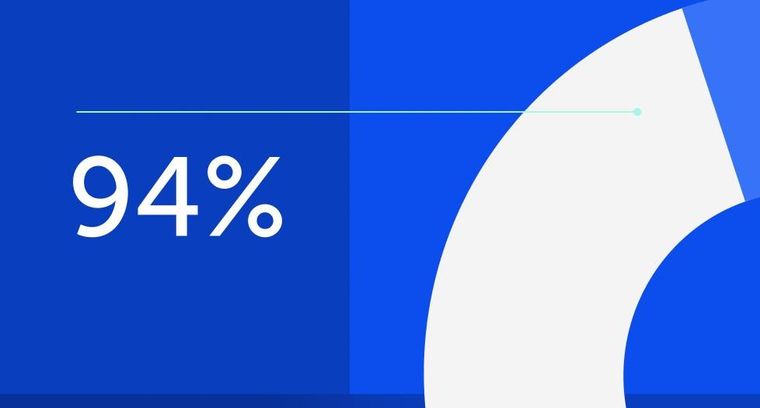
94% of researchers rate our articles as excellent or good
Learn more about the work of our research integrity team to safeguard the quality of each article we publish.
Find out more
ORIGINAL RESEARCH article
Front. Neurosci., 04 July 2023
Sec. Sleep and Circadian Rhythms
Volume 17 - 2023 | https://doi.org/10.3389/fnins.2023.1194996
Artificial light at night (ALAN) is a pervasive pollutant that alters physiology and behavior. However, the underlying mechanisms triggering these alterations are unknown, as previous work shows that dim levels of ALAN may have a masking effect, bypassing the central clock. Light stimulates neuronal activity in numerous brain regions which could in turn activate downstream effectors regulating physiological response. In the present study, taking advantage of immediate early gene (IEG) expression as a proxy for neuronal activity, we determined the brain regions activated in response to ALAN. We exposed zebra finches to dim ALAN (1.5 lux) and analyzed 24 regions throughout the brain. We found that the overall expression of two different IEGs, cFos and ZENK, in birds exposed to ALAN were significantly different from birds inactive at night. Additionally, we found that ALAN-exposed birds had significantly different IEG expression from birds inactive at night and active during the day in several brain areas associated with vision, movement, learning and memory, pain processing, and hormone regulation. These results give insight into the mechanistic pathways responding to ALAN that underlie downstream, well-documented behavioral and physiological changes.
A continued rise in global urbanization also increases artificial light at night (ALAN), with light pollution now recognized as a disruptive pollutant (Dominoni and Nelson, 2018). ALAN, even at dim levels, disrupts physiological and behavioral processes (Ouyang et al., 2018). However, these changes appear uncoupled from canonical circadian genes, which synchronize behavior and physiology to the natural photoperiod (Spoelstra et al., 2018; Alaasam et al., 2021), but (see Dominoni et al., 2020). Therefore, how dim ALAN affects neuronal activity to disrupt downstream physiological and behavioral processes remains unknown. This knowledge gap hinders our ability to predict and ameliorate responses to light pollution.
As ALAN disrupts hormone regulation, immune function, and nighttime activity, its effect could be linked to many corresponding brain regions (Alaasam et al., 2018; Mishra et al., 2019), especially if central circadian pacemakers are not disrupted. For example, ALAN disrupts melatonin and diurnal corticosterone production (Mishra et al., 2019), which are produced by the adrenal and pineal glands and directly regulate the hypothalamus, septum, and hippocampus (Chabot et al., 1998; El-Sherif et al., 2003; Kus et al., 2013; Zhang et al., 2017). ALAN also disrupts immune gene expression, neuronal survival, and plasticity in the hippocampus and caudal nidopallium (Mishra et al., 2019; Taufique et al., 2019; Moaraf et al., 2020; Namgyal et al., 2020). Lastly, ALAN recruits new neurons to the medial striatum, theorized to replace dying neurons (Moaraf et al., 2021).
Neuronal activity induces immediate early gene (IEG) expression for new protein synthesis (Greenberg et al., 1986; Flavell and Greenberg, 2008). Therefore, IEGs indicate neuronal activation by associating firing with gene expression and have successfully been used to map neuronal pathways (Guzowski et al., 2005; Feenders et al., 2008). IEGs, such as cFos and ZENK, have been shown to respond to different stimuli. cFos expression is stimulated by cAMP and calcium, and ZENK expression by injury, stress, etc. (Morgan and Curran, 1988; Sagar et al., 1988; O’Donovan et al., 1999). Using both IEGs can generate a holistic, detailed map of brain activity for a more representative analysis (Feenders et al., 2008; Nordmann et al., 2020).
We analyzed ALAN’s impact on IEG expression throughout the whole brain of Zebra finches (Taeniopygia guttata), an excellent diurnal model organism, as they translate external light similarly to most vertebrates (Durstewitz et al., 1999; Nakane and Yoshimura, 2014). Since ALAN initiates nighttime activity, we predicted activation in the visual and motor pathways, but that these areas would be similar to birds awake during the day. We also predicted, based on previous research, activation in areas involved in learning and memory, particularly the hippocampus, caudal nidopallium, and striatum (Taufique et al., 2019; Moaraf et al., 2021). We found that ALAN significantly altered IEG expression of cFos and ZENK in the hyperpallium, mesopallium, nidopallium, para-hippocampalis, striatum, entopallium, arcopallium, hippocampus, and septum compared to day and/or night birds.
Thirteen male zebra finches (~100 days old) were kept in outdoor aviaries at the University of Nevada, Reno with no previous exposure to ALAN. When they were ~ 140 days old, we moved them to individually housed indoor 47 cm × 31 cm × 36 cm cages and entrained them to 12 h light and 12 h dark (12 L,12D) for 4 weeks. For daylight, we used 1.4-Watt 5,000 K light emitting diode (LED) rated at 95 Lumens lights at 0:00 (zeitgeber time (ZT) 0) and lights off at 12:00 (ZT 12). Birds were given food and water ad libitum. Each cage contained a mechanized perch that relayed hop activity to MATLAB every minute. Cages had individual light-occlusion shades and constant white noise in the background to limit visual and acoustic cues.
We video recorded 30 min of behavior 90 min before perfusion and activity via automated perches (Alaasam et al., 2018). An observer blind to the treatments determined time spent eating/drinking, grooming, hopping, or no movement for the video recordings. We conducted a power analysis based on previously collected behavior data from control and ALAN exposed birds and determined that at least 3 birds were needed per treatment group (Power = 0.8, α = 0.05, effect size = 2, number of groups = 3).
Birds were randomly assigned to one of 3 conditions: control night (12 h light: 12 h dark; 12 L:12D sacrificed at dark night: ZT 14, n = 4), control day (12 L:12D sacrificed at day: ZT 10, n = 4), and experimental ALAN (12 h light: 12 h dim light; 12 L:12Ldim sacrificed at night with artificial light: ZT 14, n = 5). We chose the control day timepoint as close as possible to the night timepoints to be certain in capturing awake birds but also avoiding larger differences in circadian activity. As determined by One-Way ANOVA, groups did not differ in initial mass (p = 0.62). After the 4-week entertainment period, we sacrificed the control night group during the dark period (ZT 14) and the control day group during the light period (ZT 10). We sacrificed individuals in the ALAN group 2 h after the bird’s 1st exposure to ALAN (ZT 14), to obtain peak protein expression and avoid overlap from the light period. ALAN was standardized to around 1.5 lux ±0.01 from a 20 cm × 1.5 cm 5000 K broad spectrum LED strip. This was done with an Extech Easyview Digital Light Meter (model EA13) and lux was calculated using a mean measurement at perch height and two opposing base corners. For a full-spectrum description of the lights, please (see Alaasam et al., 2021).
We anesthetized birds with 0.1 ml of anesthesia made from 30 mg Ketamine HCl, 105 mg Xylazine, and 8.25 ml saline. After no response to a hard toe pinch, weight was taken, and we perfused birds with 1X PBS for 5 min and 4% paraformaldehyde in 1X PBS (PFA) for 13 min. Brains were removed, left in 4% PFA for 24 h, switched to a 15% sucrose solution for 4–12 h, followed by a 30% sucrose solution overnight, and then flash frozen with powdered dry ice and stored in −80°C until slicing.
We cut the left hemisphere of the brain sagittally at 45 μm thickness in six series. Series 1 was stained for imaging (total slices analyzed: ALAN = 90, control day = 67, control night = 67) and 2–6 were stored in cryoprotectant (3.3% sucrose, 0.01% Polyvinyl-pyrrolidone (PVP-40), 30% ethylene glycol in 0.1 M Phosphate Buffer) in −80°C.
We incubated brain slices in a blocking solution (4% BSA, 0.4% triton, 0.05% Na-Azide in 1x PBS) for 3 h and then with primary antibody diluted in blocking solution (c-Fos anti-rabbit polyclonal from ABCAM (ab190289) diluted 1:1000, ZENK anti-mouse monoclonal received from Dr. Keays’ lab (Nordmann et al., 2020) diluted 1:300) in 4°C for ~46 h. We washed slices 3 times in 1X PBS for 25 min each at room temp and incubated overnight at 4° C with secondary antibodies (anti-rabbit 488 (from ABCAM ab150081) diluted 1:1000 and anti-mouse 594 (from ABCAM ab150116) diluted 1:1000), protected from light. We then incubated slices with DAPI for 15–25 min at room temperature and washed them in 1X PBS 3 times. Slices were mounted with antifade mounting medium (VECTASHIELD®) on slides. We imaged tile scans of full slices within 1 week of mounting on a Leica TCS SP8 confocal microscope.
We analyzed images on ImageJ. We determined brain regions using anatomical locations with DAPI staining and a reference atlas from zebrafinchatlas.org. Cells were determined positive for cFos or ZENK if they were three times the mean brightness and overlapped with DAPI. We divided the number of positive IEG cells by the total DAPI cell count to determine expression percentage in representative areas measured over several slices.
We performed statistical analyses in R, version 4.1.2 (R Development Core Team, 2011). We ran generalized linear mixed-effect models to assess if IEG expression levels were affected by the treatment group as a fixed effect (lme4 package). Slice number and bird ID were included as random effects. We used a Kruskal-Wallis test to analyze the interaction of behaviors and treatment groups. We ran a correlation matrix for all brain regions in each treatment for both cFos and ZENK (Supplementary Figure S1).
All procedures were conducted in accordance with the National Institute of Health Ethical Use of Animals and approved by the University of Nevada, Reno Institutional Animal Care and Use Committee.
There was a significant difference in the interaction between behavior and treatment group (Kruskal-Wallis test: Grooming: chi-squared = 6.22, p = 0.05, Feeding: chi-squared = 11.51, p < 0.01, Hopping: chi-squared = 6.45, p = 0.04, Inactive: chi-squared = 7.20, p = 0.03). Hop activity measured via perch recordings also showed that birds had significantly lower nocturnal activity (control night) than daytime activity (control day) and nocturnal activity under ALAN (Kruskal-Wallis test: chi-squared = 6.47, p = 0.04, Figure 1).
Figure 1. Types of behavior 75 to 105 min before perfusion for birds exposed to ALAN and control birds collected during the day and night. A 30-min window of time 90 min before perfusion (75 to 105 min) was analyzed and broken down into four different behaviors: feeding (eating or drinking), grooming, hopping, and inactive. Shown are means ± 1 SE.
The ALAN group was significantly different from the control night (cFos p = 0.027, ZENK: p = 0.037) but not the control day (cFos: p = 0.17, ZENK: p = 0.66) when all 24 brain regions were analyzed together (Figure 2A). We broke down the analysis by looking into two major pathways—motor and visual—as well as additional areas. There was no significant difference between cFos and ZENK expression between the ALAN group and either control in all combined areas analyzed in the motor pathway (cFos-Day: p = 0.40, cFos-Night: p = 0.14, ZENK-Day: p = 0.72, ZENK-Night: p = 0.14). Similarly, we saw no significant difference for all areas analyzed in the visual pathway (cFos-Day: p = 0.08, cFos-Night: p = 0.07, ZENK-Day: p = 0.61, ZENK-Night: p = 0.11). However, individual areas in both pathways were significantly different (Table 1; Supplementary Figures S2, S3).
Figure 2. Immediate early gene expression of cFos and ZENK throughout the brain for birds exposed to ALAN, and control birds collected during subjective day and night. (A) Total cFos and ZENK expression, shown in percentages. Expression is significantly higher in the ALAN treatment group compared to the night controls but not the day controls. (B) cFos expression (percentage) comparing birds exposed to ALAN to control day and control night groups in three brain regions: posterior hyperpallium, anterior mesopallium dorsal, and entopallium. (C) ZENK expression (percentage) comparing birds exposed to ALAN to control day and control night groups in three brain regions: hippocampus, medial dorsal mesopallium, and entopallium. Displayed are representative brain regions from a priori hypotheses, please see Supplementary Figures S2, S3 for all brain regions. Shown are means ±1 SE. Significance stars: *p < 0.05, **p < 0.01, ***p < 0.001.
Table 1. Analysis of IEG expression, arranged alphabetically by region, for individual brain regions comparing control night and control day groups against birds exposed to ALAN.
To determine if the expression was based on activity, we reanalyzed expression with birds separated into only two groups of active (n = 7) or inactive (n = 6; total minutes of activity <1 min) 90 min before perfusion. Active birds included the control day group and non-active included the control night, with the ALAN group split between the two, based on activity. There was no significant difference in cFos or ZENK expression overall between active and non-active birds (cFos: z = 1.18, p = 0.24, ZENK: z = 1.70, p = 0.09). Additionally, there was no significant difference between active and non-active birds in the whole motor (cFos: z = 1.28, p = 0.20, ZENK: z = 1.81, p = 0.07) or visual (cFos: z = 0.65, p = 0.51, ZENK: z = 1.37, p = 0.17) pathways.
In the visual pathway, the ALAN group showed significantly higher cFos expression in the striatum adjacent to the core of the entopallium, posterior hyperpallium (Figure 2B), and ventral mesopallium adjacent to the core of the entopallium than the control night group, and significantly higher ZENK expression in the nidopallium adjacent to the core of the entopallium but lower in the core of the entopallium. The ALAN group also showed significantly higher cFos expression than the control day group in the striatum adjacent to the core of the entopallium and posterior hyperpallium areas (Table 1).
In the motor pathway, the ALAN group showed significantly higher cFos expression than the control night group in the anterior mesopallium dorsal (Figure 2B) and anterior mesopallium ventral regions and significantly higher ZENK expression in the anterior mesopallium dorsal and nidopallium caudolateral regions (Figure 3). The ALAN group also had significantly higher levels of cFos expression compared to the control day group in the anterior mesopallium dorsal and lateral int arcopallium and higher ZENK expression in the nidopallium caudolateral. However, the ALAN group had significantly lower ZENK expression in the anterior striatum, nidopallium adjacent to the basorostral nucleus, and ventral mesopallium adjacent to the basorostral nucleus (Table 1). There was no significant difference between active and non-active birds in the anterior mesopallium dorsal, nidopallium caudolateral, or nidopallium adjacent to the basorostral nucleus.
Figure 3. Brain slices with cFos and ZENK staining in the anterior mesopallium dorsal. (A) A sagittal slice of a representative zebra finch brain 1 mm from the center, showing the anterior mesopallium dorsal. Blue is DAPI, green is cFos, and red is ZENK expression. (B) Images from the anterior mesopallium dorsal of cFos, ZENK, and the overlay of both with DAPI for a bird exposed to ALAN, a bird collected during the day (control day), and a bird collected at night (control night).
The ALAN group also showed higher cFos expression in the area parahippocampalis, medial dorsal mesopallium, entopallium (Figure 2B), and lateral ventral mesopallium and higher expression of ZENK in the caudal striatum, medial dorsal mesopallium (Figure 2C), entopallium (Figure 2C), and lateral ventral mesopallium as compared to the control night group, but lower levels of ZENK expression in the hippocampus (Figure 2C) and septum. The ALAN group also showed higher levels of cFos expression in the entopallium and higher ZENK expression in the area para-hippocampalis, medial dorsal mesopallium, and entopallium as compared to the control day group (Table 1).
Although ALAN is a pervasive pollutant, the neuronal response remains unclear. We imaged IEG expression of 24 brain regions during the day, night, and ALAN exposure in birds and found various regions were significantly differentially activated among the treatment groups. Overall, ALAN-treated birds were more like control-day birds in total IEG expression. However, six brain regions differed among all three treatment groups: anterior mesopallium dorsal, entopallium, medial dorsal mesopallium, posterior hyperpallium, nidopallium caudolateral, and striatum adjacent to the core of the entopallium.
In the visual pathway, control night birds (LD sacrificed during the night) were significantly different from control day (LD sacrificed during the day) and ALAN birds (LLdim sacrificed during the night). These large differences are to be expected as LD control night birds were inactive. However, we still found two areas had significantly stronger cFos expression for ALAN birds than both control groups: posterior hyperpallium and striatum adjacent to the core of the entopallium. ALAN was a novel visual stimulus for the birds, likely employing a visual neuronal response.
The entopallium, the most prominent area to emerge, was significantly different from both controls and both IEGs. The entopallium is involved in visual pattern recognition (Watanabe et al., 2008, 2011). Surprisingly, we found that birds exposed to ALAN had different IEG expression in visual pathways compared to day controls. We see that even very dim levels of ALAN (around 1.5 lux) elicit a clear response in recognizing this visual input.
Out of the seven regions of the motor pathway analyzed, ALAN birds were significantly different from the day controls in either cFos or ZENK in six of them. However, when accounting for activity, the ALAN group remained significantly different with increased expression in the anterior mesopallium dorsal and nidopallium caudolateral and decreased in the nidopallium adjacent to the basorostral nucleus. Although the nidopallium caudolateral has additional functions, the anterior mesopallium dorsal and nidopallium adjacent to the basorostral nucleus are differentially activated under ALAN and not associated with hopping. These areas may be picking up movement we did not track, such as head turns and flapping wings, or associated with other functions we are unaware of.
We found birds exposed to ALAN were significantly different from both controls in areas associated with learning and memory. The ALAN group had significantly higher IEG expression than the day and night controls in the area para-hippocampalis and medial dorsal mesopallium, which are involved in spatial and object recognition and associative learning, respectively, (He et al., 2010; Damphousse et al., 2022). The ALAN birds also had significantly lower IEG expression than the night controls in the hippocampus, which is involved in spatial memory and learning (Bingman et al., 1990; Mayer et al., 2013). Dim ALAN dampens behavioral measures of learning and memory which have also been correlated with structural alterations in the hippocampus (Taufique et al., 2018, 2019; Liu et al., 2022). Lower nocturnal IEG expression in the hippocampus may partially explain why dim ALAN suppresses gene expression in the hippocampus (Taufique et al., 2018, 2019). It is believed that sleeping activates the hippocampus for memory consolidation (Klinzing et al., 2019). Indeed, we see higher IEG expression in our control night birds than day. A nocturnal suppression of hippocampal activity may impair memory consolidation and learning under ALAN.
ALAN treatment birds had significantly higher IEG expression in the nidopallium caudolateral than either of the controls. This aligns with previous research that has found dim ALAN alters the neuroarchitecture of the nidopallium caudolateral, the avian equivalent of the prefrontal cortex (Gunturkun, 2005; Gunturkun and Bugnyar, 2016; Taufique et al., 2019). The nidopallium caudolateral has been implemented in mimicking prefrontal area structures by having the same receptor architecture as the Brodmann Area 10 in humans, which is involved in many processes including reward and conflict, working memory, and pain (Herold et al., 2011; Peng et al., 2018). IEG activation in areas associated with memory support previous findings that ALAN impairs learning and memory (Liu et al., 2022). Additionally, the avian nidopallium caudolateral along with the entopallium have been shown to display attentional mechanisms (Johnston et al., 2017), implying an alert state in our ALAN exposed birds.
Another association to emerge was pain processing. Dim ALAN has been shown to alter pain reception in mice (Bumgarner et al., 2020). ALAN treatment birds had significantly higher activity in the caudal striatum from night controls and significantly higher activity in the nidopallium caudolateral from both controls. Although not much is known about the avian caudal striatum, this area is related to anxiety and pain in mice (Jin et al., 2020). Additionally, the nidopallium caudolateral has been associated with the Brodmann Area 10 in humans, also involved in pain reception (Herold et al., 2011; Peng et al., 2018).
Birds under ALAN had significantly decreased IEG expression compared to the control night birds in the septum and hippocampus, which directly regulate hormones leading to downstream physiological changes (Carreras et al., 1984). The hippocampal-septal pathway regulates hormones involved in stress and immune function including; corticotropin-releasing hormone (Vale et al., 1981; Nagarajan et al., 2017), thyrotropin-releasing hormone (Fabris et al., 1995; Ballard et al., 1996), and corticosterone (Garces et al., 1968; Nyakas et al., 1979).
Our results show that ALAN typically increases IEG expression in differentially activated areas compared to both controls. However, reduction of ZENK expression in the septum and hippocampus implies reduced neuronal activation in co-regulated functions—such as hormonal control. This is supported by previous research that ALAN alters hormone production (Ouyang et al., 2018; Moaraf et al., 2020).
In summary, through fine analyses of IEG expression, we found that initial ALAN exposure activates brain areas involved in vision, movement, learning and memory, pain processing, and hormone regulation, which may be differentially regulated under prolonged sleep loss or long-term exposure to ALAN. Additionally, first time exposure to ALAN at a different time in the night may produce differential responses from those we observed. Although ALAN may not be eliciting changes through circadian regulation, we still see substantial responses across brain areas that warrant further study. ALAN creates a unique brain state that is significantly different from day or nighttime brain activity. Dim light creates a novel environment, different from birds active in the day or sleeping at night, which produced widespread differential brain activity.
The datasets presented in this study can be found in online repositories. The names of the repository/repositories and accession number(s) can be found at: https://datadryad.org/stash/share/uIyGiK3KEm3Cng_fNAvg9m5pFE65ZJyG4kFOpBO5W3M.
The animal study was reviewed and approved by National Institute of Health Ethical Use of Animals.
CH, VA, and JO designed the experiments. CH, NC, and AC conducted the experiment and completed data analysis. SP and JO oversaw the project, provided training, and reviewed analyses. JO, NC, and AC provided funding. All authors contributed to and reviewed the writing.
JO is supported by NIH National Institutes of Health R15 ES030548. NC and AC were supported by the Nevada Undergraduate Research Award and AC by the NEXUS award through the Undergraduate Research Opportunity Program. Research reported in this publication used the Cellular and Molecular Imaging Core facility supported by the National Institute of General Medical Sciences of the National Institutes of Health under grant number P20 GM103650.
We thank Kerstyn Countryman for logistical help, Vladimir Pravosudov who shared equipment and expertise, Melanie Kimball for guidance, and three reviewers for their feedback on previous versions of the manuscript.
The authors declare that the research was conducted in the absence of any commercial or financial relationships that could be construed as a potential conflict of interest.
All claims expressed in this article are solely those of the authors and do not necessarily represent those of their affiliated organizations, or those of the publisher, the editors and the reviewers. Any product that may be evaluated in this article, or claim that may be made by its manufacturer, is not guaranteed or endorsed by the publisher.
The Supplementary material for this article can be found online at: https://www.frontiersin.org/articles/10.3389/fnins.2023.1194996/full#supplementary-material
Alaasam, V. J., Duncan, R., Casagrande, S., Davies, S., Sidher, A., Seymoure, B., et al. (2018). Light at night disrupts nocturnal rest and elevates glucocorticoids at cool color temperatures. J. Exper. Zool. A Ecol. Integr. Physiol. 329, 465–472. doi: 10.1002/jez.2168
Alaasam, V. J., Liu, X., Niu, Y., Habibian, J. S., Pieraut, S., Ferguson, B. S., et al. (2021). Effects of dim artificial light at night on locomotor activity, cardiovascular physiology, and circadian clock genes in a diurnal songbird. Environ. Pollut. 282:117036. doi: 10.1016/j.envpol.2021.117036
Ballard, T. M., Hunter, A. J., and Bennett, G. W. (1996). Effect of a thyrotrophin-releasing hormone analogue, RX77368, an AMPA-induced septal hippocampal lesioned rats in an operant delayed non-matching to position test. Psychopharmacology 127, 265–275. doi: 10.1007/BF02806002
Bingman, V., Ioale, P., Casini, G., and Bagnoli, P. (1990). The avian hippocampus: evidence for a role in the development of the homing pigeon navigational map. Behav. Neurosci. 104:906. doi: 10.1037/0735-7044.104.6.906
Bumgarner, J. R., Walker, W. H., Liu, J. A., Walton, J. C., and Nelson, R. J. (2020). Dim light at night exposure induces cold hyperalgesia and mechanical allodynia in male mice. Neuroscience 434, 111–119. doi: 10.1016/j.neuroscience.2020.03.022
Carreras, A., Robles, R., Ruiz, E., Ortega, E., Mendoza, C., and Osorio, C. (1984). Role of the septum on LH, FSH and prolactin secretion in male-rats. Brain Res. Bull. 13, 339–342. doi: 10.1016/0361-9230(84)90136-9
Chabot, V., Caldani, M., de Reviers, M. M., and Pelletier, J. (1998). Localization and quantification of melatonin receptors in the diencephalon and posterior telencephalon of the sheep brain. J. Pineal Res. 24, 50–57. doi: 10.1111/j.1600-079X.1998.tb00365.x
Damphousse, C. C., Miller, N., and Marrone, D. F. (2022). Dissociation of spatial and object memory in the hippocampal formation of Japanese quail. Iscience 25. doi: 10.1016/j.isci.2022.103805
Dominoni, D. M., de Jong, M., van Oers, K., O’Shaughnessy, P., Blackburn, G., Atema, E., et al. (2020). Artificial light at night leads to circadian disruption in a songbird: integrated evidence from behavioural, genomic and metabolomic data. bio Rxiv. doi: 10.1101/2020.12.18.423473
Dominoni, D. M., and Nelson, R. J. (2018). Artificial light at night as an environmental pollutant: an integrative approach across taxa, biological functions, and scientific disciplines. J. Exp. Zool. A Ecol. Integr. Physiol. 329, 387–393. doi: 10.1002/jez.2241
Durstewitz, D., Kroner, S., and Gunturkun, O. (1999). The dopaminergic innervation of the avian telencephalon. Prog. Neurobiol. 59, 161–195. doi: 10.1016/S0301-0082(98)00100-2
El-Sherif, Y., Tesoriero, J., Hogan, M. V., and Wieraszko, A. (2003). Melatonin regulates neuronal plasticity in the hippocampus. J. Neurosci. Res. 72, 454–460. doi: 10.1002/jnr.10605
Fabris, N., Mocchegiani, E., and Provinciali, M. (1995). Pituitary-thyroid AXIS and immune-system-a reciprocal neuroendocrine-immune interaction. Horm. Res. 43, 29–38. doi: 10.1159/000184234
Feenders, G., Liedvogel, M., Rivas, M., Zapka, M., Horita, H., Hara, E., et al. (2008). Molecular mapping of movement-associated areas in the avian brain: a motor theory for vocal learning origin. PLoS One 3:e1768. doi: 10.1371/journal.pone.0001768
Flavell, S. W., and Greenberg, M. E. (2008). Signaling mechanisms linking neuronal activity to gene expression and plasticity of the nervous system. Annu. Rev. Neurosci. 31, 563–590. doi: 10.1146/annurev.neuro.31.060407.125631
Garces, L. Y., Iturzaet, N. F., Richards, C., Drash, A., and Kenny, F. M. (1968). Effect of various stresses on cortisol secretion rate (CSR). J. Pediatr. 72:566.
Greenberg, M. E., Hermanowski, A. L., and Ziff, E. B. (1986). Effect of protein-synthesis inhibitors on growth-factor activation of c-FOS, c-MYC, and actin gene-transcription. Mol. Cell. Biol. 6, 1050–1057. doi: 10.1128/mcb.6.4.1050-1057.1986
Gunturkun, O. (2005). The avian 'prefrontal cortex' and cognition. Curr. Opin. Neurobiol. 15, 686–693. doi: 10.1016/j.conb.2005.10.003
Gunturkun, O., and Bugnyar, T. (2016). Cognition without cortex. Trends Cogn. Sci. 20, 291–303. doi: 10.1016/j.tics.2016.02.001
Guzowski, J. F., Timlin, J. A., Roysam, B., McNaughton, B. L., Worley, P. F., and Barnes, C. A. (2005). Mapping behaviorally relevant neural circuits with immediate-early gene expression. Curr. Opin. Neurobiol. 15, 599–606. doi: 10.1016/j.conb.2005.08.018
He, X. G., Xiao, L., and Sui, N. (2010). Effects of SCH23390 and spiperone administered into medial striatum and intermediate medial mesopallium on rewarding effects of morphine in day-old chicks. Eur. J. Pharmacol. 627, 136–141. doi: 10.1016/j.ejphar.2009.10.041
Herold, C., Palomero-Gallagher, N., Hellmann, B., Kröner, S., Theiss, C., Güntürkün, O., et al. (2011). The receptor architecture of the pigeons' nidopallium caudolaterale: an avian analogue to the mammalian prefrontal cortex. Brain Struct. Funct. 216, 239–254. doi: 10.1007/s00429-011-0301-5
Jin, Y., Meng, Q., Mei, L., Zhou, W., Zhu, X., Mao, Y., et al. (2020). A somatosensory cortex input to the caudal dorsolateral striatum controls comorbid anxiety in persistent pain. Pain 161, 416–428. doi: 10.1097/j.pain.0000000000001724
Johnston, M., Anderson, C., and Colombo, M. (2017). Neural correlates of sample-coding and reward-coding in the delay activity of neurons in the entopallium and nidopallium caudolaterale of pigeons (Columba livia). Behav. Brain Res. 317, 382–392. doi: 10.1016/j.bbr.2016.10.003
Klinzing, J. G., Niethard, N., and Born, J. (2019). Mechanisms of systems memory consolidation during sleep. Nat. Neurosci. 22, 1598–1610. doi: 10.1038/s41593-019-0467-3
Kus, M. A., Sarsilmaz, M., Karaca, O., Acar, T., Gülcen, B., Hismiogullari, A. A., et al. (2013). Effects of melatonin hormone on hippocampus in pinealectomized rats: an immunohistochemical and biochemical study. Neuroendocrinol. Lett. 34, 418–425.
Liu, Q., Wang, Z. X., Cao, J., Dong, Y. L., and Chen, Y. X. (2022). Dim blue light at night induces spatial memory impairment in mice by hippocampal neuroinflammation and oxidative stress. Antioxidants 11. doi: 10.3390/antiox11122415
Mayer, U., Watanabe, S., and Bischof, H. J. (2013). Spatial memory and the avian hippocampus: research in zebra finches. J. Physiol. 107, 2–12. doi: 10.1016/j.jphysparis.2012.05.002
Mishra, I., Knerr, R. M., Stewart, A. A., Payette, W. I., Richter, M. M., and Ashley, N. T. (2019). Light at night disrupts diel patterns of cytokine gene expression and endocrine profiles in zebra finch (Taeniopygia guttata). Sci. Rep. 9:15833. doi: 10.1038/s41598-019-51791-9
Moaraf, S., Heiblum, R., Okuliarová, M., Hefetz, A., Scharf, I., Zeman, M., et al. (2021). Evidence that artificial light at night induces structure-specific changes in brain plasticity in a diurnal bird. Biomol. Ther. 11. doi: 10.3390/biom11081069
Moaraf, S., Heiblum, R., Vistoropsky, Y., Okuliarová, M., Zeman, M., and Barnea, A. (2020). Artificial light at night increases recruitment of new neurons and differentially affects various brain regions in female Zebra finches. Int. J. Mol. Sci. 21. doi: 10.3390/ijms21176140
Moaraf, S., Vistoropsky, Y., Pozner, T., Heiblum, R., Okuliarová, M., Zeman, M., et al. (2020). Artificial light at night affects brain plasticity and melatonin in birds. Neurosci. Lett. 716:134639. doi: 10.1016/j.neulet.2019.134639
Morgan, J. I., and Curran, T. (1988). Calcium as a modulator of the immediate-early gene cascade in neurons. Cell Calcium 9, 303–311. doi: 10.1016/0143-4160(88)90011-5
Nagarajan, G., Jurkevich, A., Kang, S. W., and Kuenzel, W. J. (2017). Anatomical and functional implications of corticotrophin-releasing hormone neurones in a septal nucleus of the avian brain: an emphasis on glial-neuronal interaction via V1a receptors in vitro. J. Neuroendocrinol. 29. doi: 10.1111/jne.12494
Nakane, Y., and Yoshimura, T. (2014). Universality and diversity in the signal transduction pathway that regulates seasonal reproduction in vertebrates. Front. Neurosci. 8:115. doi: 10.3389/fnins.2014.00115
Namgyal, D., Chandan, K., Sultan, A., Aftab, M., Ali, S., Mehta, R., et al. (2020). Dim light at night induced neurodegeneration and ameliorative effect of curcumin. Cells 9. doi: 10.3390/cells9092093
Nordmann, G. C., Malkemper, E. P., Landler, L., Ushakova, L., Nimpf, S., Heinen, R., et al. (2020). A high sensitivity ZENK monoclonal antibody to map neuronal activity in Aves. Sci. Rep. 10:915. doi: 10.1038/s41598-020-57757-6
Nyakas, C., Kloet, E. R. D., and Bohus, B. (1979). Hippocampal function and putative corticosterone receptors-effect of septal-lesions. Neuroendocrinology 29, 301–312.
O’Donovan, K. J., Tourtellotte, W. G., Milbrandt, J., and Baraban, J. M. (1999). The EGR family of transcription-regulatory factors: progress at the interface of molecular and systems neuroscience. Trends Neurosci. 22, 167–173. doi: 10.1016/S0166-2236(98)01343-5
Ouyang, J. Q., Davies, S., and Dominoni, D. (2018). Hormonally mediated effects of artificial light at night on behavior and fitness: linking endocrine mechanisms with function. J. Exp. Biol. 221. doi: 10.1242/jeb.156893
Peng, K., Steele, S. C., Becerra, L., and Borsook, D. (2018). Brodmann area 10: collating, integrating and high level processing of nociception and pain. Prog. Neurobiol. 161, 1–22. doi: 10.1016/j.pneurobio.2017.11.004
Sagar, S. M., Sharp, F. R., and Curran, T. (1988). Expression of c-FOS protein in brain-metabolic mapping at the cellular-level. Science 240, 1328–1331. doi: 10.1126/science.3131879
Spoelstra, K., Verhagen, I., Meijer, D., and Visser, M. E. (2018). Artificial light at night shifts daily activity patterns but not the internal clock in the great tit (Parus major). Proc. R. Soc. B Biol. Sci. 285. doi: 10.1098/rspb.2017.2751
Taufique, S. K. T., Prabhat, A., and Kumar, V. (2018). Illuminated night alters hippocampal gene expressions and induces depressive-like responses in diurnal corvids. Eur. J. Neurosci. 48, 3005–3018. doi: 10.1111/ejn.14157
Taufique, S. T., Prabhat, A., and Kumar, V. (2019). Light at night affects hippocampal and nidopallial cytoarchitecture: implication for impairment of brain function in diurnal corvids. J. Exp. Zool. A Ecol. Integr. Physiol. 331, 149–156. doi: 10.1002/jez.2238
Vale, W., Spiess, J., Rivier, C., and Rivier, J. (1981). Characterization of a 41-residue ovine hypothalamic peptide that stimulates secretion of CORTICOTROPIN and BETA-endorphin. Science 213, 1394–1397. doi: 10.1126/science.6267699
Watanabe, S., Mayer, U., and Bischof, H. J. (2008). Pattern discrimination is affected by entopallial but not by hippocampal lesions in zebra finches. Behav. Brain Res. 190, 201–205. doi: 10.1016/j.bbr.2008.02.035
Watanabe, S., Mayer, U., and Bischof, H. J. (2011). Visual Wulst analyses “where” and entopallium analyses “what” in the zebra finch visual system. Behav. Brain Res. 222, 51–56. doi: 10.1016/j.bbr.2011.03.035
Keywords: cFos, ZENK, zebra finch, Taeniopygia guttata , light pollution
Citation: Hui CK, Chen N, Chakraborty A, Alaasam V, Pieraut S and Ouyang JQ (2023) Dim artificial light at night alters immediate early gene expression throughout the avian brain. Front. Neurosci. 17:1194996. doi: 10.3389/fnins.2023.1194996
Received: 27 March 2023; Accepted: 15 June 2023;
Published: 04 July 2023.
Edited by:
Andrew J. K. Phillips, Monash University, AustraliaReviewed by:
Jacqueline Van Der Meij, Radboud University, NetherlandsCopyright © 2023 Hui, Chen, Chakraborty, Alaasam, Pieraut and Ouyang. This is an open-access article distributed under the terms of the Creative Commons Attribution License (CC BY). The use, distribution or reproduction in other forums is permitted, provided the original author(s) and the copyright owner(s) are credited and that the original publication in this journal is cited, in accordance with accepted academic practice. No use, distribution or reproduction is permitted which does not comply with these terms.
*Correspondence: Cassandra K. Hui, Y2h1aUBuZXZhZGEudW5yLmVkdQ==
Disclaimer: All claims expressed in this article are solely those of the authors and do not necessarily represent those of their affiliated organizations, or those of the publisher, the editors and the reviewers. Any product that may be evaluated in this article or claim that may be made by its manufacturer is not guaranteed or endorsed by the publisher.
Research integrity at Frontiers
Learn more about the work of our research integrity team to safeguard the quality of each article we publish.