- 1The First School of Clinical Medicine, Zhejiang Chinese Medical University, Hangzhou, China
- 2College of Pharmaceutical Science, Zhejiang Chinese Medical University, Hangzhou, China
This review provides insight into the complex network of signaling pathways and mechanisms involved in stroke pathophysiology. It summarizes the historical progress of stroke-related signaling pathways, identifying potential interactions between them and emphasizing that stroke is a complex network disease. Of particular interest are the Hippo signaling pathway and ferroptosis signaling pathway, which remain understudied areas of research, and are therefore a focus of the review. The involvement of multiple signaling pathways, including Sonic Hedgehog (SHH), nuclear factor erythroid 2-related factor 2 (Nrf2)/antioxidant response element (ARE), hypoxia-inducible factor-1α (HIF-1α), PI3K/AKT, JAK/STAT, and AMPK in pathophysiological mechanisms such as oxidative stress and apoptosis, highlights the complexity of stroke. The review also delves into the details of traditional Chinese medicine (TCM) therapies such as Rehmanniae and Astragalus, providing an analysis of the recent status of western medicine in the treatment of stroke and the advantages and disadvantages of TCM and western medicine in stroke treatment. The review proposes that since stroke is a network disease, TCM has the potential and advantages of a multi-target and multi-pathway mechanism of action in the treatment of stroke. Therefore, it is suggested that future research should explore more treasures of TCM and develop new therapies from the perspective of stroke as a network disease.
1. Introduction
Stroke is a serious medical condition that occurs when brain tissue is damaged due to cerebrovascular accidents. Different types of stroke include ischemic stroke, cerebral hemorrhage, and subarachnoid hemorrhage, which are classified based on their causes and symptoms. Ischemic stroke, the most common type, occurs when brain tissue is damaged due to a lack of oxygen and nutrients caused by thrombosis, embolism, or systemic under perfusion. In contrast, a cerebral hemorrhage occurs when a blood vessel ruptures in the brain, resulting in tissue underperfusion. About fifteen percent of cerebral hemorrhages result from ruptured blood vessels, structural abnormalities of blood vessels, or post-hypertensive small vessel abnormalities. Subarachnoid hemorrhage, which accounts for about 5% of strokes, is primarily caused by ruptured saccular aneurysms (Dirnagl et al., 1999; Donnan et al., 2008).
Stroke, along with severe conditions such as ischemic heart disease, chronic obstructive pulmonary disease, and Coronavirus Disease 2019 (COVID-19), is a significant cause of mortality worldwide. The pathophysiology of stroke comprises multiple mechanisms, including inflammatory response, oxidative stress, apoptosis, angiogenesis, and autophagy (Krupinski et al., 1994; Dirnagl et al., 1999; Allen and Bayraktutan, 2009). The Global Burden of Disease study data indicates that the number of stroke cases increased to 12.2 million in 2019, which is a substantial rise of 70.0% in comparison to the total number of stroke cases recorded in 1990 (GBD 2019 Stroke Collaborators, 2021) In low-income countries, the age-standardized mortality rate for stroke is 3.6 times higher compared to high-income countries, and stroke also poses a greater burden in low-income countries. Moreover, the incidence of stroke exhibits regional disparities, as well as variations in age and gender distribution (GBD 2019 Stroke Collaborators, 2021; Vyas et al., 2021). Stroke can be treated with a variety of interventions such as anticoagulation, antiplatelet medication, blood pressure control, lipid reduction, thrombolysis, carotid endarterectomy, and stem cell transplantation, all of which can help reduce the risk of stroke and provide some relief to patients (O’Rourke et al., 2004; Donnan et al., 2008; Barthels and Das, 2020). Research on epidemiology and preventive medicine has suggested that maintaining appropriate levels of metal elements in plasma is crucial for reducing the risk of stroke. Studies have identified high concentrations of iron, copper, and selenium in plasma as risk factors for stroke development (Alim et al., 2019; Mirończuk et al., 2021; Shi et al., 2021; Zhang et al., 2021). Further research has confirmed that stroke occurrence, progression, and prognosis can be significantly affected by cell death resulting from iron-related mechanisms (Zhou et al., 2021). Selenium (Se) therapy has been found to mitigate the detrimental effects of stroke by targeting the three primary factors involved in iron-related cell death, namely lipid peroxidation, generation of reactive oxygen species, and iron metabolism (Alim et al., 2019).
The signaling pathways associated with stroke are intricate, comprehensive, and interconnected systems. The year 2011 witnessed the emergence of a new field of study known as cyber medicine (Barabási et al., 2011). Stroke rarely results from abnormalities in the product of a single gene target, but rather is a multifaceted outcome of complex network interactions (Qin et al., 2022). The main focus of this paper is the concept of “network disease”, which seeks to provide novel insights into the exploration of better drug targets. Stroke is a complex network disease that involves multiple signaling pathways, and relying solely on single-target Western drugs may not be effective in treating it or have side effects. The benefit of TCM and its formulations lies in their ability to provide a synergistic effect on multiple pathways and targets, making them effective in acting on various signaling pathways involved in stroke (Lou et al., 2022). This paper aims to explore the molecular mechanisms of stroke-related signaling pathways, provide an overview of the historical process, development, and relationship between each signaling pathway and stroke, and highlight the intricate and interconnected relationships among them. In addition, we will review the current status of TCM in improving and treating stroke through various molecular mechanisms such as angiogenesis, inflammatory response, and oxidative stress. TCM holds great potential for pharmacological studies of network diseases such as stroke.
2. Historical progression of stroke’s classical signaling pathways
2.1. Stroke related signaling pathways: historical progress and current research
Given the complex pathogenesis of stroke, this paper provides a macroscopic overview of its pathophysiological basis. The pathophysiological mechanisms that contribute to stroke include angiogenesis, oxidative stress, autophagy formation, inflammatory response, and apoptosis (as summarized in Supplementary Table 1). Next, we provide a summary and elaboration of stroke-related signaling pathways from various molecular mechanisms such as angiogenesis, oxidative stress, autophagy behavior, inflammatory response, cell proliferation, and apoptosis (as summarized in Supplementary Table 2). A summary diagram of stroke-related signaling pathways is shown in Figure 1. Supplementary Table 2 summarizes the historical process and research progress of signaling pathways related to different pathophysiological mechanisms of stroke. Understanding the historical development process of each signaling pathway related to stroke can enhance our comprehension of the research process and development status of a particular signaling pathway. Currently, scientific research tends to explore the correlation between two signaling pathways, as the signal axis can impact the occurrence, development, and treatment of stroke (Zhan et al., 2022). In recent years, due to the network disease characteristics of stroke, the potential crosstalk between a signaling pathway and other signaling pathways has been gradually uncovered (Lou et al., 2022).
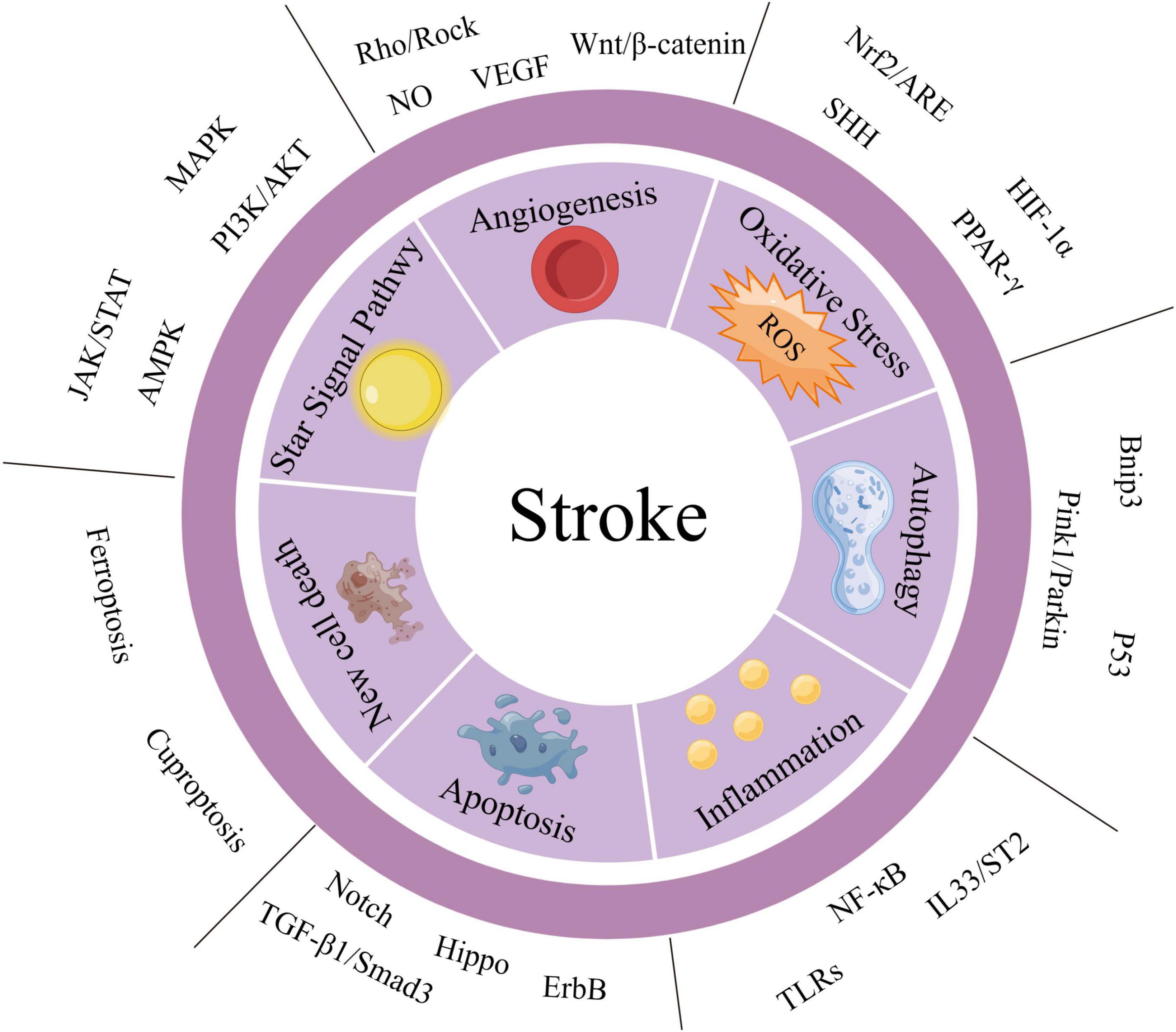
Figure 1. Summary diagram of stroke-related signaling pathways. We have categorized the important signaling pathways involved in stroke into two major groups: the “STAR” signaling pathway and other signaling pathways. Additionally, based on the pathophysiological mechanisms of stroke, we have divided the other signaling pathways into six aspects: angiogenesis, oxidative stress, autophagy, inflammatory response, apoptosis, and new cell death. The STAR signaling pathways include JAK/STAT, AMPK, mitogen-activated protein kinase (MAPK), and PI3K/AKT. These pathways can affect several pathophysiological mechanisms of stroke and are listed separately for emphasis.
2.2. From the historical process: the Hippo signaling pathway and iron death signaling pathway in stroke should be focused
Previously, we summarized Supplementary Table 2, which summarized the historical course of stroke-related signaling pathways. Since its discovery in 2017, ferroptosis, a newly identified programmed cell death (PCD) pathway, has shown a potential relationship with stroke (Dixon et al., 2012; Zille et al., 2017). The signaling pathways involved in ferroptosis mainly include lipid peroxidation, iron metabolism, and amino acid metabolism, which can affect the generation of reactive oxygen species (ROS). Ferroptosis has gained significant attention in the fields of neuroscience and medicine, and it is now considered an important area of research (Hirschhorn and Stockwell, 2019). Ferroptosis has been implicated in the development and progression of a range of diseases, including stroke. Inhibition of ferroptosis, a newly identified form of cell death, has been shown to reduce lesion damage to some extent and even improve prognosis. However, the existing research on ferroptosis, particularly in relation to stroke, is limited. Therefore, we highlight the significance of ferroptosis and draw attention to its importance in stroke-related signaling pathways.
The Hippo signaling pathway is known to play a crucial role in regulating cell behavior, growth, proliferation, and tissue homeostasis (Meng et al., 2016). The historical studies on this pathway are mainly focused on cancer, and it regulates organ regeneration and cell plasticity (Harvey et al., 2013). Regeneration is also very important for the prognosis of stroke, affecting tissue recovery and cell regeneration after stroke injury. Therefore, it is very important to study the role of this signaling pathway in stroke. Recently, the important role of Sonic Hedgehog signaling pathway in brain repair and functional recovery after stroke suggests that the regulation of Sonic Hedgehog signaling pathway is a potential strategy to extend the therapeutic window after stroke. The molecular mechanism of regulating the Hippo signaling pathway can affect ischemia-reperfusion injury (Jin et al., 2017; Gong et al., 2019, 2021). Therefore, we take it out of the historical process of stroke and emphasize the potential of this pathway in stroke with a lot of pen and ink, suggesting that new and more research should be carried out.
It is crucial to consider the aforementioned pathways, however, there is limited strong historical research on their specific mechanisms and effects in the treatment of stroke. Additionally, it has been revealed that ferroptosis has crosstalk with other pathways such as necroptosis and oxidative stres (Yan et al., 2021). Furthermore, there is a potential crosstalk between the Hippo signaling pathway and the ferroptosis signaling pathway (He et al., 2022). Therefore, in the following sections, we will provide a summary of the historical progression of the Hippo signaling pathway and the ferroptosis signaling pathway, their underlying mechanisms, the interplay between pathways, and their relationship to stroke.
2.3. Hippo signaling pathway and stroke: regeneration potential
2.3.1. Research history of Hippo signaling pathway
The Hippo signaling pathway, also referred to as the Salvador/Warts/Hippo (SWH) pathway, has gained attention as a research area in recent years. It was initially discovered in tissues of the fruit fly Drosophila melanogaster. The research history of Hippo signaling pathway is shown in Figure 2. The first crucial gene of the Hippo signaling pathway, known as Warts (Wts or Lats), was identified through genetic screening in 1995 in Drosophila. This gene encodes a protein serine/threonine kinase and acts as a newly identified tumor suppressor gene. Inhibition of its expression can lead to excessive proliferation and abnormal differentiation (Justice et al., 1995; Xu et al., 1995). Subsequently, in 2002, the Hariharan and Halder labs identified the second gene of the Hippo pathway, Sav. This gene contains two domains associated with the Wts gene and promotes apoptosis. Mutations in the Sav gene are associated with abnormal proliferation and cancer (Kango-Singh et al., 2002; Tapon et al., 2002). Since then, there have been several discoveries in the Hippo signaling pathway. For instance, the tumor suppressor Mat was found to interact with the Wts gene to enhance Wts kinase activity (Lai et al., 2005). Additionally, the Hpo gene encodes a Ste-20 family protein that links the Wts gene with the Sav gene to control growth (Harvey et al., 2003; Udan et al., 2003; Wu et al., 2003). Among them, the Sav gene can be phosphorylated by the Hpo gene to promote the interaction with the Wts gene (Pantalacci et al., 2003). Furthermore, the Yap gene was found in yeast hybridization, which makes up for the missing link of downstream transduction of the Wts gene to regulate the cell cycle and activate cell death regulators (Huang et al., 2005). The identification of Mat, Hpo, Yap, and other genes has enabled the linkage of previously identified pathway components, resulting in the discovery of the Hippo signaling pathway, which regulates organ size by controlling cell number. The pathway was first identified in Drosophila tissues, and more than 30 components have been identified through various studies. Research has advanced from the initial studies in Drosophila to studies in mammals, with the pathway being evolutionarily conserved, as the key molecules identified in Drosophila have homologous genes in mammals (Meng et al., 2016). The Hippo signaling pathway is crucial for maintaining the balance between cell apoptosis and proliferation (Harvey et al., 2003). Furthermore, the Hippo signaling pathway is involved in embryonic development, tissue regeneration, and the regulation of organ size (Grijalva et al., 2014; Elbediwy et al., 2016; Moya and Halder, 2019). The Hippo signaling pathway has been implicated in a wide range of diseases, including cancer and cardiovascular diseases, due to its important function (Moroishi et al., 2015; Zanconato et al., 2016; Zheng and Pan, 2019).
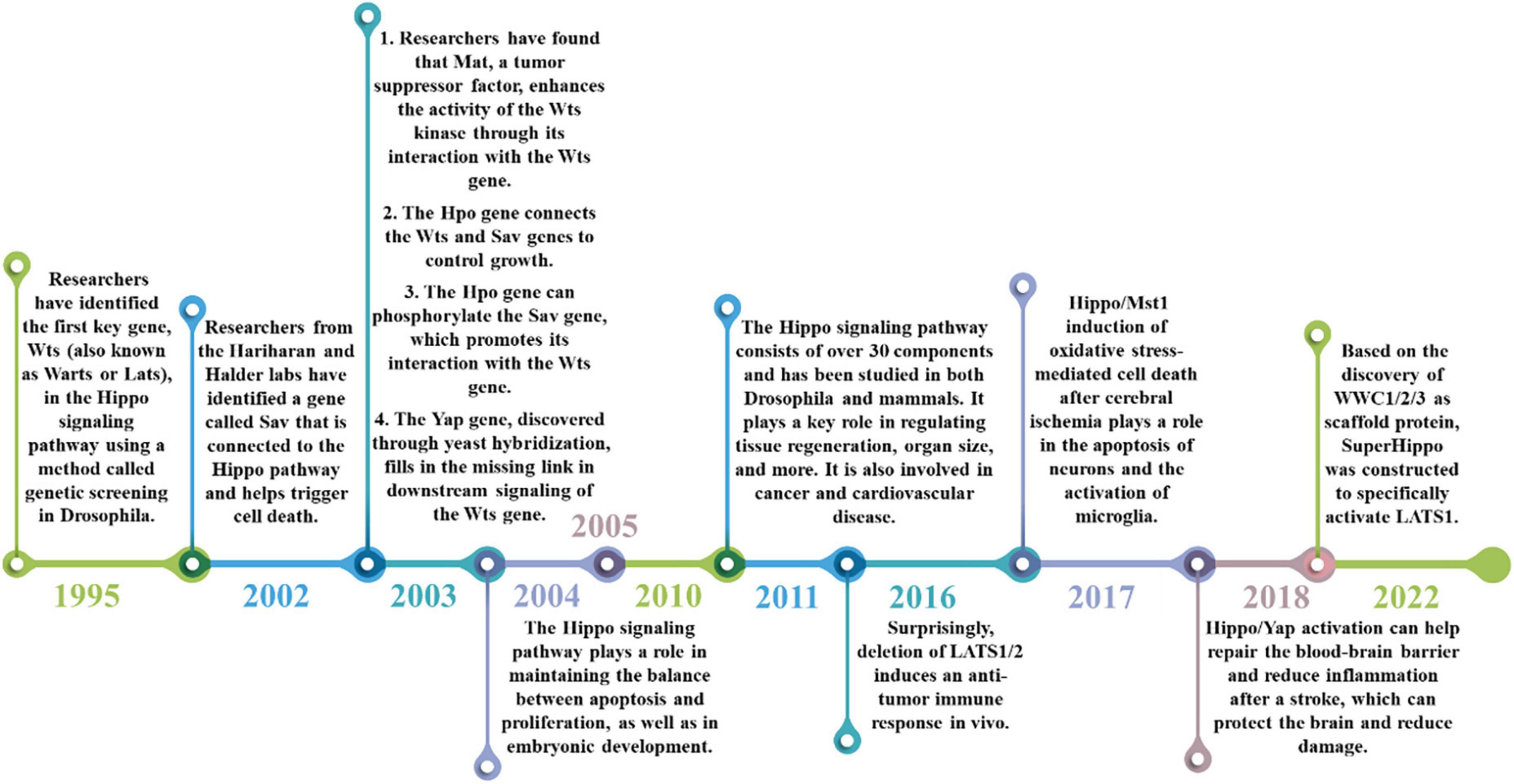
Figure 2. The historical process of the Hippo signaling pathway. The discovery of the Hippo signaling pathway began with the identification of the Wts gene in 1995, which was followed by the discovery of other genes such as Sav, YAP, and Mst. These findings helped to improve our understanding of the composition and function of the Hippo signaling pathway. Over the years, the Hippo signaling pathway has been found to regulate a range of biological processes, including cell proliferation, apoptosis, tissue development, and more (2004–2010). More recently, it has been implicated in cardiovascular disease, cancer (2011–2016), and its potential role in stroke treatment has been discovered (2018–2022).
2.3.2. The key regulatory mechanisms of Hippo signaling pathway
The Hippo signaling pathway is regulated through a series of steps. In mammals, the pathway is activated by upstream membrane protein receptors in response to extracellular signals. These receptors are subsequently phosphorylated by a sequence of conserved kinases, which ultimately regulate the activity of downstream effectors, namely Yes-associated protein (YAP)/PDZ-binding motif (TAZ) (Meng et al., 2016). Upon activation, YAP/TAZ may relocate to the nucleus and engage with TEA domain family members (TEAD) 1-4, triggering the activation of downstream transcription factors and consequent transcriptional activation (Lian et al., 2010; Meng et al., 2016; Pobbati and Hong, 2020).
The regulatory mechanism of Hippo signaling is shown in Figure 3. The Hippo signaling pathway is stimulated by various upstream signals, including mechanical signals originating from cell contact (predominantly from the extracellular matrix or ECM), G protein-coupled receptors (GPCRs), stress signals, as well as signals linked to cell cycle, polarity, and structure (Yu et al., 2012; Meng et al., 2016; Zheng and Pan, 2019). A kinase phosphorylation cascade constitutes the core of the pathway through which these inputs are transmitted.
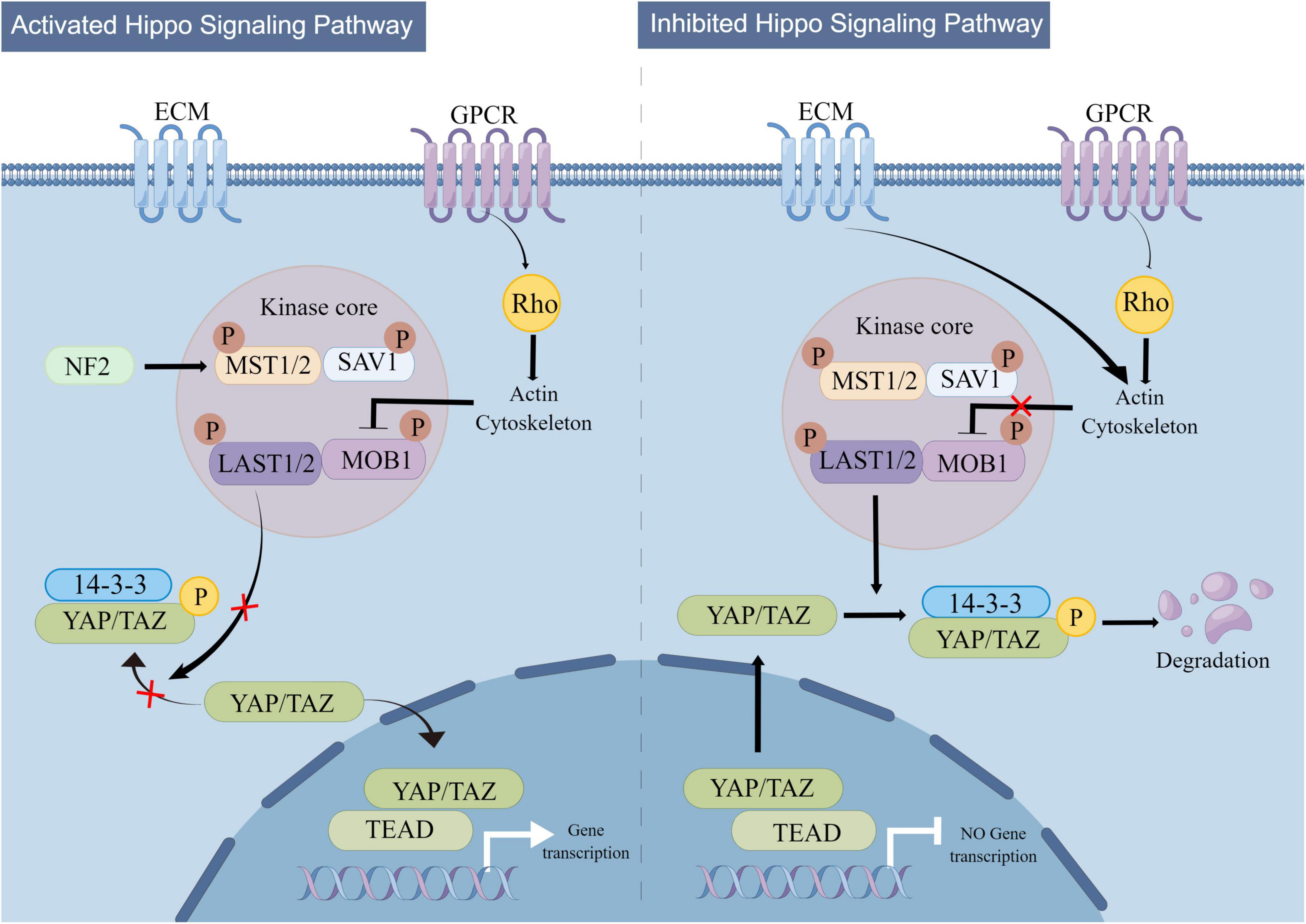
Figure 3. Mechanistic diagram of the Hippo signaling pathway. The Hippo signaling pathway is activated by a variety of upstream signals, such as mechanical signals from cell contacts and G protein-coupled receptor (GPCR) signaling, among others. Two key proteins, MST1/2 and LATS1/2, form the core of the kinase phosphorylation cascade in the Hippo signaling pathway. Various signals that stimulate the Hippo signaling pathway can affect the localization of YAP/TAZ within the cell, leading to changes in their binding to TEAD and regulation of downstream Hippo signaling pathway targets.
Two critical proteins, MST1 and its interacting protein LATS1/2, are involved in the kinase phosphorylation cascade that forms the central part of the Hippo signaling pathway (Lian et al., 2010; Meng et al., 2016). By interacting with LATS1/2, the MST1/2 protein contributes to the inhibition of cell proliferation and differentiation. The C-terminal SAV (Sav/Rassf/Hpo) domain of MST1/2, a serine/threonine kinase, can boost its activity when it forms a complex with the scaffold protein SAV1. Upon activation by specific molecules in cells, the MST1 protein forms a complex with LATS1/2, leading to the suppression of cell proliferation and differentiation and ensuring the continuity of the kinase phosphorylation cascade (Grijalva et al., 2014; Meng et al., 2016). The modulation of the MST1 and LATS1/2 proteins’ activities by the Hippo signaling pathway is pivotal in regulating cell growth and differentiation. The transcriptional coactivators Yes-associated protein (YAP) and PDZ-binding motif (TAZ) are the downstream effectors of the Hippo signaling pathway (Moya and Halder, 2019). YAP/TAZ have the ability to move back and forth between the nucleus and cytoplasm. In the absence of Hippo signaling pathway activity, YAP/TAZ relocate to the nucleus and serve as transcriptional coactivators by binding to DNA with TEAD 1-4. On the other hand, upon Hippo pathway activation, YAP/TAZ become phosphorylated and are prevented from entering the nucleus, which in turn promotes their function as transcriptional corepressors (Meng et al., 2016). The activation of the Hippo pathway leads to the suppression of YAP/TAZ function due to phosphorylation mediated by LATS1/2. Conversely, in the absence of Hippo pathway activity, YAP/TAZ become dephosphorylated and move to the nucleus, where they can engage with the transcription factors TEAD1-4 to trigger gene expression (Meng et al., 2016).
Normally, the Hippo signaling pathway proficiently manages cell growth and averts uncontrolled proliferation. Nevertheless, in specific instances, the pathway may lose its functionality, triggering abnormal growth and tumor formation. Various research studies have uncovered that the Hippo signaling pathway is frequently deactivated in tumor tissues, which facilitates cell proliferation and ultimately results in tumor advancement (Zheng and Pan, 2019). Enhancing our comprehension of the Hippo signaling pathway’s regulatory mechanisms could pave the way for innovative approaches to treat tumors (Lian et al., 2010; Elbediwy et al., 2016; Pobbati and Hong, 2020).
2.3.3. Molecular mechanisms of Hippo signaling pathway in stroke
The relationship between the Hippo signaling pathway and stroke is primarily centered around the two core targets of YAP/TAZ and MST1. While previous research has primarily focused on the role of the Hippo signaling pathway in regulating cell proliferation and differentiation in cancer, there is growing interested in its potential involvement in stroke (Zanconato et al., 2016). Recently, its therapeutic potential in cardiovascular diseases has been discovered (Dey et al., 2020). Microglial activation in the infarcted area following a stroke is a critical factor that can mediate oxidative stress-induced cell death (Davalos et al., 2005). After being recognized as a crucial pro-apoptotic factor in neuronal death triggered by oxidative stress, the emerging evidence of MST1’s potential participation in ischemia-reperfusion injury implies that it could be a potential therapeutic target for the treatment of neurodegenerative disorders (Li D. et al., 2018).
Siqi Zhao et al. established a correlation between cerebral ischemia-induced microglial activation and the Hippo/MST1 signaling pathway. They discovered that Src kinase functions as an upstream factor that facilitates this association (Zhao et al., 2016). Furthermore, YAP/TAZ, which is another key site within the Hippo signaling pathway, also appears to play an important role following a stroke (Zhao et al., 2016). Activation of YAP/TAZ by dexamethasone has been shown to reduce brain damage, and infarct size, improve neurological function and decrease blood-brain barrier permeability following a stroke (Gong et al., 2019). In a study by Luping Huang et al., it was found that XMU-MP-1 could induce the nuclear localization of YAP in astrocytes, resulting in reduced brain damage, decreased release of inflammatory factors such as Interleukin-1β (IL-1β) and Interleukin-6 (IL-6), and a decrease in astrogliosis (Huang et al., 2020). Other studies have demonstrated that verteporfin, a drug used in photodynamic therapy, can reduce Blood-Brain Barrier (BBB) permeability after stroke by inhibiting the nuclear expression of YAP. This helps to maintain BBB integrity and reduce brain damage (Gong et al., 2021).
2.3.4. Crosstalk between Hippo signaling and other signaling pathways
There is likely a crosstalk between the Hippo signaling pathway and other signaling pathways, such as the Wnt, Notch, and SHH pathways. Some of the most notable examples of pathway crosstalk are summarized below.
2.3.4.1. Crosstalk between Hippo signaling and Wnt signaling
The regulation of cell proliferation, differentiation, migration, and apoptosis relies significantly on Wnt signaling (Varelas et al., 2010). Wnt proteins serve as signaling molecules in this pathway, and the critical proteins and receptors involved include β-catenin, Dishevelled (Dvl), and Frizzled (Fzd) (Mccrea et al., 1991; Siegfried et al., 1994; Bhanot et al., 1996). The extranuclear negative regulator YAP can limit the activity of the Wnt/β-catenin signaling pathway by interacting with Dvl, modulating Glycogen Synthase Kinase 3 beta (GSK-3β) activity, and binding to β-catenin, affecting its nuclear translocation (Varelas et al., 2010; Tsai et al., 2012; Wang Y. et al., 2017). However, upon activation of the Wnt/β-catenin signaling pathway, β-catenin can evade degradation and inhibit TAZ degradation outside the nucleus, resulting in the co-accumulation of TAZ and β-catenin (Azzolin et al., 2012). Furthermore, through binding to the DNA enhancer located in the first intron of the YAP gene, the β-catenin / Transcription Factor 4 (TCF4) complex can trigger the expression of YAP, its downstream factor, in cells (Konsavage et al., 2012; Park et al., 2015). A study published in Cell has confirmed that YAP interacts with the Wnt/β-catenin signaling pathway, involving the transcription factor Thromboxane B5 (TXB5) and the beta-transducin repeat-containing protein E3 (b-TrCP E3) ligase (Azzolin et al., 2012, 2014; Tsai et al., 2012; Park et al., 2015).
2.3.4.2. Crosstalk between Hippo signaling and Notch signaling
The Notch signaling pathway is a crucial mechanism that governs cell differentiation and proliferation. This pathway holds immense significance in the field of biology, as it is responsible for regulating cell fate decisions and influencing embryonic development and stem cell differentiation (Andersson and Lendahl, 2014). By acting as a critical regulator of cellular differentiation, the Notch signaling pathway helps to ensure that cells develop into the correct types and that tissues and organs form correctly (Totaro et al., 2018). Several investigations have verified the substantial involvement of YAP1 in controlling the Notch signaling pathway in liver cancer. In particular, YAP1’s activation of Jag-1, the ligand responsible for instigating the Notch signaling cascade, has been demonstrated (Tschaharganeh et al., 2013). In addition, the conjugate of YAP and TEAD has also been found to exert regulatory effects on Notch signaling and other genes within the Notch signaling pathways (Yimlamai et al., 2014; Hansen et al., 2015).
Recent studies have shown that the activation of YAP/TAZ through mechanical cues, in conjunction with distant enhancers, can stimulate the expression of delta ligands and promote epidermal differentiation through the Notch signaling pathway. This process has a direct impact on the properties of somatic stem cells (SC), influencing their ability to differentiate and self-renew (Totaro et al., 2017). The interplay between YAP/TAZ and the Notch signaling pathway’s downstream effector is crucial in multiple biological processes, such as the development of hepatobiliary ducts, epidermis, and the pathogenesis of cancer (Totaro et al., 2018). Through its interaction with the Notch signaling pathway, YAP/TAZ influences cell fate decisions and regulates cellular proliferation, differentiation, and apoptosis, highlighting the complex interplay between different signaling pathways in various biological contexts. The emerging understanding of the role of YAP/TAZ and the Notch signaling pathway in various cellular processes underscores the need for further research into their mechanisms of action and potential therapeutic implications.
2.3.4.3. Crosstalk between Hippo signaling and SHH signaling
The SHH signaling pathway is accountable for specifying the body axis, arranging tissues and organs, and sustaining appropriate cell proliferation in tissues. By serving as a vital modulator of embryonic development, the SHH signaling pathway helps ensure the accurate differentiation of cells and the proper formation of tissues and organs (Varjosalo and Taipale, 2008). Recent studies have demonstrated that Yes-associated protein (YAP) is a target of oncogenic activation induced by the Sonic hedgehog (SHH) pathway (Fernandez et al., 2009). In cerebellar granule neuron precursors (CGNP), SHH signaling prompts the nuclear translocation of YAP1, which stimulates their proliferation (Fernandez et al., 2009) FoxO6–/– mouse studies have shown that the loss of SHH is associated with Hippo signaling (Sun et al., 2018). Furthermore, YAP has been shown to upregulate the expression of SHH, thereby contributing to bronchial morphogenesis (Isago et al., 2020). The exploration of the complex interplay between the Hippo signaling pathway and other signaling pathways in stroke remains incomplete. Our research builds upon prior studies to scrutinize plausible mechanisms of crosstalk between the Hippo signaling pathway and other pathways in the context of stroke. We provide a map below illustrating potential crosstalk between the Hippo signaling pathway and Wnt, Notch, and SHH signaling pathways (Figure 4):
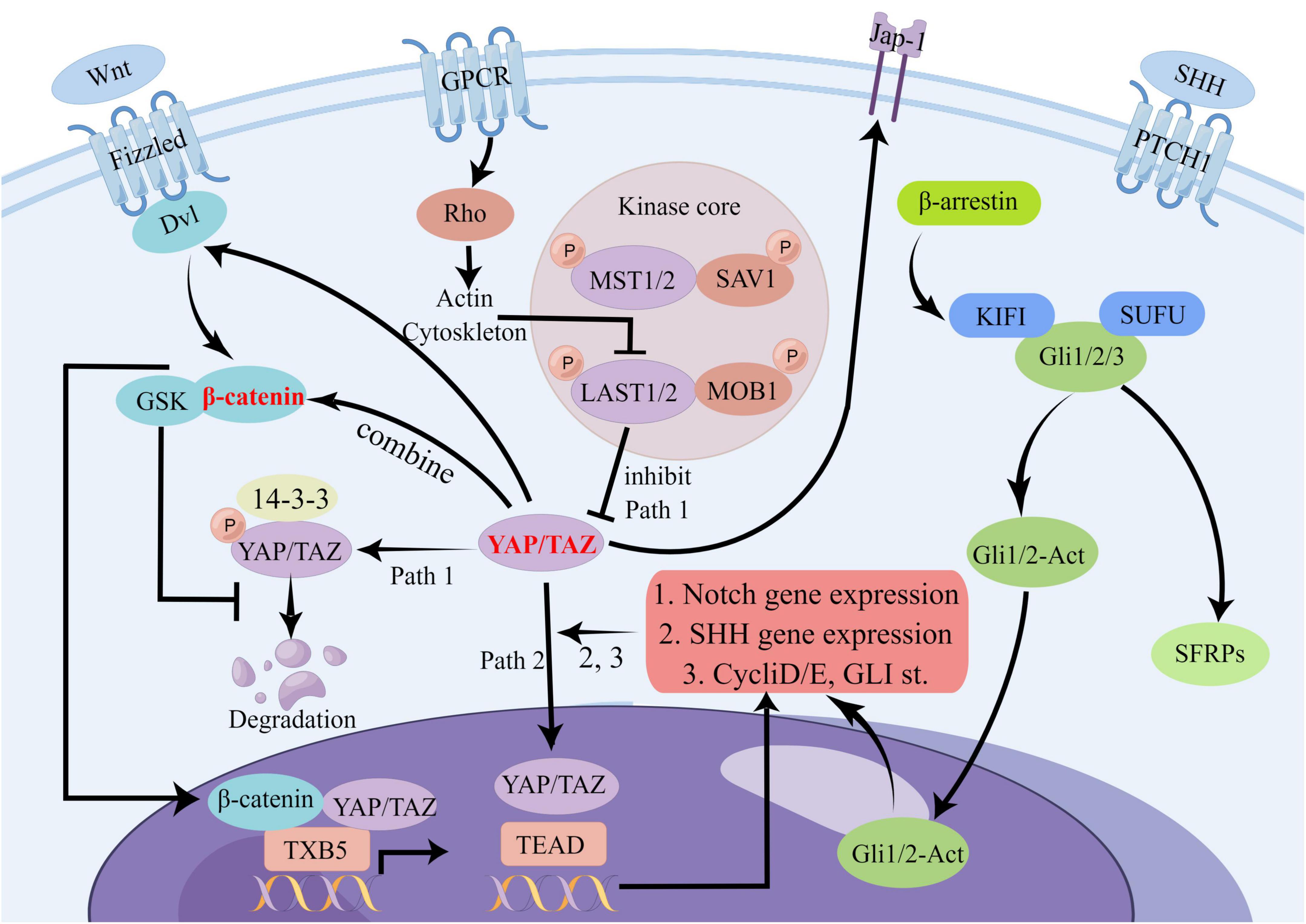
Figure 4. Potential crosstalk diagram between Hippo signaling pathway and Wnt, Notch, and SHH signaling pathways. In the Hippo signaling pathway, YAP/TAZ can influence several other pathways through crosstalk. For example, it can affect Dvl and β-catenin signaling in the Wnt pathway, JAP-1 receptor and downstream gene transduction in the SHH pathway, and the Notch pathway through binding to TEAD. This complex network of crosstalk represents a potential, albeit unproven, mechanism for stroke. There are many other points of crosstalk involving the Hippo signaling pathway in stroke, and we have highlighted some of the most significant ones.
2.4. Ferroptosis signaling pathway and stroke: regulating cell death
2.4.1. Research progress on ferroptosis of new cell death
In 1980, Bannai et al. made a groundbreaking discovery by identifying the antiporter protein cystine/glutamate transporter (xCT/SCL7A11), commonly known as system xC– (Bannai and Kitamura, 1980). Later on, Murphy’s research revealed that system xC- also has the potential to induce glutamate toxicity, a condition that damages brain cells and can lead to neurological disorders (Hirschhorn and Stockwell, 2019). Descriptions of the unique cell death caused by cystine deprivation, which is now known as ferroptosis, existed before its official naming. These descriptions included the role of reduced glutathione (Ratan et al., 1994), ceramide-induced non-apoptotic ROS-dependent cell death (D’Autréaux and Toledano, 2007), and the involvement of polyunsaturated fatty acids in glutathione peroxidase 4 (GPX4) knockdown-mediated cell death (Seiler et al., 2008). In 2003, Dolma et al. discovered that Erastin induces iron-dependent cell death (Dolma et al., 2003). In 2012, Dixon et al. officially identified ferroptosis as a distinct mode of cell death with unique mechanisms that differentiate it from traditional apoptosis (Dixon et al., 2012). Subsequently, Yang et al. identified GPX4 as a crucial target of ferroptosis (Yang et al., 2014). Acyl-CoA synthetase long-chain family member 4 (ACSL4) is recognized as a pivotal regulator of ferroptosis and is responsible for mediating sensitivity to this process (Doll et al., 2017). In 2019, James A. Olzmann, Marcus Conrad, and Jose Pedro Friedmann Angeli identified a new repressor of ferroptosis, Ferroptosis inhibitor protein 1 (FSP1) (Doll et al., 2019). Ferroptosis is thought to have a considerable impact on numerous diseases, such as neurodegenerative diseases, cardiovascular diseases, and cancer. As a result, scientists are investigating approaches to impede ferroptosis with the aim of creating potent therapeutic interventions. The research progress on ferroptosis of new cell death is shown in Figure 5.
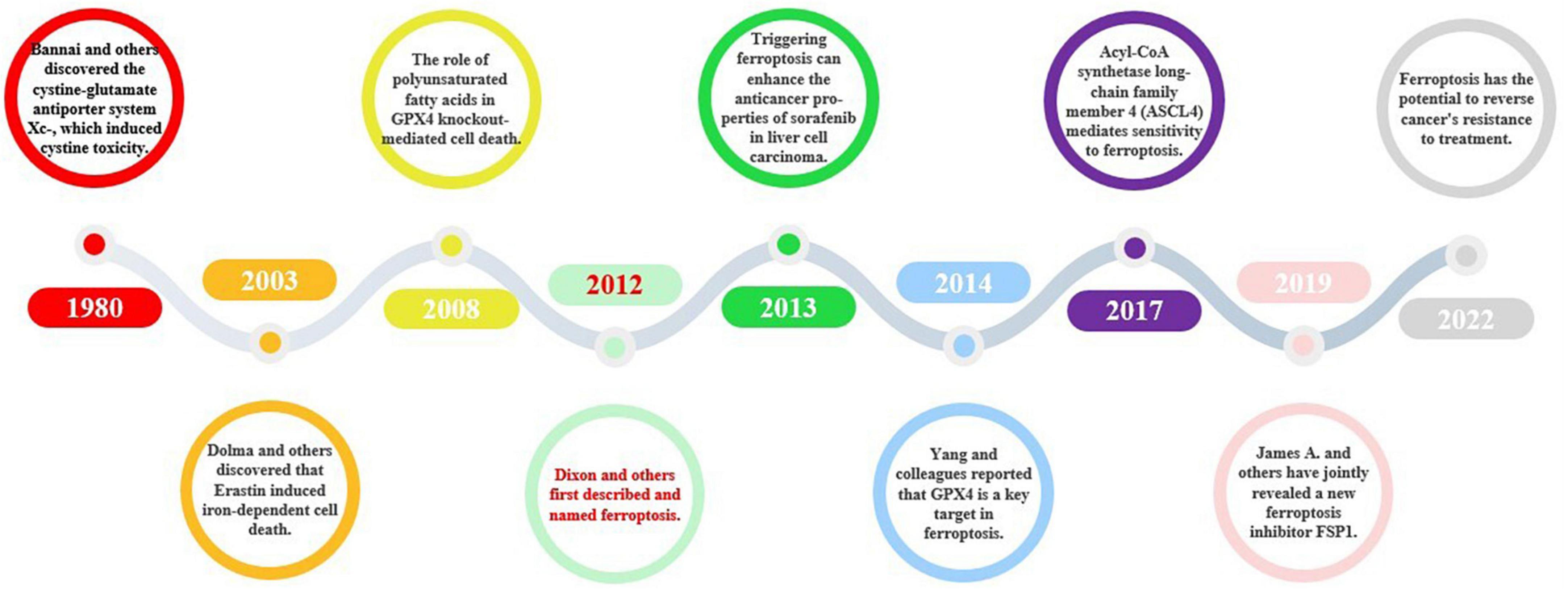
Figure 5. The historical course of ferroptosis. Ferroptosis, a novel form of cell death, was named in 2012, and since then, some conduits in the ferroptosis signaling pathway, such as the xC- system and GPX4, have been described. Further discoveries of key targets, such as Acyl-CoA synthetase long-chain family member 4 (ASCL4) and FSP1, have improved our understanding of the ferroptosis signaling pathway and elevated its importance. More recently, ferroptosis and its associated signaling pathways have been found to play a role in cancer, cardiovascular disease, and other fields.
2.4.2. Regulatory mechanisms of ferroptosis siganling pathway
Ferroptosis is an orchestrated process of cell death that encompasses various mechanisms and pathways, including iron metabolism, lipid peroxidation, and amino acid metabolism (Li J. et al., 2020; Zhang et al., 2021). The buildup of intracellular iron ions (Fe2+) can activate ferroptosis, underscoring its significance in the regulation of this process. Transferrin (TFRC) is a pivotal protein that facilitates the translocation of iron from extracellular to intracellular compartments and plays a crucial role in regulating iron-induced cell death (Yang and Stockwell, 2016; Li J. et al., 2020).
The cystine/glutamate antiporter xC- operates by exchanging glutamate with cystine in a 1:1 proportion. Nevertheless, excessive levels of glutamate can impede xC-’s function, inducing ferroptosis (Yang and Stockwell, 2016). Cystine is an indispensable component necessary for the biosynthesis of glutathione (GSH), a process catalyzed by glutamate-cysteine ligase (GCL) and glutathione synthetase (GSS). Nevertheless, curtailing xC-’s activity can diminish the uptake of cystine, ultimately impairing GSH synthesis (Yang and Stockwell, 2016; Zhang et al., 2021). As a consequence, the decrease in cystine uptake leads to a reduction in the activity of GPX4, an enzyme responsible for membrane lipid repair, as well as a decrease in the antioxidant capacity of cells. Ultimately, these effects promote the onset of ferroptosis (Zhang et al., 2021). Reactive Species-Generating Compound 3 (RSL3) is an influential ferroptosis elicitor that directly hinders the activity of GPX4, culminating in the diminished cellular antioxidant capability and buildup of reactive oxygen species (ROS), eventually instigating ferroptosis (Yang et al., 2014; Yang and Stockwell, 2016; Hirschhorn and Stockwell, 2019).
The quantity and distribution of polyunsaturated fatty acids (PUFAs) in a cell determine the degree of lipid oxidation and influence the occurrence of ferroptosis. Free PUFAs play a role in synthesizing lipid signaling molecules and are incorporated into membrane phospholipids. Following lipid oxidation, PUFAs transmit ferroptosis signals that induce cellular death (Hirschhorn and Stockwell, 2019; Li J. et al., 2020; Chen et al., 2021a). ACSL4 and lysophosphatidylcholine acyltransferase 3 (LPCAT3) are two critical enzymes involved in the synthesis and restructuring of PUFAs in membrane phospholipids (Doll et al., 2017; Chen et al., 2021a). These enzymes facilitate the incorporation of PUFAs into phospholipids, resulting in the formation of polyunsaturated fatty acid phospholipids (PUFA-PLs). PUFA-PLs are highly susceptible to free radical-induced oxidation, which is mediated by lipoxygenases (ALOXs). The oxidation of PUFA-PLs eventually leads to the breakdown of the lipid bilayer and disrupts membrane function, ultimately promoting ferroptosis (Hirschhorn and Stockwell, 2019; Li J. et al., 2020; Chen et al., 2021a).
2.4.3. Research progress on ferroptosis signaling pathway in stroke
Ferroptosis has gained significant attention in neuroscience and medicine and is now an important area of research (Hirschhorn and Stockwell, 2019). Research has indicated that ferroptosis inhibitors possess the potential to shield against degenerative brain illnesses such as Parkinson’s disease (PD), Huntington’s disease (HD), and Alzheimer’s disease (AD), alongside other types of neurodegenerative diseases and traumatic and hemorrhagic brain injuries (Stockwell et al., 2017; Hirschhorn and Stockwell, 2019; Zhang et al., 2021). Recently, there has been growing interest in the therapeutic potential of ferroptosis in treating heart disease and cancer (Chen et al., 2021a; Li N. et al., 2021).
Ferroptosis has been suggested to be linked to stroke, as the reduced blood supply to the brain during a stroke can lead to a depletion of intracellular iron ions (Fe2+), which may ultimately promote ferroptosis (Zhang et al., 2021). Additionally, The accumulation of intracellular reactive oxygen species (ROS) can be caused by a stroke, which can further promote ferroptosis. While the precise role of ferroptosis in stroke remains unclear, recent studies have suggested that inhibiting ferroptosis may help reduce stroke-related damage (Zille et al., 2017; Li Q. et al., 2017).
Several studies have shown the role and therapeutic potential of ferroptosis in stroke. For instance, ZILLE, M et al. demonstrated that both ferroptosis and necrosis markers were increased following in vitro and in vivo stroke, and the inhibition of these pathways led to increased cell survival (Zille et al., 2017). In their study, Yu Cui et al. showed that protecting against cerebral ischemia-induced ferroptosis can be achieved by knocking down ACSL4, a crucial enzyme that regulates the synthesis of PUFA. Conversely, the risk of cerebral ischemia was observed to increase with the overexpression of ACSL4 (Cui et al., 2021). The inhibitory effect of baicalein on ferroptosis has been demonstrated in two models—an in vitro model of oxygen-glucose deprivation/reperfusion (OGD/R) in HT22 cells, and a rat model of transient middle cerebral artery occlusion (tMCAO) induced by RSL3. Baicalein achieves this effect primarily by regulating the expression levels of GPX4, ACSL4, and ASCL3, which are key enzymes involved in ferroptosis (Duan et al., 2021). Astragaloside IV has demonstrated potential neuroprotective effects against ferroptosis induced brain injury after subarachnoid hemorrhage (SAH) by activating the Nrf2/HO-1 signaling pathway. This pathway reduces lipid peroxidation and increases antioxidant enzyme levels, including glutathione peroxidase 4 (GPX4). As a result, the reduction of lipid peroxidation can prevent ferroptosis from occurring (Liu Z. et al., 2022). Sikai Zhan et al. showed that Danhong injection has the potential to alleviate nerve cell ferroptosis after ischemic stroke in permanent middle cerebral artery occlusion (pMCAO) mice by activating the SLC7A11/HO-1 pathway (Zhan et al., 2022). The findings of these studies indicate that the targeting of ferroptosis may hold promise as a therapeutic strategy for treating stroke in the future.
2.4.4. Crosstalk between ferroptosis siganling pathway and other signaling pathways
Several cellular pathways, such as the AMPK, Wnt, and Hippo signaling pathways, may interact with ferroptosis, indicating a possibility of crosstalk between them.
2.4.4.1. Crosstalk between ferroptosis siganling and Wnt signaling
Currently, the connection between ferroptosis and Wnt signaling is not yet fully understood by researchers. However, studies suggest that there could potentially be an association between Wnt signaling and cell death, indicating a possible relationship between the two pathways. Specifically, research has shown that β-catenin may bind to the TCF4 transcription factor and activate the expression of GPX4 by binding to the promoter region of GPX4. This activation can then inhibit ferroptosis (Wang H. et al., 2022).
2.4.4.2. Crosstalk between ferroptosis siganling and AMPK signaling
The crosstalk between ferroptosis and the AMP-activated protein kinase (AMPK) signaling pathway has been extensively studied and established. Ferroptosis requires the phosphorylation of Beclin 1 (BECN1), and AMPK facilitates this process by directly activating the activity of BECN1. This activation leads to the initiation of autophagy, which subsequently inhibits ferroptosis by removing iron from the cell (Kang et al., 2018; Song et al., 2018). In a mouse model of renal ischemia/reperfusion injury, it was observed that activating AMPK during energy stress could reduce the pathological damage caused by ferroptosis and lower the levels of polyunsaturated fatty acids. Conversely, inactivating AMPK increased cell sensitivity to ferroptosis, suggesting the potential therapeutic significance of targeting the AMPK pathway in diseases related to ferroptosis (Lee et al., 2020; Li C. et al., 2020).
2.4.4.3. Crosstalk between ferroptosis siganling and Hippo signaling
YAP, a protein involved in the Hippo signaling pathway, plays a role in regulating the lipid peroxidation process of ferroptosis. It does so by acting on the ASCL4 target in the ferroptosis pathway, as well as through its action on NADPH Oxidase 4 (NOX4) (Yang W. H. et al., 2019; He et al., 2022). Although the intricate crosstalk mechanisms between ferroptosis and other signaling pathways in stroke have been sparsely investigated, our study delves into this area based on prior research. Our objective is to explore potential crosstalk mechanisms between ferroptosis and other signaling pathways in the context of stroke. The following is a map of potential crosstalk of signaling pathways between ferroptosis and Wnt, AMPK, and Hippo signaling pathways (Figure 6).
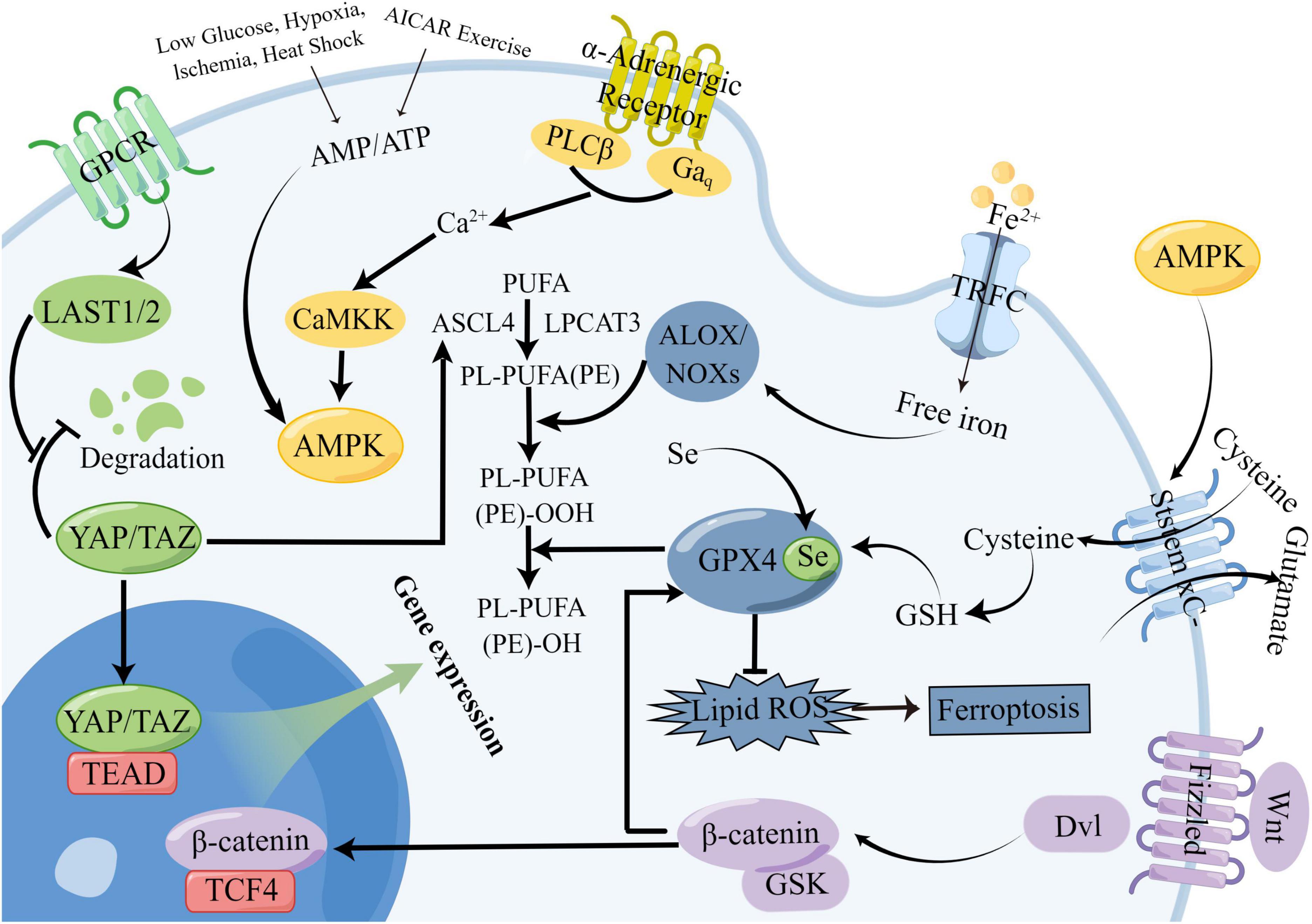
Figure 6. Potential crosstalk diagram between ferroptosis signaling pathway and Wnt, AMPK, and Hippo signaling pathways. The crosstalk between ferroptosis and other signaling pathways involves several components. One aspect involves β-catenin in the Wnt signaling pathway, which participates in the dialogue with ferroptosis signaling. Additionally, AMPK kinase affects glutamate transduction in ferroptosis signaling, while YAP/TAZ, key targets of the Hippo signaling pathway, impact the ASCL4 target in the ferroptosis signaling pathway.
2.5. Stroke is supposed to be a network disease: a complex network of pathways
Stroke is a complex network disease. The relationship and crosstalk among the signaling pathways involved in stroke are intricate and multifaceted. Apart from the Hippo signaling pathway and ferroptosis, pathways such as Wnt, AMPK, and Notch also contribute to stroke pathology. Upon scrutinizing the interplay between ferroptosis, Hippo signaling, and stroke, it is clear that the stroke signaling pathway encompasses a complex network of signaling pathways that interact and cross-talk with each other. This phenomenon of crosstalk is also evident in the historical evolution of stroke signaling pathways (Huang et al., 2013). Individual signaling pathways in stroke are involved in multiple pathophysiologies. For example, the Rho/Rock, Wnt/β-catenin, NO, and Vascular Endothelial Growth Factor (VEGF) signaling pathways, which contribute to angiogenesis, also play a role in neurogenesis, cell proliferation, and cell apoptosis (Menet et al., 2020; Lu et al., 2021; Hu Y. et al., 2022; Wang H. et al., 2022). Similarly, the SHH signaling pathway, which is associated with oxidative stress, affects anti-oxidation, anti-apoptosis, and the promotion of neurogenesis and angiogenesis (Huang et al., 2013). The Nrf2/ARE signaling pathway, which is involved in oxidative stress, is also associated with the inflammatory response and exhibits cross-talk with the NF-κB signaling pathway (Ahmed et al., 2017). The signaling pathway of HIF-1α plays a role in stroke-related processes such as inflammatory response, angiogenesis, and neuroprotection (Cheng et al., 2014; He et al., 2021). Peroxisome proliferator-activated receptor gamma (PPAR-α) agonists have the potential to protect against excessive oxidative stress, inflammation, and apoptosis following stroke (Collino et al., 2006; Luo et al., 2006; Fong et al., 2010). The signaling pathway of NF-κB plays a role in processes related to the inflammatory and immune response, as well as apoptosis (Brand et al., 1996; Pahl, 1999). The Notch signaling pathway, commonly associated with cell apoptosis, has been found to be increasingly associated with organogenesis and angiogenesis (Campos et al., 2002; Ito et al., 2002). The Hippo signaling pathway regulates organ volume and affects tissue regeneration by controlling apoptosis (Moya and Halder, 2019). The signaling pathway of TGF-β1/Smad3 serves a dual purpose of regulating both cell proliferation and apoptosis. In addition, it also holds significant importance in several physiological processes, including but not limited to inflammation, tissue repair, and the onset of cancer (Kang et al., 2009; Gough et al., 2021). The star signaling pathways in stroke, including the PI3K/AKT, JAK/STAT, AMPK, and MAPK pathways, play a role in angiogenesis, apoptosis, inflammation, autophagy, and oxidative stress (Jiang S. et al., 2018; Shariati and Meric-Bernstam, 2019; Arnold et al., 2021; Li N. et al., 2021). These pathways have multiple downstream targets and crosstalk with other pathways, contributing to various functions in stroke. Hence, exploring the mechanisms of these signaling pathways in stroke is crucial as they hold potential for treating stroke.
Secondly, taking individual signaling pathways as examples, it is also shown that there is a complex crosstalk relationship between stroke-related signaling pathways. The PI3K/AKT signaling pathway, considered a star pathway, participates in multiple functions such as oxidative stress, apoptosis, inflammation, and angiogenesis (Shariati and Meric-Bernstam, 2019). The mechanism of action is complex and involves a wide range of signaling pathways. Moreover, there are connections and crosstalk among the PI3K/AKT signaling pathway, HIF signaling pathway, and angiogenic NO signaling pathway (Ho et al., 2012; Szabo, 2017). Additionally, activated AKT has been found to protect against oxidative damage after stroke through the Nrf2/ARE pathway (Chan, 2005). The PI3K/AKT signaling pathway activation can inhibit the expression of pro-inflammatory factors stimulated by NF-κB, thereby reducing the inflammatory response (Xian et al., 2021). Studies have demonstrated that the PI3K/AKT signaling pathway promotes VEGF production, which induces angiogenesis after a stroke. Additionally, the activation of mTOR, a downstream target of PI3K/AKT, inhibits autophagy via the PI3K/AKT/mTOR signaling pathway (Chen J. et al., 2019; Yang et al., 2021). The Nrf2 signaling pathway, involved in oxidative stress, exhibits possible crosstalk with the MAPK and PI3K/AKT signaling pathways (Alfieri et al., 2011). The Notch signaling pathway has the potential for crosstalk with various other signaling pathways, including but not limited to Wnt, TGF-β/BMP, GSK-3β, Ras/MAPK, and autophagy signaling pathways (Hansson et al., 2004; Andersson et al., 2011; Sarin and Marcel, 2017). Stroke-related signaling pathways can affect other pathways through various axes, suggesting that stroke is a network disease with complex cellular signaling mechanisms.
Finally, the complex crosstalk relationship between signaling pathways forms a network of stroke signaling pathways. For example, in stroke, taurine can reduce ferroptosis after subarachnoid hemorrhage by affecting the crosstalk between the GABA/AKT/GSK3β/β-catenin axis and the Wnt and ferroptosis signaling pathways (Liu C. et al., 2022). Additionally, artesunate can inhibit the inflammatory response after ICH through the AMPK/mTORC1/GPX4 pathway by affecting the crosstalk between the AMPK signaling pathway and the ferroptosis signaling pathway (Xie et al., 2023). However, few experimental studies have investigated the crosstalk of the Hippo signaling pathway in stroke, which is a potential area of crosstalk that requires further investigation. Meanwhile, even though the crosstalk study of other signaling pathways has not been conducted in stroke, it demonstrates the complexity of signaling pathways in the human body. For example, the PI3K/AKT signaling pathway affects stroke through various pathophysiological mechanisms, such as oxidative stress, apoptosis, inflammation, and angiogenesis, and these pathways are not affected alone but overlap with each other (Shariati and Meric-Bernstam, 2019). When western drugs are used to treat stroke, they often target a single signaling pathway, which can lead to crosstalk between multiple pathways and affect only one aspect of stroke, such as inflammatory response or angiogenesis, without addressing the various pathophysiological mechanisms of stroke (Rikitake et al., 2005; Zacharek et al., 2009). This can result in poor therapeutic outcomes and potential side effects. In contrast, TCM has the characteristics of targeting multiple pathways and can act on stroke from multiple angles (Lou et al., 2022). Therefore, in proposing that stroke should be viewed as a network disease, new therapies need to be explored. Traditional Chinese medicine has the potential to play a multi-effect role in the treatment and improvement of stroke due to its multi-target and multi-pathway action. Research has shown that Compound Tongluo Decoction can inhibit endoplasmic reticulum stress and blepharoptosis, activate the SHH signaling pathway, and promote angiogenesis. This suggests that there may be potential crosstalk between the ferroptosis signaling pathway and the SHH signaling pathway in stroke (Hui et al., 2022). Although some scholars have proposed the network disease perspective for stroke, feasible evidence is still lacking (Lehnertz et al., 2023). Therefore, we have summarized the current status, advantages, and limitations of Western and traditional Chinese medicine in treating stroke, as well as the potential for combining these two approaches. Using the network disease perspective to view stroke can facilitate the development of new therapies, the discovery of the vast potential of traditional Chinese medicine, and the exploration of new possibilities for integrating traditional Chinese and Western medicine in stroke treatment.
3. Recent western medicine key treatment and trials of stroke
We summarize and outline the recent mature methods of western medicine in the treatment of stroke and the methods in the research stage. The prevention and treatment of ischemic stroke remains a challenging issue in the field of neurology. Advancing our understanding of the disease’s pathogenesis, developing effective treatment methods, and discovering novel drugs hold significant economic value and practical importance. Current clinical treatment options for stroke mainly focus on thrombolysis, antiplatelet and anticoagulant therapies, lipid-lowering medications, and non-surgical treatments such as alteplase and tissue plasminogen (tPA) administration, as well as surgical interventions like craniotomy thrombectomy and ventricular drainage (Donnan et al., 2008; Powers et al., 2019). Despite this, the existing western medicine treatment options still primarily rely on thrombolysis and vascular intervention.
3.1. Current existing clinical treatment methods of western medicine
Supplementary Table 3 summarizes the current clinical treatment methods for stroke, which primarily include intravenous thrombolysis, endovascular therapy, and drug therapy. Intravenous thrombolysis typically involves the use of alteplase, urokinase, and tirofiban. This method helps to reduce the incidence of stroke and increase blood perfusion in the ischemic area, but it carries a serious risk of bleeding. Additionally, the treatment time window for stroke is crucial in intravenous thrombolysis, as appropriate treatment timing plays a significant role in neurological recovery following a stroke (Wardlaw et al., 2014; Powers et al., 2019). The emergence of the third-generation thrombolytic enzyme, tirofiban, with a faster injection time and improved efficacy, poses a challenge to the primary clinical use of alteplase. However, its clinical application remains controversial due to a lack of sufficient clinical evidence to support its use (Singh et al., 2023). Mechanical thrombectomy is considered the preferred option for endovascular treatment, though the clinical effectiveness of arterial thrombectomy requires further evaluation (Hlavica et al., 2015). Drug therapy for stroke mainly consists of antiplatelet and neuroprotective medications. Aspirin and other antiplatelet drugs are limited in their efficacy due to the risk of bleeding, while the clinical effectiveness of neuroprotective drugs needs to be further evaluated in larger clinical trials (Greer, 2010; Martí-Carvajal et al., 2020). However, it is challenging to avoid the toxic side effects associated with drug therapy. For instance, edaravone is known to cause kidney and liver toxicity (Lapchak, 2010). Other treatments, such as oxygen therapy, anticoagulation, volume expansion, vascular dilation, and defibrination, have minimal evidence of effectiveness in treating stroke and are considered marginal treatments in clinical practice. Due to their limitations, these treatments are rarely used in clinical practice (Sandercock et al., 2008; Chang and Jensen, 2014; Powers et al., 2019). Figure 7 presents a summary of the historical evolution of key Western medical treatment approaches.
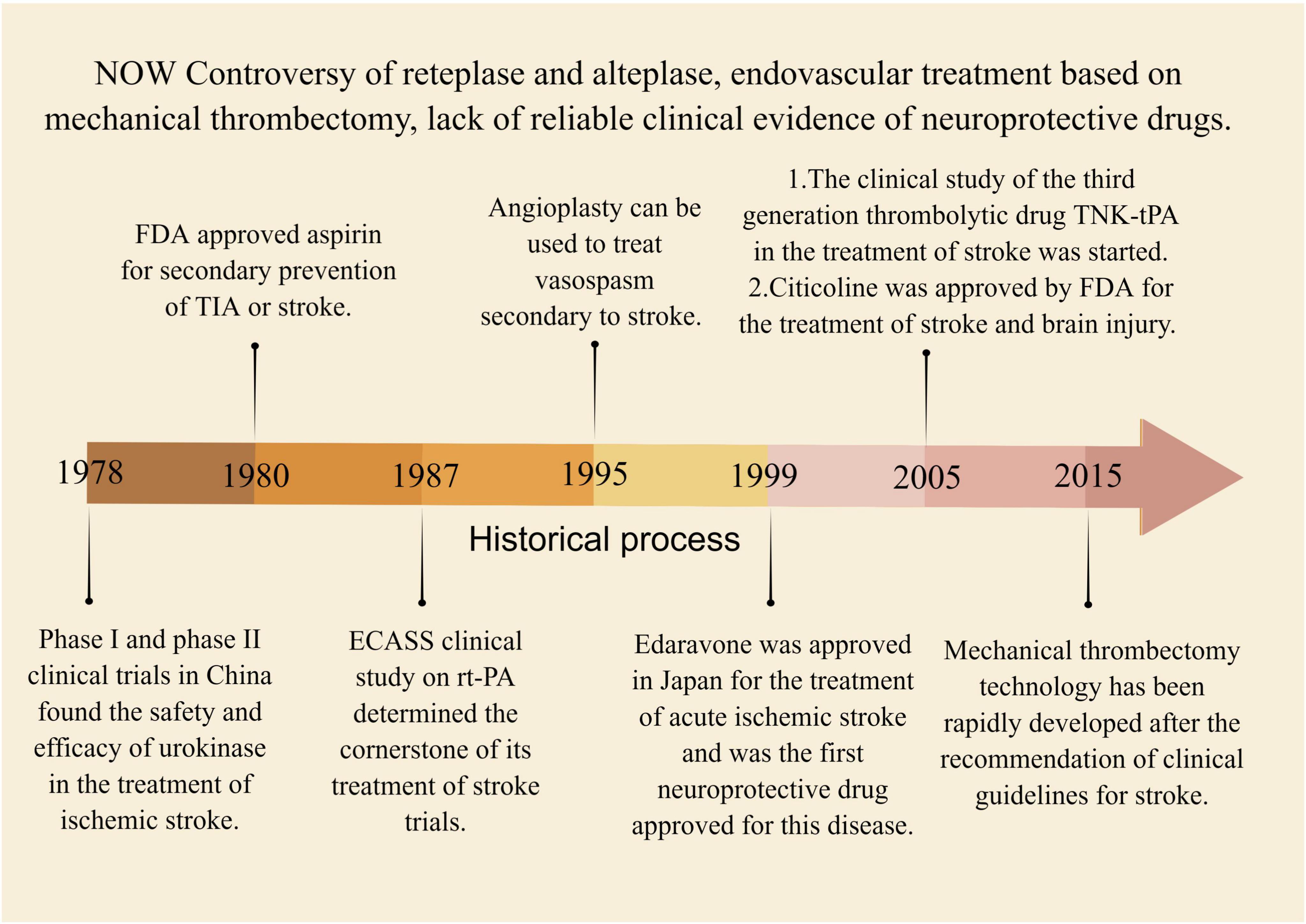
Figure 7. The historical process of key western medical methods in the treatment of stroke. Between 1978 and 2015, several intravenous thrombolytic therapies were discovered, including urokinase, alteplase, and tenecteplase. Concurrently, endovascular therapy emerged as a potential treatment for stroke, but lacked high-quality evidence to be confirmed by clinical trials.
3.2. Current western drug treatment in the research and development stage
Supplementary Table 4 provides a summary of various western drugs for the treatment of stroke that are currently undergoing animal experiments or clinical trials. Among them, Fasudil, a Rock inhibitor, has shown promising results in reducing the area of cerebral infarction and is used to treat subarachnoid hemorrhage by targeting the Rho/Rock signaling pathway of angiogenesis in stroke (Rikitake et al., 2005; Shibuya et al., 2005; Shimokawa and Takeshita, 2005). Another drug, rosiglitazone (RSG), has demonstrated the ability to reduce the release of inflammatory factors and decrease the damage of recurrent stroke by activating PPAR-γ, but its clinical use is limited (Culman et al., 2007; Li et al., 2019). Metformin has demonstrated potential to reduce the risk of stroke by activating AMPK phosphorylation, suppressing NF-κB activation, and decreasing the levels of inflammatory factors such as IL-6, IL-1β, Tumor Necrosis Factor alpha (TNF-α), and Intercellular Adhesion Molecule 1 (ICAM-1) (Liu et al., 2014). Clinical drugs with specific pharmacological effects can have additional targets and sites of action, highlighting the importance of careful monitoring during clinical use to discover new applications that may improve therapeutic outcomes for patients with multiple diseases and reduce drug development costs. While Western medicine is frequently utilized for ischemic stroke treatment, it typically targets only one aspect of the disease and may have significant adverse effects. Despite the emergence of new drugs, few have been proven to be effective, and many Western drug trials focus predominantly on animal studies rather than clinical translation. Western medicine’s efficacy in treating stroke is limited, and it faces obstacles such as high research and development expenses, lengthy clinical trial periods, and restricted therapeutic benefits (Liu et al., 2018). Stroke is a multifaceted neurological disorder that involves numerous signaling pathways. As a result, Western drugs that target a single aspect of stroke have limited efficacy and often cause unwanted side effects.
4. Current TCM treatment improves and treats stroke through multiple targets and pathways
Ischemic stroke, from the perspective of TCM, falls under the category of “stroke”. It is considered a syndrome of deficiency of essence and standard, where the accumulation of phlegm and blood stasis and the obstruction of brain vessels are the main pathogenesis. Therefore, promoting blood circulation and removing stasis is the main treatment approach. TCM is known for its multi-target and multi-pathway treatment approach. By acting on multiple targets of stroke signaling pathways and affecting various pathophysiological mechanisms, it can exert multi-angle treatment and improvement effects (Chen S. et al., 2022; Lou et al., 2022). Chinese patent drugs like Danhong injection and Danqi capsule have shown promising results in the prevention and treatment of ischemic stroke. Acupuncture, another TCM treatment, has also shown potential in the treatment of ischemic stroke (Liu et al., 2018; Chen S. et al., 2022). TCM is gaining wide recognition and acceptance globally (Liu et al., 2018). During the outbreak of COVID-19, TCM played a significant role in epidemic prevention, treatment, and rehabilitation. The unique benefits of TCM in preventing and treating chronic and complex multifactorial conditions, especially in cardiovascular and cerebrovascular diseases such as stroke, have gained significant attention. With a mature theoretical foundation, TCM has shown promising clinical outcomes in the prevention and treatment of these diseases (Liu et al., 2018; Zhan et al., 2022). Supplementary Table 5 summarizes TCM, and their active components for the treatment and improvement of stroke. The following provides an overview of the research status of key TCM, such as Scutellaria baicalensis, Astragalus membranaceus, Rehmanniae radix, and their active components in the treatment of stroke. The treatment of stroke with key TCM and its active ingredients is shown in Figure 8.
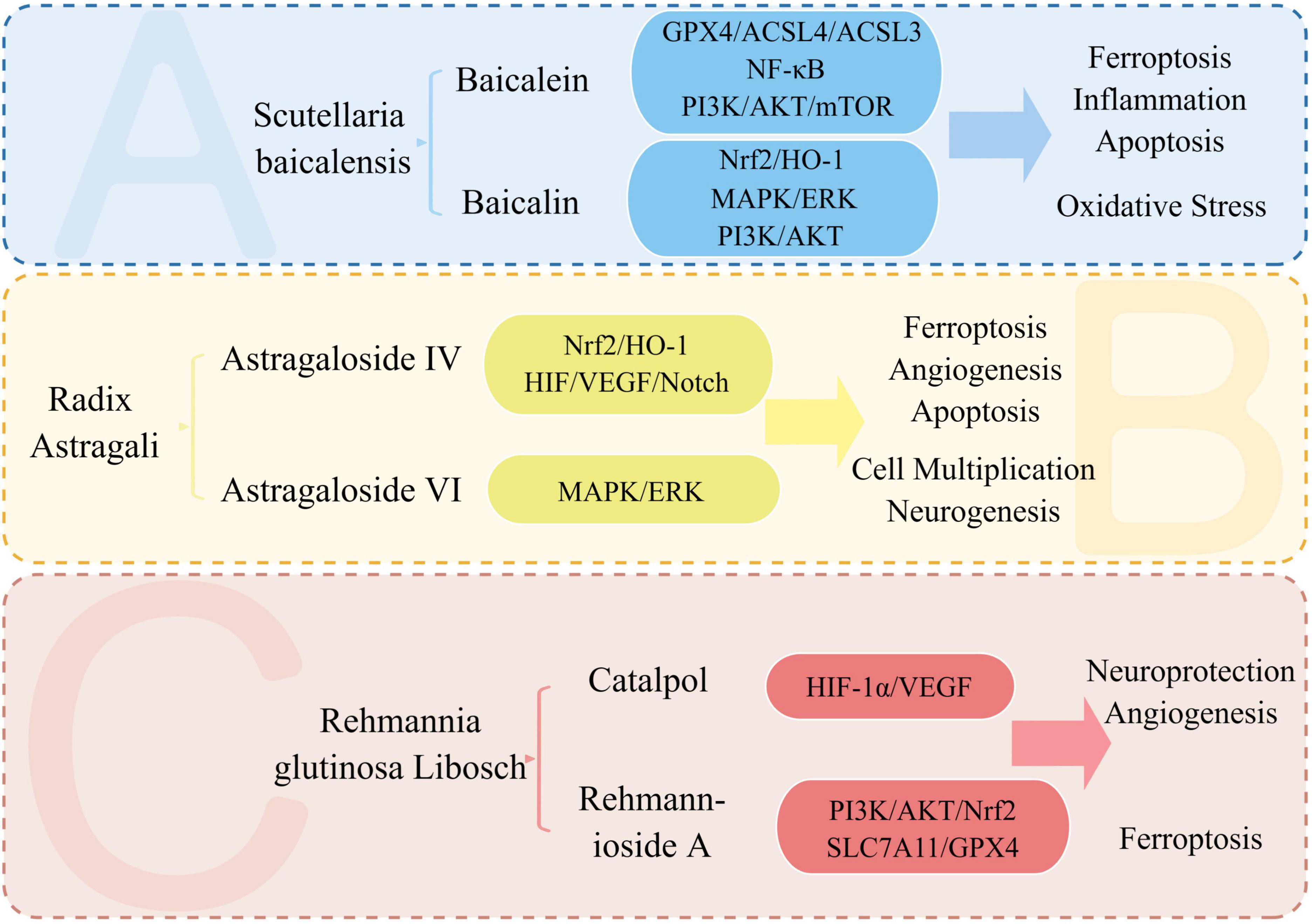
Figure 8. Treatment of stroke with key TCM and its active ingredients. We summarize the signaling pathways and pathophysiological mechanisms involved in the treatment of stroke with the active ingredients of Astragalus, Scutellaria, and Rehmanniae. Baicalein and baicalin, two active components, impact the pathophysiological mechanisms of stroke, including ferroptosis, oxidative stress, inflammation, and apoptosis. Meanwhile, Astragalus’ active ingredient, astragaloside IV, plays a neuroprotective role by affecting ferroptosis and angiogenesis in stroke.
4.1. Scutellaria baicalensis
Scutellaria baicalensis, also known as Scutellaria Baicalensis, is a Chinese medicine used for clearing heat and drying dampness. According to the Compendium of Materia Medica, it is used to treat various conditions, such as wind and heat, dampness and heat, headache, heat pain of running the dolphin, asthenia of lung, fishy throat, and blood loss. The plant contains baicalin, baicalein, astragaloside iv, and other compounds that have hemostatic and fetal safety properties. Baicalein, a flavonoid with the highest content in Scutellaria baicalensis, has been found to improve blood-cerebral circulation and possess anticoagulant properties. Studies by Li, M. et al. and Yang, S. et al. have demonstrated that baicalein can inhibit ferroptosis and neuronal apoptosis, reduce cerebral infarction area, and regulate signaling pathways such as GPX4/ACSL4/ACSL3 and NF-κB (Yang S. et al., 2019; Li et al., 2022). Baicalin, another flavonoid in Scutellaria baicalensis, has anti-thrombotic and anti-inflammatory activities. Research conducted by Huang, Z. et al. and Duan, L. et al. has demonstrated that baicalin has the ability to activate the Nrf2-HO-1 signaling pathway, reduce reactive oxygen species, and inhibit ferroptosis, resulting in a reduction of brain injury (Duan et al., 2021; Huang et al., 2021a). Baicalin has also been found to activate the PI3K/AKT signaling pathway, up-regulate glutamate transporter 1, increase the release of Brain-Derived Neurotrophic Factor (BDNF) and Tropomyosin Receptor Kinase B (TrKB), and exert antioxidant, anti-inflammatory, and neuroprotective effects during OGD/R, according to studies by Zhou et al. (2017) and Li C. et al. (2020).
4.2. Astragalus mongholicus
”Qi is the beauty of blood, and blood is the mother of qi” is a TCM theory that emphasizes the interdependence of qi and blood. To activate blood circulation, it is necessary to first promote the movement of qi. Astragalus membranaceus, known for its qi-promoting properties, can tonify qi, discharge pus, and benefit water, which indirectly helps to promote blood circulation and remove blood stasis. Its main component is astragaloside IV. Sun et al. conducted a study where they found that Astragaloside IV promoted neurogenesis and neural stem cell proliferation after stroke in a photochemical ischemia model (Sun et al., 2020). Liang, C. et al. discovered that Astragaloside IV can significantly reduce infarct size in the MACO/R model by activating the HIF/VEGF/Notch signaling pathway, increasing miRNA-210 expression, and promoting angiogenesis and cell proliferation (Liang et al., 2020). In a study by Liu et al., it was discovered that Astragaloside IV could activate the Nrf2/HO-1 signaling pathway, which in turn increased the levels of SLC7A11, GPX4, and ROS. This activation led to an enhanced antioxidant capacity and inhibition of lipid peroxidation in an ICH model with intravascular perforation (Liu Z. et al., 2022). Chen, X. et al. discovered that Astragaloside VI has the ability to target the MAPK signaling pathway that is EGF-mediated. Through activation of the EGFR/MAPK cascade, this promotes functional repair, neurogenesis, and nerve cell proliferation in MCAO models (Chen J. et al., 2019).
4.3. Rehmannia glutinosa
Rehmanniae is a form of TCM available in raw and processed forms that provides benefits such as improved blood circulation, elimination of blood stagnation, and nourishing Yin while promoting body fluids. Studies by Wang, H et al. have found that Catalpol, a compound extracted from Rehmanniae, exhibits neuroprotective properties by reducing brain damage in both in vivo MCAO/R models and in vitro OGD/R models, while also encouraging the growth, movement, and formation of blood vessels in brain microvascular endothelial cells (Wang et al., 2020). Fu, Y. et al. found that Rehmannioside A, in the MCAO/R model, can reduce cognitive dysfunction, nerve damage, and suppress ferroptosis by activating the PI3K/AKT/Nrf2 and SLC7A11/GPX4 signaling pathways (Fu et al., 2022). Astragalus membranaceus, Scutellaria baicalensis, and Rehmanniae rehmanniae are important Chinese herbs that have been used to treat cardiovascular and cerebrovascular diseases. Studies have shown that the active ingredients in these herbs have multiple therapeutic effects on stroke by targeting various pathways, including angiogenesis, inflammatory response, apoptosis, and oxidative stress. The treatment of stroke with TCM and its active components involves multiple signaling pathways, including the widely-acting PI3K/AKT and ferroptosis signaling pathways. This suggests that the treatment of stroke with TCM and its active components involves overlapping effects on multiple signaling pathways, rather than targeting a single pathway.
5. The difference and combination of traditional Chinese and Western medicine in the treatment of stroke
In the treatment of stroke, there are differences, advantages, disadvantages, and similarities between the pharmacological mechanisms of traditional Chinese medicine and Western medicine. Integrated traditional Chinese and Western medicine is becoming more common in the treatment of stroke. It is important to continue exploring the potential of traditional Chinese medicine and discovering new possibilities for combining traditional Chinese and Western medicine to provide better treatment options for stroke patients.
Western medicine utilizes drug therapy (such as intravenous thrombolysis and antiplatelets) and surgical treatments (like mechanical thrombectomy) in the treatment of stroke. These methods can help improve blood circulation and lower blood pressure, thus reducing the risk of stroke. For example, thrombolytic agents can dissolve clots, restore blood flow, and reduce the risk of ischemia. Antihypertensive drugs can lower blood pressure and reduce the risk of brain hemorrhage. However, drug therapy may also lead to adverse reactions, such as bleeding caused by thrombolytic agents (Wardlaw et al., 2014; Powers et al., 2019). Some drugs used in the treatment of stroke require long-term use, which can lead to drug resistance and dependence. In addition, surgical treatment is also an option, which can help reduce the risk of stroke by removing vascular stenosis or repairing aneurysms. For example, aneurysm surgery can prevent rupture and thus reduce the risk of intracerebral hemorrhage. However, surgical treatment may also carry surgical risks and complications, such as postoperative infection and bleeding (Powers et al., 2019; Krishnan et al., 2021). Surgical treatment may also require a longer period of rehabilitation compared to drug therapy. Additionally, Western medicine generally follows a single-target, single-pathway approach in the treatment of stroke, such as the use of intravenous thrombolytic drugs (Powers et al., 2019).
Traditional Chinese Medicine (TCM) can treat stroke through multiple targets and pathways with less risk of adverse reactions. TCM can act on various signaling pathways, such as the PI3K/AKT and SLC7A11/GPX4 pathways, which are involved in ferroptosis, angiogenesis, and neuroprotection. This multi-target approach may lead to more comprehensive and effective treatment of stroke (Fu et al., 2022). However, due to the individualized nature of TCM intervention and the lack of feasible blinding methods, it can be difficult to conduct randomized controlled trials, leading to a limited number of high-quality clinical trials for TCM in the treatment of stroke (Yang et al., 2016; Feng et al., 2022). Despite these challenges, acupuncture, a traditional Chinese medicine treatment, has gained recognition and has been shown to effectively treat stroke (Yang et al., 2016).
In the practice of integrated traditional Chinese and Western medicine for the treatment of stroke, traditional Chinese medicine, acupuncture, massage, and other TCM therapies are often combined with conventional Western medicine and surgical methods. When used in stroke rehabilitation, the combination of TCM and Western medicine has been shown to be more effective than Western medicine alone, as it can improve neurological deficits, reduce adverse reactions, and lead to better patient outcomes. This also highlights the superior efficacy of acupuncture when combined with Western medicine (Zhong L. L. et al., 2022; Hao et al., 2023). Traditional Chinese herbal medicine (TCHM) has been used as a single or adjuvant treatment for stroke, working through a variety of mechanisms such as anti-inflammation, anti-oxidative stress, anti-apoptosis, regulation of BBB, inhibition of platelet activation, and promotion of neurogenesis and angiogenesis. TCHM provides a valuable resource for the development of therapeutic drugs to treat stroke and for the discovery of more effective and safer combination therapy or individual treatment methods (Hao et al., 2023).
6. Problems and prospects
A variety of signaling pathways are associated with stroke, including angiogenesis (such as the Rho/Rock, Wnt/β-catenin, and NO signaling pathways), oxidative stress (including the Nrf2/ARE and SHH signaling pathways), immune inflammation (such as the NF-κB and TLRs signaling pathways), autophagy (including the Bnip3 signaling pathways), apoptosis (including the Notch and Hippo signaling pathways), ferroptosis, cuproptosis, and others such as the PI3K/AKT, MAPK, AMPK, and JAK/STAT signaling pathways. All of these pathways are interconnected, creating a complex network of signaling pathways involved in the pathophysiology of stroke. Stroke is a complex network disease that involves a diverse range of pathophysiological mechanisms and signaling pathways. As a result, investigating stroke requires thorough examination of various cross-talk issues.
Western medicines typically treat stroke through a single target, but due to differences in genotype and phenotype among patients, there can be poor effectiveness and significant variation in the treatment’s effects. Moreover, some drugs can cause liver damage, renal toxicity, and other side effects (O’Rourke et al., 2004). Currently, thrombolytic therapy and intravascular therapy, such asrecombinant tissue plasminogen activato (rt-PA), are still the most widely recognized treatments for stroke, often combined with auxiliary measures that have a broad range of indications (Herpich and Rincon, 2020). However, the bleeding risk to patients and the time window for thrombolysis are significant issues that cannot be ignored. Therefore, stroke centers have been established, and basic and complete pre-hospital management of stroke has been introduced in many countries (Barthels and Das, 2020). The opening of “stroke green channels” and the use of internet technology to assist with hospital admissions have also been implemented. However, these measures can be challenging to implement in developing countries, such as those in Asia and Africa, where there is limited investment and insufficient technical support. Therefore, reducing the onset symptoms of patients and extending the time window for treatment could provide significant support for the diagnosis, treatment, and prognosis of stroke (Powers et al., 2019). Due to the network nature of stroke, Western drugs that target a single pathway or target may have limitations and potential side effects.
TCM compounds or preparations and their effective ingredients have the advantage of targeting multiple pathways and targets simultaneously, including PI3K/AKT, NF-κB, and iron death pathways, and regulating various pathophysiological mechanisms such as apoptosis, oxidative stress, and inflammation in stroke (Liu et al., 2018). However, the mechanism of action of TCM remains unclear and involves effects that are multi-component, involve multiple pathways, and target multiple aspects, which is a bottleneck for the modernization and internationalization of TCM (Liu et al., 2018; Zhu et al., 2022). To overcome this, modern scientific and technological means such as network pharmacology, metabolomics, and proteomics can be used to study the effect and mechanism of TCM in stroke treatment (Kibble et al., 2015). Researchers need to clarify the signaling pathway network of stroke, identify the active ingredients in TCM, and strengthen pharmacodynamic and pharmacokinetic studies to clarify their pharmacological and toxic effects. Compound Chinese medicines have shown neuroprotective and damage-reducing effects on stroke in animal experiments, but their regional promotion is limited to clinical trials in China. Therefore, it is crucial to develop these compound Chinese medicines and promote them globally (Liu et al., 2018; Lou et al., 2022). TCM has shown to have fewer side effects and can be widely promoted in developing countries, especially in Asia and Africa, for the prevention and treatment of stroke. Moreover, TCM can also be used to assist the diagnosis, treatment, and recovery of stroke in developed countries (Sarfo et al., 2023). It is crucial to develop TCM suitable for stroke treatment, as it can be used as a means to extend the time window of stroke, retard the advancement of the ailment, and improve the prognosis and rehabilitation (Liu et al., 2018; Zhu et al., 2022). Therefore, the multi-target and multi-pathway treatment approach of TCM aligns with the complex network nature of stroke as a disease.
Network pharmacology has emerged for drug target discovery, experimental design, mechanism study, and efficacy evaluation in the exploration of TCM treatment strategies for stroke (Kibble et al., 2015; Zheng et al., 2022). It is a necessary, conditional, directional, and innovative approach. However, while network pharmacology is a hot topic, the credibility and professionalism of the network pharmacology databases need to be improved, and some network pharmacology articles remain limited to data mining. Although network pharmacology combined with experimental verification is reliable for TCM treatment of stroke, research on TCM in stroke models is still limited to preliminary experimental research and lacks clinical translation (Zheng et al., 2022).
In summary, stroke is a complex disease involved with numerous signal pathways, which makes it difficult to treat. Some western drugs like tissue plasminase (tPA) and aspirin have been used clinically, they have limited effects and side effects. TCM possesses significant potential in the treatment of stroke and is a valuable resource in this regard. However, stroke treatment research has been mostly limited to animal experiments and lacks clinical transformation (Liu et al., 2018; Powers et al., 2019; Zhu et al., 2022). It is essential to explore the potential therapy of integrated traditional Chinese and western medicine in the treatment of stroke. By combining the advantages of both approaches, this therapy can complement each other’s shortcomings, add high-quality evidence, reduce adverse reactions, and increase drug efficacy. TCM is a vast treasure trove, and there are many potential drugs that can be developed from it. Therefore, in the future, efforts should be directed towards excavating and developing the TCM treasure trove (Duan T. et al., 2022; Feng et al., 2022; Zhong L. L. et al., 2022; Hao et al., 2023). Additionally, it is essential to focus on the development of TCM placebo and randomized controlled trials to improve the credibility of TCM for stroke treatment. Network pharmacology and metabolomics can be used to mine data and conduct theoretical research in combination with Chinese traditional medical codes to find strong evidence to support the potential therapeutic effect of TCM. It is crucial to carry out clinical practice of TCM and its effective ingredients and to discover the possibility of treating stroke with integrated Chinese and western medicine. Multi-mode treatment of stroke can be explored to improve the possible direction of stroke treatment (Yang et al., 2016; Liu et al., 2018; Duan T. et al., 2022; Zhu et al., 2022). In conclusion, we propose embracing a network disease perspective when considering stroke, as it highlights the significance of acknowledging the multifaceted nature of stroke, including its multiple targets, pathways, and channels. By adopting this approach, we can facilitate the development of novel therapeutic strategies to effectively treat stroke.
Author contributions
BC drafted the manuscript. WJ provided the analysis and helped in the interpretation of results. Both authors approved the final version of the manuscript.
Funding
This work was jointly supported by the National Natural Science Foundation of China (81904083) and Natural Science Foundation of Zhejiang Province (LY22H270002). Figures 1, 3, 4, 6–8 are made by Figdraw (www.figdraw.com).
Conflict of interest
The authors declare that the research was conducted in the absence of any commercial or financial relationships that could be construed as a potential conflict of interest.
Publisher’s note
All claims expressed in this article are solely those of the authors and do not necessarily represent those of their affiliated organizations, or those of the publisher, the editors and the reviewers. Any product that may be evaluated in this article, or claim that may be made by its manufacturer, is not guaranteed or endorsed by the publisher.
Supplementary material
The Supplementary Material for this article can be found online at: https://www.frontiersin.org/articles/10.3389/fnins.2023.1200061/full#supplementary-material
Abbreviations
TCM, traditional Chinese medicine; Hippo, Salvador/Warts/Hippo (SWH); SHH, Sonic Hedgehog (SHH) signaling pathway; Nrf2/ARE, nuclear factor erythroid 2-related factor 2/antioxidant response element; HIF-1 α, hypoxia-inducible factor 1 alpha; PI3K/AKT, phosphatidylinositol 3-kinase/protein kinase B; JAK/STAT, Janus kinase/signal transducer and activator of transcription; AMPK, AMP-activated protein kinase; COVID-19, coronavirus disease 2019; MAPK, mitogen-activated protein kinase; ROS, reactive oxygen species; PCD, programmed cell death; YAP/TAZ, yes-associated protein/transcriptional co-activator with PDZ-binding motif; TEAD 1-4, TEA domain family members 1-4; LATS1/2, the hippo pathway is a key kinase that relays phosphorylation signals to effector molecules; MST1/2, the mammalian homolog of the core kinase Hippo protein; BBB, blood-brain barrier; IL-1 β, interleukin-1 β; IL-6, interleukin-6; TCF4, transcription Factor 4; GSK-3 β, glycogen synthase kinase 3 beta; β -catenin, a protein that plays a key role in the Wnt signaling pathway; TXB5, thromboxane B5; b-TrCP E3, beta-transducin repeat-containing protein E3; GPX4, glutathione peroxidase 4; FSP1, ferroptosis inhibitor protein 1; RSL3, reactive species-generating compound 3; xCT/SCL7A11, xCT, also known as SLC7A11, stands for cystine/glutamate transporter; OGD/R, oxygen-glucose deprivation/reperfusion; ASCL4, acyl-CoA synthetase long-chain family member 4; tMCAO, transient middle cerebral artery occlusion; pMCAO, permanent middle cerebral artery occlusion; SAH, subarachnoid hemorrhage; BECN1, Beclin 1 is a protein that plays a key role in the regulation of autophagy; NOX4, NADPH Oxidase 4; VEGF, vascular endothelial growth factor; TNF- α, tumor necrosis factor alpha; ICAM-1, intercellular adhesion molecule 1; BDNF, brain-derived neurotrophic factor; TrKB, tropomyosin receptor kinase B; ICH, intracerebral hemorrhage; rt-PA, recombinant tissue plasminogen activator; PPAR- γ, peroxisome proliferator-activated receptor gamma; TNK-tPA, tenecteplase.
References
Abdel-Latif, R. G., Rifaai, R. A., and Amin, E. F. (2020). Empagliflozin alleviates neuronal apoptosis induced by cerebral ischemia/reperfusion injury through HIF-1α/VEGF signaling pathway. Arch. Pharm. Res. 43, 514–525. doi: 10.1007/s12272-020-01237-y
Agashe, R. P., Lippman, S. M., and Kurzrock, R. (2022). JAK: Not just another kinase. Mol. Cancer Ther. 21, 1757–1764. doi: 10.1158/1535-7163.MCT-22-0323
Ahmed, S. M., Luo, L., Namani, A., Wang, X. J., and Tang, X. (2017). Nrf2 signaling pathway: Pivotal roles in inflammation. Biochim. Biophys. Acta Mol. Basis Dis. 1863, 585–597. doi: 10.1016/j.bbadis.2016.11.005
Aktories, K., Weller, U., and Chhatwal, G. S. (1987). Clostridium botulinum type C produces a novel ADP-ribosyltransferase distinct from botulinum C2 toxin. FEBS Lett. 212, 109–113. doi: 10.1016/0014-5793(87)81566-1
Alam, J., Stewart, D., Touchard, C., Boinapally, S., Choi, A. M., and Cook, J. L. (1999). Nrf2, a Cap’n’Collar transcription factor, regulates induction of the heme oxygenase-1 gene. J. Biol. Chem. 274, 26071–26078.
Albéri, L., Chi, Z., Kadam, S. D., Mulholland, J. D., Dawson, V. L., Gaiano, N., et al. (2010). Neonatal stroke in mice causes long-term changes in neuronal Notch-2 expression that may contribute to prolonged injury. Stroke 41, S64–S71. doi: 10.1161/STROKEAHA.110.595298
Alfieri, A., Srivastava, S., Siow, R. C. M., Cash, D., Modo, M., Duchen, M. R., et al. (2013). Sulforaphane preconditioning of the Nrf2/HO-1 defense pathway protects the cerebral vasculature against blood-brain barrier disruption and neurological deficits in stroke. Free Radic. Biol. Med. 65, 1012–1022. doi: 10.1016/j.freeradbiomed.2013.08.190
Alfieri, A., Srivastava, S., Siow, R. C., Modo, M., Fraser, P. A., and Mann, G. E. (2011). Targeting the Nrf2-Keap1 antioxidant defence pathway for neurovascular protection in stroke. J. Physiol. 589, 4125–4136. doi: 10.1113/jphysiol.2011.210294
Ali, C., Docagne, F., Nicole, O., LESNé, S., Toutain, J., Young, A., et al. (2001). Increased expression of transforming growth factor-beta after cerebral ischemia in the baboon: An endogenous marker of neuronal stress? J. Cereb. Blood Flow Metab. 21, 820–827. doi: 10.1097/00004647-200107000-00007
Alim, I., Caulfield, J. T., Chen, Y., Swarup, V., Geschwind, D. H., Ivanova, E., et al. (2019). Selenium drives a transcriptional adaptive program to block ferroptosis and treat stroke. Cell 177, 1262–1279.e25. doi: 10.1016/j.cell.2019.03.032
Allen, C. L., and Bayraktutan, U. (2009). Oxidative stress and its role in the pathogenesis of ischaemic stroke. Int. J. Stroke 4, 461–470.
Amerongen, V. N., Koolwijk, P., Versteilen, A., and Van, H. (2003). Involvement of RhoA/Rho kinase signaling in VEGF-induced endothelial cell migration and angiogenesis in vitro. Arterioscler. Thromb. Vasc. Biol. 23, 211–217. doi: 10.1161/01.atv.0000054198.68894.88
Anderson, K. V., Jürgens, G., and Nüsslein-Volhard, C. (1985). Establishment of dorsal-ventral polarity in the Drosophila embryo: Genetic studies on the role of the Toll gene product. Cell 42, 779–789.
Andersson, E. R., and Lendahl, U. (2014). Therapeutic modulation of Notch signalling–are we there yet? Nat. Rev. Drug Discov. 13, 357–378. doi: 10.1038/nrd4252
Andersson, E. R., Sandberg, R., and Lendahl, U. (2011). Notch signaling: Simplicity in design, versatility in function. Development 138, 3593–3612. doi: 10.1242/dev.063610
Arnold, R., Vehns, E., Randl, H., and Djabali, K. (2021). Baricitinib, a JAK-STAT inhibitor, reduces the cellular toxicity of the farnesyltransferase inhibitor lonafarnib in progeria cells. Int. J. Mol. Sci. 22:7474. doi: 10.3390/ijms22147474
Artavanis-Tsakonas, S., Muskavitch, M. A., and Yedvobnick, B. (1983). Molecular cloning of Notch, a locus affecting neurogenesis in Drosophila melanogaster. Proc. Natl. Acad. Sci. U. S. A. 80, 1977–1981. doi: 10.1073/pnas.80.7.1977
Arumugam, T. V., Chan, S. L., Jo, D. G., Yilmaz, G., Tang, S. C., Cheng, A., et al. (2006). Gamma secretase-mediated notch signaling worsens brain damage and functional outcome in ischemic stroke. Nat. Med. 12, 621–623. doi: 10.1038/nm1403
Arvin, B., Neville, L. F., Barone, F. C., and Feuerstein, G. Z. (1996). The role of inflammation and cytokines in brain injury. Neurosci. Biobehav. Rev. 20, 445–452.
Attisano, L., and Wrana, J. L. (2002). Signal transduction by the TGF-beta superfamily. Science 296, 1646–1647.
Austin, J., and Kimble, J. (1989). Transcript analysis of glp-1 and lin-12, homologous genes required for cell interactions during development of C. elegans. Cell 58, 565–571. doi: 10.1016/0092-8674(89)90437-6
Aylon, Y., and Oren, M. (2007). Living with p53, dying of p53. Cell 130, 597–600. doi: 10.1016/j.cell.2007.08.005
Azzolin, L., Panciera, T., Soligo, S., Enzo, E., Bicciato, S., Dupont, S., et al. (2014). YAP/TAZ incorporation in the β-catenin destruction complex orchestrates the Wnt response. Cell 158, 157–170. doi: 10.1016/j.cell.2014.06.013
Azzolin, L., Zanconato, F., Bresolin, S., Forcato, M., Basso, G., Bicciato, S., et al. (2012). Role of TAZ as mediator of Wnt signaling. Cell 151, 1443–1456. doi: 10.1016/j.cell.2012.11.027
Bannai, S., and Kitamura, E. (1980). Transport interaction of L-cystine and L-glutamate in human diploid fibroblasts in culture. J. Biol. Chem. 255, 2372–2376. doi: 10.1016/S0021-9258(19)85901-X
Barabási, A. L., Gulbahce, N., and Loscalzo, J. (2011). Network medicine: A network-based approach to human disease. Nat. Rev. Genet. 12, 56–68. doi: 10.1038/nrg2918
Barone, F. C., and Feuerstein, G. Z. (1999). Inflammatory mediators and stroke: New opportunities for novel therapeutics. J. Cereb. Blood Flow Metab. 19, 819–834. doi: 10.1097/00004647-199908000-00001
Barone, F. C., Irving, E. A., Ray, A. M., Lee, J. C., Kassis, S., Kumar, S., et al. (2001). Inhibition of p38 mitogen-activated protein kinase provides neuroprotection in cerebral focal ischemia. Med. Res. Rev. 21, 129–145.
Barrat, F. J., Crow, M. K., and Ivashkiv, L. B. (2019). Interferon target-gene expression and epigenomic signatures in health and disease. Nat. Immunol. 20, 1574–1583. doi: 10.1038/s41590-019-0466-2
Barthels, D., and Das, H. (2020). Current advances in ischemic stroke research and therapies. Biochim. Biophys. Acta Mol. Basis Dis. 1866:165260. doi: 10.1016/j.bbadis.2018.09.012
Bartlett, J. D., Close, G. L., Drust, B., and Morton, J. P. (2014). The emerging role of p53 in exercise metabolism. Sports Med. 44, 303–309. doi: 10.1007/s40279-013-0127-9
Bermudez, O., PAGèS, G., and Gimond, C. (2010). The dual-specificity MAP kinase phosphatases: Critical roles in development and cancer. Am. J. Physiol. Cell Physiol. 299, C189–C202. doi: 10.1152/ajpcell.00347.2009
Bhanot, P., Brink, M., Samos, C. H., Hsieh, J. C., Wang, Y., Macke, J. P., et al. (1996). A new member of the frizzled family from Drosophila functions as a wingless receptor. Nature 382, 225–230. doi: 10.1038/382225a0
Boulton, T. G., Yancopoulos, G. D., Gregory, J. S., Slaughter, C., Moomaw, C., Hsu, J., et al. (1990). An insulin-stimulated protein kinase similar to yeast kinases involved in cell cycle control. Science 249, 64–67. doi: 10.1126/science.2164259
Boutros, T., Chevet, E., and Metrakos, P. (2008). Mitogen-activated protein (MAP) kinase/MAP kinase phosphatase regulation: Roles in cell growth, death, and cancer. Pharmacol. Rev. 60, 261–310.
Boyd, J. M., Malstrom, S., Subramanian, T., Venkatesh, L. K., Schaeper, U., Elangovan, B., et al. (1994). Adenovirus E1B 19 kDa and Bcl-2 proteins interact with a common set of cellular proteins. Cell 79, 341–351. doi: 10.1016/0092-8674(94)90202-X
Brand, K., Page, S., Rogler, G., Bartsch, A., Brandl, R., Knuechel, R., et al. (1996). Activated transcription factor nuclear factor-kappa B is present in the atherosclerotic lesion. J. Clin. Invest. 97, 1715–1722. doi: 10.1172/JCI118598
Bridges, C. B. (1916). Non-disjunction as proof of the chromosome theory of heredity (concluded). Genetics 1, 107–163. doi: 10.1093/genetics/1.2.107
Bruggen, V., Thibodeaux, H., Palmer, J. T., Lee, W. P., Fu, L., Cairns, B., et al. (1999). VEGF antagonism reduces edema formation and tissue damage after ischemia/reperfusion injury in the mouse brain. J. Clin. Invest. 104, 1613–1620. doi: 10.1172/JCI8218
Burgering, B. M., and Coffer, P. J. (1995). Protein kinase B (c-Akt) in phosphatidylinositol-3-OH kinase signal transduction. Nature 376, 599–602.
Burton, T. R., and Gibson, S. B. (2009). The role of Bcl-2 family member BNIP3 in cell death and disease: NIPping at the heels of cell death. Cell Death Differ. 16, 515–523. doi: 10.1038/cdd.2008.185
Campos, A. H., Wang, W., Pollman, M. J., and Gibbons, G. H. (2002). Determinants of Notch-3 receptor expression and signaling in vascular smooth muscle cells: Implications in cell-cycle regulation. Circ. Res. 91, 999–1006. doi: 10.1161/01.res.0000044944.99984.25
Cano, E., and Mahadevan, L. C. (1995). Parallel signal processing among mammalian MAPKs. Trends Biochem. Sci. 20, 117–122. doi: 10.1016/s0968-0004(00)88978-1
Carling, D., Zammit, V. A., and Hardie, D. G. (1987). A common bicyclic protein kinase cascade inactivates the regulatory enzymes of fatty acid and cholesterol biosynthesis. FEBS Lett. 223, 217–222. doi: 10.1016/0014-5793(87)80292-2
Carmeliet, P., Dor, Y., Herbert, J. M., Fukumura, D., Brusselmans, K., Dewerchin, M., et al. (1998). Role of HIF-1alpha in hypoxia-mediated apoptosis, cell proliferation and tumour angiogenesis. Nature 394, 485–490. doi: 10.1038/28867
Carriere, V., Roussel, L., Ortega, N., Lacorre, D. A., Americh, L., Aguilar, L., et al. (2007). IL-33, the IL-1-like cytokine ligand for ST2 receptor, is a chromatin-associated nuclear factor in vivo. Proc. Natl. Acad. Sci. U. S. A. 104, 282–287.
Chan, J. Y., Kwong, M., Lu, R., Chang, J., Wang, B., Yen, T. S., et al. (1998). Targeted disruption of the ubiquitous CNC-bZIP transcription factor, Nrf-1, results in anemia and embryonic lethality in mice. Embo J. 17, 1779–1787. doi: 10.1093/emboj/17.6.1779
Chan, P. H. (2005). Mitochondrial dysfunction and oxidative stress as determinants of cell death/survival in stroke. Ann. N. Y. Acad. Sci. 1042, 203–209.
Chang, H., Lin, C., Li, Z., Shen, Y., Zhang, G., Mao, L., et al. (2022). T3 alleviates neuroinflammation and reduces early brain injury after subarachnoid haemorrhage by promoting mitophagy via PINK 1-parkin pathway. Exp. Neurol. 357:114175. doi: 10.1016/j.expneurol.2022.114175
Chang, T. S., and Jensen, M. B. (2014). Haemodilution for acute ischaemic stroke. Cochrane Database Syst. Rev. 2014:Cd000103.
Chen, B., Cao, P., Guo, X., Yin, M., Li, X., Jiang, L., et al. (2022). Maraviroc, an inhibitor of chemokine receptor type 5, alleviates neuroinflammatory response after cerebral Ischemia/reperfusion injury via regulating MAPK/NF-κB signaling. Int. Immunopharmacol. 108:108755.
Chen, G., Ray, R., Dubik, D., Shi, L., Cizeau, J., Bleackley, R. C., et al. (1997). The E1B 19K/Bcl-2-binding protein Nip3 is a dimeric mitochondrial protein that activates apoptosis. J. Exp. Med. 186, 1975–1983. doi: 10.1084/jem.186.12.1975
Chen, J., Lin, X., Yao, C., Bingwa, L. A., Wang, H., Lin, Z., et al. (2022). Transplantation of Roxadustat-preconditioned bone marrow stromal cells improves neurological function recovery through enhancing grafted cell survival in ischemic stroke rats. CNS Neurosci. Ther. 28, 1519–1531. doi: 10.1111/cns.13890
Chen, J., Zacharek, A., Li, A., Cui, X., Roberts, C., Lu, M., et al. (2008). Atorvastatin promotes presenilin-1 expression and Notch1 activity and increases neural progenitor cell proliferation after stroke. Stroke 39, 220–226. doi: 10.1161/STROKEAHA.107.490946
Chen, J., Zhang, X., Liu, X., Zhang, C., Shang, W., Xue, J., et al. (2019). Ginsenoside Rg1 promotes cerebral angiogenesis via the PI3K/Akt/mTOR signaling pathway in ischemic mice. Eur. J. Pharmacol. 856:172418. doi: 10.1016/j.ejphar.2019.172418
Chen, L., Min, J., and Wang, F. (2022). Copper homeostasis and cuproptosis in health and disease. Signal. Transduct. Target Ther. 7:378. doi: 10.1038/s41392-022-01229-y
Chen, R. L., Ogunshola, O. O., Yeoh, K. K., Jani, A., Papadakis, M., Nagel, S., et al. (2014). HIF prolyl hydroxylase inhibition prior to transient focal cerebral ischaemia is neuroprotective in mice. J. Neurochem. 131, 177–189. doi: 10.1111/jnc.12804
Chen, S., Peng, J., Sherchan, P., Ma, Y., Xiang, S., Yan, F., et al. (2020). TREM2 activation attenuates neuroinflammation and neuronal apoptosis via PI3K/Akt pathway after intracerebral hemorrhage in mice. J. Neuroinflammation 17:168. doi: 10.1186/s12974-020-01853-x
Chen, S., Zhang, J., Li, M., Zhou, J., and Zhang, Y. (2022). Danhong injection combined with tPA protects the BBB through Notch-VEGF signaling pathway on long-term outcomes of thrombolytic therapy. Biomed. Pharmacother. 153:113288. doi: 10.1016/j.biopha.2022.113288
Chen, X., Kang, R., Kroemer, G., and Tang, D. (2021a). Broadening horizons: The role of ferroptosis in cancer. Nat. Rev. Clin. Oncol. 18, 280–296. doi: 10.1038/s41571-020-00462-0
Chen, X., Kang, R., Kroemer, G., and Tang, D. (2021b). Ferroptosis in infection, inflammation, and immunity. J. Exp. Med. 218:e20210518.
Chen, X., Wu, H., Chen, H., Wang, Q., Xie, X. J., and Shen, J. (2019). Astragaloside VI promotes neural stem cell proliferation and enhances neurological function recovery in transient cerebral ischemic injury via activating EGFR/MAPK signaling cascades. Mol. Neurobiol. 56, 3053–3067. doi: 10.1007/s12035-018-1294-3
Cheng, Y. L., Park, J. S., Manzanero, S., Choi, Y., Baik, S. H., Okun, E., et al. (2014). Evidence that collaboration between HIF-1α and Notch-1 promotes neuronal cell death in ischemic stroke. Neurobiol. Dis. 62, 286–295.
Chien, M. Y., Chuang, C. H., Chern, C. M., Liou, K. T., Liu, D. Z., Hou, Y. C., et al. (2016). Salvianolic acid A alleviates ischemic brain injury through the inhibition of inflammation and apoptosis and the promotion of neurogenesis in mice. Free Radic. Biol. Med. 99, 508–519. doi: 10.1016/j.freeradbiomed.2016.09.006
Chinnadurai, G., Vijayalingam, S., and Gibson, S. B. (2008). BNIP3 subfamily BH3-only proteins: Mitochondrial stress sensors in normal and pathological functions. Oncogene 27, S114–S127. doi: 10.1038/onc.2009.49
Chio, I. I. C., Jafarnejad, S. M., Ponz-Sarvise, M., Park, Y., Rivera, K., Palm, W., et al. (2016). NRF2 promotes tumor maintenance by modulating mRNA translation in pancreatic cancer. Cell 166, 963–976. doi: 10.1016/j.cell.2016.06.056
Chou, W. C., Rampanelli, E., Li, X., and Ting, J. P. (2022). Impact of intracellular innate immune receptors on immunometabolism. Cell Mol. Immunol. 19, 337–351.
Cinelli, M. A., Do, H. T., Miley, G. P., and Silverman, R. B. (2020). Inducible nitric oxide synthase: Regulation, structure, and inhibition. Med. Res. Rev. 40, 158–189.
Clark, I. E., Dodson, M. W., Jiang, C., Cao, J. H., Huh, J. R., Seol, J. H., et al. (2006). Drosophila pink1 is required for mitochondrial function and interacts genetically with parkin. Nature 441, 1162–1166.
Cochrane, A., Chen, C., Stephen, J., RøNNING, O. M., Anderson, C. S., Hankey, G. J., et al. (2023). Antithrombotic treatment after stroke due to intracerebral haemorrhage. Cochrane Database Syst. Rev. 1:Cd012144.
Coffman, C., Harris, W., and Kintner, C. (1990). Xotch, the xenopus homolog of drosophila notch. Science 249, 1438–1441.
Collino, M., Aragno, M., Mastrocola, R., Benetti, E., Gallicchio, M., Dianzani, C., et al. (2006). Oxidative stress and inflammatory response evoked by transient cerebral ischemia/reperfusion: Effects of the PPAR-alpha agonist WY14643. Free Radic. Biol. Med. 41, 579–589. doi: 10.1016/j.freeradbiomed.2006.04.030
Cook, D. N., Pisetsky, D. S., and Schwartz, D. A. (2004). Toll-like receptors in the pathogenesis of human disease. Nat. Immunol. 5, 975–979.
Cooper, J. A., Bowen-Pope, D. F., Raines, E., Ross, R., and Hunter, T. (1982). Similar effects of platelet-derived growth factor and epidermal growth factor on the phosphorylation of tyrosine in cellular proteins. Cell 31, 263–273.
Cooperative Group for Reassessment of Defibrase (2005). Reassessment of defibrase in treatment of acute cerebral infarction: A multicenter, randomized, double-blind, placebo-controlled trial. Chin. Med. Sci. J. 20, 151–158.
Coyle, J. T., and Puttfarcken, P. (1993). Oxidative stress, glutamate, and neurodegenerative disorders. Science 262, 689–695.
Croll, S. D., and Wiegand, S. J. (2001). Vascular growth factors in cerebral ischemia. Mol. Neurobiol. 23, 121–135.
Cui, H. Y., Zhang, X. J., Yang, Y., Zhang, C., Zhu, C. H., Miao, J. Y., et al. (2018). Rosmarinic acid elicits neuroprotection in ischemic stroke via Nrf2 and heme oxygenase 1 signaling. Neural Regen. Res. 13, 2119–2128. doi: 10.4103/1673-5374.241463
Cui, Y., Zhang, Y., Zhao, X., Shao, L., Liu, G., Sun, C., et al. (2021). ACSL4 exacerbates ischemic stroke by promoting ferroptosis-induced brain injury and neuroinflammation. Brain Behav. Immun. 93, 312–321. doi: 10.1016/j.bbi.2021.01.003
Culman, J., Zhao, Y., Gohlke, P., and Herdegen, T. (2007). PPAR-gamma: Therapeutic target for ischemic stroke. Trends Pharmacol. Sci. 28, 244–249.
Darnell, J. E. Jr., Kerr, I. M., and Stark, G. R. (1994). Jak-STAT pathways and transcriptional activation in response to IFNs and other extracellular signaling proteins. Science 264, 1415–1421. doi: 10.1126/science.8197455
D’Autréaux, B., and Toledano, M. B. (2007). ROS as signalling molecules: Mechanisms that generate specificity in ROS homeostasis. Nat. Rev. Mol. Cell Biol. 8, 813–824. doi: 10.1038/nrm2256
Dávalos, A., and Secades, J. (2011). Citicoline preclinical and clinical update 2009-2010. Stroke 42, S36–S39. doi: 10.1161/STROKEAHA.110.605568
Davalos, D., Grutzendler, J., Yang, G., Kim, J. V., Zuo, Y., Jung, S., et al. (2005). ATP mediates rapid microglial response to local brain injury in vivo. Nat. Neurosci. 8, 752–758. doi: 10.1038/nn1472
Dave, K. D., De, S., Sheth, N. P., Ramboz, S., Beck, M. J., Quang, C., et al. (2014). Phenotypic characterization of recessive gene knockout rat models of Parkinson’s disease. Neurobiol. Dis. 70, 190–203. doi: 10.1016/j.nbd.2014.06.009
Davis, R. J. (1994). MAPKs: New JNK expands the group. Trends Biochem. Sci. 19, 470–473. doi: 10.1016/0968-0004(94)90132-5
Deleo, A. B., Jay, G., Appella, E., Dubois, G. C., Law, L. W., and Old, L. J. (1979). Detection of a transformation-related antigen in chemically induced sarcomas and other transformed cells of the mouse. Proc. Natl. Acad. Sci. U. S. A. 76, 2420–2424.
Dey, A., Varelas, X., and Guan, K. L. (2020). Targeting the Hippo pathway in cancer, fibrosis, wound healing and regenerative medicine. Nat. Rev. Drug Discov. 19, 480–494. doi: 10.1038/s41573-020-0070-z
Didonato, J. A., Mercurio, F., and Karin, M. (2012). NF-κB and the link between inflammation and cancer. Immunol. Rev. 246, 379–400.
Ding, J. Y., Pan, L. Q., Hu, Y. Y., Rajah, G. B., Zhou, D., Bai, C. B., et al. (2019). Batroxobin in combination with anticoagulation may promote venous sinus recanalization in cerebral venous thrombosis: A real-world experience. CNS Neurosci. Ther. 25, 638–646. doi: 10.1111/cns.13093
Ding, Y., Chen, M., Wang, M., Li, Y., and Wen, A. (2015). Posttreatment with 11-Keto-β-boswellic acid ameliorates cerebral ischemia-reperfusion injury: Nrf2/HO-1 pathway as a potential mechanism. Mol. Neurobiol. 52, 1430–1439.
Ding, Y., Qian, J., Li, H., Shen, H., Li, X., Kong, Y., et al. (2019). Effects of SC99 on cerebral ischemia-perfusion injury in rats: Selective modulation of microglia polarization to M2 phenotype via inhibiting JAK2-STAT3 pathway. Neurosci. Res. 142, 58–68. doi: 10.1016/j.neures.2018.05.002
Dirnagl, U., Iadecola, C., and Moskowitz, M. A. (1999). Pathobiology of ischaemic stroke: An integrated view. Trends Neurosci. 22, 391–397. doi: 10.1016/s0166-2236(99)01401-0
Dixon, S. J., Lemberg, K. M., Lamprecht, M. R., Skouta, R., Zaitsev, E. M., Gleason, C. E., et al. (2012). Ferroptosis: An iron-dependent form of nonapoptotic cell death. Cell 149, 1060–1072.
Doll, S., Freitas, F. P., Shah, R., Aldrovandi, M., Da, S., Ingold, I., et al. (2019). FSP1 is a glutathione-independent ferroptosis suppressor. Nature 575, 693–698.
Doll, S., Proneth, B., Tyurina, Y. Y., Panzilius, E., Kobayashi, S., Ingold, I., et al. (2017). ACSL4 dictates ferroptosis sensitivity by shaping cellular lipid composition. Nat. Chem. Biol. 13, 91–98. doi: 10.1038/nchembio.2239
Dolma, S., Lessnick, S. L., Hahn, W. C., and Stockwell, B. R. (2003). Identification of genotype-selective antitumor agents using synthetic lethal chemical screening in engineered human tumor cells. Cancer Cell 3, 285–296. doi: 10.1016/s1535-6108(03)00050-3
Dong, W., Xian, Y., Yuan, W., Huifeng, Z., Tao, W., Zhiqiang, L., et al. (2016). Catalpol stimulates VEGF production via the JAK2/STAT3 pathway to improve angiogenesis in rats’ stroke model. J. Ethnopharmacol. 191, 169–179. doi: 10.1016/j.jep.2016.06.030
Duan, C., Wang, H., Jiao, D., Geng, Y., Wu, Q., Yan, H., et al. (2022). Curcumin restrains oxidative stress of after intracerebral hemorrhage in rat by activating the Nrf2/HO-1 Pathway. Front. Pharmacol. 13:889226. doi: 10.3389/fphar.2022.889226
Duan, J., Cui, J., Yang, Z., Guo, C., Cao, J., Xi, M., et al. (2019). Neuroprotective effect of Apelin 13 on ischemic stroke by activating AMPK/GSK-3β/Nrf2 signaling. J. Neuroinflammation 16, 24.
Duan, L., Zhang, Y., Yang, Y., Su, S., Zhou, L., Lo, P. C., et al. (2021). Baicalin inhibits ferroptosis in intracerebral hemorrhage. Front. Pharmacol. 12:629379. doi: 10.3389/fphar.2021.629379
Duan, T., Li, L., Yu, Y., Li, T., Han, R., Sun, X., et al. (2022). Traditional Chinese medicine use in the pathophysiological processes of intracerebral hemorrhage and comparison with conventional therapy. Pharmacol. Res. 179:106200. doi: 10.1016/j.phrs.2022.106200
Durrant, T. N., and Hers, I. (2020). PI3K inhibitors in thrombosis and cardiovascular disease. Clin. Transl. Med. 9:8.
Echelard, Y., Epstein, D. J., St-Jacques, B., Shen, L., Mohler, J., Mcmahon, J. A., et al. (1993). Sonic hedgehog, a member of a family of putative signaling molecules, is implicated in the regulation of CNS polarity. Cell 75, 1417–1430. doi: 10.1016/0092-8674(93)90627-3
Elbediwy, A., Vincent-Mistiaen, Z. I., Spencer-Dene, B., Stone, R. K., Boeing, S., Wculek, S. K., et al. (2016). Integrin signalling regulates YAP and TAZ to control skin homeostasis. Development 143, 1674–1687. doi: 10.1242/dev.133728
Endres, M., Laufs, U., Liao, J. K., and Moskowitz, M. A. (2004). Targeting eNOS for stroke protection. Trends Neurosci. 27, 283–289.
Erlich, S., Shohami, E., and Pinkas-Kramarski, R. (2000). Closed head injury induces up-regulation of ErbB-4 receptor at the site of injury. Mol. Cell Neurosci. 16, 597–608. doi: 10.1006/mcne.2000.0894
Fann, D. Y., Lim, Y. A., Cheng, Y. L., Lok, K. Z., Chunduri, P., Baik, S. H., et al. (2018). Evidence that NF-κB and MAPK Signaling Promotes NLRP Inflammasome Activation in Neurons Following Ischemic Stroke. Mol. Neurobiol. 55, 1082–1096.
Fedorowicz, M. A., De, V., RüB, C., Becker, D., Huang, Y., Zhou, C., et al. (2014). Cytosolic cleaved PINK1 represses Parkin translocation to mitochondria and mitophagy. Embo Rep. 15, 86–93. doi: 10.1002/embr.201337294
Feng, C. N., Ji, Z. C., Peng, D. H., Hu, H. Y., Zhang, J. H., and Pang, B. (2022). [Clinical trials and evaluation of Chinese patent medicine for stroke]. Zhongguo Zhong Yao Za Zhi 47, 2330–2337.
Feng, S., Yang, Q., Liu, M., Li, W., Yuan, W., Zhang, S., et al. (2011). Edaravone for acute ischaemic stroke. Cochrane Database Syst. Rev. 7:CD007230. doi: 10.1002/14651858.CD007230.pub2
Fernandez, L. A., Northcott, P. A., Dalton, J., Fraga, C., Ellison, D., Angers, S., et al. (2009). YAP1 is amplified and up-regulated in hedgehog-associated medulloblastomas and mediates Sonic hedgehog-driven neural precursor proliferation. Genes Dev. 23, 2729–2741. doi: 10.1101/gad.1824509
Ferrara, N. (2001). Role of vascular endothelial growth factor in regulation of physiological angiogenesis. Am. J. Physiol. Cell Physiol. 280, C1358–C1366.
Ferrara, N., and Henzel, W. J. (1989). Pituitary follicular cells secrete a novel heparin-binding growth factor specific for vascular endothelial cells. Biochem. Biophys. Res. Commun. 161, 851–858.
Fong, W. H., Tsai, H. D., Chen, Y. C., Wu, J. S., and Lin, T. N. (2010). Anti-apoptotic actions of PPAR-gamma against ischemic stroke. Mol. Neurobiol. 41, 180–186. doi: 10.1007/s12035-010-8103-y
Foster-Goldman, A., and Mccarthy, D. (2013). Angioedema from recombinant TPA administration: Case report and pathophysiology review. Am. J. Ther. 20, 691–693. doi: 10.1097/MJT.0b013e3182799083
Frame, S., and Cohen, P. (2001). GSK3 takes centre stage more than 20 years after its discovery. Biochem. J. 359, 1–16. doi: 10.1042/0264-6021:3590001
Franke, T. F., Yang, S. I., Chan, T. O., Datta, K., Kazlauskas, A., Morrison, D. K., et al. (1995). The protein kinase encoded by the Akt proto-oncogene is a target of the PDGF-activated phosphatidylinositol 3-kinase. Cell 81, 727–736. doi: 10.1016/0092-8674(95)90534-0
Fresno, V. J., Casado, E., de Castro, J., Cejas, P., Belda-Iniesta, C., and González-Barón, M. (2004). PI3K/Akt signalling pathway and cancer. Cancer Treat Rev. 30, 193–204.
Fu, A., Eberhard, C. E., and Screaton, R. A. (2013). Role of AMPK in pancreatic beta cell function. Mol. Cell Endocrinol. 366, 127–134.
Fu, C., Wu, Y., Liu, S., Luo, C., Lu, Y., Liu, M., et al. (2022). Rehmannioside a improves cognitive impairment and alleviates ferroptosis via activating PI3K/AKT/Nrf2 and SLC7A11/GPX4 signaling pathway after ischemia. J. Ethnopharmacol. 289:115021. doi: 10.1016/j.jep.2022.115021
Furchgott, R. F., and Zawadzki, J. V. (1980). The obligatory role of endothelial cells in the relaxation of arterial smooth muscle by acetylcholine. Nature 288, 373–376.
Furlan, A., Higashida, R., Wechsler, L., Gent, M., Rowley, H., Kase, C., et al. (1999). Intra-arterial prourokinase for acute ischemic stroke. The PROACT II study: A randomized controlled trial. Prolyse in acute cerebral thromboembolism. JAMA 282, 2003–2011.
GBD 2019 Stroke Collaborators (2021). Global, regional, and national burden of stroke and its risk factors, 1990-2019: A systematic analysis for the global burden of disease study 2019. Lancet Neurol. 20, 795–820.
Geisler, S., HOLMSTRöM, K. M., Skujat, D., Fiesel, F. C., Rothfuss, O. C., Kahle, P. J., et al. (2010). PINK1/Parkin-mediated mitophagy is dependent on VDAC1 and p62/SQSTM1. Nat. Cell Biol. 12, 119–131. doi: 10.1038/ncb2012
Ghigo, A., Laffargue, M., Li, M., and Hirsch, E. (2017). PI3K and calcium signaling in cardiovascular disease. Circ. Res. 121, 282–292. doi: 10.1161/CIRCRESAHA.117.310183
Gold, R., Kappos, L., Arnold, D. L., Bar-Or, A., Giovannoni, G., Selmaj, K., et al. (2012). Placebo-controlled phase 3 study of oral BG-12 for relapsing multiple sclerosis. N. Engl. J. Med. 367, 1098–1107. doi: 10.1056/NEJMoa1114287
Gong, P., Zhang, Z., Zou, C., Tian, Q., Chen, X., Hong, M., et al. (2019). Hippo/YAP signaling pathway mitigates blood-brain barrier disruption after cerebral ischemia/reperfusion injury. Behav. Brain Res. 356, 8–17. doi: 10.1016/j.bbr.2018.08.003
Gong, S., Ma, H., Zheng, F., Huang, J., Zhang, Y., Yu, B., et al. (2021). Inhibiting YAP in endothelial cells from entering the nucleus attenuates blood-brain barrier damage during ischemia-reperfusion injury. Front. Pharmacol. 12:777680. doi: 10.3389/fphar.2021.777680
Gough, N. R., Xiang, X., and Mishra, L. (2021). TGF-β signaling in liver, pancreas, and gastrointestinal diseases and cancer. Gastroenterology 161, 434–452.e15. doi: 10.1053/j.gastro.2021.04.064
Gracey, E., Hromadová, D., Lim, M., Qaiyum, Z., Zeng, M., Yao, Y., et al. (2020). TYK2 inhibition reduces type 3 immunity and modifies disease progression in murine spondyloarthritis. J. Clin. Invest. 130, 1863–1878. doi: 10.1172/JCI126567
Greenlund, A. C., Farrar, M. A., Viviano, B. L., and Schreiber, R. D. (1994). Ligand-induced IFN gamma receptor tyrosine phosphorylation couples the receptor to its signal transduction system (p91). Embo J. 13, 1591–1600. doi: 10.1002/j.1460-2075.1994.tb06422.x
Greer, D. M. (2010). Aspirin and antiplatelet agent resistance: Implications for prevention of secondary stroke. CNS Drugs 24, 1027–1040. doi: 10.2165/11539160-0000000000-00000
Greten, F. R., Eckmann, L., Greten, T. F., Park, J. M., Li, Z. W., Egan, L. J., et al. (2004). IKKbeta links inflammation and tumorigenesis in a mouse model of colitis-associated cancer. Cell 118, 285–296. doi: 10.1016/j.cell.2004.07.013
Griffith, T. M., Edwards, D. H., Lewis, M. J., Newby, A. C., and Henderson, A. H. (1984). The nature of endothelium-derived vascular relaxant factor. Nature 308, 645–647. doi: 10.1038/308645a0
Grijalva, J. L., Huizenga, M., Mueller, K., Rodriguez, S., Brazzo, J., Camargo, F., et al. (2014). Dynamic alterations in Hippo signaling pathway and YAP activation during liver regeneration. Am. J. Physiol. Gastrointest. Liver Physiol. 307, G196–G204. doi: 10.1152/ajpgi.00077.2014
Gross, S. S., and Wolin, M. S. (1995). Nitric oxide: Pathophysiological mechanisms. Annu. Rev. Physiol. 57, 737–769. doi: 10.1146/annurev.ph.57.030195.003513
Guo, H., Zhang, W., Wang, Z., Li, Z., Zhou, J., and Yang, Z. (2022). Dexmedetomidine post-conditioning protects blood-brain barrier integrity by modulating microglia/macrophage polarization via inhibiting NF-κB signaling pathway in intracerebral hemorrhage. Front. Mol. Neurosci. 15:977941. doi: 10.3389/fnmol.2022.977941
Hansen, C. G., Moroishi, T., and Guan, K. L. (2015). YAP and TAZ: A nexus for Hippo signaling and beyond. Trends Cell Biol. 25, 499–513. doi: 10.1016/j.tcb.2015.05.002
Hanson, L. R., Roeytenberg, A., Martinez, P. M., Coppes, V. G., Sweet, D. C., Rao, R. J., et al. (2009). Intranasal deferoxamine provides increased brain exposure and significant protection in rat ischemic stroke. J. Pharmacol. Exp. Ther. 330, 679–686. doi: 10.1124/jpet.108.149807
Hansson, E. M., Lendahl, U., and Chapman, G. (2004). Notch signaling in development and disease. Semin. Cancer Biol. 14, 320–328. doi: 10.1016/j.semcancer.2004.04.011
Hao, D. L., Li, J. M., Xie, R., Huo, H. R., Xiong, X. J., Sui, F., et al. (2023). The role of traditional herbal medicine for ischemic stroke: From bench to clinic-A critical review. Phytomedicine 109:154609. doi: 10.1016/j.phymed.2022.154609
Hardie, G. (2016). Regulation of AMP-activated protein kinase by natural and synthetic activators. Acta Pharm. Sin. B 6, 1–19. doi: 10.1016/j.apsb.2015.06.002
Harris, C. C. (1993). p53: At the crossroads of molecular carcinogenesis and risk assessment. Science 262, 1980–1981. doi: 10.1126/science.8266092
Harvey, K. F., Pfleger, C. M., and Hariharan, I. K. (2003). The drosophila Mst ortholog, hippo, restricts growth and cell proliferation and promotes apoptosis. Cell 114, 457–467. doi: 10.1016/s0092-8674(03)00557-9
Harvey, K. F., Zhang, X., and Thomas, D. M. (2013). The Hippo pathway and human cancer. Nat. Rev. Cancer 13, 246–257. doi: 10.1038/nrc3458
Hashimoto, C., Hudson, K. L., and Anderson, K. V. (1988). The Toll gene of Drosophila, required for dorsal-ventral embryonic polarity, appears to encode a transmembrane protein. Cell 52, 269–279. doi: 10.1016/0092-8674(88)90516-8
Hayes, J. D., Mcmahon, M., Chowdhry, S., and Dinkova-Kostova, A. T. (2010). Cancer chemoprevention mechanisms mediated through the Keap1-Nrf2 pathway. Antioxid. Redox Signal. 13, 1713–1748.
He, Q. W., Xia, Y. P., Chen, S. C., Wang, Y., Huang, M., Huang, Y., et al. (2013). Astrocyte-derived sonic hedgehog contributes to angiogenesis in brain microvascular endothelial cells via RhoA/ROCK pathway after oxygen-glucose deprivation. Mol. Neurobiol. 47, 976–987. doi: 10.1007/s12035-013-8396-8
He, Q., Ma, Y., Liu, J., Zhang, D., Ren, J., Zhao, R., et al. (2021). Biological Functions and Regulatory Mechanisms of Hypoxia-Inducible Factor-1α in Ischemic Stroke. Front. Immunol. 12:801985. doi: 10.3389/fimmu.2021.801985
He, S., Li, R., Peng, Y., Wang, Z., Huang, J., Meng, H., et al. (2022). ACSL4 contributes to ferroptosis-mediated rhabdomyolysis in exertional heat stroke. J. Cachexia Sarcopenia Muscle 13, 1717–1730. doi: 10.1002/jcsm.12953
Helton, R., Cui, J., Scheel, J. R., Ellison, J. A., Ames, C., Gibson, C., et al. (2005). Brain-specific knock-out of hypoxia-inducible factor-1alpha reduces rather than increases hypoxic-ischemic damage. J. Neurosci. 25, 4099–4107. doi: 10.1523/JNEUROSCI.4555-04.2005
Henke, N., Albrecht, P., Bouchachia, I., Ryazantseva, M., Knoll, K., Lewerenz, J., et al. (2013). The plasma membrane channel ORAI1 mediates detrimental calcium influx caused by endogenous oxidative stress. Cell Death Dis. 4:e470. doi: 10.1038/cddis.2012.216
Herpich, F., and Rincon, F. (2020). Management of Acute Ischemic Stroke. Crit. Care Med. 48, 1654–1663.
Herr, I., and Debatin, K. M. (2001). Cellular stress response and apoptosis in cancer therapy. Blood 98, 2603–2614.
Herzig, S., and Shaw, R. J. (2018). AMPK: Guardian of metabolism and mitochondrial homeostasis. Nat. Rev. Mol. Cell Biol. 19, 121–135.
Higashida, R. T., Tsai, F. Y., Halbach, V. V., Barnwell, S. L., Dowd, C. F., and Hieshima, G. B. (1995). Interventional neurovascular techniques in the treatment of stroke–state-of-the-art therapy. J. Intern. Med. 237, 105–115. doi: 10.1111/j.1365-2796.1995.tb01147.x
Hirooka, Y., and Shimokawa, H. (2005). Therapeutic potential of rho-kinase inhibitors in cardiovascular diseases. Am. J. Cardiovasc. Drugs 5, 31–39.
Hirschhorn, T., and Stockwell, B. R. (2019). The development of the concept of ferroptosis. Free Radic. Biol. Med. 133, 130–143.
Hlavica, M., Diepers, M., Garcia-Esperon, C., Ineichen, B. V., Nedeltchev, K., Kahles, T., et al. (2015). Pharmacological recanalization therapy in acute ischemic stroke - evolution, current state and perspectives of intravenous and intra-arterial thrombolysis. J. Neuroradiol. 42, 30–46. doi: 10.1016/j.neurad.2014.11.004
Ho, J. J., Man, H. S., and Marsden, P. A. (2012). Nitric oxide signaling in hypoxia. J. Mol. Med. 90, 217–231.
Hollstein, M., Sidransky, D., Vogelstein, B., and Harris, C. C. (1991). p53 mutations in human cancers. Science 253, 49–53.
Homma, T., Kobayashi, S., Sato, H., and Fujii, J. (2019). Edaravone, a free radical scavenger, protects against ferroptotic cell death in vitro. Exp. Cell Res. 384:111592. doi: 10.1016/j.yexcr.2019.111592
Hong, L., Chen, W., He, L., Tan, H., Peng, D., Zhao, G., et al. (2021). Effect of Naoluoxintong on the NogoA/RhoA/ROCK pathway by down-regulating DNA methylation in MCAO rats. J. Ethnopharmacol. 281:114559. doi: 10.1016/j.jep.2021.114559
Hou, Y., Wang, K., Wan, W., Cheng, Y., Pu, X., and Ye, X. (2018). Resveratrol provides neuroprotection by regulating the JAK2/STAT3/PI3K/AKT/mTOR pathway after stroke in rats. Genes Dis. 5, 245–255. doi: 10.1016/j.gendis.2018.06.001
Hsu, C. C., Peng, D., Cai, Z., and Lin, H. K. (2022). AMPK signaling and its targeting in cancer progression and treatment. Semin. Cancer Biol. 85, 52–68.
Hu, G. Q., Du, X., Li, Y. J., Gao, X. Q., Chen, B. Q., and Yu, L. (2017). Inhibition of cerebral ischemia/reperfusion injury-induced apoptosis: Nicotiflorin and JAK2/STAT3 pathway. Neural Regen. Res. 12, 96–102. doi: 10.4103/1673-5374.198992
Hu, Q., Liu, L., Zhou, L., Lu, H., Wang, J., Chen, X., et al. (2020). Effect of fluoxetine on HIF-1α- Netrin/VEGF cascade, angiogenesis and neuroprotection in a rat model of transient middle cerebral artery occlusion. Exp. Neurol. 329:113312.
Hu, Q., Zuo, T., Deng, L., Chen, S., Yu, W., Liu, S., et al. (2022). β-Caryophyllene suppresses ferroptosis induced by cerebral ischemia reperfusion via activation of the NRF2/HO-1 signaling pathway in MCAO/R rats. Phytomedicine 102:154112.
Hu, S., Wu, Y., Zhao, B., Hu, H., Zhu, B., Sun, Z., et al. (2018). Panax notoginseng saponins protect cerebral microvascular endothelial cells against oxygen-glucose deprivation/reperfusion-induced barrier dysfunction via activation of pi3k/akt/nrf2 antioxidant signaling pathway. Molecules 23:2781. doi: 10.3390/molecules23112781
Hu, Y., Zheng, Y., Wang, T., Jiao, L., and Luo, Y. (2022). VEGF, a key factor for blood brain barrier injury after cerebral ischemic stroke. Aging Dis. 13, 647–654. doi: 10.14336/AD.2021.1121
Huang, J., Wu, S., Barrera, J., Matthews, K., and Pan, D. (2005). The Hippo signaling pathway coordinately regulates cell proliferation and apoptosis by inactivating Yorkie, the Drosophila Homolog of YAP. Cell 122, 421–434. doi: 10.1016/j.cell.2005.06.007
Huang, L., Li, S., Dai, Q., Zhang, A., Yu, Q., Du, W., et al. (2020). Astrocytic Yes-associated protein attenuates cerebral ischemia-induced brain injury by regulating signal transducer and activator of transcription 3 signaling. Exp. Neurol. 333:113431. doi: 10.1016/j.expneurol.2020.113431
Huang, S. S., Cheng, H., Tang, C. M., Nien, M. W., Huang, Y. S., Lee, I. H., et al. (2013). Anti-oxidative, anti-apoptotic, and pro-angiogenic effects mediate functional improvement by sonic hedgehog against focal cerebral ischemia in rats. Exp. Neurol. 247, 680–688. doi: 10.1016/j.expneurol.2013.03.004
Huang, Z., Guo, L., Huang, L., Shi, Y., Liang, J., and Zhao, L. (2021a). Baicalin-loaded macrophage-derived exosomes ameliorate ischemic brain injury via the antioxidative pathway. Mater. Sci. Eng. C Mater. Biol. Appl. 126:112123. doi: 10.1016/j.msec.2021.112123
Huang, Z., Zhou, X., Zhang, X., Huang, L., Sun, Y., Cheng, Z., et al. (2021b). Pien-Tze-Huang, a Chinese patent formula, attenuates NLRP3 inflammasome-related neuroinflammation by enhancing autophagy via the AMPK/mTOR/ULK1 signaling pathway. Biomed. Pharmacother. 141:111814. doi: 10.1016/j.biopha.2021.111814
Huber, O., Korn, R., Mclaughlin, J., Ohsugi, M., Herrmann, B. G., and Kemler, R. (1996). Nuclear localization of beta-catenin by interaction with transcription factor LEF-1. Mech. Dev. 59, 3–10.
Hui, Z., Wang, S., Li, J., Wang, J., and Zhang, Z. (2022). Compound tongluo decoction inhibits endoplasmic reticulum stress-induced ferroptosis and promoted angiogenesis by activating the Sonic Hedgehog pathway in cerebral infarction. J. Ethnopharmacol. 283:114634. doi: 10.1016/j.jep.2021.114634
Ignarro, L. J., Byrns, R. E., Buga, G. M., and Wood, K. S. (1987). Endothelium-derived relaxing factor from pulmonary artery and vein possesses pharmacologic and chemical properties identical to those of nitric oxide radical. Circ. Res. 61, 866–879. doi: 10.1161/01.res.61.6.866
Infante, P., Alfonsi, R., Botta, B., Mori, M., and Di, M. (2015). Targeting GLI factors to inhibit the hedgehog pathway. Trends Pharmacol. Sci. 36, 547–558. doi: 10.1016/j.tips.2015.05.006
Irving, E. A., Barone, F. C., Reith, A. D., Hadingham, S. J., and Parsons, A. A. (2000). Differential activation of MAPK/ERK and p38/SAPK in neurones and glia following focal cerebral ischaemia in the rat. Brain Res. Mol. Brain Res. 77, 65–75. doi: 10.1016/s0169-328x(00)00043-7
Isaacs, A., and Burke, D. C. (1958). Mode of action of interferon. Nature 182, 1073–1074. doi: 10.1038/1821073a0
Isago, H., Mitani, A., Mikami, Y., Horie, M., Urushiyama, H., Hamamoto, R., et al. (2020). Epithelial expression of YAP and TAZ Is sequentially required in lung development. Am. J. Respir. Cell Mol. Biol. 62, 256–266. doi: 10.1165/rcmb.2019-0218OC
Ishikawa, H., Tajiri, N., Shinozuka, K., Vasconcellos, J., Kaneko, Y., Lee, H. J., et al. (2013). Vasculogenesis in experimental stroke after human cerebral endothelial cell transplantation. Stroke 44, 3473–3481. doi: 10.1161/STROKEAHA.113.001943
Issemann, I., and Green, S. (1990). Activation of a member of the steroid hormone receptor superfamily by peroxisome proliferators. Nature 347, 645–650. doi: 10.1038/347645a0
Ito, D., Tanahashi, N., Murata, M., Sato, H., Saito, I., Watanabe, K., et al. (2002). Notch3 gene polymorphism and ischaemic cerebrovascular disease. J. Neurol. Neurosurg. Psychiatry 72, 382–384.
Itoh, K., Wakabayashi, N., Katoh, Y., Ishii, T., Igarashi, K., Engel, J. D., et al. (1999). Keap1 represses nuclear activation of antioxidant responsive elements by Nrf2 through binding to the amino-terminal Neh2 domain. Genes Dev. 13, 76–86. doi: 10.1101/gad.13.1.76
Ivan, M., Kondo, K., Yang, H., Kim, W., Valiando, J., Ohh, M., et al. (2001). HIFalpha targeted for VHL-mediated destruction by proline hydroxylation: Implications for O2 sensing. Science 292, 464–468. doi: 10.1126/science.1059817
Jaakkola, P., Mole, D. R., Tian, Y. M., Wilson, M. I., Gielbert, J., Gaskell, S. J., et al. (2001). Targeting of HIF-alpha to the von Hippel-Lindau ubiquitylation complex by O2-regulated prolyl hydroxylation. Science 292, 468–472. doi: 10.1126/science.1059796
Jia, J., Cheng, J., Ni, J., and Zhen, X. (2015). Neuropharmacological actions of metformin in stroke. Curr. Neuropharmacol. 13, 389–394.
Jiang, M., Liu, X., Zhang, D., Wang, Y., Hu, X., Xu, F., et al. (2018). Celastrol treatment protects against acute ischemic stroke-induced brain injury by promoting an IL-33/ST2 axis-mediated microglia/macrophage M2 polarization. J. Neuroinflammation 15:78. doi: 10.1186/s12974-018-1124-6
Jiang, S., Li, T., Ji, T., Yi, W., Yang, Z., Wang, S., et al. (2018). AMPK: Potential therapeutic target for ischemic stroke. Theranostics 8, 4535–4551.
Jiang, T., Yu, J. T., Zhu, X. C., Zhang, Q. Q., Tan, M. S., Cao, L., et al. (2015). Ischemic preconditioning provides neuroprotection by induction of AMP-activated protein kinase-dependent autophagy in a rat model of ischemic stroke. Mol. Neurobiol. 51, 220–229. doi: 10.1007/s12035-014-8725-6
Jin, Y., Barnett, A., Zhang, Y., Yu, X., and Luo, Y. (2017). Poststroke sonic hedgehog agonist treatment improves functional recovery by enhancing neurogenesis and angiogenesis. Stroke 48, 1636–1645. doi: 10.1161/STROKEAHA.117.016650
Jovanovic, I. P., Pejnovic, N. N., Radosavljevic, G. D., Arsenijevic, N. N., and Lukic, M. L. (2012). IL-33/ST2 axis in innate and acquired immunity to tumors. Oncoimmunology 1, 229–231.
Justice, R. W., Zilian, O., Woods, D. F., Noll, M., and Bryant, P. J. (1995). The Drosophila tumor suppressor gene warts encodes a homolog of human myotonic dystrophy kinase and is required for the control of cell shape and proliferation. Genes Dev. 9, 534–546. doi: 10.1101/gad.9.5.534
Justicia, C., Gabriel, C., and Planas, A. M. (2000). Activation of the JAK/STAT pathway following transient focal cerebral ischemia: Signaling through Jak1 and Stat3 in astrocytes. Glia 30, 253–270. doi: 10.1002/(sici)1098-1136(200005)30:3<253::aid-glia5>3.0.co;2-o
Kader, A., Frazzini, V. I., Solomon, R. A., and Trifiletti, R. R. (1993). Nitric oxide production during focal cerebral ischemia in rats. Stroke 24, 1709–1716.
Kang, J. S., Liu, C., and Derynck, R. (2009). New regulatory mechanisms of TGF-beta receptor function. Trends Cell Biol. 19, 385–394.
Kang, R., Zhu, S., Zeh, H. J., Klionsky, D. J., and Tang, D. (2018). BECN1 is a new driver of ferroptosis. Autophagy 14, 2173–2175. doi: 10.1080/15548627.2018.1513758
Kango-Singh, M., Nolo, R., Tao, C., Verstreken, P., Hiesinger, P. R., Bellen, H. J., et al. (2002). Shar-pei mediates cell proliferation arrest during imaginal disc growth in Drosophila. Development 129, 5719–5730. doi: 10.1242/dev.00168
Karaman, S., Leppänen, V. M., and Alitalo, K. (2018). Vascular endothelial growth factor signaling in development and disease. Development 145:dev151019.
Karikó, K., Weissman, D., and Welsh, F. A. (2004). Inhibition of toll-like receptor and cytokine signaling–a unifying theme in ischemic tolerance. J. Cereb. Blood Flow Metab. 24, 1288–1304. doi: 10.1097/01.WCB.0000145666.68576.71
Kawai, T., and Akira, S. (2007). Signaling to NF-kappaB by Toll-like receptors. Trends Mol. Med. 13, 460–469.
Kemp, B. E., Mitchelhill, K. I., Stapleton, D., Michell, B. J., Chen, Z. P., and Witters, L. A. (1999). Dealing with energy demand: The AMP-activated protein kinase. Trends Biochem. Sci. 24, 22–25. doi: 10.1016/s0968-0004(98)01340-1
Khwaja, A., Rodriguez-Viciana, P., Wennström, S., Warne, P. H., and Downward, J. (1997). Matrix adhesion and Ras transformation both activate a phosphoinositide 3-OH kinase and protein kinase B/Akt cellular survival pathway. Embo J. 16, 2783–2793. doi: 10.1093/emboj/16.10.2783
Kibble, M., Saarinen, N., Tang, J., Wennerberg, K., Mäkelä, S., and Aittokallio, T. (2015). Network pharmacology applications to map the unexplored target space and therapeutic potential of natural products. Nat. Prod. Rep. 32, 1249–1266. doi: 10.1039/c5np00005j
Kidd, S., Kelley, M. R., and Young, M. W. (1986). Sequence of the notch locus of Drosophila melanogaster: Relationship of the encoded protein to mammalian clotting and growth factors. Mol. Cell Biol. 6, 3094–3108. doi: 10.1128/mcb.6.9.3094-3108.1986
Kim, H. J., Hawke, N., and Baldwin, A. S. (2006). NF-kappaB and IKK as therapeutic targets in cancer. Cell Death Differ. 13, 738–747.
Kishi, K., Sasaki, T., Kuroda, S., Itoh, T., and Takai, Y. (1993). Regulation of cytoplasmic division of Xenopus embryo by rho p21 and its inhibitory GDP/GTP exchange protein (rho GDI). J. Cell Biol. 120, 1187–1195. doi: 10.1083/jcb.120.5.1187
Kishore, R., Qin, G., Luedemann, C., Bord, E., Hanley, A., Silver, M., et al. (2005). The cytoskeletal protein ezrin regulates EC proliferation and angiogenesis via TNF-alpha-induced transcriptional repression of cyclin A. J. Clin. Invest. 115, 1785–1796. doi: 10.1172/JCI22849
Kitada, T., Asakawa, S., Hattori, N., Matsumine, H., Yamamura, Y., Minoshima, S., et al. (1998). Mutations in the parkin gene cause autosomal recessive juvenile parkinsonism. Nature 392, 605–608. doi: 10.1038/33416
Klaus, A., and Birchmeier, W. (2008). Wnt signalling and its impact on development and cancer. Nat. Rev. Cancer 8, 387–398.
Klein, C., and Westenberger, A. (2012). Genetics of Parkinson’s disease. Cold Spring Harb. Perspect. Med. 2:a008888.
Klempner, S. J., Myers, A. P., and Cantley, L. C. (2013). What a tangled web we weave: Emerging resistance mechanisms to inhibition of the phosphoinositide 3-kinase pathway. Cancer Discov. 3, 1345–1354. doi: 10.1158/2159-8290.CD-13-0063
Konsavage, W. M. Jr., Kyler, S. L., Rennoll, S. A., Jin, G., and Yochum, G. S. (2012). Wnt/β-catenin signaling regulates Yes-associated protein (YAP) gene expression in colorectal carcinoma cells. J. Biol. Chem. 287, 11730–11739.
Kraft, A. D., Johnson, D. A., and Johnson, J. A. (2004). Nuclear factor E2-related factor 2-dependent antioxidant response element activation by tert-butylhydroquinone and sulforaphane occurring preferentially in astrocytes conditions neurons against oxidative insult. J. Neurosci. 24, 1101–1112.
Krishnan, R., Mays, W., and Elijovich, L. (2021). Complications of mechanical thrombectomy in acute ischemic stroke. Neurology 97, S115–S125.
Krupinski, J., Issa, R., Bujny, T., Slevin, M., Kumar, P., Kumar, S., et al. (1997). A putative role for platelet-derived growth factor in angiogenesis and neuroprotection after ischemic stroke in humans. Stroke 28, 564–573. doi: 10.1161/01.str.28.3.564
Krupinski, J., Kaluza, J., Kumar, P., Kumar, S., and Wang, J. M. (1994). Role of angiogenesis in patients with cerebral ischemic stroke. Stroke 25, 1794–1798.
Krupinski, J., Kumar, P., Kumar, S., and Kaluza, J. (1996). Increased expression of TGF-beta 1 in brain tissue after ischemic stroke in humans. Stroke 27, 852–857.
Kumar, A., Takada, Y., Boriek, A. M., and Aggarwal, B. B. (2004). Nuclear factor-kappaB: Its role in health and disease. J. Mol. Med. 82, 434–448.
Lagna, G., Hata, A., Hemmati-Brivanlou, A., and Massagué, J. (1996). Partnership between DPC4 and SMAD proteins in TGF-beta signalling pathways. Nature 383, 832–836. doi: 10.1038/383832a0
Lai, M., Wang, D., Lin, Z., and Zhang, Y. (2016). Small molecule copper and its relative metabolites in serum of cerebral ischemic stroke patients. J. Stroke Cerebrovasc. Dis. 25, 214–219. doi: 10.1016/j.jstrokecerebrovasdis.2015.09.020
Lai, Z. C., Wei, X., Shimizu, T., Ramos, E., Rohrbaugh, M., Nikolaidis, N., et al. (2005). Control of cell proliferation and apoptosis by mob as tumor suppressor, mats. Cell 120, 675–685. doi: 10.1016/j.cell.2004.12.036
Lapchak, P. A. (2002). Development of thrombolytic therapy for stroke: A perspective. Expert Opin. Investig. Drugs 11, 1623–1632. doi: 10.1517/13543784.11.11.1623
Lapchak, P. A. (2010). A critical assessment of edaravone acute ischemic stroke efficacy trials: Is edaravone an effective neuroprotective therapy? Expert Opin. Pharmacother. 11, 1753–1763. doi: 10.1517/14656566.2010.493558
Lau, A., Villeneuve, N. F., Sun, Z., Wong, P. K., and Zhang, D. D. (2008). Dual roles of Nrf2 in cancer. Pharmacol. Res. 58, 262–270.
Lee, H., Zandkarimi, F., Zhang, Y., Meena, J. K., Kim, J., Zhuang, L., et al. (2020). Energy-stress-mediated AMPK activation inhibits ferroptosis. Nat. Cell Biol. 22, 225–234. doi: 10.1038/s41556-020-0461-8
Lehnardt, S., Lehmann, S., Kaul, D., Tschimmel, K., Hoffmann, O., Cho, S., et al. (2007). Toll-like receptor 2 mediates CNS injury in focal cerebral ischemia. J. Neuroimmunol. 190, 28–33. doi: 10.1016/j.jneuroim.2007.07.023
Lehnertz, K., BRöHL, T., and Wrede, R. V. (2023). Epileptic-network-based prediction and control of seizures in humans. Neurobiol. Dis. 181:106098. doi: 10.1016/j.nbd.2023.106098
Leker, R. R., Aharonowiz, M., Greig, N. H., and Ovadia, H. (2004). The role of p53-induced apoptosis in cerebral ischemia: Effects of the p53 inhibitor pifithrin alpha. Exp. Neurol. 187, 478–486. doi: 10.1016/j.expneurol.2004.01.030
Lemaitre, B., Nicolas, E., Michaut, L., Reichhart, J. M., and Hoffmann, J. A. (1996). The dorsoventral regulatory gene cassette spätzle/Toll/cactus controls the potent antifungal response in Drosophila adults. Cell 86, 973–983. doi: 10.1016/s0092-8674(00)80172-5
Leung, D. W., Cachianes, G., Kuang, W. J., Goeddel, D. V., and Ferrara, N. (1989). Vascular endothelial growth factor is a secreted angiogenic mitogen. Science 246, 1306–1309. doi: 10.1126/science.2479986
Leung, S. W., Lai, J. H., Wu, J. C., Tsai, Y. R., Chen, Y. H., Kang, S. J., et al. (2020). Neuroprotective effects of emodin against ischemia/reperfusion injury through activating ERK-1/2 signaling pathway. Int. J. Mol. Sci. 21:2899. doi: 10.3390/ijms21082899
Levine, A. J. (2020). p53: 800 million years of evolution and 40 years of discovery. Nat. Rev. Cancer 20, 471–480. doi: 10.1038/s41568-020-0262-1
Levine, A. J., Momand, J., and Finlay, C. A. (1991). The p53 tumour suppressor gene. Nature 351, 453–456.
Lezoualc’h, F., and Behl, C. (1998). Transcription factor NF-kappaB: Friend or foe of neurons? Mol. Psychiatry 3, 15–20. doi: 10.1038/sj.mp.4000295
Li, C., Dong, X., Du, W., Shi, X., Chen, K., Zhang, W., et al. (2020). LKB1-AMPK axis negatively regulates ferroptosis by inhibiting fatty acid synthesis. Signal. Transduct. Target Ther. 5:187. doi: 10.1038/s41392-020-00297-2
Li, C., Sui, C., Wang, W., Yan, J., Deng, N., Du, X., et al. (2021). Baicalin attenuates oxygen-glucose deprivation/reoxygenation-induced injury by modulating the BDNF-TrkB/PI3K/Akt and MAPK/Erk1/2 signaling axes in neuron-astrocyte cocultures. Front. Pharmacol. 12:599543. doi: 10.3389/fphar.2021.599543
Li, D., Ni, H., Rui, Q., Gao, R., and Chen, G. (2018). Mst1: Function and mechanism in brain and myocardial ischemia reperfusion injury. Curr. Neuropharmacol. 16, 1358–1364. doi: 10.2174/1570159X16666180516095949
Li, J., Cao, F., Yin, H. L., Huang, Z. J., Lin, Z. T., Mao, N., et al. (2020). Ferroptosis: Past, present and future. Cell Death Dis. 11:88.
Li, J., Zeng, Z., Viollet, B., Ronnett, G. V., and Mccullough, L. D. (2007). Neuroprotective effects of adenosine monophosphate-activated protein kinase inhibition and gene deletion in stroke. Stroke 38, 2992–2999. doi: 10.1161/STROKEAHA.107.490904
Li, L., Sun, L., Qiu, Y., Zhu, W., Hu, K., and Mao, J. (2020). Protective effect of stachydrine against cerebral ischemia-reperfusion injury by reducing inflammation and apoptosis through P65 and JAK2/STAT3 signaling pathway. Front. Pharmacol. 11:64. doi: 10.3389/fphar.2020.00064
Li, M., Meng, Z., Yu, S., Li, J., Wang, Y., Yang, W., et al. (2022). Baicalein ameliorates cerebral ischemia-reperfusion injury by inhibiting ferroptosis via regulating GPX4/ACSL4/ACSL3 axis. Chem. Biol. Interact. 366:110137. doi: 10.1016/j.cbi.2022.110137
Li, N., Jiang, W., Wang, W., Xiong, R., Wu, X., and Geng, Q. (2021). Ferroptosis and its emerging roles in cardiovascular diseases. Pharmacol. Res. 166:105466.
Li, Q. Q., Ding, D. H., Wang, X. Y., Sun, Y. Y., and Wu, J. (2021). Lipoxin A4 regulates microglial M1/M2 polarization after cerebral ischemia-reperfusion injury via the Notch signaling pathway. Exp. Neurol. 339:113645. doi: 10.1016/j.expneurol.2021.113645
Li, Q., Han, X., Lan, X., Gao, Y., Wan, J., Durham, F., et al. (2017). Inhibition of neuronal ferroptosis protects hemorrhagic brain. JCI Insight 2:e90777.
Li, Y., Chopp, M., Zhang, Z. G., Zaloga, C., Niewenhuis, L., and Gautam, S. (1994). p53-immunoreactive protein and p53 mRNA expression after transient middle cerebral artery occlusion in rats. Stroke 25, 849–855. doi: 10.1161/01.str.25.4.849
Li, Y., Suo, L., Liu, Y., Li, H., and Xue, W. (2017). Protective effects of ginsenoside Rg1 against oxygen-glucose-deprivation-induced apoptosis in neural stem cells. J. Neurol. Sci. 373, 107–112. doi: 10.1016/j.jns.2016.12.036
Li, Y., Zhu, Z. Y., Lu, B. W., Huang, T. T., Zhang, Y. M., Zhou, N. Y., et al. (2019). Rosiglitazone ameliorates tissue plasminogen activator-induced brain hemorrhage after stroke. CNS Neurosci. Ther. 25, 1343–1352. doi: 10.1111/cns.13260
Li, Z., Yan, Z., Xu, C., Dong, Y., Xiong, Y., and Dai, Y. (2018). Acetylshikonin attenuates angiotensin II-induced proliferation and motility of human brain smooth muscle cells by inhibiting Wnt/β-catenin signaling. Hum. Cell 31, 242–250.
Lian, I., Kim, J., Okazawa, H., Zhao, J., Zhao, B., Yu, J., et al. (2010). The role of YAP transcription coactivator in regulating stem cell self-renewal and differentiation. Genes Dev. 24, 1106–1118.
Liang, C., Ni, G. X., Shi, X. L., Jia, L., and Wang, Y. L. (2020). Astragaloside IV regulates the HIF/VEGF/Notch signaling pathway through miRNA-210 to promote angiogenesis after ischemic stroke. Restor. Neurol. Neurosci. 38, 271–282. doi: 10.3233/RNN-201001
Liu, C., He, P., Guo, Y., Tian, Q., Wang, J., Wang, G., et al. (2022). Taurine attenuates neuronal ferroptosis by regulating GABA(B)/AKT/GSK3β/β-catenin pathway after subarachnoid hemorrhage. Free Radic. Biol. Med. 193, 795–807.
Liu, F., Hata, A., Baker, J. C., Doody, J., Cárcamo, J., Harland, R. M., et al. (1996). A human Mad protein acting as a BMP-regulated transcriptional activator. Nature 381, 620–623. doi: 10.1038/381620a0
Liu, H., Wu, X., Luo, J., Wang, X., Guo, H., Feng, D., et al. (2019). Pterostilbene attenuates astrocytic inflammation and neuronal oxidative injury after ischemia-reperfusion by inhibiting NF-κB phosphorylation. Front. Immunol. 10:2408. doi: 10.3389/fimmu.2019.02408
Liu, J., and Wang, L. N. (2017). Peroxisome proliferator-activated receptor gamma agonists for preventing recurrent stroke and other vascular events in people with stroke or transient ischaemic attack. Cochrane Database Syst. Rev. 12:Cd010693.
Liu, T., Ding, Y., and Wen, A. (2018). Traditional Chinese medicine for ischaemic stroke. Lancet Neurol. 17:745.
Liu, Y., Tang, G., Li, Y., Wang, Y., Chen, X., Gu, X., et al. (2014). Metformin attenuates blood-brain barrier disruption in mice following middle cerebral artery occlusion. J. Neuroinflammation 11:177. doi: 10.1186/s12974-014-0177-4
Liu, Z., Zhou, Z., Ai, P., Zhang, C., Chen, J., and Wang, Y. (2022). Astragaloside IV attenuates ferroptosis after subarachnoid hemorrhage via Nrf2/HO-1 signaling pathway. Front. Pharmacol. 13:924826. doi: 10.3389/fphar.2022.924826
Lou, Y., Ma, M., Jiang, Y., Xu, H., Gao, Z., Gao, L., et al. (2022). Ferroptosis: A new strategy for traditional Chinese medicine treatment of stroke. Biomed. Pharmacother. 156:113806.
Louandre, C., Ezzoukhry, Z., Godin, C., Barbare, J. C., Mazière, J. C., Chauffert, B., et al. (2013). Iron-dependent cell death of hepatocellular carcinoma cells exposed to sorafenib. Int. J. Cancer 133, 1732–1742. doi: 10.1002/ijc.28159
Lu, W., Chen, Z., and Wen, J. (2021). RhoA/ROCK signaling pathway and astrocytes in ischemic stroke. Metab. Brain Dis. 36, 1101–1108. doi: 10.1007/s11011-021-00709-4
Luo, Y., Kuo, C. C., Shen, H., Chou, J., Greig, N. H., Hoffer, B. J., et al. (2009). Delayed treatment with a p53 inhibitor enhances recovery in stroke brain. Ann. Neurol. 65, 520–530.
Luo, Y., Yin, W., Signore, A. P., Zhang, F., Hong, Z., Wang, S., et al. (2006). Neuroprotection against focal ischemic brain injury by the peroxisome proliferator-activated receptor-gamma agonist rosiglitazone. J. Neurochem. 97, 435–448.
Lv, H., Liu, X., Zeng, X., Liu, Y., Zhang, C., Zhang, Q., et al. (2022). Comprehensive analysis of cuproptosis-related genes in immune infiltration and prognosis in melanoma. Front. Pharmacol. 13:930041. doi: 10.3389/fphar.2022.930041
Mao, Z., Tian, L., Liu, J., Wu, Q., Wang, N., Wang, G., et al. (2022). Ligustilide ameliorates hippocampal neuronal injury after cerebral ischemia reperfusion through activating PINK1/Parkin-dependent mitophagy. Phytomedicine 101:154111. doi: 10.1016/j.phymed.2022.154111
Martí-Carvajal, A. J., Valli, C., Martí-Amarista, C. E., Solà, I., Martí-Fàbregas, J., and Bonfill, C. (2020). Citicoline for treating people with acute ischemic stroke. Cochrane Database Syst. Rev. 8:Cd013066.
Mastroiacovo, F., Busceti, C. L., Biagioni, F., Moyanova, S. G., Meisler, M. H., Battaglia, G., et al. (2009). Induction of the Wnt antagonist, Dickkopf-1, contributes to the development of neuronal death in models of brain focal ischemia. J. Cereb. Blood Flow Metab. 29, 264–276. doi: 10.1038/jcbfm.2008.111
Matise, M. P., and Joyner, A. L. (1999). Gli genes in development and cancer. Oncogene 18, 7852–7859.
Matsuda, N., Sato, S., Shiba, K., Okatsu, K., Saisho, K., Gautier, C. A., et al. (2010). PINK1 stabilized by mitochondrial depolarization recruits Parkin to damaged mitochondria and activates latent Parkin for mitophagy. J. Cell Biol. 189, 211–221. doi: 10.1083/jcb.200910140
Matsushima, M., Fujiwara, T., Takahashi, E., Minaguchi, T., Eguchi, Y., Tsujimoto, Y., et al. (1998). Isolation, mapping, and functional analysis of a novel human cDNA (BNIP3L) encoding a protein homologous to human NIP3. Genes Chromosomes Cancer 21, 230–235.
Mccrea, P. D., Turck, C. W., and Gumbiner, B. (1991). A homolog of the armadillo protein in Drosophila (plakoglobin) associated with E-cadherin. Science 254, 1359–1361. doi: 10.1126/science.1962194
Mccullough, L. D., Zeng, Z., Li, H., Landree, L. E., Mcfadden, J., and Ronnett, G. V. (2005). Pharmacological inhibition of AMP-activated protein kinase provides neuroprotection in stroke. J. Biol. Chem. 280, 20493–20502.
Menet, R., Lecordier, S., and Elali, A. (2020). Wnt Pathway: An emerging player in vascular and traumatic mediated brain injuries. Front. Physiol. 11:565667. doi: 10.3389/fphys.2020.565667
Meng, Z., Moroishi, T., and Guan, K. L. (2016). Mechanisms of Hippo pathway regulation. Genes Dev. 30, 1–17.
Mengesdorf, T., Jensen, P. H., Mies, G., Aufenberg, C., and Paschen, W. (2002). Down-regulation of parkin protein in transient focal cerebral ischemia: A link between stroke and degenerative disease? Proc. Natl. Acad. Sci. U. S. A. 99, 15042–15047. doi: 10.1073/pnas.232588799
Metz, C. W., and Bridges, C. B. (1917). Incompatibility of mutant races in drosophila. Proc. Natl. Acad. Sci. U. S. A. 3, 673–678. doi: 10.1073/pnas.3.12.673
Mi, D. H., Fang, H. J., Zheng, G. H., Liang, X. H., Ding, Y. R., Liu, X., et al. (2019). DPP-4 inhibitors promote proliferation and migration of rat brain microvascular endothelial cells under hypoxic/high-glucose conditions, potentially through the SIRT1/HIF-1/VEGF pathway. CNS Neurosci. Ther. 25, 323–332. doi: 10.1111/cns.13042
Minhas, J. S., Chithiramohan, T., Wang, X., Barnes, S. C., Clough, R. H., Kadicheeni, M., et al. (2022). Oral antiplatelet therapy for acute ischaemic stroke. Cochrane Database Syst. Rev. 1:Cd000029.
Mirończuk, A., Kapica-Topczewska, K., Socha, K., Soroczyńska, J., Jamiołkowski, J., Kułakowska, A., et al. (2021). Selenium, copper, zinc concentrations and Cu/Zn, Cu/Se molar ratios in the serum of patients with acute ischemic stroke in northeastern poland-a new insight into stroke pathophysiology. Nutrients 13:2139. doi: 10.3390/nu13072139
Moi, P., Chan, K., Asunis, I., Cao, A., and Kan, Y. W. (1994). Isolation of NF-E2-related factor 2 (Nrf2), a NF-E2-like basic leucine zipper transcriptional activator that binds to the tandem NF-E2/AP1 repeat of the beta-globin locus control region. Proc. Natl. Acad. Sci. U. S. A. 91, 9926–9930. doi: 10.1073/pnas.91.21.9926
Morii, N., Sekine, A., Ohashi, Y., Nakao, K., Imura, H., Fujiwara, M., et al. (1988). Purification and properties of the cytosolic substrate for botulinum ADP-ribosyltransferase. Identification as an Mr 22,000 guanine nucleotide-binding protein. J. Biol. Chem. 263, 12420–12426.
Moroishi, T., Hansen, C. G., and Guan, K. L. (2015). The emerging roles of YAP and TAZ in cancer. Nat. Rev. Cancer 15, 73–79.
Moya, I. M., and Halder, G. (2019). Hippo-YAP/TAZ signalling in organ regeneration and regenerative medicine. Nat. Rev. Mol. Cell Biol. 20, 211–226. doi: 10.1038/s41580-018-0086-y
Muller, P. A., and Vousden, K. H. (2014). Mutant p53 in cancer: New functions and therapeutic opportunities. Cancer Cell 25, 304–317.
Munday, M. R., Campbell, D. G., Carling, D., and Hardie, D. G. (1988). Identification by amino acid sequencing of three major regulatory phosphorylation sites on rat acetyl-CoA carboxylase. Eur. J. Biochem. 175, 331–338. doi: 10.1111/j.1432-1033.1988.tb14201.x
Nalamolu, K. R., Challa, S. R., Fornal, C. A., Grudzien, N. A., Jorgenson, L. C., Choudry, M. M., et al. (2021). Attenuation of the Induction of TLRs 2 and 4 mitigates inflammation and promotes neurological recovery after focal cerebral ischemia. Transl. Stroke Res. 12, 923–936. doi: 10.1007/s12975-020-00884-z
Narumiya, S., Sekine, A., and Fujiwara, M. (1988). Substrate for botulinum ADP-ribosyltransferase, Gb, has an amino acid sequence homologous to a putative rho gene product. J. Biol. Chem. 263, 17255–17257.
Ni, J., Yao, M., Wang, L. H., Yu, M., Li, R. H., Zhao, L. H., et al. (2021). Human urinary kallidinogenase in acute ischemic stroke: A single-arm, multicenter, phase IV study (RESK study). CNS Neurosci. Ther. 27, 1493–1503.
Nitatori, T., Sato, N., Waguri, S., Karasawa, Y., Araki, H., Shibanai, K., et al. (1995). Delayed neuronal death in the CA1 pyramidal cell layer of the gerbil hippocampus following transient ischemia is apoptosis. J. Neurosci. 15, 1001–1011.
Nomura, N., Miyajima, N., Sazuka, T., Tanaka, A., Kawarabayasi, Y., Sato, S., et al. (1994). Prediction of the coding sequences of unidentified human genes. I. The coding sequences of 40 new genes (KIAA0001-KIAA0040) deduced by analysis of randomly sampled cDNA clones from human immature myeloid cell line KG-1 (supplement). DNA Res. 1, 47–56. doi: 10.1093/dnares/1.1.47
Noordermeer, J., Klingensmith, J., Perrimon, N., and Nusse, R. (1994). Dishevelled and armadillo act in the wingless signalling pathway in Drosophila. Nature 367, 80–83.
Nusse, R., and Varmus, H. E. (1982). Many tumors induced by the mouse mammary tumor virus contain a provirus integrated in the same region of the host genome. Cell 31, 99–109.
Nusse, R., Brown, A., Papkoff, J., Scambler, P., Shackleford, G., Mcmahon, A., et al. (1991). A new nomenclature for int-1 and related genes: The Wnt gene family. Cell 64:231. doi: 10.1016/0092-8674(91)90633-a
Nüsslein-Volhard, C., and Wieschaus, E. (1980). Mutations affecting segment number and polarity in Drosophila. Nature 287, 795–801.
Oda, T., Elkahloun, A. G., Pike, B. L., Okajima, K., Krantz, I. D., Genin, A., et al. (1997). Mutations in the human Jagged1 gene are responsible for Alagille syndrome. Nat. Genet. 16, 235–242.
Ohashi, Y., and Narumiya, S. (1987). ADP-ribosylation of a Mr 21,000 membrane protein by type D botulinum toxin. J. Biol. Chem. 262, 1430–1433.
O’Neill, L. A., and Hardie, D. G. (2013). Metabolism of inflammation limited by AMPK and pseudo-starvation. Nature 493, 346–355. doi: 10.1038/nature11862
O’Rourke, F., Dean, N., Akhtar, N., and Shuaib, A. (2004). Current and future concepts in stroke prevention. CMAJ 170, 1123–1133.
O’Shea, J. J., and Murray, P. J. (2008). Cytokine signaling modules in inflammatory responses. Immunity 28, 477–487.
Pahl, H. L. (1999). Activators and target genes of Rel/NF-kappaB transcription factors. Oncogene 18, 6853–6866.
Palmer, G., and Gabay, C. (2011). Interleukin-33 biology with potential insights into human diseases. Nat. Rev. Rheumatol. 7, 321–329.
Palmer, R. M., Ferrige, A. G., and Moncada, S. (1987). Nitric oxide release accounts for the biological activity of endothelium-derived relaxing factor. Nature 327, 524–526.
Pan, B., Sun, J., Liu, Z., Wang, L., Huo, H., Zhao, Y., et al. (2021). Longxuetongluo capsule protects against cerebral ischemia/reperfusion injury through endoplasmic reticulum stress and MAPK-mediated mechanisms. J. Adv. Res. 33, 215–225. doi: 10.1016/j.jare.2021.01.016
Pantalacci, S., Tapon, N., and Léopold, P. (2003). The Salvador partner Hippo promotes apoptosis and cell-cycle exit in Drosophila. Nat. Cell Biol. 5, 921–927. doi: 10.1038/ncb1051
Park, H. W., Kim, Y. C., Yu, B., Moroishi, T., Mo, J. S., Plouffe, S. W., et al. (2015). Alternative Wnt signaling activates YAP/TAZ. Cell 162, 780–794.
Pepicelli, C. V., Lewis, P. M., and Mcmahon, A. P. (1998). Sonic hedgehog regulates branching morphogenesis in the mammalian lung. Curr. Biol. 8, 1083–1086.
Philips, R. L., Wang, Y., Cheon, H., Kanno, Y., Gadina, M., Sartorelli, V., et al. (2022). The JAK-STAT pathway at 30: Much learned, much more to do. Cell 185, 3857–3876. doi: 10.1016/j.cell.2022.09.023
Pikarsky, E., Porat, R. M., Stein, I., Abramovitch, R., Amit, S., Kasem, S., et al. (2004). NF-kappaB functions as a tumour promoter in inflammation-associated cancer. Nature 431, 461–466.
Pobbati, A. V., and Hong, W. (2020). A combat with the YAP/TAZ-TEAD oncoproteins for cancer therapy. Theranostics 10, 3622–3635. doi: 10.7150/thno.40889
Powers, W. J., Rabinstein, A. A., Ackerson, T., Adeoye, O. M., Bambakidis, N. C., Becker, K., et al. (2019). Guidelines for the early management of patients with acute ischemic stroke: 2019 update to the 2018 guidelines for the early management of acute ischemic stroke: A guideline for healthcare professionals from the american heart association/american stroke association. Stroke 50, e344–e418.
Qin, C., Yang, S., Chu, Y. H., Zhang, H., Pang, X. W., Chen, L., et al. (2022). Signaling pathways involved in ischemic stroke: Molecular mechanisms and therapeutic interventions. Signal. Transduct. Target Ther. 7, 215.
Ran, Y., Su, W., Gao, F., Ding, Z., Yang, S., Ye, L., et al. (2021). Curcumin ameliorates white matter injury after ischemic stroke by inhibiting microglia/macrophage pyroptosis through NF-κB suppression and NLRP3 inflammasome inhibition. Oxid. Med. Cell Longev. 2021:1552127.
Ratan, R. R., Murphy, T. H., and Baraban, J. M. (1994). Macromolecular synthesis inhibitors prevent oxidative stress-induced apoptosis in embryonic cortical neurons by shunting cysteine from protein synthesis to glutathione. J. Neurosci. 14, 4385–4392. doi: 10.1523/JNEUROSCI.14-07-04385.1994
Ray, L. B., and Sturgill, T. W. (1988). Insulin-stimulated microtubule-associated protein kinase is phosphorylated on tyrosine and threonine in vivo. Proc. Natl. Acad. Sci. U. S. A. 85, 3753–3757. doi: 10.1073/pnas.85.11.3753
Ridley, A. J., and Hall, A. (1992). The small GTP-binding protein rho regulates the assembly of focal adhesions and actin stress fibers in response to growth factors. Cell 70, 389–399. doi: 10.1016/0092-8674(92)90163-7
Ridnour, L. A., Cheng, R. Y., Switzer, C. H., Heinecke, J. L., Ambs, S., Glynn, S., et al. (2013). Molecular pathways: Toll-like receptors in the tumor microenvironment–poor prognosis or new therapeutic opportunity. Clin. Cancer Res. 19, 1340–1346. doi: 10.1158/1078-0432.CCR-12-0408
Rijken, D. C., and Collen, D. (1981). Purification and characterization of the plasminogen activator secreted by human melanoma cells in culture. J. Biol. Chem. 256, 7035–7041. doi: 10.1016/S0021-9258(19)69095-2
Rikitake, Y., Kim, H. H., Huang, Z., Seto, M., Yano, K., Asano, T., et al. (2005). Inhibition of Rho kinase (ROCK) leads to increased cerebral blood flow and stroke protection. Stroke 36, 2251–2257. doi: 10.1161/01.STR.0000181077.84981.11
Roberts, A. B., Anzano, M. A., Wakefield, L. M., Roche, N. S., Stern, D. F., and Sporn, M. B. (1985). Type beta transforming growth factor: A bifunctional regulator of cellular growth. Proc. Natl. Acad. Sci. U. S. A. 82, 119–123. doi: 10.1073/pnas.82.1.119
Ronkina, N., and Gaestel, M. (2022). MAPK-activated protein kinases: Servant or partner? Annu. Rev. Biochem. 91, 505–540. doi: 10.1146/annurev-biochem-081720-114505
Salminen, A., and Kaarniranta, K. (2012). AMP-activated protein kinase (AMPK) controls the aging process via an integrated signaling network. Ageing Res. Rev. 11, 230–241. doi: 10.1016/j.arr.2011.12.005
Sandercock, P. A., Counsell, C., and Kamal, A. K. (2008). Anticoagulants for acute ischaemic stroke. Cochrane Database Syst. Rev. 10:Cd000024. doi: 10.1002/14651858.CD000024.pub3
Sarfo, F. S., Nichols, M., Opare-Addo, P. A., and Ovbiagele, B. (2023). Polypill programs to prevent stroke and cut costs in low income countries: Moving from clinical efficacy to pragmatic implementation. Stroke 54, 407–414. doi: 10.1161/STROKEAHA.122.039567
Sarin, A., and Marcel, N. (2017). The NOTCH1-autophagy interaction: Regulating self-eating for survival. Autophagy 13, 446–447. doi: 10.1080/15548627.2016.1268303
Sasaki, H., Nishizaki, Y., Hui, C., Nakafuku, M., and Kondoh, H. (1999). Regulation of Gli2 and Gli3 activities by an amino-terminal repression domain: Implication of Gli2 and Gli3 as primary mediators of Shh signaling. Development 126, 3915–3924. doi: 10.1242/dev.126.17.3915
Sawe, N., Steinberg, G., and Zhao, H. (2008). Dual roles of the MAPK/ERK1/2 cell signaling pathway after stroke. J. Neurosci. Res. 86, 1659–1669. doi: 10.1002/jnr.21604
Schindler, C. W. (2002). Series introduction. JAK-STAT signaling in human disease. J. Clin. Invest. 109, 1133–1137. doi: 10.1172/JCI0215644
Schmitz, J., Owyang, A., Oldham, E., Song, Y., Murphy, E., Mcclanahan, T. K., et al. (2005). IL-33, an interleukin-1-like cytokine that signals via the IL-1 receptor-related protein ST2 and induces T helper type 2-associated cytokines. Immunity 23, 479–490. doi: 10.1016/j.immuni.2005.09.015
Schneider, A., Martin-Villalba, A., Weih, F., Vogel, J., Wirth, T., and Schwaninger, M. (1999). NF-kappaB is activated and promotes cell death in focal cerebral ischemia. Nat. Med. 5, 554–559. doi: 10.1038/8432
Seiler, A., Schneider, M., FöRSTER, H., Roth, S., Wirth, E. K., Culmsee, C., et al. (2008). Glutathione peroxidase 4 senses and translates oxidative stress into 12/15-lipoxygenase dependent- and AIF-mediated cell death. Cell Metab. 8, 237–248. doi: 10.1016/j.cmet.2008.07.005
Semenza, G. L., Nejfelt, M. K., Chi, S. M., and Antonarakis, S. E. (1991). Hypoxia-inducible nuclear factors bind to an enhancer element located 3’ to the human erythropoietin gene. Proc. Natl. Acad. Sci. U. S. A. 88, 5680–5684. doi: 10.1073/pnas.88.13.5680
Sen, R., and Baltimore, D. (1986). Multiple nuclear factors interact with the immunoglobulin enhancer sequences. Cell 46, 705–716.
Senger, D. R., Galli, S. J., Dvorak, A. M., Perruzzi, C. A., Harvey, V. S., and Dvorak, H. F. (1983). Tumor cells secrete a vascular permeability factor that promotes accumulation of ascites fluid. Science 219, 983–985. doi: 10.1126/science.6823562
Shariati, M., and Meric-Bernstam, F. (2019). Targeting AKT for cancer therapy. Expert. Opin. Investig. Drugs 28, 977–988. doi: 10.1080/13543784.2019.1676726
Shi, L., Yuan, Y., Xiao, Y., Long, P., Li, W., Yu, Y., et al. (2021). Associations of plasma metal concentrations with the risks of all-cause and cardiovascular disease mortality in Chinese adults. Environ. Int. 157:106808. doi: 10.1016/j.envint.2021.106808
Shi, S., Wang, M., Liu, X., Han, S., and Zhu, P. (2022). Scalp electroacupuncture promotes angiogenesis after stroke in rats by activation of Wnt/β-catenin signal pathway. Evid. Based Complement Alternat. Med. 2022:1649605.
Shibuya, M., Hirai, S., Seto, M., Satoh, S., and Ohtomo, E. (2005). Effects of fasudil in acute ischemic stroke: Results of a prospective placebo-controlled double-blind trial. J. Neurol. Sci. 238, 31–39. doi: 10.1016/j.jns.2005.06.003
Shih, A. Y., Li, P., and Murphy, T. H. (2005). A small-molecule-inducible Nrf2-mediated antioxidant response provides effective prophylaxis against cerebral ischemia in vivo. J. Neurosci. 25, 10321–10335. doi: 10.1523/JNEUROSCI.4014-05.2005
Shim, J. W., and Madsen, J. R. (2018). VEGF signaling in neurological disorders. Int. J. Mol. Sci. 19:275.
Shimokawa, H., and Takeshita, A. (2005). Rho-kinase is an important therapeutic target in cardiovascular medicine. Arterioscler. Thromb. Vasc. Biol. 25, 1767–1775.
Shin, H. K., Salomone, S., Potts, E. M., Lee, S. W., Millican, E., Noma, K., et al. (2007). Rho-kinase inhibition acutely augments blood flow in focal cerebral ischemia via endothelial mechanisms. J. Cereb. Blood Flow Metab. 27, 998–1009. doi: 10.1038/sj.jcbfm.9600406
Shruster, A., Ben-Zur, T., Melamed, E., and Offen, D. (2012). Wnt signaling enhances neurogenesis and improves neurological function after focal ischemic injury. PLoS One 7:e40843. doi: 10.1371/journal.pone.0040843
Siegfried, E., Wilder, E. L., and Perrimon, N. (1994). Components of wingless signalling in Drosophila. Nature 367, 76–80.
Sim, A. T., and Hardie, D. G. (1988). The low activity of acetyl-CoA carboxylase in basal and glucagon-stimulated hepatocytes is due to phosphorylation by the AMP-activated protein kinase and not cyclic AMP-dependent protein kinase. FEBS Lett. 233, 294–298. doi: 10.1016/0014-5793(88)80445-9
Singh, N., Menon, B. K., Dmytriw, A. A., Regenhardt, R. W., Hirsch, J. A., and Ganesh, A. (2023). Replacing alteplase with tenecteplase: Is the time ripe? J. Stroke 25, 72–80. doi: 10.5853/jos.2022.02880
Son, T. G., Camandola, S., Arumugam, T. V., Cutler, R. G., Telljohann, R. S., Mughal, M. R., et al. (2010). Plumbagin, a novel Nrf2/ARE activator, protects against cerebral ischemia. J. Neurochem. 112, 1316–1326. doi: 10.1111/j.1471-4159.2009.06552.x
Song, X., Zhu, S., Chen, P., Hou, W., Wen, Q., Liu, J., et al. (2018). AMPK-mediated BECN1 phosphorylation promotes ferroptosis by directly blocking system X(c)(-) activity. Curr. Biol. 28, 2388–2399e5. doi: 10.1016/j.cub.2018.05.094
Speer, R. E., Karuppagounder, S. S., Basso, M., Sleiman, S. F., Kumar, A., Brand, D., et al. (2013). Hypoxia-inducible factor prolyl hydroxylases as targets for neuroprotection by “antioxidant” metal chelators: From ferroptosis to stroke. Free Radic. Biol. Med. 62, 26–36. doi: 10.1016/j.freeradbiomed.2013.01.026
Stockwell, B. R., Friedmann, A., Bayir, H., Bush, A. I., Conrad, M., Dixon, S. J., et al. (2017). Ferroptosis: A regulated cell death nexus linking metabolism, redox biology, and disease. Cell 171, 273–285.
Sun, E., Zhang, J., Deng, Y., Wang, J., Wu, Q., Chen, W., et al. (2022). Docosahexaenoic acid alleviates brain damage by promoting mitophagy in mice with ischaemic stroke. Oxid. Med. Cell Longev. 2022:3119649. doi: 10.1155/2022/3119649
Sun, F. L., Wang, W., Zuo, W., Xue, J. L., Xu, J. D., Ai, H. X., et al. (2014). Promoting neurogenesis via Wnt/β-catenin signaling pathway accounts for the neurorestorative effects of morroniside against cerebral ischemia injury. Eur. J. Pharmacol. 738, 214–221.
Sun, L., Zhang, H., Wang, W., Chen, Z., Wang, S., Li, J., et al. (2020). Astragaloside IV exerts cognitive benefits and promotes hippocampal neurogenesis in stroke mice by downregulating interleukin-17 expression via wnt pathway. Front. Pharmacol. 11:421. doi: 10.3389/fphar.2020.00421
Sun, Z., Da, F., Moreno, M., Holton, N. E., Sweat, M., Sweat, Y., et al. (2018). FoxO6 regulates Hippo signaling and growth of the craniofacial complex. PLoS Genet. 14:e1007675. doi: 10.1371/journal.pgen.1007675
Suzuki, S., Tanaka, K., Nogawa, S., Dembo, T., Kosakai, A., and Fukuuchi, Y. (2001). Phosphorylation of signal transducer and activator of transcription-3 (Stat3) after focal cerebral ischemia in rats. Exp. Neurol. 170, 63–71.
Szabo, C. (2017). Hydrogen sulfide, an enhancer of vascular nitric oxide signaling: Mechanisms and implications. Am. J. Physiol. Cell Physiol. 312, C3–C15.
Tachibana, E., Harada, T., Shibuya, M., Saito, K., Takayasu, M., Suzuki, Y., et al. (1999). Intra-arterial infusion of fasudil hydrochloride for treating vasospasm following subarachnoid haemorrhage. Acta Neurochir. 141, 13–19.
Tam, J. P., Marquardt, H., Rosberger, D. F., Wong, T. W., and Todaro, G. J. (1984). Synthesis of biologically active rat transforming growth factor I. Nature 309, 376–378.
Tan, J., Luo, J., Meng, C., Jiang, N., Cao, J., and Zhao, J. (2021). Syringin exerts neuroprotective effects in a rat model of cerebral ischemia through the FOXO3a/NF-κB pathway. Int. Immunopharmacol. 90:107268.
Tang, C., Hong, J., Hu, C., Huang, C., Gao, J., Huang, J., et al. (2021). Palmatine protects against cerebral ischemia/reperfusion injury by activation of the AMPK/Nrf2 pathway. Oxid. Med. Cell Longev. 2021:6660193. doi: 10.1155/2021/6660193
Tang, D., Chen, X., and Kroemer, G. (2022). Cuproptosis: A copper-triggered modality of mitochondrial cell death. Cell Res. 32, 417–418.
Tang, J., Hu, Z., Tan, J., Yang, S., and Zeng, L. (2016). Parkin protects against oxygen-glucose deprivation/reperfusion insult by promoting Drp1 degradation. Oxid. Med. Cell Longev. 2016:8474303. doi: 10.1155/2016/8474303
Tang, S. C., Yeh, S. J., Li, Y. I., Wang, Y. C., Baik, S. H., Santro, T., et al. (2013). Evidence for a detrimental role of TLR8 in ischemic stroke. Exp. Neurol. 250, 341–347. doi: 10.1016/j.expneurol.2013.10.012
Tao, G., Kahr, P. C., Morikawa, Y., Zhang, M., Rahmani, M., Heallen, T. R., et al. (2016). Pitx2 promotes heart repair by activating the antioxidant response after cardiac injury. Nature 534, 119–123. doi: 10.1038/nature17959
Tapon, N., Harvey, K. F., Bell, D. W., Wahrer, D. C., Schiripo, T. A., Haber, D., et al. (2002). Salvador promotes both cell cycle exit and apoptosis in Drosophila and is mutated in human cancer cell lines. Cell 110, 467–478. doi: 10.1016/s0092-8674(02)00824-3
Taylor, J. M., Cohen, S., and Mitchell, W. M. (1970). Epidermal growth factor: High and low molecular weight forms. Proc. Natl. Acad. Sci. U. S. A. 67, 164–171.
Thompson, C. B. (2016). Into thin air: How we sense and respond to hypoxia. Cell 167, 9–11. doi: 10.1016/j.cell.2016.08.036
Tomas, A., Futter, C. E., and Eden, E. R. (2014). EGF receptor trafficking: Consequences for signaling and cancer. Trends Cell Biol. 24, 26–34.
Totaro, A., Castellan, M., Battilana, G., Zanconato, F., Azzolin, L., Giulitti, S., et al. (2017). YAP/TAZ link cell mechanics to Notch signalling to control epidermal stem cell fate. Nat. Commun. 8:15206. doi: 10.1038/ncomms15206
Totaro, A., Castellan, M., Di, B., and Piccolo, S. (2018). Crosstalk between YAP/TAZ and Notch Signaling. Trends Cell Biol. 28, 560–573.
Tsai, B. P., Hoverter, N. P., and Waterman, M. L. (2012). Blending hippo and WNT: Sharing messengers and regulation. Cell 151, 1401–1403. doi: 10.1016/j.cell.2012.12.007
Tschaharganeh, D. F., Chen, X., Latzko, P., Malz, M., Gaida, M. M., Felix, K., et al. (2013). Yes-associated protein up-regulates Jagged-1 and activates the Notch pathway in human hepatocellular carcinoma. Gastroenterology 144, 1530–1542.e12. doi: 10.1053/j.gastro.2013.02.009
Tsivgoulis, G., Kadlecová, P., Kobayashi, A., Czlonkowska, A., Brozman, M., Švigelj, V., et al. (2015). Safety of statin pretreatment in intravenous thrombolysis for acute ischemic stroke. Stroke 46, 2681–2684.
Tuo, Q. Z., Liu, Y., Xiang, Z., Yan, H. F., Zou, T., Shu, Y., et al. (2022). Thrombin induces ACSL4-dependent ferroptosis during cerebral ischemia/reperfusion. Signal. Transduct. Target Ther. 7:59. doi: 10.1038/s41392-022-00917-z
Udan, R. S., Kango-Singh, M., Nolo, R., Tao, C., and Halder, G. (2003). Hippo promotes proliferation arrest and apoptosis in the Salvador/Warts pathway. Nat. Cell. Biol. 5, 914–920. doi: 10.1038/ncb1050
Uehata, M., Ishizaki, T., Satoh, H., Ono, T., Kawahara, T., Morishita, T., et al. (1997). Calcium sensitization of smooth muscle mediated by a Rho-associated protein kinase in hypertension. Nature 389, 990–994.
Ugolini, F., Charafe-Jauffret, E., Bardou, V. J., Geneix, J., Adélaïde, J., Labat-Moleur, F., et al. (2001). WNT pathway and mammary carcinogenesis: Loss of expression of candidate tumor suppressor gene SFRP1 in most invasive carcinomas except of the medullary type. Oncogene 20, 5810–5817. doi: 10.1038/sj.onc.1204706
Valente, E. M., Abou-Sleiman, P. M., Caputo, V., Muqit, M. M., Harvey, K., Gispert, S., et al. (2004). Hereditary early-onset Parkinson’s disease caused by mutations in PINK1. Science 304, 1158–1160.
Varelas, X., Miller, B. W., Sopko, R., Song, S., Gregorieff, A., Fellouse, F. A., et al. (2010). The Hippo pathway regulates Wnt/beta-catenin signaling. Dev. Cell 18, 579–591.
Varelas, X., Sakuma, R., Samavarchi-Tehrani, P., Peerani, R., Rao, B. M., Dembowy, J., et al. (2008). TAZ controls Smad nucleocytoplasmic shuttling and regulates human embryonic stem-cell self-renewal. Nat. Cell Biol. 10, 837–848. doi: 10.1038/ncb1748
Varjosalo, M., and Taipale, J. (2008). Hedgehog: Functions and mechanisms. Genes Dev. 22, 2454–2472.
Veikkola, T., and Alitalo, K. (1999). VEGFs, receptors and angiogenesis. Semin. Cancer Biol. 9, 211–220.
Vousden, K. H., and Lu, X. (2002). Live or let die: The cell’s response to p53. Nat. Rev. Cancer 2, 594–604.
Vyas, M. V., Silver, F. L., Austin, P. C., Yu, A. Y. X., Pequeno, P., Fang, J., et al. (2021). Stroke incidence by sex across the lifespan. Stroke 52, 447–451.
Wabnitz, A., and Chimowitz, M. (2017). Angioplasty, stenting and other potential treatments of atherosclerotic stenosis of the intracranial arteries: Past, present and future. J. Stroke 19, 271–276. doi: 10.5853/jos.2017.01837
Wagner, E. F., and Nebreda, A. R. (2009). Signal integration by JNK and p38 MAPK pathways in cancer development. Nat. Rev. Cancer 9, 537–549.
Waldner, M. J., and Neurath, M. F. (2012). Targeting the VEGF signaling pathway in cancer therapy. Expert. Opin. Ther. Targets 16, 5–13.
Wan, D., Zhou, Y., Wang, K., Hou, Y., Hou, R., and Ye, X. (2016). Resveratrol provides neuroprotection by inhibiting phosphodiesterases and regulating the cAMP/AMPK/SIRT1 pathway after stroke in rats. Brain Res. Bull. 121, 255–262. doi: 10.1016/j.brainresbull.2016.02.011
Wang, G., Chen, Z., Song, Y., Wu, H., Chen, M., Lai, S., et al. (2022). Xueshuantong injection alleviates cerebral microcirculation disorder in middle cerebral artery occlusion/reperfusion rats by suppressing inflammation via JNK mediated JAK2/STAT3 and NF-κB signaling pathways. J. Ethnopharmacol. 298:115592.
Wang, H., Chen, S., Zhang, Y., Xu, H., and Sun, H. (2019). Electroacupuncture ameliorates neuronal injury by Pink1/Parkin-mediated mitophagy clearance in cerebral ischemia-reperfusion. Nitric Oxide 91, 23–34. doi: 10.1016/j.niox.2019.07.004
Wang, H., Guo, M., Wei, H., and Chen, Y. (2023). Targeting p53 pathways: Mechanisms, structures, and advances in therapy. Signal. Transduct. Target Ther. 8:92. doi: 10.1038/s41392-023-01347-1
Wang, H., Xu, X., Yin, Y., Yu, S., Ren, H., Xue, Q., et al. (2020). Catalpol protects vascular structure and promotes angiogenesis in cerebral ischemic rats by targeting HIF-1α/VEGF. Phytomedicine 78:153300.
Wang, H., Ye, K., Li, D., Liu, Y., and Wang, D. (2022). DL-3-n-butylphthalide for acute ischemic stroke: An updated systematic review and meta-analysis of randomized controlled trials. Front. Pharmacol. 13:963118. doi: 10.3389/fphar.2022.963118
Wang, L. P., Pan, J., Li, Y., Geng, J., Liu, C., Zhang, L. Y., et al. (2022). Oligodendrocyte precursor cell transplantation promotes angiogenesis and remyelination via Wnt/β-catenin pathway in a mouse model of middle cerebral artery occlusion. J. Cereb. Blood Flow Metab. 42, 757–770.
Wang, P. R., Wang, J. S., Zhang, C., Song, X. F., Tian, N., and Kong, L. Y. (2013). Huang-Lian-Jie-Du-Decotion induced protective autophagy against the injury of cerebral ischemia/reperfusion via MAPK-mTOR signaling pathway. J. Ethnopharmacol. 149, 270–280. doi: 10.1016/j.jep.2013.06.035
Wang, S., Shi, X., Li, H., Pang, P., Pei, L., Shen, H., et al. (2017). DAPK1 signaling pathways in stroke: From mechanisms to therapies. Mol. Neurobiol. 54, 4716–4722.
Wang, S., Yin, J., Ge, M., Dai, Z., Li, Y., Si, J., et al. (2016). Transforming growth-beta 1 contributes to isoflurane postconditioning against cerebral ischemia-reperfusion injury by regulating the c-Jun N-terminal kinase signaling pathway. Biomed. Pharmacother. 78, 280–290. doi: 10.1016/j.biopha.2016.01.030
Wang, W., Li, M., Wang, Y., Li, Q., Deng, G., Wan, J., et al. (2016). GSK-3β inhibitor TWS119 attenuates rtPA-induced hemorrhagic transformation and activates the Wnt/β-catenin signaling pathway after acute ischemic stroke in rats. Mol. Neurobiol. 53, 7028–7036.
Wang, X., Li, X., Xu, Y., Li, R., Yang, Q., Zhao, Y., et al. (2021). Effectiveness of intravenous r-tPA versus UK for acute ischaemic stroke: A nationwide prospective Chinese registry study. Stroke Vasc. Neurol. 6, 603–609. doi: 10.1136/svn-2020-000640
Wang, X., Pei, L., Yan, H., Wang, Z., Wei, N., Wang, S., et al. (2014). Intervention of death-associated protein kinase 1-p53 interaction exerts the therapeutic effects against stroke. Stroke 45, 3089–3091. doi: 10.1161/STROKEAHA.114.006348
Wang, Y., Hong, F., and Yang, S. (2022a). Roles of nitric oxide in brain ischemia and reperfusion. Int. J. Mol. Sci. 23:4243.
Wang, Y., Pan, P., Wang, Z., Zhang, Y., Xie, P., Geng, D., et al. (2017). β-catenin-mediated YAP signaling promotes human glioma growth. J. Exp. Clin. Cancer Res. 36:136.
Wang, Y., Zheng, L., Shang, W., Yang, Z., Li, T., Liu, F., et al. (2022b). Wnt/beta-catenin signaling confers ferroptosis resistance by targeting GPX4 in gastric cancer. Cell Death Differ. 29, 2190–2202. doi: 10.1038/s41418-022-01008-w
Wardlaw, J. M., Murray, V., Berge, E., and Del, Z. (2014). Thrombolysis for acute ischaemic stroke. Cochrane Database Syst. Rev. 2014:Cd000213. doi: 10.3389/fmedt.2022.946367
Wei, R., Song, L., Miao, Z., Liu, K., Han, G., Zhang, H., et al. (2022). Hydroxysafflor yellow a exerts neuroprotective effects via HIF-1α/BNIP3 pathway to activate neuronal autophagy after OGD/R. Cells 11:3726.
Wei, Z., Chigurupati, S., Arumugam, T. V., Jo, D. G., Li, H., and Chan, S. L. (2011). Notch activation enhances the microglia-mediated inflammatory response associated with focal cerebral ischemia. Stroke 42, 2589–2594. doi: 10.1161/STROKEAHA.111.614834
Weiland, A., Wang, Y., Wu, W., Lan, X., Han, X., Li, Q., et al. (2019). Ferroptosis and its role in diverse brain diseases. Mol. Neurobiol. 56, 4880–4893.
Wharton, K. A., Johansen, K. M., Xu, T., and Artavanis-Tsakonas, S. (1985). Nucleotide sequence from the neurogenic locus notch implies a gene product that shares homology with proteins containing EGF-like repeats. Cell 43, 567–581. doi: 10.1016/0092-8674(85)90229-6
Whitman, M., Downes, C. P., Keeler, M., Keller, T., and Cantley, L. (1988). Type I phosphatidylinositol kinase makes a novel inositol phospholipid, phosphatidylinositol-3-phosphate. Nature 332, 644–646. doi: 10.1038/332644a0
Willmot, M. R., and Bath, P. M. (2003). The potential of nitric oxide therapeutics in stroke. Expert Opin. Investig. Drugs 12, 455–470.
Wu, F., Zhang, Y., Sun, B., Mcmahon, A. P., and Wang, Y. (2017). Hedgehog signaling: From basic biology to cancer therapy. Cell Chem. Biol. 24, 252–280.
Wu, L., Wang, H. M., Li, J. L., Feng, H. X., Zhao, W. M., and Zhang, H. Y. (2017). Dual anti-ischemic effects of rosmarinic acid n-butyl ester via alleviation of DAPK-p53-mediated neuronal damage and microglial inflammation. Acta Pharmacol. Sin. 38, 459–468. doi: 10.1038/aps.2016.156
Wu, S., Huang, J., Dong, J., and Pan, D. (2003). hippo encodes a Ste-20 family protein kinase that restricts cell proliferation and promotes apoptosis in conjunction with salvador and warts. Cell 114, 445–456. doi: 10.1016/s0092-8674(03)00549-x
Wu, X., Li, X., Liu, Y., Yuan, N., Li, C., Kang, Z., et al. (2018). Hydrogen exerts neuroprotective effects on OGD/R damaged neurons in rat hippocampal by protecting mitochondrial function via regulating mitophagy mediated by PINK1/Parkin signaling pathway. Brain Res. 1698, 89–98. doi: 10.1016/j.brainres.2018.06.028
Xian, M., Cai, J., Zheng, K., Liu, Q., Liu, Y., Lin, H., et al. (2021). Aloe-emodin prevents nerve injury and neuroinflammation caused by ischemic stroke via the PI3K/AKT/mTOR and NF-κB pathway. Food Funct. 12, 8056–8067.
Xiao, G., Lyu, M., Li, Z., Cao, L., Liu, X., Wang, Y., et al. (2021). Restoration of early deficiency of axonal guidance signaling by guanxinning injection as a novel therapeutic option for acute ischemic stroke. Pharmacol. Res. 165:105460. doi: 10.1016/j.phrs.2021.105460
Xie, G., Liang, Y., Gao, W., Wu, L., Zhang, Y., Ye, Z., et al. (2023). Artesunate alleviates intracerebral haemorrhage secondary injury by inducing ferroptosis in M1-polarized microglia and suppressing inflammation through AMPK/mTORC1/GPX4 pathway. Basic Clin. Pharmacol. Toxicol. 132, 369–383. doi: 10.1111/bcpt.13848
Xin, N., Yang, F. J., Li, Y., Li, Y. J., Dai, R. J., Meng, W. W., et al. (2013). Dragon’s blood dropping pills have protective effects on focal cerebral ischemia rats model. Phytomedicine 21, 68–74. doi: 10.1016/j.phymed.2013.08.007
Xu, D., Hou, K., Li, F., Chen, S., Fang, W., and Li, Y. (2019). XQ-1H alleviates cerebral ischemia in mice through inhibition of apoptosis and promotion of neurogenesis in a Wnt/β-catenin signaling dependent way. Life Sci. 235:116844.
Xu, F., Na, L., Li, Y., and Chen, L. (2020). Roles of the PI3K/AKT/mTOR signalling pathways in neurodegenerative diseases and tumours. Cell Biosci. 10:54.
Xu, T., Wang, W., Zhang, S., Stewart, R. A., and Yu, W. (1995). Identifying tumor suppressors in genetic mosaics: The Drosophila lats gene encodes a putative protein kinase. Development 121, 1053–1063.
Xu, Y., Zhang, G., Kang, Z., Xu, Y., Jiang, W., and Zhang, S. (2016). Cornin increases angiogenesis and improves functional recovery after stroke via the Ang1/Tie2 axis and the Wnt/β-catenin pathway. Arch. Pharm. Res. 39, 133–142.
Xu, Z., and Ford, B. D. (2005). Upregulation of erbB receptors in rat brain after middle cerebral arterial occlusion. Neurosci. Lett. 375, 181–186. doi: 10.1016/j.neulet.2004.11.039
Yagita, Y., Kitagawa, K., Sasaki, T., Terasaki, Y., Todo, K., Omura-Matsuoka, E., et al. (2007). Rho-kinase activation in endothelial cells contributes to expansion of infarction after focal cerebral ischemia. J. Neurosci. Res. 85, 2460–2469. doi: 10.1002/jnr.21375
Yamaguchi, A., Taniguchi, M., Hori, O., Ogawa, S., Tojo, N., Matsuoka, N., et al. (2002). Peg3/Pw1 is involved in p53-mediated cell death pathway in brain ischemia/hypoxia. J. Biol. Chem. 277, 623–629. doi: 10.1074/jbc.M107435200
Yan, H. F., Zou, T., Tuo, Q. Z., Xu, S., Li, H., Belaidi, A. A., et al. (2021). Ferroptosis: Mechanisms and links with diseases. Signal. Transduct. Target Ther. 6:49.
Yang, A., Wu, H. M., Tang, J. L., Xu, L., Yang, M., and Liu, G. J. (2016). Acupuncture for stroke rehabilitation. Cochrane Database Syst. Rev. 2016:Cd004131.
Yang, B., Li, Y., Ma, Y., Zhang, X., Yang, L., Shen, X., et al. (2021). Selenium attenuates ischemia/reperfusion injury-induced damage to the blood-brain barrier in hyperglycemia through PI3K/AKT/mTOR pathway-mediated autophagy inhibition. Int. J. Mol. Med. 48:178. doi: 10.3892/ijmm.2021.5011
Yang, L., Tao, L. Y., and Chen, X. P. (2007). Roles of NF-kappaB in central nervous system damage and repair. Neurosci. Bull. 23, 307–313.
Yang, S., Wang, H., Yang, Y., Wang, R., Wang, Y., Wu, C., et al. (2019). Baicalein administered in the subacute phase ameliorates ischemia-reperfusion-induced brain injury by reducing neuroinflammation and neuronal damage. Biomed. Pharmacother. 117:109102. doi: 10.1016/j.biopha.2019.109102
Yang, W. H., Ding, C. C., Sun, T., Rupprecht, G., Lin, C. C., Hsu, D., et al. (2019). The Hippo pathway effector TAZ regulates ferroptosis in renal cell carcinoma. Cell Rep. 28, 2501–2508.e4.
Yang, W. S., and Stockwell, B. R. (2016). Ferroptosis: Death by lipid peroxidation. Trends Cell Biol. 26, 165–176.
Yang, W. S., Sriramaratnam, R., Welsch, M. E., Shimada, K., Skouta, R., Viswanathan, V. S., et al. (2014). Regulation of ferroptotic cancer cell death by GPX4. Cell 156, 317–331.
Yang, Y., He, B., Zhang, X., Yang, R., Xia, X., Chen, L., et al. (2022). Geraniin protects against cerebral ischemia/reperfusion injury by suppressing oxidative stress and neuronal apoptosis via regulation of the Nrf2/HO-1 pathway. Oxid. Med. Cell Longev. 2022:2152746. doi: 10.1155/2022/2152746
Yeh, S. H., Ou, L. C., Gean, P. W., Hung, J. J., and Chang, W. C. (2011). Selective inhibition of early–but not late–expressed HIF-1α is neuroprotective in rats after focal ischemic brain damage. Brain Pathol. 21, 249–262.
Yimlamai, D., Christodoulou, C., Galli, G. G., Yanger, K., Pepe-Mooney, B., Gurung, B., et al. (2014). Hippo pathway activity influences liver cell fate. Cell 157, 1324–1338. doi: 10.1016/j.cell.2014.03.060
Yochem, J., Weston, K., and Greenwald, I. (1988). The Caenorhabditis elegans lin-12 gene encodes a transmembrane protein with overall similarity to Drosophila Notch. Nature 335, 547–550. doi: 10.1038/335547a0
Yonish-Rouach, E., Resnitzky, D., Lotem, J., Sachs, L., Kimchi, A., and Oren, M. (1991). Wild-type p53 induces apoptosis of myeloid leukaemic cells that is inhibited by interleukin-6. Nature 352, 345–347.
Yu, F. X., Zhao, B., Panupinthu, N., Jewell, J. L., Lian, I., Wang, L. H., et al. (2012). Regulation of the Hippo-YAP pathway by G-protein-coupled receptor signaling. Cell 150, 780–791. doi: 10.1016/j.cell.2012.06.037
Yu, J., Wang, W. N., Matei, N., Li, X., Pang, J. W., Mo, J., et al. (2020). Ezetimibe attenuates oxidative stress and neuroinflammation via the AMPK/Nrf2/TXNIP pathway after MCAO in rats. Oxid. Med. Cell Longev. 2020:4717258. doi: 10.1155/2020/4717258
Yu, L., Liu, Z., He, W., Chen, H., Lai, Z., Duan, Y., et al. (2020). Hydroxysafflor yellow a confers neuroprotection from focal cerebral ischemia by modulating the crosstalk between JAK2/STAT3 and SOCS3 signaling pathways. Cell Mol. Neurobiol. 40, 1271–1281. doi: 10.1007/s10571-020-00812-7
Yu, P., Wang, L., Tang, F., Guo, S., Liao, H., Fan, C., et al. (2021). Resveratrol-mediated neurorestoration after cerebral ischemic injury - Sonic Hedgehog signaling pathway. Life Sci. 280:119715. doi: 10.1016/j.lfs.2021.119715
Yu, P., Wang, L., Tang, F., Zeng, L., Zhou, L., Song, X., et al. (2017). Resveratrol pretreatment decreases ischemic injury and improves neurological function via sonic hedgehog signaling after stroke in rats. Mol. Neurobiol. 54, 212–226. doi: 10.1007/s12035-015-9639-7
Yu, Y., Li, J., Zhou, H., Xiong, Y., Wen, Y., and Li, H. (2018). Functional importance of the TGF-β1/Smad3 signaling pathway in oxygen-glucose-deprived (OGD) microglia and rats with cerebral ischemia. Int. J. Biol. Macromol. 116, 537–544.
Yuan, Y., Zhai, Y., Chen, J., Xu, X., and Wang, H. (2021). Kaempferol ameliorates oxygen-glucose deprivation/reoxygenation-induced neuronal ferroptosis by activating Nrf2/SLC7A11/GPX4 axis. Biomolecules 11:923. doi: 10.3390/biom11070923
Yuan, Y., Zheng, Y., Zhang, X., Chen, Y., Wu, X., Wu, J., et al. (2017). BNIP3L/NIX-mediated mitophagy protects against ischemic brain injury independent of PARK2. Autophagy 13, 1754–1766. doi: 10.1080/15548627.2017.1357792
Zacharek, A., Chen, J., Cui, X., Yang, Y., and Chopp, M. (2009). Simvastatin increases notch signaling activity and promotes arteriogenesis after stroke. Stroke 40, 254–260. doi: 10.1161/STROKEAHA.108.524116
Zanconato, F., Cordenonsi, M., and Piccolo, S. (2016). YAP/TAZ at the roots of cancer. Cancer Cell 29, 783–803.
Zanin-Zhorov, A., Weiss, J. M., Nyuydzefe, M. S., Chen, W., Scher, J. U., Mo, R., et al. (2014). Selective oral ROCK2 inhibitor down-regulates IL-21 and IL-17 secretion in human T cells via STAT3-dependent mechanism. Proc. Natl. Acad. Sci. U. S. A. 111, 16814–16819. doi: 10.1073/pnas.1414189111
Zeng, J., Zheng, S., Chen, Y., Qu, Y., Xie, J., Hong, E., et al. (2021). Puerarin attenuates intracerebral hemorrhage-induced early brain injury possibly by PI3K/Akt signal activation-mediated suppression of NF-κB pathway. J. Cell Mol. Med. 25, 7809–7824.
Zerlin, M., Julius, M. A., and Kitajewski, J. (2008). Wnt/Frizzled signaling in angiogenesis. Angiogenesis 11, 63–69.
Zhan, S., Liang, J., Lin, H., Cai, J., Yang, X., Wu, H., et al. (2022). SATB1/SLC7A11/HO-1 axis ameliorates ferroptosis in neuron cells after ischemic stroke by danhong injection. Mol. Neurobiol. 60, 413–427. doi: 10.1007/s12035-022-03075-z
Zhang, C., Tao, W., Liu, M., and Wang, D. (2012). Efficacy and safety of human urinary kallidinogenase injection for acute ischemic stroke: A systematic review. J. Evid. Based Med. 5, 31–39. doi: 10.1111/j.1756-5391.2012.01167.x
Zhang, D. D. (2006). Mechanistic studies of the Nrf2-Keap1 signaling pathway. Drug Metab. Rev. 38, 769–789.
Zhang, W., Song, J. K., Yan, R., Li, L., Xiao, Z. Y., Zhou, W. X., et al. (2018). Diterpene ginkgolides protect against cerebral ischemia/reperfusion damage in rats by activating Nrf2 and CREB through PI3K/Akt signaling. Acta Pharmacol. Sin. 39, 1259–1272. doi: 10.1038/aps.2017.149
Zhang, W., Song, J., Li, W., Kong, D., Liang, Y., Zhao, X., et al. (2020). Salvianolic acid D alleviates cerebral ischemia-reperfusion injury by suppressing the cytoplasmic translocation and release of HMGB1-triggered NF-κB activation to inhibit inflammatory response. Med. Inflamm. 2020:9049614.
Zhang, Y., and Miao, J. M. (2018). Ginkgolide K promotes astrocyte proliferation and migration after oxygen-glucose deprivation via inducing protective autophagy through the AMPK/mTOR/ULK1 signaling pathway. Eur. J. Pharmacol. 832, 96–103. doi: 10.1016/j.ejphar.2018.05.029
Zhang, Y., Janssens, S. P., Wingler, K., Schmidt, H. H., and Moens, A. L. (2011). Modulating endothelial nitric oxide synthase: A new cardiovascular therapeutic strategy. Am. J. Physiol. Heart Circ. Physiol. 301, H634–H646.
Zhang, Y., Liu, D., Hu, H., Zhang, P., Xie, R., and Cui, W. (2019). HIF-1α/BNIP3 signaling pathway-induced-autophagy plays protective role during myocardial ischemia-reperfusion injury. Biomed. Pharmacother. 120:109464.
Zhang, Y., Lu, X., Tai, B., Li, W., and Li, T. (2021). Ferroptosis and Its Multifaceted Roles in Cerebral Stroke. Front. Cell Neurosci. 15:615372. doi: 10.3389/fncel.2021.615372
Zhang, Z. G., Zhang, L., Jiang, Q., Zhang, R., Davies, K., Powers, C., et al. (2000). VEGF enhances angiogenesis and promotes blood-brain barrier leakage in the ischemic brain. J. Clin. Invest. 106, 829–838.
Zhang, Z., Yang, X., Zhang, S., Ma, X., and Kong, J. (2007). BNIP3 upregulation and EndoG translocation in delayed neuronal death in stroke and in hypoxia. Stroke 38, 1606–1613. doi: 10.1161/STROKEAHA.106.475129
Zhao, S., Yin, J., Zhou, L., Yan, F., He, Q., Huang, L., et al. (2016). Hippo/MST1 signaling mediates microglial activation following acute cerebral ischemia-reperfusion injury. Brain Behav. Immun. 55, 236–248. doi: 10.1016/j.bbi.2015.12.016
Zhao, X., Sun, G., Zhang, J., Ting, S. M., Gonzales, N., and Aronowski, J. (2015). Dimethyl Fumarate protects brain from damage produced by intracerebral hemorrhage by mechanism involving Nrf2. Stroke 46, 1923–1928. doi: 10.1161/STROKEAHA.115.009398
Zhao, Y., Chen, F., Chen, S., Liu, X., Cui, M., and Dong, Q. (2013). The Parkinson’s disease-associated gene PINK1 protects neurons from ischemic damage by decreasing mitochondrial translocation of the fission promoter Drp1. J. Neurochem. 127, 711–722. doi: 10.1111/jnc.12340
Zhao, Y., Qian, Y., Sun, Z., Shen, X., Cai, Y., Li, L., et al. (2021). Role of PI3K in the progression and regression of atherosclerosis. Front. Pharmacol. 12:632378. doi: 10.3389/fphar.2021.632378
Zheng, Y., and Pan, D. (2019). The hippo signaling pathway in development and disease. Dev. Cell 50, 264–282.
Zheng, Y., Li, R., Zhou, Y., Zhang, S., and Fan, X. (2022). Investigation on the potential targets of Astragaloside IV against intracerebral hemorrhage based on network pharmacology and experimental validation. Bioorg. Chem. 127:105975. doi: 10.1016/j.bioorg.2022.105975
Zhong, L. L., Zheng, Y., Lau, A. Y., Wong, N., Yao, L., Wu, X., et al. (2022). Would integrated Western and traditional Chinese medicine have more benefits for stroke rehabilitation? A systematic review and meta-analysis. Stroke Vasc. Neurol. 7, 77–85. doi: 10.1136/svn-2020-000781
Zhong, W. J., Yang, X. S., Zhou, H., Xie, B. R., Liu, W. W., and Li, Y. (2022). Role of mitophagy in the pathogenesis of stroke: From mechanism to therapy. Oxid. Med. Cell Longev. 2022:6232902.
Zhou, G., Myers, R., Li, Y., Chen, Y., Shen, X., Fenyk-Melody, J., et al. (2001). Role of AMP-activated protein kinase in mechanism of metformin action. J. Clin. Invest. 108, 1167–1174.
Zhou, K., Chen, J., Wu, J., Wu, Q., Jia, C., Xu, Y. X. Z., et al. (2019). Atractylenolide III ameliorates cerebral ischemic injury and neuroinflammation associated with inhibiting JAK2/STAT3/Drp1-dependent mitochondrial fission in microglia. Phytomedicine 59:152922. doi: 10.1016/j.phymed.2019.152922
Zhou, Y., Liao, J., Mei, Z., Liu, X., and Ge, J. (2021). Insight into crosstalk between ferroptosis and necroptosis: Novel therapeutics in ischemic stroke. Oxid. Med. Cell Longev. 2021:9991001. doi: 10.1155/2021/9991001
Zhou, Z. Q., Li, Y. L., Ao, Z. B., Wen, Z. L., Chen, Q. W., Huang, Z. G., et al. (2017). Baicalin protects neonatal rat brains against hypoxic-ischemic injury by upregulating glutamate transporter 1 via the phosphoinositide 3-kinase/protein kinase B signaling pathway. Neural Regen. Res. 12, 1625–1631. doi: 10.4103/1673-5374.217335
Zhou, Z. X., Cui, Q., Zhang, Y. M., Yang, J. X., Xiang, W. J., Tian, N., et al. (2023). Withaferin A inhibits ferroptosis and protects against intracerebral hemorrhage. Neural Regen. Res. 18, 1308–1315. doi: 10.4103/1673-5374.355822
Zhu, H., Jian, Z., Zhong, Y., Ye, Y., Zhang, Y., Hu, X., et al. (2021). Janus kinase inhibition ameliorates ischemic stroke injury and neuroinflammation through reducing NLRP3 inflammasome activation via JAK2/STAT3 pathway inhibition. Front. Immunol. 12:714943. doi: 10.3389/fimmu.2021.714943
Zhu, J., Cao, D., Guo, C., Liu, M., Tao, Y., Zhou, J., et al. (2019). Berberine facilitates angiogenesis against ischemic stroke through modulating microglial polarization via AMPK signaling. Cell Mol. Neurobiol. 39, 751–768. doi: 10.1007/s10571-019-00675-7
Zhu, T., Wang, L., Wang, L. P., and Wan, Q. (2022). Therapeutic targets of neuroprotection and neurorestoration in ischemic stroke: Applications for natural compounds from medicinal herbs. Biomed. Pharmacother. 148:112719. doi: 10.1016/j.biopha.2022.112719
Zille, M., Karuppagounder, S. S., Chen, Y., Gough, P. J., Bertin, J., Finger, J., et al. (2017). Neuronal death after hemorrhagic stroke in vitro and in vivo shares features of ferroptosis and necroptosis. Stroke 48, 1033–1043. doi: 10.1161/STROKEAHA.116.015609
Keywords: stroke, crosstalk, network disease, traditional Chinese medicine, pathway
Citation: Chen B and Jin W (2023) A comprehensive review of stroke-related signaling pathways and treatment in western medicine and traditional Chinese medicine. Front. Neurosci. 17:1200061. doi: 10.3389/fnins.2023.1200061
Received: 04 April 2023; Accepted: 19 May 2023;
Published: 07 June 2023.
Edited by:
Marcia Inês Goettert, University of Tübingen, GermanyCopyright © 2023 Chen and Jin. This is an open-access article distributed under the terms of the Creative Commons Attribution License (CC BY). The use, distribution or reproduction in other forums is permitted, provided the original author(s) and the copyright owner(s) are credited and that the original publication in this journal is cited, in accordance with accepted academic practice. No use, distribution or reproduction is permitted which does not comply with these terms.
*Correspondence: Weifeng Jin, amluX3dlaWZlbmdAMTI2LmNvbQ==