- 1The First Affiliated Hospital of Dalian Medical University, Dalian, China
- 2Department of Histology and Embryology, College of Basic Medicine, Dalian Medical University, Dalian, China
Diabetic retinopathy (DR) is a common complication of diabetes mellitus and has been considered a microvascular disease for a long time. However, recent evidence suggests that diabetic retinal neurodegeneration (DRN), which manifests as neuronal apoptosis, a decrease in optic nerve axons, and reactive gliosis, occurs prior to retinal microvascular alterations. Thioredoxin-interacting protein (TXNIP) is an endogenous inhibitor of thioredoxin (Trx), and it acts by inhibiting its reducing capacity, thereby promoting cellular oxidative stress. In addition, it participates in regulating multiple signaling pathways as a member of the α-arrestin family of proteins. Accumulating evidence suggests that TXNIP is upregulated in diabetes and plays a pivotal role in the pathophysiological process of DR. In this review, we summarized the role of TXNIP in DRN, aiming to provide evidence for DR treatment in the future.
Introduction
Diabetic retinopathy (DR) is a leading complication of diabetes and affects millions of individuals globally (Cheung et al., 2010). Earlier studies have linked DR to microvascular disease. DR is classified and treated clinically according to its vascular features (Hammes et al., 2011), which include microaneurysms, dot blot bleeding, and hard exudates in non-proliferative DR (NPDR), and retinal neovascularization and vitreous hemorrhage in proliferative DR (PDR) (Wilkinson et al., 2003). The treatment of DR focuses on vascular phenotypes by injecting anti-vascular endothelial growth factor (VEGF), and the effects are limited (Antonetti et al., 2021).
Structural and functional abnormalities of the retina occur in the early stage of DR, such as a decrease in optic nerve axons and reactive gliosis, which is described as diabetic retinal neurodegeneration (DRN) (Lynch and Abràmoff, 2017). An increasing number of studies have shown that DRN plays a pivotal role in the pathogenesis of DR and occurs earlier and more insidiously than microvascular lesions (Jonsson et al., 2016; Kadłubowska et al., 2016). There is considerable evidence that DRN contributes to retinal microvascular abnormalities in the early stage, suggesting that DRN is the precursor to some extent (Rossino et al., 2019). Our previous study revealed that early control of DRN is highly effective in delaying the progression of DR (Ren et al., 2017).
Thioredoxin-interacting protein (TXNIP) was first refined from leukemia cells (HL-60) treated with 1,25-dihydroxyvitamin D3 (vitamin D3) and named vitamin D3-upregulated protein-1 (VDUP1) (Chen and DeLuca, 1994). It was later identified as a protein that binds to thioredoxin (Trx) and inhibits its redox function (Schulze et al., 2004). Hence, it is also known as thioredoxin-binding protein-2 (TBP-2). Human TXNIP is a universally expressed protein that contains 391 amino acid residues. The TXNIP gene is encoded on chromosome 1q21.1 and is highly conserved among different species (Ludwig et al., 2001). As an endogenous inhibitor of the Trx system, cys63 and cys247 in TXNIP can form a mixed disulfide bond with mercaptan at the Trx active site and promote oxidative stress by inhibiting Trx function (Lu and Holmgren, 2014). TXNIP belongs to the α-arrestin protein family, and these intermediate scaffold proteins play an essential role in several signaling pathways (Patwari et al., 2009). Therefore, TXNIP plays many physiological roles independent of Trx binding (Spindel et al., 2012). It was previously confirmed that the downregulation of TXNIP or the upregulation of Trx reduces the production of intracellular reactive oxygen species (ROS) and alleviates retinal cell apoptosis (Ren et al., 2018). However, these mechanisms are complicated and require further investigation. This article summarizes the role of TXNIP in the pathogenesis of DRN and proposes new potential therapies based on the existing literature.
TXNIP in diabetes
Thioredoxin-interacting protein is upregulated in response to glucose and has been identified as the highest glucose-induced gene in gene expression microarrays (Shalev et al., 2002). The expression of TXNIP is increased in the retina (Singh, 2013), pancreas (Shalev et al., 2002), kidney (Han et al., 2018), heart (Shen et al., 2018), peripheral nerves (Gao et al., 2020), and many other organs in patients with diabetes.
Physiologically, TXNIP functions as a dynamic nutrient sensor and contributes substantially to the dynamic balance of glucose regulation. To mitigate excessive glucose influx into the cell, TXNIP creates a negative feedback loop to regulate glucose uptake by binding to the glucose transporter (GLUT) (Wu et al., 2013). Under energy stress or control by insulin, AMP-activated protein kinase (AMPK) and Akt can phosphorylate TXNIP and cause its degeneration respectively, thereby preventing TXNIP-mediated endocytosis of GLUT transporters and leading to an increase in glucose influx (Dykstra et al., 2021). However, under pathophysiological conditions, such as diabetes, TXNIP is continuously induced by many factors, such as high glucose levels, causes β-cell dysfunction, and breaks this feedback loop.
Translational level
The promoter of TXNIP contains multiple transcription factor binding sites, such as carbohydrate response element (ChoRE) and antioxidant element (ARE) (Dong et al., 2016). The expression of the carbohydrate-responsive element-binding protein (ChREBP) and MondoA increases when the glucose levels are high. The signal is transmitted to the nucleus, binding to ChoRE to activate the TXNIP promoter and promoting TXNIP gene transcription (Poungvarin et al., 2012; Richards et al., 2017). ChREBP is predominantly expressed in beta cells, the liver, and adipose tissue, whereas MondoA is mainly expressed in the skeletal muscle and heart (Thielen and Shalev, 2018). Forkhead box O1 transcription factor (FoxO1) competitively binds to the ChoRE sequence associated with the inhibition of TXNIP transcription (Kibbe et al., 2013; Zhong et al., 2021). Nuclear factor E2-related factor 2 (Nrf2) inhibits the expression of TXNIP and prevents its induction by high glucose and MondoA by binding to ARE (He and Ma, 2012). It has been confirmed that sulforaphane (SF), an Nrf2 activator (Russo et al., 2018), can alleviate mouse photoreceptor-derived (661w) cell apoptosis by reducing the expression of TXNIP (Lv et al., 2020).
As a branch of glycolysis, hexosamine pathway (HBP) flux increases in a chronic high glucose environment. The transcription of the TXNIP gene is mediated by HBP to some extent, which involves the recruitment of p300 histone acetyltransferase at the TXNIP promoter (Perrone et al., 2010). Moreover, Li et al. (2018) identified that p38 mitogen-activated protein kinase (MAPK) can be activated by ROS and induces TXNIP expression in the retinas of diabetic rats treated with high glucose. Streptozotocin (STZ)-induced hyperglycemia directly stimulates TXNIP expression through increased intracellular calcium ion (Ca2+) levels in the retina (Patwari et al., 2006). Insulin and glucagon-like peptide 1 (GLP-1) reduce β-cell TXNIP expression (Chen et al., 2006; Shaked et al., 2009). TXNIP can inhibit insulin production directly or by destroying the islet β-cells (Xu et al., 2013; Thielen and Shalev, 2018), forming a vicious cycle of TXNIP increase. Moreover, topical administration of GLP-1 receptor agonists has been proven to prevent retinal neurodegeneration in animal models (Hernández et al., 2016).
MicroRNAs (miRNAs) are small non-coding RNA molecules that participate in many vital biological processes by inhibiting messenger RNA (mRNAs) or by binding to the 3’untranslated region (3’UTR) of mRNA to limit protein synthesis (Krol et al., 2010). High glucose levels induce TXNIP upregulation, mediated by the downregulation of several miRNAs. Dong et al. (2016) demonstrated that microRNA-17 (miR-17) is downregulated by high glucose levels and stabilizes TXNIP. Moreover, Wang et al. (2020) showed that microRNA-20b-3p (miR-20b-3p), which inhibits inflammatory factors by inhibiting TXNIP to reduce pathological changes in the retina of DR rats, is expressed at low levels. Furthermore, high glucose levels induce the upregulation of circRNA_0084043, a type of circular RNA that mediates the downregulation of miR-128-3p in adult retinal pigment epithelial cells (ARPE-19) and causes TXNIP overexpression (Zhang et al., 2021; Figure 1A).
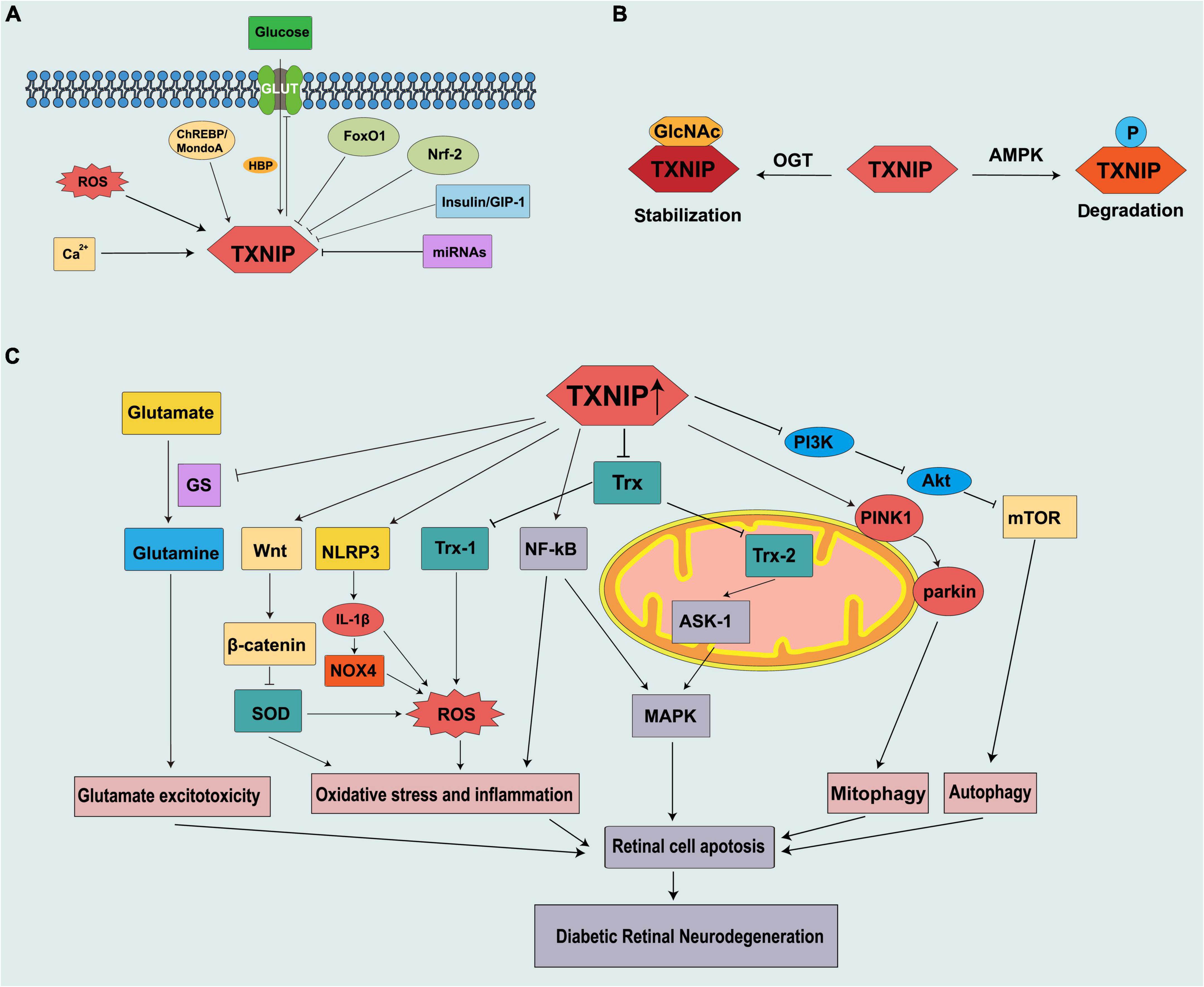
Figure 1. Schematic representation of thioredoxin-interacting protein (TXNIP) being regulated in diabetes and contributing to the pathogenesis of DRN. (A) Mechanism of TXNIP regulation at the translational level. (B) Mechanism of TXNIP regulation at the post-translational level. (C) TXNIP participates in different signaling pathways to promote DRN.
Post-translational level
Thioredoxin-interacting protein can be modified by phosphorylation and O-GlcNAcylation. These two modifications are similar in many aspects and compete with each other. Thus, they are described as the “yin-yang” model (Hart et al., 1995, 2011). Energy stress leads to the phosphorylation of TXNIP by AMPK, resulting in its rapid degradation (Wu et al., 2013). As a nutrient sensor, like TXNIP, O-GlcNAcylation is also upregulated via HBP in the diabetic retina and high glucose conditions and is involved in the development of DR (Gurel and Sheibani, 2018). A recent study reported that the O-GlcNAcylation of TXNIP is increased in β cells, and an increased level of TXNIP is also observed in a diabetes model (Filhoulaud et al., 2019; Figure 1B).
TXNIP in diabetic retinal neurodegeneration
Vascular abnormalities are the most evident clinical features of DR; however, evidence shows that retinal optic neuropathy occurs prior to vasculopathy. In db/db mice, the biological defect in the neural structure exists as early as the eighth week, which is characterized by a thinning of the outer nuclear layer (ONL) (Bogdanov et al., 2014). DRN results from neuronal apoptosis, reactive gliosis, glutamate excitotoxicity, and decreased neuroprotective factors (van Dijk et al., 2012; Chakravarthy and Devanathan, 2018). Excessive TXNIP production is observed in the retina that includes the retinal ganglion cells (RGCs) (Eissa et al., 2021), Müller cells (Devi et al., 2012), pericytes (PCs) (Devi et al., 2013), retinal pigment epithelium (RPEs) (Devi et al., 2019), and endothelial cells (ECs) (Perrone et al., 2009). However, it also has deleterious effects on these cells. TXNIP deficiency can reduce excessive autophagy and the ratio of B-cell lymphoma protein 2-associated X (Bax)/B-cell lymphoma protein 2 (Bcl-2) and inhibits retinal cell apoptosis (Yu et al., 2013). TXNIP ubiquitously exists in the cytoplasm and different organelles that include the nucleus and mitochondria, thereby affecting the function of various organelles and mediating multiple pathological processes.
TXNIP regulates oxidative stress and inflammation in RDN
The retina is a unique organ that contains high concentrations of polyunsaturated fatty acids and requires high oxygen levels. Therefore, the retina and its vascular system are more susceptible to oxidative stress. Some evidence suggests that oxidative stress is the main cause of sterile retinal inflammation in diabetes (Rodríguez et al., 2019). Oxidative stress can contribute to and result from metabolic abnormalities induced by hyperglycemia in the retina.
Cellular redox balance (reduction/oxidation) is strongly regulated by the activities of many antioxidant systems, such as the Trx, glutathione, and glutaredoxin systems (Spindel et al., 2012). Trx1 and its reductase TrxR1 are expressed in the cytoplasm and nucleus, whereas Trx2/TrxR2 is a mitochondrial subtype (Lillig and Holmgren, 2007). During cell stress, TXNIP migrates to the mitochondria and releases apoptosis signal kinase 1 (ASK1) from Trx2 capture, which results in the release of cytochrome c from the mitochondria, cleavage of caspase-3, and apoptosis (Saxena et al., 2010). Many experimental studies have demonstrated an increase in ASK1 induced by TXNIP, which is associated with retinal neurodegeneration and diabetes (Sreekumar et al., 2009; Abdelsaid et al., 2014). TXNIP also induces the activation of nuclear factor-kappa B (NF-κB), which plays a pivotal role in inflammation and colocalizes with retinal Müller cells and ECs (Perrone et al., 2009; Al-Gayyar et al., 2011). NF-κB and ASK1 activate the apoptotic p38 MAPK/c-Jun N-terminal kinase (JNK) pathway and promote retinal cell death (Al-Gayyar et al., 2011).
Thioredoxin-interacting protein is a multifunctional and inducible protein with functions independent of its combination with Trx. The NOD-like receptor protein-3 (NLRP3) inflammasome, composed of NLRP3, adaptor protein apoptosis associated speck-like protein containing a CARD (ASC), and procaspase-1, is responsible for the production and secretion of interleukin (IL)-1β (Martinon et al., 2002). IL-1β can induce retinal mitochondrial dysfunction, mitochondrial DNA damage, and apoptosis (Gu et al., 2019). IL-1β reduces the promoter activity of pri-miR-590 DNA to downregulate miR-590-3p, thereby targeting NLRP1 and activating the NADPH oxidase 4 (NOX4)-mediated pathway to promote retinal cell apoptosis. In addition, NOX4 further promotes TXNIP expression by inducing ROS (Gu et al., 2019). TXNIP plays a potential role in sterile inflammatory processes and innate immunity through the activation and releasing of IL-1β by the NLRP3 inflammasome in diabetes and oxidative stress. Furthermore, TXNIP can be induced by ROS, leading to NLRP3 activation in diabetes (Schroder et al., 2010; Lu et al., 2018). TXNIP can also activate the Wnt/β-catenin signal pathway (Zhang et al., 2021). The activation of the Wnt/β-catenin pathway downregulates the expression of antioxidant enzymes, such as superoxide dismutase (SOD)1 and SOD2, which further exacerbates cellular oxidative stress (Liu et al., 2019).
TXNIP regulates autophagy/mitophagy in RDN
Autophagy is the primary physiological process by which cells eliminate damaged organelles and defective proteins and is considered an important regulator of homeostasis (Levine and Kroemer, 2019). However, under extreme pressure, it can lead to programmed cell death (Maiuri et al., 2007). Autophagy plays a “double-edged sword” role in DR (Gong et al., 2021). The unc-51 like autophagy activating kinase 1 (ULK1) complex participates in the initiation of autophagy, and the mammalian target of rapamycin (mTOR) functions as the negative regulator of autophagy by phosphating ULK1 (Jung et al., 2009). Excessive autophagic flux is induced by retinal neuronal cells under high glucose exposure (Rosa et al., 2016; Adornetto et al., 2021). Müller cells are located from the outer layer to the inner layer of the retina. They are responsible for the communication between retinal blood vessels and neurons and provide nutrition for the retinal nerve cells. TXNIP promotes autophagy by inhibiting the PI3K/Akt/mTOR pathway in Müller cells, which leads to Müller cell damage and disease progression. A decrease in the fluorescence intensity of LC3B was observed in the retina after the knockout of TXNIP in STZ-induced diabetic rats, indicating that autophagy was downregulated (Ao et al., 2021). In addition, we observed the overexpression of Trx, which shows the inhibitory activity of TXNIP, ameliorated photoreceptor cell degeneration in DR via AMPK-mediated autophagy, and exosome secretion (Ren et al., 2022).
Mitophagy is a specialized process that removes damaged, obsolete, and dysfunctional mitochondria through lysosomal degradation. It is a cytoprotective mechanism that ensures cell survival and tissue protection (Levine and Kroemer, 2019). However, excessive mitophagy flux may lead to the damage to mitochondria, which continue to produce ROS while producing less adenosine triphosphate (ATP), leading to oxidative stress and retinal apoptosis, which eventually develops DRN (Singh et al., 2017). PINK1 accumulates on the outer membrane of depolarized mitochondria, recruits E3 ubiquitin ligase parkin, mediates ubiquitination of mitochondrial membrane proteins, and activates mitophagy (Skeie et al., 2021). TXNIP and ROS cause mitochondrial damage and fragmentation through dynamin-related protein (Drp1)-mediated mitochondrial (MT) fission and PINK1/parkin-mediated mitophagy in Müller cells and TXNIP knockout prevents these events (Devi et al., 2017). Similar results are seen for RPEs (Devi et al., 2019).
TXNIP regulates glutamate excitotoxicity in RDN
Glutamate is the most abundant and important amino acid in the nervous system. It plays an important role in synaptic transmission and maintaining the normal function of nerve cells. Glutamate metabolism is very important in maintaining a healthy retina and its dysregulation can lead to the development of DR. Moreover, under pathological conditions, such as diabetes, the accumulation of glutamate in the retina causes the overexcitation of N-methyl-D-aspartate receptors (NMDARs), which causes calcium influx and eventually leads to DRN (Yu et al., 2008). Glutamine synthetase (GS) benefits glutamate clearance through metabolizing glutamate to glutamine. Moreover, glutamate is an excitatory neurotransmitter, and it can accumulate in the retina to toxic levels due to diabetes and thus cause excitotoxicity damage to the retinal neurons (Rolev et al., 2021). Another mechanism of how glutamate excitotoxicity mediates neurodegeneration in the retina is partly through nitric oxide (NO), which causes mitochondria fragmentation in RGCs, leading to NMDA receptor upregulation and enhanced oxidative stress, thus triggering neurodegeneration (Nguyen et al., 2011). Zhou et al. (2016) reported that TXNIP suppresses the expression of GS by IL-1β, which leads to Müller cell dysfunction and further induces ganglion cell apoptosis (Figure 1C).
TXNIP: as novel potential therapeutic target for diabetic retinopathy
Substantial evidence suggests that hyperglycemia promotes the expression of TXNIP through multiple pathways. An increased TXNIP level has emerged as a novel key factor in DRN (Figure 1), and the inhibition of TXNIP expression in vivo and in vitro effectively prevents early pathology of DR (Perrone et al., 2010; Ren et al., 2018). We summarized the potential moderators targeting TXNIP to treat DRN by blocking the expression and downstream pathways of TXNIP (Table 1).
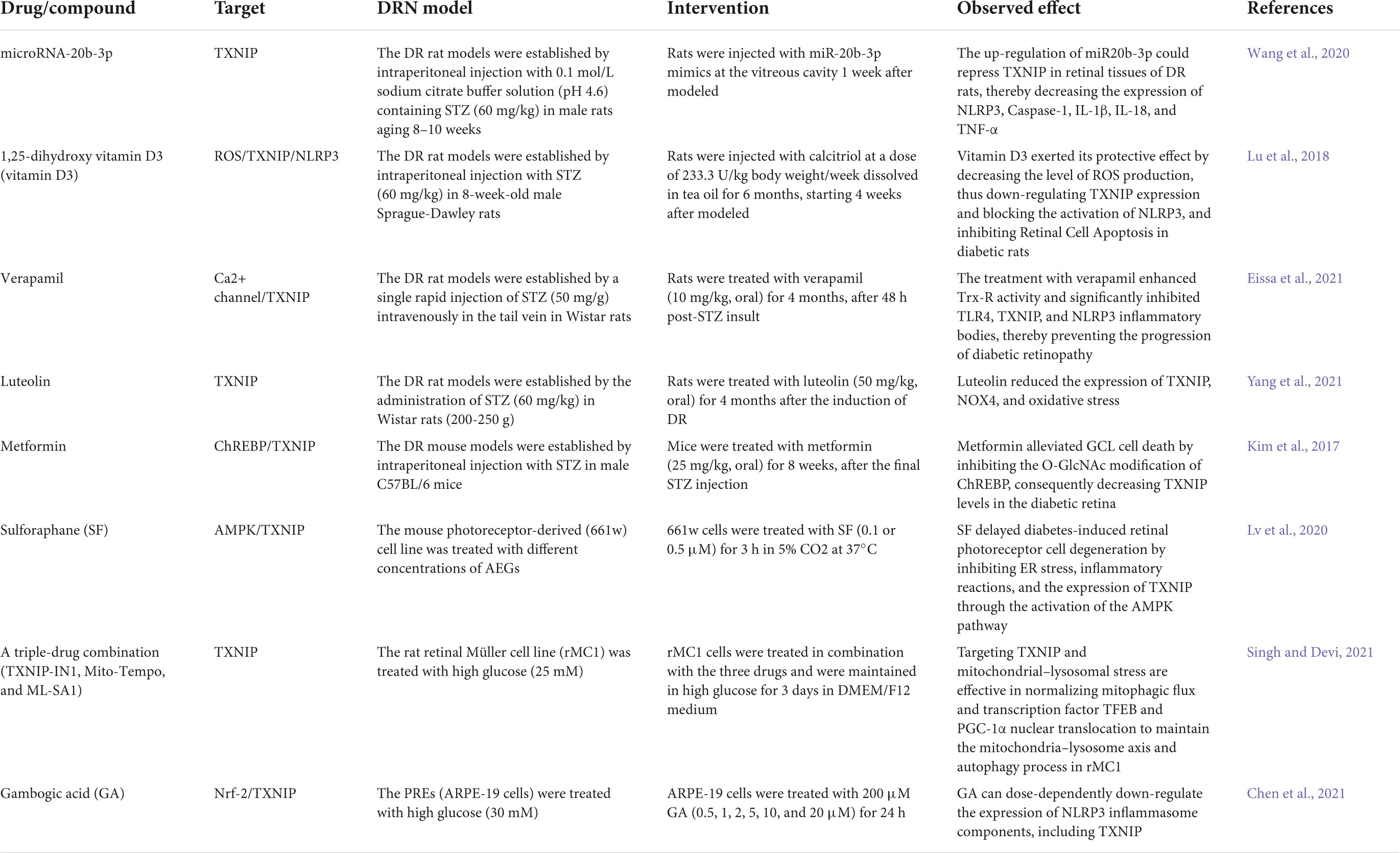
Table 1. List of drugs/compounds that have been identified to alleviate diabetic retinal neurodegeneration (DRN) in experimental models.
Given the beneficial effects of TXNIP deficiency, gene therapy using vectors, such as lentivirus and adeno-associated viral vectors, to transfer genes to the retina may be another choice. Nucleic acid constructs containing a proximal TXNIP promoter linked to a redox gene or shRNA that reduces oxidative stress and inflammation may be used to treat DR (Lalit et al., 2018). Clustered regularly interspaced short palindromic repeats (CRISPR)-associated 9 (CRISPR/cas9) an emerging technology, is used for genome editing in animals and cultured cells. Recent reports showed its progress in clinical trials of some genetic diseases (Arnold, 2021). Devi et al. (2017) applied the CRISPR/cas9 method to knockout TXNIP and observed that it can prevent high glucose-induced Müller cell line rMC1 mitochondrial damage and mitochondrial autophagy.
Thioredoxin-interacting protein is notably upregulated and is not restricted to specific cell types in the diabetic retina; therefore, intraocular administration is theoretically feasible. Nevertheless, the dose needs to be controlled within the physiological level and varies from person to person. A report also shows the adverse effects of excessive downregulation of TXNIP. In a study on age-related macular degeneration (AMD), researchers observed that sustained oxidative stress mediates TXNIP downregulation and disrupts RPE cell function, thereby aggravating AMD (Ji Cho et al., 2019).
The inhibition of TXNIP promotes endogenous cell mass and insulin production, thereby becoming an attractive new candidate drug target for diabetes (Shalev, 2014; Wondafrash et al., 2020). Promising results have been obtained in experimental models of diabetes with anti-TXNIP agents, such as S-Equol (Chen et al., 2020), SRI-37330 (Thielen et al., 2020), MiR-17-5p (Liu et al., 2021), and fluoromethyl ketone (FMK) (Han et al., 2019). Moreover, TXNIP also participates in microangiopathy in DR, such as neovascularization (Duan et al., 2018), vascular permeability, and inner blood-retinal barrier (iBRB) breakdown (Perrone et al., 2009). Extensive literature is available regarding promising drugs that prevent retinal microvascular dysfunction induced by diabetes, directed at TXNIP. Minocycline and fenofibrate have demonstrated a protective effect on retinal vascular permeability by reducing ROS-induced TXNIP expression in diabetes (Chen et al., 2017; Li et al., 2018). Tang et al. (2021) revealed that melatonin, an ancient antioxidant, could maintain the iBRB integrity by upregulating the expression of tight junction proteins via inhibiting the p38MAPK/TXNIP/NF-κB pathway, thus decreasing the production of inflammatory factors to reduce retinal damage. Given the similar pharmacological effects of these drugs on inhibiting TXNIP expression, repurposing them for DRN treatment may be a direction for future research. Since DRN precedes microangiopathy in DR, revealing the mechanism of DRN is of great significance for the early screening and intervention of DR. We have attempted to review the evidence from experimental models that support the vital role of TXNIP in the etiology of DRN and potential treatment targeting TXNIP. Therefore, there is an urgent need to develop and evaluate the efficacy of these therapies in clinical trials. In summary, TXNIP played a vital in DRN and it could be a novel potential therapeutic target for DR.
Author contributions
CL and XR conceived of this review topic. WD and CL retrieved and analyzed relevant documents. CL prepared the illustrations and wrote the manuscript. XR revised the manuscript. ZL, LK, and XR provided the funding support. All authors listed have made a substantial, direct, and intellectual contribution to the work, and approved it for publication.
Funding
This work was supported by grant no. 31371218 from the National Natural Science Foundation of China and grant no. 2020-BS-189 from the Natural Science Foundation of Liaoning Province and the Liaoning Provincial Program for Top Discipline of Basic Medical Sciences.
Conflict of interest
The authors declare that the research was conducted in the absence of any commercial or financial relationships that could be construed as a potential conflict of interest.
Publisher’s note
All claims expressed in this article are solely those of the authors and do not necessarily represent those of their affiliated organizations, or those of the publisher, the editors and the reviewers. Any product that may be evaluated in this article, or claim that may be made by its manufacturer, is not guaranteed or endorsed by the publisher.
Abbreviations
DR, Diabetic retinopathy; DRN, diabetic retinal neurodegeneration; TXNIP, Thioredoxin-interacting protein; Trx, thioredoxin; NPDR, non-proliferative DR; PDR, proliferative DR; VEGF, anti-vascular endothelial growth factor; HL-60, Leukemia cell-60; vitamin D3, 1,25-dihydroxyvitamin D3; VDUP1, vitamin D3-upregulated protein-1; TBP-2, thioredoxin-binding protein-2; ROS, reactive oxygen species; ChoRE, carbohydrate response element; ARE, antioxidant element; ChREBP, carbohydrate-responsive element-binding protein; FoxO1, Forkhead box O1 transcription factor; Nrf2, Nuclear factor E2-related factor 2; SF, sulforaphane; HBP, hexosamine pathway; MAPK, mitogen-activated protein kinase; Ca2+, calcium ion; miRNAs, MicroRNAs; 3'UTR, 3'untranslated region; MmiR-17, icroRNA-17; miR-20b-3p, MicroRNA-20b-3p; ARPE-19, adult retinal pigment epithelial cells; AMPK, AMP-activated protein kinase; ONL, outer nuclear layer; RGC, retinal ganglion cell; PC, pericytes; RPE, retinal pigment epithelium; EC, endothelial cell; ASK1, apoptosis signal kinase 1; NLRP3, NOD-like receptor protein-3; ASC, apoptosis associated speck-like protein containing a CARD; IL, interleukin; NOX4, NADPH oxidase 4; mTOR, mammalian target of rapamycin; NMDAR, N-methyl-D-aspartate receptor; GS, Glutamine synthetase; NO, nitric oxide; CRISPR/cas9, clustered regularly interspaced short palindromic repeats/CRISPR-associated 9; AMD, age-related macular degeneration; AFMK, fluoromethyl ketone; iBRB, inner blood-retinal barrier.
References
Abdelsaid, M. A., Matragoon, S., Ergul, A., and El-Remessy, A. B. (2014). Deletion of thioredoxin interacting protein (TXNIP) augments hyperoxia-induced vaso-obliteration in a mouse model of oxygen induced-retinopathy. PLoS One 9:e110388. doi: 10.1371/journal.pone.0110388
Adornetto, A., Gesualdo, C., Laganà, M. L., Trotta, M. C., Rossi, S., and Russo, R. (2021). Autophagy: a novel pharmacological target in diabetic retinopathy. Front. Pharmacol. 12:695267. doi: 10.3389/fphar.2021.695267
Al-Gayyar, M. M., Abdelsaid, M. A., Matragoon, S., Pillai, B. A., and El-Remessy, A. B. (2011). Thioredoxin interacting protein is a novel mediator of retinal inflammation and neurotoxicity. Br. J. Pharmacol. 164, 170–180. doi: 10.1111/j.1476-5381.2011.01336.x
Antonetti, D. A., Silva, P. S., and Stitt, A. W. (2021). Current understanding of the molecular and cellular pathology of diabetic retinopathy. Nat. Rev. Endocrinol. 17, 195–206. doi: 10.1038/s41574-020-00451-4
Ao, H., Li, H., Zhao, X., Liu, B., and Lu, L. (2021). TXNIP positively regulates the autophagy and apoptosis in the rat müller cell of diabetic retinopathy. Life Sci. 267:118988. doi: 10.1016/j.lfs.2020.118988
Arnold, C. (2021). What’s new in clinical CRISPR? Nat. Med. 27, 184–185. doi: 10.1038/s41591-020-01222-4
Bogdanov, P., Corraliza, L., Villena, J. A., Carvalho, A. R., Garcia-Arumí, J., Ramos, D., et al. (2014). The db/db mouse: a useful model for the study of diabetic retinal neurodegeneration. PLoS One 9:e97302. doi: 10.1371/journal.pone.0097302
Chakravarthy, H., and Devanathan, V. (2018). Molecular mechanisms mediating diabetic retinal neurodegeneration: potential research avenues and therapeutic targets. J. Mol. Neurosci. 66, 445–461. doi: 10.1007/s12031-018-1188-x
Chen, J., Couto, F. M., Minn, A. H., and Shalev, A. (2006). Exenatide inhibits beta-cell apoptosis by decreasing thioredoxin-interacting protein. Biochem. Biophys. Res. Commun. 346, 1067–1074. doi: 10.1016/j.bbrc.2006.06.027
Chen, J., Li, L., Zhou, Y., Zhang, J., and Chen, L. (2021). Gambogic acid ameliorates high glucose- and palmitic acid-induced inflammatory response in ARPE-19 cells via activating Nrf2 signaling pathway: ex vivo. Cell Stress Chaperones 26, 367–375. doi: 10.1007/s12192-020-01182-1
Chen, K. S., and DeLuca, H. F. (1994). Isolation and characterization of a novel cDNA from HL-60 cells treated with 1,25-dihydroxyvitamin D-3. Biochim. Biophys. Acta 1219, 26–32. doi: 10.1016/0167-4781(94)90242-9
Chen, K., Lang, H., Wang, L., Liu, K., Zhou, Y., and Mi, M. (2020). S-Equol ameliorates insulin secretion failure through Chrebp/Txnip signaling via modulating PKA/PP2A activities. Nutr. Metab. 17:7. doi: 10.1186/s12986-020-0426-8
Chen, W., Zhao, M., Zhao, S., Lu, Q., Ni, L., Zou, C., et al. (2017). Activation of the TXNIP/NLRP3 inflammasome pathway contributes to inflammation in diabetic retinopathy: a novel inhibitory effect of minocycline. Inflamm. Res. 66, 157–166. doi: 10.1007/s00011-016-1002-6
Cheung, N., Mitchell, P., and Wong, T. Y. (2010). Diabetic retinopathy. Lancet 376, 124–136. doi: 10.1016/s0140-6736(09)62124-3
Devi, T. S., Hosoya, K., Terasaki, T., and Singh, L. P. (2013). Critical role of TXNIP in oxidative stress, DNA damage and retinal pericyte apoptosis under high glucose: implications for diabetic retinopathy. Exp. Cell Res. 319, 1001–1012. doi: 10.1016/j.yexcr.2013.01.012
Devi, T. S., Lee, I., Hüttemann, M., Kumar, A., Nantwi, K. D., and Singh, L. P. (2012). TXNIP links innate host defense mechanisms to oxidative stress and inflammation in retinal Muller glia under chronic hyperglycemia: implications for diabetic retinopathy. Exp. Diabetes Res. 2012:438238. doi: 10.1155/2012/438238
Devi, T. S., Somayajulu, M., Kowluru, R. A., and Singh, L. P. (2017). TXNIP regulates mitophagy in retinal Müller cells under high-glucose conditions: implications for diabetic retinopathy. Cell Death Dis. 8:e2777. doi: 10.1038/cddis.2017.190
Devi, T. S., Yumnamcha, T., Yao, F., Somayajulu, M., Kowluru, R. A., and Singh, L. P. (2019). TXNIP mediates high glucose-induced mitophagic flux and lysosome enlargement in human retinal pigment epithelial cells. Biol. Open 8:bio038521. doi: 10.1242/bio.038521
Dong, D., Fu, N., and Yang, P. (2016). MiR-17 downregulation by high glucose stabilizes thioredoxin-interacting protein and removes thioredoxin inhibition on ASK1 leading to apoptosis. Toxicol. Sci. 150, 84–96. doi: 10.1093/toxsci/kfv313
Duan, J., Du, C., Shi, Y., Liu, D., and Ma, J. (2018). Thioredoxin-interacting protein deficiency ameliorates diabetic retinal angiogenesis. Int. J. Biochem. Cell Biol. 94, 61–70. doi: 10.1016/j.biocel.2017.11.013
Dykstra, H., LaRose, C., Fisk, C., Waldhart, A., Meng, X., Zhao, G., et al. (2021). TXNIP interaction with GLUT1 depends on PI(4,5)P(2). Biochim. Biophys. Acta Biomembr. 1863:183757. doi: 10.1016/j.bbamem.2021.183757
Eissa, L. D., Ghobashy, W. A., and El-Azab, M. F. (2021). Inhibition of thioredoxin-interacting protein and inflammasome assembly using verapamil mitigates diabetic retinopathy and pancreatic injury. Eur. J. Pharmacol. 901:174061. doi: 10.1016/j.ejphar.2021.174061
Filhoulaud, G., Benhamed, F., Pagesy, P., Bonner, C., Fardini, Y., Ilias, A., et al. (2019). O-GlcNacylation links TxNIP to inflammasome activation in pancreatic β cells. Front. Endocrinol. 10:291. doi: 10.3389/fendo.2019.00291
Gao, Y., Chen, S., Peng, M., Wang, Z., Ren, L., Mu, S., et al. (2020). Correlation between thioredoxin-interacting protein and nerve conduction velocity in patients with type 2 diabetes mellitus. Front. Neurol. 11:733. doi: 10.3389/fneur.2020.00733
Gong, Q., Wang, H., Yu, P., Qian, T., and Xu, X. (2021). Protective or harmful: the dual roles of autophagy in diabetic retinopathy. Front. Med. 8:644121. doi: 10.3389/fmed.2021.644121
Gu, C., Draga, D., Zhou, C., Su, T., Zou, C., Gu, Q., et al. (2019). miR-590-3p inhibits pyroptosis in diabetic retinopathy by targeting NLRP1 and inactivating the NOX4 signaling pathway. Invest. Ophthalmol. Vis. Sci. 60, 4215–4223. doi: 10.1167/iovs.19-27825
Gurel, Z., and Sheibani, N. (2018). O-Linked β-N-acetylglucosamine (O-GlcNAc) modification: a new pathway to decode pathogenesis of diabetic retinopathy. Clin. Sci. 132, 185–198. doi: 10.1042/cs20171454
Hammes, H. P., Feng, Y., Pfister, F., and Brownlee, M. (2011). Diabetic retinopathy: targeting vasoregression. Diabetes 60, 9–16. doi: 10.2337/db10-0454
Han, J. H., Kim, S., Kim, S., Lee, H., Park, S. Y., and Woo, C. H. (2019). FMK, an inhibitor of p90RSK, inhibits high glucose-induced TXNIP expression via regulation of ChREBP in pancreatic β cells. Int. J. Mol. Sci. 20:4424. doi: 10.3390/ijms20184424
Han, Y., Xu, X., Tang, C., Gao, P., Chen, X., Xiong, X., et al. (2018). Reactive oxygen species promote tubular injury in diabetic nephropathy: the role of the mitochondrial ros-txnip-nlrp3 biological axis. Redox Biol. 16, 32–46. doi: 10.1016/j.redox.2018.02.013
Hart, G. W., Greis, K. D., Dong, L. Y., Blomberg, M. A., Chou, T. Y., Jiang, M. S., et al. (1995). O-linked N-acetylglucosamine: the “yin-yang” of Ser/Thr phosphorylation? Nuclear and cytoplasmic glycosylation. Adv. Exp. Med. Biol. 376, 115–123.
Hart, G. W., Slawson, C., Ramirez-Correa, G., and Lagerlof, O. (2011). Cross talk between O-GlcNAcylation and phosphorylation: roles in signaling, transcription, and chronic disease. Annu. Rev. Biochem. 80, 825–858. doi: 10.1146/annurev-biochem-060608-102511
He, X., and Ma, Q. (2012). Redox regulation by nuclear factor erythroid 2-related factor 2: gatekeeping for the basal and diabetes-induced expression of thioredoxin-interacting protein. Mol. Pharmacol. 82, 887–897. doi: 10.1124/mol.112.081133
Hernández, C., Bogdanov, P., Corraliza, L., García-Ramírez, M., Solà-Adell, C., Arranz, J. A., et al. (2016). Topical administration of GLP-1 receptor agonists prevents retinal neurodegeneration in experimental diabetes. Diabetes 65, 172–187. doi: 10.2337/db15-0443
Ji Cho, M., Yoon, S. J., Kim, W., Park, J., Lee, J., Park, J. G., et al. (2019). Oxidative stress-mediated TXNIP loss causes RPE dysfunction. Exp. Mol. Med. 51, 1–13. doi: 10.1038/s12276-019-0327-y
Jonsson, K. B., Frydkjaer-Olsen, U., and Grauslund, J. (2016). Vascular changes and neurodegeneration in the early stages of diabetic retinopathy: which comes first? Ophthalmic Res. 56, 1–9. doi: 10.1159/000444498
Jung, C. H., Jun, C. B., Ro, S. H., Kim, Y. M., Otto, N. M., Cao, J., et al. (2009). ULK-Atg13-FIP200 complexes mediate mTOR signaling to the autophagy machinery. Mol. Biol. Cell 20, 1992–2003. doi: 10.1091/mbc.e08-12-1249
Kadłubowska, J., Malaguarnera, L., Wa̧ż, P., and Zorena, K. (2016). Neurodegeneration and neuroinflammation in diabetic retinopathy: potential approaches to delay neuronal loss. Curr. Neuropharmacol. 14, 831–839. doi: 10.2174/1570159x14666160614095559
Kibbe, C., Chen, J., Xu, G., Jing, G., and Shalev, A. (2013). FOXO1 competes with carbohydrate response element-binding protein (ChREBP) and inhibits thioredoxin-interacting protein (TXNIP) transcription in pancreatic beta cells. J. Biol. Chem. 288, 23194–23202. doi: 10.1074/jbc.M113.473082
Kim, Y. S., Kim, M., Choi, M. Y., Lee, D. H., Roh, G. S., Kim, H. J., et al. (2017). Metformin protects against retinal cell death in diabetic mice. Biochem. Biophys. Res. Commun. 492, 397–403. doi: 10.1016/j.bbrc.2017.08.087
Krol, J., Loedige, I., and Filipowicz, W. (2010). The widespread regulation of microRNA biogenesis, function and decay. Nat. Rev. Genet. 11, 597–610. doi: 10.1038/nrg2843
Lalit, P. S., Thangal, Y., Fayi, Y., and Takhellambam, S. D. (2018). Potentials of gene therapy for diabetic retinopathy: the use of nucleic acid constructs containing a TXNIP promoter. Open Access J. Ophthalmol. 3:147. doi: 10.23880/oajo-16000147
Levine, B., and Kroemer, G. (2019). Biological functions of autophagy genes: a disease perspective. Cell 176, 11–42. doi: 10.1016/j.cell.2018.09.048
Li, J., Wang, P., Chen, Z., Yu, S., and Xu, H. (2018). Fenofibrate ameliorates oxidative stress-induced retinal microvascular dysfunction in diabetic rats. Curr. Eye Res. 43, 1395–1403. doi: 10.1080/02713683.2018.1501072
Lillig, C. H., and Holmgren, A. (2007). Thioredoxin and related molecules–from biology to health and disease. Antioxid. Redox Signal. 9, 25–47. doi: 10.1089/ars.2007.9.25
Liu, Q., Zhang, X., Cheng, R., Ma, J. X., Yi, J., and Li, J. (2019). Salutary effect of fenofibrate on type 1 diabetic retinopathy via inhibiting oxidative stress-mediated Wnt/β-catenin pathway activation. Cell Tissue Res. 376, 165–177. doi: 10.1007/s00441-018-2974-z
Liu, S., Tang, G., Duan, F., Zeng, C., Gong, J., Chen, Y., et al. (2021). MiR-17-5p inhibits TXNIP/NLRP3 inflammasome pathway and suppresses pancreatic β-cell pyroptosis in diabetic mice. Front. Cardiovasc. Med. 8:768029. doi: 10.3389/fcvm.2021.768029
Lu, J., and Holmgren, A. (2014). The thioredoxin antioxidant system. Free Radic. Biol. Med. 66, 75–87. doi: 10.1016/j.freeradbiomed.2013.07.036
Lu, L., Lu, Q., Chen, W., Li, J., Li, C., and Zheng, Z. (2018). Vitamin D(3) Protects against diabetic retinopathy by inhibiting high-glucose-induced activation of the ROS/TXNIP/NLRP3 inflammasome pathway. J. Diabetes Res. 2018, 8193523. doi: 10.1155/2018/8193523
Ludwig, D. L., Kotanides, H., Le, T., Chavkin, D., Bohlen, P., and Witte, L. (2001). Cloning, genetic characterization, and chromosomal mapping of the mouse VDUP1 gene. Gene 269, 103–112. doi: 10.1016/s0378-1119(01)00455-3
Lv, J., Bao, S., Liu, T., Wei, L., Wang, D., Ye, W., et al. (2020). Sulforaphane delays diabetes-induced retinal photoreceptor cell degeneration. Cell Tissue Res. 382, 477–486. doi: 10.1007/s00441-020-03267-w
Lynch, S. K., and Abràmoff, M. D. (2017). Diabetic retinopathy is a neurodegenerative disorder. Vision Res. 139, 101–107. doi: 10.1016/j.visres.2017.03.003
Maiuri, M. C., Zalckvar, E., Kimchi, A., and Kroemer, G. (2007). Self-eating and self-killing: crosstalk between autophagy and apoptosis. Nat. Rev. Mol. Cell Biol. 8, 741–752. doi: 10.1038/nrm2239
Martinon, F., Burns, K., and Tschopp, J. (2002). The inflammasome: a molecular platform triggering activation of inflammatory caspases and processing of proIL-beta. Mol. Cell 10, 417–426. doi: 10.1016/s1097-2765(02)00599-3
Nguyen, D., Alavi, M. V., Kim, K. Y., Kang, T., Scott, R. T., Noh, Y. H., et al. (2011). A new vicious cycle involving glutamate excitotoxicity, oxidative stress and mitochondrial dynamics. Cell Death Dis. 2:e240. doi: 10.1038/cddis.2011.117
Patwari, P., Chutkow, W. A., Cummings, K., Verstraeten, V. L., Lammerding, J., Schreiter, E. R., et al. (2009). Thioredoxin-independent regulation of metabolism by the alpha-arrestin proteins. J. Biol. Chem. 284, 24996–25003. doi: 10.1074/jbc.M109.018093
Patwari, P., Higgins, L. J., Chutkow, W. A., Yoshioka, J., and Lee, R. T. (2006). The interaction of thioredoxin with Txnip. Evidence for formation of a mixed disulfide by disulfide exchange. J. Biol. Chem. 281, 21884–21891. doi: 10.1074/jbc.M600427200
Perrone, L., Devi, T. S., Hosoya, K. I., Terasaki, T., and Singh, L. P. (2010). Inhibition of TXNIP expression in vivo blocks early pathologies of diabetic retinopathy. Cell Death Dis. 1:e65. doi: 10.1038/cddis.2010.42
Perrone, L., Devi, T. S., Hosoya, K., Terasaki, T., and Singh, L. P. (2009). Thioredoxin interacting protein (TXNIP) induces inflammation through chromatin modification in retinal capillary endothelial cells under diabetic conditions. J. Cell Physiol. 221, 262–272. doi: 10.1002/jcp.21852
Poungvarin, N., Lee, J. K., Yechoor, V. K., Li, M. V., Assavapokee, T., Suksaranjit, P., et al. (2012). Carbohydrate response element-binding protein (ChREBP) plays a pivotal role in beta cell glucotoxicity. Diabetologia 55, 1783–1796. doi: 10.1007/s00125-012-2506-4
Ren, X., Li, C., Liu, J., Zhang, C., Fu, Y., Wang, N., et al. (2017). Thioredoxin plays a key role in retinal neuropathy prior to endothelial damage in diabetic mice. Oncotarget 8, 61350–61364. doi: 10.18632/oncotarget.18134
Ren, X., Lv, J., Wang, N., Liu, J., Gao, C., Wu, X., et al. (2022). Thioredoxin upregulation delays diabetes-induced photoreceptor cell degeneration via AMPK-mediated autophagy and exosome secretion. Diabetes Res. Clin. Pract. 185:109788. doi: 10.1016/j.diabres.2022.109788
Ren, X., Wang, N. N., Qi, H., Qiu, Y. Y., Zhang, C. H., Brown, E., et al. (2018). Up-regulation thioredoxin inhibits advanced glycation end products-induced neurodegeneration. Cell Physiol. Biochem. 50, 1673–1686. doi: 10.1159/000494787
Richards, P., Ourabah, S., Montagne, J., Burnol, A. F., Postic, C., and Guilmeau, S. (2017). MondoA/ChREBP: the usual suspects of transcriptional glucose sensing; Implication in pathophysiology. Metabolism 70, 133–151. doi: 10.1016/j.metabol.2017.01.033
Rodríguez, M. L., Pérez, S., Mena-Mollá, S., Desco, M. C., and Ortega, ÁL. (2019). Oxidative stress and microvascular alterations in diabetic retinopathy: future therapies. Oxid. Med. Cell Longev. 2019:4940825. doi: 10.1155/2019/4940825
Rolev, K. D., Shu, X. S., and Ying, Y. (2021). Targeted pharmacotherapy against neurodegeneration and neuroinflammation in early diabetic retinopathy. Neuropharmacology 187:108498. doi: 10.1016/j.neuropharm.2021.108498
Rosa, M. D., Distefano, G., Gagliano, C., Rusciano, D., and Malaguarnera, L. (2016). Autophagy in diabetic retinopathy. Curr. Neuropharmacol. 14, 810–825. doi: 10.2174/1570159x14666160321122900
Rossino, M. G., Dal Monte, M., and Casini, G. (2019). Relationships between neurodegeneration and vascular damage in diabetic retinopathy. Front. Neurosci. 13:1172. doi: 10.3389/fnins.2019.01172
Russo, M., Spagnuolo, C., Russo, G. L., Skalicka-Woźniak, K., Daglia, M., Sobarzo-Sánchez, E., et al. (2018). Nrf2 targeting by sulforaphane: a potential therapy for cancer treatment. Crit. Rev. Food Sci. Nutr. 58, 1391–1405. doi: 10.1080/10408398.2016.1259983
Saxena, G., Chen, J., and Shalev, A. (2010). Intracellular shuttling and mitochondrial function of thioredoxin-interacting protein. J. Biol. Chem. 285, 3997–4005. doi: 10.1074/jbc.M109.034421
Schroder, K., Zhou, R., and Tschopp, J. (2010). The NLRP3 inflammasome: a sensor for metabolic danger? Science 327, 296–300. doi: 10.1126/science.1184003
Schulze, P. C., Yoshioka, J., Takahashi, T., He, Z., King, G. L., and Lee, R. T. (2004). Hyperglycemia promotes oxidative stress through inhibition of thioredoxin function by thioredoxin-interacting protein. J. Biol. Chem. 279, 30369–30374. doi: 10.1074/jbc.M400549200
Shaked, M., Ketzinel-Gilad, M., Ariav, Y., Cerasi, E., Kaiser, N., and Leibowitz, G. (2009). Insulin counteracts glucotoxic effects by suppressing thioredoxin-interacting protein production in INS-1E beta cells and in Psammomys obesus pancreatic islets. Diabetologia 52, 636–644. doi: 10.1007/s00125-009-1274-2
Shalev, A. (2014). Minireview: thioredoxin-interacting protein: regulation and function in the pancreatic β-cell. Mol. Endocrinol. 28, 1211–1220. doi: 10.1210/me.2014-1095
Shalev, A., Pise-Masison, C. A., Radonovich, M., Hoffmann, S. C., Hirshberg, B., Brady, J. N., et al. (2002). Oligonucleotide microarray analysis of intact human pancreatic islets: identification of glucose-responsive genes and a highly regulated TGFbeta signaling pathway. Endocrinology 143, 3695–3698. doi: 10.1210/en.2002-220564
Shen, M., Bai, D., Liu, B., Lu, X., Hou, R., Zeng, C., et al. (2018). Dysregulated Txnip-ROS-Wnt axis contributes to the impaired ischemic heart repair in diabetic mice. Biochim. Biophys. Acta Mol. Basis Dis. 1864, 3735–3745. doi: 10.1016/j.bbadis.2018.09.029
Singh, L. P. (2013). Thioredoxin Interacting Protein (TXNIP) and Pathogenesis of Diabetic Retinopathy. J. Clin. Exp. Ophthalmol. 4:10.4172/2155–9570.1000287. doi: 10.4172/2155-9570.1000287
Singh, L. P., and Devi, T. S. (2021). Potential combination drug therapy to prevent redox stress and mitophagy dysregulation in retinal müller cells under high glucose conditions: implications for diabetic retinopathy. Diseases 9:91. doi: 10.3390/diseases9040091
Singh, L. P., Devi, T. S., and Yumnamcha, T. (2017). The role of Txnip in mitophagy dysregulation and inflammasome activation in diabetic retinopathy: a New Perspective. JOJ Ophthalmol. 4:10.19080/jojo.2017.04.555643. doi: 10.19080/jojo.2017.04.555643
Skeie, J. M., Nishimura, D. Y., Wang, C. L., Schmidt, G. A., Aldrich, B. T., and Greiner, M. A. (2021). Mitophagy: an emerging target in ocular pathology. Invest. Ophthalmol. Vis. Sci. 62:22. doi: 10.1167/iovs.62.3.22
Spindel, O. N., World, C., and Berk, B. C. (2012). Thioredoxin interacting protein: redox dependent and independent regulatory mechanisms. Antioxid. Redox Signal 16, 587–596. doi: 10.1089/ars.2011.4137
Sreekumar, P. G., Ding, Y., Ryan, S. J., Kannan, R., and Hinton, D. R. (2009). Regulation of thioredoxin by ceramide in retinal pigment epithelial cells. Exp. Eye Res. 88, 410–417. doi: 10.1016/j.exer.2008.10.009
Tang, L., Zhang, C., Yang, Q., Xie, H., Liu, D., Tian, H., et al. (2021). Melatonin maintains inner blood-retinal barrier via inhibition of p38/TXNIP/NF-κB pathway in diabetic retinopathy. J. Cell Physiol. 236, 5848–5864. doi: 10.1002/jcp.30269
Thielen, L. A., Chen, J., Jing, G., Moukha-Chafiq, O., Xu, G., Jo, S., et al. (2020). Identification of an anti-diabetic, orally available small molecule that regulates TXNIP expression and glucagon action. Cell Metab 32, 353.e358–365.e358. doi: 10.1016/j.cmet.2020.07.002
Thielen, L., and Shalev, A. (2018). Diabetes pathogenic mechanisms and potential new therapies based upon a novel target called TXNIP. Curr. Opin. Endocrinol. Diabetes Obes. 25, 75–80. doi: 10.1097/med.0000000000000391
van Dijk, H. W., Verbraak, F. D., Kok, P. H., Stehouwer, M., Garvin, M. K., Sonka, M., et al. (2012). Early neurodegeneration in the retina of type 2 diabetic patients. Invest. Ophthalmol. Vis. Sci. 53, 2715–2719. doi: 10.1167/iovs.11-8997
Wang, S., Du, S., Lv, Y., Wang, W., and Zhang, F. (2020). Elevated microRNA-20b-3p and reduced thioredoxin-interacting protein ameliorate diabetic retinopathy progression by suppressing the NLRP3 inflammasomes. IUBMB Life 72, 1433–1448. doi: 10.1002/iub.2267
Wilkinson, C. P., Ferris, F. L. III, Klein, R. E., Lee, P. P., Agardh, C. D., Davis, M., et al. (2003). Proposed international clinical diabetic retinopathy and diabetic macular edema disease severity scales. Ophthalmology 110, 1677–1682. doi: 10.1016/s0161-6420(03)00475-5
Wondafrash, D. Z., Nire’a, A. T., Tafere, G. G., Desta, D. M., Berhe, D. A., and Zewdie, K. A. (2020). Thioredoxin-interacting protein as a novel potential therapeutic target in diabetes mellitus and its underlying complications. Diabetes Metab. Syndr. Obes. 13, 43–51. doi: 10.2147/dmso.S232221
Wu, N., Zheng, B., Shaywitz, A., Dagon, Y., Tower, C., Bellinger, G., et al. (2013). AMPK-dependent degradation of TXNIP upon energy stress leads to enhanced glucose uptake via GLUT1. Mol. Cell 49, 1167–1175. doi: 10.1016/j.molcel.2013.01.035
Xu, G., Chen, J., Jing, G., and Shalev, A. (2013). Thioredoxin-interacting protein regulates insulin transcription through microRNA-204. Nat. Med. 19, 1141–1146. doi: 10.1038/nm.3287
Yang, Y., Zhou, M., and Liu, H. (2021). Luteolin, an aryl hydrocarbon receptor antagonist, alleviates diabetic retinopathy by regulating the NLRP/NOX4 signalling pathway: experimental and molecular docking study. Physiol. Int. Online ahead of print doi: 10.1556/2060.2021.00148
Yu, X., Xu, Z., Mi, M., Xu, H., Zhu, J., Wei, N., et al. (2008). Dietary taurine supplementation ameliorates diabetic retinopathy via anti-excitotoxicity of glutamate in streptozotocin-induced Sprague-Dawley rats. Neurochem. Res. 33, 500–507. doi: 10.1007/s11064-007-9465-z
Yu, Y., Xing, K., Badamas, R., Kuszynski, C. A., Wu, H., and Lou, M. F. (2013). Overexpression of thioredoxin-binding protein 2 increases oxidation sensitivity and apoptosis in human lens epithelial cells. Free Radic. Biol. Med. 57, 92–104. doi: 10.1016/j.freeradbiomed.2012.12.022
Zhang, Y., Zheng, L., Xu, H., and Ling, L. (2021). Circ_0084043 facilitates high glucose-induced retinal pigment epithelial cell injury by activating miR-128-3p/TXNIP-mediated Wnt/β-catenin signaling pathway. J. Cardiovasc. Pharmacol. 78, e112–e121. doi: 10.1097/fjc.0000000000001039
Zhong, L., Liu, Q., Liu, Q., Zhang, S., Cao, Y., Yang, D., et al. (2021). W2476 represses TXNIP transcription via dephosphorylation of FOXO1 at Ser319. Chem. Biol. Drug Des. 97, 1089–1099. doi: 10.1111/cbdd.13828
Keywords: TXNIP (thioredoxin-interacting protein), diabetic retinal neurodegeneration, diabetic retinopathy, thioredoxin, TXNIP modulators
Citation: Liu C, Dong W, Lv Z, Kong L and Ren X (2022) Thioredoxin-interacting protein in diabetic retinal neurodegeneration: A novel potential therapeutic target for diabetic retinopathy. Front. Neurosci. 16:957667. doi: 10.3389/fnins.2022.957667
Received: 31 May 2022; Accepted: 19 July 2022;
Published: 09 August 2022.
Edited by:
Markus Tschopp, University of Bern, SwitzerlandReviewed by:
Dario Rusciano, Fidia Farmaceutici, ItalyMari Luz Moreno, Catholic University of Valencia San Vicente Mártir, Spain
Copyright © 2022 Liu, Dong, Lv, Kong and Ren. This is an open-access article distributed under the terms of the Creative Commons Attribution License (CC BY). The use, distribution or reproduction in other forums is permitted, provided the original author(s) and the copyright owner(s) are credited and that the original publication in this journal is cited, in accordance with accepted academic practice. No use, distribution or reproduction is permitted which does not comply with these terms.
*Correspondence: Zhengshuai Lv, bHZ6aGVuZ3NodWFpMjAwN0AxNjMuY29t; Li Kong, a29uZ2xpQGRtdS5lZHUuY24=; Xiang Ren, eGlhbmdyZW5AZG11LmVkdS5jbg==