- 1Department of Cell and Developmental Biology, University of Illinois Urbana-Champaign, Urbana, IL, United States
- 2Neuroscience Program, University of Illinois Urbana-Champaign, Urbana, IL, United States
- 3Beckman Institute for Advanced Science and Technology, University of Illinois Urbana-Champaign, Urbana, IL, United States
- 4Department of Molecular and Integrative Physiology, University of Illinois Urbana-Champaign, Urbana, IL, United States
- 5Carle-Illinois College of Medicine, University of Illinois Urbana-Champaign, Urbana, IL, United States
The neurovascular system forms the interface between the tissue of the central nervous system (CNS) and circulating blood. It plays a critical role in regulating movement of ions, small molecules, and cellular regulators into and out of brain tissue and in sustaining brain health. The neurovascular unit (NVU), the cells that form the structural and functional link between cells of the brain and the vasculature, maintains the blood–brain interface (BBI), controls cerebral blood flow, and surveils for injury. The neurovascular system is dynamic; it undergoes tight regulation of biochemical and cellular interactions to balance and support brain function. Development of an intrinsic circadian clock enables the NVU to anticipate rhythmic changes in brain activity and body physiology that occur over the day-night cycle. The development of circadian neurovascular function involves multiple cell types. We address the functional aspects of the circadian clock in the components of the NVU and their effects in regulating neurovascular physiology, including BBI permeability, cerebral blood flow, and inflammation. Disrupting the circadian clock impairs a number of physiological processes associated with the NVU, many of which are correlated with an increased risk of dysfunction and disease. Consequently, understanding the cell biology and physiology of the NVU is critical to diminishing consequences of impaired neurovascular function, including cerebral bleeding and neurodegeneration.
1. Introduction
The neurovasculature regulates the flow of blood through the arteries, veins, and capillaries within the brain. It is composed of cells of the neurovascular unit (NVU), which include neuroendothelial cells, mural cells (smooth muscle and pericytes), astrocytes, and microglia as well as extracellular matrix components within the basement membrane. Arteries arising from the subarachnoid space vascularize the brain. They form NVUs comprised of smooth muscle cells, endothelial cells, pia mater, the perivascular space, and astrocytic end feet. As these arterial vessels penetrate deeper into the brain, they lose smooth muscle cells and pia mater. Pericytes, which are contractile, assume positions between the endothelial cells and astrocytic endfeet (Figure 1).
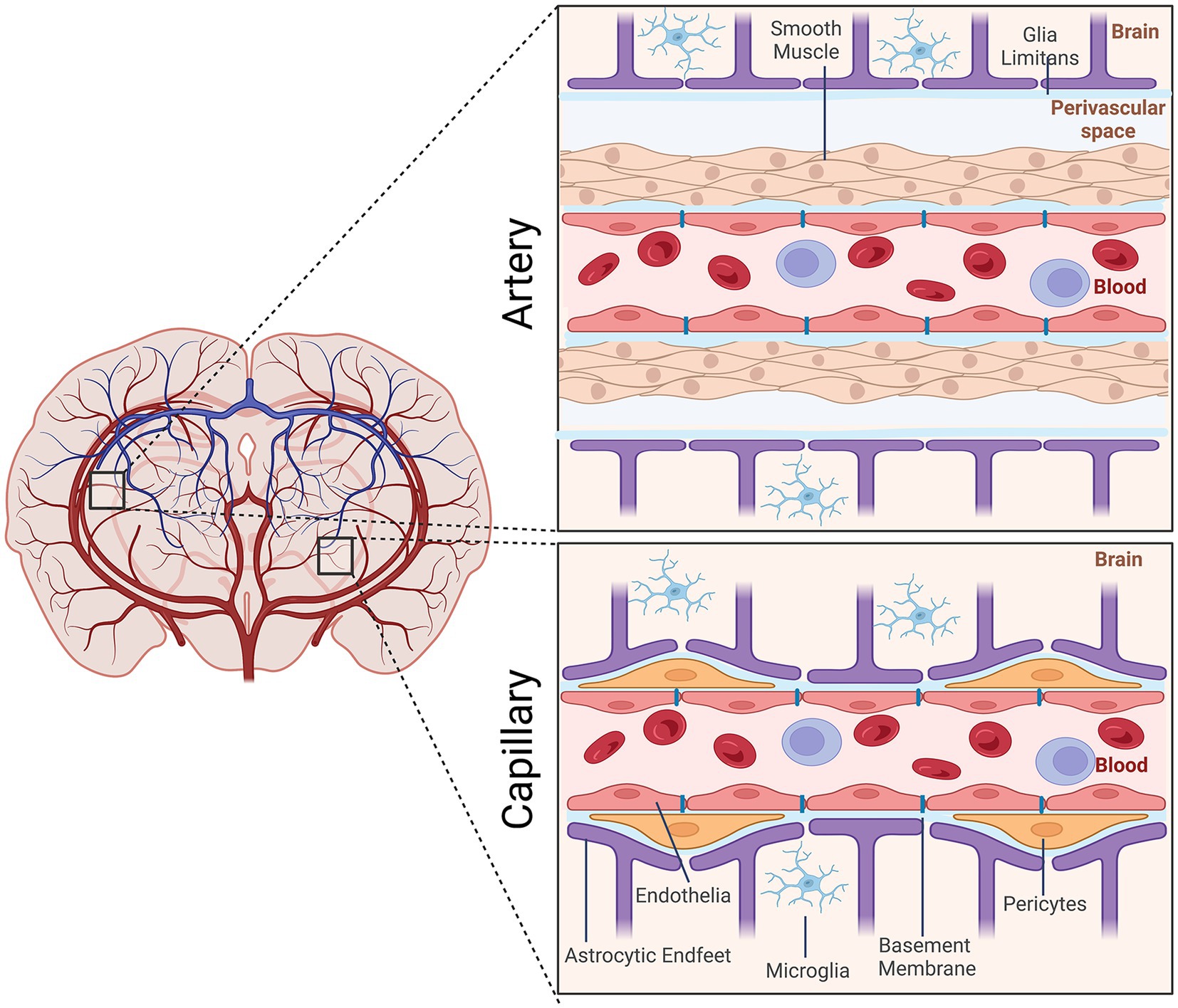
Figure 1. Schematic representation of the cellular elements of the neurovascular unit (NVU). Elements of the NVU include neuroendothelial cells, mural cells (vascular smooth muscle cells, pericytes), microglia, astrocytic endfeet. The cellular composition differs along the vascular tree. At the level of the artery/arteriole (top), the NVU is composed of neuroendothelial cells making up the inner layer of the vessel wall covered by a thin extracellular basement membrane, ringed by vascular smooth muscle cells, and ensheathed by a glial limitans. The perivascular space containing the cerebrospinal fluid is between pia and the glia limitans formed by astrocytic endfeet. At the capillary level (bottom), the NVU is composed of neuroendothelial cells that share a common basement membrane with pericytes. Pericytes stretch their processes along and around capillaries. Pericytes and endothelial cells are covered by astrocyte endfeet. Created by Biorender.com.
The cells of the NVU to undergo dynamic daily and environmental changes, making regulatory adjustments to maintain homeostasis. Interactions of the NVU with neuronal networks are responsible for the regulating cerebral blood flow (CBF), maintaining the integrity of the blood–brain interface (BBI), and immune surveilling-, which recognizes harmful pathogens, responds to injury, recruits resident microglia to clear cellular debris, and releases neuroprotective factors (Kaplan et al., 2020).
1.1. Blood–brain interface (BBI)
The cerebrovasculature continuously provides resources supporting the brain’s high metabolic rate, the necessary rapid, on-demand delivery of oxygen and energy supporting neuronal activity, as well as efficient clearance of waste products. The brain’s high metabolic rate requires the continuous supply of nutrients and oxygen by the blood. The blood–brain interface (BBI), a multicellular structure separating the CNS from systemic circulation, regulates this interaction. Tight junctions between endothelial cells of the NVU form a physical barrier, restricting permeability. The surrounding pericytes and astrocytic endfeet are encased in the extracellular matrix-containing basement membrane, enhancing this structural barrier (Abbott et al., 2010; Keaney and Campbell, 2015; Liebner et al., 2018). Vesicular transport across these endothelial cells is very low compared to vascular endothelial cells of other organs, distinguishing them as neuroendothelium (Brightman and Reese, 1969). The BBI restricts the exchange of material between the brain and the blood, except for small molecules and gases such as oxygen and carbon dioxide. Larger molecules can cross the BBI only through transporters and endocytic vesicles (pinocytosis). Loss of BBI integrity results not only in reduced oxygen and nutrient flux into the brain, but also diminished clearance of neurotoxic substances. Other components, such as astrocytic endfeet and pericytes, also express some transporters and receptors that contribute to the BBI transport system.
Neuroendothelial cells are the central regulatory components of the BBI. Characterized by few transporters, low level of paracellular diffusion and pinocytotic activity, neuroendothelial cells are joined laterally by tight junctions. Pericytes wrap around the vessels and are embedded in extra cellular matrix, the basement membrane (Abbott et al., 2010). The restrictive properties of the BBI are determined primarily by tight-junction proteins, which form a physical barrier between adjacent neuroendothelial cells (Kniesel and Wolburg, 2000). The BBI restricts entry of cytokines and antibodies, which can impair neurotransmission, into the brain (Abbott et al., 2006), as well as participates in the clearance of cellular metabolites from the brain to the blood (Winkler et al., 2011).
1.2. Cerebral blood flow
Cerebral blood flow (CBF) in the human brain is accomplished through a network of interconnected blood vessels over 400-miles long (Zlokovic, 2011; Kisler et al., 2017). Irreversible brain damage can occur within minutes if proper CBF is compromised (Moskowitz et al., 2010). Mural cells, vascular smooth muscle cells (vSMC), and pericytes are involved in regulating cerebral blood flow (Peterson et al., 2011; Hall et al., 2014; Hill et al., 2015; Attwell et al., 2016). Active contraction and relaxation of vSMCs around the larger arterioles controls flow by altering the vascular diameter (Hill et al., 2015). Pericytes are spatially isolated contractile cells in the microvasculature that are responsible for regulating the cerebral blood flow within capillaries (Attwell et al., 2016; Cai et al., 2017; Mughal et al., 2023; Figure 2). The capillary dilation created by pericyte relaxation is regulated by the blood pressure from upstream arterioles. Pericytes alter capillary diameter by actively responding to neuronal activity (Wu et al., 2003; Peppiatt et al., 2006). The dilation response in capillaries is rapid, completing prior to arteriole dilation (Hall et al., 2014).
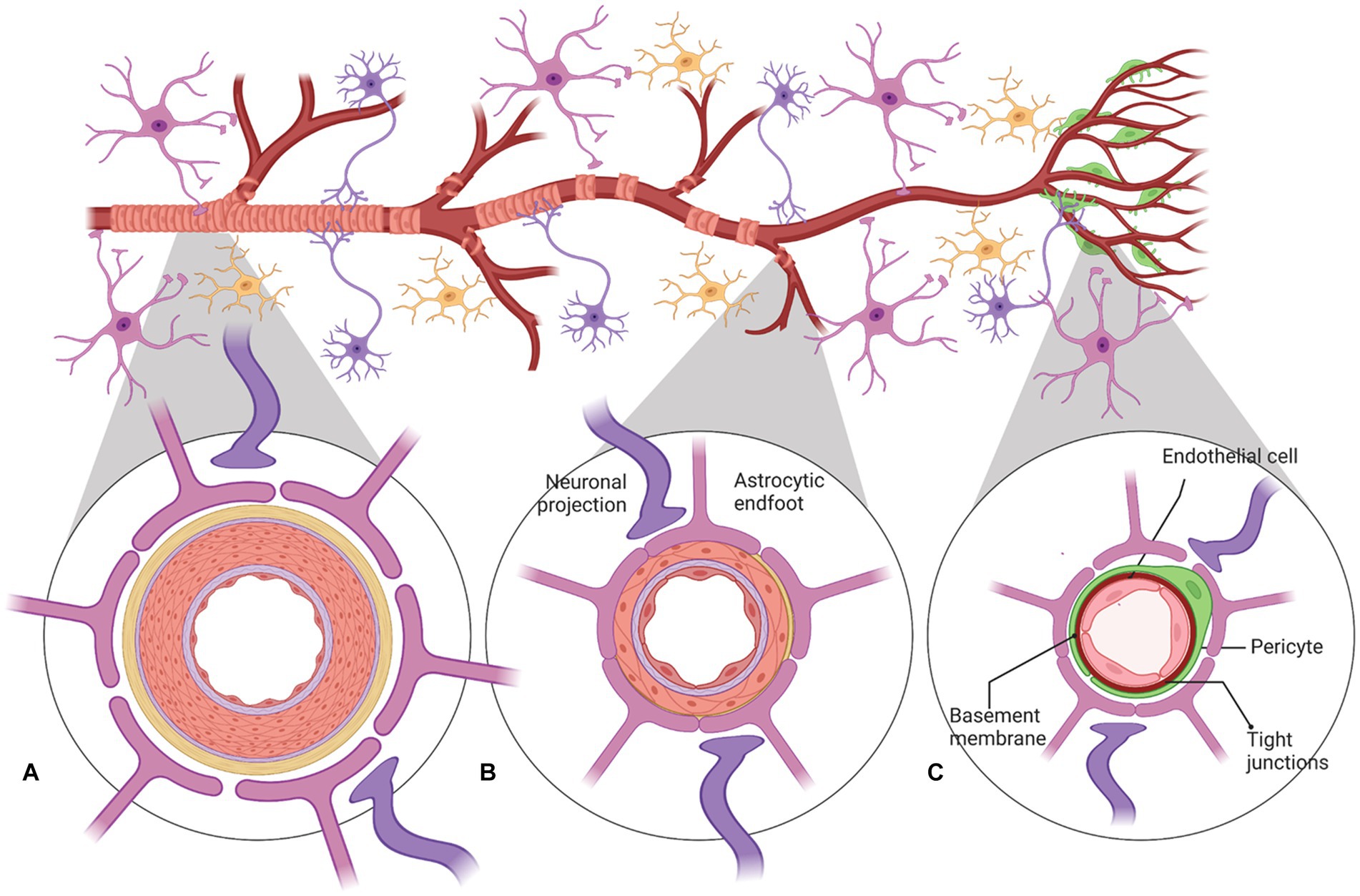
Figure 2. A schematic representation of neurovascular units with cellular elements that regulate cerebral blood flow along the vascular tree. The various types of cells that form the neurovascular unit (NVU) (neurons, astrocytes, mural cells – vascular smooth muscle cells (VSMCs) and pericytes, and neuroendothelium) regulate cerebral blood flow throughout the vascular tree. The cellular composition of the NVU differs along the vascular tree, but the principal cellular components all remain represented, as illustrated here. (A) At the level of penetrating arteries, the NVU is composed of neuroendothelial cells, making up the inner layer of the vessel wall, covered by a thin extracellular basement membrane, ringed by one to three layers of VSMCs, and ensheathed by pia. The Virchow-Robin space containing the cerebrospinal fluid is between the pia and glia limitans formed by astrocytic endfeet. Both VSMCs and astrocytes are innervated by local neurons. (B) Arterioles differ in that (i) there is only one layer of VSMCs, (ii) astrocyte coverage and innervation of the vessel wall and endothelial inner layer are continuous with penetrating arteries and brain capillaries, above and below the arteriole level, respectively. Precapillary arterioles may also contain transitional pericytes, a cell type between pericyte and VSMCs. (C) At the capillary level, the NVU is composed of endothelial cells that share a common basement membrane with pericytes. Pericytes stretch their processes along and around capillaries and make direct, interdigitated or “peg-socket”-like contacts with neuroendothelial cells. Pericytes and neuroendothelial cells are covered by astrocyte endfeet. Both astrocytes and pericytes are innervated by local neurons similar to astrocytes and VSMCs in the upper segments of the vascular tree. Created by Biorender.com.
Regulation of CBF can be grouped into three broad mechanisms: (1) Autoregulation, which maintains stable blood flow despite fluctuations in systemic blood pressure; (2) Vasomotor reactivity, which is in response to modification of the arterial pCO2/pH of the brain tissue reporting the need for oxygen; and (3) Neurovascular coupling, which is in response to local changes in neuronal and metabolic activity (Peterson et al., 2011; Silverman and Petersen, 2022). Autoregulation is largely controlled by changes in vasoconstriction and vasodilation in the smooth muscle of arterioles (Attwell et al., 2016; Hosford and Gourine, 2019; Hoiland et al., 2020; Duffin et al., 2021). This may be mediated by the release of vasoactive substances, myogenic regulation adapting vascular tone, and neurogenic regulation due to sympathetic innervation of vascular smooth muscle cells (Silverman and Petersen, 2022). Vasomotor reactivity reflects changes due to arterial CO2 pressure and coupled pH shifts of the brain microenvironment. Small arterioles are extremely sensitive, vasodilating with elevated arterial CO2 concentrations (Yoshihara et al., 1995). It has been suggested that this vasomotor response is regulated by the proton concentration in smooth muscle cells of cerebral vessels through the pH sensitivity of carbonic anhydrase activity (Budohoski et al., 2013). Neurovascular Coupling responses to elevated neuronal activity and metabolism lead to an increase in local blood flow providing the brain with enhanced blood flow for a given demand (functional hyperemia). Chemical signals secreted from cells of the NVU, which can cause vasodilation or vasoconstriction. The neuroendothelial cells produce several vasoactive factors, including nitric oxide (NO), endothelium-dependent hyperpolarization factor, eicosanoids, and endothelins; their release is regulated by cerebral blood flow (Peterson et al., 2011). Additionally, astrocytic end-feet that directly border the vessels also play key roles in regulating CBF (Peterson et al., 2011). Increased neuronal activity is can initiate the release of synaptic glutamate and thereby activate two different signaling pathways: (1) a signaling pathway that includes the activation of Ca2+-dependent enzymes, such as neuronal nitric oxide synthase (nNOS) and cyclooxygenase-2 (COX-2), can produce the vasodilators NO and prostanoids, respectively, and (2) an astrocytic-dependent signaling pathway that includes Ca2+-dependent activation of phospholipase A2 and ultimately the production of vasodilatory metabolites including epoxyeicosatrienoic acids (EETs) (Attwell et al., 2010; Stackhouse and Mishra, 2021) (Shi et al., 2008). Additionally, K+ and H+ are produced during synaptic transmission; elevation of these ions stimulates vasodilation (Paulson and Newman, 1987; Nielsen and Lauritzen, 2001). However, neural activation can also stimulate vasoconstriction as is the case via BK channels from altered astrocytic endfeet calcium signal in brain slices mimicking subarachnoid hemorrhage (Koide et al., 2012; Pappas et al., 2015).
1.3. Immune surveillance and repair
Despite barriers that prevent immune cells from traversing into the brain, the CNS is continuously monitoring for damage and agents that would disrupt normal brain function. Resident microglia and immune cells within the bordering meninges are primarily responsible for this surveillance (Ousman and Kubes, 2012). Although the border immune cells are outside the scope of this review, they have been thoroughly discussed (Kwon, 2022; Rustenhoven and Kipnis, 2022). The role of microglia in immune surveillance is facilitated by their close proximity to one another with minimal overlap between processes of neighboring microglia (Raivich, 2005; Ousman and Kubes, 2012). Under physiological conditions, microglia are quiescent (M0), yet they are constantly surveying the local environment and communicating with other cell types via their motile processes. This allows the full extracellular space to be sampled by at least one microglial process every a few hours (Nimmerjahn et al., 2005).
Within the NVU, the microglia are activated by minor alterations to the BBI, responding to various stimuli such as vascular injury, stroke, trauma, bacterial infection as well as diseases states such as Alzheimer’s disease and multiple sclerosis (Dudvarski Stankovic et al., 2016; Orihuela et al., 2016; Zhao et al., 2018). The microglial processes are recruited rapidly and form a dense, continuous, stable aggregate at the site of BBI leakage (Davalos et al., 2012; Jolivel et al., 2015; Lou et al., 2016). Microglia can be activated within minutes of tissue damage. They undergo a spectrum of responses when activated, including polarization and differentiation toward pro-inflammatory and neurotoxicity (M1) or anti-inflammatory, healing (M2) forms (Guo et al., 2022). Microglia driven toward the M1 state can produce inflammatory cytokines and chemokines as well as expressing NADPH oxidase, which produces superoxide and reactive oxygen species (ROS). The M2 state of microglia generate anti-inflammatory cytokines, growth factors, as in insulin-like growth factor 1 (IGF-1) and fibroblastic growth factor (FGF), and neurotropic growth factors, including neurotrophic growth factor (NGF) and brain-derived neurotrophic factor (BDNF). Signals shifting between M1 and M2 polarizations can have profound effects on being either neuroprotective or pathogenetic on brain physiology (Ma et al., 2017; Zhao et al., 2018; Guo et al., 2022).
2. Development of the neurovasculature
The developing CNS does not produce vascular progenitor cells; thus, blood vessels must enter into the developing CNS to form the neurovasculature (Bautch and James, 2009). Early studies demonstrate that vascularization of the brain is initiated by vessels outside of the CNS through angiogenic sprouting, namely the peri-neural vascular plexus (Feeney and Watterson, 1946; Strong, 1964). The blood vessels invade and form distinct patterns once they enter the brain (Bautch and James, 2009; Tata et al., 2015). Ultimately, the neurovasculature expands into a vast network and remodels into a vascular tree organized into a hierarchical network of arteries, arterioles, capillary beds, venules, and veins.
Early in embryogenesis, a blood–brain interface is formed to protect neural tissue from variations in blood composition, maintain ionic homeostasis, and exclude toxins. This complex developing structure involves nascent vessels emerging by ingression from endothelial cells. Tight junctions are present between these neuroendothelial cells restricting the passage of low molecular weight molecules very early in development (Ek et al., 2012) The nascent vessels recruit pericytes which are required for barrier formation (Daneman et al., 2010). Notably, this occurs before astrocyte generation (Daneman et al., 2010). Astrocytes extend processes that contact blood vessels after birth, and thus are not required to initially induce the BBI, but likely are involved in maintenance. A number of known signaling molecules between the neural and vascular cells are involved in crosstalk to regulate development of the neurovasculature, all of which allow formation of the intricate architecture through the brain (Tata et al., 2015).
3. Specialized neurovascular structures: circumventricular organs and the cerebral portal system
3.1. Circumventricular organs
The brain has a number of circumventricular organs (CVOs). CVOs possess characteristically highly permeable, fenestrated capillaries, in contrast to the barrier nature of capillaries of the BBI. As the name implies, these specializations form around (Lat., circum) ventricles, including the area postrema (AP), median eminence (ME), neurhypophyis (NP), organum vaculosum of the lamina terminalis (OVLT), pineal gland, and subfornical organ (SFO) (Miyata, 2015). The subcommissural organ (SCO) is often included among the seven CVOs, although it lacks fenestrated capillaries. On the other hand, the choroid plexus, which does have fenestrated capillaries but lacks neurons, may also be grouped with the CVO (Oldfield and McKinley, 2015). Because of the lack of the endothelial barrier, the CVOs permit the direct exchange of chemical information between the brain and circulating blood. Broadly, the CVOs can be separated into two categories, sensory and secretory. The secretory CVOs include the NH, ME, and pineal (as well as the SCO&/or choroid plexus). These neurosecretory nuclei release neurohormones and hormone-releasing factors into the blood via the fenestrated capillaries. The sensory CVOs, which are often described as “windows of the brain,” detect circulating hormones and ions and initiate responses that maintain homeostasis (Gross et al., 1987) and include the SFO, OVLT and AP.
The presence of these leaky, fenestrated capillaries may allow diffusible timing signals to entrain brain regions outside of the suprachiasmatic nucleus (SCN), the site of the central circadian clock (see section 5). To our knowledge, no prior publication reports movement of signals that traverse from the brain directly into the neurovasculature. However, the dense capillaries within the SCN, specifically, within the shell region, suggest a pathway that transports diffusible signals from the SCN into the blood (Yao et al., 2023). There is evidence that diffusible signals from the SCN can interact locally with the CSF. Taub et al. demonstrated that arginine vasopressin (AVP), an established SCN output timing signal that synchronizes 24-h rhythms of physiology and behavior, was found to contribute to AVP levels in the CSF (Taub et al., 2021). AVP levels exhibit 24-h rhythms in CSF, but not in blood (Schwartz et al., 1983). Previous reports have demonstrated a disparate regulation in neuropeptides between plasma and the CSF (Kagerbauer et al., 2013). However, direct access through CVOs to potential timing signals raises an interesting possibility for regulation of circadian oscillations in the neurovasculature.
3.2. Cerebral portal systems
Portal systems carry blood from one capillary bed to another rather than from a capillary bed to a vein. This facilitates the rapid transport of chemical signals from one region to another while maintaining high concentrations of solutes, as the fluid is not transported via the vascular system back through to the heart. The hypophyseal-pituitary portal system is a well-known portal system. Neuropeptides packaged in vesicles within the neuronal cell bodies travel along the axons of the supraoptic and paraventricular neurons that synthesize them to the capillary bed of the median eminence (ME). They are released on demand into the capillary beds of the anterior pituitary (Harris, 1948; Clarke, 2015). Notably, the capillaries and veins of this portal system are leaky due to fenestrations in their vessel walls; thus, the neurohormones pass directly from axon terminals into the blood without encountering a BBI. This portal system allows hypothalamic hormones, such as gonadotropin releasing hormone (GnRH), to stimulate the secretory cells they control.
A new brain portal system has been described that links the SCN and the OVLT (Yao et al., 2021). These portal vessels arise from the rostral SCN and join the capillaries at the base of the OVLT (Yao et al., 2021, 2023). The OVLT participates in osmotic regulation and is involved in the release of AVP (McKinley et al., 2004; Samson and Ferguson, 2015). Many OVLT functions are under circadian control, including anticipatory thirst and osmoregulation (Trudel and Bourque, 2010; Gizowski et al., 2018; Gizowski and Bourque, 2020). The SCN secretes a number of paracrine output signals (Cheng et al., 2002; Kraves and Weitz, 2006; Maywood et al., 2011). This portal system may provide a pathway by which these diffusible circadian-timing signals are maintained at levels that directly influence other brain regions.
4. The neurovascular system is dynamic and diurnally rhythmic
Modulation of blood flow, metabolism, and neural activity within the brain is dynamically regulated. The complex multicellular NVU responds accordingly. Beside the changes that occur with aging (Fabiani et al., 2021; Zimmerman et al., 2021), many of these alterations display rhythmic, anticipatory variations that are correlated with specific phases of the 24-h day-night cycle. These circadian rhythms, derived from the Latin circa (approximately) and dies (day), are driven by endogenous clocks that enable organisms to anticipate and prepare for changes over the day-night cycle. Although not restricted to the neurovasculature, one of the most notable vascular functions that displays circadian rhythmicity is blood pressure (Conroy et al., 2005; Paschos and FitzGerald, 2010). In humans, blood pressure rises before awaking, reaches a peak in midmorning and then decrease as the day progresses (Millar-Craig et al., 1978). Circadian rhythms of regulators of blood pressure, plasma epinephrine, norepinephrine, cortisol, cardiac vagal tone, and heart rate all have been noted (Kalsbeek et al., 2006). Ablation of the hypothalamic SCN disrupts the circadian variation in blood pressure, demonstrating that the central circadian clock is necessary to orchestrate these diverse vascular changes (Janssen et al., 1994; Sano et al., 1995; Witte et al., 1998).
Platelet activation, which mediates blot clot formation, and fibrinolysis, the dissolution of these clots, also displays circadian rhythmicity (Paschos and FitzGerald, 2010; Thosar et al., 2018; Budkowska et al., 2019). With regard to clot formation, platelet aggregation and platelet activation markers show strong time-of-day variation, peaking mid-morning in humans. These include factors promoting blood coagulation, such as platelet factor 4 (PF4), glycoprotein Ib (GPIb), β-thromboglobulin (βTG), P-selectin, and activated integrin αIIbβ3 (also known as GP11b/IIIa) (Tofler et al., 1987; Jafri et al., 1992; Scheer et al., 2011; Thosar et al., 2018; Crnko et al., 2019). Additionally, fibrinogen, the circulating precursor of fibrin, a protein that propagates clotting, demonstrates a 24-h variation in humans (Bremner et al., 2000). In tandem, fibrinolytic activity is significantly reduced in the morning (Andreotti and Kluft, 1991). The concentration of plasminogen activator inhibitor-1 (PAI-1), which inhibits fibrinolysis, peaks in the morning (Andreotti et al., 1988; Kluft et al., 1988; Angleton et al., 1989). This is endogenously driven, independent of the sleep cycle (Scheer and Shea, 2014). Together these findings suggest that mornings, with the increase in platelet activation and decrease in fibrinolysis, constitute an adverse stressor toward negative cardio-and neurovascular events.
Indeed, adverse neurovascular events, including ischemic stroke and hemorrhagic stroke, demonstrate daily fluctuations (Elliott, 1998; Chaturvedi et al., 1999; Manfredini et al., 2005; Butt et al., 2009; Turin et al., 2010; Ripamonti et al., 2017). In intracerebral hemorrhage and subarachnoid hemorrhage detection, a bimodality in the rhythm is present, with the peak in morning and second smaller peak in afternoon/early evening (Butt et al., 2009; Turin et al., 2010). Additionally, differential damage outcome is associated with the time of day of the stroke. In the morning, the increases in paracellular permeability and immune-cell trafficking are associated with more severe stroke phenotype and more adverse outcomes compared to strokes at that occur in the afternoon (Liu et al., 2021). Thus, circadian rhythms may influence stroke vulnerability of the NVU. A more in depth understanding could allow targeted prevention in the development and progression of neurovascular dysfunction and disease.
5. The circadian system and the molecular clock
Circadian rhythms are orchestrated by a central pacemaker within the hypothalamus, the SCN. Lesions of the SCN abolish or attenuate daily rhythms in locomotor activity, body temperature, blood pressure, and heart rate in rodents (Stephan and Zucker, 1972; Ralph et al., 1990; Witte et al., 1998), indicating that these are endogenous rhythms rather than driven by 24-h changes in the environment or daily behaviors. SCN rhythms are synchronized to the external environment primarily by light signals via the retinohypothalamic tract (Ding et al., 1994; Gooley et al., 2001). Other salient entrainers, or zeitgebers, are food and behavioral activity. The SCN is an autonomous oscillator, maintaining rhythms in vitro (Gillette and Reppert, 1987; Yamazaki and Takahashi, 2005). Notably, dissociated SCN cells also remain rhythmic in culture, demonstrating the cell-intrinsic property of rhythmicity (Welsh et al., 2004).
At its core, the endogenous circadian clock is a transcriptional-translational negative feedback loop (TTFL). Several interlocking TTFLs act in collaboration with a key negative feedback loop (Figure 3). In this key loop are the positive transcriptional regulators, the proteins BMAL1 and CLOCK. These two positive regulators heterodimerize and bind to cis-acting E-box promoter elements to activate the expression of negative regulators, which include the repressors CRYPTOCHROME 1 and 2 (CRY1, CRY2) and PERIOD 1, 2, and 3 (PER1-3). As the cycle progresses, the negative regulators accumulate, form complexes and are transported to the nucleus where they inhibit BMAL/CLOCK-activated transcription, thereby inhibiting their own transcription (Takahashi, 2017; Cox and Takahashi, 2019; Rosensweig and Green, 2020). An auxiliary loop involving REV-ERB and ROR modulates the main TTFL to further controls the expression of CLOCK and BMAL1 (Takahashi, 2017). The regulation and machinery of the molecular clock mechanism has been extensively reviewed (Takahashi, 2017; Cox and Takahashi, 2019; Rosensweig and Green, 2020).
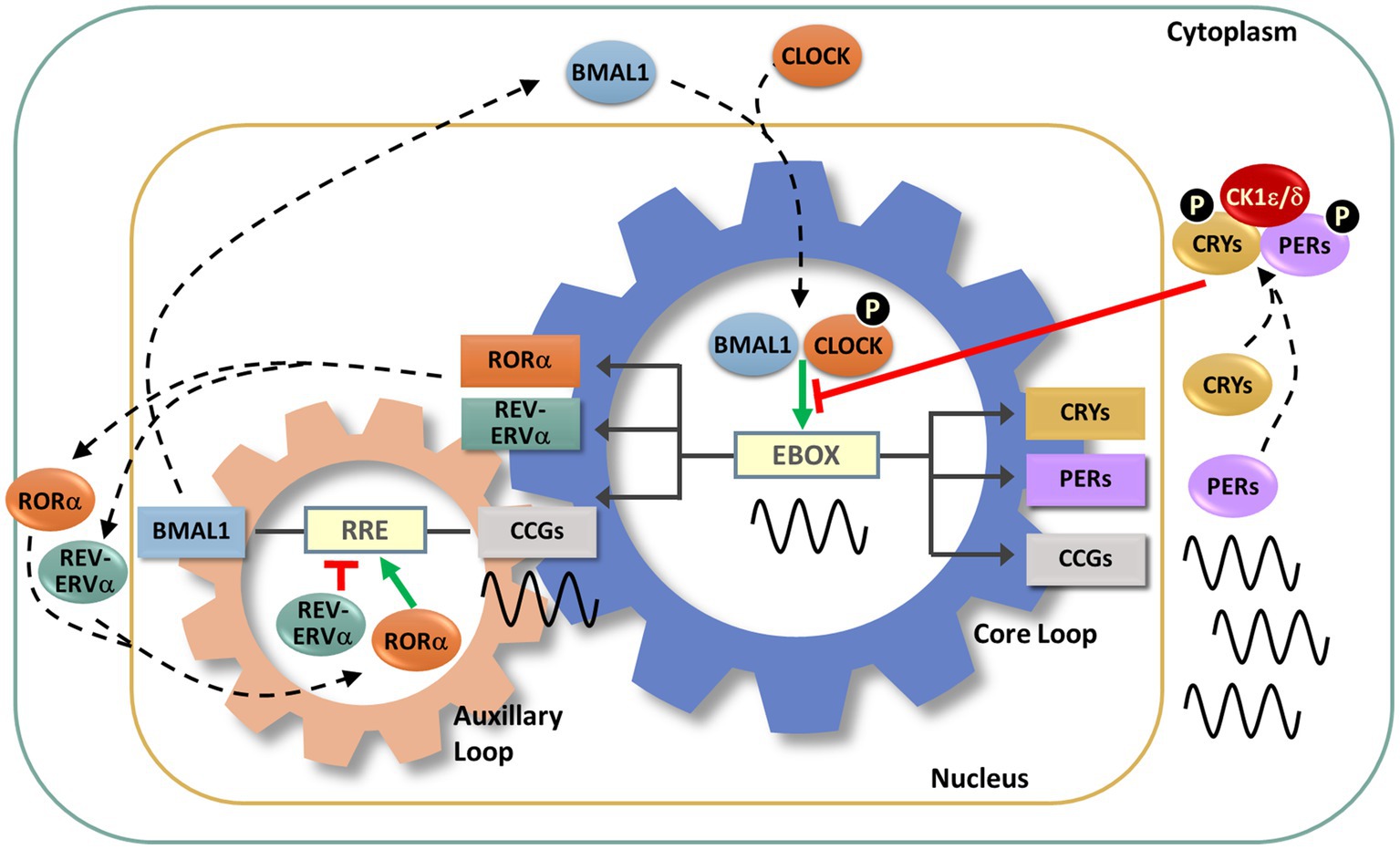
Figure 3. Molecular circadian clockwork is composed of two interlocking transcription/translation feedback loops (TTFLs). The clock proteins, CLOCK and BMAL1, are integral components of the core circadian timekeeping loop. They form a heterodimer, then induce E-box-mediated transcription of negative regulators, Period (Per1,2,3) and Cryptochrome (Cry1,2) genes. Accumulated PER and CRY proteins repress E-box-mediated transcription until their levels decrease, allowing the cycle to repeat. CLOCK and BMAL1 also control the transcription of the nuclear receptors RORα and REV-ERBα, which modulate BMAL1 mRNA levels by competitive actions on the RRE element residing in the Bmal1 promoter. The cycling of clock components collectively determines the temporal patterning and levels of clock-controlled genes (CCGs), thus generating diverse circadian rhythmic outputs. In addition, a number of signaling molecules, including kinases and ubiquitinases, fine-tune these molecular clock loops. Casein kinase Iε (CKIε) and CKIδ form a complex with PERs and CRYs, phosphorylate (P) PERs and then promote proteasome-dependent degradation of these negative regulators. Phosphorylation of CLOCK facilitates its dimerization with BMAL1 and nuclear entry.
This TTFL generates endogenous circadian rhythms even under constant conditions (absent environmental or behavioral rhythms). These endogenous rhythms are present in nearly all cells and physiological systems, including those of involved with neuronal, metabolic, immune, inflammatory, and vascular function (Thosar et al., 2018, 2019). Collapse of this TTLF leads to internal cellular desynchrony and systemic circadian rhythm disruption. Circadian rhythm disruption results in a higher risk, as well as being an early indicator, for disease (Foster, 2020).
6. Circadian influences on cells of the NVU
Although much of the circadian variation in the neurovascular system is coordinated by the pacemaker function of the SCN, there are also likely local rhythms present within the molecular clocks of the cellular components of the NVU. Each component of the NVU is closely linked. The circadian influences on cells of the NVU are numerous resulting in discrete alterations in these cells across the day night cycle (Figure 4). There is a need to investigate the free-running rhythms in cells of the NVU under constant conditions. Nevertheless, to understand these influences as they currently stand, the various cell types of the NVU will be evaluated individually.
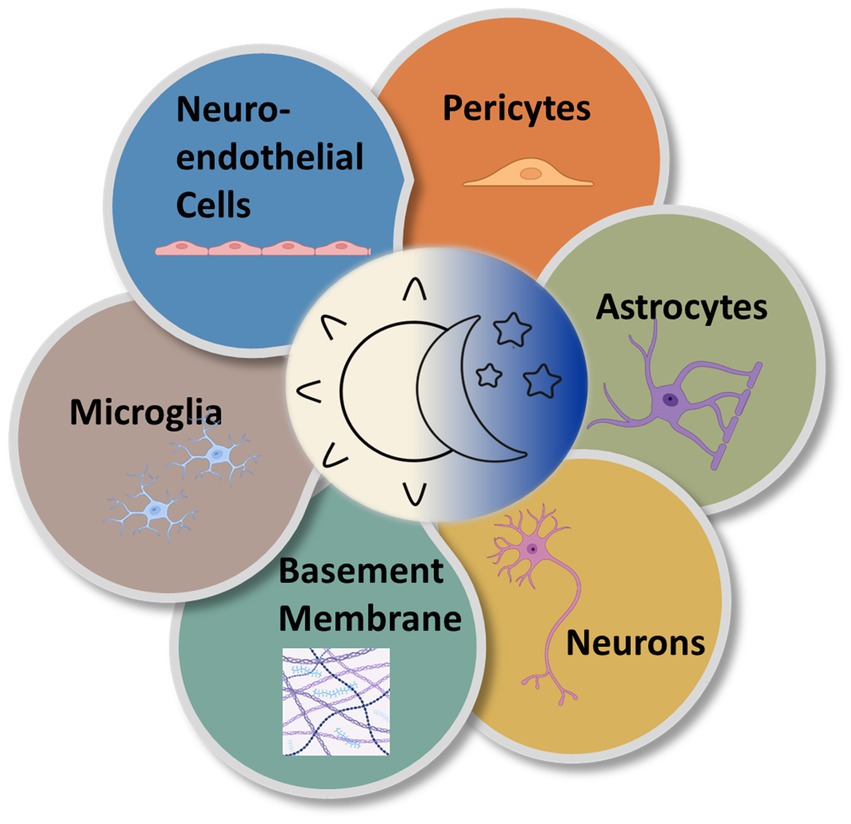
Figure 4. Circadian rhythms are expressed in the various cell types of the NVU. Multiple types of cells comprise the NVU and contribute to neurovascular function. All express near-24-h oscillations in functionality.
6.1. Neuroendothelial cells
The endothelial cells of the BBI are distinct from other endothelial cells. Rather than forming a semi-permeable conduit for the blood, as in the periphery, neuroendothelial cells form a barrier between the brain and the blood, permitting only a few types of molecules to cross. They form a cellular layer with high electrical resistance, tight intercellular junctions, specific transporters, and decreased levels of vesicular transcellular transport compared to peripheral endothelial cells (Betz and Goldstein, 1978; Betz et al., 1980; Daneman, 2012). The activity of efflux transporters is an important regulator of permeability across the BBI. Except in areas of the CVO, there is a general absence of fenestrae in the brain neuroendothelium (Abbott et al., 2010).
Neuroendothelial cells of the luminal membrane of the BBI highly express the ATPase-binding cassette (ABC) efflux transporter, ABCB1, alternatively named permeability glycoprotein (Pgp) and multi-drug resistant protein 1 (MDR1) (Cordon-Cardo et al., 1989; Schinkel et al., 1994). ABCB1 substrate-specific efflux oscillates across the circadian cycle in mouse and human neuroendothelia (Zhang et al., 2021). Although dependent on a functional molecular clock, the ABCB1 transcript family does not exhibit a circadian oscillation nor does the ABC transporter family demonstrate regulation at the transcript level by the circadian clock (Zhang et al., 2021). However, the necessary cofactor in the regulation of ABC transports, intracellular Mg2+, demonstrate circadian oscillations that persist in constant conditions in such diverse examples as a human cell line with epithelial morphology, U2OS cells, as well as a unicellular algae, Osteocossus tauri (Feeney et al., 2016). Further, the transient receptor potential cation channel, subfamily M, member 7 (Trpm7), a Mg2+ transporter expressed in neuroendothelial cells, exhibits circadian oscillations in its transcript (Zhang et al., 2021). Thus, efflux by transporters, such as ABCB1, may be regulated through its co-factor, via daily fluctuations in intracellular Mg2+.
The barrier function of the BBI is regulated by neuroendothelial tight-junction proteins. Tight junctions contain multiple protein complexes, which may include occludins, claudins, zonula occludens (ZO), and junctional adhesion molecules (JAM). Multiple studies have demonstrated circadian regulation of tight proteins at both the RNA and protein levels, although not specifically in the BBI. In retinal pigment epithelium, claudin 2 (cldn2) was found to display circadian rhythms in both protein and RNA expression (Louer et al., 2020). In the inner retinal blood vessels, claudin-5 was found to be highly dynamic and regulated in a circadian manner by the clock protein BMAL1 (Hudson et al., 2019). In intestinal epithelial cells, occludin and claudin-1 mRNA display oscillations across the day-night cycle and their expression is inversely associated with colonic permeability (Oh-oka et al., 2014). This suggests the potential for diurnal or circadian oscillations in the BBI, but this has yet to be determined.
Additionally, neuroendothelial cells can secrete molecules that affect microglia. One such molecule is the proinflammatory cytokine, tumor necrosis factor alpha (TNFα). Although the primary source of TNFα is macrophages and monocytes, TNFα production in neuroendothelial cells has been noted (Imaizumi et al., 2000). TNFα undergoes circadian oscillations in its uptake and can activate interleukin-15 (IL-15). Notably, IL-15 affects the growth of microglia as well as nitric oxide production. Further, IL-15 and its receptor can feedback, exerting anti-inflammatory roles (Pan et al., 2011).
Neuroendothelial cells contribute to the aforementioned circadian fluctuation in the coagulation/fibrinolytic factors. Thrombomodulin, an endothelial membrane protein that exerts anti-coagulation effects through protein C (de Wouwer et al., 2004), displays circadian oscillations at both the level of the RNA and protein in lung and heart (Takeda et al., 2007). Plasminogen activator inhibitor-1 (PAI-1), the principal inhibitor of fibrinolysis in vivo, exhibits significant circadian variation with peak circulating levels observed in the morning (Angleton et al., 1989), as well as activation by the core clock proteins, CLOCK and BMAL1 (Schoenhard et al., 2003). Whereas tissue plasminogen antigen (t-PA) which catalyzes the conversion of plasminogen into plasmin and thereby promoter of fibrinolysis, also demonstrated daily variations in levels with increasing concentrations found in the afternoon in humans (Liu et al., 2021).
6.2. Astrocytes
The function of astrocytes in the BBI is not fully understood; however, evidence suggests that they induce barrier-like properties in neuroendothelial cells by releasing factors that include TGFβ, glial-derived neurotrophic factor (GDNF), bFGF, and angiopoietin 1 (Abbott et al., 2006), as well as exerting an effect on BBI polarity (Beck et al., 1984). They control neurotransmitter and ion concentrations to maintain homeostatic balance. Astrocytes interface with neuroendothelial cells through the endfeet of specialized processes that line the outer side of cerebral capillaries. They contribute importantly in regulating metabolite levels and brain water content, and they modulate vasodilation. Astrocytes release several molecules that enhance and maintain barrier properties: members of the Hedgehog family, proteins of the renin-angiotensin system, and apolipoprotein (a cholesterol and phospholipid transporter).
Circadian fluctuations in astrocyte structure and function throughout the brain are well documented (McKee et al., 2020; Naseri Kouzehgarani et al., 2022; Rojo et al., 2022; Hastings et al., 2023). Astrocytes contribute to cell–cell coupling in the SCN, undergo daily changes in GFAP distribution and structural complexity, and physical coverage of neurons. Glia respond to daily oscillations in neuronal activity (McKee et al., 2020, Naseri Kouzehgarani et al., 2022, Rojo et al., 2022, Hastings et al., 2023). They may also be involved in thermoregulation, hormonal secretion, and sleep (McKee et al., 2020).
The role of circadian rhythms in astrocyte structure and function in neurovascular function is less understood. However, it is notable that the water channel, aquaporin 4 (AQP4) displays day-night differences in expression levels. AQP4 can control fluid exchange bidirectionally. It is abundant in astrocytes associated with the neurovasculature and is present in high density in astrocytic endfeet bordering blood vessels. Although no difference in the total level of AQP4 has been reported, its polarization to vascular structures is highest during the day in the cortex of rodents suggesting diurnal variation in water exchange across the BBI (Hablitz et al., 2020). Mice deficient in AQP4 display profound reduction in glymphatic clearance (Iliff et al., 2012; Lundgaard et al., 2017), however with regard to BBI integrity, studies lack consensus (Zhou et al., 2008; Saadoun et al., 2009; Haj-Yasein et al., 2011). This topic merits further careful analysis.
6.3. Neurons
There is significant heterogeneity in the innervation of the NVU. Broadly, larger, surface extracranial cerebral vessels (such as carotid arteries, vertebral arteries and jugular veins) and intracranial vessels (pial arteries, perforant arteries and pial veins) receive peripheral or extrinsic innervation by cranial autonomic ganglia. Input is derived from sympathetic nerves originating from the superior cervical ganglia, parasympathetic nerves from the sphenopalatine ganglion or otic ganglion, and sensory nerves from the trigeminal ganglion releasing a number of neuropeptides (including substance P, calcitonin gene-related peptide, vasoactive intestinal peptide, pituitary adenylate cyclase-activating peptide, neuropeptide Y, and somatostatin) (Hamel, 2006; Schaeffer and Iadecola, 2021). On the other hand, smaller penetrating arterioles and capillaries receive central or intrinsic innervation via nerve terminals from local interneurons or subcortical pathways, such as the thalamus, locus coeruleus, raphe nucleus and basal forebrain. Communication is mediated via a number of different factors, including neuropeptides, neurotransmitters, prostaglandins, ions, and NO (Giorgi et al., 2020; Schaeffer and Iadecola, 2021).
At the local level, neurons of the NVU directly and indirectly control local cerebral blood flow via neurovascular coupling or function hyperemia in response to neuronal activity, as well as impact vascular networks and BBI formation through release of vasoactive agents (Attwell et al., 2010; Whiteus et al., 2014; McConnell et al., 2017; Kugler et al., 2021). Much of the local neurovascular coupling involved the increased release of glutamate with neuronal activity. Glutamate release can activate neuronal and glial signaling pathways that release vasoactive effectors. Mediators of local neurovascular coupling through inhibitory interneurons have demonstrated the ability to either induce vasodilation or vasoconstriction (Cauli et al., 2004; Rancillac et al., 2006).
Much less is known about the role of circadian neuronal activity in regulating neurovascular function. The numerous neuronal releasates involved in NVU regulation are beyond the scope of this review. We focus on examples with regards to circadian function rather than those involved in acute, inducible alterations to the NVU. Regarding intrinsic innervations that project to cortical microvessels, a number of subcortical pathways are associated with wakefulness and undergo alterations with sleep deprivation. The locus coeruleus expresses 24-h rhythms in the noradrenaline rate-limiting enzyme tyrosine hydroxylase in animals housed under both light:dark (LD) and continuous dark (DD) conditions (Caputo et al., 2023). Additionally, functional MRI in humans has linked cerebral blood flow to diurnal modulation in regions of the default-mode network displaying a decrease in from morning into the afternoon. The default mode network is primarily composed of the medial temporal lobe, the medial prefrontal cortex, and the posterior cingulate cortex and associated with internal thought, active while not engaged in specific tasks (Hodkinson et al., 2014). One of these mediators is nitric active during oxide (NO). NO is generated by a family of three nitric oxide synthase (NOS) isoforms: neuronal NOS (nNOS); endothelial NOS (eNOS), and inducible NOS (iNOS). Although nNOS is abundantly expressed in neurons, it is also expressed in non-neuronal cells (Boulanger et al., 1998; Loesch et al., 1998; Schwarz et al., 1999). It has been argued that the nNOS form participates significantly in regulating microvascular tone (Costa et al., 2016). However, both eNOS and nNOS have been linked to control of vascular tone (Kurihara et al., 1998; Fleming, 2003; Hagioka et al., 2005; Förstermann and Münzel, 2006; Lemmer and Arraj, 2008; Seddon et al., 2008) Multiple genes (eNOS, caveolin1 and 3) and proteins (tetrahydrobiopterin, phospho-Akt, phospho-eNOS) linked to NO-induced vasodilation display circadian expression, however, their rhythmic regulation in the neurons of the NVU has not been determined (Panda et al., 2002; Rudic et al., 2005; Kunieda et al., 2008; Anea et al., 2009).
Although much research has focused on the effect of neuronal activities on CBF, recent work also has demonstrated an influence of neurons of the NVU on BBI permeability. Elevated expression and function of a major neuroendothelial ABC efflux transporter, P-glycoprotein (pgp), was found to be correlated to decreases in neuronal activity. A diurnal rhythm in pgp substrates efflux was negated in a knockout of the clock gene, Bmal1 (Pulido et al., 2020).
6.4. Pericytes
Pericytes are imbedded in the basement membrane in direct contact with neuroendothelial cells, wrapping around the tightly-connected neuroendothelial cells. They are found in the capillary bed but display a marked preference for junctional locations within branching capillaries (Mughal et al., 2023). They have been found to play a regulatory role in brain angiogenesis (the formation of new capillaries from existing ones), neuroendothelial cell-tight junction formation, and differentiation of the blood–brain interface, as well as contribute to structural stability. The CNS vasculature has a significantly higher pericyte coverage compared with peripheral vessels (Winkler et al., 2011; Hall et al., 2014).
Pericytes rhythmically express a number of circadian genes (Mastrullo et al., 2022). A mouse line lacking BMAL1 exhibited a decrease in pericyte coverage within the NVU. This decrease in pericyte coverage increased permeability within the BBI, led to a decrease in platelet-derived growth factor receptor β (PDGFRβ) transcription. The PDGFR is important for BBI integrity (Nakazato et al., 2017b). Exposure to synchronized pericytes in a contact co-culture also synchronizes neuroendothelial circadian rhythms of Bmal1:luciferase, a clock gene reporter (Mastrullo et al., 2022).
6.5. Vascular smooth muscle cells
Vascular smooth muscle cells (VSMC) of the NVU are responsible for constriction and dilation of larger arterial and arteriole blood vessels, regulating vascular resistance and blood pressure and stabilizing smaller downstream vessels from the pulsative effect of heartbeat. At this level neurovascular tone is regulated by the relaxation and contraction of VSMC. Hyperpolarization results in the relaxation of the VSMC whereas depolarization results in contraction of the VSMC and constriction of the blood vessels. A number of factors can influence VSMC, including the sympathetic nervous system (discussed in section 6.3) circulating mediators, and endothelial cells (Sandoo et al., 2010; Kisler et al., 2017).
Most work on the influence of the circadian clock in smooth muscle has focused on the peripheral vascular system and not specifically on smooth muscle within neurovascular system. Mesenteric smooth muscle and the aorta exhibit time-of-day variation in contractility in response to vasoconstricting and vasodilating stimuli (Keskil et al., 1996; Witte et al., 2001; Denniff et al., 2014). Molecular clocks have been described in smooth muscle cells (Nonaka et al., 2001) and mesenteric arteries (Denniff et al., 2014). They rhythmically display a number of genes involve in structural integrity in an immortalized vascular smooth muscle cell line, including metalloproteinase 1 and 3 (timp1/3), collagen 3a1 (col3a1), transgelin 1 (sm22alpha), and calponin 1 (cnn1) (Chalmers et al., 2008). Deletion of the clock gene, Bmal1, specifically in vascular smooth muscle cells abolishes the circadian variation in pulse pressure and attenuates the amplitude of the daily rhythm in systolic and diastolic blood pressure (Xie et al., 2015). Insights on the molecular circadian clock as well as the effect of a dysfunctional clock specifically on the VSMC within the NVU would be useful, but this has yet to be studied.
6.6. Microglia
Microglia are associated with numerous functions within the brain including trophic support, angiogenesis, the regulation of synaptic pruning and neuronal activity (Geloso and D'Ambrosi, 2021; Umpierre and Wu, 2021); however, within the context of the NVU, they are more closely linked to immune surveillance, repairing damage to the BBI as well as regulating vascular tone (Lou et al., 2016; Bisht et al., 2021). Microglia are the resident immune cells in the CNS. In this role, they actively monitor the brain parenchyma via highly motile protrusions and increase phagocytic activity and cytokine production in response to pathogens, neuroinflammation and tissue damage (Madore et al., 2020). Their role in regulating vascular tone involves capillary-associated microglia (CAMS), found in close contact with the capillary wall in the brain in areas not covered by astrocytic endfeet. Loss of CAMS increases blood flow by increasing capillary diameter and results in impaired responses to vasodilation (Bisht et al., 2021).
Microglia have been shown to contain a functional molecular clock and exhibit circadian expression of a number of clock genes, which remain rhythmic in constant conditions, as well as rhythmic expression of a number of microglial markers (Nakazato et al., 2011; Hayashi et al., 2013; Guzman-Ruiz et al., 2023). In addition, Wang et al. demonstrated daily oscillations in the expression of pro-inflammatory cytokines (IL-1β and IL-6) and oxidation-related genes (NADPH oxidase 2, Nox2), as well as molecules involved in nutrient utilization (glucose transporter member 5, Glut5) (Wang et al., 2020). Elevated levels of pro-inflammatory cytokine transcripts during the light (inactive) phase in rodents may indicate a higher innate immune activity when they are sleeping during the day.
Functionally, microglia also exhibit diurnal oscillation in phagocytic activity linked to synaptic pruning during sleep (Choudhury et al., 2020). Furthermore, just as peripheral immune cells display circadian alterations in their response to an immune challenge (Marpegan et al., 2009; Spengler et al., 2012), time-of-day effects within the CNS also have been reported. Microglial process extension are more pronounced at a rodent’s inactive state (day) compared to night (Takayama et al., 2016). Expression of microglial proinflammatory cytokines (IL-1β, TNFα and IL-6) and the inflammatory pathway are elevated with a immune challenge during a rodent’s day (inactive) as compared to night (active) (Fonken et al., 2015; Takayama et al., 2016). It will be important to determine if these day-night differences persist under constant conditions.
Intriguingly, although Cathepsin S (CatS) has not been investigated for a role in altered BBI permeability across the day-night cycle, its demonstrated circadian expression suggests a possible mechanism by which this could occur. CatS, a microglial-specific cysteine protease within the CNS, displays clock-driven expression (Hayashi et al., 2013). CatS-deficient mice exhibited impaired migration across peripheral endothelial basement membranes (Sukhova et al., 2003). It has the ability to degrade ECM molecules at neutral pH (Liuzzo et al., 1999; Vizovišek et al., 2019) and can mediate BBI permeability through proteolytic processing of the junctional adhesion molecule B (JAM-B) (Sevenich et al., 2014).
6.7. Basement membrane
The vascular basement membrane, an extracellular matrix (ECM) of structural proteins secreted by cells of the NVU, supports vessel development and maintenance of the BBI. It consists predominately of (1) glycoproteins, such as the integrins laminin, collagen, fibronectin, and vitronectin, (2) enzymes associated with ECM remodeling and processing, and (3) soluble growth factors and cytokines. It is sandwiched between neuroendothelial cells and pericytes on one side and astrocytic endfeet on the other. The ECM proteins are key regulators of cell signaling. The ECM exert its effect through adhesion signaling receptors that sense and report the environment and the surrounding cells (Streuli, 2016).
Although less is known about the role of the ECM in circadian neurovascular function, there is a link of the ECM to clock-gene expression. Changes in the stiffness of the microenvironment of the mammary epithelia can modulate BMAL1-CLOCK activity (Yang et al., 2017). The extracellular environment via integrins transmits signals to the cytoskeleton (Akhtar and Streuli, 2013) and daily oscillations in actin polymerization state have been observed in liver (Gerber et al., 2013) and brain (Gillette, unpublished). The depletion of globular (G) actin toward filamentous (F) actin assemblies release myocardin-related transcription factor (MRTF) from the cytoplasm allowing a rhythmic translocation into the nucleus. MRTF is a serum response factor (SRF) cofactor, and as such leads to 24-h cycles of transcriptional activation by genes targeted though the MRTF-SRF signaling pathway. Thus, a link between the ECM and the circadian molecular clock within the NVU may be through actin dynamics (Streuli and Meng, 2019).
7. Impaired neurovascular function with circadian dysregulation
Circadian neurovascular function is essential in balancing the influx and efflux between blood and the brain parenchyma; and thereby, modulating the exchange of nutrients and ions as well as protecting neural tissue from access by toxins and pathogens. Circadian rhythm disruption, interference with a stable circadian cycle, can have profound effects on the regulation CNS homeostasis. There has been a rise in human circadian rhythm disruption due to sleep disruption, travel to different time zones, shift work, and social jetlag that has let in an increase in neurological disorders, cancer, metabolic disorders, and mood disorders (Hatori et al., 2017; Lunn et al., 2017; Schurhoff and Toborek, 2023). Alterations of normal circadian rhythms can have profound effects on the neurovasculature, affecting the BBI, cerebral blood flow (CBF), and immune surveillance (Figure 5). These disruptions can contribute to the severity of pathology and response to treatment, most notably with stroke (Thosar et al., 2018; Ramsey et al., 2020; Liu et al., 2021) and traumatic brain injury (Li et al., 2016).
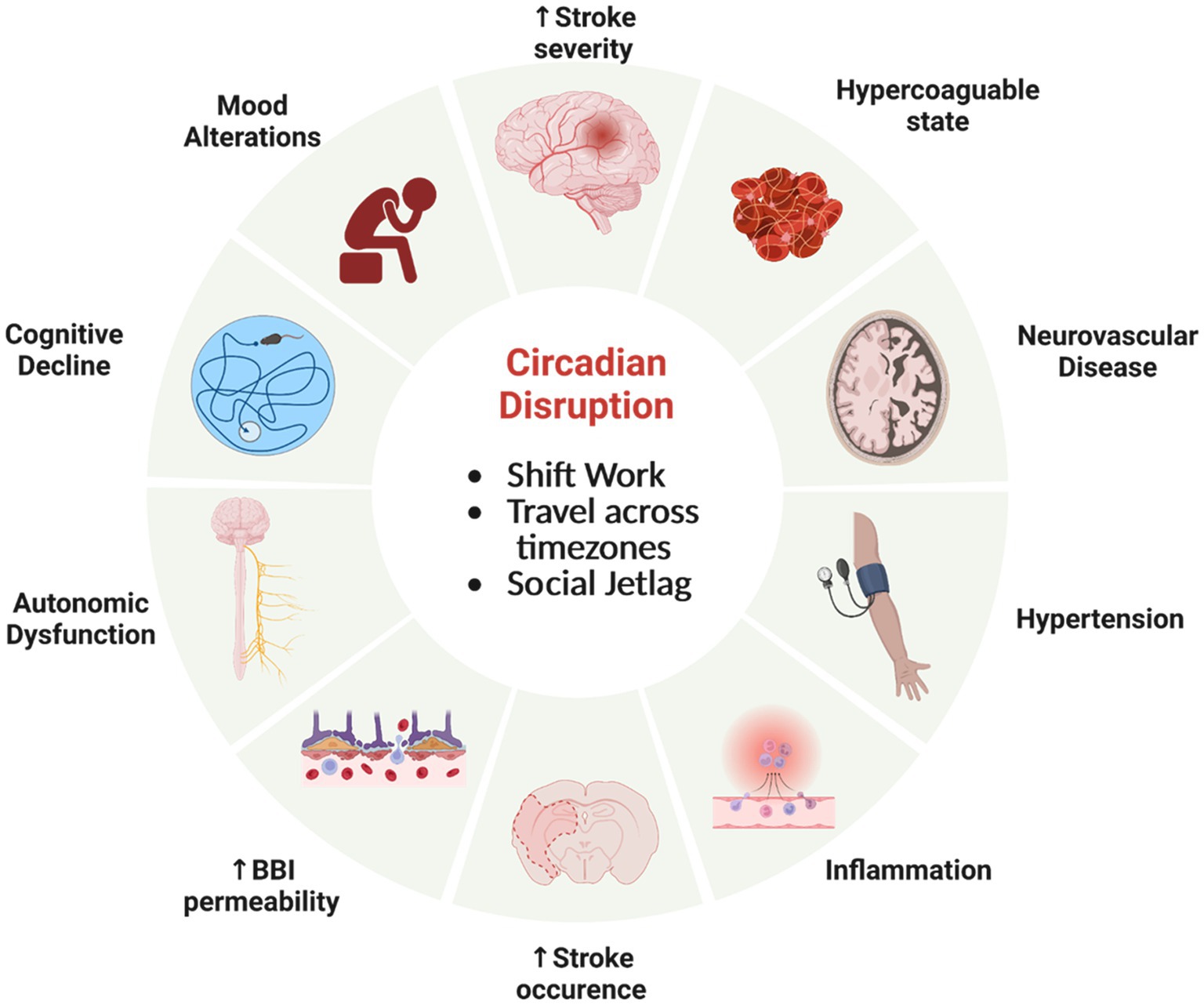
Figure 5. Circadian rhythm disfunction adversely effects physiology. Misalignment of circadian clocks between cells, brain regions, tissue, across the organism and the natural world can increase the risk of significant health consequences involving the neurovasculature and brain. Created by Biorender.com.
7.1. Blood–brain interface (BBI)
The BBI plays an integral role in maintaining tight control of brain chemical composition. Understanding the consequences of alterations to the BBI becomes paramount, considering loss of BBI integrity is present in many neurodegenerative disorders, such as Alzheimer’s disease and Parkinson’s disease (Sweeney et al., 2018). As these diseases involve the accumulation of protein that form fibrils and aggregates, it is important that BBI maintain proper regulation across the interface for removal of waste. Additionally, proteasomal activity follows a circadian rhythm and disruption of these rhythms leads to diminished neuroprotection against protein-plaque formation (Musiek and Holtzman, 2016). Loss of BBI integrity can increase vascular permeability and is associated with cell damage from impaired blood flow and the entrance of toxins into the brain, which can stimulate inflammatory and immune responses. Increases in BBI permeability have been noted with disruption of the molecular clock (Bmal1 knockout) and sleep deprivation (Gómez-González et al., 2013; He et al., 2014; Nakazato et al., 2017b).
With stroke, the blood supply is disrupted, causing essential nutrients not to reach the irreversibly damaged ischemic core, as well as the reversibly injured brain tissue around the ischemic core, the penumbra. Due to an imbalance in ion transport, secondary neuronal damage can occur. Circadian disruptions initiated by weekly phase advances prior to ischemic events exacerbate inflammation and increase in infarct volume (Earnest et al., 2016; Ramsey et al., 2020). It has been suggested that circadian rhythm disruption leads to a propensity for clotting, a prothrombotic state (Liu et al., 2021). A dysfunctional molecular clock in mice, due to either a disruption of Bmal1 expression or a Clock gene mutation, causes a more hypercoagulable state, with alterations in platelet counts and clotting components (Westgate et al., 2008; Hemmeryckx et al., 2011). Considered together, these findings suggest that circadian rhythm dysfunction elevates the risk for greater stroke damage and negative outcome.
7.2. Cerebral blood flow
Circadian regulation of blood flow is well documented in the periphery (Wang et al., 1992; Sano et al., 1995; Hermida et al., 2007; Xie et al., 2015; Douma and Gumz, 2018). Blood pressure rises sharply early in the active period and declines to its lowest levels during sleep. However, in a cohort termed “normal dippers,” the mean blood pressure during sleep is 10–20% compared to the daytime-active mean (Hermida et al., 2007). Subjects with a diminished decline in blood pressure (“non-dippers”), have a significantly higher risk for cerebrovascular disease and vascular dementia. Less is known about diurnal alterations in CBF and the consequences of circadian rhythm dysfunction on CBF. Non-invasive measurements of cerebral blood flow velocity (CBFV) in the middle cerebral artery have been used as a surrogate for CBF. Using these methods, daily rhythms in CBFV in human subjects are lower in morning than in afternoon and evening (Sawaya and Ingvar, 1989; Madsen et al., 1991; Droste et al., 1993; Conroy et al., 2005; Kotajima et al., 2005). This phenomenon is often linked with sleep–wake behaviors. CBFV in healthy humans is reduced in non-REM sleep compared to wakefulness (Madsen et al., 1991; Droste et al., 1993; Kotajima et al., 2005). However, Conroy et al. noted time-of-day variations in human CBFV is not altered following 30-h of sustained wakefulness, and therefore not dependent on sleep (Conroy et al., 2005). Similarly in rats, CBF measured a by laser-Doppler flow probe (over 3–4 days) demonstrated diurnal changes independent of peripheral arterial blood pressure or locomotion (Wauschkuhn et al., 2005). These studies were performed under 12:12-h environmental light:dark conditions. This area would benefit from further studies under constant dark conditions to establish the endogenous nature of these oscillations across the circadian cycle.
Regional CBF has been measured by perfusion MRI. CBF in specific regions was altered in workers performing shift work for >2 years. When compared to daytime workers, the cuneus, fusiform/parahippocampal gyri, and cerebellum of shift workers were significantly decreased, while the inferior occipital gyrus was increased (Park et al., 2019). Alterations in circadian rhythms of blood pressure may have significant consequences for cerebral microbleeds. Patients with cerebral microbleeds have higher nocturnal mean systolic blood pressure and lower nocturnal dipping rates (Chen et al., 2022). Patients with cerebral small vessel disease (CSVD) show disturbance of the circadian rhythms in blood pressure (non-dippers and reverse-dippers) as well as cognitive dysfunction (Xu et al., 2023).
7.3. Immune surveillance and repair
Disruption of circadian rhythms can have deleterious outcomes for immune surveillance and repair within the brain. Mice exposed to experimental jetlag exhibit increases in inflammatory markers in the blood (Castanon-Cervantes et al., 2010). Circadian rhythm disruption by irregular rest-activity cycles that involve light at night enhances pro-inflammatory cytokine expression following an immune challenge (Fonken et al., 2013). Loss of a functional molecular clock alters microglial immune responses. Pro-inflammatory cytokines were reduced in microglia isolated from Bmal1-deficient mice (Wang et al., 2020). The induction of pro-inflammatory cytokines was attenuated under lipopolysaccharide (LPS) immune challenge of a microglial cell line model with Bmal1 knockdown and in Bmal1-deficient mice (Nakazato et al., 2017a; Wang et al., 2020). Disruption of Rev-erbα caused unprompted microglial activation in the hippocampus increased expression of inflammatory transcripts (IL-1β/Trem2) and elevated the inflammatory response to LPS. Together these data suggest that disruption of the circadian clock alters shifts the pro−/anti-inflammatory balance necessary to maintain normal microglial responses to inflammatory challenges.
Circadian rhythm disruptions also can have profound effects on increasing risk and exacerbating disease states. This is likely mediate via changes in immune and inflammatory responses to damage or disease, including to ischemia, traumatic brain injury, and neurodegenerative diseases (i.e., Alzheimer’s disease, Huntington’s disease, and Parkinson’s disease) (Li et al., 2016; Ramsey et al., 2020). Alterations to immune surveillance and repair functions within the NVU have significant effects. For example, in mice subjected to cerebral artery occlusion and reperfusion, then chronic circadian rhythm-disruption group displayed increased infarcts. The interpretation is that this is due to alterations in the ratio of pro-to anti-inflammatory cytokine expression (Ramsey et al., 2020). Although circadian rhythm disruption is known to disrupt peripheral immune responses and to alter circulating proinflammatory cytokines and the complement immune system (Comas et al., 2017; Shivshankar et al., 2020), a defective central immune response also is likely to exacerbate diseases states requiring removal of pathogens and damaged cells in brain.
8. Conclusion
The development of the circadian clock in the neurovascular enables anticipation of rhythmic variations that adjust internal states to the 24-h day. In the brain, these changes include oscillations in metabolism, neurohormone secretion, and neurophysiological regulation along with neurovascular components, including cells that form the neurovascular unit and regulate blood flow and platelet aggregation/fibrinolysis. Disruption of the circadian clock has profound effects on mental and physical health. Many disease states are at elevated risk or exacerbated by misalignment of the internal circadian clock (Evans and Davidson, 2013; Foster, 2020; Schurhoff and Toborek, 2023).
Despite significant progress in understanding circadian clocks within the cells of the NVU, knowledge of how the neurovascular units and systems participate in acute responses to cerebrovascular challenges, neurodegenerative diseases, and the aging of the brain is still in its infancy. The roles and risk factors caused by alterations in the circadian system via shift work, travel, and social jet lag are now beginning to be understood. Integrated study of circadian clocks and neurovascular systems has the potential to contribute importantly to understanding cerebral vascular disease, neurodegenerative diseases, and aging, as well as identify isolated key nodes for translational interventions for chronotherapies and other therapeutic benefits.
Author contributions
JM and MG: drafting and refining the manuscript and critical reading of the manuscript. All authors contributed to the article and approved the submitted version.
Funding
The authors gratefully acknowledge support in preparation of this manuscript by the National Heart, Lung, and Blood Institute (1R61/R33HL159948) and the Beckman Institute for Advanced Science and Technology.
Conflict of interest
The authors declare that the research was conducted in the absence of any commercial or financial relationships that could be construed as a potential conflict of interest.
Publisher’s note
All claims expressed in this article are solely those of the authors and do not necessarily represent those of their affiliated organizations, or those of the publisher, the editors and the reviewers. Any product that may be evaluated in this article, or claim that may be made by its manufacturer, is not guaranteed or endorsed by the publisher.
References
Abbott, N. J., Patabendige, A. A., Dolman, D. E., Yusof, S. R., and Begley, D. J. (2010). Structure and function of the blood-brain barrier. Neurobiol. Dis. 37, 13–25. doi: 10.1016/j.nbd.2009.07.030
Abbott, N. J., Ronnback, L., and Hansson, E. (2006). Astrocyte-endothelial interactions at the blood-brain barrier. Nat. Rev. Neurosci. 7, 41–53. doi: 10.1038/nrn1824
Andreotti, F., Davies, G. J., Hackett, D. R., Khan, M. I., De Bart, A. C., Aber, V. R., et al. (1988). Major circadian fluctuations in fibrinolytic factors and possible relevance to time of onset of myocardial infarction, sudden cardiac death and stroke. Am. J. Cardiol. 62, 635–637. doi: 10.1016/0002-9149(88)90669-8
Andreotti, F., and Kluft, C. (1991). Circadian variation of fibrinolytic activity in blood. Chronobiol. Int. 8, 336–351.
Anea, C. B., Zhang, M., Stepp, D. W., Simkins, G. B., Reed, G., Fulton, D. J., et al. (2009). Vascular disease in mice with a dysfunctional circadian clock. Circulation 119, 1510–1517. doi: 10.1161/CIRCULATIONAHA.108.827477
Angleton, P., Chandler, W. L., and Schmer, G. (1989). Diurnal variation of tissue-type plasminogen activator and its rapid inhibitor (PAI-1). Circulation 79, 101–106. doi: 10.1161/01.CIR.79.1.101
Akhtar, N., and Streuli, C. H. (2013). An integrin–ILK–microtubule network orients cell polarity and lumen formation in glandular epithelium. Nat. cell bio. 15, 17–27.
Attwell, D., Buchan, A. M., Charpak, S., Lauritzen, M., Macvicar, B. A., and Newman, E. A. (2010). Glial and neuronal control of brain blood flow. Nature 468, 232–243. doi: 10.1038/nature09613
Attwell, D., Mishra, A., Hall, C. N., O’farrell, F. M., and Dalkara, T. (2016). What is a pericyte? J. Cereb. Blood Flow Metab. 36, 451–455. doi: 10.1177/0271678X15610340
Bautch, V. L., and James, J. M. (2009). Neurovascular development: the beginning of a beautiful friendship. Cell Adhes. Migr. 3, 199–204. doi: 10.4161/cam.3.2.8397
Beck, D. W., Vinters, H. V., Hart, M. N., and Cancilla, P. A. (1984). Glial cells influence polarity of the blood-brain barrier. J. Neuropathol. Exp. Neurol. 43, 219–224. doi: 10.1097/00005072-198405000-00001
Betz, A. L., Firth, J. A., and Goldstein, G. W. (1980). Polarity of the blood-brain barrier: distribution of enzymes between the luminal and antiluminal membranes of brain capillary endothelial cells. Brain Res. 192, 17–28. doi: 10.1016/0006-8993(80)91004-5
Betz, A. L., and Goldstein, G. W. (1978). Polarity of the blood-brain barrier: neutral amino acid transport into isolated brain capillaries. Science 202, 225–227. doi: 10.1126/science.211586
Bisht, K., Okojie, K. A., Sharma, K., Lentferink, D. H., Sun, Y. Y., Chen, H. R., et al. (2021). Capillary-associated microglia regulate vascular structure and function through PANX1-P2RY12 coupling in mice. Nat. Commun. 12:5289. doi: 10.1038/s41467-021-25590-8
Boulanger, C. M., Heymes, C., Benessiano, J., Geske, R. S., Lévy, B. I., and Vanhoutte, P. M. (1998). Neuronal nitric oxide synthase is expressed in rat vascular smooth muscle cells: activation by angiotensin II in hypertension. Circ. Res. 83, 1271–1278. doi: 10.1161/01.RES.83.12.1271
Bremner, W. F., Sothern, R. B., Kanabrocki, E. L., Ryan, M., Mccormick, J. B., Dawson, S., et al. (2000). Relation between circadian patterns in levels of circulating lipoprotein(a), fibrinogen, platelets, and related lipid variables in men. Am. Heart J. 139, 164–173. doi: 10.1016/S0002-8703(00)90324-7
Brightman, M. W., and Reese, T. S. (1969). Junctions between intimately apposed cell membranes in the vertebrate brain. J. Cell Biol. 40, 648–677. doi: 10.1083/jcb.40.3.648
Budkowska, M., Lebiecka, A., Marcinowska, Z., Woźniak, J., Jastrzębska, M., and Dołęgowska, B. (2019). The circadian rhythm of selected parameters of the hemostasis system in healthy people. Thromb. Res. 182, 79–88. doi: 10.1016/j.thromres.2019.08.015
Budohoski, K. P., Czosnyka, M., Kirkpatrick, P. J., Smielewski, P., Steiner, L. A., and Pickard, J. D. (2013). Clinical relevance of cerebral autoregulation following subarachnoid haemorrhage. Nat. Rev. Neurol. 9, 152–163. doi: 10.1038/nrneurol.2013.11
Butt, M. U., Zakaria, M., and Hussain, H. M. (2009). Circadian pattern of onset of ischaemic and haemorrhagic strokes, and their relation to sleep/wake cycle. J. Pak. Med. Assoc. 59, 129–132.
Cai, W., Liu, H., Zhao, J., Chen, L. Y., Chen, J., Lu, Z., et al. (2017). Pericytes in brain injury and repair after ischemic stroke. Transl. Stroke Res. 8, 107–121. doi: 10.1007/s12975-016-0504-4
Caputo, R., Poirel, V. J., Paiva, I., Boutillier, A. L., Challet, E., Meijer, J. H., et al. (2023). Circadian functioning of locus Cœruleus of the nocturnal rat and diurnal rodent Arvicanthis. Neurosci. Lett. 799:137091. doi: 10.1016/j.neulet.2023.137091
Castanon-Cervantes, O., Wu, M., Ehlen, J. C., Paul, K., Gamble, K. L., Johnson, R. L., et al. (2010). Dysregulation of inflammatory responses by chronic circadian disruption. J. Immunol. 185, 5796–5805. doi: 10.4049/jimmunol.1001026
Cauli, B., Tong, X. K., Rancillac, A., Serluca, N., Lambolez, B., Rossier, J., et al. (2004). Cortical GABA interneurons in neurovascular coupling: relays for subcortical vasoactive pathways. J. Neurosci. 24, 8940–8949. doi: 10.1523/JNEUROSCI.3065-04.2004
Chalmers, J. A., Martino, T. A., Tata, N., Ralph, M. R., Sole, M. J., and Belsham, D. D. (2008). Vascular circadian rhythms in a mouse vascular smooth muscle cell line (Movas-1). Am. J. Phys. Regul. Integr. Comp. Phys. 295, R1529–R1538. doi: 10.1152/ajpregu.90572.2008
Chaturvedi, S., Adams, H. P. Jr., and Woolson, R. F. (1999). Circadian variation in ischemic stroke subtypes. Stroke 30, 1792–1795. doi: 10.1161/01.STR.30.9.1792
Chen, Y. K., Liang, W. C., Yuan, S. L., Ni, Z. X., Li, W., Liu, Y. L., et al. (2022). Circadian rhythms of blood pressure in hypertensive patients with cerebral microbleeds. Brain Behav. 12:e2530. doi: 10.1002/brb3.2530
Cheng, M. Y., Bullock, C. M., Li, C., Lee, A. G., Bermak, J. C., Belluzzi, J., et al. (2002). Prokineticin 2 transmits the behavioural circadian rhythm of the suprachiasmatic nucleus. Nature 417, 405–410. doi: 10.1038/417405a
Choudhury, M. E., Miyanishi, K., Takeda, H., Islam, A., Matsuoka, N., Kubo, M., et al. (2020). Phagocytic elimination of synapses by microglia during sleep. Glia 68, 44–59. doi: 10.1002/glia.23698
Clarke, I. J. (2015). Hypothalamus as an endocrine organ. Compr. Physiol. 5, 217–253. doi: 10.1002/cphy.c140019
Comas, M., Gordon, C. J., Oliver, B. G., Stow, N. W., King, G., Sharma, P., et al. (2017). A circadian based inflammatory response–implications for respiratory disease and treatment. Sleep Sci. Pract. 1, 1–19. doi: 10.1186/s41606-017-0019-2
Conroy, D. A., Spielman, A. J., and Scott, R. Q. (2005). Daily rhythm of cerebral blood flow velocity. J. Circadian Rhythms 3, 1–11. doi: 10.1186/1740-3391-3-3
Cordon-Cardo, C., O’brien, J. P., Casals, D., Rittman-Grauer, L., Biedler, J. L., Melamed, M. R., et al. (1989). Multidrug-resistance gene (P-glycoprotein) is expressed by endothelial cells at blood-brain barrier sites. Proc. Natl. Acad. Sci. 86, 695–698. doi: 10.1073/pnas.86.2.695
Costa, E. D., Rezende, B. A., Cortes, S. F., and Lemos, V. S. (2016). Neuronal nitric oxide synthase in vascular physiology and diseases. Front. Physiol. 7:206. doi: 10.3389/fphys.2016.00206
Cox, K. H., and Takahashi, J. S. (2019). Circadian clock genes and the transcriptional architecture of the clock mechanism. J. Mol. Endocrinol. 63, R93–R102. doi: 10.1530/JME-19-0153
Crnko, S., Du Pré, B. C., Sluijter, J. P., and Van Laake, L. W. (2019). Circadian rhythms and the molecular clock in cardiovascular biology and disease. Nat. Rev. Cardiol. 16, 437–447. doi: 10.1038/s41569-019-0167-4
Daneman, R. (2012). The blood–brain barrier in health and disease. Ann. Neurol. 72, 648–672. doi: 10.1002/ana.23648
Daneman, R., Zhou, L., Kebede, A. A., and Barres, B. A. (2010). Pericytes are required for blood-brain barrier integrity during embryogenesis. Nature 468, 562–566. doi: 10.1038/nature09513
Davalos, D., Kyu Ryu, J., Merlini, M., Baeten, K. M., Le Moan, N., Petersen, M. A., et al. (2012). Fibrinogen-induced perivascular microglial clustering is required for the development of axonal damage in neuroinflammation. Nat. Commun. 3:1227. doi: 10.1038/ncomms2230
De Wouwer, M. V., Collen, D., and Conway, E. M. (2004). Thrombomodulin-protein C-EPCR system: integrated to regulate coagulation and inflammation. Arterioscler. Thromb. Vasc. Biol. 24, 1374–1383. doi: 10.1161/01.ATV.0000134298.25489.92
Denniff, M., Turrell, H. E., Vanezis, A., and Rodrigo, G. C. (2014). The time-of-day variation in vascular smooth muscle contractility depends on a nitric oxide signalling pathway. J. Mol. Cell. Cardiol. 66, 133–140. doi: 10.1016/j.yjmcc.2013.11.009
Ding, J. M., Chen, D., Weber, E. T., Faiman, L. E., Rea, M. A., and Gillette, M. U. (1994). Resetting the biological clock: mediation of nocturnal circadian shifts by glutamate and NO. Science 266, 1713–1717. doi: 10.1126/science.7527589
Douma, L. G., and Gumz, M. L. (2018). Circadian clock-mediated regulation of blood pressure. Free Radic. Biol. Med. 119, 108–114. doi: 10.1016/j.freeradbiomed.2017.11.024
Droste, D., Berger, W., Schuler, E., and Krauss, J. (1993). Middle cerebral artery blood flow velocity in healthy persons during wakefulness and sleep: a transcranial Doppler study. Sleep 16, 603–609.
Dudvarski Stankovic, N., Teodorczyk, M., Ploen, R., Zipp, F., and Schmidt, M. H. (2016). Microglia–blood vessel interactions: a double-edged sword in brain pathologies. Acta Neuropathol. 131, 347–363. doi: 10.1007/s00401-015-1524-y
Duffin, J., Mikulis, D. J., and Fisher, J. A. (2021). Control of cerebral blood flow by blood gases. Front. Physiol. 12:640075. doi: 10.3389/fphys.2021.640075
Earnest, D. J., Neuendorff, N., Coffman, J., Selvamani, A., and Sohrabji, F. (2016). Sex differences in the impact of shift work schedules on pathological outcomes in an animal model of ischemic stroke. Endocrinology 157, 2836–2843. doi: 10.1210/en.2016-1130
Ek, C. J., Dziegielewska, K. M., Habgood, M. D., and Saunders, N. R. (2012). Barriers in the developing brain and Neurotoxicology. Neurotoxicology 33, 586–604. doi: 10.1016/j.neuro.2011.12.009
Elliott, W. J. (1998). Circadian variation in the timing of stroke onset: a meta-analysis. Stroke 29, 992–996. doi: 10.1161/01.STR.29.5.992
Evans, J. A., and Davidson, A. J. (2013). “Chapter ten-health consequences of circadian disruption in humans and animal models” in Progress in molecular biology and translational science. ed. M. U. Gillette (Cambridge, MA: Academic Press)
Fabiani, M., Rypma, B., and Gratton, G. (2021). Aging and cerebrovascular health: structural, functional, cognitive, and methodological implications. Psychophysiology 58:e13842. doi: 10.1111/psyp.13842
Feeney, K. A., Hansen, L. L., Putker, M., Olivares-Yañez, C., Day, J., Eades, L. J., et al. (2016). Daily magnesium fluxes regulate cellular timekeeping and energy balance. Nature 532, 375–379. doi: 10.1038/nature17407
Feeney, J. F. Jr., and Watterson, R. L. (1946). The development of the vascular pattern within the walls of the central nervous system of the chick embryo. J. Morphol. 78, 231–303. doi: 10.1002/jmor.1050780205
Fleming, I. (2003). Brain in the brawn: The neuronal nitric oxide synthase as a regulator of myogenic tone. Circ Res 93, 586–588. doi: 10.1161/01.RES.0000095380.06622.D8
Fonken, L. K., Frank, M. G., Kitt, M. M., Barrientos, R. M., Watkins, L. R., and Maier, S. F. (2015). Microglia inflammatory responses are controlled by an intrinsic circadian clock. Brain Behav. Immun. 45, 171–179. doi: 10.1016/j.bbi.2014.11.009
Fonken, L. K., Weil, Z. M., and Nelson, R. J. (2013). Mice exposed to dim light at night exaggerate inflammatory responses to lipopolysaccharide. Brain Behav. Immun. 34, 159–163. doi: 10.1016/j.bbi.2013.08.011
Förstermann, U., and Münzel, T. (2006). Endothelial nitric oxide synthase in vascular disease. Circulation 113, 1708–1714. doi: 10.1161/CIRCULATIONAHA.105.602532
Foster, R. G. (2020). Sleep, circadian rhythms and health. Interface Focus 10:20190098. doi: 10.1098/rsfs.2019.0098
Geloso, M. C., and D'ambrosi, N. (2021). Microglial pruning: relevance for synaptic dysfunction in multiple sclerosis and related experimental models. Cells 10:686. doi: 10.3390/cells10030686
Gerber, A., Esnault, C., Aubert, G., Treisman, R., Pralong, F., and Schibler, U. (2013). Blood-borne circadian signal stimulates daily oscillations in actin dynamics and SRF activity. Cells 152, 492–503. doi: 10.1016/j.cell.2012.12.027
Gillette, M. U., and Reppert, S. M. (1987). The hypothalamic suprachiasmatic nuclei: circadian patterns of vasopressin secretion and neuronal activity in vitro. Brain Res. Bull. 19, 135–139. doi: 10.1016/0361-9230(87)90176-6
Giorgi, F. S., Galgani, A., Puglisi-Allegra, S., Limanaqi, F., Busceti, C. L., and Fornai, F. (2020). Locus Coeruleus and neurovascular unit: from its role in physiology to its potential role in Alzheimer’s disease pathogenesis. J. Neurosci. Res. 98, 2406–2434. doi: 10.1002/jnr.24718
Gizowski, C., and Bourque, C. W. (2020). Sodium regulates clock time and output via an excitatory GABAergic pathway. Nature 583, 421–424. doi: 10.1038/s41586-020-2471-x
Gizowski, C., Zaelzer, C., and Bourque, C. W. (2018). Activation of organum vasculosum neurones and water intake in mice by vasopressin neurones in the suprachiasmatic nucleus. J. Neuroendocrinol. 30:e12577. doi: 10.1111/jne.12577
Gómez-González, B., Hurtado-Alvarado, G., Esqueda-León, E., Santana-Miranda, R., Rojas-Zamorano, J., and Velázquez-Moctezuma, J. (2013). REM sleep loss and recovery regulates blood-brain barrier function. Curr. Neurovasc. Res. 10, 197–207. doi: 10.2174/15672026113109990002
Gooley, J. J., Lu, J., Chou, T. C., Scammell, T. E., and Saper, C. B. (2001). Melanopsin in cells of origin of the retinohypothalamic tract. Nat. Neurosci. 4:1165. doi: 10.1038/nn768
Gross, P. M., Weindl, A., and Knigge, K. M. (1987). Peering through the windows of the brain. J. Cereb. Blood Flow Metab. 7, 663–672. doi: 10.1038/jcbfm.1987.120
Guo, S., Wang, H., and Yin, Y. (2022). Microglia polarization from M1 to M2 in neurodegenerative diseases. Front. Aging Neurosci. 14:815347. doi: 10.3389/fnagi.2022.815347
Guzman-Ruiz, M. A., Guerrero-Vargas, N. N., Lagunes-Cruz, A., Gonzalez-Gonzalez, S., Garcia-Aviles, J. E., Hurtado-Alvarado, G., et al. (2023). Circadian modulation of microglial physiological processes and immune responses. Glia 71, 155–167. doi: 10.1002/glia.24261
Hablitz, L. M., Plá, V., Giannetto, M., Vinitsky, H. S., Stæger, F. F., Metcalfe, T., et al. (2020). Circadian control of brain glymphatic and lymphatic fluid flow. Nat. Commun. 11:4411. doi: 10.1038/s41467-020-18115-2
Hagioka, S., Takeda, Y., Zhang, S., Sato, T., and Morita, K. (2005). Effects of 7-nitroindazole and N-nitro-l-arginine methyl ester on changes in cerebral blood flow and nitric oxide production preceding development of hyperbaric oxygen-induced seizures in rats. Neurosci. Lett. 382, 206–210. doi: 10.1016/j.neulet.2005.01.006
Haj-Yasein, N. N., Vindedal, G. F., Eilert-Olsen, M., Gundersen, G. A., Skare, Ø., Laake, P., et al. (2011). Glial-conditional deletion of aquaporin-4 (Aqp4) reduces blood–brain water uptake and confers barrier function on perivascular astrocyte endfeet. Proc. Natl. Acad. Sci. 108, 17815–17820. doi: 10.1073/pnas.1110655108
Hall, C. N., Reynell, C., Gesslein, B., Hamilton, N. B., Mishra, A., Sutherland, B. A., et al. (2014). Capillary pericytes regulate cerebral blood flow in health and disease. Nature 508, 55–60. doi: 10.1038/nature13165
Hamel, E. (2006). Perivascular nerves and the regulation of cerebrovascular tone. J. Appl. Physiol. 1985, 1059–1064. doi: 10.1152/japplphysiol.00954.2005
Harris, G. (1948). Neural control of the pituitary gland. Physiol. Rev. 28, 139–179. doi: 10.1152/physrev.1948.28.2.139
Hastings, M. H., Brancaccio, M., Gonzalez-Aponte, M. F., and Herzog, E. D. (2023). Circadian rhythms and astrocytes: the good, the bad, and the ugly. Annu. Rev. Neurosci. 46, 123–143. doi: 10.1146/annurev-neuro-100322-112249
Hatori, M., Gronfier, C., Van Gelder, R. N., Bernstein, P. S., Carreras, J., Panda, S., et al. (2017). Global rise of potential health hazards caused by blue light-induced circadian disruption in modern aging societies. NPJ Aging Mech. Dis. 3:9. doi: 10.1038/s41514-017-0010-2
Hayashi, Y., Koyanagi, S., Kusunose, N., Okada, R., Wu, Z., Tozaki-Saitoh, H., et al. (2013). The intrinsic microglial molecular clock controls synaptic strength via the circadian expression of cathepsin S. Sci. Rep. 3:2744. doi: 10.1038/srep02744
He, J., Hsuchou, H., He, Y., Kastin, A. J., Wang, Y., and Pan, W. (2014). Sleep restriction impairs blood-brain barrier function. J. Neurosci. 34, 14697–14706. doi: 10.1523/JNEUROSCI.2111-14.2014
Hemmeryckx, B., Van Hove, C. E., Fransen, P., Emmerechts, J., Kauskot, A., Bult, H., et al. (2011). Progression of the prothrombotic state in aging Bmal1-deficient mice. Arterioscler. Thromb. Vasc. Biol. 31, 2552–2559. doi: 10.1161/ATVBAHA.111.229062
Hermida, R. C., Ayala, D. E., and Portaluppi, F. (2007). Circadian variation of blood pressure: the basis for the chronotherapy of hypertension. Adv. Drug Deliv. Rev. 59, 904–922. doi: 10.1016/j.addr.2006.08.003
Hill, R. A., Tong, L., Yuan, P., Murikinati, S., Gupta, S., and Grutzendler, J. (2015). Regional blood flow in the normal and ischemic brain is controlled by arteriolar smooth muscle cell contractility and not by capillary pericytes. Neuron 87, 95–110. doi: 10.1016/j.neuron.2015.06.001
Hodkinson, D. J., O’daly, O., Zunszain, P. A., Pariante, C. M., Lazurenko, V., Zelaya, F. O., et al. (2014). Circadian and homeostatic modulation of functional connectivity and regional cerebral blood flow in humans under normal entrained conditions. J. Cereb. Blood Flow Metab. 34, 1493–1499. doi: 10.1038/jcbfm.2014.109
Hoiland, R. L., Caldwell, H. G., Howe, C. A., Nowak-Flück, D., Stacey, B. S., Bailey, D. M., et al. (2020). Nitric oxide is fundamental to neurovascular coupling in humans. J. Physiol. 598, 4927–4939. doi: 10.1113/JP280162
Hosford, P. S., and Gourine, A. V. (2019). What is the key mediator of the neurovascular coupling response? Neurosci. Biobehav. Rev. 96, 174–181. doi: 10.1016/j.neubiorev.2018.11.011
Hudson, N., Celkova, L., Hopkins, A., Greene, C., Storti, F., Ozaki, E., et al. (2019). Dysregulated claudin-5 cycling in the inner retina causes retinal pigment epithelial cell atrophy. JCI insight 4:e130273. doi: 10.1172/jci.insight.130273
Iliff, J., Wang, M., Liao, Y., Plogg, B., Peng, W., Gundersen, G., et al. (2012, 2012). A paravascular pathway facilitates CSF flow through the brain parenchyma and the clearance of interstitial solutes, including amyloid β. Sci. Transl. Med. 4:147ra111. doi: 10.1126/scitranslmed.3003748
Imaizumi, T., Itaya, H., Fujita, K., Kudoh, D., Kudoh, S., Mori, K., et al. (2000). Expression of tumor necrosis factor-α in cultured human endothelial cells stimulated with lipopolysaccharide or interleukin-1α. Arterioscler. Thromb. Vasc. Biol. 20, 410–415. doi: 10.1161/01.ATV.20.2.410
Jafri, S. M., Vanrollins, M., Ozawa, T., Mammen, E. F., Goldberg, A. D., and Goldstein, S. (1992). Circadian variation in platelet function in healthy volunteers. Am. J. Cardiol. 69, 951–954. doi: 10.1016/0002-9149(92)90799-5
Janssen, B. J., Tyssen, C. M., Duindam, H., and Rietveld, W. J. (1994). Suprachiasmatic lesions eliminate 24-h blood pressure variability in rats. Physiol. Behav. 55, 307–311. doi: 10.1016/0031-9384(94)90138-4
Jolivel, V., Bicker, F., Binamé, F., Ploen, R., Keller, S., Gollan, R., et al. (2015). Perivascular microglia promote blood vessel disintegration in the ischemic penumbra. Acta Neuropathol. 129, 279–295. doi: 10.1007/s00401-014-1372-1
Kagerbauer, S. M., Martin, J., Schuster, T., Blobner, M., Kochs, E. F., and Landgraf, R. (2013). Plasma oxytocin and vasopressin do not predict neuropeptide concentrations in human cerebrospinal fluid. J. Neuroendocrinol. 25, 668–673. doi: 10.1111/jne.12038
Kalsbeek, A., Perreau-Lenz, S., and Buijs, R. M. (2006). A network of (autonomic) clock outputs. Chronobiol. Int. 23, 521–535. doi: 10.1080/07420520600651073
Kaplan, L., Chow, B. W., and Gu, C. (2020). Neuronal regulation of the blood–brain barrier and neurovascular coupling. Nat. Rev. Neurosci. 21, 416–432. doi: 10.1038/s41583-020-0322-2
Keaney, J., and Campbell, M. (2015). The dynamic blood-brain barrier. FEBS J. 282, 4067–4079. doi: 10.1111/febs.13412
Keskil, Z., Gorgun, C. Z., Hodoglugil, U., and Zengil, H. (1996). Twenty-four-hour variations in the sensitivity of rat aorta to vasoactive agents. Chronobiol. Int. 13, 465–475.
Kisler, K., Nelson, A. R., Montagne, A., and Zlokovic, B. V. (2017). Cerebral blood flow regulation and neurovascular dysfunction in Alzheimer disease. Nat. Rev. Neurosci. 18, 419–434. doi: 10.1038/nrn.2017.48
Kluft, C., Jie, A., Rijken, D., and Verheijen, J. (1988). Daytime fluctuations in blood of tissue-type plasminogen activator (t-PA) and its fast-acting inhibitor (PAI-1). Thromb. Haemost. 59, 329–332.
Kniesel, U., and Wolburg, H. (2000). Tight junctions of the blood–brain barrier. Cell. Mol. Neurobiol. 20, 57–76. doi: 10.1023/A:1006995910836
Koide, M., Bonev, A. D., Nelson, M. T., and Wellman, G. C. (2012). Inversion of neurovascular coupling by subarachnoid blood depends on large-conductance Ca2+−activated K+ (BK) channels. Proc. Natl. Acad. Sci. U. S. A. 109, E1387–E1395. doi: 10.1073/pnas.1121359109
Kotajima, F., Meadows, G. E., Morrell, M. J., and Corfield, D. R. (2005). Cerebral blood flow changes associated with fluctuations in alpha and theta rhythm during sleep onset in humans. J. Physiol. 568, 305–313. doi: 10.1113/jphysiol.2005.092577
Kraves, S., and Weitz, C. J. (2006). A role for cardiotrophin-like cytokine in the circadian control of mammalian locomotor activity. Nat. Neurosci. 9, 212–219. doi: 10.1038/nn1633
Kugler, E. C., Greenwood, J., and Macdonald, R. B. (2021). The “neuro-glial-vascular” unit: the role of glia in neurovascular unit formation and dysfunction. Front. Cell Develop. Biol. 9:732820. doi: 10.3389/fcell.2021.732820
Kunieda, T., Minamino, T., Miura, K., Katsuno, T., Tateno, K., Miyauchi, H., et al. (2008). Reduced nitric oxide causes age-associated impairment of circadian rhythmicity. Circ. Res. 102, 607–614. doi: 10.1161/CIRCRESAHA.107.162230
Kurihara, N., Alfie, M. E., Sigmon, D. H., Rhaleb, N.-E., Shesely, E. G., and Carretero, O. A. (1998). Role of nNOS in blood pressure regulation in eNOS null mutant mice. Hypertension 32, 856–861. doi: 10.1161/01.HYP.32.5.856
Kwon, D. (2022). Guardians of the brain: how a special immune system protects our grey matter. Nature 606, 22–24. doi: 10.1038/d41586-022-01502-8
Lemmer, B., and Arraj, M. (2008). Effect of NO synthase inhibition on cardiovascular circadian rhythms in wild-type and eNOS-knock-out mice. Chronobiol. Int. 25, 501–510. doi: 10.1080/07420520802257695
Li, D., Ma, S., Guo, D., Cheng, T., Li, H., Tian, Y., et al. (2016). Environmental circadian disruption worsens neurologic impairment and inhibits hippocampal neurogenesis in adult rats after traumatic brain injury. Cell. Mol. Neurobiol. 36, 1045–1055. doi: 10.1007/s10571-015-0295-2
Liebner, S., Dijkhuizen, R. M., Reiss, Y., Plate, K. H., Agalliu, D., and Constantin, G. (2018). Functional morphology of the blood-brain barrier in health and disease. Acta Neuropathol. 135, 311–336. doi: 10.1007/s00401-018-1815-1
Liu, J. A., Walton, J. C., Devries, A. C., and Nelson, R. J. (2021). Disruptions of circadian rhythms and thrombolytic therapy during ischemic stroke intervention. Front. Neurosci. 15:675732. doi: 10.3389/fnins.2021.766648
Liuzzo, J. P., Petanceska, S. S., Moscatelli, D., and Devi, L. A. (1999). Inflammatory mediators regulate cathepsin S in macrophages and microglia: a role in attenuating heparan sulfate interactions. Mol. Med. 5, 320–333. doi: 10.1007/BF03402068
Loesch, A., Milner, P., and Burnstock, G. (1998). Endothelin in perivascular nerves. An electronimmunocytochemical study of rat basilar artery. Neuroreport 9, 3903–3904. doi: 10.1097/00001756-199812010-00025
Lou, N., Takano, T., Pei, Y., Xavier, A. L., Goldman, S. A., and Nedergaard, M. (2016). Purinergic receptor P2RY12-dependent microglial closure of the injured blood–brain barrier. Proc. Natl. Acad. Sci. 113, 1074–1079. doi: 10.1073/pnas.1520398113
Louer, E. M. M., Günzel, D., Rosenthal, R., Carmone, C., Yi, G., Stunnenberg, H. G., et al. (2020). Differential day-night expression of tight junction components in murine retinal pigment epithelium. Exp. Eye Res. 193:107985. doi: 10.1016/j.exer.2020.107985
Lundgaard, I., Lu, M. L., Yang, E., Peng, W., Mestre, H., Hitomi, E., et al. (2017). Glymphatic clearance controls state-dependent changes in brain lactate concentration. J. Cereb. Blood Flow Metab. 37, 2112–2124. doi: 10.1177/0271678X16661202
Lunn, R. M., Blask, D. E., Coogan, A. N., Figueiro, M. G., Gorman, M. R., Hall, J. E., et al. (2017). Health consequences of electric lighting practices in the modern world: a report on the National Toxicology Program's workshop on shift work at night, artificial light at night, and circadian disruption. Sci. Total Environ. 607–608, 1073–1084. doi: 10.1016/j.scitotenv.2017.07.056
Ma, Y., Wang, J., Wang, Y., and Yang, G. Y. (2017). The biphasic function of microglia in ischemic stroke. Prog. Neurobiol. 157, 247–272. doi: 10.1016/j.pneurobio.2016.01.005
Madore, C., Yin, Z., Leibowitz, J., and Butovsky, O. (2020). Microglia, lifestyle stress, and neurodegeneration. Immunity 52, 222–240. doi: 10.1016/j.immuni.2019.12.003
Madsen, P. L., Holm, S., Vorstrup, S., Friberg, L., Lassen, N. A., and Wildschiødtz, G. (1991). Human regional cerebral blood flow during rapid-eye-movement sleep. J. Cereb. Blood Flow Metab. 11, 502–507. doi: 10.1038/jcbfm.1991.94
Manfredini, R., Boari, B., Smolensky, M. H., Salmi, R., La Cecilia, O., Maria Malagoni, A., et al. (2005). Circadian variation in stroke onset: identical temporal pattern in ischemic and hemorrhagic events. Chronobiol. Int. 22, 417–453. doi: 10.1081/CBI-200062927
Marpegan, L., Leone, M. J., Katz, M. E., Sobrero, P. M., Bekinstein, T. A., and Golombek, D. A. (2009). Diurnal variation in endotoxin-induced mortality in mice: correlation with proinflammatory factors. Chronobiol. Int. 26, 1430–1442. doi: 10.3109/07420520903408358
Mastrullo, V., Van Der Veen, D. R., Gupta, P., Matos, R. S., Johnston, J. D., Mcvey, J. H., et al. (2022). Pericytes' circadian clock affects endothelial Cells' synchronization and angiogenesis in a 3D tissue engineered scaffold. Front. Pharmacol. 13:867070. doi: 10.3389/fphar.2022.867070
Maywood, E. S., Chesham, J. E., O'Brien, J. A., and Hastings, M. H. (2011). A diversity of paracrine signals sustains molecular circadian cycling in suprachiasmatic nucleus circuits. Proc. Natl. Acad. Sci. 108, 14306–14311. doi: 10.1073/pnas.1101767108
Mcconnell, H. L., Kersch, C. N., Woltjer, R. L., and Neuwelt, E. A. (2017). The translational significance of the neurovascular unit. J. Biol. Chem. 292, 762–770. doi: 10.1074/jbc.R116.760215
Mckee, C. A., Lananna, B. V., and Musiek, E. S. (2020). Circadian regulation of astrocyte function: implications for Alzheimer's disease. Cell. Mol. Life Sci. 77, 1049–1058. doi: 10.1007/s00018-019-03314-y
Mckinley, M. J., Mathai, M. L., Mcallen, R. M., Mcclear, R. C., Miselis, R. R., Pennington, G. L., et al. (2004). Vasopressin secretion: osmotic and hormonal regulation by the lamina terminalis. J. Neuroendocrinol. 16, 340–347. doi: 10.1111/j.0953-8194.2004.01184.x
Millar-Craig, M., Bishop, C., and Raftery, E. (1978). Circadian variation of blood-pressure. Lancet 311, 795–797. doi: 10.1016/S0140-6736(78)92998-7
Miyata, S. (2015). New aspects in fenestrated capillary and tissue dynamics in the sensory circumventricular organs of adult brains. Front. Neurosci. 9:390. doi: 10.3389/fnins.2015.00390
Moskowitz, M. A., Lo, E. H., and Iadecola, C. (2010). The science of stroke: mechanisms in search of treatments. Neuron 67, 181–198. doi: 10.1016/j.neuron.2010.07.002
Mughal, A., Nelson, M. T., and Hill-Eubanks, D. (2023). The post-arteriole transitional zone: a specialized capillary region that regulates blood flow within the CNS microvasculature. J. Physiol. 601, 889–901. doi: 10.1113/JP282246
Musiek, E. S., and Holtzman, D. M. (2016). Mechanisms linking circadian clocks, sleep, and neurodegeneration. Science 354, 1004–1008. doi: 10.1126/science.aah4968
Nakazato, R., Hotta, S., Yamada, D., Kou, M., Nakamura, S., Takahata, Y., et al. (2017a). The intrinsic microglial clock system regulates interleukin-6 expression. Glia 65, 198–208. doi: 10.1002/glia.23087
Nakazato, R., Kawabe, K., Yamada, D., Ikeno, S., Mieda, M., Shimba, S., et al. (2017b). Disruption of Bmal1 impairs blood-brain barrier integrity via pericyte dysfunction. J. Neurosci. 37, 10052–10062. doi: 10.1523/JNEUROSCI.3639-16.2017
Nakazato, R., Takarada, T., Yamamoto, T., Hotta, S., Hinoi, E., and Yoneda, Y. (2011). Selective upregulation of Per1 mRNA expression by ATP through activation of P2X7 purinergic receptors expressed in microglial cells. J. Pharmacol. Sci. 116, 350–361. doi: 10.1254/jphs.11069FP
Naseri Kouzehgarani, G., Kandel, M. E., Sakakura, M., Dupaty, J. S., Popescu, G., and Gillette, M. U. (2022). Circadian volume changes in hippocampal glia studied by label-free interferometric imaging. Cells 11:2073. doi: 10.3390/cells11132073
Nielsen, A. N., and Lauritzen, M. (2001). Coupling and uncoupling of activity-dependent increases of neuronal activity and blood flow in rat somatosensory cortex. J. Physiol. 533, 773–785. doi: 10.1111/j.1469-7793.2001.00773.x
Nimmerjahn, A., Kirchhoff, F., and Helmchen, F. (2005). Resting microglial cells are highly dynamic surveillants of brain parenchyma in vivo. Science 308, 1314–1318. doi: 10.1126/science.1110647
Nonaka, H., Emoto, N., Ikeda, K., Fukuya, H., Rohman, M. S., Raharjo, S. B., et al. (2001). Angiotensin II induces circadian gene expression of clock genes in cultured vascular smooth muscle cells. Circulation 104, 1746–1748. doi: 10.1161/hc4001.098048
Oh-Oka, K., Kono, H., Ishimaru, K., Miyake, K., Kubota, T., Ogawa, H., et al. (2014). Expressions of tight junction proteins Occludin and Claudin-1 are under 634 the circadian control in the mouse large intestine: implications in intestinal permeability 635 and susceptibility to colitis. PLoS One 9:636. doi: 10.1371/journal.pone.0098016
Oldfield, B. J., and Mckinley, M. J. (2015). “Chapter 15- Circumventricular Organs” in The rat nervous system. ed. G. Paxinos. 4th ed (San Diego: Academic Press)
Orihuela, R., Mcpherson, C. A., and Harry, G. J. (2016). Microglial M1/M2 polarization and metabolic states. Br. J. Pharmacol. 173, 649–665. doi: 10.1111/bph.13139
Ousman, S. S., and Kubes, P. (2012). Immune surveillance in the central nervous system. Nat. Neurosci. 15, 1096–1101. doi: 10.1038/nn.3161
Pan, W., Wu, X., Kastin, A. J., Zhang, Y., Hsuchou, H., Halberg, F., et al. (2011). Potential protective role of IL15Rα during inflammation. J. Mol. Neurosci. 43, 412–423. doi: 10.1007/s12031-010-9459-1
Panda, S., Antoch, M. P., Miller, B. H., Su, A. I., Schook, A. B., Straume, M., et al. (2002). Coordinated transcription of key pathways in the mouse by the circadian clock. Cells 109, 307–320. doi: 10.1016/S0092-8674(02)00722-5
Pappas, A. C., Koide, M., and Wellman, G. C. (2015). Astrocyte Ca2+ signaling drives inversion of neurovascular coupling after subarachnoid hemorrhage. J. Neurosci. 35, 13375–13384. doi: 10.1523/JNEUROSCI.1551-15.2015
Park, Y. K., Kim, J. H., Choi, S. J., Kim, S. T., and Joo, E. Y. (2019). Altered regional cerebral blood flow associated with mood and sleep in shift workers: cerebral perfusion magnetic resonance imaging study. J. Clin. Neurol. 15, 438–447. doi: 10.3988/jcn.2019.15.4.438
Paschos, G. K., and Fitzgerald, G. A. (2010). Circadian clocks and vascular function. Circ. Res. 106, 833–841. doi: 10.1161/CIRCRESAHA.109.211706
Paulson, O. B., and Newman, E. A. (1987). Does the release of potassium from astrocyte endfeet regulate cerebral blood flow? Science 237, 896–898. doi: 10.1126/science.3616619
Peppiatt, C. M., Howarth, C., Mobbs, P., and Attwell, D. (2006). Bidirectional control of CNS capillary diameter by pericytes. Nature 443, 700–704. doi: 10.1038/nature05193
Peterson, E. C., Wang, Z., and Britz, G. (2011). Regulation of cerebral blood flow. J. Vasc. Med. 2011:823525. doi: 10.1155/2011/823525
Pulido, R. S., Munji, R. N., Chan, T. C., Quirk, C. R., Weiner, G. A., Weger, B. D., et al. (2020). Neuronal activity regulates blood-brain barrier efflux transport through endothelial circadian genes. Neuron 108:e7. doi: 10.1016/j.neuron.2020.09.002
Raivich, G. (2005). Like cops on the beat: the active role of resting microglia. Trends Neurosci. 28, 571–573. doi: 10.1016/j.tins.2005.09.001
Ralph, M. R., Foster, R. G., Davis, F. C., and Menaker, M. (1990). Transplanted suprachiasmatic nucleus determines circadian period. Science 247, 975–978. doi: 10.1126/science.2305266
Ramsey, A. M., Stowie, A., Castanon-Cervantes, O., and Davidson, A. J. (2020). Environmental circadian disruption increases stroke severity and dysregulates immune response. J. Biol. Rhythm. 35, 368–376. doi: 10.1177/0748730420929450
Rancillac, A., Rossier, J., Guille, M., Tong, X. K., Geoffroy, H., Amatore, C., et al. (2006). Glutamatergic control of microvascular tone by distinct GABA neurons in the cerebellum. J. Neurosci. 26, 6997–7006. doi: 10.1523/JNEUROSCI.5515-05.2006
Ripamonti, L., Riva, R., Maioli, F., Zenesini, C., and Procaccianti, G. (2017). Daily variation in the occurrence of different subtypes of stroke. Stroke Res. Treat 2017:9091250. doi: 10.1155/2017/9091250
Rojo, D., Badner, A., and Gibson, E. M. (2022). Circadian control of glial cell homeodynamics. J. Biol. Rhythm. 37, 593–608. doi: 10.1177/07487304221120966
Rosensweig, C., and Green, C. B. (2020). Periodicity, repression, and the molecular architecture of the mammalian circadian clock. Eur. J. Neurosci. 51, 139–165. doi: 10.1111/ejn.14254
Rudic, R. D., Mcnamara, P., Reilly, D., Grosser, T., Curtis, A.-M., Price, T. S., et al. (2005). Bioinformatic analysis of circadian gene oscillation in mouse aorta. Circulation 112, 2716–2724. doi: 10.1161/CIRCULATIONAHA.105.568626
Rustenhoven, J., and Kipnis, J. (2022). Brain borders at the central stage of neuroimmunology. Nature 612, 417–429. doi: 10.1038/s41586-022-05474-7
Saadoun, S., Tait, M., Reza, A., Davies, D. C., Bell, B., Verkman, A., et al. (2009). AQP4 gene deletion in mice does not alter blood–brain barrier integrity or brain morphology. Neuroscience 161, 764–772. doi: 10.1016/j.neuroscience.2009.03.069
Samson, W. K., and Ferguson, A. V. (2015). Exploring the OVLT: insight into a critically important window into the brain. Am. J. Physiol. Regul. Integr. Comp. Physiol. 309, R322–R323. doi: 10.1152/ajpregu.00305.2015
Sandoo, A., Veldhuijzen Van Zanten, J. J., Metsios, G. S., Carroll, D., and Kitas, G. D. (2010). The endothelium and its role in regulating vascular tone. Open Cardiovasc. Med. J. 4, 302–312. doi: 10.2174/1874192401004010302
Sano, H., Hayashi, H., Makino, M., Takezawa, H., Hirai, M., Saito, H., et al. (1995). Effects of suprachiasmatic lesions on circadian rhythms of blood pressure, heart rate and locomotor activity in the rat. Jpn. Circ. J. 59, 565–573. doi: 10.1253/jcj.59.565
Sawaya, R., and Ingvar, D. (1989). Cerebral blood flow and metabolism in sleep. Acta Neurol. Scand. 80, 481–491. doi: 10.1111/j.1600-0404.1989.tb03915.x
Schaeffer, S., and Iadecola, C. (2021). Revisiting the neurovascular unit. Nat. Neurosci. 24, 1198–1209. doi: 10.1038/s41593-021-00904-7
Scheer, F. A., Michelson, A. D., Frelinger Iii, A. L., Evoniuk, H., Kelly, E. E., Mccarthy, M., et al. (2011). The human endogenous circadian system causes greatest platelet activation during the biological morning independent of behaviors. PLoS One 6:e24549. doi: 10.1371/journal.pone.0024549
Scheer, F. A. J. L., and Shea, S. A. (2014). Human circadian system causes a morning peak in prothrombotic plasminogen activator inhibitor-1 (PAI-1) independent of the sleep/wake cycle. Blood 123, 590–593. doi: 10.1182/blood-2013-07-517060
Schinkel, A., Smit, J., Van Tellingen, M., Beijnen, J., Wagenaar, E., Van Deemter, L., et al. (1994). Disruption of the mouse mdr1a P-glycoprotein gene leads to a deficiency in the blood-brain barrier and to increased sensitivity to drugs. Cells 77, 491–502. doi: 10.1016/0092-8674(94)90212-7
Schoenhard, J. A., Smith, L. H., Painter, C. A., Eren, M., Johnson, C. H., and Vaughan, D. E. (2003). Regulation of the PAI-1 promoter by circadian clock components: differential activation by BMAL1 and BMAL2. J. Mol. Cell. Cardiol. 35, 473–481. doi: 10.1016/S0022-2828(03)00051-8
Schurhoff, N., and Toborek, M. (2023). Circadian rhythms in the blood-brain barrier: impact on neurological disorders and stress responses. Mol. Brain 16:5. doi: 10.1186/s13041-023-00997-0
Schwartz, W. J., Coleman, R. J., and Reppert, S. M. (1983). A daily vasopressin rhythm in rat cerebrospinal fluid. Brain Res. 263, 105–112. doi: 10.1016/0006-8993(83)91205-2
Schwarz, P. M., Kleinert, H., and FöRstermann, U. (1999). Potential functional significance of brain-type and muscle-type nitric oxide synthase I expressed in adventitia and media of rat aorta. Arterioscler. Thromb. Vasc. Biol. 19, 2584–2590. doi: 10.1161/01.ATV.19.11.2584
Seddon, M. D., Chowienczyk, P. J., Brett, S. E., Casadei, B., and Shah, A. M. (2008). Neuronal nitric oxide synthase regulates basal microvascular tone in humans in vivo. Circulation 117, 1991–1996. doi: 10.1161/CIRCULATIONAHA.107.744540
Sevenich, L., Bowman, R. L., Mason, S. D., Quail, D. F., Rapaport, F., Elie, B. T., et al. (2014). Analysis of tumour-and stroma-supplied proteolytic networks reveals a brain-metastasis-promoting role for cathepsin S. Nat. Cell Biol. 16, 876–888. doi: 10.1038/ncb3011
Shi, Y., Liu, X., Gebremedhin, D., Falck, J. R., Harder, D. R., and Koehler, R. C. (2008). Interaction of mechanisms involving epoxyeicosatrienoic acids, adenosine receptors, and metabotropic glutamate receptors in neurovascular coupling in rat whisker barrel cortex. J. Cereb. Blood Flow Metab. 28, 111–125. doi: 10.1038/sj.jcbfm.9600511
Shivshankar, P., Fekry, B., Eckel-Mahan, K., and Wetsel, R. A. (2020). Circadian clock and complement immune system—complementary control of physiology and pathology? Front. Cell. Infect. Microbiol. 10:418.
Silverman, A., and Petersen, N. H. (2022). Physiology, cerebral autoregulation, StatPearls Treasure Island, FL.
Spengler, M. L., Kuropatwinski, K. K., Comas, M., Gasparian, A. V., Fedtsova, N., Gleiberman, A. S., et al. (2012). Core circadian protein CLOCK is a positive regulator of NF-κB–mediated transcription. Proc. Natl. Acad. Sci. 109, E2457–E2465. doi: 10.1073/pnas.1206274109
Stackhouse, T. L., and Mishra, A. (2021). Neurovascular coupling in development and disease: focus on astrocytes. Front. Cell Devel. Biol. 9:702832. doi: 10.3389/fcell.2021.702832
Stephan, F. K., and Zucker, I. (1972). Circadian rhythms in drinking behavior and locomotor activity of rats are eliminated by hypothalamic lesions. Proc. Natl. Acad. Sci. 69, 1583–1586. doi: 10.1073/pnas.69.6.1583
Streuli, C. H. (2016). Integrins as architects of cell behavior. Mol. Biol. Cell 27, 2885–2888. doi: 10.1091/mbc.E15-06-0369
Streuli, C. H., and Meng, Q. J. (2019). Influence of the extracellular matrix on cell-intrinsic circadian clocks. J. Cell Sci. 132:jcs207498. doi: 10.1242/jcs.207498
Strong, L. H. (1964). The early embryonic pattern of internal vascularization of the mammalian cerebral cortex. J. Comp. Neurol. 123, 121–138. doi: 10.1002/cne.901230111
Sukhova, G. K., Zhang, Y., Pan, J. H., Wada, Y., Yamamoto, T., Naito, M., et al. (2003). Deficiency of cathepsin S reduces atherosclerosis in LDL receptor-deficient mice. J. Clin. Invest. 111, 897–906. doi: 10.1172/JCI200314915
Sweeney, M. D., Sagare, A. P., and Zlokovic, B. V. (2018). Blood–brain barrier breakdown in Alzheimer disease and other neurodegenerative disorders. Nat. Rev. Neurol. 14, 133–150. doi: 10.1038/nrneurol.2017.188
Takahashi, J. S. (2017). Transcriptional architecture of the mammalian circadian clock. Nat. Rev. Genet. 18, 164–179. doi: 10.1038/nrg.2016.150
Takayama, F., Hayashi, Y., Wu, Z., Liu, Y., and Nakanishi, H. (2016). Diurnal dynamic behavior of microglia in response to infected bacteria through the UDP-P2Y6 receptor system. Sci. Rep. 6, 1–10. doi: 10.1038/srep30006
Takeda, N., Maemura, K., Horie, S., Oishi, K., Imai, Y., Harada, T., et al. (2007). Thrombomodulin is a clock-controlled gene in vascular endothelial cells. J. Biol. Chem. 282, 32561–32567. doi: 10.1074/jbc.M705692200
Tata, M., Ruhrberg, C., and Fantin, A. (2015). Vascularisation of the central nervous system. Mech. Dev. 138, 26–36. doi: 10.1016/j.mod.2015.07.001
Taub, A., Carbajal, Y., Rimu, K., Holt, R., Yao, Y., Hernandez, A. L., et al. (2021). Arginine vasopressin-containing neurons of the suprachiasmatic nucleus project to CSF. eNeuro 8:ENEURO.0363-20.2021. doi: 10.1523/ENEURO.0363-20.2021
Thosar, S. S., Berman, A. M., Herzig, M. X., Mchill, A. W., Bowles, N. P., Swanson, C. M., et al. (2019). Circadian rhythm of vascular function in midlife adults. Arterioscler. Thromb. Vasc. Biol. 39, 1203–1211. doi: 10.1161/ATVBAHA.119.312682
Thosar, S. S., Butler, M. P., and Shea, S. A. (2018). Role of the circadian system in cardiovascular disease. J. Clin. Invest. 128, 2157–2167. doi: 10.1172/JCI80590
Tofler, G. H., Brezinski, D., Schafer, A. I., Czeisler, C. A., Rutherford, J. D., Willich, S. N., et al. (1987). Concurrent morning increase in platelet aggregability and the risk of myocardial infarction and sudden cardiac death. N. Engl. J. Med. 316, 1514–1518. doi: 10.1056/NEJM198706113162405
Trudel, E., and Bourque, C. W. (2010). Central clock excites vasopressin neurons by waking osmosensory afferents during late sleep. Nat. Neurosci. 13, 467–474. doi: 10.1038/nn.2503
Turin, T. C., Kita, Y., Rumana, N., Takashima, N., Ichikawa, M., Sugihara, H., et al. (2010). Diurnal variation in onset of hemorrhagic stroke is independent of risk factor status: Takashima stroke registry. Neuroepidemiology 34, 25–33. doi: 10.1159/000255463
Umpierre, A. D., and Wu, L. J. (2021). How microglia sense and regulate neuronal activity. Glia 69, 1637–1653. doi: 10.1002/glia.23961
Vizovišek, M., Fonović, M., and Turk, B. (2019). Cysteine cathepsins in extracellular matrix remodeling: extracellular matrix degradation and beyond. Matrix Biol. 75, 141–159. doi: 10.1016/j.matbio.2018.01.024
Wang, Z., Wang, L., Zhang, L., Liu, Q., Xue, Z., Cornélissen, G., et al. (1992). Circadian relations among cardiovascular variables of young adults. Chronobiologia 19, 111–120.
Wang, X. L., Wolff, S. E. C., Korpel, N., Milanova, I., Sandu, C., Rensen, P. C. N., et al. (2020). Deficiency of the circadian clock gene Bmal1 reduces microglial Immunometabolism. Front. Immunol. 11:586399. doi: 10.3389/fimmu.2020.586399
Wauschkuhn, C. A., Witte, K., Gorbey, S., Lemmer, B., and Schilling, L. (2005). Circadian periodicity of cerebral blood flow revealed by laser-Doppler flowmetry in awake rats: relation to blood pressure and activity. Am. J. Phys. Heart Circ. Phys. 289, H1662–H1668. doi: 10.1152/ajpheart.01242.2004
Welsh, D. K., Yoo, S. H., Liu, A. C., Takahashi, J. S., and Kay, S. A. (2004). Bioluminescence imaging of individual fibroblasts reveals persistent, independently phased circadian rhythms of clock gene expression. Curr. Biol. 14, 2289–2295. doi: 10.1016/j.cub.2004.11.057
Westgate, E. J., Cheng, Y., Reilly, D. F., Price, T. S., Walisser, J. A., Bradfield, C. A., et al. (2008). Genetic components of the circadian clock regulate thrombogenesis in vivo. Circulation 117, 2087–2095. doi: 10.1161/CIRCULATIONAHA.107.739227
Whiteus, C., Freitas, C., and Grutzendler, J. (2014). Perturbed neural activity disrupts cerebral angiogenesis during a postnatal critical period. Nature 505, 407–411. doi: 10.1038/nature12821
Winkler, E. A., Bell, R. D., and Zlokovic, B. V. (2011). Central nervous system pericytes in health and disease. Nat. Neurosci. 14, 1398–1405. doi: 10.1038/nn.2946
Witte, K., Hasenberg, T., Rueff, T., Hauptfleisch, S., Schilling, L., and Lemmer, B. (2001). Day-night variation in the in vitro contractility of aorta and mesenteric and renal arteries in transgenic hypertensive rats. Chronobiol. Int. 18, 665–681.
Witte, K., Schnecko, A., Buijs, R. M., Van Der Vliet, J., Scalbert, E., Delagrange, P., et al. (1998). Effects of Scn lesions on orcadian blood pressure rhythm in normotensive and transgenic hypertensive rats. Chronobiol. Int. 15, 135–145. doi: 10.1081/cbi-100106080
Wu, D. M., Kawamura, H., Sakagami, K., Kobayashi, M., and Puro, D. G. (2003). Cholinergic regulation of pericyte-containing retinal microvessels. Am. J. Phys. Heart Circ. Phys. 284, H2083–H2090. doi: 10.1152/ajpheart.01007.2002
Xie, Z., Su, W., Liu, S., Zhao, G., Esser, K., Schroder, E. A., et al. (2015). Smooth-muscle BMAL1 participates in blood pressure circadian rhythm regulation. J. Clin. Invest. 125, 324–336. doi: 10.1172/JCI76881
Xu, Y., Gong, C., Liao, J., Ge, Z., Tan, Y., Jiang, Y., et al. (2023). Absence of fluctuation and inverted circadian rhythm of blood pressure increase the risk of cognitive dysfunction in cerebral small vessel disease patients. BMC Neurol. 23:73. doi: 10.1186/s12883-023-03107-8
Yamazaki, S., and Takahashi, J. S. (2005). Real-time luminescence reporting of circadian gene expression in mammals. Methods Enzymol. 393, 288–301. doi: 10.1016/S0076-6879(05)93012-7
Yang, N., Williams, J., Pekovic-Vaughan, V., Wang, P., Olabi, S., Mcconnell, J., et al. (2017). Cellular mechano-environment regulates the mammary circadian clock. Nat. Commun. 8:14287. doi: 10.1038/ncomms14287
Yao, Y., Green, I. K., Taub, A. B., Tazebay, R., Lesauter, J., and Silver, R. (2023). Vasculature of the suprachiasmatic nucleus: pathways for diffusible output signals. J. Biol. Rhythms. 8:7487304231189537. doi: 10.1177/07487304231189537
Yao, Y., Taub, A. B. N., Lesauter, J., and Silver, R. (2021). Identification of the suprachiasmatic nucleus venous portal system in the mammalian brain. Nat. Commun. 12:5643. doi: 10.1038/s41467-021-25793-z
Yoshihara, M., Bandoh, K., and Marmarou, A. (1995). Cerebrovascular carbon dioxide reactivity assessed by intracranial pressure dynamics in severely head injured patients. J. Neurosurg. 82, 386–393. doi: 10.3171/jns.1995.82.3.0386
Zhang, S. L., Lahens, N. F., Yue, Z., Arnold, D. M., Pakstis, P. P., Schwarz, J. E., et al. (2021). A circadian clock regulates efflux by the blood-brain barrier in mice and human cells. Nat. Commun. 12:617. doi: 10.1038/s41467-020-20795-9
Zhao, X., Eyo, U. B., Murugan, M., and Wu, L. J. (2018). Microglial interactions with the neurovascular system in physiology and pathology. Dev. Neurobiol. 78, 604–617. doi: 10.1002/dneu.22576
Zhou, J., Kong, H., Hua, X., Xiao, M., Ding, J., and Hu, G. (2008). Altered blood–brain barrier integrity in adult aquaporin-4 knockout mice. Neuroreport 19, 1–5. doi: 10.1097/WNR.0b013e3282f2b4eb
Zimmerman, B., Rypma, B., Gratton, G., and Fabiani, M. (2021). Age-related changes in cerebrovascular health and their effects on neural function and cognition: a comprehensive review. Psychophysiology 58:e13796. doi: 10.1111/psyp.13796
Keywords: clock, blood–brain interface, neuroendothelial, tight junctions, circadian rhythm disruption
Citation: Mitchell JW and Gillette MU (2023) Development of circadian neurovascular function and its implications. Front. Neurosci. 17:1196606. doi: 10.3389/fnins.2023.1196606
Edited by:
Rae Silver, Columbia University, United StatesReviewed by:
Eric L. Bittman, University of Massachusetts Amherst, United StatesJihwan Myung, Taipei Medical University, Taiwan
Javier Stern, Georgia State University, United States
Copyright © 2023 Mitchell and Gillette. This is an open-access article distributed under the terms of the Creative Commons Attribution License (CC BY). The use, distribution or reproduction in other forums is permitted, provided the original author(s) and the copyright owner(s) are credited and that the original publication in this journal is cited, in accordance with accepted academic practice. No use, distribution or reproduction is permitted which does not comply with these terms.
*Correspondence: Martha U. Gillette, bWdpbGxldHRAaWxsaW5vaXMuZWR1