- 1Department of Molecular Medicine and Surgery and Centre of Molecular Medicine, Karolinska Institutet, Stockholm, Sweden
- 2Department of Applied Physics and Science for Life Laboratory, KTH Royal Institute of Technology, Stockholm, Sweden
- 3Department of Clinical Genetics, Karolinska University Hospital, Stockholm, Sweden
Delta-catenin (CTNND2) is an adhesive junction associated protein belonging to the family of p120 catenins. The human gene is located on the short arm of chromosome 5, the region deleted in Cri-du-chat syndrome (OMIM #123450). Heterozygous loss of CTNND2 has been linked to a wide spectrum of neurodevelopmental disorders such as autism, schizophrenia, and intellectual disability. Here we studied how heterozygous loss of ctnnd2b affects zebrafish embryonic development, and larvae and adult behavior. First, we observed a disorganization of neuronal subtypes in the developing forebrain, namely the presence of ectopic isl1-expressing cells and a local reduction of GABA-positive neurons in the optic recess region. Next, using time-lapse analysis, we found that the disorganized distribution of is1l-expressing forebrain neurons resulted from an increased specification of Isl1:GFP neurons. Finally, we studied the swimming patterns of both larval and adult heterozygous zebrafish and observed an increased activity compared to wildtype animals. Overall, this data suggests a role for ctnnd2b in the differentiation cascade of neuronal subtypes in specific regions of the vertebrate brain, with repercussions in the animal’s behavior.
1. Introduction
CTNND2, also known as delta-catenin or neural plakophilin-related armadillo protein (NPRAP), is the only member of the catenin family of proteins expressed exclusively in the nervous system (Ho et al., 2000). Genetic changes affecting this gene have been shown to result in various neurodevelopmental disorders. The most known is Cri-du-chat syndrome (OMIM #123450), resulting from a large deletion of chromosome 5p with the hallmark features including an unusual high-pitched cry at birth, facial dysmorphology, poor growth, and severe intellectual disability. Interestingly, individuals in whom CTNND2 was included in the deleted region showed more severe neurological symptoms (Medina et al., 2000; Mainardi et al., 2001; Sardina et al., 2014). Genetic changes affecting CTNND2, both structural variants and single nucleotide variants, have been associated with autism-spectrum disorder (Girirajan et al., 2013; Turner et al., 2015; Miller et al., 2020; Tuncay et al., 2022), intellectual disability with or without dyslexia-like learning difficulties (Belcaro et al., 2015; Hofmeister et al., 2015), neurodevelopmental delay (Asadollahi et al., 2014), attention deficit hyperactivity disorder or ADHD (Adegbola et al., 2020), depression (Kang et al., 2021), cerebral palsy (McMichael et al., 2014), anxiety (Nivard et al., 2014), epilepsy (van Rootselaar et al., 2017), and schizophrenia (Vrijenhoek et al., 2008). Even though it is not entirely understood how heterozygous loss or expression of CTNND2 variants result in such disorders, research done in in vitro systems, such as neurons in culture, or animal models, have provided with some understanding of the possible role of CTNND2 in neurogenesis. In brief, CTNND2 plays a role in neuronal differentiation (Jones et al., 2002; Lee et al., 2016) and dendrite and synapse formation and maintenance (Israely et al., 2004; Kim et al., 2008; Arikkath et al., 2009; Matter et al., 2009; Turner et al., 2015; Gilbert and Man, 2016; van Rootselaar et al., 2017; Baumert et al., 2020; Ligon et al., 2020). These are, at least in part, regulated by CTNND2 via its interaction with the actin cytoskeleton, the ubiquitin proteasome pathway, and autophagy (Bareiss et al., 2010; Lee et al., 2016; Ligon et al., 2020). Expression studies in mice showed that CTNND2 expression is high in neuronal precursors, downregulated during neuronal migration, and then increases again in post-mitotic neurons (Ho et al., 2000), supporting a dynamic role for CTNND2 in neurogenesis.
CTNND2 interacts with proteins such as N-Cadherin (Arikkath et al., 2009; Yuan et al., 2015; Gilbert and Man, 2016), Presenilin (Kim et al., 2006), RhoGTPases (Abu-Elneel et al., 2008; Lu et al., 2016; Donta et al., 2022), and SHANK3 (Hassani Nia et al., 2020). Interestingly, de novo point mutations and deletions affecting SHANK3 causes Phelan-McDermid syndrome (OMIM # 606232), a developmental disorder characterized by neonatal hypotonia, global developmental delay, severely delayed speech, and autistic behavior (Uchino and Waga, 2013; Ricciardello et al., 2021).
Studies in mice have shown that loss of CTNND2 results in decreased learning ability and autism-like behaviors such as reduced sociability and increased anxiety (Israely et al., 2004; Wang et al., 2021). Conversely, overexpression of CTNND2 in mice resulted in increased sociability and reduced anxiety (Ryu et al., 2019). We have previously shown that morpholino knock down of ctnnd2b but not ctnnd2a results in misplaced neural precursors. Here, we have generated stable Crispr/Cas9 ctnnd2a and ctnnd2b mutants and show that heterozygous loss of ctnnd2b affects the brain patterning of zebrafish embryos and the swimming behavior of both larvae and adults.
2. Materials and methods
2.1. Zebrafish husbandry
Adult zebrafish were maintained on a 14 h day/ 10 h night cycle at the Karolinska Institutet zebrafish core facility, KM-C. Embryos were produced by light-induced spawning, collected, and maintained in an incubator at 28.5°C until used in experiments. Tg(isl1:GFP)rw0 transgenic (isl:GFP for short) and TL strains were used. All experiments were performed according to standard procedures and with permission from the Stockholm Ethical Board for Animal Experiments (ethics permit numbers 13063-2017, 14049-2019, and 14469-2022).
2.2. CRISPR/Cas9-mediated knockout of ctnnd2 orthologues
Zebrafish mutant lines were established using a protocol adapted from Varshney et al. Varshney et al. (2015). sgRNAs and Cas9 protein mix was injected into 1-cell embryos and efficacy was tested using a PCR-STAT method (Carrington et al., 2015). The target sequence of the sgRNAs were in exon 6 of ctnnd2a 5’ GGTTCAGCGAGCGATACACC 3′ and exon 5 of ctnnd2b 5’ GGTGGAAGCGCCCGGGCCAG 3′. Primers used for genotyping were ctnnd2a-F 5’ TCACTCTCCTTACAGGTGACA 3′, ctnnd2a-R 5’ GGTTGCGTAGCCAGGTGTAT 3′, ctnnd2b-F 5’ CCAGGTCCTGAACTTTCTGC 3′, and ctnnd2b-R 5’ GGTCCTTCTGGATGCTGTCC 3′. Embryos were raised and founders identified by PCR and Sanger sequencing.
The stable ctnnd2 mutant lines presented with short indels in the coding sequence resulting in a frameshift and premature termination of the protein sequence: ctnnd2a c.812_819del, p.S270TfsX61 and ctnnd2b c.1363_1369del, p.T456RfsX38 (Supplementary Figures S1A, S2). Non-sense mediated decay of mutant allele was evaluated using primers flanking the indel in ctnnd2b 5’ GACATTCCCTCGCGCTGGTTAC 3′ and 5’ CCCTCCAGCCAAATTCCCTGGG 3′ (forward and reverse primers, respectively). Amplification of β-actin was used as the reference gene with the primers 5’ CCGTGACATCAAGGAGAAGCT 3′ and 5’ TCGTGGATACCGCAAGATTCC 3′ (Supplementary Figure S1B).
Founders were outcrossed to wildtype or Tg (isl:GFP)rw0 zebrafish and raised to adulthood. Given the lack of any severe phenotype, homozygous mutants were maintained as adults and outcrossed to generate heterozygous embryos used in the experiments.
2.3. Immunofluorescence and In situ hybridization
Embryos were fixed in 4% paraformaldehyde (PFA, HistoLabs) overnight at 4°C. Whole larvae or dissected brains were processed for immunofluorescence using standard protocol (Devine and Key, 2003). Primary antibody used was rabbit anti-GABA (Sigma, Cat# A2052, RRID: AB_477652) and secondary antibodies used were goat anti-rabbit Alexa 594 (Molecular Probes, Cat# A11012, RRID: AB_141359) and donkey anti-goat Alexa 546 (Molecular Probes, Cat#A11056, RRID: AB_142628). In situ staining was performed as previously described (Thisse and Thisse, 2008). The nkx2.1 riboprobe was synthesized using the DIG labeling kit (Roche) using the following PCR template with a T7 RNA polymerase site attached to the 5′ end of the reverse primer (forward primer: 5’ TTGCCTCGTACAGACAACCC 3′, reverse primer: 5’ TAATACGACTCACTATAGGGGTCACCACGTCCTGCCATAA 3′). Embryos were mounted in gelvatol for imaging.
2.4. Imaging and data analysis
For confocal imaging, transgenic embryos fixed in 4% PFA or immunolabeled embryos were mounted rostrally or laterally in gelvatol mounting medium and imaged using a Zeiss LSM 700,800 or Olympus FV1000. Images where blinded and isl:GFP or GABA stained cells counted throughout individual confocal sections. Cell numbers were subsequently corelated back to the genotype of the embryos as identified by Sanger sequencing. Quantifications from multiple experiments/crosses were pooled together. For GABA-positive cell quantifications, data was obtained from two separate crosses of double heterozygous adults and one cross of double homozygous adults.
For light sheet imaging, 31hpf embryos were anesthetized in tricaine (MS222) and mounted in 1% low melting point agarose containing 1:2000 F-Z 050 fluorescent microspheres (Merck) in a glass capillary. The agarose cylinder was extruded into a sample chamber containing E3 medium at 28.5°C. Two-channel images were acquired using a Light Sheet Z.1(Carl Zeiss, Germany) with a water dipping 20X detection objective (W-Plan-APOCHROMAT-1.0NA) and dual side 10X illumination objectives (LSFM, 0.2NA). Samples were illuminated from two sides and Z-stacks were acquired from 3 angles (covering 90°) every 20 min for 16 h. Data from multiple angles was registered and deconvolved in the Multiview Reconstruction Fiji plugin (Preibisch et al., 2010). Briefly, beads were detected in one channel and used to register views and correct drift. Transformations were duplicated to the GFP channel and images were fused by multi-view deconvolution, performed on the GPU (Preibisch et al., 2014). Deconvolved images were rendered, and movies created in Imaris 9.0 (Bitplane).
2.5. Larval behavior analysis
Wildtype and ctnnd2b+/− embryos were produced and maintained in an incubator with light cycle (14 h light, 10 h dark) for 5 days, i.e., until the day of recording. The day before the experiment, embryos were placed in 48-well plates, one embryo per well, with a total of 1 mL of E3 medium per well. Recording was performed in a DanioVision Observation Chamber controlled by the EthoVision XT software (version 16, Noldus).
The visual motor response test was used to evaluate larvae behavior. Briefly, following a 5-min acclimatation in light, larvae were exposed to 5 iterations of 10 min light (100% light – equivalent to 3,000 LUX) and 10 min darkness (0% light). A video recording of larvae movements was acquired at a rate of 50 frames per second, resulting in evenly spaced frames every 20 milliseconds. Automatic single point detection was used to track individuals. After each recording, the video file was saved for offline inspection. A preliminary filtering using the distance per second was performed, with distances larger than 5 mm being discarded, as they may relate to ineffective larva detection. This was investigated by visualizing the trial video and understanding the cause of abnormal values. Data was analyzed using own scripts written in Python.
For thigmotaxis evaluation, each well was subdivided into an outer and inner zone. The inner zone was defined by a circle with 6.5 mm diameter at the center of the well (total diameter of the well is 10 mm). Total distance moved in the second dark iteration for each zone of the arena was calculated.
2.6. Adult behavior analysis
Five months old wildtype and ctnnd2b+/− zebrafish were used to assess response to a new environment, known as the novel tank test. Adult zebrafish were maintained according to standard procedures, in 3 L tanks at a maximum density of 20 fish per tank, until the time of the experiment. Recording was done between 9 am-12 pm in consecutive days and, following the recording, animals were placed in new tanks. A total of 17 animals per genotype were used in the test. For the recording, two tanks (17x11x19.5 cm LxWxH) were placed side by side and filled with water. The sides of the tanks were taped to avoid external visual stimulation. A camera was placed in front of the tanks to record the animals’ lateral and vertical movements. Recording started before the fish were transferred to the tanks and set to 16 min, to guarantee a full 15-min recording of every animal. For each recording, one fish from each genotype was used and were alternated between the recording tanks. Both genders were used. Tanks were rinsed and filled with fresh water between recordings. Tracking of the movements was performed using the Ethovision XT software and corrected when needed. For the analysis, the tank was subdivided into bottom and top zones (bottom corresponding to the bottom 1/3 of the tank and top corresponding to the rest of the tank).
2.7. Statistical analysis
Quantifications were statistically compared with GraphPad Prism version 9. For quantifications that fit the normal distribution of values, parametric tests were used. If a normal distribution of values was not observed, non-parametric tests were performed instead. Tests used are named in the results section. Statistical significance was considered when p < 0.05. Non-significant differences were not plotted in the graphs.
3. Results
3.1. Loss of ctnnd2b but not ctnnd2a results in misplaced neurons
The zebrafish genome contains two orthologs for the human CTNND2, ctnnd2a and ctnnd2b, most likely due to a genome-wide duplication event that occurred during teleost evolution (Glasauer and Neuhauss, 2014). We have previously shown that reduction of ctnnd2b expression but not ctnnd2a using antisense morpholinos in zebrafish embryos results in ectopic isl1-expressing neurons in the forebrain (Hofmeister et al., 2015). The ectopic Isl1:GFP-positive cells are found in the optic recess region (ORR), which can be identified as a distinct morphological entity in the brain of 48 h post fertilization (hpf) embryos (Figure 1A), located between the telencephalon (tel) and the hypothalamus (hyp), and flanked by the anterior and the postoptic commissure (AC and POC, respectively) (Affaticati et al., 2015).
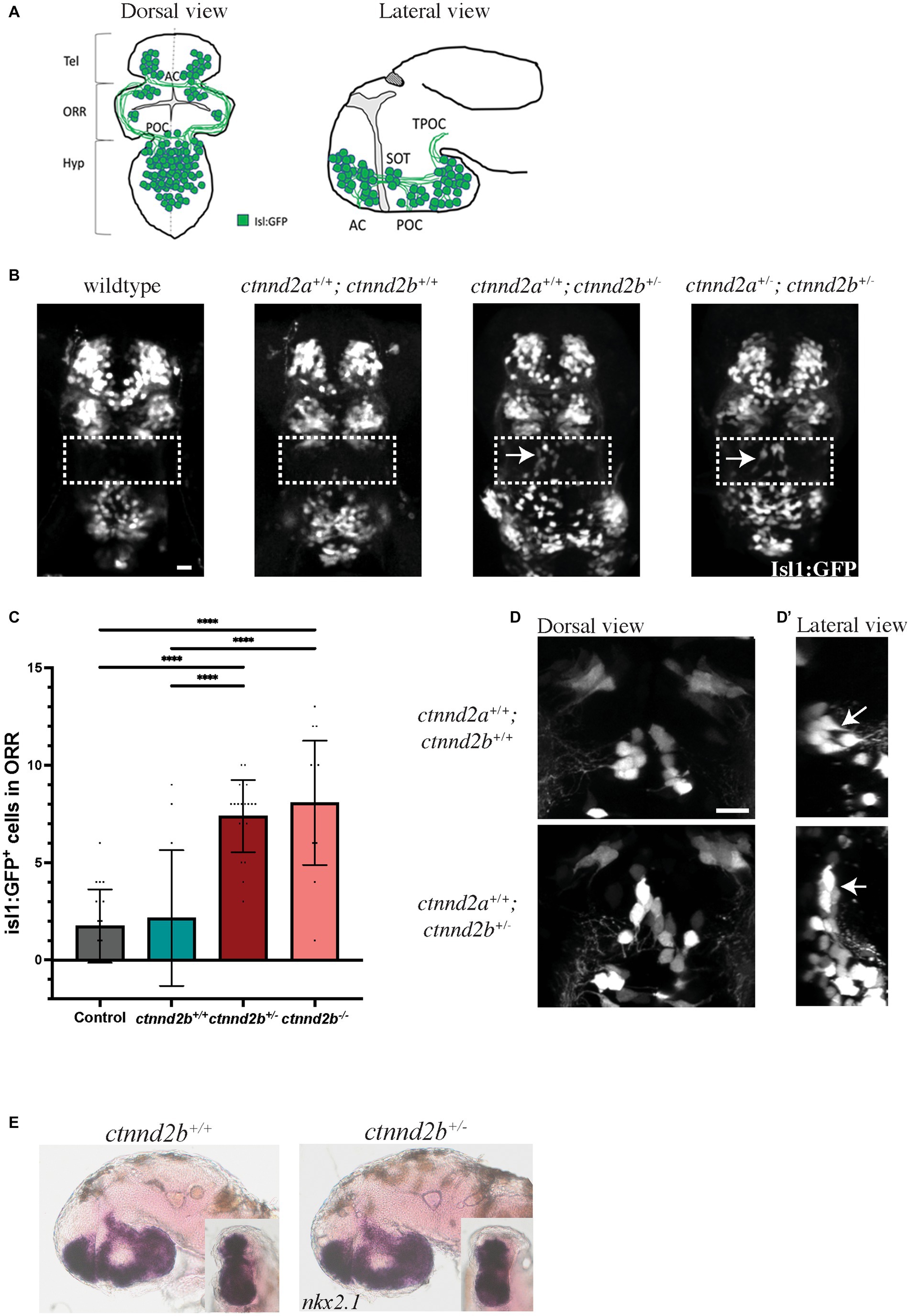
Figure 1. FIGURE 1 (Continued)(A,B) Schematic illustration of the isl1:GFP-expressing neuron population in the forebrain of wildtype 48 hpf zebrafish embryos. (B) Heterozygous loss of ctnnd2b results in ectopic isl1:GFP-expressing cells in the ORR (arrows), not detected in control embryos. Wildtype: embryos obtained from an incross of Tg(isl1:GFP) line; ctnnd2a+/+,ctnnd2b+/+: non-carriers from the incross of the ctnnd2a+/−,ctnnd2b+/− line. (C) Quantification of Isl1:GFP-positive neurons in the ORR confirmed that heterozygous and homozygous loss of ctnnd2b result in ectopic Isl1-positive cells in the region. (D) In ctnnd2b+/− embryos the ectopic Isl1:GFP-positive cells display a vertical rather than horizontal orientation (arrows, lateral view in D′) and the cluster seems more disorganized when compared to these cells in control (ctnnd2a+/+,ctnnd2b+/+) embryos. (E) Control and ctnnd2b+/− 54 hpf embryos show no difference in nkx2.1 expression, investigated in lateral and rostral (inset) views. Dashed box: ORR; Tel: telencephalon; ORR: optic recess region; Hyp: hypothalamus; AC: anterior commissure; POC: post-optic commissure; SOT: supraoptic tract; TPOC: tract of the POC; ****p < 0.0001. Scale bar =10 μm.
To further investigate these findings, we created stable knockout lines for ctnnd2 with CRISPR/Cas9. The changes generated by the genome editing resulted in frameshift mutations that introduced a premature stop codon to the coding sequence: ctnnd2a c.812_819del p.S270TfsX61, and ctnnd2b c.1363_1369del p.T456RfsX38 (Supplementary Figures S1, S2). For nomenclature simplicity, the mutant allele is represented by ‘-‘symbol, e.g., ctnnd2b−/− representing the homozygous ctnnd2b null genotype. Similarly to the knockdown of ctnnd2 with morpholinos, heterozygous loss of ctnnd2b, but not ctnnd2a, resulted in ectopic isl1-expressing neurons in the ORR, seen in 54 hpf embryos (arrows, Figure 1B, Supplementary Figure S3A). Moreover, both heterozygous and homozygous ctnnd2b null embryos showed the same phenotype (Supplementary Figure S3A). Quantification of the number of Isl1:GFP-positive cells in the ORR showed a significant increase in both ctnnd2b+/− and ctnnd2b,−/− (n = 13–21 embryos per group, one-way ANOVA, ****p < 0.0001, Figure 1C). Interestingly, confocal analysis at higher magnification showed that the ectopic neurons display a vertical rather than horizontal orientation and the cluster seems disorganized compared to neurons in wildtype embryos (Figure 1D, lateral view D′, arrows). Ectopic neurons are still present at later stages, e.g., 6 days post fertilization (dpf) (arrows, Supplementary Figure S3B), suggesting the mispatterning of the brain may be maintained throughout development and into adulthood.
3.2. Heterozygous loss of ctnnd2b results in abnormal GABAergic neurogenesis
In mammals, ISL1 has been shown to form a complex with LHX3 to promote cholinergic gene expression in basal forebrain cholinergic neurons (Cho et al., 2014). This neuronal population is involved in attention, memory, reward pathways, and motor activity (Allaway and Machold, 2017). Forebrain cholinergic neurons have an embryonic origin in the ventral telencephalon, in a region that expresses the transcription factor nkx2.1. The nkx2.1 expression domain in zebrafish where the isl1-expressing forebrain cells reside encompasses the ventral telencephalon, optic recess region, ventral diencephalon, and hypothalamus (Manoli and Driever, 2014). In situ analysis revealed no difference in patterning of the nkx2.1 expression region between wildtype and heterozygous embryos (Figure 1E), suggesting normal patterning of this region at early developmental stages.
The ventral telencephalon is also the birthplace of GABAergic neurons. The choice as to whether nkx2.1 expressing cells in the ventral telencephalon become GABAergic or cholinergic is due to a distinct expression of a combination of transcription factors. Neurons fated to become GABAergic express high levels of the transcription factor lhx6 (Liodis et al., 2007). Conversely, in post-mitotic nkx2.1 positive neurons expressing high levels of isl1 and lhx8, the expression of lhx6 is suppressed and these become forebrain cholinergic neurons (Elshatory and Gan, 2008; Cho et al., 2014). Thus, the differentiation into GABAergic neurons is inhibited by the expression of isl1 (Fragkouli et al., 2009). We therefore decided to assess the expression of GABA in ctnnd2b mutant embryos. As expected, GABAergic neurons in diencephalon and ORR are intermingled but rarely overlapping with Isl1:GFP-positive cells (ctnnd2a+/+,ctnnd2b+/+), and ectopic Isl1-positive neurons also lack GABA expression (arrows, Figures 2A,B). Moreover, the population of GABA-positive neurons in the ORR appears to be more loosely packed together in ctnnd2b+/− embryos than in controls (arrowheads, Figure 2A). Interestingly, quantification of GABAergic neurons throughout the images taken at the ORR confirmed a reduction in GABA-positive neurons in heterozygous ctnnd2b embryos in addition to the cell disorganization (n = 5–20 embryos, Mann–Whitney test, **p = 0.0017; Figure 2C).
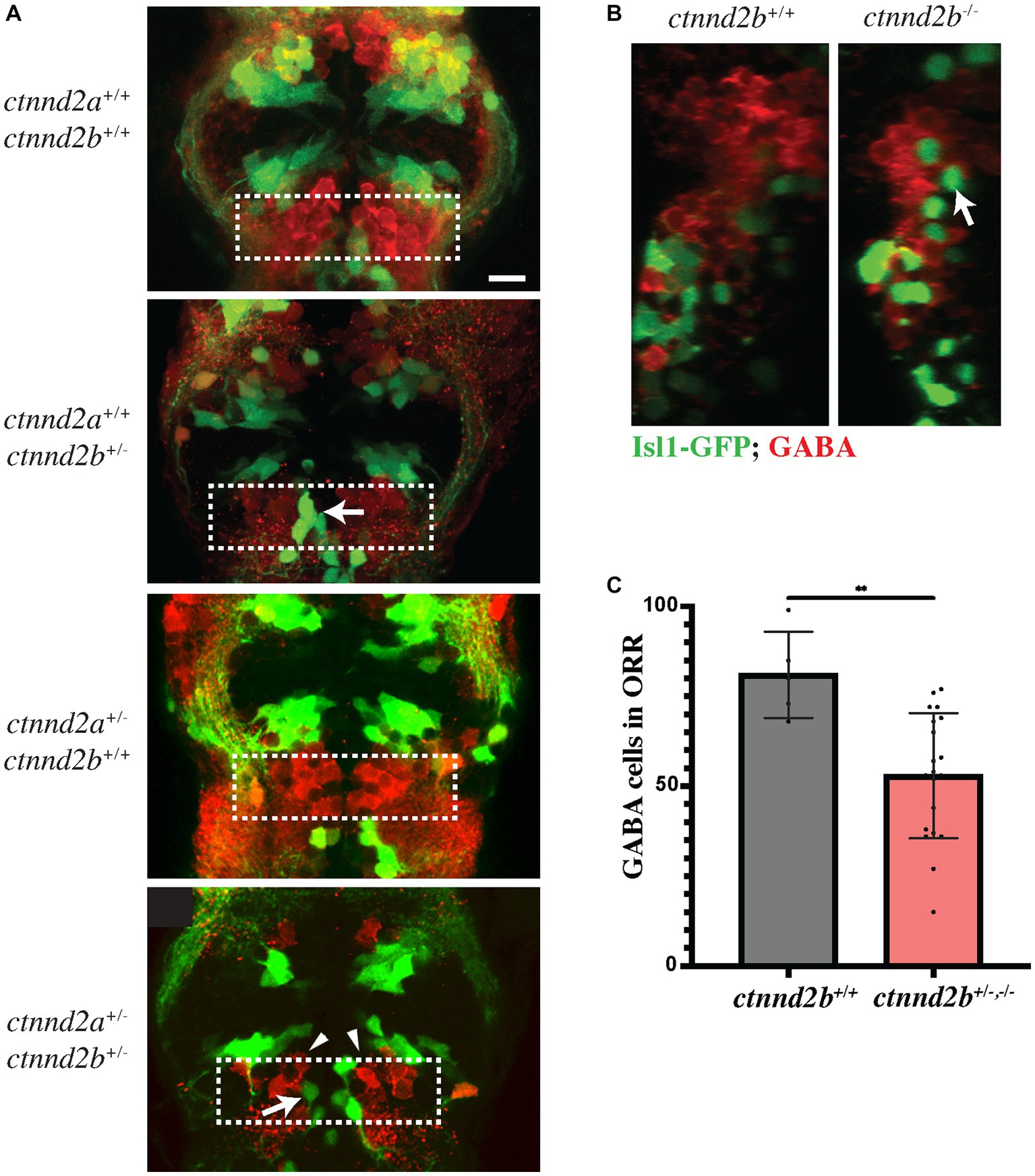
Figure 2. (A) Heterozygous loss of ctnnd2b affects GABA neuronal specification in the ORR, resulting in a significant decrease in the number and organization of GABA-positive cells in the ORR (dashed box, arrowheads in A; C). (A,B) Isl:GFP-positive cells are negative for GABA (arrows). **p = 0.0017. Scale bar =10 μm.
3.3. Increased isl1-expressing cells at ORR is due to abnormal neuronal specification
To address if the observed phenotype was a result of migration defects or abnormal specification, we imaged embryos between 32 and 48 hpf. Given the lack of phenotypes of embryos carrying the ctnnd2a+/− genotype, the following experiments were performed only on ctnnd2b wild type and mutant embryos (on a ctnnd2a+/+ background). Most patients, including those we previously reported (Hofmeister et al., 2015) present with heterozygous loss of CTNND2. Since both ctnnd2b+/− and ctnnd2b−/− zebrafish embryos developed the same phenotype, we continued the experiments on ctnnd2b+/− embryos. Staging was additionally assessed by growth of visible axon tracts such as the trigeminal and post-optic commissure. Initially, the number of isl1:GFP-expressing cells were similar between transgenic control and heterozygous embryos (ctnnd2b+/−) (compare Figures 3A–A’, Supplementary Movie S1). However, as development proceeds, we see a progressive increase in the number of Isl1:GFP-positive cells arising in the ORR of ctnnd2b+/− embryos (compare Figures 3B,C–B’,C’, Supplementary Movie S1) that are not traceable to other regions of the embryo from earlier timepoints, while in the control there were only a very limited number of Isl1:GFP-positive cells detected (compare Figures 3D–D’, Supplementary Movie S1). The finding suggests that in the mutant embryos more cells from the ORR become committed to the Isl1-lineage compared to control embryos.
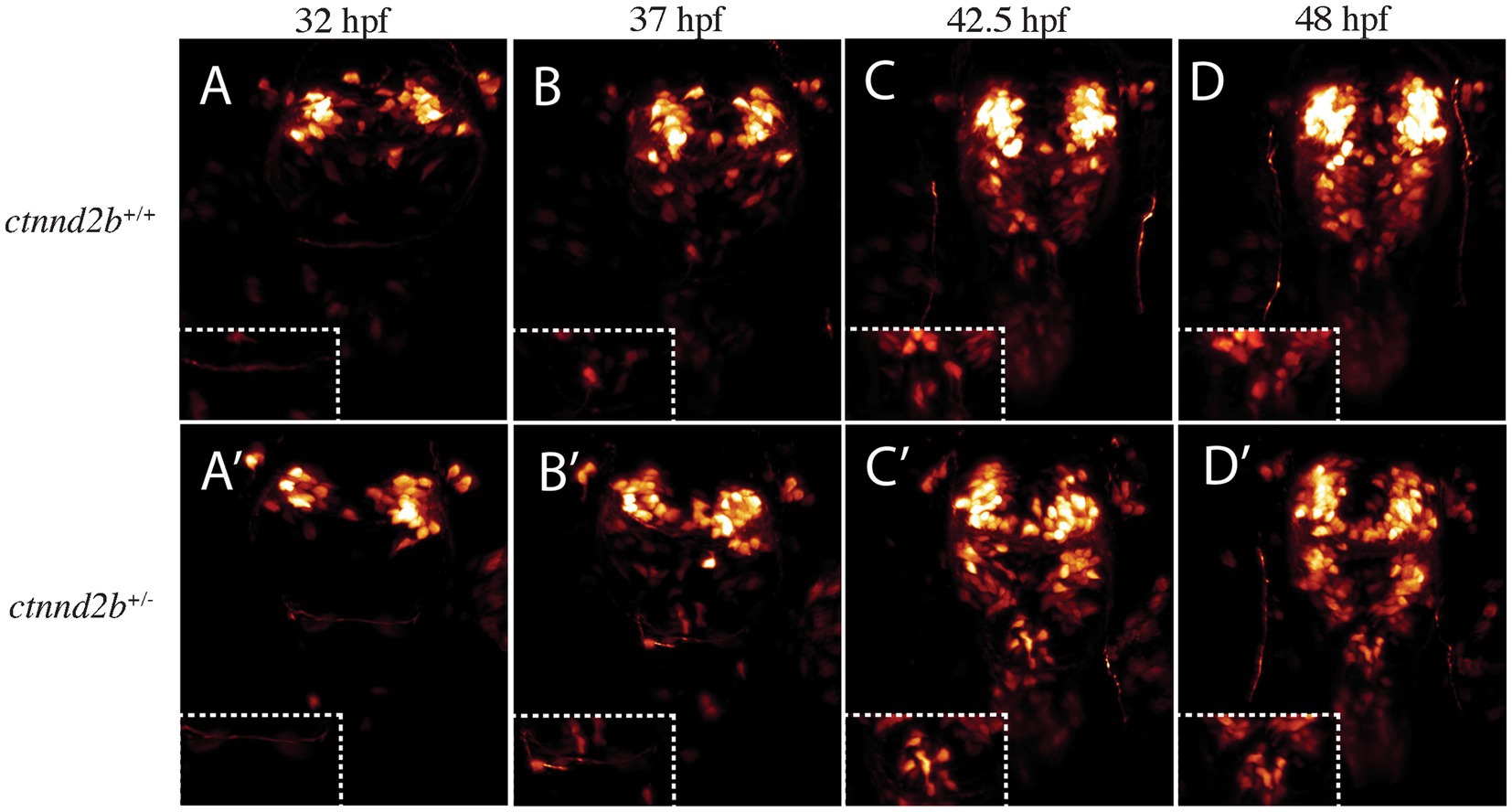
Figure 3. Increase in Isl1-positive cells is likely due to misspecification rather than migration defects. 3D renders of timelapse imaged ctnnd2b+/+ (A-D) and ctnnd2b+/− embryos (A’-D’) with highlighted ORR (insets). Embryos at 32 hpf, prior to appearance of neurons at ORR, are shown in (A,A’). Isl1:GFP-positive neurons start appearing at the ORR by 37 hpf (B,B’), and detected at 42.5 hpf (C,C’) and 48 hpf (D,D’). Insets show the ORR at the corresponding timepoints. By the end of recording only a few isl1:GFP-expressing cells are found in ctnnd2b+/+ embryos compared to ctnnd2b+/− embryos.
3.4. Reduction in Ctnnd2b affects swimming behavior
To investigate if and how the changes in the brain patterning of ctnnd2b+/− embryos may affect their swimming behavior, we performed the visual motor response test on 5 dpf larvae (Vaz et al., 2019). Briefly, larvae were exposed to five consecutive cycles of light and dark and the distance traveled throughout the test was determined. Zebrafish larvae are known to be more active in the dark than in light conditions, which we also observed (Figure 4A). Interestingly, ctnnd2b+/− presented with a larger increase in swimming activity when exposed to darkness compared to wildtype larvae (Figures 4A,B). Quantification of the distance moved for each light condition (average of the total distance moved during each 10-min light condition) per fish was calculated. We found a statistically significant increase in the distance moved in both light and dark conditions by ctnnd2b+/− than wildtype larvae (nwildtype = 138 and n ctnnd2b+/− = 133 embryos, Mann–Whitney test, ***p = 0.0006, ****p < 0.0001; Figure 4B). Another swimming behavior that can be evaluated is thigmotaxis, which is the preference for larvae to swim along the walls of the dish rather than in the center, that correlates with anxiety (Colwill and Creton, 2011; Schnorr et al., 2012). To assess if ctnnd2b+/− larvae presented with a different thigmotaxic behavior when compared to wildtype larvae, the arena was subdivided into an outer and inner zone (Figure 4C). The total distance traveled within each zone during one dark iteration of the visual motor response test was calculated. Mutant larvae presented with an overall increased activity in both zones compared to wildtype larvae (nwildtype = 140 and n ctnnd2b+/− = 137 embryos, Kruskal-Wallis test, **p = 0.0033, ****p < 0.0001; Figure 4C). While wildtype embryos showed no difference between the distance moved in the outer and inner zones (p = 0.0663), the ctnnd2b+/− larvae moved significantly more in the outer zone compared to the inner zone of the arena (****p < 0.0001; Figure 4C), suggesting an increased anxiety-like behavior from the ctnnd2b+/− larvae.
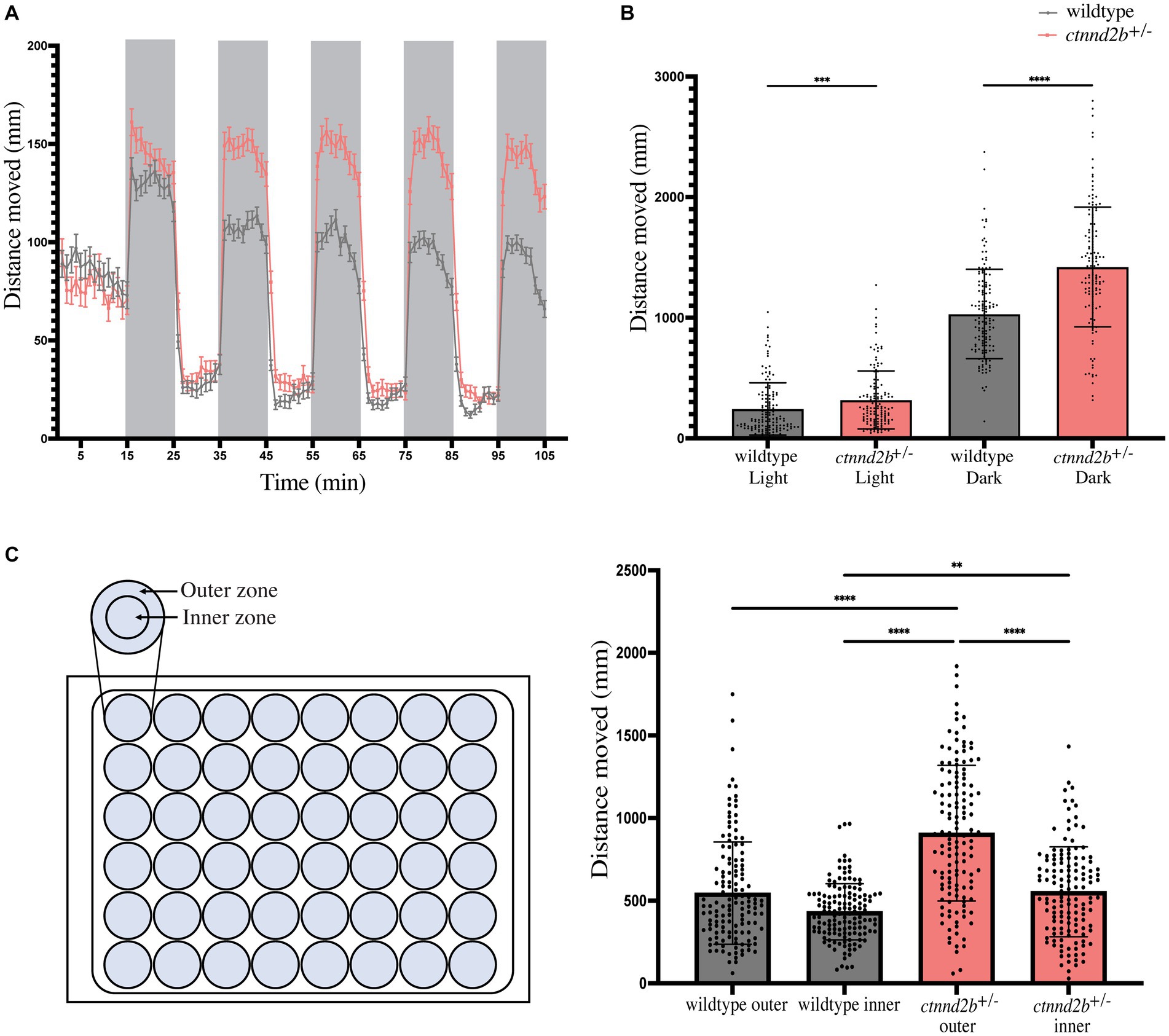
Figure 4. (A) Assessment of the swimming performance using the visual motor response test showed an increase in activity from ctnnd2b+/− 5 dpf larvae compared to wildtype larvae when exposed to darkness (shaded time windows). (B) Quantification of total distance moved per light condition showed that mutant larvae moved significantly move in both light and dark environments. (C) Evaluation of swimming patterns during a dark iteration of the test showed a statistically significant increase preference to swimming in the outer zone of the arena, or thigmotaxis, from the ctnnd2b+/− larvae compared to wildtype controls. **p = 0.0033, ***p = 0.0006, ****p < 0.0001.
Next, we wanted to assess whether adult behavior was also affected. To do so, the novel tank test was performed, where one fish was transferred to a new tank and the swimming behavior was recorded. The total distance moved per fish for the 15 min of recording analyzed was similar between genotypes (n = 17 fish per genotype, t-test, p = 0.5829; Figure 5A). Even though there was no difference in the total distance swam by the animals, we investigated whether there was a preference for any specific zone in the tank. To address this question, the tank area was divided into bottom zone, the lower third of the tank height, and top zone, the upper area of the tank (Figure 5B). Frequency (time spent on each zone, not shown), distance, and number of transitions between the zones were calculated. We found a tendency for ctnnd2b+/− zebrafish to swim more in the top zone compared to wildtype animals (dashed lines, Figure 5C), however the variability between individuals with the same genotype is large, hence the differences are not statistically significant (two-way ANOVA, p = 0.4673 and p = 0.0761 for bottom and top zones; Figure 5C). Similar findings were observed when the number of transitions between the bottom and top zones were assessed (two-way ANOVA, p ≈ 0.16 for both bottom-to-top and top-to-bottom transitions; Figure 5D). It has been widely reported that male patients present with more severe changes in behavior than females. Therefore, we analyzed males and females separately. We found no significant difference between the distance moved throughout time between females from each genotype (two-way ANOVA, p = 0.9283 and p = 0.06961 for bottom and top zones, respectively) and in the bottom zone between males (two-way ANOVA, p = 0.2077), due to a large individual variability. However, mutant males move more in the top zone of the tank than wildtype males (two-way ANOVA, *p = 0.0252; Figure 5E), resembling a reduction in novelty-induced anxiety behaviors previously reported.
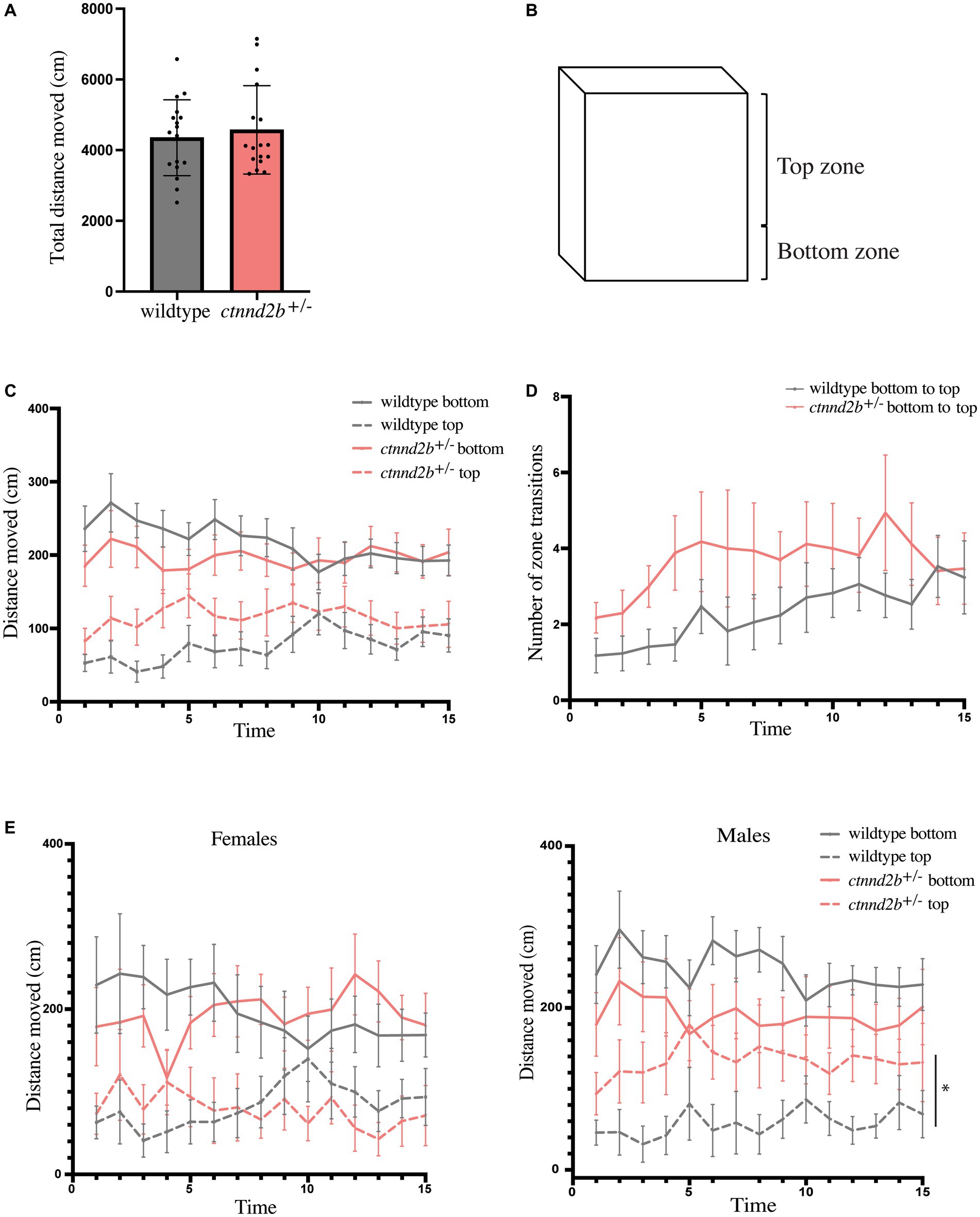
Figure 5. Behavior of adult individuals was evaluated using the novel tank test. (A) Total distance moved for the duration of the test was similar between ctnnd2b+/− and wildtype individuals. (B) Anxiety-like behavior was tested by defining a top and bottom zone of the tank used in the test. Quantification of the distance moved per minute per zone (C) and the number of bottom-to-top transitions during the test (D) showed no difference between genotypes. (E) While no difference in the distance moved per zone was found between females, heterozygous mutant males show a statistically significant increase in the distance moved in the top zone of the tank compared to wildtype individuals. Graphs: mean ± SEM, *p = 0.0252.
4. Discussion
To study how Ctnnd2 affects zebrafish development and behavior we generated and investigated a stable zebrafish knock out line. Using this approach, opposed to transient knock down of gene expression, we were able to phenotype older embryos, larvae, and adult individuals.
Our results show that, in developing embryos, ctnnd2b haploinsufficiency results in ectopic specification of Isl1-positive cells at the ORR. Since in this region of the brain cholinergic and GABAergic neurons are specified, it is plausible that an ectopic specification of Isl1-positive cells affect the neuronal pattering in the ORR. Previous publications have shown that Isl1, via its interaction with Lhx8 in the forebrain, promotes cholinergic specification (Elshatory and Gan, 2008; Cho et al., 2014). In fact, choline acetyltransferase is expressed in the region from early developmental stages (Rima et al., 2020; Ncube et al., 2022). We therefore suggest that the ectopic Isl1-positive cells may also differentiate into cholinergic neurons. In ctnnd2b+/− embryos we found a reduction of the number of GABA neurons in the ORR, suggesting that either directly or indirectly, ectopic Isl1 cells affect the local differentiation and organization of the GABA neurons in that region. Reduced GABAergic signaling has already been observed in individuals with autism (Fatemi et al., 2009; Oblak et al., 2011; Robertson et al., 2016), and genes encoding GABA receptor subunits have been associated with autism in both linkage and copy number studies (Ma et al., 2005). Mutations in CNTNAP2, an autism susceptibility gene, also results in a reduction in GABAergic neurons as well as hyperactivity, and changes in behavior in mice and zebrafish (Penagarikano et al., 2011; Hoffman et al., 2016). These findings strongly suggest that the imbalance or dysfunction of the GABAergic system is a candidate cause of autism, and that CTNND2 may have a role in the regulation of GABAergic neuron identity and the onset of this neurodevelopmental disorder.
Behavior assessment tests have been previously used in zebrafish to assess swimming patterns and test the influence of genes, toxicants, and other compounds (Egan et al., 2009; Wong et al., 2010; Ali et al., 2012; de Esch et al., 2012; Lange et al., 2012; Vignet et al., 2013; Ziv et al., 2013; Kim et al., 2014, 2017; Spulber et al., 2014; Crosby et al., 2015; Li et al., 2015). Here, we compared ctnnd2b+/− larvae and adults to wildtype individuals. At both developmental stages we found an increase in swimming activity and exploratory behavior. It has been reported previously that healthy zebrafish larvae move more when in the dark than light (MacPhail et al., 2009; Padilla et al., 2011; de Esch et al., 2012; Li et al., 2015). Interestingly, we observe that larvae missing one copy of ctnnd2b present with a more pronounced increase in activity when exposed to darkness than controls, resembling the changes reported in the Cntnap2 zebrafish autism model mentioned above (Hoffman et al., 2016), as well as in a model for lphn3.1-related ADHD (Lange et al., 2012). The changes in thigmotaxis we observe in ctnnd2b+/− larvae are also consistent with changes seen in other zebrafish models (Lockwood et al., 2004; Schnorr et al., 2012). Conversely, some studies presenting zebrafish models for Rett syndrome and autism have shown an opposite effect on behavior, namely in the distance moved in the visual motor response test and thigmotaxis (Pietri et al., 2013; Liu et al., 2018). These opposite effects are interesting but remain to be understood.
In adult ctnnd2b+/− we found an increase in the distance swam in the tank top zone compared to wildtype controls. These differences were more pronounced during the first 10 minutes of the test and were only significantly different between male zebrafish (Figures 5C,D). Other studies have shown similar findings, that the exploration to the top zone increases over time (Cachat et al., 2011; Vignet et al., 2013). Previous studies have shown that exposure to compounds that cause autism-like changes in behavior result in increased activity and exploratory behavior, resembling the ctnnd2b+/− fish’s behavior observed in our study (Maaswinkel et al., 2013). One limitation of our setup was the use of a single camera recording, instead of two cameras (from the side and the top of the tank) that would allow us to better triangulate the movements of the animals and assess other behavior features such as freezing and turn patterns. Nevertheless, supporting our observations, zebrafish models for fragile X syndrome and Down syndrome also presented with an increased vertical activity, with knockout animals spending more time in the top zone of the tank than controls (Ng et al., 2013; Kim et al., 2014, 2017). These findings suggest that there may be an imbalance between the excitatory and inhibitory neuronal signals or that the mutant animals present with a lack of impulsivity control, which we are not able to decipher yet.
Our study supports a role for CTNND2 haploinsufficiency in the etiology of neurodevelopmental disorders due to abnormal GABA neuronal differentiation in the forebrain. This haploinsufficiency affects the behavior of the carriers in similar ways to other neurodevelopmental disorders. Knowing the affected pathway causing the onset of the disorder offers the opportunity to test compounds able to rescue if not all, some phenotypes. Several compounds have efficaciously rescued abnormal behaviors in mutant animals in disorders such as ADHD (Lange et al., 2012) and ASD (Hoffman et al., 2016). Estrogens, for example, were successfully able to rescue the behavior changes in cntnap2ab-related ASD (Hoffman et al., 2016), suggesting that they could also be efficacious in our disease model.
Data availability statement
The raw data supporting the conclusions of this article will be made available by the authors, without undue reservation.
Ethics statement
The animal study was reviewed and approved by The Stockholm Ethical Board for Animal Experiments.
Author contributions
RV, WH, and AL conceptualized the work. RV, SE, AD-R, and WH performed the experiments and analyzed and interpreted the data. RV drafted the manuscript. All authors contributed to the article and approved the submitted version.
Funding
This study was funded by the Brain Foundation (Hjärnfonden, FO2022-0256), the Swedish research council (2019–02078) and Region Stockholm.
Acknowledgments
We would like to thank the staff at the Zebrafish Core facility for their help with the maintenance of the zebrafish and the staff at the BioMedicum Imaging Core for the maintenance of the microscopes. We acknowledge the ALM facility and the National Microscopy Infrastructure, NMI (VR-RFI 2019-00217) for providing assistance with the light sheet microscopy. We would also like to thank Dr. Jesper Eisfeldt for his help writing the scripts in Python. AL is a member of the European Reference Network on Rare Congenital Malformations and Rare Intellectual Disability ERN-ITHACA [EU Framework Partnership Agreement ID: 3HP-HP-FPA ERN-01-2016/739516].
Conflict of interest
The authors declare that the research was conducted in the absence of any commercial or financial relationships that could be construed as a potential conflict of interest.
Publisher’s note
All claims expressed in this article are solely those of the authors and do not necessarily represent those of their affiliated organizations, or those of the publisher, the editors and the reviewers. Any product that may be evaluated in this article, or claim that may be made by its manufacturer, is not guaranteed or endorsed by the publisher.
Supplementary material
The Supplementary material for this article can be found online at: https://www.frontiersin.org/articles/10.3389/fnins.2023.1205653/full#supplementary-material
References
Abu-Elneel, K., Ochiishi, T., Medina, M., Remedi, M., Gastaldi, L., Caceres, A., et al. (2008). A delta-catenin signaling pathway leading to dendritic protrusions. J. Biol. Chem. 283, 32781–32791. doi: 10.1074/jbc.M804688200
Adegbola, A., Lutz, R., Nikkola, E., Strom, S. P., Picker, J., and Wynshaw-Boris, A. (2020). Disruption of CTNND2, encoding delta-catenin, causes a penetrant attention deficit disorder and myopia. HGG Adv. 1:100007. doi: 10.1016/j.xhgg.2020.100007
Affaticati, P., Yamamoto, K., Rizzi, B., Bureau, C., Peyrieras, N., Pasqualini, C., et al. (2015). Identification of the optic recess region as a morphogenetic entity in the zebrafish forebrain. Sci. Rep. 5:8738. doi: 10.1038/srep08738
Ali, S., Champagne, D. L., and Richardson, M. K. (2012). Behavioral profiling of zebrafish embryos exposed to a panel of 60 water-soluble compounds. Behav. Brain Res. 228, 272–283. doi: 10.1016/j.bbr.2011.11.020
Allaway, K. C., and Machold, R. (2017). Developmental specification of forebrain cholinergic neurons. Dev. Biol. 421, 1–7. doi: 10.1016/j.ydbio.2016.11.007
Arikkath, J., Peng, I. F., Ng, Y. G., Israely, I., Liu, X., Ullian, E. M., et al. (2009). Delta-catenin regulates spine and synapse morphogenesis and function in hippocampal neurons during development. J. Neurosci. 29, 5435–5442. doi: 10.1523/JNEUROSCI.0835-09.2009
Asadollahi, R., Oneda, B., Joset, P., Azzarello-Burri, S., Bartholdi, D., Steindl, K., et al. (2014). The clinical significance of small copy number variants in neurodevelopmental disorders. J. Med. Genet. 51, 677–688. doi: 10.1136/jmedgenet-2014-102588
Bareiss, S., Kim, K., and Lu, Q. (2010). Delta-catenin/NPRAP: a new member of the glycogen synthase kinase-3beta signaling complex that promotes beta-catenin turnover in neurons. J. Neurosci. Res. 88, 2350–2363. doi: 10.1002/jnr.22414
Baumert, R., Ji, H., Paulucci-Holthauzen, A., Wolfe, A., Sagum, C., Hodgson, L., et al. (2020). Novel phospho-switch function of delta-catenin in dendrite development. J. Cell Biol. 219:909166. doi: 10.1083/jcb.201909166
Belcaro, C., Dipresa, S., Morini, G., Pecile, V., Skabar, A., and Fabretto, A. (2015). CTNND2 deletion and intellectual disability. Gene 565, 146–149. doi: 10.1016/j.gene.2015.03.054
Cachat, J., Stewart, A., Utterback, E., Hart, P., Gaikwad, S., Wong, K., et al. (2011). Three-dimensional neurophenotyping of adult zebrafish behavior. PLoS One 6:e17597. doi: 10.1371/journal.pone.0017597
Carrington, B., Varshney, G. K., Burgess, S. M., and Sood, R. (2015). CRISPR-STAT: an easy and reliable PCR-based method to evaluate target-specific sgRNA activity. Nucleic Acids Res. 43:e157. doi: 10.1093/nar/gkv802
Cho, H. H., Cargnin, F., Kim, Y., Lee, B., Kwon, R. J., Nam, H., et al. (2014). Isl1 directly controls a cholinergic neuronal identity in the developing forebrain and spinal cord by forming cell type-specific complexes. PLoS Genet. 10:e1004280. doi: 10.1371/journal.pgen.1004280
Colwill, R. M., and Creton, R. (2011). Locomotor behaviors in zebrafish (Danio rerio) larvae. Behav. Process. 86, 222–229. doi: 10.1016/j.beproc.2010.12.003
Crosby, E. B., Bailey, J. M., Oliveri, A. N., and Levin, E. D. (2015). Neurobehavioral impairments caused by developmental imidacloprid exposure in zebrafish. Neurotoxicol. Teratol. 49, 81–90. doi: 10.1016/j.ntt.2015.04.006
de Esch, C., van der Linde, H., Slieker, R., Willemsen, R., Wolterbeek, A., Woutersen, R., et al. (2012). Locomotor activity assay in zebrafish larvae: influence of age, strain and ethanol. Neurotoxicol. Teratol. 34, 425–433. doi: 10.1016/j.ntt.2012.03.002
Devine, C. A., and Key, B. (2003). Identifying axon guidance defects in the embryonic zebrafish brain. Methods Cell Sci. 25, 33–37. doi: 10.1023/B:MICS.0000006851.84998.e0
Donta, M. S., Srivastava, Y., and McCrea, P. D. (2022). Delta-catenin as a modulator of rho GTPases in neurons. Front. Cell. Neurosci. 16:939143. doi: 10.3389/fncel.2022.939143
Egan, R. J., Bergner, C. L., Hart, P. C., Cachat, J. M., Canavello, P. R., Elegante, M. F., et al. (2009). Understanding behavioral and physiological phenotypes of stress and anxiety in zebrafish. Behav. Brain Res. 205, 38–44. doi: 10.1016/j.bbr.2009.06.022
Elshatory, Y., and Gan, L. (2008). The LIM-homeobox gene Islet-1 is required for the development of restricted forebrain cholinergic neurons. J. Neurosci. 28, 3291–3297. doi: 10.1523/JNEUROSCI.5730-07.2008
Fatemi, S. H., Reutiman, T. J., Folsom, T. D., and Thuras, P. D. (2009). GABA(a) receptor downregulation in brains of subjects with autism. J. Autism Dev. Disord. 39, 223–230. doi: 10.1007/s10803-008-0646-7
Fragkouli, A., van Wijk, N. V., Lopes, R., Kessaris, N., and Pachnis, V. (2009). LIM homeodomain transcription factor-dependent specification of bipotential MGE progenitors into cholinergic and GABAergic striatal interneurons. Development 136, 3841–3851. doi: 10.1242/dev.038083
Gilbert, J., and Man, H. Y. (2016). The X-linked autism protein KIAA2022/KIDLIA regulates neurite outgrowth via N-cadherin and delta-catenin Signaling. eNeuro. 3, ENEURO.0238–ENEU16.2016. doi: 10.1523/ENEURO.0238-16.2016
Girirajan, S., Dennis, M. Y., Baker, C., Malig, M., Coe, B. P., Campbell, C. D., et al. (2013). Refinement and discovery of new hotspots of copy-number variation associated with autism spectrum disorder. Am. J. Hum. Genet. 92, 221–237. doi: 10.1016/j.ajhg.2012.12.016
Glasauer, S. M., and Neuhauss, S. C. (2014). Whole-genome duplication in teleost fishes and its evolutionary consequences. Mol. Gen. Genomics. 289, 1045–1060. doi: 10.1007/s00438-014-0889-2
Hassani Nia, F., Woike, D., Martens, V., Klussendorf, M., Honck, H. H., Harder, S., et al. (2020). Targeting of delta-catenin to postsynaptic sites through interaction with the Shank3 N-terminus. Mol. Autism. 11:85. doi: 10.1186/s13229-020-00385-8
Ho, C., Zhou, J., Medina, M., Goto, T., Jacobson, M., Bhide, P. G., et al. (2000). Delta-catenin is a nervous system-specific Adherens junction protein which undergoes dynamic Relocalization during development. J. Comp. Neurol. 420, 261–276. doi: 10.1002/(SICI)1096-9861(20000501)420:2<261::AID-CNE8>3.0.CO;2-Q
Hoffman, E. J., Turner, K. J., Fernandez, J. M., Cifuentes, D., Ghosh, M., Ijaz, S., et al. (2016). Estrogens suppress a Behavioral phenotype in zebrafish mutants of the autism risk gene, CNTNAP2. Neuron 89, 725–733. doi: 10.1016/j.neuron.2015.12.039
Hofmeister, W., Nilsson, D., Topa, A., Anderlid, B. M., Darki, F., Matsson, H., et al. (2015). CTNND2-a candidate gene for reading problems and mild intellectual disability. J. Med. Genet. 52, 111–122. doi: 10.1136/jmedgenet-2014-102757
Israely, I., Costa, R. M., Xie, C. W., Silva, A. J., Kosik, K. S., and Liu, X. (2004). Deletion of the neuron-specific protein delta-catenin leads to severe cognitive and synaptic dysfunction. Curr. Biol. 14, 1657–1663. doi: 10.1016/j.cub.2004.08.065
Jones, S. B., Lanford, G. W., Chen, Y. H., Moribito, M., Kim, K., and Lu, Q. (2002). Glutamate-induced delta-catenin redistribution and dissociation from postsynaptic receptor complexes. Neurosci. 115, 1009–1021. doi: 10.1016/S0306-4522(02)00532-8
Kang, H. J., Kim, K. T., Park, Y., Yoo, K. H., Kim, J. W., Lee, J. Y., et al. (2021). Genetic markers for depressive disorders with earlier age at onset. Prog. Neuro-Psychopharmacol. Biol. Psychiatry 108:110176. doi: 10.1016/j.pnpbp.2020.110176
Kim, J. S., Bareiss, S., Kim, K. K., Tatum, R., Han, J.-R., Jin, Y. H., et al. (2006). Presenilin-1 inhibits δ-catenin-induced cellular branching and promotes δ-catenin processing and turnover. Biochem. Biophys. Res. Commun. 351, 903–908. doi: 10.1016/j.bbrc.2006.10.135
Kim, O. H., Cho, H. J., Han, E., Hong, T. I., Ariyasiri, K., Choi, J. H., et al. (2017). Zebrafish knockout of down syndrome gene, DYRK1A, shows social impairments relevant to autism. Mol. Autism. 8:50. doi: 10.1186/s13229-017-0168-2
Kim, H., Han, J. R., Park, J., Oh, M., James, S. E., Chang, S., et al. (2008). Delta-catenin-induced dendritic morphogenesis. An essential role of p190RhoGEF interaction through Akt1-mediated phosphorylation. J. Biol. Chem. 283, 977–987. doi: 10.1074/jbc.M707158200
Kim, L., He, L., Maaswinkel, H., Zhu, L., Sirotkin, H., and Weng, W. (2014). Anxiety, hyperactivity and stereotypy in a zebrafish model of fragile X syndrome and autism spectrum disorder. Prog. Neuro-Psychopharmacol. Biol. Psychiatry 55, 40–49. doi: 10.1016/j.pnpbp.2014.03.007
Lange, M., Norton, W., Coolen, M., Chaminade, M., Merker, S., Proft, F., et al. (2012). The ADHD-susceptibility gene lphn3.1 modulates dopaminergic neuron formation and locomotor activity during zebrafish development. Mol. Psychiatry 17, 946–954. doi: 10.1038/mp.2012.29
Lee, N., Park, S. J., Haddad, G., Kim, D. K., Park, S. M., Park, S. K., et al. (2016). Interactomic analysis of REST/NRSF and implications of its functional links with the transcription suppressor TRIM28 during neuronal differentiation. Sci. Rep. 6:39049. doi: 10.1038/srep39049
Li, Q., Lin, J., Zhang, Y., Liu, X., Chen, X. Q., Xu, M. Q., et al. (2015). Differential behavioral responses of zebrafish larvae to yohimbine treatment. Psychopharmacology 232, 197–208. doi: 10.1007/s00213-014-3656-5
Ligon, C., Seong, E., Schroeder, E. J., DeKorver, N. W., Yuan, L., Chaudoin, T. R., et al. (2020). Delta-catenin engages the autophagy pathway to sculpt the developing dendritic arbor. J. Biol. Chem. 295, 10988–11001. doi: 10.1074/jbc.RA120.013058
Liodis, P., Denaxa, M., Grigoriou, M., Akufo-Addo, C., Yanagawa, Y., and Pachnis, V. (2007). Lhx6 activity is required for the normal migration and specification of cortical interneuron subtypes. J. Neurosci. 27, 3078–3089. doi: 10.1523/JNEUROSCI.3055-06.2007
Liu, X., Lin, J., Zhang, Y., Guo, N., and Li, Q. (2018). Sound shock response in larval zebrafish: a convenient and high-throughput assessment of auditory function. Neurotoxicol. Teratol. 66, 1–7. doi: 10.1016/j.ntt.2018.01.003
Lockwood, B., Bjerke, S., Kobayashi, K., and Guo, S. (2004). Acute effects of alcohol on larval zebrafish: a genetic system for large-scale screening. Pharmacol. Biochem. Behav. 77, 647–654. doi: 10.1016/j.pbb.2004.01.003
Lu, Q., Aguilar, B. J., Li, M., Jiang, Y., and Chen, Y. H. (2016). Genetic alterations of delta-catenin/NPRAP/Neurojungin (CTNND2): functional implications in complex human diseases. Hum. Genet. 135, 1107–1116. doi: 10.1007/s00439-016-1705-3
Ma, D. Q., Whitehead, P. L., Menold, M. M., Martin, E. R., Ashley-Koch, A. E., Mei, H., et al. (2005). Identification of significant association and gene-gene interaction of GABA receptor subunit genes in autism. Am. J. Hum. Genet. 77, 377–388. doi: 10.1086/433195
Maaswinkel, H., Zhu, L., and Weng, W. (2013). Assessing social engagement in heterogeneous groups of zebrafish: a new paradigm for autism-like behavioral responses. PLoS One 8:e75955. doi: 10.1371/journal.pone.0075955
MacPhail, R. C., Brooks, J., Hunter, D. L., Padnos, B., Irons, T. D., and Padilla, S. (2009). Locomotion in larval zebrafish: influence of time of day, lighting and ethanol. Neurotoxicology 30, 52–58. doi: 10.1016/j.neuro.2008.09.011
Mainardi, P. C., Perfumo, C., Cali, A., Coucourde, G., Pastore, G., Cavani, S., et al. (2001). Clinical and molecular characterisation of 80 patients with 5p deletion: genotype-phenotype correlation. J. Med. Genet. 38, 151–158. doi: 10.1136/jmg.38.3.151
Manoli, M., and Driever, W. (2014). nkx2.1 and nkx2.4 genes function partially redundant during development of the zebrafish hypothalamus, preoptic region, and pallidum. Front. Neuroanat. 8:145. doi: 10.3389/fnana.2014.00145
Matter, C., Pribadi, M., Liu, X., and Trachtenberg, J. T. (2009). Delta-catenin is required for the maintenance of neural structure and function in mature cortex in vivo. Neuron 64, 320–327. doi: 10.1016/j.neuron.2009.09.026
McMichael, G., Girirajan, S., Moreno-De-Luca, A., Gecz, J., Shard, C., Nguyen, L. S., et al. (2014). Rare copy number variation in cerebral palsy. Eur. J. Hum. Genet. 22, 40–45. doi: 10.1038/ejhg.2013.93
Medina, M., Marinescu, R. C., Overhauser, J., and Kosik, K. S. (2000). Hemizygosity of delta-catenin (CTNND2) is associated with severe mental retardation in cri-du-chat syndrome. Genomics 63, 157–164. doi: 10.1006/geno.1999.6090
Miller, D. E., Squire, A., and Bennett, J. T. (2020). A child with autism, behavioral issues, and dysmorphic features found to have a tandem duplication within CTNND2 by mate-pair sequencing. Am. J. Med. Genet. A 182, 543–547. doi: 10.1002/ajmg.a.61442
Ncube, D., Tallafuss, A., Serafin, J., Bruckner, J., Farnsworth, D. R., Miller, A. C., et al. (2022). A conserved transcriptional fingerprint of multi-neurotransmitter neurons necessary for social behavior. BMC Genomics 23:675. doi: 10.1186/s12864-022-08879-w
Ng, M. C., Yang, Y. L., and Lu, K. T. (2013). Behavioral and synaptic circuit features in a zebrafish model of fragile X syndrome. PLoS One 8:e51456. doi: 10.1371/journal.pone.0051456
Nivard, M. G., Mbarek, H., Hottenga, J. J., Smit, J. H., Jansen, R., Penninx, B. W., et al. (2014). Further confirmation of the association between anxiety and CTNND2: replication in humans. Genes Brain Behav. 13, 195–201. doi: 10.1111/gbb.12095
Oblak, A. L., Gibbs, T. T., and Blatt, G. J. (2011). Reduced GABAA receptors and benzodiazepine binding sites in the posterior cingulate cortex and fusiform gyrus in autism. Brain Res. 1380, 218–228. doi: 10.1016/j.brainres.2010.09.021
Padilla, S., Hunter, D. L., Padnos, B., Frady, S., and MacPhail, R. C. (2011). Assessing locomotor activity in larval zebrafish: influence of extrinsic and intrinsic variables. Neurotoxicol. Teratol. 33, 624–630. doi: 10.1016/j.ntt.2011.08.005
Penagarikano, O., Abrahams, B. S., Herman, E. I., Winden, K. D., Gdalyahu, A., Dong, H., et al. (2011). Absence of CNTNAP2 leads to epilepsy, neuronal migration abnormalities, and core autism-related deficits. Cells 147, 235–246. doi: 10.1016/j.cell.2011.08.040
Pietri, T., Roman, A. C., Guyon, N., Romano, S. A., Washbourne, P., Moens, C. B., et al. (2013). The first mecp2-null zebrafish model shows altered motor behaviors. Front Neural Circuits. 7:118. doi: 10.3389/fncir.2013.00118
Preibisch, S., Amat, F., Stamataki, E., Sarov, M., Singer, R. H., Myers, E., et al. (2014). Efficient Bayesian-based multiview deconvolution. Nat. Methods 11, 645–648. doi: 10.1038/nmeth.2929
Preibisch, S., Saalfeld, S., Schindelin, J., and Tomancak, P. (2010). Software for bead-based registration of selective plane illumination microscopy data. Nat. Methods 7, 418–419. doi: 10.1038/nmeth0610-418
Ricciardello, A., Tomaiuolo, P., and Persico, A. M. (2021). Genotype-phenotype correlation in Phelan-McDermid syndrome: a comprehensive review of chromosome 22q13 deleted genes. Am. J. Med. Genet. A 185, 2211–2233. doi: 10.1002/ajmg.a.62222
Rima, M., Lattouf, Y., Abi Younes, M., Bullier, E., Legendre, P., Mangin, J. M., et al. (2020). Dynamic regulation of the cholinergic system in the spinal central nervous system. Sci. Rep. 10:15338. doi: 10.1038/s41598-020-72524-3
Robertson, C. E., Ratai, E. M., and Kanwisher, N. (2016). Reduced GABAergic action in the autistic brain. Curr. Biol. 26, 80–85. doi: 10.1016/j.cub.2015.11.019
Ryu, T., Park, H. J., Kim, H., Cho, Y. C., Kim, B. C., Jo, J., et al. (2019). Improved memory and reduced anxiety in delta-catenin transgenic mice. Exp. Neurol. 318, 22–31. doi: 10.1016/j.expneurol.2019.04.006
Sardina, J. M., Walters, A. R., Singh, K. E., Owen, R. X., and Kimonis, V. E. (2014). Amelioration of the typical cognitive phenotype in a patient with the 5pter deletion associated with cri-du-chat syndrome in addition to a partial duplication of CTNND2. Am. J. Med. Genet. A 164A, 1761–1764. doi: 10.1002/ajmg.a.36494
Schnorr, S. J., Steenbergen, P. J., Richardson, M. K., and Champagne, D. L. (2012). Measuring thigmotaxis in larval zebrafish. Behav. Brain Res. 228, 367–374. doi: 10.1016/j.bbr.2011.12.016
Spulber, S., Kilian, P., Wan Ibrahim, W. N., Onishchenko, N., Ulhaq, M., Norrgren, L., et al. (2014). PFOS induces behavioral alterations, including spontaneous hyperactivity that is corrected by dexamfetamine in zebrafish larvae. PLoS One 9:e94227. doi: 10.1371/journal.pone.0094227
Thisse, C., and Thisse, B. (2008). High-resolution in situ hybridization to whole-mount zebrafish embryos. Nat. Protoc. 3, 59–69. doi: 10.1038/nprot.2007.514
Tuncay, I. O., Parmalee, N. L., Khalil, R., Kaur, K., Kumar, A., Jimale, M., et al. (2022). Analysis of recent shared ancestry in a familial cohort identifies coding and noncoding autism spectrum disorder variants. NPJ Genom. Med. 7:13. doi: 10.1038/s41525-022-00284-2
Turner, T. N., Sharma, K., Oh, E. C., Liu, Y. P., Collins, R. L., Sosa, M. X., et al. (2015). Loss of delta-catenin function in severe autism. Nature 520, 51–56. doi: 10.1038/nature14186
Uchino, S., and Waga, C. (2013). SHANK3 as an autism spectrum disorder-associated gene. Brain and Development 35, 106–110. doi: 10.1016/j.braindev.2012.05.013
van Rootselaar, A. F., Groffen, A. J., de Vries, B., Callenbach, P. M. C., Santen, G. W. E., Koelewijn, S., et al. (2017). D-catenin (CTNND2) missense mutation in familial cortical myoclonic tremor and epilepsy. Neurology 89, 2341–2350. doi: 10.1212/WNL.0000000000004709
Varshney, G. K., Sood, R., and Burgess, S. M. (2015). Understanding and editing the zebrafish genome. Adv. Genet. 92, 1–52. doi: 10.1016/bs.adgen.2015.09.002
Vaz, R., Hofmeister, W., and Lindstrand, A. (2019). Zebrafish models of neurodevelopmental disorders: limitations and benefits of current tools and techniques. Int. J. Mol. Sci. 20:61296. doi: 10.3390/ijms20061296
Vignet, C., Begout, M. L., Pean, S., Lyphout, L., Leguay, D., and Cousin, X. (2013). Systematic screening of behavioral responses in two zebrafish strains. Zebrafish 10, 365–375. doi: 10.1089/zeb.2013.0871
Vrijenhoek, T., Buizer-Voskamp, J. E., van der Stelt, I., Strengman, E., Sabatti, C., van Kessel, A. G., et al. (2008). Recurrent CNVs disrupt three candidate genes in schizophrenia patients. Am. J. Hum. Genet. 83, 504–510. doi: 10.1016/j.ajhg.2008.09.011
Wang, X., Xu, M., Xu, Q., Yang, F., Tang, H., Shao, C., et al. (2021). Rictor is involved in Ctnnd2 deletion-induced impairment of spatial learning and memory but not autism-like behaviors. Front Biosci (Landmark Ed). 26, 335–346. doi: 10.52586/4947
Wong, K., Elegante, M., Bartels, B., Elkhayat, S., Tien, D., Roy, S., et al. (2010). Analyzing habituation responses to novelty in zebrafish (Danio rerio). Behav. Brain Res. 208, 450–457. doi: 10.1016/j.bbr.2009.12.023
Yuan, L., Seong, E., Beuscher, J. L., and Arikkath, J. (2015). Delta-catenin regulates spine architecture via cadherin and PDZ-dependent interactions. J. Biol. Chem. 290, 10947–10957. doi: 10.1074/jbc.M114.632679
Keywords: CTNND2, CRISPR/Cas9, zebrafish, neuronal development, swimming behavior
Citation: Vaz R, Edwards S, Dueñas-Rey A, Hofmeister W and Lindstrand A (2023) Loss of ctnnd2b affects neuronal differentiation and behavior in zebrafish. Front. Neurosci. 17:1205653. doi: 10.3389/fnins.2023.1205653
Edited by:
Tudor Constantin Badea, Transilvania University of Brașov, RomaniaReviewed by:
Marc Ekker, University of Ottawa, CanadaPhilip Washbourne, University of Oregon, United States
Estuardo Robles, Purdue University, United States
Copyright © 2023 Vaz, Edwards, Dueñas-Rey, Hofmeister and Lindstrand. This is an open-access article distributed under the terms of the Creative Commons Attribution License (CC BY). The use, distribution or reproduction in other forums is permitted, provided the original author(s) and the copyright owner(s) are credited and that the original publication in this journal is cited, in accordance with accepted academic practice. No use, distribution or reproduction is permitted which does not comply with these terms.
*Correspondence: Raquel Vaz, raquel.vaz@ki.se
†PRESENT ADDRESS: Alfredo Dueñas-Rey, Department of Biomolecular Medicine, Ghent University, Center for Medical Genetics, University Hospital Ghent, Ghent, Belgium