- 1The Miami Project to Cure Paralysis, University of Miami, Miami, FL, United States
- 2Neuroscience Graduate Program, University of Miami, Miami, FL, United States
- 3Department of Radiology, University of Miami, Miami, FL, United States
- 4Department of Neurological Surgery, University of Miami, Miami, FL, United States
Traumatic brain injury (TBI) can lead to a variety of comorbidities, including chronic pain. Although brain tissue metabolite alterations have been extensively examined in several chronic pain populations, it has received less attention in people with TBI. Thus, the primary aim of this study was to compare brain tissue metabolite levels in people with TBI and chronic pain (n = 16), TBI without chronic pain (n = 17), and pain-free healthy controls (n = 31). The metabolite data were obtained from participants using whole-brain proton magnetic resonance spectroscopic imaging (1H-MRSI) at 3 Tesla. The metabolite data included N-acetylaspartate, myo-inositol, total choline, glutamate plus glutamine, and total creatine. Associations between N-acetylaspartate levels and pain severity, neuropathic pain symptom severity, and psychological variables, including anxiety, depression, post-traumatic stress disorder (PTSD), and post-concussive symptoms, were also explored. Our results demonstrate N-acetylaspartate, myo-inositol, total choline, and total creatine alterations in pain-related brain regions such as the frontal region, cingulum, postcentral gyrus, and thalamus in individuals with TBI with and without chronic pain. Additionally, NAA levels in the left and right frontal lobe regions were positively correlated with post-concussive symptoms; and NAA levels within the left frontal region were also positively correlated with neuropathic pain symptom severity, depression, and PTSD symptoms in the TBI with chronic pain group. These results suggest that neuronal integrity or density in the prefrontal cortex, a critical region for nociception and pain modulation, is associated with the severity of neuropathic pain symptoms and psychological comorbidities following TBI. Our data suggest that a combination of neuronal loss or dysfunction and maladaptive neuroplasticity may contribute to the development of persistent pain following TBI, although no causal relationship can be determined based on these data.
1. Introduction
Survivors of traumatic brain injury (TBI) experience a wide range of physical and mental health problems, including sensorimotor, behavioral, and neuropsychological deficits (Ponsford et al., 2014; Bramlett and Dietrich, 2015; Gardner and Zafonte, 2016; Irvine and Clark, 2018; Ng and Lee, 2019). It is estimated that TBI contributed to approximately 224,000 annual hospitalizations and over 64,000 deaths in 2020 in the United States (Centers for Disease Control and Prevention [CDC], 2022). Domestic surveillance data reveal that between 3.2 and 5.3 million individuals are currently living with at least one TBI-related disability, which further emphasizes the burden of TBI in the US population (Centers for Disease Control and Prevention [CDC], 2015). Clinical studies suggest that many TBI-associated symptoms, including pain (Prins et al., 2013; Rabinowitz and Levin, 2014; Bramlett and Dietrich, 2015; Irvine and Clark, 2018; Ng and Lee, 2019; Pavlovic et al., 2019; Capizzi et al., 2020), persist over an extended period. Incidentally, more than 50% of individuals with TBI report chronic pain (Lahz and Bryant, 1996; Nampiaparampil, 2008). While pathophysiological mechanisms underlying the development of chronic pain after TBI are not entirely understood, those individuals reporting chronic pain frequently present with a combination of characteristics that resemble those of neuropathic pain conditions, including sensory alterations and psychological comorbidities (Ofek and Defrin, 2007; Widerström-Noga et al., 2016; Irvine and Clark, 2018; Khoury and Benavides, 2018; Bouferguène et al., 2019; Leung, 2020; Robayo et al., 2022). Thus, chronic neuropathic pain can be a consequence of trauma or diseases involving the central or peripheral nervous system, such as TBI (Ofek and Defrin, 2007; Scholz et al., 2019; Robayo et al., 2022).
Findings from preclinical TBI research can offer insights into the molecular and cellular basis for developing chronic neuropathic pain symptoms, including central and peripheral sensitization, prolonged disinhibition within thalamocortical circuits, and the derangement of glial cell functions (Ji et al., 2013; Groh et al., 2018; den Boer et al., 2019). In humans, significant mechanistic insights have been gained through pain phenotyping based on symptoms, signs, and biomarkers, including brain imaging. Indeed, neuroimaging studies have identified both functional and structural abnormalities within specific pain-related brain regions in individuals reporting neuropathic pain (Geha and Apkarian, 2005; Moisset and Bouhassira, 2007; Alshelh et al., 2016; Mills et al., 2018). Numerous brain regions, including the thalamus, anterior cingulate cortex (ACC), insula, prefrontal cortex (PFC), and somatosensory cortices (S1 and S2), are involved in processing sensory-discriminative (e.g., location and intensity), cognitive-evaluative, and affective-emotional aspects of pain (Bushnell et al., 2013; Kuner and Kuner, 2021; Mercer Lindsay et al., 2021). Each of these regions uniquely contributes to the overall modulation and resulting perceptual experience (Davis and Moayedi, 2013; Bastuji et al., 2016; Garcia-Larrea and Bastuji, 2018). The prefrontal cortex, has been implicated in attentional and evaluative processes of pain, including attention, anticipation, learning, and cognitive control (Bushnell et al., 2013; Tan and Kuner, 2021). Common findings reported in the literature include increased prefrontal cortex functional activation and decreased gray matter volume in individuals with chronic pain (Seminowicz and Moayedi, 2017). Interestingly, the activity of the prefrontal cortex can be modulated by practicing mindfulness and meditation (Allen et al., 2012). The insula has been implicated in coding pain intensity, whereby electrical stimulation of this structure appears to elicit pain, and lesions affecting the insula can lead to the development of neuropathic pain (Garcia-Larrea et al., 2010; Garcia-Larrea and Peyron, 2013). The insula receives input from the spinothalamic tract and has also been implicated in mediating complex cognitive processes, including emotional awareness (Craig, 2009; Starr et al., 2009). The anterior cingulate cortex (ACC) and cingulum are components of the limbic system, thought to be involved in the affective-emotional components of the pain experience (Bushnell et al., 2013; Kuner and Kuner, 2021). The ACC can modulate both sensory and affective aspects of pain via activation of various receptor systems, including μ-opioid and gamma-aminobutyric acid (GABA) receptors, and activation of the periaqueductal gray (PAG) (Fuchs et al., 2014; Benarroch, 2020). Preclinical studies show that the ACC can undergo profound functional and structural changes during acute and chronic pain (Bliss et al., 2016), and such changes have also been associated with depression in human patients (Holtzheimer et al., 2017). The postcentral gyrus or primary somatosensory cortex is commonly activated during induced pain and implicated in the sensory-discriminative (e.g., location and intensity) components of the pain experience (Apkarian et al., 2005; Kuner and Kuner, 2021; Tan and Kuner, 2021). Greater functional connectivity between the ACC and postcentral gyrus has been observed in adults with chronic pain (Youssef et al., 2019). Lastly, the thalamus is the primary relay station for sensory information, and it also communicates with the cortex (Apkarian et al., 2005; Kuner and Kuner, 2021). Furthermore, the thalamus is rich in opioid receptors and, therefore, may also be involved in pain modulation (Apkarian et al., 2005).
Chronic neuropathic pain has been associated with increased activation of the ACC, insula, PFC, S1, and S2 and decreased thalamic activity (Moisset and Bouhassira, 2007; Friebel et al., 2011; Lin, 2014), accompanied by an altered thalamocortical rhythm (Walton and Llinás, 2010; Alshelh et al., 2016). Similarly, studies evaluating structural abnormalities found lower volume in the thalamus, ACC, PFC, and anterior insula, and greater volume in the periaqueductal gray (PAG) and posterior insula in individuals with neuropathic pain compared to healthy controls (Pan et al., 2015; Henssen et al., 2019). These findings suggest that abnormalities in cortical and subcortical brain regions may alter the perception and modulation of pain signals (i.e., maladaptive pain processing) (Kuner and Flor, 2017) and result in chronic neuropathic pain.
Brain imaging modalities such as magnetic resonance imaging (MRI) and magnetic resonance spectroscopy (MRS) are often used to identify brain tissue and metabolic abnormalities associated with TBI (Brooks W. M. et al., 2001; Croall et al., 2015; Faghihi et al., 2017; Smith et al., 2019) and neuropathic pain conditions (Geha and Apkarian, 2005; Moisset and Bouhassira, 2007; Alshelh et al., 2016; Mills et al., 2018). Specifically, proton magnetic resonance spectroscopy imaging (1H-MRSI) is a non-invasive MRI technique that allows the acquisition of spectroscopic data for several brain metabolites, including N-acetyl aspartate (NAA- a proxy for neuronal density and viability), total choline (tCho – an indicator of cell membrane integrity), glutamate and glutamine (Glx – excitatory neurotransmitter and its precursor), total creatine (tCre – a biomarker of cellular energetics), and myo-inositol (m-Ins – an indicator for glial cell density and inflammation) (Miller et al., 1996; Govindaraju et al., 2000; Brooks W. M. et al., 2001; Bak et al., 2006; Moffett et al., 2007; Govind et al., 2010; Chang et al., 2013; Croall et al., 2015; Bartnik-Olson et al., 2019). While metabolite alterations across brain regions have been examined in multiple chronic pain conditions (Fukui et al., 2006; Chang et al., 2013; Widerström-Noga et al., 2013; Ito et al., 2017; Levins et al., 2019; Peek et al., 2020), including neuropathic pain (Chang et al., 2013; Widerström-Noga et al., 2015), only a few research studies have focused on those with TBI and comorbid pain (Widerström-Noga et al., 2016; Lin et al., 2022). Moreover, prior TBI studies have utilized single-voxel or multi-voxel-single slice or multi-slice MRS techniques (Babikian et al., 2010; Widerström-Noga et al., 2016) for the evaluation of brain metabolites within a region or multiple regions of interest (ROIs). However, these limited spatial coverage MRS techniques may not be sufficient to enclose injured brain regions spread across the brain; this might limit assessments of brain metabolites in cortical and subcortical brain regions responsible for the processing of pain (Lin et al., 2022). The use of a whole-brain 1H-MRSI technique in individuals with TBI (Govind et al., 2010; Maudsley et al., 2015; Lin et al., 2022) has largely overcome the spatial coverage limitation of the brain and permits the evaluation of metabolite alterations following TBI during acute and subacute phases (Govind et al., 2010; Harris et al., 2012; DeVience et al., 2017; Bartnik-Olson et al., 2021). Yet, there remains limited research investigating changes in NAA, tCho, Glx, tCre, and m-Ins among those reporting pain in the chronic disease stage.
Our laboratory has previously assessed the concentration of metabolite levels and their respective ratios among those with TBI and subacute pain (Widerström-Noga et al., 2016). However, no studies have examined such alterations in those with chronic pain following TBI. Furthermore, there is limited prior research that evaluated brain metabolite differences among individuals after TBI with- and without pain and pain-free healthy controls. Such information may provide insight into potential mechanisms associated with the development of chronic pain in those with TBI. Therefore, the purpose of this study was to obtain whole-brain 1H-MRSI measures in participants with chronic TBI and pain, and compare their metabolite profiles to those without pain and healthy controls across several pain-relevant brain regions. It was hypothesized that participants with chronic TBI and comorbid pain would exhibit altered brain metabolism compared to those with TBI and no pain and healthy controls. We also examined associations between metabolite levels, chronic pain symptoms, and psychological measures among the TBI with pain group.
2. Materials and methods
2.1. Study participants
Sixty-eight adult participants were enrolled in this study. Of them, thirty-one were healthy controls (HC, N = 31), and thirty-seven had closed-head TBI (TBI, N = 37). Participants with TBI were categorized into those with chronic pain (TBI-P, N = 19) and those without pain (TBI-NP, N = 18). Demographic and injury characteristics for all participants are shown in Table 1. In addition, all participants were: (1) fluent in English, (2) free from significant cognitive impairment using the MMSE-2 (PAR, 2020), (3) with no recent history of alcohol or drug abuse, (4) with no severe major depression, and (5) free from other neurological diseases (e.g., multiple sclerosis) or trauma (e.g., spinal cord injury). Enrolled TBI participants had experienced their injury six to two hundred seventy-six months before the start of the study and were thus considered to be in the chronic time period following injury (Mayer et al., 2017). The severity of TBI in each participant was determined based on the Glasgow Coma Scale (GCS) score when available. Participants were recruited through advertisements posted at the University of Miami Medical Campus and the Health and Human Services/National Institute on Disability, Independent Living, and Rehabilitation Research (HHS/NIDILRR) organization, South Florida TBI Model System center, the Transforming Research and Clinical Knowledge in Traumatic Brain Injury (TRACK-TBI) study center within the University of Miami (UM), and by word of mouth. TBI participants provided medical records with proof of head/brain injury obtained from their medical care provider or insurance company unless they were directly referred from the HHS/NIDILRR, South Florida TBI Model System center, or the TRACK-TBI study center. The institutional review board of the University of Miami approved the study protocol, and all participants signed an informed consent form before participation. The data presented in this article is a subset of a more extensive study involving pain, quantitative sensory and psychological/psychosocial evaluations, and brain imaging in individuals with TBI. Pain, quantitative sensory, and psychological/psychosocial data of this larger cohort have been published (Robayo et al., 2022).
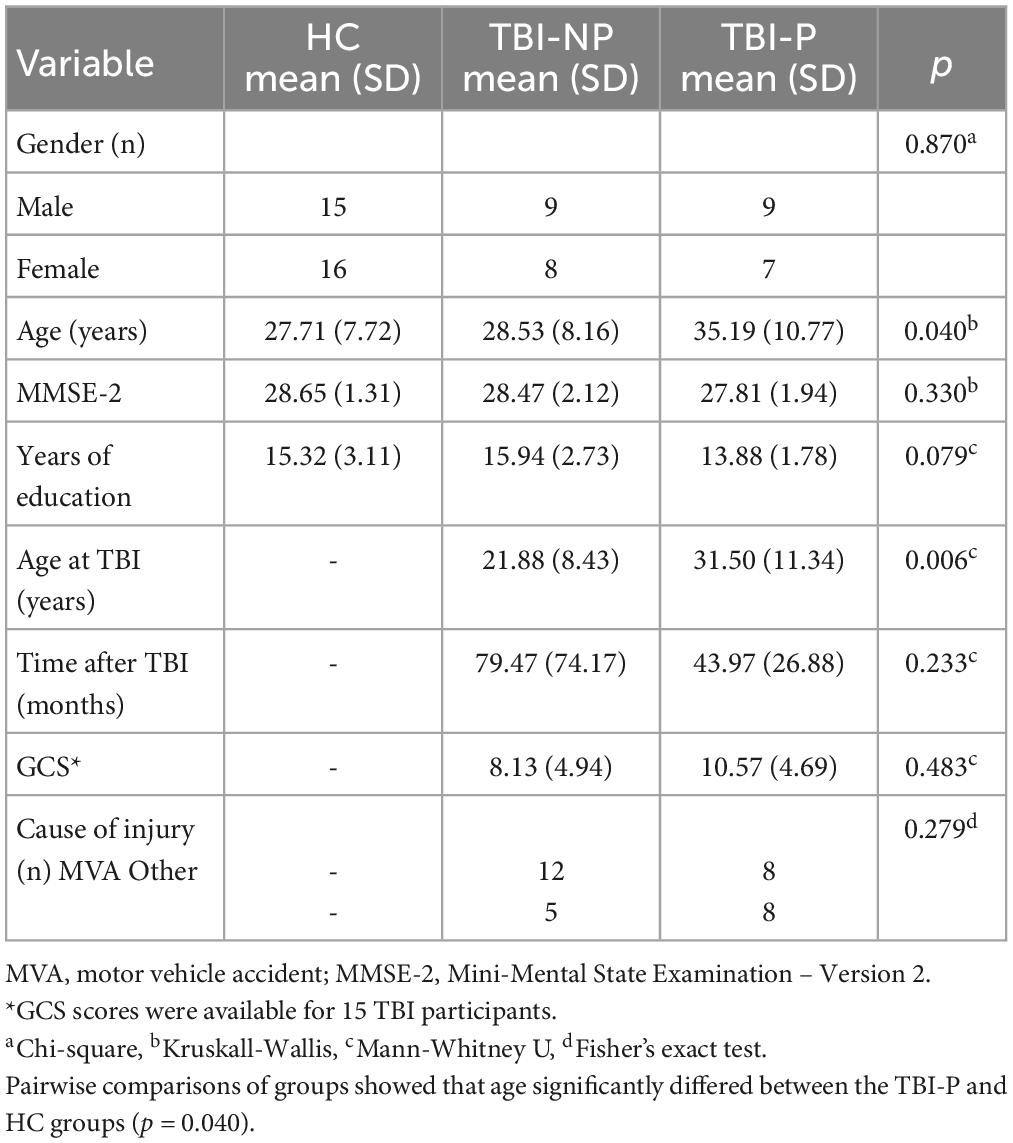
Table 1. Demographic and injury characteristics for HC (N = 31), TBI-NP (N = 17), and TBI-P (N = 16) groups.
2.2. Proton-magnetic resonance spectroscopic imaging (1H-MRSI) data
2.2.1. Data acquisition
Magnetic resonance spectroscopic imaging data from all participants (N = 68) were acquired using a 3 Tesla MRI scanner (Siemens Skyra) with a 20-channel receive-only head coil. The protocol included T1-weighted MPRAGE (magnetization-prepared acquisition rapid gradient echo; 1-mm isotropic resolution), T2-weighted gradient echo, FLAIR (fluid-attenuated inversion recovery), and whole-brain MR spectroscopic imaging (axial orientation, TE = 17.6 ms, TR = 1551 ms, field of view: 280 × 280 × 180 mm3, slab thickness: 135 mm, matrix: 50 × 50 × 18, nominal voxel volume: 0.31 cm3, and ∼ 17 min acquisition time).
2.2.2. Spectral processing
RS data were processed using the MIDAS package (Maudsley et al., 2006) to obtain signal-normalized (institutional units), and CSF partial volume corrected metabolite maps at an approximate spatial volume of 1 cm3. Metabolite concentrations were obtained for NAA, tCre, tCho, Glx, and m-Ins. Individual subject T1-weighted MRI data were spatially transformed into a T1-weighted MRI in the Montreal Neurological Institute (MNI) template space. The resulting spatial transformation was subsequently applied to the MRSI data. We used a modified version (47 regions only, see Supplementary Table 1) of the anatomic parcellation-based Automated Anatomical Labeling (AAL) atlas (Tzourio-Mazoyer et al., 2002) in the MNI template space for obtaining metabolite data for each respective brain region. The quality of MRS data used for analysis was controlled by applying criteria such as linewidth (≤ 12 Hz), tissue fraction (> 70% gray matter plus white matter), and outlier removal (> 3 SD). To improve the quality of MRS data obtained for analysis, spectra from multiple voxels within a selected brain region were summed to create a spectrum (e.g., thalamus) using the Map INTegrated spectrum (MINT) tool in the MIDAS package (Maudsley et al., 2006). In addition, metabolite ratios (NAA/tCre, m-Ins/tCre, Cho/tCre, Glx/tCre, and Glx/m-Ins) were calculated by dividing corresponding metabolite values per area. Data from four TBI participants were excluded from the final analysis due to motion artifacts, insufficient water signal suppression, and significant signal distortions.
2.2.3. Regions of interest
Data from ten pain-related brain regions of interest were selected for analysis. These included the left and right frontal, insula, cingulum anterior, postcentral, and thalamus (see Figure 1A), which are involved in the processing of sensory-discriminative (e.g., location and intensity), cognitive-evaluative, and affective-emotional aspects of nociception and pain (Bushnell et al., 2013; Kuner and Kuner, 2021; Mercer Lindsay et al., 2021). These ROIs were selected because metabolite alterations in these regions have been consistently observed in chronic pain conditions (Ito et al., 2017; Levins et al., 2019; Peek et al., 2020; Cruz-Almeida and Porges, 2021), including neuropathic pain (Chang et al., 2013; Widerström-Noga et al., 2015). The frontal region, which includes the prefrontal cortex, has been implicated in attentional and evaluative processes of pain (Bushnell et al., 2013; Kuner and Kuner, 2021; Tan and Kuner, 2021). The insula has been implicated in coding pain intensity (Garcia-Larrea et al., 2010; Garcia-Larrea and Peyron, 2013). The anterior cingulate cortex (ACC) and cingulum are components of the limbic system, thought to be involved in the affective-emotional components of the pain experience (Bushnell et al., 2013; Kuner and Kuner, 2021; Tan and Kuner, 2021). The postcentral gyrus or primary somatosensory cortex is commonly activated during induced pain and implicated in the sensory-discriminative (e.g., location and intensity) components of pain (Apkarian et al., 2005; Kuner and Kuner, 2021; Tan and Kuner, 2021). Lastly, the thalamus is the primary relay station for sensory information, communicates with the cortex and is also be involved in pain modulation (Apkarian et al., 2005).
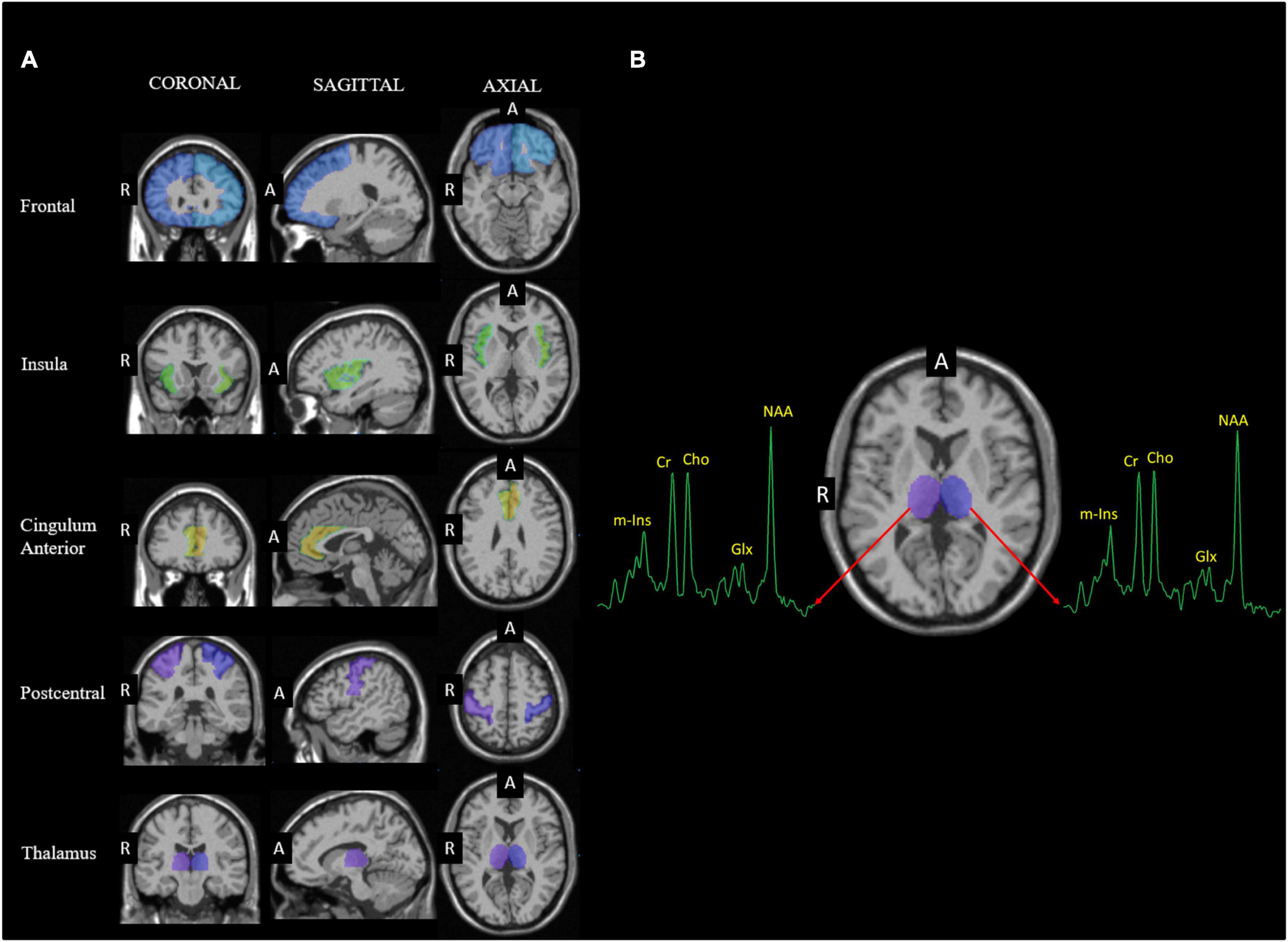
Figure 1. (A) Coronal, sagittal and axial slices showing the regions of interest (ROIs), including the frontal, cingulum anterior, insula, postcentral, and thalamus from left and right hemispheres, superimposed on template T1 MRI and obtained using the AAL atlas (63). (B) Representative spectrum from the thalamus. Abbreviations used for anterior (A) and right (R).
2.3. Clinical variables
Pain and psychological function measures were obtained by interview using validated questionnaires.
2.3.1. Pain
The pain severity subscale of the multidimensional pain inventory (MPI) (Kerns et al., 1985) was used to assess overall pain severity on a 0-6 scale. In addition, neuropathic pain symptoms were evaluated using the NPSI (Bouhassira et al., 2004). Total NPSI scores were obtained by summing all the individual scores for ten questions assessing the presence and severity of (1) spontaneous pain with burning, squeezing, or pressure characteristics; (2) painful attacks with electric shocks or stabbing characteristics; (3) pain provoked or increased by brushing, pressure, or contact with something cold on the painful area; and (4) abnormal sensations including pins and needles, and tingling.
2.3.2. Psychological function
The Beck anxiety inventory (BAI) (Beck et al., 1988) and Beck depression inventory (BDI) (Steer et al., 2001) were used to evaluate the presence and severity of 21 anxiety and depression symptoms, respectively. Participants were asked to rate their symptoms over the past two weeks using a number ranging from 0 to 3, with increasing scores reflecting greater symptomatology. Similarly, the post-traumatic stress disorder (PTSD) checklist-civilian version, PCL-C (Weathers, 1993; Ruggiero et al., 2003; Haarbauer-Krupa et al., 2017), was used to evaluate the presence and severity of PTSD symptoms. The Rivermead Post-concussion Symptoms Questionnaire Rivermead was also used to assess awareness of cognitive, emotional, and physical symptoms following TBI (King et al., 1995).
2.4. Statistical analysis
Independent variables were assessed for normality and homogeneity of variances (homoscedasticity) using Shapiro-Wilk and Levene’s tests, respectively. Mean values for each of the metabolites (NAA, m-Ins, tCho, Glx and tCre) were compared across the three groups (HC versus TBI-NP and TBI-P) using multiple non-parametric ANCOVAs (Quade, 1967) with age as a covariate. LSD pairwise comparisons were obtained. In addition, metabolite ratios (NAA/tCre, m-Ins/tCre, Cho/tCre, Glx/tCre, and Glx/m-Ins) were compared using Kruskal-Wallis Tests. Statistical analyses were conducted using SPSS. Results were considered significant if values were p ≤ 0.05 after being corrected for multiple comparisons using the Benjamini and Hochberg false discovery rate (FDR) method (Benjamini and Hochberg, 1995). Data from the frontal, anterior cingulum, insula, postcentral gyrus, and thalamic regions were analyzed separately for both the left and right hemispheres. To evaluate the associations between clinical variables (e.g., pain and psychological) and the metabolite measures in the TBI-P group, we conducted partial Pearson correlations with age as a covariate.
3. Results
3.1. Pain and psychological characteristics of participants
Pain and psychological characteristics are indicated in Table 2.
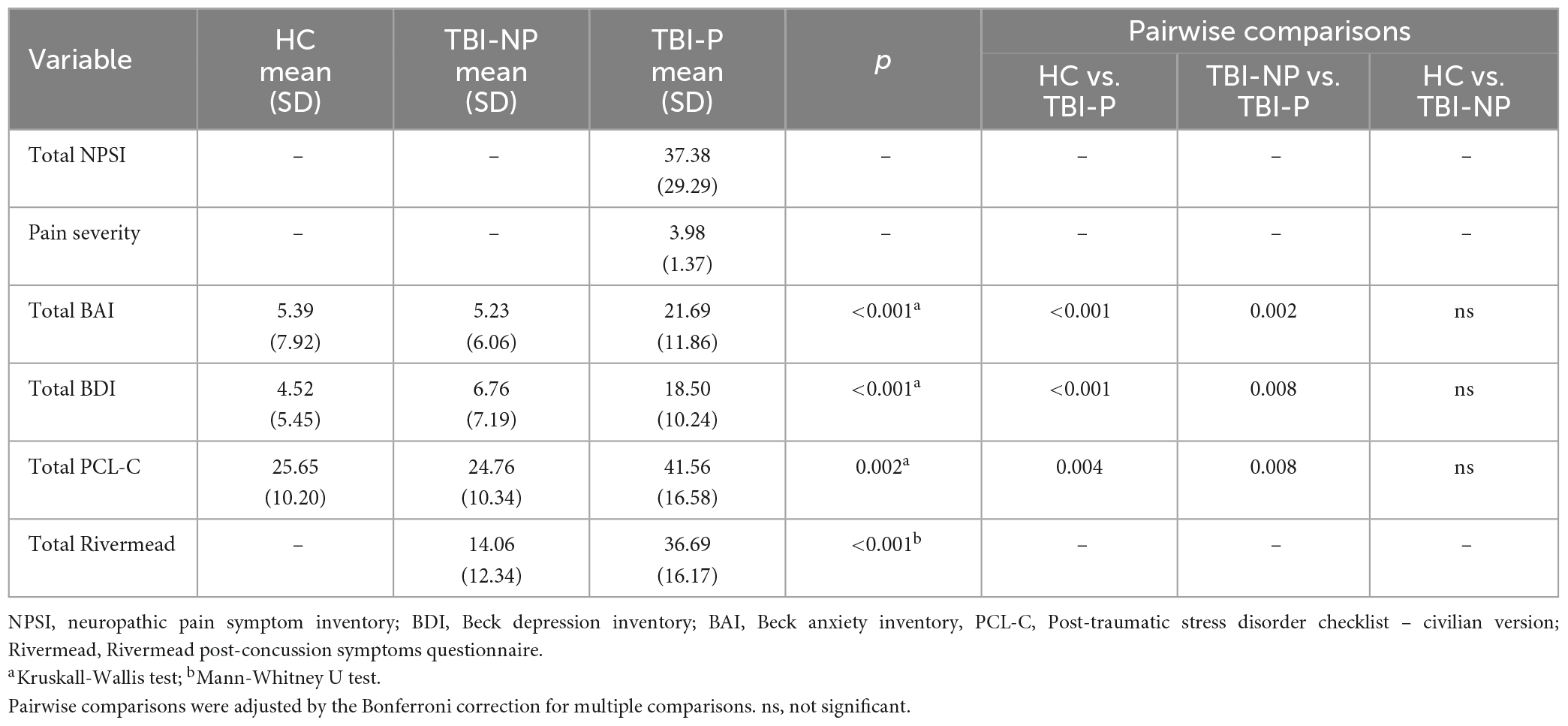
Table 2. Pain and psychological characteristics for the HC (N = 31), TBI-NP (N = 17), and TBI-P (N = 16) groups.
3.2. Metabolite differences between the HC, TBI-NP and TBI-P groups
Results from non-parametric ANCOVA are presented in Tables 3-7, with Figure 2 displaying age-corrected means and pairwise comparisons for each metabolite (i.e., NAA, tCho, m-Ins, Glx, and tCre) in the selected ROIs for each group. Relative to HC, those within the TBI-NP group showed significantly lower NAA in nearly all brain regions, excluding the left postcentral ROI. These participants also displayed significantly lower m-Ins within the right postcentral region and significantly lower Glx and tCre within the right and left thalamus. When those in the TBI-P group were compared to HC, significantly lower NAA was also observed in the left and right anterior cingulum, left postcentral region, and the right thalamus. Similarly, tCho was significantly lower in the left frontal, left postcentral, right cingulum, and left and right thalamus, with tCre showing significantly lower values in the left postcentral region.
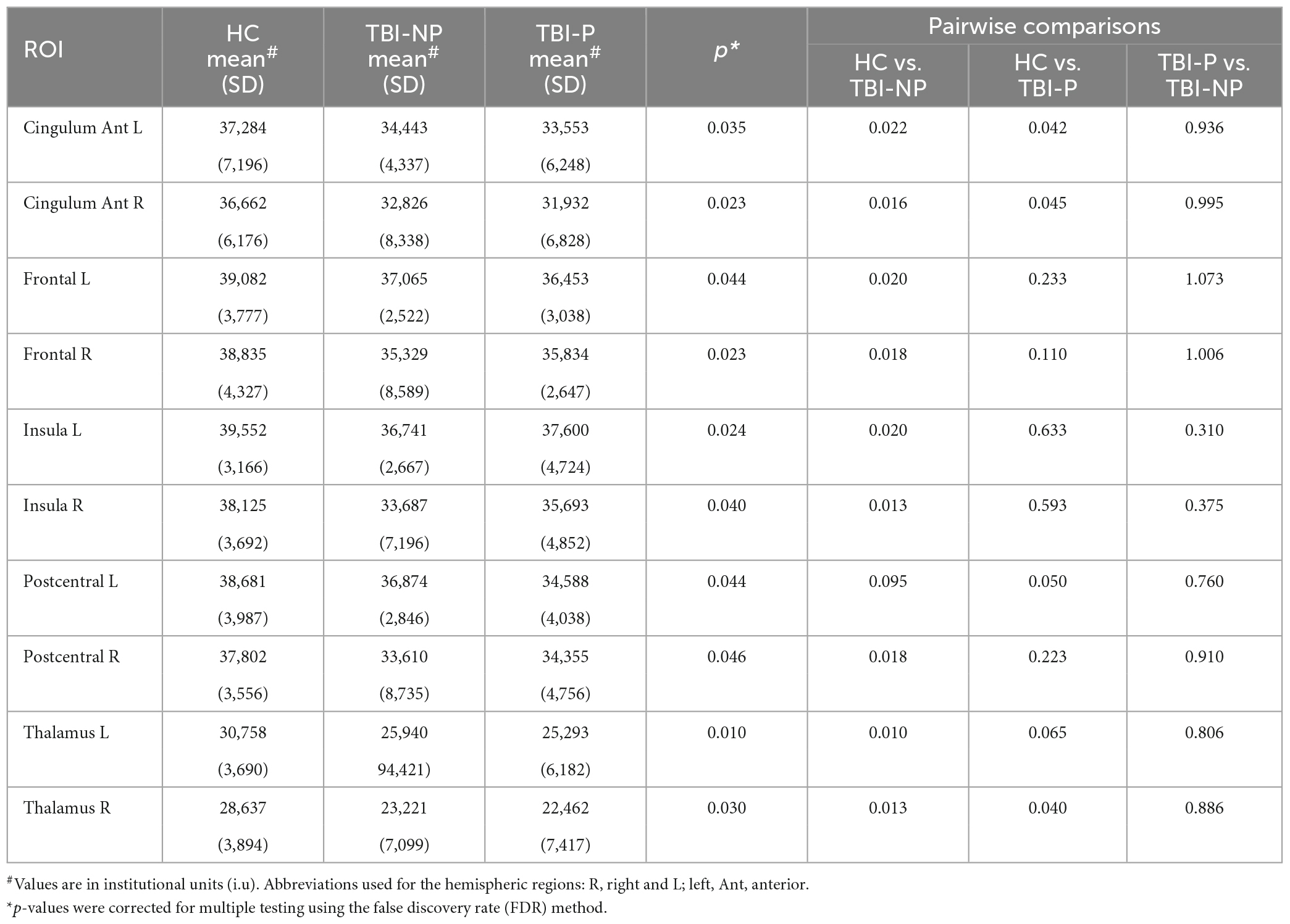
Table 3. Results from analysis of covariance (ANCOVA) controlling for age between HC (N = 31), TBI-NP (N = 17) and TBI-P (N = 16) groups for N-acetylaspartate (NAA).
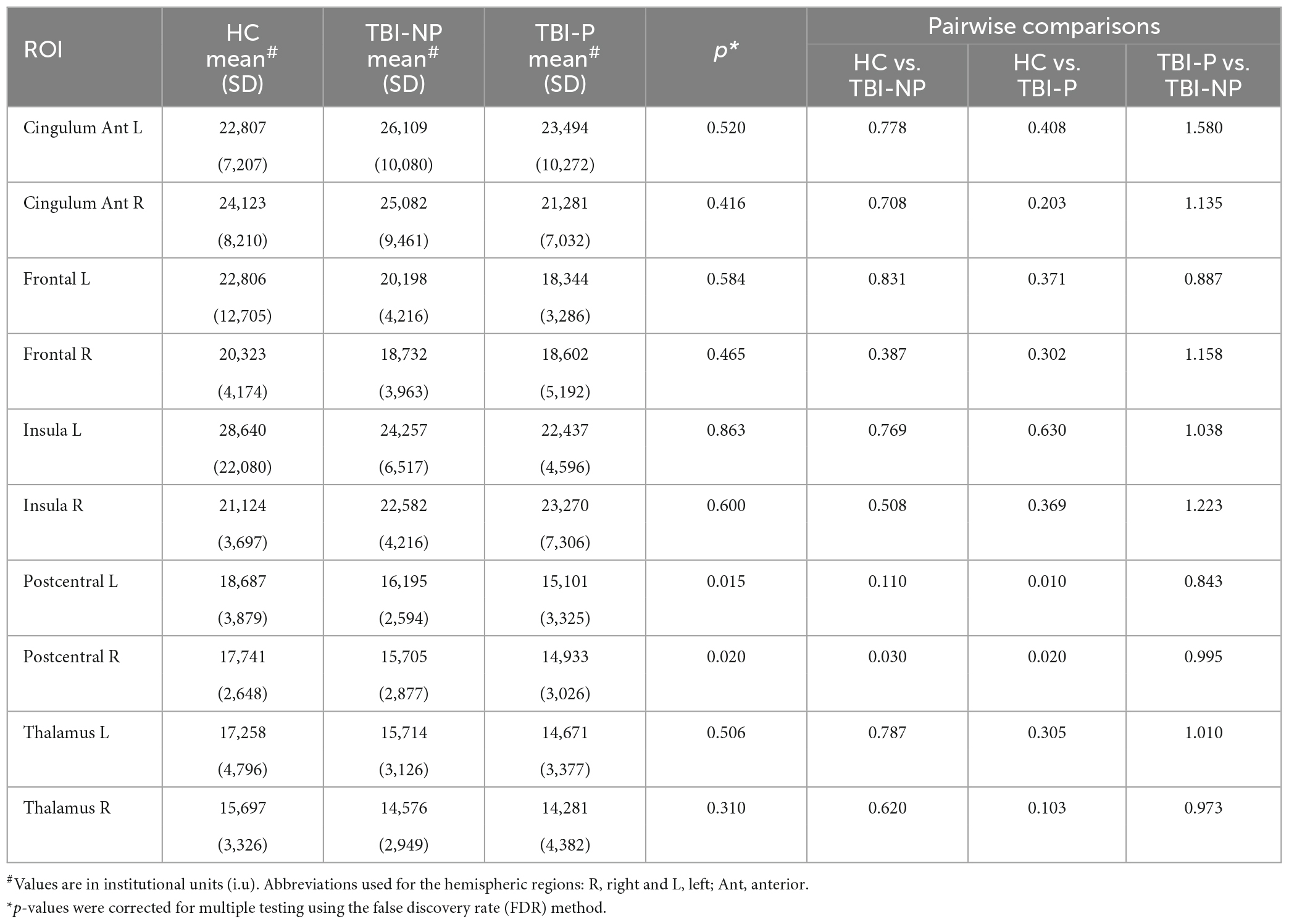
Table 4. Results from analysis of covariance (ANCOVA) controlling for age between HC (N = 31), TBI-NP (N = 17) and TBI-P (N = 16) groups for myo-inositol (m-Ins).
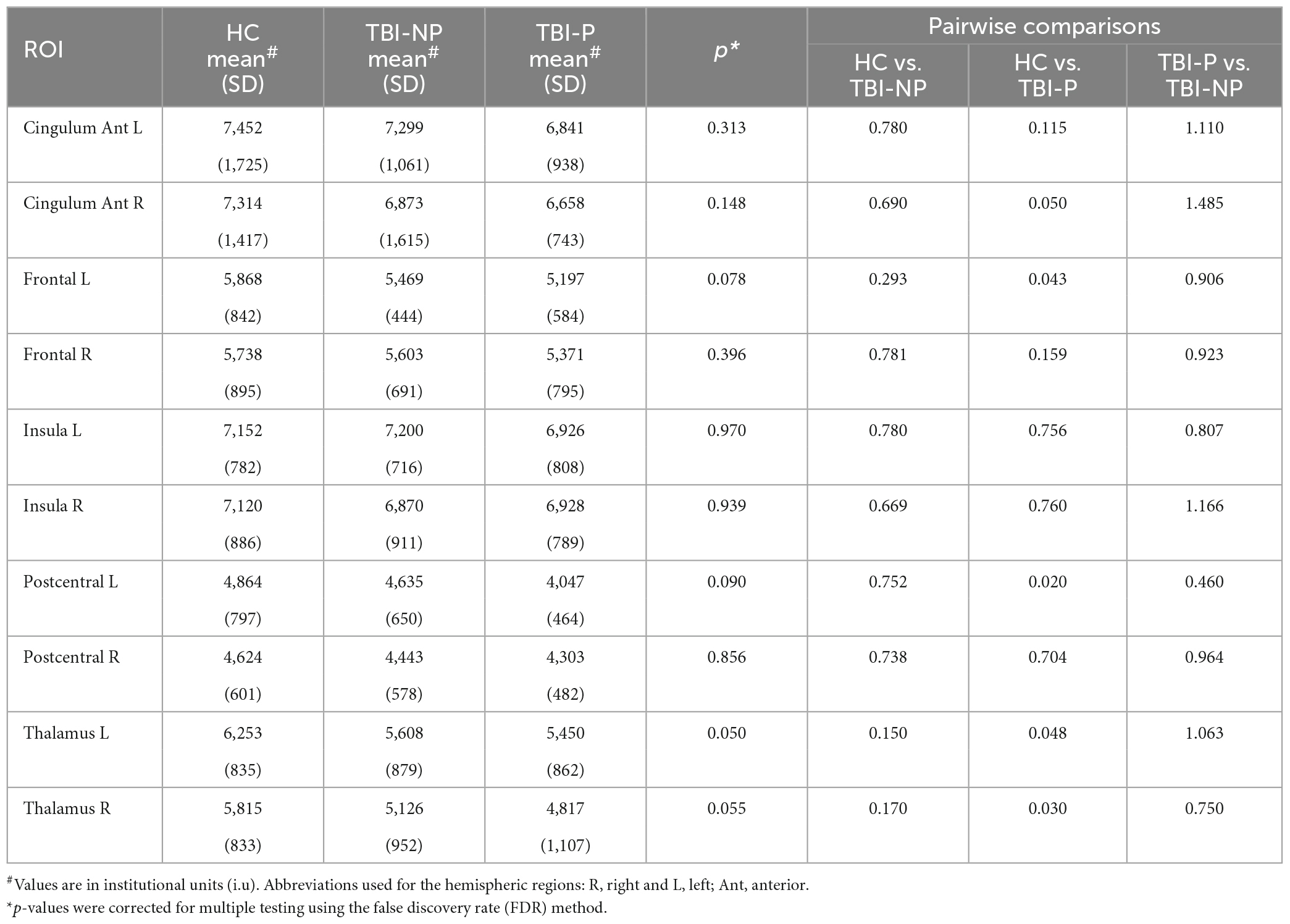
Table 5. Results from analysis of covariance (ANCOVA) controlling for age between HC (N = 31), TBI-NP (N = 17) and TBI-P (N = 16) groups for total choline (tCho).
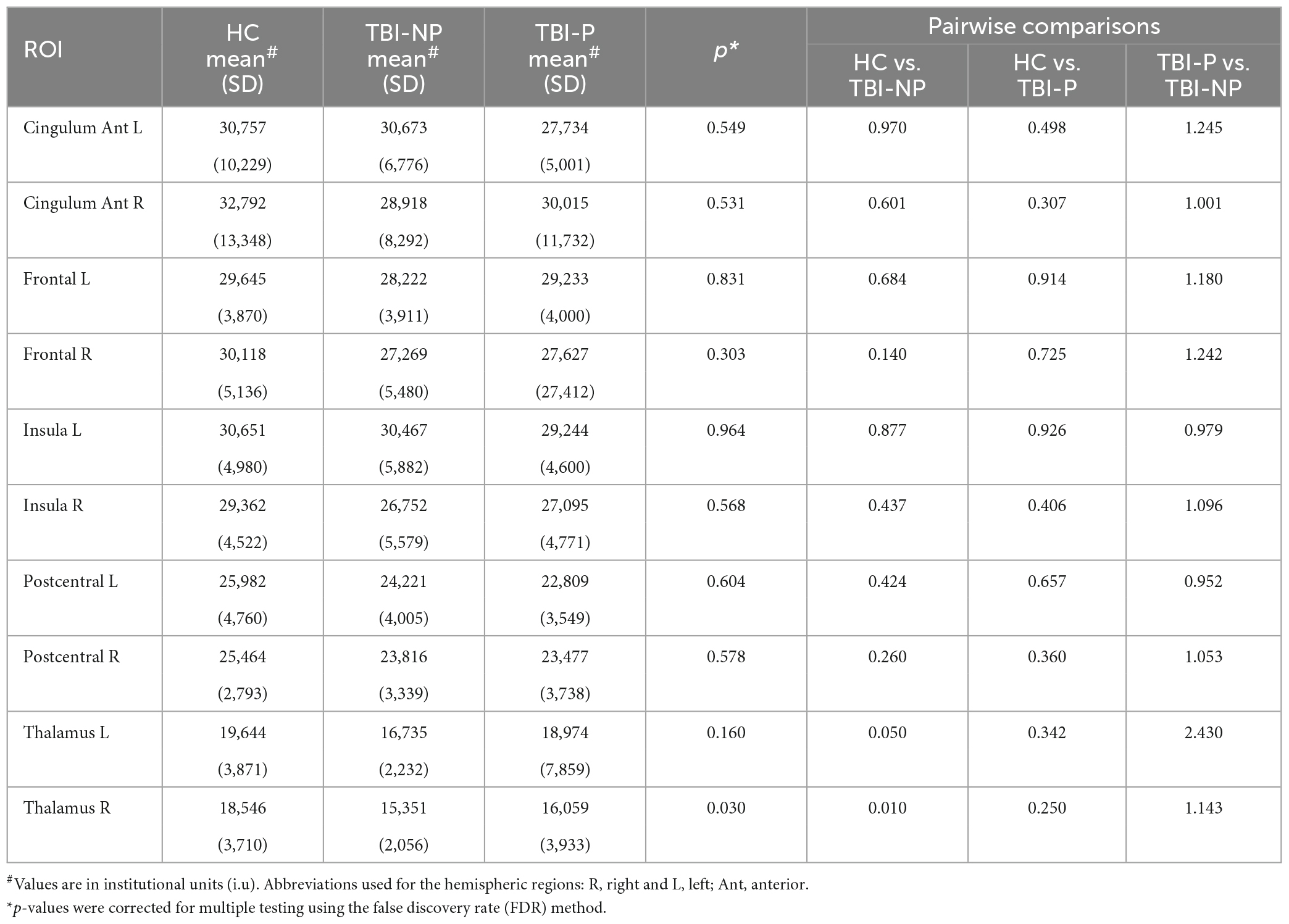
Table 6. Results from analysis of covariance (ANCOVA) controlling for age between HC (N = 31), TBI-NP (N = 17) and TBI-P (N = 16) groups for glutamate and glutamine (Glx).
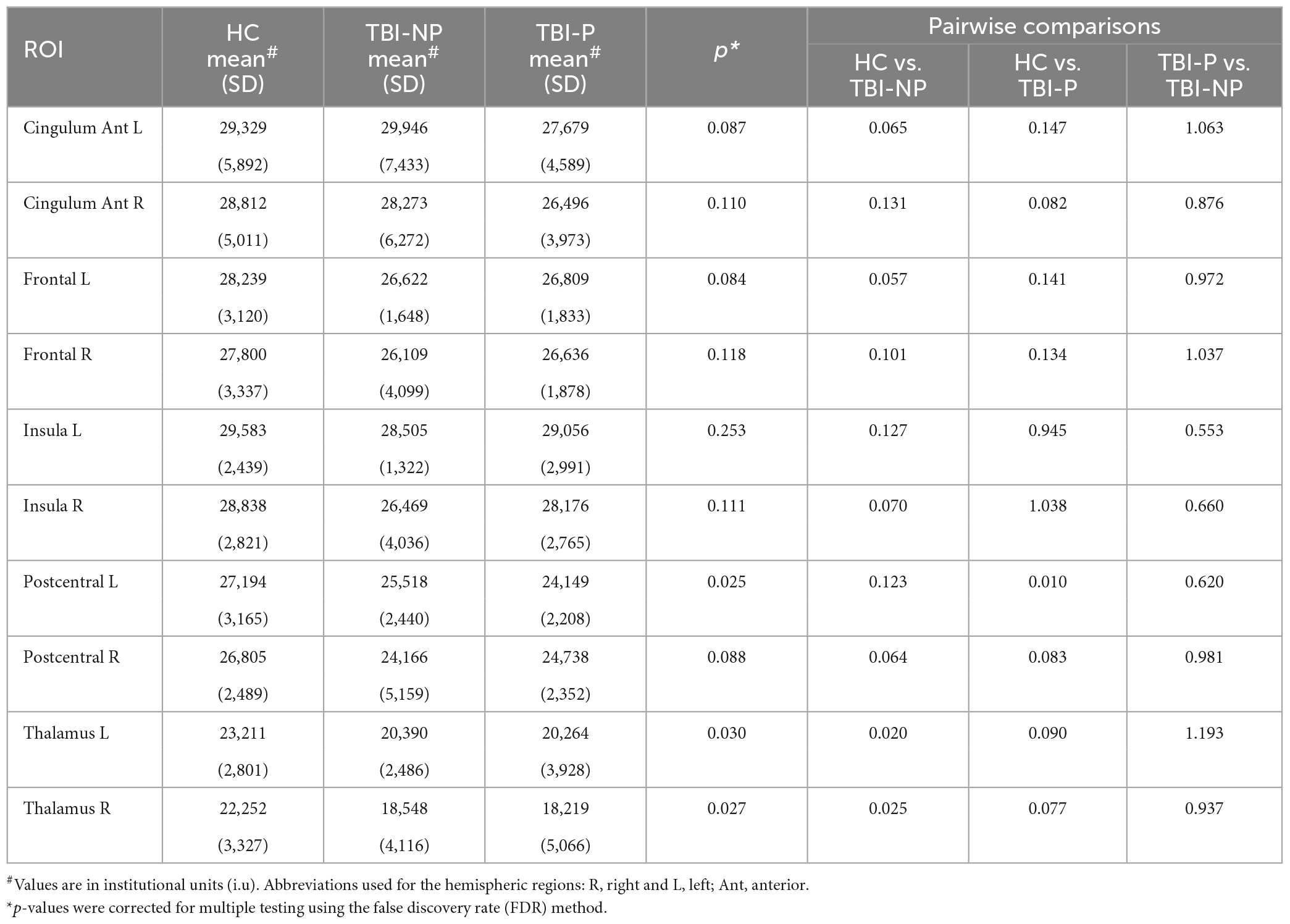
Table 7. Results from analysis of covariance (ANCOVA) controlling for age between HC (N = 31), TBI-NP (N = 17) and TBI-P (N = 16) groups for total creatine (tCre).
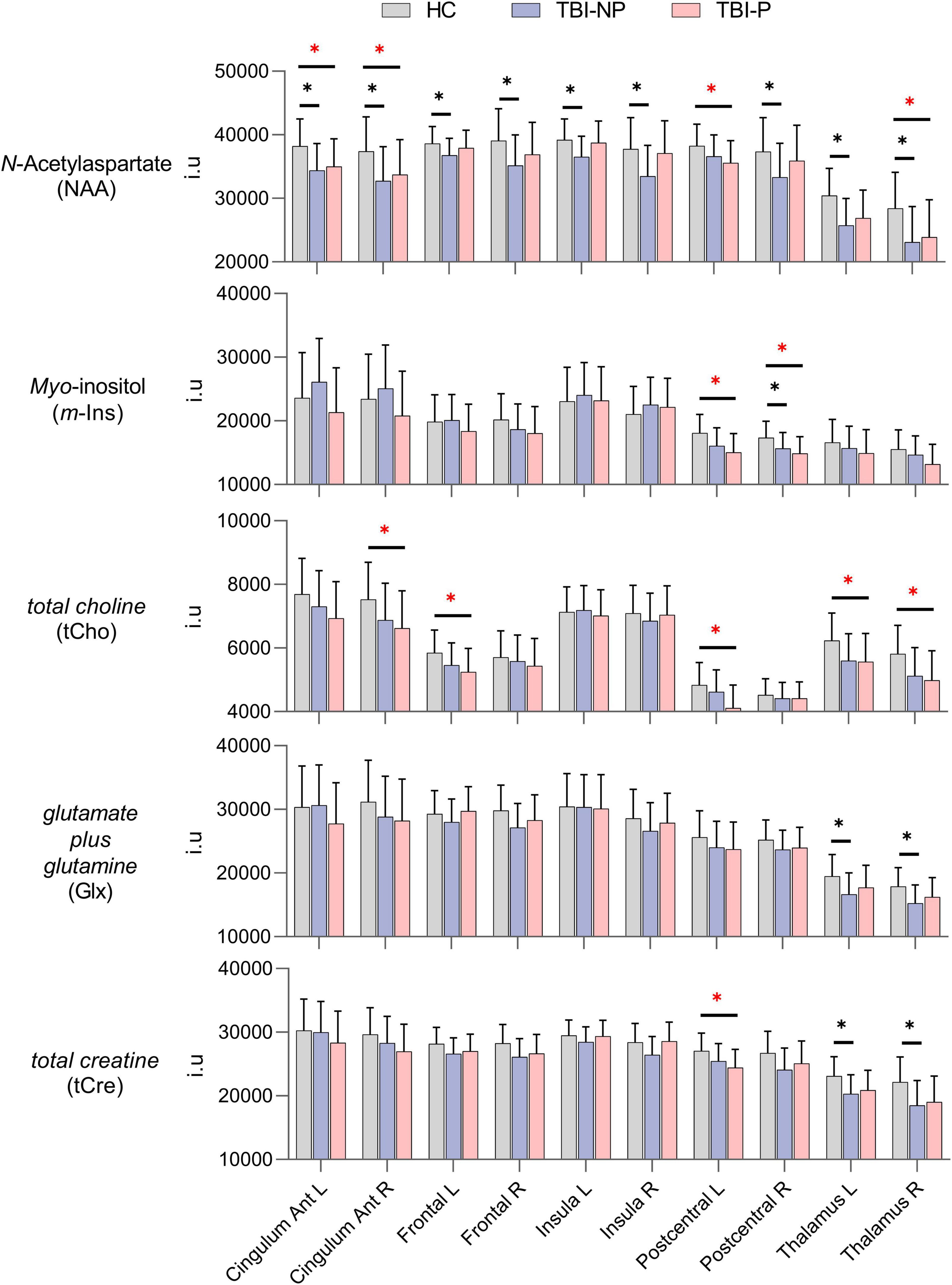
Figure 2. Mean values of the metabolites in the healthy control (HC, N = 31), traumatic brain injury with no chronic pain (TBI-NP, N = 17), and traumatic brain injury with chronic pain (TBI-P, N = 16) groups, by brain region, with error bars indicating the standard deviation (SD). False discovery rate (FDR)-corrected significant differences indicated with asterisks (*p ≤ 0.05). Red asterisks (*) indicate significant differences between TBI-P and other groups. The y-axis scale is in institutional units (i.u.). Abbreviations used for the hemispheric regions: R, right and L, left; Ant, anterior.
As indicated in Table 1, “time after TBI” is not significantly different between TBI groups (TBI-NP vs. TBI-P). However, we understand that this variable covers a large range. For that, we conducted a sub-analysis to test if “time after TBI” affect the between-group results. Independent-Samples Mann-Whitney U tests, between a short time after TBI (N = 17, mean = 24.15) vs. a long time after TBI (N = 16, mean = 102.75) groups, indicated that there was no effect of “time after TBI” on metabolite levels, except for m-Ins on the postcentral right region (U = 71.00, p = 0.02). Myo-Inositol levels were significantly higher in the group with a shorter time after TBI (mean rank = 20.82) vs. a longer time after TBI (mean rank = 12.94). We then conducted an additional non-parametric ANCOVA for myo-inositol in the postcentral right region, controlling for “age” and “time after TBI.” Similar to our previously reported results, there was no significant difference between TBI-P vs. TBI-NP groups, F (1, 31) = 0.278, p = 0.602, suggesting that even though “time after TBI” may affect myo-inositol levels, the effect was not strong.
Comparisons among the groups of NAA/tCre, Cho/tCre, Glx/tCre, m-Ins/tCre, and Glx/m-Ins ratios did not indicate significant differences between TBI-NP and TBI-P relative to controls (data not shown), except for higher m-Ins/Cre and lower Glx/m-Ins ratios in the right insula. Although uncorrected p-values were significant (p = 0.034 and p = 0.018), they did not survive FDR correction for multiple tests.
3.3. Associations with pain, psychological and injury variables
Correlation coefficients between metabolite levels and clinical variables for the TBI-P group are provided in Table 8. Age was used as a covariate in the analysis to control for any potential confounding effect that age may have on metabolite measures. Significant positive correlations were found between 1) left frontal NAA levels and total NPSI scores, BDI and PTSD symptoms, and Rivermead scores, and 2) right frontal NAA levels with pain severity and Rivermead scores. Additionally, left postcentral NAA values were significantly correlated to total NPSI scores, suggesting some relationship between the severity of neuropathic pain symptoms and altered NAA levels.
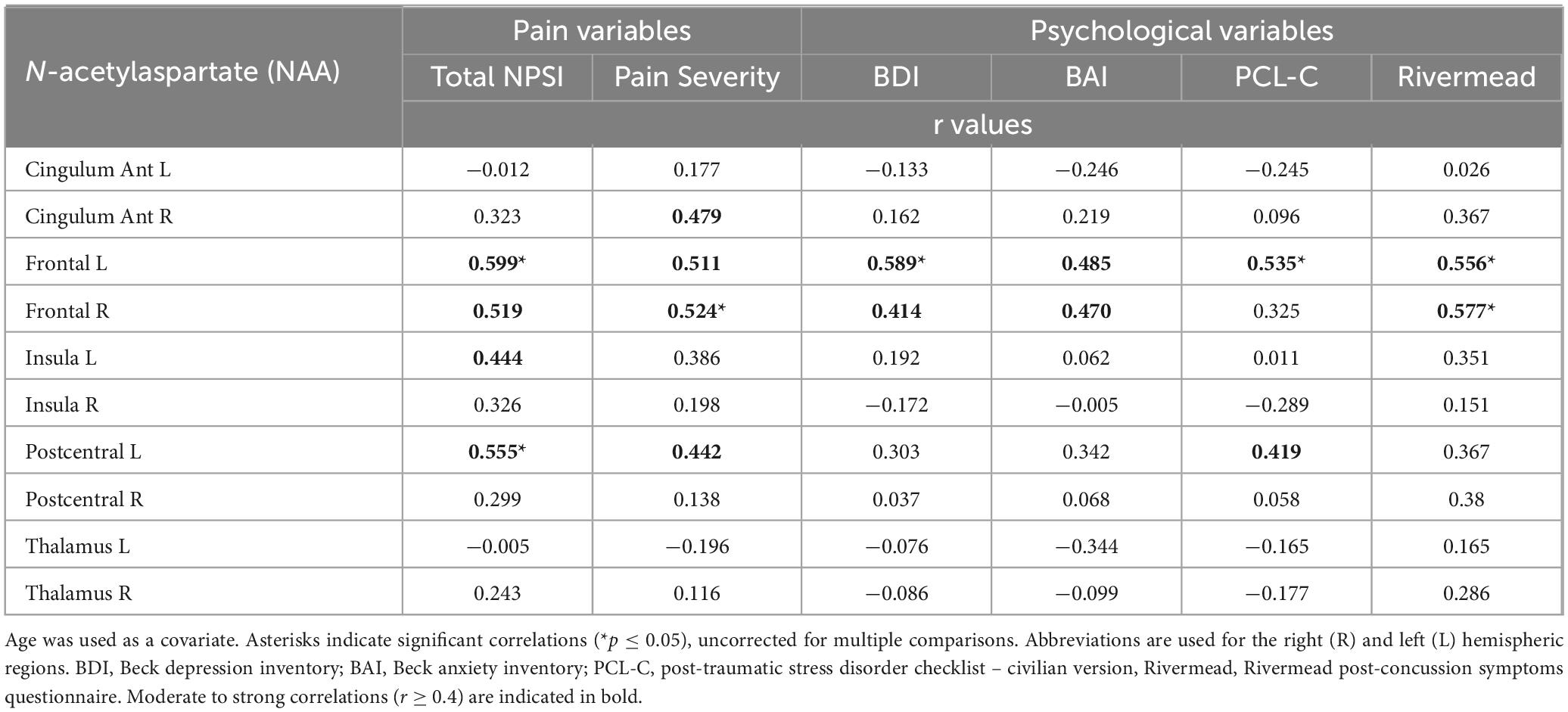
Table 8. Pearson correlation coefficients (r) of partial correlations between N-acetylaspartate (NAA) levels of the TBI pain group (TBI-P, N = 16) and pain and psychological variables, controlling for age.
4. Discussion
Whole-brain 1H-MRSI can provide in vivo brain tissue metabolite levels following TBI (Govindaraju et al., 2000; Govind et al., 2010). This type of information can help elucidate the relationship between brain tissue metabolite alterations in people with chronic TBI and co-occurring chronic pain. In this study, we compared NAA, tCho, Glx, tCre, and m-Ins levels and their respective ratios across frontal, cingulate, insula, somatosensory, and thalamic regions in participants living with chronic TBI and healthy controls. We also examined the relationships between metabolite levels and pain and psychological measures in those with chronic pain following TBI. Relative to controls, those in the TBI and chronic pain group exhibited significantly lower NAA in the left and right anterior cingulum, left postcentral region, and the right thalamus. Similarly, tCho was significantly lower in the left frontal, left postcentral, right cingulum, and left and right thalamus, with tCre showing significantly lower values in the left postcentral region. Conversely, those with TBI without chronic pain also showed significantly lower NAA in nearly all selected ROIs, excluding the left postcentral. These participants also displayed significantly lower m-Ins within the right postcentral region and significantly less Glx and tCre within the right and left thalamus. In addition, we observed significant correlations between left frontal NAA levels and poorer BDI scores, worse PTSD symptoms, and higher post-concussive symptoms among those reporting chronic pain. Similarly, left frontal NAA levels were correlated to pain severity and post-concussive symptoms, while left postcentral NAA was correlated with total NPSI scores. These findings may indicate long-term alterations among specific metabolite levels, such as NAA, that could be used as biomarkers for psychological dysfunction and chronic pain, including neuropathic pain symptoms, following TBI.
Although cross-sectional and longitudinal studies have identified acute and subacute reductions in NAA or NAA/Cr as clinical indicators of neurological dysfunction, some report recovery to values approximating healthy controls (Govind et al., 2010; Maudsley et al., 2015, 2017). In general, lower NAA levels or NAA/tCre ratios are associated with worse TBI outcomes (Govind et al., 2010; Croall et al., 2015; Holshouser et al., 2019) and with chronic neuropathic pain conditions (Pattany et al., 2002; Fukui et al., 2006; Sorensen et al., 2008). Our findings support these observations and reveal TBI-associated alterations in NAA levels across numerous ROIs relative to healthy controls. Lower NAA levels in participants with TBI during the chronic stage likely reflect a reduction in the metabolic integrity and overall viability of neurons housed across pain-related structures, as NAA is one of the most abundant and highly concentrated neuronal metabolites responsible for mitochondrial bioenergetics and the transfer of intermediary energy substrates to various glial cells, including oligodendrocytes (Govindaraju et al., 2000; Moffett et al., 2013). These findings align with those of Lin and colleagues (2022), who recently used whole-brain 1H-MRSI to examine metabolite differences in participants suffering from moderate to severe TBI at least 12 months after their initial injury. Although Lin and colleagues (2022) reported lower NAA and its ratios across several ROI, metabolite levels were not statistically different in the TBI group compared to healthy controls following FDR correction for multiple comparisons (Lin et al., 2022). Our study develops this line of inquiry further and confirms metabolite alterations in people with TBI during the chronic stage, with p values surviving multiple comparisons.
Biochemical and structural abnormalities among key pain regions, including the frontal cortex (Glodzik-Sobanska et al., 2007), insula (Widerström-Noga et al., 2016), the primary somatosensory cortex (Ferris et al., 2021), and thalamus (Gustin et al., 2014), have been identified following neurotrauma, suggesting an emergent schematic of disrupted metabolic and structural integrity, and reorganization of existing neural tissue. To our knowledge, no studies have delineated metabolite alterations in those with chronic pain following TBI. However, our findings may also indicate that brain tissue metabolite alterations following TBI are necessary but not sufficient for developing chronic pain. Thus, a combination of cellular and molecular mechanisms, including altered neuronal and glial cell function or loss within the pain circuitry, may lead to maladaptive plasticity favoring persistent pain symptoms post-TBI. Indeed, maladaptive plasticity has been associated with neuropathic pain (Costigan et al., 2009). Although not significantly different from the non-pain TBI group, apparent higher metabolite levels in the bilateral frontal and insular regions, right postcentral, and right thalamus were observed among those with chronic pain. This observation may indicate a shift toward increased metabolic activity (hyperactivity) or loss of inhibitory control associated with a state of central sensitization within pain-related brain regions, also supported by positive correlations between NAA levels in the left and right frontal and left postcentral regions and pain measures. Similarly, NAA levels in the left and right frontal regions were also positively correlated with measures of depression, post-traumatic stress disorder, and post-concussion symptoms. Neuroimaging studies suggest that abnormal frontal executive connectivity largely contributes to developing chronic pain symptoms where the intensity and temporality are often comprised of affective and motivational elements (Davis and Moayedi, 2013). Circuitry housed within frontal brain regions is thought to provide top-down attentional control of salient sensory information while also representing the emotional content of pain within more midline regions (Kragel et al., 2018).
The interpretation of tCho signal alterations within the selected ROIs is difficult to parse, mainly because the tCho signal includes contributions from free choline (Cho), glycerophosphorylcholine (GPC), and phosphorylcholine (PC) (Govindaraju et al., 2000). Cho is required for the synthesis of neuronal and glial membrane phospholipids (e.g., phosphatidylcholine) and the neurotransmitter acetylcholine (Ach), which is involved in sensory, motor, and cognitive function. GPC acts as a cerebral osmolyte, whereas PC is involved in the synthesis of myelin sheath-associated compounds (e.g., sphingomyelin), which is essential for axonal integrity (Govindaraju et al., 2000; Leipelt and Merrill, 2004; Javaid et al., 2021). Higher tCho levels have been consistently reported during the acute and subacute TBI stages, and this increase has been attributed to breakdown products of cell membranes and myelin and astrogliosis (Govind et al., 2010). Contrary to this general finding, both TBI groups showed lower tCho levels when compared to the healthy control group in the left thalamus. Moreover, those in the TBI and chronic pain group had lower tCho levels within the right cingulum, left frontal, left postcentral, and the right thalamic regions when compared to healthy controls. These reductions likely suggest permanent tissue damage and no further possibility of structural repairs, which manifest as a decreased tCho profile that differs from those in the acute and subacute injury stages. Future research with larger sample sizes is required to confirm this finding. Ultimately, our results showing lower tCho in the cingulum anterior, frontal, postcentral, and thalamic regions in the TBI and chronic pain group relative to the healthy control group may suggest either one or a combination of the following: (1) decreased membrane turnover or impaired cholinergic neurotransmission (i.e., Cho), (2) reduced cell volumes (i.e., GPC), or (3) decreased myelin synthesis (i.e., PC) in the chronic stage.
As with tCho levels, the impact of lower m-Ins is difficult to delineate, given limited findings across previously published MRS studies (Kierans et al., 2014). Indeed, prior evidence suggests that m-Ins is a crucial marker for glial cell health and also serves as an osmolyte – with higher levels reflecting greater neuronal dysfunction through alterations in calcium signaling and other ion mediated transduction pathways (Fisher et al., 2002; Croall et al., 2015). Similar to tCho, studies in acute and subacute TBI generally report elevated m-Ins levels or its ratios (Garnett et al., 2000a; Kierans et al., 2014). Our results indicate that those with TBI and chronic pain presented with lower m-Ins levels across the left postcentral, and both the right and left thalamus regions when compared to healthy controls. This result differs from those reported by Yoon and colleagues (Yoon et al., 2005), who observed elevated m-Ins/Cre ratios among those with moderate to severe TBI five-months after their injury. Once again, reduced neurometabolite levels may suggest tissue damage and metabolic dysfunction within neural networks responsible for both the gating of sensory information (i.e., thalamus) and processing of such information within higher order brain centers (i.e., left postcentral region). Additional studies in long term TBI are required to replicate such findings.
TBI-associated increases in tCre (creatine/phosphocreatine) have been documented with limited evidence suggesting that increased levels may reflect a combination of neuronal cell death paired with low grade gliosis (Garnett et al., 2001). While authors have noted altered tCre and its ratios in the acute (Gasparovic et al., 2009) and chronic TBI stages (Cadoux-Hudson et al., 1990), many assume constant levels of this metabolite when acquiring such measures, making observable variations difficult to contextualize across studies. Indeed, one study found no change in overall tCre levels in seven acute TBI cases (Garnett et al., 2001), while another observed increases in the neurometabolite that also correlated to more severe emotional distress and executive function performance in those with mild injuries (Gasparovic et al., 2009). That said, tCre represents an intracellular estimate of energy production, and when combined with the quantification of adenosine di/triphosphate (ADP and ATP), and inorganic phosphate (Pi), may provide a high-resolution marker for the metabolism of discrete brain regions associated with the processing of chronic pain symptoms. Based on the current findings and similar to those observed for m-Ins and lower tCre levels in the postcentral region of the TBI with chronic pain group relative to the healthy control group may suggest reduced glial cell density or volume (i.e., m-Ins) and reduced cellular energetics (i.e., tCre) (Govindaraju et al., 2000; Croall et al., 2015) within the left somatosensory cortex. Future studies are needed in larger cohorts to better examine variations in tCre years after TBI.
Increases in Glx levels within gray and white matter have been associated with poor neurological outcomes in individuals with TBI (Ashwal et al., 2004; Shutter et al., 2004), possibly reflecting excitotoxicity. However, lower Glx levels have also been detected in individuals with TBI compared to controls (Kubas et al., 2010; Yeo et al., 2011). Such findings may indicate downregulation of glutamate or glutamine production as a neuroprotective adaptation to neuronal death (Ramonet et al., 2004) or an overall reduced neuronal activity (Yeo et al., 2011). Based on a recent meta-analysis (Joyce et al., 2022), differences in findings may be attributed to biologically relevant (e.g., brain region) and technical factors (e.g., data acquisition). Results from Joyce et al. also showed that Glx was commonly unaffected, similar to our results, where no significant difference was found among groups with respect to all regions of interest except for the thalamus.
Ultimately, the global perception of pain is contingent on the proper integration of excitatory and inhibitory processes across brain networks, where functional and structural connectivity within these networks is essential for processing sensory-discriminative, affective-motivational, and cognitive-evaluative dimensions of pain (Treede et al., 2000; Apkarian et al., 2005; Kuner and Kuner, 2021; Mercer Lindsay et al., 2021; Tan and Kuner, 2021). Exposure of cortical and subcortical tissue to external forces of an appropriate magnitude may cause diffuse axonal injury and brain swelling (Werner and Engelhard, 2007). Coincidently, secondary injury pathologies such as neuroinflammation, abnormal monoaminergic signaling, and mitochondrial dysfunction may prompt a maladaptive reorganization of existing pain circuitry (Irvine and Clark, 2018) following TBI. This notion is further corroborated by parallel cell signaling pathologies that have been observed in those with spinal cord injury (SCI), painful diabetic neuropathy, trigeminal neuralgia, and complex regional pain syndrome (CRPS) (Chang et al., 2013).
4.1. Limitations
The associations between metabolite levels and severity of initial TBI were not investigated in this study but have been reported previously by us and others in cross-sectional and longitudinal studies (Garnett et al., 2000a,b; Govind et al., 2010), showing conflicting results. Thus, associations between metabolites in the context of initial TBI severity remain to be fully elucidated, as well as any influence of the mechanism of injury (e.g., MVA vs. sports injury), pain medication, and damage to the brain tissue microstructure. In addition, the relatively small sample size of the present study may have affected the statistical power when comparing both TBI groups (with and without chronic pain), so future studies should be conducted with larger sample sizes to further expand and validate the presented results. Furthermore, age and age at TBI were significantly different among groups. Participants in the TBI and chronic pain group were significantly older than those in the TBI without chronic pain group. For that reason, we controlled for age in our between-group comparisons to account for possible confounding effects of age on metabolite levels (Brooks J. C. W. et al., 2001; Grachev and Apkarian, 2001; Reyngoudt et al., 2012). Interestingly, age at TBI were higher in the TBI and chronic pain group than in the TBI without chronic pain group, suggesting that an older age at TBI may predispose people to chronic pain. This observation is consistent with previous literature reporting that an older age at TBI was related to greater disability (Forslund et al., 2019; Rabinowitz et al., 2021). However, any association between age at TBI and chronic pain remains to be fully elucidated, as well as the effects of age at TBI on long-term neurometabolite alterations.
5. Conclusion
The results of the present study suggest that those with chronic TBI present with significant neurometabolite alterations within pain-related brain regions. The extent of these alterations varied among the metabolite measured and the regions of interest. The presence of chronic pain was associated with significantly lower NAA, m-Ins, tCho and tCre levels across pain-related regions relative to controls, and with higher (although non-significant) NAA and tCre levels in the frontal, insula, right postcentral and thalamus regions compared to individuals without chronic pain. We also observed significant correlations between NAA levels with measures of pain and psychological factors, further emphasizing the relationship between the severity of pain and the extent of psychological distress among those with TBI. Altogether, these results may suggest that metabolite alterations are necessary but not sufficient for the maintenance of chronic pain post-injury and that additional underlying mechanisms, including maladaptive plasticity and functional reorganization in pain-related brain regions, may play a role in the development of chronic pain.
Data availability statement
The datasets presented in this study can be found in online repositories. The names of the repository/repositories and accession number(s) can be found below: Home | FITBIR (nih.gov).
Ethics statement
The studies involving human participants were reviewed and approved by University of Miami Institutional Review Board. The patients/participants provided their written informed consent to participate in this study.
Author contributions
EW-N, VG, and AM designed the study. LR, VG, TS, and SS analyzed the proton-magnetic resonance spectroscopic imaging data. LR, EW-N, and NC analyzed the pain and psychological measures. NC, LR, and TS prepared the manuscript draft. All authors substantially contributed to the interpretation of data and manuscript revision.
Funding
This work was supported by the U.S. Department of Defense (MR141214 grant) and The Miami Project to Cure Paralysis. However, these entities were not involved in the study design, collection, analysis, and interpretation of data, neither in writing the report nor in the decision to submit the article for publication.
Acknowledgments
The authors would like to acknowledge Loriann Fleming for recruiting participants and performing neuropsychological and pain assessments, Temitope Adedolapo and Madison L. Clinger for assisting with assessments and data entry.
Conflict of interest
The authors declare that the research was conducted in the absence of any commercial or financial relationships that could be construed as a potential conflict of interest.
Publisher’s note
All claims expressed in this article are solely those of the authors and do not necessarily represent those of their affiliated organizations, or those of the publisher, the editors and the reviewers. Any product that may be evaluated in this article, or claim that may be made by its manufacturer, is not guaranteed or endorsed by the publisher.
Supplementary material
The Supplementary Material for this article can be found online at: https://www.frontiersin.org/articles/10.3389/fnins.2023.1125128/full#supplementary-material
References
Allen, M., Dietz, M., Blair, K. S., van Beek, M., Rees, G., Vestergaard-Poulsen, P., et al. (2012). Cognitive-affective neural plasticity following active-controlled mindfulness intervention. J. Neurosci. 32, 15601–15610. doi: 10.1523/JNEUROSCI.2957-12.2012
Alshelh, Z., Di Pietro, F., Youssef, A. M., Reeves, J. M., Macey, P. M., Vickers, E. R., et al. (2016). Chronic neuropathic pain: it’s about the rhythm. J. Neurosci. 36, 1008–1018. doi: 10.1523/JNEUROSCI.2768-15.2016
Apkarian, A. V., Bushnell, M. C., Treede, R. D., and Zubieta, J. K. (2005). Human brain mechanisms of pain perception and regulation in health and disease. Eur. J. Pain Lond. Engl. 9, 463–484. doi: 10.1016/j.ejpain.2004.11.001
Ashwal, S., Holshouser, B., Tong, K., Serna, T., Osterdock, R., Gross, M., et al. (2004). Proton MR spectroscopy detected glutamate/glutamine is increased in children with traumatic brain injury. J. Neurotrauma 21, 1539–1552. doi: 10.1089/neu.2004.21.1539
Babikian, T., Marion, S. D., Copeland, S., Alger, J. R., O’Neill, J., Cazalis, F., et al. (2010). Metabolic levels in the corpus callosum and their structural and behavioral correlates after moderate to severe pediatric TBI. J. Neurotrauma 27, 473–481. doi: 10.1089/neu.2009.1058
Bak, L. K., Schousboe, A., and Waagepetersen, H. S. (2006). The glutamate/GABA-glutamine cycle: aspects of transport, neurotransmitter homeostasis and ammonia transfer. J. Neurochem. 98, 641–653. doi: 10.1111/j.1471-4159.2006.03913.x
Bartnik-Olson, B., Alger, J., Babikian, T., Harris, A. D., Holshouser, B., Kirov, I. I., et al. (2019). The clinical utility of magnetic resonance spectroscopy in traumatic brain injury: recommendations from the ENIGMA MRS working group. Brain Imaging Behav. 15, 504–525.
Bartnik-Olson, B. L., Alger, J. R., Babikian, T., Harris, A. D., Holshouser, B., Kirov, I. I., et al. (2021). The clinical utility of proton magnetic resonance spectroscopy in traumatic brain injury: recommendations from the ENIGMA MRS working group. Brain Imaging Behav. 15, 504–525.
Bastuji, H., Frot, M., Perchet, C., Magnin, M., and Garcia-Larrea, L. (2016). Pain networks from the inside: spatiotemporal analysis of brain responses leading from nociception to conscious perception. Hum. Brain Mapp. 37, 4301–4315. doi: 10.1002/hbm.23310
Beck, A. T., Epstein, N., Brown, G., and Steer, R. A. (1988). An inventory for measuring clinical anxiety: Psychometric properties. J. Consult. Clin. Psychol. 56, 893–897. doi: 10.1037/0022-006X.56.6.893
Benarroch, E. E. (2020). What is the role of the cingulate cortex in pain? Neurology 95, 729–732. doi: 10.1212/WNL.0000000000010712
Benjamini, Y., and Hochberg, Y. (1995). Controlling the false discovery rate: a practical and powerful approach to multiple testing. J. R. Stat. Soc. Ser. B Methodol. 57, 289–300. doi: 10.1111/j.2517-6161.1995.tb02031.x
Bliss, T. V. P., Collingridge, G. L., Kaang, B. K., and Zhuo, M. (2016). Synaptic plasticity in the anterior cingulate cortex in acute and chronic pain. Nat. Rev. Neurosci. 17, 485–496. doi: 10.1038/nrn.2016.68
Bouferguène, S., Lapierre, A., Houzé, B., Rainville, P., and Arbour, C. (2019). Chronic central pain among community-dwelling survivors of moderate-to-severe traumatic brain injury: a quantitative sensory testing study. Biol. Res. Nurs. 21, 519–531. doi: 10.1177/1099800419859078
Bouhassira, D., Attal, N., Fermanian, J., Alchaar, H., Gautron, M., Masquelier, E., et al. (2004). Development and validation of the neuropathic pain symptom inventory. Pain 108, 248–257. doi: 10.1016/j.pain.2003.12.024
Bramlett, H. M., and Dietrich, W. D. (2015). Long-term consequences of traumatic brain injury: current status of potential mechanisms of injury and neurological outcomes. J. Neurotrauma. 32, 1834–1848. doi: 10.1089/neu.2014.3352
Brooks, J. C. W., Roberts, N., Kemp, G. J., Gosney, M. A., Lye, M., and Whitehouse, G. H. (2001). A proton magnetic resonance spectroscopy study of age-related changes in frontal lobe metabolite concentrations. Cereb. Cortex 11, 598–605. doi: 10.1093/cercor/11.7.598
Brooks, W. M., Friedman, S. D., and Gasparovic, C. (2001). Magnetic resonance spectroscopy in traumatic brain injury. J. Head Trauma Rehabil. 16, 149–164. doi: 10.1097/00001199-200104000-00005
Bushnell, M. C., Èeko, M., and Low, L. A. (2013). Cognitive and emotional control of pain and its disruption in chronic pain. Nat. Rev. Neurosci. 14, 502–511. doi: 10.1038/nrn3516
Cadoux-Hudson, T. A. D., Wade, D., Taylor, D. J., Rajagopalan, B., Ledingham, J. G. G., Briggs, M., et al. (1990). Persistent metabolic sequelae of severe head injury in humans in vivo. Acta Neurochir. 104, 1–7. doi: 10.1007/BF01842884
Capizzi, A., Woo, J., and Verduzco-Gutierrez, M. (2020). Traumatic brain injury: an overview of epidemiology, pathophysiology, and medical management. Med. Clin. 104, 213–238. doi: 10.1016/j.mcna.2019.11.001
Centers for Disease Control and Prevention [CDC] (2015). Report to Congress on Traumatic Brain Injury in the United States: Epidemiology and Rehabilitation. Atlanta: CDC.
Centers for Disease Control and Prevention [CDC] (2022). Mortality Data on CDC WONDER. Available online at: https://wonder.cdc.gov/mcd.html (accessed sep 20, 2022).
Chang, L., Munsaka, S. M., Kraft-Terry, S., and Ernst, T. (2013). Magnetic resonance spectroscopy to assess neuroinflammation and neuropathic pain. J. Neuroimmune Pharmacol. 8, 576–593. doi: 10.1007/s11481-013-9460-x
Costigan, M., Scholz, J., and Woolf, C. J. (2009). Neuropathic pain: a maladaptive response of the nervous system to damage. Annu. Rev. Neurosci. 32, 1–32. doi: 10.1146/annurev.neuro.051508.135531
Craig, D. A. (2009). How do you feel — now? the anterior insula and human awareness. Nat. Rev. Neurosci. 10, 59–70. doi: 10.1038/nrn2555
Croall, I., Smith, F. E., and Blamire, A. M. (2015). Magnetic resonance spectroscopy for traumatic brain injury. Top. Magn. Reson. Imaging 24, 267–274. doi: 10.1097/RMR.0000000000000063
Cruz-Almeida, Y., and Porges, E. (2021). Additional considerations for studying brain metabolite levels across pain conditions using proton magnetic resonance spectroscopy. NeuroImage 224:117392. doi: 10.1016/j.neuroimage.2020.117392
Davis, K. D., and Moayedi, M. (2013). Central mechanisms of pain revealed through functional and structural MRI. J. Neuroimmune Pharmacol. 8, 518–534. doi: 10.1007/s11481-012-9386-8
den Boer, C., Dries, L., Terluin, B., van der Wouden, J. C., Blankenstein, A. H., van Wilgen, C. P., et al. (2019). Central sensitization in chronic pain and medically unexplained symptom research: a systematic review of definitions, operationalizations and measurement instruments. J. Psychosom. Res. 117, 32–40. doi: 10.1016/j.jpsychores.2018.12.010
DeVience, S. J., Lu, X., Proctor, J., Rangghran, P., Melhem, E. R., Gullapalli, R., et al. (2017). Metabolic imaging of energy metabolism in traumatic brain injury using hyperpolarized [1-13C]pyruvate. Sci. Rep. 7:1907. doi: 10.1038/s41598-017-01736-x
Faghihi, R., Zeinali-Rafsanjani, B., Mosleh-Shirazi, M. A., Saeedi-Moghadam, M., Lotfi, M., Jalli, R., et al. (2017). Magnetic resonance spectroscopy and its clinical applications: a review. J. Med. Imaging Radiat. Sci. 48, 233–253. doi: 10.1016/j.jmir.2017.06.004
Ferris, J. K., Neva, J. L., Vavasour, I. M., Attard, K. J., Greeley, B., Hayward, K. S., et al. (2021). Cortical N-acetylaspartate concentrations are impacted in chronic stroke but do not relate to motor impairment: a magnetic resonance spectroscopy study. Hum. Brain Mapp. 42, 3119–3130. doi: 10.1002/hbm.25421
Fisher, S. K., Novak, J. E., and Agranoff, B. W. (2002). Inositol and higher inositol phosphates in neural tissues: homeostasis, metabolism and functional significance. J. Neurochem. 82, 736–754. doi: 10.1046/j.1471-4159.2002.01041.x
Forslund, M. V., Perrin, P. B., Røe, C., Sigurdardottir, S., Hellstrøm, T., Berntsen, S. A., et al. (2019). Global outcome trajectories up to 10 years after moderate to severe traumatic brain injury. Front. Neurol. 10:219. doi: 10.3389/fneur.2019.00219
Friebel, U., Eickhoff, S. B., and Lotze, M. (2011). Coordinate-based meta-analysis of experimentally induced and chronic persistent neuropathic pain. NeuroImage 58, 1070–1080. doi: 10.1016/j.neuroimage.2011.07.022
Fuchs, P. N., Peng, Y. B., Boyette-Davis, J. A., and Uhelski, M. L. (2014). The anterior cingulate cortex and pain processing. Front. Integr. Neurosci. 8:35. doi: 10.3389/fnint.2014.00035
Fukui, S., Matsuno, M., Inubushi, T., and Nosaka, S. (2006). N-Acetylaspartate concentrations in the thalami of neuropathic pain patients and healthy comparison subjects measured with 1H-MRS. Magn. Reson. Imaging 24, 75–79. doi: 10.1016/j.mri.2005.10.021
Garcia-Larrea, L., and Bastuji, H. (2018). Pain and consciousness. Prog. Neuropsychopharmacol. Biol. Psychiatry 87, 193–199. doi: 10.1016/j.pnpbp.2017.10.007
Garcia-Larrea, L., Perchet, C., Creac’h, C., Convers, P., Peyron, R., Laurent, B., et al. (2010). Operculo-insular pain (parasylvian pain): a distinct central pain syndrome. Brain 133, 2528–2539. doi: 10.1093/brain/awq220
Garcia-Larrea, L., and Peyron, R. (2013). Pain matrices and neuropathic pain matrices: a review. Pain 154, S29–S43. doi: 10.1016/j.pain.2013.09.001
Gardner, A. J., and Zafonte, R. (2016). Neuroepidemiology of traumatic brain injury. Handb. Clin. Neurol. 138, 207–223. doi: 10.1016/B978-0-12-802973-2.00012-4
Garnett, M. R., Blamire, A. M., Corkill, R. G., Cadoux-Hudson, T. A., Rajagopalan, B., and Styles, P. (2000a). Early proton magnetic resonance spectroscopy in normal-appearing brain correlates with outcome in patients following traumatic brain injury. Brain J. Neurol. 123(Pt 10), 2046–2054. doi: 10.1093/brain/123.10.2046
Garnett, M. R., Blamire, A. M., Rajagopalan, B., Styles, P., and Cadoux-Hudson, T. A. D. (2000b). Evidence for cellular damage in normal-appearing white matter correlates with injury severity in patients following traumatic brain injury: a magnetic resonance spectroscopy study. Brain 123, 1403–1409. doi: 10.1093/brain/123.7.1403
Garnett, M. R., Corkill, R. G., Blamire, A. M., Rajagopalan, B., Manners, D. N., Young, J. D., et al. (2001). Altered cellular metabolism following traumatic brain injury: a magnetic resonance spectroscopy study. J. Neurotrauma 18, 231–240. doi: 10.1089/08977150151070838
Gasparovic, C., Yeo, R., Mannell, M., Ling, J., Elgie, R., Phillips, J., et al. (2009). Neurometabolite concentrations in gray and white matter in mild traumatic brain injury: an 1H–magnetic resonance spectroscopy study. J. Neurotrauma 26, 1635–1643. doi: 10.1089/neu.2009.0896
Geha, P. Y., and Apkarian, A. V. (2005). Brain imaging findings in neuropathic pain. Curr. Pain Headache Rep. 9, 184–188. doi: 10.1007/s11916-005-0060-1
Glodzik-Sobanska, L., Li, J., Mosconi, L., Slowik, A., Walecki, J., Szczudlik, A., et al. (2007). Prefrontal N-acetylaspartate and poststroke recovery: a longitudinal proton spectroscopy study. Am. J. Neuroradiol. 28, 470–474.
Govind, V., Gold, S., Kaliannan, K., Saigal, G., Falcone, S., Arheart, K. L., et al. (2010). Whole-brain proton MR spectroscopic imaging of mild-to-moderate traumatic brain injury and correlation with neuropsychological deficits. J. Neurotrauma 27, 483–496. doi: 10.1089/neu.2009.1159
Govindaraju, V., Young, K., and Maudsley, A. A. (2000). Proton NMR chemical shifts and coupling constants for brain metabolites. NMR Biomed. 13, 129–153. doi: 10.1002/1099-1492(200005)13:3<129::AID-NBM619>3.0.CO;2-V
Grachev, I. D., and Apkarian, A. V. (2001). Aging alters regional multichemical profile of the human brain: an in vivo1H-MRS study of young versus middle-aged subjects. J. Neurochem. 76, 582–593. doi: 10.1046/j.1471-4159.2001.00026.x
Groh, A., Krieger, P., Mease, R. A., and Henderson, L. (2018). Acute and chronic pain processing in the thalamocortical system of humans and animal models. Neuroscience 387, 58–71. doi: 10.1016/j.neuroscience.2017.09.042
Gustin, S. M., Wrigley, P. J., Youssef, A. M., McIndoe, L., Wilcox, S. L., Rae, C. D., et al. (2014). Thalamic activity and biochemical changes in individuals with neuropathic pain after spinal cord injury. Pain 155, 1027–1036. doi: 10.1016/j.pain.2014.02.008
Haarbauer-Krupa, J., Taylor, C. A., Yue, J. K., Winkler, E. A., Pirracchio, R., Cooper, S. R., et al. (2017). Screening for post-traumatic stress disorder in a civilian emergency department population with traumatic brain injury. J. Neurotrauma 34, 50–58. doi: 10.1089/neu.2015.4158
Harris, J. L., Yeh, H. W., Choi, I. Y., Lee, P., Berman, N. E., Swerdlow, R. H., et al. (2012). Altered neurochemical profile after traumatic brain injury: 1H-MRS biomarkers of pathological mechanisms. J. Cereb. Blood Flow Metab. 32, 2122–2134. doi: 10.1038/jcbfm.2012.114
Henssen, D., Dijk, J., Knepflé, R., Sieffers, M., Winter, A., and Vissers, K. (2019). Alterations in grey matter density and functional connectivity in trigeminal neuropathic pain and trigeminal neuralgia: A systematic review and meta-analysis. NeuroImage Clin. 24:102039. doi: 10.1016/j.nicl.2019.102039
Holshouser, B., Pivonka-Jones, J., Nichols, J. G., Oyoyo, U., Tong, K., Ghosh, N., et al. (2019). Longitudinal metabolite changes after traumatic brain injury: a prospective pediatric magnetic resonance spectroscopic imaging study. J. Neurotrauma 36, 1352–1360. doi: 10.1089/neu.2018.5919
Holtzheimer, P. E., Husain, M. M., Lisanby, S. H., Taylor, S. F., Whitworth, L. A., McClintock, S., et al. (2017). Subcallosal cingulate deep brain stimulation for treatment-resistant depression: a multisite, randomised, sham-controlled trial. Lancet Psychiatry 4, 839–849. doi: 10.1016/S2215-0366(17)30371-1
Irvine, K. A., and Clark, J. D. (2018). Chronic pain after traumatic brain injury: pathophysiology and pain mechanisms. Pain Med. 19, 1315–1333. doi: 10.1093/pm/pnx153
Ito, T., Tanaka-Mizuno, S., Iwashita, N., Tooyama, I., Shiino, A., Miura, K., et al. (2017). Proton magnetic resonance spectroscopy assessment of metabolite status of the anterior cingulate cortex in chronic pain patients and healthy controls. J. Pain Res. 10, 287–293. doi: 10.2147/JPR.S123403
Javaid, S., Farooq, T., Rehman, Z., Afzal, A., Ashraf, W., Rasool, M. F., et al. (2021). Dynamics of choline-containing phospholipids in traumatic brain injury and associated comorbidities. Int. J. Mol. Sci. 22:11313. doi: 10.3390/ijms222111313
Ji, R. R., Berta, T., and Nedergaard, M. (2013). Glia and pain: is chronic pain a gliopathy? Pain 154, S10–S28. doi: 10.1016/j.pain.2013.06.022
Joyce, J. M., La, P. L., Walker, R., and Harris, A. D. (2022). Magnetic resonance spectroscopy of traumatic brain injury and subconcussive hits: a systematic review and meta–analysis. J. Neurotrauma 39, 1455–1476. doi: 10.1089/neu.2022.0125
Kerns, R. D., Turk, D. C., and Rudy, T. E. (1985). The west haven-yale multidimensional pain inventory (WHYMPI). Pain 23, 345–356. doi: 10.1016/0304-3959(85)90004-1
Khoury, S., and Benavides, R. (2018). Pain with traumatic brain injury and psychological disorders. Prog. Neuropsychopharmacol. Biol. Psychiatry 87, 224–233. doi: 10.1016/j.pnpbp.2017.06.007
Kierans, A. S., Kirov, I. I., Gonen, O., Haemer, G., Nisenbaum, E., Babb, J. S., et al. (2014). Myoinositol and glutamate complex neurometabolite abnormality after mild traumatic brain injury. Neurology 82, 521–528. doi: 10.1212/WNL.0000000000000105
King, N. S., Crawford, S., Wenden, F. J., Moss, N. E. G., and Wade, D. T. (1995). The rivermead post concussion symptoms questionnaire: a measure of symptoms commonly experienced after head injury and its reliability. J. Neurol. 242, 587–592. doi: 10.1007/BF00868811
Kragel, P. A., Kano, M., Van Oudenhove, L., Ly, H. G., Dupont, P., Rubio, A., et al. (2018). Generalizable representations of pain, cognitive control, and negative emotion in medial frontal cortex. Nat. Neurosci. 21, 283–289. doi: 10.1038/s41593-017-0051-7
Kubas, B., Łebkowski, W., Łebkowska, U., Kułak, W., Tarasow, E., and Walecki, J. (2010). Proton MR spectroscopy in mild traumatic brain injury. Pol. J. Radiol. 75, 7–10.
Kuner, R., and Flor, H. (2017). Structural plasticity and reorganisation in chronic pain. Nat. Rev. Neurosci. 18, 20–30. doi: 10.1038/nrn.2016.162
Kuner, R., and Kuner, T. (2021). Cellular circuits in the brain and their modulation in acute and chronic pain. Physiol. Rev. 101, 213–258. doi: 10.1152/physrev.00040.2019
Lahz, S., and Bryant, R. A. (1996). Incidence of chronic pain following traumatic brain injury. Arch. Phys. Med. Rehabil. 77, 889–891. doi: 10.1016/S0003-9993(96)90275-0
Leipelt, M., and Merrill, A. H. (2004). “Sphingolipid Biosynthesis,” in Encyclopedia of Biological Chemistry [Internet], eds W. J. Lennarz and M. D. Lane (New York: Elsevier). doi: 10.1016/B0-12-443710-9/00725-0
Leung, A. (2020). Addressing chronic persistent headaches after MTBI as a neuropathic pain state. J. Headache Pain 21:77. doi: 10.1186/s10194-020-01133-2
Levins, K. J., Drago, T., Roman, E., Martin, A., King, R., Murphy, P., et al. (2019). Magnetic resonance spectroscopy across chronic pain disorders: a systematic review protocol synthesising anatomical and metabolite findings in chronic pain patients. Syst. Rev. 8:338. doi: 10.1186/s13643-019-1256-5
Lin, C. S. (2014). Brain signature of chronic orofacial pain: a systematic review and meta-analysis on neuroimaging research of trigeminal neuropathic pain and temporomandibular joint disorders. PLoS One 9:e94300. doi: 10.1371/journal.pone.0094300
Lin, J. C., Mueller, C., Campbell, K. A., Thannickal, H. H., Daredia, A. F., Sheriff, S., et al. (2022). Investigating whole-brain metabolite abnormalities in the chronic stages of moderate or severe traumatic brain injury. PM&R 14, 472–485. doi: 10.1002/pmrj.12623
Maudsley, A. A., Darkazanli, A., Alger, J. R., Hall, L. O., Schuff, N., Studholme, C., et al. (2006). Comprehensive processing, display and analysis for in vivo MR spectroscopic imaging. NMR Biomed. 19, 492–503. doi: 10.1002/nbm.1025
Maudsley, A. A., Govind, V., Levin, B., Saigal, G., Harris, L., and Sheriff, S. (2015). Distributions of magnetic resonance diffusion and spectroscopy measures with traumatic brain injury. J. Neurotrauma 32, 1056–1063. doi: 10.1089/neu.2014.3505
Maudsley, A. A., Govind, V., Saigal, G., Gold, S. G., Harris, L., and Sheriff, S. (2017). Longitudinal MR spectroscopy shows altered metabolism in traumatic brain injury. J. Neuroimaging 27, 562–569. doi: 10.1111/jon.12463
Mayer, A. R., Quinn, D. K., and Master, C. L. (2017). The spectrum of mild traumatic brain injury: a review. Neurology 89, 623–632. doi: 10.1212/WNL.0000000000004214
Mercer Lindsay, N., Chen, C., Gilam, G., Mackey, S., and Scherrer, G. (2021). Brain circuits for pain and its treatment. Sci. Transl. Med. 13:eabj7360. doi: 10.1126/scitranslmed.abj7360
Miller, B. L., Changl, L., Booth, R., Ernst, T., Cornford, M., Nikas, D., et al. (1996). In vivo 1H MRS choline: correlation with in vitro chemistry/histology. Life Sci. 58, 1929–1935. doi: 10.1016/0024-3205(96)00182-8
Mills, E. P., Di Pietro, F., Alshelh, Z., Peck, C. C., Murray, G. M., Vickers, E. R., et al. (2018). Brainstem pain-control circuitry connectivity in chronic neuropathic pain. J. Neurosci. 38, 465–473. doi: 10.1523/JNEUROSCI.1647-17.2017
Moffett, J. R., Arun, P., Ariyannur, P. S., and Namboodiri, A. M. (2013). N-Acetylaspartate reductions in brain injury: impact on post-injury neuroenergetics, lipid synthesis, and protein acetylation. Front. Neuroenergetics 5:11. doi: 10.3389/fnene.2013.00011
Moffett, J. R., Ross, B., Arun, P., Madhavarao, C. N., and Namboodiri, A. M. (2007). N-Acetylaspartate in the CNS: from neurodiagnostics to neurobiology. Prog. Neurobiol. 81, 89–131. doi: 10.1016/j.pneurobio.2006.12.003
Moisset, X., and Bouhassira, D. (2007). Brain imaging of neuropathic pain. NeuroImage 37, S80–S88. doi: 10.1016/j.neuroimage.2007.03.054
Nampiaparampil, D. E. (2008). Prevalence of chronic pain after traumatic brain injury: a systematic review. JAMA 300, 711–719. doi: 10.1001/jama.300.6.711
Ng, S. Y., and Lee, A. Y. W. (2019). Traumatic brain injuries: pathophysiology and potential therapeutic targets. Front. Cell Neurosci. 13:528. doi: 10.3389/fncel.2019.00528
Ofek, H., and Defrin, R. (2007). The characteristics of chronic central pain after traumatic brain injury. Pain 131, 330–340. doi: 10.1016/j.pain.2007.06.015
Pan, P. L., Zhong, J. G., Shang, H. F., Zhu, Y. L., Xiao, P. R., Dai, Z. Y., et al. (2015). Quantitative meta-analysis of grey matter anomalies in neuropathic pain. Eur. J. Pain 19, 1224–1231. doi: 10.1002/ejp.670
PAR (2020). Mini-Mental State Examination, Second Edition | MMSE-2. Available online at: https://www.parinc.com/Products/Pkey/238 (accessed Dec 7, 2020).
Pattany, P. M., Yezierski, R. P., Widerström-Noga, E. G., Bowen, B. C., Martinez-Arizala, A., Garcia, B. R., et al. (2002). Proton magnetic resonance spectroscopy of the thalamus in patients with chronic neuropathic pain after spinal cord injury. Am. J. Neuroradiol. 23, 901–905.
Pavlovic, D., Pekic, S., Stojanovic, M., and Popovic, V. (2019). Traumatic brain injury: neuropathological, neurocognitive and neurobehavioral sequelae. Pituitary 22, 270–282. doi: 10.1007/s11102-019-00957-9
Peek, A. L., Rebbeck, T., Puts, N. A. J., Watson, J., Aguila, M. E. R., and Leaver, A. M. (2020). Brain GABA and glutamate levels across pain conditions: a systematic literature review and meta-analysis of 1H-MRS studies using the MRS-Q quality assessment tool. NeuroImage 210:116532. doi: 10.1016/j.neuroimage.2020.116532
Ponsford, J. L., Downing, M. G., Olver, J., Ponsford, M., Acher, R., Carty, M., et al. (2014). Longitudinal follow-up of patients with traumatic brain injury: outcome at two, five, and ten years post-injury. J Neurotrauma. 31, 64–77. doi: 10.1089/neu.2013.2997
Prins, M., Greco, T., Alexander, D., and Giza, C. C. (2013). The pathophysiology of traumatic brain injury at a glance. Dis. Model. Mech. 6, 1307–1315. doi: 10.1242/dmm.011585
Quade, D. (1967). Rank analysis of covariance. J. Am. Stat. Assoc. 62, 1187–1200. doi: 10.1080/01621459.1967.10500925
Rabinowitz, A. R., Kumar, R., Sima, A., Venkatesan, U. M., Juengst, S., O’Neil-Pirozzi, T. M., et al. (2021). Aging with traumatic brain injury: deleterious effects of injury chronicity are most pronounced in later life. J. Neurotrauma 38, 2706–2713. doi: 10.1089/neu.2021.0038
Rabinowitz, A. R., and Levin, H. S. (2014). Cognitive sequelae of traumatic brain injury. Psychiatr. Clin. North Am. 37, 1–11. doi: 10.1016/j.psc.2013.11.004
Ramonet, D., Rodríguez, M. J., Fredriksson, K., Bernal, F., and Mahy, N. (2004). In vivo neuroprotective adaptation of the glutamate/glutamine cycle to neuronal death. Hippocampus 14, 586–594. doi: 10.1002/hipo.10188
Reyngoudt, H., Claeys, T., Vlerick, L., Verleden, S., Acou, M., Deblaere, K., et al. (2012). Age-related differences in metabolites in the posterior cingulate cortex and hippocampus of normal ageing brain: A 1H-MRS study. Eur. J. Radiol. 81:e223-31. doi: 10.1016/j.ejrad.2011.01.106
Robayo, L. E., Govind, V., Vastano, R., Felix, E. R., Fleming, L., Cherup, N. P., et al. (2022). Multidimensional pain phenotypes after traumatic brain injury. Front Pain Res. 3:947562. doi: 10.3389/fpain.2022.947562
Ruggiero, K. J., Ben, K. D., Scotti, J. R., and Rabalais, A. E. (2003). Psychometric properties of the PTSD checklist—civilian version. J. Trauma Stress 16, 495–502. doi: 10.1023/A:1025714729117
Scholz, J., Finnerup, N. B., Attal, N., Aziz, Q., Baron, R., Bennett, M. I., et al. (2019). The IASP classification of chronic pain for ICD-11: chronic neuropathic pain. Pain 160, 53–59. doi: 10.1097/j.pain.0000000000001365
Seminowicz, D. A., and Moayedi, M. (2017). The dorsolateral prefrontal cortex in acute and chronic pain. J. Pain 18, 1027–1035. doi: 10.1016/j.jpain.2017.03.008
Shutter, L., Tong, K. A., and Holshouser, B. A. (2004). Proton MRS in acute traumatic brain injury: role for glutamate/glutamine and choline for outcome prediction. J. Neurotrauma 21, 1693–1705. doi: 10.1089/neu.2004.21.1693
Smith, L. G., Milliron, E., Ho, M. L., Hu, H. H., Rusin, J., Leonard, J., et al. (2019). Advanced neuroimaging in traumatic brain injury: an overview. Neurosurg. Focus 47:E17. doi: 10.3171/2019.9.FOCUS19652
Sorensen, L., Siddall, P. J., Trenell, M. I., and Yue, D. K. (2008). Differences in metabolites in pain-processing brain regions in patients with diabetes and painful neuropathy. Diabetes Care 31, 980–981. doi: 10.2337/dc07-2088
Starr, C. J., Sawaki, L., Wittenberg, G. F., Burdette, J. H., Oshiro, Y., Quevedo, A. S., et al. (2009). Roles of the insular cortex in the modulation of pain: insights from brain lesions. J. Neurosci. 29, 2684–2694. doi: 10.1523/JNEUROSCI.5173-08.2009
Steer, R. A., Brown, G. K., Beck, A. T., and Sanderson, W. C. (2001). Mean beck depression inventory–II scores by severity of major depressive episode. Psychol. Rep. 88, 1075–1076. doi: 10.2466/pr0.2001.88.3c.1075
Tan, L. L., and Kuner, R. (2021). Neocortical circuits in pain and pain relief. Nat. Rev. Neurosci. 22, 458–471. doi: 10.1038/s41583-021-00468-2
Treede, R. D., Apkarian, A. V., Bromm, B., Greenspan, J. D., and Lenz, F. A. (2000). Cortical representation of pain: functional characterization of nociceptive areas near the lateral sulcus. Pain 87, 113–119. doi: 10.1016/S0304-3959(00)00350-X
Tzourio-Mazoyer, N., Landeau, B., Papathanassiou, D., Crivello, F., Etard, O., Delcroix, N., et al. (2002). Automated anatomical labeling of activations in SPM using a macroscopic anatomical parcellation of the MNI MRI single-subject brain. NeuroImage 15, 273–289. doi: 10.1006/nimg.2001.0978
Walton, K. D., and Llinás, R. R. (2010). “Central pain as a thalamocortical dysrhythmia: a thalamic efference disconnection?,” in Translational Pain Research: from Mouse to Man [Internet], eds L. Kruger and A. R. Light (Boca Raton, FL: CRC Press). doi: 10.1201/9781439812105-c13
Weathers, F. (1993). “The PTSD checklist : Reliability, validity and diagnostic utility,” in Paper Presented at the Annual Meeting of the International Society for Traumatic Stress Studies, (San Antonio, TX).
Werner, C., and Engelhard, K. (2007). Pathophysiology of traumatic brain injury. Br. J. Anaesth. 99, 4–9. doi: 10.1093/bja/aem131
Widerström-Noga, E., Cruz-Almeida, Y., Felix, E. R., and Pattany, P. M. (2015). Somatosensory phenotype is associated with thalamic metabolites and pain intensity after spinal cord injury. Pain 156, 166–174. doi: 10.1016/j.pain.0000000000000019
Widerström-Noga, E., Govind, V., Adcock, J. P., Levin, B. E., and Maudsley, A. A. (2016). Subacute pain after traumatic brain injury is associated with lower insular N-acetylaspartate concentrations. J. Neurotrauma 33, 1380–1389. doi: 10.1089/neu.2015.4098
Widerström-Noga, E., Pattany, P. M., Cruz-Almeida, Y., Felix, E. R., Perez, S., Cardenas, D. D., et al. (2013). Metabolite concentrations in the anterior cingulate cortex predict high neuropathic pain impact after spinal cord injury. Pain 154, 204–212. doi: 10.1016/j.pain.2012.07.022
Yeo, R. A., Gasparovic, C., Merideth, F., Ruhl, D., Doezema, D., and Mayer, A. R. (2011). A longitudinal proton magnetic resonance spectroscopy study of mild traumatic brain injury. J. Neurotrauma 28, 1–11. doi: 10.1089/neu.2010.1578
Yoon, S. J., Lee, J. H., Kim, S. T., and Chun, M. H. (2005). Evaluation of traumatic brain injured patients in correlation with functional status by localized 1H-MR spectroscopy. Clin. Rehabil. 19, 209–215. doi: 10.1191/0269215505cr813oa
Keywords: traumatic brain injury, chronic pain, N-acetylaspartate, brain metabolite, magnetic resonance spectroscopic imaging
Citation: Robayo LE, Govind V, Salan T, Cherup NP, Sheriff S, Maudsley AA and Widerström-Noga E (2023) Neurometabolite alterations in traumatic brain injury and associations with chronic pain. Front. Neurosci. 17:1125128. doi: 10.3389/fnins.2023.1125128
Received: 15 December 2022; Accepted: 07 February 2023;
Published: 23 February 2023.
Edited by:
Liangjie Lin, Philips Healthcare, ChinaReviewed by:
Yanqin Lin, Xiamen University, ChinaJin Gao, University of Illinois Chicago, United States
Copyright © 2023 Robayo, Govind, Salan, Cherup, Sheriff, Maudsley and Widerström-Noga. This is an open-access article distributed under the terms of the Creative Commons Attribution License (CC BY). The use, distribution or reproduction in other forums is permitted, provided the original author(s) and the copyright owner(s) are credited and that the original publication in this journal is cited, in accordance with accepted academic practice. No use, distribution or reproduction is permitted which does not comply with these terms.
*Correspondence: Eva Widerström-Noga, ZXdpZGVyc3Ryb20tbm9nYUBtZWQubWlhbWkuZWR1