- 1Division of Cardiology, Department of Medicine, UCLA Cardiac Arrhythmia Center, Los Angeles, CA, United States
- 2Molecular, Cellular, and Integrative Physiology Interdepartmental Program, University of California, Los Angeles, Los Angeles, CA, United States
The meticulous control of cardiac sympathetic and parasympathetic tone regulates all facets of cardiac function. This precise calibration of cardiac efferent innervation is dependent on sensory information that is relayed from the heart to the central nervous system. The vagus nerve, which contains vagal cardiac afferent fibers, carries sensory information to the brainstem. Vagal afferent signaling has been predominantly shown to increase parasympathetic efferent response and vagal tone. However, cardiac vagal afferent signaling appears to change after cardiac injury, though much remains unknown. Even though subsequent cardiac autonomic imbalance is characterized by sympathoexcitation and parasympathetic dysfunction, it remains unclear if, and to what extent, vagal afferent dysfunction is involved in the development of vagal withdrawal. This review aims to summarize the current understanding of cardiac vagal afferent signaling under in health and in the setting of cardiovascular disease, especially after myocardial infarction, and to highlight the knowledge gaps that remain to be addressed.
Introduction
The ability to perceive and respond to sensory signals is essential for maintenance of physiological homeostasis, and therefore survival (Bonaz et al., 2021; Chen et al., 2021). The vagus nerve, also known as cranial nerve X, is fundamental to visceral sensory transduction and subsequent central integration. It plays an important role in interoception (Paintal, 1973; Khalsa et al., 2018; Prescott and Liberles, 2022), the process through which the nervous system can sense, interpret, and integrate signals from the body (Khalsa et al., 2018). In addition to afferent fibers, which predominate, the vagus nerve also contains parasympathetic efferent nerves in approximately an 80:20 ratio (Foley and Dubois, 1937). Named after the Latin word for “wandering,” vagal afferent fibers transmit sensory information from visceral organs of the cardio-respiratory, gastro-intestinal, endocrine, and immune systems to the central nervous system (Berthoud and Neuhuber, 2000). Upon central integration, specific efferent signals are formulated and transmitted via vagal efferent fibers to visceral organs.
Cardiac sensory information is transmitted to the brainstem via pseudounipolar vagal sensory neurons of the inferior (nodose) vagal ganglia (Berthoud and Neuhuber, 2000; Armour and Ardell, 2004). Of note, cardiac sensory information is also relayed through spinal afferent nerves, which synapse upon second-order neurons in the spinal cord (Armour and Ardell, 2004). Functionally, whereas excitation of vagal afferent neurons appears to predominantly evoke a parasympathetic efferent response (Aviado and Guevara Aviado, 2001; Campagna and Carter, 2003; Armour, 2004; Janig, 2006; Crystal and Salem, 2012), activation of the spinal afferent pathways elicit a primarily sympathetic reflex (Malliani and Montano, 2002; Wang et al., 2017). As such, these distinct afferent pathways contribute to the adjustment and fine-tuning of cardiac sympathetic and parasympathetic tone, which antagonistically and synergistically maintain cardiac homeostasis.
Upon cardiac injury, autonomic balance becomes disrupted, characterized by increased cardiac sympathetic tone and parasympathetic withdrawal (Florea and Cohn, 2014; Ardell and Armour, 2016; Van Weperen et al., 2023). These changes subsequently lead to further progression of cardiac disease, including heart failure, and increased susceptibility to ventricular arrhythmias after myocardial infarction, and therefore, sudden cardiac death (Vaseghi and Shivkumar, 2008; Ardell and Armour, 2016; Wu and Vaseghi, 2020). Recent data suggests that the decrease in parasympathetic efferent outflow can, at least in part, result from diminished vagal afferent signaling (Salavatian et al., 2022). Understanding the physiological role of cardiac vagal afferents, how cardiac injury alters their function, and how this ultimately translates to cardiac autonomic dysfunction is, therefore, of paramount importance.
Anatomy of the vagus nerve
Peripheral terminals of cardiac vagal afferents can be found across the different layers of the atrial and ventricular myocardium and the conduction system (Foreman, 1999; Zhao et al., 2022). From the heart and mediastinum, cardiac vagal sensory neurites merge into the bilateral vagi, which span the distance between the heart and the central nervous system.
On the cranial side, just inferior to the jugular foramen or, in some species including humans, within the jugular foramen, lies the nodose, or inferior, vagal ganglion. Given the location of the nodose ganglion in humans, it is more difficult to reach surgically (Settell et al., 2020; Prescott and Liberles, 2022).
In the nodose ganglion, the somata of all vagal afferent neurons are found, interspersed with glial cells, endothelial cells, and fibroblasts (Kupari et al., 2019). Interestingly, there is no viscerotopic organization to the nodose ganglia (Akgul Caglar et al., 2021), meaning that afferent neurons of specific visceral organs are not localized to a particular region in the ganglion. Cardiac vagal afferent neurons make up approximately 5–6% of all sensory afferent neurons,(Akgul Caglar et al., 2021; Zhao et al., 2022) and given lack of a spatial distribution, there is much interest in the identification of molecular markers that are specific for the cardiac phenotype.
Superior to the nodose ganglion lies the jugular ganglion, which mainly contains vagal afferent neurons innervating the auricular and meningeal branches of the vagus. These afferent neurons/nerves are of a developmentally different origin than the vagal afferents in the nodose (neurogenic placode vs. neural crest, respectively) (Moody and Lamantia, 2015). In most species, including humans, pigs, dogs and cats, the nodose and jugular ganglia are physically separated. However, in mice and rats, the jugular and nodose ganglia are often together in one structure, interchangeably termed the nodose or jugular(-petrosal)-nodose ganglion (Kollarik et al., 2019).
Once cardiac vagal afferent nerves have passed through the jugular foramen, they project to the nuclei tractus solitarius (NTS) (Lawrence and Jarrott, 1996). In contrast to the nodose ganglion, the NTS does appear to have some viscerotopic organization; the dorsal medial part being predominantly involved in cardiovascular reflexes (Lawrence and Jarrott, 1996). From the NTS, a subset of second order neurons project to the nucleus ambiguous and dorsal motor nucleus of the vagus, from where preganglionic efferent vagal neurons project to the periphery (Standish et al., 1994). Alternatively, second order neurons can also project to higher centers of the brain, for further integration of cardiac vagal afferent information (Neff et al., 1998).
Cardiac vagal afferent neurotransmission in health
In the healthy state, sensory information on the mechanical and chemical milieu of the heart, including the atria and ventricles, is continuously transmitted to the central nervous system through specialized mechano- and/or chemosensitive cardiac vagal neuron receptors (Hainsworth, 1991; Armour and Ardell, 2004). In the ventricles, there appears to be preferential distribution of vagal receptors on the infero-posterior left ventricular wall (Thames et al., 1978; Walker et al., 1978; Felder and Thames, 1979), whereas the highest densities of cardiac spinal afferents are found to be at the left anterior wall (Quigg et al., 1988; Quigg, 1991). Correspondingly, myocardial infarcts of the inferior left ventricular wall or application of epicardial afferent chemicals on the posterior wall elicit a primarily vagal response (Inoue and Zipes, 1987; Lombardi et al., 1996). In addition, various important reflexes are mediated through the vagus, including the Bainbridge and Bezold-Jarisch reflex (Aviado and Guevara Aviado, 2001; Campagna and Carter, 2003; Crystal and Salem, 2012), which are initiated with activation of vagal atrial or ventricular receptors, respectively. Most vagal afferent fibers seem to form flower spray or plate of puncta, parallel intramuscular arrays or end-net-like endings, based on data obtained from mice and rat aorta and atria (Cheng et al., 1997a,b; Zhao et al., 2022). Distinct from the atria, vagal afferents primarily form intramuscular array-like endings within the ventricles (Kazci et al., 2022).
Mechanosensitive cardiac vagal afferents are primarily myelinated Aδ fibers that have their endings within the epi- or endocardium (Quigg, 1991; Armour and Ardell, 2004; Shenton et al., 2021). These afferent neurons are specialized in the transduction of sensory information on myocardial mechanical deformation, generally discharging one or two impulses with each cardiac cycle. Depending on the subtype, ventricular mechanosensitive neurons are either activated by changes in ventricular filling (preload) or by increased ventricular pressure during systole (afterload) (Armour and Ardell, 2004). In the experimental setting, mechanoreceptors can be activated by increases in afterload (e.g., through aortic occlusion), or preload (e.g., by blood volume expansion) (Beaumont et al., 2013; Salavatian et al., 2022).
Important receptors for sensing visceral and cardiovascular mechanical stretch, which are also involved in the baroreflex, are the PIEZO1 and PIEZO2 channels (Zeng et al., 2018; Min et al., 2019). PIEZO2 receptors play an important role in arterial pressure sensing, as Cre-guided ablation of the glossopharyngeal and vagal PIEZO2 neurons eliminates the baroreflex (Min et al., 2019). These PIEZO2 afferent neurons appear to sense aortic pressure through their distinct terminal morphology of claw-like endings, which surround most of the aortic arch (Min et al., 2019). Recent studies have also reported a potential role of TRPC5, ASIC2, and Tentonin 3 (TMEM150c) channels in the baroreflex and sensing of changes in blood pressures in the aortic arch (Lu et al., 2009, 2020; Lau et al., 2016). TRPC5, a member of the transient receptor potential (TRP) family, is a cation channel that is activated with increases in hydrostatic pressure, hypoosmolarity, and membrane stretch (Gomis et al., 2008; Jemal et al., 2014; Shen et al., 2015). In transgenic Trpc5 knockout mice, baroreflex-induced heart rate changes are attenuated, and blood pressure fluctuates drastically throughout the day (Lau et al., 2016). Similarly, ASIC2, a member of the acid sensing ion channel family, is another mechanosensitive channel that is expressed by aortic baroreceptor afferent neurons (Lu et al., 2009). ASIC2 knockout mice develop hypertension and an impaired baroreflex through decreased vagal afferent sensing of aortic pressure, highlighting the role of this channel in autonomic control of blood pressure (Lu et al., 2009). Moreover, genetic deletion of tentonin 3, a cation channel that senses aortic stretch, also results in hypertension, tachycardia, and impaired baroreflex sensitivity (Lu et al., 2020). Interestingly, subsequent overexpression of tentonin 3 in these knock-out mice reverses the observed hypertension, underscoring the importance of this channel in sensing arterial pressure (Lu et al., 2020). As such, the expression of these channels can be used as a marker for mechanosensitive neurons.
Chemosensitive cardiac vagal afferents, on the other hand, are present in much greater numbers (Armour and Ardell, 2004). The majority of this population comprises of non-myelinated C fibers, which exhibit low basal activity, do not have a rhythmic discharge pattern that is synchronized with cardiac rhythm, and demonstrate a slow response upon activation (Armour and Ardell, 2004; Shenton et al., 2021).
Vagal chemosensitive afferent neurons are activated through binding of various chemicals, including bradykinin, prostaglandins, reactive oxygen species (Recordati et al., 1971; Robertson et al., 1985; Ustinova and Schultz, 1994). The majority of chemosensitive cardiac vagal afferents are transient receptor potential vanilloid-1 (TRPV1) positive C-type nociceptors (Porszasz et al., 1955; Bencsik et al., 2020; Szabados et al., 2020). This homotetrameric non-selective cation channel is best characterized for its activation through binding of capsaicin (Caterina and Julius, 2001), the main ingredient in chili peppers. However, it can be activated through a wide range of stimuli, including contact with nociceptive compounds and noxious heat (Caterina et al., 1997; Tominaga and Julius, 2000; Caterina and Julius, 2001). Moreover, several of such nociceptive compounds are produced and released upon tissue injury and ischemia, including H+, K+, bradykinin, reactive oxygen species, and prostaglandins (Nagy et al., 2004; Randhawa and Jaggi, 2015). TRPV1 activation results in Na+ and Ca2+ influx, inducing neuronal depolarization and the local release of neuropeptides, such as calcitonin gene-related peptide (CGRP) and substance P (Holzer, 1988; Maggi and Meli, 1988). Upon binding to their respective receptors, these peptides promote neurogenic inflammation (Holzer, 1988; Maggi and Meli, 1988) by inducing vasodilation, acute phase protein activation, and immune and inflammatory cell activation through paracrine signaling (Figure 1). Interestingly, whereas TRPV1 is expressed by both neurons that can release CGRP and substance P (peptidergic neurons) and non-peptidergic neurons in rats and humans (Tominaga et al., 1998; Price and Flores, 2007), TRPV1 expression is limited to peptidergic afferents in mice (Cavanaugh et al., 2011).
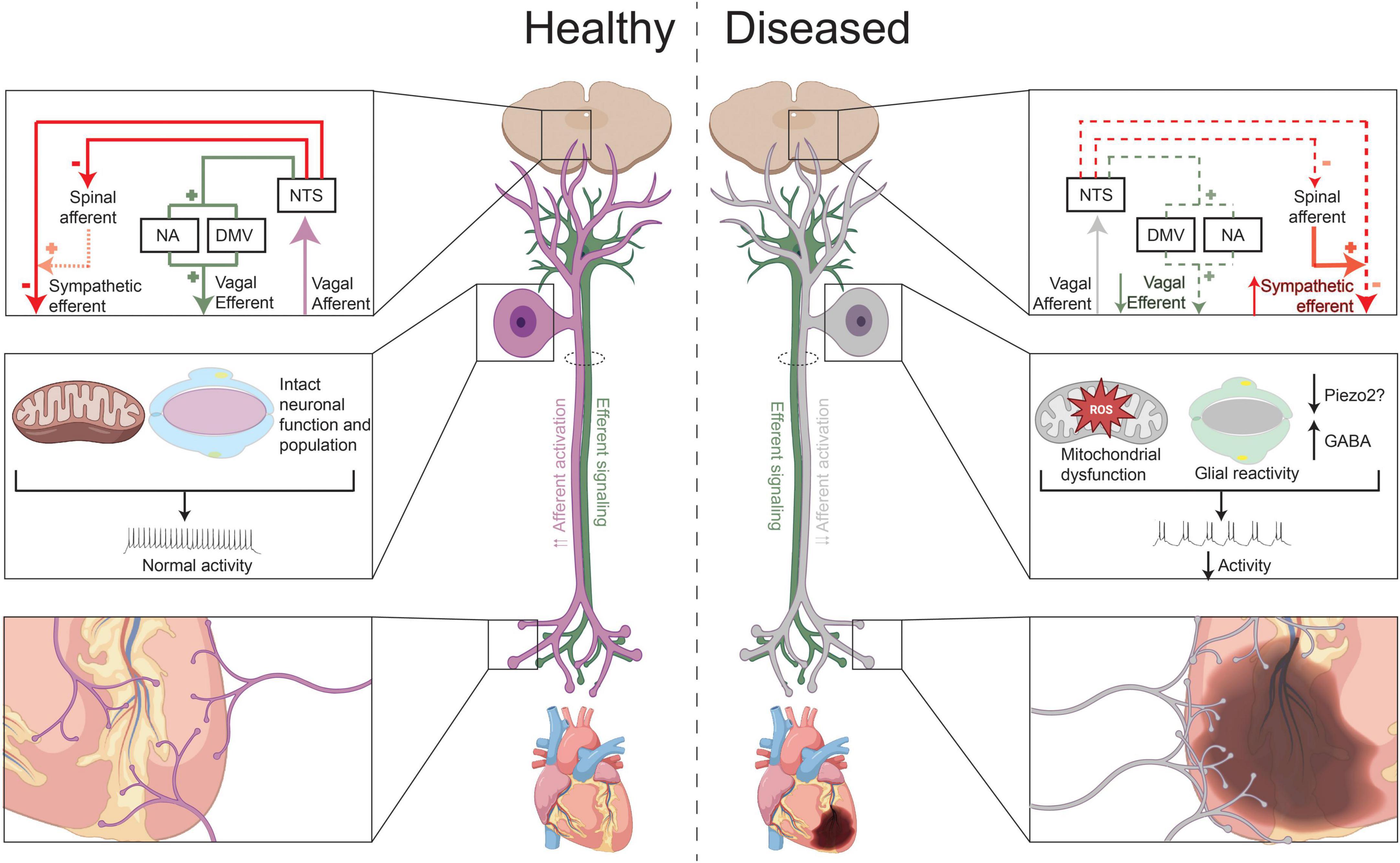
Figure 1. Vagal afferent transduction in health and after chronic myocardial injury. Under healthy conditions, release of neurochemicals that cause excitation of chemosensitive neurons, such as of TRPV1 + neurons, and activation of mechanosensitive afferent neurons (i.e., due to rises in ventricular or blood pressure), increase vagal efferent outflow to the heart and decrease sympathetic efferent activity. After chronic myocardial injury or infarction, vagal afferent transduction appears to be decreased. This is possibly due to increased expression and release of GABA after MI or decrease in PIEZO2 neurons (in setting of hypertension or ventricular hypertrophy), which can in turn, reduce activity of nociceptive and mechanosensitive neurons, respectively, development of metabolic dysfunction and oxidative in these neurons, and/or possibly due satellite glial cell reactivity. At the level of the heart, there is a pattern of vagal afferent denervation in the infarcted area, with vagal afferent nerve sprouting in the peri-infarct area and border zone regions. In the central nervous system, the functional decrease in vagal afferent signaling reduces vagal efferent outflow and indirectly increases cardiac sympathetic outflow.
Experimentally, TRPV1 expressing afferent neurons can be selectively “ablated” via the administration of high doses of capsaicin (>50 mg/kg) or resiniferatoxin (RTX) (Jancso et al., 1980; Wang et al., 2015; Teofilo et al., 2019). Chemical ablation of TRPV1 afferents has been successfully tested in both the clinical and pre-clinical setting, including as a therapy chronic pain and overactive bladder (Kissin and Szallasi, 2011).
In addition to chemosensitive and mechanosensitive neurons, a substantial number of cardiac vagal afferents are multimodal in nature (Salavatian et al., 2019), meaning that they can be activated by both mechanical distortion, as well as bradykinin, nicotine, and/or veratrum alkaloids (Thoren, 1977; Baker et al., 1980; Nerdrum et al., 1986; Armour et al., 1994; Thompson et al., 2002; Armour and Ardell, 2004). These afferents, similar to the mechanosensory neurons, are primarily Aδ fibers (Armour and Ardell, 2004).
Within the NTS, vagal afferents synapse upon second-order neurons, using glutamate as their primary neurotransmitter (Armour and Ardell, 2004). Thus, most vagal afferents express a vesicular glutamate transporter. Both vesicular glutamate 1 and 2 are expressed in the nodose ganglia (Corbett et al., 2005).
In the central nervous system, glutamate can bind to both N-methyl-D-aspartate (NMDA) and non-NMDA receptors, which induce different cardiovascular effects; activation of NMDA receptors causes bradycardia, whereas binding of glutamate to non-NMDA receptors on second-order neurons results in an increase in arterial pressure (Colombari et al., 1997). Other important neurotransmitters released by cardiac vagal afferent fibers include gamma-aminobutyric acid (GABA), nitric oxide (NO), and dopamine (Granata and Woodruff, 1982; Catelli et al., 1987; Lewis et al., 1991; Machado and Bonagamba, 1992).
Gamma-aminobutyric acid is another important neurotransmitter within the NTS, which is inhibitory and expressed by approximately 20% of vagal afferent neurons in the nodose ganglia (Stoyanova, 2004). Release of GABA in the NTS has been demonstrated to increase blood pressure (Catelli et al., 1987). NO is released by vagal afferent neurons that express either the neuronal and/or endothelial isoforms of nitric oxide synthase (nNOS or eNOS) (Lawrence et al., 1997; Yamamoto et al., 2003). Injection of NO into the NTS decreases arterial pressure and heart rate (Lewis et al., 1991; Machado and Bonagamba, 1992). Lastly, dopamine release in the NTS also increases systemic blood pressure and heart rate (Granata and Woodruff, 1982).
Finally, vagal afferent neurotransmission is also likely modulated by satellite glial cells (SGC) in the nodose ganglia. SGCs have been shown to envelop the cell bodies of neurons in the peripheral sensory ganglia and interact with neurons through gap junctions and buffering of neurotransmitters (Hanani and Spray, 2020). Moreover, in the nodose ganglion glutaminergic and GABAergic signaling between neurons and SGCs has been demonstrated to increase or decrease neuronal activity, respectively (Shoji et al., 2010). However, the extent to which SGC activity modulates vagal cardiac afferent signaling remains to be elucidated.
Cardiac vagal afferent neurotransmission in cardiovascular disease
Cardiac injury induces pathological changes in the autonomic nervous system, resulting in autonomic imbalance (Fukuda et al., 2015; Ardell and Armour, 2016; Van Weperen et al., 2023). The consequent sympathoexcitation and parasympathetic withdrawal lead to progression of heart failure and predispose to fatal ventricular arrhythmias, increasing the incidence of sudden death (Vaseghi and Shivkumar, 2008; Florea and Cohn, 2014; Shen and Zipes, 2014; Ardell and Armour, 2016; Wu and Vaseghi, 2020). It has been demonstrated that cardiac vagal efferent tone is reduced in the setting of cardiovascular disease, and that this, at least in part, is due to central vagal withdrawal manifesting as increased heart rate, altered responses to atropine, reduced heart rate variability and baroreflex sensitivity in humans and decreased vagal input to the post-ganglionic cardiac neurons of the intrinsic cardiac nervous system in large animal models of myocardial infarction (Eckberg et al., 1971; De Ferrari et al., 1991; Jouven et al., 2001; De Ferrari et al., 2007; Soliman et al., 2010; Libbus et al., 2016; Zhang et al., 2016; Vaseghi et al., 2017). Importantly, reduced vagal tone is a marker of mortality and ventricular arrhythmias (Jouven et al., 2001; De Ferrari et al., 2007; Soliman et al., 2010; Vaseghi et al., 2017). Recent data, however, suggests that these changes in parasympathetic efferent tone may at least in part, be due to reduced vagal afferent signaling (Salavatian et al., 2022).
The importance of intact vagal afferent signaling was highlighted in a study by Aydin et al. (2011) which showed that disturbed vagal afferent transduction, caused by ischemic lesions of the nodose ganglion, resulted in significant heart rhythm disturbances in all animals, and even death, in 30% of animals. The animals who died demonstrated more severe axonal degeneration of the vagus compared to the animals that survived) (Aydin et al., 2011). Moreover, under normal conditions, vagal cardiovascular afferent neural activity tonically inhibit sympathetic efferent neurotransmission through central pathways (Figure 1; Ammons et al., 1983; Ren et al., 1990; Sved et al., 2001). Impaired or decreased vagal afferent signaling can, therefore, result in increased cardiac sympathetic efferent outflow. Correspondingly, in the nucleus ambiguous and dorsal motor nucleus of the vagus, activity of cardiac vagal neurons (identified using retrograde tracers) is decreased after left ventricular hypertrophy induced heart failure, mediated by spontaneous inhibitory GABAergic neurotransmission (Cauley et al., 2015). Isolated electrical stimulation of vagal afferents (through esophageal electrical stimulation) on the other hand, decreases sympathetic and increases parasympathetic tone of the heart, as reflected by power spectral analyses (Bajwa et al., 1997).
Myocardial infarction
Nearly 850,000 people suffer from myocardial infarction (MI) each year in the United States (Tsao et al., 2022). Clinical and pre-clinical studies have detailed the resulting autonomic imbalance, with most focused on sympathetic (efferent) signaling (Chidsey et al., 1962; Ardell and Armour, 2016). Only a few anatomical studies have evaluated the effects of (chronic) ischemia on vagal afferent signaling. Barber et al. (1985) found that upon embolization of the first or second diagonal branch of the left anterior descending coronary artery, vagal afferent signaling distal from the lesion, as identified by efferent vagal responses, were diminished. Similarly, Inoue et al. (1988) found that cardiac ischemia interrupts spinal and vagal afferent signaling, but that the attenuation in afferent signaling can be reversed by reperfusion. Given that this study allowed for reperfusion after only 15 min of ischemia, it remains unknown if reperfusion would also rescue afferent nerves after longer periods of occlusion. Kazci et al. (2022) evaluated vagal afferent remodeling in a mouse model of chronic myocardial infarction. They observed vagal afferent denervation in the infarcted area, with vagal afferent nerve sprouting in the peri-infarct area and border zone regions. This reported pattern of hyperinnervation resembles those of sympathetic efferent fibers after MI, induced by a temporal increase in neuronal growth factor (Figure 1; Fukuda et al., 2015). Finally, a study by Ieda et al. (2006) showed that neuronal growth factor could rescue loss of cardiac spinal afferents in a diabetic mouse model. It is therefore, possible that neuronal growth factor could also induces reinnervation of vagal afferent fibers, though additional data is needed.
Functionally, Salavatian et al. (2022) were one of the first to study the effects of myocardial infarction on cardiac vagal afferent signaling in a large animal model. Using in vivo neural recordings from the nodose ganglia of pigs with chronic myocardial infarction, they observed an increase in the number of nociceptive neurons, but a paradoxical decrease in functional nociceptive signaling (Salavatian et al., 2022). More specifically, upon application of nociceptive chemicals on the heart, they observed a predominantly inhibitory response of vagal cardiac afferents, whereas the healthy control animals exhibited an excitatory response. This functional difference was associated a significantly greater number of CGRP-positive neurons that also expressed GABA in infarcted animals (Salavatian et al., 2022). Hence, this increase in GABA could, at least in part, be responsible for mediating the observed inhibitory responses. Other studies had previously shown that GABA can be released by nociceptive neurons (Du et al., 2017). GABA has been shown to not only reduce the activity of the releasing neuron, but to also act in a paracrine fashion to inhibit the function of nearby nociceptive neurons (Hanack et al., 2015). Therefore, the increased expression of GABA in vagal afferent nociceptive neurons could result in functionally lower activity (Du et al., 2017). Notably, this study did not observe any changes in quantity or quality of mechanosensitive neurons (PIEZO2) neurons, but did find an increase in glial fibrillary acidic protein (GFAP) expression, which could indicate increased SGC reactivity (Figure 1; Salavatian et al., 2022). However, the increase in glial fibrillary acidic protein (GFAP) expression was not selective for CGRP-expressing neurons.
Similarly, the effects of MI on parasympathetic efferent responses to vagal afferent activation were studied in a transgenic mouse model, in which glutamatergic vagal afferents could be activated through optogenetic stimulation (Lokhandwala et al., 2021). In this study, a decreased vagal efferent responses to similar levels of afferent activation were observed (Lokhandwala et al., 2021). It’s possible that in addition to a potential role for GABA, vagal dysfunction could be a result of myocardial infarction induced metabolic dysfunction in the vagal ganglia, similar to what has been observed in the central nervous system of patients with heart failure and myocardial ischemia (Fiechter et al., 2019; Bai et al., 2022). In rats with chronic heart failure, decreased baroreceptor sensitivity was associated with mitochondrial dysfunction in the nodose ganglia, leading to reduced activation of voltage-gated sodium channels in these baroreceptor neurons (Tu et al., 2012). This effect could be partially restored by in vivo transfection of manganese superoxide dismutase, which resulted in decreased mitochondria-derived superoxide levels in the nodose ganglia (Zhang et al., 2014). Therefore, mitochondrial dysfunction and oxidative stress may play an important role in the development and progression of vagal afferent dysfunction.
Since after myocardial infarction, cardiac vagal nociceptive signaling appears to be reduced, it’s possible that a subsequent nociceptive stimulus (i.e., additional ischemia) in the setting of post-myocardial infarction autonomic remodeling will result in reduced parasympathetic responses, potentially increasing the risk of ventricular arrhythmias and mortality. Premature ventricular contractions and cardiac ischemia, for example, can activate both chemosensitive and mechanosensitive vagal afferent neurons (Recordati et al., 1971; Robertson et al., 1985; Salavatian et al., 2019). In the setting of ischemia, various substances are released that excite these cardiac afferent neurons, including bradykinin, thromboxane A2, prostaglandins, free radicals, lactic acid, and adenosine triphosphate (ATP) (Ustinova and Schultz, 1994; Pan and Chen, 2004; Kumar et al., 2019). Whereas under healthy conditions, activation of these nociceptors would increase vagal afferent neuronal firing, and reflexively, increase parasympathetic efferent outflow, reduced signaling in the setting of a second ischemic insult can (Figure 1) can potentially face decreased vagal tone and predispose to life-threatening arrhythmias.
Hypertension
Chronic hypertension and consequent left ventricular hypertrophy are also associated with changes in cardiac autonomic remodeling. However, few studies have evaluated the role of vagal afferent neurotransmission in contributing to this sympathovagal imbalance. Huo et al. (2021) found a decrease in expression of PIEZO2 positive mechanosensitive neurons in the nodose ganglia of spontaneous hypertensive rats as well as in a hypertensive rat model. These results suggest diminished cardiac vagal afferent mechanotransduction in the setting of chronic hypertension (Figure 1).
Knowledge gaps
Even though data on vagal afferent (dys)function remains limited, vagal sensory neurotransmission appears to play a key role in regulating parasympathetic efferent outflow to the heart and in the development of cardiac autonomic dysfunction. Several aspects of cardiac vagal afferent neurotransmission in health and disease require further investigation, and significant knowledge gaps remain. Specific and sensitive markers of cardiac vagal afferent neurons are lacking. Most studies have used TRPV1, PIEZO1, PIEZO2, CGRP, VGLUT1, and/or VGLUT2 or a combination of these markers. However, as previously mentioned, these markers are neither specific nor sensitive for cardiac-specific sensory neurons. Recent studies have combined single cell RNA sequencing combined with cardiac tracing to specifically study the transcriptomic profile of cardiac vagal afferent neurons (Kupari et al., 2019; Zhao et al., 2022). However, this has yet to lead to the identification specific cardiac afferent neuronal molecular markers.
Multi-organ or nerve tracer studies have alluded to the presence of convergent neurons with dichotomizing afferents within the peripheral autonomic ganglia, which may receive sensory information from multiple visceral organs (Pierau et al., 1984; Christianson et al., 2007; Devarajan et al., 2022; Zhao et al., 2022). In the vagal ganglia, Devarajan et al. (2022) noted a rather large proportion of cardiac and respiratory neurons to be convergent or cardiorespiratory, as delineated via differential viral and dye tracer studies in the heart and lungs, suggesting that these neurons receive inputs from both organs. It is known that cardiorespiratory integration at the level of the brainstem is critical for regulation of heart rate changes associated with breathing. Nucleus ambiguus and dorsal motor nucleus of vagus receive inhibitory inputs from the pre-Bötzinger complex (the primary respiratory rhythm generator in mammals) and pulmonary stretch receptors via the NTS (Feher, 2012; Menuet et al., 2020). Given the presence of convergent neurons in the vagal ganglia, it’s possible that some integration of these reflexes occurs even before signaling reaches the brainstem. Examples of axonal convergence and divergence are widely present in the central nervous system (Man et al., 2013). Moreover, many pathologies that are initiated within one organ (system), such as a myocardial infarction, impact multiple organs (e.g., the respiratory system). As the vagus nerve harbors afferent fibers from several visceral organs, it is possible that multi-organ interactions may at least be partially “integrated” within the nodose sensory neurons. Elucidating how vagal neurons are involved in transducing and integrating multi-organ autonomic innervation could result in a better understanding of how multi-organ pathologies interact through their common autonomic innervation.
Few studies have assessed changes in vagal afferent dysfunction after cardiac injury. As this review has highlighted, the knowledge on altered vagal afferent signaling has been mainly derived from experimental studies looking at the changes induced by myocardial infarction or hypertension. However, the role of vagal afferent dysfunction in other cardiovascular disease, such as heart failure with preserved ejection fraction, are largely overlooked. Moreover, the molecular signals and cellular adaptations that underlie reduced vagal afferent signaling, as well as therapeutic approaches that can impede or prevent these changes, remain to be elucidated. While vagal nerve stimulation had been initially put forth as a promising neuromodulatory approach in preclinical and clinical studies of heart failure, it has been met with mixed results in large randomized clinical trials (Li et al., 2004; Schwartz and De Ferrari, 2009; Sabbah et al., 2011; Hamann et al., 2013; Premchand et al., 2014; Zannad et al., 2015; Gold et al., 2016). Studies have often over looked the fact that the vagus nerve is largely made up of afferent fibers, and effects of activating these fibers electrically remains unclear. A few large animal studies have suggested that electrical stimulation of the vagus does cause afferent fiber activation that seems to paradoxically reduce cardiac vagal efferent effects, though which subtypes of fibers are leading to this inhibition are unknown (Ardell et al., 2015; Yamakawa et al., 2015). Having a better understanding of the integrated effects of afferent and efferent vagal nerve stimulation might be beneficial in further improving and targeting this neuromodulatory therapy.
Lastly, SGC dysfunction and reactivity has been demonstrated to play a significant role in the development and progression of various neuropathic diseases, including chronic pain (Hanani and Spray, 2020). Even though SGCs have been shown to be present in the nodose ganglia, the extent to which they actively modulate vagal afferent signaling remains unknown. As SGCs can release TNFα and other excitatory cytokines (Zhang et al., 2007; Song et al., 2014), it’s possible that their activation may contributed to vagal afferent dysfunction.
Conclusion
Cardiac vagal afferent signaling is fundamental for maintenance of cardiac autonomic balance. After chronic cardiac injury, parasympathetic efferent withdrawal appears to be, at least in part, mediated through decreased cardiac vagal afferent signaling. However, compared to efferent signaling within the cardiac neuraxis, studies on parasympathetic afferent neurotransmission are still in their infancy. Additional data on the contribution of cardiac vagal afferent neurotransmission in health and in the setting of cardiovascular disease could provide key insights for the design of improved, targeted neuromodulatory therapies with reduced off-target effects.
Author contributions
Both authors contributed to the design, literature search and review, and drafting of the manuscript.
Funding
This work was supported by NIHR01HL148190 to MV.
Conflict of interest
MV has received educational consulting fees from Biosense Webster, Medtronic, Recor and has shares in NeuCures Inc.
The remaining author declares that the research was conducted in the absence of any commercial or financial relationships that could be construed as a potential conflict of interest.
Publisher’s note
All claims expressed in this article are solely those of the authors and do not necessarily represent those of their affiliated organizations, or those of the publisher, the editors and the reviewers. Any product that may be evaluated in this article, or claim that may be made by its manufacturer, is not guaranteed or endorsed by the publisher.
References
Akgul Caglar, T., Durdu, Z. B., Turhan, M. U., Gunal, M. Y., Aydin, M. S., Ozturk, G., et al. (2021). Evaluation of the bilateral cardiac afferent distribution at the spinal and vagal ganglia by retrograde labeling. Brain Res. 1751:147201. doi: 10.1016/j.brainres.2020.147201
Ammons, W. S., Blair, R. W., and Foreman, R. D. (1983). Vagal afferent inhibition of primate thoracic spinothalamic neurons. J. Neurophysiol. 50, 926–940. doi: 10.1152/jn.1983.50.4.926
Ardell, J. L., and Armour, J. A. (2016). Neurocardiology: Structure-based function. Compr. Physiol. 6, 1635–1653. doi: 10.1002/cphy.c150046
Ardell, J. L., Rajendran, P. S., Nier, H. A., Kenknight, B. H., and Armour, J. A. (2015). Central-peripheral neural network interactions evoked by vagus nerve stimulation: Functional consequences on control of cardiac function. Am. J. Physiol. Heart Circ. Physiol. 309, H1740–H1752. doi: 10.1152/ajpheart.00557.2015
Armour, J. A. (2004). Cardiac neuronal hierarchy in health and disease. Am. J. Physiol. Regul. Integr. Comp. Physiol. 287, R262–R271. doi: 10.1152/ajpregu.00183.2004
Armour, J. A., and Ardell, J. L. (2004). Basic and Clinical Neurocardiology. New York, NY: Oxford University Press.
Armour, J. A., Huang, M. H., Pelleg, A., and Sylven, C. (1994). Responsiveness of in situ canine nodose ganglion afferent neurones to epicardial mechanical or chemical stimuli. Cardiovasc. Res. 28, 1218–1225. doi: 10.1093/cvr/28.8.1218
Aviado, D. M., and Guevara Aviado, D. (2001). The Bezold-Jarisch reflex. A historical perspective of cardiopulmonary reflexes. Ann. N. Y. Acad. Sci. 940, 48–58.
Aydin, M. D., Kanat, A., Yilmaz, A., Cakir, M., Emet, M., Cakir, Z., et al. (2011). The role of ischemic neurodegeneration of the nodose ganglia on cardiac arrest after subarachnoid hemorrhage: An experimental study. Exp. Neurol. 230, 90–95. doi: 10.1016/j.expneurol.2010.09.018
Bai, Y., Yun, M., Nie, B., Shan, L., Liu, W., Hacker, M., et al. (2022). Neurometabolism and ventricular dyssynchrony in patients with heart failure and reduced ejection fraction. J. Am. Coll. Cardiol. 80, 1884–1896. doi: 10.1016/j.jacc.2022.08.801
Bajwa, A., Hollerbach, S., Kamath, M. V., Upton, A. R., Fitzpatrick, D., Fallen, E. L., et al. (1997). Neurocardiac response to esophageal electric stimulation in humans: Effects of varying stimulation frequencies. Am. J. Physiol. 272, R896–R901. doi: 10.1152/ajpregu.1997.272.3.R896
Baker, D. G., Coleridge, H. M., Coleridge, J. C., and Nerdrum, T. (1980). Search for a cardiac nociceptor: Stimulation by bradykinin of sympathetic afferent nerve endings in the heart of the cat. J. Physiol. 306, 519–536. doi: 10.1113/jphysiol.1980.sp013412
Barber, M. J., Mueller, T. M., Davies, B. G., Gill, R. M., and Zipes, D. P. (1985). Interruption of sympathetic and vagal-mediated afferent responses by transmural myocardial infarction. Circulation 72, 623–631. doi: 10.1161/01.cir.72.3.623
Beaumont, E., Salavatian, S., Southerland, E. M., Vinet, A., Jacquemet, V., Armour, J. A., et al. (2013). Network interactions within the canine intrinsic cardiac nervous system: Implications for reflex control of regional cardiac function. J. Physiol. 591, 4515–4533. doi: 10.1113/jphysiol.2013.259382
Bencsik, P., Gomori, K., Szabados, T., Santha, P., Helyes, Z., Jancso, G., et al. (2020). Myocardial ischaemia reperfusion injury and cardioprotection in the presence of sensory neuropathy: Therapeutic options. Br. J. Pharmacol. 177, 5336–5356. doi: 10.1111/bph.15021
Berthoud, H. R., and Neuhuber, W. L. (2000). Functional and chemical anatomy of the afferent vagal system. Auton. Neurosci. 85, 1–17. doi: 10.1016/S1566-0702(00)00215-0
Bonaz, B., Lane, R. D., Oshinsky, M. L., Kenny, P. J., Sinha, R., Mayer, E. A., et al. (2021). Diseases, disorders, and comorbidities of interoception. Trends Neurosci. 44, 39–51. doi: 10.1016/j.tins.2020.09.009
Campagna, J. A., and Carter, C. (2003). Clinical relevance of the Bezold-Jarisch reflex. Anesthesiology 98, 1250–1260. doi: 10.1097/00000542-200305000-00030
Catelli, J. M., Giakas, W. J., and Sved, A. F. (1987). GABAergic mechanisms in nucleus tractus solitarius alter blood pressure and vasopressin release. Brain Res. 403, 279–289. doi: 10.1016/0006-8993(87)90065-5
Caterina, M. J., and Julius, D. (2001). The vanilloid receptor: A molecular gateway to the pain pathway. Annu. Rev. Neurosci. 24, 487–517. doi: 10.1146/annurev.neuro.24.1.487
Caterina, M. J., Schumacher, M. A., Tominaga, M., Rosen, T. A., Levine, J. D., and Julius, D. (1997). The capsaicin receptor: A heat-activated ion channel in the pain pathway. Nature 389, 816–824. doi: 10.1038/39807
Cauley, E., Wang, X., Dyavanapalli, J., Sun, K., Garrott, K., Kuzmiak-Glancy, S., et al. (2015). Neurotransmission to parasympathetic cardiac vagal neurons in the brain stem is altered with left ventricular hypertrophy-induced heart failure. Am. J. Physiol. Heart Circ. Physiol. 309, H1281–H1287. doi: 10.1152/ajpheart.00445.2015
Cavanaugh, D. J., Chesler, A. T., Braz, J. M., Shah, N. M., Julius, D., and Basbaum, A. I. (2011). Restriction of transient receptor potential vanilloid-1 to the peptidergic subset of primary afferent neurons follows its developmental downregulation in nonpeptidergic neurons. J. Neurosci. 31, 10119–10127. doi: 10.1523/JNEUROSCI.1299-11.2011
Chen, W. G., Schloesser, D., Arensdorf, A. M., Simmons, J. M., Cui, C., Valentino, R., et al. (2021). The emerging science of interoception: Sensing, integrating, interpreting, and regulating signals within the self. Trends Neurosci. 44, 3–16. doi: 10.1016/j.tins.2020.10.007
Cheng, Z., Powley, T. L., Schwaber, J. S., and Doyle, F. J. III (1997a). A laser confocal microscopic study of vagal afferent innervation of rat aortic arch: Chemoreceptors as well as baroreceptors. J. Auton. Nerv. Syst. 67, 1–14. doi: 10.1016/s0165-1838(97)00085-4
Cheng, Z., Powley, T. L., Schwaber, J. S., and Doyle, F. J. III (1997b). Vagal afferent innervation of the atria of the rat heart reconstructed with confocal microscopy. J. Comp. Neurol. 381, 1–17. doi: 10.1002/(sici)1096-9861(19970428)381:1<1::aid-cne1>3.0.co;2-5
Chidsey, C. A., Harrison, D. C., and Braunwald, E. (1962). Augmentation of the plasma nor-epinephrine response to exercise in patients with congestive heart failure. N. Engl. J. Med. 267, 650–654. doi: 10.1056/NEJM196209272671305
Christianson, J. A., Liang, R., Ustinova, E. E., Davis, B. M., Fraser, M. O., and Pezzone, M. A. (2007). Convergence of bladder and colon sensory innervation occurs at the primary afferent level. Pain 128, 235–243. doi: 10.1016/j.pain.2006.09.023
Colombari, E., Bonagamba, L. G., and Machado, B. H. (1997). NMDA receptor antagonist blocks the bradycardic but not the pressor response to L-glutamate microinjected into the nucleus tractus solitarius (NTS) of unanesthetized rats. Brain Res. 749, 209–213. doi: 10.1016/S0006-8993(96)01169-9
Corbett, E. K., Sinfield, J. K., Mcwilliam, P. N., Deuchars, J., and Batten, T. F. (2005). Differential expression of vesicular glutamate transporters by vagal afferent terminals in rat nucleus of the solitary tract: Projections from the heart preferentially express vesicular glutamate transporter 1. Neuroscience 135, 133–145. doi: 10.1016/j.neuroscience.2005.06.010
Crystal, G. J., and Salem, M. R. (2012). The Bainbridge and the “reverse” Bainbridge reflexes: History, physiology, and clinical relevance. Anesth. Analg. 114, 520–532. doi: 10.1213/ANE.0b013e3182312e21
De Ferrari, G. M., Sanzo, A., Bertoletti, A., Specchia, G., Vanoli, E., and Schwartz, P. J. (2007). Baroreflex sensitivity predicts long-term cardiovascular mortality after myocardial infarction even in patients with preserved left ventricular function. J. Am. Coll. Cardiol. 50, 2285–2290. doi: 10.1016/j.jacc.2007.08.043
De Ferrari, G. M., Vanoli, E., Stramba-Badiale, M., Hull, S. S. Jr., Foreman, R. D., and Schwartz, P. J. (1991). Vagal reflexes and survival during acute myocardial ischemia in conscious dogs with healed myocardial infarction. Am. J. Physiol. 261, H63–H69. doi: 10.1152/ajpheart.1991.261.1.H63
Devarajan, A., Wang, K., Shannon, K., Su, Y., Verheyden, J., Sun, X., et al. (2022). Convergent cardiorespiratory neurons represent a significant portion of cardiac and respiratory neurons in the vagal ganglia. Front. Cardiovasc. Med. 9:959815. doi: 10.3389/fcvm.2022.959815
Du, X., Hao, H., Yang, Y., Huang, S., Wang, C., Gigout, S., et al. (2017). Local GABAergic signaling within sensory ganglia controls peripheral nociceptive transmission. J. Clin. Invest. 127, 1741–1756. doi: 10.1172/JCI86812
Eckberg, D. L., Drabinsky, M., and Braunwald, E. (1971). Defective cardiac parasympathetic control in patients with heart disease. N. Engl. J. Med. 285, 877–883. doi: 10.1056/NEJM197110142851602
Felder, R. B., and Thames, M. D. (1979). Interaction between cardiac receptors and sinoaortic baroreceptors in the control of efferent cardiac sympathetic nerve activity during myocardial ischemia in dogs. Circ. Res. 45, 728–736. doi: 10.1161/01.res.45.6.728
Fiechter, M., Roggo, A., Haider, A., Bengs, S., Burger, I. A., Maredziak, M., et al. (2019). Metabolic activity in central neural structures of patients with myocardial injury. J. Am. Heart Assoc. 8:e013070. doi: 10.1161/JAHA.119.013070
Florea, V. G., and Cohn, J. N. (2014). The autonomic nervous system and heart failure. Circ. Res. 114, 1815–1826. doi: 10.1161/CIRCRESAHA.114.302589
Foley, J. O., and Dubois, F. S. (1937). Quantitative studies of the vagus nerve in the cat. J. Comp. Neurol. 67, 49–67.
Foreman, R. D. (1999). Mechanisms of cardiac pain. Annu. Rev. Physiol. 61, 143–167. doi: 10.1146/annurev.physiol.61.1.143
Fukuda, K., Kanazawa, H., Aizawa, Y., Ardell, J. L., and Shivkumar, K. (2015). Cardiac innervation and sudden cardiac death. Circ. Res. 116, 2005–2019. doi: 10.1161/CIRCRESAHA.116.304679
Gold, M. R., Van Veldhuisen, D. J., Hauptman, P. J., Borggrefe, M., Kubo, S. H., Lieberman, R. A., et al. (2016). Vagus nerve stimulation for the treatment of heart failure: The INOVATE-HF Trial. J. Am. Coll. Cardiol. 68, 149–158. doi: 10.1016/j.jacc.2016.03.525
Gomis, A., Soriano, S., Belmonte, C., and Viana, F. (2008). Hypoosmotic- and pressure-induced membrane stretch activate TRPC5 channels. J. Physiol. 586, 5633–5649. doi: 10.1113/jphysiol.2008.161257
Granata, A. R., and Woodruff, G. N. (1982). Dopaminergic mechanisms in the nucleus tractus solitarius and effects on blood pressure. Brain Res. Bull. 8, 483–488. doi: 10.1016/0361-9230(82)90005-3
Hainsworth, R. (1991). Reflexes from the heart. Physiol. Rev. 71, 617–658. doi: 10.1152/physrev.1991.71.3.617
Hamann, J. J., Ruble, S. B., Stolen, C., Wang, M., Gupta, R. C., Rastogi, S., et al. (2013). Vagus nerve stimulation improves left ventricular function in a canine model of chronic heart failure. Eur. J. Heart Fail. 15, 1319–1326. doi: 10.1093/eurjhf/hft118
Hanack, C., Moroni, M., Lima, W. C., Wende, H., Kirchner, M., Adelfinger, L., et al. (2015). GABA blocks pathological but not acute TRPV1 pain signals. Cell 160, 759–770. doi: 10.1016/j.cell.2015.01.022
Hanani, M., and Spray, D. C. (2020). Emerging importance of satellite glia in nervous system function and dysfunction. Nat. Rev. Neurosci. 21, 485–498. doi: 10.1038/s41583-020-0333-z
Holzer, P. (1988). Local effector functions of capsaicin-sensitive sensory nerve endings: Involvement of tachykinins, calcitonin gene-related peptide and other neuropeptides. Neuroscience 24, 739–768. doi: 10.1016/0306-4522(88)90064-4
Huo, L., Gao, Y., Zhang, D., Wang, S., Han, Y., Men, H., et al. (2021). Piezo2 channel in nodose ganglia neurons is essential in controlling hypertension in a pathway regulated directly by Nedd4-2. Pharmacol. Res. 164:105391. doi: 10.1016/j.phrs.2020.105391
Ieda, M., Kanazawa, H., Ieda, Y., Kimura, K., Matsumura, K., Tomita, Y., et al. (2006). Nerve growth factor is critical for cardiac sensory innervation and rescues neuropathy in diabetic hearts. Circulation 114, 2351–2363. doi: 10.1161/CIRCULATIONAHA.106.627588
Inoue, H., Skale, B. T., and Zipes, D. P. (1988). Effects of ischemia on cardiac afferent sympathetic and vagal reflexes in dog. Am. J. Physiol. 255, H26–H35. doi: 10.1152/ajpheart.1988.255.1.H26
Inoue, H., and Zipes, D. P. (1987). Increased afferent vagal responses produced by epicardial application of nicotine on the canine posterior left ventricle. Am. Heart J. 114, 757–764. doi: 10.1016/0002-8703(87)90786-1
Jancso, G., Kiraly, E., and Jancso-Gabor, A. (1980). Direct evidence for an axonal site of action of capsaicin. Naunyn Schmiedebergs Arch. Pharmacol. 313, 91–94. doi: 10.1007/BF00505809
Janig, W. (2006). Integrative Action of the Autonomic Nervous System: Neurobiology of Homeostasis. Cambridge: Cambridge University Press.
Jemal, I., Soriano, S., Conte, A. L., Morenilla, C., and Gomis, A. (2014). G protein-coupled receptor signalling potentiates the osmo-mechanical activation of TRPC5 channels. Pflugers Arch. 466, 1635–1646. doi: 10.1007/s00424-013-1392-z
Jouven, X., Zureik, M., Desnos, M., Guerot, C., and Ducimetiere, P. (2001). Resting heart rate as a predictive risk factor for sudden death in middle-aged men. Cardiovasc. Res. 50, 373–378. doi: 10.1016/s0008-6363(01)00230-9
Kazci, Y. E., Sahoglu Goktas, S., Aydin, M. S., Karadogan, B., Nebol, A., Turhan, M. U., et al. (2022). Anatomical characterization of vagal nodose afferent innervation and ending morphologies at the murine heart using a transgenic approach. Auton. Neurosci. 242:103019. doi: 10.1016/j.autneu.2022.103019
Khalsa, S. S., Adolphs, R., Cameron, O. G., Critchley, H. D., Davenport, P. W., Feinstein, J. S., et al. (2018). Interoception and mental health: A roadmap. Biol. Psychiatry Cogn. Neurosci. Neuroimaging 3, 501–513. doi: 10.1016/j.bpsc.2017.12.004
Kissin, I., and Szallasi, A. (2011). Therapeutic targeting of TRPV1 by resiniferatoxin, from preclinical studies to clinical trials. Curr. Top. Med. Chem. 11, 2159–2170. doi: 10.2174/156802611796904924
Kollarik, M., Ru, F., and Undem, B. J. (2019). Phenotypic distinctions between the nodose and jugular TRPV1-positive vagal sensory neurons in the cynomolgus monkey. Neuroreport 30, 533–537. doi: 10.1097/WNR.0000000000001231
Kumar, A., Potts, J. D., and Dipette, D. J. (2019). Protective role of alpha-calcitonin gene-related peptide in cardiovascular diseases. Front. Physiol. 10:821. doi: 10.3389/fphys.2019.00821
Kupari, J., Haring, M., Agirre, E., Castelo-Branco, G., and Ernfors, P. (2019). An atlas of vagal sensory neurons and their molecular specialization. Cell Rep. 27:e2504. doi: 10.1016/j.celrep.2019.04.096
Lau, O. C., Shen, B., Wong, C. O., Tjong, Y. W., Lo, C. Y., Wang, H. C., et al. (2016). TRPC5 channels participate in pressure-sensing in aortic baroreceptors. Nat. Commun. 7:11947. doi: 10.1038/ncomms11947
Lawrence, A. J., and Jarrott, B. (1996). Neurochemical modulation of cardiovascular control in the nucleus tractus solitarius. Prog. Neurobiol. 48, 21–53. doi: 10.1016/0301-0082(95)00034-8
Lawrence, A. J., Krstew, E., and Jarrott, B. (1997). Complex interactions between nitric oxide and adenosine receptors in the rat isolated nodose ganglion. Eur. J. Pharmacol. 328, 83–88. doi: 10.1016/s0014-2999(97)83032-4
Lewis, S. J., Ohta, H., Machado, B., Bates, J. N., and Talman, W. T. (1991). Microinjection of S-nitrosocysteine into the nucleus tractus solitarii decreases arterial pressure and heart rate via activation of soluble guanylate cyclase. Eur. J. Pharmacol. 202, 135–136. doi: 10.1016/0014-2999(91)90269-v
Li, M., Zheng, C., Sato, T., Kawada, T., Sugimachi, M., and Sunagawa, K. (2004). Vagal nerve stimulation markedly improves long-term survival after chronic heart failure in rats. Circulation 109, 120–124. doi: 10.1161/01.CIR.0000105721.71640.DA
Libbus, I., Nearing, B. D., Amurthur, B., Kenknight, B. H., and Verrier, R. L. (2016). Autonomic regulation therapy suppresses quantitative T-wave alternans and improves baroreflex sensitivity in patients with heart failure enrolled in the ANTHEM-HF study. Heart Rhythm. 13, 721–728. doi: 10.1016/j.hrthm.2015.11.030
Lokhandwala, Z. A., Devarajan, A., Wang, K., Shannon, K., Tompkins, J. D., and Vaseghi, M. (2021). Abstract 14090: Myocardial infarction selectively decreases cardiac vagal afferent neurotransmission: Implications for mechanisms behind cardiac parasympathetic dysfunction. Circulation 144:A14090.
Lombardi, F., Sandrone, G., Spinnler, M. T., Torzillo, D., Lavezzaro, G. C., Brusca, A., et al. (1996). Heart rate variability in the early hours of an acute myocardial infarction. Am. J. Cardiol. 77, 1037–1044. doi: 10.1016/s0002-9149(96)00127-0
Lu, H. J., Nguyen, T. L., Hong, G. S., Pak, S., Kim, H., Kim, H., et al. (2020). Tentonin 3/TMEM150C senses blood pressure changes in the aortic arch. J. Clin. Invest. 130, 3671–3683. doi: 10.1172/JCI133798
Lu, Y., Ma, X., Sabharwal, R., Snitsarev, V., Morgan, D., Rahmouni, K., et al. (2009). The ion channel ASIC2 is required for baroreceptor and autonomic control of the circulation. Neuron 64, 885–897. doi: 10.1016/j.neuron.2009.11.007
Machado, B. H., and Bonagamba, L. G. (1992). Microinjection of S-nitrosocysteine into the nucleus tractus solitarii of conscious rats decreases arterial pressure but L-glutamate does not. Eur. J. Pharmacol. 221, 179–182. doi: 10.1016/0014-2999(92)90791-2
Maggi, C. A., and Meli, A. (1988). The sensory-efferent function of capsaicin-sensitive sensory neurons. Gen. Pharmacol. 19, 1–43. doi: 10.1016/0306-3623(88)90002-x
Malliani, A., and Montano, N. (2002). Emerging excitatory role of cardiovascular sympathetic afferents in pathophysiological conditions. Hypertension 39, 63–68. doi: 10.1161/hy0102.099200
Man, K., Kaplan, J., Damasio, H., and Damasio, A. (2013). Neural convergence and divergence in the mammalian cerebral cortex: From experimental neuroanatomy to functional neuroimaging. J. Comp. Neurol. 521, 4097–4111. doi: 10.1002/cne.23408
Menuet, C., Connelly, A. A., Bassi, J. K., Melo, M. R., Le, S., Kamar, J., et al. (2020). PreBotzinger complex neurons drive respiratory modulation of blood pressure and heart rate. Elife 9:e57288. doi: 10.7554/eLife.57288
Min, S., Chang, R. B., Prescott, S. L., Beeler, B., Joshi, N. R., Strochlic, D. E., et al. (2019). Arterial baroreceptors sense blood pressure through decorated aortic claws. Cell Rep. 29, 2192–2201.e3. doi: 10.1016/j.celrep.2019.10.040
Moody, S. A., and Lamantia, A. S. (2015). Transcriptional regulation of cranial sensory placode development. Curr. Top. Dev. Biol. 111, 301–350. doi: 10.1016/bs.ctdb.2014.11.009
Nagy, I., Santha, P., Jancso, G., and Urban, L. (2004). The role of the vanilloid (capsaicin) receptor (TRPV1) in physiology and pathology. Eur. J. Pharmacol. 500, 351–369. doi: 10.1016/j.ejphar.2004.07.037
Neff, R. A., Mihalevich, M., and Mendelowitz, D. (1998). Stimulation of NTS activates NMDA and non-NMDA receptors in rat cardiac vagal neurons in the nucleus ambiguus. Brain Res. 792, 277–282. doi: 10.1016/s0006-8993(98)00149-8
Nerdrum, T., Baker, D. G., Coleridge, H. M., and Coleridge, J. C. (1986). Interaction of bradykinin and prostaglandin E1 on cardiac pressor reflex and sympathetic afferents. Am. J. Physiol. 250, R815–R822. doi: 10.1152/ajpregu.1986.250.5.R815
Paintal, A. S. (1973). Vagal sensory receptors and their reflex effects. Physiol. Rev. 53, 159–227. doi: 10.1152/physrev.1973.53.1.159
Pan, H. L., and Chen, S. R. (2004). Sensing tissue ischemia: Another new function for capsaicin receptors? Circulation 110, 1826–1831. doi: 10.1161/01.CIR.0000142618.20278.7A
Pierau, F. K., Fellmer, G., and Taylor, D. C. (1984). Somato-visceral convergence in cat dorsal root ganglion neurones demonstrated by double-labelling with fluorescent tracers. Brain Res. 321, 63–70. doi: 10.1016/0006-8993(84)90681-4
Porszasz, J., Gyorgy, L., and Porszasz-Gibiszer, K. (1955). Cardiovascular and respiratory effects of capsaicin. Acta Physiol. Acad. Sci. Hung. 8, 61–76.
Premchand, R. K., Sharma, K., Mittal, S., Monteiro, R., Dixit, S., Libbus, I., et al. (2014). Autonomic regulation therapy via left or right cervical vagus nerve stimulation in patients with chronic heart failure: Results of the ANTHEM-HF trial. J. Card. Fail. 20, 808–816. doi: 10.1016/j.cardfail.2014.08.009
Prescott, S. L., and Liberles, S. D. (2022). Internal senses of the vagus nerve. Neuron 110, 579–599. doi: 10.1016/j.neuron.2021.12.020
Price, T. J., and Flores, C. M. (2007). Critical evaluation of the colocalization between calcitonin gene-related peptide, substance P, transient receptor potential vanilloid subfamily type 1 immunoreactivities, and isolectin B4 binding in primary afferent neurons of the rat and mouse. J. Pain 8, 263–272. doi: 10.1016/j.jpain.2006.09.005
Quigg, M. (1991). Distribution of vagal afferent fibers of the guinea pig heart labeled by anterograde transport of conjugated horseradish peroxidase. J. Auton. Nerv. Syst. 36, 13–24. doi: 10.1016/0165-1838(91)90125-m
Quigg, M., Elfvin, L. G., and Aldskogius, H. (1988). Distribution of cardiac sympathetic afferent fibers in the guinea pig heart labeled by anterograde transport of wheat germ agglutinin-horseradish peroxidase. J. Auton. Nerv. Syst. 25, 107–118. doi: 10.1016/0165-1838(88)90015-x
Randhawa, P. K., and Jaggi, A. S. (2015). TRPV1 and TRPV4 channels: Potential therapeutic targets for ischemic conditioning-induced cardioprotection. Eur. J. Pharmacol. 746, 180–185. doi: 10.1016/j.ejphar.2014.11.010
Recordati, G., Schwartz, P. J., Pagani, M., Malliani, A., and Brown, A. M. (1971). Activation of cardiac vagal receptors during myocardial ischemia. Experientia 27, 1423–1424. doi: 10.1007/BF02154267
Ren, K., Randich, A., and Gebhart, G. F. (1990). Electrical stimulation of cervical vagal afferents. I. Central relays for modulation of spinal nociceptive transmission. J. Neurophysiol. 64, 1098–1114. doi: 10.1152/jn.1990.64.4.1098
Robertson, D., Hollister, A. S., Forman, M. B., and Robertson, R. M. (1985). Reflexes unique to myocardial ischemia and infarction. J. Am. Coll. Cardiol. 5, 99B–104B. doi: 10.1016/s0735-1097(85)80536-2
Sabbah, H. N., Ilsar, I., Zaretsky, A., Rastogi, S., Wang, M., and Gupta, R. C. (2011). Vagus nerve stimulation in experimental heart failure. Heart Fail. Rev. 16, 171–178. doi: 10.1007/s10741-010-9209-z
Salavatian, S., Hoang, J. D., Yamaguchi, N., Lokhandwala, Z. A., Swid, M. A., Armour, J. A., et al. (2022). Myocardial infarction reduces cardiac nociceptive neurotransmission through the vagal ganglia. JCI Insight 7:e155747. doi: 10.1172/jci.insight.155747
Salavatian, S., Yamaguchi, N., Hoang, J., Lin, N., Patel, S., Ardell, J. L., et al. (2019). Premature ventricular contractions activate vagal afferents and alter autonomic tone: Implications for premature ventricular contraction-induced cardiomyopathy. Am. J. Physiol. Heart Circ. Physiol. 317, H607–H616. doi: 10.1152/ajpheart.00286.2019
Schwartz, P. J., and De Ferrari, G. M. (2009). Vagal stimulation for heart failure: Background and first in-man study. Heart Rhythm 6, S76–S81. doi: 10.1016/j.hrthm.2009.08.012
Settell, M. L., Pelot, N. A., Knudsen, B. E., Dingle, A. M., Mcconico, A. L., Nicolai, E. N., et al. (2020). Functional vagotopy in the cervical vagus nerve of the domestic pig: Implications for the study of vagus nerve stimulation. J. Neural Eng. 17:026022. doi: 10.1088/1741-2552/ab7ad4
Shen, B., Wong, C. O., Lau, O. C., Woo, T., Bai, S., Huang, Y., et al. (2015). Plasma membrane mechanical stress activates TRPC5 channels. PLoS One 10:e0122227. doi: 10.1371/journal.pone.0122227
Shen, M. J., and Zipes, D. P. (2014). Role of the autonomic nervous system in modulating cardiac arrhythmias. Circ. Res. 114, 1004–1021. doi: 10.1161/CIRCRESAHA.113.302549
Shenton, F. C., Campbell, T., Jones, J. F. X., and Pyner, S. (2021). Distribution and morphology of sensory and autonomic fibres in the subendocardial plexus of the rat heart. J. Anat. 238, 36–52. doi: 10.1111/joa.13284
Shoji, Y., Yamaguchi-Yamada, M., and Yamamoto, Y. (2010). Glutamate- and GABA-mediated neuron-satellite cell interaction in nodose ganglia as revealed by intracellular calcium imaging. Histochem. Cell Biol. 134, 13–22. doi: 10.1007/s00418-010-0711-0
Soliman, E. Z., Elsalam, M. A., and Li, Y. (2010). The relationship between high resting heart rate and ventricular arrhythmogenesis in patients referred to ambulatory 24 h electrocardiographic recording. Europace 12, 261–265. doi: 10.1093/europace/eup344
Song, D. D., Li, Y., Tang, D., Huang, L. Y., and Yuan, Y. Z. (2014). Neuron-glial communication mediated by TNF-alpha and glial activation in dorsal root ganglia in visceral inflammatory hypersensitivity. Am. J. Physiol. Gastrointest. Liver Physiol. 306, G788–G795. doi: 10.1152/ajpgi.00318.2013
Standish, A., Enquist, L. W., and Schwaber, J. S. (1994). Innervation of the heart and its central medullary origin defined by viral tracing. Science 263, 232–234. doi: 10.1126/science.8284675
Stoyanova, I. (2004). Gamma-aminobutiric acid immunostaining in trigeminal, nodose and spinal ganglia of the cat. Acta Histochem. 106, 309–314. doi: 10.1016/j.acthis.2004.05.001
Sved, A. F., Ito, S., Madden, C. J., Stocker, S. D., and Yajima, Y. (2001). Excitatory inputs to the RVLM in the context of the baroreceptor reflex. Ann. N. Y. Acad. Sci. 940, 247–258. doi: 10.1111/j.1749-6632.2001.tb03681.x
Szabados, T., Gomori, K., Palvolgyi, L., Gorbe, A., Baczko, I., Helyes, Z., et al. (2020). Capsaicin-sensitive sensory nerves and the TRPV1 Ion channel in cardiac physiology and pathologies. Int. J. Mol. Sci. 21:4472. doi: 10.3390/ijms21124472
Teofilo, T. M., Duarte, G. P., Borges, R. S., Santos, A. A., Magalhaes, P. J. C., and Lahlou, S. (2019). Stimulation of pulmonary vagal C-fibers by trans-4-methyl-beta-nitrostyrene induces bradycardiac and depressor reflex in rats: Role of vanilloid TRPV(1) receptors. Eur. J. Pharmacol. 849, 154–159. doi: 10.1016/j.ejphar.2019.01.063
Thames, M. D., Klopfenstein, H. S., Abboud, F. M., Mark, A. L., and Walker, J. L. (1978). Preferential distribution of inhibitory cardiac receptors with vagal afferents to the inferoposterior wall of the left ventricle activated during coronary occlusion in the dog. Circ. Res. 43, 512–519. doi: 10.1161/01.res.43.4.512
Thompson, G. W., Horackova, M., and Armour, J. A. (2002). Role of P(1) purinergic receptors in myocardial ischemia sensory transduction. Cardiovasc. Res. 53, 888–901. doi: 10.1016/s0008-6363(01)00542-9
Thoren, P. N. (1977). Characteristics of left ventricular receptors with nonmedullated vagal afferents in cats. Circ. Res. 40, 415–421. doi: 10.1161/01.res.40.4.415
Tominaga, M., Caterina, M. J., Malmberg, A. B., Rosen, T. A., Gilbert, H., Skinner, K., et al. (1998). The cloned capsaicin receptor integrates multiple pain-producing stimuli. Neuron 21, 531–543. doi: 10.1016/s0896-6273(00)80564-4
Tominaga, M., and Julius, D. (2000). Capsaicin receptor in the pain pathway. Jpn. J. Pharmacol. 83, 20–24. doi: 10.1254/jjp.83.20
Tsao, C. W., Aday, A. W., Almarzooq, Z. I., Alonso, A., Beaton, A. Z., Bittencourt, M. S., et al. (2022). Heart disease and stroke statistics-2022 update: A report from the American Heart Association. Circulation 145, e153–e639. doi: 10.1161/CIR.0000000000001052
Tu, H., Liu, J., Zhu, Z., Zhang, L., Pipinos, I. I., and Li, Y. L. (2012). Mitochondria-derived superoxide and voltage-gated sodium channels in baroreceptor neurons from chronic heart-failure rats. J. Neurophysiol. 107, 591–602. doi: 10.1152/jn.00754.2011
Ustinova, E. E., and Schultz, H. D. (1994). Activation of cardiac vagal afferents in ischemia and reperfusion. Prostaglandins versus oxygen-derived free radicals. Circ. Res. 74, 904–911. doi: 10.1161/01.res.74.5.904
Van Weperen, V. Y. H., Ripplinger, C. M., and Vaseghi, M. (2023). Autonomic control of ventricular function in health and disease: Current state of the art. Clin. Auton. Res. [Online ahead of print]. doi: 10.1007/s10286-023-00948-8
Vaseghi, M., Salavatian, S., Rajendran, P. S., Yagishita, D., Woodward, W. R., Hamon, D., et al. (2017). Parasympathetic dysfunction and antiarrhythmic effect of vagal nerve stimulation following myocardial infarction. JCI Insight 2:e86715. doi: 10.1172/jci.insight.86715
Vaseghi, M., and Shivkumar, K. (2008). The role of the autonomic nervous system in sudden cardiac death. Prog. Cardiovasc. Dis. 50, 404–419. doi: 10.1016/j.pcad.2008.01.003
Walker, J. L., Thames, M. D., Abboud, F. M., Mark, A. L., and Klopfenstein, H. S. (1978). Preferential distribution of inhbititory cardiac receptors in left ventricle of the dog. Am. J. Physiol. 235, H188–H192. doi: 10.1152/ajpheart.1978.235.2.H188
Wang, H. J., Rozanski, G. J., and Zucker, I. H. (2017). Cardiac sympathetic afferent reflex control of cardiac function in normal and chronic heart failure states. J. Physiol. 595, 2519–2534. doi: 10.1113/JP273764
Wang, X. L., Tian, B., Huang, Y., Peng, X. Y., Chen, L. H., Li, J. C., et al. (2015). Hydrogen sulfide-induced itch requires activation of Cav3.2 T-type calcium channel in mice. Sci. Rep. 5:16768. doi: 10.1038/srep16768
Wu, P., and Vaseghi, M. (2020). The autonomic nervous system and ventricular arrhythmias in myocardial infarction and heart failure. Pacing Clin. Electrophysiol. 43, 172–180. doi: 10.1111/pace.13856
Yamakawa, K., Rajendran, P. S., Takamiya, T., Yagishita, D., So, E. L., Mahajan, A., et al. (2015). Vagal nerve stimulation activates vagal afferent fibers that reduce cardiac efferent parasympathetic effects. Am. J. Physiol. Heart Circ. Physiol. 309, H1579–H1590. doi: 10.1152/ajpheart.00558.2015
Yamamoto, Y., Henrich, M., Snipes, R. L., and Kummer, W. (2003). Altered production of nitric oxide and reactive oxygen species in rat nodose ganglion neurons during acute hypoxia. Brain Res. 961, 1–9. doi: 10.1016/s0006-8993(02)03826-x
Zannad, F., De Ferrari, G. M., Tuinenburg, A. E., Wright, D., Brugada, J., Butter, C., et al. (2015). Chronic vagal stimulation for the treatment of low ejection fraction heart failure: Results of the NEural Cardiac TherApy foR Heart Failure (NECTAR-HF) randomized controlled trial. Eur. Heart J. 36, 425–433. doi: 10.1093/eurheartj/ehu345
Zeng, W. Z., Marshall, K. L., Min, S., Daou, I., Chapleau, M. W., Abboud, F. M., et al. (2018). PIEZOs mediate neuronal sensing of blood pressure and the baroreceptor reflex. Science 362, 464–467. doi: 10.1126/science.aau6324
Zhang, D., Liu, J., Tu, H., Muelleman, R. L., Cornish, K. G., and Li, Y. L. (2014). In vivo transfection of manganese superoxide dismutase gene or nuclear factor kappaB shRNA in nodose ganglia improves aortic baroreceptor function in heart failure rats. Hypertension 63, 88–95. doi: 10.1161/HYPERTENSIONAHA.113.02057
Zhang, D., Shen, X., and Qi, X. (2016). Resting heart rate and all-cause and cardiovascular mortality in the general population: A meta-analysis. CMAJ 188, E53–E63. doi: 10.1503/cmaj.150535
Zhang, X., Chen, Y., Wang, C., and Huang, L. Y. (2007). Neuronal somatic ATP release triggers neuron-satellite glial cell communication in dorsal root ganglia. Proc. Natl. Acad. Sci. U. S. A. 104, 9864–9869. doi: 10.1073/pnas.0611048104
Keywords: autonomic, vagus, afferent, cardiovascular disease, parasympathetic, myocardial infarction
Citation: van Weperen VYH and Vaseghi M (2023) Cardiac vagal afferent neurotransmission in health and disease: review and knowledge gaps. Front. Neurosci. 17:1192188. doi: 10.3389/fnins.2023.1192188
Received: 23 March 2023; Accepted: 23 May 2023;
Published: 07 June 2023.
Edited by:
Martin Gerbert Frasch, University of Washington, United StatesReviewed by:
Camilo Toledo, Austral University of Chile, ChilePolina Nedoboy, The Heart Research Institute, Australia
Copyright © 2023 van Weperen and Vaseghi. This is an open-access article distributed under the terms of the Creative Commons Attribution License (CC BY). The use, distribution or reproduction in other forums is permitted, provided the original author(s) and the copyright owner(s) are credited and that the original publication in this journal is cited, in accordance with accepted academic practice. No use, distribution or reproduction is permitted which does not comply with these terms.
*Correspondence: Marmar Vaseghi, bXZhc2VnaGlAbWVkbmV0LnVjbGEuZWR1