- 1Facultad de Medicina y Ciencia, Universidad San Sebastián, Concepción, Chile
- 2Research and Development Department, Bay Pines VAHCS, Bay Pines, FL, United States
- 3Facultad de Odontologia y Ciencias de la Rehabilitacion, Universidad San Sebastián, Concepción, Chile
Animal survival depends on cognitive abilities such as learning and memory to adapt to environmental changes. Memory functions require an enhanced activity and connectivity of a particular arrangement of engram neurons, supported by the concerted action of neurons, glia, and vascular cells. The deterioration of the cholinergic system is a common occurrence in neurological conditions exacerbated by aging such as traumatic brain injury (TBI), posttraumatic stress disorder (PTSD), Alzheimer’s disease (AD), and Parkinson’s disease (PD). Cotinine is a cholinergic modulator with neuroprotective, antidepressant, anti-inflammatory, antioxidant, and memory-enhancing effects. Current evidence suggests Cotinine’s beneficial effects on cognition results from the positive modulation of the α7-nicotinic acetylcholine receptors (nAChRs) and the inhibition of the toll-like receptors (TLRs). The α7nAChR affects brain functions by modulating the function of neurons, glia, endothelial, immune, and dendritic cells and regulates inhibitory and excitatory neurotransmission throughout the GABA interneurons. In addition, Cotinine acting on the α7 nAChRs and TLR reduces neuroinflammation by inhibiting the release of pro-inflammatory cytokines by the immune cells. Also, α7nAChRs stimulate signaling pathways supporting structural, biochemical, electrochemical, and cellular changes in the Central nervous system during the cognitive processes, including Neurogenesis. Here, the mechanisms of memory formation as well as potential mechanisms of action of Cotinine on memory preservation in aging and neurological diseases are discussed.
1. Introduction
Learning and memory are not only essential tools for adaptation and survival but give us identity and the opportunity to build our individual and collective advancement based on previous experiences. During a learning experience, we gather sensorimotor, spatial, and emotional information and combine them with similar memories stored in the past.
Learning and memory comprise acquisition, memory consolidation, recall, and reconsolidation or extinction. During memory consolidation, temporary memory transforms into long-term memory (LTM), the mechanism by which acquired memories gain stability or become stronger over time and resistant to interference (Brashers-Krug et al., 1996). At the beginning of the last century, Richard Semon introduced two main theories of memory consolidation, the engram and synaptic plasticity theories. The concept of engrams describes the neural bases for memory storage and hypothesizes that an experience activates a specific group of hippocampal cells that undergo stable biochemical and structural changes to become engrams or memory traces (Semon and Simon, 1921; Thompson, 1976; Thompson, 2005; Thompson, 2013).
Donald Hebb stated that transforming a temporary memory into LTM required a concurrent activation and strengthening of pre-and postsynaptic neurons, a concept broadly supported by the scientific community (Kandel and Schwartz, 1982; Kandel, 2001; Day and Sweatt, 2011; Mayford et al., 2012; Kandel et al., 2014; Day et al., 2015; Asok et al., 2019).
Although still in its initial steps, a deeper understanding of brain functions opens the gates to an unknown and uncertain world where artificial intelligence arrives as an ethical and technological challenge rapidly impacting our society in its social and philosophical foundations. On the other side, however, the same advances promise to improve the quality of life of a crescent population of elderly people worldwide.
In the last decades, when life expectancy grew steadily, cognitive dysfunction during aging has devastated individuals and their significant ones.
The cognitive decline during aging is characterized by hippocampal dysfunction induced by neuronal loss and synaptic loss. These losses seem to be caused by the activation of immune cells, provoking neuroinflammation, mitochondria abnormalities, oxidative stress, decreased autophagy, and diminished Neurogenesis (Echeverria, 2016; Echeverria et al., 2016a; Yanai et al., 2022; Bathini et al., 2023; Chen et al., 2023; Choi and Tanzi, 2023; Dranovsky et al., 2023; Wang et al., 2023; Zhou et al., 2023). Thus, every year, the battle to preserve or augment cognitive abilities in the elderly becomes more critical for humanity. This review will discuss the potential mechanism of the alkaloid Cotinine, a positive cholinergic modulator, in preventing memory loss during aging and disease.
2. Memory mechanisms and engram cells
Episodic memory tells us what happened, when, and where it occurred (Reis et al., 2016). Unfortunately, this type of memory is the first to disappear during aging or dementia (Nyberg et al., 1996a). Dr. Brenda Milner and her team discovered that the hippocampus is the central brain region required to form episodic LTMs (Nyberg et al., 1996b). Almost 30 years later, Bliss and Lomo described long-term potentiation (LTP), a cellular mechanism of LTM (Bliss and Collingridge, 1993). In agreement with Hebbian’s theory, LTP entails increased presynaptic transmission, a concomitant rise of postsynaptic excitability, and the depolarization of the N-methyl-D-aspartic acid receptors (NMDARs). Simultaneous activation of these inotropic receptors at the presynaptic and postsynaptic terminals enhances the influx of calcium ions into neurons, triggering calcium waves and stimulating the expression of genes involved in memory acquisition and consolidation (Nicoll and Malenka, 1999).
The introduction of LTP as a molecular mechanism of memory was followed by the description of a second mechanism named long-term depression (LTD), both considered fundamental mechanisms of memory (Nicoll and Malenka, 1999; Castellano et al., 2001; Frey, 2001; Nakazawa et al., 2004). Finally, the spike-timing-dependent plasticity mechanism shaped the view of other cellular processes underlying both the acquisition and consolidation of LTM (Bi and Poo, 1998; Feldman, 2012).
The role of LTP and LTD in vivo in learning and memory is an unfinished discussion; however, the scientific community agrees that the formation and consolidation of episodic memory involve new mRNA transcription and protein synthesis, two processes also required in the late phases of LTP (Feldman, 2009; Alberini and Kandel, 2014; Fioriti et al., 2015). In addition, LTM consolidation demands metabolic, electric, and structural changes that reinforce particular synaptic connections and consolidate memories by establishing distinct neuronal networks.
As stated above, at the beginning of the last century, Dr. Semon introduced the term engram to describe the neural bases for memory storage (Thompson, 1976, 2013; Tonegawa et al., 2015, 2018; Josselyn and Tonegawa, 2020). According to Semon, an experience activates a specific group of cells that undergo stable biochemical and structural changes to become engrams or memory traces (Semon and Simon, 1921; Thompson, 1976, 2005, 2013; Josselyn and Tonegawa, 2020). Semon’s ideas were ignored during his lifetime; however, in the past decade, the access to transgenic animal models and techniques such as optogenetics, electrophysiology, and in vivo brain imaging, permitted to identify of the engram neurons activated during learning and forming part of the neural circuits involved in LTM consolidation and retrieval. Previous studies have shown that consolidation of LTM requires synaptic plasticity changes, which demand cellular changes in the metabolism, neurotransmission, and cell structure to reinforce synaptic connections establishing distinct neuronal networks (Izquierdo and Medina, 1997; Kandel, 1997; Kandel, 2001; Bailey et al., 2004). New technologies including gene editing (Nihongaki et al., 2015), single-cell RNA sequencing (Landry et al., 2013), in vivo transcriptional imaging (Das et al., 2018), and the optical control of epigenetic marks, have permitted to investigate posttranscriptional mechanisms controlling LTM storage and retrieval during aging and brain disease (Rajasethupathy et al., 2012; Landry et al., 2013).
In agreement with Semon’s theory, the engram neurons seem to acquire a stable biochemical and cellular state after activation, which is susceptible to being reactivated to retrieve, inhibit, or modify a particular memory content. For example, after the acquisition of a conditioned contextual memory, in the absence of a conditioned stimulus, it is possible to induce a false fear memory and conditioned fear reaction by pairing the activation of engram cells with exposure to a new context (Tonegawa et al., 2015, 2018; Josselyn and Tonegawa, 2020).
In the fifties, more than 73 years ago, a visionary scientist, Karl Spencer Lashley, defined the main concepts of memory storage as engrams throughout the cortex forming a sophisticated network connecting specialized brain cells preserving the representation of memories in the brain (Bruce, 2001). Additional technical advances such as the dual-eGRASP (green fluorescent protein reconstitution across synaptic partners; Choi et al., 2018) permitted the visualization of synaptic changes between engram cells during memory formation. These studies showed potential regional neuronal sites for memory processing and storage, including the hippocampus, ventromedial prefrontal cortex, anterior temporal lobe, amygdala (Choi et al., 2021), and posterior cortical areas with engrams in the dentate gyrus participating in spatial navigation memory. More importantly, they reported that partially nonoverlapping engram ensembles in the dentate gyrus encoded different paths during spatial navigation in rodents (Kim et al., 2018; Choi et al., 2021; Choi and Kaang, 2022; Roy et al., 2022).
2.1. The receptor mosaic hypothesis for memory trace formation
Other hypotheses were later developed, further enriching our understanding of memory mechanisms, including the receptor mosaic hypothesis for memory trace formation. This hypothesis postulated that receptors form complex aggregates in the plasma membrane. These aggregates would develop as the result of specific interactions between the receptors promoted by the input of neurotransmitters a neuron receives because of the cognitive process. These receptors form a distinctive mosaic of receptors stabilized by adaptor proteins. The expression and transport of the adaptors would be regulated by the neurochemical codes produced by the summation of neuronal inputs codifying a particular memory (Agnati et al., 1982, 2003, 2004; Zoli et al., 1996; Fuxe et al., 2007).
Also, the order and timing of spikes between pre-and postsynaptic neurons called spike-timing-dependent plasticity (STDP), defines the sign and magnitude of the synaptic plasticity event (Bell et al., 1997; Markram et al., 1997; Bi, 2002; Kepecs et al., 2002; Dan and Poo, 2004; Tazerart et al., 2020). For example, in pyramidal neurons, when a presynaptic spike precedes the postsynaptic one within a limited time, LTP is triggered; if the inverse occurs, the result will be LTD (Bi and Poo, 1998).
Recently, Dr.Tazerart used a spine STDP protocol with two-photon glutamate uncaging (pre) paired with postsynaptic spikes in the cortical layer V of pyramidal neurons to investigate how timing influences LTP in mice (Tazerart et al., 2020). They found that pre-post pairings triggered timing-dependent LTP (t-LTP), neck contraction in the activated spine, and increased synapse strength. On the contrary, post-pre pairings elicited timing-dependent LTD (t-LTD) and decreased synaptic strength without inducing a detectable change in spine structure (Tazerart et al., 2020). Also, they found that triggering t-LTP in clustered spines (<5 μm apart) enhanced LTP by an NMDAR-dependent increase of calcium inside the spine. The NMDAR-dependent t-LTD was interrupted due to the activation of clustered spines but normalized when the distance between spines was at a distance >40 μm. The authors concluded that synaptic cooperativity prevented t-LTD by extending the period permitted for STDP and LTP induction, thus increasing the chance of synaptic plasticity changes underlying LTM to occur (Tazerart et al., 2020). Later, an impairment of STDP was detected in the hippocampus of an APP/PS1 mouse model of AD, overexpressing the Amyloid beta peptide (Aβ) (Garad et al., 2021).
Initially, memory storage was understood as a linear and unidirectional process, transmitting memories from the hippocampus to cortical regions that, in turn, send them for storage in other cortical areas. Recently, Gilboa and Moscovitch (2021), argued that memory might have interconnected representations (abstract semantic, sensory, episodic, and emotional) (Gilboa and Moscovitch, 2021; Wing et al., 2021).
Memories are susceptible to modification after retrieval and reconsolidation, a characteristic exploited to extinguish fear memory using exposure therapy (Nijdam and Vermetten, 2018; Fazel et al., 2020; Gandelman et al., 2022; Wilker et al., 2023); However, fear memory usually reinstates, after exposure to events stimulating the unextinguished components of the memory even long time after extinction (Schiller et al., 2008; Lin et al., 2011; Ishii et al., 2012; Yang et al., 2012).
A better understanding of the detailed effects of fear and different therapies on engram cell’s connectivity and signaling factors (Han et al., 2022; Lee et al., 2023; Wilker et al., 2023) may guide the finding of effective and safe treatments for conditions associated with fear memory, such as PTSD and phobias.
In addition, we may consider non-synaptic transmission, glial cells such as the astrocytes, the extracellular space organization, and volume transmission (Vargova and Sykova, 2014). Similarly, other actors such as non-synaptic receptors (Vizi et al., 2010) metal ions (Chang, 2017), lipids (Wan et al., 2020), nucleic acid (Shao et al., 2020; Jana et al., 2023), and hormones (Hsu et al., 2021; Ghosh-Swaby et al., 2022; Harrington et al., 2022; Krinke et al., 2022; Su et al., 2022; Szczurowska et al., 2022; Wu et al., 2022; Zhang et al., 2022; Zhu D. et al., 2022; Kling et al., 2023; Lacasse et al., 2023) that simultaneously influence the brain response to external stimuli as well as internal abstract conceptualizations, to generate new or modified memories or ideas.
3. Role of the nAChRs in learning and memory
The cholinergic system is critical in keeping high-order cognitive processes in the brain by modulating inflammation, neurotransmitter release, and the expression of many essential genes participating in learning and memory. The cholinergic system involves the muscarinic AChRs (mAChRs) and nicotinic AChRs (nAChRs) in neuronal and non-neuronal cells (Echeverria et al., 2016b; Maurer and Williams, 2017; Mendoza et al., 2018a; Fuenzalida et al., 2020; Galvin et al., 2020; Yousuf et al., 2023). During the formation of new memories, ACh, an endogenous ligand of the AChRs, stimulates inhibitory and excitatory synapses in the hippocampus, adding flexibility to the brain networks during complex behaviors (Haam and Yakel, 2017). In addition, ACh modulates STDP by mechanisms influenced by the firing rate of neurons (Sjostrom et al., 2001) and synapse location and distance to the excitatory inputs (Kampa et al., 2006; Letzkus et al., 2006; Kampa et al., 2007; Letzkus et al., 2007; Froemke et al., 2010).
The hippocampus and the medial prefrontal cortex are brain regions, vastly populated with α7nAChRs, playing a fundamental role in acquiring, storing, and recalling working and episodic memory. In addition, one of its ligands, acetylcholine (ACh), influences STDP and adds flexibility to the brain networks during complex behaviors. The pentameric α7nAChRs are ligand-gated ion channels expressed pre- and postsynaptically in neurons (Cordero-Erausquin et al., 2000). The activation of presynaptic nAChRs promotes neurotransmitter release (Girod et al., 2000; Schilstrom et al., 2000), while activation of postsynaptic nAChRs controls cell signaling cascades supporting learning and memory, such as the PKA/CREB pathway (Kenney and Gould, 2008). In addition, the activation of the nAChRs induces LTP (Matsuyama et al., 2000; Matsuyama and Matsumoto, 2003).
The nAChRs are expressed in the dopaminergic, GABAergic, glutamatergic, norepinephrinergic, and serotonergic neurons (Albuquerque et al., 2009; Hernandez-Lopez et al., 2013; Wallace and Bertrand, 2013). When located on the inhibitory GABAergic, and excitatory glutamatergic neurons, the nAChRs regulate the circuits supporting episodic memory and the associated emotional content (Karadsheh et al., 2004). The nAChRs induce calcium to flow into the cell, triggering signaling cascades promoting synaptic plasticity (McKay et al., 2000; Karadsheh et al., 2004). New evidence suggests that the nAChRs play a specific role in reconsolidating long-term object memories (Wideman et al., 2022).
The nAChRs in neuronal, immune, and glial cells mediate the anti-inflammatory effect. Also, the nAChRs stimulate the expression of genes codifying for transcription factors involved in brain plasticity, such as the brain-derived neurotrophic factor (BDNF) and the cAMP response element-binding protein (CREB), and protein kinases such as the protein kinase A (PKA), the extracellular-signal-regulated kinases (ERKs), the protein kinases C (PKC), the phosphoinositide 3-kinases (PI3K), the protein kinase B (Akt), and the Calcium/calmodulin-dependent protein kinase II (CaMKII). These factors promote the expression of synaptic proteins, including Synapsin I/II, postsynaptic density protein-95 (PSD95), and Synaptophysin (Garad et al., 2021).
3.1. The nAChRs, engrams, and fear memory
Studies of the engrams in the hippocampus found that contextual fear memory formation involved enhanced connectivity between CA3 -and CA1 engram cells, which showed an increased number and size of synaptic spines. Furthermore, they concluded that higher synaptic connectivity between engram cells from adjacent brain regions of the hippocampus is part of the contextual fear memory formation mechanism.
It is known that the genetic background and environment influence a subject’s high-order cognitive abilities and behavior.
Further studies, using genetically modified mice not expressing the α7, β3, or β4 nAChRs subunits, showed that these mice have normal contextual and cued fear conditioning. This evidence suggests that these receptors are not required for fear memory acquisition and storage in mice (Davis and Gould, 2007). Recently, Lotfipour and colleagues (2013) showed that α2 nAChR KO mice showed regular contextual fear conditioning and no effect of nicotine on contextual or trace fear conditioning. However, sex-related differences in their role in fear conditioning were later discovered (Mohammadi-Farani et al., 2022). For example, Semenova et al. reported that male β4 KO mice presented deficits in cued fear conditioning while females did not (Semenova et al., 2012).
Our results working with the modulator of the nAChRs, Cotinine, acting as a positive allosteric modulator of the α7nAChRs enhanced contextual fear memory extinction without altering the acquisition and short-term retention of fear memory in a nAChR dependent-manner (Lotfipour et al., 2013). Based on th current evidence, we have postulated that cotinine-induced enhancement of extinction is mediated by increased neuronal plasticity and neurogenesis in rodents (Echeverria et al., 2017; Sadigh-Eteghad et al., 2020; Bastianini et al., 2021; Raghu et al., 2021). Few years ago, Sadigh-Eteghad et al. (2020) found that chronic cotinine ameliorated learning and memory in aged animals in the MWM and novel object recognition (NOR) tasks (Sadigh-Eteghad et al., 2020), and also increased the expression of (a) antioxidant factors such as the superoxide dismutase (SOD), (b) synaptic proteins (PSD95, GAP-43, and synaptophysin), (c) neurotrophic factors brain-derived neurotrophic factor (BDNF), and neural growth factor (NGF), while decreasing cytokines (TNFα and IL1β) in the hippocampus of aged mice (Sadigh-Eteghad et al., 2020). The α7nAChRs antagonist MLA blocked cotinine effects, in agreement with our results on mice models of PTSD (Echeverria et al., 2016a; Mendoza et al., 2018a; Oliveros-Matus et al., 2020).
A study of the effect of nicotine on contextual fear conditioning in β2 knockout mice did not show enhancement or impairment of fear conditioning compared to wild-type mice (Davis and Gould, 2007). Another study found slight contextual fear-conditioning deficits in the β2 knockout mice (Wehner et al., 2004; Wehner and Radcliffe, 2004). Interestingly, aging seems to change the role of β2 subunits in fear conditioning, as young β2 KO mice showed normal contextual and cued fear conditioning, in contrast to aged β2 KO mice that were impaired in both forms of fear learning (Caldarone et al., 2000). In this respect, β2 nAChR subunits may compensate for the decreased NMDA receptors observed in the aged mice, a function absent in the young β2 KO mice. This study also showed that while α2, α7, and β2 KO mice showed regular trace fear conditioning, β2 KO mice did not show the enhancing effect of nicotine over trace conditioning (Davis and Gould, 2007; Lotfipour et al., 2013).
On the other hand, new studies have shown that transcriptional regulation is also essential in modulating experience-directed behavior. In addition, epigenetic mechanisms can regulate nAChRs expression during memory acquisition through gene methylation and acetylation of chromatin. Changes in nAChRs expression should affect behavior and susceptibility to stress (Morrison et al., 2022).
4. Changes in cholinergic function in neurodegenerative conditions
The cholinergic neurotransmission occurs throughout the entire brain, controlling critical physiological processes such as attention, learning and memory, stress response, wakefulness, and sensory information acquisition (Nees, 2015). For many years, cholinergic dysfunction has been recognized as the leading cause of synaptic deficits leading to cognitive impairment in AD. Several studies using imaging and immunohistochemical approaches have shown degeneration of the cholinergic neurons in the basal forebrain (Jurgensen and Ferreira, 2010; Foucault-Fruchard and Antier, 2017) and a progressive loss of cholinergic receptors in the synaptic sites a primary cause of cognitive impairment during aging and neurodegenerative conditions (Bubser et al., 2012; Yakel, 2013; Okada et al., 2022; Yousuf et al., 2023).
Based on the accumulated evidence, it is reasonable to propose that due to the powerful anti-inflammatory effects of the α7nAChR, its reduced expression in AD and other neurological conditions is a crucial factor inducing synaptic deficits and neuroinflammation (Egea et al., 2015; Heneka et al., 2015; Lykhmus et al., 2015).
The abnormalities initially express as mild cognitive impairment (MCI) that may or not advance to dementia. The leading cause of dementia, Alzheimer’s disease (AD), is characterized by the progressive degeneration of cholinergic neurons in the basal forebrain and loss of nAChRs (Tiwari et al., 2019; Lacalle-Aurioles and Iturria-Medina, 2023; Tryon et al., 2023). In animal AD models, memory loss is characterized by neuronal mitochondrial dysfunction and deficits in the axonal transport of proteins and organelles. These defects are mainly attributed to the aggregation of the microtubule protein Tau and amyloid-beta peptides (Aβ). The intracellular accumulation of oligomeric forms of Aβ, is followed by the intracellular accumulation of aggregated forms of hyperphosphorylated Tau (Echeverria et al., 2002, 2004, 2007; Echeverria and Cuello, 2002). These abnormalities trigger the release of cytokines, such as the Transforming Neurotrophic Factor α (TNFα), neuroinflammation, oxidative stress, and mitochondrial dysfunction. This results in energy deficits and neuron cell death in brain regions involved in learning and memory (Bathini et al., 2023; Teoh et al., 2023; Wang et al., 2023). Activation of the α7nAChRs inhibits cytokine release by microglia, macrophages, astrocytes, and T cells in the CNS (McKay et al., 2007; Hernandez and Dineley, 2012; Sadigh-Eteghad et al., 2016; Morioka et al., 2018). Inhibition of cytokines release prevents neuroinflammation and neuronal damage induced by toxic protein aggregates in AD. Counteracting neuroinflammation and oxidative stress reduces Aβ peptides aggregation, abnormal tau phosphorylation, and conversion into intracellular neurofibrillary tangles (Echeverria et al., 2016a). Furthermore, re-establishing brain homeostasis reduces the metabolic deficit, oxidative stress, and mitochondria dysfunction (Echeverria et al., 2016b). Similar positive effects are expected by enhancing nAChRs activity to reduce neuroinflammation and neurodegeneration in other neurological conditions presenting a decrease in nAChRs, such as PD (Majdi et al., 2018; Iarkov et al., 2020).
5. Cognitive impairment during traumatic brain injury
TBI is commonly associated with the deterioration of executive functions, spatiotemporal memory, and recognition memory in the absence of dementia due to stress response and vascular pathology (Brett et al., 2021; Lippa et al., 2021; Steward et al., 2022; Williams et al., 2022).
Also, loss of cognitive abilities in TBI patients results from using drugs to diminish pain or anxiety, such as using anesthetics during and opioids after surgery, respectively (Stratmann et al., 2010; Lin et al., 2012; Devick et al., 2020). In addition, with few exceptions such as sertraline, most antidepressants, anxiolytic, and antipsychotics drugs radiation or chemotherapy, or new treatments such as ketamine investigated for treating PTSD, drug-resistant depression, diminishes cognitive abilities (Yasar et al., 2008; Mitchell and Turton, 2011; White et al., 2013; Hohman et al., 2015). Triggering events associated with a vulnerable genetic background work together to produce neuroinflammation and progressive deterioration of cognitive functions by reducing synaptic plasticity and the connectivity between brain regions supporting healthy cognitive functions (Rowan et al., 2007).
A recent study shows that single whole-body exposure to radiation decreased Neurogenesis, synaptic potentiation, and learning abilities in adult mice (Miry et al., 2021). Moreover, 2 months post-exposure, mice still showed plasticity deficits compared to sham-irradiated and age-matched mice. Unexpectedly, 6-, 12-, and 20 months after radiation, mice showed enhanced synaptic potentiation, spatial learning, and adult neurogenesis (Miry et al., 2021). Furthermore, synaptic plasticity and spatial learning remained enhanced 20 months post-exposure, indicating a life-long influence on plasticity and cognition from a single exposure to radiation in young adulthood. The authors speculated that galactic radiation exposure might alter brain health and cognitive function during or after a long trip in space (Miry et al., 2021). To our best knowledge, the mechanisms underlying the long-term effects of radiation on cognitive abilities and their impact on older individuals are not entirely understood.
6. Memory loss during aging
The preservation of learning and memory capabilities is one of the most challenging and needed healthcare goals to achieve, considering the growth of the aged population (Dinius et al., 2023). Various clinical trials have found numerous changes in the brain that may explain the negative impact of unhealthy aging on cognitive abilities. Cognitive impairment during aging is associated with hormonal deficits (Amore, 2005; Henderson, 2011; Winczyk et al., 2022), inflammation (Engler-Chiurazzi et al., 2023; Teoh et al., 2023; Zhou et al., 2023), epigenetic modifications affecting gene expression and adult neurogenesis (Zocher and Toda, 2023), and changes in the cholinergic, glutamatergic, dopaminergic, and histaminergic neurotransmission (Gasiorowska et al., 2021). These changes reduce synaptic and brain plasticity and memory loss (Shen and Barnes, 1996; Echeverria et al., 2017; Kumar et al., 2019b; Gasiorowska et al., 2021; Sanchez-Sarasua et al., 2022).
Incorporating brain imaging, optogenetic, and electric brain recording techniques in aging studies has allowed us to understand better the compensatory mechanisms preserving brain functionality and evaluate different therapeutic strategies. Magnetic resonance imaging (MRI) studies have shown that structural changes, such as the decline in the volume of the prefrontal areas and hippocampus during aging, can predict a person’s cognitive performance (Raz et al., 1997; Morrison and Baxter, 2012). New approaches tested to maintain or improve cognitive abilities during aging include hormonal supplementation (Foster et al., 2003), magnetic brain stimulation, oxygen therapy (Yang C. et al., 2023), cognitive stimulation using computer-assisted programs (Curlik 2nd and Shors, 2013; Clemenson and Stark, 2015; Nebot et al., 2022), guided exercise, music and dance therapy (Thumuluri et al., 2021; Aguinaga et al., 2022; Ambegaonkar et al., 2022; Kennedy et al., 2022; Li et al., 2022; Rodziewicz-Flis et al., 2022; Vega-Avila et al., 2022; Wang et al., 2022; Zhu Y. et al., 2022; Blanco-Rambo et al., 2023), and diets containing natural antioxidant compounds (Kumar et al., 2012; Maki, 2012; Smith et al., 2014; Barreto et al., 2017; Phipps et al., 2021; Tao et al., 2023; Tchekalarova and Tzoneva, 2023).
Most pathological conditions that provoke loss of cognitive abilities, including learning and memory deficits, can be triggered or exacerbated by aging (Murman, 2015). Brain atrophy during aging starts in the more plastic regions that are involved in learning and memory, such as the prefrontal cortex (PFC), the hippocampus (Hipp), the cingulate cortex (CC), and the entorhinal cortex (EC) to later extend throughout the brain (Blinkouskaya et al., 2021; Blinkouskaya and Weickenmeier, 2021).
6.1. Role of neuroinflammation in memory loss during aging
During aging, changes in the levels of neurotransmitters and hormones seem to make the brain more prone to suffer cerebrovascular accidents and white matter lesions, leading to memory impairment and dementia (Jett et al., 2022; Kanoni et al., 2022; Hogervorst et al., 2023; Kerstens et al., 2023). Cognitive impairment results from complex mechanisms affecting the brain’s function during aging. Mutations, genetic predisposition, biological clock, and a mix of extrinsic factors, including infectious diseases, chronic stress, and environmental toxins, enhance brain aging. These factors transform the glial cells to stop releasing neuroprotective factors, and release apoptotic cytokines, ultimately provoking energy deficits, oxidative stress, neuroinflammation, and neuronal loss.
Neuroinflammation is a common feature of most CNS diseases and a recognized mediator of brain dysfunction (Echeverria et al., 2016b). Neuroinflammation and oxidative stress accompany cognitive deterioration during aging by mechanisms altering the structure and function of glial cells (Echeverria, 2016). Glial cells, under pathological conditions, decrease the production of neurotrophic factors such as BDNF and show higher expression and release of cytokines. Cytokines are proteins that, when secreted, regulate the immune response and control cell growth, differentiation, and function. Cytokines released by macrophages, glial cells, and lymphocytes induce the innate immune response and a persistent cellular response by B cells and T cells. In addition, the binding of the cytokines to their receptors activates signaling pathways, enhancing the expression of genes that further increase the immune response (Jones and Thomsen, 2013). The cellular changes induced by inflammatory cytokines such as TNF-α and IL-1β, alter the connectivity of brain regions involved in information accurate acquisition and recall or interpretation of facts, leading to abnormal thinking and behaviors (Bowirrat, 2022). After TBI, infection, depression, and neurodegeneration, high levels of cytokines negatively affect brain homeostasis and plasticity, learning, memory, and emotional behavior, especially during aging (Ladak et al., 2019; Bourgognon and Cavanagh, 2020; Kalra et al., 2022).
Chronic neuroinflammation is common in most neurological or psychiatric conditions, leading to cognitive impairment and dementia (McGeer et al., 2000; McGeer and McGeer, 2010). Brain inflammation, oxidative stress, and energy deficits co-occur and can result from exposure to neurotoxins, physical and mental trauma, abnormal protein aggregates, stress hormones altering neurotransmission, and autoimmune reactions.
A complex combination of genetic factors, life events, and lifestyles triggers these pathological processes. Mental triggers include traumatic events, depression (Gu et al., 2022), physical stressors such as radiotherapy (Gu et al., 2022; Gutierrez-Quintana et al., 2022), traumatic brain injury, viral or bacterial infections (Akinci et al., 2022; Francis et al., 2023), and exposure to toxins such as toxic heavy metals (Mani et al., 2022; Xu et al., 2022), insecticides, chemotherapy agents, medicaments, and a fatty diet. A recent study showed that neuroinflammation could also be associated with epigenetic changes induced by Posttraumatic Stress Disorder (PTSD) (Wolf et al., 2018) (see Figure 1).
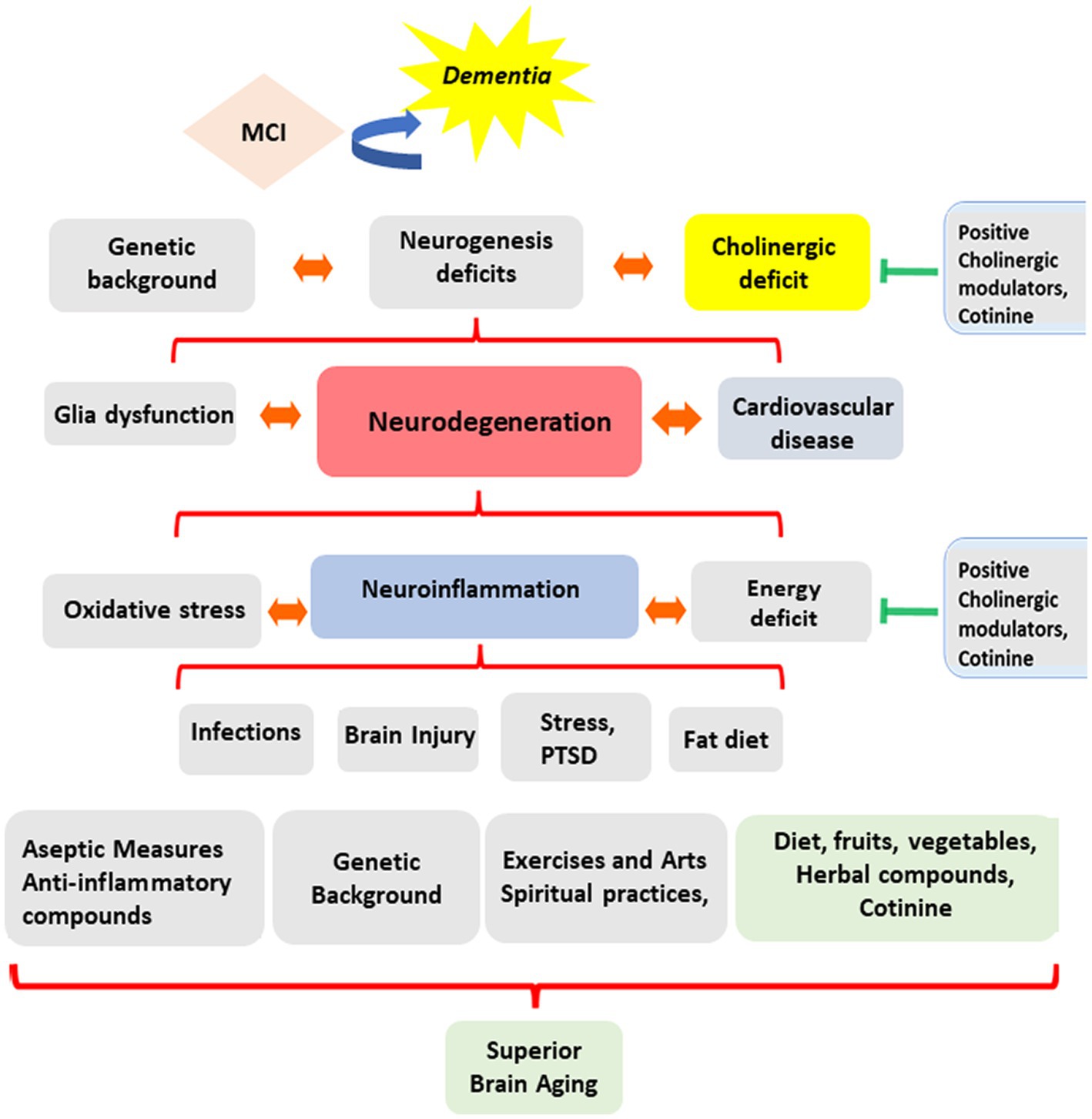
Figure 1. Evolution of cognitive abilities during aging. The diagram summarizes the complexity of the factors influencing the direction of mental changes during aging. These factors include experiencing inflammatory diseases, a person’s lifestyle, genetic background, diet, exercise habits, religious practices, and environmental, social, and personal stress factors affecting brain function.
7. Targeting adult neurogenesis to enhance memory abilities using positive modulators of the nAChRs
The CNS controls all body processes, from thinking, movement, and memory to regulating body temperature and hormone secretion. Therefore, one of the most exciting questions in medicine is why neurons have a limited regenerative capacity compared to other body parts. The CNS’s limited regenerative ability may help prevent inadequate integration of new neurons in a complex system, preventing disorganized behavior and altered perception that may endanger the organism’s survival. Almost 60 years have passed since the first reports suggesting that the mammalian brain could regenerate through the proliferation and differentiation of adult neural stem cells (Brett et al., 2021; Lippa et al., 2021; Steward et al., 2022).
Adult Neurogenesis is a lifelong process by which neural stem cells (NSCs) differentiate, mature, and integrate into local circuits (Aimone et al., 2014). Currently, two points of Neurogenesis in the brain are well known: the subventricular zone (SVZ), adjacent to the lateral ventricles, and the subgranular zone (SGZ) of the dentate gyrus (DG) of the hippocampus (Altman and Das, 1966; Altman, 1969a,b; Altmann et al., 2019). In addition, according to some data, Neurogenesis can also occur in the hippocampus, cerebral cortex, striatum, amygdala, and substantia nigra after brain damage (Gould et al., 2000; Li et al., 2023; Woitke et al., 2023; Yang J.L. et al., 2023). For example, some of the developing cells from the SVZ migrate as neuroblasts to the olfactory bulb, where they develop into olfactory interneurons (Doetsch et al., 1997, 1999; Doetsch and Scharff, 2001; Doetsch and Hen, 2005). In addition, part of these immature neuronal cells migrates to areas of the cerebral cortex where they may participate in cell repair or renewal (Parent, 2002). Similarly, newly formed cells migrate into the granular cell layer transforming into fully integrated mature dentate granular neurons (Kempermann et al., 2018). Significantly, newly generated DG granular neurons form synapses and attach axons to their correct part of the CA3 region, generating action potentials and functional synaptic inputs like mature DG neurons (Hastings and Gould, 1999).
According to some quantitative studies of the neurogenesis level in rodents, it is pretty high. For example, rat dentate gyrus produces up to 270,000 new cells per month, up to a quarter of the total number of granule cells (Cameron and McKay, 2001; Cameron and Glover, 2015). It is supposed that this level of new cell addition will undoubtedly change the function of the network. Indeed, numerous evidence supports the involvement of adult hippocampal Neurogenesis in learning and memory (Clelland et al., 2009). Fortunately, physical activity Increases the generation of new neurons, accelerates spatial learning, and enhances long-term potentiation (van Praag et al., 1999).
Conversely, reduced Neurogenesis in the hippocampus as a result of antimitotic drugs, radiation, or genetic manipulation impairs hippocampal function (Shors et al., 2001), long-term spatial memory (Rola et al., 2004; Snyder et al., 2005), and contextual fear conditioning and environmental enrichment (Meshi et al., 2006; Saxe et al., 2006). Compared to hippocampal Neurogenesis, the function of SVZ-olfactory Neurogenesis is less well-defined. However, a few studies have shown that new neurons in the olfactory bulb play a significant role in the maintenance of olfactory tissue and are involved in olfactory discrimination and olfactory perception functions (Kageyama et al., 2012).
7.1. Neurogenesis and aging
Neurogenesis happens, to a low extent, in specific brain regions and promoted by acetylcholine (ACh), a neurotransmitter pivotal in cognition (Madrid et al., 2021). Trying to understand how a small number of new hippocampal neurons can influence brain function, a study investigated adult neurogenesis across the lifespan. They found that chronic suppression of hippocampal Neurogenesis, as observed after exposure to chronic stress and aging, results in a hippocampal cholinergic deficit that parallels a progressive loss of working memory (Madrid et al., 2021). Also, they observed compensatory structural and functional changes in the cholinergic innervation of the hippocampal formation supported by new adult neurons that provide increased cholinergic inputs to the ventral hippocampus from ventrally projecting neurons that are recruited by the dorsal projection. They hypothesize that Neurogenesis supports memory by supporting the septohippocampal cholinergic circuit throughout the lifespan. They concluded that even aberrant new connections can be recruited to restore memory functions in aged subjects. Furthermore, neurogenesis in the hippocampus is critical to maintain social memory and can be by social factors Oxytocin, a hormone stimulated by social interaction, stimulates neurogenesis under common and stressful conditions and social isolation has the opposite effect (Leuner et al., 2012; Holmes, 2016). Neurogenesis occurs at a calculated rate of 1.75% per year (Spalding et al., 2013) and persists throughout aging (Boldrini et al., 2018; Tartt et al., 2018).
Some scientists in the field argued that there is insufficient evidence to sustain the existence of adult neurogenesis in humans, and that generation of neurons and their migration are limited to early childhood (Curtis et al., 2012; Kumar et al., 2019a, 2020). Despite these suggestions, the research on adult neurogenesis continues growing, holding great promise for brain rehabilitation, and helping to modifying stressful memories (Scott et al., 2021). Stimulating endogenous brain repair mechanisms would be more effective from an immunological and physiological point of view than using exogenous cell implantation approaches (Sun, 2014; Poulose et al., 2017; Scott et al., 2021). Opening opportunities stimulate scientists to study Neurogenesis in various aspects, including the search for controlling and modulating signals (Shabani et al., 2021). Other therapeutic approaches include the use of plant extracts, plant compounds or nutritional factors (functional food), neurotransmitters (serotonin, acetylcholine), sex hormones (estrogen and testosterone), glucocorticoids, and neuronal growth factors (NGF, BDNF) to target biochemical factors modulating neuronal cell proliferation or differentiation (Poulose et al., 2017; Setel et al., 2022; Shengkai et al., 2022; Zheng et al., 2022).
Current evidence suggests that the most promising factors to stimulate neurogenesis are the ones that positively modulate the cholinergic system, which has a vital role in adult neurogenesis (Harrist et al., 2004; Mohapel et al., 2005; John et al., 2015). We and others propose that acetylcholine receptors, especially the α7 and α4β2 subtypes, are the most promising targets to promote neurogenesis in the hippocampus (Harrist et al., 2004; Campbell et al., 2010; Hernandez-Miranda et al., 2010; John et al., 2015; Otto and Yakel, 2019; Webster et al., 2019). The α7nAChRs are expressed in embryonic and adult NSCs and play an essential role in activating its proliferation and differentiation, thus regulating hippocampal neurogenesis in a sex-dependent manner (Otto and Yakel, 2019). Furthermore, the nAChRs may influence Neurogenesis by inhibiting neuroinflammation and regulating fibroblast growth factor-1 (FGF-1) and FGF-2 signaling (Narla S. et al., 2013; Narla S.T. et al., 2013). The subgranular zone and the inner granular cell layer, which are neural stem cell niches, have rich cholinergic innervation and solid responses to cholinergic stimulation suggesting a role of the nAChRs in neurogenesis (Doetsch and Hen, 2005; Riquelme et al., 2008).
In addition, evidence showed that degeneration of the cholinergic system suppresses Neurogenesis in the hippocampus (Kotani et al., 2006; Ijomone and Nwoha, 2015). The survival and development of neurons in the hippocampus require Ca2+-dependent activation of the ERK and CREB by the nAChRs (Shabani et al., 2020; Shen et al., 2022). The enhancement of Neurogenesis involves the trafficking of α7nAChR, which requires increased phosphorylation of Protein Kinase C (PKC) and a decrease in the level of farnesyl pyrophosphate (Chen et al., 2018). In the brain, α7nAChR activation stimulates neuroprotection and neuronal survival by increasing Bcl-2 expression via the PI3K/Akt pathway (Kihara et al., 2001). Overall, current evidence suggests that partial agonists, antagonists, and positive modulators of the α7nAChRs can stimulate and inhibit adult neurogenesis. Thus, the positive impact of cotinine on neurogenesis can be explained at least partly by the positive modulation of the α7nAChRs.
8. Cognitive-enhancing effects of cotinine in mice models of PTSD, aging, and Alzheimer’s disease
We and others have shown evidence suggesting that hippocampal cholinergic receptors are fundamental to regulating the extinction of fear memories showing that antagonists of the nAChRs or mAChRs impair fear memory extension when directly administered in the hippocampus using stereotaxic techniques (Oliveros-Matus et al., 2020; Rosa et al., 2023).
Cotinine is a member of a family of >60 alkaloid derivatives of nicotine and its primary metabolite (Grizzell and Echeverria, 2015). Cotinine is produced by the oxidation of nicotine by the Cytochrome P450 and aldehyde oxidases and further modified by other metabolic enzymes to be eliminated in the urine (Siu and Tyndale, 2007; Zhou et al., 2010). As a result, cotinine accumulates in the brain and has a better safety profile than nicotine and its derivatives, having minimal side effects in humans (Grizzell and Echeverria, 2015).
In animals, Cotinine has been administered via oral, intranasal, intravenously, and by intramuscular or intracerebral injection showing strong memory and mood-enhancing effects under normal and pathological conditions in rodents (Dar et al., 1993; Schroff et al., 2000; Zeitlin et al., 2012; Grizzell et al., 2014a; Patel et al., 2014; Wildeboer-Andrud et al., 2014; Grizzell et al., 2017; Perez-Urrutia et al., 2017; Alvarez-Ricartes et al., 2018a; Mendoza et al., 2018b; Oliveros-Matus et al., 2020), monkeys (Terry et al., 2005; O'Leary et al., 2008), humans, and zebrafish (Boiangiu et al., 2021). In addition, Cotinine has shown unprecedented transversal benefits for cognitive abilities in animal models of mental illnesses or developmental, including schizophrenia (Moran, 2012; Echeverria et al., 2016a), autism (Wang et al., 2015), depression (Grizzell et al., 2014b; Patel et al., 2014; Mendoza et al., 2018b), chronic stress (Alvarez-Ricartes et al., 2018b), anxiety (Barreto et al., 2017), PTSD (Barreto et al., 2015), AD (Patel et al., 2014; Echeverria et al., 2016a, 2017), aging (Terry et al., 2005; Moran, 2012) and cellular models of PD (Soto-Otero et al., 2002; O'Leary et al., 2008; Barreto et al., 2014). Furthermore, Cotinine improved cognitive abilities and mood with no significant adverse events in rodents, monkeys, fish, and humans (Hatsukami et al., 1992, 1997, 1998a,b; Keenan et al., 1994).
Despite various studies showing the safety of cotinine in humans (Hatsukami et al., 1997; Terry et al., 2005), no clinical studies of Cotinine as a treatment for psychiatric and neurological conditions have been performed. Nevertheless, last year a nasal spray containing a nicotinic Cholinergic Agonists Mixture (CAM), that included Cotinine, anatabine, and nicotine, was tested as a therapy in 80 adult Colombian patients with confirmed SARS-CoV-2 (Sharma and Mehan, 2021). The results showed that in these critical patients, after 2 weeks of treatment, the patients showed a statistically and clinically significant reduction of five symptoms, including dyspnea, cough, general malaise, muscle fatigue, and cephalea (Sharma and Mehan, 2021). In this and other conditions alleviated by Cotinine, there was an underlying deficit in nAChRs, neuroinflammation, and oxidative stress (Tan et al., 2021).
8.1. Current therapies for cognitive impairment in AD
Currently, the amyloid hypothesis stating that Aβ aggregation is the leading cause of AD (McGeer and McGeer, 2013) is subjected to severe scrutiny, mainly because the therapies directed to reduce the senile Aβ plaques or the peptide aggregation have failed or proven minimally effective (Hilund-Carlsen et al., 2022). In fact, for long time, it has been recognized that brains with significant amyloid Aβ burden can still function well when neuroinflammation is under control (Echeverria et al., 2009). The abnormalities of the microtubule protein Tau have proven to be better at predicting brain atrophy and cognitive deficits in AD (Malpetti et al., 2022). Although, considerable evidence supports the view that aggregated forms of Aβ are part of the pathological equation (Hill, 2022). The Aβ peptide induces oxidative stress, tau hyperphosphorylation, synaptic plasticity deficits, and neuroinflammation (Choi and Gandhi, 2018; Rejc et al., 2022). To overcome similar paradoxes, new diagnostic tools such as the 18F-THK5351, a PET tau tracer that binds to the astroglial monoamine oxidase B, are being characterized to detect tau aggregation and neuroinflammation simultaneously (Imabayashi et al., 2022).
The drugs approved by the US Food and Drug Administration (FDA) to treat cognitive impairment include acetylcholinesterase inhibitors (AChEI), donepezil, galantamine, and rivastigmine, the NMDA receptor antagonist memantine and a new immunotherapeutic treatment; these drugs delay the progression of the disease but do not stop its progression (Porsteinsson et al., 2008; Kavanagh et al., 2011; Tayeb et al., 2012). The treatment for immunotherapy against Aβ peptides aggregation with aducanumab has been controversial, being one of the arguments that quantification of amyloid deposition by PET was imprecise due to the lack of specificity of the PET imaging probe (Hilund-Carlsen et al., 2022).
Another approach would be using positive allosteric modulators (PAM) to enhance the nAChRs activation in brain cells, to increase brain plasticity and Neurogenesis, and prevent the activation of glial cells and peripheric immune cells, such as macrophages and T cells, that reach the brain. Altogether this strategy would prevent neuroinflammation, neurodegeneration, and memory loss during aging and pathological conditions affecting the brain (Echeverria et al., 2016a).
Clinical trials have tested many pro-cholinergic compounds showing adverse side effects or lower efficacy. However, there are reasons to believe that these failures were because these therapies primarily involved agonists of these receptors, many of which rapidly desensitize the nAChRs eliciting undesired side effects. In addition, these compounds did not prevent tau hyperphosphorylation and Amyloid β peptide (Aβ) aggregation in the brains of people with dementia (Echeverria et al., 2016b).
Galantamine Hs shown to be superior to donepezil, improving cognitive impairment, most likely because, in addition to inhibiting ACh degradation, it is a PAM of the α7nAChR and inhibits the toll-like receptors (TLRs) (Bagaitkar et al., 2012). Other clinical studies investigating galantamine plus memantine did not show a superior effect of this combination in improving memory than galantamine as a single therapy in AD (Thancharoen et al., 2019). Galantamine and memantine increased the circulating level of the brain-derived neurotrophic factor (BDNF), most likely through the modulation/activation of the α7nAChRs and NMDARs, which can explain at least in part its pro-cognitive and neuroprotective effects (Bozon et al., 2003; Josselyn et al., 2004; Josselyn and Nguyen, 2005).
Based on our results investigating the effect of cotinine on learning and memory consolidation and extinction in vivo and the response of the human a7nAChR to ACh or nicotine, our team was the first to propose that a deficit in the expression and activity of the α7nAChRs was vital in promoting cognitive deficits and brain neurodegeneration during aging and after traumatic experiences (Sabandal et al., 2022). After more than a decade, all new evidence supports the idea that Cotinine acting on the nAChRs present in neurons, immune and glial cells prevents the release of inflammatory factors and neuroinflammation. Also, by modulating these receptors in neurons, endothelial cells, and neuronal precursor cells, cotinine can enhance Neurogenesis and strengthens the synapsis in the brain (Valles et al., 2014). Recent studies have shown that an enhanced risk of PTSD and AD during aging relates to differences in susceptibility genes involved in immune function, neuroinflammation, and stress responses. This evidence further supports the view that cotinine controlling neuroinflammation can prevent aging-induced cognitive decline (Abbatecola et al., 2011; Sabbagh et al., 2014; Criado-Marrero et al., 2018; Sawyer et al., 2022; Bathini et al., 2023).
9. Conclusion
Based on the evidence described in this review, it is reasonable to propose that correcting the nicotinic receptor deficits during aging may normalize neurogenesis, prevent the abnormal aggregation of Tau, APP, and α-synuclein and consequently, neuroinflammation, and neurodegeneration. The prevention of the accumulation of these pathogenic protein aggregates may delay or completely revert cognitive decline during aging (Maelicke, 2000; Coyle and Kershaw, 2001; Jurgensen and Ferreira, 2010; Toyohara and Hashimoto, 2010; Geerts, 2012; Echeverria et al., 2016b).
Author contributions
All authors listed have made a substantial, direct, and intellectual contribution to the work and approved it for publication.
Funding
This study was supported by the grant Fondecyt, ANID 1190264 (VE and AI).
Conflict of interest
VE is the inventor of five patents granted for the use of cotinine for posttraumatic stress disorder, Alzheimer’s disease, and other neurological and psychiatric conditions.
The remaining authors declare that the research was conducted in the absence of any commercial or financial relationships that could be construed as a potential conflict of interest.
Publisher’s note
All claims expressed in this article are solely those of the authors and do not necessarily represent those of their affiliated organizations, or those of the publisher, the editors and the reviewers. Any product that may be evaluated in this article, or claim that may be made by its manufacturer, is not guaranteed or endorsed by the publisher.
References
Abbatecola, A. M., Olivieri, F., Corsonello, A., Antonicelli, R., Corica, F., and Lattanzio, F. (2011). Genome-wide association studies: is there a genotype for cognitive decline in older persons with type 2 diabetes? Curr. Pharm. Des. 17, 347–356. doi: 10.2174/1381612821666150130120747
Agnati, L. F., Ferre, S., Leo, G., Lluis, C., Canela, E. I., Franco, R., et al. (2004). On the molecular basis of the receptor mosaic hypothesis of the engram. Cell. Mol. Neurobiol. 24, 501–516. doi: 10.1023/B:CEMN.0000023626.35717.5d
Agnati, L. F., Franzen, O., Ferre, S., Leo, G., Franco, R., and Fuxe, K. (2003). Possible role of intramembrane receptor-receptor interactions in memory and learning via formation of long-lived heteromeric complexes: focus on motor learning in the basal ganglia. J. Neural Transm. Suppl. 1–28. doi: 10.1007/978-3-7091-0643-3_1
Agnati, L. F., Fuxe, K., Zoli, M., Rondanini, C., and Ogren, S. O. (1982). New vistas on synaptic plasticity: the receptor mosaic hypothesis of the engram. Med. Biol. 60, 183–190.
Aguinaga, S., Kaushal, N., Balbim, G. M., Wilson, R. S., Wilbur, J. E., Hughes, S., et al. (2022). Latin dance and working memory: the mediating effects of physical activity among middle-aged and older Latinos. Front. Aging Neurosci. 14:755154. doi: 10.3389/fnagi.2022.755154
Aimone, J. B., Li, Y., Lee, S. W., Clemenson, G. D., Deng, W., and Gage, F. H. (2014). Regulation and function of adult neurogenesis: from genes to cognition. Physiol. Rev. 94, 991–1026. doi: 10.1152/physrev.00004.2014
Akinci, M., Pena-Gomez, C., Operto, G., Fuentes-Julian, S., Deulofeu, C., Sanchez-Benavides, G., et al. (2022). A. Study, Prepandemic Alzheimer disease biomarkers and anxious-depressive symptoms during the COVID-19 confinement in cognitively unimpaired adults. Neurology 99, e1486–e1498. doi: 10.1212/WNL.0000000000200948
Alberini, C. M., and Kandel, E. R. (2014). The regulation of transcription in memory consolidation. Cold Spring Harb. Perspect. Biol. 7:a021741. doi: 10.1101/cshperspect.a021741
Albuquerque, E. X., Pereira, E. F., Alkondon, M., and Rogers, S. W. (2009). Mammalian nicotinic acetylcholine receptors: from structure to function. Physiol. Rev. 89, 73–120. doi: 10.1152/physrev.00015.2008
Altman, J. (1969a). Autoradiographic and histological studies of postnatal neurogenesis. IV. Cell proliferation and migration in the anterior forebrain, with special reference to persisting neurogenesis in the olfactory bulb. J. Comp. Neurol. 137, 433–457. doi: 10.1002/cne.901370404
Altman, J. (1969b). Autoradiographic and histological studies of postnatal neurogenesis. 3. Dating the time of production and onset of differentiation of cerebellar microneurons in rats. J. Comp. Neurol. 136, 269–293. doi: 10.1002/cne.901360303
Altman, J., and Das, G. D. (1966). Autoradiographic and histological studies of postnatal neurogenesis. I. a longitudinal investigation of the kinetics, migration and transformation of cells incorporating tritiated thymidine in neonate rats, with special reference to postnatal neurogenesis in some brain regions. J. Comp. Neurol. 126, 337–389.
Altmann, C., Keller, S., and Schmidt, M. H. H. (2019). The Role of SVZ stem cells in glioblastoma. Cancers (Basel) 11:448. doi: 10.3390/cancers11040448
Alvarez-Ricartes, N., Oliveros-Matus, P., Mendoza, C., Perez-Urrutia, N., Echeverria, F., Iarkov, A., et al. (2018a). Intranasal cotinine plus krill oil facilitates fear extinction, decreases depressive-like behavior, and increases hippocampal Calcineurin a levels in mice. Mol. Neurobiol. 55:7961. doi: 10.1007/s12035-018-1095-8
Alvarez-Ricartes, N., Oliveros-Matus, P., Mendoza, C., Perez-Urrutia, N., Echeverria, F., Iarkov, A., et al. (2018b). Intranasal cotinine plus krill oil facilitates fear extinction, decreases depressive-like behavior, and increases hippocampal Calcineurin a levels in mice. Mol. Neurobiol. 55, 7949–7960. doi: 10.1007/s12035-018-0916-0
Ambegaonkar, J. P., Matto, H., Ihara, E. S., Tompkins, C., Caswell, S. V., Cortes, N., et al. (2022). Dance, music, and social conversation program participation positively affects physical and mental health in community-dwelling older adults: a randomized controlled trial. J. Dance Med. Sci. 26, 255–264. doi: 10.12678/1089-313X.121522f
Amore, M. (2005). Partial androgen deficiency and neuropsychiatric symptoms in aging men. J. Endocrinol. Investig. 28, 49–54.
Asok, A., Leroy, F., Rayman, J. B., and Kandel, E. R. (2019). Molecular mechanisms of the memory trace. Trends Neurosci. 42, 14–22. doi: 10.1016/j.tins.2018.10.005
Bagaitkar, J., Zeller, I., Renaud, D. E., and Scott, D. A. (2012). Cotinine inhibits the pro-inflammatory response initiated by multiple cell surface toll-like receptors in monocytic THP cells. Tob. Induc. Dis. 10:18. doi: 10.1186/1617-9625-10-18
Bailey, C. H., Kandel, E. R., and Si, K. (2004). The persistence of long-term memory: a molecular approach to self-sustaining changes in learning-induced synaptic growth. Neuron 44, 49–57. doi: 10.1016/j.neuron.2004.09.017
Barreto, G. E., Avila-Rodriguez, M., Foitzick, M., Aliev, G., and Echeverria, V. (2017). Advances in medicinal plants with effects on anxiety behavior associated to mental and health conditions. Curr. Med. Chem. 24, 411–423. doi: 10.2174/0929867323666161101140908
Barreto, G. E., Iarkov, A., and Moran, V. E. (2014). Beneficial effects of nicotine, cotinine and its metabolites as potential agents for Parkinson's disease. Front. Aging Neurosci. 6:340. doi: 10.3389/fnagi.2014.00340
Barreto, G. E., Yarkov, A., Avila-Rodriguez, M., Aliev, G., and Echeverria, V. (2015). Nicotine-derived compounds as therapeutic tools against post-traumatic stress disorder. Curr. Pharm. Des. 21, 3589–3595. doi: 10.2174/1381612821666150710145250
Bastianini, S., Lo Martire, V., Alvente, S., Berteotti, C., Matteoli, G., Rullo, L., et al. (2021). Early-life nicotine or cotinine exposure produces long-lasting sleep alterations and downregulation of hippocampal corticosteroid receptors in adult mice. Sci. Rep. 11:23897. doi: 10.1038/s41598-021-03468-5
Bathini, P., Dupanloup, I., Zenaro, E., Terrabuio, E., Fischer, A., Ballabani, E., et al. (2023). Systemic inflammation causes microglial dysfunction with a vascular AD phenotype. Brain Behav. Immun. Health 28:100568. doi: 10.1016/j.bbih.2022.100568
Bell, C. C., Han, V. Z., Sugawara, Y., and Grant, K. (1997). Synaptic plasticity in a cerebellum-like structure depends on temporal order. Nature 387, 278–281. doi: 10.1038/387278a0
Bi, G. Q. (2002). Spatiotemporal specificity of synaptic plasticity: cellular rules and mechanisms. Biol. Cybern. 87, 319–332. doi: 10.1007/s00422-002-0349-7
Bi, G. Q., and Poo, M. M. (1998). Synaptic modifications in cultured hippocampal neurons: dependence on spike timing, synaptic strength, and postsynaptic cell type. J. Neurosci. 18, 10464–10472. doi: 10.1523/JNEUROSCI.18-24-10464.1998
Blanco-Rambo, E., Izquierdo, M., and Cadore, E. L. (2023). Letter to the editor: dance as an intervention to improve physical and cognitive functioning in older adults. J. Nutr. Health Aging 27, 75–76. doi: 10.1007/s12603-022-1873-x
Blinkouskaya, Y., Cacoilo, A., Gollamudi, T., Jalalian, S., and Weickenmeier, J. (2021). Brain aging mechanisms with mechanical manifestations. Mech. Ageing Dev. 200:111575. doi: 10.1016/j.mad.2021.111575
Blinkouskaya, Y., and Weickenmeier, J. (2021). Brain shape changes associated with cerebral atrophy in healthy aging and Alzheimer's disease. Front. Mech. Eng. 7:705653. doi: 10.3389/fmech.2021.705653
Bliss, T. V., and Collingridge, G. L. (1993). A synaptic model of memory: long-term potentiation in the hippocampus. Nature 361, 31–39. doi: 10.1038/361031a0
Boiangiu, R. S., Mihasan, M., Gorgan, D. L., Stache, B. A., and Hritcu, L. (2021). Anxiolytic, Promnesic, anti-acetylcholinesterase and antioxidant effects of cotinine and 6-Hydroxy-L-nicotine in scopolamine-induced zebrafish (Danio rerio) model of Alzheimer's disease. Antioxidants (Basel) 10:212. doi: 10.3390/antiox10020212
Boldrini, M., Fulmore, C. A., Tartt, A. N., Simeon, L. R., Pavlova, I., Poposka, V., et al. (2018). Human hippocampal neurogenesis persists throughout aging. Cell Stem Cell 22, 589–599 e5. doi: 10.1016/j.stem.2018.03.015
Bourgognon, J. M., and Cavanagh, J. (2020). The role of cytokines in modulating learning and memory and brain plasticity. Brain Neurosci. Adv. 4:2398212820979802. doi: 10.1177/2398212820979802
Bowirrat, A. (2022). Immunosenescence and aging: neuroinflammation is a prominent feature of Alzheimer's disease and is a likely contributor to neurodegenerative disease pathogenesis. J. Pers. Med. 12:1817. doi: 10.3390/jpm12111817
Bozon, B., Kelly, A., Josselyn, S. A., Silva, A. J., Davis, S., and Laroche, S. (2003). MAPK, CREB and zif268 are all required for the consolidation of recognition memory. Philos. Trans. R. Soc. Lond. Ser. B Biol. Sci. 358, 805–814. doi: 10.1098/rstb.2002.1224
Brashers-Krug, T., Shadmehr, R., and Bizzi, E. (1996). Consolidation in human motor memory. Nature 382, 252–255. doi: 10.1038/382252a0
Brett, B. L., Kramer, M. D., Whyte, J., McCrea, M. A., Stein, M. B., Giacino, J. T., et al. (2021). Latent profile analysis of neuropsychiatric symptoms and cognitive function of adults 2 weeks after traumatic brain injury: findings from the TRACK-TBI study. JAMA Netw. Open 4:e213467. doi: 10.1001/jamanetworkopen.2021.3467
Bruce, D. (2001). Fifty years since Lashley's in search of the engram: refutations and conjectures. J. Hist. Neurosci. 10, 308–318. doi: 10.1076/jhin.10.3.308.9086
Bubser, M., Byun, N., Wood, M. R., and Jones, C. K. (2012). Muscarinic receptor pharmacology and circuitry for the modulation of cognition. Handb. Exp. Pharmacol. 37, 121–166. doi: 10.1007/978-3-642-23274-9_7
Caldarone, B. J., Duman, C. H., and Picciotto, M. R. (2000). Fear conditioning and latent inhibition in mice lacking the high affinity subclass of nicotinic acetylcholine receptors in the brain. Neuropharmacology 39, 2779–2784. doi: 10.1016/S0028-3908(00)00137-4
Cameron, H. A., and Glover, L. R. (2015). Adult neurogenesis: beyond learning and memory. Annu. Rev. Psychol. 66, 53–81. doi: 10.1146/annurev-psych-010814-015006
Cameron, H. A., and McKay, R. D. (2001). Adult neurogenesis produces a large pool of new granule cells in the dentate gyrus. J. Comp. Neurol. 435, 406–417. doi: 10.1002/cne.1040
Campbell, N. R., Fernandes, C. C., Halff, A. W., and Berg, D. K. (2010). Endogenous signaling through alpha7-containing nicotinic receptors promotes maturation and integration of adult-born neurons in the hippocampus. J. Neurosci. 30, 8734–8744. doi: 10.1523/JNEUROSCI.0931-10.2010
Castellano, C., Cestari, V., and Ciamei, A. (2001). NMDA receptors and learning and memory processes. Curr. Drug Targets 2, 273–283. doi: 10.2174/1389450013348515
Chang, C. J. (2017). Bioinorganic life and neural activity: toward a chemistry of consciousness? Acc. Chem. Res. 50, 535–538. doi: 10.1021/acs.accounts.6b00531
Chen, P., Guo, Z., and Zhou, B. (2023). Insight into the role of adult hippocampal neurogenesis in aging and Alzheimer's disease. Ageing Res. Rev. 84:101828. doi: 10.1016/j.arr.2022.101828
Chen, T., Wang, Y., Zhang, T., Zhang, B., Chen, L., Zhao, L., et al. (2018). Simvastatin enhances activity and trafficking of alpha7 nicotinic acetylcholine receptor in hippocampal neurons through PKC and CaMKII signaling pathways. Front. Pharmacol. 9:362. doi: 10.3389/fphar.2018.00362
Choi, M. L., and Gandhi, S. (2018). Crucial role of protein oligomerization in the pathogenesis of Alzheimer's and Parkinson's diseases. FEBS J. 285, 3631–3644. doi: 10.1111/febs.14587
Choi, D. I., and Kaang, B. K. (2022). Interrogating structural plasticity among synaptic engrams. Curr. Opin. Neurobiol. 75:102552. doi: 10.1016/j.conb.2022.102552
Choi, D. I., Kim, J., Lee, H., Kim, J. I., Sung, Y., Choi, J. E., et al. (2021). Synaptic correlates of associative fear memory in the lateral amygdala. Neuron 109, 2717–2726 e3. doi: 10.1016/j.neuron.2021.07.003
Choi, J. H., Sim, S. E., Kim, J. I., Choi, D. I., Oh, J., Ye, S., et al. (2018). Interregional synaptic maps among engram cells underlie memory formation. Science 360, 430–435. doi: 10.1126/science.aas9204
Choi, S. H., and Tanzi, R. E. (2023). Adult neurogenesis in Alzheimer's disease. Hippocampus. 33, 307–321. doi: 10.1002/hipo.23504
Clelland, C. D., Choi, M., Romberg, C., Clemenson, G. D. Jr., Fragniere, A., Tyers, P., et al. (2009). A functional role for adult hippocampal neurogenesis in spatial pattern separation. Science 325, 210–213. doi: 10.1126/science.1173215
Clemenson, G. D., and Stark, C. E. (2015). Virtual environmental enrichment through video games improves hippocampal-associated memory. J. Neurosci. 35, 16116–16125. doi: 10.1523/JNEUROSCI.2580-15.2015
Cordero-Erausquin, M., Marubio, L. M., Klink, R., and Changeux, J. P. (2000). Nicotinic receptor function: new perspectives from knockout mice. Trends Pharmacol. Sci. 21, 211–217. doi: 10.1016/S0165-6147(00)01489-9
Coyle, J., and Kershaw, P. (2001). Galantamine, a cholinesterase inhibitor that allosterically modulates nicotinic receptors: effects on the course of Alzheimer's disease. Biol. Psychiatry 49, 289–299. doi: 10.1016/S0006-3223(00)01101-X
Criado-Marrero, M., Rein, T., Binder, E. B., Porter, J. T., Koren, J. 3rd, and Blair, L. J. (2018). Hsp90 and FKBP51: complex regulators of psychiatric diseases. Philos. Trans. R. Soc. Lond. Ser. B Biol. Sci. 373:20160532. doi: 10.1098/rstb.2016.0532
Curlik, D. M. 2nd, and Shors, T. J. (2013). Training your brain: do mental and physical (MAP) training enhance cognition through the process of neurogenesis in the hippocampus? Neuropharmacology 64, 506–514. doi: 10.1016/j.neuropharm.2012.07.027
Curtis, M. A., Low, V. F., and Faull, R. L. (2012). Neurogenesis and progenitor cells in the adult human brain: a comparison between hippocampal and subventricular progenitor proliferation. Dev. Neurobiol. 72, 990–1005. doi: 10.1002/dneu.22028
Dan, Y., and Poo, M. M. (2004). Spike timing-dependent plasticity of neural circuits. Neuron 44, 23–30. doi: 10.1016/j.neuron.2004.09.007
Dar, M. S., Li, C., and Bowman, E. R. (1993). Central behavioral interactions between ethanol, (−)-nicotine, and (−)-cotinine in mice. Brain Res. Bull. 32, 23–28. doi: 10.1016/0361-9230(93)90314-2
Das, S., Moon, H. C., Singer, R. H., and Park, H. Y. (2018). A transgenic mouse for imaging activity-dependent dynamics of endogenous arc mRNA in live neurons. Sci. Adv. 4:eaar3448. doi: 10.1126/sciadv.aar3448
Davis, J. A., and Gould, T. J. (2007). beta2 subunit-containing nicotinic receptors mediate the enhancing effect of nicotine on trace cued fear conditioning in C57BL/6 mice. Psychopharmacology 190, 343–352. doi: 10.1007/s00213-006-0624-8
Day, J. J., Kennedy, A. J., and Sweatt, J. D. (2015). DNA methylation and its implications and accessibility for neuropsychiatric therapeutics. Annu. Rev. Pharmacol. Toxicol. 55, 591–611. doi: 10.1146/annurev-pharmtox-010814-124527
Day, J. J., and Sweatt, J. D. (2011). Epigenetic mechanisms in cognition. Neuron 70, 813–829. doi: 10.1016/j.neuron.2011.05.019
Devick, K. L., Sprung, J., Mielke, M., Petersen, R. C., and Schulte, P. J. (2020). Association between surgery with anesthesia and cognitive decline in older adults: analysis using shared parameter models for informative dropout. J. Clin. Transl. Sci. 5:e27. doi: 10.1017/cts.2020.519
Dinius, C. J., Pocknell, C. E., Caffrey, M. P., and Roche, R. A. P. (2023). Cognitive interventions for memory and psychological well-being in aging and dementias. Front. Psychol. 14:1070012. doi: 10.3389/fpsyg.2023.1070012
Doetsch, F., Garcia-Verdugo, J. M., and Alvarez-Buylla, A. (1997). Cellular composition and three-dimensional organization of the subventricular germinal zone in the adult mammalian brain. J. Neurosci. 17, 5046–5061. doi: 10.1523/JNEUROSCI.17-13-05046.1997
Doetsch, F., Garcia-Verdugo, J. M., and Alvarez-Buylla, A. (1999). Regeneration of a germinal layer in the adult mammalian brain. Proc. Natl. Acad. Sci. U. S. A. 96, 11619–11624. doi: 10.1073/pnas.96.20.11619
Doetsch, F., and Hen, R. (2005). Young and excitable: the function of new neurons in the adult mammalian brain. Curr. Opin. Neurobiol. 15, 121–128. doi: 10.1016/j.conb.2005.01.018
Doetsch, F., and Scharff, C. (2001). Challenges for brain repair: insights from adult neurogenesis in birds and mammals. Brain Behav. Evol. 58, 306–322. doi: 10.1159/000057572
Dranovsky, A., Kirshenbaum, G., Chang, C. Y., Bompolaki, M., Bradford, V., Bell, J., et al. (2023). Adult-born neurons maintain hippocampal cholinergic inputs and support working memory during aging. Res. Sq. rs.3.rs-1851645. doi: 10.21203/rs.3.rs-1851645/v1
Echeverria, V. (2016). Neuroinflammation a common link in neurodegenerative neurological and psychiatric disorders. Curr. Pharm. Des. 22:1293. doi: 10.2174/1381612822999151228105750
Echeverria, V., Barreto, G. E., Avila-Rodriguezc, M., Tarasov, V. V., and Aliev, G. (2017). Is VEGF a key target of cotinine and other potential therapies against Alzheimer disease? Curr. Alzheimer Res. 14, 1155–1163. doi: 10.2174/1567205014666170329113007
Echeverria, V., Berman, D. E., and Arancio, O. (2007). Oligomers of beta-amyloid peptide inhibit BDNF-induced arc expression in cultured cortical neurons. Curr. Alzheimer Res. 4, 518–521. doi: 10.2174/156720507783018190
Echeverria, V., Burgess, S., Gamble-George, J., Zeitlin, R., Lin, X., Cao, C., et al. (2009). Sorafenib inhibits nuclear factor kappa B, decreases inducible nitric oxide synthase and cyclooxygenase-2 expression, and restores working memory in APPswe mice. Neuroscience 162, 1220–1231. doi: 10.1016/j.neuroscience.2009.05.019
Echeverria, V., and Cuello, A. C. (2002). Intracellular A-beta amyloid, a sign for worse things to come? Mol. Neurobiol. 26, 299–316. doi: 10.1385/MN:26:2-3:299
Echeverria, V., Dowd, E., Ducatenzeiler, A., Grant, S., Szyf, M., Cuello, A., et al. (2002). A rat transgenic model expressing human amyloidgenic a beta peptide intraneuronarly in the hippocampus and cortex shows up-regulation of activated MAPKs and tau phosphorylation. Neurobiol. Aging 23:S235. doi: 10.3233/JAD-2010-1349
Echeverria, V., Ducatenzeiler, A., Dowd, E., Janne, J., Grant, S. M., Szyf, M., et al. (2004). Altered mitogen-activated protein kinase signaling, tau hyperphosphorylation and mild spatial learning dysfunction in transgenic rats expressing the beta-amyloid peptide intracellularly in hippocampal and cortical neurons. Neuroscience 129, 583–592. doi: 10.1016/j.neuroscience.2004.07.036
Echeverria, V., Grizzell, J. A., and Barreto, G. E. (2016a). Neuroinflammation: a therapeutic target of cotinine for the treatment of psychiatric disorders? Curr. Pharm. Des. 22, 1324–1333. doi: 10.2174/138161282210160304112511
Echeverria, V., Yarkov, A., and Aliev, G. (2016b). Positive modulators of the alpha7 nicotinic receptor against neuroinflammation and cognitive impairment in Alzheimer's disease. Prog. Neurobiol. 144, 142–157. doi: 10.1016/j.pneurobio.2016.01.002
Egea, J., Buendia, I., Parada, E., Navarro, E., Leon, R., and Lopez, M. G. (2015). Anti-inflammatory role of microglial alpha7 nAChRs and its role in neuroprotection. Biochem. Pharmacol. 97, 463–472. doi: 10.1016/j.bcp.2015.07.032
Engler-Chiurazzi, E. B., Russell, A. E., Povroznik, J. M., McDonald, K. O., Porter, K. N., Wang, D. S., et al. (2023). Intermittent systemic exposure to lipopolysaccharide-induced inflammation disrupts hippocampal long-term potentiation and impairs cognition in aging male mice. Brain Behav. Immun. 108, 279–291. doi: 10.1016/j.bbi.2022.12.013
Fazel, M., Stratford, H. J., Rowsell, E., Chan, C., Griffiths, H., and Robjant, K. (2020). Five applications of narrative exposure therapy for children and adolescents presenting with post-traumatic stress disorders. Front. Psychiatry 11:19. doi: 10.3389/fpsyt.2020.00019
Feldman, D. E. (2009). Synaptic mechanisms for plasticity in neocortex. Annu. Rev. Neurosci. 32, 33–55. doi: 10.1146/annurev.neuro.051508.135516
Feldman, D. E. (2012). The spike-timing dependence of plasticity. Neuron 75, 556–571. doi: 10.1016/j.neuron.2012.08.001
Fioriti, L., Myers, C., Huang, Y. Y., Li, X., Stephan, J. S., Trifilieff, P., et al. (2015). The persistence of hippocampal-based memory requires protein synthesis mediated by the prion-like protein CPEB3. Neuron 86, 1433–1448. doi: 10.1016/j.neuron.2015.05.021
Foster, T. C., Sharrow, K. M., Kumar, A., and Masse, J. (2003). Interaction of age and chronic estradiol replacement on memory and markers of brain aging. Neurobiol. Aging 24, 839–852. doi: 10.1016/S0197-4580(03)00014-9
Foucault-Fruchard, L., and Antier, D. (2017). Therapeutic potential of alpha7 nicotinic receptor agonists to regulate neuroinflammation in neurodegenerative diseases. Neural Regen. Res. 12, 1418–1421. doi: 10.4103/1673-5374.215244
Francis, A. G., Elhadd, K., Camera, V., Ferreira Dos Santos, M., Rocchi, C., Adib-Samii, P., et al. (2023). Acute inflammatory diseases of the central nervous system after SARS-CoV-2 vaccination. Neurol. Neuroimmunol. Neuroinflamm. 10:e200063. doi: 10.1212/NXI.0000000000200063
Frey, J. U. (2001). Long-lasting hippocampal plasticity: cellular model for memory consolidation? Results Probl. Cell Differ. 34, 27–40. doi: 10.1007/978-3-540-40025-7_2
Froemke, R. C., Letzkus, J. J., Kampa, B. M., Hang, G. B., and Stuart, G. J. (2010). Dendritic synapse location and neocortical spike-timing-dependent plasticity. Front. Synaptic Neurosci. 2:29. doi: 10.3389/fnsyn.2010.00029
Fuenzalida, M., Chiu, C. Q., and Chavez, A. E. (2020). Muscarinic regulation of spike timing dependent synaptic plasticity in the hippocampus. Neuroscience 456, 50–59. doi: 10.1016/j.neuroscience.2020.08.015
Fuxe, K., Canals, M., Torvinen, M., Marcellino, D., Terasmaa, A., Genedani, S., et al. (2007). Intramembrane receptor-receptor interactions: a novel principle in molecular medicine. J. Neural Transm. (Vienna) 114, 49–75. doi: 10.1007/s00702-006-0589-0
Galvin, V. C., Arnsten, A. F. T., and Wang, M. (2020). Involvement of nicotinic receptors in working memory function. Curr. Top. Behav. Neurosci. 45, 89–99. doi: 10.1007/7854_2020_142
Gandelman, E. M., Miller, S. A., and Back, S. E. (2022). Imaginal exposure processing during concurrent treatment of PTSD and substance use disorders using prolonged exposure (COPE) therapy: examination of linguistic markers of cohesiveness. J. Trauma. Stress. 35, 682–693. doi: 10.1002/jts.22786
Garad, M., Edelmann, E., and Lessmann, V. (2021). Impairment of spike-timing-dependent plasticity at Schaffer collateral-CA1 synapses in adult APP/PS1 mice depends on proximity of Abeta plaques. Int. J. Mol. Sci. 22:1378. doi: 10.3390/ijms22031378
Gasiorowska, A., Wydrych, M., Drapich, P., Zadrozny, M., Steczkowska, M., Niewiadomski, W., et al. (2021). The biology and pathobiology of glutamatergic, cholinergic, and dopaminergic signaling in the aging brain. Front. Aging Neurosci. 13:654931. doi: 10.3389/fnagi.2021.654931
Geerts, H. (2012). α7 nicotinic receptor modulators for cognitive deficits in schizophrenia and Alzheimer's disease. Expert Opin. Investig. Drugs 21, 59–65. doi: 10.1517/13543784.2012.633510
Ghosh-Swaby, O. R., Reichelt, A. C., Sheppard, P. A. S., Davies, J., Bussey, T. J., and Saksida, L. M. (2022). Metabolic hormones mediate cognition. Front. Neuroendocrinol. 66:101009. doi: 10.1016/j.yfrne.2022.101009
Gilboa, A., and Moscovitch, M. (2021). No consolidation without representation: correspondence between neural and psychological representations in recent and remote memory. Neuron 109, 2239–2255. doi: 10.1016/j.neuron.2021.04.025
Girod, R., Barazangi, N., McGehee, D., and Role, L. W. (2000). Facilitation of glutamatergic neurotransmission by presynaptic nicotinic acetylcholine receptors. Neuropharmacology 39, 2715–2725. doi: 10.1016/S0028-3908(00)00145-3
Gould, E., Tanapat, P., Rydel, T., and Hastings, N. (2000). Regulation of hippocampal neurogenesis in adulthood. Biol. Psychiatry 48, 715–720. doi: 10.1016/S0006-3223(00)01021-0
Grizzell, J. A., and Echeverria, V. (2015). New insights into the mechanisms of action of cotinine and its distinctive effects from nicotine. Neurochem. Res. 40, 2032–2046. doi: 10.1007/s11064-014-1359-2
Grizzell, J. A., Iarkov, A., Holmes, R., Mori, T., and Echeverria, V. (2014a). Cotinine reduces depressive-like behavior, working memory deficits, and synaptic loss associated with chronic stress in mice. Behav. Brain Res. 268, 55–65. doi: 10.1016/j.bbr.2014.03.047
Grizzell, J. A., Mullins, M., Iarkov, A., Rohani, A., Charry, L. C., and Echeverria, V. (2014b). Cotinine reduces depressive-like behavior and hippocampal vascular endothelial growth factor downregulation after forced swim stress in mice. Behav. Neurosci. 128, 713–721. doi: 10.1037/bne0000021
Grizzell, J. A., Patel, S., Barreto, G. E., and Echeverria, V. (2017). Cotinine improves visual recognition memory and decreases cortical tau phosphorylation in the Tg6799 mice. Prog. Neuro-Psychopharmacol. Biol. Psychiatry 78, 75–81. doi: 10.1016/j.pnpbp.2017.05.010
Gu, S., Li, Y., Jiang, Y., Huang, J. H., and Wang, F. (2022). Glymphatic dysfunction induced oxidative stress and neuro-inflammation in major depression disorders. Antioxidants (Basel) 11:2296. doi: 10.3390/antiox11112296
Gutierrez-Quintana, R., Walker, D. J., Williams, K. J., Forster, D. M., and Chalmers, A. J. (2022). Radiation-induced neuroinflammation: a potential protective role for poly(ADP-ribose) polymerase inhibitors? Neurooncol. Adv. 4:vdab190. doi: 10.1093/noajnl/vdab190
Haam, J., and Yakel, J. L. (2017). Cholinergic modulation of the hippocampal region and memory function. J. Neurochem. 142, 111–121. doi: 10.1111/jnc.14052
Han, D. H., Park, P., Choi, D. I., Bliss, T. V. P., and Kaang, B. K. (2022). The essence of the engram: cellular or synaptic? Semin. Cell Dev. Biol. 125, 122–135. doi: 10.1016/j.semcdb.2021.05.033
Harrington, Y. A., Parisi, J. M., Duan, D., Rojo-Wissar, D. M., Holingue, C., and Spira, A. P. (2022). Sex hormones, sleep, and memory: interrelationships across the adult female lifespan. Front. Aging Neurosci. 14:800278. doi: 10.3389/fnagi.2022.800278
Harrist, A., Beech, R. D., King, S. L., Zanardi, A., Cleary, M. A., Caldarone, B. J., et al. (2004). Alteration of hippocampal cell proliferation in mice lacking the beta 2 subunit of the neuronal nicotinic acetylcholine receptor. Synapse 54, 200–206. doi: 10.1002/syn.20081
Hastings, N., and Gould, E. (1999). Erratum: rapid extension of axons into the CA3 region by adult-generated granule cells. J. Comp. Neurol. 413, 146–154. doi: 10.1002/(sici)1096-9861(19991011)413:1<146::aid-cne10>3.0.co;2-b
Hatsukami, D., Anton, D., Keenan, R., and Callies, A. (1992). Smokeless tobacco abstinence effects and nicotine gum dose. Psychopharmacology 106, 60–66. doi: 10.1007/BF02253589
Hatsukami, D. K., Grillo, M., Pentel, P. R., Oncken, C., and Bliss, R. (1997). Safety of cotinine in humans: physiologic, subjective, and cognitive effects. Pharmacol. Biochem. Behav. 57, 643–650. doi: 10.1016/S0091-3057(97)80001-9
Hatsukami, D., Lexau, B., Nelson, D., Pentel, P. R., Sofuoglu, M., and Goldman, A. (1998a). Effects of cotinine on cigarette self-administration. Psychopharmacology 138, 184–189. doi: 10.1007/s002130050661
Hatsukami, D., Pentel, P. R., Jensen, J., Nelson, D., Allen, S. S., Goldman, A., et al. (1998b). Cotinine: effects with and without nicotine. Psychopharmacology 135, 141–150. doi: 10.1007/s002130050495
Henderson, V. W. (2011). Gonadal hormones and cognitive aging: a midlife perspective. Women's Health (Lond. Engl.) 7, 81–93. doi: 10.2217/WHE.10.87
Heneka, M. T., Carson, M. J., Khoury, J. E., Landreth, G. E., Brosseron, F., Feinstein, D. L., et al. (2015). Neuroinflammation in Alzheimer's disease. Lancet Neurol. 14, 388–405. doi: 10.1016/S1474-4422(15)70016-5
Hernandez, C. M., and Dineley, K. T. (2012). alpha7 nicotinic acetylcholine receptors in Alzheimer's disease: neuroprotective, neurotrophic or both? Curr. Drug Targets 13, 613–622. doi: 10.2174/138945012800398973
Hernandez-Lopez, S., Garduno, J., and Mihailescu, S. (2013). Nicotinic modulation of serotonergic activity in the dorsal raphe nucleus. Rev. Neurosci. 24, 455–469. doi: 10.1515/revneuro-2013-0012
Hernandez-Miranda, L. R., Parnavelas, J. G., and Chiara, F. (2010). Molecules and mechanisms involved in the generation and migration of cortical interneurons. ASN Neuro 2:e00031. doi: 10.1042/AN20090053
Hill, A. M. (2022). Alzheimer disease and the evolving treatment landscape. Am. J. Manag. Care 28, S179–S187. doi: 10.37765/ajmc.2022.89235
Hilund-Carlsen, P. F., Revheim, M. E., Alavi, A., Satyamurthy, N., and Barrio, J. R. (2022). Amyloid PET: a questionable single primary surrogate efficacy measure on Alzheimer immunotherapy trials. J. Alzheimers Dis. 90, 1395–1399. doi: 10.3233/JAD-220841
Hogervorst, E., Temple, S., and O'Donnell, E. (2023). Sex differences in dementia. Curr. Top. Behav. Neurosci. 62, 309–331. doi: 10.1007/7854_2022_408
Hohman, T. J., Bell, S. P., and Jefferson, A. L., I. Alzheimer's Disease Neuroimaging (2015). The role of vascular endothelial growth factor in neurodegeneration and cognitive decline: exploring interactions with biomarkers of Alzheimer disease. JAMA Neurol. 72, 520–529. doi: 10.1001/jamaneurol.2014.4761
Holmes, M. M. (2016). Social regulation of adult neurogenesis: a comparative approach. Front. Neuroendocrinol. 41, 59–70. doi: 10.1016/j.yfrne.2016.02.001
Hsu, C. K., Ney, L. J., Honan, C., and Felmingham, K. L. (2021). Gonadal steroid hormones and emotional memory consolidation: a systematic review and meta-analysis. Neurosci. Biobehav. Rev. 130, 529–542. doi: 10.1016/j.neubiorev.2021.09.010
Iarkov, A., Barreto, G. E., Grizzell, J. A., and Echeverria, V. (2020). Strategies for the treatment of Parkinson's disease: beyond dopamine. Front. Aging Neurosci. 12:4. doi: 10.3389/fnagi.2020.00004
Ijomone, O. M., and Nwoha, P. U. (2015). Nicotine inhibits hippocampal and striatal acetylcholinesterase activities, and demonstrates dual action on adult neuronal proliferation and maturation. Pathophysiology 22, 231–239. doi: 10.1016/j.pathophys.2015.09.002
Imabayashi, E., Saitoh, Y., Tsukamoto, T., Sakata, M., and Takano, H. (2022). Combination of Astrogliosis and phosphorylated tau for the preclinical diagnosis of Alzheimer disease using 3-dimensional stereotactic surface projection images with 18F-THK5351. Clin. Nucl. Med. 47, 1066–1068. doi: 10.1097/RLU.0000000000004425
Ishii, D., Matsuzawa, D., Matsuda, S., Tomizawa, H., Sutoh, C., and Shimizu, E. (2012). No erasure effect of retrieval-extinction trial on fear memory in the hippocampus-independent and dependent paradigms. Neurosci. Lett. 523, 76–81. doi: 10.1016/j.neulet.2012.06.048
Izquierdo, I., and Medina, J. H. (1997). Memory formation: the sequence of biochemical events in the hippocampus and its connection to activity in other brain structures. Neurobiol. Learn. Mem. 68, 285–316. doi: 10.1006/nlme.1997.3799
Jana, S., Dines, M., Lalzar, M., and Lamprecht, R. (2023). Fear conditioning leads to enduring alterations in RNA transcripts in hippocampal neuropil that are dependent on EphB2 forward signaling. Mol. Neurobiol. 60, 2320–2329. doi: 10.1007/s12035-022-03191-w
Jett, S., Dyke, J. P., Andy, C., Schelbaum, E., Jang, G., Boneu Yepez, C., et al. (2022). Sex and menopause impact (31)P-magnetic resonance spectroscopy brain mitochondrial function in association with (11)C-PiB PET amyloid-beta load. Sci. Rep. 12:22087. doi: 10.1038/s41598-022-26573-5
John, D., Shelukhina, I., Yanagawa, Y., Deuchars, J., and Henderson, Z. (2015). Functional alpha7 nicotinic receptors are expressed on immature granule cells of the postnatal dentate gyrus. Brain Res. 1601, 15–30. doi: 10.1016/j.brainres.2014.12.041
Jones, K. A., and Thomsen, C. (2013). The role of the innate immune system in psychiatric disorders. Mol. Cell. Neurosci. 53, 52–62. doi: 10.1016/j.mcn.2012.10.002
Josselyn, S. A., Kida, S., and Silva, A. J. (2004). Inducible repression of CREB function disrupts amygdala-dependent memory. Neurobiol. Learn. Mem. 82, 159–163. doi: 10.1016/j.nlm.2004.05.008
Josselyn, S. A., and Nguyen, P. V. (2005). CREB, synapses and memory disorders: past progress and future challenges. Curr. Drug Targets CNS Neurol. Disord. 4, 481–497. doi: 10.2174/156800705774322058
Josselyn, S. A., and Tonegawa, S. (2020). Memory engrams: recalling the past and imagining the future. Science 367:eaaw4325. doi: 10.1126/science.aaw4325
Jurgensen, S., and Ferreira, S. T. (2010). Nicotinic receptors, amyloid-beta, and synaptic failure in Alzheimer's disease. J. Mol. Neurosci. 40, 221–229. doi: 10.1007/s12031-009-9237-0
Kageyama, R., Imayoshi, I., and Sakamoto, M. (2012). The role of neurogenesis in olfaction-dependent behaviors. Behav. Brain Res. 227, 459–463. doi: 10.1016/j.bbr.2011.04.038
Kalra, S., Malik, R., Singh, G., Bhatia, S., Al-Harrasi, A., Mohan, S., et al. (2022). Pathogenesis and management of traumatic brain injury (TBI): role of neuroinflammation and anti-inflammatory drugs. Inflammopharmacology 30, 1153–1166. doi: 10.1007/s10787-022-01017-8
Kampa, B. M., Letzkus, J. J., and Stuart, G. J. (2006). Requirement of dendritic calcium spikes for induction of spike-timing-dependent synaptic plasticity. J. Physiol. 574, 283–290. doi: 10.1113/jphysiol.2006.111062
Kampa, B. M., Letzkus, J. J., and Stuart, G. J. (2007). Dendritic mechanisms controlling spike-timing-dependent synaptic plasticity. Trends Neurosci. 30, 456–463. doi: 10.1016/j.tins.2007.06.010
Kandel, E. R. (1997). Genes, synapses, and long-term memory. J. Cell. Physiol. 173, 124–125. doi: 10.1002/(SICI)1097-4652(199711)173:2<124::AID-JCP6>3.0.CO;2-P
Kandel, E. R. (2001). The molecular biology of memory storage: a dialogue between genes and synapses. Science 294, 1030–1038. doi: 10.1126/science.1067020
Kandel, E. R., Dudai, Y., and Mayford, M. R. (2014). The molecular and systems biology of memory. Cells 157, 163–186. doi: 10.1016/j.cell.2014.03.001
Kandel, E. R., and Schwartz, J. H. (1982). Molecular biology of learning: modulation of transmitter release. Science 218, 433–443. doi: 10.1126/science.6289442
Kanoni, S., Graham, S. E., Wang, Y., Surakka, I., Ramdas, S., Zhu, X., et al. (2022). Implicating genes, pleiotropy, and sexual dimorphism at blood lipid loci through multi-ancestry meta-analysis. Genome Biol. 23:268. doi: 10.1186/s13059-022-02837-1
Karadsheh, M. S., Shah, M. S., Tang, X., Macdonald, R. L., and Stitzel, J. A. (2004). Functional characterization of mouse alpha4beta2 nicotinic acetylcholine receptors stably expressed in HEK293T cells. J. Neurochem. 91, 1138–1150. doi: 10.1111/j.1471-4159.2004.02801.x
Kavanagh, S., Van Baelen, B., and Schauble, B. (2011). Long-term effects of galantamine on cognitive function in Alzheimer's disease: a large-scale international retrospective study. J. Alzheimers Dis. 27, 521–530. doi: 10.3233/JAD-2011-110417
Keenan, R. M., Hatsukami, D. K., Pentel, P. R., Thompson, T. N., and Grillo, M. A. (1994). Pharmacodynamic effects of cotinine in abstinent cigarette smokers. Clin. Pharmacol. Ther. 55, 581–590. doi: 10.1038/clpt.1994.72
Kempermann, G., Gage, F. H., Aigner, L., Song, H., Curtis, M. A., Thuret, S., et al. (2018). Human adult neurogenesis: evidence and remaining questions. Cell Stem Cell 23, 25–30. doi: 10.1016/j.stem.2018.04.004
Kennedy, C. C., Hewston, P., Ioannidis, G., Egbujie, B., Marr, S., Negm, A., et al. (2022). Effect of the GERAS DANcing for cognition and exercise program on physical function in older adults. J. Aging Phys. Act. 31, 182–190. doi: 10.1123/japa.2021-0504
Kenney, J. W., and Gould, T. J. (2008). Modulation of hippocampus-dependent learning and synaptic plasticity by nicotine. Mol. Neurobiol. 38, 101–121. doi: 10.1007/s12035-008-8037-9
Kepecs, A., van Rossum, M. C., Song, S., and Tegner, J. (2002). Spike-timing-dependent plasticity: common themes and divergent vistas. Biol. Cybern. 87, 446–458. doi: 10.1007/s00422-002-0358-6
Kerstens, C., Wildiers, H., Schroyen, G., Almela, M., Mark, R. E., Lambrecht, M., et al. (2023). A systematic review on the potential acceleration of neurocognitive aging in older cancer survivors. Cancers (Basel) 15:1215. doi: 10.3390/cancers15041215
Kihara, T., Shimohama, S., Sawada, H., Honda, K., Nakamizo, T., Shibasaki, H., et al. (2001). Alpha 7 nicotinic receptor transduces signals to phosphatidylinositol 3-kinase to block a beta-amyloid-induced neurotoxicity. J. Biol. Chem. 276, 13541–13546. doi: 10.1074/jbc.M008035200
Kim, J. I., Choi, D. I., and Kaang, B. K. (2018). Strengthened connections between engrams encode specific memories. BMB Rep. 51, 369–370. doi: 10.5483/BMBRep.2018.51.8.176
Kling, J. M., Dowling, N. M., Bimonte-Nelson, H., Gleason, C. E., Kantarci, K., Stonnington, C. M., et al. (2023). Associations between pituitary-ovarian hormones and cognition in recently menopausal women independent of type of hormone therapy. Maturitas 167, 113–122. doi: 10.1016/j.maturitas.2022.10.002
Kotani, S., Yamauchi, T., Teramoto, T., and Ogura, H. (2006). Pharmacological evidence of cholinergic involvement in adult hippocampal neurogenesis in rats. Neuroscience 142, 505–514. doi: 10.1016/j.neuroscience.2006.06.035
Krinke, E., Held, U., Steigmiller, K., Felmingham, K., and Kleim, B. (2022). Sex hormones and cortisol during experimental trauma memory consolidation: prospective association with intrusive memories. Eur. J. Psychotraumatol. 13:2040818. doi: 10.1080/20008198.2022.2040818
Kumar, H., More, S. V., Han, S. D., Choi, J. Y., and Choi, D. K. (2012). Promising therapeutics with natural bioactive compounds for improving learning and memory–a review of randomized trials. Molecules 17, 10503–10539. doi: 10.3390/molecules170910503
Kumar, A., Pareek, V., Faiq, M. A., Ghosh, S. K., and Kumari, C. (2019a). Adult neurogenesis in humans: a review of basic concepts, history, current research, and clinical implications. Innov. Clin. Neurosci. 16, 30–37.
Kumar, A., Pareek, V., Faiq, M. A., Kumar, P., Kumari, C., Singh, H. N., et al. (2020). Transcriptomic analysis of the signature of neurogenesis in human hippocampus suggests restricted progenitor cell progression post-childhood. IBRO Rep. 9, 224–232. doi: 10.1016/j.ibror.2020.08.003
Kumar, A., Thinschmidt, J. S., and Foster, T. C. (2019b). Subunit contribution to NMDA receptor hypofunction and redox sensitivity of hippocampal synaptic transmission during aging. Aging 11, 5140–5157. doi: 10.18632/aging.102108
Lacalle-Aurioles, M., and Iturria-Medina, Y. (2023). Fornix degeneration in risk factors of Alzheimer's disease, possible trigger of cognitive decline. Cereb. Circ. Cogn. Behav. 4:100158. doi: 10.1016/j.cccb.2023.100158
Lacasse, J. M., Boulos, V., Fisher, C., Hamilton, S., Heron, M., Mac Cionnaith, C. E., et al. (2023). Combined effects of the contraceptive hormones, ethinyl estradiol and levonorgestrel, on the use of place and response memory in gonadally-intact female rats. Psychoneuroendocrinology 147:105974. doi: 10.1016/j.psyneuen.2022.105974
Ladak, A. A., Enam, S. A., and Ibrahim, M. T. (2019). A review of the molecular mechanisms of traumatic brain injury. World Neurosurg. 131, 126–132. doi: 10.1016/j.wneu.2019.07.039
Landry, C. D., Kandel, E. R., and Rajasethupathy, P. (2013). New mechanisms in memory storage: piRNAs and epigenetics. Trends Neurosci. 36, 535–542. doi: 10.1016/j.tins.2013.05.004
Lee, C., Lee, B. H., Jung, H., Lee, C., Sung, Y., Kim, H., et al. (2023). Hippocampal engram networks for fear memory recruit new synapses and modify pre-existing synapses in vivo. Curr. Biol. 33, 507–516 e3. doi: 10.1016/j.cub.2022.12.038
Letzkus, J. J., Kampa, B. M., and Stuart, G. J. (2006). Learning rules for spike timing-dependent plasticity depend on dendritic synapse location. J. Neurosci. 26, 10420–10429. doi: 10.1523/JNEUROSCI.2650-06.2006
Letzkus, J. J., Kampa, B. M., and Stuart, G. J. (2007). Does spike timing-dependent synaptic plasticity underlie memory formation? Clin. Exp. Pharmacol. Physiol. 34, 1070–1076. doi: 10.1111/j.1440-1681.2007.04724.x
Leuner, B., Caponiti, J. M., and Gould, E. (2012). Oxytocin stimulates adult neurogenesis even under conditions of stress and elevated glucocorticoids. Hippocampus 22, 861–868. doi: 10.1002/hipo.20947
Li, M., Chen, X., Yang, Q., Cao, S., Wyler, S., Yuan, R., et al. (2023). Single-nucleus profiling of adult mice sub-ventricular zone after blast-related traumatic brain injury. Sci. Data 10:13. doi: 10.1038/s41597-022-01925-y
Li, K., Cui, C., Zhang, H., Jia, L., Li, R., and Hu, H. Y. (2022). Exploration of combined physical activity and music for patients with Alzheimer's disease: a systematic review. Front. Aging Neurosci. 14:962475. doi: 10.3389/fnagi.2022.1090665
Lin, D., Cao, L., Wang, Z., Li, J., Washington, J. M., and Zuo, Z. (2012). Lidocaine attenuates cognitive impairment after isoflurane anesthesia in old rats. Behav. Brain Res. 228, 319–327. doi: 10.1016/j.bbr.2011.12.010
Lin, H. C., Tseng, Y. C., Mao, S. C., Chen, P. S., and Gean, P. W. (2011). GABAA receptor endocytosis in the basolateral amygdala is critical to the reinstatement of fear memory measured by fear-potentiated startle. J. Neurosci. 31, 8851–8861. doi: 10.1523/JNEUROSCI.0979-11.2011
Lippa, S. M., Gill, J., Brickell, T. A., French, L. M., and Lange, R. T. (2021). Blood biomarkers relate to cognitive performance years after traumatic brain injury in service members and veterans. J. Int. Neuropsychol. Soc. 27, 508–514. doi: 10.1017/S1355617720001071
Lotfipour, S., Byun, J. S., Leach, P., Fowler, C. D., Murphy, N. P., Kenny, P. J., et al. (2013). Targeted deletion of the mouse alpha2 nicotinic acetylcholine receptor subunit gene (Chrna2) potentiates nicotine-modulated behaviors. J. Neurosci. 33, 7728–7741. doi: 10.1523/JNEUROSCI.4731-12.2013
Lykhmus, O., Voytenko, L., Koval, L., Mykhalskiy, S., Kholin, V., Peschana, K., et al. (2015). alpha7 nicotinic acetylcholine receptor-specific antibody induces inflammation and amyloid beta42 accumulation in the mouse brain to impair memory. PLoS One 10:e0122706. doi: 10.1371/journal.pone.0122706
Madrid, L. I., Jimenez-Martin, J., Coulson, E. J., and Jhaveri, D. J. (2021). Cholinergic regulation of adult hippocampal neurogenesis and hippocampus-dependent functions. Int. J. Biochem. Cell Biol. 134:105969. doi: 10.1016/j.biocel.2021.105969
Maelicke, A. (2000). Allosteric modulation of nicotinic receptors as a treatment strategy for Alzheimer's disease. Dement. Geriatr. Cogn. Disord. 11, 11–18. doi: 10.1159/000051227
Majdi, A., Kamari, F., Sadigh-Eteghad, S., and Gjedde, A. (2018). Molecular insights into memory-enhancing metabolites of nicotine in brain: a systematic review. Front. Neurosci. 12:1002. doi: 10.3389/fnins.2018.01002
Maki, P. M. (2012). Minireview: effects of different HT formulations on cognition. Endocrinology 153, 3564–3570. doi: 10.1210/en.2012-1175
Malpetti, M., Joie, R., and Rabinovici, G. D. (2022). Tau beats amyloid in predicting brain atrophy in Alzheimer disease: implications for prognosis and clinical trials. J. Nucl. Med. 63, 830–832. doi: 10.2967/jnumed.121.263694
Mani, V., Arfeen, M., Rabbani, S. I., Shariq, A., and Amirthalingam, P. (2022). Levetiracetam ameliorates doxorubicin-induced Chemobrain by enhancing cholinergic transmission and reducing Neuroinflammation using an experimental rat model and molecular docking study. Molecules 27:7364. doi: 10.3390/molecules27217364
Markram, H., Lubke, J., Frotscher, M., and Sakmann, B. (1997). Regulation of synaptic efficacy by coincidence of postsynaptic APs and EPSPs. Science 275, 213–215. doi: 10.1126/science.275.5297.213
Matsuyama, S., and Matsumoto, A. (2003). Epibatidine induces long-term potentiation (LTP) via activation of alpha4beta2 nicotinic acetylcholine receptors (nAChRs) in vivo in the intact mouse dentate gyrus: both alpha7 and alpha4beta2 nAChRs essential to nicotinic LTP. J. Pharmacol. Sci. 93, 180–187. doi: 10.1254/jphs.93.180
Matsuyama, S., Matsumoto, A., Enomoto, T., and Nishizaki, T. (2000). Activation of nicotinic acetylcholine receptors induces long-term potentiation in vivo in the intact mouse dentate gyrus. Eur. J. Neurosci. 12, 3741–3747. doi: 10.1046/j.1460-9568.2000.00259.x
Maurer, S. V., and Williams, C. L. (2017). The cholinergic system modulates memory and hippocampal plasticity via its interactions with non-neuronal cells. Front. Immunol. 8:1489. doi: 10.3389/fimmu.2017.01489
Mayford, M., Siegelbaum, S. A., and Kandel, E. R. (2012). Synapses and memory storage. Cold Spring Harb. Perspect. Biol. 4:a005751. doi: 10.1101/cshperspect.a005751
McGeer, E. G., and McGeer, P. L. (2010). Neuroinflammation in Alzheimer's disease and mild cognitive impairment: a field in its infancy. J. Alzheimers Dis. 19, 355–361. doi: 10.3233/JAD-2010-1219
McGeer, P. L., and McGeer, E. G. (2013). The amyloid cascade-inflammatory hypothesis of Alzheimer disease: implications for therapy. Acta Neuropathol. 126, 479–497. doi: 10.1007/s00401-013-1177-7
McGeer, P. L., McGeer, E. G., and Yasojima, K. (2000). Alzheimer disease and neuroinflammation. J. Neural Transm. Suppl. 59, 53–57. doi: 10.1007/978-3-7091-6781-6_8
McKay, B. E., Persinger, M. A., and Koren, S. A. (2000). Exposure to a theta-burst patterned magnetic field impairs memory acquisition and consolidation for contextual but not discrete conditioned fear in rats. Neurosci. Lett. 292, 99–102. doi: 10.1016/S0304-3940(00)01437-3
McKay, B. E., Placzek, A. N., and Dani, J. A. (2007). Regulation of synaptic transmission and plasticity by neuronal nicotinic acetylcholine receptors. Biochem. Pharmacol. 74, 1120–1133. doi: 10.1016/j.bcp.2007.07.001
Mendoza, C., Barreto, G. E., Iarkov, A., Tarasov, V. V., Aliev, G., and Echeverria, V. (2018a). Cotinine: a therapy for memory extinction in post-traumatic stress disorder. Mol. Neurobiol. 55, 6700–6711. doi: 10.1007/s12035-018-0869-3
Mendoza, C., Perez-Urrutia, N., Alvarez-Ricartes, N., Barreto, G. E., Perez-Ordas, R., Iarkov, A., et al. (2018b). Cotinine plus krill oil decreased depressive behavior, and increased astrocytes survival in the hippocampus of mice subjected to restraint stress. Front. Neurosci. 12:952. doi: 10.3389/fnins.2018.00952
Meshi, D., Drew, M. R., Saxe, M., Ansorge, M. S., David, D., Santarelli, L., et al. (2006). Hippocampal neurogenesis is not required for behavioral effects of environmental enrichment. Nat. Neurosci. 9, 729–731. doi: 10.1038/nn1696
Miry, O., Zhang, X. L., Vose, L. R., Gopaul, K. R., Subah, G., Moncaster, J. A., et al. (2021). Life-long brain compensatory responses to galactic cosmic radiation exposure. Sci. Rep. 11:4292. doi: 10.1038/s41598-021-83447-y
Mitchell, T., and Turton, P. (2011). 'Chemobrain': concentration and memory effects in people receiving chemotherapy – a descriptive phenomenological study. Eur. J. Cancer Care 20, 539–548. doi: 10.1111/j.1365-2354.2011.01244.x
Mohammadi-Farani, A., Farhangian, S., and Shirooie, S. (2022). Sex differences in acetylcholinesterase modulation during spatial and fear memory extinction in the amygdala; an animal study in the single prolonged stress model of PTSD. Res. Pharm. Sci. 17, 686–696. doi: 10.4103/1735-5362.359435
Mohapel, P., Leanza, G., Kokaia, M., and Lindvall, O. (2005). Forebrain acetylcholine regulates adult hippocampal neurogenesis and learning. Neurobiol. Aging 26, 939–946. doi: 10.1016/j.neurobiolaging.2004.07.015
Moran, V. E. (2012). Cotinine: beyond that expected, More than a biomarker of tobacco consumption. Front. Pharmacol. 3:173. doi: 10.3389/fphar.2012.00173
Morioka, N., Hisaoka-Nakashima, K., and Nakata, Y. (2018). “Regulation by nicotinic acetylcholine receptors of microglial glutamate transporters: role of microglia in neuroprotection” in Nicotinic acetylcholine receptor signaling in neuroprotection, Singapore. eds. A. Akaike, S. Shimohama, and Y. Misu, (Singapore: Springer), 73–88.
Morrison, J. H., and Baxter, M. G. (2012). The ageing cortical synapse: hallmarks and implications for cognitive decline. Nat. Rev. Neurosci. 13, 240–250. doi: 10.1038/nrn3200
Morrison, T. A., Hudson, W. H., Chisolm, D. A., Kanno, Y., Shih, H. Y., Ahmed, R., et al. (2022). Evolving views of long noncoding RNAs and Epigenomic control of lymphocyte state and memory. Cold Spring Harb. Perspect. Biol. 14:a037952. doi: 10.1101/cshperspect.a037952
Murman, D. L. (2015). The impact of age on cognition. Semin. Hear. 36, 111–121. doi: 10.1055/s-0035-1555115
Nakazawa, K., McHugh, T. J., Wilson, M. A., and Tonegawa, S. (2004). NMDA receptors, place cells and hippocampal spatial memory. Nat. Rev. Neurosci. 5, 361–372. doi: 10.1038/nrn1385
Narla, S. T., Klejbor, I., Birkaya, B., Lee, Y. W., Morys, J., Stachowiak, E. K., et al. (2013). Activation of developmental nuclear fibroblast growth factor receptor 1 signaling and neurogenesis in adult brain by alpha7 nicotinic receptor agonist. Stem Cells Transl. Med. 2, 776–788. doi: 10.5966/sctm.2012-0103
Narla, S., Klejbor, I., Birkaya, B., Lee, Y. W., Morys, J., Stachowiak, E. K., et al. (2013). alpha7 nicotinic receptor agonist reactivates neurogenesis in adult brain. Biochem. Pharmacol. 86, 1099–1104. doi: 10.1016/j.bcp.2013.07.028
Nebot, A., Domenech, S., Albino-Pires, N., Mugica, F., Benali, A., Porta, X., et al. (2022). LONG-REMI: an AI-based technological application to promote healthy mental longevity grounded in reminiscence therapy. Int. J. Environ. Res. Public Health 19:5997. doi: 10.3390/ijerph19105997
Nees, F. (2015). The nicotinic cholinergic system function in the human brain. Neuropharmacology 96, 289–301. doi: 10.1016/j.neuropharm.2014.10.021
Nicoll, R. A., and Malenka, R. C. (1999). Expression mechanisms underlying NMDA receptor-dependent long-term potentiation. Ann. N. Y. Acad. Sci. 868, 515–525. doi: 10.1111/j.1749-6632.1999.tb11320.x
Nihongaki, Y., Kawano, F., Nakajima, T., and Sato, M. (2015). Photoactivatable CRISPR-Cas9 for optogenetic genome editing. Nat. Biotechnol. 33, 755–760. doi: 10.1038/nbt.3245
Nijdam, M. J., and Vermetten, E. (2018). Moving forward in treatment of posttraumatic stress disorder: innovations to exposure-based therapy. Eur. J. Psychotraumatol. 9:1458568. doi: 10.1080/20008198.2018.1458568
Nyberg, L., Backman, L., Erngrund, K., Olofsson, U., and Nilsson, L. G. (1996a). Age differences in episodic memory, semantic memory, and priming: relationships to demographic, intellectual, and biological factors. J. Gerontol. B Psychol. Sci. Soc. Sci. 51, P234–P240. doi: 10.1093/geronb/51b.4.p234
Nyberg, L., McIntosh, A. R., Cabeza, R., Habib, R., Houle, S., and Tulving, E. (1996b). General and specific brain regions involved in encoding and retrieval of events: what, where, and when. Proc. Natl. Acad. Sci. U. S. A. 93, 11280–11285. doi: 10.1073/pnas.93.20.11280
Okada, K., Hashimoto, K., and Kobayashi, K. (2022). Cholinergic regulation of object recognition memory. Front. Behav. Neurosci. 16:996089. doi: 10.3389/fnbeh.2022.996089
O'Leary, K., Parameswaran, N., McIntosh, J. M., and Quik, M. (2008). Cotinine selectively activates a subpopulation of alpha3/alpha6beta2 nicotinic receptors in monkey striatum. J. Pharmacol. Exp. Ther. 325, 646–654. doi: 10.1124/jpet.108.136838
Oliveros-Matus, P., Perez-Urrutia, N., Alvarez-Ricartes, N., Echeverria, F., Barreto, G. E., Elliott, J., et al. (2020). Cotinine enhances fear extinction and astrocyte survival by mechanisms involving the nicotinic acetylcholine receptors signaling. Front. Pharmacol. 11:303. doi: 10.3389/fphar.2020.00303
Otto, S. L., and Yakel, J. L. (2019). The alpha7 nicotinic acetylcholine receptors regulate hippocampal adult-neurogenesis in a sexually dimorphic fashion. Brain Struct. Funct. 224, 829–846. doi: 10.1007/s00429-018-1799-6
Parent, J. M. (2002). The role of seizure-induced neurogenesis in epileptogenesis and brain repair. Epilepsy Res. 50, 179–189. doi: 10.1016/S0920-1211(02)00078-5
Patel, S., Grizzell, J. A., Holmes, R., Zeitlin, R., Solomon, R., Sutton, T. L., et al. (2014). Cotinine halts the advance of Alzheimer's disease-like pathology and associated depressive-like behavior in Tg6799 mice. Front. Aging Neurosci. 6:162. doi: 10.3389/fnagi.2014.00162
Perez-Urrutia, N., Mendoza, C., Alvarez-Ricartes, N., Oliveros-Matus, P., Echeverria, F., Grizzell, J. A., et al. (2017). Intranasal cotinine improves memory, and reduces depressive-like behavior, and GFAP+ cells loss induced by restraint stress in mice. Exp. Neurol. 295, 211–221. doi: 10.1016/j.expneurol.2017.06.016
Phipps, C. J., Murman, D. L., and Warren, D. E. (2021). Stimulating memory: reviewing interventions using repetitive transcranial magnetic stimulation to enhance or restore memory abilities. Brain Sci. 11:1283. doi: 10.3390/brainsci11101283
Porsteinsson, A. P., Grossberg, G. T., Mintzer, J., and Olin, J. T. (2008). Memantine treatment in patients with mild to moderate Alzheimer's disease already receiving a cholinesterase inhibitor: a randomized, double-blind, placebo-controlled trial. Curr. Alzheimer Res. 5, 83–89. doi: 10.2174/156720508783884576
Poulose, S. M., Miller, M. G., Scott, T., and Shukitt-Hale, B. (2017). Nutritional factors affecting adult neurogenesis and cognitive function. Adv. Nutr. 8, 804–811. doi: 10.3945/an.117.016261
Raghu, S. V., Kudva, A. K., Rao, S., Prasad, K., Mudgal, J., and Baliga, M. S. (2021). Dietary agents in mitigating chemotherapy-related cognitive impairment (chemobrain or chemofog): first review addressing the benefits, gaps, challenges and ways forward. Food Funct. 12, 11132–11153. doi: 10.1039/D1FO02391H
Rajasethupathy, P., Antonov, I., Sheridan, R., Frey, S., Sander, C., Tuschl, T., et al. (2012). A role for neuronal piRNAs in the epigenetic control of memory-related synaptic plasticity. Cells 149, 693–707. doi: 10.1016/j.cell.2012.02.057
Raz, N., Gunning, F. M., Head, D., Dupuis, J. H., McQuain, J., Briggs, S. D., et al. (1997). Selective aging of the human cerebral cortex observed in vivo: differential vulnerability of the prefrontal gray matter. Cereb. Cortex 7, 268–282. doi: 10.1093/cercor/7.3.268
Reis, J., Portugal, A. M., Fernandes, L., Afonso, N., Pereira, M., Sousa, N., et al. (2016). An alpha and theta Intensive and short neurofeedback protocol for healthy aging working-memory training. Front. Aging Neurosci. 8:157. doi: 10.3389/fnagi.2016.00157
Rejc, L., Gomez-Vallejo, V., Joya, A., Arsequell, G., Egimendia, A., Castellnou, P., et al. (2022). Longitudinal evaluation of neuroinflammation and oxidative stress in a mouse model of Alzheimer disease using positron emission tomography. Alzheimers Res. Ther. 14:80. doi: 10.1186/s13195-022-01016-5
Riquelme, P. A., Drapeau, E., and Doetsch, F. (2008). Brain micro-ecologies: neural stem cell niches in the adult mammalian brain. Philos. Trans. R. Soc. Lond. Ser. B Biol. Sci. 363, 123–137. doi: 10.1098/rstb.2006.2016
Rodziewicz-Flis, E. A., Kawa, M., Skrobot, W. R., Flis, D. J., Wilczynska, D., Szaro-Truchan, M., et al. (2022). The positive impact of 12 weeks of dance and balance training on the circulating amyloid precursor protein and serotonin concentration as well as physical and cognitive abilities in elderly women. Exp. Gerontol. 162:111746. doi: 10.1016/j.exger.2022.111746
Rola, R., Raber, J., Rizk, A., Otsuka, S., VandenBerg, S. R., Morhardt, D. R., et al. (2004). Radiation-induced impairment of hippocampal neurogenesis is associated with cognitive deficits in young mice. Exp. Neurol. 188, 316–330. doi: 10.1016/j.expneurol.2004.05.005
Rosa, J., de Carvalho Myskiw, J., Fiorenza, N. G., Furini, C. R. G., Sapiras, G. G., and Izquierdo, I. (2023). Hippocampal cholinergic receptors and the mTOR participation in fear-motivated inhibitory avoidance extinction memory. Behav. Brain Res. 437:114129. doi: 10.1016/j.bbr.2022.114129
Rowan, M. J., Klyubin, I., Wang, Q., Hu, N. W., and Anwyl, R. (2007). Synaptic memory mechanisms: Alzheimer's disease amyloid beta-peptide-induced dysfunction. Biochem. Soc. Trans. 35, 1219–1223. doi: 10.1042/BST0351219
Roy, D. S., Park, Y. G., Kim, M. E., Zhang, Y., Ogawa, S. K., DiNapoli, N., et al. (2022). Brain-wide mapping reveals that engrams for a single memory are distributed across multiple brain regions. Nat. Commun. 13:1799. doi: 10.1038/s41467-022-29384-4
Sabandal, P. R., Saldes, E. B., and Han, K. A. (2022). Acetylcholine deficit causes dysfunctional inhibitory control in an aging-dependent manner. Sci. Rep. 12:20903. doi: 10.1038/s41598-022-25402-z
Sabbagh, J. J., O'Leary, J. C. 3rd, Blair, L. J., Klengel, T., Nordhues, B. A., Fontaine, S. N., et al. (2014). Age-associated epigenetic upregulation of the FKBP5 gene selectively impairs stress resiliency. PLoS One 9:e107241. doi: 10.1371/journal.pone.0107241
Sadigh-Eteghad, S., Majdi, A., Mahmoudi, J., Golzari, S. E. J., and Talebi, M. (2016). Astrocytic and microglial nicotinic acetylcholine receptors: an overlooked issue in Alzheimer's disease. J. Neural Transm. (Vienna) 123, 1359–1367. doi: 10.1007/s00702-016-1580-z
Sadigh-Eteghad, S., Vatandoust, S. M., Mahmoudi, J., Rahigh Aghsan, S., and Majdi, A. (2020). Cotinine ameliorates memory and learning impairment in senescent mice. Brain Res. Bull. 164, 65–74. doi: 10.1016/j.brainresbull.2020.08.010
Sanchez-Sarasua, S., Meseguer-Beltran, M., Garcia-Diaz, C., Beltran-Bretones, M. T., ElMlili, N., and Sanchez-Perez, A. M. (2022). IRS1 expression in hippocampus is age-dependent and is required for mature spine maintenance and neuritogenesis. Mol. Cell. Neurosci. 118:103693. doi: 10.1016/j.mcn.2021.103693
Sawyer, R. P., Demel, S. L., Comeau, M. E., Marion, M., Rosand, J., Langefeld, C. D., et al. (2022). Alzheimer's disease related single nucleotide polymorphisms and correlation with intracerebral hemorrhage incidence. Medicine 101:e30782. doi: 10.1097/MD.0000000000030782
Saxe, M. D., Battaglia, F., Wang, J. W., Malleret, G., David, D. J., Monckton, J. E., et al. (2006). Ablation of hippocampal neurogenesis impairs contextual fear conditioning and synaptic plasticity in the dentate gyrus. Proc. Natl. Acad. Sci. U. S. A. 103, 17501–17506. doi: 10.1073/pnas.0607207103
Schiller, D., Cain, C. K., Curley, N. G., Schwartz, J. S., Stern, S. A., Ledoux, J. E., et al. (2008). Evidence for recovery of fear following immediate extinction in rats and humans. Learn. Mem. 15, 394–402. doi: 10.1101/lm.909208
Schilstrom, B., Fagerquist, M. V., Zhang, X., Hertel, P., Panagis, G., Nomikos, G. G., et al. (2000). Putative role of presynaptic alpha7* nicotinic receptors in nicotine stimulated increases of extracellular levels of glutamate and aspartate in the ventral tegmental area. Synapse 38, 375–383. doi: 10.1002/1098-2396(20001215)38:4<375::AID-SYN2>3.0.CO;2-Y
Schroff, K. C., Lovich, P., Schmitz, O., Aschhoff, S., Richter, E., and Remien, J. (2000). Effects of cotinine at cholinergic nicotinic receptors of the sympathetic superior cervical ganglion of the mouse. Toxicology 144, 99–105. doi: 10.1016/S0300-483X(99)00195-X
Scott, G. A., Terstege, D. J., Roebuck, A. J., Gorzo, K. A., Vu, A. P., Howland, J. G., et al. (2021). Adult neurogenesis mediates forgetting of multiple types of memory in the rat. Mol. Brain 14:97. doi: 10.1186/s13041-021-00808-4
Semenova, S., Contet, C., Roberts, A. J., and Markou, A. (2012). Mice lacking the beta4 subunit of the nicotinic acetylcholine receptor show memory deficits, altered anxiety- and depression-like behavior, and diminished nicotine-induced analgesia. Nicotine Tob. Res. 14, 1346–1355. doi: 10.1093/ntr/nts107
Semon, R. W., and Simon, L. (1921). The mneme. G. Allen & Unwin Ltd.; The Macmillan company., London, New York, NY
Setel, D. D., Beker, M., Terzioglu-Usak, S., and Elibol, B. (2022). Astragalus membranaceus treatment combined with caloric restriction may enhance genesis factors and decrease apoptosis in the hippocampus of rats. Arch. Gerontol. Geriatr. 99:104584. doi: 10.1016/j.archger.2021.104584
Shabani, Z., Ghadiri, T., Karimipour, M., Sadigh-Eteghad, S., Mahmoudi, J., Mehrad, H., et al. (2021). Modulatory properties of extracellular matrix glycosaminoglycans and proteoglycans on neural stem cells behavior: highlights on regenerative potential and bioactivity. Int. J. Biol. Macromol. 171, 366–381. doi: 10.1016/j.ijbiomac.2021.01.006
Shabani, Z., Mahmoudi, J., Farajdokht, F., and Sadigh-Eteghad, S. (2020). An overview of nicotinic cholinergic system signaling in neurogenesis. Arch. Med. Res. 51, 287–296. doi: 10.1016/j.arcmed.2020.03.014
Shao, X., Cui, W., Xie, X., Ma, W., Zhan, Y., and Lin, Y. (2020). Treatment of Alzheimer's disease with framework nucleic acids. Cell Prolif. 53:e12787. doi: 10.1111/cpr.12787
Sharma, T., and Mehan, S. (2021). Possible therapeutic interventions in COVID-19 induced ARDS by cotinine as an ACE-2 promoter and AT-1R blocker. Infect. Disord. Drug Targets 21:e170721189261. doi: 10.2174/1871526520666201218153554
Shen, J., and Barnes, C. A. (1996). Age-related decrease in cholinergic synaptic transmission in three hippocampal subfields. Neurobiol. Aging 17, 439–451. doi: 10.1016/0197-4580(96)00020-6
Shen, J., Yang, L., and Wei, W. (2022). Dl-3-n-butylphthalide prevents chronic restraint stress-induced depression-like behaviors and cognitive impairment via regulating CaMKII/CREB/BDNF signaling pathway in hippocampus. Neuroreport 33, 597–603. doi: 10.1097/WNR.0000000000001819
Shengkai, D., Qianqian, L., and Yazhen, S. (2022). The effects and regulatory mechanism of flavonoids from stems and leaves of Scutellaria baicalensis Georgi in promoting neurogenesis and improving memory impairment mediated by the BDNF-ERK-CREB signaling pathway in rats. CNS Neurol. Disord. Drug Targets 21, 354–366. doi: 10.2174/1871527320666210827112048
Shors, T. J., Miesegaes, G., Beylin, A., Zhao, M., Rydel, T., and Gould, E. (2001). Neurogenesis in the adult is involved in the formation of trace memories. Nature 410, 372–376. doi: 10.1038/35066584
Siu, E. C., and Tyndale, R. F. (2007). Characterization and comparison of nicotine and cotinine metabolism in vitro and in vivo in DBA/2 and C57BL/6 mice. Mol. Pharmacol. 71, 826–834. doi: 10.1124/mol.106.032086
Sjostrom, P. J., Turrigiano, G. G., and Nelson, S. B. (2001). Rate, timing, and cooperativity jointly determine cortical synaptic plasticity. Neuron 32, 1149–1164. doi: 10.1016/S0896-6273(01)00542-6
Smith, R. N., Agharkar, A. S., and Gonzales, E. B. (2014). A review of creatine supplementation in age-related diseases: more than a supplement for athletes. F1000Research 3:222. doi: 10.12688/f1000research.5218.1
Snyder, J. S., Hong, N. S., McDonald, R. J., and Wojtowicz, J. M. (2005). A role for adult neurogenesis in spatial long-term memory. Neuroscience 130, 843–852. doi: 10.1016/j.neuroscience.2004.10.009
Soto-Otero, R., Méndez-Alvarez, E., Hermida-Ameijeiras, A., López-Real, A. M., and Labandeira-García, J. L. (2002). Effects of (−)-nicotine and (−)-cotinine on 6-hydroxydopamine-induced oxidative stress and neurotoxicity: relevance for Parkinson's disease. Biochem. Pharmacol. 64, 125–135. doi: 10.1016/S0006-2952(02)01070-5
Spalding, K. L., Bergmann, O., Alkass, K., Bernard, S., Salehpour, M., Huttner, H. B., et al. (2013). Dynamics of hippocampal neurogenesis in adult humans. Cells 153, 1219–1227. doi: 10.1016/j.cell.2013.05.002
Steward, K. A., Silva, M. A., Maduri, P., Tang, X., Wittine, L., Dams-O'Connor, K., et al. (2022). Obstructive sleep apnea is associated with worse cognitive outcomes in acute moderate-to-severe traumatic brain injury: a TBI model systems study. Sleep Med. 100, 454–461. doi: 10.1016/j.sleep.2022.09.012
Stratmann, G., Sall, J. W., Bell, J. S., Alvi, R. S., May, L., Ku, B., et al. (2010). Isoflurane does not affect brain cell death, hippocampal neurogenesis, or long-term neurocognitive outcome in aged rats. Anesthesiology 112, 305–315. doi: 10.1097/ALN.0b013e3181ca33a1
Su, Y., Yang, X., Yang, L., Liu, X., She, Z., Zhang, Y., et al. (2022). Thyroid hormones regulate reelin expression in neuropsychiatric disorders. Can. J. Physiol. Pharmacol. 100. doi: 10.1139/cjpp-2022-0270
Sun, D. (2014). The potential of endogenous neurogenesis for brain repair and regeneration following traumatic brain injury. Neural Regen. Res. 9, 688–692. doi: 10.4103/1673-5374.131567
Szczurowska, E., Szanti-Pinter, E., Chetverikov, N., Randakova, A., Kudova, E., and Jakubik, J. (2022). Modulation of muscarinic Signalling in the central nervous system by steroid hormones and Neurosteroids. Int. J. Mol. Sci. 24:507. doi: 10.3390/ijms24010507
Tan, X., Vrana, K., and Ding, Z. M. (2021). Cotinine: pharmacologically active metabolite of nicotine and neural mechanisms for its actions. Front. Behav. Neurosci. 15:758252. doi: 10.3389/fnbeh.2021.758252
Tao, W., Ruan, J., Wu, R., Zhao, M., Zhao, T., Qi, M., et al. (2023). A natural carotenoid crocin exerts antidepressant action by promoting adult hippocampal neurogenesis through Wnt/beta-catenin signaling. J. Adv. Res. 43, 219–231. doi: 10.1016/j.jare.2022.02.015
Tartt, A. N., Fulmore, C. A., Liu, Y., Rosoklija, G. B., Dwork, A. J., Arango, V., et al. (2018). Considerations for assessing the extent of hippocampal neurogenesis in the adult and aging human brain. Cell Stem Cell 23, 782–783. doi: 10.1016/j.stem.2018.10.025
Tayeb, H. O., Yang, H. D., Price, B. H., and Tarazi, F. I. (2012). Pharmacotherapies for Alzheimer's disease: beyond cholinesterase inhibitors. Pharmacol. Ther. 134, 8–25. doi: 10.1016/j.pharmthera.2011.12.002
Tazerart, S., Mitchell, D. E., Miranda-Rottmann, S., and Araya, R. (2020). A spike-timing-dependent plasticity rule for dendritic spines. Nat. Commun. 11:4276. doi: 10.1038/s41467-020-17861-7
Tchekalarova, J., and Tzoneva, R. (2023). Oxidative stress and aging as risk factors for Alzheimer's disease and Parkinson's disease: the Role of the antioxidant melatonin. Int. J. Mol. Sci. 24:3022. doi: 10.3390/ijms24033022
Teoh, N. S. N., Gyanwali, B., Lai, M. K. P., Chai, Y. L., Chong, J. R., Chong, E. J. Y., et al. (2023). Association of Interleukin-6 and Interleukin-8 with cognitive decline in an Asian memory clinic population. J. Alzheimers Dis. 92, 445–455. doi: 10.3233/JAD-220971
Terry, A. V. Jr., Hernandez, C. M., Hohnadel, E. J., Bouchard, K. P., and Buccafusco, J. J. (2005). Cotinine, a neuroactive metabolite of nicotine: potential for treating disorders of impaired cognition. CNS Drug Rev. 11, 229–252. doi: 10.1111/j.1527-3458.2005.tb00045.x
Thancharoen, O., Limwattananon, C., Waleekhachonloet, O., Rattanachotphanit, T., Limwattananon, P., and Limpawattana, P. (2019). Ginkgo biloba extract (EGb761), cholinesterase inhibitors, and Memantine for the treatment of mild-to-moderate Alzheimer's disease: a network meta-analysis. Drugs Aging 36, 435–452. doi: 10.1007/s40266-019-00648-x
Thompson, R. F. (1976). The search for the engram. Am. Psychol. 31, 209–227. doi: 10.1037/0003-066X.31.3.209
Thompson, R. F. (2005). In search of memory traces. Annu. Rev. Psychol. 56, 1–23. doi: 10.1146/annurev.psych.56.091103.070239
Thompson, R. F. (2013). An essential memory trace found. Behav. Neurosci. 127, 669–675. doi: 10.1037/a0033978
Thumuluri, D., Lyday, R., Babcock, P., Ip, E. H., Kraft, R. A., Laurienti, P. J., et al. (2021). Improvisational movement to improve quality of life in older adults with early-stage dementia: a pilot study. Front. Sports Act. Living 3:796101. doi: 10.3389/fspor.2021.796101
Tiwari, S., Atluri, V., Kaushik, A., Yndart, A., and Nair, M. (2019). Alzheimer's disease: pathogenesis, diagnostics, and therapeutics. Int. J. Nanomedicine 14, 5541–5554. doi: 10.2147/IJN.S200490
Tonegawa, S., Morrissey, M. D., and Kitamura, T. (2018). The role of engram cells in the systems consolidation of memory. Nat. Rev. Neurosci. 19, 485–498. doi: 10.1038/s41583-018-0031-2
Tonegawa, S., Pignatelli, M., Roy, D. S., and Ryan, T. J. (2015). Memory engram storage and retrieval. Curr. Opin. Neurobiol. 35, 101–109. doi: 10.1016/j.conb.2015.07.009
Toyohara, J., and Hashimoto, K. (2010). alpha7 nicotinic receptor agonists: potential therapeutic drugs for treatment of cognitive impairments in schizophrenia and Alzheimer's disease. Open Med. Chem. J. 4, 37–56. doi: 10.2174/1874104501004010037
Tryon, S. C., Bratsch-Prince, J. X., Warren, J. W., Jones, G. C., McDonald, A. J., and Mott, D. D. (2023). Differential regulation of Prelimbic and thalamic transmission to the basolateral amygdala by acetylcholine receptors. J. Neurosci. 43, 722–735. doi: 10.1523/JNEUROSCI.2545-21.2022
Valles, A. S., Borroni, M. V., and Barrantes, F. J. (2014). Targeting brain alpha7 nicotinic acetylcholine receptors in Alzheimer's disease: rationale and current status. CNS Drugs 28, 975–987. doi: 10.1007/s40263-014-0201-3
van Praag, H., Christie, B. R., Sejnowski, T. J., and Gage, F. H. (1999). Running enhances neurogenesis, learning, and long-term potentiation in mice. Proc. Natl. Acad. Sci. U. S. A. 96, 13427–13431. doi: 10.1073/pnas.96.23.13427
Vargova, L., and Sykova, E. (2014). Astrocytes and extracellular matrix in extrasynaptic volume transmission. Philos. Trans. R. Soc. Lond. Ser. B Biol. Sci. 369:20130608. doi: 10.1098/rstb.2013.0608
Vega-Avila, G. C., Afanador-Restrepo, D. F., Rivas-Campo, Y., Garcia-Garro, P. A., Hita-Contreras, F., Carcelen-Fraile, M. D. C., et al. (2022). Rhythmic physical activity and global cognition in older adults with and without mild cognitive impairment: a systematic review. Int. J. Environ. Res. Public Health 19:12230. doi: 10.3390/ijerph191912230
Vizi, E. S., Fekete, A., Karoly, R., and Mike, A. (2010). Non-synaptic receptors and transporters involved in brain functions and targets of drug treatment. Br. J. Pharmacol. 160, 785–809. doi: 10.1111/j.1476-5381.2009.00624.x
Wallace, T. L., and Bertrand, D. (2013). Importance of the nicotinic acetylcholine receptor system in the prefrontal cortex. Biochem. Pharmacol. 85, 1713–1720. doi: 10.1016/j.bcp.2013.04.001
Wan, L., Lu, J., Huang, J., Huo, Y., Jiang, S., and Guo, C. (2020). Association between peripheral adiponectin and lipids levels and the therapeutic response to donepezil treatment in Han Chinese patients with Alzheimer's disease. Front. Aging Neurosci. 12:532386. doi: 10.3389/fnagi.2020.532386
Wang, L., Almeida, L. E., Spornick, N. A., Kenyon, N., Kamimura, S., Khaibullina, A., et al. (2015). Modulation of social deficits and repetitive behaviors in a mouse model of autism: the role of the nicotinic cholinergic system. Psychopharmacology 232, 4303–4316. doi: 10.1007/s00213-015-4058-z
Wang, L. L., Sun, C. J., Wang, Y., Zhan, T. T., Yuan, J., Niu, C. Y., et al. (2022). Effects of dance therapy on non-motor symptoms in patients with Parkinson's disease: a systematic review and meta-analysis. Aging Clin. Exp. Res. 34, 1201–1208. doi: 10.1007/s40520-021-02030-7
Wang, M., Tang, G., Zhou, C., Guo, H., Hu, Z., Hu, Q., et al. (2023). Revisiting the intersection of microglial activation and neuroinflammation in Alzheimer's disease from the perspective of ferroptosis. Chem. Biol. Interact. 375, 110387. doi: 10.1016/j.cbi.2023.110387
Webster, M. K., Barnett, B. J., Stanchfield, M. L., Paris, J. R., Webster, S. E., Cooley-Themm, C. A., et al. (2019). Stimulation of retinal pigment epithelium with an alpha7 nAChR agonist leads to Muller glia dependent neurogenesis in the adult mammalian retina. Invest. Ophthalmol. Vis. Sci. 60, 570–579. doi: 10.1167/iovs.18-25722
Wehner, J. M., Keller, J. J., Keller, A. B., Picciotto, M. R., Paylor, R., Booker, T. K., et al. (2004). Role of neuronal nicotinic receptors in the effects of nicotine and ethanol on contextual fear conditioning. Neuroscience 129, 11–24. doi: 10.1016/j.neuroscience.2004.07.016
Wehner, J. M., and Radcliffe, R. A. (2004). Cued and contextual fear conditioning in mice. Curr. Protoc. Neurosci. Chapter 8:Unit 8.5C. doi: 10.1002/0471142301.ns0805cs27
White, W. B., Marfatia, R., Schmidt, J., Wakefield, D. B., Kaplan, R. F., Bohannon, R. W., et al. (2013). INtensive versus standard ambulatory blood pressure lowering to prevent functional DeclINe in the ElderlY (INFINITY). Am. Heart J. 165, 258–265 e1. doi: 10.1016/j.ahj.2012.11.008
Wideman, C. E., Minard, E. P., Zakaria, J. M., Capistrano, J. D. R., Scott, G. A., and Winters, B. D. (2022). Dissociating the involvement of muscarinic and nicotinic cholinergic receptors in object memory destabilization and reconsolidation. Neurobiol. Learn. Mem. 195:107686. doi: 10.1016/j.nlm.2022.107686
Wildeboer-Andrud, K. M., Zheng, L., Choo, K. S., and Stevens, K. E. (2014). Cotinine impacts sensory processing in DBA/2 mice through changes in the conditioning amplitude. Pharmacol. Biochem. Behav. 117, 144–150. doi: 10.1016/j.pbb.2013.12.005
Wilker, S., Vukojevic, V., Schneider, A., Pfeiffer, A., Inerle, S., Pauly, M., et al. (2023). Epigenetics of traumatic stress: the association of NR3C1 methylation and posttraumatic stress disorder symptom changes in response to narrative exposure therapy. Transl. Psychiatry 13:14. doi: 10.1038/s41398-023-02316-6
Williams, H. C., Carlson, S. W., and Saatman, K. E. (2022). A role for insulin-like growth factor-1 in hippocampal plasticity following traumatic brain injury. Vitam. Horm. 118, 423–455. doi: 10.1016/bs.vh.2021.11.009
Winczyk, K., Kostka, T., Pisarek, H., Pisarek, H., Guligowska, A., Guligowska, A., et al. (2022). Hormones and depression in the advanced age. Endokrynol. Pol. 73, 917–921. doi: 10.5603/EP.a2022.0094
Wing, E. A., D'Angelo, M. C., Gilboa, A., and Ryan, J. D. (2021). The Role of the ventromedial prefrontal cortex and basal forebrain in relational memory and inference. J. Cogn. Neurosci. 33, 1976–1989. doi: 10.1162/jocn_a_01722
Woitke, F., Blank, A., Fleischer, A. L., Zhang, S., Lehmann, G. M., Broesske, J., et al. (2023). Post-stroke environmental enrichment improves neurogenesis and cognitive function and reduces the generation of aberrant neurons in the mouse hippocampus. Cells 12:652. doi: 10.3390/cells12040652
Wolf, E. J., Maniates, H., Nugent, N., Maihofer, A. X., Armstrong, D., Ratanatharathorn, A., et al. (2018). Traumatic stress and accelerated DNA methylation age: a meta-analysis. Psychoneuroendocrinology 92, 123–134. doi: 10.1016/j.psyneuen.2017.12.007
Wu, Y., Hang, Z., Lei, T., and Du, H. (2022). Intestinal Flora affect Alzheimer's disease by regulating endogenous hormones. Neurochem. Res. 47, 3565–3582. doi: 10.1007/s11064-022-03784-w
Xu, Y., Liu, J., Tian, Y., Wang, Z., Song, Z., Li, K., et al. (2022). Wnt/beta-catenin signaling pathway is strongly implicated in cadmium-induced developmental neurotoxicity and Neuroinflammation: clues from zebrafish Neurobehavior and in vivo neuroimaging. Int. J. Mol. Sci. 23:11434. doi: 10.3390/ijms232415508
Yakel, J. L. (2013). Cholinergic receptors: functional role of nicotinic ACh receptors in brain circuits and disease. Pflugers Arch. 465, 441–450. doi: 10.1007/s00424-012-1200-1
Yanai, S., Tago, T., Toyohara, J., Arasaki, T., and Endo, S. (2022). Reversal of spatial memory impairment by phosphodiesterase 3 inhibitor cilostazol is associated with reduced neuroinflammation and increased cerebral glucose uptake in aged male mice. Front. Pharmacol. 13:1031637. doi: 10.3389/fphar.2022.1031637
Yang, J. L., Fan, H., Fu, F. F., Guo, B. L., Huang, Y., Sun, L., et al. (2023). Transient neurogenesis in ischemic cortex from Sox2(+) astrocytes. Neural Regen. Res. 18, 1521–1526. doi: 10.4103/1673-5374.357910
Yang, C. H., Shi, H. S., Zhu, W. L., Wu, P., Sun, L. L., Si, J. J., et al. (2012). Venlafaxine facilitates between-session extinction and prevents reinstatement of auditory-cue conditioned fear. Behav. Brain Res. 230, 268–273. doi: 10.1016/j.bbr.2012.02.023
Yang, C., Yang, Q., Xiang, Y., Zeng, X. R., Xiao, J., and Le, W. D. (2023). The neuroprotective effects of oxygen therapy in Alzheimer's disease: a narrative review. Neural Regen. Res. 18, 57–63. doi: 10.4103/1673-5374.343897
Yasar, S., Zhou, J., Varadhan, R., and Carlson, M. C. (2008). The use of angiotensin-converting enzyme inhibitors and diuretics is associated with a reduced incidence of impairment on cognition in elderly women. Clin. Pharmacol. Ther. 84, 119–126. doi: 10.1038/sj.clpt.6100483
Yousuf, H., Girardi, E. M., Crouse, R. B., and Picciotto, M. R. (2023). Muscarinic antagonists impair multiple aspects of operant discrimination learning and performance. Neurosci. Lett. 794:137025. doi: 10.1016/j.neulet.2022.137025
Zeitlin, R., Patel, S., Solomon, R., Tran, J., Weeber, E. J., and Echeverria, V. (2012). Cotinine enhances the extinction of contextual fear memory and reduces anxiety after fear conditioning. Behav. Brain Res. 228, 284–293. doi: 10.1016/j.bbr.2011.11.023
Zhang, Y., Huang, B., Yang, W., Zhong, S., Lai, S., Zhao, H., et al. (2022). Correlations between endocrine hormones and cognitive function in patients with obesity: a cross-sectional study. Obes. Surg. 32, 2299–2308. doi: 10.1007/s11695-022-06076-y
Zheng, T., Bielinski, D. F., Fisher, D. R., Zhang, J., and Shukitt-Hale, B. (2022). Protective effects of a polyphenol-rich blueberry extract on adult human neural progenitor cells. Molecules 27:6152. doi: 10.3390/molecules27196152
Zhou, J., Zhang, C., Fang, X., Zhang, N., Zhang, X., and Zhu, Z. (2023). Activation of autophagy inhibits the activation of NLRP3 inflammasome and alleviates sevoflurane-induced cognitive dysfunction in elderly rats. BMC Neurosci. 24:9. doi: 10.1186/s12868-023-00777-5
Zhou, X., Zhuo, X., Xie, F., Kluetzman, K., Shu, Y. Z., Humphreys, W. G., et al. (2010). Role of CYP2A5 in the clearance of nicotine and cotinine: insights from studies on a Cyp2a5-null mouse model. J. Pharmacol. Exp. Ther. 332, 578–587. doi: 10.1124/jpet.109.162610
Zhu, Y., Gao, Y., Guo, C., Qi, M., Xiao, M., Wu, H., et al. (2022). Effect of 3-month aerobic dance on hippocampal volume and cognition in elderly people with amnestic mild cognitive impairment: a randomized controlled trial. Front. Aging Neurosci. 14:771413. doi: 10.3389/fnagi.2022.771413
Zhu, D., Zhang, M., He, B., Wan, Y., Wang, L., and Gao, F. (2022). The role of sex and ovarian hormones in hippocampal damage and cognitive deficits induced by chronic exposure to hypobaric hypoxia. Front. Neurosci. 16:953417. doi: 10.3389/fnins.2022.953417
Zocher, S., and Toda, T. (2023). Epigenetic aging in adult neurogenesis. Hippocampus 33, 347–359. doi: 10.1002/hipo.23494
Keywords: nicotinic acetylcholine receptors, learning and memory, aging, cotinine, neurogenesis, posttraumatic stress disorder, traumatic brain injury
Citation: Echeverria V, Mendoza C and Iarkov A (2023) Nicotinic acetylcholine receptors and learning and memory deficits in Neuroinflammatory diseases. Front. Neurosci. 17:1179611. doi: 10.3389/fnins.2023.1179611
Edited by:
Jae Kyu Ryu, Gladstone Institutes, United StatesReviewed by:
Gerard Marx, MX Biotech Ltd., IsraelRaviye Ozen Koca, Necmettin Erbakan University, Türkiye
Copyright © 2023 Echeverria, Mendoza and Iarkov. This is an open-access article distributed under the terms of the Creative Commons Attribution License (CC BY). The use, distribution or reproduction in other forums is permitted, provided the original author(s) and the copyright owner(s) are credited and that the original publication in this journal is cited, in accordance with accepted academic practice. No use, distribution or reproduction is permitted which does not comply with these terms.
*Correspondence: Valentina Echeverria, ZWNoZXZlcnJpYS52YWxlbnRpbmFAZ21haWwuY29t