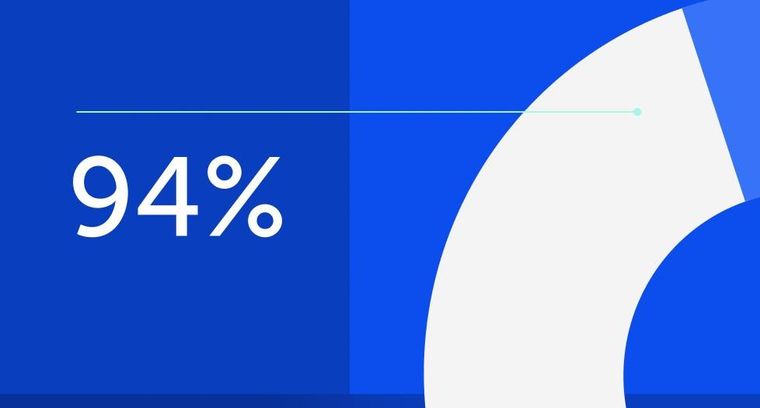
94% of researchers rate our articles as excellent or good
Learn more about the work of our research integrity team to safeguard the quality of each article we publish.
Find out more
BRIEF RESEARCH REPORT article
Front. Neurosci., 02 June 2023
Sec. Neural Technology
Volume 17 - 2023 | https://doi.org/10.3389/fnins.2023.1125476
This article is part of the Research TopicInvertebrate Neurophysiology - of Currents, Cells, and CircuitsView all 15 articles
Cilia are the major effectors in Ctenophores, but very little is known about their transmitter control and integration. Here, we present a simple protocol to monitor and quantify cilia activity and provide evidence for polysynaptic control of cilia coordination in ctenophores. We also screened the effects of several classical bilaterian neurotransmitters (acetylcholine, dopamine, L-DOPA, serotonin, octopamine, histamine, gamma-aminobutyric acid (GABA), L-aspartate, L-glutamate, glycine), neuropeptide (FMRFamide), and nitric oxide (NO) on cilia beating in Pleurobrachia bachei and Bolinopsis infundibulum. NO and FMRFamide produced noticeable inhibitory effects on cilia activity, whereas other tested transmitters were ineffective. These findings further suggest that ctenophore-specific neuropeptides could be major candidates for signal molecules controlling cilia activity in representatives of this early-branching metazoan lineage.
The phylum Ctenophora represents descendants of the earliest animal group, sister to the rest of Metazoa (e.g., Moroz et al., 2014; Whelan et al., 2017; Li et al., 2021; Schultz et al., 2023). Ctenophores or comb jellies have the largest cilia in the animal kingdom, primarily used for complex locomotion in most species within this phylum. Moreover, cilia contribute to the majority of functions and behaviors of ctenophores (Tamm, 1982; Tamm, 2014). One primary example is ctene rows, which consist of the large mechanically fused swim cilia (ctene plates) used by ctenophores to move in the water column. The coordination of multiple behaviors in ctenophores is controlled by variations in the activity of swim cilia, and these mechanisms were under intensive investigation (Tamm and Tamm, 1981; Tamm, 1983, 1984; Nakamura and Tamm, 1985; Moss and Tamm, 1986, 1987; Tamm, 1988; Tamm and Tamm, 1988; Tamm and Terasaki, 1994).
Although cilia are the main effectors in ctenophores, with presumed neuronal control and different subtypes of synapses detected by electron microscopy (Hernandez-Nicaise, 1991; Burkhardt et al., 2023), little is known about synaptic regulation and neurotransmitters controlling cilia movement. Initial identification of glutamate as a small signal molecule and neurotransmitter candidate in ctenophores (Moroz et al., 2014, 2020b, 2021) targeted muscular systems. Still, in early experiments, glutamate did not change cilia beating (Moroz et al., 2014), and some ionotropic glutamate receptors were sensitive to glycine (Alberstein et al., 2015; Yu et al., 2016).
It was proposed that neural systems evolved independently in ctenophores by developing a unique molecular and structural organization (Moroz, 2014; Dabe et al., 2015; Kohn et al., 2015; Moroz, 2015; Moroz and Kohn, 2015; Whelan et al., 2015), including a subset of ctenophore-specific secretory peptides that could act as signal molecules (Moroz, 2014; Moroz et al., 2014; Moroz, 2021). Multiple candidates were identified in Pleurobrachia and Mnemiopsis (Moroz et al., 2014; Moroz and Kohn, 2016). The recent genome-wide and mass spectroscopy survey further expanded the list of secretory peptide candidates and identified some (neuro)peptides involved in the control of cilia beating in juvenile Mnemiopsis (Sachkova et al., 2021) and Bolinopsis (Hayakawa et al., 2022). However, cellular bases of ctenophore behavior are unknown.
Quantitative recording of cilia activity in ctenophores is equally essential for behavioral and functional analyses in both juvenile and adult animals. First, we described a simple protocol successfully used to quantify the frequency of cilia beating in ctenophores. This protocol can be practical for screening and investigating the physiological roles of different transmitters. Second, we provided initial evidence of (i) polysynaptic control of cilia coordination using chemical transmission, (ii) confirmed negative results of classical bilaterian neurotransmitter action on cilia, and (iii) showed a potential regulatory role of the gaseous molecule, nitric oxide (NO), in cilia beating.
Large, 1-to-2 cm, Pleurobrachia bachei and medium-size, 3-to-4 cm, Bolinopsis infundibulum [the updated valid name for this species is currently Bolinopsis microptera (Johnson et al., 2023)] were collected from the dock at Friday Harbor Laboratories, University of Washington, in the Pacific Northwest. The animals were tightly pinned to a Sylgard-coated Petri dish (World Precision Instruments, Sylgard Silicone Elastomer, SYLG184) with small steel insect pins to prevent all body movements other than cilia beating. Most animals were used as whole-mount preparations without dissection. However, the larger animals (>2 cm Pleurobrachia and 4 cm Bolinopsis) were dissected, and parts of a body wall with 2–3 cilia rows were pinned the same way to the Petri dish. Prior to dissection, freshly caught animals were incubated in high magnesium seawater (300 mM MgCl2 added to filtered seawater at a 1:1 ratio) for about 15 min. After dissection, the preparation was washed several times in a regular seawater for over 10 min before the experiment started.
The Petri dish was placed in a standard electrophysiological rig on a recording platform and connected to the Ag/AgCl reference electrode. We used glass microelectrodes (borosilicate glass micropipettes for intracellular recording from World Precision Instruments – standard glass capillaries 2 mm diameter with a thin filament, 1B200F-4), filled with 3 M potassium acetate to record cilia beating. The sharp microelectrodes were pulled using Microelectrode Puller (Sutter Instruments, Flaming/Brown Micropipette Puller P-97). The original resistance of sharp microelectrodes (made for intracellular recordings) was around 30 MΩ. A narrow strip of thin paper was used to carefully touch the tip of the electrode to break off the most fragile sharp end. The resulting electrode was more stable to further mechanical contact and had a resistance of 5–15 MΩ. Electrodes with very low resistances (below 1 MΩ) and wider tips were unsuitable. The electrodes were then connected to the micromanipulators (Warner Instruments, Standard Manual Control Micromanipulators, MM-33) and the intracellular amplifiers (Neuroprobe 1,600, A-M Systems).
With the help of micromanipulators and under visual control via a dissecting microscope (Nikon stereoscopic microscope SMZ-10A), the tip of the electrode was carefully placed next to the cilia combs so that during cilia beating, cilia were touching the end of the electrode (Figure 1A). This physical contact created a brief electrical signal picked up by amplifiers and recorded on paper and in digital form using Gould Recorder (WindoGraf 980). Thus, each cilia beat was translated into a fast electrical spike. Combining electrophysiology with microscopy, we observed a one-to-one relationship between a cilia strike and a recorded electrical signal/spike, which allowed a digital recording of cilia beat frequency. It is important to note that this technique did not allow quantification of cilia beating amplitude and forces – only the frequency. It was crucial for stable recording to have the ctenophore body wall tightly pinned to the Sylgard-coated Petri dish, with no movements except cilia beating.
Figure 1. Recording cilia activity in Bolinopsis and Pleurobrachia. (A) Schematic diagram showing the position of a recording microelectrode near comb plates in Bolinopsis (see text for details). Illustrative examples of cilia beating recording in Pleurobrachia (B) and Bolinopsis (C–E).
Most recordings were conducted in freshly filtered seawater. We used high MgCl2 seawater only to suppress chemical/synaptic transmission and excitability in particular experiments described in the results. Muscle contractions were not a problem for cilia recording in tightly pinned preparations. Only in some very active Bolinopsis specimens a temporary withdrawal of the entire cilia row inside the body sometimes did interrupt the continuous cilia recording.
To test the possible role of different neurotransmitter candidates in cilia control, we applied them to the recording dish using a graduated 1 mL pipette attached to a long, small-diameter tube. The final concentrations were calculated from the known volume of injected solution and the known volume of the recording dish.
The following candidates for signal molecules were used in these experiments: GABA, acetylcholine, serotonin, glutamate, dopamine, histamine, glycine, aspartate, octopamine, FMRFamide, and two donors of nitric oxide (NOC-9 [6-(2-Hydroxy-1-methyl-2-nitrosohydrazino)-N-methyl-1-hexanamine], diethylamine NONOate [DEA NO or Diethylammonium (Z)-1-(N,N-diethylamino)diazen-1-ium-1,2-diolate], see details in (Maragos et al., 1991; Keefer et al., 1996; Braga et al., 2009; Li et al., 2020). All chemicals were obtained from Sigma. Specifically, we used the following concentrations on both Pleurobrachia bachei and Bolinopsis infundibulum. Gamma-aminobutyric acid (GABA), total semi-intact preparations n = 6, at concentrations: 0.1 mM, 0.2 mM and 0.5 mM (4 independent tests for each concentration– no effect); Acetylcholine (ACh), n = 5, at concentrations: 0.1 mM, 0.2 mM, 0.5 mM and (2 independent tests for each concentration – no effect); Serotonin (5-HT), n = 5 preparations, at concentrations: 0.1 mM and 0.5 mM (2 independent tests for each concentration – no effect); L-Glutamate, n = 3 preparations, at concentrations: 0.5 mM and 0.2 mM (2 independent tests for each concentration – no effect); Dopamine (DA), n = 3, at concentrations: 0.1 mM, 0.2 mM and 0.4 mM (3 independent tests for each concentration – no effect); L-DOPA (DA precursor); once at 0.5 mM – no effect; Histamine, n = 3; at concentrations: 0.5 mM, 0.4 mM and 0.1 mM (3 independent tests for each concentration – no effect); Glycine, n = 2; at concentrations: 0.4 mM, and 0.2 mM (2 independent tests for each concentration – no effect); L-Aspartate, n = 2, 0.5 mM and 0.2 mM (2 independent tests for each concentration – no effect); Octopamine, n = 2, 0.4 mM and 0.2 mM (2 independent tests for each concentration – no effect); FMRFamide, n = 8 preparations at concentrations: 0.2 mM and 0.1 mM (3–5 independent tests for each concentration – suppression of complex patterns of cilia activity in combs). Effects of NO donors: NOC-9, n = 5, at 0.1 mM and 0.2 mM (3 independent tests for each concentration –inhibition of comb’s cilia beating); Diethylamine NONOate, n = 13 at subsequent 0.02 mM, 0.06 mM, 0.1 mM, and 0.2 mM in seawater, and n = 3 in high MgCl2 (3 independent tests for each condition – inhibition of comb’s cilia beating). Details about NO donors and FMRFamide are described in the result section.
To understand whether the possible effect was direct on the cilia cells or indirect via potential interneurons and due to chemical transmission, ‘chemical isolation’ was used by bathing the preparation in high Mg2+ saline for 5–15 min (333 mM MgCl2 was added to filtered seawater at a 1:1 ratio). Elevated magnesium chloride solution suppresses synaptic chemical transmission and is widely used in comparative neurobiology (Del Castillo and Engbaek, 1954; Hutter and Kostial, 1954). All solutions were prepared immediately before use. In all experiments, we checked the effect of a candidate neurotransmitter on the frequency of cilia beating and the occurrence and intensity of bursts. The cilia beating was compared before transmitter application, after application for about 5–30 min, and then after washing in seawater for about 5–15 min (the entire volume of the experimental chamber was replaced by fresh seawater at least 5 times).
Immunohistochemical labeling was performed as described elsewhere using anti-FMRFamide antibody (Cat # AB15348, Sigma-Aldrich). See details about the protocol and Pleurobrachia neuroanatomy (Norekian and Moroz, 2019a, 2020).
In semi-intact preparations, patterns of cilia beating in Pleurobrachia were variable, with periods of bursts and inhibitory episodes (Figure 2). Such activity might represent intact behaviors in free-moving Pleurobrachia as an ambush predator. In contrast, Bolinopsis had more regular cilia beating with fewer activity patterns (Figures 1C–E), also reminiscent of its free-moving behavior. The maximum cilia beat frequency recorded during high-intensity bursting was around 40 Hz in Pleurobrachia and 20 Hz in Bolinopsis (Figures 1B,E).
Figure 2. Cilia beating in Pleurobrachia bachei was very variable and complex, similar to intact behaviors in free-moving animals. For example, (A) regular episodes of high-frequency bursting with periods of inhibition between them, (B) long-duration powerful bursts of comb cilia strikes, (C) irregular unstructured bursting of cilia movements, (D) regular cilia beating with possible brief episodes of acceleration. Numbers under all traces show the duration of recordings.
The irregular patterns of cilia activity were eliminated in the presence of a high concentration of Mg2+, known to suppress synaptic inputs (Del Castillo and Engbaek, 1954; Hutter and Kostial, 1954). The effect of high Mg2+ solution was a regular uniform cilia beating without any high-frequency bursts or inhibitory episodes, which was quickly washed out by rinses in regular seawater to restore the initial patterned activity (Figure 3). These findings indicate the presence of multifaceted regulatory chemical inputs and likely neuronal/secretory control of cilia, which was anticipated from ultrastructural data and neuro-ciliary synapses (Hernandez-Nicaise, 1991).
Figure 3. High Mg2+ seawater (B) blocked complex patterns of cilia activity [(A) – control], suggesting that synaptic inputs initiated high-frequency bursting and inhibition episodes. The regular unvarying cilia beating in high Mg2+ solution (B) was removed by washing in normal seawater, fully restoring (C) previously observed episodes of bursting and inhibition. Numbers under all traces show the time of recordings.
Cilia excitatory and cilia inhibitory transmitters are unknown for most ctenophore species. A few neuropeptides have been recently identified in Mnemiopsis leidyi (Sachkova et al., 2021) and Bolinopsis (Hayakawa et al., 2022) as putative signal molecules controlling ciliated locomotion in these species. We performed pharmacological screening of low molecular weight transmitter candidates in our ctenophore preparations. The effects of different signal molecules on cilia beating were similar in Pleurobrachia and Bolinopsis: no observable effects on the application of selected low molecular weight transmitters and inhibitory action of FMRFamide and nitric oxide donors (see below). Our screening showed an apparent lack of involvement of bilaterian neurotransmitters in the ctenophore cilia activity.
Previous pharmacological and electrophysiological tests were consistent with the hypothesis that L-glutamate could be a neuromuscular transmitter in ctenophores because of its higher efficiency in inducing muscle contractions than D-glutamate and L-aspartate (Moroz et al., 2014). However, neither L-glutamate, L-aspartate, nor any other bilaterian amino acid-derived neurotransmitters tested here (glycine, GABA, acetylcholine, serotonin, dopamine, octopamine, and histamine) could noticeably change the frequency of cilia beating in Pleurobrachia and Bolinopsis in concentrations up to 0.5 mM (see methods). These observations also support the hypothesis that acetylcholine and monoamines are bilaterian innovations (Moroz and Kohn, 2015; Moroz et al., 2021).
The first neural systems might have mainly been peptidergic (Moroz, 2009, 2021). Peptidergic signaling can significantly affect interneuronal communication in ctenophores (Moroz et al., 2014; Sachkova et al., 2021; Hayakawa et al., 2022). Pleurobrachia and Mnemiopsis genomes do not encode FMRFamide (Moroz and Kohn, 2016). However, this versatile tetrapeptide might be used as a tool to mimic the action of some other endogenous short neuropeptides. Specifically, these peptides have different conformational states (Edison et al., 1999; Espinoza et al., 2000; Dossey et al., 2006) with affinity to various receptors because they are short. When the complete list of endogenous peptides is not determined precisely (as in ctenophores), RFamide related peptides can be efficiently used as a model for initial screening for the presence of peptidergic neurons and their actions. This approach was applied here as a part of screening for modulatory action on cilia activity in Pleurobrachia.
FMRFamide had an apparent inhibitory effect on high-frequency bursts of activity in cilia (Figure 4). In 10–20 s after application, the frequency of cilia beating in bursts was reduced, and the appearance of bursts was also decreased. The whole effect could be observed within 1–2 min. Of note, there was no effect of FMRFamide in high Mg2+ seawater (Figure 5, n = 2). It suggests that the observed action of FMRFamide was indirect and polysynaptic.
Figure 4. In Pleurobrachia FMRFamide reversibly inhibited the intensity of cilia bursting activity, repressing or even eliminating the occurrence of bursts, significantly weakening the degree of cilia acceleration in the remaining bursts (A–C). Numbers under all traces show the time of recordings.
Figure 5. In high Mg2+ seawater, which blocked the variable bursting activity of Pleurobrachia cilia (A), FMRFamide did not demonstrate any noticeable effect on locomotory cilia (B), suggesting that its target was not comb’s cilia themselves but an external source controlling inputs to ciliated cells. Numbers under the traces show the time of recordings.
Because FMRFamide and other short peptides have confirmation dynamic in solutions (Edison et al., 1999; Espinoza et al., 2000; Dossey et al., 2006), we also assumed they might be cross-reactive with many endogenous peptides. We tested this situation using immunohistochemistry and revealed a distinct subset of peptidergic neurons, not reported previously (Jager et al., 2011; Norekian and Moroz, 2016, 2019, 2020). This is consistent with an observation that RFamide immunoreactivity was also detected in specific cells of the polar field in Pleurobrachia (Figure 6). These potentially chemoreceptive cells might use short neuropeptides as afferent components of neural circuits controlling locomotion via still-unknown interneurons and motoneurons.
Figure 6. FMRF-like immunoreactivity in the polar field, a putative chemosensory organ of Pleurobrachia bachei. The left image shows regularly positioned stained putative chemoreceptive cells at lower magnification. Scale bar – 100 μm. Images on the right show the same cells are at higher magnification. See details about the protocol and Pleurobrachia neuroanatomy (Norekian and Moroz, 2019a, 2020).
Nitric oxide (NO) is an ancient and versatile signal molecule (Moroz and Kohn, 2011), recently proposed as a transmitter candidate in ctenophores (Moroz and Kohn, 2016; Moroz et al., 2023). In contrast to classical transmitters, the application of NO donors (NOC-9 and Diethylamine NONOate, 0.02–0.2 mM) caused inhibition of comb cilia beating both in Pleurobrachia and Bolinopsis with a complete arrest of cilia activity in most cases at higher concentrations, 100 μM and above (Figure 7). The effect developed slowly over 1–2 min after NO-donor solution applications [half-life of NO release is reached 10–15 min after diluting the NO-donors in the seawater (Maragos et al., 1991; Keefer et al., 1996; Li et al., 2020)]. This inhibitory effect was always reversible and was washed out in the seawater with a complete restoration of pre-application activity in about 5 min. Of note, this inhibitory action of NO donors persisted in high Mg2+ seawater, suggesting the direct action of NO on the cilia in combs (Figure 8).
Figure 7. Nitric Oxide (NO) donors, such as NOC-9, reversibly suppressed cilia beating, completely inhibiting cilia movements in Pleurobrachia (A,B). This effect was reversible, and cilia beating was restored within 5 min of washing (C). Numbers under all traces show the time of recordings.
Figure 8. In high Mg2+ seawater, which blocked variable synaptic inputs and chemical transmission (A), Nitric Oxide (NO) donors noticeably inhibited cilia beating in Pleurobrachia (B), suggesting that NO targets can be comb’s cilia themselves.
Our results imply that endogenous or environmental NO suppresses the cilia beating in ctenophores. Gaseous NO is one of the smallest and most diffusible signal molecules, with multiple non-enzymatic and enzymatic synthetic pathways, including nitric oxide synthase (NOS) in both host cells and microbiome (Moroz and Kohn, 2011). Interestingly, the screening of the sequenced genome in Pleurobrachia and several transcriptomes from this species did not recover any NOS-like enzymes (Moroz et al., 2014). However, NOS was detected in basal and more derived species of ctenophores, such as Mnemiopsis leidyi (Moroz and Kohn, 2016; Moroz et al., 2020a) and Bolinopsis (Moroz et al., 2023). These comparative analyses illustrate the mosaic nature of NOS distribution within the phylum Ctenophora and provide evidence for the secondary loss of NOS in Pleurobrachia from the common ancestor of ctenophores (Moroz et al., 2023). However, Pleurobrachia has soluble guanylyl cyclases and possibly other receptors for NO, which might sense this molecule from alternative endogenous and exogenous sources (e.g., microbiomes and/or food).
Ctenophores is the earliest lineage of metazoans (Whelan et al., 2015, 2017; Li et al., 2021; Schultz et al., 2023), central to understanding the origins and fundamental principles of animal organization. The life of ctenophores is entirely based on cilia, with dozens of populations of ciliated cells (Tamm, 1982; Hernandez-Nicaise, 1991; Tamm, 2014; Norekian and Moroz, 2019b). As a result, multi-transmitter control of cilia activity is paramount to ctenophore organization and behaviors.
NO-cilia interactions can be one of the ancient signaling pathways in the evolution of animals, but this is a little investigated direction, with no comparative data (Saternos and Aboualaiwi, 2018). Thus, it would be essential to identify both sources and mechanisms of the action of NO on cilia in different ecological groups of ctenophores. Experiments on other ctenophore species are imperative because of the mosaic distribution of NOS across species, with examples of secondary loss of this enzyme in many lineages (Moroz et al., 2023).
Second, the observed suppression of complex ciliary patterns by MgCl2 indicates the significance of steady-state chemical transmission in generating ctenophore behaviors. This experiment is important because of the recently discovered syncytial organization of five ctenophore neurons in the subepithelial nerve net of early developmental stages of Mnemiopsis (Burkhardt et al., 2023). The finding might be interpreted as support for the widespread role of non-synaptic and non-chemical transmission in ctenophores (Dunn, 2023). However, the majority of neurons in ctenophores and external control of cilia activities are likely mediated by chemical transmission. Specifically, distinct ctenophore neural systems can employ well-recognized synapses already detected by electron microscopy (Hernandez-Nicaise, 1991; Burkhardt et al., 2023) and volume-type intercellular transmission (Moroz et al., 2021) mediated by small peptides, nitric oxide and, perhaps, additional low molecular weight messengers to be determined in future studies.
The precise balance and complementary contributions of different transmitter mechanisms in ctenophores are the areas of exciting discoveries essential for fundamental neuroscience and deciphering the evolution of alternative integrative systems across basal metazoan lineages (Jekely, 2021; Moroz et al., 2021; Moroz and Romanova, 2022; Moroz et al., 2004; Nikitin et al., 2023).
In conclusion, we would like to add that both Pleurobrachia and Bolinopsis (but especially Pleurobrachia) have complicated cilia activity patterns, including high-frequency bursts, periods of inhibition, and more regular activity. Some of these are reported in illustrative figures. Those patterns and frequencies could also be different across animals and maybe also depend on the age, size, reproductive, and nutrition status of wild animals collected in nature. The overall scope of ctenophore behaviors is comparable to bilaterian animals and is currently understudied. We understood that more detailed and systematic future analysis might reveal some subtle modulatory effects of various chemical signals (neuronal-derived and non-neuronal transmitters), which is the subject of future, more detailed studies. Considering the variability of animals and their states, we anticipate that this direction would include future analysis of hundreds of individuals. The proposed protocol is one of the first steps in this direction to be expanded and correlated with detailed and complex behaviors of intact free-swimming animals in natural habitats.
The raw data supporting the conclusions of this article will be made available by the authors, without undue reservation.
TPN and LLM designed the study, jointly performed experiments, wrote the manuscript, reviewed, and edited the manuscript. All authors contributed to the article and approved the submitted version.
This work was supported in part by the Human Frontiers Science Program (RGP0060/2017) and National Science Foundation (1146575, 1557923, 1548121 and 1645219) grants to LLM. Research reported in this publication was also supported in part by the National Institute of Neurological Disorders and Stroke of the National Institutes of Health under Award Number R01NS114491 (to LM). The content is solely the authors’ responsibility and does not necessarily represent the official views of the National Institutes of Health.
The authors declare that the research was conducted in the absence of any commercial or financial relationships that could be construed as a potential conflict of interest.
All claims expressed in this article are solely those of the authors and do not necessarily represent those of their affiliated organizations, or those of the publisher, the editors and the reviewers. Any product that may be evaluated in this article, or claim that may be made by its manufacturer, is not guaranteed or endorsed by the publisher.
Alberstein, R., Grey, R., Zimmet, A., Simmons, D. K., and Mayer, M. L. (2015). Glycine activated ion channel subunits encoded by ctenophore glutamate receptor genes. Proc. Natl. Acad. Sci. U. S. A. 112, E6048–E6057. doi: 10.1073/pnas.1513771112
Braga, A. A., Aguiar, D. C., and Guimaraes, F. S. (2009). NOC-9, a selective nitric oxide donor, induces flight reactions in the dorsolateral periaqueductal gray of rats by activating soluble guanylate cyclase. Neurosci. Lett. 459, 79–83. doi: 10.1016/j.neulet.2009.05.009
Burkhardt, P., Colgren, J., Medhus, A., Digel, L., Naumann, B., Soto-Angel, J. J., et al. (2023). Syncytial nerve net in a ctenophore adds insights on the evolution of nervous systems. Science 380, 293–297. doi: 10.1126/science.ade5645
Dabe, E. C., Sanford, R. S., Kohn, A. B., Bobkova, Y., and Moroz, L. L. (2015). DNA methylation in basal metazoans: insights from ctenophores. Integr. Comp. Biol. 55, 1096–1110. doi: 10.1093/icb/icv086
Del Castillo, J., and Engbaek, L. (1954). The nature of the neuromuscular block produced by magnesium. J. Physiol. 124, 370–384. doi: 10.1113/jphysiol.1954.sp005114
Dossey, A. T., Reale, V., Chatwin, H., Zachariah, C., Debono, M., Evans, P. D., et al. (2006). NMR analysis of Caenorhabditis elegans FLP-18 neuropeptides: implications for NPR-1 activation. Biochemistry 45, 7586–7597. doi: 10.1021/bi0603928
Dunn, C. (2023). Neurons that connect without synapses. Science 380, 241–242. doi: 10.1126/science.adh0542
Edison, A. S., Espinoza, E., and Zachariah, C. (1999). Conformational ensembles: the role of neuropeptide structures in receptor binding. J. Neurosci. 19, 6318–6326. doi: 10.1523/JNEUROSCI.19-15-06318.1999
Espinoza, E., Carrigan, M., Thomas, S. G., Shaw, G., and Edison, A. S. (2000). A statistical view of FMRFamide neuropeptide diversity. Mol. Neurobiol. 21, 35–56. doi: 10.1385/MN:21:1-2:035
Hayakawa, E., Guzman, C., Horiguchi, O., Kawano, C., Shiraishi, A., Mohri, K., et al. (2022). Mass spectrometry of short peptides reveals common features of metazoan peptidergic neurons. Nat. Ecol. Evol. 6, 1438–1448. doi: 10.1038/s41559-022-01835-7
Hernandez-Nicaise, M.-L. (1991). “Ctenophora” in Microscopic Anatomy of Invertebrates: Placozoa, Porifera, Cnidaria, and Ctenophora. eds. F. W. F. W. Harrison and J. A. Westfall (New York: Wiley), 359–418.
Hutter, O. F., and Kostial, K. (1954). Effect of magnesium and calcium ions on the release of acetylcholine. J. Physiol. 124, 234–241. doi: 10.1113/jphysiol.1954.sp005102
Jager, M., Chiori, R., Alié, A., Dayraud, C., Quéinnec, E., and Manuel, M. (2011). New insights on ctenophore neural anatomy: immunofluorescence study in Pleurobrachia pileus (Müller, 1776). J. Exp. Zool. B Mol. Dev. Evol. 316B, 171–187. doi: 10.1002/jez.b.21386
Jekely, G. (2021). The chemical brain hypothesis for the origin of nervous systems. Philos. Trans. R. Soc. Lond. Ser. B Biol. Sci. 376:20190761. doi: 10.1098/rstb.2019.0761
Johnson, S. B., Winnikoff, J. R., Schultz, D. T., Christianson, L. M., Patry, W. L., Mills, C. E., et al. (2022). Speciation of pelagic zooplankton: Invisible boundaries can drive isolation of oceanic ctenophores. Front Genet 13:970314. doi: 10.3389/fgene.2022.970314
Keefer, L. K., Nims, R. W., Davies, K. M., and Wink, D. A. (1996). "NONOates" (1-substituted diazen-1-ium-1,2-diolates) as nitric oxide donors: convenient nitric oxide dosage forms. Methods Enzymol. 268, 281–293. doi: 10.1016/s0076-6879(96)68030-6
Kohn, A. B., Sanford, R. S., Yoshida, M. A., and Moroz, L. L. (2015). Parallel evolution and lineage-specific expansion of RNA editing in ctenophores. Integr. Comp. Biol. 55, 1111–1120. doi: 10.1093/icb/icv065
Li, B., Ming, Y., Liu, Y., Xing, H., Fu, R., Li, Z., et al. (2020). Recent developments in pharmacological effect, mechanism and application Prospect of Diazeniumdiolates. Front. Pharmacol. 11:923. doi: 10.3389/fphar.2020.00923
Li, Y., Shen, X. X., Evans, B., Dunn, C. W., and Rokas, A. (2021). Rooting the animal tree of life. Mol. Biol. Evol. 38, 4322–4333. doi: 10.1093/molbev/msab170
Maragos, C. M., Morley, D., Wink, D. A., Dunams, T. M., Saavedra, J. E., Hoffman, A., et al. (1991). Complexes of.NO with nucleophiles as agents for the controlled biological release of nitric oxide. Vasorelaxant effects. J. Med. Chem. 34, 3242–3247. doi: 10.1021/jm00115a013
Moroz, L. L. (2009). On the independent origins of complex brains and neurons. Brain Behav. Evol. 74, 177–190. doi: 10.1159/000258665
Moroz, L. L. (2014). The genealogy of genealogy of neurons. Commun. Integr. Biol. 7:e993269. doi: 10.4161/19420889.2014.993269
Moroz, L. L. (2015). Convergent evolution of neural systems in ctenophores. J. Exp. Biol. 218, 598–611. doi: 10.1242/jeb.110692
Moroz, L. L. (2021). Multiple origins of neurons from secretory cells. Front. Cell Dev. Biol. 9:669087. doi: 10.3389/fcell.2021.669087
Moroz, L. L., Kocot, K. M., Citarella, M. R., Dosung, S., Norekian, T. P., Povolotskaya, I. S., et al. (2014). The ctenophore genome and the evolutionary origins of neural systems. Nature 510, 109–114. doi: 10.1038/nature13400
Moroz, L. L., and Kohn, A. B. (2011). Parallel evolution of nitric oxide signaling: diversity of synthesis and memory pathways. Front. Biosci. (Landmark Ed) 16, 2008–2051. doi: 10.2741/3837
Moroz, L. L., and Kohn, A. B. (2015). Unbiased view of synaptic and neuronal gene complement in ctenophores: are there Pan-neuronal and Pan-synaptic genes across Metazoa? Integr. Comp. Biol. 55, icv104–icv1049. doi: 10.1093/icb/icv104
Moroz, L. L., and Kohn, A. B. (2016). Independent origins of neurons and synapses: insights from ctenophores. Philos. Trans. R. Soc. Lond. Ser. B Biol. Sci. 371:20150041. doi: 10.1098/rstb.2015.0041
Moroz, L. L., Meech, R. W., Sweedler, J. V., and Mackie, G. O. (2004). Nitric oxide regulates swimming in the jellyfish Aglantha digitale. J Comp Neurol 471, 26–36. doi: 10.1002/cne.20023
Moroz, L. L., Mukherjee, K., and Romanova, D. Y. (2023). Nitric oxide signaling in ctenophores. Front. Neurosci. 17:1125433. doi: 10.3389/fnins.2023.1125433
Moroz, L. L., and Romanova, D. Y. (2022). Alternative neural systems: what is a neuron? (ctenophores, sponges and placozoans). Front. Cell Dev. Biol. 10:1071961. doi: 10.3389/fcell.2022.1071961
Moroz, L. L., Romanova, D. Y., and Kohn, A. B. (2021). Neural versus alternative integrative systems: molecular insights into origins of neurotransmitters. Philos. Trans. R. Soc. Lond. Ser. B Biol. Sci. 376:20190762. doi: 10.1098/rstb.2019.0762
Moroz, L. L., Romanova, D. Y., Nikitin, M. A., Sohn, D., Kohn, A. B., Neveu, E., et al. (2020a). The diversification and lineage-specific expansion of nitric oxide signaling in Placozoa: insights in the evolution of gaseous transmission. Sci. Rep. 10:13020. doi: 10.1038/s41598-020-69851-w
Moroz, L. L., Sohn, D., Romanova, D. Y., and Kohn, A. B. (2020b). Microchemical identification of enantiomers in early-branching animals: lineage-specific diversification in the usage of D-glutamate and D-aspartate. Biochem. Biophys. Res. Commun. 527, 947–952. doi: 10.1016/j.bbrc.2020.04.135
Moss, A. G., and Tamm, S. L. (1986). Electrophysiological control of ciliary motor responses in the ctenophore Pleurobrachia. J. Comp. Physiol. A 158, 311–330. doi: 10.1007/BF00603615
Moss, A. G., and Tamm, S. L. (1987). A calcium regenerative potential controlling ciliary reversal is propagated along the length of ctenophore comb plates. Proc. Natl. Acad. Sci. U. S. A. 84, 6476–6480. doi: 10.1073/pnas.84.18.6476
Nakamura, S., and Tamm, S. L. (1985). Calcium control of ciliary reversal in ionophore-treated and ATP-reactivated comb plates of ctenophores. J. Cell Biol. 100, 1447–1454. doi: 10.1083/jcb.100.5.1447
Nikitin, M. A., Romanova, D. Y., Borman, S. I., and Moroz, L. L. (2023). Amino acids integrate behaviors in nerveless placozoans. Front. Neurosci. 17, 1–21. doi: 10.3389/fnins.2023.1125624
Norekian, T. P., and Moroz, L. L. (2016). Development of neuromuscular organization in the ctenophore Pleurobrachia bachei. J. Comp. Neurol. 524, 136–151. doi: 10.1002/cne.23830
Norekian, T. P., and Moroz, L. L. (2019a). Neuromuscular organization of the ctenophore Pleurobrachia bachei. J. Comp. Neurol. 527, 406–436. doi: 10.1002/cne.24546
Norekian, T. P., and Moroz, L. L. (2019b). Neural system and receptor diversity in the ctenophore Beroe abyssicola. J Comp Neurol 527, 1986–2008. doi: 10.1002/cne.24633
Norekian, T. P., and Moroz, L. L. (2020). Comparative neuroanatomy of ctenophores: neural and muscular systems in Euplokamis dunlapae and related species. J. Comp. Neurol. 528, 481–501. doi: 10.1002/cne.24770
Sachkova, M. Y., Nordmann, E. L., Soto-Angel, J. J., Meeda, Y., Gorski, B., Naumann, B., et al. (2021). Neuropeptide repertoire and 3D anatomy of the ctenophore nervous system. Curr. Biol. 31:e5276, 5274–5285.e6. doi: 10.1016/j.cub.2021.09.005
Saternos, H. C., and Aboualaiwi, W. A. (2018). Signaling interplay between primary cilia and nitric oxide: a mini review. Nitric Oxide 80, 108–112. doi: 10.1016/j.niox.2018.08.003
Schultz, D. T., Haddock, S. H. D., Bredeson, J. V., Green, R. E., Simakov, O., and Rokhsar, D. S. (2023). Ancient gene linkages support ctenophores as sister to other animals. Nature. doi: 10.1038/s41586-023-05936-6
Tamm, S. L. (1982). “Ctenophora” in Electrical conduction and behavior in simple invertebrates (Oxford: Clarendon Press), 266–358.
Tamm, S. L. (1983). Motility and mechanosensitivity of macrocilia in the ctenophore Beroe. Nature 305, 430–433. doi: 10.1038/305430a0
Tamm, S. L. (1984). Mechanical synchronization of ciliary beating within comb plates of ctenophores. J. Exp. Biol. 113, 401–408. doi: 10.1242/jeb.113.1.401
Tamm, S. L. (1988). Calcium activation of macrocilia in the ctenophore Beroe. J. Comp. Physiol. A 163, 23–31. doi: 10.1007/BF00611993
Tamm, S. L. (2014). Cilia and the life of ctenophores. Invertebr. Biol. 133, 1–46. doi: 10.1111/ivb.12042
Tamm, S. L., and Tamm, S. (1981). Ciliary reversal without rotation of axonemal structures in ctenophore comb plates. J. Cell Biol. 89, 495–509. doi: 10.1083/jcb.89.3.495
Tamm, S. L., and Tamm, S. (1988). Development of macrociliary cells in Beroe. II. Formation of macrocilia. J. Cell Sci. 89, 81–95. doi: 10.1242/jcs.89.1.81
Tamm, S. L., and Terasaki, M. (1994). Visualization of calcium transients controlling orientation of ciliary beat. J. Cell Biol. 125, 1127–1135. doi: 10.1083/jcb.125.5.1127
Whelan, N. V., Kocot, K. M., Moroz, L. L., and Halanych, K. M. (2015). Error, signal, and the placement of Ctenophora sister to all other animals. Proc. Natl. Acad. Sci. U. S. A. 112, 5773–5778. doi: 10.1073/pnas.1503453112
Whelan, N. V., Kocot, K. M., Moroz, T. P., Mukherjee, K., Williams, P., Paulay, G., et al. (2017). Ctenophore relationships and their placement as the sister group to all other animals. Nat. Ecol. Evol. 1, 1737–1746. doi: 10.1038/s41559-017-0331-3
Keywords: Ctenophora, electrophysiology, behavior, neuropeptides, nitric oxide, Pleurobrachia, Bolinopsis, Mnemiopsis
Citation: Norekian TP and Moroz LL (2023) Recording cilia activity in ctenophores: effects of nitric oxide and low molecular weight transmitters. Front. Neurosci. 17:1125476. doi: 10.3389/fnins.2023.1125476
Received: 16 December 2022; Accepted: 03 May 2023;
Published: 02 June 2023.
Edited by:
James Newcomb, New England College, United StatesReviewed by:
Kei Jokura, University of Exeter, United KingdomCopyright © 2023 Norekian and Moroz. This is an open-access article distributed under the terms of the Creative Commons Attribution License (CC BY). The use, distribution or reproduction in other forums is permitted, provided the original author(s) and the copyright owner(s) are credited and that the original publication in this journal is cited, in accordance with accepted academic practice. No use, distribution or reproduction is permitted which does not comply with these terms.
*Correspondence: Leonid L. Moroz, bW9yb3pAd2hpdG5leS51ZmwuZWR1
†These authors have contributed equally to this work
Disclaimer: All claims expressed in this article are solely those of the authors and do not necessarily represent those of their affiliated organizations, or those of the publisher, the editors and the reviewers. Any product that may be evaluated in this article or claim that may be made by its manufacturer is not guaranteed or endorsed by the publisher.
Research integrity at Frontiers
Learn more about the work of our research integrity team to safeguard the quality of each article we publish.