- 1Molecular Neurogenetics, Max Planck Institute of Psychiatry, Munich, Germany
- 2Department of Animal Model Development, Brain Research Institute, Niigata University, Niigata, Japan
- 3Super-Network Brain Physiology, Graduate School of Life Sciences, Tohoku University, Sendai, Japan
Corticotropin-releasing hormone expressing (CRH+) neurons are distributed throughout the brain and play a crucial role in shaping the stress responses. Mouse models expressing site-specific recombinases (SSRs) or reporter genes are important tools providing genetic access to defined cell types and have been widely used to address CRH+ neurons and connected brain circuits. Here, we investigated a recently generated CRH-FlpO driver line expanding the CRH system-related tool box. We directly compared it to a previously established and widely used CRH-Cre line with respect to the FlpO expression pattern and recombination efficiency. In the brain, FlpO mRNA distribution fully recapitulates the expression pattern of endogenous Crh. Combining both Crh locus driven SSRs driver lines with appropriate reporters revealed an overall coherence of respective spatial patterns of reporter gene activation validating CRH-FlpO mice as a valuable tool complementing existing CRH-Cre and reporter lines. However, a substantially lower number of reporter-expressing neurons was discerned in CRH-FlpO mice. Using an additional CRH reporter mouse line (CRH-Venus) and a mouse line allowing for conversion of Cre into FlpO activity (CAG-LSL-FlpO) in combination with intersectional and subtractive mouse genetic approaches, we were able to demonstrate that the reduced number of tdTomato reporter expressing CRH+ neurons can be ascribed to the lower recombination efficiency of FlpO compared to Cre recombinase. This discrepancy particularly manifests under conditions of low CRH expression and can be overcome by utilizing homozygous CRH-FlpO mice. These findings have direct experimental implications which have to be carefully considered when targeting CRH+ neurons using CRH-FlpO mice. However, the lower FlpO-dependent recombination efficiency also entails advantages as it provides a broader dynamic range of expression allowing for the visualization of cells showing stress-induced CRH expression which is not detectable in highly sensitive CRH-Cre mice as Cre-mediated recombination has largely been completed in all cells generally possessing the capacity to express CRH. These findings underscore the importance of a comprehensive evaluation of novel SSR driver lines prior to their application.
1. Introduction
Genetically engineered mouse models (GEMMs) are potent tools used in neuroscience research and crucial for understanding mechanisms underlying neurological and psychiatric disorders. Cre-loxP and Flp-FRT are site-specific recombinase (SSR) systems widely used in GEMMs. The combination of Cre-/Flp-expressing mouse strains with recombinase-dependent reporters or effectors has proven as a versatile and efficient approach offering a myriad of options ranging from manipulation of gene expression and cell activity to visualization of molecules, cells and circuits (Deussing, 2013; Luo et al., 2018; Arias et al., 2022).
Corticotropin-releasing hormone (CRH; also known as corticotropin-releasing factor, CRF) is a neuropeptide playing a critical role in different aspects of the body’s stress response. CRH in the paraventricular nucleus of the hypothalamus (PVN) controls the neuroendocrine response by activating the hypothalamic–pituitary–adrenal (HPA) axis and regulating adrenocorticotropic hormone (ACTH) secretion from the pituitary which, in turn, triggers the synthesis and release of glucocorticoids from the adrenal glands as ultimate effectors of the stress response (Ulrich-Lai and Herman, 2009). Parvocellular CRH neurons in the PVN are localized at the apex of the HPA axis and represent a specialized subpopulation of excitatory CRH neurons co-expressing the vesicular glutamate transporter 2 (VGLUT2) (Dabrowska et al., 2013; Romanov et al., 2017; Dedic et al., 2018b).
Beyond the PVN, CRH is widely expressed throughout the brain (Peng et al., 2017). Here, in contrast to the PVN, CRH is predominantly found in inhibitory neurons expressing GABAergic markers, such as glutamic acid decarboxylase (also known as glutamate decarboxylase) 65 and 67 (GAD65/GAD67) (Romanov et al., 2017; Dedic et al., 2018b, 2019). Inhibitory CRH neurons show a high degree of morphological and physiological diversity (Wang et al., 2021). For instance, in the cortex, CRH neurons co-express markers of different subpopulations of GABAergic neurons, such as vasoactive intestinal peptide (VIP), calretinin (CR), cholecystokinin (CCK), and somatostatin (SOM), but not parvalbumin (PV) or neuropeptide Y (NPY) (Kubota et al., 2011). In the hippocampus, CRH neurons were found to be positive for PV, CR, and CCK but not for calbindin (CB) or SOM (Gunn et al., 2019). In subregions of the extended amygdala encompassing the central amygdala (CeA), the lateral part of the interstitial nucleus of the posterior limb of the anterior commissure (IPACL) and the bed nucleus of the stria terminalis (BNST), CRH neurons again express a diverse and region-specific set of GABAergic markers including protein kinase C δ (PKCδ), SOM, neurokinin B (NKB), neurotensin (NTS) or CB (Sanford et al., 2017; Dedic et al., 2018b; Chang et al., 2022; Soden et al., 2022). Cortical and hippocampal CRH neurons possess typical features of interneurons. The extended amygdala, however, harbors a large fraction of CRH neurons representing GABAergic long-range projection neurons which co-express calcium/calmodulin-dependent protein kinase 2α (CAMK2A) and innervate midbrain structures, such as the ventral tegmental area and the substantia nigra (Dedic et al., 2018b; Chang et al., 2022; Soden et al., 2022). The heterogeneity of neuronal CRH populations suggests a unique role of these neurons in the regulation of responses to salient stimuli in different brain regions. Accordingly, the CRH system has been shown to bidirectionally modulate anxiety- and stress-related behaviors in dependence of its localization and prior stress experience (Refojo et al., 2011; Lemos et al., 2012; Dedic et al., 2018b).
As a neuropeptide, CRH is sorted into large dense core vesicles and immediately transported to be stored at its release sites, making reliable detection of CRH+ soma somewhat difficult. Moreover, it is of note that even with the most efficient antiserum raised against CRH more than 4 decades ago in the Vale lab (Bloom et al., 1982), the accessibility of CRH by immunohistochemistry is largely unsatisfying (Itoi et al., 2014). Hence, extensive efforts have been undertaken in the past years to gain genetic access to CRH+ cells by developing respective reporter and SSR mouse lines (Table 1). Different strategies have been applied, ranging from classic transgenic approaches using small promoter fragments to large bacterial artificial chromosome (BAC)-based constructs and knock-in approaches. The first transgenic β-galactosidase reporter mice, carrying a lacZ construct flanked by 8.7 kb of Crh promoter region, already revealed difficulties in fully recapitulating the endogenous CRH expression pattern (Keegan et al., 1994). This has been further corroborated by some of the subsequently generated transgenic reporter or Cre driver lines revealing limitations in their use because of ectopic expression and/or failure of expression (Alon et al., 2009; Martin et al., 2010; Sarkar et al., 2011; Chen et al., 2015; Korecki et al., 2019). These observations underscore the utter importance to extensively characterize SSR mouse models to validate spatial and temporal expression patterns as a prerequisite for a meaningful interpretation of studies using these GEMMs (Heffner et al., 2012; Luo et al., 2020). In this regard, knock-in strategies, also in the case of CRH, have proven their superiority as reflected by reliable and reproducible reporter and Cre expression (Taniguchi et al., 2011; Itoi et al., 2014; Krashes et al., 2014; Kono et al., 2017). Accordingly, CRH-Cre knock-in mice have been widely applied to explore the physiology of CRH neurons in the central nervous system (Krashes et al., 2014; Marcinkiewcz et al., 2016; Fadok et al., 2017; Sanford et al., 2017; Dedic et al., 2018b; Wang et al., 2021; Chang et al., 2022).
The Flp-FRT system was initially introduced to remove selection markers from Cre-loxP-dependent conditional alleles (Rodríguez et al., 2000) but with the concerted improvement of its recombination efficiency (Buchholz et al., 1998; Raymond and Soriano, 2007) the Flp-FRT system has developed into a powerful alternative SSR which, in combination with the Cre-loxP system, allows for parallel, intersectional or subtractive approaches. Only recently, a first CRH-FlpO mouse line was established, thus expanding the CRH system-related tool box (Salimando et al., 2020) and providing alternative access to CRH neurons, which is highly demanded considering their enormous diversity. However, a systematic characterization of this novel mouse line has not been reported so far which to some extent limits its immediate use. In particular, considering repeated accounts on the diversity of reporter expression conveyed by previously established CRH-Cre lines demands a comprehensive assessment of the recombination pattern in this novel CRH-FlpO mouse line (Chen et al., 2015; Dedic et al., 2018a).
Here, we characterized CRH-FlpO mice and directly compared their recombination properties throughout the brain with a well-established CRH-Cre mouse line as a reference (Taniguchi et al., 2011) using 9 different reporter mouse models. We focused our evaluation of SSR-activated reporter gene expression patterns on brain regions well-known to possess CRH+ neurons involved in the neuroendocrine and behavioral stress response. Our analysis revealed similarities but also discrepancies between CRH-FlpO and CRH-Cre mice that have direct implications for the use of SSRs driven by the Crh locus.
2. Materials and methods
2.1. Animals
All animal experiments were conducted with the approval of and in accordance with the Guide of the Care and Use of Laboratory Animals of the Government of Upper Bavaria, Germany. Mice were group- housed under standard lab conditions (22 ± 1°C, 55 ± 5% humidity) and maintained under a 12 h light–dark cycle with food and water ad libitum. All experiments were conducted with adult male or female mice (age: 2–5 months). For each mouse line 3–7 animals were analyzed of which representative images are included in the figures.
The following transgenic mouse lines were used in this study: CRH-Cre (Crhtm1(cre)Zjh, Jackson Laboratory stock no. 012704) (Taniguchi et al., 2011), CRH-FlpO (Crhtm1.1(flpo)Bsab, Jackson Laboratory stock no. 031559; Supplementary Material, provided by Bernado Sabatini and Caiying Guo) (Salimando et al., 2020), CRH-Venus (Crhtm1.1Ksak, RIKEN BRC stock no. BRC09893) (Kono et al., 2017), Ai9 (Gt(ROSA)26Sortm9(CAG-tdTomato)Hze, Jackson Laboratory stock no. 007909) (Madisen et al., 2010), Ai65 (Gt(ROSA)26Sortm65.1(CAG-tdTomato)Hze, Jackson Laboratory stock no. 021875) (Madisen et al., 2015) and CAG-LSL-FlpO (Gt(ROSA)26Sortm5(CAG-flpo)Zjh, Jackson Laboratory stock no. 028584) (He et al., 2016). A pure Flp reporter line was generated by breeding the intersectional Ai65 reporter to a Deleter-Cre line removing the loxP-flanked transcriptional terminator (STOP). This novel mouse line, reporting only Flp recombinase activity, was designated as Ai65F. In this study, the following double and triple transgenic lines were generated by cross-breeding of single transgenic lines: CRH-Cre::Ai9, CRH-FlpO::Ai65F, CRH-Venus::CRH-Cre::Ai9, CRH-Venus::CRH-FlpO::Ai65F, CRH-Cre::CRH-FlpO::Ai65, CRH-Cre::CRH-FlpO::Ai9, CRH-Cre::CAG-LSL-FlpO::Ai65 and CRH-Cre::CAG-LSL-FlpO::Ai9. All transgenic alleles involving the Rosa26 locus were heterozygous. Detailed breeding schemes, primer sequences and protocols for genotyping are available upon request.
2.2. Preparation of brain sections
Vibratome sections: mice were sacrificed by an overdose of isoflurane and transcardially perfused with 20 mL 0.1 M PBS (4°C) supplemented with heparin and subsequently with 20 mL of 4% paraformaldehyde (PFA) in 0.1 M PBS (4°C). After recovery, brains were post-fixed overnight in 4% PFA at 4°C and coronal 50 μm sections were prepared using a vibratome (Microm HM 650 V, Thermo-Fisher Scientific). Cryosections: perfused and dissected brains were post-fixed in 4% PFA for 6 h. After 48 h in 30% sucrose in 0.1 M PBS at 4°C, brains were snap frozen on dry ice and 40 μm sections were prepared using a cryostat (Microm, Walldorf, Germany). All sections were stored at −20°C in cryopreservation solution (25% glycerol, 25% ethylene glycol, 50% 0.1 M PBS, pH 7.4) until further use.
2.3. Immunohistochemistry
Vibratome sections were blocked with 10% normal goat serum (NGS) and 0.15% Triton in PBS for 30 min at RT and then washed in 0.1 M PBS for 3 × 5 min. Subsequently, sections were incubated with anti-GFP primary antibody (chicken polyclonal antibody; 1:500; abcam) diluted in 0.15% NGS and 0.15% Triton in PBS overnight at 4°C. After another washing step, sections were incubated with an Alexa Fluor 488 secondary antibody (goat anti-chicken IgG; 1:250; Invitrogen) while shaking for 2 h at RT. Following 3 more washing steps, sections were mounted on glass slides (epredia) with Fluoromount-G (SouthernBiotech).
2.4. In situ hybridization
Freshly dissected brains were snap frozen on dry ice and stored at −80°C until further use. Brains were sectioned coronally at 20 μm using a cryostat. Sections were mounted onto SuperFrost slides and kept at −80°C until further use. ISH was performed as previously described (Dedic et al., 2018b). The following FlpO-specific riboprobe was used: bp 311–641 of MH493814.
2.5. Acute restraint stress
To test the capacity of the CRH-FlpO mouse line to induce reporter gene expression in CRH+ cells following stress exposure, CRH-FlpO::Ai65F mice were exposed to acute restraint stress. On the day of the experiment, animals were placed for 15 min in a 50 ml conical tube with the bottom removed. Animals were sacrificed 1 week later and brains were used for the assessment of stress-induced expression of tdTomato.
2.6. Image acquisition
Vibratome sections and cryosections were imaged using a slidescanner or confocal microscope. Slidescanner: overviews of whole brain sections and region-specific scans were performed with an Olympus VS120 Slide Scanner. Image capturing and initial processing was conducted with the Olympus VS Software. Briefly, overview images of the whole slide were acquired in the batch scanning mode. Subsequently, region-specific scans were taken with the 20×, whole sections images with the 10× objective. The scan region was adjusted to the size of the region. Exposure time and focus were set to automated mode. Sections were acquired with the Cy3 (TdTomato) channel or the FITC (GFP) channel. Confocal microscopy: for higher resolution, confocal images of regions of interest were acquired with a Zeiss Axioplan2 fluorescence microscope using Axio Vision 4.5 software. Fluorescently labeled cells were excited at 488 nm (GFP Alexa-488) and 559 nm (tdTomato, Alexa-594), respectively, using a 20× objective. After acquisition in virtual slide (.VSI file) or Carl Zeiss (.CZI) format, images were extracted and saved as Tagged Image File Format (.tiff) for further processing. Images were analyzed using Fiji1 and Inkscape (version 1.2.2.).
3. Results
3.1. FlpO expression pattern in CRH-FlpO mice recapitulates endogenous CRH expression
CRH-FlpO mice were generated by targeted insertion of a FlpO expression cassette into the murine Crh locus immediately downstream of the stop codon. FlpO expression driven by the Crh locus is conveyed by an internal ribosomal entry site (IRES). As a selection marker, a self-excising neomycin cassette driven by the testis-specific angiotensin converting enzyme promotor (tACE) was used, which removed itself in the male germline leaving a single loxP2272 site behind (Figure 1A and Supplementary Material). To validate FlpO expression, we used heterozygous CRH-FlpO mice and performed an in situ hybridization (ISH) against FlpO. The spatial pattern of FlpO mRNA expression fully recapitulated the expression of endogenous Crh as indicated by the detection of distinct signals in all regions of salient CRH expression including the piriform cortex (Pir), IPACL, BNST, CeA, and PVN. The expression of FlpO appeared generally weaker, as CRH-FlpO mice were only heterozygous (Figure 1B).
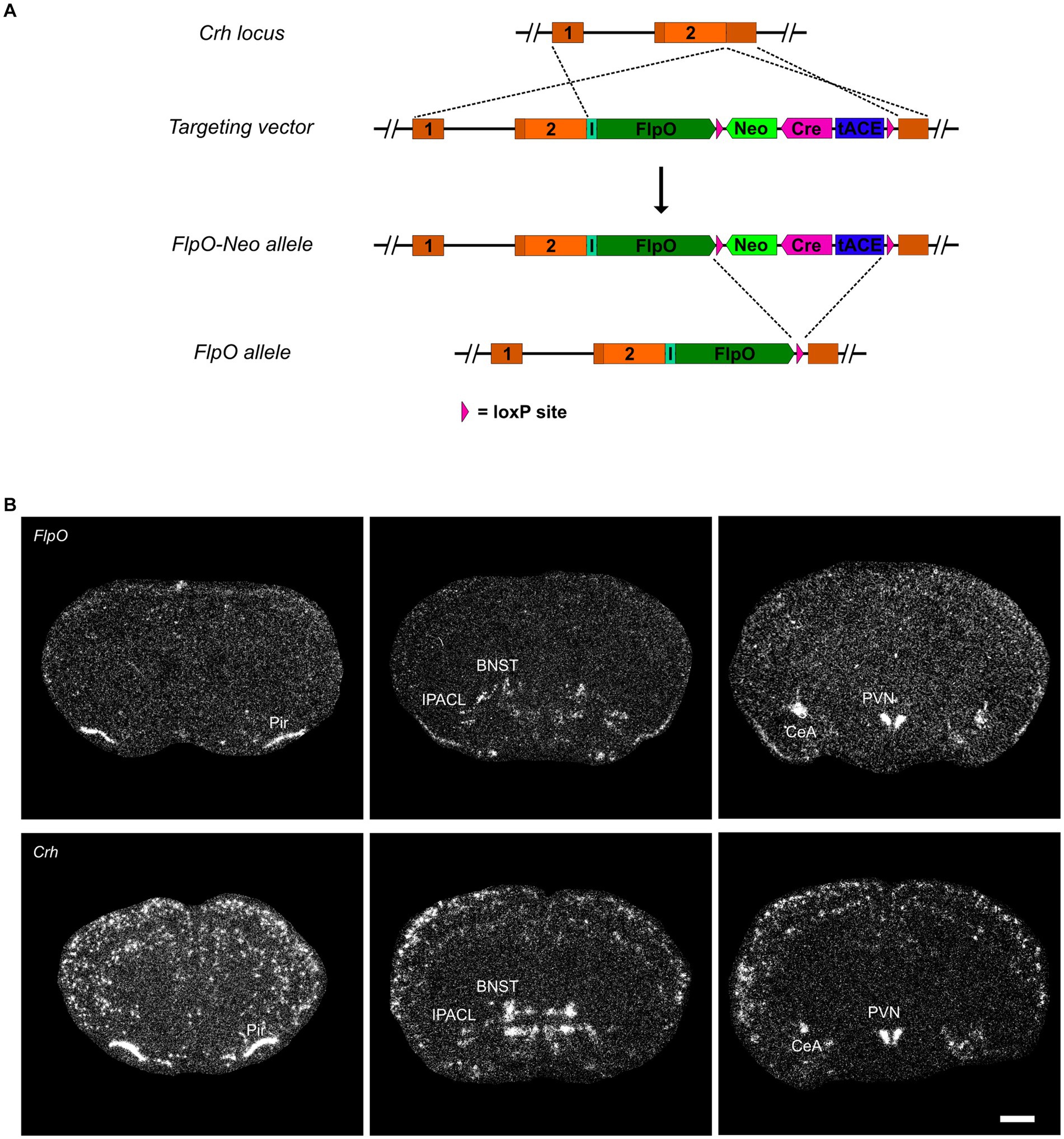
Figure 1. FlpO expression pattern in CRH-FlpO mice recapitulates endogenous CRH expression. (A) Scheme illustrating the generation of CRH-FlpO mice by homologous recombination-mediated gene targeting. (B) In situ hybridization (ISH) on coronal brain sections of heterozygous (Crh+/FlpO) (n = 3) and wild-type (Crh+/+) CRH-FlpO (n = 3) mice using FlpO- and Crh-specific riboprobes showing comparable expression patterns, e.g., the in piriform cortex (Pir), lateral part of the interstitial nucleus of the anterior commissure (IPACL), bed nucleus of the stria terminalis (BNST), central amygdala (CeA) and paraventricular nucleus of the hypothalamus (PVN). I, internal ribosomal entry site; loxP, loxP2272 Neo, neomycine resistance gene. Scale bar, 1,000 μm.
3.2. Comparison of FlpO- and Cre-activated reporter gene expression
To compare the pattern and efficiency of FlpO- and Cre-mediated recombination driven by the Crh locus, we bred CRH-FlpO and CRH-Cre mice to appropriate reporter lines (Figures 2A,B). The reporter alleles in Ai65F and Ai9 mice are ideally suited for direct comparison of FlpO and Cre activity, as both are based on the Rosa26 locus harboring an identical CAG promotor-driven tdTomato expression unit, which is preceded by an FRT- and a loxP-flanked transcriptional terminator, respectively (Figures 2A,B). Similarly, the Crh alleles harboring either FlpO or Cre recombinase were designed in the same way, i.e., the recombinase was integrated immediately downstream of the Crh STOP codon and is driven by an IRES.
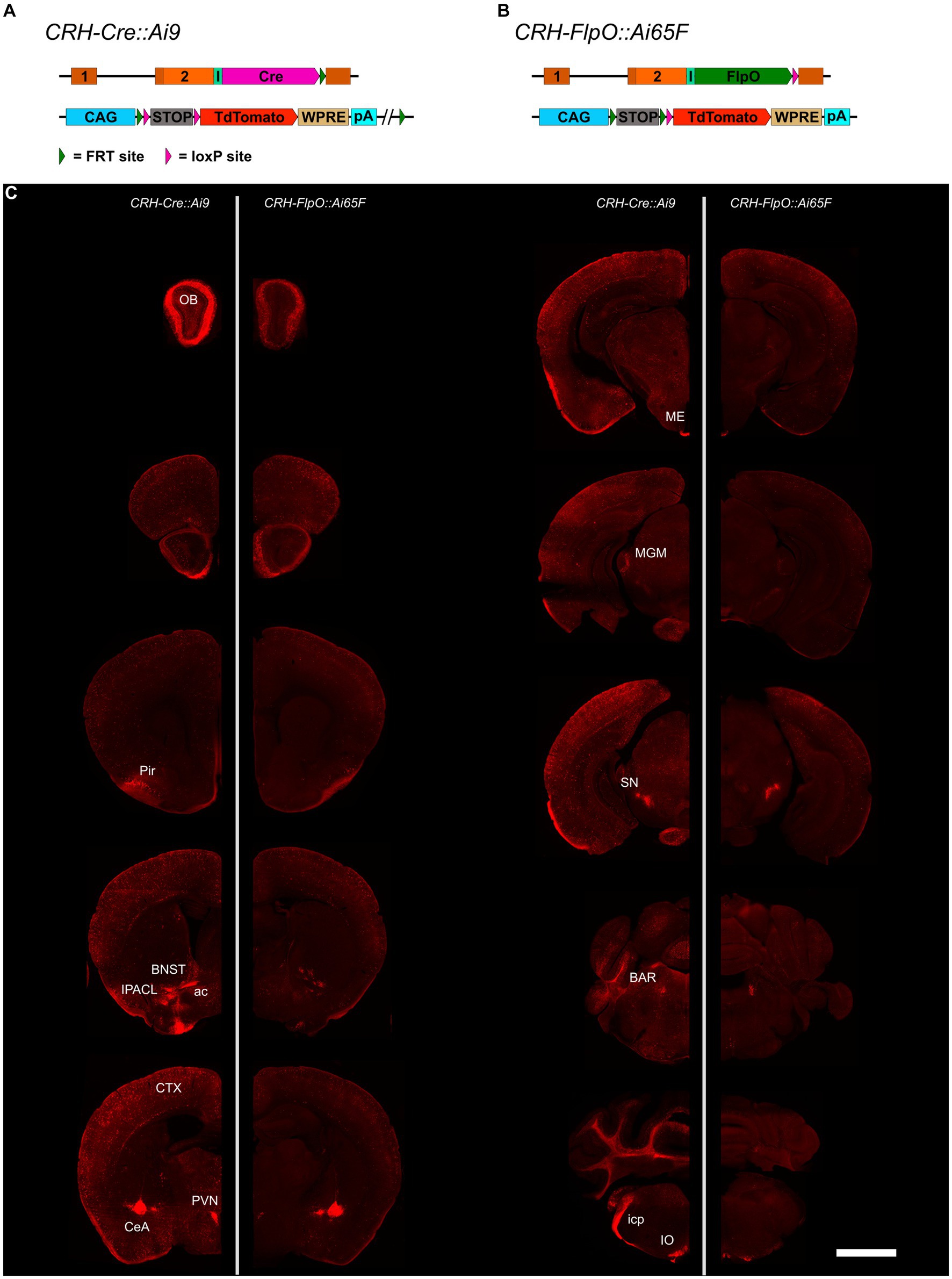
Figure 2. Comparison of the distribution of tdTomato+ cells and fibers in the brain of CRH-Cre::Ai9 and CRH-FlpO::Ai65F mice. (A,B) Schematic illustration combining photomicrographs of CRH-Cre::Ai9 (left, n = 5) and CRH-FlpO::Ai65F (right, n = 4) reporter mice. (C) Distribution of tdTomato+ cells and fibers in CRH-Cre::Ai9 and CRH-FlpO::Ai65F mice. ac, anterior commissure; BAR, Barrington’s nucleus; BNST, bed nucleus of the stria terminalis; CeA, central amygdala; I, internal ribosomal entry site; icp, inferior cerebellar peduncle; IO, inferior olivary nucleus; IPACL, lateral part of the interstitial nucleus of the anterior commissure; ME, median eminence; MGM, medial division of the medial geniculate; OB, olfactory bulb; Pir, piriform cortex; PVN, paraventricular nucleus of the hypothalamus; SN, substantia nigra. WRPE, woodchuck hepatitis virus posttranscriptional regulatory element. Scale bar, 1,000 μm.
The tdTomato expression pattern was analyzed throughout the brain of CRH-FlpO::Ai65F and CRH-Cre::Ai9 mice. In both mouse lines, tdTomato-positive neurons were detectable in all brain regions which have previously been reported to endogenously express CRH (Kono et al., 2017; Wang et al., 2021), including the olfactory bulb (OB), cortex (CTX), IPACL, BNST, PVN, CeA, Barrington’s nucleus (BAR) and inferior olivary nucleus (IO) (Figure 2C). Moreover, characteristic tdTomato-labeled CRH+ fibers were visible in the anterior commissure (ac) and the inferior cerebellar peduncle (icp), the latter representing climbing fibers originating in the IO and innervating the cerebellum (Ezra-Nevo et al., 2018). In addition, prominent tdTomato-labeled terminals were detectable in the ME and substantia nigra (SN), sites well-known for their massive CRH afferents (Chang et al., 2022). Taken together, our results indicate that the CRH-FlpO mice are able to promote site-specific recombination at endogenous CRH expression sites comparable with CRH-Cre mice. However, imaging with identical exposure times revealed an overall lower fluorescence intensity detectable throughout brain sections of CRH-FlpO::Ai65F compared to CRH-Cre::Ai9 mice (Figure 2C). This discrepancy in intensity likely originates from a reduced number of tdTomato-labeled soma and fibers present in CRH-FlpO::Ai65F mice, an observation which necessitated closer inspection.
3.3. FlpO-dependent recombination is inferior to Cre-mediated recombination
To interrogate the discrepancy in tdTomato expression between CRH-FlpO::Ai65F and CRH-Cre::Ai9 mice in more detail, we focused our analysis on CRH-expressing brain structures involved in the neuroendocrine (HPA axis: PVN, ME) and behavioral (extended amygdala: CeA, IPACL, BNST) stress response as well as on the CTX, because of its homogeneous and scattered distribution of CRH+ neurons. First, we tested the degree of overlapping Cre and FlpO expression, comparing the occurrence of tdTomato+ cells in the intersectional reporter mouse line CRH-Cre::CRH-FlpO::Ai65 with CRH-Cre::Ai9 mice (Figure 3A). In all analyzed structures, CRH-Cre::CRH-FlpO::Ai65 mice showed a markedly reduced number of tdTomato+ cells compared to CRH-Cre::Ai9 mice. Instead, the number of tdTomato+ cells was comparable to CRH-FlpO::Ai65F mice, suggesting that the FlpO-mediated recombination in CRH+ cells was less efficient compared to Cre-mediated recombination driven by the Crh locus (Figure 3B).
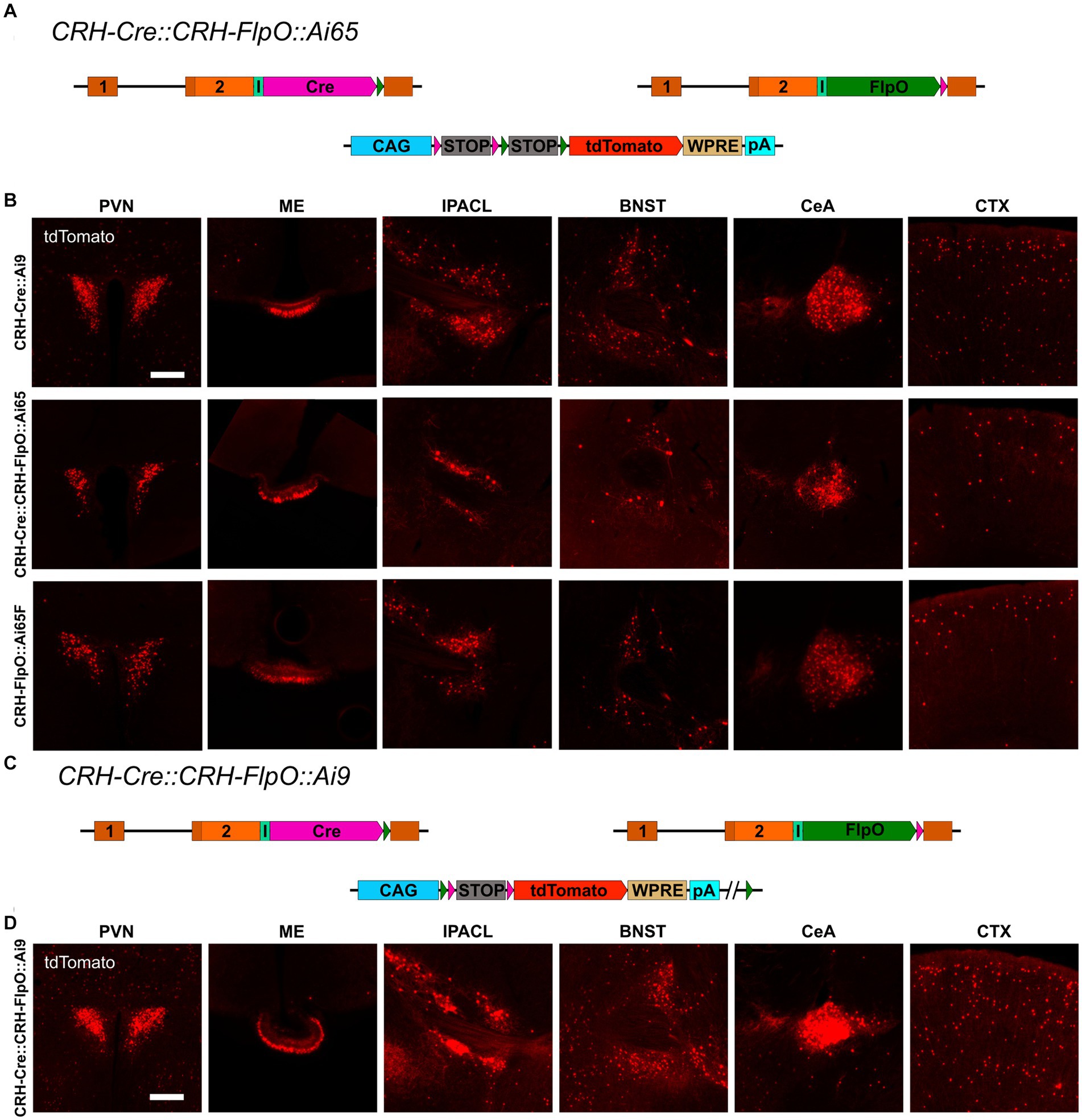
Figure 3. Reporter mice reveal diverging FlpO- and Cre-mediated recombination efficiency. (A) Schematic illustration of CRH-Cre::CRH-FlpO::Ai65 reporter mice. (B) Comparison of the distribution of tdTomato+ cells and fibers in regions of interest in CRH-Cre::Ai9 (n = 5), CRH-Cre::CRH-FlpO::Ai65 (n = 4) and CRH-FlpO::Ai65F (n = 4) mice. (C) Schematic illustration of CRH-Cre::CRH-FlpO::Ai9 reporter mice. (D) Distribution of tdTomato+ cells and fibers in regions of interest in CRH-Cre::CRH-FlpO::Ai9 mice (n = 6). BNST, bed nucleus of the stria terminalis; CeA, central amygdala; I, internal ribosomal entry site; CTX, cortex; IPACL, lateral part of the interstitial nucleus of the anterior commissure; ME, median eminence; pA, BGH polyA signal; PVN, paraventricular nucleus of the hypothalamus; WRPE, woodchuck hepatitis virus posttranscriptional regulatory element. Scale bars, 200 μm.
To rule out the possibility that differences in the genetic composition in which the tdTomato reporter is embedded in Ai9 vs. Ai65F mice was causal for the differential reporting, we used the subtractive capacity of the Ai9 reporter line. This reporter line still contains the neomycin selection marker and a downstream FRT site, which makes the tdTomato expression cassette vulnerable to deletion via Flp recombinase, resulting in a loss of tdTomato expression in cells previously activated by Cre-mediated recombination (Figure 3C). However, the analysis of the PVN, ME, IPACL, BNST, CeA, and CTX in CRH-Cre::CRH-FlpO::Ai9 mice did not reveal any substantial reduction in tdTomato expression but instead showed similar numbers of tdTomato expressing cells as detected in CRH-Cre::Ai9 mice (Figure 3D).
Taken together, these data confirm a high degree of co-expression of Cre and FlpO driven from the Crh locus but also provide evidence that the Cre recombinase is more efficiently activating reporter gene expression compared to the FlpO recombinase.
3.4. Discrepancies between direct and indirect reporting of CRH expression
The recombination efficiency is determined by intrinsic properties of the respective SSR itself and by environmental conditions, e.g., the accessibility of recognition sites within the chromatin structure. Another important aspect is the expression, which needs to reach a certain threshold to allow site-specific recombination. To evaluate the influence of the expression level, we used CRH-Venus reporter mice in which the fluorescent protein Venus was integrated at the Crh start codon. This direct reporter allele readily reports CRH expression and is at the same time a CRH knockout allele (Figure 4A), whereas CRH-Cre::Ai9 and CRH-FlpO::Ai65F mice serve as indirect reporters enabling the identification of CRH+ cells which are labeled by accumulating and enduring tdTomato expression subsequent to CRH expression. Generally, the CRH expression level is rather low and does not allow reliable detection of Venus expression without antibody staining (Figure 4B). We generated CRH-Venus::CRH-Cre::Ai9 and CRH-Venus::CRH-FlpO::Ai65F mice to directly compare CRH-Venus expression with Cre/FlpO-induced tdTomato expression, i.e., direct versus indirect reporting. Detailed analysis revealed a largely congruent expression in all regions of interest in both mouse lines (Figures 4C,D), i.e., a significant proportion of tdTomato+ cells showed co-expression of Venus. The distribution of Venus+ cells in both mouse lines shows a high degree of concordance indicating similar levels of CRH expression. Thus, the differences observed in tdTomato+ cells cannot be attributed to general variations in CRH expression in the two mouse lines. Moreover, we observed in CRH-Venus::CRH-Cre::Ai9 mice an excess of tdTomato+ cells compared to Venus expressing cells (Supplementary Figure S1). The detection of tdTomato+/Venus− cells suggests that either (i) Cre caused ectopic CRH reporting by tdTomato, (ii) CRH expression remained below a certain threshold, preventing reliable visualization by antibody staining, or (iii) CRH expression occurred only transiently during development but had ceased in adulthood. To evaluate the influence of the Venus expression level on the number of reported neurons, we bred CRH-Venus mice to homozygosity, which resulted in a significantly enhanced Venus signal and an increased number of Venus+ cells in all regions of interest. This result indicated that tdTomato+/Venus− neurons were not a consequence of ectopic Cre expression but rather reflected their low level of CRH expression. Accordingly, the Venus levels of these cells were too low to be detected by antibody staining, whereas the similarly low amount of Cre recombinase was sufficient to activate tdTomato expression (Figure 4E). Of note, a small proportion of Venus+ cells did not express tdTomato, which additionally suggests some limitations of CAG promoter-based reporters located in the Rosa26 locus (Figure 4C; Supplementary Figure S1). CRH-Venus::CRH-FlpO::Ai65F mice generally displayed less tdTomato+/Venus+ cells. However, no clear correlation between the detected Venus expression level and expression of tdTomato was observed (Figure 4D).
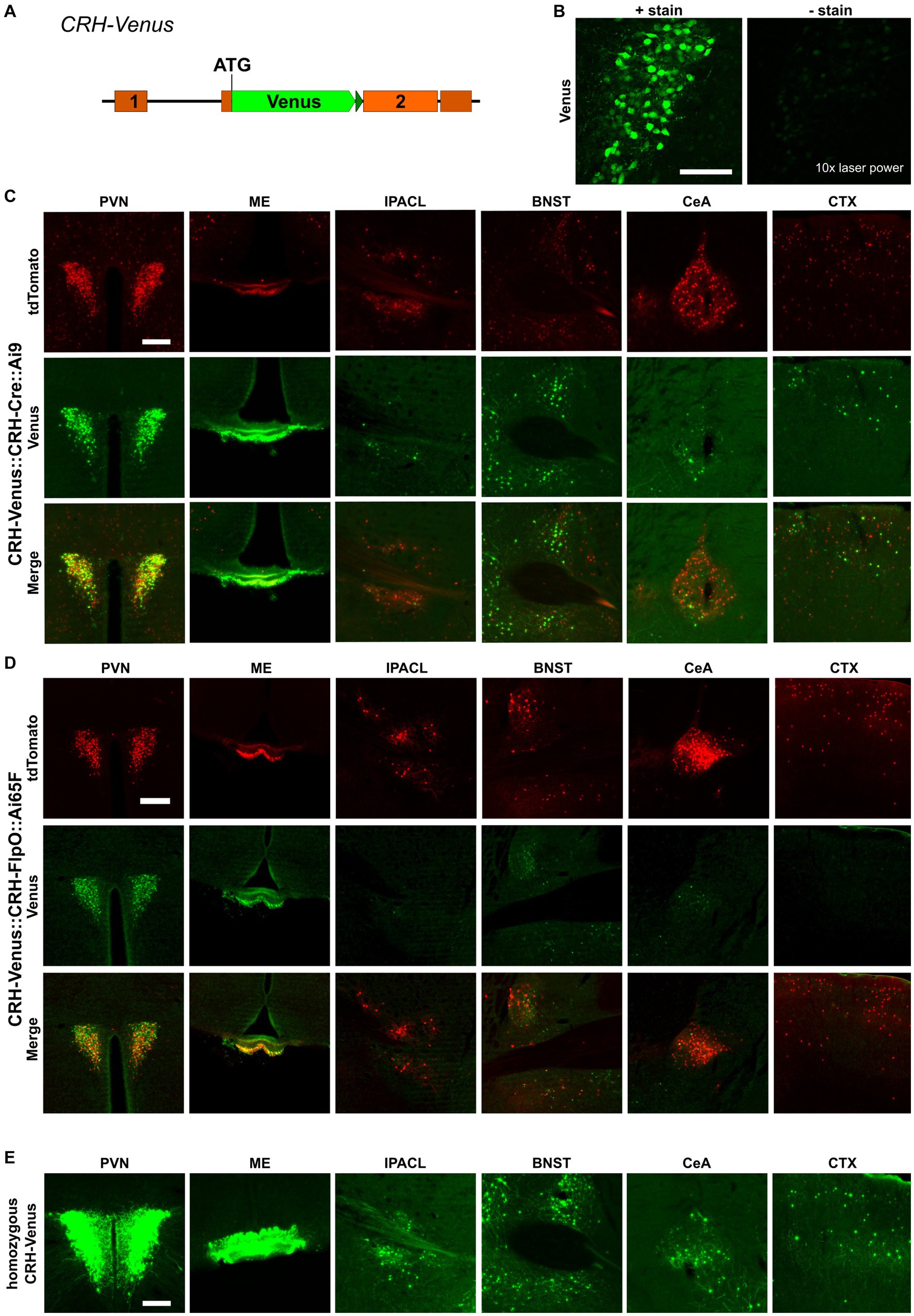
Figure 4. Cre recombinase possesses high recombination efficiency, even at low expression levels. (A) Schematic illustration of CRH-Venus reporter mice. (B) Visualization of Venus+ cells with or without GFP antibody staining. Scale bar, 100 μm. (C) Comparison of the distribution of tdTomato+ and Venus+ cells and fibers in regions of interest in CRH-Venus::CRH-Cre::Ai9 mice (n = 3). Scale bar, 200 μm. (D) Comparison of the distribution of tdTomato+ and Venus+ cells and fibers in regions of interest in CRH-Venus::CRH-FlpO::Ai65F mice (n = 6). Scale bar, 200 μm. (E) Distribution of Venus+ cells and fibers in regions of interest in homozygous CRH-Venus mice (n = 3). Scale bar, 200 μm. BNST, bed nucleus of the stria terminalis; CeA, central amygdala; CTX, cortex; IPACL, lateral part of the interstitial nucleus of the anterior commissure; ME, median eminence; PVN, paraventricular nucleus of the hypothalamus.
3.5. FlpO recombination efficacy in CRH cells depends on the FlpO expression level
The previous results revealed that the strength of the Crh promoter differs strongly between neurons, even in the same region of interest. Therefore, CAG-LSL-FlpO mice were used to functionally validate the impact of the expression level on FlpO recombination efficiency. Since the recombination efficiency of a specific recombinase is dependent on its nuclear availability, it is also influenced by its expression level and therefore by the strength of its driving promoter. CAG-LSL-FlpO mice allow to convert any Cre activity into FlpO activity, which is then driven by the strong CAG promoter. Accordingly, CRH-Cre::CAG-LSL-FlpO::Ai65 mice express high levels of FlpO in all CRH+ cells previously expressing Cre (Figure 5A). Careful analysis of brain regions of interest revealed a considerable increase of tdTomato-expressing CRH neurons throughout the brain resembling the pattern and extent of expression previously observed in CRH-Ai9 mice (Figure 5C). These findings provide strong evidence for a direct correlation of FlpO recombination efficacy with its expression level in CRH+ neurons. Along those lines, and in contrast to CRH-Cre::CRH-FlpO::Ai9 mice, CRH-Cre::CAG-LSL-FlpO::Ai9 mice (Figure 5D) showed a substantial reduction of tdTomato-expressing CRH+ cells, which was most prominent in the PVN, ME, and CeA (Figure 5C). Along these lines, homozygous mice expressing FlpO from both Crh alleles, showed a considerable increase in tdTomato+ cells compared heterozygous CRH-FlpO mice. This result directly proves that the level of FlpO expression determines the efficiency of FlpO recombination in CRH+ cells.
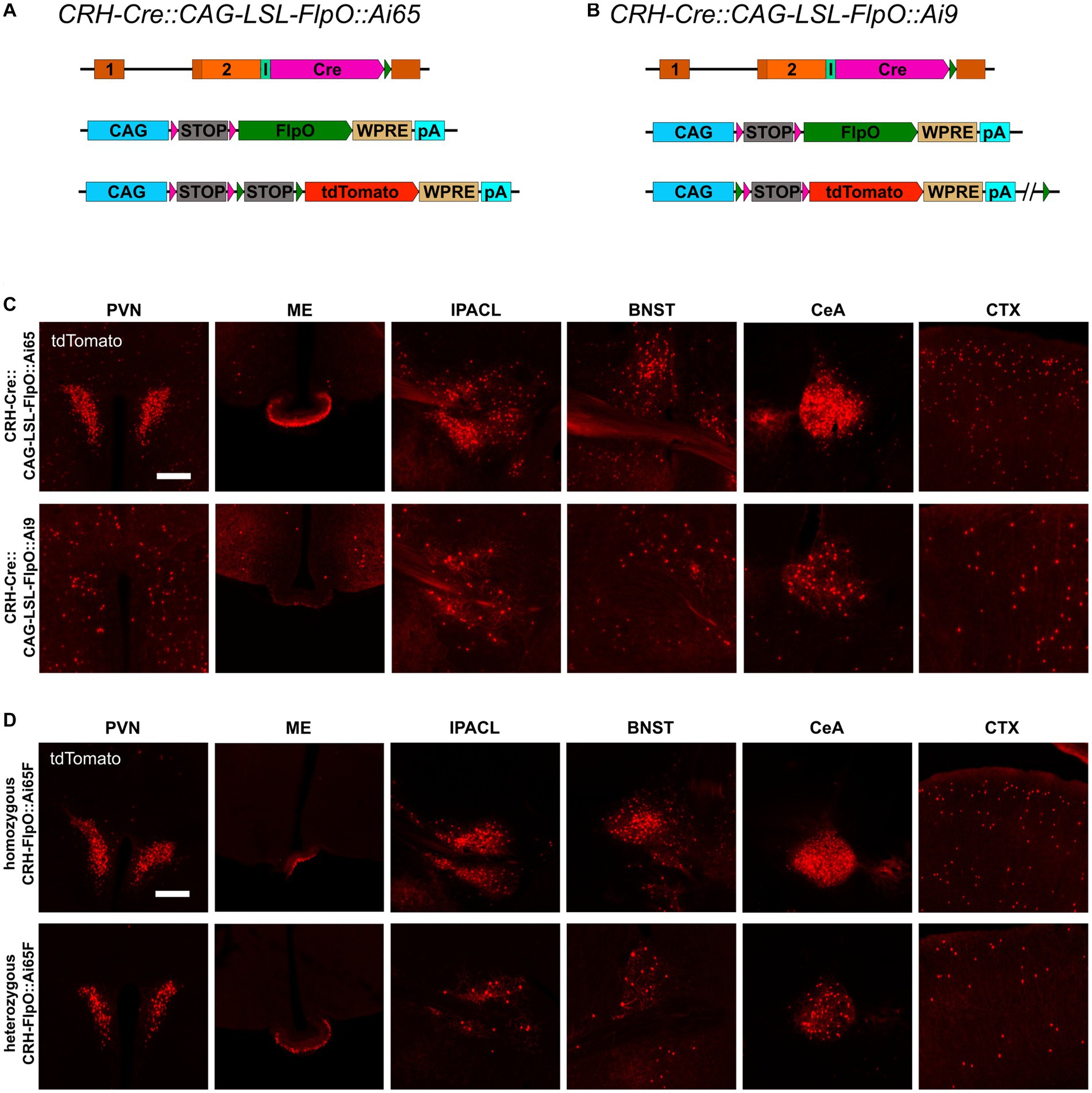
Figure 5. FlpO recombination efficiency depends on strength of driving promoter. (A,B) Schematic illustration of CRH-Cre::CAG-LSL-FlpO::Ai65 and CRH-Cre::CAG-LSL-FlpO::Ai9 reporter mice. (C) Distribution of tdTomato+ cells and fibers in regions of interest in CRH-Cre::CAG-LSL-FlpO::Ai65 (n = 3) and CRH-Cre::CAG-LSL-FlpO::Ai9 (n = 7) mice. (D) Distribution of tdTomato+ cells and fibers in regions of interest in homozygous CRH-FlpO::Ai65F mice (n = 4) and heterozygous CRH-FlpO::Ai65F mice (n = 4). BNST, bed nucleus of the stria terminalis; CeA, central amygdala; CTX, cortex; I, internal ribosomal entry site; IPACL, lateral part of the interstitial nucleus of the anterior commissure; ME, median eminence; pA, BGH polyA signal; PVN, paraventricular nucleus of the hypothalamus. Scale bars, 200 μm.
Taken together, our results demonstrate that the FlpO-FRT system possesses a considerably lower sensitivity compared to the Cre-loxP system. Nevertheless, the attenuated sensitivity can be compensated by a higher expression level allowing the FlpO-FRT system to reach an efficacy which is comparable to the Cre-loxP system.
3.6. CRH-FlpO mice allow the visualization of stress-induced CRH expression
While previous studies have demonstrated the faithful reproduction of endogenous CRH expression when combining CRH-Cre mice (Taniguchi et al., 2011) with Cre-dependent reporters, such as CRH-Cre::Ai9, CRH-Cre::Ai14 and CRH-Cre::Ai32 (Chen et al., 2015; Dedic et al., 2018b; Wang et al., 2021; Chang et al., 2022), Walker et al. (2019) reported that there were no significant changes in the number of tdTomato-expressing neurons in CRH-Cre::Ai14 mice after exposure to stress (Walker et al., 2019). In order to interrogate the suitability of the CRH-FlpO-based reporting system for evaluating the response to stress, we exposed CRH-FlpO::Ai65F mice to acute restraint stress. Analysis of brain sections revealed a considerable increase in tdTomato-expressing CRH neurons in the investigated brain region which was most prominent in the PVN of stressed compared to unstressed CRH-FlpO::Ai65F mice (Figure 6A). These results indicate an upregulation of CRH and concomitant FlpO expression in CRH+ neurons following acute stress suggesting that the CRH-FlpO-based reporter mouse model provides a more dynamic range of recombination that overcomes the reported limitations of the CRH-Cre line (Walker et al., 2019).
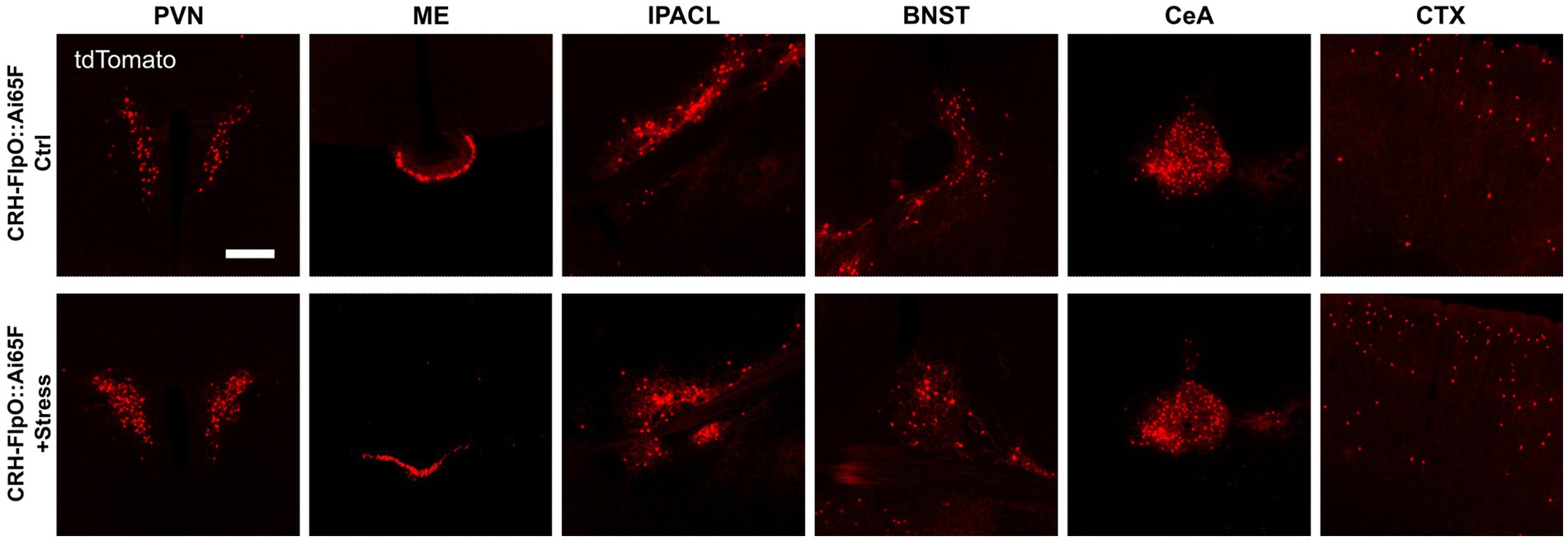
Figure 6. FlpO recombination efficiency is enhanced by acute restraint stress. Comparison of distribution of tdTomato+ cells and fibers in regions of interest in stressed and unstressed CRH-FlpO::Ai65F mice (each n = 5). BNST, bed nucleus of the stria terminalis; CeA, central amygdala; CTX, cortex; I, internal ribosomal entry site; IPACL, lateral part of the interstitial nucleus of the anterior commissure; ME, median eminence; PVN, paraventricular nucleus of the hypothalamus. Scale bar, 200 μm.
4. Discussion
CRH+ neurons are widely distributed throughout the brain and possess a high diversity with regards to their identity and physiology. The limited accessibility of CRH neurons by antibody-based approaches and particularly the quest to disentangle CRH neuron function has raised increasing interest in CRH-specific SSR and reporter mouse lines. While reliable CRH-specific Cre mouse lines have been available for some time (Taniguchi et al., 2011), only recently, the development of a novel CRH-FlpO line (Salimando et al., 2020) has opened up additional opportunities to address the CRH system with increasing precision. To meaningfully apply CRH-specific SSR mouse lines, it is utterly important to understand their capacity to recapitulate endogenous CRH expression. In this study, we therefore compared the recently developed CRH-FlpO line with endogenous CRH expression and a well-established and widely used CRH-Cre line (Taniguchi et al., 2011). We demonstrated that the overall reporting pattern of CRH-FlpO mice is very similar to CRH-Cre mice. The efficiency of FlpO, however, is strongly correlated with its expression level in the analyzed population of CRH+ neurons.
The comparison of FlpO expression with endogenous Crh expression on mRNA level and the evaluation of tdTomato induction in CRH-FlpO::Ai65F compared to CRH-Cre::Ai9 mice both confirmed that the FlpO expression and recombination pattern strongly align with that of endogenous Crh. These results corroborate the high potential of the chosen knock-in strategy to reliably convey properties of the endogenous Crh locus to an integrated transgene.
Nevertheless, the overall intensity of FlpO-activated tdTomato fluorescence appeared somewhat decreased in CRH-FlpO::Ai65F compared to CRH-Cre::Ai9 mice, raising the question whether these discrepancies were caused by differences in their reporting capacity in general. The in-depth analysis of CRH-expressing nuclei revealed that, indeed, the number of tdTomato+ labeled cells and fibers were lower in FlpO- than in Cre-dependent reporter animals, strengthening the hypothesis of a differential reporting capacity. Although CRH-Cre::Ai9 and CRH-FlpO::Ai65 both utilize tdTomato as a reporter in combination with the CAG promoter followed by an identical floxed STOP cassette, the genetic composition in which the tdTomato reporter is embedded differs slightly between the Ai9 and Ai65F lines (Madisen et al., 2010, 2015). Thus, we wondered whether the Ai65F reporter, rather than the FlpO recombinase itself, was responsible for the observed differences. Unexpectedly, the intersectional reporter mice CRH-Cre::CRH-FlpO::Ai65 and CRH-Cre::CRH-FlpO::Ai9 revealed that FlpO was neither able to report all CRH+ cells in the Ai65-based model nor did it deplete tdTomato from Cre-activated cells in the Ai9-based model. These results imply that not the used Ai65F reporter line but FlpO recombination efficiency itself could be the limiting factor. To rule out that the higher cell numbers were not caused by ectopic Cre activity in CRH-Cre mice, the CRH-Venus mouse line as a direct reporter of CRH expression was used to compare tdTomato and Venus expression in the same individual. It is worth noting that Cre-mediated recombination, resulting in cumulative tdTomato expression, effectively reflects the cellular history of Crh gene transcription over the time. In contrast, the Venus+ knock-in allele reports a snapshot of the current protein content above a certain detection threshold, providing a glimpse into the present state rather than the developmental timeline. Despite the differences between the direct and indirect reporting systems and the distinct characteristics of the two applied fluorescent proteins, such as their disparate fluorescent protein lifetimes (Sarkar et al., 2009; Drobizhev et al., 2011), the comparable number of Venus+ cells observed in the analyzed brain regions of CRH-Venus::CRH-Cre::Ai9 and CRH-Venus::CRH-FlpO::Ai65F mice suggests that there is no variation in CRH expression between the two mouse lines. Accordingly, this indicates that the disparity of tdTomato+ cells in CRH-Cre::Ai9 and CRH-FlpO::Ai65F mice may be attributed to the inherent properties of these two recombinases. Indirect reporting via Cre recombinase-mediated activation of tdTomato showed a high degree of co-localization with Venus expression. Interestingly, also a substantial number of tdTomato+/Venus− cells were detectable, which left open the possibility that tdTomato expression in Venus− neurons represent ectopic or legacy expression due to transient activation of CRH and, thus, Cre expression, e.g., during development or in response to experienced stressors, which would not be detected by the Venus reporter. In this regard, homozygous CRH-Venus mice were instructive, as we were able to visualize an increased number of Venus+ cells in all analyzed brain regions compared to heterozygous CRH-Venus mice. This observation suggests that more cells reached the detection threshold, supporting the notion that CRH neurons possess a broad spectrum of expression levels, even within the same brain region. This result additionally confirms that Cre-mediated reporter activation is substantially more sensitive than the detection of Venus via antibody staining, as the tdTomato is driven by the strong and ubiquitously active CAG promoter. Seemingly, a few molecules of Cre are sufficient to activate tdTomato expression. Although a compensatory upregulation of CRH, caused by the de facto knockout of CRH in homozygous CRH-Venus mice, may also have contributed to the higher number of Venus+ neurons, the differences in intensity still confirm a broad diversity of CRH expression levels in respective neurons. In contrast to the PVN, CRH expression in the CeA has been reported to be positively regulated by corticosterone (Makino et al., 1994). Consequentially, CRH inactivation, resulting in low corticosterone levels, should lead to a decreased Venus expression in the CeA. Of note, in our experiments we observed a general increase of Venus expression in all regions analyzed, including the CeA. This observation indicates that homozygosity of the reporter is potentially overriding the hypothesized downregulation of CRH by the low corticosterone levels entailed by the CRH knockout.
These results suggest that, if FlpO-mediated recombination, and therefore reporter efficacy, depends on the expression level of the driving promoter, an enhanced expression should be able to elevate the number of reported cells. Indeed, the combination of FlpO with the CAG promoter, a strong synthetic promoter integrated in the Rosa26 locus, did not only increase reporting efficacy in the CRH-Cre::CAG-LSL-FlpO::Ai65 model to a level comparable to CRH-Cre::Ai9 mice but was also able to vastly decrease the reporting in the CRH-Cre::CAG-LSL-FlpO::Ai9 model. These results also demonstrate that the larger distance between the FRT sites flanking the tdTomato cassette in the Ai9 line (3.9 kb) is not limiting FlpO recombination activity in general (Coppoolse et al., 2005; Liu et al., 2013). Ultimately, homozygous CRH-FlpO::Ai65F mice, expressing FlpO from both Crh alleles, confirmed that the level of FlpO expression is directly correlated with its recombination efficiency. Along these lines, while CRH-Cre males show 100% germline recombination when combined with a floxed allele, the respective frequency observed in CRH-FlpO males is also significantly lower.
Thermo-instability of yeast-derived Flp-related recombinases has been shown to decrease their recombination efficiency in mammalian cells (Buchholz et al., 1996). Despite previous optimization efforts (Buchholz et al., 1998; Raymond and Soriano, 2007) our results clearly demonstrate a persistent difference in recombination efficiency between Cre and FlpO, which particularly matters in low gene expression conditions. Whether this discrepancy is related to differences in protein thermo-stability or the intrinsic reaction rate of Cre and FlpO, remains to be investigated. To what extent other recombinases, such as Dre, can keep up with Cre recombinase efficiency, requires further examination (Anastassiadis et al., 2009; Karimova et al., 2018). The utilized IRES might further aggravate the situation, as IRES-dependent gene expression of the second gene in a bicistronic construct is up to 10-fold lower than cap-dependent expression of the first gene (Mizuguchi et al., 2000; Martin et al., 2006). Today, 2A-related self-cleaving peptides are used to overcome the drawbacks of IRES (Liu et al., 2017). However, 2A peptides leave a couple of amino acids to the N-terminally located protein. This would not be compatible with CRH maturation which involves proteolytic processing by prohormone convertases at predetermined recognition sites which will be disturbed by remnants of 2A peptides (Hook et al., 2008). In fact, this poses a general difficulty for the expression of neuropeptides using multicistronic expression vectors.
Mouse models targeting the CRH system, particularly those employing Cre-dependent reporters, are widely utilized to study the neurobiology of stress and related circuits. On the one hand, the robust efficacy of Cre recombinase provides a reliable tool for investigating the spatial distribution and connectivity of CRH neurons throughout the brain. On the other hand, the high efficacy of Cre recombination seemingly labels almost all cells which have the potential to express CRH in the course of their lifetime. Consequently, the recruitment of new stress-induced tdTomato+ cells in CRH-Cre::Ai9 cells is very limited (Walker et al., 2019). Our results demonstrate that this particular limitation of CRH-Cre-based models can be overcome by using CRH-FlpO mice. Following exposure to acute stress, we observed an increased number of tdTomato+ cells in CRH-FlpO::Ai65F mice. Thus, de novo tdTomato expression can serve as a proxy for stress-responsive neurons characterized by the upregulation of the stress peptide CRH. These results highlight the utility of CRH-FlpO mice as a valuable tool for functional analysis of the CRH system under both physiological and pathological conditions.
In conclusion, we show a difference in the recombination efficiency of Cre and FlpO in CRH+ neurons and demonstrate that the expression level of FlpO contributes to discrepancies in reporter gene activation observed between CRH-Cre and CRH-FlpO mice. These results have strong implications for future experiments using the investigated mouse lines but also for SSR-based approaches in general. (i) When using and comparing FlpO- and Cre-based reporter systems, the strength of the driving promoter has to be taken into account. (ii) In intersectional approaches, the number of reported co-expressing cells is limited by the efficacy of the FlpO. (iii) In approaches involving knockout or overexpression of a specific target gene, the efficacy is highly dependent on the used SSR. These results are essential to interpret and reconcile divergent scientific discoveries which will ultimately advance our comprehension of the physiological role of CRH in the central nervous system.
Data availability statement
The raw data supporting the conclusions of this article will be made available by the authors, without undue reservation.
Ethics statement
The animal study was reviewed and approved by Government of Upper Bavaria, Germany.
Author contributions
CZ, CR, and YD designed and performed all experiments, analyzed and interpreted data, drafted figures, and wrote the manuscript. JZ performed a subset of experiments and reviewed the manuscript. KS and KI provided materials, edited the manuscript and contributed scientific advice. JD designed the project, supervised all experiments and analyses, edited the manuscript, and provided scientific advice, guidance and support. All authors contributed to the article and approved the submitted version.
Funding
The project was supported by the Max Planck Society (JD) and the Federal Ministry of Education and Research (FKZ 01KU1901, JD). JZ and YD received funding from the Chinese Scholarship Council. CR received funding from the International Max Planck Research School for Translational Psychiatry and the Gunter Sachs Donation.
Acknowledgments
The authors thank Stefanie Unkmeir and Sabrina Bauer of the scientific core unit Genetically Engineered Mouse Models for genotyping support; Rainer Stoffel for excellent technical support; Elfi Fesl for proofreading and editing of the manuscript. We thank Minsuk Huyn, Caiying Guo, and Bernado Sabatini for sharing details on the generation of CRH-FlpO mice.
Conflict of interest
The authors declare that the research was conducted in the absence of any commercial or financial relationships that could be construed as a potential conflict of interest.
Publisher’s note
All claims expressed in this article are solely those of the authors and do not necessarily represent those of their affiliated organizations, or those of the publisher, the editors and the reviewers. Any product that may be evaluated in this article, or claim that may be made by its manufacturer, is not guaranteed or endorsed by the publisher.
Supplementary material
The Supplementary material for this article can be found online at: https://www.frontiersin.org/articles/10.3389/fnins.2023.1163462/full#supplementary-material
Footnotes
References
Alon, T., Zhou, L., Perez, C. A., Garfield, A. S., Friedman, J. M., and Heisler, L. K. (2009). Transgenic mice expressing green fluorescent protein under the control of the corticotropin-releasing hormone promoter. Endocrinology 150, 5626–5632. doi: 10.1210/en.2009-0881
Anastassiadis, K., Fu, J., Patsch, C., Hu, S., Weidlich, S., Duerschke, K., et al. (2009). Dre recombinase, like Cre, is a highly efficient site-specific recombinase in E. coli, mammalian cells and mice. Dis. Model. Mech. 2, 508–515. doi: 10.1242/dmm.003087
Arias, A., Manubens-Gil, L., and Dierssen, M. (2022). Fluorescent transgenic mouse models for whole-brain imaging in health and disease. Front. Mol. Neurosci. 15:958222. doi: 10.3389/fnmol.2022.958222
Bloom, F. E., Battenberg, E. L. F., Rivier, J., and Vale, W. (1982). Corticotropin releasing factor (CRF): immunoreactive neurones and fibers in rat hypothalamus. Regul. Pept. 4, 43–48. doi: 10.1016/0167-0115(82)90107-0
Buchholz, F., Angrand, P. O., and Stewart, A. F. (1998). Improved properties of FLP recombinase evolved by cycling mutagenesis. Nat. Biotechnol. 16, 657–662. doi: 10.1038/nbt0798-657
Buchholz, F., Ringrose, L., Angrand, P. O., Rossi, F., and Stewart, A. F. (1996). Different thermostabilities of FLP and Cre recombinases: implications for applied site-specific recombination. Nucleic Acids Res. 24, 4256–4262. doi: 10.1093/nar/24.21.4256
Chang, S., Fermani, F., Lao, C. L., Huang, L., Jakovcevski, M., Di Giaimo, R., et al. (2022). Tripartite extended amygdala-basal ganglia CRH circuit drives locomotor activation and avoidance behavior. Sci. Adv. 8:eabo1023. doi: 10.1126/sciadv.abo1023
Chen, Y., Molet, J., Gunn, B. G., Ressler, K., and Baram, T. Z. (2015). Diversity of reporter expression patterns in transgenic mouse lines targeting corticotropin-releasing hormone-expressing neurons. Endocrinology 156, 4769–4780. doi: 10.1210/en.2015-1673
Coppoolse, E. R., de Vroomen, M. J., van Gennip, F., Hersmus, B. J., and van Haaren, M. J. (2005). Size does matter: Cre-mediated somatic deletion efficiency depends on the distance between the target lox-sites. Plant Mol. Biol. 58, 687–698. doi: 10.1007/s11103-005-7705-7
Dabrowska, J., Hazra, R., Guo, J. D., Dewitt, S., and Rainnie, D. G. (2013). Central CRF neurons are not created equal: phenotypic differences in CRF-containing neurons of the rat paraventricular hypothalamus and the bed nucleus of the stria terminalis. Front. Neurosci. 7:156. doi: 10.3389/fnins.2013.00156
Dedic, N., Chen, A., and Deussing, J. M. (2018a). The CRF family of neuropeptides and their receptors—mediators of the central stress response. Curr. Mol. Pharmacol. 11, 4–31. doi: 10.2174/1874467210666170302104053
Dedic, N., Kuhne, C., Gomes, K. S., Hartmann, J., Ressler, K. J., Schmidt, M. V., et al. (2019). Deletion of CRH from GABAergic forebrain neurons promotes stress resilience and dampens stress-induced changes in neuronal activity. Front. Neurosci. 13:986. doi: 10.3389/fnins.2019.00986
Dedic, N., Kühne, C., Jakovcevski, M., Hartmann, J., Genewsky, A. J., Gomes, K. S., et al. (2018b). Chronic CRH depletion from GABAergic, long-range projection neurons in the extended amygdala reduces dopamine release and increases anxiety. Nat. Neurosci. 21, 803–807. doi: 10.1038/s41593-018-0151-z
Deussing, J. M. (2013). Targeted mutagenesis tools for modelling psychiatric disorders. Cell Tissue Res. 354, 9–25. doi: 10.1007/s00441-013-1708-5
Drobizhev, M., Makarov, N. S., Tillo, S. E., Hughes, T. E., and Rebane, A. (2011). Two-photon absorption properties of fluorescent proteins. Nat. Methods 8, 393–399. doi: 10.1038/nmeth.1596
Ezra-Nevo, G., Volk, N., Ramot, A., Kuehne, C., Tsoory, M., Deussing, J., et al. (2018). Inferior olive CRF plays a role in motor performance under challenging conditions. Transl. Psychiatry 8:107. doi: 10.1038/s41398-018-0145-3
Fadok, J. P., Krabbe, S., Markovic, M., Courtin, J., Xu, C., Massi, L., et al. (2017). A competitive inhibitory circuit for selection of active and passive fear responses. Nature 542, 96–100. doi: 10.1038/nature21047
Gunn, B. G., Sanchez, G. A., Lynch, G., Baram, T. Z., and Chen, Y. (2019). Hyper-diversity of CRH interneurons in mouse hippocampus. Brain Struct. Funct. 224, 583–598. doi: 10.1007/s00429-018-1793-z
He, M., Tucciarone, J., Lee, S., Nigro, M. J., Kim, Y., Levine, J. M., et al. (2016). Strategies and tools for combinatorial targeting of GABAergic neurons in mouse cerebral cortex. Neuron 91, 1228–1243. doi: 10.1016/j.neuron.2016.08.021
Heffner, C. S., Herbert Pratt, C., Babiuk, R. P., Sharma, Y., Rockwood, S. F., Donahue, L. R., et al. (2012). Supporting conditional mouse mutagenesis with a comprehensive Cre characterization resource. Nat. Commun. 3:1218. doi: 10.1038/ncomms2186
Hook, V., Funkelstein, L., Lu, D., Bark, S., Wegrzyn, J., and Hwang, S. R. (2008). Proteases for processing proneuropeptides into peptide neurotransmitters and hormones. Annu. Rev. Pharmacol. Toxicol. 48, 393–423. doi: 10.1146/annurev.pharmtox.48.113006.094812
Itoi, K., Talukder, A. H., Fuse, T., Kaneko, T., Ozawa, R., Sato, T., et al. (2014). Visualization of corticotropin-releasing factor neurons by fluorescent proteins in the mouse brain and characterization of labeled neurons in the paraventricular nucleus of the hypothalamus. Endocrinology 155, 4054–4060. doi: 10.1210/en.2014-1182
Karimova, M., Baker, O., Camgoz, A., Naumann, R., Buchholz, F., and Anastassiadis, K. (2018). A single reporter mouse line for Vika, Flp, Dre, and Cre-recombination. Sci. Rep. 8:14453. doi: 10.1038/s41598-018-32802-7
Keegan, C. E., Herman, J. P., Karolyi, I. J., O’Shea, K. S., Camper, S. A., and Seasholtz, A. F. (1994). Differential expression of corticotropin-releasing hormone in developing mouse embryos and adult brain. Endocrinology 134, 2547–2555. doi: 10.1210/endo.134.6.8194481
Kono, J., Konno, K., Talukder, A. H., Fuse, T., Abe, M., Uchida, K., et al. (2017). Distribution of corticotropin-releasing factor neurons in the mouse brain: a study using corticotropin-releasing factor-modified yellow fluorescent protein knock-in mouse. Brain Struct. Funct. 222, 1705–1732. doi: 10.1007/s00429-016-1303-0
Korecki, A. J., Hickmott, J. W., Lam, S. L., Dreolini, L., Mathelier, A., Baker, O., et al. (2019). Twenty-seven tamoxifen-inducible iCre-driver mouse strains for eye and brain, including seventeen carrying a new inducible-first constitutive-ready allele. Genetics 211, 1155–1177. doi: 10.1534/genetics.119.301984
Krashes, M. J., Shah, B. P., Madara, J. C., Olson, D. P., Strochlic, D. E., Garfield, A. S., et al. (2014). An excitatory paraventricular nucleus to AgRP neuron circuit that drives hunger. Nature 507, 238–242. doi: 10.1038/nature12956
Kubota, Y., Shigematsu, N., Karube, F., Sekigawa, A., Kato, S., Yamaguchi, N., et al. (2011). Selective coexpression of multiple chemical markers defines discrete populations of neocortical GABAergic neurons. Cereb. Cortex 21, 1803–1817. doi: 10.1093/cercor/bhq252
Lemos, J. C., Wanat, M. J., Smith, J. S., Reyes, B. A., Hollon, N. G., Van Bockstaele, E. J., et al. (2012). Severe stress switches CRF action in the nucleus accumbens from appetitive to aversive. Nature 490, 402–406. doi: 10.1038/nature11436
Liu, Z., Chen, O., Wall, J. B. J., Zheng, M., Zhou, Y., Wang, L., et al. (2017). Systematic comparison of 2A peptides for cloning multi-genes in a polycistronic vector. Sci. Rep. 7:2193. doi: 10.1038/s41598-017-02460-2
Liu, J., Willet, S. G., Bankaitis, E. D., Xu, Y., Wright, C. V., and Gu, G. (2013). Non-parallel recombination limits Cre-LoxP-based reporters as precise indicators of conditional genetic manipulation. Genesis 51, 436–442. doi: 10.1002/dvg.22384
Luo, L., Ambrozkiewicz, M. C., Benseler, F., Chen, C., Dumontier, E., Falkner, S., et al. (2020). Optimizing nervous system-specific gene targeting with Cre driver lines: prevalence of germline recombination and influencing factors. Neuron 106, 37–65.e5. doi: 10.1016/j.neuron.2020.01.008
Luo, L., Callaway, E. M., and Svoboda, K. (2018). Genetic dissection of neural circuits: a decade of progress. Neuron 98, 256–281. doi: 10.1016/j.neuron.2018.03.040
Madisen, L., Garner, A. R., Shimaoka, D., Chuong, A. S., Klapoetke, N. C., Li, L., et al. (2015). Transgenic mice for intersectional targeting of neural sensors and effectors with high specificity and performance. Neuron 85, 942–958. doi: 10.1016/j.neuron.2015.02.022
Madisen, L., Zwingman, T. A., Sunkin, S. M., Oh, S. W., Zariwala, H. A., Gu, H., et al. (2010). A robust and high-throughput Cre reporting and characterization system for the whole mouse brain. Nat. Neurosci. 13, 133–140. doi: 10.1038/nn.2467
Makino, S., Gold, P. W., and Schulkin, J. (1994). Corticosterone effects on corticotropin-releasing hormone mRNA in the central nucleus of the amygdala and the parvocellular region of the paraventricular nucleus of the hypothalamus. Brain Res. 640, 105–112. doi: 10.1016/0006-8993(94)91862-7
Marcinkiewcz, C. A., Mazzone, C. M., D’Agostino, G., Halladay, L. R., Hardaway, J. A., DiBerto, J. F., et al. (2016). Serotonin engages an anxiety and fear-promoting circuit in the extended amygdala. Nature 537, 97–101. doi: 10.1038/nature19318
Martin, P., Albagli, O., Poggi, M. C., Boulukos, K. E., and Pognonec, P. (2006). Development of a new bicistronic retroviral vector with strong IRES activity. BMC Biotechnol. 6:4. doi: 10.1186/1472-6750-6-4
Martin, E. I., Ressler, K. J., Jasnow, A. M., Dabrowska, J., Hazra, R., Rainnie, D. G., et al. (2010). A novel transgenic mouse for gene-targeting within cells that express corticotropin-releasing factor. Biol. Psychiatry 67, 1212–1216. doi: 10.1016/j.biopsych.2010.01.026
Mizuguchi, H., Xu, Z., Ishii-Watabe, A., Uchida, E., and Hayakawa, T. (2000). IRES-dependent second gene expression is significantly lower than cap-dependent first gene expression in a bicistronic vector. Mol. Ther. 1, 376–382. doi: 10.1006/mthe.2000.0050
Peng, J., Long, B., Yuan, J., Peng, X., Ni, H., Li, X., et al. (2017). A quantitative analysis of the distribution of CRH neurons in whole mouse brain. Front. Neuroanat. 11:63. doi: 10.3389/fnana.2017.00063
Raymond, C. S., and Soriano, P. (2007). High-efficiency FLP and PhiC31 site-specific recombination in mammalian cells. PLoS One 2:e162. doi: 10.1371/journal.pone.0000162
Refojo, D., Schweizer, M., Kuehne, C., Ehrenberg, S., Thoeringer, C., Vogl, A. M., et al. (2011). Glutamatergic and dopaminergic neurons mediate anxiogenic and anxiolytic effects of CRHR1. Science 333, 1903–1907. doi: 10.1126/science.1202107
Rodríguez, C. I., Buchholz, F., Galloway, J., Sequerra, R., Kasper, J., Ayala, R., et al. (2000). High-efficiency deleter mice show that FLPe is an alternative to Cre-loxP. Nat. Genet. 25, 139–140. doi: 10.1038/75973
Romanov, R. A., Zeisel, A., Bakker, J., Girach, F., Hellysaz, A., Tomer, R., et al. (2017). Molecular interrogation of hypothalamic organization reveals distinct dopamine neuronal subtypes. Nat. Neurosci. 20, 176–188. doi: 10.1038/nn.4462
Salimando, G. J., Hyun, M., Boyt, K. M., and Winder, D. G. (2020). BNST GluN2D-containing NMDA receptors influence anxiety- and depressive-like behaviors and modulate cell-specific excitatory/inhibitory synaptic balance. J. Neurosci. 40, 3949–3968. doi: 10.1523/JNEUROSCI.0270-20.2020
Sanford, C. A., Soden, M. E., Baird, M. A., Miller, S. M., Schulkin, J., Palmiter, R. D., et al. (2017). A central amygdala CRF circuit facilitates learning about weak threats. Neuron 93, 164–178. doi: 10.1016/j.neuron.2016.11.034
Sarkar, P., Koushik, S. V., Vogel, S. S., Gryczynski, I., and Gryczynski, Z. (2009). Photophysical properties of Cerulean and Venus fluorescent proteins. J. Biomed. Opt. 14:034047. doi: 10.1117/1.3156842
Sarkar, J., Wakefield, S., MacKenzie, G., Moss, S. J., and Maguire, J. (2011). Neurosteroidogenesis is required for the physiological response to stress: role of neurosteroid-sensitive GABAA receptors. J. Neurosci. 31, 18198–18210. doi: 10.1523/JNEUROSCI.2560-11.2011
Soden, M. E., Yee, J. X., Cuevas, B., Rastani, A., Elum, J., and Zweifel, L. S. (2022). Distinct encoding of reward and aversion by peptidergic BNST inputs to the VTA. Front. Neural Circuits 16:918839. doi: 10.3389/fncir.2022.918839
Taniguchi, H., He, M., Wu, P., Kim, S., Paik, R., Sugino, K., et al. (2011). A resource of Cre driver lines for genetic targeting of GABAergic neurons in cerebral cortex. Neuron 71, 995–1013. doi: 10.1016/j.neuron.2011.07.026
Ulrich-Lai, Y. M., and Herman, J. P. (2009). Neural regulation of endocrine and autonomic stress responses. Nat. Rev. Neurosci. 10, 397–409. doi: 10.1038/nrn2647
Walker, L. C., Cornish, L. C., Lawrence, A. J., and Campbell, E. J. (2019). The effect of acute or repeated stress on the corticotropin releasing factor system in the CRH-IRES-Cre mouse: a validation study. Neuropharmacology 154, 96–106. doi: 10.1016/j.neuropharm.2018.09.037
Keywords: corticotropin-releasing hormone, CRH, CRF, Cre, Flp, reporter, stress
Citation: Zhao C, Ries C, Du Y, Zhang J, Sakimura K, Itoi K and Deussing JM (2023) Differential CRH expression level determines efficiency of Cre- and Flp-dependent recombination. Front. Neurosci. 17:1163462. doi: 10.3389/fnins.2023.1163462
Edited by:
Nandini Vasudevan, University of Reading, United KingdomReviewed by:
Hideki Miwa, National Center of Neurology and Psychiatry, JapanRyoji Fukabori, Fukushima Medical University, Japan
Copyright © 2023 Zhao, Ries, Du, Zhang, Sakimura, Itoi and Deussing. This is an open-access article distributed under the terms of the Creative Commons Attribution License (CC BY). The use, distribution or reproduction in other forums is permitted, provided the original author(s) and the copyright owner(s) are credited and that the original publication in this journal is cited, in accordance with accepted academic practice. No use, distribution or reproduction is permitted which does not comply with these terms.
*Correspondence: Jan M. Deussing, ZGV1c3NpbmdAcHN5Y2gubXBnLmRl
†These authors have contributed equally to this work