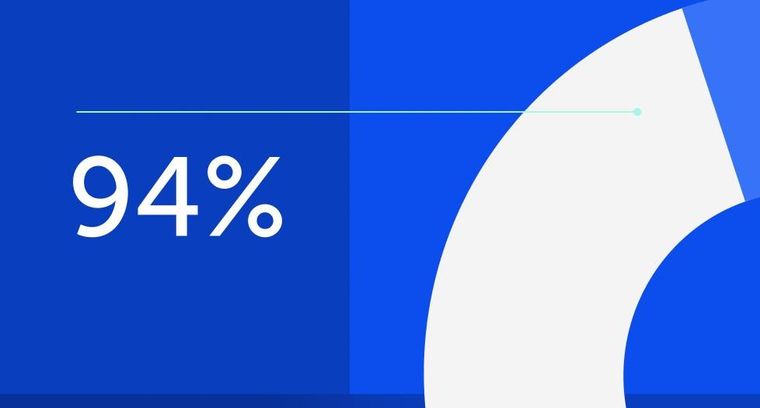
94% of researchers rate our articles as excellent or good
Learn more about the work of our research integrity team to safeguard the quality of each article we publish.
Find out more
REVIEW article
Front. Neurosci., 07 March 2023
Sec. Neuroendocrine Science
Volume 17 - 2023 | https://doi.org/10.3389/fnins.2023.1082449
This article is part of the Research TopicGrowth Hormone in the Nervous System: From Biology to TherapyView all 5 articles
Advanced age is typically associated with a decrease in cognitive function including impairment in the formation and retention of new memories. The hippocampus is critical for learning and memory, especially spatial learning, and is particularly affected by ageing. With advanced age, multiple neural components can be detrimentally affected including a reduction in the number of neural stem and precursor cells, a decrease in the formation of adult born neurons (neurogenesis), and deficits in neural circuitry, all of which ultimately contribute to impaired cognitive function. Importantly, physical exercise has been shown to ameliorate many of these impairments and is able to improve learning and memory. Relevantly, growth hormone (GH) is an important protein hormone that decreases with ageing and increases following physical exercise. Originally described due to its role in longitudinal growth, GH has now been identified to play several additional key roles, especially in relation to the brain. Indeed, the regular decrease in GH levels following puberty is one of the most well documented components of neuroendocrine ageing. Growth hormone deficiency (GHD) has been described to have adverse effects on brain function, which can be ameliorated via GH replacement therapy. Physical exercise has been shown to increase circulating GH levels. Furthermore, we recently demonstrated the increase in exercise-mediated GH is critical for improved cognitive function in the aged mouse. Here we examine the multiple roles that GH plays, particularly in the aged brain and following trauma, irradiation and stroke, and how increasing GH levels can ameliorate deficits in cognition.
It has long been known that the pituitary gland is critical for growth. In 1912, Cushing postulated the existence of a “hormone of growth” within the pituitary gland. Experiments in the 1920s, in which intraperitoneal injections of bovine pituitary gland extracts increased the growth of rats (Evans and Long, 1922; Johnson and Everett, 1929), confirmed this hypothesis. The discovery and successful purification of growth hormone (GH), however, took several subsequent decades starting with the isolation of oxen GH in 1944 (Li and Evans, 1944) and culminating with the purification of human GH (hGH) in 1956 (Li and Papkoff, 1956). Within 2 years of this discovery, the positive effect of GH treatment on human growth was reported (Raben, 1958). Treatment of patients with purified hGH required obtaining human pituitary glands and therefore GH therapy was reserved for the most severe cases of GH deficiency (GHD) (Ayyar, 2011). The identification of the hGH sequence in the 1960s (Li et al., 1966, 1969) and the sequence of bovine GH soon after (Graf and Li, 1974), allowed for the successful cloning and expression of hGH, thereby allowing a wider range of individuals to be treated with GH. This was followed by the cloning of the receptor itself and the elucidation of its signalling processes (reviewed in Waters, 2016).
The availability of pure hGH made it possible to measure circulating hGH levels by immunoassay, beginning in the 1960s. The pulsatile release of GH was then documented by Tannenbaum and Martin (1976). It was discovered that both males and females had multiple GH peaks throughout the day and night with young females possessing the highest peaks (Ho et al., 1987). Soon after, it became apparent that circulating levels of GH changed throughout a person’s lifetime with peak GH levels reached at puberty for both genders. In both genders, this is followed by an age-dependent decrease in both general baseline levels and pulsatile peak heights (Ho et al., 1987). It is now accepted that peak levels of GH decrease approximately 10–15% every consecutive decade beyond the age of 30 (Garcia et al., 2019). This decrease, coupled with a general decrease in metabolism and body composition, is collectively referred to as Somatopause (Lanfranco et al., 2003; Roelfsema and Veldhuis, 2016). Individuals over 60 have been routinely diagnosed as being GHD. Those suffering from adult onset GHD typically have deficits in body composition, cognitive processing and general wellbeing (Sattler, 2013) compared to age-matched controls.
Concurrent with the age-dependent decrease in GH levels is an age-associated decrease in certain domains of cognitive ability. The formation and retention of new memories, especially those related to spatial learning, appear to be especially sensitive to ageing (Gallagher and Pelleymounter, 1988). The hippocampus is a region of the brain critical for a range of cognitive processes including learning and successful retention of memories (Lisman et al., 2017). It is also one of two main regions in the mammalian brain that continue to generate new neurons during adulthood in a multi-step process referred to as neurogenesis (Eriksson et al., 1998). Neurons generated in the dentate gyrus of the hippocampus are critical for spatial learning (Vukovic et al., 2013). The second region that continues neurogenesis into adulthood is the subventricular zone (SVZ) of the lateral ventricle. Immature neurons generated in the SVZ migrate to the olfactory bulb where they integrate and contribute to olfaction. Both brain regions are detrimentally affected with advanced age, resulting in deficits at each stage of neurogenesis including; a reduction in the number of activated neural stem cells (NSCs) (Walker et al., 2008; Blackmore et al., 2009), a decrease in adult born neurons (Blackmore et al., 2021), deficits in cognitive function including spatial learning (van Praag et al., 2005) and reduced fine olfactory discrimination (Enwere et al., 2004).
Physical exercise is one of the strongest positive stimulators of both cognitive function and circulating GH. The beneficial effects of physical exercise on the brain, especially in relation to hippocampal neurogenesis and spatial learning, was originally discovered in adult mice by the seminal work of van Praag et al. (1999a,b). This was then extended to aged mice some years later (van Praag et al., 2005). Similarly, physical exercise has been described to be beneficial to human cognition in both young (Winter et al., 2007) and aged individuals (Cotman and Berchtold, 2002). Importantly, physical exercise has also been clearly demonstrated to increase circulating GH levels. Studies in humans reported that exercise-mediated increases in GH levels (Sutton and Lazarus, 1976) are exercise intensity-dependent (Felsing et al., 1992; Pritzlaff et al., 1999). Importantly, exercise, even in individuals of advanced age, continues to mediate increases in GH levels of both males and females (Giannoulis et al., 2005; Weltman et al., 2006). Similarly, in rodents, it was found that GH levels increased in the rat following treadmill exercise (Bigbee et al., 2000). We recently confirmed comparable results in adult and aged mice (Blackmore et al., 2021).
Even though there are coincident decreases in GH levels and cognitive function during ageing, and both respond positively to exercise, it was originally believed that GH affected peripheral tissues exclusively and not the brain. Therefore, any effect of GH on the brain was assumed to be indirect. Work in the late 1970s and early 1980s began to alter this view with the finding that intravenous injection of rGH in hypophysectomised rat resulted in decreased catecholamine secretion from the hypothalamus (Andersson et al., 1977, 1983). The finding that the GH receptor (GHR) is expressed in the rodent (Lobie et al., 1993) and human (Castro et al., 2000) brain and the demonstration that GH is transported into the brain (Pan et al., 2005), further established the potential for a direct role of GH mediating effects within the brain. It is now well established that GH directly affects several components within the brain, including stem cell activation and neural plasticity. This manifests as altered brain structure in children with isolated GHD, notably in reduced hippocampus, thalamus, and globus pallidum volumes as assessed by MRI (Webb et al., 2012). Ultimately these GH-mediated changes culminate in improvement of some components of cognitive ability in GH replacement models of GHD and clinical GHD, particularly those related to fluid memory to which spatial learning belongs. There is also an improvement in processing speed and movement-associated battery scores in isolated GHD children receiving GH replacement (Webb et al., 2012). It should be noted that adult-onset GHD is not limited to disorders of the pituitary or physiological age but can also be induced through injury including stroke or following trauma including whole brain irradiation. Here we review the effects of manipulating GH, either naturally or pharmacologically, within the brain with particular reference to age and injury. It should be noted that the effect of altering GH levels is often described interchangeably with changes in the GH/IGF-1 axis. This is because IGF-1 was originally believed to be responsible for mediating all of GH actions, a view that has since altered to include direct effects of GH (Sonntag et al., 2005). This original assumption was likely because IGF-1 can pass through the blood–brain barrier (BBB) and has also been shown to enhance NSC proliferation, neurogenesis (Aberg, 2010), and improve cognitive function (Aleman et al., 1999). For the purposes of this review, the focus will be on the direct effect of GH within the brain.
The first identification and mapping of murine GHRs within the brain was published in 1993 (Lobie et al., 1993). This finding was surprising as prior to this, the role of GH was considered to involve only peripheral tissues. Notably, this study found that GHRs were strongly expressed during neurogenesis and decreased subsequently, intimating at a potential role during brain development. Consequent studies also identified the presence of GHR within embryonic rat cerebral cortical cells (Ajo et al., 2003) and the dentate gyrus of the adult rat hippocampus (Fujikawa et al., 2000). GHR was identified within neural tissue of other species including chicken (Harvey et al., 2002) and humans (Castro et al., 2000). The first indication that GH is capable of crossing the BBB came when adult-onset GHD patients treated with recombinant human GH (rhGH) demonstrated a dose-dependent increase of GH within the CSF (Johansson et al., 1995; Burman et al., 1996). Confirmation of GH influx across the BBB of rodents was then achieved by measuring 125I-labelled rat GH in brain homogenates of both mice and rats (Pan et al., 2005). This finding further solidified the potential for a direct role of GH within the central nervous system (CNS). Of note, Pan et al. (2005) ruled out a specific active transport system for GH as they were unable to identify a saturable active transport system. Interestingly, whilst the majority of GH is synthesised within the anterior pituitary gland, it is now established that GH is also synthesised within non-pituitary regions of the brain of birds (Ramesh et al., 2000) and rodents (Donahue et al., 2006), including the Ames dwarf mouse that is deficient in pituitary-derived GH (Sun et al., 2005). The finding of extrapituitary GH in the brain supports a role for a GH brain axis separate from the periphery but does not exclude input from circulating pituitary GH through its uptake via the BBB.
In order to determine the identity of neural GHR+ve cells, we subsequently used FACS to isolate these cells from the SVZ of the lateral ventricles of adult mice (Blackmore et al., 2012a). This region of the lateral ventricles has been demonstrated to be the primary location of SVZ neural stem and precursor cells (Lois and Alvarez-Buylla, 1994). In vitro culture of these cells confirmed the ability of GHR+ve cells to display the main characteristics attributed to stem cells (Gage, 2000): form neurospheres, undergo long-term proliferation and become multipotent upon differentiation (Blackmore et al., 2012a). More recently we showed that the GHR transcript was enriched in Nestin-GFP+ve/EGFR+ve precursor cells purified from the adult murine hippocampus (Blackmore et al., 2021). Together, these results demonstrate that GHR is expressed within the adult brain, including in neural precursor cell populations from the two main neurogenic regions of the brain. It is interesting to note that, as with the decrease in GH with advancing age, reports demonstrate age-dependent decreases in GHR density within the human brain (Lai et al., 1993) and throughout the CNS of rats, including the hippocampus (Lobie et al., 1993; Zhai et al., 1994).
Given GH’s known role regarding the growth of internal organs and peripheral tissue during development, it is perhaps not surprising that GH has also been demonstrated to be involved in the induction of embryonic NSC proliferation. Initial experiments conducted on rat embryonic cerebral cortical tissue demonstrated that addition of GH increased proliferation of cortical precursors (Ajo et al., 2003). Similarly, in vitro GH treatment increased the rate of proliferation in passaged embryonic mouse SVZ neurospheres (Christophidis et al., 2009). The ability of GH to increase the rate of proliferation in NSCs was confirmed for humans using human embryonic-derived NSCs (Pathipati et al., 2011). Addition of GH to adult, wild-type (WT) SVZ primary cultures both increased neurosphere number and proliferation rate (McLenachan et al., 2009), demonstrating that GH continues to activate NSCs in adult neural tissue. In these studies, however, unsorted primary neural tissue was used to examine the effect of GH on stem cell proliferation. It was therefore important to determine if GH directly activated NSC populations by examining the effect of GH on purified subpopulations of NSCs.
We therefore took advantage of our sub-populations of neural precursor and stem cells that expressed GHR+ve purified from both the SVZ and hippocampal regions of adult WT mice (Blackmore et al., 2012a,2021) to examine this. In vitro addition of GH to purified adult SVZ GHR+ve cells increased neurosphere number and expansion rate during long-term serial passaging, indicating that GH directly activated NSCs (Blackmore et al., 2012a). Direct activation by GH was confirmed when primary SVZ tissue was cultured in vitro and the N-CFC assay was used to identify stem cell-derived colonies based on size (Reynolds and Rietze, 2005). A higher number of large, stem cell-derived colonies followed the addition of exogenous GH (Blackmore et al., 2012a). Purified, clonal adult hippocampal Nestin-GFP+ve/EGFR+ve precursor cells were also found to be responsive to in vitro GH treatment (Blackmore et al., 2021). Taken together, these results indicate that GH can act directly on NSCs in adult brain samples.
Unfortunately, during ageing, there is a dramatic decrease in NSC number within both the hippocampus (Walker et al., 2008) and SVZ (Blackmore et al., 2009), making it technically impossible to obtain purified stem cell populations from aged brains on which to test directly the effect of GH in vitro. However, unsorted neurosphere cultures from aged tissue confirmed that ageing NSCs retain their ability to be activated with GH treatment in both the SVZ and hippocampus (Blackmore et al., 2012b,2021). Interestingly, similar to the ageing brain, there is also a marked decrease in stem cell number in GHR knockout (GHRKO) mice (McLenachan et al., 2009; Deleyrolle et al., 2011; Blackmore et al., 2012a). This decreased number of stem cells may contribute to the decreased size of brains from GHRKO animals compared to WT littermates. SVZ precursor cells from GHRKO animals possess a reduced neurosphere size (McLenachan et al., 2009), a decrease in passaged proliferation rate (Blackmore et al., 2012a), and as one would expect, do not respond to exogenous GH in in vitro culture (Blackmore et al., 2012b).
As described above, whilst adult GHRKO animals show a decreased proportion of NSCs, they do retain a population of stem cells. Similarly, inhibition of GH action in vitro via addition of a GH antagonist to adult (Blackmore et al., 2012b; Devesa et al., 2014) and aged brains (Blackmore et al., 2021) reduces precursor cell number to non-activated, control levels. This suggests that GH activates a subpopulation of endogenous neural precursor cells. It appears that at least two distinct sub-populations of precursor cells reside within the adult brain that can be activated by alternative stimuli, a finding that was confirmed within the hippocampus of adult mice (Jhaveri et al., 2015).
Having demonstrated that GH activates NSC proliferation in embryonic, adult and aged primary tissue using in vitro tissue culture techniques, attention moved to the effect of exogenous GH treatment on proliferation in vivo. One of the first studies to address this demonstrated that a 10-week, twice daily subcutaneous (s.c.) injection of rhGH significantly increased the number of hippocampal neurons in aged Wistar rats (Azcoitia et al., 2005). However, in this study, it was not possible to determine if GH treatment altered proliferation rates or increased neuronal survival. This was subsequently addressed when adult hypophysectomised rats were injected s.c. daily with bovine GH (bGH) for 6 or 28 days in combination with intraperitoneal BrdU injections (Aberg et al., 2009). This approach was undertaken to remove the confound of pituitary derived GH and to induce GHD in the rat. Interestingly, both injection paradigms successfully increased the number of BrdU+ve cells in the hippocampus, however, only the longer, 28-day bGH treatment increased proliferation within the SVZ. This suggests differences in precursor cell activation timing between these two neurogenic regions. Subsequently, Aberg et al. (2010) also examined the effect of peripheral bGH treatment on neural proliferation in the intact adult rat brain. In this instance, a 5-day s.c. injection paradigm induced a doubling in BrdU+ve cells within the dentate gyrus, thus demonstrating that GH supplementation can augment hippocampal cell proliferation above normal levels. To examine the direct effect of GH supplementation, we conducted intercranial ventricular (ICV) infusion of GH into adult (Blackmore et al., 2012a) and aged (Blackmore et al., 2012b) WT mice. In both instances, we observed significant increases in NSC activation as measured by increased neurosphere number. Interestingly, we also noted that an acute, 7-day infusion appeared to increase the number of NSCs for at least 120 days (Blackmore et al., 2012a), suggesting a reprogramming of stem cell activation status. This may occur by altering the methylation or epigenetic status within these cells, as was recently demonstrated in a human study in which a combinational injection paradigm that included GH, DHEA and metformin reversed the epigenetic age of a small cohort of human subjects (Fahy et al., 2019).
Control of GH secretion is complex. It continues to be the focus of extensive research and has been comprehensively addressed in multiple excellent reviews (Muller et al., 1999; Pombo et al., 2001). Contributing to the complexity of GH release are the physiological components of ageing, which continue to be elucidated. The age-related decrease in circulating GH levels is the culmination of several inter-connected cascading events, with the main components including a change in: (1) the relative abundance, sensitivity and responsiveness between the two main positive and negative regulators of GH secretion, GH releasing hormone (GHRH) and somatostatin (SRIF) respectively; (2) an age-dependent change in the principal form of SRIF; and (3) morphological changes to the pituitary gland (Console et al., 1993).
Whilst there is little change in the frequency of GH pulsatile release, the pulse amplitude is dramatically reduced during ageing for both genders (Ho et al., 1987). Induction of a GH pulse requires appropriate timing of upstream regulatory components, which include both the release of GHRH and an inhibition of somatostatin tone (Hindmarsh et al., 1991). Early work demonstrated an age-dependent decrease in the number of GHRH producing neurons and GHRH mRNA levels within the hypothalamus of ageing rats (DeGennaro Colonna et al., 1989). A decrease in GHRH was therefore considered to contribute to the reduced amount of GH being secreted with advanced age.
A change in somatostatin level was also touted as being the predominant mechanism by which GH levels were reduced. Initial experiments showed that the pharmacological induction of GH release was significantly blunted in aged rats (Sonntag et al., 1981). Co-injection of somatostatin antiserum, however, resulted in comparable secreted GH levels between adult and aged male rats, leading the authors to two possible conclusions – the first being that SRIF becomes more abundant during ageing whilst the second was that the pituitary becomes more sensitive to SRIF (Sonntag et al., 1981). Subsequent work by this group appeared to point to the latter as induction of GH release was reduced in aged rats in vivo. However, when pituitary gland slices from adult and aged rats were removed and treated in vitro with synthetic GH releasing factor, similar levels of secreted GH were obtained from the two preparations following stimulation (Sonntag et al., 1983), a finding that demonstrated an increased influence of hypothalamic control of GH release during ageing. Interestingly, whilst the concentration of basal levels of SRIF remain constant during ageing (Sonntag et al., 1986), the relative concentrations of the two main isoforms, somatostatin-14 and somatostatin-28 (SRIF-14 and -28, respectively), do not. Following the discovery of multiple isoforms of SRIF (Meyers et al., 1980; Patel et al., 1981), Tannenbaum et al. (1982) showed that SRIF-28 is longer lasting on the pituitary. Soon after, Sonntag et al. (1986) demonstrated an age-dependent increase in the prevalence of SRIF-28 in male rats. Taken together, it appears that SRIF, especially the age-associated SRIF-28 isoform, plays a key role in the diminution of GH secretion from the pituitary gland during ageing. This is compounded by significant age-dependent decreases in both the number and volume of individual somatotroph cells within the pituitary gland (Console et al., 1993), a finding we recently confirmed in ageing WT mice (Blackmore et al., 2021).
As can be seen from above, the aetiology of an age-dependent decrease of GH secretion is multifactorial. A decrease in the presence of GHRH-containing neurons in the hypothalamus (DeGennaro Colonna et al., 1989), coupled with an increase in the more potent bioactive, age-associated SRIF-28 somatostatin isoform (Tannenbaum et al., 1982), likely contribute to morphological changes observed in the pituitary gland (Console et al., 1993). This culminates in a significant decrease in GH secretion, which has been demonstrated to have multiple deleterious effects in physiological ageing. Furthermore, as described above, there is also a decrease in the distribution of GHRs within the aged brain (Lobie et al., 1993; Zhai et al., 1994). This constellation of events is collectively referred to as somatopause. Importantly however, even at an advanced age, it is still possible to stimulate GH release naturally with physical exercise being one of the most effective physiological approaches by which to achieve this.
Slowing the age-dependent decrease in GH under physiological conditions has been shown to improve multiple health parameters. These include a change in body composition, improved heart health, metabolism and importantly, altered cognitive health in terms of mood and enhancement of multiple components of learning and memory. One of the most effective ways to increase GH secretion is via physical exercise. Some of the earliest work noted that aerobic physical exercise increased GH levels in humans in an intensity-dependent manner (Sutton and Lazarus, 1976). Indeed, as testament to the effectiveness of exercise increasing GH levels, this approach surpassed the pharmacological induction of GH release induced by arginine and L-DOPA (Sutton and Lazarus, 1976). Continued research in this field proposed key components that govern the amount of GH released during and immediately after physical exercise. These include gender (Pritzlaff-Roy et al., 2002), obesity (Kanaley et al., 1999), physical fitness (Holt et al., 2001), exercise intensity (Felsing et al., 1992; Pritzlaff et al., 1999), and age (Hagberg et al., 1988; Craig et al., 1989a,b; Pyka et al., 1992).
To better understand the age-associated diminution of GH responses to exercise, research groups began to interrogate further these potential regulatory components. For example, some have shown that increased adiposity can result in a significant attenuation of exercise-mediated GH release in young adult men (Weltman et al., 2001) and women (Kanaley et al., 1999). However, another group found that under comparable exercise conditions, overweight young male adults retained the ability to increase GH levels (Holt et al., 2001). In contrast, aged overweight males showed a reduced GH response to exercise compared to age-matched, lean individuals. Using multivariate analysis, these authors determined that age, and not adiposity, was the main determining factor in the decreased response. They also determined that during ageing, maintenance of physical fitness as assessed by VO2 max, is also critical to the preservation of GH secretion levels (Holt et al., 2001). Interestingly, endurance training, which can improve physical fitness, significantly enhances GH release after 1 year of training in young adults, especially when exercise is conducted above the Lactate threshold (Weltman et al., 1992). This response is blunted relatively early. Middle-aged men following a 4-month progressive endurance training paradigm exhibited a significant age-related decline in exercise-mediated GH secretion levels that was not enhanced following extended training (Zaccaria et al., 1999). Directly comparing gender and ages during a graded exercise testing paradigm similarly revealed an overall decrease in GH response in the aged participants (Weltman et al., 2006). This study also found no gender differences in response to exercise for the aged participants and that only the highest intensities of exercise resulted in statistically significant elevations in GH levels (Weltman et al., 2006). The latter decreased sensitivity to exercise suggests that ageing may increase the degree of inhibition of exercise-mediated GH release.
As discussed earlier, it is known that somatostatin, particularly the age-associated form, SRIF-28 (Sonntag et al., 1986), is a potent inhibitor of GH release from the pituitary. It is upregulated in response to raised GH levels (Giustina and Veldhuis, 1998). Previous studies have also shown that GH release in response to exercise is mediated by lowered somatostatin release (Thompson et al., 1993). To directly examine the influence of SRIF on GH release, Marcell et al. (1999) inhibited SRIF during exercise in adult and aged individuals. They found that administration of pyridostigmine bromide (PYR), an acetylcholine esterase inhibitor that inhibits SRIF secretion (Muller, 1997; Obermayr et al., 2005), enhanced the GH response to exercise in both adult and aged males. Whilst PYR administration in the aged participants significantly increased exercise-mediated GH secretion, it did not restore GH to youthful levels. This finding led the authors to conclude that additional components beyond somatostatin are involved in the age-dependent decrease in GH secretion following exercise.
Of note, studies examining the effect of exercise on GH levels in aged individuals also revealed that the exercise-induced increase in GH seen in aged individuals occurs later than in young adults (Marcell et al., 1999). Similarly, we recently showed that in young mice, GH levels are increased following acute periods of exercise whilst a prolonged period of voluntary wheel running was required to increase GH levels both in the blood and the pituitary gland of 24-month-old mice (Blackmore et al., 2021). This exercise-mediated increase in GH coincided with morphological changes in the pituitary gland, including an increase in both the total number of cells and the density of GH-containing cells in the pituitary of 24-month-old mice. A coincident decrease in SRIF levels was also observed in these animals. We found that pharmacological treatment to reduce somatostatin tone using donepezil, an acetylcholine esterase inhibitor, extended circulating GH levels during prolonged exercise (Blackmore et al., 2021). Finally, as with human studies (Weltman et al., 2006), we noted that a lower exercise intensity, achieved by using low resistance running discs, failed to increase GH levels in aged mice (Blackmore et al., 2021).
The optimal increase in exercise-mediated GH levels in adult and aged mice also coincided with an increase in NSCs. Indeed, we and others have found that optimisation of exercise to increase NSCs appeared to be dependent on age, with younger animals requiring acute exercise (Blackmore et al., 2009; Overall et al., 2013; Leiter et al., 2022) and older animals requiring a prolonged exercise duration (Kohman et al., 2012; Blackmore et al., 2021; Zhou et al., 2021). To determine if the exercise-mediated increase in stem cell number was due to increased GH activation, we directly infused competitive GH antagonists during exercise and found NSC number to remain at sedentary, non-run levels in SVZ and hippocampal neurogenic regions (Blackmore et al., 2012b,2021).
It is clear that advanced age results in decreased circulating GH levels, especially GH peaks, and physical exercise retains its ability to increase GH levels in aged humans and animals. Whilst peak GH levels in the aged fail to reach youthful levels, they appear to be sufficient to improve several measures of health and wellbeing, including measures of cognitive function. Animal models have further elucidated the mechanisms involved in these processes and shed light on how manipulation of GH levels contributes to improved learning and memory in those suffering from GHD, during ageing and following traumatic brain injury (TBI), stroke, and whole-brain irradiation therapy.
As stated above, animal models have been intrinsic to understanding the effect of GH on cognitive function. Induction of GHD, either in spontaneous GHD rats (Li E. et al., 2011) or more typically via hypophysectomising animals, robustly induces cognitive deficits in rodents. These deficits, typically expressed as decreased spatial learning ability, can be reversed via GH administration (Le Grevès et al., 2006; Ramis et al., 2013). In parallel with animal studies investigating the effect of GHD and GH replacement therapy on cognition, clinical studies also examined this as a potential therapeutic approach. A relationship between cognitive measures and those suffering from GHD in humans was established by the early work of Deijen et al. (1996). They compared differences in psychological complaints between adult males suffering from GHD as a result of either isolated growth hormone deficiency (IGHD) or multiple pituitary hormone deficiency (MPHD). This wide-ranging study identified that MPHD subjects possessed a more severe deficit in GH secretion than IGHD patients. The MPHD subjects also displayed a wider range of psychological issues including decreased vigour and self-esteem whilst also possessing higher anxiety levels. These psychological issues were primarily attributed to deficiencies in hormones, including testosterone and ACTH. Both MPHD and IGHD groups, however, also displayed deficits in specific domains of cognitive function including iconic, short-term and long-term memory (Deijen et al., 1996). The effect of GH supplementation on psychological and cognitive outcomes was assessed in adult male GHD patients subjected to a 2-year GH replacement therapy regimen (Deijen et al., 1998). This study demonstrated both a time-dependent and GH-dose response effect in improving specific components of cognitive function including short-term and long-term memory abilities. Patients receiving a supraphysiological GH dose displayed a more rapid improvement in cognitive function than those receiving lower GH doses. However, both cohorts showed significant improvements after 12 months of GH treatment, improvements that were maintained for the subsequent 12 months (Deijen et al., 1998). Similar results were found by Soares et al. (1999) who showed a 6-month treatment of rhGH improved measures of attention, cognitive efficiency, comprehension and vocabulary in adults suffering from GHD. Importantly, the long-term benefits of GH replacement for GHD patients appear to be stable with a longitudinal study showing prolonged positive outcomes in health parameters and cognitive function (Arwert et al., 2005). Indeed, both short-term and long-term associative tasks remained significantly improved 10 years following initiation of GH replacement. More recently it was shown that an acute, 3-week period of GH treatment of an elderly female suffering from cognitive decline was sufficient to improve learning ability. A PET-SCAN revealed a normalisation of cerebral metabolism within the hippocampus (Devesa et al., 2018).
It should be noted that side effects from GH replacement therapy have been commonly reported and include fluid retention (Lanfranco et al., 2003), peripheral oedema and carpel tunnel syndrome (Hersch and Merriam, 2008). Accordingly, researchers altered their experimental approach to induce a more physiological release of GH via GHRH and GHRH-analogue treatments, especially in instances of adult onset GHD or physiological ageing. Initial work in the aged rat demonstrated that GHRH administration is effective at attenuating age-related deficits in spatial memory, especially during the probe portion of Morris water maze (MWM) trials (Thornton et al., 2000). In humans, treatment with GHRH and analogues typically resulted in both a lowered incidence and severity of any reported side effects compared to GH replacement therapy (Merriam et al., 2003). Examining the ability of GHRH treatment to overcome the age-dependent decrease in GH in aged individuals typically revealed improvements in specific domains of cognitive function, especially those related to areas of fluid intelligence such as comprehension and reasoning. For example, a 6-month treatment with GHRH in healthy aged participants resulted in improved cognitive domains that included problem solving and working memory (Vitiello et al., 2006). Importantly, this latter study also demonstrated comparable improvements for both males and females, highlighting the utility of such an approach in aged individuals. The clinical importance of GH levels in physiologically aged individuals is further highlighted by the fact that GH levels induced via GH secretagogues positively correlate with the above-mentioned domains of cognitive function including attention and short-term memory (Quik et al., 2012a). Focus was then extended to comparing the effect of GHRH treatment on healthy older adults versus those suffering from mild cognitive impairment (MCI). In accordance with previous work, GHRH treatment enhanced cognitive outcomes in healthy aged individuals following a 20-week trial (Baker et al., 2012). MCI patients also displayed comparable improvements in cognitive function, however, it should be noted that these individuals did not reach non-MCI levels. Similar findings were also found in aged MCI individuals following a 20-week, self-administration treatment of the GHRH analogue tesamorelin, with improvements in cognitive function reported (Friedman et al., 2013).
The success of GH therapy on cognitive function for GHD adults and the elderly encouraged research to be conducted on the potential of GH to help recovery from forms of neurotrauma including stroke, TBI and whole brain irradiation-induced injury and will be briefly summarised below.
Several experimental stroke models have been designed to test the effectiveness of neuroprotection or cognitive recovery paradigms before translation to human subjects. One of the most common of these is the induction of a hypoxic-ischaemic stroke via carotid artery ligation. Stroke severity can be increased by lengthening the time animals are exposed to hypoxic conditions following carotid artery ligation, enabling careful control of stroke severity. Initial studies by Scheepens et al. (1999) used this approach to examine the distribution of GH receptor immunoreactive cells following injury. These investigators then determined the effect of direct ICV injection of recombinant rat GH (rrGH) in adult rats following moderate to severe stroke (Scheepens et al., 2001). Interestingly, there was a time- and severity-dependent increase in GH receptor immunoreactive cells within regions of damage. These regions included the hippocampus, the cortex and the walls of the lateral ventricles where GH receptor immunoreactivity was predominantly localised to neurons. More severe stroke samples showed more intense staining earlier. Direct ICV injection of rrGH, delivered 2-h post stroke, significantly reduced neuronal cell loss in several regions of the brain, including the cortex and hippocampus. This finding shows that GH treatment is neuroprotective in regions known to express GHR (Scheepens et al., 2001). Delaying ICV delivery of GH in rats to 4-days post stroke resulted in only moderate improvements in some measures of motor function and in spatial learning as measured by the MWM, a cognitive paradigm known to rely on the hippocampus (Pathipati et al., 2009). This diminished response of outcomes following a delay in GH supplementation may indicate that GH treatment is required immediately following injury in order to minimise tissue loss and serve as a neuroprotective agent, likely through activation of endogenous NSCs. More recently, a photothrombotic occlusion stroke model in adult mice revealed that a 28-day GH treatment, achieved via sub cutaneous osmotic pump delivery, resulted in reduced neural tissue loss and significant improvement in the hippocampal-dependent paired associate learning task and motor function (Ong et al., 2018). Moreover, chronic hypoxia induced hippocampal GH mRNA and protein expression while GH administration induced EPO, VEGF, and IGF-1 (Li R. C. et al., 2011). Moving to human patients, a recent trial examined the effect of treating stroke patients suffering from cognitive deficits with rhGH for a period of 6-months (Feng et al., 2020). The rhGH group displayed significant improvements (measured by the Montreal Cognitive Assessment score) relative to placebo controls, providing the tantalising prospect of utilising GH treatment in post stroke cognitive recovery. Similarly, exercise has been demonstrated to improve cognition post stroke. We also recently demonstrated improved hippocampal outcomes after 21-days of voluntary exercise in young adult mice following an endothelin-induced stroke (Codd et al., 2020), a period of exercise shown to increase circulating GH levels in young adult mice (Blackmore et al., 2021).
With an ageing population, TBI is becoming more prevalent with falls being the leading cause of TBI in aged individuals (Thompson et al., 2006). For the purpose of this discussion, TBI, refers to the acquisition of a sudden trauma that causes damage to the brain. It is interesting to note that following TBI, many patients suffer from some form of pituitary dysfunction (Bondanelli et al., 2007; Devesa et al., 2013). Indeed, similar to aged individuals in whom successful induction of GH secretion correlates with increased cognitive function (Quik et al., 2012a), induced GH secretion levels can also predict positive outcomes and recovery following TBI (Bondanelli et al., 2007). To examine the prospective role of GH on cognitive recovery following TBI, a pilot study examined GHD TBI patients following a 12-month GH replacement therapy period and found significant improvement in several cognitive domains relative to placebo controls (High et al., 2010). These findings were replicated soon after (Reimunde et al., 2011) and a third study noted that the greatest improvements in GHD TBI patients occurred in those that had the most severe deficits prior to initiation of the trial (Moreau et al., 2013). These pre-clinical findings resulted in a recommendation by the US DOD for Phase II/III trials of GH after TBI (Diaz-Arrastia et al., 2014). Animal models examining the effect of TBI also revealed that TBI causes an induction of GHD and there was improved cognitive function following GH replacement therapy, whilst also offering some mechanistic insight as to how this occurs. Inducing a cortical concussion impact (CCI) via an impactor resulted in roughly 50% of rats displaying reduced serum GH levels (Zhang et al., 2014). A 2-week treatment period with rhGH improved spatial learning, as measured by the MWM, and increased BDNF and TrkB mRNA levels in the hippocampus (Zhang et al., 2014). BDNF has well-established roles in learning and memory functions and the recent finding that GH directly induces BDNF expression (Martinez-Moreno et al., 2018) further illustrates the pivotal role that GH plays in neural function. Recent studies have also begun to examine novel delivery approaches of GH following injury, including delivery to the spinal cord where effective delivery is considered difficult. Indeed, there is increasing evidence that nanowired delivery of GH may offer prolonged neuroprotection and reduced spinal injury compared to traditional cannula-based delivery (Muresanu et al., 2015). Moving forward, approaches such as nanowired delivery may dramatically improve outcomes following injury and is worthy of continued investigation.
Cranial radiation therapy is often used in the treatment of patients suffering from a variety of malignant diseases of the brain (Barlind et al., 2010). Unfortunately, whole brain irradiation frequently causes GHD in patients (Ogilvy-Stuart et al., 1994) and a subsequent manifestation of neurocognitive dysfunction (Quik et al., 2012b). The severity of GHD appears to be related to both the dose of radiation received (Merchant et al., 2002) and the age of the patient at time of treatment, with younger patients displaying greater cognitive deficits (Chin and Maruyama, 1984). Pharmacological induction of GH secretion using GHRH in irradiation-induced GHD patients indicates that the main contributing factor of irradiation-induced GHD is hypothalamic dysregulation rather than ablation of the pituitary gland (Ogilvy-Stuart et al., 1994; Darzy and Shalet, 2006).
Animal models have also clearly demonstrated that irradiation has severe effects on the brain with greater effects apparent in developing brains. These effects include increased rates of apoptosis, greater loss of progenitor cells and a prolonged decrease in proliferation rates within the neurogenic regions (Fukuda et al., 2005). As in humans, the severity of irradiation-induced damage in animal models is dose-dependent (Mizumatsu et al., 2003). Mechanistically, it is now well known that endogenous NSCs in both the SVZ and hippocampus are exquisitely sensitive to irradiation, especially those that are mitotically active (McGinn et al., 2008). There is an increase in apoptosis (Peissner et al., 1999) and a reduction in neurogenesis rates in both the SVZ (Hellstrom et al., 2009) and dentate gyrus (Mizumatsu et al., 2003), with the dentate gyrus appearing to be more severely affected than the SVZ long-term (McGinn et al., 2008; Hellstrom et al., 2009). The consequences of irradiation-induced neural damage culminate in cognitive impairments, including spatial learning deficits (Madsen et al., 2003; Raber et al., 2004; Rola et al., 2004). These likely contribute to the observed severity of cognitive dysfunction within younger patients (Chin and Maruyama, 1984).
We previously demonstrated that direct ICV infusion of GH resulted in long-term activation of NSCs as measured by neurosphere number (Blackmore et al., 2012b). However, irradiation of animals after GH treatment resulted in incomplete regeneration of the SVZ, similar to that observed following a double dose of irradiation. This suggests that the GH-activated NSCs are more sensitive and vulnerable to irradiation. GH treatment following irradiation however, resulted in an augmented recovery of SVZ precursor cell numbers beyond non-irradiated levels (Blackmore et al., 2012b), indicating that GH can be effectively utilised in neural recovery following irradiation treatment. Similarly, Barlind et al. (2010) found that irradiated mice treated with the GH secretagogue hexarelin demonstrated an augmented hippocampal neurogenesis response.
Concerns regarding GH treatment in relation to the potential re-occurrence of malignant tumours has likely limited research in the use of GH treatment following irradiation therapy. Alternatives have therefore been investigated to aid in neural recovery following irradiation-induced damage, including physical exercise. Multiple groups have demonstrated that voluntary exercise has augmented neural recovery following irradiation-induced damage including increased neural precursor cell proliferation (Blackmore et al., 2012b), elevated neurogenesis levels (Clark et al., 2008; Naylor et al., 2008; Wong-Goodrich et al., 2010) and improved spatial learning (Wong-Goodrich et al., 2010). Considering the established role that physical exercise has in elevating GH levels, it may be an attractive alternative to GH replacement following irradiation induced damage in human subjects.
The known roles of GH have rapidly evolved. It is now clear that GH plays a critical function in the brain. Through the use of animal models, it has been possible to interrogate the complex mechanisms involved in GH-mediated changes within the brain. This includes brain development where GH aids in the proliferation of NSCs. In the adult brain, exogenous GH can activate otherwise quiescent endogenous NSCs, giving rise to increased neuron number within key regions of the brain, particularly the hippocampus. Even in ageing and following injury, GH supplementation has now been clearly demonstrated to improve cognitive outcomes. These steps and the contribution that GH plays in neural function is summarised in Figure 1. Identifying changes in the regulation of GH release allows for refinement in treatment strategies for those suffering from GHD, be it genetic or due to ageing or injury. Ultimately, the knowledge gained from these studies will allow for the tailoring of strategies to minimise side-effects and maximise treatment outcomes. With a worldwide ageing population and a concomitant increase in age-related cognitive deficits, continued research in this field is essential in order to minimise the impact for people now and in the immediate future.
Figure 1. Schematics summarising the neural effects due to GHD and following GH replacement therapy in mice. GHD due to ageing or brain injury can result in hypothalamic dysfunction where GHRH is decreased and somatostatin, including somatostatin-28 (SRIF-28) is increased. This results in a decrease in GH secretion from the pituitary gland. Downstream of this reduction is a decrease in endogenous neural precursor cell activation, a decrease in neurogenesis and deficits in cognitive function, including spatial learning. Increasing GH levels either through physical exercise or GH therapy increases neural precursor cell proliferation, increases neurogenesis and improves cognitive function.
Both authors listed have made a substantial, direct, and intellectual contribution to the work, and approved it for publication.
The figure was generated with BioRender.com.
The authors declare that the research was conducted in the absence of any commercial or financial relationships that could be construed as a potential conflict of interest.
All claims expressed in this article are solely those of the authors and do not necessarily represent those of their affiliated organizations, or those of the publisher, the editors and the reviewers. Any product that may be evaluated in this article, or claim that may be made by its manufacturer, is not guaranteed or endorsed by the publisher.
Aberg, D. (2010). Role of the growth hormone/insulin-like growth factor 1 axis in neurogenesis. Endocr. Dev. 17, 63–76. doi: 10.1159/000262529
Aberg, D. N., Lind, J., Isgaard, J., and Georg Kuhn, H. (2010). Peripheral growth hormone induces cell proliferation in the intact adult rat brain. Growth Horm. IGF Res. 20, 264–269. doi: 10.1016/j.ghir.2009.12.003
Aberg, N. D., Johansson, I., Aberg, M. A., Lind, J., Johansson, U. E., Cooper-Kuhn, C. M., et al. (2009). Peripheral administration of GH induces cell proliferation in the brain of adult hypophysectomized rats. J. Endocrinol. 201, 141–150.
Ajo, R., Cacicedo, L., Navarro, C., and Sanchez-Franco, F. (2003). Growth hormone action on proliferation and differentiation of cerebral cortical cells from fetal rat. Endocrinology 144, 1086–1097.
Aleman, A., Verhaar, H. J., De Haan, E. H., De Vries, W. R., Samson, M. M., Drent, M. L., et al. (1999). Insulin-like growth factor-I and cognitive function in healthy older men. J. Clin. Endocrinol. Metab. 84, 471–475.
Andersson, K., Fuxe, K., Eneroth, P., Gustafsson, J. A., and Skett, P. (1977). Catecholamine control of growth-hormone regulation—evidence for discrete changes in dopamine and noradrenaline turnover following growth-hormone administration. Neurosci. Lett. 5, 83–89.
Andersson, K., Fuxe, K., Eneroth, P., Isaksson, O., Nyberg, F., and Roos, P. (1983). Rat growth hormone and hypothalamic catecholamine nerve terminal systems. Evidence for rapid and discrete reductions in dopamine and noradrenaline levels and turnover in the median eminence of the hypophysectomized male rat. Eur. J. Pharmacol. 95, 271–275.
Arwert, L. I., Deijen, J. B., Muller, M., and Drent, M. L. (2005). Long-term growth hormone treatment preserves GH-induced memory and mood improvements: A 10-year follow-up study in GH-deficient adult men. Horm. Behav. 47, 343–349. doi: 10.1016/j.yhbeh.2004.11.015
Azcoitia, I., Perez-Martin, M., Salazar, V., Castillo, C., Ariznavarreta, C., Garcia-Segura, L. M., et al. (2005). Growth hormone prevents neuronal loss in the aged rat hippocampus. Neurobiol. Aging 26, 697–703.
Baker, L. D., Barsness, S. M., Borson, S., Merriam, G. R., Friedman, S. D., Craft, S., et al. (2012). Effects of growth hormone-releasing hormone on cognitive function in adults with mild cognitive impairment and healthy older adults: Results of a controlled trial. Arch. Neurol. 69, 1420–1429. doi: 10.1001/archneurol.2012.1970
Barlind, A., Karlsson, N., Aberg, N. D., Bjork-Eriksson, T., Blomgren, K., and Isgaard, J. (2010). The growth hormone secretagogue hexarelin increases cell proliferation in neurogenic regions of the mouse hippocampus. Growth Horm. IGF Res. 20, 49–54. doi: 10.1016/j.ghir.2009.09.001
Bigbee, A. J., Gosselink, K. L., Roy, R. R., Grindeland, R. E., and Edgerton, V. R. (2000). Bioassayable growth hormone release in rats in response to a single bout of treadmill exercise. J. Appl. Physiol. 89, 2174–2178. doi: 10.1152/jappl.2000.89.6.2174
Blackmore, D. G., Golmohammadi, M. G., Large, B., Waters, M. J., and Rietze, R. L. (2009). Exercise increases neural stem cell number in a growth hormone-dependent manner, augmenting the regenerative response in aged mice. Stem Cells 27, 2044–2052. doi: 10.1002/stem.120
Blackmore, D. G., Reynolds, B. A., Golmohammadi, M. G., Large, B., Aguilar, R. M., Haro, L. S., et al. (2012a). Growth hormone responsive neural precursor cells reside within the adult mammalian brain. Sci. Rep. 2:250. doi: 10.1038/srep00250
Blackmore, D. G., Steyn, F. J., Carlisle, A., O’Keeffe, I., Vien, K. Y., Zhou, X., et al. (2021). An exercise “sweet spot” reverses cognitive deficits of aging by growth-hormone-induced neurogenesis. iScience 24:103275. doi: 10.1016/j.isci.2021.103275
Blackmore, D. G., Vukovic, J., Waters, M. J., and Bartlett, P. F. (2012b). GH mediates exercise-dependent activation of SVZ neural precursor cells in aged mice. PLoS One 7:e49912. doi: 10.1371/journal.pone.0049912
Bondanelli, M., Ambrosio, M. R., Cavazzini, L., Bertocchi, A., Zatelli, M. C., Carli, A., et al. (2007). Anterior pituitary function may predict functional and cognitive outcome in patients with traumatic brain injury undergoing rehabilitation. J. Neurotrauma 24, 1687–1697. doi: 10.1089/neu.2007.0343
Burman, P., Hetta, J., Wide, L., Mansson, J. E., Ekman, R., and Karlsson, F. A. (1996). Growth hormone treatment affects brain neurotransmitters and thyroxine [see comment]. Clin. Endocrinol. 44, 319–324.
Castro, J. R., Costoya, J. A., Gallego, R., Prieto, A., Arce, V. M., and Senaris, R. (2000). Expression of growth hormone receptor in the human brain. Neurosci. Lett. 281, 147–150.
Chin, H. W., and Maruyama, Y. (1984). Age at treatment and long-term performance results in medulloblastoma. Cancer 53, 1952–1958.
Christophidis, L. J., Gorba, T., Gustavsson, M., Williams, C. E., Werther, G. A., Russo, V. C., et al. (2009). Growth hormone receptor immunoreactivity is increased in the subventricular zone of juvenile rat brain after focal ischemia: A potential role for growth hormone in injury-induced neurogenesis. Growth Horm. IGF Res. 19, 497–506. doi: 10.1016/j.ghir.2009.05.001
Clark, P. J., Brzezinska, W. J., Thomas, M. W., Ryzhenko, N. A., Toshkov, S. A., and Rhodes, J. S. (2008). Intact neurogenesis is required for benefits of exercise on spatial memory but not motor performance or contextual fear conditioning in C57BL/6J mice. Neuroscience 155, 1048–1058.
Codd, L. N., Blackmore, D. G., Vukovic, J., and Bartlett, P. F. (2020). Exercise reverses learning deficits induced by hippocampal injury by promoting neurogenesis. Sci. Rep. 10:19269. doi: 10.1038/s41598-020-76176-1
Console, G. M., Gomez Dumm, C. L., and Goya, R. G. (1993). Impact of aging on the morphology and function of the somatotroph cell population in rats. Mech. Ageing Dev. 70, 45–51.
Cotman, C. W., and Berchtold, N. C. (2002). Exercise: A behavioral intervention to enhance brain health and plasticity. Trends Neurosci. 25, 295–301. doi: 10.1016/s0166-2236(02)02143-4
Craig, B. W., Brown, R., and Everhart, J. (1989a). Effects of progressive resistance training on growth hormone and testosterone levels in young and elderly subjects. Mech. Ageing Dev. 49, 159–169. doi: 10.1016/0047-6374(89)90099-7
Craig, B. W., Everhart, J., and Brown, R. (1989b). The influence of high-resistance training on glucose tolerance in young and elderly subjects. Mech. Ageing Dev. 49, 147–157. doi: 10.1016/0047-6374(89)90098-5
Darzy, K. H., and Shalet, S. M. (2006). Pathophysiology of radiation-induced growth hormone deficiency: Efficacy and safety of GH replacement. Growth Horm. IGF Res. 16, S30–S40. doi: 10.1016/j.ghir.2006.03.002
DeGennaro Colonna, V., Zoli, M., Cocchi, D., Maggi, A., Marrama, P., Agnati, L. F., et al. (1989). Reduced growth hormone releasing factor (GHRF)-like immunoreactivity and GHRF gene expression in the hypothalamus of aged rats. Peptides 10, 705–708. doi: 10.1016/0196-9781(89)90164-2
Deijen, J., de Boer, H., and van der Veen, E. (1998). Cognitive changes during growth hormone replacement in adult men. Psychoneuroendocrinology 23, 45–55.
Deijen, J., de Boer, H., Blok, G., and van der Veen, E. (1996). Cognitive impairments and mood disturbances in growth hormone deficient men. Psychoneuroendocrinology 21, 313–322. doi: 10.1016/0306-4530(95)00050-x
Deleyrolle, L. P., Ericksson, G., Morrison, B. J., Lopez, J. A., Burrage, K., Burrage, P., et al. (2011). Determination of somatic and cancer stem cell self-renewing symmetric division rate using sphere assays. PLoS One 6:e15844. doi: 10.1371/journal.pone.0015844
Devesa, J., Nunez, I., Agra, C., Bejarano, A., and Devesa, P. (2018). Treatment with growth hormone (GH) increased the metabolic activity of the brain in an elder patient, not GH-deficient, who suffered mild cognitive alterations and had an ApoE 4/3 genotype. Int. J. Mol. Sci. 19:2294. doi: 10.3390/ijms19082294
Devesa, J., Reimunde, P., Devesa, P., Barbera, M., and Arce, V. (2013). Growth hormone (GH) and brain trauma. Horm. Behav. 63, 331–344.
Devesa, P., Agasse, F., Xapelli, S., Almenglo, C., Devesa, J., Malva, J. O., et al. (2014). Growth hormone pathways signaling for cell proliferation and survival in hippocampal neural precursors from postnatal mice. BMC Neurosci. 15:100. doi: 10.1186/1471-2202-15-100
Diaz-Arrastia, R., Kochanek, P. M., Bergold, P., Kenney, K., Marx, C. E., Grimes, C. J., et al. (2014). Pharmacotherapy of traumatic brain injury: State of the science and the road forward: Report of the department of defense neurotrauma pharmacology workgroup. J. Neurotrauma 31, 135–158.
Donahue, C. P., Kosik, K. S., and Shors, T. J. (2006). Growth hormone is produced within the hippocampus where it responds to age, sex, and stress. Proc. Natl. Acad. Sci. U.S.A. 103, 6031–6036. doi: 10.1073/pnas.0507776103
Enwere, E., Shingo, T., Gregg, C., Fujikawa, H., Ohta, S., and Weiss, S. (2004). Aging results in reduced epidermal growth factor receptor signaling, diminished olfactory neurogenesis, and deficits in fine olfactory discrimination. J. Neurosci. 24, 8354–8365. doi: 10.1523/JNEUROSCI.2751-04.2004
Eriksson, P. S., Perfilieva, E., Bjork-Eriksson, T., Alborn, A. M., Nordborg, C., Peterson, D. A., et al. (1998). Neurogenesis in the adult human hippocampus. Nat. Med. 4, 1313–1317.
Evans, H. M., and Long, J. A. (1922). Characteristic effects upon growth, oestrus and ovulation induced by the intraperitoneal administration of fresh anterior hypophyseal substance. Proc. Natl. Acad. Sci. U.S.A. 8, 38–39. doi: 10.1073/pnas.8.3.38
Fahy, G. M., Brooke, R. T., Watson, J. P., Good, Z., Vasanawala, S. S., Maecker, H., et al. (2019). Reversal of epigenetic aging and immunosenescent trends in humans. Aging Cell 18:e13028.
Felsing, N. E., Brasel, J. A., and Cooper, D. M. (1992). Effect of low and high intensity exercise on circulating growth hormone in men. J. Clin. Endocrinol. Metab. 75, 157–162.
Feng, X., Li, G., Wu, W., Xu, Y., Lin, H., and Fan, J. (2020). Recombinant human growth hormone ameliorates cognitive impairment in stroke patients. J. Comput. Assist. Tomogr. 44, 255–261.
Friedman, S. D., Baker, L. D., Borson, S., Jensen, J. E., Barsness, S. M., Craft, S., et al. (2013). Growth hormone-releasing hormone effects on brain gamma-aminobutyric acid levels in mild cognitive impairment and healthy aging. JAMA Neurol. 70, 883–890. doi: 10.1001/jamaneurol.2013.1425
Fujikawa, T., Soya, H., Fukuoka, H., Alam, K. S., Yoshizato, H., Mcewen, B. S., et al. (2000). A biphasic regulation of receptor mRNA expressions for growth hormone, glucocorticoid and mineralocorticoid in the rat dentate gyrus during acute stress. Brain Res. 874, 186–193.
Fukuda, A., Fukuda, H., Swanpalmer, J., Hertzman, S., Lannering, B., Marky, I., et al. (2005). Age-dependent sensitivity of the developing brain to irradiation is correlated with the number and vulnerability of progenitor cells. J. Neurochem. 92, 569–584. doi: 10.1111/j.1471-4159.2004.02894.x
Gallagher, M., and Pelleymounter, M. A. (1988). Spatial learning deficits in old rats: A model for memory decline in the aged. Neurobiol. Aging 9, 549–556. doi: 10.1016/s0197-4580(88)80112-x
Garcia, J. M., Merriam, G. R., and Kargi, A. Y. (2019). “Growth hormone in aging,” in Endotext, eds K. R. Feingold, B. Anawalt, M. R. Blackman, A. Boyce, G. Chrousos, E. Corpas, et al. (South Dartmouth, MA: MDText.com, Inc).
Giannoulis, M. G., Boroujerdi, M. A., Powrie, J., Dall, R., Napoli, R., Ehrnborg, C., et al. (2005). Gender differences in growth hormone response to exercise before and after rhGH administration and the effect of rhGH on the hormone profile of fit normal adults. Clin. Endocrinol. 62, 315–322. doi: 10.1111/j.1365-2265.2005.02216.x
Giustina, A., and Veldhuis, J. D. (1998). Pathophysiology of the neuroregulation of growth hormone secretion in experimental animals and the human. Endocr. Rev. 19, 717–797. doi: 10.1210/edrv.19.6.0353
Graf, L., and Li, C. H. (1974). On the primary structure of pituitary bovine growth hormone. Biochem. Biophys. Res. Commun. 56, 168–176.
Hagberg, J. M., Seals, D. R., Yerg, J. E., Gavin, J., Gingerich, R., Premachandra, B., et al. (1988). Metabolic responses to exercise in young and older athletes and sedentary men. J. Appl. Physiol. 65, 900–908. doi: 10.1152/jappl.1988.65.2.900
Harvey, S., Lavelin, I., and Pines, M. (2002). Growth hormone (GH) action in the brain: Neural expression of a GH-response gene. J. Mol. Neurosci. 18, 89–95. doi: 10.1385/JMN:18:1-2:89
Hellstrom, N. A., Bjork-Eriksson, T., Blomgren, K., and Kuhn, H. G. (2009). Differential recovery of neural stem cells in the subventricular zone and dentate gyrus after ionizing radiation. Stem Cells 27, 634–641. doi: 10.1634/stemcells.2008-0732
Hersch, E. C., and Merriam, G. R. (2008). Growth hormone (GH)-releasing hormone and GH secretagogues in normal aging: Fountain of youth or pool of tantalus? Clin. Interv. Aging 3, 121–129.
High, W. M. Jr., Briones-Galang, M., Clark, J. A., Gilkison, C., Mossberg, K. A., Zgaljardic, D. J., et al. (2010). Effect of growth hormone replacement therapy on cognition after traumatic brain injury. J. Neurotrauma 27, 1565–1575.
Hindmarsh, P. C., Brain, C. E., Robinson, I. C., Matthews, D. R., and Brook, C. G. (1991). The interaction of growth hormone releasing hormone and somatostatin in the generation of a GH pulse in man. Clin. Endocrinol. 35, 353–360.
Ho, K. Y., Evans, W. S., Blizzard, R. M., Veldhuis, J. D., Merriam, G. R., Samojlik, E., et al. (1987). Effects of sex and age on the 24-hour profile of growth hormone secretion in man: Importance of endogenous estradiol concentrations. J. Clin. Endocrinol. Metab. 64, 51–58. doi: 10.1210/jcem-64-1-51
Holt, R. I., Webb, E., Pentecost, C., and Sonksen, P. H. (2001). Aging and physical fitness are more important than obesity in determining exercise-induced generation of GH. J. Clin. Endocrinol. Metab. 86, 5715–5720. doi: 10.1210/jcem.86.12.8092
Jhaveri, D. J., O’Keeffe, I., Robinson, G. J., Zhao, Q. Y., Zhang, Z. H., Nink, V., et al. (2015). Purification of neural precursor cells reveals the presence of distinct, stimulus-specific subpopulations of quiescent precursors in the adult mouse hippocampus. J. Neurosci. 35, 8132–8144. doi: 10.1523/JNEUROSCI.0504-15.2015
Johansson, J. O., Larson, G., Andersson, M., Elmgren, A., Hynsjo, L., Lindahl, A., et al. (1995). Treatment of growth hormone-deficient adults with recombinant human growth hormone increases the concentration of growth hormone in the cerebrospinal fluid and affects neurotransmitters. Neuroendocrinology 61, 57–66. doi: 10.1159/000126813
Johnson, G. E., and Everett, D. S. (1929). The effects of daily injections of bovine anterior pituitary extract upon the developing albino rat. Physiol. Zool. 2, 285–301.
Kanaley, J. A., Weatherup-Dentes, M. M., Jaynes, E. B., and Hartman, M. L. (1999). Obesity attenuates the growth hormone response to exercise. J. Clin. Endocrinol. Metab. 84, 3156–3161.
Kohman, R. A., Deyoung, E. K., Bhattacharya, T. K., Peterson, L. N., and Rhodes, J. S. (2012). Wheel running attenuates microglia proliferation and increases expression of a proneurogenic phenotype in the hippocampus of aged mice. Brain Behav. Immun. 26, 803–810. doi: 10.1016/j.bbi.2011.10.006
Lai, Z., Roos, P., Zhai, O., Olsson, Y., Fholenhag, K., Larsson, C., et al. (1993). Age-related reduction of human growth hormone-binding sites in the human brain. Brain Res. 621, 260–266.
Lanfranco, F., Gianotti, L., Giordano, R., Pellegrino, M., Maccario, M., and Arvat, E. (2003). Ageing, growth hormone and physical performance. J. Endocrinol. Invest. 26, 861–872.
Le Grevès, M., Zhou, Q., Berg, M., Le Grevès, P., Fhölenhag, K., Meyerson, B., et al. (2006). Growth hormone replacement in hypophysectomized rats affects spatial performance and hippocampal levels of NMDA receptor subunit and PSD-95 gene transcript levels. Exp. Brain Res. 173, 267–273. doi: 10.1007/s00221-006-0438-2
Leiter, O., Zhuo, Z., Rust, R., Wasielewska, J. M., Gronnert, L., Kowal, S., et al. (2022). Selenium mediates exercise-induced adult neurogenesis and reverses learning deficits induced by hippocampal injury and aging. Cell Metab. 34, 408–423.e8. doi: 10.1016/j.cmet.2022.01.005
Li, C. H., and Papkoff, H. (1956). Preparation and properties of growth hormone from human and monkey pituitary glands. Science 124, 1293–1294.
Li, C. H., Dixon, J. S., and Liu, W. K. (1969). Human pituitary growth hormone. 19. Primary structure of hormone. Arch. Biochem. Biophy. 133, 70–91.
Li, C. H., Liu, W. K., and Dixon, J. S. (1966). Human pituitary growth hormone. 12. Amino acid sequence of Hormone. J. Am. Chem. Soc. 88:2050.
Li, E., Kim, D., Cai, M., Lee, S., Kim, Y., Lim, E., et al. (2011). Hippocampus-dependent spatial learning and memory are impaired in growth hormone-deficient spontaneous dwarf rats. Endocr. J. 58, 257–267. doi: 10.1507/endocrj.k11e-006
Li, R. C., Guo, S. Z., Raccurt, M., Moudilou, E., Morel, G., Brittian, K. R., et al. (2011). Exogenous growth hormone attenuates cognitive deficits induced by intermittent hypoxia in rats. Neuroscience 196, 237–250. doi: 10.1016/j.neuroscience.2011.08.029
Lisman, J., Buzsaki, G., Eichenbaum, H., Nadel, L., Ranganath, C., and Redish, A. D. (2017). Viewpoints: How the hippocampus contributes to memory, navigation and cognition. Nat. Neurosci. 20, 1434–1447.
Lobie, P. E., Garcia-Aragon, J., Lincoln, D. T., Barnard, R., Wilcox, J. N., and Waters, M. J. (1993). Localization and ontogeny of growth hormone receptor gene expression in the central nervous system. Brain Res. Dev. Brain Res. 74, 225–233.
Lois, C., and Alvarez-Buylla, A. (1994). Long-distance neuronal migration in the adult mammalian brain. Science 264, 1145–1148.
Madsen, T. M., Kristjansen, P. E., Bolwig, T. G., and Wortwein, G. (2003). Arrested neuronal proliferation and impaired hippocampal function following fractionated brain irradiation in the adult rat. Neuroscience 119, 635–642.
Marcell, T. J., Wiswell, R. A., Hawkins, S. A., and Tarpenning, K. M. (1999). Age-related blunting of growth hormone secretion during exercise may not be soley due to increased somatostatin tone. Metabolism 48, 665–670.
Martinez-Moreno, C. G., Fleming, T., Carranza, M., Avila-Mendoza, J., Luna, M., Harvey, S., et al. (2018). Growth hormone protects against kainate excitotoxicity and induces BDNF and NT3 expression in chicken neuroretinal cells. Exp. Eye Res. 166, 1–12. doi: 10.1016/j.exer.2017.10.005
McGinn, M. J., Sun, D., and Colello, R. J. (2008). Utilizing X-irradiation to selectively eliminate neural stem/progenitor cells from neurogenic regions of the mammalian brain. J. Neurosci. Methods 170, 9–15. doi: 10.1016/j.jneumeth.2007.12.012
McLenachan, S., Lum, M. G., Waters, M. J., and Turnley, A. M. (2009). Growth hormone promotes proliferation of adult neurosphere cultures. Growth Horm. IGF Res. 19, 212–218. doi: 10.1016/j.ghir.2008.09.003
Merchant, T. E., Goloubeva, O., Pritchard, D. L., Gaber, M. W., Xiong, X., Danish, R. K., et al. (2002). Radiation dose-volume effects on growth hormone secretion. Int. J. Radiat. Oncol. Biol. Phys. 52, 1264–1270.
Merriam, G. R., Schwartz, R. S., and Vitiello, M. V. (2003). Growth hormone-releasing hormone and growth hormone secretagogues in normal aging. Endocrine 22, 41–48.
Meyers, C. A., Murphy, W. A., Redding, T. W., Coy, D. H., and Schally, A. V. (1980). Synthesis and biological actions of prosomatostatin. Proc. Natl. Acad. Sci. U.S.A. 77, 6171–6174.
Mizumatsu, S., Monje, M. L., Morhardt, D. R., Rola, R., Palmer, T. D., and Fike, J. R. (2003). Extreme sensitivity of adult neurogenesis to low doses of X-irradiation. Cancer Res. 63, 4021–4027.
Moreau, O. K., Cortet-Rudelli, C., Yollin, E., Merlen, E., Daveluy, W., and Rousseaux, M. (2013). Growth hormone replacement therapy in patients with traumatic brain injury. J. Neurotrauma 30, 998–1006.
Muller, E. E. (1997). Cholinergic function and neural control of GH secretion. A critical re-appraisal. Eur. J. Endocrinol. 137, 338–342. doi: 10.1530/eje.0.1370338
Muller, E. E., Locatelli, V., and Cocchi, D. (1999). Neuroendocrine control of growth hormone secretion. Physiol. Rev. 79, 511–607.
Muresanu, D. F., Sharma, A., Lafuente, J. V., Patnaik, R., Tian, Z. R., Nyberg, F., et al. (2015). Nanowired delivery of growth hormone attenuates pathophysiology of spinal cord injury and enhances insulin-like growth factor-1 concentration in the plasma and the spinal cord. Mol. Neurobiol. 52, 837–845. doi: 10.1007/s12035-015-9298-8
Naylor, A. S., Bull, C., Nilsson, M. K., Zhu, C., Bjork-Eriksson, T., Eriksson, P. S., et al. (2008). Voluntary running rescues adult hippocampal neurogenesis after irradiation of the young mouse brain. Proc. Natl. Acad. Sci. U.S.A. 105, 14632–14637. doi: 10.1073/pnas.0711128105
Obermayr, R. P., Mayerhofer, L., Knechtelsdorfer, M., Mersich, N., Huber, E. R., Geyer, G., et al. (2005). The age-related down-regulation of the growth hormone/insulin-like growth factor-1 axis in the elderly male is reversed considerably by donepezil, a drug for Alzheimer’s disease. Exp. Gerontol. 40, 157–163. doi: 10.1016/j.exger.2004.11.001
Ogilvy-Stuart, A. L., Wallace, W. H., and Shalet, S. M. (1994). Radiation and neuroregulatory control of growth hormone secretion. Clin. Endocrinol. 41, 163–168. doi: 10.1111/j.1365-2265.1994.tb02525.x
Ong, L. K., Chow, W. Z., Tebay, C., Kluge, M., Pietrogrande, G., Zalewska, K., et al. (2018). Growth hormone improves cognitive function after experimental stroke. Stroke 49, 1257–1266.
Overall, R. W., Walker, T. L., Leiter, O., Lenke, S., Ruhwald, S., and Kempermann, G. (2013). Delayed and transient increase of adult hippocampal neurogenesis by physical exercise in DBA/2 mice. PLoS One 8:e83797. doi: 10.1371/journal.pone.0083797
Pan, W., Yu, Y., Cain, C. M., Nyberg, F., Couraud, P. O., and Kastin, A. J. (2005). Permeation of growth hormone across the blood-brain barrier. Endocrinology 146, 4898–4904.
Patel, Y. C., Wheatley, T., and Ning, C. (1981). Multiple forms of immunoreactive somatostatin: Comparison of distribution in neural and nonneural tissues and portal plasma of the rat. Endocrinology 109, 1943–1949. doi: 10.1210/endo-109-6-1943
Pathipati, P., Gorba, T., Scheepens, A., Goffin, V., Sun, Y., and Fraser, M. (2011). Growth hormone and prolactin regulate human neural stem cell regenerative activity. Neuroscience 190, 409–427. doi: 10.1016/j.neuroscience.2011.05.029
Pathipati, P., Surus, A., Williams, C. E., and Scheepens, A. (2009). Delayed and chronic treatment with growth hormone after endothelin-induced stroke in the adult rat. Behav. Brain Res. 204, 93–101. doi: 10.1016/j.bbr.2009.05.023
Peissner, W., Kocher, M., Treuer, H., and Gillardon, F. (1999). Ionizing radiation-induced apoptosis of proliferating stem cells in the dentate gyrus of the adult rat hippocampus. Brain Res. Mol. Brain Res. 71, 61–68.
Pombo, M., Pombo, C. M., Garcia, A., Caminos, E., Gualillo, O., Alvarez, C. V., et al. (2001). Hormonal control of growth hormone secretion. Horm. Res. 55, 11–16.
Pritzlaff, C. J., Wideman, L., Weltman, J. Y., Abbott, R. D., Gutgesell, M. E., Hartman, M. L., et al. (1999). Impact of acute exercise intensity on pulsatile growth hormone release in men. J. Appl. Physiol. 87, 498–504. doi: 10.1152/jappl.1999.87.2.498
Pritzlaff-Roy, C. J., Widemen, L., Weltman, J. Y., Abbott, R., Gutgesell, M., Hartman, M. L., et al. (2002). Gender governs the relationship between exercise intensity and growth hormone release in young adults. J. Appl. Physiol. 92, 2053–2060. doi: 10.1152/japplphysiol.01018.2001
Pyka, G., Wiswell, R. A., and Marcus, R. (1992). Age-dependent effect of resistance exercise on growth hormone secretion in people. J. Clin. Endocrinol. Metab. 75, 404–407. doi: 10.1210/jcem.75.2.1639942
Quik, E. H., Conemans, E. B., Valk, G. D., Kenemans, J. L., Koppeschaar, H. P., and van Dam, P. (2012a). Cognitive performance in older males is associated with growth hormone secretion. Neurobiol. Aging 33, 582–587.
Quik, E. H., Valk, G. D., Drent, M. L., Stalpers, L. J., Kenemans, J. L., and Koppeschaar, H. P. (2012b). Reduced growth hormone secretion after cranial irradiation contributes to neurocognitive dysfunction. Growth Horm. IGF Res. 22, 42–47. doi: 10.1016/j.ghir.2011.12.007
Raben, M. S. (1958). Treatment of a pituitary dwarf with human growth hormone. J. Clin. Endocrinol. Metab. 18, 901–903.
Raber, J., Rola, R., Lefevour, A., Morhardt, D., Curley, J., Mizumatsu, S., et al. (2004). Radiation-induced cognitive impairments are associated with changes in indicators of hippocampal neurogenesis. Radiat. Res. 162, 39–47.
Ramesh, R., Kuenzel, W. J., Buntin, J. D., and Proudman, J. A. (2000). I dentification of growth-hormone- and prolactin-containing neurons within the avian brain. Cell Tissue Res. 299, 371–383.
Ramis, M., Sarubbo, F., Sola, J., Aparicio, S., Garau, C., Miralles, A., et al. (2013). Cognitive improvement by acute growth hormone is mediated by NMDA and AMPA receptors and MEK pathway. Prog. Neuropsychopharmacol. Biol. Psychiatry 45, 11–20. doi: 10.1016/j.pnpbp.2013.04.005
Reimunde, P., Quintana, A., Castanon, B., Casteleiro, N., Vilarnovo, Z., Otero, A., et al. (2011). Effects of growth hormone (GH) replacement and cognitive rehabilitation in patients with cognitive disorders after traumatic brain injury. Brain Injury 25, 65–73.
Reynolds, B. A., and Rietze, R. L. (2005). Neural stem cells and neurospheres–re-evaluating the relationship. Nat. Methods 2, 333–336. doi: 10.1038/nmeth758
Roelfsema, F., and Veldhuis, J. D. (2016). Growth hormone dynamics in healthy adults are related to age and sex and strongly dependent on body mass index. Neuroendocrinology 103, 335–344. doi: 10.1159/000438904
Rola, R., Raber, J., Rizk, A., Otsuka, S., Vandenberg, S. R., Morhardt, D. R., et al. (2004). Radiation-induced impairment of hippocampal neurogenesis is associated with cognitive deficits in young mice. Exp. Neurol. 188, 316–330.
Sattler, F. R. (2013). Growth hormone in the aging male. Best Pract. Res. Clin. Endocrinol. Metab. 27, 541–555.
Scheepens, A., Sirimanne, E. S., Breier, B. H., Clark, R. G., Gluckman, P. D., and Williams, C. E. (2001). Growth hormone as a neuronal rescue factor during recovery from CNS injury. Neuroscience 104, 677–687. doi: 10.1016/s0306-4522(01)00109-9
Scheepens, A., Sirimanne, E., Beilharz, E., Breier, B. H., Waters, M. J., Gluckman, P. D., et al. (1999). Alterations in the neural growth hormone axis following hypoxic-ischemic brain injury. Brain Res. Molec. Brain Res. 68, 88–100. doi: 10.1016/s0169-328x(99)00051-0
Soares, C. N., Musolino, N. R., Cunha Neto, M., Caires, M. A., Rosenthal, M. C., Camargo, C. P., et al. (1999). Impact of recombinant human growth hormone (RH-GH) treatment on psychiatric, neuropsychological and clinical profiles of GH deficient adults. A placebo-controlled trial. Arq. Neuropsiquiatr. 57, 182–189. doi: 10.1590/s0004-282x1999000200003
Sonntag, W. E., Forman, L. J., Miki, N., Steger, R. W., Ramos, T., Arimura, A., et al. (1981). Effects of CNS active drugs and somatostatin antiserum on growth hormone release in young and old male rats. Neuroendocrinology 33, 73–78. doi: 10.1159/000123205
Sonntag, W. E., Gottschall, P. E., and Meites, J. (1986). Increased secretion of somatostatin-28 from hypothalamic neurons of aged rats in vitro. Brain Res. 380, 229–234. doi: 10.1016/0006-8993(86)90217-9
Sonntag, W. E., Hylka, V. W., and Meites, J. (1983). Impaired ability of old male rats to secrete growth hormone in vivo but not in vitro in response to hpGRF(1-44). Endocrinology 113, 2305–2307. doi: 10.1210/endo-113-6-2305
Sonntag, W. E., Ramsey, M., and Carter, C. S. (2005). Growth hormone and insulin-like growth factor-1 (IGF-1) and their influence on cognitive aging. Ageing Res. Rev. 4, 195–212.
Sun, L. Y., Al-Regaiey, K., Masternak, M. M., Wang, J., and Bartke, A. (2005). Local expression of GH and IGF-1 in the hippocampus of GH-deficient long-lived mice. Neurobiol. Aging 26, 929–937. doi: 10.1016/j.neurobiolaging.2004.07.010
Sutton, J., and Lazarus, L. (1976). Growth hormone in exercise: Comparison of physiological and pharmacological stimuli. J. Appp. Physiol. 41, 523–527.
Tannenbaum, G. S., and Martin, J. B. (1976). Evidence for an endogenous ultradian rhythm governing growth hormone secretion in the rat. Endocrinology 98, 562–570. doi: 10.1210/endo-98-3-562
Tannenbaum, G. S., Ling, N., and Brazeau, P. (1982). Somatostatin-28 is longer acting and more selective than somatostatin-14 on pituitary and pancreatic hormone release. Endocrinology 111, 101–107. doi: 10.1210/endo-111-1-101
Thompson, H. J., Mccormick, W. C., and Kagan, S. H. (2006). Traumatic brain injury in older adults: Epidemiology, outcomes, and future implications. J. Am. Geriatr. Soc. 54, 1590–1595.
Thompson, D. L., Weltman, J. Y., Rogol, A. D., Metzger, D. L., Veldhuis, J. D., and Weltman, A. (1993). Cholinergic and opioid involvement in release of growth hormone during exercise and recovery. J. Appl. Physiol. 75, 870–878. doi: 10.1152/jappl.1993.75.2.870
Thornton, P. L., Ingram, R. L., and Sonntag, W. E. (2000). Chronic [D-Ala2]-growth hormone-releasing hormone administration attenuates age-related deficits in spatial memory. J. Gerontol. 55, B106–B112. doi: 10.1093/gerona/55.2.b106
van Praag, H., Christie, B. R., Sejnowski, T. J., and Gage, F. H. (1999a). Running enhances neurogenesis, learning, and long-term potentiation in mice. Proc. Natl. Acad. Sci. U.S.A. 96, 13427–13431.
van Praag, H., Kempermann, G., and Gage, F. H. (1999b). Running increases cell proliferation and neurogenesis in the adult mouse dentate gyrus. Nat. Neurosci. 2, 266–270.
van Praag, H., Shubert, T., Zhao, C., and Gage, F. H. (2005). Exercise enhances learning and hippocampal neurogenesis in aged mice. J. Neurosci. 25, 8680–8685.
Vitiello, M. V., Moe, K. E., Merriam, G. R., Mazzoni, G., Buchner, D. H., and Schwartz, R. S. (2006). Growth hormone releasing hormone improves the cognition of healthy older adults. Neurobiol. Aging 27, 318–323. doi: 10.1016/j.neurobiolaging.2005.01.010
Vukovic, J., Borlikova, G. G., Ruitenberg, M. J., Robinson, G. J., Sullivan, R. K., Walker, T. L., et al. (2013). Immature doublecortin-positive hippocampal neurons are important for learning but not for remembering. J. Neursci. 33, 6603–6613.
Walker, T. L., White, A., Black, D. M., Wallace, R. H., Sah, P., and Bartlett, P. F. (2008). Latent stem and progenitor cells in the hippocampus are activated by neural excitation. J. Neurosci. 28, 5240–5247. doi: 10.1523/JNEUROSCI.0344-08.2008
Webb, E. A., O’reilly, M. A., Clayden, J. D., Seunarine, K. K., Chong, W. K., Dale, N., et al. (2012). Effect of growth hormone deficiency on brain structure, motor function and cognition. Brain 135, 216–227.
Weltman, A., Weltman, J. Y., Roy, C. P., Wideman, L., Patrie, J., Evans, W. S., et al. (2006). Growth hormone response to graded exercise intensities is attenuated and the gender difference abolished in older adults. J. Appl. Physiol. 100, 1623–1629. doi: 10.1152/japplphysiol.01312.2005
Weltman, A., Weltman, J. Y., Schurrer, R., Evans, W. S., Veldhuis, J. D., and Rogol, A. D. (1992). Endurance training amplifies the pulsatile release of growth hormone: Effects of training intensity. J. Appl. Physiol. 72, 2188–2196.
Weltman, A., Weltman, J. Y., Veldhuis, J. D., and Hartman, M. L. (2001). Body composition, physical exercise, growth hormone and obesity. Eat. Weight Disord 6, 28–37.
Winter, B., Breitenstein, C., Mooren, F. C., Voelker, K., Fobker, M., Lechtermann, A., et al. (2007). High impact running improves learning. Neurobiol. Learn. Mem. 87, 597–609.
Wong-Goodrich, S. J., Pfau, M. L., Flores, C. T., Fraser, J. A., Williams, C. L., and Jones, L. W. (2010). Voluntary running prevents progressive memory decline and increases adult hippocampal neurogenesis and growth factor expression after whole-brain irradiation. Cancer Res. 70, 9329–9338. doi: 10.1158/0008-5472.CAN-10-1854
Zaccaria, M., Varnier, M., Piazza, P., Noventa, D., and Ermolao, A. (1999). Blunted growth hormone response to maximal exercise in middle-aged versus young subjects and no effect of endurance training. J. Clin. Endocrinol. Metab. 84, 2303–2307. doi: 10.1210/jcem.84.7.5853
Zhai, Q., Lai, Z., Roos, P., and Nyberg, F. (1994). Characterization of growth hormone binding sites in rat brain. Acta Paediatr. Suppl. 406, 92–95.
Zhang, H., Han, M., Zhang, X., Sun, X., and Ling, F. (2014). The effect and mechanism of growth hormone replacement on cognitive function in rats with traumatic brain injury. PLoS One 9:e108518. doi: 10.1371/journal.pone.0108518
Keywords: growth hormone, ageing, cognition, neural function, neural stem cells, exercise
Citation: Blackmore DG and Waters MJ (2023) The multiple roles of GH in neural ageing and injury. Front. Neurosci. 17:1082449. doi: 10.3389/fnins.2023.1082449
Received: 28 October 2022; Accepted: 17 February 2023;
Published: 07 March 2023.
Edited by:
Jesús Devesa, University of Santiago de Compostela, SpainReviewed by:
Junjie Zhuo, Hainan University, ChinaCopyright © 2023 Blackmore and Waters. This is an open-access article distributed under the terms of the Creative Commons Attribution License (CC BY). The use, distribution or reproduction in other forums is permitted, provided the original author(s) and the copyright owner(s) are credited and that the original publication in this journal is cited, in accordance with accepted academic practice. No use, distribution or reproduction is permitted which does not comply with these terms.
*Correspondence: Michael J. Waters, bS53YXRlcnNAdXEuZWR1LmF1
Disclaimer: All claims expressed in this article are solely those of the authors and do not necessarily represent those of their affiliated organizations, or those of the publisher, the editors and the reviewers. Any product that may be evaluated in this article or claim that may be made by its manufacturer is not guaranteed or endorsed by the publisher.
Research integrity at Frontiers
Learn more about the work of our research integrity team to safeguard the quality of each article we publish.