- 1Department of Psychiatry, Harvard Medical School, Beth Israel Deaconess Medical Center, Boston, MA, United States
- 2Department of Mathematics and Statistics, Boston University, Boston, MA, United States
Introduction
Disturbances in smell emerged at the very beginning of the pandemic as the predominant neurological symptom of COVID-19 (Boscolo-Rizzo et al., 2020; Menni et al., 2020) providing evidence of COVID-19 related neurological abnormalities originating from pathology of the olfactory epithelium. The very first prospective imaging studies (MRI scans 3–4 months after COVID-19 hospitalization in Wuhan) reported significant changes in gray matter volume correlated with loss of smell and memory loss, primarily found in cingulate gyrus, piriform cortex, and hippocampus (Lu et al., 2020). Since then, the available data have substantially expanded and the focus shifted to long-term sequelae in which cognitive and mental functioning are prominently featured in post-COVID and long-COVID conditions [see rev. (Rogers et al., 2020; Xydakis et al., 2021; Batiha et al., 2022; Doty, 2022; Hasegawa et al., 2022; Kay, 2022; Lippi et al., 2023)]. Research in this area is rapidly progressing; the most recent articles are being collected in this Special Issue underlining that investigation of the neuropsychiatric sequelae of olfactory dysfunction related to COVID-19 infection is particularly critical to characterize the pathological effects of COVID-19 on brain function and to develop strategies to improve patient's quality of life and mental wellbeing.
In this paper we call attention to potential benefits these studies may gain from a wider approach, including respiratory related oscillations (RRO) in forebrain structures induced by rhythmic nasal airflow. It may help in two major aspects of this research, concerning the two “ends” of the pathology of the central olfactory processing networks, extending from the olfactory bulb (OB) all the way to cortical networks (Xydakis et al., 2021). These are two points where processing of distinct sensory inputs from the OB significantly overlaps and where investigating RRO mechanisms may help to understand (1) how smell loss is caused by SARS-2-COV infection which does not directly attack olfactory sensory neurons (OSN) (Cooper et al., 2020; Iadecola et al., 2020; Doty, 2022; Las Casas Lima et al., 2022; Rodriguez-Sevilla et al., 2022; Butowt et al., 2023) and (2) how olfactory dysfunction advances to a complex condition of diverse cognitive and emotional disturbances (Putri et al., 2021; Soltani et al., 2021; Vanderlind et al., 2021; Batiha et al., 2022; Kay, 2022; Crook et al., 2023).
Respiratory rhythmic modulation of a wide range of cognitive functions has been reported both in rodents and human, from sensory processing and motor coordination to various memory functions [rev. (Heck et al., 2019)]—i.e. not directly related to gas exchange. In rodents, during exploration associated with sniffing, respiratory rate accelerates to match the frequency of hippocampal (HPC) theta rhythm, an intrinsic brain oscillation. During these episodes, RRO play a key role in synchronizing sensory sampling in OB on one hand and rhythmic fluctuations in excitability of neurons involved in central processing in HPC and piriform cortex, on the other. Outside of sniffing episodes, when respiration is in the delta range, OB RRO synchronize instead with frontal cortical delta oscillations. In rats and mice, these waking delta oscillations include task-related intrinsic oscillations (Fujisawa and Buzsaki, 2011; Dejean et al., 2016; Karalis et al., 2016; Furtunato et al., 2020) and are markedly different from the broad-band thalamo-cortical delta rhythms of deep sleep (Pittman-Polletta et al., 2018), being spectrally narrow-band, cortically generated, hierarchically nested with gamma oscillations (Hunt et al., 2017; Pittman-Polletta et al., 2018), and associated with various cognitive functions (Nacher et al., 2013; Hall et al., 2014; Riecke et al., 2015; Hunt et al., 2017). Since respiration is slower in humans than rodents, whereas the frequencies of brain rhythms are evolutionary wellpreserved (Buzsaki and Draguhn, 2004), RRO in humans exhibit a different form of coupling with forebrain oscillations. It periodically modulates the levels of oscillatory activity in forebrain circuits, including both “slow” delta and theta rhythms as well as “fast” beta and gamma rhythms activity known to be involved in cognitive processes (Zelano et al., 2016). Data demonstrating the potential role of RRO in cognitive processing has accumulated in recent years also from human studies (Zelano et al., 2016; Arshamian et al., 2018; Perl et al., 2019). In humans, behaviors modulated by respiratory phase include eye (Rittweger and Popel, 1998; Rassler and Raabe, 2003) and finger (Ebert et al., 2002; Nassrallah et al., 2013) movements, visual (Li et al., 2012)and auditory (Gallego et al., 1991)reaction times, grip-force (Li and Laskin, 2006), olfactory memory consolidation (Arshamian et al., 2018), aversive associative learning (Waselius et al., 2019), visuospatial cognition (Perl et al., 2019), and visual working memory retrieval (Nakamura et al., 2018).
The strategic use of brain oscillations as a mesoscale mechanistic link between cellular and circuit-level neurophysiology and brain-wide network activity giving rise to cognition and behavior has borne fruit in research on schizophrenia (Siok et al., 2006; Ford et al., 2007; Hajos et al., 2008; Lanre-Amos and Kocsis, 2010; Kocsis, 2012; Driesen et al., 2013; Harvey et al., 2013; Kocsis et al., 2013, 2014; Khlestova et al., 2016; Pittman-Polletta et al., 2018; Parker et al., 2019; Hamilton et al., 2020; Thorn et al., 2022), Parkinson's disease (Brown, 2003; Oswal et al., 2013; Little and Brown, 2014; Li and Zhang, 2015; Johnson et al., 2021), and many other pathological conditions, e.g., epilepsy (Buzsaki et al., 1990; Steriade, 2005; Beenhakker and Huguenard, 2009; Takeuchi and Berenyi, 2020), autism (Ben-Ari, 2015; Casanova et al., 2020; Kayarian et al., 2020; Jia et al., 2021), dyslexia (Hancock et al., 2017; Vidyasagar, 2019), and neurodegeneration (Rossini et al., 2007; Nimmrich et al., 2015). The functions, dynamics, and key features including characteristic frequencies of brain oscillations are similar in humans and rodents, and they have been shown to be not only robust but heritable (van Pelt et al., 2012) and responsive to interventions, making them a valuable tool for translational research.
We believe that RRO may provide mechanistic insight into both ends of COVID-19 related olfactory dysfunction, shedding light on both questions posed above (see Figure 1) and may be important for investigations of olfactory processing in general and its COVID-19 related pathology, in particular. RRO adheres to the principle of hierarchical organization of brain oscillations in which slow rhythms (delta, theta, alpha, etc.) modulate local gamma oscillations to facilitate functional coupling of local and distant networks. Gamma is present in all cortical networks and in the OB (Beshel et al., 2007; Brea et al., 2009), as well. RRO couples with slow rhythms intrinsically generated in cortical networks (Kocsis et al., 2018; Mofleh and Kocsis, 2021a) and modulates cortical gamma (Cavelli et al., 2020; Gonzalez et al., 2023). It was recently shown that RRO-gamma coupling in the piriform cortex acted to select and amplify the best set of neurons for representing the odor sensed during a sniff, and to quieten less relevant neurons (Gonzalez et al., 2023), pointing to the strong involvement of RRO in olfactory processing at every level of organization from the OB to higher structures (Figure 1). Thus, our hypothesis concerning RRO does not suggest a separate channel to COVID-19 pathology, alternative to olfactory disfunction. It may rather suggest that considering RRO may provide a significant contribution to investigations of the neuropsychiatric sequelae of olfactory dysfunction related to COVID-19 infection. This latter is rapidly progressing, extending rigorously designed longitudinal MRI studies (Douaud et al., 2022) to comparing COVID-19 patients with or without olfactory dysfunction (Delgado-Alonso et al., 2022; Yus et al., 2022; Caroli et al., 2023) and alterations in functional connections between parahippocampal gyrus and orbitofrontal cortex or other brain regions associated with sensory processing and cognitive functioning in groups of healthy controls, vs. COVID-19 with vs. without smell loss (Díez-Cirarda et al., 2022; Wingrove et al., 2023). Below, we describe the potential links between RRO and cognitive function and dysfunction, and between olfactory dysfunction and impaired RRO (about which less is known), in greater detail.
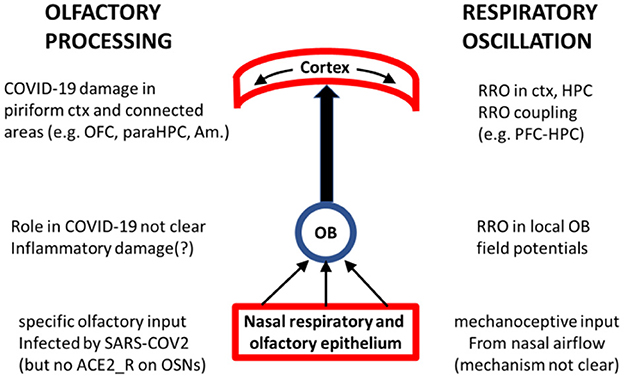
Figure 1. Schematics of key nodes of the olfactory processing system (middle) with their involvement in COVID-19 (left) and in RRO (right). Olfactory and respiratory epithelium plays crucial role in generating both signals and is the primary site of SARS-CoV-2 infection. OB is transmitting ascending olfactory sensation as well as mechanoceptive signal of rhythmic nasal airflow and is receiving “top-down” information related to olfactory processing (from ctx, HPC, Amygdala) as well as oscillatory drive (e.g. theta, gamma from HPC); reports of its possible COVID-19 inflammatory damage are not fully consistent (Sherif et al., 2022; Abdou et al., 2023; Muccioli et al., 2023). Piriform cortex is the primary target of the olfactory tract where cortical processing of olfactory information involves RRO-driven local gamma oscillations (Gonzalez et al., 2023) and is transmitted to other cortical structures, oscillatory coupled at different frequencies [including RRO (Mofleh and Kocsis, 2021a)], for further processing in the context of different cognitive functions. COVID-19 impairment of these cortical structures is well-documented.
Non-olfactory RRO input from OB is strongly involved in cortical processing and cognitive function
Large potential waves in OB and piriform cortex rhythmically occurring at each inspiration have been demonstrated over 80 years ago (Adrian, 1942) and adjustment of the respiratory rate to the frequency of HPC theta rhythm, invariably present during stereotyped sniffing bouts, was reported several decades later (Macrides, 1975; Macrides et al., 1982; Semba and Komisaruk, 1984). These findings initiated highly productive research clarifying the cellular mechanisms involved, how they are adapted to different behaviors and cognitive tasks, and how they are affected by numerous pharmacological compounds [rev. (Klemm, 1976; Kepecs et al., 2006; Kay et al., 2009; Kay, 2014; Tort et al., 2018a; Heck et al., 2019)]. As a result, the vital engagement of HPC in olfactory processing is well established. Theta rhythm generated in HPC controls multiple processes in the olfactory system from the OB (Kepecs et al., 2006; Rojas-Libano et al., 2018; Liu et al., 2020) to the piriform cortex in both rodents (Wilson et al., 2011; Xu and Wilson, 2012; Morrison et al., 2013; Kay, 2014; Trieu et al., 2015; Dupin et al., 2020; Iravani et al., 2021; Sheriff et al., 2021; Poo et al., 2022) and human (Jiang et al., 2017; Iravani et al., 2021; Yang et al., 2022). Theta rhythm from HPC also synchronizes the olfactory system with multiple non-olfactory sensory channels and associated motor control of rhythmic nasal, whisker, and head movements to further optimize odor perception. Thus, theta rhythm synchronized with RRO occupies a central position in a complex system considered a “paradigmatic example” of active sensing (Wachowiak, 2011; Corcoran et al., 2018) aimed at processing synchronized streams of olfactory and other (e.g. tactile, visual, etc.) information.
More recently, an explosion of findings firmly demonstrated that brain activity and cognitive function are also modulated by respiratory rhythm outside of sniffing episodes, as well [rev. (Tort et al., 2018a; Heck et al., 2019)]. Slow, non-theta RRO were detected in numerous brain structures, including higher order cognitive centers as the prefrontal cortex (Biskamp et al., 2017; Zhong et al., 2017) and HPC (Yanovsky et al., 2014; Chi et al., 2016; Lockmann et al., 2016). RRO coupling with wide-spread forebrain activity was confirmed using advanced techniques, including single unit firing (Rojas-Libano and Kay, 2008; Chi et al., 2016; Biskamp et al., 2017; Zhong et al., 2017; Koszeghy et al., 2018; Jung et al., 2022, 2023), current source density (Rojas-Libano and Kay, 2008; Chi et al., 2016; Lockmann et al., 2016), and phase modulation of local gamma activity (Ito et al., 2014; Biskamp et al., 2017; Zhong et al., 2017; Rojas-Libano et al., 2018; Cavelli et al., 2020). It was firmly established that RRO derives from rhythmic nasal airflow in the OB (Yanovsky et al., 2014), which dynamically couples with intrinsic network oscillations in higher brain structures (Kocsis et al., 2018) either: (1) by coherence, when the frequency of RRO matches that of local field potentials such as delta and theta activity in rodents (Ito et al., 2014; Yanovsky et al., 2014; Chi et al., 2016; Lockmann et al., 2016; Biskamp et al., 2017; Tort et al., 2018b), or (2) by phase-amplitude modulation when the frequencies diverge, as in gamma high frequency oscillations (HFO; >100 Hz) in rodents (Ito et al., 2014; Yanovsky et al., 2014; Biskamp et al., 2017; Zhong et al., 2017) or all characteristic EEG rhythms in human (which have frequencies comparable to those in rodents, but faster than human respiration) (Zelano et al., 2016).
Importantly, the effect of RRO driven by mechanoceptive input from the OB goes well beyond rhythmic modulation of the level of activity in higher brain structures; it is deeply involved in complex circuit mechanisms of neural network function. This is an area of intense on-going investigations on different levels of network organization, from cellular to interregional communication (Rojas-Libano and Kay, 2008; Ito et al., 2014; Chi et al., 2016; Lockmann et al., 2016; Biskamp et al., 2017; Zhong et al., 2017; Koszeghy et al., 2018; Rojas-Libano et al., 2018; Cavelli et al., 2020; Mofleh and Kocsis, 2021a; Jung et al., 2022, 2023; Gonzalez et al., 2023). Although OB projection to different higher brain regions is not direct (Hoover and Vertes, 2007; Mori et al., 2013; Yanovsky et al., 2014; Moberly et al., 2018), mostly mediated by the piriform cortex, RRO appears in functionally different areas dynamically coupled in a complex behavior- and task-related manner. As RRO depends on vigilance state (Girin et al., 2020; Mofleh and Kocsis, 2021a), it appears coincident with various state-dependent intrinsic brain oscillations which exhibit characteristic spatial distributions. For example, transient time windows of long-range cortico-cortical coupling of gamma activity (a phenomenon implicated in visual perception, attention, and bottom-up information transfer) are regularly evoked at a specific time during each breathing cycle, as high frequency oscillations e.g. in the frontal cortex are phase-coupled with OB and consequently with piriform cortex (González et al., 2023). Frontal cortex and HPC, typically generating delta and theta oscillations, respectively, are accessible for rhythmic OB input depending on the behavior-dependent respiratory rate, and this has strong implications for their communication. We have shown recently that in resting states, slow (~2 Hz) respiration firmly couples with frontal cortex providing a delta communication channel toward HPC with weaker and variable RRO (Mofleh and Kocsis, 2021a,b)—i.e. in contrast with the well known dominant theta-driven communication controlled by HPC during exploration. In association areas, e.g. in parietal cortex (recorded far caudal from primary olfactory areas) where RRO and intrinsic brain oscillations are driven by converging extrinsic inputs transmitted from different sources, the two rhythms may simultaneously activate partially overlapping cellular populations at different strengths depending on vigilant states, even though the laminar profiles of theta and RRO diploes (a different level of organization) are in different layers (Jung et al., 2022, 2023).
COVID-19 mechanisms in the olfactory epithelium, affecting smell and possibly RRO
Potential pathomechanisms of COVID-19 related olfactory dysfunction have been extensively studied and regularly reviewed in the past several years. At the very beginning (Summer of 2020) for example, Cooper et al. (2020) pointed out, that the natural history of COVID-19-associated anosmia argues that SARS-CoV-2 attacks the olfactory system through mechanisms distinct from those used by the more benign endemic coronaviruses (Giacomelli et al., 2020; Spinato et al., 2020). In fact, imaging studies of the olfactory bulb in COVID-19 patients were either normal or revealed focal inflammation (Eliezer et al., 2020). According to current understanding, SARS-CoV-2 does not directly infect OSNs; COVID-19 induced OSN dysfunction is mediated instead by alterations to the microenvironment maintained by angiotensin converting enzyme-2 (ACE2) receptor-expressing cells in the olfactory epithelium [rev. (Cooper et al., 2020; Iadecola et al., 2020; Las Casas Lima et al., 2022; Rodriguez-Sevilla et al., 2022; Butowt et al., 2023)]. It is believed that the primary target of SARS-CoV-2 infection in the olfactory mucosa are sustentacular cells, known to express ACE2 receptors (Bilinska and Butowt, 2020; Bilinska et al., 2020; Brann et al., 2020; Fodoulian et al., 2020; Klingenstein et al., 2020; Ye et al., 2021; Shahbaz et al., 2022). Moreover, the virus was demonstrated directly in these cells while still replicating in COVID-19 patients who died a few days after infection (Khan et al., 2021).
Rodent sustentacular cells have been ascribed myriad roles collectively referred to as “supporting”: absorptive, detoxifying, metabolic, nourishing, phagocytic, physical, secretory, structural (Getchell et al., 1989; Hansel et al., 2001; Kam et al., 2014; Liang, 2020; Butowt and von Bartheld, 2021; Khan et al., 2021). The tight anatomical and functional links between OSNs and sustentacular cells is a strong indication that impairment of the latter would affect the function of the former. Through changes in extracellular ionic concentrations, nutrient bioavailability, or structural support, alterations in the microenvironment of OSNs could easily cause disruptions in both the perception of odorants and the synchronization of brain-wide electrical activity. The cellular mechanisms of RRO generation in the OB are not yet clear at this level of detail, and we are not aware of published research on whether impaired RRO are associated with COVID-19 pathology. But it seems plausible that impaired function of non-sensory olfactory epithelial cells may negatively affect RRO. Indeed, the potential for metabolic factors to instigate changes in brain rhythms has been demonstrated, e.g., in recent biophysical models of burst suppression under propofol anesthesia (Ching et al., 2012) and the sleep-stage architecture of thalamocortical spindles (Roberts, 2007).
The causal relationship between OSN dysfunction and potential dysrhythmias is not clear a priori. Disruption of OSN function might directly lead to impaired RRO, as OSNs can respond not only to odorants but also to mechanical stimuli (Connelly et al., 2015; Grosmaitre et al., 2021) and transmit both odor and air flow-driven mechanical signals (Carey et al., 2009; Iwata et al., 2017). Such mechanosensory activity has been extensively studied as a mechanism in sniffing-related synchronization of brain activity. However, its role in the generation of lower (i.e., delta) frequency RRO targeting a wider range of forebrain regions remains unidentified. In the opposite direction, mechano-sensation of rhythmic airflow and its deficits (i.e., the disorganization of RRO) may directly affect odor perception already at the level of OB. Odor encoding occurs relative to the phase of respiration (Kepecs et al., 2006; Cury and Uchida, 2010), i.e. during inhalation, and its frequency determines many aspects of OB activity (David et al., 2015; Short et al., 2016). Olfactory external tufted cells exhibit rhythmic bursting activity in several frequency ranges synchronized within olfactory glomeruli (Hayar et al., 2004; De Saint Jan et al., 2009) by multiple mechanisms including gap junction connectivity, slow (dendritic) excitatory currents, and slow recurrent inhibition from periglomerular cells (Hayar et al., 2005), and most likely mediate the phase-locking of OB output to respiration (Buonviso et al., 2003). Thus, impaired RRO may directly contribute to COVID-19 associated olfactory deficits.
Discussion
In this opinion paper we advocated for the investigation of potentially impaired non-olfactory inputs arising from the olfactory epithelium and involved in cognitive function (e.g., RRO) as a potential mechanistic factor underlying the neuropsychiatric consequences of SARS-CoV-2 infection and linking them to COVID-19 related olfactory dysfunction. We should mention however, that long-Covid is a new and very complex condition, which includes many mechanisms—viral, inflammation, signaling pathways and, of course, non-homogeneous, depending on the acute phase, virus & patients particularities. RRO is one potential component in this puzzle that should be considered.
Additionally, given the well established links between olfactory function and mental health, RRO are likely to play a significant role in other medical conditions (MacKay et al., 2018) as well, when these oscillations may be disrupted for different reasons, or when this extrinsic synchronizing input remain functional while intrinsic brain oscillations are disturbed. An obvious example of the first, besides impairments of the olfactory epithelium, is the condition of long-term intubation, necessary in the context of a variety of medical indication and treatment. Its potential consequences on cognition are hard to distinguish from those directly related to the basic pathology. However, promoting RRO in sensory and motor cortex through the activation of oro-facial and neck muscle activity in synchrony with respiration (Wachowiak, 2011; Corcoran et al., 2018) may have therapeutic benefits for both weaning procedures prior to extubation, and subsequent rehab. As for the second possibility, abnormal brain oscillations, “oscillopathies”, are commonly found in a wide variety of psychiatric diseases associated with severe cognitive deficits (see e.g. Katsuki et al., 2022; Shu et al., 2022; Sohal, 2022; Syed et al., 2022; Beste et al., 2023; Ramos et al., 2023; Wischnewski et al., 2023 for recent reviews). Rhythmic nasal airflow continues uninterrupted, but the potential alterations to the functionality of cortical RRO remain unclear. We have shown recently for example that normal RRO patterns (Mofleh and Kocsis, 2021a,b) remain functional after severe disruption of intrinsic cortical and HPC oscillations under the psychotomimetic Ketamine, in a state characterized by “psychotic-like” behavior and abnormal cortical gamma activity, even with a highly unstable respiratory rate (Staszelis et al., 2022). Whether and to what extent this source of extrinsic oscillatory drive provides a mechanism for interregional long-range oscillatory coupling between cortical networks requires further investigations in specific disorders.
Author contributions
All authors listed have made a substantial, direct, and intellectual contribution to the work and approved it for publication.
Funding
This work was supported by the National Institute of Health (MH127483 and MH125242).
Conflict of interest
The authors declare that the research was conducted in the absence of any commercial or financial relationships that could be construed as a potential conflict of interest.
Publisher's note
All claims expressed in this article are solely those of the authors and do not necessarily represent those of their affiliated organizations, or those of the publisher, the editors and the reviewers. Any product that may be evaluated in this article, or claim that may be made by its manufacturer, is not guaranteed or endorsed by the publisher.
References
Abdou, E. H. E., Ebada, H. A., Salem, M. A., Ghoneim, M. M. R., Sherif, F., and Kamal, E. (2023). Clinical and imaging evaluation of COVID-19-related olfactory dysfunction. Am. J. Rhinol. Allergy 1945, 8924231163969. doi: 10.1177/19458924231163969
Adrian, E. D. (1942). Olfactory reactions in the brain of the hedgehog. J. Physiol. 100, 459–473. doi: 10.1113/jphysiol.1942.sp003955
Arshamian, A., Iravani, B., Majid, A., and Lundstrom, J. N. (2018). Respiration modulates olfactory memory consolidation in humans. J. Neurosci. 38, 10286–10294. doi: 10.1523/JNEUROSCI.3360-17.2018
Batiha, G. E., Al-Kuraishy, H. M., Al-Gareeb, A. I., and Welson, N. N. (2022). Pathophysiology of Post-COVID syndromes: a new perspective. Virol. J. 19, 158. doi: 10.1186/s12985-022-01891-2
Beenhakker, M. P., and Huguenard, J. R. (2009). Neurons that fire together also conspire together: is normal sleep circuitry hijacked to generate epilepsy? Neuron 62, 612–632. doi: 10.1016/j.neuron.2009.05.015
Ben-Ari, Y. (2015). Is birth a critical period in the pathogenesis of autism spectrum disorders? Nat. Rev. Neurosci. 16, 498–505. doi: 10.1038/nrn3956
Beshel, J., Kopell, N., and Kay, L. M. (2007). Olfactory bulb gamma oscillations are enhanced with task demands. J. Neurosci. 27, 8358–8365. doi: 10.1523/JNEUROSCI.1199-07.2007
Beste, C., Munchau, A., and Frings, C. (2023). Towards a systematization of brain oscillatory activity in actions. Commun. Biol. 6, 137. doi: 10.1038/s42003-023-04531-9
Bilinska, K., and Butowt, R. (2020). Anosmia in COVID-19: A bumpy road to establishing a cellular mechanism. ACS Chem. Neurosci. 11, 2152–2155. doi: 10.1021/acschemneuro.0c00406
Bilinska, K., Jakubowska, P., Von Bartheld, C. S., and Butowt, R. (2020). Expression of the SARS-CoV-2 Entry Proteins, ACE2 and TMPRSS2, in cells of the olfactory epithelium: identification of cell types and trends with age. ACS Chem. Neurosci. 11, 1555–1562. doi: 10.1021/acschemneuro.0c00210
Biskamp, J., Bartos, M., and Sauer, J. F. (2017). Organization of prefrontal network activity by respiration-related oscillations. Sci. Rep. 7, 45508. doi: 10.1038/srep45508
Boscolo-Rizzo, P., Borsetto, D., Fabbris, C., Spinato, G., Frezza, D., Menegaldo, A., et al. (2020). Evolution of altered sense of smell or taste in patients with mildly symptomatic COVID-19. JAMA Otolaryngol. Head Neck Surg. 20, 253 doi: 10.1001/jamaoto.2020.1379
Brann, D. H., Tsukahara, T., Weinreb, C., Lipovsek, M., Van den Berge, K., Gong, B., et al. (2020). Non-neuronal expression of SARS-CoV-2 entry genes in the olfactory system suggests mechanisms underlying COVID-19-associated anosmia. Sci. Adv. 6, 202. doi: 10.1126/sciadv.abc5801
Brea, J. N., Kay, L. M., and Kopell, N. J. (2009). Biophysical model for gamma rhythms in the olfactory bulb via subthreshold oscillations. Proc. Natl. Acad. Sci. U S A 106, 21954–21959. doi: 10.1073/pnas.0910964106
Brown, P. (2003). Oscillatory nature of human basal ganglia activity: relationship to the pathophysiology of Parkinson's disease. Mov. Disord. 18, 357–363. doi: 10.1002/mds.10358
Buonviso, N., Amat, C., Litaudon, P., Roux, S., Royet, J.-P., Farget, V., et al. (2003). Rhythm sequence through the olfactory bulb layers during the time window of a respiratory cycle. Eur. J. Neurosci. 17, 1811–1819. doi: 10.1046/j.1460-9568.2003.02619.x
Butowt, R., Bilinska, K., and von Bartheld, C. S. (2023). Olfactory dysfunction in COVID-19: new insights into the underlying mechanisms. Trends. Neurosci. 46, 75–90. doi: 10.1016/j.tins.2022.11.003
Butowt, R., and von Bartheld, C. S. (2021). Anosmia in COVID-19: underlying mechanisms and assessment of an olfactory route to brain infection. Neuroscientist 27, 582–603. doi: 10.1177/1073858420956905
Buzsaki, G., and Draguhn, A. (2004). Neuronal oscillations in cortical networks. Science 304, 1926–1929. doi: 10.1126/science.1099745
Buzsaki, G., Smith, A., Berger, S., Fisher, L. J., and Gage, F. H. (1990). Petit mal epilepsy and parkinsonian tremor: hypothesis of a common pacemaker. Neuroscience 36, 1–14. doi: 10.1016/0306-4522(90)90345-5
Carey, R. M., Verhagen, J. V., Wesson, D. W., Pirez, N., and Wachowiak, M. (2009). Temporal structure of receptor neuron input to the olfactory bulb imaged in behaving rats. J. Neurophysiol. 101, 1073–1088. doi: 10.1152/jn.90902.2008
Caroli, A., Capelli, S., Napolitano, A., Cabrini, G., Arrigoni, A., Pezzetti, G., et al. (2023). Brain diffusion alterations in patients with COVID-19 pathology and neurological manifestations. Neuroimage Clin. 37, 103338. doi: 10.1016/j.nicl.2023.103338
Casanova, M. F., Sokhadze, E. M., Casanova, E. L., Opris, I., Abujadi, C., Marcolin, M. A., et al. (2020). Translational neuroscience in autism: from neuropathology to transcranial magnetic stimulation therapies. Psychiatr. Clin. North Am. 43, 229–248. doi: 10.1016/j.psc.2020.02.004
Cavelli, M., Castro-Zaballa, S., Gonzalez, J., Rojas-Líbano, D., Rubido, N., Velásquez, N., et al. (2020). Nasal respiration entrains neocortical long-range gamma coherence during wakefulness. Eur. J. Neurosci. 51, 1463–1477. doi: 10.1111/ejn.14560
Chi, V. N., Müller, C., Wolfenstetter, T., Yanovsky, Y., Draguhn, A., Tort, A. B. L., et al. (2016). Hippocampal respiration-driven rhythm distinct from theta oscillations in awake mice. J. Neurosci. 36, 162–177. doi: 10.1523/JNEUROSCI.2848-15.2016
Ching, S., Purdon, P. L., Vijayan, S., Kopell, N. J., and Brown, E. N. (2012). A neurophysiological-metabolic model for burst suppression. Proc. Natl. Acad. Sci. U S A 109, 3095–3100. doi: 10.1073/pnas.1121461109
Connelly, T., Yu, Y., Grosmaitre, X., Wang, J., Santarelli, L. C., Savigner, A., et al. (2015). G protein-coupled odorant receptors underlie mechanosensitivity in mammalian olfactory sensory neurons. Proc. Natl. Acad. Sci. U S A 112, 590–595. doi: 10.1073/pnas.1418515112
Cooper, K. W., Brann, D. H., Farruggia, M. C., Bhutani, S., Pellegrino, R., Tsukahara, T., et al. (2020). COVID-19 and the chemical senses: supporting players take center stage. Neuron 107, 219–233. doi: 10.1016/j.neuron.2020.06.032
Corcoran, A. W., Pezzulo, G., and Hohwy, J. (2018). Commentary: respiration-entrained brain rhythms are global but often overlooked. Front. Syst. Neurosci. 12, 25. doi: 10.3389/fnsys.2018.00025
Crook, H., Ramirez, A., Hosseini, A., Vavougyios, G., Lehmann, C., Bruchfeld, J., et al. (2023). European Working Group on SARS-CoV-2: Current Understanding, Unknowns, and Recommendations on the Neurological Complications of COVID-19. Brain Connect. 23, 252. doi: 10.1089/brain.2022.0058
Cury, K. M., and Uchida, N. (2010). Robust odor coding via inhalation-coupled transient activity in the mammalian olfactory bulb. Neuron 68, 570–585. doi: 10.1016/j.neuron.2010.09.040
David, F., Courtiol, E., Buonviso, N., and Fourcaud-Trocme, N. (2015). Competing Mechanisms of Gamma and Beta Oscillations in the Olfactory Bulb Based on Multimodal Inhibition of Mitral Cells Over a Respiratory Cycle. eNeuro 2, 215. doi: 10.1523/ENEURO.0018-15.2015
De Saint Jan, D., Hirnet, D., Westbrook, G. L., and Charpak, S. (2009). External tufted cells drive the output of olfactory bulb glomeruli. J. Neurosci. 29, 2043–2052. doi: 10.1523/JNEUROSCI.5317-08.2009
Dejean, C., Courtin, J., Karalis, N., Chaudun, F., Wurtz, H., Bienvenu, T. C. M., et al. (2016). Prefrontal neuronal assemblies temporally control fear behaviour. Nature 535, 420–424. doi: 10.1038/nature18630
Delgado-Alonso, C., Valles-Salgado, M., Delgado-Álvarez, A., Yus, M., Gómez-Ruiz, N., Jorquera, M., et al. (2022). Cognitive dysfunction associated with COVID-19: A comprehensive neuropsychological study. J. Psychiatr. Res. 150, 40–46. doi: 10.1016/j.jpsychires.2022.03.033
Díez-Cirarda, M., Yus, M., Gómez-Ruiz, N., Polidura, C., Gil-Martínez, L., Delgado-Alonso, C., et al. (2022). Multimodal neuroimaging in post-COVID syndrome and correlation with cognition. Brain. 22:525. doi: 10.1093/brain/awac384
Doty, R. L. (2022). Olfactory dysfunction in COVID-19: pathology and long-term implications for brain health. Trends Mol. Med. 28, 781–794. doi: 10.1016/j.molmed.2022.06.005
Douaud, G., Lee, S., Alfaro-Almagro, F., Arthofer, C., Wang, C., McCarthy, P., et al. (2022). SARS-CoV-2 is associated with changes in brain structure in UK Biobank. Nature 604, 697–707. doi: 10.1038/s41586-022-04569-5
Driesen, N. R., McCarthy, G., Bhagwagar, Z., Bloch, M., Calhoun, V., D'Souza, D. C., et al. (2013). Relationship of resting brain hyperconnectivity and schizophrenia-like symptoms produced by the NMDA receptor antagonist ketamine in humans. Mol. Psychiatr. 18, 1199–1204. doi: 10.1038/mp.2012.194
Dupin, M., Garcia, S., Messaoudi, B., Doyere, V., and Mouly, A. M. (2020). Respiration and brain neural dynamics associated with interval timing during odor fear learning in rats. Sci. Rep. 10, 17643. doi: 10.1038/s41598-020-74741-2
Ebert, D., Hefter, H., Binkofski, F., and Freund, H. J. (2002). Coordination between breathing and mental grouping of pianistic finger movements. Percept. Mot. Skills 95, 339–353. doi: 10.2466/pms.2002.95.2.339
Eliezer, M., Hautefort, C., Hamel, A.-L., Verillaud, B., Herman, P., Houdart, E., et al. (2020). Sudden and complete olfactory loss of function as a possible symptom of COVID-19. JAMA Otolaryngol. Head Neck. Surg. 146, 674–675. doi: 10.1001/jamaoto.2020.0832
Fodoulian, L., Tuberosa, J., Rossier, D., Boillat, M., Kan, C., Pauli, V., et al. (2020). SARS-CoV-2 receptors and entry genes are expressed in the human olfactory neuroepithelium and brain. iScience 23, 101839. doi: 10.1016/j.isci.2020.101839
Ford, J. M., Krystal, J. H., and Mathalon, D. H. (2007). Neural synchrony in schizophrenia: from networks to new treatments. Schizophr. Bull. 33, 848–852. doi: 10.1093/schbul/sbm062
Fujisawa, S., and Buzsaki, G. (2011). A 4 Hz oscillation adaptively synchronizes prefrontal, VTA, and hippocampal activities. Neuron 72, 153–165. doi: 10.1016/j.neuron.2011.08.018
Furtunato, A. M. B., Lobao-Soares, B., Tort, A. B. L., and Belchior, H. (2020). Specific increase of hippocampal delta oscillations across consecutive treadmill runs. Front. Behav. Neurosci. 14, 101. doi: 10.3389/fnbeh.2020.00101
Gallego, J., Perruchet, P., and Camus, J. F. (1991). Assessing attentional control of breathing by reaction time. Psychophysiology 28, 217–224. doi: 10.1111/j.1469-8986.1991.tb00413.x
Getchell, M. L., Bouvet, J. F., Finger, T. E., Holley, A., and Getchell, T. V. (1989). Peptidergic regulation of secretory activity in amphibian olfactory mucosa: immunohistochemistry, neural stimulation, and pharmacology. Cell Tissue Res. 256, 381–389. doi: 10.1007/BF00218896
Giacomelli, A., Pezzati, L., Conti, F., Bernacchia, D., Siano, M., Oreni, L., et al. (2020). Self-reported olfactory and taste disorders in patients with severe acute respiratory coronavirus 2 infection: a cross-sectional study. Clin. Infect. Dis. 71, 889–890. doi: 10.1093/cid/ciaa330
Girin, B., Juventin, M., Garcia, S., Lefèvre, L., Amat, C., Fourcaud-Trocm,é, N., et al. (2020). The deep and slow breathing characterizing rest favors brain respiratory-drive. BioRxiv. doi: 10.1101/2020.07.30.226563
González, J., Cavelli, M., Mondino, A., Castro-Zaballa, S., Brankačk, J., Draguhn, A., et al. (2023). Breathing modulates gamma synchronization across species. Pflugers Arch. 475, 49–63. doi: 10.1007/s00424-022-02753-0
Gonzalez, J., Torterolo, P., and Tort, A. B. L. (2023). Mechanisms and functions of respiration-driven gamma oscillations in the primary olfactory cortex. Elife 12, 82. doi: 10.7554/eLife.83044.sa2
Grosmaitre, X., Santarelli, L. C., Tan, J., Luo, M., and Ma, M. (2021). Dual functions of mammalian olfactory sensory neurons as odor detectors and mechanical sensors. Nat. Neurosci. 10, 348–354. doi: 10.1038/nn1856
Hajos, M., Hoffmann, W. E., and Kocsis, B. (2008). Activation of cannabinoid-1 receptors disrupts sensory gating and neuronal oscillation: relevance to schizophrenia. Biol. Psychiatr. 63, 1075–1083. doi: 10.1016/j.biopsych.2007.12.005
Hall, T. M., de Carvalho, F., and Jackson, A. (2014). A common structure underlies low-frequency cortical dynamics in movement, sleep, and sedation. Neuron 83, 1185–1199. doi: 10.1016/j.neuron.2014.07.022
Hamilton, H. K., Roach, B. J., Cavus, I., Teyler, T. J., Clapp, W. C., Ford, J. M., et al. (2020). Impaired potentiation of theta oscillations during a visual cortical plasticity paradigm in individuals with schizophrenia. Front. Psychiatr. 11, 590567. doi: 10.3389/fpsyt.2020.590567
Hancock, R., Pugh, K. R., and Hoeft, F. (2017). Neural noise hypothesis of developmental dyslexia. Trends Cogn. Sci. 21, 434–448. doi: 10.1016/j.tics.2017.03.008
Hansel, D. E., Eipper, B. A., and Ronnett, G. V. (2001). Neuropeptide Y functions as a neuroproliferative factor. Nature 410, 940–944. doi: 10.1038/35073601
Harvey, B. D., Siok, C. J., Kiss, T., Volfson, D., Grimwood, S., Shaffer, C. L., et al. (2013). Neurophysiological signals as potential translatable biomarkers for modulation of metabotropic glutamate 5 receptors. Neuropharmacology 75, 19–30. doi: 10.1016/j.neuropharm.2013.06.020
Hasegawa, Y., Ma, M., Sawa, A., Lane, A. P., and Kamiya, A. (2022). Olfactory impairment in psychiatric disorders: does nasal inflammation impact disease psychophysiology? Transl. Psychiatr. 12, 314. doi: 10.1038/s41398-022-02081-y
Hayar, A., Karnup, S., Shipley, M. T., and Ennis, M. (2004). Olfactory bulb glomeruli: external tufted cells intrinsically burst at theta frequency and are entrained by patterned olfactory input. J. Neurosci. 24, 1190–1199. doi: 10.1523/JNEUROSCI.4714-03.2004
Hayar, A., Shipley, M. T., and Ennis, M. (2005). Olfactory bulb external tufted cells are synchronized by multiple intraglomerular mechanisms. J. Neurosci. 25, 8197–8208. doi: 10.1523/JNEUROSCI.2374-05.2005
Heck, D. H., Kozma, R., and Kay, L. M. (2019). The rhythm of memory: how breathing shapes memory function. J. Neurophysiol. 122, 563–571. doi: 10.1152/jn.00200.2019
Hoover, W. B., and Vertes, R. P. (2007). Anatomical analysis of afferent projections to the medial prefrontal cortex in the rat. Brain. Struct. Funct. 212, 149–179. doi: 10.1007/s00429-007-0150-4
Hunt, M. J., Kopell, N. J., Traub, R. D., and Whittington, M. A. (2017). Aberrant network activity in schizophrenia. Trends Neurosci. 40, 371–382. doi: 10.1016/j.tins.2017.04.003
Iadecola, C., Anrather, J., and Kamel, H. (2020). Effects of COVID-19 on the nervous system. Cell 183, 16–27. doi: 10.1016/j.cell.2020.08.028
Iravani, B., Arshamian, A., Lundqvist, M., Kay, L. M., Wilson, D. A., and Lundström, J. N. (2021). Odor identity can be extracted from the reciprocal connectivity between olfactory bulb and piriform cortex in humans. Neuroimage 237, 118130. doi: 10.1016/j.neuroimage.2021.118130
Ito, J., Roy, S., Liu, Y., Cao, Y., Fletcher, M., Lu, L., et al. (2014). Whisker barrel cortex delta oscillations and gamma power in the awake mouse are linked to respiration. Nat. Commun. 5, 3572. doi: 10.1038/ncomms4572
Iwata, R., Kiyonari, H., and Imai, T. (2017). Mechanosensory-based phase coding of odor identity in the olfactory bulb. Neuron 96, 1139–1152. doi: 10.1016/j.neuron.2017.11.008
Jia, H., Gao, F., and Yu, D. (2021). Altered Temporal Structure of Neural Phase Synchrony in Patients With Autism Spectrum Disorder. Front. Psychiatry 12, 618573. doi: 10.3389/fpsyt.2021.618573
Jiang, H., Schuele, S., Rosenow, J., Zelano, C., Parvizi, J., Tao, J. X., et al. (2017). theta oscillations rapidly convey odor-specific content in human piriform cortex. Neuron 94, 207-219 e204. doi: 10.1016/j.neuron.2017.03.021
Johnson, L. A., Aman, J. E., Yu, Y., Sanabria, D. E., Wang, J., Hill, M., et al. (2021). High-frequency oscillations in the pallidum: a pathophysiological biomarker in parkinson's disease? Mov. Disord. 36, 1332–1341. doi: 10.1002/mds.28566
Jung, F., Witte, V., Yanovsky, Y., Klumpp, M., Brankačk, J., Tort, A. B. L., et al. (2022). Differential modulation of parietal cortex activity by respiration and theta oscillations. J. Neurophysiol. 127, 801–817. doi: 10.1152/jn.00376.2021
Jung, F., Yanovsky, Y., Brankack, J., Tort, A. B. L., and Draguhn, A. (2023). Respiratory entrainment of units in the mouse parietal cortex depends on vigilance state. Pflugers Arch. 475, 65–76. doi: 10.1007/s00424-022-02727-2
Kam, J. W., Raja, R., and Cloutier, J. F. (2014). Cellular and molecular mechanisms regulating embryonic neurogenesis in the rodent olfactory epithelium. Int. J. Dev. Neurosci. 37, 76–86. doi: 10.1016/j.ijdevneu.2014.06.017
Karalis, N., Dejean, C., Chaudun, F., Khoder, S., Rozeske, R. R., Wurtz, H., et al. (2016). 4-Hz oscillations synchronize prefrontal-amygdala circuits during fear behavior. Nat. Neurosci. 19, 605–612. doi: 10.1038/nn.4251
Katsuki, F., Gerashchenko, D., and Brown, R. E. (2022). Alterations of sleep oscillations in Alzheimer's disease: a potential role for GABAergic neurons in the cortex, hippocampus, and thalamus. Brain Res. Bull. 187, 181–198. doi: 10.1016/j.brainresbull.2022.07.002
Kay, L. M. (2014). Circuit oscillations in odor perception and memory. Prog. Brain Res. 208, 223–251. doi: 10.1016/B978-0-444-63350-7.00009-7
Kay, L. M. (2022). COVID-19 and olfactory dysfunction: a looming wave of dementia? J. Neurophysiol. 128, 436–444. doi: 10.1152/jn.00255.2022
Kay, L. M., Beshel, J., Brea, J., Martin, C., Rojas-Líbano, D., and Kopell, N. (2009). Olfactory oscillations: the what, how and what for. Trends Neurosci. 32, 207–214. doi: 10.1016/j.tins.2008.11.008
Kayarian, F. B., Jannati, A., Rotenberg, A., and Santarnecchi, E. (2020). Targeting gamma-related pathophysiology in autism spectrum disorder using transcranial electrical stimulation: opportunities and challenges. Autism Res. 13, 1051–1071. doi: 10.1002/aur.2312
Kepecs, A., Uchida, N., and Mainen, Z. F. (2006). The sniff as a unit of olfactory processing. Chem. Senses 31, 167–179. doi: 10.1093/chemse/bjj016
Khan, M., Yoo, S.-J., Clijsters, M., Backaert, W., Vanstapel, A., Speleman, K., et al. (2021). Visualizing in deceased COVID-19 patients how SARS-CoV-2 attacks the respiratory and olfactory mucosae but spares the olfactory bulb. Cell 184, 5932–5949 e5915. doi: 10.1016/j.cell.2021.10.027
Khlestova, E., Johnson, J. W., Krystal, J. H., and Lisman, J. (2016). The Role of GluN2C-Containing NMDA receptors in ketamine's psychotogenic action and in schizophrenia models. J. Neurosci. 36, 11151–11157. doi: 10.1523/JNEUROSCI.1203-16.2016
Klemm, W. R. (1976). Hippocampal EEG and information processing: a special role for theta rhythm. Prog. Neurobiol. 7, 197–214. doi: 10.1016/0301-0082(76)90007-1
Klingenstein, M., Klingenstein, S., Neckel, P. H., Mack, A. F., Wagner, A. P., Kleger, A., et al. (2020). Evidence of SARS-CoV2 Entry Protein ACE2 in the human nose and olfactory bulb. Cells Tissues Organs 209, 155–164. doi: 10.1159/000513040
Kocsis, B. (2012). Differential role of NR2A and NR2B subunits in N-methyl-D-aspartate receptor antagonist-induced aberrant cortical gamma oscillations. Biol. Psychiatr. 71, 987–995. doi: 10.1016/j.biopsych.2011.10.002
Kocsis, B., Brown, R. E., McCarley, R. W., and Hajos, M. (2013). Impact of ketamine on neuronal network dynamics: translational modeling of schizophrenia-relevant deficits. CNS Neurosci. Ther. 19, 437–447. doi: 10.1111/cns.12081
Kocsis, B., Lee, P., and Deth, R. (2014). Enhancement of gamma activity after selective activation of dopamine D4 receptors in freely moving rats and in a neurodevelopmental model of schizophrenia. Brain Struct. Funct. 219, 2173–2180. doi: 10.1007/s00429-013-0607-6
Kocsis, B., Pittman-Polletta, B. R., and Roy, A. (2018). Respiration-coupled rhythms in prefrontal cortex: beyond if, to when, how, and why. Brain Struct. Funct. 223, 11–16. doi: 10.1007/s00429-017-1587-8
Koszeghy, A., Lasztoczi, B., Forro, T., and Klausberger, T. (2018). Spike-timing of orbitofrontal neurons is synchronized with breathing. Front. Cell Neurosci. 12, 105. doi: 10.3389/fncel.2018.00105
Lanre-Amos, T., and Kocsis, B. (2010). Hippocampal oscillations in the rodent model of schizophrenia induced by amygdala GABA receptor blockade. Front Psychiatry 1, 132. doi: 10.3389/fpsyt.2010.00132
Las Casas Lima, M. H., Cavalcante, A. L. B., and Leao, S. C. (2022). Pathophysiological relationship between COVID-19 and olfactory dysfunction: a systematic review. Braz. J. Otorhinolaryngol. 88, 794–802. doi: 10.1016/j.bjorl.2021.04.001
Li, M., and Zhang, W. (2015). Oscillations in pedunculopontine nucleus in Parkinson's disease and its relationship with deep brain stimulation. Front. Neural Circuits. 9, 47. doi: 10.3389/fncir.2015.00047
Li, S., and Laskin, J. J. (2006). Influences of ventilation on maximal isometric force of the finger flexors. Muscle Nerve 34, 651–655. doi: 10.1002/mus.20592
Li, S., Park, W. H., and Borg, A. (2012). Phase-dependent respiratory-motor interactions in reaction time tasks during rhythmic voluntary breathing. Motor Contr. 16, 493–505. doi: 10.1123/mcj.16.4.493
Liang, F. (2020). Sustentacular cell enwrapment of olfactory receptor neuronal dendrites: an Update. Genes (Basel) 11, 20. doi: 10.3390/genes11050493
Lippi, G., Sanchis-Gomar, F., and Henry, B. M. (2023). COVID-19 and its long-term sequelae: what do we know in 2023? Pol. Arch. Intern. Med. 16, 462. doi: 10.20452/pamw.16402
Little, S., and Brown, P. (2014). The functional role of beta oscillations in Parkinson's disease. Parkinsonism Relat. Disord. 20, S44–48. doi: 10.1016/S1353-8020(13)70013-0
Liu, P., Cao, T., Xu, J., Mao, X., Wang, D., and Li, A. (2020). Plasticity of sniffing pattern and neural activity in the olfactory bulb of behaving mice during odor sampling, anticipation, and reward. Neurosci. Bull. 36, 598–610. doi: 10.1007/s12264-019-00463-9
Lockmann, A. L., Laplagne, D. A., Leao, R. N., and Tort, A. B. (2016). A Respiration-coupled rhythm in the rat hippocampus independent of theta and slow oscillations. J. Neurosci. 36, 5338–5352. doi: 10.1523/JNEUROSCI.3452-15.2016
Lu, Y., Li, X., Geng, D., Mei, N., Wu, P.-Y., Huang, C.-C., et al. (2020). Cerebral Micro-Structural Changes in COVID-19 Patients—An MRI-based 3-month Follow-up Study. EClinical. Med. 25, 100484. doi: 10.1016/j.eclinm.2020.100484
MacKay, M.-A. B., Paylor, J. W., Wong, J. T. F., Winship, I. R., Baker, G. B., and Dursun, S. M. (2018). Multidimensional connectomics and treatment-resistant schizophrenia: linking phenotypic circuits to targeted therapeutics. Front. Psychiatr. 9, 537. doi: 10.3389/fpsyt.2018.00537
Macrides, F. (1975). Temporal relationships between hippocampal slow waves and exploratory sniffing in hamsters. Behav. Biol. 14, 295–308. doi: 10.1016/S0091-6773(75)90419-8
Macrides, F., Eichenbaum, H. B., and Forbes, W. B. (1982). Temporal relationship between sniffing and the limbic theta rhythm during odor discrimination reversal learning. J. Neurosci. 2, 1705–1717. doi: 10.1523/JNEUROSCI.02-12-01705.1982
Menni, C., Valdes, A. M., Freidin, M. B., Sudre, C. H., Nguyen, L. H., Drew, D. A., et al. (2020). Real-time tracking of self-reported symptoms to predict potential COVID-19. Nat. Med. 26, 1037–1040. doi: 10.1038/s41591-020-0916-2
Moberly, A. H., Schreck, M., Bhattarai, J. P., Zweifel, L. S., Luo, W., and Ma, M. (2018). Olfactory inputs modulate respiration-related rhythmic activity in the prefrontal cortex and freezing behavior. Nat. Commun. 9, 1528. doi: 10.1038/s41467-018-03988-1
Mofleh, R., and Kocsis, B. (2021a). Delta-range coupling between prefrontal cortex and hippocampus supported by respiratory rhythmic input from the olfactory bulb in freely behaving rats. Sci. Rep. 11, 8100. doi: 10.1038/s41598-021-87562-8
Mofleh, R., and Kocsis, B. (2021b). Respiratory coupling between prefrontal cortex and hippocampus of rats anaesthetized with urethane in theta and non-theta states. Eur. J. Neurosci. 54, 5507–5517. doi: 10.1111/ejn.15384
Mori, K., Manabe, H., Narikiyo, K., and Onisawa, N. (2013). Olfactory consciousness and gamma oscillation couplings across the olfactory bulb, olfactory cortex, and orbitofrontal cortex. Front. Psychol. 4, 743. doi: 10.3389/fpsyg.2013.00743
Morrison, G. L., Fontaine, C. J., Harley, C. W., and Yuan, Q. (2013). A role for the anterior piriform cortex in early odor preference learning: evidence for multiple olfactory learning structures in the rat pup. J. Neurophysiol. 110, 141–152. doi: 10.1152/jn.00072.2013
Muccioli, L., Sighinolfi, G., Mitolo, M., Ferri, L., Rochat, M. J., Pensato, U., et al. (2023). Cognitive and functional connectivity impairment in post-COVID-19 olfactory dysfunction. Neuroimage Clin. 38, 103410. doi: 10.1016/j.nicl.2023.103410
Nacher, V., Ledberg, A., Deco, G., and Romo, R. (2013). Coherent delta-band oscillations between cortical areas correlate with decision making. Proc. Natl. Acad. Sci. U S A. 110, 15085–15090. doi: 10.1073/pnas.1314681110
Nakamura, N. H., Fukunaga, M., and Oku, Y. (2018). Respiratory modulation of cognitive performance during the retrieval process. PLoS One 13, e0204021. doi: 10.1371/journal.pone.0204021
Nassrallah, F., Comeau, G., Russell, D., and Cossette, I. (2013). Coordination of breathing and various movement markers during pianists' performance tasks. Percept Mot. Skills 116, 1–20. doi: 10.2466/22.25.26.PMS.116.1.1-20
Nimmrich, V., Draguhn, A., and Axmacher, N. (2015). Neuronal Network oscillations in neurodegenerative diseases. Neuromolecular. Med. 17, 270–284. doi: 10.1007/s12017-015-8355-9
Oswal, A., Brown, P., and Litvak, V. (2013). Synchronized neural oscillations and the pathophysiology of Parkinson's disease. Curr. Opin. Neurol. 26, 662–670. doi: 10.1097/WCO.0000000000000034
Parker, D. A., Hamm, J. P., McDowell, J. E., Keedy, S. K., Gershon, E. S., Ivleva, E. I., et al. (2019). Auditory steady-state EEG response across the schizo-bipolar spectrum. Schizophr. Res. 209, 218–226. doi: 10.1016/j.schres.2019.04.014
Perl, O., Ravia, A., Rubinson, M., Eisen, A., Soroka, T., Mor, N., et al. (2019). Human non-olfactory cognition phase-locked with inhalation. Nat. Hum. Behav. 3, 501–512. doi: 10.1038/s41562-019-0556-z
Pittman-Polletta, B., Hu, K., and Kocsis, B. (2018). Subunit-specific NMDAR antagonism dissociates schizophrenia subtype-relevant oscillopathies associated with frontal hypofunction and hippocampal hyperfunction. Sci. Rep. 8, 11588. doi: 10.1038/s41598-018-29331-8
Poo, C., Agarwal, G., Bonacchi, N., and Mainen, Z. F. (2022). Spatial maps in piriform cortex during olfactory navigation. Nature 601, 595–599. doi: 10.1038/s41586-021-04242-3
Putri, C., Arisa, J., Hananto, J. E., Hariyanto, T. I., and Kurniawan, A. (2021). Psychiatric sequelae in COVID-19 survivors: a narrative review. World J. Psychiatr. 11, 821–829. doi: 10.5498/wjp.v11.i10.821
Ramos, C. G. L., Tan, H., Yamamoto, E. A., Cleary, D. R., Mazur-Hart, D. J., Shahin, M. N., et al. (2023). Stereotactic electroencephalography in epilepsy patients for mapping of neural circuits related to emotional and psychiatric behaviors: a systematic review. Neurosurgical. focus 54, E4. doi: 10.3171/2022.11.FOCUS22615
Rassler, B., and Raabe, J. (2003). Co-ordination of breathing with rhythmic head and eye movements and with passive turnings of the body. Eur. J. Appl. Physiol. 90, 125–130. doi: 10.1007/s00421-003-0876-5
Riecke, L., Sack, A. T., and Schroeder, C. E. (2015). Endogenous delta/theta sound-brain phase entrainment accelerates the buildup of auditory streaming. Curr. Biol. 25, 3196–3201. doi: 10.1016/j.cub.2015.10.045
Rittweger, J., and Popel, A. (1998). Respiratory-like periodicities in slow eye movements during sleep onset. Clin. Physiol. 18, 471–478. doi: 10.1046/j.1365-2281.1998.00128.x
Roberts, E. A. (2007). “Global neural rhythm control by local neuromodulation,” in College of Engineering (Boston University, Boston 2019).
Rodriguez-Sevilla, J. J., Guerri-Fernadez, R., and Bertran Recasens, B. (2022). Is there less alteration of smell sensation in patients with omicron SARS-CoV-2 Variant infection? Front. Med. (Lausanne) 9, 852998. doi: 10.3389/fmed.2022.852998
Rogers, J. P., Chesney, E., Oliver, D., Pollak, T. A., McGuire, P., Fusar-Poli, P., et al. (2020). Psychiatric and neuropsychiatric presentations associated with severe coronavirus infections: a systematic review and meta-analysis with comparison to the COVID-19 pandemic. Lancet Psychiatr. 7, 611–627. doi: 10.1016/S2215-0366(20)30203-0
Rojas-Libano, D., and Kay, L. M. (2008). Olfactory system gamma oscillations: the physiological dissection of a cognitive neural system. Cogn. Neurodyn. 2, 179–194. doi: 10.1007/s11571-008-9053-1
Rojas-Libano, D., Wimmer Del Solar, J., Aguilar-Rivera, M., Montefusco-Siegmund, R., and Maldonado, P. E. (2018). Local cortical activity of distant brain areas can phase-lock to the olfactory bulb's respiratory rhythm in the freely behaving rat. J. Neurophysiol. 120, 960–972. doi: 10.1152/jn.00088.2018
Rossini, P. M., Rossi, S., Babiloni, C., and Polich, J. (2007). Clinical neurophysiology of aging brain: from normal aging to neurodegeneration. Prog. Neurobiol. 83, 375–400. doi: 10.1016/j.pneurobio.2007.07.010
Semba, K., and Komisaruk, B. R. (1984). Neural substrates of two different rhythmical vibrissal movements in the rat. Neuroscience 12, 761–774. doi: 10.1016/0306-4522(84)90168-4
Shahbaz, M. A., De Bernardi, F., Alatalo, A., Sachana, M., Clerbaux, L.-A., Muñoz, A., et al. (2022). Mechanistic understanding of the olfactory neuroepithelium involvement leading to short-term anosmia in COVID-19 using the adverse outcome pathway framework. Cells 11, 22. doi: 10.3390/cells11193027
Sherif, F., Elmokadem, A. H., Razek, A. A., Kamal, E., Abdou, E. H. E., Salem, M. A., et al. (2022). DTI of the Olfactory Bulb in COVID-19-Related Anosmia: A Pilot Study. AJNR Am. J. Neuroradiol. 43, 1180–1183. doi: 10.3174/ajnr.A7590
Sheriff, A., Pandolfi, G., Nguyen, V. S., and Kay, L. M. (2021). Long-range respiratory and theta oscillation networks depend on spatial sensory context. J. Neurosci. 41, 9957–9970. doi: 10.1523/JNEUROSCI.0719-21.2021
Short, S. M., Morse, T. M., McTavish, T. S., Shepherd, G. M., and Verhagen, J. V. (2016). Respiration gates sensory input responses in the mitral cell layer of the olfactory bulb. PLoS One 11, e0168356. doi: 10.1371/journal.pone.0168356
Shu, I. W., Granholm, E. L., and Singh, F. (2022). Targeting Frontal Gamma Activity with Neurofeedback to Improve Working Memory in Schizophrenia. Curr. Top Behav. Neurosci. 22, 377. doi: 10.1007/7854_2022_377
Siok, C. J., Rogers, J. A., Kocsis, B., and Hajos, M. (2006). Activation of alpha7 acetylcholine receptors augments stimulation-induced hippocampal theta oscillation. Eur. J. Neurosci. 23, 570–574. doi: 10.1111/j.1460-9568.2005.04560.x
Sohal, V. S. (2022). Transforming discoveries about cortical microcircuits and gamma oscillations into new treatments for cognitive deficits in schizophrenia. Am. J. Psychiatr. 179, 267–276. doi: 10.1176/appi.ajp.20220147
Soltani, S., Tabibzadeh, A., Zakeri, A., Zakeri, A. M., Latifi, T., Shabani, M., et al. (2021). COVID-19 associated central nervous system manifestations, mental and neurological symptoms: a systematic review and meta-analysis. Rev. Neurosci. 32, 351–361. doi: 10.1515/revneuro-2020-0108
Spinato, G., Fabbris, C., Polesel, J., Cazzador, D., Borsetto, D., Hopkins, C., et al. (2020). Alterations in smell or taste in mildly symptomatic outpatients with SARS-CoV-2 infection. JAMA 323, 2089–2090. doi: 10.1001/jama.2020.6771
Staszelis, A., Mofleh, R., and Kocsis, B. (2022). The effect of ketamine on delta-range coupling between prefrontal cortex and hippocampus supported by respiratory rhythmic input from the olfactory bulb. Brain Res. 1791, 147996. doi: 10.1016/j.brainres.2022.147996
Steriade, M. (2005). Sleep, epilepsy and thalamic reticular inhibitory neurons. Trends Neurosci. 28, 317–324. doi: 10.1016/j.tins.2005.03.007
Syed, S. A., Schnakenberg Martin, A. M., Cortes-Briones, J. A., and Skosnik, P. D. (2022). The relationship between cannabinoids and neural oscillations: how cannabis disrupts sensation, perception, and cognition. Clin EEG Neurosci. 155, 38280. doi: 10.1177/15500594221138280
Takeuchi, Y., and Berenyi, A. (2020). Oscillotherapeutics—time-targeted interventions in epilepsy and beyond. Neurosci. Res. 152, 87–107. doi: 10.1016/j.neures.2020.01.002
Thorn, C. W., Kafetzopoulos, V., and Kocsis, B. (2022). Differential effect of dopamine d4 receptor activation on low-frequency oscillations in the prefrontal cortex and hippocampus may bias the bidirectional prefrontal-hippocampal coupling. Int. J. Mol. Sci. 23, 705. doi: 10.3390/ijms231911705
Tort, A. B. L., Brankack, J., and Draguhn, A. (2018a). Respiration-Entrained Brain Rhythms Are Global but Often Overlooked. Trends Neurosci. 41, 186–197. doi: 10.1016/j.tins.2018.01.007
Tort, A. B. L., Ponsel, S., Jessberger, J., Yanovsky, Y., Brankačk, J., and Draguhn, A. (2018b). Parallel detection of theta and respiration-coupled oscillations throughout the mouse brain. Sci. Rep. 8, 6432. doi: 10.1038/s41598-018-24629-z
Trieu, B. H., Kramár, E. A., Cox, C. D., Jia, Y., Wang, W., Gall, C. M., et al. (2015). Pronounced differences in signal processing and synaptic plasticity between piriform-hippocampal network stages: a prominent role for adenosine. J. Physiol. 593, 2889–2907. doi: 10.1113/JP270398
van Pelt, S., Boomsma, D. I., and Fries, P. (2012). Magnetoencephalography in twins reveals a strong genetic determination of the peak frequency of visually induced gamma-band synchronization. J. Neurosci. 32, 3388–3392. doi: 10.1523/JNEUROSCI.5592-11.2012
Vanderlind, W. M., Rabinovitz, B. B., Miao, I. Y., Oberlin, L. E., Bueno-Castellano, C., Fridman, C., et al. (2021). A systematic review of neuropsychological and psychiatric sequalae of COVID-19: implications for treatment. Curr. Opin. Psychiatr. 34, 420–433. doi: 10.1097/YCO.0000000000000713
Vidyasagar, T. R. (2019). Visual attention and neural oscillations in reading and dyslexia: are they possible targets for remediation? Neuropsychologia 130, 59–65. doi: 10.1016/j.neuropsychologia.2019.02.009
Wachowiak, M. (2011). All in a sniff: olfaction as a model for active sensing. Neuron 71, 962–973. doi: 10.1016/j.neuron.2011.08.030
Waselius, T., Wikgren, J., Penttonen, M., and Nokia, M. S. (2019). Breathe out and learn: Expiration-contingent stimulus presentation facilitates associative learning in trace eyeblink conditioning. Psychophysiology 56, e13387. doi: 10.1111/psyp.13387
Wilson, D. A., Peterson, J., Basavaraj, B. S., and Saito, M. (2011). Local and regional network function in behaviorally relevant cortical circuits of adult mice following postnatal alcohol exposure. Alcohol Clin. Exp. Res. 35, 1974–1984. doi: 10.1111/j.1530-0277.2011.01549.x
Wingrove, J., Makaronidis, J., Prados, F., Kanber, B., Yiannakas, M. C., Magee, C., et al. (2023). Aberrant olfactory network functional connectivity in people with olfactory dysfunction following COVID-19 infection: an exploratory, observational study. EClinicalMedicine 58, 101883. doi: 10.1016/j.eclinm.2023.101883
Wischnewski, M., Alekseichuk, I., and Opitz, A. (2023). Neurocognitive, physiological, and biophysical effects of transcranial alternating current stimulation. Trends. Cogn. Sci. 27, 189–205. doi: 10.1016/j.tics.2022.11.013
Xu, W., and Wilson, D. A. (2012). Odor-evoked activity in the mouse lateral entorhinal cortex. Neuroscience 223, 12–20. doi: 10.1016/j.neuroscience.2012.07.067
Xydakis, M. S., Albers, M. W., Holbrook, E. H., Lyon, D. M., Shih, R. Y., Frasnelli, J. A., et al. (2021). Post-viral effects of COVID-19 in the olfactory system and their implications. Lancet Neurol. 20, 753–761. doi: 10.1016/S1474-4422(21)00182-4
Yang, Q., Zhou, G., Noto, T., Templer, J. W., Schuele, S. U., Rosenow, J. M., et al. (2022). Smell-induced gamma oscillations in human olfactory cortex are required for accurate perception of odor identity. PLoS Biol. 20, e3001509. doi: 10.1371/journal.pbio.3001509
Yanovsky, Y., Ciatipis, M., Draguhn, A., Tort, A. B., and Brankack, J. (2014). Slow oscillations in the mouse hippocampus entrained by nasal respiration. J. Neurosci. 34, 5949–5964. doi: 10.1523/JNEUROSCI.5287-13.2014
Ye, Q., Zhou, J., He, Q., Li, R.-T., Yang, G., Zhang, Y., et al. (2021). SARS-CoV-2 infection in the mouse olfactory system. Cell. Discov. 7, 49. doi: 10.1038/s41421-021-00290-1
Yus, M., Matias-Guiu, J. A., Gil-Martínez, L., Gómez-Ruiz, N., Polidura, C., Jorquera, M., et al. (2022). Persistent olfactory dysfunction after COVID-19 is associated with reduced perfusion in the frontal lobe. Acta. Neurol. Scand. 146, 194–198. doi: 10.1111/ane.13627
Zelano, C., Jiang, H., Zhou, G., Arora, N., Schuele, S., Rosenow, J., et al. (2016). Nasal respiration entrains human limbic oscillations and modulates cognitive function. J. Neurosci. 36, 12448–12467. doi: 10.1523/JNEUROSCI.2586-16.2016
Keywords: respiratory-related coupling, mechanoceptive OB input, cortical oscillations, brain network activity, rhythmic neural synchronization
Citation: Kocsis B and Pittman-Polletta B (2023) Neuropsychiatric consequences of COVID-19 related olfactory dysfunction: could non-olfactory cortical-bound inputs from damaged olfactory bulb also contribute to cognitive impairment? Front. Neurosci. 17:1164042. doi: 10.3389/fnins.2023.1164042
Received: 11 February 2023; Accepted: 24 May 2023;
Published: 22 June 2023.
Edited by:
Dongdong Qin, Yunnan University of Traditional Chinese Medicine, ChinaReviewed by:
Jordi A. Matias-Guiu, Hospital Clínico San Carlos, SpainCopyright © 2023 Kocsis and Pittman-Polletta. This is an open-access article distributed under the terms of the Creative Commons Attribution License (CC BY). The use, distribution or reproduction in other forums is permitted, provided the original author(s) and the copyright owner(s) are credited and that the original publication in this journal is cited, in accordance with accepted academic practice. No use, distribution or reproduction is permitted which does not comply with these terms.
*Correspondence: Bernat Kocsis, YmtvY3Npc0BobXMuaGFydmFyZC5lZHU=
†These authors have contributed equally to this work