. Recently, miRNA dysregulation has been found in neurodegenerative diseases such as Alzheimer’s disease (AD) and Parkinson’s disease (PD). The diagnosis of Alzheimer’s and Parkinson’s is currently challenging, mainly occurring when pathology is already present, and although treatments are available for both diseases, the role of treatment is primarily to prevent or delay the progress of the diseases instead of fully overcoming the diseases. Therefore, the challenge in the near future will be to determine effective drugs to tackle the dysregulated biological pathways in neurodegenerative diseases. In the present study, we describe the dysregulation of miRNAs in blood of Alzheimer’s and Parkinson’s patients with the aim to identify common mechanisms between the 2 pathologies and potentially to identify common therapeutic targets which can stop or delay the progression of two most frequent neuropathologies. Two independent systematic reviews, bioinformatic analysis, and experiment validation were performed to identify whether AD and PD share common pathways. A total of 15 common miRNAs were found in the literature and 13 common KEGG pathways. Among the common miRNAs, two were selected for validation in a small cohort of AD and PD patients. Let-7f-5p and miR-29b-3p showed to be good predictors in blood of PD patients.
Introduction
Neurodegeneration is a term that can be applied to several conditions which share, as common features, the progressive loss of structure and function of central nervous system (Wareham et al., 2022). Neurodegenerative diseases include a variety of pathological disease entities and clinical presentations, among these, the most prevalent are Alzheimer’s disease (AD, progressive dementia), Parkinson disease (PD, progressive movement disorder with or without dementia; Poewe et al., 2017; Leng and Edison, 2021). Although AD and PD are two distinct diseases they often appear together and share several risk factors contributing to the development and progression of the two pathologies, including aging, genetic and epigenetic factors, environmental factors (Poirier et al., 1993; Hou et al., 2019; Businaro et al., 2021; Marques-Aleixo et al., 2021). AD and PD are neurodegenerative diseases which affect memory, movement and communication. PD typically presents clinically with a tremor at rest, Bradykinesia, rigidity and gait impairments with pathological features of dopaminergic neuron loss and presence of Lewy bodies, whereas AD typically presents clinically as learning and memory loss, reductions in executive function and speech and, pathologically, is associated with the presence of amyloid-beta protein and neurofibrillary tangles. Despite their clinical and pathological features, there are common pathological mechanisms which occur. These include disturbances in iron metabolism (Oshiro et al., 2011), build-up α-Synuclein and Tau protein (Kim et al., 2004; Sengupta and Kayed, 2022), higher levels of oxidative stress and mitochondrial dysfunction (Alqahtani et al., 2023), reductions of noradrenergic neurons in the Locus Coeruleus and increased levels of neuroinflammation (Sakakibara et al., 2021; Zhou et al., 2021). However, despite the considerable effort in studying these two pathologies, our understanding of the mechanisms involved and the link between them remains rudimentary at best.
With this study, we investigated the commonalities of these two pathologies looking at similarities and differences in microRNA (miRNA, miR) expression patterns and pathways regulated by them. The emerging developments in the field of miRNAs has led to the investigation of their function in the nervous system physiology and pathology. High throughput sequencing experiments have reported that almost 50% of the miRNAs are expressed in the mammalian brain (Shao et al., 2010) and can play a role locally in synaptic activity, neuronal connectivity and neuroplasticity (Aksoy-Aksel et al., 2014; Ryan et al., 2015).
There is also an increased interest in miRNA roles in neurodegenerative conditions. In AD it has been reported that Aβ production involves miRNA-mediated regulation and that dysregulation of miR-29a/29b-1 alters the expression of β-secretase (BACE-1) (Hébert et al., 2008; Wang W. X. et al., 2008; Geekiyanage and Chan, 2011). MiRNAs have also been found to play an important role in PD, for example, dysregulation of miR-7 and miR-34b/c was associated with PD mitochondrial dysfunction and oxidative stress (Junn et al., 2009; Miñones-Moyano et al., 2011). Additionally, miR-133b, which plays a key role in the differentiation of dopaminergic neurones, was found to be downregulated in PD (Kim et al., 2007).
This review has the aim to identify the common features of two most common neurodegenerative diseases, AD and PD, improving our understanding of how critical biological pathways impact AD and PD and influence the neurodegenerative processes and the clinical outcomes. Common and altered AD and PD pathways can also be used to identify promising new targets for drug development as well as to identify new molecules as non-invasive biomarkers.
Results
Search results
There were two separate searches conducted for AD and PD in the systematic review. Through PubMed, EMBASE and Web of Science, 605 searches were retrieved for the AD search. 112 of these entries were unique, without duplicates. Through screening against the inclusion and exclusion criteria, 19 papers were included (Figure 1). Of the papers excluded, many did not describe AD and looked at other neurodegenerative disorders or mild cognitive impairment (n = 31). Also, many papers used animal models, which were not relevant to this study (n = 22). Lastly, another large reason for exclusion was that many of the papers were review articles, and this study required primary articles for analysis (n = 11).
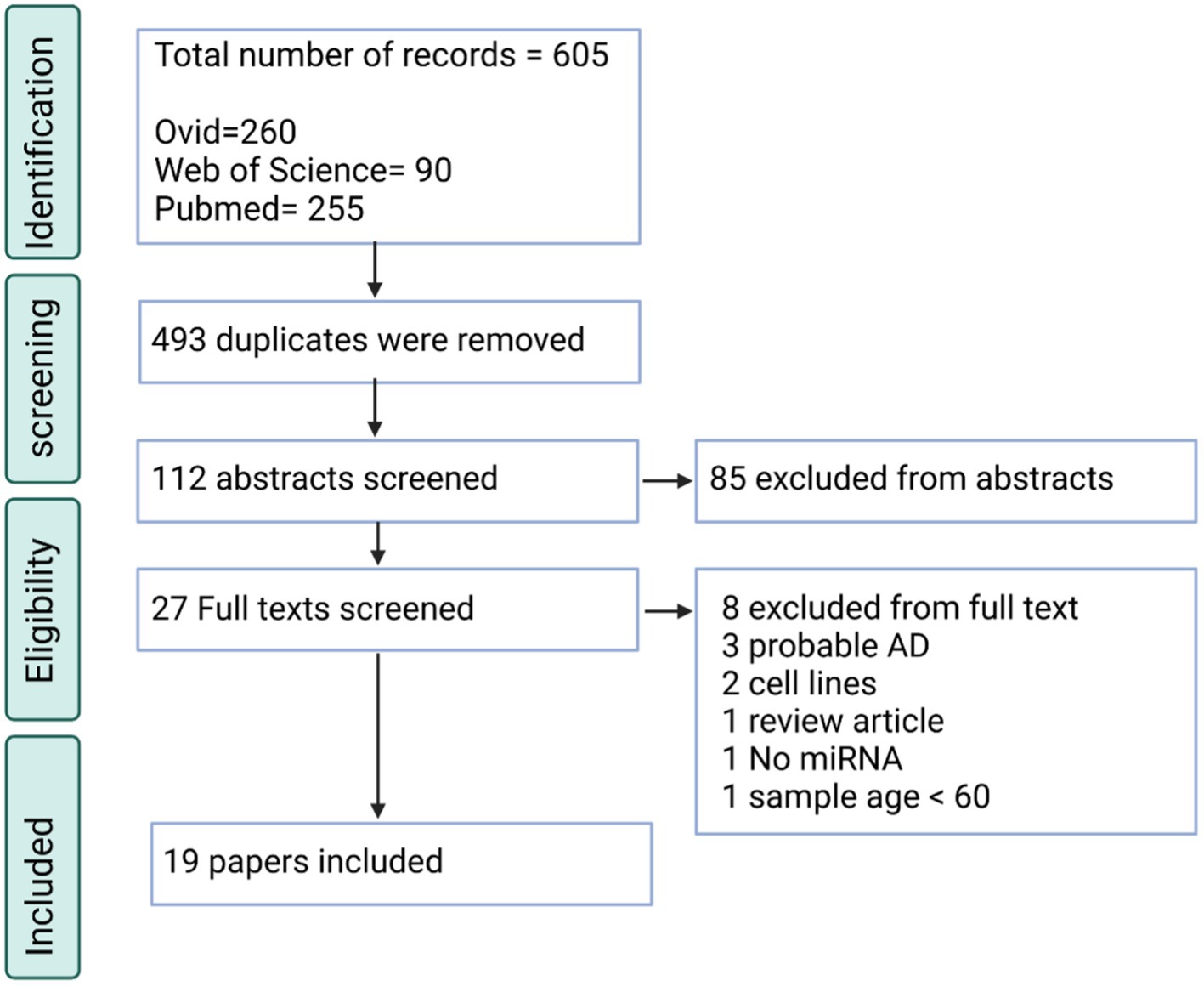
Figure 1. PRISMA flow chart for the AD systematic review displaying the screening process utilized to acquire the articles containing miRNAs.
Regarding the PD searches, 584 total entries were inputted, of which 92 were unique. Through screening, the total included papers were 19 papers altogether (Figure 2). The significant reasons for exclusion were similar to the AD searches. For this search, it was also found that many papers did not describe PD (n = 28), some were review articles (n = 17), and some did not quantify the miRNAs in the blood sample (n = 17).
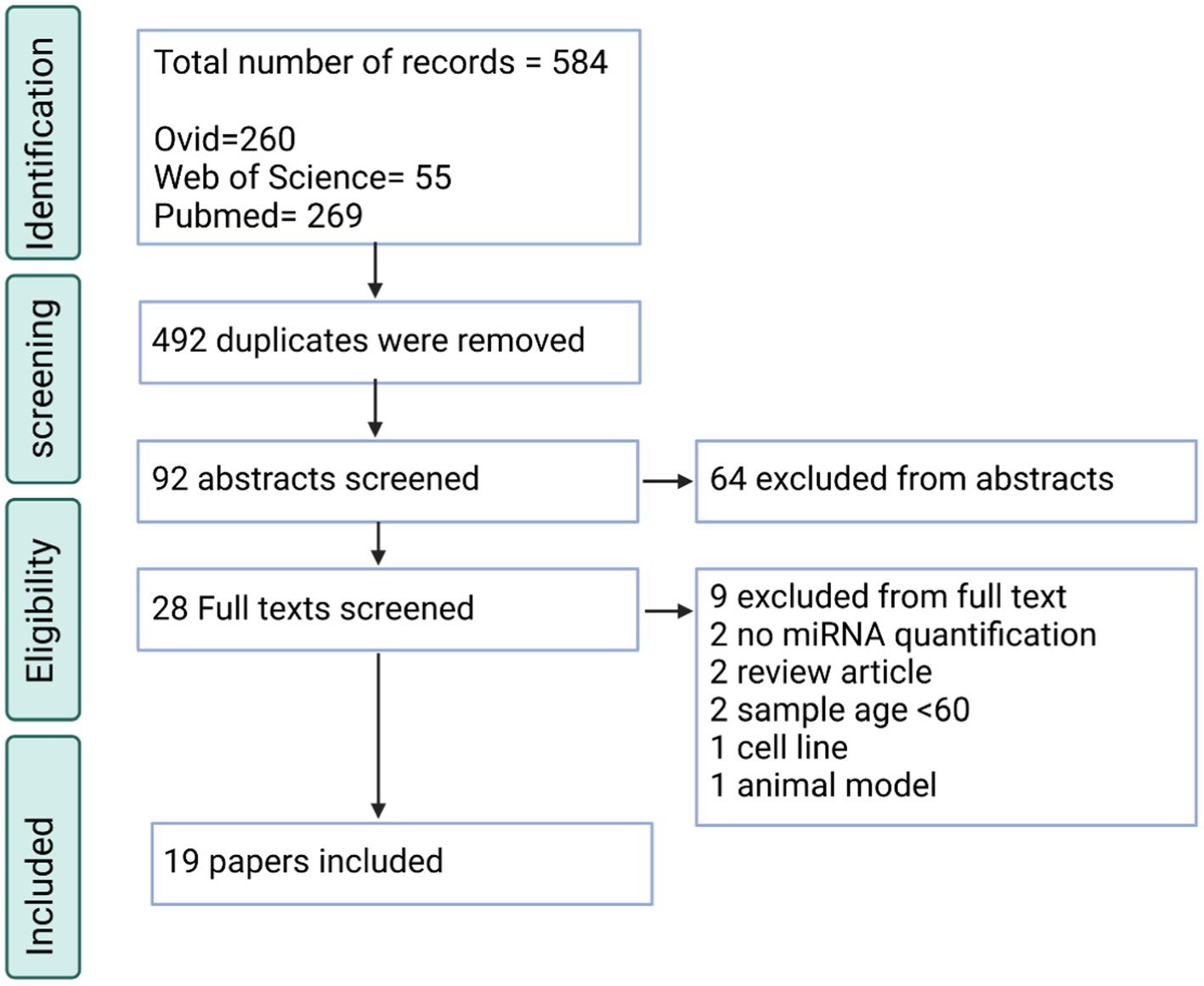
Figure 2. PRISMA flow chart for the PD systematic review displaying the screening process utilized to acquire the articles containing miRNAs.
Data extraction
From the two independent systematic reviews, data regarding the sample population and findings were extracted (Tables 1 2). 19 papers describing blood biomarkers in AD and 19 in PD were identified through two independent systematic reviews. The extraction of these data allows the research question to be investigated, further analyzing if there are any common miRNAs between the two diseases. 15 common dysregulated miRNAs, including 5 showing a different directionality in the 2 pathologies, were finally found (Table 3). A problem encountered at this stage is that not all papers described the study population in fullness, some did not mention how many males and females there were and did not identify the severity of the AD or PD. Where the severity of the disease was not specified, it was assumed they had late or severe, and any other information which was unavailable was written as “NA.”
Pathway analysis
The miRNAs identified for AD and PD were separately inputted into the DIANA database, observing pathways regulated by the miRNAs. The miRNAs obtained from the search regarding AD resulted in 162 KEGG pathways regulated by the miRNAs (Supplementary Table S1). To ensure the pathways are associated, a Fisher exact test was applied at p < 0.05. The most significantly affected pathways include the TGF β signaling pathway, Pancreatic secretion and the Calcium signaling pathway. The same was done for the systematic review regarding PD, which obtained 146 KEGG pathways (Supplementary Table S2). Some affected pathways include the ECM receptor interaction, Ras signaling pathways and PI3K-Akt pathway. The common miRNAs between PD and AD were also assessed to identify common pathways and gene targets, whereby 13 KEGG pathways were identified (Table 4). List of generated predicted targets was further evaluated using ShinyGO 0.77 software for enriched pathway and Gene Ontology (GO) analyses. A chart diagram generated with the list of predicted targets is represented in Figure 3. In addition, GO analyses for biological process, cellular component and molecular function are represented in Supplementary Tables S3–S5 respectively.
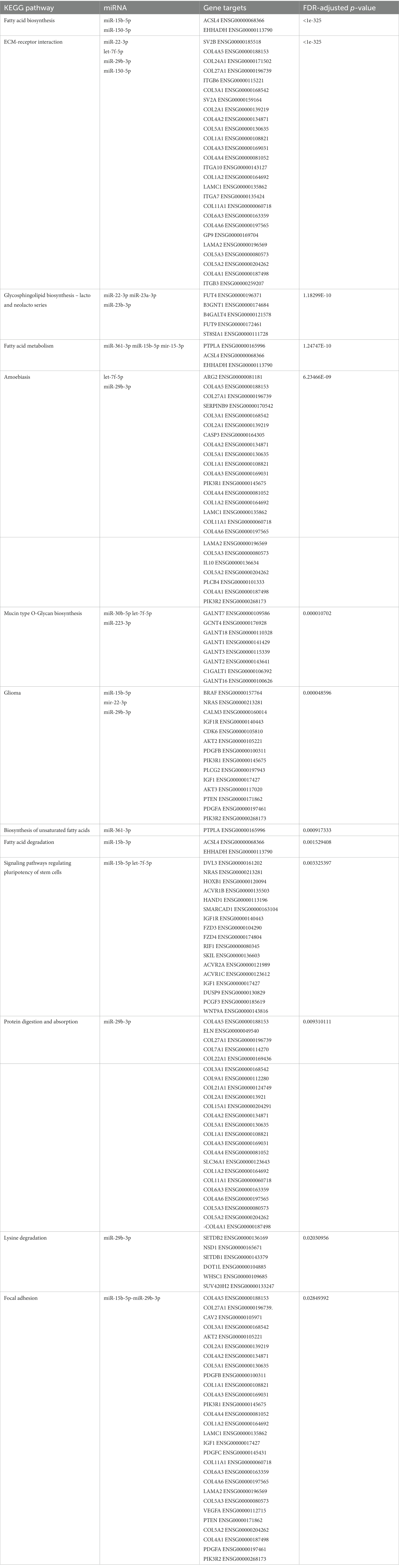
Table 4. The identified KEGG pathways associated with the common miRNAs, gene targets, and correspondent FDR-adjusted p values are also shown in this table.
Quality appraisal
For both systematic reviews, quality appraisals were performed on the papers that were used for the analysis (Table 5). As a result, all of the papers that were included were of a suitable quality. All scored a greater quality than 60%. All papers included had statistical analysis, a reliable methodology and overall low risk of bias. Some papers lacked a clear identification of confounding factors and also did not provide clear inclusion criteria for their study population.
Pilot study: samples validation
Let-7f-5p and miR-29b-3p were selected for the pilot study, among the common miRNAs in 2 pathologies and their expression was analyzed in 5 whole blood samples of AD patients and 10 whole blood samples of PD patients, both groups compared to control (C) whole blood samples (N = 7). Both miRs did not show any significant expression in AD samples compared to controls, while both were significantly downregulated in PD showing a p value of 0.01 and < 0.001, respectively, (Figure 4). One-way ANOVA with post-hoc Tukey’s multiple comparison test was also performed among the 3 different groups and showed adjusted value of ps of 0.997, 0.005, and 0.013 when let-7f was compared between CvsAD; CvsPD; and AD vs PD, respectively. Same analysis was performed for miR-29b-3p and showed adjusted value of ps of 0.434; 0.0002; 0.010 for the same comparisons.
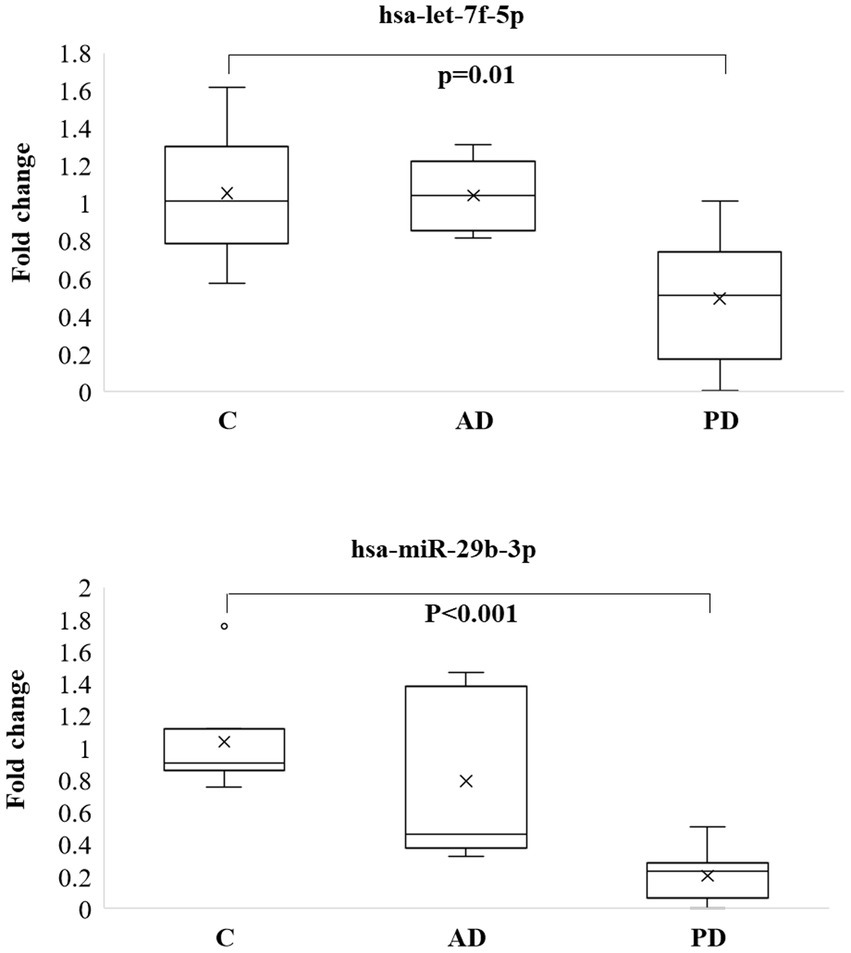
Figure 4. Box plots of let-7f-5p and miR-29b-3p analyzed by qPCR in blood of 5 AD and 10 PD patients and compared to 7 controls.
Conclusion
MiRNAs are non-coding RNAs whose function is to regulate the expression of genes via miRNA-induced silencing complex, in both physiological and pathological conditions and including neurological diseases. The ability of miRNA to regulate several physiological pathways makes it appealing in revealing new molecular mechanisms and suggesting new potential treatments. The aim of this work was to identify common miRNAs in 2 of the most important neurodegenerative diseases, potentially unveiling pathways that may be affected by both disorders and determining the link that irreversible lead to neurodegeneration.
In this study we have identified 15 common miRNAs. Five of them showed dysregulation in opposite direction in the 2 pathologies, suggesting a potential different response in the same altered pathway. Interestingly, our data showed the fatty acid (FA) biosynthesis, metabolism and degradation among the most highly significant and dysregulated pathways in both conditions. This is not surprising as brain is the second most lipid-rich organ in the body, contributing to many fundamental cellular processes, such as membrane synthesis, energy storage, signaling, and complex protein modifications (Sastry, 1985), therefore, FA homeostasis is an essential determinant of neural development, neurotransmission, and receptor activation. In AD research, cholesterol was demonstrated to contribute to the development of amyloid plaques by facilitating the formation of β-sheets (Zhou and Xu, 2012), as well as decreased concentrations of docosahexaenoic acid (DHA) were demonstrated to contribute to increased production of Amyloid β (Grimm et al., 2011).
In PD also, a disruption of FA homeostasis was reported, suggesting a role of polyunsaturated fatty acids in the formation of Lewy bodies, through the interactions of the polyunsaturated fats and the N-terminal of α-synuclein forming oligomers (Karube et al., 2008). Furthermore, a previous study supported that polyunsaturated and saturated fatty acids stabilize soluble α-synuclein oligomers (Sharon et al., 2003).
Another importantly compromised pathway that was identified by our bioinformatic analysis, is the extracellular matrix (ECM) receptor interaction pathway. ECM, which is estimated to constitute 20% of the brain, is involved in essential roles such as cell migration, proliferation and differentiation. This pathway is known to be affected in both AD and PD (Lam et al., 2019; Crapser et al., 2020) and glycosaminoglycans (GAGs), a component of the ECM, are seen in Lewy bodies and might play a role in accumulating α-synuclein by impeding its degradation and thereby facilitating Lewy bodies’ formation (Raghunathan et al., 2020).
Another pathway related to the ECM is the focal adhesion pathway which was also identified as a commonly dysregulated pathway. Focal adhesions play an essential role in cell migration, allowing cells to respond to stimuli. Under normal conditions, focal adhesion kinases (FAK) signal regulate the formation of these adhesions, and the focal adhesion molecules interact with the ECM through integrins whose activation may assist amyloid β plaque formation (Wright et al., 2007). Another study (Wang Q. et al., 2008) showed that αv integrins could also cause inhibition of long-term potentiation, disrupting synaptic plasticity and resulting in neurodegenerative effects.
Within PD, there is less evidence for the involvement of focal adhesions in pathogenesis. However, focal adhesions are critical to the neuronal interactions between other neurons and their environment. The cell adhesion pathway is hypothesized to be disrupted in PD, which affects the interaction with synaptic vesicles (Chapman, 2014). The rapid firing nature of dopaminergic neurons in the substantia nigra creates a greater demand for neurotransmitter release from the vesicles; therefore, this hypothesized dysfunction profoundly impacts neuronal communication. This process is believed to be affected by actin as actin interacts with vesicles and affects their fusion to the synaptic membrane. In addition, microtubules are thought to be involved in the trafficking of vesicles between neurons, affecting neuronal communication (Chapman, 2014). There is evidence that synaptic dysfunction occurs early in PD with decreased dopamine synthesis, storage and release; therefore, these hypothesized mechanisms could be involved in the pathogenesis of PD (Nikolaus et al., 2009). Nonetheless, further research is needed into the potential impact of the focal adhesion pathway on the pathology of PD.
Among the common list of differentially regulated miRNAs, in our pilot study we chose to validate, in a small cohort of patients, two miRNAs, let-7f-5p and miR-29b-3p and explore their potential role as non-invasive biomarkers. Let-7f-5p showed a different trend in the 2 diseases, being upregulated in AD and downregulated in PD (Hara et al., 2017; Chen et al., 2018), its target genes are involved in the cell cycle, apoptosis and cell adhesion (Ghanbari et al., 2015). However, the mechanisms through which let-7f-5p plays a role in pathogenesis are yet to be understood. An overexpression of let-7f-5p was seen in cells undergoing oxidative damage in vitro (Li K. et al., 2020). In this study, the authors using let-7f mimic have found the viability of the cells undergoing oxidative stress to improve while also decreasing apoptosis. It was discovered that AKT-2 can be repressed by let-7f, which is involved in the PI3K-Akt pathway, affecting cell proliferation and apoptosis (Li K. et al., 2020). Through this mechanism, it is speculated that the hsa-let-7f mimic can improve cell viability and reduce apoptosis.
Let-7f-5p also has a role in inflammation, targeting NLRP3 and pro-IL-1β, and repressing their expression (Tan et al., 2019). MiR-29b-3p, instead, is highly expressed in the brain and spinal cord (Smirnova et al., 2005; Hébert et al., 2008). It is involved in different mechanisms (extracellular matrix, insulin signaling, angiogenesis) (Cushing et al., 2011; Yang et al., 2014; Zhang et al., 2014) and regulates distinct cell population or pathologies (Park et al., 2009; Kwon et al., 2019) can also play crucial role during neuronal maturation, or can target BH3 protein levels in favor of neuronal degeneration (Liu et al., 2015; Huang et al., 2018). Its downregulation has been previously described in peripheral blood mononuclear cells (Villa et al., 2013), plasma (Lugli et al., 2015) and brain of subjects(Hébert et al., 2008) with AD whereby up-regulation has been seen in the cerebrospinal fluid of AD patients (Kiko et al., 2014). In addition, positive correlations were described between miR-29b concentration in serum and cortical thickness and cortical glucose metabolism (Maldonado-Lasuncion et al., 2019), which both decrease in AD. Finally, there are also studies showing the use of miR-29b as potential therapeutic agent in AD (Pereira et al., 2017).
Our data, despite the small sample size, support the existing literature, showing the downregulation of both miRNAs, let-7f and miR-29b in blood of PD patients and therefore confirming their use as potential biomarkers. On the contrary, both microRNA did not show any significant results in AD patients, although several limitations must be noted.
First of all, samples size is minimal, the number of patients that composes the validation cohorts is very low, especially for AD cohort. Moreover, AD patients were all diagnosed with an early stage of the disease and previous work showed a less strong signature of miRNA profile in the initial phase (Watson et al., 2022). Hence the large standard deviation and the consequent low statistical power for the validation studies. Therefore, a larger cohort is necessary to validate these findings.
Furthermore, there is an evident lack of age matching that may confound the interpretation of miRNA data. Therefore an age-matched control group is required for this study. The samples were extracted from whole blood, and most of the studies in the SRs reported the expression in serum/plasma. Therefore, the miRNA expression is influenced by intracellular miRNA-content. Finally, only 2 miRNAs were selected and tested in this pilot study. These two were selected on the basis of our previous results showing potential long-term implications in neurodegenerative process after a brain injury (Pietro et al., 2021).
In conclusion, this study did identify possible ways in which AD and PD share similar pathways leading to pathology, providing potential targets for future research into the disease and its treatments. A crosstalk interaction study between miRNAs and their targets related to the identified pathways is now necessary in order to propose potential area of intervention. Finally, this work was also able to show the use of miR-29b-3p as a non-invasive biomarker for PD.
Materials and methods
Study design
Two parallel and independent systematic reviews were undertaken using the Preferred Reporting Items for Systematic Reviews and Meta-Analyses (PRISMA) guidelines (Liberati et al., 2009). The research question is as follows: “To identify the differentially expressed miRNAs in blood/serum/plasma of Alzheimer’s and Parkinson’s diseases.” From the research question focused keywords were used as search terms in order to gather relevant records from three databases. Records eligible for inclusion were deciphered through a strict inclusion and exclusion criteria. The inclusion and exclusion criteria’s were used to complete an abstract and full-text screening. This was carried out manually by a primary reviewer and then included articles were checked by a second reviewer (B.W., V.DP.). Data extracted and miRNAs identified through the literature search were entered into DIANA database, for in silico bioinformatic analysis of predicted gene targets and Kyoto Encyclopedia of Genes and Genomes (KEGG) pathway identification. List of predicted targets were further evaluated by ShinyGo 0.77 for Gene Ontology (GO) enrichment analyses.
Two common miRNAs were selected from this review and used for a pilot study. Let-7f-5p and miR-29b-3p were analyzed in whole blood of 5 AD and 10 PD patients to confirm their validity as potential blood biomarkers.
Search strategy
The Population, Intervention, Comparison (s), and Outcome (s) (PICOs) framework was used to determine a focused research question for the systematic review. This Cochrane Collaboration-recommended system enables a quantitative analysis of the results by the comprehensive gathering of the evidence within the defined parameters.
Population
Patients over 60 diagnosed with AD and PD of all races and genders.
Cut-off of 60 years old was chosen, since a stronger miRNA signature is identified in late AD stage (Watson et al., 2022). While people with Parkinson’s are generally diagnosed at an average age of 60 (Samii et al., 2004).
Intervention
Patients have received an AD or PD diagnosis against the clinical criteria.
Comparison
Healthy age-matched individuals who do not meet the clinical requirements for AD, PD or cognitive impairment diagnosis.
Outcome
The dysregulation of miRNA in serum/plasma/blood of AD and PD compared to the healthy controls to identify potential common pathways.
Search terms and database
In order to comply with PRISMA guidelines, a selection of keywords were created. To ensure all relevant titles were covered, all possible spellings and abbreviations were used as keywords. These were searched using three different databases, PubMed, EMBASE and Web of Science. Separate searches were done for papers concerning PD and AD using separate keywords. The keywords used are as follows: “Alzheimer’s disease” AND “microRNA” OR “miRNA” OR “miR” AND “dysregulation” OR “Upregulation” OR “downregulation” AND “human” AND “blood” OR “plasma” OR “serum.” For the PD search, the keywords used are as follows: “Parkinson’s disease” AND “microRNA” OR “miRNA” OR “miR” AND “dysregulation” OR “upregulation” OR “downregulation” AND “human” AND “blood” OR “plasma” OR “serum.” The records retrieved were collated in Endnote 20 (Clarivate, Philadelphia, PA, USA) where duplicates were screened and any identified were removed. Using the defined inclusion and exclusion criteria, the remaining abstracts were then manually evaluated. Two independent reviewers evaluated the eligible records as outlined in Table 6. Papers analyzed were filtered between 2011 and Feb 2022 to guarantee that all searches were up to date with research.
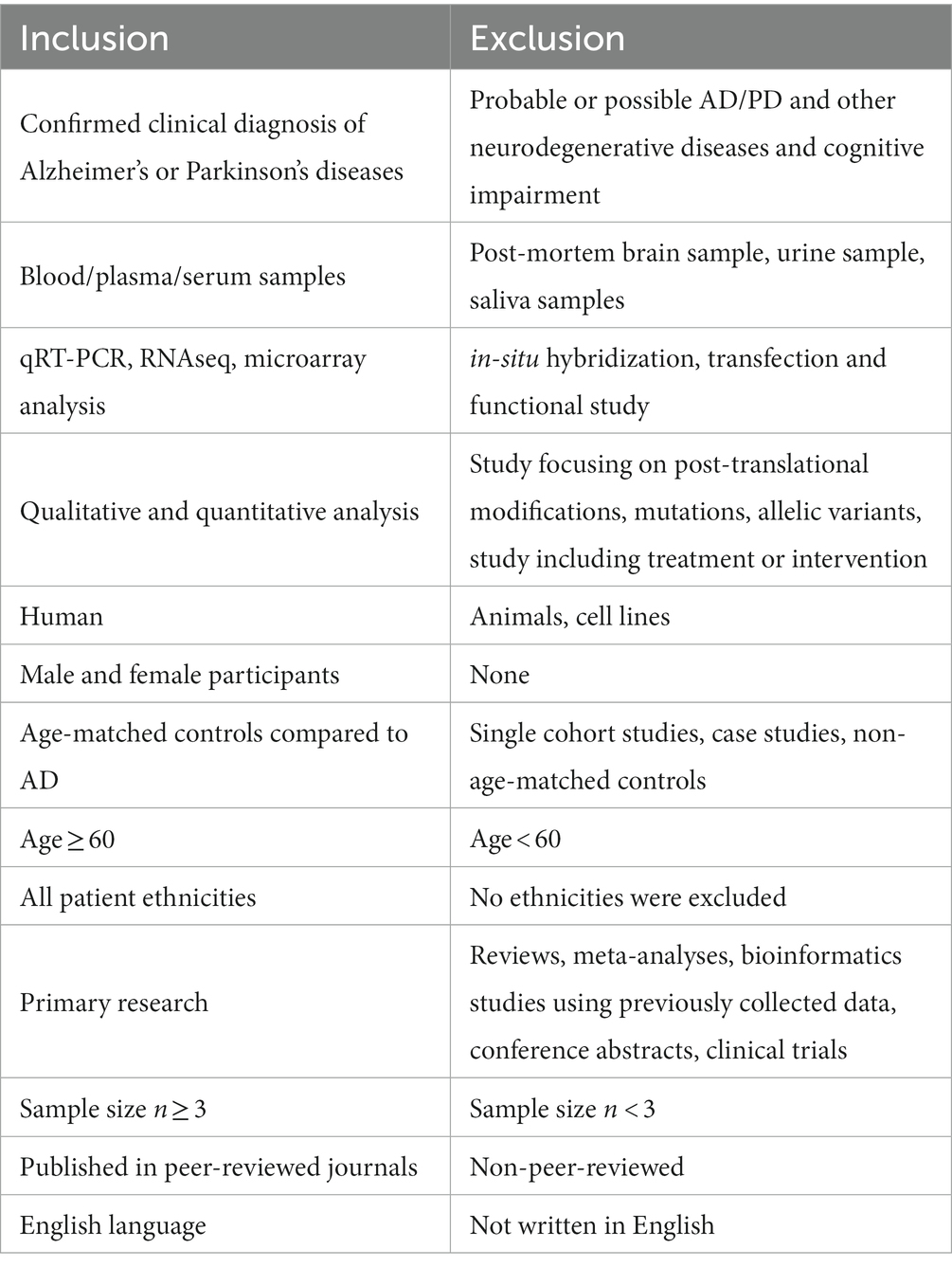
Table 6. Inclusion and exclusion criteria utilized to direct the miRNA in AD and PD systematic literature search.
Data collection
After screening, the papers that fit the inclusion criteria were saved into a separate excel file. The excel datafile included the title, the authors, the year published, and the URL. Two separate files were made for the papers describing AD and those describing PD. Then the text was screened further to identify the population of those with AD/PD and the controls, the gender, age, and AD or PD severity. Further, the miRNAs were identified, whether they were up or downregulated, and lastly, the technique used was identified. This step ensured that the relevant information from each study was included. Data were extracted from the final included studies and imported into Microsoft Excel (Microsoft Corporation, Redmond, WA, USA).
Quality appraisal
A critical appraisal was conducted using the JBI checklist tool for systematic reviews to ensure the studies quality and determine if there was any bias (Brackett and Batten, 2022). The JBI checklist included seven questions that were answered with either yes, no, unclear or not applicable. Q3 “Exposure measures in a valid and reliable manner” has been removed since it is not applicable to this study. The articles were then given a quality score calculated by (number of yes responses/7) *100. For this study, the quality included was greater than 60%.
Pathway analysis
Pathway analysis was performed using DIANA tools miRpaths v.3 to search the associated pathways extensively1 (Paraskevopoulou et al., 2013). By using this system, the significantly associated miRNA-regulated pathways were identified. The miRNAs identified through the systematic review were added to the system independently. The bioinformatic analysis was done separately for the miRNAs reported in AD and PD papers. Then the identified common miRNAs were also inputted into the system to analyze the common pathways affected. Based on the DIANA-micro-T-CDS algorithm, miRNA-mRNA interactions were predicted in silicon (Vlachos et al., 2015).
List of predicted targets was further evaluated using ShinyGO 0.77 software for enriched pathway and GO analyses2 (Luo and Brouwer, 2013; Ge et al., 2020; Kanehisa et al., 2021).
Pilot study: sample validation
The study was carried out in accordance with the recommendations of the University of Birmingham Research Ethics Committee (Ethics Ref 18-315). All participants gave written informed consent in accordance with the Declaration of Helsinki. Participants were consented for blood samples from clinical staff from the Human Biomaterials Resource Center, University of Birmingham, at routine clinical appointments undertaken at the Queen Elizabeth Hospital Birmingham. Blood samples were collected in EDTA and frozen whole at-80c until analysis. Male and female participants with a confirmed diagnosis of AD or PD, were enrolled in this study. Diagnosis was made on individual’s history, symptoms, physical exam and evaluation of Mini-Mental State Examination (MMSE). Demographic data is detailed in Table 7.
RNA isolation
Total RNA was isolated from 200 μL of whole blood by using Qiagen miRNeasy Mini Kit (Qiagen, GmbH, Hilden, Germany), according to Qiagen Protocol. RNA was quantified using a NanoDrop® ND-1000 spectrophotometer (Thermo Scientific, Wilmington, DE, United States). An Agilent 2,100 Bioanalyzer (Santa Clara, CA, United States) was used to detect the size distribution of total RNA, as well as determine the quality of the RNA.
Single TaqMan assays
Two differentially expressed miRNA were chosen among the common miRNAs of the systematic reviews. Samples were analyzed by single TaqMan assays (Applied Biosystems, Life Technologies™). Samples were retrotranscribed (Applied Biosystems, Life Technologies™) and RT-qPCR analysis was performed in a Bio-Rad iQ5 Real-time PCR Detection System (Bio-Rad, CA, United States). Expression fold changes were calculated by the 2-ΔΔCT method by using miR-16 as reference gene (Shahid et al., 2019).
Statistical analysis
A non-parametric test (Mann–Whitney U test) was used to compare the level of miRNAs in the independent groups (C vs. AD; C vs. PD). A value of p <0.05 was accepted as significant. One-way Anova with post-hoc Tukey for multiple comparison analysis for the 3 groups was also performed. All statistical analyses were carried on SPSS v.22 (IBM).
Data availability statement
The original contributions presented in the study are included in the article/Supplementary material, further inquiries can be directed to the corresponding author.
Ethics statement
The studies involving humans were approved by the study was carried out in accordance with the recommendations of the University of Birmingham Research Ethics Committee (Ethics Ref 18-315). The studies were conducted in accordance with the local legislation and institutional requirements. The participants provided their written informed consent to participate in this study.
Author contributions
AW, LH, and VD: conceptualization and resources. BA-D, LH, and VD: methodology, formal analysis, data curation, and writing – original draft preparation. VD: validation. BW and AW: writing – review and editing. LH: project administration. LH and VD: funding acquisition. All authors contributed to the article and approved the submitted version.
Funding
This project was funded by The Midland Neuroscience Teaching and Research Fund (Charity number 313446), Queen Elizabeth Hospital Birmingham.
Acknowledgments
The authors would like to acknowledge Zsuzsa Nagy, University of Birmingham, for intellectual input and guidance for the project.
Conflict of interest
The authors declare that the research was conducted in the absence of any commercial or financial relationships that could be construed as a potential conflict of interest.
Publisher’s note
All claims expressed in this article are solely those of the authors and do not necessarily represent those of their affiliated organizations, or those of the publisher, the editors and the reviewers. Any product that may be evaluated in this article, or claim that may be made by its manufacturer, is not guaranteed or endorsed by the publisher.
Supplementary material
The Supplementary material for this article can be found online at: https://www.frontiersin.org/articles/10.3389/fnins.2023.1228927/full#supplementary-material
Footnotes
1. ^http://snf-515788.vm.okeanos.grnet.gr/is last accessed 13 March 2022.
2. ^http://bioinformatics.sdstate.edu/go/ last accessed 19 May 2023.
References
Aksoy-Aksel, A., Zampa, F., and Schratt, G. (2014). MicroRNAs and synaptic plasticity—a mutual relationship. Philos. Trans. R. Soc. Lond. Ser. B Biol. Sci. 369:20130515. doi: 10.1098/rstb.2013.0515
Alqahtani, T., Deore, S. L., Kide, A. A., Shende, B. A., Sharma, R., Dadarao Chakole, R., et al. (2023). Mitochondrial dysfunction and oxidative stress in Alzheimer’s disease, and Parkinson’s disease, Huntington’s disease and amyotrophic lateral sclerosis-An updated review. Mitochondrion 71, 83–92. doi: 10.1016/j.mito.2023.05.007
Bai, X., Tang, Y., Yu, M., Wu, L., Liu, F., Ni, J., et al. (2017). Downregulation of blood serum microRNA 29 family in patients with Parkinson’s disease. Sci. Rep. 7:5411. doi: 10.1038/s41598-017-03887-3
Barbagallo, C., Mostile, G., Baglieri, G., Giunta, F., Luca, A., Raciti, L., et al. (2020). Specific signatures of serum miRNAs as potential biomarkers to discriminate clinically similar neurodegenerative and vascular-related diseases. Cell. Mol. Neurobiol. 40, 531–546. doi: 10.1007/s10571-019-00751-y
Behbahanipour, M., Peymani, M., Salari, M., Hashemi, M. S., Nasr-Esfahani, M. H., and Ghaedi, K. (2019). Expression profiling of blood microRNAs 885, 361, and 17 in the patients with the Parkinson’s disease: integrating interaction data to uncover the possible triggering age-related mechanisms. Sci. Rep. 9, 1–11. doi: 10.1038/s41598-019-50256-3
Brackett, A., and Batten, J. (2022). Ensuring rigor in systematic reviews: part 7, critical appraisal of systematic review quality. Heart Lung 53, 32–35. doi: 10.1016/j.hrtlng.2022.01.008
Businaro, R., Vauzour, D., Sarris, J., Münch, G., Gyengesi, E., Brogelli, L., et al. (2021). Therapeutic opportunities for food supplements in neurodegenerative disease and depression. Front. Nutr. 8:669846. doi: 10.3389/fnut.2021.669846
Cai, M., Chai, S., Xiong, T., Wei, J., Mao, W., Zhu, Y., et al. (2021). Aberrant expression of circulating MicroRNA leads to the dysregulation of alpha-synuclein and other pathogenic genes in Parkinson’s disease. Front. Cell Dev. Biol. 9:695007. doi: 10.3389/fcell.2021.695007
Cao, X. Y., Lu, J. M., Zhao, Z. Q., Li, M. C., Lu, T., An, X. S., et al. (2017). MicroRNA biomarkers of Parkinson’s disease in serum exosome-like microvesicles. Neurosci. Lett. 644, 94–99. doi: 10.1016/j.neulet.2017.02.045
Chapman, M. A. (2014). Interactions between cell adhesion and the synaptic vesicle cycle in Parkinson’s disease. Med. Hypotheses 83, 203–207. doi: 10.1016/j.mehy.2014.04.029
Chen, Y., Gao, C., Sun, Q., Pan, H., Huang, P., Ding, J., et al. (2017). MicroRNA-4639 is a regulator of DJ-1 expression and a potential early diagnostic marker for Parkinson’s disease. Front. Aging Neurosci. 9:232. doi: 10.3389/fnagi.2017.00232
Chen, L., Yang, J., Lü, J., Cao, S., Zhao, Q., and Yu, Z. (2018). Identification of aberrant circulating mi RNA s in Parkinson’s disease plasma samples. Brain Behav. 8:e00941. doi: 10.1002/brb3.941
Cheng, L., Doecke, J. D., Sharples, R. A., Villemagne, V. L., Fowler, C. J., Rembach, A., et al. (2015). Prognostic serum miRNA biomarkers associated with Alzheimer’s disease shows concordance with neuropsychological and neuroimaging assessment. Mol. Psychiatry 20, 1188–1196. doi: 10.1038/mp.2014.127
Crapser, J. D., Spangenberg, E. E., Barahona, R. A., Arreola, M. A., Hohsfield, L. A., and Green, K. N. (2020). Microglia facilitate loss of perineuronal nets in the Alzheimer’s disease brain. EBioMedicine 58:102919. doi: 10.1016/j.ebiom.2020.102919
Cushing, L., Kuang, P. P., Qian, J., Shao, F., Wu, J., Little, F., et al. (2011). miR-29 is a major regulator of genes associated with pulmonary fibrosis. Am. J. Respir. Cell Mol. Biol. 45, 287–294. doi: 10.1165/rcmb.2010-0323OC
De Felice, B., Montanino, C., Oliva, M., Bonavita, S., Di Onofrio, V., Coppola, C., et al. (2020). MicroRNA expression signature in mild cognitive impairment due to Alzheimer’s disease. Mol. Neurobiol. 57, 4408–4416. doi: 10.1007/s12035-020-02029-7
Ding, H., Huang, Z., Chen, M., Wang, C., Chen, X., Chen, J., et al. (2016). Identification of a panel of five serum miRNAs as a biomarker for Parkinson’s disease. Parkinsonism Relat. Disord. 22, 68–73. doi: 10.1016/j.parkreldis.2015.11.014
Dong, Z., Gu, H., Guo, Q., Liang, S., Xue, J., Yao, F., et al. (2021). Profiling of serum exosome MiRNA reveals the potential of a MiRNA panel as diagnostic biomarker for Alzheimer’s disease. Mol. Neurobiol. 58, 3084–3094. doi: 10.1007/s12035-021-02323-y
Dong, L. H., Sun, L., Zhang, W. J., Wang, X. Y., and Li, J. M. (2021). Reduced serum miR-202 may promote the progression of Alzheimer’s disease patients via targeting amyloid precursor protein. Kaohsiung J. Med. Sci. 37, 730–738. doi: 10.1002/kjm2.12391
Fazeli, S., Motovali-Bashi, M., Peymani, M., Hashemi, M. S., Etemadifar, M., Hossein Nasr-Esfahani, M., et al. (2020). Correction: a compound downregulation of SRRM2 and miR-27a-3p with upregulation of miR-27b-3p in PBMCs of Parkinson’s patients is associated with the early stage onset of disease. PLoS One 15:e0244776. doi: 10.1371/journal.pone.0244776
Ge, S. X., Jung, D., and Yao, R. (2020). ShinyGO: a graphical gene-set enrichment tool for animals and plants. Bioinformatics 36, 2628–2629. doi: 10.1093/bioinformatics/btz931
Geekiyanage, H., and Chan, C. (2011). MicroRNA-137/181c regulates serine palmitoyltransferase and in turn amyloid β, novel targets in sporadic Alzheimer’s disease. J. Neurosci. 31, 14820–14830. doi: 10.1523/JNEUROSCI.3883-11.2011
Ghanbari, R., Mosakhani, N., Sarhadi, V. K., Armengol, G., Nouraee, N., Mohammadkhani, A., et al. (2015). Simultaneous underexpression of let-7a-5p and let-7f-5p microRNAs in plasma and stool samples from early stage colorectal carcinoma: supplementary issue: biomarkers for colon cancer. Biomarkers Cancer 7s1, BIC.S25252–BIC.S25248. doi: 10.4137/BIC.S25252
Grimm, M. O., Kuchenbecker, J., Grösgen, S., Burg, V. K., Hundsdörfer, B., Rothhaar, T. L., et al. (2011). Docosahexaenoic acid reduces amyloid β production via multiple pleiotropic mechanisms. J. Biol. Chem. 286, 14028–14039. doi: 10.1074/jbc.M110.182329
Grossi, I., Radeghieri, A., Paolini, L., Porrini, V., Pilotto, A., Padovani, A., et al. (2021). MicroRNA-34a-5p expression in the plasma and in its extracellular vesicle fractions in subjects with Parkinson’s disease: An exploratory study. Int. J. Mol. Med. 47, 533–546. doi: 10.3892/ijmm.2020.4806
Han, L., Tang, Y., Bai, X., Liang, X., Fan, Y., Shen, Y., et al. (2020). Association of the serum microRNA-29 family with cognitive impairment in Parkinson’s disease. Aging (Albany NY) 12, 13518–13528. doi: 10.18632/aging.103458
Hara, N., Kikuchi, M., Miyashita, A., Hatsuta, H., Saito, Y., Kasuga, K., et al. (2017). Serum microRNA miR-501-3p as a potential biomarker related to the progression of Alzheimer’s disease. Acta Neuropathol. Commun. 5:10. doi: 10.1186/s40478-017-0414-z
Hébert, S. S., Horré, K., Nicolaï, L., Papadopoulou, A. S., Mandemakers, W., Silahtaroglu, A. N., et al. (2008). Loss of microRNA cluster miR-29a/b-1 in sporadic Alzheimer’s disease correlates with increased BACE1/β-secretase expression. Proc. Natl. Acad. Sci. 105, 6415–6420. doi: 10.1073/pnas.0710263105
Heydari, M., Hojati, Z., and Dehbashi, M. (2021). Identification of circulating hsa-miR-324-3p and hsa-miR-331-3p exchanges in the serum of Alzheimer’s patients and insights into the pathophysiological pathways. Cell J. 23:211. doi: 10.22074/cellj.2021.7047
Hojati, Z., Omidi, F., Dehbashi, M., and Mohammad Soltani, B. (2021). The highlighted roles of metabolic and cellular response to stress pathways engaged in circulating hsa-miR-494-3p and hsa-miR-661 in Alzheimer’s disease. Iran. Biomed. J. 25, 62–67. doi: 10.29252/ibj.25.1.62
Hou, Y., Dan, X., Babbar, M., Wei, Y., Hasselbalch, S. G., Croteau, D. L., et al. (2019). Ageing as a risk factor for neurodegenerative disease. Nat. Rev. Neurol. 15, 565–581. doi: 10.1038/s41582-019-0244-7
Huang, Z., Lu, L., Jiang, T., Zhang, S., Shen, Y., Zheng, Z., et al. (2018). miR-29b affects neurocyte apoptosis by targeting MCL-1 during cerebral ischemia/reperfusion injury. Exp. Ther. Med. 16, 3399–3404. doi: 10.3892/etm.2018.6622
Jia, L., Zhu, M., Yang, J., Pang, Y., Wang, Q., Li, Y., et al. (2021). Prediction of P-tau/Aβ42 in the cerebrospinal fluid with blood microRNAs in Alzheimer’s disease. BMC Med. 19:264. doi: 10.1186/s12916-021-02142-x
Junn, E., Lee, K. W., Jeong, B. S., Chan, T. W., Im, J. Y., and Mouradian, M. M. (2009). Repression of alpha-synuclein expression and toxicity by microRNA-7. Proc. Natl. Acad. Sci. U. S. A. 106, 13052–13057. doi: 10.1073/pnas.0906277106
Kanehisa, M., Furumichi, M., Sato, Y., Ishiguro-Watanabe, M., and Tanabe, M. (2021). KEGG: integrating viruses and cellular organisms. Nucleic Acids Res. 49, D545–D551. doi: 10.1093/nar/gkaa970
Karube, H., Sakamoto, M., Arawaka, S., Hara, S., Sato, H., Ren, C. H., et al. (2008). N-terminal region of α-synuclein is essential for the fatty acid-induced oligomerization of the molecules. FEBS Lett. 582, 3693–3700. doi: 10.1016/j.febslet.2008.10.001
Kiko, T., Nakagawa, K., Tsuduki, T., Furukawa, K., Arai, H., and Miyazawa, T. (2014). MicroRNAs in plasma and cerebrospinal fluid as potential markers for Alzheimer’s disease. J. Alzheimers Dis. 39, 253–259. doi: 10.3233/JAD-130932
Kim, S. H., Choi, K. Y., Park, Y., McLean, C., Park, J., Lee, J. H., et al. (2021). Enhanced expression of microRNA-1273g-3p contributes to Alzheimer’s disease pathogenesis by regulating the expression of mitochondrial genes. Cells 10:2697. doi: 10.3390/cells10102697
Kim, J., Inoue, K., Ishii, J., Vanti, W. B., Voronov, S. V., Murchison, E., et al. (2007). A MicroRNA feedback circuit in midbrain dopamine neurons. Science 317, 1220–1224. doi: 10.1126/science.1140481
Kim, S., Seo, J. H., and Suh, Y. H. (2004). Alpha-synuclein, Parkinson’s disease, and Alzheimer’s disease. Parkinsonism Relat. Disord. 10, S9–S13. doi: 10.1016/j.parkreldis.2003.11.005
Kumar, S., Vijayan, M., and Reddy, P. H. (2017). MicroRNA-455-3p as a potential peripheral biomarker for Alzheimer’s disease. Hum. Mol. Genet. 26, 3808–3822. doi: 10.1093/hmg/ddx267
Kwon, J. J., Factora, T. D., Dey, S., and Kota, J. (2019). A systematic review of miR-29 in cancer. Mol. Ther. Oncolytics 12, 173–194. doi: 10.1016/j.omto.2018.12.011
Lam, D., Enright, H. A., Cadena, J., Peters, S. K. G., Sales, A. P., Osburn, J. J., et al. (2019). Tissue-specific extracellular matrix accelerates the formation of neural networks and communities in a neuron-glia co-culture on a multi-electrode array. Sci. Rep. 9, 1–15. doi: 10.1038/s41598-019-40128-1
Leng, F., and Edison, P. (2021). Neuroinflammation and microglial activation in Alzheimer disease: where do we go from here? Nat. Rev. Neurol. 17, 157–172. doi: 10.1038/s41582-020-00435-y
Li, L., Ren, J., Pan, C., Li, Y., Xu, J., Dong, H., et al. (2021). Serum miR-214 serves as a biomarker for prodromal Parkinson’s disease. Front. Aging Neurosci. 13:700959. doi: 10.3389/fnagi.2021.700959
Li, K., Wang, Z. Q., Zhang, J. L., and Lv, P. Y. (2020). MicroRNA let-7f protects against H2O2-induced oxidative damage in neuroblastoma cells by targeting AKT-2. Arch. Med. Sci. 16:94490. doi: 10.5114/aoms.2020.94490
Li, H., Yu, L., Li, M., Chen, X., Tian, Q., Jiang, Y., et al. (2020). MicroRNA-150 serves as a diagnostic biomarker and is involved in the inflammatory pathogenesis of Parkinson’s disease. Mol. Genet. Genom. Med. 8:e1189. doi: 10.1002/mgg3.1189
Liberati, A., Altman, D. G., Tetzlaff, J., Mulrow, C., Gotzsche, P. C., Ioannidis, J. P. A., et al. (2009). The PRISMA statement for reporting systematic reviews and meta-analyses of studies that evaluate healthcare interventions: explanation and elaboration. BMJ 339:b2700. doi: 10.1136/bmj.b2700
Liu, X.-J., Zheng, X. P., Zhang, R., Guo, Y. L., and Wang, J. H. (2015). Combinatorial effects of miR-20a and miR-29b on neuronal apoptosis induced by spinal cord injury. Int. J. Clin. Exp. Pathol. 8, 3811–3818.
Ludwig, N., Fehlmann, T., Kern, F., Gogol, M., Maetzler, W., Deutscher, S., et al. (2019). Machine learning to detect Alzheimer’s disease from circulating non-coding RNAs. Genom. Proteom. Bioinform. 17, 430–440. doi: 10.1016/j.gpb.2019.09.004
Lugli, G., Cohen, A. M., Bennett, D. A., Shah, R. C., Fields, C. J., Hernandez, A. G., et al. (2015). Plasma exosomal miRNAs in persons with and without Alzheimer disease: altered expression and prospects for biomarkers. PLoS One 10:e0139233. doi: 10.1371/journal.pone.0139233
Luo, W., and Brouwer, C. (2013). Pathview: an R/Bioconductor package for pathway-based data integration and visualization. Bioinformatics 29, 1830–1831. doi: 10.1093/bioinformatics/btt285
Madadi, S., Saidijam, M., Yavari, B., and Soleimani, M. (2022). Downregulation of serum miR-106b: a potential biomarker for Alzheimer disease. Arch. Physiol. Biochem. 128, 875–879. doi: 10.1080/13813455.2020.1734842
Maldonado-Lasuncion, I., Atienza, M., Sanchez-Espinosa, M. P., and Cantero, J. L. (2019). Aging-related changes in cognition and cortical integrity are associated with serum expression of candidate microRNAs for Alzheimer disease. Cereb. Cortex 29, 4426–4437. doi: 10.1093/cercor/bhy323
Mancuso, R., Agostini, S., Hernis, A., Zanzottera, M., Bianchi, A., and Clerici, M. (2019). Circulatory miR-223-3p discriminates between Parkinson’s and Alzheimer’s patients. Sci. Rep. 9:9393. doi: 10.1038/s41598-019-45687-x
Marques-Aleixo, I., Beleza, J., Sampaio, A., Stevanović, J., Coxito, P., Gonçalves, I., et al. (2021). Preventive and therapeutic potential of physical exercise in neurodegenerative diseases. Antioxid. Redox Signal. 34, 674–693. doi: 10.1089/ars.2020.8075
Miñones-Moyano, E., Porta, S., Escaramís, G., Rabionet, R., Iraola, S., Kagerbauer, B., et al. (2011). MicroRNA profiling of Parkinson’s disease brains identifies early downregulation of miR-34b/c which modulate mitochondrial function. Hum. Mol. Genet. 20, 3067–3078. doi: 10.1093/hmg/ddr210
Nikolaus, S., Antke, C., and Müller, H. W. (2009). In vivo imaging of synaptic function in the central nervous system: II. Mental and affective disorders. Behav. Brain Res. 204, 32–66. doi: 10.1016/j.bbr.2009.06.009
Oliveira, S. R., Dionísio, P. A., Correia Guedes, L., Gonçalves, N., Coelho, M., Rosa, M. M., et al. (2020). Circulating inflammatory miRNAs associated with Parkinson’s disease pathophysiology. Biomol. Ther. 10:945. doi: 10.3390/biom10060945
Oshiro, S., Morioka, M. S., and Kikuchi, M. (2011). Dysregulation of iron metabolism in Alzheimer’s disease, Parkinson’s disease, and amyotrophic lateral sclerosis. Adv. Pharmacol. Sci. 2011:378278, 1–8. doi: 10.1155/2011/378278
Paraskevopoulou, M. D., Georgakilas, G., Kostoulas, N., Vlachos, I. S., Vergoulis, T., Reczko, M., et al. (2013). DIANA-microT web server v5.0: service integration into miRNA functional analysis workflows. Nucleic Acids Res. 41, W169–W173. doi: 10.1093/nar/gkt393
Park, S.-Y., Lee, J. H., Ha, M., Nam, J. W., and Kim, V. N. (2009). miR-29 miRNAs activate p53 by targeting p85α and CDC42. Nat. Struct. Mol. Biol. 16, 23–29. doi: 10.1038/nsmb.1533
Pereira, P., Pedro, A. Q., Queiroz, J. A., Figueiras, A. R., and Sousa, F. (2017). New insights for therapeutic recombinant human miRNAs heterologous production: Rhodovolum sulfidophilum vs Escherichia coli. Bioengineered 8, 670–677. doi: 10.1080/21655979.2017.1284710
Pietro, V. D., O’Halloran, P., and Watson, C. N. (2021). Unique diagnostic signatures of concussion in the saliva of male athletes: the study of concussion in Rugby union through MicroRNAs (SCRUM). Br. J. Sports Med. 55, 1395–1404. doi: 10.1136/bjsports-2020-103274
Poewe, W., Seppi, K., Tanner, C. M., Halliday, G. M., Brundin, P., Volkmann, J., et al. (2017). Parkinson disease. Nat. Rev. Dis. Primers. 3:17013. doi: 10.1038/nrdp.2017.13
Poirier, J., Bertrand, P., Poirier, J., Kogan, S., Gauthier, S., Poirier, J., et al. (1993). Apolipoprotein E polymorphism and Alzheimer’s disease. Lancet 342, 697–699. doi: 10.1016/0140-6736(93)91705-Q
Raghunathan, R., Hogan, J. D., Labadorf, A., Myers, R. H., and Zaia, J. (2020). A glycomics and proteomics study of aging and Parkinson’s disease in human brain. Sci. Rep. 10, 1–9. doi: 10.1038/s41598-020-69480-3
Ruf, W. P., Freischmidt, A., Grozdanov, V., Roth, V., Brockmann, S. J., Mollenhauer, B., et al. (2021). Protein binding partners of dysregulated miRNAs in Parkinson’s disease serum. Cells 10:791. doi: 10.3390/cells10040791
Ryan, B., Joilin, G., and Williams, J. M. (2015). Plasticity-related microRNA and their potential contribution to the maintenance of long-term potentiation. Front. Mol. Neurosci. 8:4. doi: 10.3389/fnmol.2015.00004
Sakakibara, Y., Hirota, Y., Ibaraki, K., Takei, K., Chikamatsu, S., Tsubokawa, Y., et al. (2021). Widespread reduced density of noradrenergic locus coeruleus axons in the app knock-in mouse model of amyloid-β amyloidosis. J. Alzheimers Dis. 82, 1513–1530. doi: 10.3233/JAD-210385
Samii, A., Nutt, J. G., and Ransom, B. R. (2004). Parkinson’s disease. Lancet 363, 1783–1793. doi: 10.1016/S0140-6736(04)16305-8
Sastry, P. S. (1985). Lipids of nervous tissue: composition and metabolism. Prog. Lipid Res. 24, 69–176. doi: 10.1016/0163-7827(85)90011-6
Sengupta, U., and Kayed, R. (2022). Amyloid β, tau, and α-Synuclein aggregates in the pathogenesis, prognosis, and therapeutics for neurodegenerative diseases. Prog. Neurobiol. 214:102270. doi: 10.1016/j.pneurobio.2022.102270
Serpente, M., Fenoglio, C., D’Anca, M., Arcaro, M., Sorrentino, F., Visconte, C., et al. (2020). MiRNA profiling in plasma neural-derived small extracellular vesicles from patients with Alzheimer’s disease. Cells 9:1443. doi: 10.3390/cells9061443
Shahid, S., Shaheen, J., Shahid, W., Akhtar, M. W., and Sadaf, S. (2019). Mir-16-5p as a suitable reference gene for normalization of quantitative real time PCR in acute lymphoblastic leukemia. Pak. J. Zool. 51:747. doi: 10.17582/journal.pjz/2019.51.2.747.754
Shao, N.-Y., Hu, H. Y., Yan, Z., Xu, Y., Hu, H., Menzel, C., et al. (2010). Comprehensive survey of human brain microRNA by deep sequencing. BMC Genomics 11:409. doi: 10.1186/1471-2164-11-409
Sharon, R., Bar-Joseph, I., Frosch, M. P., Walsh, D. M., Hamilton, J. A., and Selkoe, D. J. (2003). The formation of highly soluble oligomers of α-synuclein is regulated by fatty acids and enhanced in Parkinson’s disease. Neuron 37, 583–595. doi: 10.1016/S0896-6273(03)00024-2
Siedlecki-Wullich, D., Català-Solsona, J., Fábregas, C., Hernández, I., Clarimon, J., Lleó, A., et al. (2019). Altered microRNAs related to synaptic function as potential plasma biomarkers for Alzheimer’s disease. Alzheimers Res. Ther. 11:46. doi: 10.1186/s13195-019-0501-4
Smirnova, L., Grafe, A., Seiler, A., Schumacher, S., Nitsch, R., and Wulczyn, F. G. (2005). Regulation of miRNA expression during neural cell specification. Eur. J. Neurosci. 21, 1469–1477. doi: 10.1111/j.1460-9568.2005.03978.x
Tan, W., Gu, Z., Leng, J., Zou,, Chen, H., Min, F., et al. (2019). Let-7f-5p ameliorates inflammation by targeting NLRP3 in bone marrow-derived mesenchymal stem cells in patients with systemic lupus erythematosus. Biomed. Pharmacother. 118:109313. doi: 10.1016/j.biopha.2019.109313
Uwatoko, H., Hama, Y., Iwata, I. T., Shirai, S., Matsushima, M., Yabe, I., et al. (2019). Identification of plasma microRNA expression changes in multiple system atrophy and Parkinson’s disease. Mol. Brain 12:49. doi: 10.1186/s13041-019-0471-2
Vallelunga, A., Iannitti, T., Capece, S., Somma, G., Russillo, M. C., Foubert-Samier, A., et al. (2021). Serum miR-96-5P and miR-339-5P are potential biomarkers for multiple system atrophy and Parkinson’s disease. Front. Aging Neurosci. 13:632891. doi: 10.3389/fnagi.2021.632891
Villa, C., Ridolfi, E., Fenoglio, C., Ghezzi, L., Vimercati, R., Clerici, F., et al. (2013). Expression of the transcription factor Sp1 and its regulatory hsa-miR-29b in peripheral blood mononuclear cells from patients with Alzheimer’s disease. J. Alzheimers Dis. 35, 487–494. doi: 10.3233/JAD-122263
Vlachos, I. S., Zagganas, K., Paraskevopoulou, M. D., Georgakilas, G., Karagkouni, D., Vergoulis, T., et al. (2015). DIANA-miRPath v3. 0: deciphering microRNA function with experimental support. Nucleic Acids Res. 43, W460–W466. doi: 10.1093/nar/gkv403
Wang, Q., Klyubin, I., Wright, S., Griswold-Prenner, I., Rowan, M. J., and Anwyl, R. (2008). αv integrins mediate beta-amyloid induced inhibition of long-term potentiation. Neurobiol. Aging 29, 1485–1493. doi: 10.1016/j.neurobiolaging.2007.03.018
Wang, Z., Qin, W., Wei, C. B., Tang, Y., Zhao, L. N., Jin, H. M., et al. (2018). The microRNA-1908 up-regulation in the peripheral blood cells impairs amyloid clearance by targeting ApoE. Int. J. Geriatr. Psychiatry 33, 980–986. doi: 10.1002/gps.4881
Wang, W. X., Rajeev, B. W., Stromberg, A. J., Ren, N., Tang, G., Huang, Q., et al. (2008). The expression of microRNA miR-107 decreases early in Alzheimer’s disease and may accelerate disease progression through regulation of beta-site amyloid precursor protein-cleaving enzyme 1. J. Neurosci. 28, 1213–1223. doi: 10.1523/JNEUROSCI.5065-07.2008
Wareham, L. K., Liddelow, S. A., Temple, S., Benowitz, L. I., di Polo, A., Wellington, C., et al. (2022). Solving neurodegeneration: common mechanisms and strategies for new treatments. Mol. Neurodegener. 17:23. doi: 10.1186/s13024-022-00524-0
Watson, C. N., Begum, G., Ashman, E., Thorn, D., Yakoub, K. M., Hariri, M. A., et al. (2022). Co-expression analysis of microRNAs and proteins in brain of Alzheimer’s disease patients. Cells 11:163. doi: 10.3390/cells11010163
Wright, S., Malinin, N. L., Powell, K. A., Yednock, T., Rydel, R. E., Griswold-Prenner, I., et al. (2007). α2β1 and αVβ1 integrin signaling pathways mediate amyloid-β-induced neurotoxicity. Neurobiol. Aging 28, 226–237. doi: 10.1016/j.neurobiolaging.2005.12.002
Yang, W.-M., Jeong, H. J., Park, S. Y., and Lee, W. (2014). Induction of miR-29a by saturated fatty acids impairs insulin signaling and glucose uptake through translational repression of IRS-1 in myocytes. FEBS Lett. 588, 2170–2176. doi: 10.1016/j.febslet.2014.05.011
Zago, E., Dal Molin, A., Dimitri, G. M., Xumerle, L., Pirazzini, C., Bacalini, M. G., et al. (2022). Early downregulation of hsa-miR-144-3p in serum from drug-naïve Parkinson’s disease patients. Sci. Rep. 12:1330. doi: 10.1038/s41598-022-05227-6
Zeng, Q., Zou, L., Qian, L., Zhou, F., Nie, H., Yu, S., et al. (2017). Expression of microRNA-222 in serum of patients with Alzheimer’s disease. Mol. Med. Rep. 16, 5575–5579. doi: 10.3892/mmr.2017.7301
Zhang, X., Gong, X., Han, S., and Zhang, Y. (2014). MiR-29b protects dorsal root ganglia neurons from diabetic rat. Cell Biochem. Biophys. 70, 1105–1111. doi: 10.1007/s12013-014-0029-y
Zhou, C., Guo, T., Bai, X., Wu, J. J., Gao, T., Guan, X., et al. (2021). Locus coeruleus degeneration is associated with disorganized functional topology in Parkinson’s disease. NeuroImage Clin. 32:102873. doi: 10.1016/j.nicl.2021.102873
Keywords: microRNA, neurodegeneration, Alzheimer’s disease, Parkinson’s disease, biomarkers, therapeutic targets
Citation: Awuson-David B, Williams AC, Wright B, Hill LJ and Di Pietro V (2023) Common microRNA regulated pathways in Alzheimer’s and Parkinson’s disease. Front. Neurosci. 17:1228927. doi: 10.3389/fnins.2023.1228927
Edited by:
Wang-Xia Wang, University of Kentucky, United StatesReviewed by:
Ursula S. Sandau, Oregon Health and Science University, United StatesLucas Caldi Gomes, Technical University of Munich, Germany
Copyright © 2023 Awuson-David, Williams, Wright, Hill and Di Pietro. This is an open-access article distributed under the terms of the Creative Commons Attribution License (CC BY). The use, distribution or reproduction in other forums is permitted, provided the original author(s) and the copyright owner(s) are credited and that the original publication in this journal is cited, in accordance with accepted academic practice. No use, distribution or reproduction is permitted which does not comply with these terms.
*Correspondence: Lisa J. Hill, bC5qLmhpbGxAYmhhbS5hYy51aw==; Valentina Di Pietro, di5kaXBpZXRyb0BiaGFtLmFjLnVr