- Department of Neurosurgery, Affiliated Union Hospital, Fujian Medical University, Fuzhou, China
Background: Although some previous studies have indicated that extracellular vesicles (EVs) secreted from miRNA-modified mesenchymal stem cells (MSCs) may be more effective as compared with control EVs in the treatment of rats with spinal cord injuries (SCI), the efficacy of this treatment modality remains controversial.
Objectives: The current study comprehensively evaluated the efficacy of different administered doses of EVs, including miRNA-overexpressing MSCs-derived EVs, among SCI rats. The efficacy of EVs' treatment was evaluated in different SCI models to provide evidence for preclinical trials.
Methods: We extensively searched the following databases to identify relevant studies: PubMed, Embase, Scopus, The Cochrane Library, and Web of Science (from inception to July 20, 2022). Two trained investigators independently screened literature, extracted the data, and evaluated literature quality.
Results: Thirteen studies were included in this network meta-analysis. The results demonstrated that miRNA-overexpressing MSCs-derived EVs (100 and 200 μg of total protein of EVs) significantly improved hind limb motor function in rats at early stages of SCI (i.e., at 3 days after injury) as compared with EVs (100 and 200 μg of total protein of EVs, respectively). However, in the middle and late stages (14 and 28 days), there were no statistically significant differences between EVs with 200 μg dosages and miRNA-loaded EVs with 100 μg dosages. In the late stages (28 days), there were no statistically significant differences between EVs with 100 μg dosages and miRNA-loaded EVs with 200 μg dosages. We found that miRNA-overexpressing MSCs-derived EVs significantly improved motor function among early-stage SCI rats in a compression and contusion model (3 days) as compared with MSCs-derived EVs and miRNA-overexpressing MSCs-derived EVs likewise significantly improved motor function among SCI rats in a contusion model at middle and late stages (14 and 28 days).
Conclusion: Our results suggest that miRNA-overexpressing MSCs-derived EVs (200 μg of total protein of EVs) may be the best choice for the effective treatment of SCI, and miRNA-overexpressing MSCs-derived EVs may likewise be the best choice for treating contusions. However, there are some risks of bias in our included studies, and the mechanisms underlying the efficacy of EVs remain unclear.
Systematic review registration: https://www.crd.york.ac.uk/prospero/display_record.php?RecordID=282051, identifier: CRD42021282051.
Introduction
Spinal cord injury (SCI) is a disease associated with a high disability rate. SCI not only affects patient quality of life, but also imposes a heavy burden on society. Hence, it is essential to develop effective treatments for SCI (Ahuja et al., 2017). Early surgical decompression is considered an effective treatment (Ramakonar and Fehlings, 2021). However, there is currently a lack of effective drugs for inhibiting secondary injury. SCI is characterized by progressive loss of neurologic function, with secondary injuries causing severe damage such as release of toxic chemicals and proinflammatory cytokines, hemorrhage and ischemia of blood vessels, and neuronal apoptosis (McDonald and Sadowsky, 2002). Thus, it is critical to develop effective drugs. Currently, melatonin (Zhang et al., 2018), riluzole (Fehlings et al., 2021a), Rho inhibitors (Fehlings et al., 2021b), and methylprednisolone (Liu et al., 2020) have been evaluated in clinical and preclinical trials. The efficacy of these drugs remains controversial. Generally speaking, the clinical application of new drugs necessitates preclinical trials including drugs for SCI. Currently, an array of new drugs are being developed within animal models of SCI (Kabu et al., 2015).
Recently, various major breakthroughs have been made in cell therapy with respect to treating SCI, including treatment with mesenchymal stem cells (MSCs) (Huang W. et al., 2021). However, immune rejection and tumorigenicity limit the clinical application of cell therapy. Studies suggest that the remarkable effects of cell therapy can be attributed to its dominant paracrine effect (Liu et al., 2021). Extracellular vesicles (EVs), an intercellular communication tool, play a critical role in this putative mechanism. Exosomes, ectosomes or shedding microvesicles (SMVs), and apoptotic bodies are the main subtypes of EVs and differ for their biogenesis, functions and properties. Exosomes are small EVs 40–150 nm in size. Moreover, exosomes are endosome-derived molecules secreted in most cells and are reported to carry proteins, lipids, DNA, and RNAs (Jeppesen et al., 2019). In contrast to exosomes, ectosomes or shedding microvesicles (SMVs) are large vesicles between 100 and 1,000 nm in diameter that are secreted by outward budding of the plasma membrane. Apoptotic bodies are heterogeneous vesicles released from apoptotic cells and are thought to be ~50–5,000 nm in diameter. These vesicles are between 50 and 1,000 nm in size and partially overlap with the exosome size. For this reason, it has not been possible to isolate pure subtypes of EVs to date. For further information on the different origins of these types of vesicles, their content, and their functions, the reader is referred to several comprehensive reviews (Kalra et al., 2016; Thery et al., 2018; van Niel et al., 2022). There are many different sources of EVs, including MSCs, neural stem cells (NSCs) (Rong et al., 2019), macrophage (Zhang B. et al., 2021), pericyte (Yuan et al., 2019), Schwann cells (SCs) (Pan et al., 2021), and olfactory ensheathing cells (OECs) (Tu and Hsueh, 2020). Among them, MSC-derived EVs were widely studied and were considered the most appropriate source (Ren et al., 2020). MSCs originate from bone marrow, adipose tissue, and umbilical cord, having pluripotent differentiation ability and low immunogenicity (Liau et al., 2020). It is reported that MSC-derived EVs could significantly reduce neuronal apoptosis and the expression levels of inflammatory markers TNF-α, IL-1β, and IL-6 (Gu et al., 2020). Moreover, they could inhibit fibrotic and glial scar formation by reducing CSPG deposition and the activation of A1 neurotoxic reactive astrocytes (Liu et al., 2019).
A meta-analysis showed that EVs improve locomotor function in rodents with SCI; however, there are still many controversial issues in the treatment of SCI via EVs, especially with respect to differential effects in humans and animal models as well as controversies with regard to the most effective administered EVs' dose (Yi and Wang, 2021). Some studies indicate that EVs secreted from miRNA-modified MSCs are more effective within SCI as compared with control EVs (Huang et al., 2020; Li et al., 2020; Zhang A. et al., 2021). Both miR-181c (Zhang M. et al., 2021) and miR-26a (Chen et al., 2021) can inhibit the expression of the target gene PTEN, which attenuates the inflammatory response. Nevertheless, the efficacy of this treatment modality remains controversial. To the best of our knowledge, there are no trials comparing different EVs' doses and no trials evaluating miRNA-loaded EVs in the treatment of SCI. There are likewise no trials comparing the efficacy of miRNA-loaded and non-miRNA-loaded EVs within different SCI models. MiRNA-modified MSCs are MSCs that were transfected with miRNA mimic in cell culture. EVs derived from MSCs over-expressing a specific miRNA were named miRNA-loaded EVs in the following studies.
Thus, we conducted a systematic review of the literature and network meta-analysis of data from literature research to evaluate the efficacy of different administered EVs' doses and the effects of miRNA-loaded EVs among SCI rats. We likewise evaluated the efficacy of EVs and miRNA-loaded EVs in different SCI models to provide rigorous evidence for preclinical trials.
Methods
We adhered to PRISMA (Preferred Reporting Items for Systematic Reviews and Meta-Analysis) guidelines in conducting this systematic review (Page et al., 2021). The study protocol was registered in the PROSPERO database with registration number: CRD42021282051. The following search terms were used: (“spinal cord injury” OR “Hemisection” OR “contusion injury” OR “dorsal column injury” OR “complete transection” OR “corticospinal tract injury” OR “Paraplegia” OR “Quadriplegia” OR “Hemiplegia” OR “tetraplegia” OR “Monoplegia” OR “spinal cord trauma” OR “spinal cord transection” OR “spinal cord laceration” OR “spinal cord compromise” OR “spinal cord lesion” OR “spinal cord rupture” OR “spinal cord contusion” OR “spinal cord compression” OR “spinal cord hemisection” OR “traumatic myelopath”) AND (“extracellular vesicles” OR “ exosomes” OR “nano sized vesicles” OR “microvesicles” OR “shedding vesicles” OR “apoptotic bodies”). A total of 197 studies were identified according to search terms. We screened a total of 166 studies, extracted the data of 13 included studies, and evaluated the literature quality of 13 included studies.
Search strategies
We extensively searched the following databases from inception to July 20, 2022 to identify relevant studies: PubMed, Embase, Scopus, The Cochrane Library, and Web of Science databases. We likewise searched the references within identified reviews and manuscripts. Language restriction was English. The comprehensive search strategy is presented in Supplementary File 1.
Study selection
Two investigators (ZLY and JR) screened literature separately according to the inclusion and exclusion criteria specified below. If the two researchers had different opinions, a decision was negotiated. If this negotiation was not effective, it was arbitrated by a third party (CMC).
Eligibility criteria
We developed inclusion and exclusion criteria for this study in strict accordance with PICOS (population, intervention, comparison, outcome, study design) principles. The inclusion and exclusion criteria are as follows.
Types of participants (P): All studies on SCI rats were included. Studies in other animals and in humans with SCI were excluded.
Types of interventions (I): All studies using EVs derived from miRNA-modified MSCs in the treatment of SCI were included. Studies using EVs derived from cells without miRNA modification were excluded.
Types of comparisons (C): Studies in which animals were divided into at least three groups were included. The control group received phosphate buffered saline (PBS) following SCI, and the two intervention groups received EVs and miRNA-loaded EVs, respectively.
Types of outcomes (O): The outcome indicator used in this review was the Basso, Beattie & Bresnahan (BBB) scoring system, which can be used to assay the hind limb motor function among rats.
Types of studies (S): All studies evaluating miRNA-loaded EVs with respect to improving locomotor function among rats with SCI were included.
Data extraction and quality assessment
Two trained investigators (ZLY and JR) independently extracted the data from studies screened according to the aforementioned inclusion and exclusion criteria and cross-checked the identified studies. In the case of any disputes, a third party (CMC) was consulted to solve these disputes through negotiation. The extracted contents included (a) study characteristics: author, year, animal models, sex, weight, anesthetics, SCI models, the origins of the EVs, administered doses, and routes of administration; and (b) outcome indicators (i.e., BBB scores). The SYRCLE (Systematic Review Center for Laboratory Animal Experimentation) risk of bias tool was used to assay the quality of the included studies, with respect to selection bias, performance bias, detection bias, attrition bias, reporting bias, and other considerations from a list of 10 entries (Hooijmans et al., 2014).
Outcome measurements
BBB scores were implemented to assay the hind limb motor function of rats. Rats were placed in an open field and allowed to move freely for 5 min. Two independent researchers blinded to the rats' grouping then recorded the movements of the hip, knee, and ankle joints. The scores ranged from 0 (flaccid paralysis) to 21 (normal gait). The average score of the two observers was used as the final score for each outcome.
Statistical analysis
An R version 4.1.1 based on Bayesian model (The R Project for Statistical Computing, Vienna, Austria) was used to perform the current network meta-analysis. Mean differences (MD) and associated 95% confidence intervals (CI) were utilized for continuous outcomes. The adjusted indirect comparisons were performed based on MD and 95% CI values to assess the indirect comparisons with respect to the efficacy of different animal models and the administered EVs' dose. I2 tests were used to assess statistical heterogeneity. I2 values >50% were considered statistically significant heterogeneity. A random-effects model was used in the current study. The results are shown via forest plots. The surface under the cumulative ranking curve (SUCRA) was used to estimate ranking probabilities (Salanti et al., 2011).
There are three key assumptions within network meta-analysis: similarity, transitivity, and consistency (Cipriani et al., 2013). The inclusion and exclusion criteria were tightly controlled to meet the similarity and transitivity assumptions, including with respect to the severity of illness at baseline, sample size, sex, weight, EVs' origins, and routes of administration (Salanti, 2012). The animal models and interventions in all included studies within the network meta-analysis were likewise evaluated via meta-regression. By definition, inconsistency could not occur within a multi-arm study (Higgins et al., 2012). Goodness-of-fit was assessed via the deviance information criterion (DIC). Model fit was evaluated by comparing the DIC values between the consistency and inconsistency models.
Results
Article selection process
A total of 197 studies were identified in searching the PubMed database. However, no relevant studies were found from other databases (Supplementary File 1). Two duplicate studies were excluded. After screening the title and abstract, 166 studies were removed because of the study types, animal models, and interventions. The full text of the remaining 29 studies was meticulously retrieved for assessment; 16 studies were excluded due to reasons specified in the flow chart (Figure 1). A total of 13 studies were included in this network meta-analysis.
Study characteristics
The characteristics of the 13 included studies are summarized in Table 1. The sample size for each group ranged from n = 6 to n = 24. Most (92.3%) animal models were Sprague Dawley (SD) rats. Most (69.23%) were male. The body weights ranged from 80 to 300 g, and ages varied from 2 to 8 weeks. The SCI models included transection, contusion, and compression models. All studies assessed the thoracic spinal cord (T10) level at which damaged. Compression injury was induced using an aneurysm clip in two studies, and contusion injury was impacted using a standard striking device in four studies. In all these studies, all EVs originated from mesenchymal stem cells (MSCs) and almost all EVs were injected through the tail vein. Three studies used the ExoQuick-TC kit, and 10 used ultracentrifugations to isolate EVs. Ultimately, the administered EVs' dose ranged from 100 to 700 μg. In nine studies, the time of dosing was delayed (i.e., administration within 24 h after surgery). In four studies, the EV was administered immediately after surgery. Although EVs derived from MSCs overexpress specific miRNAs (miR-181c, miR-26a, miR-126, miR-29b, miR-133b, miR-544, miR-494, miR-329-3p, miR-29b, miR-511-3p, miR-22, miR-9-5p, and let-7a-5p) differently, their role in promoting SCI repair is the same. The MSCs were transfected with miRNA with Lipofectamine in eight studies, with siRNA in three studies, and with Lentiviral vector in one study. However, there is one study that did not specify the method.
Quality assessment and publication bias
The SYRCLE tool was used to assay the risk of bias in all included studies. The methodologic quality of the results from each study are summarized in Figure 2. There was no high risk of bias in the 13 included studies. All included studies had a low risk of sequence generation with respect to selection bias. However, all included studies had an unclear risk of incomplete outcome data for attrition bias and 12 (92%) had an unclear risk of selective outcomes for reporting bias. Moreover, most included studies showed low risk with respect to many items, including allocation concealment in terms of selection bias, baseline characteristics for selection bias, random animal housing and blinding with regard to performance bias, random outcome assessment and blinding for detection bias, and other sources of bias. The methodologic quality of all the included trials was satisfactory. According to Cochrane Group guidelines, funnel plots are not recommended for detecting publication bias when fewer than 10 studies are included in a meta-analysis (Stang, 2010). However, this study included 13 studies. Therefore, we used funnel plots and Egger regression tests to assess publication bias. As shown in the Figure 3A, there is a significant funnel plot asymmetry visually, indicating publication bias. Egger's test (P = 0.0217) was used to test for potential publication bias in the primary results. The trim and fill analysis (Figure 3B) estimated five “missing” unpublished studies on the right side of the funnel plot.
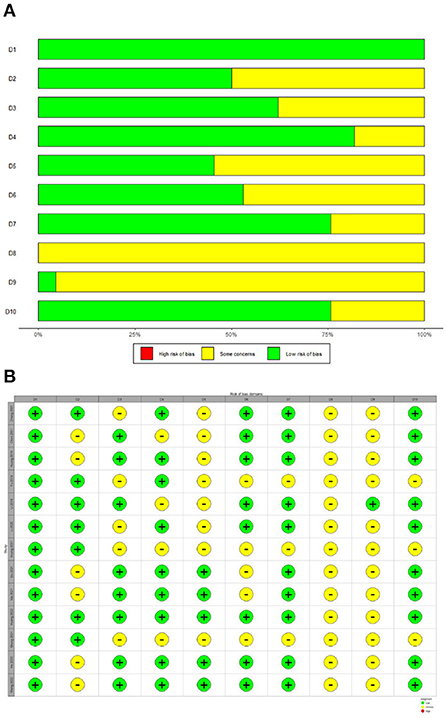
Figure 2. Risk of bias with SYRCLE tool. (A) Risk of bias graph. (B) Risk of bias summary. D1 (Selection bias): Was the allocation sequence adequately generated and applied? D2 (Selection bias): Were the groups similar at baseline or were they adjusted for confounders in the analysis? D3 (Selection bias): Was the allocation adequately concealed? D4 (Performance bias): Were the animals randomly housed during the experiment? D5 (Performance bias): Were the caregivers and/or investigators blinded from knowledge which intervention each animal received during the experiment? D6 (Detection bias): Were animals selected at random for outcome assessment? D7 (Detection bias): Was the outcome assessor blinded? D8 (Attrition bias): Were incomplete outcome data adequately addressed? D9 (Reporting bias): Are reports of the study free of selective outcome reporting? D10 (Other): Was the study apparently free of other problems that could result in high risk of bias?
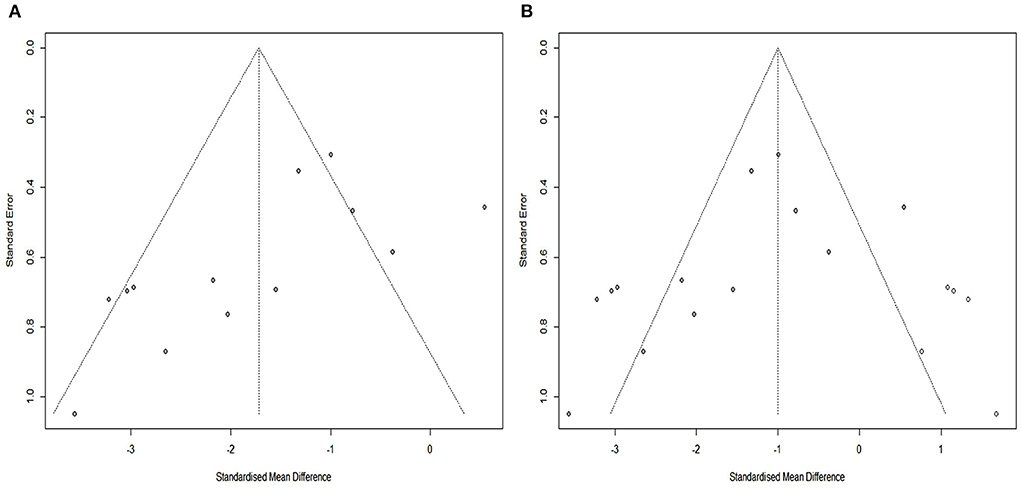
Figure 3. Assessment of publication bias. (A) Funnel plots showed pronounced asymmetry. (B) Trim-and-fill analysis predicted 5 “missing” studies (unfilled circles).
Network meta-analysis of BBB scores at different dosages
First, with respect to findings 3 days after administration, one study (Wang et al., 2022) was excluded because a polyethylene catheter was placed at the injured level for continuous intrathecal injection and two studies (Huang W. et al., 2021; He et al., 2022) were excluded because the administered dose had changed at 3 days. Therefore, 10 included studies (Li et al., 2018, 2020; Yu et al., 2019; Huang et al., 2020, 2022; Chen et al., 2021; Nie and Jiang, 2021; Sheng et al., 2021; Zhang M. et al., 2021; Liu et al., 2022) were used for the data analysis. The results of this network meta-analysis suggest that the number of studies evaluating EVs and miRNA-loaded EVs was higher with the 100 μg dose as compared with the 200 μg dose (Figure 4A). Moreover, forest plots (Figure 4B) and league tables (Table 2) show that miRNA-loaded EVs at 100 μg dosages presented with a statistically significant advantage over PBS (MD = 1.3; 95% CI: 0.79, 1.84; p < 0.01) and EVs at 100 μg dosages (MD = 0.87; 95% CI: 0.36, 1.39; p < 0.01) and 200 μg dosages (MD = 0.86; 95% CI: 0.07, 1.67; p < 0.01), respectively. Additionally, miRNA-loaded EVs at 200 μg dosages presented with a statistically significant advantage over PBS (MD = 1.53; 95% CI: 0.91, 2.15; p < 0.01) and EVs at 100 μg dosages (MD = 1.1; 95% CI: 0.29, 1.9; p < 0.01) and 200 μg dosages (MD = 1.09; 95% CI: 0.47, 1.71; p < 0.01), respectively. However, the differences were not statistically significant with respect to the other comparisons. Finally, the SUCRA ranking graph showed that miRNA-loaded EVs at 200 μg dosages (92.93%) ranked highest, followed by miRNA-loaded EVs at 100 μg dosages (81.43%), EVs at 200 μg dosages (36.71%), EVs at 100 μg dosages (36.07%), and PBS (2.87%) (Figure 4C).
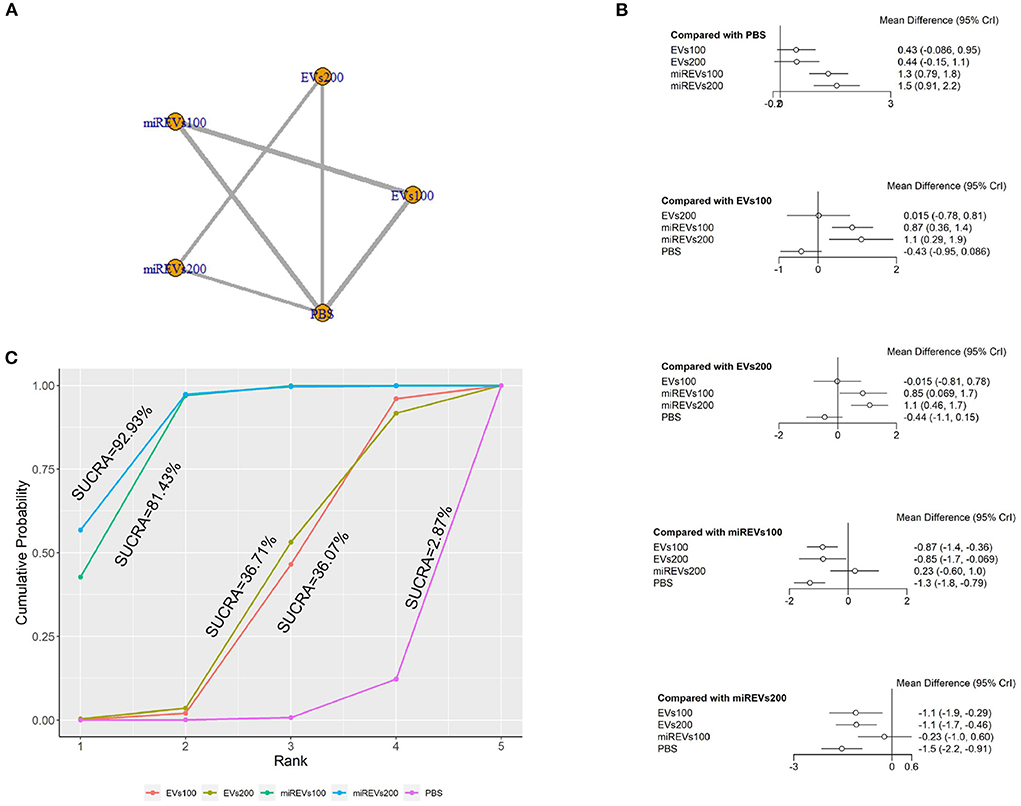
Figure 4. The network meta-analysis of BBB scores at different dosages at 3 days after administration. (A) Network plot; (B) Forest plot; (C) SUCRA plot. EVs100, EVs at 100 μg dosages; EVs200, EVs at 200 μg dosages; miREVs100, miRNAs-loaded EVs at 100 μg dosages; miREVs200, miRNAs-loaded EVs at 200 μg dosages.
Second, with respect to findings 14 days after administration, two studies (Li et al., 2020; Liu et al., 2022) were excluded because BBB scores were not assessed at 14 days and four studies (Huang W. et al., 2021; Sheng et al., 2021; Zhang M. et al., 2021) were excluded because the administered dose had changed at 14 days. Furthermore, one study (Wang et al., 2022) was excluded because a polyethylene catheter was placed at the injured level for continuous intrathecal injection. Therefore, six studies (Li et al., 2018; Yu et al., 2019; Huang et al., 2020, 2022; Chen et al., 2021; Nie and Jiang, 2021) were included for data analysis. The results of this network meta-analysis suggest that the number of studies with EVs and miRNA-loaded EVs was higher with the 100 μg dose as compared with the 200 μg dose (Figure 5A). The forest plot (Figure 5B) and league table (Table 3) show that miRNA-loaded EVs at 100 μg dosages presented with a statistically significant advantage over PBS (MD = 4.85; 95% CI: 2.94, 6.74; p < 0.01) and EVs at 100 μg dosages (MD = 3.24; 95% CI: 1.33, 5.13; p < 0.01), respectively. Additionally, miRNA-loaded EVs at 200 μg dosages presented with a statistically significant advantage over PBS (MD = 5.11; 95% CI: 2.42, 7.79; p < 0.01) and EVs at 100 μg dosages (MD = 3.5; 95% CI: 0.2, 6.78; p < 0.01) and 200 μg dosages (MD = 3.38; 95% CI: 0.68, 6.09; p < 0.01), respectively. However, the differences were not statistically significant among the other comparisons. The SUCRA ranking graph showed that miRNA-loaded EVs at 200 μg dosages (88.46%) ranked highest, followed by the miRNA-loaded EVs at 100 μg dosages (84.92%), EVs at 200 μg dosages (37.18%), EVs at 100 μg dosages (36.19%), and PBS (3.25%) (Figure 5C).
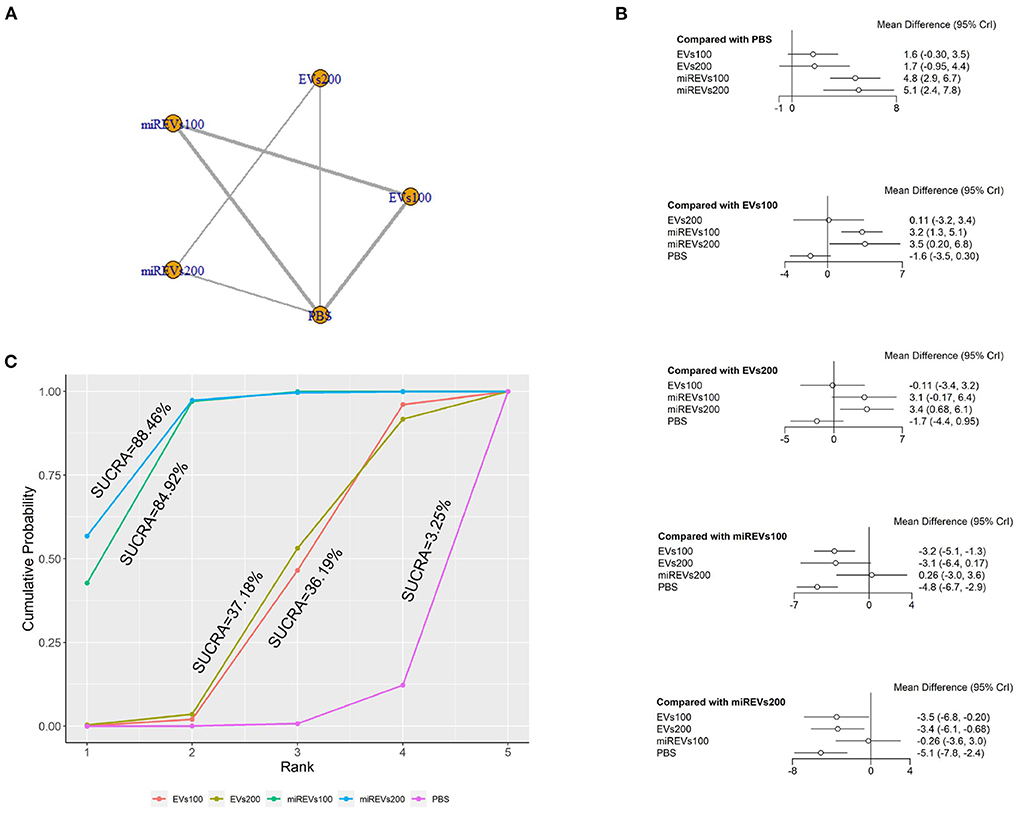
Figure 5. The network meta-analysis of BBB scores at different dosages at 14 days after administration. (A) Network plot; (B) Forest plot; (C) SUCRA plot. EVs100, EVs at 100 μg dosages; EVs200, EVs at 200 μg dosages; miRExos100, miRNAs-loaded EVs at 100 μg dosages; miREVs200, miRNAs-loaded EVs at 200 μg dosages.
Finally, with respect to findings 28 days after administration, three studies (Li et al., 2018, 2020; Liu et al., 2022) were excluded because BBB scores were not assessed at 28 days after administration and four studies (Huang W. et al., 2021; Sheng et al., 2021; Zhang A. et al., 2021; He et al., 2022) were excluded because the administered dose had changed at 28 days. Moreover, one study (Wang et al., 2022) was excluded because a polyethylene catheter was placed at the injured level for continuous intrathecal injection. Thus, five studies (Yu et al., 2019; Huang et al., 2020, 2022; Chen et al., 2021; Nie and Jiang, 2021) were included for data analysis. The results of this network meta-analysis suggest that the number of studies evaluating EVs and miRNAs-loaded EVs was higher with the 100 μg dose than with the 200 μg dose (Figure 6A). The forest plot (Figure 6B) and league table (Table 4) show that miRNA-loaded EVs at 100 μg dosages presented with a statistically significant advantage over PBS (MD = 7.22; 95% CI: 4.14, 10.29; p < 0.01) and EVs at 100 μg dosages (MD = 3.9; 95% CI: 0.82, 6.98; p < 0.01), respectively. Additionally, miRNA-loaded EVs at 200 μg dosages presented with a statistically significant advantage over PBS (MD = 7.81; 95% CI: 4.03, 11.57; p < 0.01) and EVs at 200 μg dosages (MD = 4.33; 95% CI: 0.57, 8.12; p < 0.01), respectively. Moreover, EVs at 100 μg dosages presented with a statistically significant advantage over PBS (MD = 3.31; 95% CI: 0.24, 6.42; p < 0.01). However, the differences were not statistically significant among the other comparisons. The SUCRA ranking graph showed that miRNA-loaded EVs at 200 μg dosages (89.15%) ranked highest, followed by the miRNA-loaded EVs at 100 μg dosages (82.97%), EVs at 200 μg dosages (39.25%), EVs at 100 μg dosages (37.25%), and PBS (1.37%) (Figure 6C).
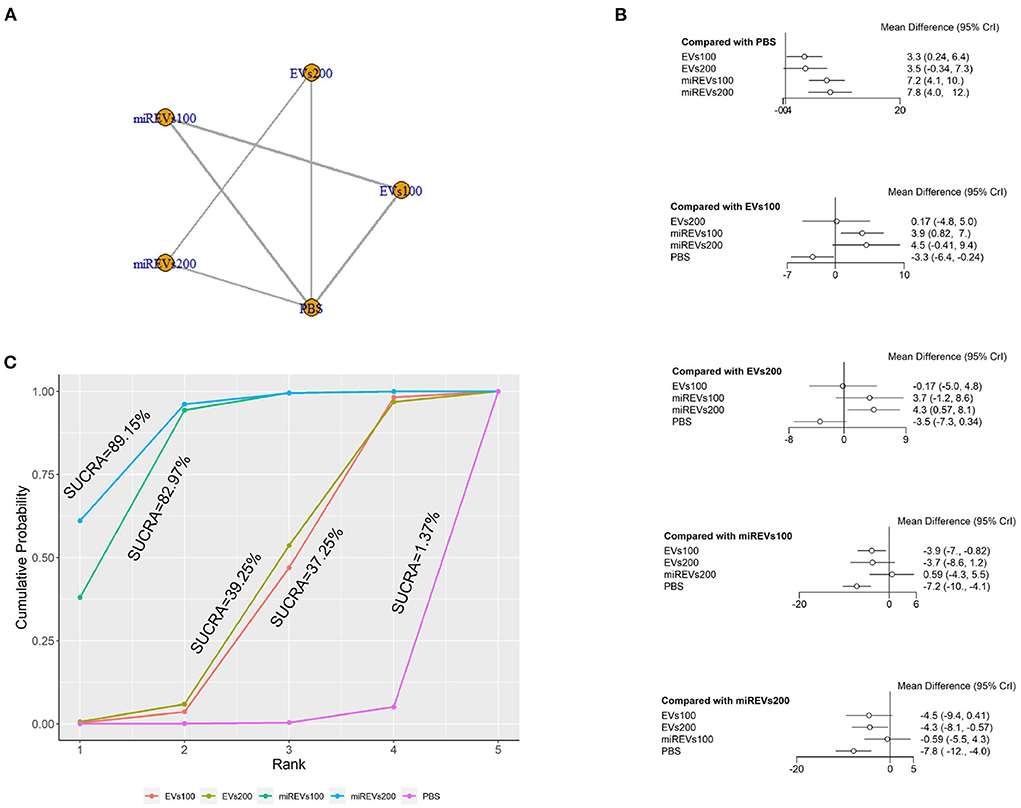
Figure 6. The network meta-analysis of BBB scores at different dosages at 28 days after administration. (A) Network plot; (B) Forest plot; (C) SUCRA plot. EVs100, EVs at 100 μg dosages; EVs200, EVs at 200 μg dosages; miREVs100, miRNAs-loaded EVs at 100 μg dosages; miREVs200, miRNAs-loaded EVs at 200 μg dosages.
The network meta-analysis of BBB scores in different models
First, with respect to findings 3 days after administration, two studies (Huang W. et al., 2021; Wang et al., 2022) were excluded because BBB scores were not assessed at 3 days and one study (Nie and Jiang, 2021) was excluded because the SCI model was transection model. Therefore, 10 included studies (Li et al., 2018, 2020; Yu et al., 2019; Huang et al., 2020, 2022; Chen et al., 2021; Sheng et al., 2021; Zhang M. et al., 2021; He et al., 2022; Liu et al., 2022) were included for data analysis. The results of this network meta-analysis suggest that EVs and miRNA-loaded EVs in contusion models had more studies than in compression models (Figure 7A). Moreover, the forest plot (Figure 7B) and league table (Table 5) showed that the miRNA-loaded EVs in contusion models presented with a statistically significant advantage over PBS (MD = 1.42; 95% CI: 0.96, 1.89; p < 0.01) and the EVs in compression models (MD = 1.18; 95% CI: 0.31, 2.05; p < 0.01) and contusion models (MD = 0.94; 95% CI: 0.47, 1.41; p < 0.01), respectively. Additionally, the miRNAs-loaded EVs in compression models likewise had a statistically significant advantage over PBS (MD = 1.35; 95% CI: 0.61, 2.11; p < 0.01) and EVs in compression models (MD = 1.12; 95% CI: 0.37, 1.86; p < 0.01) and contusion models (MD = 0.87; 95% CI: 0, 1.76; p < 0.01), respectively. Furthermore, EVs in contusion models presented with a statistically significant advantage over PBS (MD = 0.48; 95% CI: 0.02, 0.94; p < 0.01). However, the differences were not statistically significant among the other comparisons. Finally, the SUCRA ranking graph showed that miRNA-loaded EVs in contusion models (88.83%) ranked highest, followed by the miRNA-loaded EVs in compression models (85.32%), EVs in contusion models (43.16%), EVs in compression models (25.94%), and PBS (6.75%) (Figure 7C).
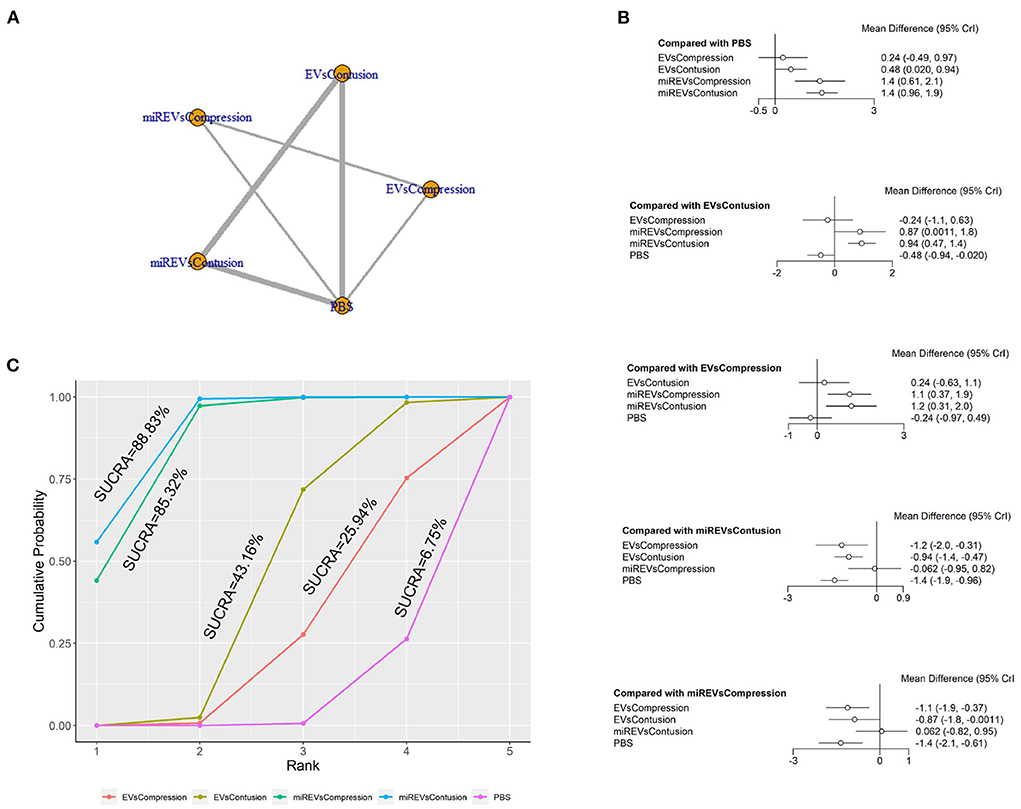
Figure 7. The network meta-analysis of BBB scores in different models at 3 days after administration. (A) Network plot; (B) Forest plot; (C) SUCRA plot. EVsCompression, EVs in compression models; EVsContusion, EVs in contusion models; miREVsCompression, miRNAs-loaded EVs in compression models; miREVsContusion, miRNAs-loaded EVs in contusion models.
Next, with respect to findings 14 days after administration, three studies (Li et al., 2020; Sheng et al., 2021; Liu et al., 2022) were excluded because BBB scores were not assessed at 14 days and one study (Nie and Jiang, 2021) was excluded because the SCI model was transection model. Therefore, a total of nine studies (Li et al., 2018; Yu et al., 2019; Huang et al., 2020, 2022; Chen et al., 2021; Huang L. et al., 2021; Zhang M. et al., 2021; He et al., 2022; Wang et al., 2022) were included for data analysis. The results of this network meta-analysis suggest that EVs and miRNA-loaded EVs had more studies within contusion models as compared with compression models (Figure 8A). The forest plot (Figure 8B) and league table (Table 6) showed that EVs in contusion models (MD = 2.42; 95% CI: 0.86, 3.96; p < 0.01) and miRNA-loaded EVs in compression models (MD = 3.66; 95% CI: 0.8, 6.53; p < 0.01) and contusion models (MD = 4.52; 95% CI: 2.95, 6.04; p < 0.01) presented with a statistically significant advantage over PBS and that miRNA-loaded EVs in contusion models had a statistically significant advantage over the EVs in compression models (MD = 3.65; 95% CI: 0.39, 6.89; p < 0.01) and contusion models (MD = 2.1; 95% CI: 0.53, 3.62; p < 0.01). The differences were not statistically significant among the other comparisons. The SUCRA ranking graph showed that the miRNA-loaded EVs in contusion models (92.19%) ranked highest, followed by the miRNA-loaded EVs in compression models (76.08%), EVs in contusion models (51.38%), EVs in compression models (23.57%), and PBS (6.79%) (Figure 8C).
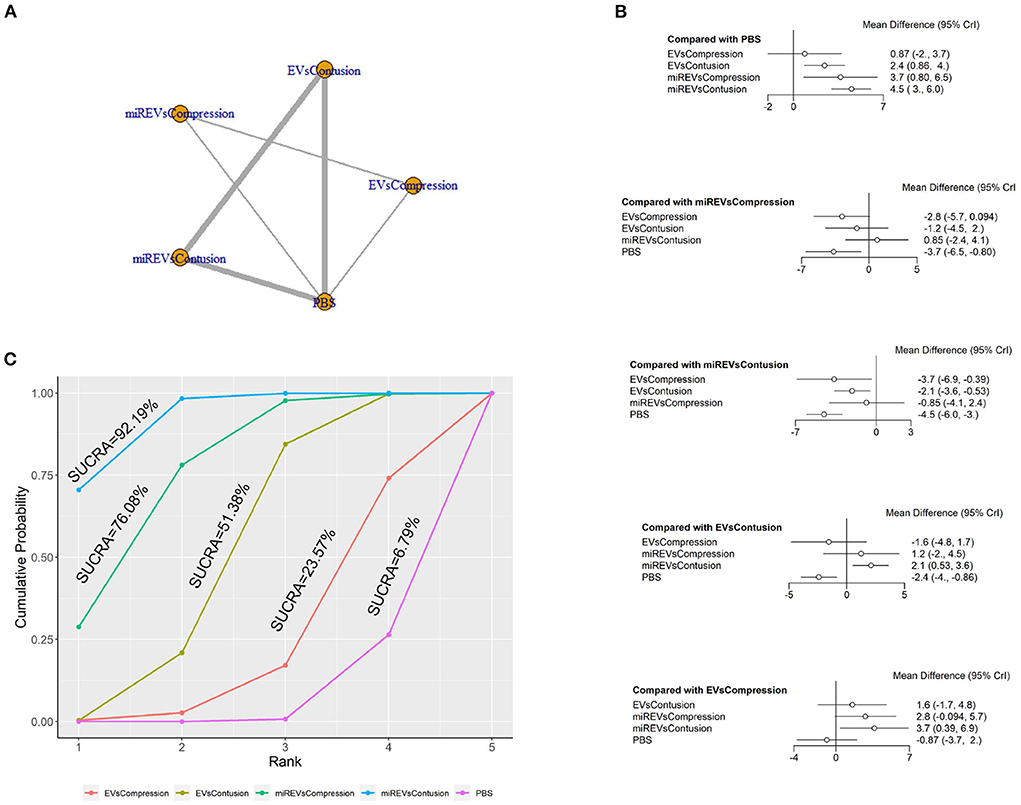
Figure 8. The network meta-analysis of BBB scores in different models at 14 days after administration. (A) Network plot; (B) Forest plot; (C) SUCRA plot. EVsCompression, EVs in compression models; EVsContusion, EVs in contusion models; miREVsCompression, miRNAs-loaded EVs in compression models; miREVsContusion, miRNAs-loaded EVs in contusion models.
Finally, with respect to findings 28 days after administration, four studies (Li et al., 2018, 2020; Sheng et al., 2021; Liu et al., 2022) were excluded because BBB scores were not assessed at 28 days and one study (Nie and Jiang, 2021) was excluded because the SCI model was transection model. Therefore, eight included studies (Yu et al., 2019; Huang et al., 2020; Chen et al., 2021; Huang L. et al., 2021; Huang W. et al., 2021; Zhang M. et al., 2021; He et al., 2022; Wang et al., 2022) were used for data analysis. The results of this network meta-analysis suggest that more studies evaluated EVs and miRNA-loaded EVs in the contusion model as compared with the compression model (Figure 9A). The forest plot (Figure 9B) and league table (Table 7) showed that EVs in contusion models (MD = 4.05; 95% CI: 2.23, 5.87; p < 0.01) and miRNA-loaded EVs in compression models (MD = 5.99; 95% CI: 1.16, 10.82; p < 0.01) and contusion models (MD = 6.83; 95% CI: 4.96, 8.63; p < 0.01) presented with a statistically significant advantage over PBS and that miRNA-loaded EVs in contusion models had a statistically significant advantage over the EVs in contusion models (MD = 2.78; 95% CI: 0.92, 4.58; p < 0.01). The differences were not statistically significant among the other comparisons. The SUCRA ranking graph showed that the miRNA-loaded EVs in contusion models (89.79%) ranked highest, followed by the miRNA-loaded EVs in compression models (77.15%), EVs in contusion models (49.41%), EVs in compression models (29.55%), and PBS (4.1%) (Figure 9C).
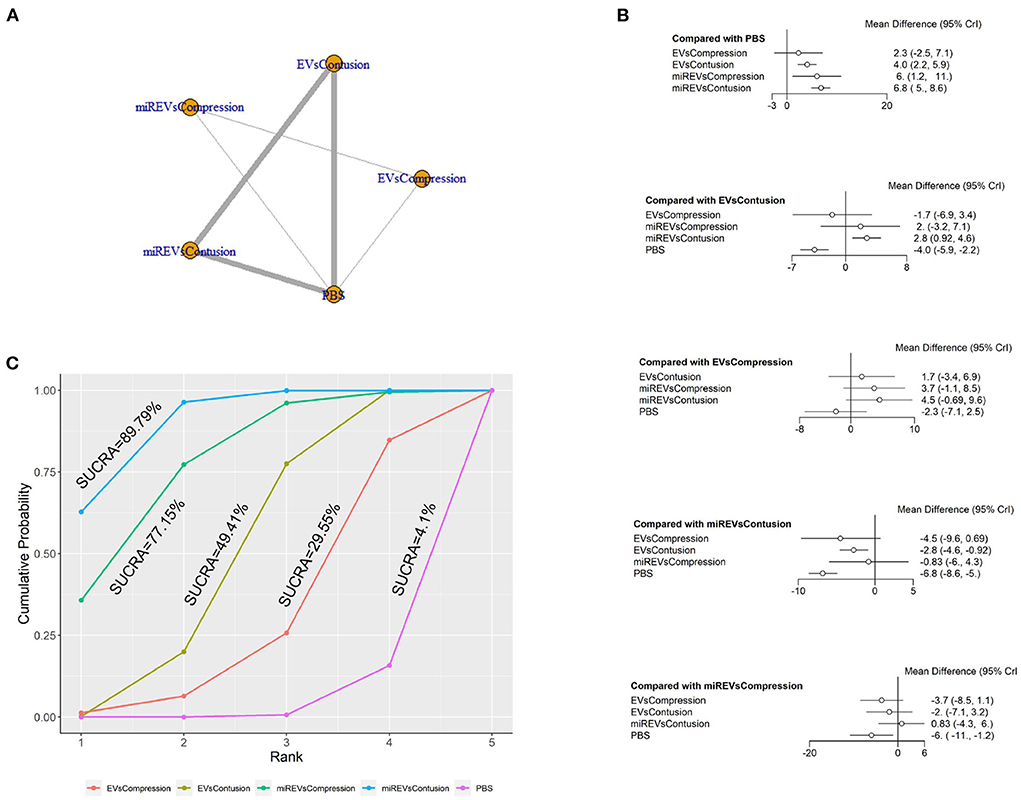
Figure 9. The network meta-analysis of BBB scores in different models at 28 days after administration. (A) Network plot; (B) Forest plot; (C) SUCRA plot. EVsCompression, EVs in compression models; EVsContusion, EVs in contusion models; miREVsCompression, miRNAs-loaded EVs in compression models; miREVsContusion, miRNAs-loaded EVs in contusion models.
Inconsistency assessment
The following factors were not evaluated because the severity of illness at baseline, sex, weight, EVs' origins, and routes of administration were almost entirely consistent. Because there was no inconsistency in the multi-arm studies (Higgins et al., 2012) (all studies included in the network meta-analysis were multi-arm studies), the inconsistency could not be evaluated. The DIC difference between the consistency and inconsistency models was less than five, which showed that the results of the consistency model were robust (Supplementary File 3).
Discussion
In summary, this systematic review and network meta-analysis of EVs derived from miRNA-modified MSCs for the treatment of SCI included data from 13 preclinical trials among a total of 396 rats.
Summary of the evidence
Summary of comparison of EVs and miRNA-loaded EVs at different dosages
This study indirectly compared the efficacy of different doses of EVs and miRNA-loaded EVs in the treatment of SCI. We likewise indirectly compared the efficacy of EVs and miRNA-loaded EVs in different SCI models. The results of this network meta-analysis showed that miRNA-loaded EVs with 100 and 200 μg dosages statistically significantly improved hind limb motor function among SCI rats at the early stage (3 days), as compared with EVs with 100 and 200 μg dosages. However, in the middle and late stages (14 and 28 days), there were no statistically significant differences between EVs with 200 μg dosages and miRNA-loaded EVs with 100 μg dosages. In the late stages (28 days), there were no statistically significant differences between EVs with 100 μg dosages and miRNA-loaded EVs with 200 μg dosages. This may be related to the half-life of EVs. Although EVs presented with the properties of immune privilege, they were also inevitably removed by macrophages. The circulation half-life of the EVs has been reported as only ~30 min following intravenous administration (Yang et al., 2020). Therefore, it is likely that the results of this network meta-analysis were not statistically significant in the late stage of treatment because the EVs had been depleted. Moreover, the SUCRA ranking graph suggested that miRNA-loaded EVs at 200 μg dosages may be most effective for treatment in early, middle, and late stages. A study by Dumbrava et al. conducted in 2021 demonstrated that low-dose EVs promote nerve regeneration following ischemia, in contrast to high-dose EVs (Dumbrava et al., 2022). This phenomenon may be associated with the biodistribution of exogenous EVs in vivo following intravenous administration. Studies report that EVs are normally distributed in organs of the mononuclear phagocyte system and accumulate most in the liver, followed by the spleen, gastrointestinal tract, and lungs. However, some studies show that their distribution and clearance are affected by the size of the particles. For example, small EVs are less susceptible to extravasate through the discontinuous endothelium in the spleen, which makes them enter into the red pulp smoothly (Wiklander et al., 2015). Appropriate administered doses are likely safe and efficacious with EV therapy, while high doses may produce toxicity and ineffective therapeutic effects. These results guide physicians in selecting the proper therapeutic dose for EVs and miRNA-loaded EVs. The main EV delivery methods to damaged tissue include intrathecal and tail vein injection. Most studies have used the tail vein injection method because of its simplicity and minimally invasive (Yi and Wang, 2021). Interestingly, a study showed that EVs could improve significant locomotor recovery by intranasal injection (Guo et al., 2019). Furthermore, some scholars designed MSC-derived EV fibrin glue to treat spinal cord injury (Mu et al., 2021). Although EV delivery methods are important for the treatment of SCI, their extraction methods also play a key role. However, there is no uniform standard for the EV extraction methods. The most commonly used is ultracentrifugation. Various other techniques include density gradients, filtration, and precipitation (Thery et al., 2018).
Summary of comparison of EVs and miRNA-loaded EVs in different models
Additionally, the results of this network meta-analysis showed that miRNA-loaded EVs statistically significantly improved hind limb motor function among SCI rats at early stages in compression and contusion models, as compared with EVs. Moreover, there are results show that miRNA-loaded EVs statistically significantly improved hind limb motor function among SCI rats in the contusion model at the middle and late stages as compared with EVs. EVs could be used to treat spinal cord contusion, compression, and transection (Mu et al., 2021). Furthermore, they could treat spinal cord ischemia/reperfusion injury because of their ability to pass the blood-spinal barrier (Wu et al., 2022). A meta-analysis showed that EVs were more effective in treating contusion models as compared with compression models (Yi and Wang, 2021). Zhang found that MSC-derived EVs could inhibit inflammation and apoptosis by suppressing the PTEN and NF-κB signal (Zhang M. et al., 2021). Li found that MSC-derived EVs could activate ERK1/2, CREB, and STAT3, which play a critical part in the survival of neurons and the regeneration of axons (Li et al., 2018). Our network meta-analysis findings show that EVs derived from miRNA-modified MSCs can significantly improve hind limb motor function in SCI rats. Our study likewise found that miRNA-loaded EVs were more effective in treating contusion models than compression models. These results may be associated with the miRNA species found in EVs. Even so, these results could be partly explained by the fact that only three articles used the compression model and eight articles used the contusion model. On the other hand, it may be because the compression model is more unstable and prone to various biases, while the contusion model, as a classical SCI model, is more stable, so that the risk of various biases is relatively low in the process of modeling or treatment. However, direct comparative evidence is still needed to verify these results. Moreover, we found that miRNA-loaded EVs were more effective than EVs in treating SCI, consistent with the results of all included studies (Li et al., 2018, 2020; Yu et al., 2019; Huang et al., 2020; Chen et al., 2021; Zhang M. et al., 2021). The SUCRA ranking graph suggested that miRNA-loaded EVs may be most effective for the treatment of contusion models at the early, middle, and late stages. Therefore, miRNA-loaded EVs have tremendous therapeutic potential within SCI. Although there is no clear consensus with respect to SCI models, our study found that miRNA-loaded EVs are effective in compression and contusion models. These results meaningfully inform preclinical studies.
Strengths and limitations
In terms of motor function scores on day 28 after SCI, this study found that miRNA-modified EVs therapy was effective in improving motor function scores compared with EVs alone or control therapy, and this is consistent with a recent study by Hu et al. (2021). However, our network meta-analysis also investigated motor function scores on day 3 and day 14 after SCI, whereas the recent meta-analysis only examined motor function scores on day 28. The results of our network meta-analysis indicate that miRNA-modified EVs therapy is more effective in improving motor function scores than EVs alone or control therapy in the early, middle and late stages of SCI. Therefore, the conclusions of our study are more comprehensive. To the best of our knowledge, this network meta-analysis is the first to compare the efficacy of different administered doses of EVs and miRNA-loaded EVs in different SCI models. Three nodes were selected for the network meta-analysis according to the pathological stage of the SCI (day 3 in the subacute stage, day 14 in the subacute and intermediate stages, and day 28 in the intermediate stage) (Rowland et al., 2008). Thus, we comprehensively compared the efficacy of administered EVs and miRNA-loaded EVs with respect to administered doses and different SCI models. The internationally recognized SYRCLE risk of bias tool was implemented to assay the quality of the included studies (Hooijmans et al., 2014). All included studies were of high methodological quality and had a low associated risk with respect to many items.
However, we acknowledge some limitations of this network meta-analysis. First, the anesthetic used for surgery and miRNA were not specified. Second, the number of included studies and their associated sample sizes are modest, which may pose a risk of bias. Third, all included studies had an unclear risk of blinding with respect to performance bias. Fourth, the BBB score was the only index of efficacy evaluation. This score is not sufficiently thorough or comprehensive, though it has remarkable objectivity. Fifth, using the SUCRA ranking graph to estimate ranking probabilities has inherent limitations and the results should be interpreted with caution (Salanti et al., 2011). Sixth, we only included SCI model of rats in the study. We had ever tried to extend the proposed analyses to the other studies, and to make a comparison with the results obtained in rats. However, we found that in addition to the SCI rats model, the other is SCI model in mice. The measure of motor function in mice is the Basso Mouse Scale for Locomotion (BMS), which is different from the Basso, Beattie, Bresnahan Locomotor Rating Scale (BBB) in rats. A study (Basso et al., 2006) showed that the recovery of motor function in mice is different from that in rats, and different behavioral indicators should be used. Moreover, the number of studies using the SCI mouse model was too small to conduct a network meta-analysis. Therefore, we included SCI model of rats in the study. Seventh, different miRNAs play different roles in SCI, including inhibiting inflammation, promoting axonal regeneration and promoting angiogenesis. However, in the complex environment in vivo, different miRNAs may exert direct or indirect effects on inhibiting inflammation, promoting axonal regeneration and promoting angiogenesis, which is not clear in all the studies included so far. Nevertheless, what we can identify is the macroscopic role they play (i.e., BBB motor function assessment), and therefore, we collected BBB functional score data to perform network meta-analysis. Even if these factors can affect the results of the network meta-analysis, this is inevitable. In conclusion, we consider that these factors may affect the results of the network meta-analysis, but the impact is not significant.
Impact on future studies
Preclinical studies are essential for the application of interventions to clinical studies. Translating these studies in animal models to the delivery and effects of such an allogeneic source in human SCI therapies should focus on the difference between animal models and human SCI, such as the characteristics of disease, drug administration time, and drug efficacy. While it has shown that MSC-derived EVs could limit rejection in allogeneic transplantation, it is still necessary to carefully consider whether it is autologous or allogeneic when selecting their source (Lee et al., 2014). Many studies have compared the efficacy of EVs and miRNA-loaded EVs for the treatment of SCI. However, to the best of our knowledge, no trials have directly compared different EVs' doses of EVs and miRNA-loaded EVs. There are likewise no trials directly comparing the efficacy of EVs and miRNAs-loaded EVs in different SCI models. These respective efficacies can be compared in future studies. Moreover, the studies included in this network meta-analysis only measured efficacy indicators. None of the studies reported on safety indicators. Therefore, safety should be considered in future relevant preclinical studies in order to avoid adverse reactions upon clinical application.
Conclusion
Our results indicate that miRNA-loaded EVs at 200 μg dosages may be the potential choice for the treatment of SCI. Moreover, miRNAs-loaded EVs may be the potential choice in contusion. However, there are some risks of bias in our included study. The mechanisms underlying the efficacy of EVs and the requirement for further clinical trials remain unclear. Therefore, additional preclinical trials are necessary to directly compare the efficacy of different therapeutic EVs' doses as well as the implementation of miRNA-loaded EVs in different SCI models. Such preclinical trials are beneficial in clinical transformation.
Data availability statement
The original contributions presented in the study are included in the article/Supplementary material, further inquiries can be directed to the corresponding authors.
Author contributions
ZY first presented the idea and designed the outline of the article. JR and ZL were responsible for all data extraction and analysis. The first version was written by ZY. FL was involved in article revision. The final version was revised by CC. All of authors reviewed and approved the final article proof for submission.
Conflict of interest
The authors declare that the research was conducted in the absence of any commercial or financial relationships that could be construed as a potential conflict of interest.
Publisher's note
All claims expressed in this article are solely those of the authors and do not necessarily represent those of their affiliated organizations, or those of the publisher, the editors and the reviewers. Any product that may be evaluated in this article, or claim that may be made by its manufacturer, is not guaranteed or endorsed by the publisher.
Supplementary material
The Supplementary Material for this article can be found online at: https://www.frontiersin.org/articles/10.3389/fnins.2022.989295/full#supplementary-material
References
Ahuja, C. S., Wilson, J. R., Nori, S., Kotter, M. R. N., Druschel, C., Curt, A., et al. (2017). Traumatic spinal cord injury. Nat. Rev. Dis. Primers 3, 17018. doi: 10.1038/nrdp.2017.18
Basso, D. M., Fisher, L. C., Anderson, A. J., Jakeman, L. B., McTigue, D. M., and Popovich, P. G. (2006). Basso Mouse Scale for locomotion detects differences in recovery after spinal cord injury in five common mouse strains. J. Neurotrauma 23, 635–659. doi: 10.1089/neu.2006.23.635
Chen, Y., Tian, Z., He, L., Liu, C., Wang, N., Rong, L., et al. (2021). Exosomes derived from miR-26a-modified MSCs promote axonal regeneration via the PTEN/AKT/mTOR pathway following spinal cord injury. Stem Cell Res. Ther. 12, 224. doi: 10.1186/s13287-021-02282-0
Cipriani, A., Higgins, J. P., Geddes, J. R., and Salanti, G. (2013). Conceptual and technical challenges in network meta-analysis. Ann Intern Med. 159, 130–137. doi: 10.7326/0003-4819-159-2-201307160-00008
Dumbrava, D. A., Surugiu, R., Börger, V., Ruscu, M., Tertel, T., Giebel, B., et al. (2022). Mesenchymal stromal cell-derived small extracellular vesicles promote neurological recovery and brain remodeling after distal middle cerebral artery occlusion in aged rats. Geroscience 44, 293–310. doi: 10.1007/s11357-021-00483-2
Fehlings, M. G., Badhiwala, J. H., Ahn, H., Farhadi, H. F., Shaffrey, C. I., Nassr, A., et al. (2021a). Safety and efficacy of riluzole in patients undergoing decompressive surgery for degenerative cervical myelopathy (CSM-Protect): a multicentre, double-blind, placebo-controlled, randomised, phase 3 trial. Lancet Neurol. 20, 98–106. doi: 10.1016/S1474-4422(20)30407-5
Fehlings, M. G., Chen, Y., Aarabi, B., Ahmad, F., Anderson, K. D., Dumont, T., et al. (2021b). A randomized controlled trial of local delivery of a rho inhibitor (VX-210) in patients with acute traumatic cervical spinal cord injury. J. Neurotrauma 38, 2065–2072. doi: 10.1089/neu.2020.7096
Gu, J., Jin, Z. S., Wang, C. M., Yan, X. F., Mao, Y. Q., and Chen, S. (2020). Bone marrow mesenchymal stem cell-derived exosomes improves spinal cord function after injury in rats by activating autophagy. Drug Des. Devel. Ther. 14, 1621–1631. doi: 10.2147/DDDT.S237502
Guo, S., Perets, N., Betzer, O., Ben-Shaul, S., Sheinin, A., Michaelevski, I., et al. (2019). Intranasal delivery of mesenchymal stem cell derived exosomes loaded with phosphatase and tensin homolog sirna repairs complete spinal cord injury. ACS Nano 13, 10015–10028. doi: 10.1021/acsnano.9b01892
He, X., Zhang, J., Guo, Y., Yang, X., Huang, Y., and Hao, D. (2022). Exosomal miR-9-5p derived from BMSCs alleviates apoptosis, inflammation and endoplasmic reticulum stress in spinal cord injury by regulating the HDAC5/FGF2 axis. Mol. Immunol. 145, 97–108. doi: 10.1016/j.molimm.2022.03.007
Higgins, J.P., Jackson, D., Barrett, J.K., Lu, G., Ades, A.E., and White, I.R. (2012). Consistency and inconsistency in network meta-analysis: concepts and models for multi-arm studies. Res Synth Methods. 3, 98–110. doi: 10.1002/jrsm.1044
Hooijmans, C. R., Rovers, M. M., de Vries, R. B., Leenaars, M., Ritskes-Hoitinga, M., and Langendam, M. W. (2014). SYRCLE's risk of bias tool for animal studies. BMC Med Res Methodol. 14, 43. doi: 10.1186/1471-2288-14-43
Hu, M., Cao, Z., and Jiang, D. (2021). The effect of miRNA-modified exosomes in animal models of spinal cord injury: a meta-analysis. Front. Bioeng. Biotechnol. 9, 819651. doi: 10.3389/fbioe.2021.819651
Huang, J. H., Xu, Y., Yin, X. M., and Lin, F. Y. (2020). Exosomes derived from miR-126-modified MSCs promote angiogenesis and neurogenesis and attenuate apoptosis after spinal cord injury in rats. Neuroscience 424, 133–145. doi: 10.1016/j.neuroscience.2019.10.043
Huang, L., Fu, C., Xiong, F., He, C., and Wei, Q. (2021). Stem cell therapy for spinal cord injury. Cell Transplant. 30, 963689721989266. doi: 10.1177/0963689721989266
Huang, T., Jia, Z., Fang, L., Cheng, Z., Qian, J., Xiong, F., et al. (2022). Extracellular vesicle-derived miR-511-3p from hypoxia preconditioned adipose mesenchymal stem cells ameliorates spinal cord injury through the TRAF6/S1P axis. Brain Res. Bull. 180, 73–85. doi: 10.1016/j.brainresbull.2021.12.015
Huang, W., Lin, M., Yang, C., Wang, F., Zhang, M., Gao, J., et al. (2021). Rat bone mesenchymal stem cell-derived exosomes loaded with miR-494 promoting neurofilament regeneration and behavioral function recovery after spinal cord injury. Oxid. Med. Cell. Longev. 2021, 1634917. doi: 10.1155/2021/1634917
Jeppesen, D. K., Fenix, A. M., Franklin, J. L., Higginbotham, J. N., Zhang, Q., Zimmerman, L. J., et al. (2019). Reassessment of exosome composition. Cell 177, 428–445 e418. doi: 10.1016/j.cell.2019.02.029
Kabu, S., Gao, Y., Kwon, B. K., and Labhasetwar, V. (2015). Drug delivery, cell-based therapies, and tissue engineering approaches for spinal cord injury. J. Control. Release 219, 141–154. doi: 10.1016/j.jconrel.2015.08.060
Kalra, H., Drummen, G. P., and Mathivanan, S. (2016). Focus on extracellular vesicles: introducing the next small big thing. Int. J. Mol. Sci. 17, 170. doi: 10.3390/ijms17020170
Lee, M., Jeong, S. Y., Ha, J., Kim, M., Jin, H. J., Kwon, S. J., et al. (2014). Low immunogenicity of allogeneic human umbilical cord blood-derived mesenchymal stem cells in vitro and in vivo. Biochem. Biophys. Res. Commun. 446, 983–989. doi: 10.1016/j.bbrc.2014.03.051
Li, C., Li, X., Zhao, B., and Wang, C. (2020). Exosomes derived from miR-544-modified mesenchymal stem cells promote recovery after spinal cord injury. Arch. Physiol. Biochem. 126, 369–375. doi: 10.1080/13813455.2019.1691601
Li, D., Zhang, P., Yao, X., Li, H., Shen, H., Li, X., et al. (2018). Exosomes derived from miR-133b-modified mesenchymal stem cells promote recovery after spinal cord injury. Front. Neurosci. 12, 845. doi: 10.3389/fnins.2018.00845
Liau, L. L., Looi, Q. H., Chia, W. C., Subramaniam, T., Ng, M. H., and Law, J. X. (2020). Treatment of spinal cord injury with mesenchymal stem cells. Cell Biosci. 10, 112. doi: 10.1186/s13578-020-00475-3
Liu, J., Lin, M., Qiao, F., and Zhang, C. (2022). Exosomes derived from lncRNA TCTN2-modified mesenchymal stem cells improve spinal cord injury by miR-329-3p/IGF1R Axis. J. Mol. Neurosci. 72, 482–495. doi: 10.1007/s12031-021-01914-7
Liu, L. J. W., Rosner, J., and Cragg, J. J. (2020). Journal club: high-dose methylprednisolone for acute traumatic spinal cord injury: a meta-analysis. Neurology 95, 272–274. doi: 10.1212/WNL.0000000000009263
Liu, W., Wang, Y., Gong, F., Rong, Y., Luo, Y., Tang, P., et al. (2019). Exosomes derived from bone mesenchymal stem cells repair traumatic spinal cord injury by suppressing the activation of A1 neurotoxic reactive astrocytes. J. Neurotrauma 36, 469–484. doi: 10.1089/neu.2018.5835
Liu, W. Z., Ma, Z. J., Li, J. R., and Kang, X. W. (2021). Mesenchymal stem cell-derived exosomes: therapeutic opportunities and challenges for spinal cord injury. Stem Cell Res. Ther. 12, 102. doi: 10.1186/s13287-021-02153-8
McDonald, J. W., and Sadowsky, C. (2002). Spinal-cord injury. Lancet 359, 417–425. doi: 10.1016/S0140-6736(02)07603-1
Mu, J., Wu, J., Cao, J., Ma, T., Li, L., Feng, S., et al. (2021). Rapid and effective treatment of traumatic spinal cord injury using stem cell derived exosomes. Asian J. Pharm. Sci. 16, 806–815. doi: 10.1016/j.ajps.2021.10.002
Nie, H., and Jiang, Z. (2021). Bone mesenchymal stem cell-derived extracellular vesicles deliver microRNA-23b to alleviate spinal cord injury by targeting toll-like receptor TLR4 and inhibiting NF-kappaB pathway activation. Bioengineered 12, 8157–8172. doi: 10.1080/21655979.2021.1977562
Page, M. J., McKenzie, J. E., Bossuyt, P. M., Boutron, I., Hoffmann, T. C., Mulrow, C. D., et al. (2021). The PRISMA 2020 statement: an updated guideline for reporting systematic reviews. Bmj 372, n71. doi: 10.1136/bmj.n71
Pan, D., Li, Y., Yang, F., Lv, Z., Zhu, S., Shao, Y., et al. (2021). Increasing toll-like receptor 2 on astrocytes induced by Schwann cell-derived exosomes promotes recovery by inhibiting CSPGs deposition after spinal cord injury. J. Neuroinflamm. 18, 172. doi: 10.1186/s12974-021-02215-x
Ramakonar, H., and Fehlings, M. G. (2021). 'Time is Spine': new evidence supports decompression within 24 h for acute spinal cord injury. Spinal Cord 59, 933–934. doi: 10.1038/s41393-021-00654-0
Ren, Z., Qi, Y., Sun, S., Tao, Y., and Shi, R. (2020). Mesenchymal stem cell-derived exosomes: hope for spinal cord injury repair. Stem Cells Dev. 29, 1467–1478. doi: 10.1089/scd.2020.0133
Rong, Y., Liu, W., Wang, J., Fan, J., Luo, Y., Li, L., et al. (2019). Neural stem cell-derived small extracellular vesicles attenuate apoptosis and neuroinflammation after traumatic spinal cord injury by activating autophagy. Cell Death Dis. 10, 340. doi: 10.1038/s41419-019-1571-8
Rowland, J. W., Hawryluk, G. W., Kwon, B., and Fehlings, M. G. (2008). Current status of acute spinal cord injury pathophysiology and emerging therapies: promise on the horizon. Neurosurg Focus 25, E2. doi: 10.3171/foc.2008.25.11.E2
Salanti, G. (2012). Indirect and mixed-treatment comparison, network, or multiple-treatments meta-analysis: many names, many benefits, many concerns for the next generation evidence synthesis tool. Res. Synth. Methods 3, 80–97. doi: 10.1002/jrsm.1037
Salanti, G., Ades, A. E., and Ioannidis, J. P. (2011). Graphical methods and numerical summaries for presenting results from multiple-treatment meta-analysis: an overview and tutorial. J. Clin. Epidemiol. 64, 163–171. doi: 10.1016/j.jclinepi.2010.03.016
Sheng, Y., Zhou, X., Wang, J., Shen, H., Wu, S., Guo, W., et al. (2021). MSC derived EV loaded with miRNA-22 inhibits the inflammatory response and nerve function recovery after spinal cord injury in rats. J. Cell. Mol. Med. 25, 10268–10278. doi: 10.1111/jcmm.16965
Stang, A. (2010). Critical evaluation of the Newcastle-Ottawa scale for the assessment of the quality of nonrandomized studies in meta-analyses. Eur. J. Epidemiol. 25, 603–605. doi: 10.1007/s10654-010-9491-z
Thery, C., Witwer, K. W., Aikawa, E., Alcaraz, M. J., Anderson, J. D., Andriantsitohaina, R., et al. (2018). Minimal information for studies of extracellular vesicles 2018 (MISEV2018): a position statement of the International Society for Extracellular Vesicles and update of the MISEV2014 guidelines. J. Extracell Vesicles 7, 1535750. doi: 10.1080/20013078.2018.1535750
Tu, Y. K., and Hsueh, Y. H. (2020). Extracellular vesicles isolated from human olfactory ensheathing cells enhance the viability of neural progenitor cells. Neurol. Res. 42, 959–967. doi: 10.1080/01616412.2020.1794371
van Niel, G., Carter, D. R. F., Clayton, A., Lambert, D. W., Raposo, G., and Vader, P. (2022). Challenges and directions in studying cell-cell communication by extracellular vesicles. Nat. Rev. Mol. Cell Biol. 23, 369–82. doi: 10.1038/s41580-022-00460-3
Wang, Y., Han, T., Guo, R., Song, P., Liu, Y., Wu, Z., et al. (2022). Micro-RNA let-7a-5p derived from mesenchymal stem cell-derived extracellular vesicles promotes the regrowth of neurons in spinal-cord-injured rats by targeting the HMGA2/SMAD2 Axis. Front. Mol. Neurosci. 15, 850364. doi: 10.3389/fnmol.2022.850364
Wiklander, O. P., Nordin, J. Z., O'Loughlin, A., Gustafsson, Y., Corso, G., Mäger, I., et al. (2015). Extracellular vesicle in vivo biodistribution is determined by cell source, route of administration and targeting. J. Extracell. Vesicles 4, 26316. doi: 10.3402/jev.v4.26316
Wu, C., Zhu, Q., Yao, Y., Shi, Z., Jin, C., and Chen, L. (2022). Exosome miR-23a-3p from osteoblast alleviates spinal cord ischemia/reperfusion injury by down-regulating KLF3-activated CCNL2 transcription. Dev. Neurosci. 44, 121–30. doi: 10.1159/000521167
Yang, Z., Shi, J., Xie, J., Wang, Y., Sun, J., Liu, T., et al. (2020). Large-scale generation of functional mRNA-encapsulating exosomes via cellular nanoporation. Nat. Biomed. Eng. 4, 69–83. doi: 10.1038/s41551-019-0485-1
Yi, H., and Wang, Y. (2021). A meta-analysis of exosome in the treatment of spinal cord injury. Open Med. 16, 1043–1060. doi: 10.1515/med-2021-0304
Yu, T., Zhao, C., Hou, S., Zhou, W., Wang, B., and Chen, Y. (2019). Exosomes secreted from miRNA-29b-modified mesenchymal stem cells repaired spinal cord injury in rats. Braz. J. Med. Biol. Res. 52, e8735. doi: 10.1590/1414-431x20198735
Yuan, X., Wu, Q., Wang, P., Jing, Y., Yao, H., Tang, Y., et al. (2019). Exosomes derived from pericytes improve microcirculation and protect blood-spinal cord barrier after spinal cord injury in mice. Front. Neurosci. 13, 319. doi: 10.3389/fnins.2019.00319
Zhang, A., Bai, Z., Yi, W., Hu, Z., and Hao, J. (2021). Overexpression of miR-338-5p in exosomes derived from mesenchymal stromal cells provides neuroprotective effects by the Cnr1/Rap1/Akt pathway after spinal cord injury in rats. Neurosci. Lett. 761, 136124. doi: 10.1016/j.neulet.2021.136124
Zhang, B., Lin, F., Dong, J., Liu, J., Ding, Z., and Xu, J. (2021). Peripheral macrophage-derived exosomes promote repair after spinal cord injury by inducing local anti-inflammatory type microglial polarization via increasing autophagy. Int. J. Biol. Sci. 17, 1339–1352. doi: 10.7150/ijbs.54302
Zhang, M., Wang, L., Huang, S., and He, X. (2021). Exosomes with high level of miR-181c from bone marrow-derived mesenchymal stem cells inhibit inflammation and apoptosis to alleviate spinal cord injury. J. Mol. Histol. 52, 301–311. doi: 10.1007/s10735-020-09950-0
Keywords: spinal cord injury, extracellular vesicles, network meta-analysis, miRNAs, animal
Citation: Yang Z, Rao J, Liang Z, Xu X, Lin F, Lin Y, Wang C and Chen C (2022) Efficacy of miRNA-modified mesenchymal stem cell extracellular vesicles in spinal cord injury: A systematic review of the literature and network meta-analysis. Front. Neurosci. 16:989295. doi: 10.3389/fnins.2022.989295
Received: 08 July 2022; Accepted: 12 September 2022;
Published: 05 October 2022.
Edited by:
Jiake Xu, University of Western Australia, AustraliaReviewed by:
Karthik Prabhakara, Thermo Fisher Scientific, United StatesXiaocheng Lu, The First Affiliated Hospital of Soochow University, China
Copyright © 2022 Yang, Rao, Liang, Xu, Lin, Lin, Wang and Chen. This is an open-access article distributed under the terms of the Creative Commons Attribution License (CC BY). The use, distribution or reproduction in other forums is permitted, provided the original author(s) and the copyright owner(s) are credited and that the original publication in this journal is cited, in accordance with accepted academic practice. No use, distribution or reproduction is permitted which does not comply with these terms.
*Correspondence: Chunmei Chen, MTczMTAxMjk0OEBxcS5jb20=; Chunhua Wang, d2NobWFpbEAxMjYuY29t
†These authors have contributed equally to this work and share first authorship