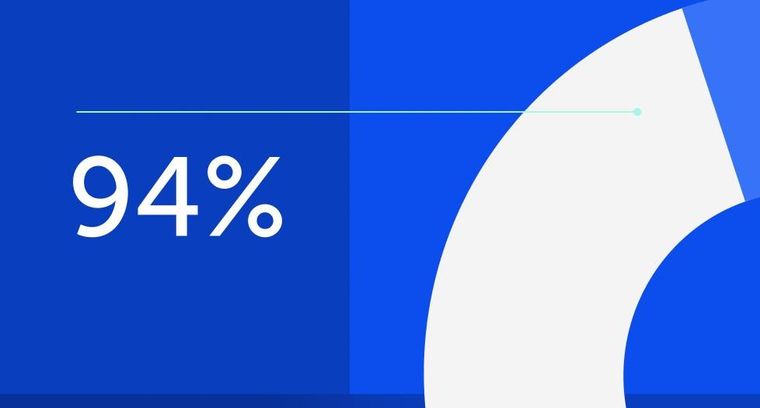
94% of researchers rate our articles as excellent or good
Learn more about the work of our research integrity team to safeguard the quality of each article we publish.
Find out more
ORIGINAL RESEARCH article
Front. Neurosci., 07 July 2023
Sec. Neurodevelopment
Volume 17 - 2023 | https://doi.org/10.3389/fnins.2023.1170090
This article is part of the Research TopicApplications of Transcranial Electrical Stimulation Across the Lifespan: Advances, Challenges, and OpportunitiesView all articles
Introduction: Attention-deficit/hyperactivity disorder (ADHD) is characterized by an inappropriate, pervasive and persistent pattern of inattention, hyperactivity, and/or impulsivity and associated with substantial functional impairment. Despite considerable advances in the understanding and management of ADHD, some patients do not respond well to methylphenidate (MPH), the first-choice pharmacological treatment. Over the past decades, among non-invasive brain stimulation techniques, transcranial direct current stimulation (tDCS) has proven to be an effective and safe technique to improve behavior and cognition in children with neurodevelopmental disorders, including ADHD, by modifying cortical excitability. However, the effect of tDCS has never been directly compared with that of the MPH. The present randomized sham-controlled trial evaluated the effect of a single session of anodal tDCS compared with the administration of a single dose of MPH in children and adolescents with ADHD.
Methods: After completing baseline assessment (T0), 26 children and adolescents with ADHD were exposed to 3 conditions with a 24-h interval-sessions: (A) a single session of anodal tDCS over the left dorsolateral prefrontal cortex (DLPFC); (B) a single session of sham tDCS over the left DLPFC; (C) a single dose of MPH.
Results: Our results showed that after administering a single dose of MPH, children and adolescents with ADHD improved inhibitory control and visual–spatial WM compared with baseline, anodal, and sham tDCS. However, a single session of active tDCS over the left DLPFC was not effective compared with either baseline or sham tDCS.
Discussion: In conclusion, our protocol in ADHD involving a single tDCS session did not demonstrate consistent improvements in neurocognitive features compared with baseline, sham tDCS, or single MPH administration. Different protocols need to be developed to further test the effectiveness of tDCS in improving ADHD symptoms.
Attention-deficit/hyperactivity disorder (ADHD) is characterized by an inappropriate, pervasive and persistent pattern of severe inattention, hyperactivity, and/or impulsivity (American Psychiatric Association, 2013) with a worldwide prevalence of ~6–16% in children and adolescents (Danielson et al., 2022). ADHD develops during childhood, and persists into adulthood in most cases where it is associated with psychiatric comorbidities and poor quality of academic, social and professional life (Thomas et al., 2015).
Most people with ADHD have impairments in high-level cognitive functions necessary for goal-directed behaviors, such as inhibitory control, working memory (WM), and cognitive flexibility (Nigg and Casey, 2005; Sonuga-Barke et al., 2010; Willcutt, 2012). Neuroimaging studies have documented several abnormalities in the dorsolateral prefrontal cortex (DLPFC), orbital frontal cortex (OFC), anterior cingulate cortex, and basal ganglia (Cortese et al., 2012; McCarthy et al., 2014; Faraone et al., 2015; Norman et al., 2016; Lukito et al., 2020), probably due to dysfunction of dopaminergic and noradrenergic neurotransmission in the fronto-striatal pathway (Castellanos, 2002).
Methylphenidate (MPH) is the most commonly used drug for ADHD (Cortese et al., 2018). Although the specific mechanisms of action are not fully defined, MPH is thought to inhibit the protein responsible for dopamine reuptake into the synaptic space, DAT-1, thereby modulating the transmission of catecholaminergic neurotransmitters in fronto-striatal and fronto-parietal pathways (Arnsten, 2006; Schiffer et al., 2006; Volkow et al., 2007). Neuroimaging studies in both healthy individuals and patients with ADHD indicate that acute doses of MPH up-regulate and normalize brain regions known to be under-functioning in ADHD (Rubia et al., 2009).
More than 100 randomized placebo-controlled clinical trials ascertain that MPH is one of the most successful interventions for ADHD, reducing symptoms of inattention, hyperactivity, and impulsivity (Stein et al., 2003) as well as improving executive functions (Coghill et al., 2014; Guven et al., 2018). Nevertheless, about 30 percent of individuals do not respond well to MPH, have no long-term benefits, experience side effects (Faraone et al., 2015; Cortese et al., 2018) and, especially in adolescence, adhere poorly to treatment (Taylor et al., 2004). Moreover, non-pharmacological interventions such as cognitive-behavioral psychotherapy (Knouse et al., 2017), cognitive training (Scionti et al., 2020), parent training for preschool children (Rimestad et al., 2019) or dietary interventions (Li et al., 2020) exhibit small to moderate clinical effectiveness (Faraone et al., 2021). In this context, the development of safe brain-centered drug-free treatments for children and adolescents with ADHD merits undoubtedly further investigation.
For example, transcranial direct current stimulation (tDCS) directly and non-invasively modulates cortical excitability and, consequently, associated behavior and cognitive functions via a weak electrical current (generally between 0.5 and 2 mA) delivered through one or more electrodes placed on the scalp (Jamil et al., 2017). Specifically, the current modulates spontaneous discharge rates and generates subthreshold polarity-dependent shifts in resting membrane potentials, increasing (anode electrode) or decreasing (cathode electrode) the excitability of underlying neurons and leading to respective increases or decreases in cortical function and synaptic strength (Jamil et al., 2017). In this way, tDCS significantly enhances neuroplasticity since its neurophysiological effects last for over an hour (Nitsche and Paulus, 2000; Kuo et al., 2016), especially when combined with a training or cognitive task (Fritsch et al., 2010).
The application of tDCS in pediatric population is an emerging area of intensive research, supported by promising results in a wide range of neurodevelopmental disorders (Costanzo et al., 2016; Finisguerra et al., 2019; Lazzaro et al., 2021; Battisti et al., 2022). Over the past decades, studies exploring the effects of tDCS in children and adolescents with ADHD have increased significantly. Most studies have examined the effects of tDCS on ADHD-related executive function deficits (inhibitory control, WM, attention, cognitive flexibility) and reward processing (Salehinejad et al., 2022). However, results are still sparse and mixed. Some studies documented null effects of tDCS (Westwood et al., 2021b; Salehinejad et al., 2022), whereas other studies reported an improvement of inhibitory control and WM after anodal tDCS over the left DLPFC (Munz et al., 2015; Soltaninejad et al., 2019; Nejati et al., 2020; Berger et al., 2021; Klomjai and Aneksan, 2022). In line with this evidence, studies applying tDCS in adults without ADHD promote left DLPFC as one of the key target area for modulating executive functions, especially inhibitory control (Friehs et al., 2021) and WM (Brunoni et al., 2014; Hill et al., 2016; Mancuso et al., 2016).
Further studies are still needed to understand the actual potential of this technique in the treatment of ADHD. In addition, direct comparison between tDCS and MPH could provide interesting insights into the magnitude of the effect on cognitive function of brain stimulation compared with MPH treatment.
The aim of the present study is to test whether tDCS can induce improvement in inhibitory control and WM of children and adolescents with ADHD and to explore whether the effect of a single session of tDCS can be comparable to that obtained after a single dose of MPH.
All participants received a comprehensive neuropsychiatric assessment at the Child and Adolescent Neuropsychiatry Unit of the Bambino Gesù Children’s Hospital (Rome). Neuropsychiatrists and development psychologists conducted clinical eligibility screenings, evaluating cognitive level, ADHD symptoms and the occurrence of comorbid psychiatric disorders.
Cognitive level was assessed using the Wechsler Intelligence Scale for Children Fourth Edition (perceptual reasoning index) (Orsini et al., 2012) or the Raven Progressive Matrices (Raven and Court, 1998).
The diagnosis of ADHD and psychiatric comorbidities was based on the developmental history, a thorough clinical examination, the semi-structured interview Kiddie Schedule for Affective Disorders and Schizophrenia Present and Lifetime Version for DSM-5 (K-SADS-PL DSM-5) (Kaufman, 2019) and parent-report questionnaires (CBCL, Child Behavior Checklist; CPRS, Conners’ Parent Rating Scales; SNAP-IV; C-GAS, Children Global Assessment Scale) (Swanson et al., 1981; Shaffer, 1983; Achenbach, 1991; Conners et al., 1998).
Children and adolescents with the following characteristics were considered for the inclusion in the study:
1) diagnosis of ADHD (combined presentation) according to the DSM-5 criteria (1);
2) Intelligence Quotient (IQ): higher than or equal to 85 (IQ ≥ 85);
3) age between 8 years to 13 years and 11 months;
4) normal or corrected-to-normal vision;
5) being drug naïve and needing drug treatment for ADHD symptoms.
Patients were excluded if they presented:
6) autism spectrum disorders or specific psychiatric disorders (e.g., bipolar disorders, schizophrenia spectrum disorders, or adjustment disorder) as comorbid conditions;
7) a history of neurological (e.g., seizures, migraines, injury resulting in a loss of consciousness) or medical (e.g., scalp or skin condition such as psoriasis or eczema, the presence of any metallic implants including intracranial electrodes, surgical clips, shrapnel or a pacemaker) or genetic conditions;
8) a pre-existing medical condition (e.g., heart, kidney, or liver diseases) contraindicated for the administration of MPH.
After clinical eligibility screening, 26 children and adolescents (24 males and 2 females, age: 10.63 ± 1.41 years, IQ: 106.42 ± 10.85) with combined presentation of ADHD were included in the study. All participants and their parents were fully instructed about the procedures and the purpose of the study, and both parents and adolescents aged 12 years and older provided written consent before entering the study.
Ethical approval for the study was granted by the local research ethics committee (process number 2185_OPBG_2020) and was registered at ClinicalTrials.gov (ID: NCT04964427) on the 13th of July 2021. The rationale and design of this trial was discussed in a published Study Protocol (D’Aiello et al., 2022a). The study was performed following the Declaration of Helsinki.
A randomized, single-blind, within-subjects, and sham-controlled design was conducted.
After clinical eligibility screening at baseline (Day 0), participants were exposed to three conditions with a 24-h interval-sessions (Day 1, Day 2, Day 3, see Figure 1A) a single session of active anodal tDCS; Figure 1B a single session of sham tDCS; and Figure 1C a single dose of MPH (Ritalin®) administered according to the National Institute for Clinical Excellence (NICE) guidelines for ADHD (Atkinson and Hollis, 2010). After recruitment, participants were assigned to one of six possible sequences: ABC (4 participants), ACB (4 participants), BAC (5 participants), BCA (4 participants), CBA (5 participants), or CAB (5 participants). Assignment to one of six possible combinations was according to a computer-generated randomization order. Randomization information was stored by an independent researcher until the data collection was completed.
Figure 1. Overview of the study design. DAY 0, Baseline; DAY 1, DAY 2, DAY 3, Day of conditions administration; (A) single session of anodal tDCS; (B) single session of sham tDCS; (C) single dose of MPH (Ritalin®); CBCL, Child Behavior Checklist; CPRS, Conners’ Parent Rating Scales; SNAP-IV; K-SADS-PL DSM-5, Kiddie Schedule for Affective Disorders and Schizophrenia Present and Lifetime Version for DSM-5; C-GAS, Children Global Assessment Scale; ABAS-II, Adaptive Behavior Assessment System; N-Back; SST, Stop Signal Task; Safety and Tolerability Questionnaire.
The participants and their families were blinded to the type of tDCS sessions (sham/active). Outcome measures were recorded at Day 0, Day 1, Day 2, and Day 3.
Direct current was delivered by a battery-operated direct current stimulator (Brain-Stim stimulation by E.M.S. S.R.L-Bologna, Italy) through a pair of identical square (25 cm2) saline sponge electrodes held in place by elastic bands. The anodal electrode was placed over the left DLPFC, in accordance with the International System 10–20, on the sites corresponding to F3, while the cathodal electrode was located over the contralateral supraorbital area (OFC), corresponding to Fp2.
During the anodal tDCS condition, the current slowly increased during the first 30 s to 1 mA (ramp-up) and, at the end of stimulation, the current slowly decreased to 0 mA during the last 30 s (ramp-down). Based on previous studies conducted on children and adolescents with neurodevelopmental disorders (Minhas et al., 2012; Kessler et al., 2013; Costanzo et al., 2016; Lazzaro et al., 2021), a current intensity of 1 mA was applied, reduced from what is generally administered to adults, to take into account the characteristics of the pediatric population, such as smaller head size, thinner scalp, and less cerebrospinal fluid than adults, which would influence current distribution and density at the site of stimulation (Minhas et al., 2012; Kessler et al., 2013; Opitz et al., 2015).
Between ramp-up and ramp-down, constant current was delivered for 20 min, with a density of 0.04 mA/cm2.
During the sham tDCS condition, stimulation was delivered the same positioning as active tDCS, respectively left anodal on DLPFC and right electrode reference on OFC. The stimulation intensity was set to 1 mA, but the current was applied for 30 s and was reduced without participant awareness. This placebo condition induces sensations (e.g., tingling) associated with tDCS and, is therefore indistinguishable by participants from the active condition (Gandiga et al., 2006).
tDCS set-up was based on previous studies in children and adolescents with ADHD (Salehinejad et al., 2020b) demonstrating that anodal but not cathodal DLPFC significantly improves inhibitory control and WM in participants with ADHD (Salehinejad et al., 2019). Furthermore, the left anodal DLPFC/right cathodal OFC (reference electrode) montage was proved to be the most effective electrode placement (Salehinejad et al., 2019).
Depending on the age and weight of the child, a single dose of 5–10 mg of immediate-release MPH (Ritalin®) was administered by a neuropsychiatrist in accordance with NICE and AIFA (Agenzia Italiana del Farmaco) guidelines for the treatment of ADHD. Neuropsychiatric preventive and naturalistic assessment (anamnestic history, the mental state examination, and the neurological examination) according to the AIFA guidelines for ADHD was conducted by a neuropsychiatrist before recruitment. At that time, cardiovascular risk factors associated with MPH assumption (e.g., Brugada syndrome) were excluded by clinicians. After this first evaluation, the participants underwent medical examinations. Specifically, the electrocardiogram and the correction of the QT segment were preventively evaluated by a cardiologist. Moreover, blood exams were carried out to exclude any other medical condition associated with ADHD and that may mime this disorder’s symptoms (e.g., thyroiditis).
The primary outcome measure was response inhibition (Stop Signal Reaction Time—SSRT, see Figure 2) measured with the Stop Signal Task – SST (Logan and Cowan, 1984). The SST is a go no-go task that assesses the ability to suppress a dominant response. The SST was performed with PsychoPy® software (Open Science Tools Ltd., Nottingham, United Kingdom) (Peirce et al., 2019), and was designed in accordance with the SST consensus guide (Verbruggen et al., 2019). It consisted of randomly intermixed go and stop trials (75 and 25%, respectively). All trials began with the presentation of a cross in the center of the computer monitor. After 1,000 ms, a stimulus target (go signal) replaced the cross. In the go trials, children were instructed to press the space bar as fast as possible after the go signal appeared. The go signal persisted on the screen up to a maximum of 1,500 ms. In the stop trials, after a variable delay, a stop stimulus target appeared following the go signal (Stop-Signal Delay, SSD). Children were required to abstain from responding. The duration of SSD was monitored with a simple staircase procedure (50 ms step) to maintain the probability of inhibition around 50% of the trials. SSD was increased or decreased by a single step after a successful or failed arrest. When the participant repeatedly failed to inhibit the response, the SSD could reach a minimum delay of 0 ms, thus making the stop signal a no-go signal (a no-go signal can be considered a stop signal with SSD = 0); when the participant repeatedly succeeded in inhibiting the response, the SSD could increase to a maximum of the entire duration of the target stimulus presentation (1,500 ms). However, among subjects, the maximum value reached was 814.79 ms, while the average of the max SSD across participants was 412.95 ± 123.85. SSRT was calculated (in ms) by subtracting a mean estimate of SSD from the observed mean reaction times (RTs) in no-stop trials. The duration of the task was about 14 min.
Because intra-individual variability in RTs is often observed in ADHD (Castellanos et al., 2005, 2006; Fassbender et al., 2009; Tamm et al., 2012), an additional analysis was performed on the variability of reaction times (VRTs) in correct go trials to see if different interventions reduced performance fluctuations.
The outputs of the SST consisted of the following parameters: Accuracy (percentage of corrected trials, including go and no-go trials), SSRT, RTs, VRTs.
In Figure 2, participants respond to the direction of arrows by pressing the corresponding arrow key in the go task. In one of the trials, the arrow is replaced by a stop symbol after a variable SSD. Each response is followed by positive or negative feedback.
The N-Back task is one of the most frequently used culture-free instruments to assess WM. The visual–spatial condition consists of presenting a series of visual stimuli (blue boxes) at a given position on the screen. After a training phase, participants had to indicate whether the position of each box presented was the same as that presented in previous trials. For example, in 2-Back task, participants had to indicate whether the current position was the same as the position in trial n − 2. When the accuracy was higher than 80%, the difficulty of the N-Back task increased (e.g., going from 1-Back to 2-Back). The N-Back index was determined based on the last unachieved span (i.e., when the accuracy percentage < 80%). For example, if the participant reached the 1-Back span (accuracy percentage ≥ 80%) and achieved only 30% accuracy in the 2-Back span, the N-Back index would be 2.3. The task took about 2 min to complete.
Outcome measures were collected at Day 0 and 10 min after the start of stimulation (Martin et al., 2014) or 90 min after MPH administration (maximum peak for MPH effects as mentioned in Ritalin® label), when each treatment achieved its maximum effect.
N-back and SST tasks were administered during tDCS, in agreement with studies (Miniussi and Vallar, 2011; Martin et al., 2014; Dedoncker et al., 2016) that have shown that accuracy in online tasks seems to be more effective than in offline tasks.
The order of N-back and SST was counterbalanced across sessions.
Overall, the evaluation took about 20 min to complete.
Safety and tolerability are important dimensions for validating and translating a treatment in the daily clinical routine. Therefore, symptoms and side effects were evaluated by using a standard questionnaire (Brunoni et al., 2011) completed by each participant after each tDCS session. The questionnaire assesses adverse effects such as headache, neck pain, scalp pain, tingling, itching, burning sensation, skin redness, sleepiness, trouble concentrating, and acute mood change. Participants quantified the intensity of the symptoms or side effects related to tDCS (1—absent; 2—mild; 3—moderate; 4—severe).
Sample size was calculated using a priori analysis in G*Power, version 3.1.9.7 (Faul et al., 2007). As previously reported in the study protocol (D’Aiello et al., 2022a), the sample size of 24 was calculated using a repeated-measures Analysis of Variance (ANOVA) model with four within-factors (baseline, anodal tDCS, sham tDCS, and MPH), considering an estimated f = 0.25, α value = 0.05 (i.e., probability of false positives of 5%), and β = 0.80 (i.e., at least 80% power).
Repeated-measures ANOVAs were conducted separately on SSRT, VRTs and Accuracy of SST with Condition (Day 0, anodal tDCS, sham tDCS, single dose of MPH) as a within-subjects factor. Post-hoc comparisons were run using Tukey’s honest significance test. Partial eta square (ηp2) was used as a measure of effect size.
The Friedman’s ANOVAs were employed to conduct nonparametric tests on the RTs of the SST and the visual–spatial N-Back index, as they did not follow a normal distribution. Post-hoc analyses were conducted by using Wilcoxon signed-rank tests to check for potential differences among conditions (Day 0, anodal tDCS, sham tDCS, and MPH). Cohens’ d was used as a measure of effect size.
To exclude sequences effect, non-parametric analysis was run for each outcome measure (see Supplementary materials: 1. Sequence effect analysis and 2. Sequence effect result).
A p value ≤0.05 was considered statistically significant.
Repeated-measures ANOVA documented a significant Condition Effect (F3,75 = 3.92, p = 0.011, ηp2 = 0.13) in the primary outcome, the SSRT (see Figure 3 panel A). Post-hoc comparisons showed a significant reduction of SSRT after a single dose of MPH compared with Day 0 (p = 0.006). However, no significant differences were found between MPH and anodal tDCS (p = 0.11) or between MPH and sham tDCS (p = 0.15).
Figure 3. Graph of outcome measures (SSRT, RTs, VRTs, Accuracy) at Day 0 and in anodal tDCS, sham tDCS and MPH conditions. (A) A significant reduction of SSRT was found after a single dose of MPH compared with Day 0. (B) No difference was found in RTs. (C) A significant reduction of VRTs after a single dose of MPH compared with Day 0, anodal tDCS, and sham tDCS. (D) No difference was found in the percentage of Accuracy of SST.
No further significant differences were found (anodal tDCS vs Day 0: p = 0.71; anodal tDCS vs sham tDCS: p = 0.99; sham tDCS vs Day 0: p = 0.62).
When considering RTs, Friedman’s ANOVA did not reveal significant differences (χ2(3) = 0.88, p = 0.83) among the conditions (see Figure 3 panel B).
Repeated-measures ANOVA showed a significant Condition effect (F3,75 = 7.90, p = 0.001, ηp2 = 0.24) in VRTs (see Figure 3 panel C). Post-hoc documented a significant reduction of VRTs after a single dose of MPH compared with Day 0 (p = 0.001), anodal tDCS (p = 0.0002), and sham tDCS (p = 0.047). No further significant differences were found (anodal tDCS vs Day 0: p = 0.92; anodal tDCS vs sham tDCS: p = 0.26; sham tDCS vs Day 0: p = 0.61).
Repeated-measures ANOVA showed no significant Condition effect (F3, 75 = 0.14, p = 0.93) in the Accuracy of SST (see Figure 3 panel D).
Friedman’s ANOVA revealed a significant difference among the conditions (χ2(3) = 17.75, p = 0.0005). Wilcoxon signed-rank tests documented a significant increment of visual–spatial N-Back index after a single dose of MPH compared with Day 0 (Z = 3.88, p < 0.0001, Cohens’ d = 2.3), anodal tDCS (Z = 2.62, p = 0.008, Cohens’ d = 1.2) and sham tDCS (Z = 3.06, p = 0.0002, Cohens’ d = 1.5). No further significant differences were found (anodal tDCS vs Day 0: p = 0.23; anodal tDCS vs sham tDCS: p = 0.57; sham tDCS vs Day 0: p = 0.22) (see Figure 4).
Figure 4. Graph of outcome measures (Visual–spatial N-Back) at Day 0 and in anodal tDCS, sham tDCS and MPH conditions. A significant increment of visual–spatial N-Back index was found after a single dose of MPH compared with Day 0, anodal tDCS and sham tDCS.
Supplementary Table S4 depicts mean and SD of outcome measures (SSRT, RTs, VRTs, Accuracy, Visual–spatial N-Back index) for Day 0, anodal tDCS, sham tDCS and MPH conditions (see Supplementary materials).
Regarding the safety questionnaire used to assess post-stimulation adverse effects (Miniussi and Vallar, 2011), most participants (24/26) did not experience any adverse effects other than itching during both active and sham stimulation. Only two participants with a very fair skin complexion experienced redness after the active stimulation session.
The present study is the first attempt to compare the effects of tDCS with those of established pharmacological treatment.
In this randomized, single-blind, sham-controlled study, children and adolescents with ADHD were exposed to a single session of anodal tDCS, sham tDCS, and a single dose of MPH.
After a single dose of MPH, results showed a significant improvement in inhibitory control (in terms of VRTs and SSRT) and visual–spatial WM compared with baseline, one session of anodal tDCS and sham tDCS. These findings corroborate previous evidence, showing MPH beneficial effects on inhibitory control and visual–spatial WM (Bedard et al., 2004; Spencer et al., 2009; Campez et al., 2022; D’Aiello et al., 2022b; Vertessen et al., 2022).
However, several explanations could be proposed for the lack of results on cognitive variables examined after left anodal tDCS on DLPFC. First, a possible reason may lay into the stimulated brain region – i.e., DLPFC. The choice of stimulating this brain region was based on previous studies demonstrating that the DLPFC abnormalities are physiologically associated with the cognitive deficits of ADHD (Faraone et al., 2015). For these reasons, the left DLPFC was widely targeted via tDCS to improve both inhibitory control and WM in ADHD (Salehinejad et al., 2019, 2020b; Breitling et al., 2020). During inhibitory tasks, such as SST, hypo-activation of the DLPFC has been observed in ADHD (Inoue et al., 2012; Monden et al., 2015) and the bilateral hypoactivity of the DLPFC (Lei et al., 2015) at the resting state (Hoekzema et al., 2014) and during cognitive tasks (Langleben et al., 2001). However, it should be considered that inhibitory control and visual–spatial WM involve a widespread network of fronto-parietal areas (Hart et al., 2012), the cerebellum, as documented by functional neuroimaging and tDCS studies in individuals without ADHD (Mannarelli et al., 2020) as well as the temporo-parietal regions (Garavan et al., 1999; Pliszka et al., 2006; Rubia et al., 2008, 2011; Sripada et al., 2014; Mencarelli et al., 2022). These brain regions have been little targeted (Westwood et al., 2021b) and should, therefore, be further explored in tDCS studies with children and adolescents with ADHD. Indeed, a recent review (Westwood et al., 2021b) underlined that out of 17 tDCS studies conducted in children and adolescents with ADHD, the majority have stimulated DLPFC (10/17), only one study targeted the ventromedial prefrontal cortex (Nejati et al., 2020), another one applied tDCS to the posterior parietal cortex (Salehinejad et al., 2020a), and the last delivered anodal tDCS to the right inferior frontal gyrus (Breitling et al., 2016, 2020; Breitling-Ziegler et al., 2021; Westwood et al., 2021a, 2022).
Second, a potential explanation could be due to the specificity of the neurophysiological actions associated with tDCS. Indeed, it is well-known that tDCS can induce the alteration of cortical excitability that depends on the dendro-axonic orientation of the pyramidal cells (Das et al., 2016; Yavari et al., 2018). However, once passing via the scalp, current flow through the brain is diffuse, reaching both the brain regions covered by the electrodes with a peak of intensity as well as large swaths of cortical and subcortical structures, although with lower intensity (Kronberg et al., 2017). Whereas, compared to tDCS, MPH has a stronger and direct modulatory effect on subcortical structures such as the striatum and putamen (Rubia et al., 2011) – which are mostly associated with inhibitory control and visual–spatial WM (Haber, 2017).
Third, a single stimulation of the anodal tDCS may not have been sufficient to give an effect. Although a single dose of MPH has been shown to affect brain network connectivity and cognitive symptoms (Epstein et al., 2007; Rubia et al., 2009, 2011; Kaiser et al., 2022), evidence converges that non-invasive brain stimulation techniques achieve their maximum effectiveness when the stimulation sessions are repeated (Fecteau et al., 2014; Meron et al., 2015; Lefaucheur et al., 2017). Multiple sessions of tDCS induce cumulative neurobiological effects over time, generating more robust neuroplasticity processes (Nitsche and Paulus, 2000; Boggio et al., 2009; Monte-Silva et al., 2013) that have greater efficacy on behavioral symptoms and neurocognitive measures.
The current results have helped confirm the safety and feasibility of tDCS in the pediatric population (Fritsch et al., 2010; Salehinejad et al., 2019, 2020b) as side effects are negligible, with at most scalp redness.
Finally, online administration of tDCS may have influenced the results. Although tDCS during task performance has been shown to increase the synaptic strength of neural networks already activated by concurrent tasks (Miniussi and Vallar, 2011; Martin et al., 2014; Dedoncker et al., 2016), some studies have documented that behaviors are more influenced by offline than online tDCS (Stagg et al., 2011; Amadi et al., 2015; Grasso et al., 2020, 2021).
Our study has some limitations. The first refers to the absence of a placebo pharmacological-control intervention to compare the effects obtained after MPH administration. Future studies involving four conditions (MPH, pharmacological placebo, anodal tDCS, and sham tDCS) could be useful to clarify the contribution of a single dose of MPH compared with placebo and the non-invasive brain stimulation conditions in the same group of children with ADHD.
The number of participants can also be considered a limitation of the present study. To confirm the superiority of the effects of MPH over tDCS, it will be necessary to collect data from larger groups.
In addition, neurophysiological and neuroimaging studies, such as EEG and/or MRI studies, could help explain the reduced effect of a single non-invasive brain stimulation session.
In conclusion, our protocol involving a single tDCS session in ADHD did not demonstrate consistent improvements in neuropsychological measures. Different protocols could be developed that provide significant effects. Different montages should be implemented to target other potential brain regions involved in inhibitory control and visual–spatial WM (i.e., posterior parietal cortex) beyond DLPFC. Other transcranial electrical stimulation techniques (e.g., high-definition tDCS) could be used so as to reach more deeply and focally the brain structures involved in ADHD symptoms. In addition, multi-session tDCS protocols could make more significant changes than a single session for ADHD, possibly in combination with usual treatments such as cognitive-behavioral psychotherapy and medication. However, our study has the merit of directly comparing tDCS with a pharmacological intervention (D’Aiello et al., 2022a), whereas most studies have examined the effects of tDCS as an adjunct to pharmacological treatment (Brunoni et al., 2013; Bennabi and Haffen, 2018; McLaren et al., 2018; Rigi Kooteh et al., 2019; Wang et al., 2021a). Direct comparison between tDCS and pharmacological intervention is important because it allows comparison of the parameters of tolerability, safety, and feasibility, which are crucial aspects of translating scientific findings into actual clinical practice.
In light of previous considerations, it should not be therefore excluded that tDCS could represent a promising treatment for ADHD-associated executive dysfunctions, and it is still an open area of research which merits to be further investigated.
The original contributions presented in the study are included in the article/Supplementary material, further inquiries can be directed to the corresponding author.
The studies involving human participants were reviewed and approved by the Ethics Committee of the Bambino Gesù Children Hospital (2185_OPBG_2020). Written informed consent to participate in this study was provided by the participants’ legal guardian/next of kin.
BD, DM, and SV: conceptualization. BD, DM, GL, PP, and SV: methodology. SV and DM: supervision. BD, GL, and AB: writing original draft. BD, DM, GL, AB, PDR, SDV, IP, FC, and SV: writing review and editing. All authors have read and agreed to the published version of the manuscript.
This work was supported by the Italian Ministry of Health with “Current Research” funds.
The authors declare that the research was conducted in the absence of any commercial or financial relationships that could be construed as a potential conflict of interest.
All claims expressed in this article are solely those of the authors and do not necessarily represent those of their affiliated organizations, or those of the publisher, the editors and the reviewers. Any product that may be evaluated in this article, or claim that may be made by its manufacturer, is not guaranteed or endorsed by the publisher.
The Supplementary material for this article can be found online at: https://www.frontiersin.org/articles/10.3389/fnins.2023.1170090/full#supplementary-material
Achenbach, T. M. (1991). Manual for the youth self-report and 1991 profile. Burlington: Department of Psychiatry, University of Vermont.
Amadi, U., Allman, C., Johansen-Berg, H., and Stagg, C. J. (2015). The homeostatic interaction between anodal transcranial direct current stimulation and motor learning in humans is related to GABAA activity. Brain Stimul. 8, 898–905. doi: 10.1016/j.brs.2015.04.010
American Psychiatric Association (2013) Diagnostic and statistical manual of mental disorders (5th). Virginia: American Psychiatric Association.
Arnsten, A. F. T. (2006). Stimulants: therapeutic actions in ADHD. Neuropsychopharmacology 31, 2376–2383. doi: 10.1038/sj.npp.1301164
Atkinson, M., and Hollis, C. (2010). NICE guideline: attention deficit hyperactivity disorder. Arch. Dis. Childh. Educ. Pract. 95, 24–27. doi: 10.1136/adc.2009.175943
Battisti, A., Lazzaro, G., Costanzo, F., Varuzza, C., Rossi, S., Vicari, S., et al. (2022). Effects of a short and intensive transcranial direct current stimulation treatment in children and adolescents with developmental dyslexia: a crossover clinical trial. Front. Psychol. 13:986242. doi: 10.3389/fpsyg.2022.986242
Bedard, A. C., Martinussen, R., Ickowicz, A., and Tannock, R. (2004). Methylphenidate improves visual-spatial memory in children with attention-deficit/hyperactivity disorder. J. Am. Acad. Child Adolesc. Psychiatry 43, 260–268. doi: 10.1097/00004583-200403000-00006
Bennabi, D., and Haffen, E. (2018). Transcranial direct current stimulation (tDCS): a promising treatment for major depressive disorder? Brain Sci. 8:81. doi: 10.3390/brainsci8050081
Berger, I., Dakwar-Kawar, O., Grossman, E. S., Nahum, M., and Cohen, K. R. (2021). Scaffolding the attention-deficit/hyperactivity disorder brain using transcranial direct current and random noise stimulation: a randomized controlled trial. Clin. Neurophysiol. 132, 699–707. doi: 10.1016/j.clinph.2021.01.005
Boggio, P. S., Zaghi, S., and Fregni, F. (2009). Modulation of emotions associated with images of human pain using anodal transcranial direct current stimulation (tDCS). Neuropsychologia 47, 212–217. doi: 10.1016/j.neuropsychologia.2008.07.022
Breitling, C., Zaehle, T., Dannhauer, M., Bonath, B., Tegelbeckers, J., Flechtner, H. H., et al. (2016). Improving interference control in ADHD patients with transcranial direct current stimulation (tDCS). Front. Cell Neurosci. 10:e0072. doi: 10.3389/fncel.2016.00072
Breitling, C., Zaehle, T., Dannhauer, M., Tegelbeckers, J., Flechtner, H. H., and Krauel, K. (2020). Comparison between conventional and HD-tDCS of the right inferior frontal gyrus in children and adolescents with ADHD. Clin. Neurophysiol. 131, 1146–1154. doi: 10.1016/j.clinph.2019.12.412
Breitling-Ziegler, C., Zaehle, T., Wellnhofer, C., Dannhauer, M., Tegelbeckers, J., Baumann, V., et al. (2021). Effects of a five-day HD-tDCS application to the right IFG depend on current intensity: a study in children and adolescents with ADHD. Prog. Brain Res., 117–150.
Brunoni, A. R., Amadera, J., Berbel, B., Volz, M. S., Rizzerio, B. G., and Fregni, F. (2011). A systematic review on reporting and assessment of adverse effects associated with transcranial direct current stimulation. Int. J. Neuropsychopharmacol. 14, 1133–1145. doi: 10.1017/S1461145710001690
Brunoni, A. R., Boggio, P. S., De Raedt, R., Benseñor, I. M., Lotufo, P. A., Namur, V., et al. (2014). Cognitive control therapy and transcranial direct current stimulation for depression: a randomized, double-blinded, controlled trial. J. Affect. Disord. 162, 43–49. doi: 10.1016/j.jad.2014.03.026
Brunoni, A. R., Ferrucci, R., Bortolomasi, M., Scelzo, E., Boggio, P. S., Fregni, F., et al. (2013). Interactions between transcranial direct current stimulation (tDCS) and pharmacological interventions in the major depressive episode: findings from a naturalistic study. Eur. Psychiatry 28, 356–361. doi: 10.1016/j.eurpsy.2012.09.001
Campez, M., Raiker, J. S., Little, K., Altszuler, A. R., Merrill, B. M., Macphee, F. L., et al. (2022). An evaluation of the effect of methylphenidate on working memory, time perception, and choice impulsivity in children with ADHD. Exp. Clin. Psychopharmacol. 30, 209–219. doi: 10.1037/pha0000446
Castellanos, F. X. (2002). Developmental trajectories of brain volume abnormalities in children and adolescents with attention-deficit/hyperactivity disorder. JAMA 288, 1740–1748. doi: 10.1001/jama.288.14.1740
Castellanos, F. X., Sonuga-Barke, E. J. S., Milham, M. P., and Tannock, R. (2006). Characterizing cognition in ADHD: beyond executive dysfunction. Trends Cogn. Sci. 10, 117–123. doi: 10.1016/j.tics.2006.01.011
Castellanos, F. X., Sonuga-Barke, E. J. S., Scheres, A., Di Martino, A., Hyde, C., and Walters, J. R. (2005). Varieties of attention-deficit/hyperactivity disorder-related intra-individual variability. Biol. Psychiatry 57, 1416–1423. doi: 10.1016/j.biopsych.2004.12.005
Coghill, D. R., Seth, S., Pedroso, S., Usala, T., Currie, J., and Gagliano, A. (2014). Effects of methylphenidate on cognitive functions in children and adolescents with attention-deficit/hyperactivity disorder: evidence from a systematic review and a Meta-analysis. Biol. Psychiatry 76, 603–615. doi: 10.1016/j.biopsych.2013.10.005
Conners, C. K., Sitarenios, G., Parker, J. D. A., and Epstein, J. N. (1998). The revised Conners’ Parent Rating Scale (CPRS-R): factor structure, reliability, and criterion validity. J. Abnorm. Child Psychol. 26, 257–268. doi: 10.1023/a:1022602400621
Cortese, S., Adamo, N., Del Giovane, C., Mohr-Jensen, C., Hayes, A. J., Carucci, S., et al. (2018). Comparative efficacy and tolerability of medications for attention-deficit hyperactivity disorder in children, adolescents, and adults: a systematic review and network meta-analysis. Lancet Psychiatry 5, 727–738. doi: 10.1016/S2215-0366(18)30269-4
Cortese, S., Kelly, C., Chabernaud, C., Proal, E., Di Martino, A., Milham, M. P., et al. (2012). Toward systems neuroscience of ADHD: a Meta-analysis of 55 fMRI studies. AJP 169, 1038–1055. doi: 10.1176/appi.ajp.2012.11101521
Costanzo, F., Varuzza, C., Rossi, S., Sdoia, S., Varvara, P., Oliveri, M., et al. (2016). Reading changes in children and adolescents with dyslexia after transcranial direct current stimulation. Neuroreport 27, 295–300. doi: 10.1097/WNR.0000000000000536
D’Aiello, B., Battisti, A., Lazzaro, G., Pani, P., De Rossi, P., Di Vara, S., et al. (2022a). Comparing the effect of methylphenidate and anodal tDCS on inhibitory control and working-memory in children and adolescents with attention deficit/hyperactivity disorder: a study protocol for a randomized, within-subject trial. IJERPH 19:4575. doi: 10.3390/ijerph19084575
D’Aiello, B., Di Vara, S., De Rossi, P., Pretelli, I., Vicari, S., and Menghini, D. (2022b). Moderators and other predictors of methylphenidate response in children and adolescents with ADHD. IJERPH 19:1640. doi: 10.3390/ijerph19031640
Danielson, M. L., Holbrook, J. R., Bitsko, R. H., Newsome, K., Charania, S. N., McCord, R. F., et al. (2022). State-level estimates of the prevalence of parent-reported ADHD diagnosis and treatment among U.S. children and adolescents, 2016 to 2019. J. Atten. Disord. 26, 1685–1697. doi: 10.1177/10870547221099961
Das, S., Holland, P., Frens, M. A., and Donchin, O. (2016). Impact of transcranial direct current stimulation (tDCS) on neuronal functions. Front. Neurosci. 10:550. doi: 10.3389/fnins.2016.00550
Dedoncker, J., Brunoni, A. R., Baeken, C., and Vanderhasselt, M. A. (2016). A systematic review and Meta-analysis of the effects of transcranial direct current stimulation (tDCS) over the dorsolateral prefrontal cortex in healthy and neuropsychiatric samples: influence of stimulation parameters. Brain Stimul. 9, 501–517. doi: 10.1016/j.brs.2016.04.006
Epstein, J. N., Casey, B. J., Tonev, S. T., Davidson, M. C., Reiss, A. L., Garrett, A., et al. (2007). ADHD- and medication-related brain activation effects in concordantly affected parent-child dyads with ADHD: ADHD frontostriatal dysfunction. J. Child Psychol. Psychiatry 48, 899–913. doi: 10.1111/j.1469-7610.2007.01761.x
Faraone, S. V., Asherson, P., Banaschewski, T., Biederman, J., Buitelaar, J. K., Ramos-Quiroga, J. A., et al. (2015). Attention-deficit/hyperactivity disorder. Nat. Rev. Dis. Primers. 1:15020. doi: 10.1038/nrdp.2015.20
Faraone, S. V., Banaschewski, T., Coghill, D., Zheng, Y., Biederman, J., Bellgrove, M. A., et al. (2021). The world federation of ADHD international consensus statement: 208 evidence-based conclusions about the disorder. Neurosci. Biobehav. Rev. 128, 789–818. doi: 10.1016/j.neubiorev.2021.01.022
Fassbender, C., Zhang, H., Buzy, W. M., Cortes, C. R., Mizuiri, D., Beckett, L., et al. (2009). A lack of default network suppression is linked to increased distractibility in ADHD. Brain Res. 1273, 114–128. doi: 10.1016/j.brainres.2009.02.070
Faul, F., Erdfelder, E., Lang, A. G., and Buchner, A. (2007). G*power 3: a flexible statistical power analysis program for the social, behavioral, and biomedical sciences. Behav. Res. Methods 39, 175–191. doi: 10.3758/BF03193146
Fecteau, S., Agosta, S., Hone-Blanchet, A., Fregni, F., Boggio, P., Ciraulo, D., et al. (2014). Modulation of smoking and decision-making behaviors with transcranial direct current stimulation in tobacco smokers: a preliminary study. Drug Alcohol Depend. 140, 78–84. doi: 10.1016/j.drugalcdep.2014.03.036
Finisguerra, A., Borgatti, R., and Urgesi, C. (2019). Non-invasive brain stimulation for the rehabilitation of children and adolescents with neurodevelopmental disorders: a systematic review. Front. Psychol. 10:135. doi: 10.3389/fpsyg.2019.00135
Friehs, M. A., Frings, C., and Hartwigsen, G. (2021). Effects of single-session transcranial direct current stimulation on reactive response inhibition. Neurosci. Biobehav. Rev. 128, 749–765. doi: 10.1016/j.neubiorev.2021.07.013
Fritsch, B., Reis, J., Martinowich, K., Schambra, H. M., Ji, Y., Cohen, L. G., et al. (2010). Direct current stimulation promotes BDNF-dependent synaptic plasticity: potential implications for motor learning. Neuron 66, 198–204. doi: 10.1016/j.neuron.2010.03.035
Gandiga, P. C., Hummel, F. C., and Cohen, L. G. (2006). Transcranial DC stimulation (tDCS): a tool for double-blind sham-controlled clinical studies in brain stimulation. Clin. Neurophysiol. 117, 845–850. doi: 10.1016/j.clinph.2005.12.003
Garavan, H., Ross, T. J., and Stein, E. A. (1999). Right hemispheric dominance of inhibitory control: an event-related functional MRI study. Proc. Natl. Acad. Sci. U. S. A. 96, 8301–8306. doi: 10.1073/pnas.96.14.8301
Grasso, P. A., Tonolli, E., Bortoletto, M., and Miniussi, C. (2021). tDCS over posterior parietal cortex increases cortical excitability but decreases learning: an ERPs and TMS-EEG study. Brain Res. 1753:147227. doi: 10.1016/j.brainres.2020.147227
Grasso, P. A., Tonolli, E., and Miniussi, C. (2020). Effects of different transcranial direct current stimulation protocols on visuo-spatial contextual learning formation: evidence of homeostatic regulatory mechanisms. Sci. Rep. 10:4622. doi: 10.1038/s41598-020-61626-7
Guven, A., Altinkaynak, M., Dolu, N., Demirci, E., Ozmen, S., Izzetoglu, M., et al. (2018). Effects of methylphenidate on reaction time in children with attention deficit hyperactivity disorder. Arch. Neuropsych. 56, 27–31. doi: 10.29399/npa.22873
Haber, S. N. (2017). Anatomy and connectivity of the reward circuit. Decision Neurosci. 3–19. doi: 10.1016/B978-0-12-805308-9.00001-4
Hart, H., Radua, J., Mataix-Cols, D., and Rubia, K. (2012). Meta-analysis of fMRI studies of timing in attention-deficit hyperactivity disorder (ADHD). Neurosci. Biobehav. Rev. 36, 2248–2256. doi: 10.1016/j.neubiorev.2012.08.003
Hill, A. T., Fitzgerald, P. B., and Hoy, K. E. (2016). Effects of anodal transcranial direct current stimulation on working memory: a systematic review and Meta-analysis of findings from healthy and neuropsychiatric populations. Brain Stimul. 9, 197–208. doi: 10.1016/j.brs.2015.10.006
Hoekzema, E., Carmona, S., Ramos-Quiroga, J. A., Richarte Fernández, V., Bosch, R., Soliva, J. C., et al. (2014). An independent components and functional connectivity analysis of resting state fMRI data points to neural network dysregulation in adult ADHD: neural network dysregulation in adult ADHD. Hum. Brain Mapp. 35, 1261–1272. doi: 10.1002/hbm.22250
Inoue, Y., Sakihara, K., Gunji, A., Ozawa, H., Kimiya, S., Shinoda, H., et al. (2012). Reduced prefrontal hemodynamic response in children with ADHD during the go/NoGo task: a NIRS study. Neuroreport 23, 55–60. doi: 10.1097/WNR.0b013e32834e664c
Jamil, A., Batsikadze, G., Kuo, H. I., Labruna, L., Hasan, A., Paulus, W., et al. (2017). Systematic evaluation of the impact of stimulation intensity on neuroplastic after-effects induced by transcranial direct current stimulation: effects of DC intensity on cortical excitability. J. Physiol. 595, 1273–1288. doi: 10.1113/JP272738
Kaiser, A., Broeder, C., Cohen, J. R., Douw, L., Reneman, L., and Schrantee, A. (2022). Effects of a single-dose methylphenidate challenge on resting-state functional connectivity in stimulant-treatment naive children and adults with ADHD. Hum. Brain Mapp. 43, 4664–4675. doi: 10.1002/hbm.25981
Kaufman, J. K-SADS-PL DSM-5®: intervista diagnostica per la valutazione dei disturbi psicopatologici in bambini e adolescenti. Trento: Erickson (2019).
Kessler, S. K., Minhas, P., Woods, A. J., Rosen, A., Gorman, C., and Bikson, M. (2013). Dosage considerations for transcranial direct current stimulation in children: a computational modeling study. Chambers C, editor. PLoS One 8:e76112. doi: 10.1371/journal.pone.0076112
Klomjai, W., and Aneksan, B. (2022). A randomized sham-controlled trial on the effects of dual-tDCS “during” physical therapy on lower limb performance in sub-acute stroke and a comparison to the previous study using a “before” stimulation protocol. BMC Sports Sci. Med. Rehabil. 14:68. doi: 10.1186/s13102-022-00463-9
Knouse, L. E., Teller, J., and Brooks, M. A. (2017). Meta-analysis of cognitive–behavioral treatments for adult ADHD. J. Consult. Clin. Psychol. 85, 737–750. doi: 10.1037/ccp0000216
Kronberg, G., Bridi, M., Abel, T., Bikson, M., and Parra, L. C. (2017). Direct current stimulation modulates LTP and LTD: activity dependence and dendritic effects. Brain Stimul. 10, 51–58. doi: 10.1016/j.brs.2016.10.001
Kuo, M. F., Polanía, R., and Nitsche, M. (2016). “Physiology of transcranial direct and alternating current stimulation” in Transcranial direct current stimulation in neuropsychiatric disorders [internet]. eds. A. Brunoni, M. Nitsche, and C. Loo (Cham: Springer International Publishing), 29–46.
Langleben, D. D., Austin, G., Krikorian, G., Ridlehuber, H. W., Goris, M. L., and Strauss, H. W. (2001). Interhemispheric asymmetry of regional cerebral blood flow in prepubescent boys with attention deficit hyperactivity disorder. Nucl. Med. Commun. 22, 1333–1340. doi: 10.1097/00006231-200112000-00009
Lazzaro, G., Costanzo, F., Varuzza, C., Rossi, S., Vicari, S., and Menghini, D. (2021). Effects of a short, intensive, multi-session tDCS treatment in developmental dyslexia: preliminary results of a sham-controlled randomized clinical trial. Progr. Brain Res., 191–210. doi: 10.1016/bs.pbr.2021.01.015
Lefaucheur, J. P., Antal, A., Ayache, S. S., Benninger, D. H., Brunelin, J., Cogiamanian, F., et al. (2017). Evidence-based guidelines on the therapeutic use of transcranial direct current stimulation (tDCS). Clin. Neurophysiol. 128, 56–92. doi: 10.1016/j.clinph.2016.10.087
Lei, D., Du, M., Wu, M., Chen, T., Huang, X., Du, X., et al. (2015). Functional MRI reveals different response inhibition between adults and children with ADHD. Neuropsychology 29, 874–881. doi: 10.1037/neu0000200
Li, L., Taylor, M. J., Bälter, K., Kuja-Halkola, R., Chen, Q., Hegvik, T., et al. (2020). Attention-deficit/hyperactivity disorder symptoms and dietary habits in adulthood: a large population-based twin study in Sweden. Am. J. Med. Genet. 183, 475–485. doi: 10.1002/ajmg.b.32825
Logan, G. D., and Cowan, W. B. (1984). On the ability to inhibit thought and action: a theory of an act of control. Psychol. Rev. 91, 295–327. doi: 10.1037/0033-295X.91.3.295
Lukito, S., Norman, L., Carlisi, C., Radua, J., Hart, H., Simonoff, E., et al. (2020). Comparative meta-analyses of brain structural and functional abnormalities during cognitive control in attention-deficit/hyperactivity disorder and autism spectrum disorder. Psychol. Med. 50, 894–919. doi: 10.1017/S0033291720000574
Mancuso, L. E., Ilieva, I. P., Hamilton, R. H., and Farah, M. J. (2016). Does transcranial direct current stimulation improve healthy working memory?: a Meta-analytic review. J. Cogn. Neurosci. 28, 1063–1089. doi: 10.1162/jocn_a_00956
Mannarelli, D., Pauletti, C., Petritis, A., Delle Chiaie, R., Currà, A., Trompetto, C., et al. (2020). Effects of cerebellar tDCS on inhibitory control: evidence from a go/NoGo task. Cerebellum 19, 788–798. doi: 10.1007/s12311-020-01165-z
Martin, D. M., Liu, R., Alonzo, A., Green, M., and Loo, C. K. (2014). Use of transcranial direct current stimulation (tDCS) to enhance cognitive training: effect of timing of stimulation. Exp. Brain Res. 232, 3345–3351. doi: 10.1007/s00221-014-4022-x
McCarthy, H., Skokauskas, N., and Frodl, T. (2014). Identifying a consistent pattern of neural function in attention deficit hyperactivity disorder: a meta-analysis. Psychol. Med. 44, 869–880. doi: 10.1017/S0033291713001037
McLaren, M. E., Nissim, N. R., and Woods, A. J. (2018). The effects of medication use in transcranial direct current stimulation: a brief review. Brain Stimul. 11, 52–58. doi: 10.1016/j.brs.2017.10.006
Mencarelli, L., Romanella, S. M., Di Lorenzo, G., Demchenko, I., Bhat, V., Rossi, S., et al. (2022). Neural correlates of N-back task performance and proposal for corresponding neuromodulation targets in psychiatric and neurodevelopmental disorders. Psychiatry Clin. Neurosci. 76, 512–524. doi: 10.1111/pcn.13442
Meron, D., Hedger, N., Garner, M., and Baldwin, D. S. (2015). Transcranial direct current stimulation (tDCS) in the treatment of depression: systematic review and meta-analysis of efficacy and tolerability. Neurosci. Biobehav. Rev. 57, 46–62. doi: 10.1016/j.neubiorev.2015.07.012
Minhas, P, Bikson, M, Woods, AJ, Rosen, AR, and Kessler, SK. Transcranial direct current stimulation in pediatric brain: a computational modeling study. In: 2012 Annual International Conference of the IEEE Engineering in Medicine and Biology Society [Internet]. San Diego, CA: IEEE (2012).
Miniussi, C., and Vallar, G. (2011). Brain stimulation and behavioural cognitive rehabilitation: a new tool for neurorehabilitation? Neuropsychol. Rehabil. 21, 553–559. doi: 10.1080/09602011.2011.622435
Monden, Y., Dan, I., Nagashima, M., Dan, H., Uga, M., Ikeda, T., et al. (2015). Individual classification of ADHD children by right prefrontal hemodynamic responses during a go/no-go task as assessed by fNIRS. NeuroImage 9, 1–12. doi: 10.1016/j.nicl.2015.06.011
Monte-Silva, K., Kuo, M. F., Hessenthaler, S., Fresnoza, S., Liebetanz, D., Paulus, W., et al. (2013). Induction of late LTP-like plasticity in the human motor cortex by repeated non-invasive brain stimulation. Brain Stimul. 6, 424–432. doi: 10.1016/j.brs.2012.04.011
Munz, M. T., Prehn-Kristensen, A., Thielking, F., Mölle, M., Göder, R., and Baving, L. (2015). Slow oscillating transcranial direct current stimulation during non-rapid eye movement sleep improves behavioral inhibition in attention-deficit/hyperactivity disorder. Front. Cell Neurosci. 9:307. doi: 10.3389/fncel.2015.00307
Nejati, V., Salehinejad, M. A., Nitsche, M. A., Najian, A., and Javadi, A. H. (2020). Transcranial direct current stimulation improves executive dysfunctions in ADHD: implications for inhibitory control, interference control, working memory, and cognitive flexibility. J. Atten. Disord. 24, 1928–1943. doi: 10.1177/1087054717730611
Nigg, J. T., and Casey, B. J. (2005). An integrative theory of attention-deficit/ hyperactivity disorder based on the cognitive and affective neurosciences. Dev. Psychopathol. 17, 785–806. doi: 10.1017/S0954579405050376
Nitsche, M. A., and Paulus, W. (2000). Excitability changes induced in the human motor cortex by weak transcranial direct current stimulation. J. Physiol. 527, 633–639. doi: 10.1111/j.1469-7793.2000.t01-1-00633.x
Norman, L. J., Carlisi, C., Lukito, S., Hart, H., Mataix-Cols, D., Radua, J., et al. (2016). Structural and functional brain abnormalities in attention-deficit/hyperactivity disorder and obsessive-compulsive disorder: a comparative Meta-analysis. JAMA Psychiat. 73, 815–825. doi: 10.1001/jamapsychiatry.2016.0700
Opitz, A., Paulus, W., Will, S., Antunes, A., and Thielscher, A. (2015). Determinants of the electric field during transcranial direct current stimulation. NeuroImage 109, 140–150. doi: 10.1016/j.neuroimage.2015.01.033
Orsini, A., Pezzuti, L., and Picone, L. (2012). WISC-IV. Contributo alla taratura italiana. Firenze: Giunti O.S.
Peirce, J., Gray, J. R., Simpson, S., MacAskill, M., Höchenberger, R., Sogo, H., et al. (2019). PsychoPy2: experiments in behavior made easy. Behav Res. 51, 195–203. doi: 10.3758/s13428-018-01193-y
Pliszka, G. D. C., Semrud-Clikman, M., Franklin, C., Perez, R., Xiong, J., and Liotti, M. (2006). Neuroimaging of inhibitory control areas in children with attention deficit hyperactivity disorder who were treatment naive or in long-term treatment. Am. J. Psychiatry 163, 1052–1060. doi: 10.1176/ajp.2006.163.6.1052
Raven, J. C., and Court, John Hugh. (1998). Raven’s progressive matrices and vocabulary scales Oxford: Oxford Psychologists Press.
Rigi Kooteh, B., Bakhshani, N. M., Nosratabadi, M., and Dolatshahi, B. (2019). Effectiveness of transcranial direct-current stimulation (tDCS) and emotion regulation training in reducing current drug craving and drug-use thoughts and fantasies in opioid-dependent patients: the issue of precedence. Int. J. High. Risk Behav. Addict. 8. doi: 10.5812/ijhrba.94499
Rimestad, M. L., Lambek, R., Zacher Christiansen, H., and Hougaard, E. (2019). Short- and long-term effects of parent training for preschool children with or at risk of ADHD: a systematic review and Meta-analysis. J. Atten. Disord. 23, 423–434. doi: 10.1177/1087054716648775
Rubia, K., Halari, R., Cubillo, A., Mohammad, A. M., Brammer, M., and Taylor, E. (2009). Methylphenidate normalises activation and functional connectivity deficits in attention and motivation networks in medication-naïve children with ADHD during a rewarded continuous performance task. Neuropharmacology 57, 640–652. doi: 10.1016/j.neuropharm.2009.08.013
Rubia, K., Halari, R., Cubillo, A., Smith, A. B., Mohammad, A. M., Brammer, M., et al. (2011). Methylphenidate normalizes Fronto-striatal Underactivation during interference inhibition in medication-Naïve boys with attention-deficit hyperactivity disorder. Neuropsychopharmacology 36, 1575–1586. doi: 10.1038/npp.2011.30
Rubia, K., Halari, R., Smith, A. B., Mohammed, M., Scott, S., Giampietro, V., et al. (2008). Dissociated functional brain abnormalities of inhibition in boys with pure conduct disorder and in boys with pure attention deficit hyperactivity disorder. AJP 165, 889–897. doi: 10.1176/appi.ajp.2008.07071084
Salehinejad, MA, Ghanavati, E, Glinski, B, Hallajian, AH, and Azarkolah, A. A systematic review of randomized controlled trials on efficacy and safety of transcranial direct current stimulation in major neurodevelopmental disorders ADHD, Autism, and dyslexia [Internet] PsyArXiv (2022).
Salehinejad, M. A., Ghayerin, E., Nejati, V., Yavari, F., and Nitsche, M. A. (2020a). Domain-specific involvement of the right posterior parietal cortex in attention network and attentional control of ADHD: a randomized, cross-over Sham-controlled tDCS Study. Neuroscience 444, 149–159. doi: 10.1016/j.neuroscience.2020.07.037
Salehinejad, M. A., Nejati, V., Mosayebi-Samani, M., Mohammadi, A., Wischnewski, M., Kuo, M. F., et al. (2020b). Transcranial direct current stimulation in ADHD: a systematic review of efficacy, safety, and protocol-induced electrical field modeling results. Neurosci. Bull. 36, 1191–1212. doi: 10.1007/s12264-020-00501-x
Salehinejad, M. A., Wischnewski, M., Nejati, V., Vicario, C. M., and Nitsche, M. A. (2019). Transcranial direct current stimulation in attention-deficit hyperactivity disorder: a meta-analysis of neuropsychological deficits. Avenanti a, editor. PLoS One 14:e0215095. doi: 10.1371/journal.pone.0215095
Schiffer, W. K., Volkow, N. D., Fowler, J. S., Alexoff, D. L., Logan, J., and Dewey, S. L. (2006). Therapeutic doses of amphetamine or methylphenidate differentially increase synaptic and extracellular dopamine. Synapse 59, 243–251. doi: 10.1002/syn.20235
Scionti, N., Cavallero, M., Zogmaister, C., and Marzocchi, G. M. (2020). Is cognitive training effective for improving executive functions in preschoolers? A systematic review and Meta-analysis. Front. Psychol. 10:2812. doi: 10.3389/fpsyg.2019.02812
Shaffer, D. (1983). A Children’s global assessment scale (CGAS). Arch. Gen. Psychiatry 40:1228. doi: 10.1001/archpsyc.1983.01790100074010
Soltaninejad, Z., Nejati, V., and Ekhtiari, H. (2019). Effect of anodal and cathodal transcranial direct current stimulation on DLPFC on modulation of inhibitory control in ADHD. J. Atten. Disord. 23, 325–332. doi: 10.1177/1087054715618792
Sonuga-Barke, E., Bitsakou, P., and Thompson, M. (2010). Beyond the dual pathway model: evidence for the dissociation of timing, inhibitory, and delay-related impairments in attention-deficit/hyperactivity disorder. J. Am. Acad. Child Adolesc. Psychiatry 49, 345–355. doi: 10.1097/00004583-201004000-00009
Spencer, S. V., Hawk, L. W., Richards, J. B., Shiels, K., Pelham, W. E., and Waxmonsky, J. G. (2009). Stimulant treatment reduces lapses in attention among children with ADHD: the effects of methylphenidate on intra-individual response time distributions. J. Abnorm. Child Psychol. 37, 805–816. doi: 10.1007/s10802-009-9316-2
Sripada, C. S., Kessler, D., and Angstadt, M. (2014). Lag in maturation of the brain’s intrinsic functional architecture in attention-deficit/hyperactivity disorder. Proc. Natl. Acad. Sci. U. S. A. 111, 14259–14264. doi: 10.1073/pnas.1407787111
Stagg, C. J., Jayaram, G., Pastor, D., Kincses, Z. T., Matthews, P. M., and Johansen-Berg, H. (2011). Polarity and timing-dependent effects of transcranial direct current stimulation in explicit motor learning. Neuropsychologia 49, 800–804. doi: 10.1016/j.neuropsychologia.2011.02.009
Stein, M. A., Sarampote, C. S., Waldman, I. D., Robb, A. S., Conlon, C., Pearl, P. L., et al. (2003). A dose-response study of OROS methylphenidate in children with attention-deficit/hyperactivity disorder. Pediatrics 112:e404. doi: 10.1542/peds.112.5.e404
Swanson, J, Nolan, W, and Pelham, WE. The SNAP rating scale for the diagnosis of attention deficit disorder. Paper presented at the meeting of the American Psychological Association Los Angeles. (1981).
Tamm, L., Narad, M. E., Antonini, T. N., O’Brien, K. M., Hawk, L. W., and Epstein, J. N. (2012). Reaction time variability in ADHD: a review. Neurotherapeutics 9, 500–508. doi: 10.1007/s13311-012-0138-5
Taylor, E., Dpfner, M., Sergeant, J., Asherson, P., Banaschewski, T., Buitelaar, J., et al. (2004). European clinical guidelines for hyperkinetic disorder? First upgrade. Euro. Child Adolesc. Psychiatry 13, I7–I30. doi: 10.1007/s00787-004-1002-x
Thomas, R., Sanders, S., Doust, J., Beller, E., and Glasziou, P. (2015). Prevalence of attention-deficit/hyperactivity disorder: a systematic review and Meta-analysis. Pediatrics 135, e994–e1001. doi: 10.1542/peds.2014-3482
Verbruggen, F., Aron, A. R., Band, G. P., Beste, C., Bissett, P. G., Brockett, A. T., et al. (2019). A consensus guide to capturing the ability to inhibit actions and impulsive behaviors in the stop-signal task. elife 8:e46323. doi: 10.7554/eLife.46323
Vertessen, K., Luman, M., Staff, A., Bet, P., de Vries, R., Twisk, J., et al. (2022). Meta-analysis: dose-dependent effects of methylphenidate on neurocognitive functioning in children with attention-deficit/hyperactivity disorder. J. Am. Acad. Child Adolesc. Psychiatry 61, 626–646. doi: 10.1016/j.jaac.2021.08.023
Volkow, N. D., Wang, G. J., Newcorn, J., Fowler, J. S., Telang, F., Solanto, M. V., et al. (2007). Brain dopamine transporter levels in treatment and drug naïve adults with ADHD. NeuroImage 34, 1182–1190. doi: 10.1016/j.neuroimage.2006.10.014
Wang, J., Luo, H., Schülke, R., Geng, X., Sahakian, B. J., and Wang, S. (2021). Is transcranial direct current stimulation, alone or in combination with antidepressant medications or psychotherapies, effective in treating major depressive disorder? A systematic review and meta-analysis. BMC Med. 19:319. doi: 10.1186/s12916-021-02181-4
Westwood, S. J., Bozhilova, N., Criaud, M., Lam, S. L., Lukito, S., Wallace-Hanlon, S., et al. (2022). The effect of transcranial direct current stimulation (tDCS) combined with cognitive training on EEG spectral power in adolescent boys with ADHD: a double-blind, randomized, sham-controlled trial. IBRO Neurosci. Rep. 12, 55–64. doi: 10.1016/j.ibneur.2021.12.005
Westwood, S. J., Criaud, M., Lam, S. L., Lukito, S., Wallace-Hanlon, S., Kowalczyk, O. S., et al. (2021a). Transcranial direct current stimulation (tDCS) combined with cognitive training in adolescent boys with ADHD: a double-blind, randomised, sham-controlled trial. Psychol. Med. 1–16, 1–16. doi: 10.1017/S0033291721001859
Westwood, S. J., Radua, J., and Rubia, K. (2021b). Noninvasive brain stimulation in children and adults with attention-deficit/hyperactivity disorder: a systematic review and meta-analysis. JPN 46, E14–E33. doi: 10.1503/jpn.190179
Willcutt, E. G. (2012). The prevalence of DSM-IV attention-deficit/hyperactivity disorder: a Meta-analytic review. Neurotherapeutics 9, 490–499. doi: 10.1007/s13311-012-0135-8
Keywords: MPH, drug treatments, non-invasive brain stimulation, transcranial direct current stimulation, executive functions, evidence-based medicine, neurodevelopmental disorders
Citation: D’Aiello B, Lazzaro G, Battisti A, Pani P, Di Vara S, De Rossi P, Pretelli I, Costanzo F, Vicari S and Menghini D (2023) Methylphenidate is more effective to improve inhibitory control and working memory compared to tDCS in children and adolescents with attention deficit/hyperactivity disorder: a proof-of-concept study. Front. Neurosci. 17:1170090. doi: 10.3389/fnins.2023.1170090
Received: 20 February 2023; Accepted: 22 June 2023;
Published: 07 July 2023.
Edited by:
Michael Siniatchkin, Department of Child and Adolescent Psychiatry, GermanyReviewed by:
Paolo Antonino Grasso, University of Florence, ItalyCopyright © 2023 D’Aiello, Lazzaro, Battisti, Pani, Di Vara, De Rossi, Pretelli, Costanzo, Vicari and Menghini. This is an open-access article distributed under the terms of the Creative Commons Attribution License (CC BY). The use, distribution or reproduction in other forums is permitted, provided the original author(s) and the copyright owner(s) are credited and that the original publication in this journal is cited, in accordance with accepted academic practice. No use, distribution or reproduction is permitted which does not comply with these terms.
*Correspondence: Deny Menghini, ZGVueS5tZW5naGluaUBvcGJnLm5ldA==
Disclaimer: All claims expressed in this article are solely those of the authors and do not necessarily represent those of their affiliated organizations, or those of the publisher, the editors and the reviewers. Any product that may be evaluated in this article or claim that may be made by its manufacturer is not guaranteed or endorsed by the publisher.
Research integrity at Frontiers
Learn more about the work of our research integrity team to safeguard the quality of each article we publish.