- 1Department of Biosciences, Durham University, Durham, United Kingdom
- 2MSc in Neuroscience Programme, University of Oxford, Oxford, United Kingdom
- 3Institute of Functional Genomics, University of Montpellier, CNRS, INSERM, Montpellier, France
Although most neurons are generated embryonically, neurogenesis is maintained at low rates in specific brain areas throughout adulthood, including the dentate gyrus of the mammalian hippocampus. Episodic-like memories encoded in the hippocampus require the dentate gyrus to decorrelate similar experiences by generating distinct neuronal representations from overlapping inputs (pattern separation). Adult-born neurons integrating into the dentate gyrus circuit compete with resident mature cells for neuronal inputs and outputs, and recruit inhibitory circuits to limit hippocampal activity. They display transient hyperexcitability and hyperplasticity during maturation, making them more likely to be recruited by any given experience. Behavioral evidence suggests that adult-born neurons support pattern separation in the rodent dentate gyrus during encoding, and they have been proposed to provide a temporal stamp to memories encoded in close succession. The constant addition of neurons gradually degrades old connections, promoting generalization and ultimately forgetting of remote memories in the hippocampus. This makes space for new memories, preventing saturation and interference. Overall, a small population of adult-born neurons appears to make a unique contribution to hippocampal information encoding and removal. Although several inconsistencies regarding the functional relevance of neurogenesis remain, in this review we argue that immature neurons confer a unique form of transience on the dentate gyrus that complements synaptic plasticity to help animals flexibly adapt to changing environments.
Introduction
Brain plasticity enables animals to encode novel information and adapt to changing environments. A leading hypothesis suggests that memory representations are stored within connected neuronal ensembles called engrams in each brain region and throughout the brain (Semon, 1921). Neuronal ensembles activated together by a learning experience undergo persistent functional modifications upon learning and are reactivated together during memory recall. Learning triggers lasting changes in synaptic strength between co-activated neurons (Hebb, 2005), often underlain by long-term potentiation (LTP) of relevant synapses (Bliss and Lømo, 1973).
In the mammalian brain, episodic-like memories are stored in the hippocampus (Figure 1A). The dentate gyrus (DG) of the hippocampus integrates spatio-temporal and event-specific information from the medial and lateral entorhinal cortex (EC), respectively, and converts them into sparse neuronal representations (Engin et al., 2015). Pattern separation enables highly analogous memories to be stored with little interference in distinct cell ensembles in the CA3 subfield (Leutgeb et al., 2007). The DG also attenuates the generalization of remote fear memories and may be involved in the remote memory retrieval (Bernier et al., 2017). CA3 performs the complementary process of pattern completion, enabling behavioral expression of a memory trace, even when the context or inputs of memory recall are different from encoding or incomplete (Leutgeb et al., 2007). Outputs from CA3 are compared with direct EC inputs in CA1 and sent back to the EC to be distributed across the neocortex for long-term storage. The EC also has direct connections with CA3, which are involved in discrimination of distinct stimuli (Fyhn et al., 2007).
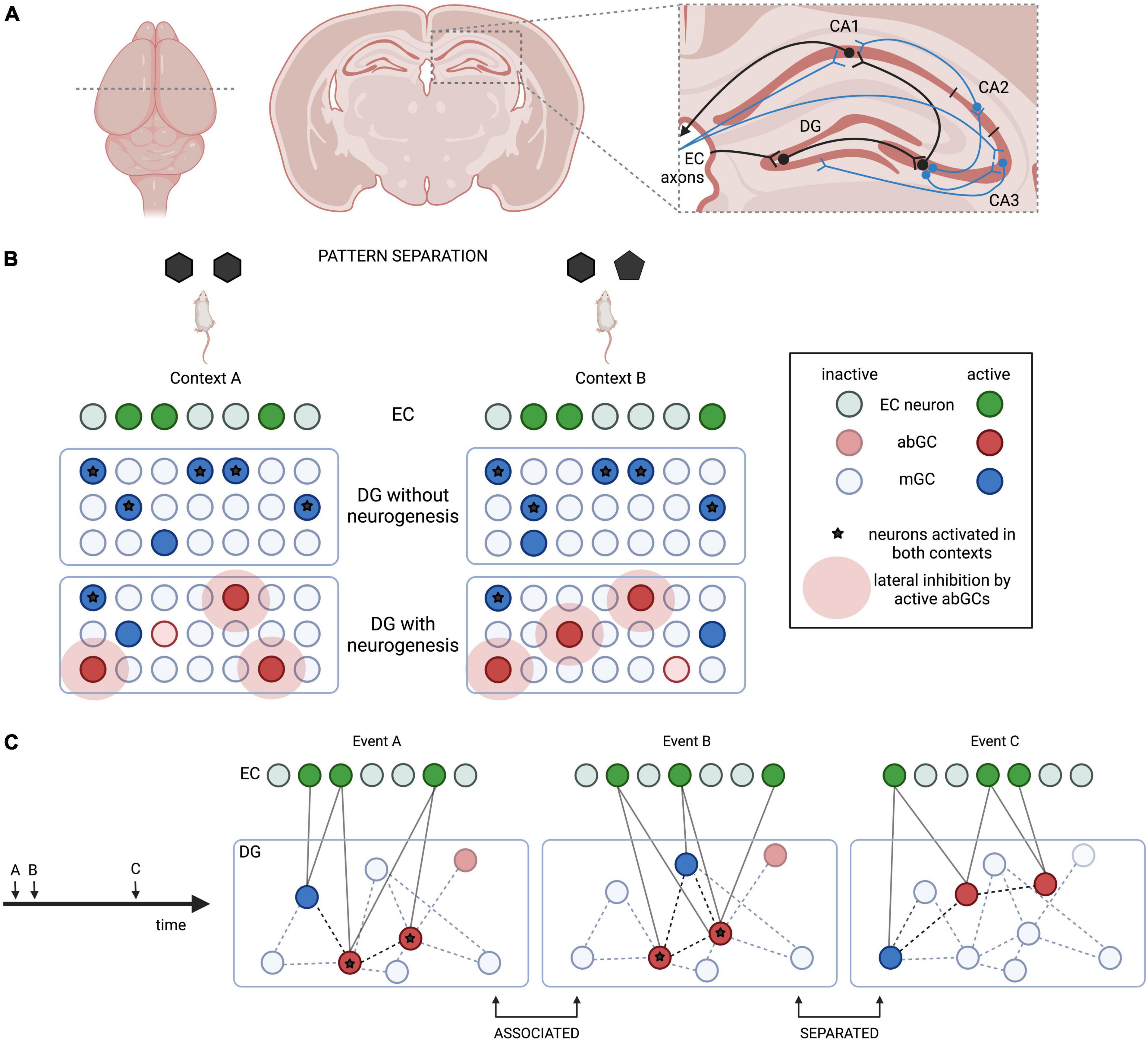
Figure 1. Simplified hippocampal circuitry and the role of adult neurogenesis in pattern separation in the dentate gyrus (DG). (A) Schematic hippocampal circuitry from a mouse brain coronal section. Beyond the basic trisynaptic loop (EC-DG-CA3-CA1, black), some entorhinal cortex (EC) inputs go directly to CA1/CA3, while some CA3 axons project to CA2, send collaterals to other CA3 neurons, and feed back to the DG (blue). (B) Adult-born GCs (abGCs) achieve pattern separation by limiting the activation of the same mature GCs (mGCs) in similar contexts. (C) “Time-stamping” could link contemporary events A and B, but separate remote event C by activating a changing population of abGCs.
In most mammals, hippocampal circuitry is constantly reformed by a unique form of structural and functional plasticity involving neurogenesis. Adult neurogenesis in rodents is present in at least two areas: the subventricular zone lining the lateral ventricles, and the subgranular zone of the DG (Lois and Alvarez-Buylla, 1993; Kempermann et al., 1997a). The former gives rise to cells that migrate to the olfactory bulb and differentiate into inhibitory olfactory neurons, while the latter generates excitatory glutamatergic granule cells (GCs). The vast majority of DG GCs are born perinatally, after which neurogenesis declines and is maintained at varying levels throughout adulthood (Ngwenya et al., 2015; Hochgerner et al., 2018). Of all DG GCs, ∼0.2% are generated daily in rats and ∼0.06% in mice (Kempermann et al., 1997a; Cameron and Mckay, 2001). Around half of adult-born GCs (abGCs) generated are eliminated through waves of programmed cell death during their maturation (Dayer et al., 2003; Ryu et al., 2016; Pilz et al., 2018). Surviving cells are stably maintained and ultimately become indistinguishable from developmental GCs (Dayer et al., 2003; Kempermann et al., 2003). Neurogenesis is balanced by the continuous removal of mostly perinatally-generated mature GCs (mGCs) (Ciric et al., 2019), resulting in a constant or slightly expanding DG cell number (Rapp and Gallagher, 1996; Kempermann et al., 1997b). In this review, we argue that integration of abGCs into the DG network confers plasticity to the classical cortico-hippocampal circuit.
Functional integration of adult-born neurons
Proliferation of neural progenitor cells (NPCs) in the subgranular zone of the DG produces neuronal fate-committed cells that undergo stereotypic stages of maturation (Hochgerner et al., 2018; Pilz et al., 2018). Maturing abGCs extend dendrites and an axon toward CA3 (or CA2; Llorens-Martín et al., 2015) of the hippocampus (Zhao et al., 2006), shifting excitation-inhibition balance. The ensuing critical period of hyperexcitability (Mongiat et al., 2009; Danielson et al., 2016; Li L. et al., 2017) is characterized by lower LTP induction threshold and higher LTP amplitude compared to mature mGCs (Schmidt-Hieber et al., 2004; Ge et al., 2007; Li et al., 2013). Maturing abGCs continuously reform their connections with the local circuitry, in an activity-dependent manner (Toni et al., 2007; Jungenitz et al., 2018).
During early maturation, abGCs receive inhibitory inputs from local interneurons and form transient direct connections with mGCs (Hendricks et al., 2017; Gozel and Gerstner, 2021). Electrophysiological recordings show that abGCs receiving lateral EC inputs inhibit mGCs, while abGCs receiving medial EC inputs excite mGCs (Luna et al., 2019). Later, abGCs switch from direct interactions to synaptic competition with mGCs for EC inputs and CA3 targets. Electron microscopy evidence shows that abGCs initially contact pre-existing axon terminals occupied by other neurons, but later outcompete mGC axons to become unique synaptic partners (Toni et al., 2007). Similar processes occur dendritically (Toni et al., 2008; McAvoy et al., 2016). Firing connections are stably maintained while inactive ones are pruned, therefore hyperexcitable abGCs tend to prevail, driving the elimination of existing mGC connections (Tashiro et al., 2007; Yasuda et al., 2011; Restivo et al., 2015; Murray et al., 2020). The selective survival of abGCs may also be regulated by synaptic activity, and in an information-specific manner (Tashiro et al., 2006). abGCs additionally form dynamic connections with hippocampal interneurons that inhibit neighboring mGCs (lateral inhibition), and exert inhibition even on CA3 and CA1 (feedforward inhibition) (Chawla et al., 2005; Guo et al., 2018; Berdugo-Vega et al., 2020). This results in the overall sparsification of population firing across the hippocampus (Lacefield et al., 2012; Temprana et al., 2015; McHugh et al., 2022).
Excitation or artificial LTP induction, as well as exposure to new experiences, such as spatial learning, voluntary exercise, or increased sensory stimulation (e.g., animal housing in groups, novel toys in home cages etc.) promote neurogenesis (Kempermann et al., 1997b; Gould et al., 1999; van Praag et al., 1999; Deisseroth et al., 2004; Bruel-Jungerman et al., 2006; Darcy et al., 2014). Stress, aging, and some neuropsychiatric conditions decrease proliferation rates and responsiveness of abGCs (Ben Abdallah et al., 2010; Snyder et al., 2011). This may contribute to intensified stress responses (Snyder et al., 2011), and decreased learning abilities in older animals (Montaron et al., 2020).
Contribution of adult-born neurons to memory encoding
The DG converts EC inputs into highly decorrelated representations in CA3 (Fyhn et al., 2007). This is achieved by changes in the correlated activity of the same sparse subset of GCs between similar contexts, such as when rats explore enclosures of slightly different shapes. Niibori et al. (2012) measured the overlap between CA3 cells activated during encoding and re-exposure using a cellular imaging approach, and found that suppressing neurogenesis disrupts the decorrelation of highly overlapping (but not dissimilar) contexts. Behavioral evidence suggests that critical period abGCs are important for tasks requiring separation of highly similar contexts, such as contextual fear discrimination or re-learning of a shock zone location (Table 1); however, these cells appear dispensable for learning a location in the water maze (Sahay et al., 2011, Burghardt et al., 2012). Intriguingly, blocking all outputs from GCs older than 3–4 weeks improves contextual fear conditioning (CFC) performance, suggesting that pattern separation not only relies on abGCs, but could be counteracted by mGCs (Nakashiba et al., 2012). abGCs might recruit inhibitory circuits to limit the activation of the same GCs in similar contexts via lateral and feedback inhibition, providing a potential mechanism for pattern separation (Kitamura et al., 2009; Engin et al., 2015; Temprana et al., 2015; Figure 1B).
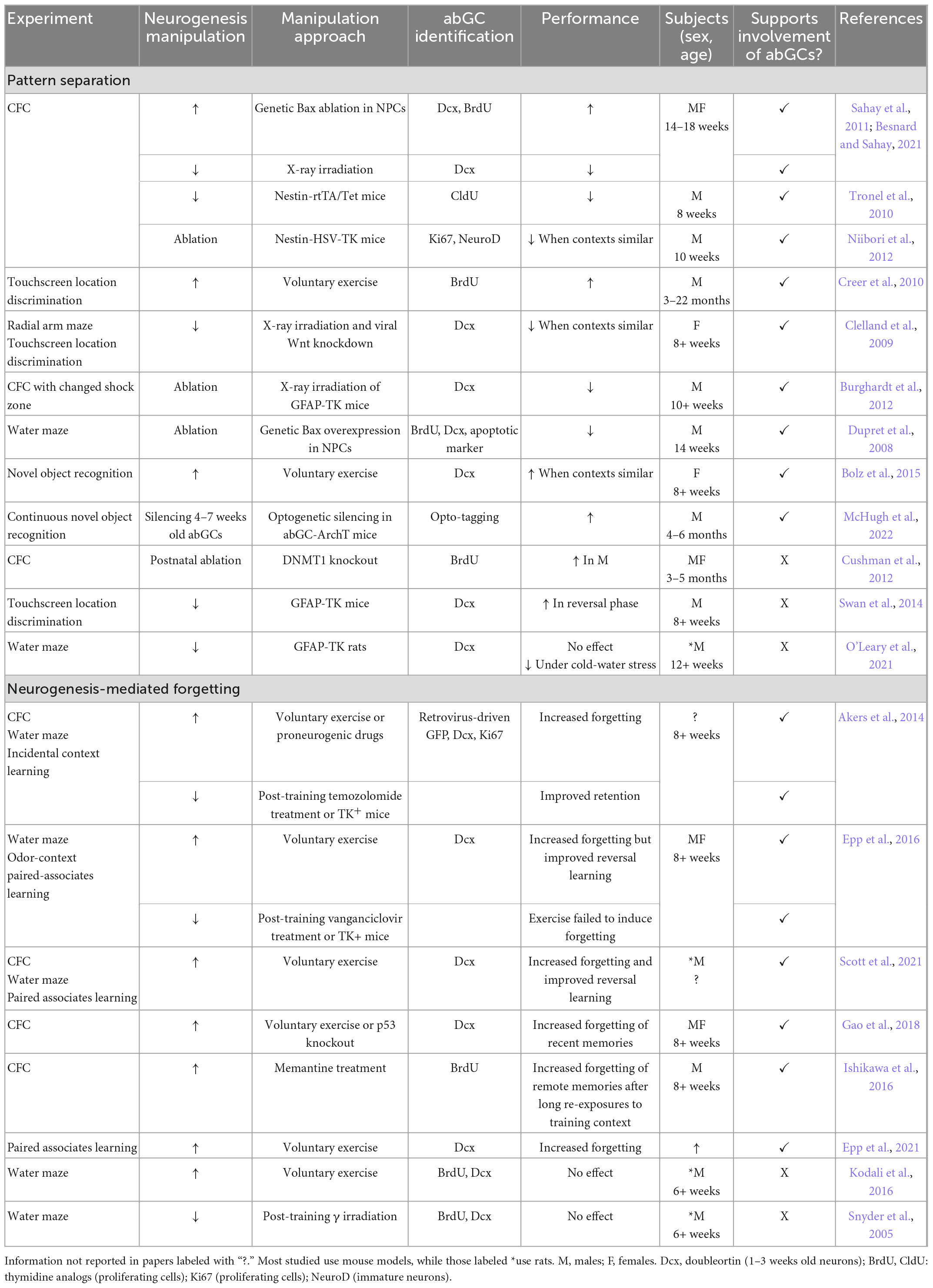
Table 1. Key studies on the effect of hippocampal neurogenesis on behavioral pattern separation and forgetting.
Despite these advances, the extent of abGC contribution to pattern separation remains ambiguous, largely due to inconsistencies around defining and manipulating relevant neuronal populations, and in the behavioral paradigms used (Table 1). More specific DG-based pattern separation paradigms, optogenetic manipulations, and simultaneous recordings of abGCs and mature hippocampal cell types may help elucidate the precise role of abGCs in memory formation. Disrupted pattern separation in some, but improved performance in other hippocampus-based tasks suggests that abGCs may serve additional functions beyond pattern separation, depending on the behavioral paradigm.
Aimone et al. (2006) proposed that abGCs link memories encoded in close succession, while separating remote memories (Figure 1C). Because hyperexcitable DG cells are preferentially included into engrams (Park et al., 2016), critical period abGCs may be more readily recruited into any memory trace. This generates overlapping representations in CA3 that can be activated by the context represented in any of the temporally associated engrams. Therefore, integration of young abGCs into hippocampal engrams may help connect contemporary memories (Cai et al., 2016), while more mature abGC populations support pattern separation (Aimone et al., 2010). The involvement of juvenile-born GCs (Kesner et al., 2014) in “time-stamping” has been described in a task where animals use spatial cues to generate preference for a temporally paired spatial location. Lesions in either cell population eliminated preference for the cued location, suggesting disrupted associations between events occurring close in time.
Recently, new findings have questioned the validity of the “time-stamping” hypothesis. Whereas abGCs are indeed more likely to be recruited during spatial memory encoding and activated during retrieval (Kee et al., 2007; Trouche et al., 2009; Stone et al., 2011; Gu et al., 2012; Martinez-Canabal et al., 2013), little overlap was found between abGCs activated during encoding or retrieval of contextual fear memories (Kumar et al., 2020). This suggests that either abGCs are activated by behavioral states rather than specific events or contexts (Erwin et al., 2020), or that limited overlap between the two populations could be a general property of DG engrams (Denny et al., 2014). Another line of recent findings shows that maturing abGCs maintain their hyperexcitable properties for several months beyond the proposed critical period. These studies injected rats with various thymidine analogs to birthdate GC populations before quantifying their activity using immediate early gene expression. abGCs remained excitable especially in younger animals and animals that were offered environmental stimulation, and their activation supported learning even in older animals (Ohline et al., 2018; Montaron et al., 2020). This questions the idea of temporal integration and the long-standing view that abGCs exert their memory-related functions merely during their first weeks of existence (Alme et al., 2010).
Adult-born neurons and memory consolidation
abGCs stably integrated into the hippocampal circuitry may also influence later stages of memory processing. Classical views of systems consolidation have held that after encoding, memories progressively lose their hippocampal dependence before transferring completely to neocortex (Frankland and Bontempi, 2005). Prefrontal cortex engrams are strengthened by CA3 and CA1 ripples (Nakashiba et al., 2009), while hippocampal engrams are gradually silenced (Kitamura et al., 2017; Gao et al., 2018).
Some evidence suggests that abGCs promote memory consolidation during sleep, when hippocampal and neocortical engrams are reactivated, and synapses are selectively strengthened or renormalized by dendritic remodeling (Mirescu et al., 2006; de Vivo et al., 2017; Li W. et al., 2017). abGCs active during CFC learning are reactivated during rapid eye movement (REM) sleep, and both optogenetic stimulation and silencing of reactivated abGCs disrupt consolidation (Kumar et al., 2020). Blocking neurogenesis reduces non-REM sleep and disrupts consolidation-related oscillations and cortex-hippocampus interaction, leading to poor spatial memory performance (Sippel et al., 2020). Further, the rate of neurogenesis also seems to determine the hippocampus-dependent period of memories (Kitamura et al., 2009). Although none of these studies directly links abGC engrams to these changes, or specifically accounts for sleep-induced changes in neurogenesis levels, they do demonstrate that abGCs are involved in sleep-related consolidation.
Neurogenesis affects memory stability and causes forgetting
Hippocampal representations of consolidated remote memories are reactivated upon retrieval. This destabilizes engrams, allowing protein synthesis-dependent reconsolidation processes to update, strengthen, or silence them (Suzuki et al., 2004). Both immature and critical period abGCs are reactivated during retrieval, however, blocking protein synthesis in the immature population alone affects reconsolidation (Lods et al., 2021). Updating of memories is impaired in the novel object recognition task when an even younger abGC population is ablated, further supporting that highly immature abGCs mediate reconsolidation (Suárez-Pereira and Carrión, 2015). The emerging unique roles of abGCs in post-encoding memory strengthening was recently demonstrated; chemogenetic stimulation during retrieval of abGCs, but not mGCs, improved remote memory strength and accuracy in rats (Lods et al., 2022).
A growing body of evidence suggests that increased neurogenesis after memory encoding promotes forgetting (Table 1). For instance, pharmacologically enhancing neurogenesis increases the forgetting of remote CFC memories after long re-exposures to the original context make them return to the hippocampus (Ishikawa et al., 2016). This has been explained by gradual elimination of existing connections through synaptic competition with abGCs (Murray et al., 2020), and reduction in LTP persistence through feedback and feedforward inhibition (Alam et al., 2018). Neurogenesis also disrupts perineuronal nets in CA1, which otherwise protect memories from degradation by limiting interneuron activity (Evans et al., 2022).
Removal of a small number of connections may only reduce memory precision, allowing recall by cues slightly different from the original encoding context (generalization) (Ko and Frankland, 2021). This might involve pruning of synapses that mediate feedforward inhibition (Ruediger et al., 2011). Once more connections are weakened, memories become inaccessible. High postnatal neurogenesis may even explain why early childhood memories are forgotten in many species (infantile amnesia) (Akers et al., 2014). Memory representations are not fully erased, as optogenetic reactivation of DG engrams can partially recover these memories (Guskjolen et al., 2018).
Replacement of old memories with updated novel memories can occur in similar contexts without interference. Indeed, neurogenesis is involved specifically in tasks requiring high cognitive flexibility, such as re-learning of a changed spatial location. In this case, ablation of neurogenesis prevents, while expansion of the abGC population promotes better search strategies (Garthe et al., 2009; Burghardt et al., 2012; Swan et al., 2014; Berdugo-Vega et al., 2021). Increased post-training neurogenesis weakens memories acquired in the water maze, which ultimately enables later re-learning of the task (Epp et al., 2016). Therefore, abGCs might promote forgetting to subsequently support encoding of novel memories.
Discussion
Neural circuits require flexibility to adapt to changing environments, and stability to preserve information. The brain uses two main approaches to achieve transience: synaptic plasticity and cellular plasticity, or neurogenesis. Turnover of dendritic spines is undoubtedly the primary mechanism of structural plasticity behind learning, raising the question of why the DG needs neurogenesis beyond the synaptic modulation of mGCs.
Memory encoding by abGCs adds an anterograde form of transience to the hippocampus. Computational models support that abGCs optimize the balance between pattern separation and completion (Becker, 2005; Weisz and Argibay, 2009; O’Donnell and Sejnowski, 2014; Finnegan and Becker, 2015). abGCs might be used specifically to incorporate information about new experiences into engrams in familiar contexts (Aimone et al., 2009). Importantly, the inherent temporality of neurogenesis could hardly be replicated by synaptic plasticity. Most research into temporal sequence generation in the hippocampus has focused on CA1 and CA2 (MacDonald et al., 2013; Mankin et al., 2015), but the contribution of DG abGCs merits further investigations.
abGCs also confer retrograde transience on DG engrams through weakening and elimination of existing connections. Novel DG engrams may be “overfitted” and thus require generalization for optimal expression through neurogenesis, which acts as a regularizer in neuronal networks (Richards and Frankland, 2017; Tran et al., 2022). By eliminating unnecessary details while maintaining core features, neurogenesis may make memories easier to recall in changing or noisy environments. Neurogenesis also helps “clear up” remnants of remote hippocampal engrams already consolidated in the cortex, similar to sleep that serves the same function on a shorter timescale (Alam et al., 2018). Models support that neurogenesis makes room for new memories and prevents interferences (Wiskott et al., 2006).
As brains have become more complex throughout evolution, neurogenesis in the DG was maintained and repurposed. Some argue that it confers key functional benefits that underpin the evolutionary success of mammals (Kempermann, 2012), while others dismiss it as an evolutionary remnant, given its low rates, especially in highly cognitively developed species. As neurogenesis is associated with energy costs, oxidative stress, and oncogenesis (Walton et al., 2012; Batista et al., 2014), its maintenance may only be beneficial in animals that need to flexibly adapt to rapidly changing or enriched environments (Abrous et al., 2021). Indeed, most generalists (e.g., rodents) show neurogenesis, but mammals living in stable or homogenous environments do not (e.g., cetaceans).
The outstanding cognitive abilities of the human brain are thought to result from plasticity. Yet, the maintenance of DG neurogenesis throughout adulthood remains debated (Boldrini et al., 2018; Sorrells et al., 2018), mainly due to a lack of non-invasive research methods. Single-nucleus RNA sequencing recently verified the presence of scarce immature GCs in the adult human DG, with a marked reduction in Alzheimer’s disease (Zhou et al., 2022). Whether these cells are actively generated during adulthood or retained in an immature state is unclear. Further research is required to establish if human neurogenesis has any cognitive benefits or functional implications in neuropsychiatric conditions (Mishra et al., 2022).
This review supports the idea that abGCs can participate in the formation of hippocampal memories and influence mGCs to help encoding, generalization, and forgetting. abGCs bring transience to the hippocampus both by adding and removing information about new events, experiences, or environments. Experimental standardization and technological advances can help resolve contradictions in the literature, for example, by combining abGC labeling, in vivo recording with engram cell- and synapse-tagging (Choi et al., 2018), and more advanced DG-specific behavioral paradigms. Standardized definitions of abGC versus mGC populations should also help draw clearer conclusions. Nevertheless if one accepts that, in addition to preserving information, a major goal of memory is to optimize behavior, a large body of evidence now supports adult neurogenesis as a meaningful contributor to hippocampal memory functions.
Author contributions
This work was originally written by OF as part of a 3rd year literature review assignment at Durham University. OF and VC: conceptualization. OF, ST, and VC: writing. VC: supervision. All authors contributed to the article and approved the submitted version.
Funding
Research in ST’s laboratory is funded by the French National Research Agency (ANR-19-CE37-00036 and ANR-21-CE16-0015), the NARSAD BBRF (award 24925), and the Foundation NRJ-Institut de France. Research in VC’s laboratory is funded by BBSRC (BB/W007347/1), the Royal Society (RGS\R1\221097), and Durham University Seedcorn Funds.
Conflict of interest
The authors declare that the research was conducted in the absence of any commercial or financial relationships that could be construed as a potential conflict of interest.
Publisher’s note
All claims expressed in this article are solely those of the authors and do not necessarily represent those of their affiliated organizations, or those of the publisher, the editors and the reviewers. Any product that may be evaluated in this article, or claim that may be made by its manufacturer, is not guaranteed or endorsed by the publisher.
References
Abrous, D. N., Koehl, M., and Lemoine, M. (2021). A Baldwin interpretation of adult hippocampal neurogenesis: From functional relevance to physiopathology. Mol. Psychiatry 2021, 1–20. doi: 10.1038/s41380-021-01172-4
Aimone, J. B., Deng, W., and Gage, F. H. (2010). Adult neurogenesis: Integrating theories and separating functions. Trends Cogn. Sci. 14, 325–337. doi: 10.1016/J.TICS.2010.04.003
Aimone, J. B., Wiles, J., and Gage, F. H. (2006). Potential role for adult neurogenesis in the encoding of time in new memories. Nat. Neurosci. 9, 723–727. doi: 10.1038/NN1707
Aimone, J. B., Wiles, J., and Gage, F. H. (2009). Computational influence of adult neurogenesis on memory encoding. Neuron 61, 187–202. doi: 10.1016/J.NEURON.2008.11.026
Akers, K. G., Martinez-Canabal, A., Restivo, L., Yiu, A. P., de Cristofaro, A., Hsiang, H.-L., et al. (2014). Hippocampal neurogenesis regulates forgetting during adulthood and infancy. Science 344, 598–602. doi: 10.1126/SCIENCE.1248903
Alam, M. J., Kitamura, T., Saitoh, Y., Ohkawa, N., Kondo, T., and Inokuchi, K. (2018). Adult neurogenesis conserves hippocampal memory capacity. J. Neurosci. 38, 6854–6863. doi: 10.1523/JNEUROSCI.2976-17.2018
Alme, C. B., Buzzetti, R. A., Marrone, D. F., Leutgeb, J. K., Chawla, M. K., Schaner, M. J., et al. (2010). Hippocampal granule cells opt for early retirement. Hippocampus 20, 1109–1123. doi: 10.1002/HIPO.20810
Batista, C. M., Mariano, E. D., Barbosa, B. J. A. P., Morgalla, M., Marie, S. K. N., Teixeira, M. J., et al. (2014). Adult neurogenesis and glial oncogenesis: When the process fails. Biomed. Res. Int. 2014:438639. doi: 10.1155/2014/438639
Becker, S. (2005). A computational principle for hippocampal learning and neurogenesis. Hippocampus 15, 722–738. doi: 10.1002/HIPO.20095
Ben Abdallah, N., Slomianka, L., Vyssotski, A. L., and Lipp, H. P. (2010). Early age-related changes in adult hippocampal neurogenesis in C57 mice. Neurobiol. Aging 31, 151–161. doi: 10.1016/J.NEUROBIOLAGING.2008.03.002
Berdugo-Vega, G., Arias-Gil, G., López-Fernández, A., Artegiani, B., Wasielewska, J. M., Lee, C. C., et al. (2020). Increasing neurogenesis refines hippocampal activity rejuvenating navigational learning strategies and contextual memory throughout life. Nat. Commun. 11, 1–12. doi: 10.1038/s41467-019-14026-z
Berdugo-Vega, G., Lee, C. C., Garthe, A., Kempermann, G., and Calegari, F. (2021). Adult-born neurons promote cognitive flexibility by improving memory precision and indexing. Hippocampus 31, 1068–1079. doi: 10.1002/HIPO.23373
Bernier, B. E., Lacagnina, A. F., Ayoub, A., Shue, F., Zemelman, B. V., Krasne, F. B., et al. (2017). Dentate gyrus contributes to retrieval as well as encoding: Evidence from context fear conditioning, recall, and extinction. J. Neurosci. 37:6359. doi: 10.1523/JNEUROSCI.3029-16.2017
Besnard, A., and Sahay, A. (2021). Enhancing adult neurogenesis promotes contextual fear memory discrimination and activation of hippocampal-dorsolateral septal circuits. Behav. Brain Res. 399:112917. doi: 10.1016/J.BBR.2020.112917
Bliss, T. V. P., and Lømo, T. (1973). Long-lasting potentiation of synaptic transmission in the dentate area of the anaesthetized rabbit following stimulation of the perforant path. J. Physiol. 232, 331–356. doi: 10.1113/JPHYSIOL.1973.SP010273
Boldrini, M., Fulmore, C. A., Tartt, A. N., Simeon, L. R., Pavlova, I., Poposka, V., et al. (2018). Human hippocampal neurogenesis persists throughout aging. Cell Stem Cell 22, 589–599.e5. doi: 10.1016/J.STEM.2018.03.015
Bolz, L., Heigele, S., and Bischofberger, J. (2015). Running improves pattern separation during novel object recognition. Brain Plast. 1:129. doi: 10.3233/BPL-150010
Bruel-Jungerman, E., Davis, S., Rampon, C., and Laroche, S. (2006). Long-term potentiation enhances neurogenesis in the adult dentate gyrus. J. Neurosci. 26, 5888–5893. doi: 10.1523/JNEUROSCI.0782-06.2006
Burghardt, N. S., Park, E. H., Hen, R., and Fenton, A. A. (2012). Adult-born hippocampal neurons promote cognitive flexibility in mice. Hippocampus 22, 1795–1808. doi: 10.1002/HIPO.22013
Cai, D. J., Aharoni, D., Shuman, T., Shobe, J., Biane, J., Song, W., et al. (2016). A shared neural ensemble links distinct contextual memories encoded close in time. Nature 534, 115–118. doi: 10.1038/nature17955
Cameron, H. A., and Mckay, R. D. G. (2001). Adult neurogenesis produces a large pool of new granule cells in the dentate gyrus. J. Comp. Neurol. 435, 406–417. doi: 10.1002/CNE.1040
Chawla, M. K., Guzowski, J. F., Ramirez-Amaya, V., Lipa, P., Hoffman, K. L., Marriott, L. K., et al. (2005). Sparse, environmentally selective expression of Arc RNA in the upper blade of the rodent fascia dentata by brief spatial experience. Hippocampus 15, 579–586. doi: 10.1002/HIPO.20091
Choi, J., Sim, S., Kim, J., Choi, D., Oh, J., Ye, S., et al. (2018). Interregional synaptic maps among engram cells underlie memory formation. Science 360, 430–435. doi: 10.1126/SCIENCE.AAS9204
Ciric, T., Cahill, S. P., and Snyder, J. S. (2019). Dentate gyrus neurons that are born at the peak of development, but not before or after, die in adulthood. Brain Behav. 9:e01435. doi: 10.1002/BRB3.1435
Clelland, C. D., Choi, M., Romberg, C., Clemenson, G. D., Fragniere, A., Tyers, P., et al. (2009). A functional role for adult hippocampal neurogenesis in spatial pattern separation. Science 325, 210–213. doi: 10.1126/SCIENCE.1173215/SUPPL_FILE/CLELLAND.SOM-.PDF
Creer, D. J., Romberg, C., Saksida, L. M., van Praag, H., and Bussey, T. J. (2010). Running enhances spatial pattern separation in mice. Proc. Natl. Acad. Sci. U.S.A. 107, 2367–2372. doi: 10.1073/PNAS.0911725107
Cushman, J. D., Maldonado, J., Kwon, E. E., Denise Garcia, A., Fan, G., Imura, T., et al. (2012). Juvenile neurogenesis makes essential contributions to adult brain structure and plays a sex-dependent role in fear memories. Front. Behav. Neurosci. 6:3. doi: 10.3389/FNBEH.2012.00003
Danielson, N. B. B., Kaifosh, P., Zaremba, J. D. D., Lovett-Barron, M., Tsai, J., Denny, C. A. A., et al. (2016). Distinct contribution of adult-born hippocampal granule cells to context encoding. Neuron 90, 101–112. doi: 10.1016/J.NEURON.2016.02.019
Darcy, M. J., Trouche, S., Jin, S. X., and Feig, L. A. (2014). Ras-GRF2 mediates long-term potentiation, survival, and response to an enriched environment of newborn neurons in the hippocampus. Hippocampus 24, 1317–1329. doi: 10.1002/HIPO.22313
Dayer, A. G., Ford, A. A., Cleaver, K. M., Yassaee, M., and Cameron, H. A. (2003). Short-term and long-term survival of new neurons in the rat dentate gyrus. J. Comp. Neurol. 460, 563–572. doi: 10.1002/CNE.10675
de Vivo, L., Bellesi, M., Marshall, W., Bushong, E. A., Ellisman, M. H., Tononi, G., et al. (2017). Ultrastructural evidence for synaptic scaling across the wake/sleep cycle. Science 355, 507–510. doi: 10.1126/SCIENCE.AAH5982
Deisseroth, K., Singla, S., Toda, H., Monje, M., Palmer, T. D., and Malenka, R. C. (2004). Excitation-neurogenesis coupling in adult neural stem/progenitor cells. Neuron 42, 535–552. doi: 10.1016/S0896-6273(04)00266-1
Denny, C. A., Kheirbek, M. A., Alba, E. L., Tanaka, K. F., Brachman, R. A., Laughman, K. B., et al. (2014). Hippocampal memory traces are differentially modulated by experience, time, and adult neurogenesis. Neuron 83, 189–201. doi: 10.1016/J.NEURON.2014.05.018
Dupret, D., Revest, J. M., Koehl, M., Ichas, F., de Giorgi, F., Costet, P., et al. (2008). Spatial relational memory requires hippocampal adult neurogenesis. PLoS One 3:e1959. doi: 10.1371/JOURNAL.PONE.0001959
Engin, E., Zarnowska, E. D., Benke, D., Tsvetkov, E., Sigal, M., Keist, R., et al. (2015). Tonic inhibitory control of dentate gyrus granule cells by α5-containing GABAA receptors reduces memory interference. J. Neurosci. 35, 13698–13712. doi: 10.1523/JNEUROSCI.1370-15.2015
Epp, J. R., Botly, L. C. P., Josselyn, S. A., and Frankland, P. W. (2021). Voluntary exercise increases neurogenesis and mediates forgetting of complex paired associates memories. Neuroscience 475, 1–9. doi: 10.1016/J.NEUROSCIENCE.2021.08.022
Epp, J. R., Mera, R. S., Köhler, S., Josselyn, S. A., and Frankland, P. W. (2016). Neurogenesis-mediated forgetting minimizes proactive interference. Nat. Commun. 7, 1–8. doi: 10.1038/ncomms10838
Erwin, S. R., Sun, W., Copeland, M., Lindo, S., Spruston, N., and Cembrowski, M. S. (2020). A sparse, spatially biased subtype of mature granule cell dominates recruitment in hippocampal-associated behaviors. Cell Rep. 31:107551. doi: 10.1016/J.CELREP.2020.107551
Evans, A., Terstege, D. J., Scott, G. A., Tsutsui, M., and Epp, J. R. (2022). Neurogenesis mediated plasticity is associated with reduced neuronal activity in CA1 during context fear memory retrieval. Sci. Rep. 12, 1–16. doi: 10.1038/s41598-022-10947-w
Finnegan, R., and Becker, S. (2015). Neurogenesis paradoxically decreases both pattern separation and memory interference. Front. Syst. Neurosci. 9:136. doi: 10.3389/FNSYS.2015.00136
Frankland, P. W., and Bontempi, B. (2005). The organization of recent and remote memories. Nat. Rev. Neurosci. 6, 119–130. doi: 10.1038/nrn1607
Fyhn, M., Hafting, T., Treves, A., Moser, M. B., and Moser, E. I. (2007). Hippocampal remapping and grid realignment in entorhinal cortex. Nature 446, 190–194. doi: 10.1038/NATURE05601
Gao, A., Xia, F., Guskjolen, A. J., Ramsaran, A. I., Santoro, A., Josselyn, S. A., et al. (2018). Elevation of hippocampal neurogenesis induces a temporally graded pattern of forgetting of contextual fear memories. J. Neurosci. 38, 3190–3198. doi: 10.1523/JNEUROSCI.3126-17.2018
Garthe, A., Behr, J., and Kempermann, G. (2009). Adult-generated hippocampal neurons allow the flexible use of spatially precise learning strategies. PLoS One 4:e5464. doi: 10.1371/JOURNAL.PONE.0005464
Ge, S., Yang, C., Hsu, K., Ming, G., and Song, H. (2007). A critical period for enhanced synaptic plasticity in newly generated neurons of the adult brain. Neuron 54, 559–566. doi: 10.1016/J.NEURON.2007.05.002
Gould, E., Beylin, A., Tanapat, P., Reeves, A., and Shors, T. J. (1999). Learning enhances adult neurogenesis in the hippocampal formation. Nat. Neurosci. 2, 260–265. doi: 10.1038/6365
Gozel, O., and Gerstner, W. (2021). A functional model of adult dentate gyrus neurogenesis. Elife 10:e66463. doi: 10.1016/j.neulet.2021.136176
Gu, Y., Arruda-Carvalho, M., Wang, J., Janoschka, S. R., Josselyn, S. A., Frankland, P. W., et al. (2012). Optical controlling reveals time-dependent roles for adult-born dentate granule cells. Nat. Neurosci. 15, 1700–1706. doi: 10.1038/NN.3260
Guo, N., Soden, M. E., Herber, C., Kim, M. T. W., Besnard, A., Lin, P., et al. (2018). Dentate granule cell recruitment of feedforward inhibition governs engram maintenance and remote memory generalization. Nat. Med. 24, 438–449. doi: 10.1038/nm.4491
Guskjolen, A., Kenney, J., de la Parra, J., Yeung, B., Josselyn, S., and Frankland, P. (2018). Recovery of “Lost” infant memories in mice. Curr. Biol. 28, 2283–2290.e3. doi: 10.1016/J.CUB.2018.05.059
Hebb, D. O. (2005). The organization of behavior: A neuropsychological theory. New York, NY: Psychology Press. doi: 10.4324/9781410612403
Hendricks, W. D., Chen, Y., Bensen, A. L., Westbrook, G. L., and Schnell, E. (2017). Short-term depression of sprouted mossy fiber synapses from adult-born granule cells. J. Neurosci. 37, 5722–5735. doi: 10.1523/JNEUROSCI.0761-17.2017
Hochgerner, H., Zeisel, A., Lönnerberg, P., and Linnarsson, S. (2018). Conserved properties of dentate gyrus neurogenesis across postnatal development revealed by single-cell RNA sequencing. Nat. Neurosci. 21, 290–299. doi: 10.1038/s41593-017-0056-2
Ishikawa, R., Fukushima, H., Frankland, P. W., and Kida, S. (2016). Hippocampal neurogenesis enhancers promote forgetting of remote fear memory after hippocampal reactivation by retrieval. Elife 5:e17464. doi: 10.7554/ELIFE.17464
Jungenitz, T., Beining, M., Radic, T., Deller, T., Cuntz, H., Jedlicka, P., et al. (2018). Structural homo- and heterosynaptic plasticity in mature and adult newborn rat hippocampal granule cells. Proc. Natl. Acad. Sci. U.S.A. 115, E4670–E4679. doi: 10.1073/PNAS.1801889115
Kee, N., Teixeira, C. M., Wang, A. H., and Frankland, P. W. (2007). Preferential incorporation of adult-generated granule cells into spatial memory networks in the dentate gyrus. Nat. Neurosci. 10, 355–362. doi: 10.1038/NN1847
Kempermann, G. (2012). New neurons for “survival of the fittest.”. Nat. Rev. Neurosci. 13, 727–736. doi: 10.1038/nrn3319
Kempermann, G., Gast, D., Kronenberg, G., Yamaguchi, M., and Gage, F. H. (2003). Early determination and long-term persistence of adult-generated new neurons in the hippocampus of mice. Development 130, 391–399. doi: 10.1242/DEV.00203
Kempermann, G., Kuhn, H. G., and Gage, F. H. (1997a). Genetic influence on neurogenesis in the dentate gyrus of adult mice. Proc. Natl. Acad. Sci. U.S.A. 94, 10409–10414.
Kempermann, G., Kuhn, H. G., and Gage, F. H. (1997b). More hippocampal neurons in adult mice living in an enriched environment. Nature 386, 493–495. doi: 10.1038/386493a0
Kesner, R. P., Hui, X., Sommer, T., Wright, C., Barrera, V. R., and Fanselow, M. S. (2014). The role of postnatal neurogenesis in supporting remote memory and spatial metric processing. Hippocampus 24, 1663–1671. doi: 10.1002/HIPO.22346
Kitamura, T., Ogawa, S. K., Roy, D. S., Okuyama, T., Morrissey, M. D., Smith, L. M., et al. (2017). Engrams and circuits crucial for systems consolidation of a memory. Science 356, 73–78. doi: 10.1126/SCIENCE.AAM6808
Kitamura, T., Saitoh, Y., Takashima, N., Murayama, A., Niibori, Y., Ageta, H., et al. (2009). Adult neurogenesis modulates the hippocampus-dependent period of associative fear memory. Cell 139, 814–827. doi: 10.1016/J.CELL.2009.10.020
Ko, S. Y., and Frankland, P. W. (2021). Neurogenesis-dependent transformation of hippocampal engrams. Neurosci. Lett. 762:136176. doi: 10.1016/J.NEULET.2021.136176
Kodali, M., Megahed, T., Mishra, V., Shuai, B., Hattiangady, B., and Shetty, A. K. (2016). Voluntary running exercise-mediated enhanced neurogenesis does not obliterate retrograde spatial memory. J. Neurosci. 36, 8112–8122. doi: 10.1523/JNEUROSCI.0766-16.2016
Kumar, D., Koyanagi, I., Carrier-Ruiz, A., Vergara, P., Srinivasan, S., Sugaya, Y., et al. (2020). Sparse activity of hippocampal adult-born neurons during REM sleep is necessary for memory consolidation. Neuron 107, 552–565.e10. doi: 10.1016/J.NEURON.2020.05.008
Lacefield, C. O., Itskov, V., Reardon, T., Hen, R., and Gordon, J. A. (2012). Effects of adult-generated granule cells on coordinated network activity in the dentate gyrus. Hippocampus 22, 106–116. doi: 10.1002/HIPO.20860
Leutgeb, J. K., Leutgeb, S., Moser, M. B., and Moser, E. I. (2007). Pattern separation in the dentate gyrus and CA3 of the hippocampus. Science 315, 961–966.
Li, L., He, Y., Zhao, M., and Jiang, J. (2013). Collective cell migration: Implications for wound healing and cancer invasion. Burns Trauma 1, 2321–3868.
Li, L., Sultan, S., Heigele, S., Schmidt-Salzmann, C., Toni, N., and Bischofberger, J. (2017). Silent synapses generate sparse and orthogonal action potential firing in adult-born hippocampal granule cells. Elife 6:e23612. doi: 10.7554/ELIFE.23612
Li, W., Ma, L., Yang, G., and Gan, W. B. (2017). REM sleep selectively prunes and maintains new synapses in development and learning. Nat. Neurosci. 20, 427–437. doi: 10.1038/nn.4479
Llorens-Martín, M., Jurado-Arjona, J., Avila, J., and Hernández, F. (2015). Novel connection between newborn granule neurons and the hippocampal CA2 field. Exp. Neurol. 263, 285–292. doi: 10.1016/J.EXPNEUROL.2014.10.021
Lods, M., Mortessagne, P., Pacary, E., Terral, G., Farrugia, F., Mazier, W., et al. (2022). Chemogenetic stimulation of adult neurogenesis, and not neonatal neurogenesis, is sufficient to improve long-term memory accuracy. Prog. Neurobiol. 219:102364. doi: 10.1016/J.PNEUROBIO.2022.102364
Lods, M., Pacary, E., Mazier, W., Farrugia, F., Mortessagne, P., Masachs, N., et al. (2021). Adult-born neurons immature during learning are necessary for remote memory reconsolidation in rats. Nat. Commun. 12, 1–14. doi: 10.1038/s41467-021-22069-4
Lois, C., and Alvarez-Buylla, A. (1993). Proliferating subventricular zone cells in the adult mammalian forebrain can differentiate into neurons and glia. Proc. Natl. Acad. Sci. U.S.A. 90, 2074–2077. doi: 10.1073/pnas.90.5.2074
Luna, V. M., Anacker, C., Burghardt, N. S., Khandaker, H., Andreu, V., Millette, A., et al. (2019). Adult-born hippocampal neurons bidirectionally modulate entorhinal inputs into the dentate gyrus. Science 364, 578–583. doi: 10.1126/SCIENCE.AAT8789
MacDonald, C. J., Carrow, S., Place, R., and Eichenbaum, H. (2013). Distinct hippocampal time cell sequences represent odor memories in immobilized rats. J. Neurosci. 33, 14607–14616. doi: 10.1523/JNEUROSCI.1537-13.2013
Mankin, E. A., Diehl, G. W., Sparks, F. T., Leutgeb, S., and Leutgeb, J. K. (2015). Hippocampal CA2 activity patterns change over time to a larger extent than between spatial contexts. Neuron 85, 190–201. doi: 10.1016/J.NEURON.2014.12.001
Martinez-Canabal, A., Akers, K. G., Josselyn, S. A., and Frankland, P. W. (2013). Age-dependent effects of hippocampal neurogenesis suppression on spatial learning. Hippocampus 23, 66–74. doi: 10.1002/HIPO.22054
McAvoy, K. M., Scobie, K. N., Berger, S., Russo, C., Guo, N., Decharatanachart, P., et al. (2016). Modulating neuronal competition dynamics in the dentate gyrus to rejuvenate aging memory circuits. Neuron 91, 1356. doi: 10.1016/J.NEURON.2016.08.009
McHugh, S. B., Lopes-dos-Santos, V., Gava, G. P., Hartwich, K., Tam, S. K. E., Bannerman, D. M., et al. (2022). Adult-born dentate granule cells promote hippocampal population sparsity. Nat. Neurosci. 25, 1481–1491. doi: 10.1038/s41593-022-01176-5
Mirescu, C., Peters, J. D., Noiman, L., and Gould, E. (2006). Sleep deprivation inhibits adult neurogenesis in the hippocampus by elevating glucocorticoids. Proc. Natl. Acad. Sci. U.S.A. 103, 19170–19175. doi: 10.1073/pnas.0608644103
Mishra, R., Phan, T., Kumar, P., Morrissey, Z., Gupta, M., Hollands, C., et al. (2022). Augmenting neurogenesis rescues memory impairments in Alzheimer’s disease by restoring the memory-storing neurons. J. Exp. Med. 219:e20220391. doi: 10.1084/JEM.20220391
Mongiat, L. A., Espósito, M. S., Lombardi, G., and Schinder, A. F. (2009). Reliable activation of immature neurons in the adult hippocampus. PLoS One 4:e5320. doi: 10.1371/JOURNAL.PONE.0005320
Montaron, M. F., Charrier, V., Blin, N., Garcia, P., and Abrous, D. N. (2020). Responsiveness of dentate neurons generated throughout adult life is associated with resilience to cognitive aging. Aging Cell 19:e13161. doi: 10.1111/ACEL.13161
Murray, K. D., Liu, X. B., King, A. N., Luu, J. D., and Cheng, H. J. (2020). Age-related changes in synaptic plasticity associated with mossy fiber terminal integration during adult neurogenesis. eNeuro 7, 1–16. doi: 10.1523/ENEURO.0030-20.2020
Nakashiba, T., Buhl, D. L., McHugh, T. J., and Tonegawa, S. (2009). Hippocampal CA3 output is crucial for ripple-associated reactivation and consolidation of memory. Neuron 62, 781–787. doi: 10.1016/j.neuron.2009.05.013
Nakashiba, T., Cushman, J. D., Pelkey, K. A., Renaudineau, S., Buhl, D. L., McHugh, T. J., et al. (2012). Young dentate granule cells mediate pattern separation, whereas old granule cells facilitate pattern completion. Cell 149, 188–201. doi: 10.1016/J.CELL.2012.01.046
Ngwenya, L. B., Heyworth, N. C., Shwe, Y., Moore, T. L., and Rosene, D. L. (2015). Age-related changes in dentate gyrus cell numbers, neurogenesis, and associations with cognitive impairments in the rhesus monkey. Front. Syst. Neurosci. 9:102. doi: 10.3389/FNSYS.2015.00102
Niibori, Y., Yu, T. S., Epp, J. R., Akers, K. G., Josselyn, S. A., and Frankland, P. W. (2012). Suppression of adult neurogenesis impairs population coding of similar contexts in hippocampal CA3 region. Nat. Commun. 3, 1–7. doi: 10.1038/ncomms2261
O’Donnell, C., and Sejnowski, T. J. (2014). Selective memory generalization by spatial patterning of protein synthesis. Neuron 82, 398–412. doi: 10.1016/J.NEURON.2014.02.028
O’Leary, T. P., Askari, B., Lee, B., Darby, K., Knudson, C., Ash, A. M., et al. (2021). Inhibiting adult neurogenesis differentially affects spatial learning in females and males. bioRxiv [Preprint]. doi: 10.1101/2021.10.27.466135
Ohline, S. M., Wake, K. L., Hawkridge, M. V., Dinnunhan, M. F., Hegemann, R. U., Wilson, A., et al. (2018). Adult-born dentate granule cell excitability depends on the interaction of neuron age, ontogenetic age and experience. Brain Struct. Funct. 223, 3213–3228. doi: 10.1007/S00429-018-1685-2/FIGURES/6
Park, S., Kramer, E. E., Mercaldo, V., Rashid, A. J., Insel, N., Frankland, P. W., et al. (2016). Neuronal allocation to a hippocampal engram. Neuropsychopharmacology 41, 2987–2993. doi: 10.1038/npp.2016.73
Pilz, G. A., Bottes, S., Betizeau, M., Jörg, D. J., Carta, S., Simons, B. D., et al. (2018). Live imaging of neurogenesis in the adult mouse hippocampus. Science 359, 658–662.
Rapp, P. R., and Gallagher, M. (1996). Preserved neuron number in the hippocampus of aged rats with spatial learning deficits. Proc. Natl. Acad. Sci. U.S.A. 93, 9926–9930. doi: 10.1073/PNAS.93.18.9926
Restivo, L., Niibori, Y., Mercaldo, V., Josselyn, S. A., and Frankland, P. W. (2015). Development of adult-generated cell connectivity with excitatory and inhibitory cell populations in the hippocampus. J. Neurosci. 35, 10600–10612. doi: 10.1523/JNEUROSCI.3238-14.2015
Richards, B. A., and Frankland, P. W. (2017). The persistence and transience of memory. Neuron 94, 1071–1084. doi: 10.1016/J.NEURON.2017.04.037
Ruediger, S., Vittori, C., Bednarek, E., Genoud, C., Strata, P., Sacchetti, B., et al. (2011). Learning-related feedforward inhibitory connectivity growth required for memory precision. Nature 473, 514–518. doi: 10.1038/nature09946
Ryu, J. R., Hong, C. J., Kim, J. Y., Kim, E. K., Sun, W., and Yu, S. W. (2016). Control of adult neurogenesis by programmed cell death in the mammalian brain. Mol. Brain 9, 1–20. doi: 10.1186/S13041-016-0224-4
Sahay, A., Scobie, K. N., Hill, A. S., O’Carroll, C. M., Kheirbek, M. A., Burghardt, N. S., et al. (2011). Increasing adult hippocampal neurogenesis is sufficient to improve pattern separation. Nature 472, 466–470. doi: 10.1038/nature09817
Schmidt-Hieber, C., Jones, P., and Bischofberger, J. (2004). Enhanced synaptic plasticity in newly generated granule cells of the adult hippocampus. Nature 429, 184–187. doi: 10.1038/nature02553
Scott, G. A., Terstege, D. J., Roebuck, A. J., Gorzo, K. A., Vu, A. P., Howland, J. G., et al. (2021). Adult neurogenesis mediates forgetting of multiple types of memory in the rat. Mol. Brain 14, 1–13. doi: 10.1186/S13041-021-00808-4/FIGURES/4
Semon, R. (1921). The mneme. G. Allen & Unwin Limited. Available online at: https://books.google.com/books?hl=en&lr=&id=rFfuAgAAQBAJ&oi=fnd&pg=PA17&ots=4e3JbfekP2&sig=JteMg1F1IJQZNIsq8zU6xIhvXuc (accessed February 1, 2022).
Sippel, D., Schwabedal, J., Snyder, J. C., Oyanedel, C. N., Bernas, S. N., Garthe, A., et al. (2020). Disruption of NREM sleep and sleep-related spatial memory consolidation in mice lacking adult hippocampal neurogenesis. Sci. Rep. 10, 1–14. doi: 10.1038/s41598-020-72362-3
Snyder, J. S., Hong, N. S., McDonald, R. J., and Wojtowicz, J. M. (2005). A role for adult neurogenesis in spatial long-term memory. Neuroscience 130, 843–852. doi: 10.1016/J.NEUROSCIENCE.2004.10.009
Snyder, J. S., Soumier, A., Brewer, M., Pickel, J., and Cameron, H. A. (2011). Adult hippocampal neurogenesis buffers stress responses and depressive behaviour. Nature 476, 458–461. doi: 10.1038/nature10287
Sorrells, S. F., Paredes, M. F., Cebrian-Silla, A., Sandoval, K., Qi, D., Kelley, K. W., et al. (2018). Human hippocampal neurogenesis drops sharply in children to undetectable levels in adults. Nature 555, 377–381. doi: 10.1038/nature25975
Stone, S. S. D., Teixeira, C. M., Zaslavsky, K., Wheeler, A. L., Martinez-Canabal, A., Wang, A. H., et al. (2011). Functional convergence of developmentally and adult-generated granule cells in dentate gyrus circuits supporting hippocampus-dependent memory. Hippocampus 21, 1348–1362. doi: 10.1002/HIPO.20845
Suárez-Pereira, I., and Carrión, ÁM. (2015). Updating stored memory requires adult hippocampal neurogenesis. Sci. Rep. 5, 1–7. doi: 10.1038/srep13993
Suzuki, A., Josselyn, S. A., Frankland, P. W., Masushige, S., Silva, A. J., and Kida, S. (2004). Memory Reconsolidation and Extinction Have Distinct Temporal and Biochemical Signatures. J. Neurosci. 24, 4787–4795. doi: 10.1523/JNEUROSCI.5491-03.2004
Swan, A. A., Clutton, J. E., Chary, P. K., Cook, S. G., Liu, G. G., and Drew, M. R. (2014). Characterization of the role of adult neurogenesis in touch-screen discrimination learning. Hippocampus 24, 1581–1591. doi: 10.1002/HIPO.22337
Tashiro, A., Makino, H., and Gage, F. H. (2007). Experience-specific functional modification of the dentate gyrus through adult neurogenesis: A critical period during an immature stage. J. Neurosci. 27, 3252–3259. doi: 10.1523/JNEUROSCI.4941-06.2007
Tashiro, A., Sandler, V. M., Toni, N., Zhao, C., and Gage, F. H. (2006). NMDA-receptor-mediated, cell-specific integration of new neurons in adult dentate gyrus. Nature 442, 929–933. doi: 10.1038/nature05028
Temprana, S. G., Mongiat, L. A., Yang, S. M., Trinchero, M. F., Alvarez, D. D., Kropff, E., et al. (2015). Delayed coupling to feedback inhibition during a critical period for the integration of adult-born granule cells. Neuron 85, 116–130. doi: 10.1016/J.NEURON.2014.11.023
Toni, N., Laplagne, D. A., Zhao, C., Lombardi, G., Ribak, C. E., Gage, F. H., et al. (2008). Neurons born in the adult dentate gyrus form functional synapses with target cells. Nat. Neurosci. 11, 901–907. doi: 10.1038/nn.2156
Toni, N., Teng, E. M., Bushong, E. A., Aimone, J. B., Zhao, C., Consiglio, A., et al. (2007). Synapse formation on neurons born in the adult hippocampus. Nat. Neurosci. 10, 727–734. doi: 10.1038/NN1908
Tran, L. M., Santoro, A., Liu, L., Josselyn, S. A., Richards, B. A., and Frankland, P. W. (2022). Adult neurogenesis acts as a neural regularizer. Proc. Natl. Acad. Sci. U.S.A. 119, e2206704119.
Tronel, S., Fabre, A., Charrier, V., Oliet, S. H. R., Gage, F. H., and Abrous, D. N. (2010). Spatial learning sculpts the dendritic arbor of adult-born hippocampal neurons. Proc. Natl. Acad. Sci. U.S.A. 107, 7963–7968. doi: 10.1073/PNAS.0914613107
Trouche, S., Bontempi, B., Roullet, P., and Rampon, C. (2009). Recruitment of adult-generated neurons into functional hippocampal networks contributes to updating and strengthening of spatial memory. Proc. Natl. Acad. Sci. U.S.A. 106, 5919–5924. doi: 10.1073/PNAS.0811054106
van Praag, H., Christie, B. R., Sejnowski, T. J., and Gage, F. H. (1999). Running enhances neurogenesis, learning, and long-term potentiation in mice. Proc. Natl. Acad. Sci. U.S.A. 96, 13427–13431. doi: 10.1073/PNAS.96.23.13427
Walton, N. M., Shin, R., Tajinda, K., Heusner, C. L., Kogan, J. H., Miyake, S., et al. (2012). Adult neurogenesis transiently generates oxidative stress. PLoS One 7:e35264. doi: 10.1371/JOURNAL.PONE.0035264
Weisz, V. I., and Argibay, P. F. (2009). A putative role for neurogenesis in neuro-computational terms: Inferences from a hippocampal model. Cognition 112, 229–240. doi: 10.1016/J.COGNITION.2009.05.001
Wiskott, L., Rasch, M. J., and Kempermann, G. (2006). A functional hypothesis for adult hippocampal neurogenesis: Avoidance of catastrophic interference in the dentate gyrus. Hippocampus 16, 329–343. doi: 10.1002/HIPO.20167
Yasuda, M., Johnson-Venkatesh, E. M., Zhang, H., Parent, J. M., Sutton, M. A., and Umemori, H. (2011). Multiple forms of activity-dependent competition refine hippocampal circuits in vivo. Neuron 70, 1128–1142. doi: 10.1016/J.NEURON.2011.04.027
Zhao, C., Teng, E. M., Summers, R. G., Ming, G. L., and Gage, F. H. (2006). Distinct morphological stages of dentate granule neuron maturation in the adult mouse hippocampus. J. Neurosci. 26, 3–11. doi: 10.1523/JNEUROSCI.3648-05.2006
Keywords: neurogenesis, memory, hippocampus, forgetting, pattern separation, flexibility
Citation: Fölsz O, Trouche S and Croset V (2023) Adult-born neurons add flexibility to hippocampal memories. Front. Neurosci. 17:1128623. doi: 10.3389/fnins.2023.1128623
Received: 20 December 2022; Accepted: 30 January 2023;
Published: 15 February 2023.
Edited by:
Ashok K. Shetty, Texas A&M University College of Medicine, United StatesReviewed by:
Shawn Fletcher Sorrells, University of Pittsburgh, United StatesCopyright © 2023 Fölsz, Trouche and Croset. This is an open-access article distributed under the terms of the Creative Commons Attribution License (CC BY). The use, distribution or reproduction in other forums is permitted, provided the original author(s) and the copyright owner(s) are credited and that the original publication in this journal is cited, in accordance with accepted academic practice. No use, distribution or reproduction is permitted which does not comply with these terms.
*Correspondence: Vincent Croset, dmluY2VudC5jcm9zZXRAZHVyaGFtLmFjLnVr