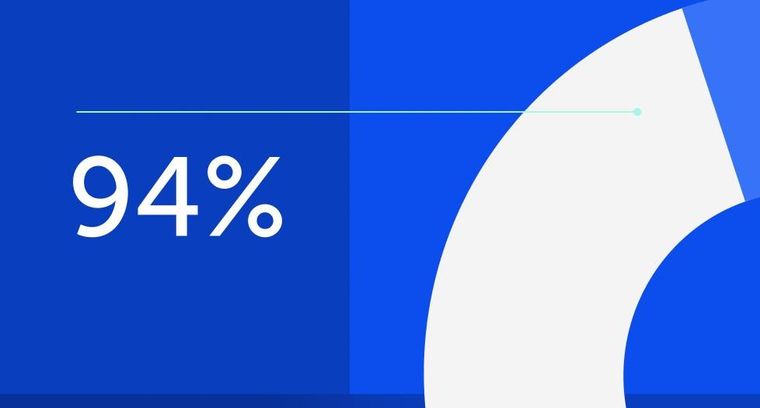
94% of researchers rate our articles as excellent or good
Learn more about the work of our research integrity team to safeguard the quality of each article we publish.
Find out more
SYSTEMATIC REVIEW article
Front. Neurosci., 29 March 2023
Sec. Neuropharmacology
Volume 17 - 2023 | https://doi.org/10.3389/fnins.2023.1147194
This article is part of the Research TopicNovel Therapeutic Target and Drug Discovery for Neurological Diseases, Volume IIView all 33 articles
Background: There was still no effective treatment for traumatic brain injury (TBI). Recently, many preclinical studies had shown promising efficacy of extracellular vesicles (EVs) from various cell sources. Our aim was to compare which cell-derived EVs were most effective in treating TBI through a network meta-analysis.
Methods: We searched four databases and screened various cell-derived EVs for use in preclinical studies of TBI treatment. A systematic review and network meta-analysis were conducted for two outcome indicators, modified Neurological Severity Score (mNSS) and Morris Water Maze (MWM), and they were ranked by the surface under the cumulative ranking curves (SUCRA). Bias risk assessment was performed with SYRCLE. R software (version 4.1.3, Boston, MA, USA) was used for data analysis.
Results: A total of 20 studies were included in this study, involving 383 animals. Astrocyte-derived extracellular vesicles (AEVs) ranked first in response to mNSS at day 1 (SUCRA: 0.26%), day 3 (SUCRA: 16.32%), and day 7 (SUCRA: 9.64%) post-TBI. Extracellular vesicles derived from mesenchymal stem cells (MSCEVs) were most effective in mNSS assessment on day 14 (SUCRA: 21.94%) and day 28 (SUCRA: 6.26%), as well as MWM’s escape latency (SUCRA: 6.16%) and time spent in the target quadrant (SUCRA: 86.52%). The result of mNSS analysis on day 21 showed that neural stem cell-derived extracellular vesicles (NSCEVs) had the best curative effect (SUCRA: 6.76%).
Conclusion: AEVs may be the best choice to improve early mNSS recovery after TBI. The efficacy of MSCEVs may be the best in the late mNSS and MWM after TBI.
Systematic review registration: https://www.crd.york.ac.uk/prospero/, identifier CRD42023377350.
Traumatic brain injury (TBI) is a kind of neurological or even motor dysfunction caused by brain trauma, which can be life-threatening in severe cases (Menon et al., 2010). In the epidemiological report of 2016, more than 8 million TBI patients will lead to Years Lived with Disability (YLDs), which brings a huge burden to society (Badhiwala et al., 2019). Therefore, the treatment of TBI has great significance for individuals, societies and countries.
To date, the recommended treatment for TBI includes hypertonic therapy, neurotrophic drug therapy, surgical decompression, and nutritional support (Maas et al., 2017, 2022). However, these methods do not work well. Therefore, new therapies are urgently needed to enhance the efficacy and improve the prognosis. As early as 20 years ago, there were studies showing that cell therapy has good promise (Mahmood et al., 2001; Chopp and Li, 2002). Therefore, a large number of studies have promoted the progress of cell therapy (Harvey and Chopp, 2003; Mahmood et al., 2004; Osanai et al., 2012). However, tumorigenicity and immune rejection are still troubling researchers when cell therapy is used in clinical studies (Liu et al., 2021).
Cell free therapy, an emerging therapy that is expected to replace cell therapy, has attracted a large number of researchers’ attention (Dutta et al., 2021; Pischiutta et al., 2022). The so-called cell-free therapy is the treatment of various diseases with products secreted by cells (also known as extracellular vesicles) (Han et al., 2022). Extracellular vesicle (EV) is a vesicle with membrane structure and rich contents produced by cells through endocytosis or exocytosis (van Niel et al., 2018). It has multiple subtypes and plays an irreplaceable role in the physiological and pathological processes of various diseases (Kalra et al., 2016). Specific information can be referred to the relevant review (Colombo et al., 2014; Thery et al., 2018).
In recent years, a large number of studies have shown that EVs can effectively treat various neurodegenerative diseases, including TBI (Guedes et al., 2020; Mot et al., 2022). There have also been conventional meta-analysis showing that EVs can improve neurological dysfunction after TBI (Muhammad et al., 2022). However, in the study of cell therapy, it has been found that the efficacy of different cells varies (Xu et al., 2022). This makes it all the more important to select a particular cell to treat a particular disease. To date, no studies have shown which cell-derived EVs work better. Therefore, the purpose of this study is to provide a cell source with the best curative effect by conducting a network meta-analysis of the efficacy of EVs from different cell sources in TBI, so as to provide ideas for preclinical research and promote the progress of cell-free therapies into clinical research.
The literature search was conducted in strict accordance with the expanded statement of the Preferred Reporting Items for Systematic Reviews and Meta-Analyses statement (PRISMA) (Hutton et al., 2015). The PROSPERO database registration number was CRD42023377350. A comprehensive search was conducted on PubMed, Ovid-Embase, The Cochrane Library, and Web of Science databases until November 10, 2022. The search strategy was based on English keywords and free words. Details could be found in Supplementary Table 1.
Two researchers independently screened the study based on the inclusion and exclusion criteria established in strict accordance with the PICOS principle. Controversial studies were evaluated by a third investigator for resolution. Preclinical studies of EVs for the treatment of TBI were included regardless of the cellular origin of EVs. The outcome measures included modified Neurological Severity Score (mNSS) and Morris Water Maze (MWM) (Gold et al., 2013). The mNSS had been used to assess neural function after TBI, and MWM had been used to test learning and memory ability in animals. Exclusion criteria included: (1) in vitro and clinical studies; (2) Reviews, letters and comments; (3) There was no mNSS or MWM outcome indicator.
The data were extracted independently by two specialized researchers and examined by another trained researcher. A third researcher was also involved in discussing and resolving their conflicting data. Information extracted included authors, year, country, groups, sample size, animal species, sex, age, weight, type of model, EVs source, immunocompatibility, EVs separation method, time, dose, frequency and method of administration, and outcome indicators. The raw data for outcome metrics was extracted using GetData Graph Digitizer (version 2.24). Two evaluators independently assessed the quality of each included study using the Systematic Review Center for Laboratory Animal Experimentation (SYRCLE) bias risk assessment tool1 (Hooijmans et al., 2014). Review Manager 5.4.1 was used to draw bias risk maps and summaries.
The network meta-analysis based on the Bayesian approach was performed using R software (version 4.1.3, Boston, MA, USA) for statistical analysis (Jansen et al., 2008). The nodes in the network diagram represent the intervention and the control group, and the sample size is represented by the node size. The line between nodes represents a direct comparison between two nodes, and the number of studies of direct comparison can be seen in the width of the line. When Markov Chain Monte Carlo (MCMC) method was used, the annealing times were 20,000, and the iteration times were 50,000 (Gressani et al., 2022). The continuous variables were evaluated using mean difference (MD) and 95% confidence interval (95% CI), and the corresponding results were presented in the form of forest map. League tables were used to show the results of direct and indirect comparisons. The surface under the cumulative ranking curves (SUCRA) was used to evaluate the ranking of different interventions (Salanti et al., 2011). The tightly controlled inclusion and exclusion criteria make this network meta-analysis maintain good similarity (Salanti, 2012). The consistency of data can be judged by comparing the deviance information criterion (DIC) values of different models (Zhu et al., 2014). The smaller the difference value is, the higher the consistency is. By definition, there are no local inconsistencies in a closed-loop study (White et al., 2012). Heterogeneity between studies could be assessed by the I2 value. Non-statistically significant heterogeneity results showed an I2 value <50%. The publication bias was checked by using funnel plot and Egger’s regression test. Testing for publication bias was not required for meta-analyses with fewer than 10 studies (Stang, 2010).
A total of 1,479 studies were obtained through a comprehensive search of relevant databases. 128 duplicates were excluded. After preliminary screening of 1313 studies by title and abstract, 38 studies remained after excluding 1313 studies. After reading the full text, 18 studies without relevant outcome indicators were excluded. Finally, a total of 20 eligible studies were included in the network meta-analysis (Figure 1).
The included studies were published between 2015 and 2022, with three from the United States (15%), one from Iran (5%), and the rest all from China (80%). The study included a sample size of 383 animals. Seven of the studies involved mice (35%) and 13 involved rats (65%). All used male animals (90%), except for one study (5%) in which the animals were not gender-specific and one (5%) in which female rats were used. The ages ranged from 6 to 14 weeks, with mice weighing 20–25 g and rats weighing 200–352.8 g. The majority of the studies (80%) chose the CCI model, while a small percentage (10%) chose the WD model. Only one study chose the FPI model (5%). There’s even one study (5%) that didn’t exactly describe how it’s made. Cell sources for EVs were mesenchymal stem cell (MSC, 65%), astrocyte (20%), microglia (10%) and neural stem cell (NSC, 5%). Allogeneic (50%) and xenogeneic (50%) were used for EVs cell origin studies. The majority of studies (65%) used traditional ultracentrifugation methods to isolate EVs. Some (25%) used EVs isolation kits. EVs had also been obtained using ultrafiltration (5%) and density-gradient ultracentrifugation (5%) methods. Most studies (80%) gave the EVs within 1 day of injury. One study (5%) administered drugs 35 days after injury. Another study (5%) compared 1, 4, and 7 days of administration. However, there were also two studies (10%) that did not specify the timing of dosing. The majority of studies (85%) were dosed in a single dose of 3−200 μg. One study (5%) quantified EVs dose using particle count. Another study (5%) quantified the number of EVs-derived cells. Of course, one study (5%) did not specify dose or frequency. EVs was administered intravenously (65%), intraventricular (30%), and retroorbital (5%). Table 1 summarized the research characteristics. By comparing the DIC values of different models (Supplementary Table 2), it was found that the consistency of all outcome indexes was high.
The mNSS neurological function assessment at day 1 after TBI, a total of 12 studies were eligible (Zhang et al., 2015, 2017, 2020; Li et al., 2019; Ni et al., 2019; Chen et al., 2020; Long et al., 2020; Xu et al., 2020; He et al., 2021; Zhang W. et al., 2021; Zhang Y. et al., 2021; Cui et al., 2022). The network diagram (Figure 2A) showed three interventions and control comparisons, including astrocyte-derived extracellular vesicles (AEVs), microglia-derived extracellular vesicles (MEVs) and mesenchymal stem cell-derived extracellular vesicles (MSCEVs). The results of forest map (Figure 2B) showed that, compared with control [MD: −2.7, 95%CI: (−3.6, −1.6)], MEVs [MD: −2.6, 95%CI: (−4.2, −0.71)] and MSCEVs [MD: −2.4, 95%CI: (−3.5, −1.2)], AEVs were more effective. The result of SUCRA (Figure 2C) showed that AEVs (0.26%) had the best curative effect, followed by MSCEVs (52.32%), MEVs (65.98%) and Control (81.44%). For mNSS, the lower the score, the better the recovery of neural function. Therefore, the closer the SUCRA value is to 0%, the better the efficacy is. The funnel plot (Figure 2D) and Egger’s test (Table 2, p-value = 0.0986) showed that publication bias did not exist.
Figure 2. Comparison of the efficacy of extracellular vesicles (EVs) from different cell sources in modified Neurological Severity Score (mNSS) at day 1 post-traumatic brain injury (TBI). (A) Network plot; (B) Forest plot; (C) Surface under the cumulative ranking curves (SUCRA); (D) Funnel plot. The dotted lines represent 95% confidence interval (95% CI). AEVs, astrocyte-derived extracellular vesicles; MEVs, microglia-derived extracellular vesicles; MSCEVs, mesenchymal stem cell-derived extracellular vesicles; NSCEVs, neural stem cell-derived extracellular vesicles.
Six studies qualified for the mNSS neurological function assessment at day 3 after TBI (Li et al., 2019; Ni et al., 2019; Chen et al., 2020; Long et al., 2020; He et al., 2021; Cui et al., 2022). The network diagram (Figure 3A) showed three interventions and control comparisons, including AEVs, MEVs, and MSCEVs. No statistically significant comparison was found between the results of forest map (Figure 3B). The result of SUCRA (Figure 3C) showed that AEVs (16.32%) had the best curative effect, followed by MSCEVs (27.67%), MEVs (73.47%), and Control (82.54%).
Figure 3. Comparison of the efficacy of extracellular vesicles (EVs) from different cell sources in modified Neurological Severity Score (mNSS) at day 3 post-traumatic brain injury (TBI). (A) Network plot; (B) Forest plot; (C) Surface under the cumulative ranking curves (SUCRA). AEVs, astrocyte-derived extracellular vesicles; MEVs, microglia-derived extracellular vesicles; MSCEVs, mesenchymal stem cell-derived extracellular vesicles; NSCEVs, neural stem cell-derived extracellular vesicles.
The mNSS neurological function assessment at day 7 after TBI, a total of 15 studies were eligible (Zhang et al., 2015, 2017, 2020; Li et al., 2019; Ni et al., 2019; Yang et al., 2019; Chen et al., 2020; Long et al., 2020; Xu et al., 2020; He et al., 2021; Zhang W. et al., 2021; Zhang Y. et al., 2021; Abedi et al., 2022; Cui et al., 2022; Zhang et al., 2022). The network diagram (Figure 4A) showed four interventions and control comparisons, including AEVs, MEVs, neural stem cell-derived extracellular vesicles (NSCEVs) and MSCEVs. The results of forest map (Figure 4B) showed that compared with the control, AEVs [MD: −3.1, 95% CI: (−4.2, −2.0)], MSCEVs [MD: −2.8, 95% CI: (−3.4, −2.1)] and NSCEVs [MD: −2.0, 95% CI: (−3.9, −0.14)] could effectively improve the neurological function after TBI. Compared with MEVs, AEVs [MD: −3.9, 95% CI: (−6.2, −1.5)] and MSCEVs [MD: −3.5, 95% CI: (−5.7, −1.3)] were more beneficial to neurological recovery after TBI. The result of SUCRA (Figure 4C) showed that AEVs (9.64%) had the best curative effect, followed by MSCEVs (23.87%), NSCEVs (42.76%), Control (80.2%), and MEVs (93.53%). The funnel plot (Figure 4D) and Egger’s test (Table 2, p-value = 0.0926) showed that publication bias did not exist.
Figure 4. Comparison of the efficacy of extracellular vesicles (EVs) from different cell sources in mNSS at day 7 post-traumatic brain injury (TBI). (A) Network plot; (B) Forest plot; (C) Surface under the cumulative ranking curves (SUCRA); (D) Funnel plot. The dotted lines represent 95% confidence interval (95% CI). AEVs, astrocyte-derived extracellular vesicles; MEVs, microglia-derived extracellular vesicles; MSCEVs, mesenchymal stem cell-derived extracellular vesicles; NSCEVs, neural stem cell-derived extracellular vesicles.
The mNSS neurological function assessment at day 14 after TBI, a total of 14 studies were eligible (Zhang et al., 2015, 2017, 2020; Li et al., 2019; Ni et al., 2019; Yang et al., 2019; Chen et al., 2020; Long et al., 2020; Xu et al., 2020; He et al., 2021; Zhang Y. et al., 2021; Abedi et al., 2022; Cui et al., 2022; Zhang et al., 2022). The network diagram (Figure 5A) showed four interventions and control comparisons, including AEVs, MEVs, NSCEVs, and MSCEVs. The results of forest map (Figure 5B) showed that, compared with the control, AEVs [MD: −2.7, 95% CI: (−4.1, −1.2)], MSCEVs [MD: −2.7, 95% CI: (−3.4, −2.1)] and NSCEVs [MD: −2.5, 95% CI: (−4.5, −0.45)] could effectively improve the neurological function after TBI. Compared with MEVs, AEVs [MD: −2.7, 95% CI: (−5.3, −0.017)] and MSCEVs [MD: −2.7, 95% CI: (−5.0, −0.46)] significantly improved mNSS neural function scores. The results of SUCRA (Figure 5C) showed that MSCEVs (21.94%) had the best curative effect, followed by AEVs (25.11%), NSCEVs (30.32%), MEVs (85.5%), and Control (87.12%). The results of funnel plot (Figure 5D) and Egger’s test (Table 2, p-value = 0.0289) indicated that publication bias might exist. Therefore, we found four “missing” studies on the right side of the funnel plot by the trim and fill analysis method (Figure 5E).
Figure 5. Comparison of the efficacy of extracellular vesicles (EVs) from different cell sources in modified Neurological Severity Score (mNSS) at day 14 post-traumatic brain injury (TBI). (A) Network plot; (B) Forest plot; (C) Surface under the cumulative ranking curves (SUCRA); (D) Funnel plot; (E) Funnel plot by the trim and fill analysis method. The dotted lines represent 95% confidence interval (95% CI). AEVs, astrocyte-derived extracellular vesicles; MEVs, microglia-derived extracellular vesicles; MSCEVs, mesenchymal stem cell-derived extracellular vesicles; NSCEVs, neural stem cell-derived extracellular vesicles.
The mNSS neurological function assessment at day 21 after TBI, a total of 10 studies were eligible (Zhang et al., 2015, 2017, 2020; Li et al., 2019; Yang et al., 2019; Chen et al., 2020; Zhang Y. et al., 2021; Abedi et al., 2022; Cui et al., 2022; Zhang et al., 2022). The network diagram (Figure 6A) showed three interventions and control comparisons, including MEVs, NSCEVs, and MSCEVs. The results of forest map (Figure 6B) showed that MSCEVs [MD: −3.1, 95% CI: (−4.2, −2.0)] and NSCEVs [MD: −4.5, 95% CI: (−7.6, −1.4)] could effectively improve the neurological function after TBI compared with the control. The results of SUCRA (Figure 6C) showed that NSCEVs (6.76%) had the best curative effect, followed by MSCEVs (28.82%), MEVs (80.07%), and Control (84.35%). The funnel plot (Figure 6D) and Egger’s test (Table 2, p-value = 0.3315) showed that publication bias did not exist.
Figure 6. Comparison of the efficacy of extracellular vesicles (EVs) from different cell sources in modified Neurological Severity Score (mNSS) at day 21 post-traumatic brain injury (TBI). (A) Network plot; (B) Forest plot; (C) Surface under the cumulative ranking curves (SUCRA); (D) Funnel plot. The dotted lines represent 95% confidence interval (95% CI). AEVs, astrocyte-derived extracellular vesicles; MEVs, microglia-derived extracellular vesicles; MSCEVs, mesenchymal stem cell-derived extracellular vesicles; NSCEVs, neural stem cell-derived extracellular vesicles.
A total of 8 studies were eligible for mNSS neurological function assessment at day 28 after TBI (Zhang et al., 2015, 2017, 2020; Yang et al., 2019; Chen et al., 2020; Zhang Y. et al., 2021; Abedi et al., 2022; Zhang et al., 2022). The network diagram (Figure 7A) showed two interventions and control comparisons, including NSCEVs and MSCEVs. The results of forest map (Figure 7B) showed that MSCEVs [MD: −3.0, 95% CI: (−4.0, −1.9)] had significant curative effect compared with the control. The results of SUCRA (Figure 7C) showed that MSCEVs (6.26%) had the best curative effect, followed by NSCEVs (50.5%) and Control (93.24%).
Figure 7. Comparison of the efficacy of extracellular vesicles (EVs) from different cell sources in modified Neurological Severity Score (mNSS) at day 28 post- traumatic brain injury (TBI). (A) Network plot; (B) Forest plot; (C) Surface under the cumulative ranking curves (SUCRA). AEVs, astrocyte-derived extracellular vesicles; MEVs, microglia-derived extracellular vesicles; MSCEVs, mesenchymal stem cell-derived extracellular vesicles; NSCEVs, neural stem cell-derived extracellular vesicles.
We extracted data from the last day of escape latency in MWM for evaluation. A total of 13 studies were included in the network meta-analysis (Kim et al., 2016; Zhang et al., 2017, 2020; Li et al., 2019; Wang and Han, 2019; Yang et al., 2019; Ge et al., 2020; Liu et al., 2020; Xu et al., 2020; Zhang W. et al., 2021; Zhang Y. et al., 2021; Zhang et al., 2022; Zhuang et al., 2022). The network diagram (Figure 8A) showed three interventions and control comparisons, including AEVs, MEVs, and MSCEVs. The results of forest map (Figure 8B) showed that AEVs [MD: −10.0, 95% CI: (−19.0, −1.5)] and MSCEVs [MD: −15.0, 95% CI: (−19.0, −11.0)] reduced the escape latency time more than the control group, indicating that they could effectively improve the spatial memory ability after TBI. Compared with MEVs, MSCEVs [MD: −12.0, 95% CI: (−22.0, −2.4)] showed better efficacy. The shorter the escape latency time is, the better the spatial memory ability is. Therefore, the closer the SUCRA value is to 0%, the better the efficacy is. The results of SUCRA (Figure 8C) showed that MSCEVs (6.16%) had the best curative effect, followed by AEVs (31.06%), MEVs (71.97%), and Control (90.82%). The funnel plot (Figure 8D) and Egger’s test (Table 2, p-value = 0.2978) showed that publication bias did not exist.
Figure 8. Comparison of the efficacy of extracellular vesicles (EVs) from different cell sources in escape latency of Morris Water Maze (MWM). (A) Network plot; (B) Forest plot; (C) Surface under the cumulative ranking curves (SUCRA); (D) Funnel plot. The dotted lines represent 95% confidence interval (95% CI). AEVs, astrocyte-derived extracellular vesicles; MEVs, microglia-derived extracellular vesicles; MSCEVs, mesenchymal stem cell-derived extracellular vesicles; NSCEVs, neural stem cell-derived extracellular vesicles.
We extracted data from the last day of time spent in the goal quadrant in MWM for evaluation. A total of 14 studies were included in the network meta-analysis (Zhang et al., 2015, 2017, 2020; Kim et al., 2016; Li et al., 2019; Wang and Han, 2019; Yang et al., 2019; Ge et al., 2020; Liu et al., 2020; Xu et al., 2020; Zhang W. et al., 2021; Zhang Y. et al., 2021; Zhang et al., 2022; Zhuang et al., 2022). The network diagram (Figure 9A) shows three interventions and control comparisons, including AEVs, MEVs and MSCEVs. The results of forest map (Figure 9B) showed that AEVs [MD: 8.3, 95% CI: (1.2, 16.0)] and MSCEVs [MD: 9.3, 95% CI: (6.1, 13.0)] spent more time in the target quadrant than the control group, indicating that they could effectively improve the spatial memory ability after TBI. Compared with MEVs, MSCEVs [MD: 8.7, 95% CI: (0.91, 16.0)] showed better efficacy. The longer the time spent in the target quadrant, the better the spatial memory. Therefore, the closer the SUCRA value is to 100%, the better the efficacy is. The results of SUCRA (Figure 9C) showed that MSCEVs (86.52%) had the best curative effect, followed by AEVs (77.2%), MEVs (21.63%), and Control (14.65%). The funnel plot (Figure 9D) and Egger’s test (Table 2, p-value = 0.0820) showed that publication bias did not exist.
Figure 9. Comparison of the efficacy of extracellular vesicles (EVs) from different cell sources in time spent in the goal quadrant in Morris Water Maze (MWM). (A) Network plot; (B) Forest plot; (C) Surface under the cumulative ranking curves (SUCRA); (D) Funnel plot. The dotted lines represent 95% confidence interval (95% CI). AEVs, astrocyte-derived extracellular vesicles; MEVs, microglia-derived extracellular vesicles; MSCEVs, mesenchymal stem cell-derived extracellular vesicles; NSCEVs, neural stem cell-derived extracellular vesicles.
The results of the risk of bias assessment in this study were shown in Supplementary Figures 1A, B. Only two studies (10%) did not account for random sequence generation. About 50% of the studies were low risk for selection bias, performance bias, and detection bias. However, for all the included studies, it was not clear whether they processed incomplete outcome data. Therefore, all studies had the unclear risk of attrition bias. Moreover, only one study (5%) reported low risk, and the rest (95%) were unclear. For other bias, nine studies (45%) showed low risk of bias and five studies (25%) showed unclear risk of bias. However, there was a high risk of bias in six studies (30%). All in all, the included studies were of average quality.
A total of 20 studies and 383 animals were included in this network meta-analysis. Our results suggested that AEVs, MEVs, NSCEVs, and MSCEVs were all effective in mNSS at day 1, 3, and 7 after TBI. Among them, the efficacy of AEVs was the most significant. Astrocytes are the most abundant glial cells in the central nervous system, and they play a crucial role in many neurodegenerative diseases (Hara et al., 2017; Escartin et al., 2021). After TBI, they play a role in maintaining the homeostasis of the internal environment and transmitting signals and communication between cells (Li et al., 2022; Yuan and Wu, 2022). In recent years, EV has been widely studied as a popular intercellular communication tool. It contains lipids, proteins, RNA and DNA and is responsible for its functional diversity (Colombo et al., 2014; Thery et al., 2018). A large number of studies have also shown that AEVs can be used to treat TBI, which can not only reduce the lesion volume, but also reduce cell apoptosis (Wang and Han, 2019; Long et al., 2020). In the early stages of TBI, astrocytes play an important role, which explains why AEVs are the most effective (Perez et al., 2017). However, due to the non-renewable nature of astrocytes, with the aggravation of secondary damage, the efficacy of AEVs diminishes with its depletion (Ridet et al., 1997; Sofroniew and Vinters, 2010). Therefore, it is not surprising that the efficacy of AEVs did not rank first in the late stage of TBI. However, NSCEVs ranked first in mNSS at day 21 after TBI. This result should be considered with caution as only one of the included studies used NSCEVs. Although studies have shown that NSCEVs can enhance the function of endogenous NSC after TBI, thus contributing to the recovery of neural function (Sun et al., 2020). However, the result of network meta-analysis showed NSCEVs were statistically significant compared with the control group, and there was no statistical difference between direct and indirect comparisons with other interventions in mNSS at day 21 after TBI. Therefore, more studies are needed to prove the efficacy of NSCEVs.
Moreover, AEVs, MEVs, NSCEVs, and MSCEVs were equally effective in mNSS at day 14 and 28 after TBI. Among them, MSCEVs ranked first in efficacy. As for the results of the network meta-analysis of the MWM test, AEVs, MEVs, and MSCEVs all had some effect. Among them, MSCEVs ranked first in the outcome analysis of escape latency and spent time in the target quadrant. As is well-known, MSC is a pluripotent stem cell with differentiation ability and therapeutic potential, which has been widely studied in various fields (Das et al., 2019; Shao et al., 2019). Stem cell therapies have also been used in clinical trials (Shahror et al., 2020). The efficacy of MSC in neurodegenerative diseases has also been widely reported (Peruzzaro et al., 2019; Köhli et al., 2021). However, although MSC has also been found to improve neurological function after TBI, inhibit the expression of apoptosis-related proteins Bax and Caspase−3, and also inhibit the secretion of pro-inflammatory and anti-inflammatory factors (Zhang et al., 2013; Shahror et al., 2019). Although MSC is easy to isolate and has homing function (Hsuan et al., 2016). However, it still has some unavoidable shortcomings such as tumorigenicity, easy contamination and immune rejection (Liu et al., 2021). Luckily, the discovery of MSCEVs is a bright spot. After TBI, the use of MSCEVs significantly improved the motor function and spatial memory ability of TBI animals (Patel et al., 2018; Moss et al., 2021). At the cellular level, MSCEVs can not only reduce apoptosis and neuroinflammatory response, but also promote the growth of progenies (Wen et al., 2022). One study has also shown that MSCEVs play a neuroprotective role by regulating the interaction between astrocytes and neurons through the PI3K/AKT signaling pathway (Turovsky et al., 2022). MSC contributes to the functional recovery of various endogenous cells and plays a more indirect role through continuous retention in the body (Andrzejewska et al., 2021). It also has the potential to differentiate into various cells, helping to replenish the cells consumed after TBI, thus exerting its curative effect. Therefore, it is natural that its efficacy ranked first in the late evaluation of mNSS and MWM. For another MEVs with therapeutic potential, it has been found to be useful in the treatment of early TBI. And they can also significantly inhibit inflammation. As resident macrophages of the central nervous system, microglia play an important role in the early stage of injury (Liddelow et al., 2017). The early inflammatory cascade reaction will affect axon regeneration, and microglia will also produce two polarization states of M1 and M2, affecting the secretion of inflammatory factors (Kigerl et al., 2009). One study has shown that EVs derived from microglia can promote axon regeneration through overexpression of miRNA, thus improving motor function after TBI (Zhao et al., 2021). Although MEVs were statistically significant compared to controls, they were less effective than other cell-derived EVs. It is possible that the intense early inflammatory response requires a large number of microglia, which are in short supply. It is also possible that some MEVs are affected by M1 microglia and turn into EVs that are not effective, thus playing a harmful role. Therefore, more studies are needed to further support the conclusion that MEVs can be used in the treatment of TBI.
Our Bayesian network meta-analysis provided a basis for future research by comparing EVs from different cell sources for TBI to select the EVs with the most significant efficacy. Our study not only assessed neural function, but also analyzed spatial memory ability. It is helpful for researchers to choose EVs which is more suitable for their research direction. To explore the mechanism of early efficacy, the use of MEVs and AEVs as intervention may be a better choice. MSCEVs and NSCEVs may be the best solution for researchers who want to further study the mechanism of neural function recovery after injury, or who want to compare the efficacy of stem cells and EVs. While providing strong evidence for preclinical research, it can reduce the detours in the transformation to clinical research.
There were some limitations to our Bayesian network meta-analysis. First, the number of studies included and the sample size were too small. The number of studies on some outcome measures was so small that some interventions had only been studied in one study, compromising the confidence of the results. Secondly, the quality of the included studies was uneven, and there were still studies with a high risk of bias. This might have something to do with the types of studies included. The unsatisfactory quality of preclinical research was still common due to the lack of systematic guidelines and inconsistent laboratory conditions. Moreover, the outcome indexes were all subjective results. The two outcome indicators, mNSS and MWM, were not objective enough, which might increase the risk of bias. Finally, SUCRA results used to assess efficacy rankings might have their own limitations affecting the results.
The results of our network meta-analysis suggested that AEVs might be the best option in the treatment of early TBI in mNSS. In the later stages of TBI, MSCEVs might have the best efficacy, both in neural function and spatial memory. However, more research is needed to confirm our findings and provide sufficient basis for its transformation into clinical research.
The raw data supporting the conclusions of this article will be made available by the authors, without undue reservation.
Z-LY and Z-YL developed the ideas, wrote the frameworks and full texts, searched the literatures, and collected the data. Y-KL and F-BL analyzed and organized the data. JR and X-JX formatted the pictures and tables. C-HW and C-MC revised the article and reviewed the final version. All authors contributed to the article and approved the submitted version.
The authors declare that the research was conducted in the absence of any commercial or financial relationships that could be construed as a potential conflict of interest.
All claims expressed in this article are solely those of the authors and do not necessarily represent those of their affiliated organizations, or those of the publisher, the editors and the reviewers. Any product that may be evaluated in this article, or claim that may be made by its manufacturer, is not guaranteed or endorsed by the publisher.
The Supplementary Material for this article can be found online at: https://www.frontiersin.org/articles/10.3389/fnins.2023.1147194/full#supplementary-material
Abedi, M., Hajinejad, M., Atabi, F., and Sahab-Negah, S. (2022). Exosome derived from human neural stem cells improves motor activity and neurogenesis in a traumatic brain injury model. Biomed. Res. Int. 2022:6409346. doi: 10.1155/2022/6409346
Andrzejewska, A., Dabrowska, S., Lukomska, B., and Janowski, M. (2021). Mesenchymal stem cells for neurological disorders. Adv. Sci. 8:2002944. doi: 10.1002/advs.202002944
Badhiwala, J. H., Wilson, J. R., and Fehlings, M. G. (2019). Global burden of traumatic brain and spinal cord injury. Lancet Neurol. 18, 24–25. doi: 10.1016/s1474-4422(18)30444-7
Chen, Y., Li, J., Ma, B., Li, N., Wang, S., Sun, Z., et al. (2020). MSC-derived exosomes promote recovery from traumatic brain injury via microglia/macrophages in rat. Aging 12, 18274–18296. doi: 10.18632/aging.103692
Chopp, M., and Li, Y. (2002). Treatment of neural injury with marrow stromal cells. Lancet Neurol. 1, 92–100. doi: 10.1016/s1474-4422(02)00040-6
Colombo, M., Raposo, G., and Thery, C. (2014). Biogenesis, secretion, and intercellular interactions of exosomes and other extracellular vesicles. Annu. Rev. Cell Dev. Biol. 30, 255–289. doi: 10.1146/annurev-cellbio-101512-122326
Cui, L., Luo, W., Jiang, W., Li, H., Xu, J., Liu, X., et al. (2022). Human umbilical cord mesenchymal stem cell-derived exosomes promote neurological function recovery in rat after traumatic brain injury by inhibiting the activation of microglia and astrocyte. Regen. Ther. 21, 282–287. doi: 10.1016/j.reth.2022.07.005
Das, M., Mayilsamy, K., Mohapatra, S. S., and Mohapatra, S. (2019). Mesenchymal stem cell therapy for the treatment of traumatic brain injury: progress and prospects. Rev. Neurosci. 30, 839–855. doi: 10.1515/revneuro-2019-0002
Dutta, D., Khan, N., Wu, J., and Jay, S. M. (2021). Extracellular vesicles as an emerging frontier in spinal cord injury pathobiology and therapy. Trends Neurosci. 44, 492–506. doi: 10.1016/j.tins.2021.01.003
Escartin, C., Galea, E., Lakatos, A., O’Callaghan, J. P., Petzold, G. C., Serrano-Pozo, A., et al. (2021). Reactive astrocyte nomenclature, definitions, and future directions. Nat. Neurosci. 24, 312–325. doi: 10.1038/s41593-020-00783-4
Ge, X., Guo, M., Hu, T., Li, W., Huang, S., Yin, Z., et al. (2020). Increased microglial exosomal miR-124-3p alleviates neurodegeneration and improves cognitive outcome after rmTBI. Mol. Ther. 28, 503–522. doi: 10.1016/j.ymthe.2019.11.017
Gold, E. M., Su, D., López-Velázquez, L., Haus, D. L., Perez, H., Lacuesta, G. A., et al. (2013). Functional assessment of long-term deficits in rodent models of traumatic brain injury. Regen. Med. 8, 483–516. doi: 10.2217/rme.13.41
Gressani, O., Faes, C., and Hens, N. (2022). Laplacian-P-splines for Bayesian inference in the mixture cure model. Stat. Med. 41, 2602–2626. doi: 10.1002/sim.9373
Guedes, V. A., Devoto, C., Leete, J., Sass, D., Acott, J. D., Mithani, S., et al. (2020). Extracellular vesicle proteins and MicroRNAs as biomarkers for traumatic brain injury. Front. Neurol. 11:663. doi: 10.3389/fneur.2020.00663
Han, Q. F., Li, W. J., Hu, K. S., Gao, J., Zhai, W. L., Yang, J. H., et al. (2022). Exosome biogenesis: machinery, regulation, and therapeutic implications in cancer. Mol. Cancer 21:207. doi: 10.1186/s12943-022-01671-0
Hara, M., Kobayakawa, K., Ohkawa, Y., Kumamaru, H., Yokota, K., Saito, T., et al. (2017). Interaction of reactive astrocytes with type I collagen induces astrocytic scar formation through the integrin-N-cadherin pathway after spinal cord injury. Nat. Med. 23, 818–828. doi: 10.1038/nm.4354
Harvey, R. L., and Chopp, M. (2003). The therapeutic effects of cellular therapy for functional recovery after brain injury. Phys. Med. Rehabil. Clin. N. Am. 14 (1 Suppl), S143–S151. doi: 10.1016/s1047-9651(02)00058-x
He, B., Chen, W., Zeng, J., Tong, W., and Zheng, P. (2021). Long noncoding RNA NKILA transferred by astrocyte-derived extracellular vesicles protects against neuronal injury by upregulating NLRX1 through binding to mir-195 in traumatic brain injury. Aging 13, 8127–8145. doi: 10.18632/aging.202618
Hooijmans, C. R., Rovers, M. M., de Vries, R. B. M., Leenaars, M., Ritskes-Hoitinga, M., and Langendam, M. W. (2014). SYRCLE’s risk of bias tool for animal studies. BMC Med. Res. Methodol. 14:43. doi: 10.1186/1471-2288-14-43
Hsuan, Y. C., Lin, C. H., Chang, C. P., and Lin, M. T. (2016). Mesenchymal stem cell-based treatments for stroke, neural trauma, and heat stroke. Brain Behav. 6:e00526. doi: 10.1002/brb3.526
Hutton, B., Salanti, G., Caldwell, D. M., Chaimani, A., Schmid, C. H., Cameron, C., et al. (2015). The PRISMA extension statement for reporting of systematic reviews incorporating network meta-analyses of health care interventions: checklist and explanations. Ann. Intern. Med. 162, 777–784. doi: 10.7326/m14-2385
Jansen, J. P., Crawford, B., Bergman, G., and Stam, W. (2008). Bayesian meta-analysis of multiple treatment comparisons: an introduction to mixed treatment comparisons. Value Health 11, 956–964. doi: 10.1111/j.1524-4733.2008.00347.x
Kalra, H., Drummen, G. P., and Mathivanan, S. (2016). Focus on extracellular vesicles: introducing the next small big thing. Int. J. Mol. Sci. 17:170. doi: 10.3390/ijms17020170
Kigerl, K. A., Gensel, J. C., Ankeny, D. P., Alexander, J. K., Donnelly, D. J., and Popovich, P. G. (2009). Identification of two distinct macrophage subsets with divergent effects causing either neurotoxicity or regeneration in the injured mouse spinal cord. J. Neurosci. 29, 13435–13444. doi: 10.1523/jneurosci.3257-09.2009
Kim, D. K., Nishida, H., An, S. Y., Shetty, A. K., Bartosh, T. J., and Prockop, D. J. (2016). Chromatographically isolated CD63(+) CD81(+) extracellular vesicles from mesenchymal stromal cells rescue cognitive impairments after TBI. Proc Natl. Acad. Sci. U S A. 113, 170–175. doi: 10.1073/pnas.1522297113
Köhli, P., Otto, E., Jahn, D., Reisener, M. J., Appelt, J., Rahmani, A., et al. (2021). Future perspectives in spinal cord repair: brain as saviour? TSCI with concurrent TBI: pathophysiological interaction and impact on MSC treatment. Cells 10:2955. doi: 10.3390/cells10112955
Li, B., Zhang, D., and Verkhratsky, A. (2022). Astrocytes in post-traumatic stress disorder. Neurosci. Bull. 38, 953–965. doi: 10.1007/s12264-022-00845-6
Li, D., Huang, S., Yin, Z., Zhu, J., Ge, X., Han, Z., et al. (2019). Increases in miR-124-3p in microglial exosomes confer neuroprotective effects by targeting FIP200-Mediated neuronal autophagy following traumatic brain injury. Neurochem. Res. 44, 1903–1923. doi: 10.1007/s11064-019-02825-1
Liddelow, S. A., Guttenplan, K. A., Clarke, L. E., Bennett, F. C., Bohlen, C. J., Schirmer, L., et al. (2017). Neurotoxic reactive astrocytes are induced by activated microglia. Nature 541, 481–487. doi: 10.1038/nature21029
Liu, W. Z., Ma, Z. J., Li, J. R., and Kang, X. W. (2021). Mesenchymal stem cell-derived exosomes: therapeutic opportunities and challenges for spinal cord injury. Stem Cell Res. Ther. 12:102. doi: 10.1186/s13287-021-02153-8
Liu, X.-Y., Wei, M.-G., Liang, J., Xu, H.-H., Wang, J.-J., Wang, J., et al. (2020). Injury-preconditioning secretome of umbilical cord mesenchymal stem cells amplified the neurogenesis and cognitive recovery after severe traumatic brain injury in rats. J. Neurochem. 153, 230–251. doi: 10.1111/jnc.14859
Long, X., Yao, X., Jiang, Q., Yang, Y., He, X., Tian, W., et al. (2020). Astrocyte-derived exosomes enriched with miR-873a-5p inhibit neuroinflammation via microglia phenotype modulation after traumatic brain injury. J. Neuroinflamm. 17:89. doi: 10.1186/s12974-020-01761-0
Maas, A. I. R., Fitzgerald, M., Gao, G., Gupta, D., Hutchinson, P., Manley, G. T., et al. (2022). Traumatic brain injury over the past 20 years: research and clinical progress. Lancet Neurol. 21, 768–770. doi: 10.1016/s1474-4422(22)00307-6
Maas, A. I. R., Menon, D. K., Adelson, P. D., Andelic, N., Bell, M. J., Belli, A., et al. (2017). Traumatic brain injury: integrated approaches to improve prevention, clinical care, and research. Lancet Neurol. 16, 987–1048. doi: 10.1016/s1474-4422(17)30371-x
Mahmood, A., Lu, D., and Chopp, M. (2004). Marrow stromal cell transplantation after traumatic brain injury promotes cellular proliferation within the brain. Neurosurgery 55, 1185–1193. doi: 10.1227/01.neu.0000141042.14476.3c
Mahmood, A., Lu, D., Wang, L., Li, Y., Lu, M., and Chopp, M. (2001). Treatment of traumatic brain injury in female rats with intravenous administration of bone marrow stromal cells. Neurosurgery 49:1196-1203; discussion 1203-1194.
Menon, D. K., Schwab, K., Wright, D. W., and Maas, A. I. (2010). Position statement: definition of traumatic brain injury. Arch. Phys. Med. Rehabil. 91, 1637–1640. doi: 10.1016/j.apmr.2010.05.017
Moss, L. D., Sode, D., Patel, R., Lui, A., Hudson, C., Patel, N. A., et al. (2021). Intranasal delivery of exosomes from human adipose derived stem cells at forty-eight hours post injury reduces motor and cognitive impairments following traumatic brain injury. Neurochem. Int. 150:105173. doi: 10.1016/j.neuint.2021.105173
Mot, Y. Y., Moses, E. J., Mohd Yusoff, N., Ling, K. H., Yong, Y. K., and Tan, J. J. (2022). Mesenchymal stromal cells-derived exosome and the roles in the treatment of traumatic brain injury. Cell Mol. Neurobiol. 43, 469–489. doi: 10.1007/s10571-022-01201-y
Muhammad, S. A., Abbas, A. Y., Imam, M. U., Saidu, Y., and Bilbis, L. S. (2022). Efficacy of stem cell secretome in the treatment of traumatic brain injury: a systematic review and meta-analysis of preclinical studies. Mol. Neurobiol. 59, 2894–2909. doi: 10.1007/s12035-022-02759-w
Ni, H., Yang, S., Siaw-Debrah, F., Hu, J., Wu, K., He, Z., et al. (2019). Exosomes derived from bone mesenchymal stem cells ameliorate early inflammatory responses following traumatic brain injury. Front. Neurosci. 13:14. doi: 10.3389/fnins.2019.00014
Osanai, T., Kuroda, S., Sugiyama, T., Kawabori, M., Ito, M., Shichinohe, H., et al. (2012). Therapeutic effects of intra-arterial delivery of bone marrow stromal cells in traumatic brain injury of rats–in vivo cell tracking study by near-infrared fluorescence imaging. Neurosurgery 70, 435–444; discussion 444. doi: 10.1227/NEU.0b013e318230a795.
Patel, N. A., Moss, L. D., Lee, J. Y., Tajiri, N., Acosta, S., Hudson, C., et al. (2018). Long noncoding RNA MALAT1 in exosomes drives regenerative function and modulates inflammation-linked networks following traumatic brain injury. J. Neuroinflamm. 15:204. doi: 10.1186/s12974-018-1240-3
Perez, E. J., Tapanes, S. A., Loris, Z. B., Balu, D. T., Sick, T. J., Coyle, J. T., et al. (2017). Enhanced astrocytic d-serine underlies synaptic damage after traumatic brain injury. J. Clin. Invest. 127, 3114–3125. doi: 10.1172/jci92300
Peruzzaro, S. T., Andrews, M. M. M., Al-Gharaibeh, A., Pupiec, O., Resk, M., Story, D., et al. (2019). Transplantation of mesenchymal stem cells genetically engineered to overexpress interleukin-10 promotes alternative inflammatory response in rat model of traumatic brain injury. J. Neuroinflamm. 16:2. doi: 10.1186/s12974-018-1383-2
Pischiutta, F., Caruso, E., Cavaleiro, H., Salgado, A. J., Loane, D. J., and Zanier, E. R. (2022). Mesenchymal stromal cell secretome for traumatic brain injury: focus on immunomodulatory action. Exp. Neurol. 357:114199. doi: 10.1016/j.expneurol.2022.114199
Ridet, J. L., Malhotra, S. K., Privat, A., and Gage, F. H. (1997). Reactive astrocytes: cellular and molecular cues to biological function. Trends Neurosci. 20, 570–577. doi: 10.1016/s0166-2236(97)01139-9
Salanti, G. (2012). Indirect and mixed-treatment comparison, network, or multiple-treatments meta-analysis: many names, many benefits, many concerns for the next generation evidence synthesis tool. Res. Synth. Methods 3, 80–97. doi: 10.1002/jrsm.1037
Salanti, G., Ades, A. E., and Ioannidis, J. P. (2011). Graphical methods and numerical summaries for presenting results from multiple-treatment meta-analysis: an overview and tutorial. J. Clin. Epidemiol. 64, 163–171. doi: 10.1016/j.jclinepi.2010.03.016
Shahror, R. A., Ali, A. A. A., Wu, C. C., Chiang, Y. H., and Chen, K. Y. (2019). Enhanced homing of mesenchymal stem cells overexpressing fibroblast growth factor 21 to injury site in a mouse model of traumatic brain injury. Int. J. Mol. Sci. 20:2624. doi: 10.3390/ijms20112624
Shahror, R. A., Wu, C. C., Chiang, Y. H., and Chen, K. Y. (2020). Genetically modified mesenchymal stem cells: the next generation of stem cell-based therapy for TBI. Int. J. Mol. Sci. 21:4051. doi: 10.3390/ijms21114051
Shao, A., Tu, S., Lu, J., and Zhang, J. (2019). Crosstalk between stem cell and spinal cord injury: pathophysiology and treatment strategies. Stem Cell Res. Ther. 10:238. doi: 10.1186/s13287-019-1357-z
Sofroniew, M. V., and Vinters, H. V. (2010). Astrocytes: biology and pathology. Acta Neuropathol. 119, 7–35. doi: 10.1007/s00401-009-0619-8
Stang, A. (2010). Critical evaluation of the Newcastle-Ottawa scale for the assessment of the quality of nonrandomized studies in meta-analyses. Eur. J. Epidemiol. 25, 603–605. doi: 10.1007/s10654-010-9491-z
Sun, M. K., Passaro, A. P., Latchoumane, C. F., Spellicy, S. E., Bowler, M., Goeden, M., et al. (2020). Extracellular vesicles mediate neuroprotection and functional recovery after traumatic brain injury. J. Neurotrauma 37, 1358–1369. doi: 10.1089/neu.2019.6443
Thery, C., Witwer, K. W., Aikawa, E., Alcaraz, M. J., Anderson, J. D., Andriantsitohaina, R., et al. (2018). Minimal information for studies of extracellular vesicles 2018 (MISEV2018): a position statement of the International Society for Extracellular Vesicles and update of the MISEV2014 guidelines. J. Extracell. Vesicles 7:1535750. doi: 10.1080/20013078.2018.1535750
Turovsky, E. A., Golovicheva, V. V., Varlamova, E. G., Danilina, T. I., Goryunov, K. V., Shevtsova, Y. A., et al. (2022). Mesenchymal stromal cell-derived extracellular vesicles afford neuroprotection by modulating PI3K/AKT pathway and calcium oscillations. Int. J. Biol. Sci. 18, 5345–5368. doi: 10.7150/ijbs.73747
van Niel, G., D’Angelo, G., and Raposo, G. (2018). Shedding light on the cell biology of extracellular vesicles. Nat. Rev. Mol. Cell Biol. 19, 213–228. doi: 10.1038/nrm.2017.125
Wang, B., and Han, S. (2019). Modified exosomes reduce apoptosis and ameliorate neural deficits induced by traumatic brain injury. ASAIO J. 65, 285–292. doi: 10.1097/MAT.0000000000000810
Wen, L., Wang, Y.-D., Shen, D.-F., Zheng, P.-D., Tu, M.-D., You, W.-D., et al. (2022). Exosomes derived from bone marrow mesenchymal stem cells inhibit neuroinflammation after traumatic brain injury. Neural Regenerat. Res. 17, 2717–2724.
White, I. R., Barrett, J. K., Jackson, D., and Higgins, J. P. (2012). Consistency and inconsistency in network meta-analysis: model estimation using multivariate meta-regression. Res. Synth. Methods 3, 111–125. doi: 10.1002/jrsm.1045
Xu, H., Jia, Z., Ma, K., Zhang, J., Dai, C., Yao, Z., et al. (2020). Protective effect of BMSCs-derived exosomes mediated by BDNF on TBI via miR-216a-5p. Med. Sci. Monit. 26:e920855. doi: 10.12659/msm.920855
Xu, X., Liang, Z., Lin, Y., Rao, J., Lin, F., Yang, Z., et al. (2022). Comparing the efficacy and safety of cell transplantation for spinal cord injury: a systematic review and bayesian network meta-analysis. Front. Cell Neurosci. 16:860131. doi: 10.3389/fncel.2022.860131
Yang, Y., Ye, Y., Kong, C., Su, X., Zhang, X., Bai, W., et al. (2019). MiR-124 enriched exosomes promoted the M2 polarization of microglia and enhanced hippocampus neurogenesis after traumatic brain injury by inhibiting TLR4 pathway. Neurochem. Res. 44, 811–828. doi: 10.1007/s11064-018-02714-z
Yuan, M., and Wu, H. (2022). Astrocytes in the traumatic brain injury: the good and the bad. Exp. Neurol. 348:113943. doi: 10.1016/j.expneurol.2021.113943
Zhang, R., Liu, Y., Yan, K., Chen, L., Chen, X. R., Li, P., et al. (2013). Anti-inflammatory and immunomodulatory mechanisms of mesenchymal stem cell transplantation in experimental traumatic brain injury. J. Neuroinflamm. 10:106. doi: 10.1186/1742-2094-10-106
Zhang, W., Hong, J., Zhang, H., Zheng, W., and Yang, Y. (2021). Astrocyte-derived exosomes protect hippocampal neurons after traumatic brain injury by suppressing mitochondrial oxidative stress and apoptosis. Aging 13, 21642–21658. doi: 10.18632/aging.203508
Zhang, Y., Zhang, Y., Chopp, M., Pang, H., Zhang, Z. G., Mahmood, A., et al. (2021). MiR-17-92 cluster-enriched exosomes derived from human bone marrow mesenchymal stromal cells improve tissue and functional recovery in rats after traumatic brain injury. J. Neurotrauma 38, 1535–1550. doi: 10.1089/neu.2020.7575
Zhang, Y., Chopp, M., Meng, Y., Katakowski, M., Xin, H., Mahmood, A., et al. (2015). Effect of exosomes derived from multipluripotent mesenchymal stromal cells on functional recovery and neurovascular plasticity in rats after traumatic brain injury. J. Neurosurg. 122, 856–867. doi: 10.3171/2014.11.Jns14770
Zhang, Y., Chopp, M., Zhang, Z. G., Katakowski, M., Xin, H., Qu, C., et al. (2017). Systemic administration of cell-free exosomes generated by human bone marrow derived mesenchymal stem cells cultured under 2D and 3D conditions improves functional recovery in rats after traumatic brain injury. Neurochem. Int. 111, 69–81. doi: 10.1016/j.neuint.2016.08.003
Zhang, Y., Zhang, Y., Chopp, M., Zhang, Z. G., Mahmood, A., and Xiong, Y. (2020). Mesenchymal stem cell-derived exosomes improve functional recovery in rats after traumatic brain injury: a dose-response and therapeutic window study. Neurorehabil. Neural Repair. 34, 616–626. doi: 10.1177/1545968320926164
Zhang, Z. W., Wei, P., Zhang, G. J., Yan, J. X., Zhang, S., Liang, J., et al. (2022). Intravenous infusion of the exosomes derived from human umbilical cord mesenchymal stem cells enhance neurological recovery after traumatic brain injury via suppressing the NF-kappa B pathway. Open Life Sci. 17, 189–201. doi: 10.1515/biol-2022-0022
Zhao, C., Deng, Y., He, Y., Huang, X., Wang, C., and Li, W. (2021). Decreased level of exosomal miR-5121 released from microglia suppresses neurite outgrowth and synapse recovery of neurons following traumatic brain injury. Neurotherapeutics 18, 1273–1294. doi: 10.1007/s13311-020-00999-z
Zhu, H., Ibrahim, J. G., and Chen, Q. (2014). Bayesian case-deletion model complexity and information criterion. Stat. Interface 7, 531–542. doi: 10.4310/SII.2014.v7.n4.a9
Zhuang, Z., Liu, M., Luo, J., Zhang, X., Dai, Z., Zhang, B., et al. (2022). Exosomes derived from bone marrow mesenchymal stem cells attenuate neurological damage in traumatic brain injury by alleviating glutamate-mediated excitotoxicity. Exp. Neurol. 357:114182. doi: 10.1016/j.expneurol.2022.114182
Keywords: traumatic brain injury, network meta-analysis, extracellular vesicles, preclinical research, systematic review
Citation: Yang Z-L, Liang Z-Y, Lin Y-K, Lin F-B, Rao J, Xu X-J, Wang C-H and Chen C-M (2023) Efficacy of extracellular vesicles of different cell origins in traumatic brain injury: A systematic review and network meta-analysis. Front. Neurosci. 17:1147194. doi: 10.3389/fnins.2023.1147194
Received: 18 January 2023; Accepted: 14 March 2023;
Published: 29 March 2023.
Edited by:
Zhuorong Li, Institute of Medicinal Biotechnology (CAS), ChinaReviewed by:
Changmeng Cui, Jining Medical University, ChinaCopyright © 2023 Yang, Liang, Lin, Lin, Rao, Xu, Wang and Chen. This is an open-access article distributed under the terms of the Creative Commons Attribution License (CC BY). The use, distribution or reproduction in other forums is permitted, provided the original author(s) and the copyright owner(s) are credited and that the original publication in this journal is cited, in accordance with accepted academic practice. No use, distribution or reproduction is permitted which does not comply with these terms.
*Correspondence: Chun-Mei Chen, Y21jaGVuMjAwOUBzaW5hLmNvbQ==; Chun-Hua Wang, d2NobWFpbEAxMjYuY29t
†These authors have contributed equally to this work and share first authorship
Disclaimer: All claims expressed in this article are solely those of the authors and do not necessarily represent those of their affiliated organizations, or those of the publisher, the editors and the reviewers. Any product that may be evaluated in this article or claim that may be made by its manufacturer is not guaranteed or endorsed by the publisher.
Research integrity at Frontiers
Learn more about the work of our research integrity team to safeguard the quality of each article we publish.