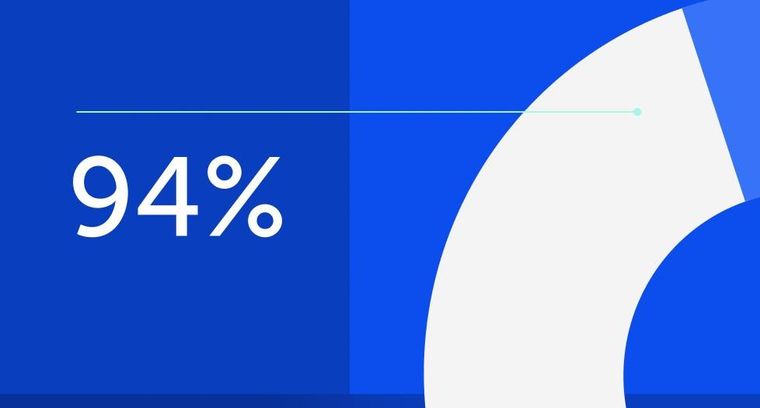
94% of researchers rate our articles as excellent or good
Learn more about the work of our research integrity team to safeguard the quality of each article we publish.
Find out more
REVIEW article
Front. Neurosci., 24 August 2023
Sec. Sleep and Circadian Rhythms
Volume 17 - 2023 | https://doi.org/10.3389/fnins.2023.1152959
This article is part of the Research TopicDevelopment of Circadian Clock Functions, volume IIView all 11 articles
Previous studies suggest the importance of stable circadian environments for fetuses to achieve sound physiology and intrauterine development. This idea is also supported by epidemiological and animal studies, in which pregnant females exposed to repeated shifting of light–dark cycles had increased rates of reproductive abnormalities and adverse pregnancy outcomes. In response to such findings, artificial circadian environments with light–dark (LD) cycles have been introduced to NICUs to promote better physical development of preterm infants. Such LD cycles, however, may not be fully effective for preterm infants who are less than 30 weeks gestational age (WGA) since they are too premature to be adequately responsive to light. Instead, circadian rhythmicity of incubated preterm infants less than 30 WGA may be able to be developed through stimulation of the non-visual senses such as touch and sound.
British author Aldous Huxley used his genius to predict future Neonatal Intensive Care Units (NICUs) in his 1932 fictional novel ‘Brave New World’ (Huxley, 1932). In the NICU he describes, human embryos are incubated in transparent bottles under a dim red light similar to the red colored sunshine we see when we close our eyelids outside on a summer afternoon. The NICU described in his novel also has an artificially-controlled environment high in temperature and humidity, like in some tropical areas.
Huxley’s description of temperature and humidity for the fetuses as being “tropical” matches well with the present settings for preterm infants in modern incubators. In Japan, preterm infants with a weight of <1,000 g are usually accommodated in incubators at a temperature of approximately 34–35°C and a humidity of 60–70% for their first week of life after birth. However, the lighting conditions he states – a constant dim red light – differs from the light–dark cycle which several circadian studies and the American Academy of Pediatrics (AAP) and American Colleges of Obstetrician and Gynecologists (ACOG) recommend for preterm infants in NICUs to encourage circadian entrainment (White, 2013; AAP Committee on Fetus and Newborn and ACOG Committee on Obstetric Practice, 2017). More recent studies have suggested that appropriate multisensory exposures to preterm infants in the NICU may also improve their postnatal development (Pineda et al., 2021; Schmidt Mellado et al., 2022). However, further research on achieving sound physiology and extrauterine development of preterm infants though NICU environment is still required. This review focuses on constructing possibly better NICU environments, particularly paying attention to circadian effects on the body growth of preterm infants.
Among past literature, only two types of studies seem to report the possibility that biological clocks affect fetal body growth. One is an animal study in which rat fetal growth was measured in pregnant mother rats exposed to different lighting conditions. According to the study, the fetal weights in pregnant mother rats exposed to constant light were decreased compared to the fetal weights in pregnant rats exposed to light–dark (LD) cycles (Mendez et al., 2012). In LD conditions, the mother rats had circadian peaks of melatonin at night while the mothers in constant light lost their melatonin circadian rhythms. Moreover, restoration of maternal melatonin rhythmicity via night-time melatonin injection in mother rats exposed to constant light rescued their fetal growth restriction. The authors suggest that maternal circadian rhythm of melatonin leads to fetal circadian rhythm of corticosterone in the adrenal gland and that fetal corticosterone rhythm then contributes to fetal growth. The physiological mechanism linking fetal corticosterone rhythm and body growth, however, remains unclear.
The other type of study is clinical studies in which weight gains of human preterm infants were found to be better in LD conditions than in constant light or dark conditions (Watanabe et al., 2013; Morag and Ohlsson, 2016). Preterm infants are born before term and raised in incubators with a 24-h monitoring system, and an artificial ventilator or an ECMO (extra-corporeal membrane oxygenator), depending on their medical situation. The 24-h monitoring systems tell us that no circadian rhythms exist in the heart rate, respiratory rate, oxygen saturation or EEG of preterm infants (Glotzbach et al., 1995) in non-LD conditions. This is in contrast to human fetuses, who have been reported to have circadian rhythms in heart rates and fetal movements at around 20–22 weeks gestational age (WGA) (de Vries et al., 1987; Lunshof et al., 1998). Previous studies suggest that the circadian systems of fetuses are still premature and dependent on maternal circadian signals such as hormonal or nutritional signals, or on signals through maternal physical activity (Seron-Ferre et al., 2007; Watanabe et al., 2013). The researchers suggest a possibility that LD conditions support the immature biological clocks of preterm infants and premature animals by directly affecting their suprachiasmatic nuclei (SCN) through their photoreceptors, the intrinsically-photosensetive RGCs (ipRGCs) and/or rods, in their eyes, leading to stable circadian rhythms within the SCN (Sekaran et al., 2005; Sernagor, 2005; Schmidt et al., 2008; Van Gelder, 2008; Watanabe et al., 2013; Hazelhoff et al., 2021; Caval-Holme et al., 2022; Polese et al., 2022). Mouse studies have also revealed that the circadian systems of the SCN are disrupted by constant light at the cellular level (Ohta et al., 2005, 2006). The physiological mechanism linking LD conditions and body growth in preterm infants, however, remains unclear and further research in this area is still required.
If these animal and clinical studies present correct results, at least the following three hypotheses can be derived. First, the clinical studies suggest that the circadian mechanism for the growth of preterm infants does not need to depend on maternal signals. Since human preterm infants in incubators, who are completely separated from their mother, are able to achieve appropriate growth in an LD cycle, it can be assumed that maternal signals are not absolutely necessary for the appropriate growth of preterm infants.
Second, clinical studies also suggest that melatonin does not directly contribute to the circadian mechanism for the growth of preterm infants. In humans, melatonin does not have circadian rhythms at birth - at around 40 WGA - and do not exist until approximately 3 months of age (Kennaway, 2000; Rivkees, 2003). Although nighttime increase in melatonin concentration of breast milk in a circadian manner has been reported to occur 9 days after delivery and may support development of the circadian rhythms of infants (Illnerová et al., 1993), their circadian rhythms in sleep–wake only start to be observed after approximately 2 months of age (Rivkees, 2003). Since preterm infants do not have circadian rhythms of melatonin, circadian signals from external or internal factors, such as lighting, nutrition or other hormones must affect the biological clocks of preterm infants. Detection of these, however, is difficult to achieve, as it would require blood samples to be taken from infants every three to 4 h for examination, which is ethically unacceptable (Dupont-Thibodeau and Janvier, 2016).
Third, the animal study with melatonin injections into pregnant rats (Mendez et al., 2012) suggests that the SCN or the organ biological clocks, which modulate circadian phases through melatonin receptors, contribute to the circadian mechanism for the body growth of preterm infants, although melatonin itself does not seem to directly affect the body growth of preterm infants (Gombert and Codoñer-Franch, 2021). To date, two membrane-bound melatonin receptors have been identified and characterized - MT1 and MT2. MT1 receptors are expressed in the brain, cardiovascular system (including peripheral blood vessels, aorta and heart), immune system, testes, ovary, skin, liver, kidney, adrenal cortex, placenta, breast, retina, pancreas and spleen (Dubocovich and Markowska, 2005; Slominski et al., 2005; Fischer et al., 2008; Pandi-Perumal et al., 2008; Slominski et al., 2008). In the brain, the MT1 receptor is predominantly found in the SCN, hypothalamus, cerebellum, hippocampus, substantia nigra and ventral tegmental area (Pandi-Perumal et al., 2008). MT2 has been found in the brain (SCN, hypothalamus and pituitary), retina, immune system, blood vessels, testes, kidney, gastrointestinal tract, mammary glands, adipose tissue, and the skin (Reppert et al., 1995; Roca et al., 1996; Dubocovich and Markowska, 2005; Slominski et al., 2005).
These three hypotheses lead us to a possible conclusion that the retina, the SCN, and the adrenal gland, each of which can be affected by both light and melatonin, may each possibly be part of the pathway for the circadian mechanism for the growth of preterm infants.
Regarding the retina and SCN, the retina has a wide range of efferent visual and non-visual pathways to the brain. The visual pathways, which contribute to both image formation and light–dark detection, are based on the nerve innervations from the rods and cones in the retina. The visual pathways have direct projections to the dorsal lateral geniculate nucleus (dLGN) and superior colliculus (SC). Non-visual pathways, which contribute to only light–dark detection, are composed of intrinsically-photosensetive RGCs (ipRGCs). The non-visual pathways have direct projections to the suprachiasmatic nucleus (SCN), supraoptic nucleus (SON), subparaventricular zone (SPZ), ventral lateral preoptic area (VLPO), lateral hypothalamic area (LH), anterior hypothalamus (AH), medial amygdaloid nucleus (MA), habenula (Hb), bed nucleus of the stria terminalis (BST), dorsal lateral geniculate nucleus (dLGN), ventral lateral geniculate nucleus (vLGN), intergeniculate leaflet (IGL), periaqueductal gray (PAG), olivary pretectal nucleus core (OPN), and superior colliculus (SC). Via the SCN, the non-visual pathways also have indirect projections to the paraventricular nucleus (PVN), dorsomedial hypothalamus (DMH), locus coeruleus (LC), ventral tegmental area (VTA), and septal area (Sept) (Dacey et al., 2005; Hattar et al., 2006; Brown et al., 2010; Noseda et al., 2010; Milosavljevic, 2019; Aranda and Schmidt, 2021).
No direct efferent pathway from the retina to the visual or frontal cortex has been anatomically detected (Gaillard et al., 2013). A human EEG study, however, using light stimulus on blind people, in whom no visual pathway but only the non-visual pathway is supposed to function, suggests that the non-visual pathway may have possible projections to the visual cortex (Vandewalle et al., 2018). A mouse study also indicates that the non-visual pathway can affect cortical synaptogenesis at early developmental stages via the SON and PVN by light-dependent oxytocin secretion (Hu et al., 2022). The ipRGCs-dependent non-visual pathway contributes to various physiological functions such as entrainment of circadian rhythms (Panda et al., 2002; Ruby et al., 2002), sleep–wake regulations (Lupi et al., 2008), pupil constriction (Hattar et al., 2003; Watanabe et al., 2013; Adhikari et al., 2015; Ikeda et al., 2015), alertness (Milosavljevic, 2019), mood (LeGates et al., 2012; Fernandez et al., 2018), learning (Fernandez et al., 2018), and visual perception (Aranda and Schmidt, 2021). In particular, in terms of circadian rhythms and sleep–wake regulation, the SCN directly controls circadian rhythms and the IGL also indirectly entrains circadian rhythms (Hatori and Panda, 2010; Aranda and Schmidt, 2021). In addition, the lateral and posterior hypothalamus, the VLPO, the Hb, and the SPZ are believed to mediate the effects of light on the hypothalamic regulation of sleep, behavior, and other physiological functions (Hatori and Panda, 2010; Aranda and Schmidt, 2021). These facts imply that the retina and/or SCN may contribute to the circadian mechanisms for the body growth of preterm infants.
The adrenal cortex is another candidate partial pathway since light information is conveyed from the SCN to the adrenal gland via the SCN-sympathetic nervous system (Ishida et al., 2005) and MT1 receptors are also detected in the adrenal cortex in adult animals (Torres-Farfan et al., 2003; Richter et al., 2008). Like the development of melatonin circadian rhythms, however, no clear cortisol circadian rhythms are detected in humans until approximately 1 month of age (Ivars et al., 2015, 2017), suggesting that cortisol may not contribute to the circadian mechanism for the body growth of preterm infants until that time.
Overall, the above suggests that we might be able to improve the body growth of preterm infants by providing the SCN and retina with artificial circadian signals, such as a light–dark (LD) cycle, or nighttime-injected melatonin.
The concept of a stable circadian uterine environment having positive effects on fetal development is also supported by clinical studies in which pregnant females exposed to repeated shifting of their LD cycle had increased rates of reproductive abnormalities and adverse pregnancy outcomes such as preterm delivery and particularly low birth weight of offspring. Several epidemiological studies on humans have identified associations between shift work or repeated travel across time zones and reduced fertility (Bisanti et al., 1996) as well as negative pregnancy outcomes, including increased incidence of low birth weight, preterm birth and miscarriage (Cone et al., 1998; Aspholm et al., 1999; Knutsson, 2003). However, whether these adverse outcomes are due to circadian dysregulation or some other lifestyle factor associated with shift work has not been clearly determined.
Also, the study mentioned earlier in which pregnant mother rats were exposed to different lighting conditions reported that exposure of pregnant rats to constant light, which disrupts the circadian environment for fetuses, induced intrauterine growth restriction and also lowered corticosterone production in fetal adrenal glands (Mendez et al., 2012). Other studies using rodent models indicated that repeated shifting of the light–dark cycle of pregnant rats and mice increased rates of miscarriage and induced hyperleptinemia and hyperinsulinemia in offspring lasting into adulthood (Varcoe et al., 2011; Summa et al., 2012). Shifts in rats’ LD cycle are known to transiently disrupt normal phase relationships between the SCN and peripheral biological clocks (Yamazaki et al., 2000), thus desynchronized circadian rhythms, either between central and peripheral maternal tissues or between maternal and fetal tissues (or both), may in part contribute to the adverse effects of chronic environmentally-mediated circadian disruption on pregnancy outcomes.
In theory, an LD cycle might best be introduced to preterm infants at around 26–30 WGA, when the eyes of preterm infants can start to perceive light. Robinson and Fielder (1990) reported that preterm infants can respond to light from 30 weeks postmenstrual age (WPA), equivalent to 30 WGA, based on measurements they had made of the pupillary light reflex (PLR). Another study by Hao and Rivkees (1999) using a baboon model indicated that the SCN in the baboon fetus of an age corresponding to human 24 weeks conceptional age (WCA), approximately equivalent to 26 WGA in the human fetus, was already responsive to light stimuli. The data suggest that preterm infants might start to respond to light from around 26–30 WGA.
In practice, however, an LD cycle would best be introduced to preterm infants just after birth without considering when preterm infants start to perceive light. The reason for this is the large individual difference in when preterm infants start to respond to light. In a study of the development of PLR, Robinson and Fielder reported that the earliest that some preterm infants start to respond to light is at 30 WGA, while others start to respond to light as late as 34 WGA (Robinson and Fielder, 1990), indicating that the onset of the PLR could vary up to 5 weeks between individual preterm infants. It is quite time-consuming and stress inducing to examine preterm infants’ PLR every week to confirm whether the infants have started to respond to light. Instead, if the NICU is always set to an LD cycle, each preterm infant will automatically entrain their biological clock to the LD cycle as their biological clocks start to respond to light without any need for regular PLR checks.
Strict light control of LD cycles in NICUs, however, might not provide the best benefit for preterm infants of <30 WGA being intensively treated for serious medical conditions just after birth. Since they are in a critical physiological state and have minimal sensitivity to light because of the immaturity of their photoreceptors, a constantly lit environment would allow easier performance of intensive medical treatments and cares, operation of medical equipment and observation of the infants’ physiological condition and behavior without any major adverse effect on the rhythm of their biological clock.
To design appropriate lighting conditions for preterm infants in NICUs, we have to take into account at least the following three factors: duration, light intensity, and light wavelengths of light/dark periods.
Previous studies suggest that a 12 L:12D LD cycle would be safe as a NICU lighting cycle. However, no clinical study in human neonatal physiology has investigated an optimum ratio of light/dark period duration for NICUs. Adult studies, however, have demonstrated that humans maintain stable circadian rhythms of behavior and hormonal secretions by adapting to different day lengths (photoperiods) between short day (8 h light period: 16 h dark period (8 L:16D)) and long day (14 L,10D). Compared to the long-day photoperiod, the short-day photoperiod provides us with longer sleep durations, longer nocturnal periods of low rectal temperature, longer nocturnal periods of active melatonin secretion, and higher levels of prolactin and cortisol secretion (Thomas et al., 2002).
Animal studies have also examined the effects of photoperiod during early infancy on development of circadian clocks and other physiological parameters. Ciarleglio et al. (2011) found that mice reared in long days (16 L:8D) exhibited a shorter free-running period of locomotor activity rhythms compared to mice reared in short days (8 L:16D). This is consistent with their results from Per1, a clock gene expression in the SCN, in which perinatal exposure to long days (16 L:8D) induced a narrower Per1 expression waveform and shorter rhythm period compared to short-day (8 L:16D) exposure. Using the same animal model, Ohta and coworkers demonstrated that perinatal exposure to constant light (24 L:0D) leads mice to have disrupted Per1 expression waveform in the developing SCN with desynchronization of the circadian rhythms of individual clock neurons within the SCN, but without reduction in the ability of single neurons to generate circadian rhythms (Ohta et al., 2005, 2006). Another group found that mice reared in constant light (24 L:0D) showed a higher locomotor activity rhythm amplitude and lower levels of vasoactive intestinal peptide (VIP) and arginine vasopressin (AVP) in the SCN compared to mice reared in constant darkness (0 L:24D) or 12 L:12D LD cycles (Smith and Canal, 2009). These results suggest that postnatal light experiences affect the SCN neuronal network that regulates seasonal adaptation by affecting the clock functions and output of SCN neurons. Recently, the same group found that mice raised in constant light showed stronger and shorter-period circadian rhythms of body temperature compared to mice raised in constant darkness and also displayed a greater tendency for depression-like behavior compared to 12 L:12D LD cycle-raised mice (Coleman et al., 2016). Moreover, compared to 12 L:12D LD cycle-raised mice, both constant-light and constant-dark-raised mice showed a decreased glucocorticoid receptor expression in the hippocampus and increased plasma corticosterone concentration at the onset of night. This demonstrates a possibility that the postnatal light environment induces long-term effects on the hypothalamic–pituitary–adrenal axis and circadian system leading to a depressive phenotype in adulthood.
These clinical and animal studies suggest that a 12 L:12D LD cycle would be safe as a NICU lighting cycle for the promotion of sleep and psychological development of preterm infants.
Lighting recommendations by the American Academy of Pediatrics (AAP) and American College of Obstetricians and Gynecologists (ACOG) suggest that the light intensity of NICU illumination should range between 10 and 600 lux to allow continuous assessment of infants, as well as examination of skin color and perfusion (White, 2013; AAP Committee on Fetus and Newborn and ACOG Committee on Obstetric Practice, 2017). The Australian Health Infrastructure Alliance (AHIA) recommends an ambient light range of 100–600 lux [The Australian Health Infrastructure Alliance (AHIA), 2014]. Villalba et al. (2018) also stated that when using individual procedure lights, which are capable of reaching 2000 lux, care should be taken to avoid exposing the neonate’s eyes to high levels of light.
However, none of these guidelines specify optimal intensity ranges for varying gestational ages (Best et al., 2018). For light intensity during night, studies demonstrated that adult humans are responsive to a light intensity of approximately 30 lux and above when assessed by melatonin phase delay response to light and also responsive to a light intensity of approximately 50 lux and above when assessed by the acute melatonin suppressive effects of light (the phase change of melatonin circadian rhythm and suppression of plasma melatonin level have been used as the gold standard to evaluate the effects of light on biological clock) (St. Hilaire et al., 2012). The data indicate that human adults biologically recognize brightness at night when light intensity is 30–50 lux or above. The adult data suggest that the light intensity in NICUs at night should be lower than 30 lux, which preterm infants would not biologically perceive as brightness. The light sensitivity of preterm infants at each gestational age, however, has not been investigated. The light sensitivity of preterm infants can vary depending on their developmental stage. According to our own preterm study (Watanabe et al., 2013), 5 lux does not induce pupillary light reflex in preterm infants between 30 and 38 WGA, suggesting a light intensity of <5 lux, which was recommended for night NICUs by the American Academy of Pediatrics (AAP) and American College of Obstetricians and Gynecologists (ACOG), would be safe for preterm infants of all gestational ages in a NICU lighting environment (White, 2013; AAP Committee on Fetus and Newborn and ACOG Committee on Obstetric Practice, 2017).
For light intensity during daytime, an intensity between 30 and 440 lux would be safe for a NICU lighting environment. As earlier mentioned, adult data on phase change of daily melatonin rhythm suggest that preterm infants are likely to perceive daytime brightness at a light intensity of ≥30 lux. A safe brightness level during daytime would be 440 lux since a US multicenter study demonstrated that no clinical damage was detected in the eyes of preterm infants who were raised in constant light at an average light intensity of 447 lux (Reynolds et al., 1998).
Previous clinical studies indicate that the optimum light wavelengths for light/dark periods depend on the developmental stages of the preterm infants since the three types of visual photoreceptors in the retina (ipRGCs, rods, and cones) each start to function at a different time somewhere between approximately 26 WGA and 1 month after birth (where birth occurs at 40 WGA). Studies of the pupillary light reflex (PLR) of preterm infants revealed that only ipRGCs provide visual perception at the early stages of human development – at approximately 30 WGA – and contributes to the detection of changes in environmental light radiance (Robinson and Fielder, 1990; Hanita et al., 2009; Watanabe et al., 2013). Rods start to function at around 34 WGA and provides image detection (Figure 1; Watanabe et al., 2013; Ikeda et al., 2015). Cones begin to function 1 month after birth (where birth occurs at 40 WGA) (Field et al., 1982; Teller, 1997) and perform image and color detection.
Figure 1. Bed-site illumination before and after introduction of a monochromatic light red filter. (A) In a former NICU, continuous lighting was selected to allow instant reaction to emergency situations such as respiratory and cardiovascular dysfunctions, brain hemorriage, and infections. The background lighting was generally between 50 and 600 lux. (B) Artificial night was achieved by covering the isolette or crib every night with a light filter, which specifically cuts the wavelength of light that the ipRGCs and rods of preterm infants detect. During daytime, the light filter was removed from the isolette (Watanabe et al., 2013).
Adult studies have clarified the properties of the three types of photoreceptors and indicate that ipRGCs detect light of wave lengths up to 580 nm (maximum light sensitivity: 480 nm), rods detect wave lengths up to 610 nm (maximum light sensitivity: 500 nm), and cones detect wave lengths up to 690 nm (maximum light sensitivities of S, M, and L cones: 420, 530, and 560 nm, respectively) (Figure 2; Zaidi et al., 2007). A PLR study of preterm infants of 38 WGA, the age at which most preterm infants are discharged from hospital, demonstrated that they did not respond to 635 nm light but responded to 470 nm (Ikeda et al., 2015). This indicates that cones do not function at that age since 635 nm falls within the wave-length range normally detected by cones. However, since the preterm infants could respond to 470 nm light, this also indicates they are very likely to perceive light through ipRGCs and/or rods, which detect wave lengths up to 580 nm and 610 nm, respectively. Since ipRGCs starts to function earlier than rods and cones and convey light information well enough to control the SCN, the light wave length of <580 nm might be best for use in the design of NICU light–dark cycles.
Figure 2. Spectral properties of human photoreceptors and spectral transmission characteristics of the red filter. (A) Spectral sensitivity of rods, cones and ipRGCs measured by pupil responses to light. Maximum light sensitivity wavelengths of human rods (R), S cones (SW), M cones (MW) and L cones (LW) are approximately 500 nm, 420 nm, 530 nm and 560 nm, respectively. The ipRGCs (depicted as pRGCs in the figure) exhibit their peak sensitivity at around 480 nm. The dashed line on the figure indicates 610 nanometers. Figure cited from Zaidi et al. (2007). White circles indicate the pupil responses to light in a blind woman. (B) Spectral transmission characteristics of the red filter in Figure 1B. Note that the red filter cut light of wave lengths <610 nm perceived by ipRGCs and rods, which function in preterm infants, while allowing wave lengths >610 nm perceived by adult MW and LW to pass through (Watanabe et al., 2013).
Since preterm infants are still in the process of developing the visual system and biological clock, further research should be carefully explored to understand their light sensitivity and entrainment (Hazelhoff et al., 2021; Polese et al., 2022). Light is the most powerful stimuli for the SCN, the biological clock, to synchronize to the environment. However, in the absence of light (i.e., constant darkness), the SCN of human adults can be synchronized by tactile and auditory cues. The physiological conditions of human adults in constant darkness are quite similar to the conditions of preterm infants less than 30 WGA, who do not yet fully respond to light. Human fetuses start to develop their tactile and auditory perceptions at around 16 and 28 WGA, respectively. Their tactile perception starts to function earlier than light perception and their auditory perception starts at approximately the same developmental age as light perception (Humphrey, 1964; Birnholz and Benacerraf, 1983; Lary et al., 1985; Schaal et al., 2004; Table 1).
Despite few dedicated human neonate trials on the influence of tactile contact on the circadian clock, tactile stimulation has been medically performed on preterm infants in NICUs through massage therapy since the 1980s (Figure 3; Field et al., 2006; Badr et al., 2015). Randomized controlled trials (RCTs) on the effects of massage therapy in preterm infants confirm improved weight gain and shortened length of hospital stay (Badr et al., 2015; Niemi, 2017). Previous studies have reported that tactile stimulation leads to higher salivary concentration of both norepinephrine and epinephrine and an increase in the alert state (Kuhn et al., 1991; White-traut et al., 1997), suggesting that tactile stimuli may indeed contribute to the formation of circadian sleep/wake rhythms with more arousal during wake-time period of preterm infants of around 33–34 WGA. In a study of term infants equivalent to 40–42 WGA, massage therapy was reported to enhance their circadian rest-activity rhythms and elevated their nighttime excretion of urinary 6-sulphatoxymelatonin, the melatonin metabolite (Ferber et al., 2002). Massage therapy has also been reported to decrease infant crying and salivary cortisol, suggesting that tactile stimuli may also contribute to reducing psychophysiological stress in preterm infants. This is consistent with an animal study in which elevated cortisol levels caused by maternal deprivation were reduced in response to tactile stimulation (Pauk et al., 1986).
Figure 3. Preterm infant being massaged. Figure cited from Imura et al. (2014).
One means of improving the efficacy of massage therapy is to perform it with the infant in intermittent kangaroo position. It has been reported that massage therapy in intermittent kangaroo position leads to greater daily weight gain compared to massage therapy in incubators (Aldana Acosta et al., 2019). Kangaroo care is well known for decreasing mortality among preterm infants and improving rates of exclusive breast feeding in both developed and developing countries (Boundy et al., 2016). Tactile stimulation provided by kangaroo care, as well as continuous interaction with the parent, may also contribute to the organization of sleep–wake cyclicity and better modulation of the arousal system (Feldman et al., 2002). There is also a possibility that parental circadian body temperature supports the formation of the infant’s own circadian rhythms.
Auditory stimuli can also have a circadian synchronization effect on preterm infants. Auditory stimuli are known to influence the circadian rhythms of a variety of animals. For instance, the behavioral rhythms of the house sparrow (Passer domesticus) can be entrained by conspecific bird songs (Menaker and Eskin, 1966) and even by white noise (Reebs, 1989). Mice also show circadian responses to auditory cues, while other rodents like rats and hamsters do not (Davidson and Menaker, 2003). The efficacy of auditory stimuli entrainment thus varies among species. In human adults, presentation of an auditory stimulus at nighttime can phase shift circadian rhythms of melatonin and core body temperature (Goel, 2005), as well as synchronize activity rhythms in constant darkness but not in LD conditions (Davidson and Menaker, 2003). Preterm infants whose eyes are still immature (< 30 WGA) do not yet have matured ability to perceive light and therefore experience near-constant darkness. In the absence of visual cues, it is possible that such infants’ circadian systems can instead influenced by auditory stimuli.
Regarding sense of smell, no olfactory stimuli have been reported to phase-shift human behavioral or hormonal circadian rhythms. In animals, however, olfactory stimuli are known to affect the circadian clocks of a number of both invertebrate and vertebrate species. A study of social interaction effects on circadian rhythms suggests that olfactory signals can function as synchronizers in fruit flies (Drosophila melanogaster). Olfactory cues (possibly pheromonal) have also been implicated as synchronizers in a mammalian species, the degu (Octogon degus), a diurnal rodent. In addition, a mammary pheromone, aldehyde 2-methylbut-2-enal (2 MB2), was reported to act as a maternal olfactory cue to synchronize the circadian clock of artificially raised European newborn rabbits (Montúfar-Chaveznava et al., 2013).
Among the above mentioned three social cues of touch, sound, and smell, both tactile and auditory stimuli can phase-shift circadian clocks of preterm infants in normal lighting conditions: a light–dark cycle. Since tactile sensitivity is the first of the human senses to appear (at 16 WGA), appropriate tactile stimuli may be able to act as signals to synchronize the circadian clocks of preterm infants before they begin to perceive light at around 26–30 WGA. Auditory stimuli may also potentially support the stability of circadian rhythmicity once infants become sensitive to sound at approximately 28 WGA.
Preterm infants of approximately 22 WGA, the earliest age at which artificial ventilators are able to be applied, can be saved with medical treatments in Japan. Past literature suggests that the circadian rhythms of preterm infants of >26–30 WGA can be safely and effectively supported by LD cycles of 12 L:12D at a light intensity of >50 lux during daytime and < 5 lux during nighttime with wavelengths of up to 580 nm, which the ipRGCs of preterm infants, the first functioning photoreceptors in life, can detect.
The circadian rhythms of preterm infants of <30 WGA, however, are not likely to be fully assisted by LD cycles since their visual photoreceptors are still premature and do not yet function effectively. At such an age, tactile stimuli such as massage therapy in a circadian manner might be an effective way to entrain the circadian rhythms of preterm infants, leading to better body growth, since human tactile perception starts to function at 16 WGA, far before 26 WGA when ipRGCs start to function. In addition, auditory stimuli, such as parents’ voices may also entrain preterm infants’ circadian rhythms since auditory stimuli has been found to affect human circadian clocks in constant darkness, which preterm infants of <30 WGA experience due to immature visual function.
Moreover, a multisensory approach such as the combination of touch, sound and smell might be an important strategy for generating circadian rhythmicity in preterm infants more effectively in the future (Ferber et al., 2002; Neel et al., 2019). Animal neonate studies using rodents such as hamsters, mice and rats, clearly demonstrated that maternal multisensory stimuli such as touch, sound, smell and feeding phase-shifts neonatal circadian rhythms of behavior, hormones, and clock gene expressions. In one such study, newborn rats of mothers kept in an LD cycle were blinded immediately after birth and reared by foster mothers under either LD (LD blind pups) or a reversed light–dark (DL) cycle (DL blind pups). At postnatal day 6, significant phase differences were observed in the circadian gene expression rhythms of the SCN between the LD and DL blind pups, indicating that the two different maternal circadian behavioral patterns with multisensory stimuli in antiphase affected the circadian rhythms of the two different groups of blind pups, respectively, (Ohta et al., 2002). Recent studies on preterm and term infants have also suggested that not only circadian synchronization (entrainment) but also general brain functional connectivity can be affected by multisensory systems when they are exposed to various stimuli in extrauterine environments (Gatti et al., 2012; De Asis-Cruz et al., 2020; Polese et al., 2021; Pineda et al., 2021; Schmidt Mellado et al., 2022).
If, using circadian science, clinicians and researchers are able to redesign the presently employed protocol of kangaroo care, which is composed of parental touch, voice, and smell, the resulting modified form of kangaroo care may be a strong synchronizer for the circadian clock of preterm infants.
TA, RF, MK, K-iT, HG, MH, SO, and HO conceived of the review. TA, RF, MK, HS, YY, K-iT, HG, YS, HAd, HAr, MH, SO, and HO wrote the paper. All authors contributed to the article and approved the submitted version.
This work was supported by Grants-in-Aid for Scientific Research from the Ministry of Education, Culture, Sports, Science and Technology (to TA, RF, MK, YY, K-iT, HG, HAd, HAr, SO, and HO), from the Japan Science and Technology Agency (to HO), and also from the Uhehara Memorial Foundation (to HO).
We thank members of the Japan Developmental Care study group for helpful discussions and comments on the manuscript. We would also like to acknowledge the excellent mentoring by Professor Hiroshi Nishida, who has supported the clinical and research activities of the Japan Developmental Care study group.
The authors declare that the research was conducted in the absence of any commercial or financial relationships that could be construed as a potential conflict of interest.
All claims expressed in this article are solely those of the authors and do not necessarily represent those of their affiliated organizations, or those of the publisher, the editors and the reviewers. Any product that may be evaluated in this article, or claim that may be made by its manufacturer, is not guaranteed or endorsed by the publisher.
AAP Committee on Fetus and Newborn and ACOG Committee on Obstetric Practice (2017). Guidelines for perinatal care, 8th. Itasca: AAP Books.
Adhikari, P., Pearson, C. A., Anderson, A. M., Zele, A. J., and Feigl, B. (2015). Effect of age and refractive error on the melanopsin mediated post-illumination pupil response (PIPR). Sci. Rep. 5:17610. doi: 10.1038/srep17610
The Australian Health Infrastructure Alliance (AHIA) (2014). Australasian health facility guidelines, part B-health facility briefing and planning, 390 – Intensive care-neonatal care/special care nursery. Adelaide, Australia.
Aldana Acosta, A. C., Tessier, R., Charpak, N., and Tarabulsy, G. (2019). Randomised controlled trial on the impact of kinesthetic stimulation on early somatic growth of preterm infants in kangaroo position. Acta Paediatr. 108, 1230–1236. doi: 10.1111/apa.14675
Aranda, M. L., and Schmidt, T. M. (2021). Diversity of intrinsically photosensitive retinal ganglion cells: circuits and functions. Cell. Mol. Life Sci. 78, 889–907. doi: 10.1007/s00018-020-03641-5
Aspholm, R., Lindbohm, M. L., Paakkulainen, H., Taskinen, H., Nurminen, T., and Tiitinen, A. (1999). Spontaneous abortions among Finnish flight attendants. J. Occup. Environ. Med. 41, 486–491. doi: 10.1097/00043764-199906000-00015
Badr, L. K., Abdallah, B., and Kahale, L. (2015). A meta-analysis of preterm infant massage: an ancient practice with contemporary applications. MCN Am. J. Matern. Child Nurs. 40, 344–358. doi: 10.1097/NMC.0000000000000177
Best, K., Bogossian, F., and New, K. (2018). Sensory exposure of neonates in single-room environments (SENSE): an observational study of light. Arch. Dis. Child. Fetal Neonatal Ed. 103, F436–F440. doi: 10.1136/archdischild-2017-312977
Birnholz, J. C., and Benacerraf, B. R. (1983). The development of human fetal hearing. Science 222, 516–518. doi: 10.1126/science.6623091
Bisanti, L., Olsen, J., Basso, O., Thonneau, P., and Karmaus, W. (1996). Shift work and subfecundity: a European multicenter study. European study group on infertility and subfecundity. J. Occup. Environ. Med. 38, 352–358. doi: 10.1097/00043764-199604000-00012
Boundy, E. O., Dastjerdi, R., Spiegelman, D., Fawzi, W. W., Missmer, S. A., Lieberman, E., et al. (2016). Kangaroo mother care and neonatal outcomes: a meta-analysis. Pediatrics 137:e20152238. doi: 10.1542/peds.2015-2238
Brown, T. M., Gias, C., Hatori, M., Keding, S. R., Semo, M., Coffey, P. J., et al. (2010). Melanopsin contributions to irradiance coding in the thalamo-cortical visual system. PLoS Biol. 8:e1000558. doi: 10.1371/journal.pbio.1000558
Caval-Holme, F. S., Aranda, M. L., Chen, A. Q., Tiriac, A., Zhang, Y., Smith, B., et al. (2022). The retinal basis of light aversion in neonatal mice. J. Neurosci. 42, 4101–4115. doi: 10.1523/JNEUROSCI.0151-22.2022
Ciarleglio, C. M., Axley, J. C., Strauss, B. R., Gamble, K. L., and McMahon, D. G. (2011). Perinatal photoperiod imprints the circadian clock. Nat. Neurosci. 14, 25–27. doi: 10.1038/nn.2699
Coleman, G., Gigg, J., and Canal, M. M. (2016). Postnatal light alters hypothalamic-pituitary-adrenal axis function and induces a depressive-like phenotype in adult mice. Eur. J. Neurosci. 44, 2807–2817. doi: 10.1111/ejn.13388
Cone, J. E., Vaughan, L. M., Huete, A., and Samuels, S. J. (1998). Reproductive health outcomes among female flight attendants: an exploratory study. J. Occup. Environ. Med. 40, 210–216. doi: 10.1097/00043764-199803000-00002
Dacey, D. M., Liao, H. W., Peterson, B. B., Robinson, F. R., Smith, V. C., Pokorny, J., et al. (2005). Melanopsin-expressing ganglion cells in primate retina signal colour and irradiance and project to the LGN. Nature 433, 749–754. doi: 10.1038/nature03387
Davidson, A. J., and Menaker, M. (2003). Birds of a feather clock together--sometimes: social synchronization of circadian rhythms. Curr. Opin. Neurobiol. 13, 765–769. doi: 10.1016/j.conb.2003.10.011
De Asis-Cruz, J., Kapse, K., Basu, S. K., Said, M., Scheinost, D., Murnick, J., et al. (2020). Functional brain connectivity in ex utero premature infants compared to in utero fetuses. NeuroImage 219:117043. doi: 10.1016/j.neuroimage.2020.117043
de Vries, J. I., Visser, G. H., Mulder, E. J., and Prechtl, H. F. (1987). Diurnal and other variations in fetal movement and heart rate patterns at 20-22 weeks. Early Hum. Dev. 15, 333–348. doi: 10.1016/0378-3782(87)90029-6
Dubocovich, M. L., and Markowska, M. (2005). Functional MT1 and MT2 melatonin receptors in mammals. Endocrine 27, 101–110. doi: 10.1385/ENDO:27:2:101
Dupont-Thibodeau, A., and Janvier, A. (2016). “When do we become a person and why should it matter to pediatricians?” in Ethical dilemmas for critically III babies. eds. E. Verhagen and A. Janvier (Dordrecht: Springer), 13–24.
Feldman, R., Weller, A., Sirota, L., and Eidelman, A. I. (2002). Skin-to-skin contact (Kangaroo care) promotes self-regulation in premature infants: sleep-wake cyclicity, arousal modulation, and sustained exploration. Dev. Psychol. 38, 194–207. doi: 10.1037/0012-1649.38.2.194
Ferber, S. G., Laudon, M., Kuint, J., Weller, A., and Zisapel, N. (2002). Massage therapy by mothers enhances the adjustment of circadian rhythms to the nocturnal period in full-term infants. J. Dev. Behav. Pediatr. 23, 410–415. doi: 10.1097/00004703-200212000-00003
Fernandez, D. C., Fogerson, P. M., Lazzerini Ospri, L., Thomsen, M. B., Layne, R. M., Severin, D., et al. (2018). Light affects mood and learning through distinct retina-brain pathways. Cells 175, 71–84.e18. doi: 10.1016/j.cell.2018.08.004
Field, T., Diego, M. A., Hernandez-Reif, M., Deeds, O., and Figuereido, B. (2006). Moderate versus light pressure massage therapy leads to greater weight gain in preterm infants. Infant Behav. Dev. 29, 574–578. doi: 10.1016/j.infbeh.2006.07.011
Field, T. M., Woodson, R., Greenberg, R., and Cohen, D. (1982). Discrimination and imitation of facial expression by neonates. Science 218, 179–181. doi: 10.1126/science.7123230
Fischer, T. W., Slominski, A., Tobin, D. J., and Paus, R. (2008). Melatonin and the hair follicle. J. Pineal Res. 14, 1–15. doi: 10.1111/j.1600-079X.2007.00512.x
Gaillard, F., Karten, H. J., and Sauvé, Y. (2013). Retinorecipient areas in the diurnal murine rodent Arvicanthis niloticus: a disproportionally large superior colliculus. J. Comp. Neurol. 521:Spc1. doi: 10.1002/cne.23330
Gatti, M. G., Becucci, E., Fargnoli, F., Fagioli, M., Ådén, U., and Buonocore, G. (2012). Functional maturation of neocortex: a base of viability. J. Matern. Fetal Neonatal Med. 25, 101–103. doi: 10.3109/14767058.2012.664351
Glotzbach, S. F., Edgar, D. M., and Ariagno, R. L. (1995). Biological rhythmicity in preterm infants prior to discharge from neonatal intensive care. Pediatrics 95, 231–237. doi: 10.1542/peds.95.2.231
Goel, N. (2005). Late-night presentation of an auditory stimulus phase delays human circadian rhythms. Am. J. Physiol. Regul. Integr. Comp. Physiol. 289, R209–16. doi: 10.1152/ajpregu.00754.2004
Gombert, M., and Codoñer-Franch, P. (2021). Melatonin in early nutrition: long-term effects on cardiovascular system. Int. J. Mol. Sci. 22:6809. doi: 10.3390/ijms22136809
Hanita, T., Ohta, H., Matsuda, T., and Miyazawa, H. (2009). Monitoring preterm infants' vision development with light-only melanopsin is functional. J. Pediatr. 155, 596–596.e1. doi: 10.1016/j.jpeds.2009.03.005
Hao, H., and Rivkees, S. A. (1999). The biological clock of very premature primate infants is responsive to light. Proc. Natl. Acad. Sci. U. S. A. 96, 2426–2429. doi: 10.1073/pnas.96.5.2426
Hatori, M., and Panda, S. (2010). The emerging roles of melanopsin in behavioral adaptation to light. Trends Mol. Med. 16, 435–446. doi: 10.1016/j.molmed.2010.07.005
Hattar, S., Kumar, M., Park, A., Tong, P., Tung, J., Yau, K. W., et al. (2006). Central projections of melanopsin-expressing retinal ganglion cells in the mouse. J. Comp. Neurol. 497, 326–349. doi: 10.1002/cne.20970
Hattar, S., Lucas, R. J., Mrosovsky, N., Thompson, S., Douglas, R. H., Hankins, M. W., et al. (2003). Melanopsin and rod-cone photoreceptive systems account for all major accessory visual functions in mice. Nature 424, 76–81. doi: 10.1038/nature01761
Hazelhoff, E. M., Dudink, J., Meijer, J. H., and Kervezee, L. (2021). Beginning to see the light: lessons learned from the development of the circadian system for optimizing light conditions in the neonatal intensive care unit. Front. Neurosci. 15:634034. doi: 10.3389/fnins.2021.634034
Hu, J., Shi, Y., Zhang, J., Huang, X., Wang, Q., Zhao, H., et al. (2022). Melanopsin retinal ganglion cells mediate light-promoted brain development. Cells 185, 3124–3137.e15. doi: 10.1016/j.cell.2022.07.009
Humphrey, T. (1964). Embryology of the central nervous system: with some correlations with functional development. Ala. J. Med. Sci. 1, 60–64.
Huxley, A., Herman Finkelstein Collection (Library of Congress). (1932). Brave new world, a novel. Garden City, NY. Doubleday, Doran & company, Inc.
Ikeda, T., Ishikawa, H., Shimizu, K., Asakawa, K., and Goseki, T. (2015). Pupillary size and light reflex in premature infants. Neuroophthalmology 39, 175–178. doi: 10.3109/01658107.2015.1055363
Illnerová, H., Buresová, M., and Presl, J. (1993). Melatonin rhythm in human milk. J. Clin. Endocrinol. Metab. 77, 838–841. doi: 10.1210/jcem.77.3.8370707
Imura, M., Suzuki, C., and Fuse, K. (2014). “Massage thrapy” in Standard developmental care. eds. H. Nishida, S. Ohgi, T. Watanabe, and H. Kihara (Osaka: Medicus Shuppan), 231–248.
Ishida, A., Mutoh, T., Ueyama, T., Bando, H., Masubuchi, S., Nakahara, D., et al. (2005). Light activates the adrenal gland: timing of gene expression and glucocorticoid release. Cell Metab. 2, 297–307. doi: 10.1016/j.cmet.2005.09.009
Ivars, K., Nelson, N., Theodorsson, A., Theodorsson, E., Ström, J. O., and Mörelius, E. (2015). Development of salivary cortisol circadian rhythm and reference intervals in full-term infants. PLoS One 10:e0129502. doi: 10.1371/journal.pone.0129502
Ivars, K., Nelson, N., Theodorsson, A., Theodorsson, E., Ström, J. O., and Mörelius, E. (2017). Development of salivary cortisol circadian rhythm in preterm infants. PLoS One 12:e0182685. doi: 10.1371/journal.pone.0182685
Kennaway, D. J. (2000). Melatonin and development: physiology and pharmacology. Semin. Perinatol. 24, 258–266. doi: 10.1053/sper.2000.8594
Knutsson, A. (2003). Health disorders of shift workers. Occup. Med. (Lond.) 53, 103–108. doi: 10.1093/occmed/kqg048
Kuhn, C. M., Schanberg, S. M., Field, T., Symanski, R., Zimmerman, E., Scafidi, F., et al. (1991). Tactile-kinesthetic stimulation effects on sympathetic and adrenocortical function in preterm infants. J. Pediatr. 119, 434–440. doi: 10.1016/s0022-3476(05)82059-1
Lary, S., Briassoulis, G., de Vries, L., Dubowitz, L. M., and Dubowitz, V. (1985). Hearing threshold in preterm and term infants by auditory brainstem response. J. Pediatr. 107, 593–599. doi: 10.1016/s0022-3476(85)80030-5
LeGates, T. A., Altimus, C. M., Wang, H., Lee, H. K., Yang, S., Zhao, H., et al. (2012). Aberrant light directly impairs mood and learning through melanopsin-expressing neurons. Nature 491, 594–598. doi: 10.1038/nature11673
Lunshof, S., Boer, K., Wolf, H., van Hoffen, G., Bayram, N., and Mirmiran, M. (1998). Fetal and maternal diurnal rhythms during the third trimester of normal pregnancy: outcomes of computerized analysis of continuous twenty-four-hour fetal heart rate recordings. Am. J. Obstet. Gynecol. 178, 247–254. doi: 10.1016/s0002-9378(98)80008-2
Lupi, D., Oster, H., Thompson, S., and Foster, R. G. (2008). The acute light-induction of sleep is mediated by OPN4-based photoreception. Nat. Neurosci. 11, 1068–1073. doi: 10.1038/nn.2179
Menaker, M., and Eskin, A. (1966). Entrainment of circadian rhythms by sound in Passer domesticus. Science 154, 1579–1581. doi: 10.1126/science.154.3756.1579
Mendez, N., Abarzua-Catalan, L., Vilches, N., Galdames, H. A., Spichiger, C., Richter, H. G., et al. (2012). Timed maternal melatonin treatment reverses circadian disruption of the fetal adrenal clock imposed by exposure to constant light. PLoS One 7:e42713. doi: 10.1371/journal.pone.0042713
Milosavljevic, N. (2019). How does light regulate mood and behavioral state? Clocks Sleep 1, 319–331. doi: 10.3390/clockssleep1030027
Montúfar-Chaveznava, R., Trejo-Muñoz, L., Hernández-Campos, O., Navarrete, E., and Caldelas, I. (2013). Maternal olfactory cues synchronize the circadian system of artificially raised newborn rabbits. PLoS One 8:e74048. doi: 10.1371/journal.pone.0074048
Morag, I., and Ohlsson, A. (2016). Cycled light in the intensive care unit for preterm and low birth weight infants. Cochrane Database Syst. Rev. 2020:CD006982. doi: 10.1002/14651858.CD006982.pub4
Neel, M. L., Yoder, P., Matusz, P. J., Murray, M. M., Miller, A., Burkhardt, S., et al. (2019). Randomized controlled trial protocol to improve multisensory neural processing, language and motor outcomes in preterm infants. BMC Pediatr. 19:81. doi: 10.1186/s12887-019-1455-1
Niemi, A. K. (2017). Review of randomized controlled trials of massage in preterm infants. Children (Basel). 4:21. doi: 10.3390/children4040021
Noseda, R., Kainz, V., Jakubowski, M., Gooley, J. J., Saper, C. B., Digre, K., et al. (2010). A neural mechanism for exacerbation of headache by light. Nat. Neurosci. 13, 239–245. doi: 10.1038/nn.2475
Ohta, H., Honma, S., Abe, H., and Honma, K. (2002). Effects of nursing mothers on rPer1 and rPer2 circadian expressions in the neonatal rat suprachiasmatic nuclei vary with developmental stage. Eur. J. Neurosci. 15, 1953–1960. doi: 10.1046/j.1460-9568.2002.02016.x
Ohta, H., Mitchell, A. C., and McMahon, D. G. (2006). Constant light disrupts the developing mouse biological clock. Pediatr. Res. 60, 304–308. doi: 10.1203/01.pdr.0000233114.18403.66
Ohta, H., Yamazaki, S., and McMahon, D. G. (2005). Constant light desynchronizes mammalian clock neurons. Nat. Neurosci. 8, 267–269. doi: 10.1038/nn1395
Panda, S., Sato, T. K., Castrucci, A. M., Rollag, M. D., DeGrip, W. J., Hogenesch, J. B., et al. (2002). Melanopsin (Opn4) requirement for normal light-induced circadian phase shifting. Science 298, 2213–2216. doi: 10.1126/science.1076848
Pandi-Perumal, S. R., Trakht, I., Srinivasan, V., Spence, D. W., Maestroni, G. J., Zisapel, N., et al. (2008). Physiological effects of melatonin: role of melatonin receptors and signal transduction pathways. Prog. Neurobiol. 85, 335–353. doi: 10.1016/j.pneurobio.2008.04.001
Pauk, J., Kuhn, C. M., Field, T. M., and Schanberg, S. M. (1986). Positive effects of tactile versus kinesthetic or vestibular stimulation on neuroendocrine and ODC activity in maternally-deprived rat pups. Life Sci. 39, 2081–2087. doi: 10.1016/0024-3205(86)90359-0
Pineda, R., Roussin, J., Kwon, J., Heiny, E., Colditz, G., and Smith, J. (2021). Applying the RE-AIM framework to evaluate the implementation of the supporting and enhancing NICU sensory experiences (SENSE) program. BMC Pediatr. 21:137. doi: 10.1186/s12887-021-02594-3
Polese, D., Fagioli, M., Virgili, F., and Nastro, P. F. (2021). Something must happen before first breath. BMC Med. Ethics. 22:57. doi: 10.1186/s12910-021-00624-4
Polese, D., Riccio, M. L., Fagioli, M., Mazzetta, A., Fagioli, F., and Parisi, P. (2022). The Newborn's reaction to light as the determinant of the Brain's activation at human birth. Front. Integr. Neurosci. 16:933426. doi: 10.3389/fnint.2022.933426
Reebs, S. G. (1989). Acoustical entrainment of circadian activity rhythms in house sparrows: constant light is not necessary. Ethology 80, 172–181.
Reppert, S. M., Godson, C., Mahle, C. D., Weaver, D. R., Slaugenhaupt, S. A., and Gusella, J. F. (1995). Molecular characterization of a second melatonin receptor expressed in human retina and brain: the Mel 1b melatonin receptor. Proc. Natl. Acad. Sci. U. S. A. 92, 8734–8738. doi: 10.1073/pnas.92.19.8734
Reynolds, J. D., Hardy, R. J., Kennedy, K. A., Spencer, R., van Heuven, W. A., and Fielder, A. R. (1998). Lack of efficacy of light reduction in preventing retinopathy of prematurity. Light reduction in retinopathy of prematurity (LIGHT-ROP) cooperative group. N. Engl. J. Med. 338, 1572–1576. doi: 10.1056/NEJM199805283382202
Richter, H. G., Torres-Farfan, C., Garcia-Sesnich, J., Abarzua-Catalan, L., Henriquez, M. G., Alvarez-Felmer, M., et al. (2008). Rhythmic expression of functional MT1 melatonin receptors in the rat adrenal gland. Endocrinology 149, 995–1003. doi: 10.1210/en.2007-1009
Rivkees, S. A. (2003). Developing circadian rhythmicity in infants. Pediatrics 112, 373–381. doi: 10.1542/peds.112.2.373
Rivkees, S. A., Mayes, L., Jacobs, H., and Gross, I. (2004). Rest-activity patterns of premature infants are regulated by cycled lighting. Pediatrics 113, 833–839. doi: 10.1542/peds.113.4.833
Robinson, J., and Fielder, A. R. (1990). Pupillary diameter and reaction to light in preterm neonates. Arch. Dis. Child. 65, 35–38. doi: 10.1136/adc.65.1_Spec_No.35
Roca, A. L., Godson, C., Weaver, D. R., and Reppert, S. M. (1996). Structure, characterization, and expression of the gene encoding the mouse Mel1a melatonin receptor. Endocrinology 137, 3469–3477. doi: 10.1210/endo.137.8.8754776
Ruby, N. F., Brennan, T. J., Xie, X., Cao, V., Franken, P., Heller, H. C., et al. (2002). Role of melanopsin in circadian responses to light. Science 298, 2211–2213. doi: 10.1126/science.1076701
Schaal, B., Hummel, T., and Soussignan, R. (2004). Olfaction in the fetal and premature infant: functional status and clinical implications. Clin. Perinatol. 31, 261–285. doi: 10.1016/j.clp.2004.04.003
Schmidt Mellado, G., Pillay, K., Adams, E., Alarcon, A., Andritsou, F., Cobo, M. M., et al. (2022). The impact of premature extrauterine exposure on infants' stimulus-evoked brain activity across multiple sensory systems. Neuroimage Clin. 33:102914. doi: 10.1016/j.nicl.2021.102914
Schmidt, T. M., Taniguchi, K., and Kofuji, P. (2008). Intrinsic and extrinsic light responses in melanopsin-expressing ganglion cells during mouse development. J. Neurophysiol. 100, 371–384. doi: 10.1152/jn.00062.2008
Sekaran, S., Lupi, D., Jones, S. L., Sheely, C. J., Hattar, S., Yau, K. W., et al. (2005). Melanopsin-dependent photoreception provides earliest light detection in the mammalian retina. Curr. Biol. 15, 1099–1107. doi: 10.1016/j.cub.2005.05.053
Sernagor, E. (2005). Retinal development: second sight comes first. Curr. Biol. 15, R556–R559. doi: 10.1016/j.cub.2005.07.004
Seron-Ferre, M., Valenzuela, G. J., and Torres-Farfan, C. (2007). Circadian clocks during embryonic and fetal development. Birth Defects Res. C. Embryo. Today 81, 204–214. doi: 10.1002/bdrc.20101
Slominski, A., Fischer, T. W., Zmijewski, M. A., Wortsman, J., Semak, I., Zbytek, B., et al. (2005). On the role of melatonin in skin physiology and pathology. Endocrine 27, 137–148. doi: 10.1385/ENDO:27:2:137
Slominski, A., Tobin, D. J., Zmijewski, M. A., Wortsman, J., and Paus, R. (2008). Melatonin in the skin: synthesis, metabolism and functions. Trends Endocrinol. Metab. 19, 17–24. doi: 10.1016/j.tem.2007.10.007
Smith, L., and Canal, M. M. (2009). Expression of circadian neuropeptides in the hypothalamus of adult mice is affected by postnatal light experience. J. Neuroendocrinol. 21, 946–953. doi: 10.1111/j.1365-2826.2009.01914.x
St. Hilaire, M. A., Gooley, J. J., Khalsa, S. B., Kronauer, R. E., Czeisler, C. A., and Lockley, S. W. (2012). Human phase response curve to a 1 h pulse of bright white light. J. Physiol. 590, 3035–3045. doi: 10.1113/jphysiol.2012.227892
Summa, K. C., Vitaterna, M. H., and Turek, F. W. (2012). Environmental perturbation of the circadian clock disrupts pregnancy in the mouse. PLoS One 7:e37668. doi: 10.1371/journal.pone.0037668
Teller, D. Y. (1997). First glances: the vision of infants. The Friedenwald lecture. Invest. Ophthalmol. Vis. Sci. 38, 2183–2203.
Thomas, L., Purvis, C. C., Drew, J. E., Abramovich, D. R., and Williams, L. M. (2002). Melatonin receptors in human fetal brain: 2-[(125)I]iodomelatonin binding and MT1 gene expression. J. Pineal Res. 33, 218–224. doi: 10.1034/j.1600-079X.2002.02921.x
Torres-Farfan, C., Richter, H. G., Rojas-García, P., Vergara, M., Forcelledo, M. L., Valladares, L. E., et al. (2003). mt1 melatonin receptor in the primate adrenal gland: inhibition of adrenocorticotropin-stimulated cortisol production by melatonin. J. Clin. Endocrinol. Metab. 88, 450–458. doi: 10.1210/jc.2002-021048
Vandewalle, G., van Ackeren, M. J., Daneault, V., Hull, J. T., Albouy, G., Lepore, F., et al. (2018). Light modulates oscillatory alpha activity in the occipital cortex of totally visually blind individuals with intact non-image-forming photoreception. Sci. Rep. 8:16968. doi: 10.1038/s41598-018-35400-9
Van Gelder, R. N. (2008). Non-visual photoreception: sensing light without sight. Curr. Biol. 18, R38–9. doi: 10.1016/j.cub.2007.11.027
Varcoe, T. J., Wight, N., Voultsios, A., Salkeld, M. D., and Kennaway, D. J. (2011). Chronic phase shifts of the photoperiod throughout pregnancy programs glucose intolerance and insulin resistance in the rat. PLoS One 6:e18504. doi: 10.1371/journal.pone.0018504
Villalba, A., Monteoliva, J. R. R., and Pattini, A. (2018). A dynamic performance analysis of passive sunlight control strategies in a neonatal intensive care unit. Light. Res. Technol. 50, 191–204. doi: 10.1177/1477153516656225
Watanabe, S., Akiyama, S., Hanita, T., Li, H., Nakagawa, M., Kaneshi, Y., et al. (2013). Designing artificial environments for preterm infants based on circadian studies on pregnant uterus. Front. Endocrinol. 4:113. doi: 10.3389/fendo.2013.00113
White, R. D. (2013). Recommended NICU design standards and the physical environment of the NICU. J. Perinatol. 33:S1. doi: 10.1038/jp.2013.9
White-Traut, R. C., Nelson, M. N., Silvestri, J. M., Cunningham, N., and Patel, M. (1997). Responses of preterm infants to unimodal and multimodal sensory intervention. Pediatr. Nurs. 23:193.
Yamazaki, S., Numano, R., Abe, M., Hida, A., Takahashi, R., Ueda, M., et al. (2000). Resetting central and peripheral circadian oscillators in transgenic rats. Science 288, 682–685. doi: 10.1126/science.288.5466.682
Keywords: designing artificial environments, NICUs, preterm infants, circadian rhythm, multisensory cares
Citation: Arimitsu T, Fukutomi R, Kumagai M, Shibuma H, Yamanishi Y, Takahashi K-i, Gima H, Seto Y, Adachi H, Arai H, Higuchi M, Ohgi S and Ohta H (2023) Designing artificial circadian environments with multisensory cares for supporting preterm infants’ growth in NICUs. Front. Neurosci. 17:1152959. doi: 10.3389/fnins.2023.1152959
Received: 28 January 2023; Accepted: 26 July 2023;
Published: 24 August 2023.
Edited by:
Daisuke Ono, Nagoya University, JapanReviewed by:
Daniela Polese, Sant'Andrea University Hospital, ItalyCopyright © 2023 Arimitsu, Fukutomi, Kumagai, Shibuma, Yamanishi, Takahashi, Gima, Seto, Adachi, Arai, Higuchi, Ohgi and Ohta. This is an open-access article distributed under the terms of the Creative Commons Attribution License (CC BY). The use, distribution or reproduction in other forums is permitted, provided the original author(s) and the copyright owner(s) are credited and that the original publication in this journal is cited, in accordance with accepted academic practice. No use, distribution or reproduction is permitted which does not comply with these terms.
*Correspondence: Hidenobu Ohta, aGlkZW9odGFAbWVkLmFraXRhLXUuYWMuanA=
Disclaimer: All claims expressed in this article are solely those of the authors and do not necessarily represent those of their affiliated organizations, or those of the publisher, the editors and the reviewers. Any product that may be evaluated in this article or claim that may be made by its manufacturer is not guaranteed or endorsed by the publisher.
Research integrity at Frontiers
Learn more about the work of our research integrity team to safeguard the quality of each article we publish.