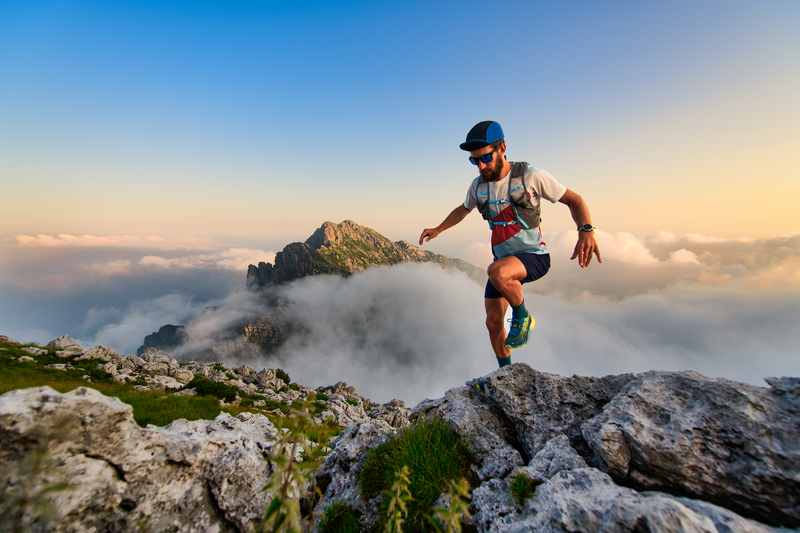
95% of researchers rate our articles as excellent or good
Learn more about the work of our research integrity team to safeguard the quality of each article we publish.
Find out more
REVIEW article
Front. Neurosci. , 10 June 2022
Sec. Neuropharmacology
Volume 16 - 2022 | https://doi.org/10.3389/fnins.2022.901360
This article is part of the Research Topic Advances in Neuroprotective Agents for Cerebral Ischemia Treatment View all 24 articles
Cerebral ischemia reperfusion injury is a debilitating medical condition, currently with only a limited amount of therapies aimed at protecting the cerebral parenchyma. Micro RNAs (miRNAs) are small, non-coding RNA molecules that via the RNA-induced silencing complex either degrade or prevent target messenger RNAs from being translated and thus, can modulate the synthesis of target proteins. In the neurological field, miRNAs have been evaluated as potential regulators in brain development processes and pathological events. Following ischemic hypoxic stress, the cellular and molecular events initiated dysregulate different miRNAs, responsible for long-terming progression and extension of neuronal damage. Because of their ability to regulate the synthesis of target proteins, miRNAs emerge as a possible therapeutic strategy in limiting the neuronal damage following a cerebral ischemic event. This review aims to summarize the recent literature evidence of the miRNAs involved in signaling and modulating cerebral ischemia-reperfusion injuries, thus pointing their potential in limiting neuronal damage and repair mechanisms. An in-depth overview of the molecular pathways involved in ischemia reperfusion injury and the involvement of specific miRNAs, could provide future perspectives in the development of neuroprotective agents targeting these specific miRNAs.
Stroke represents the third leading cause of death and a major debilitating medical condition. It is responsible for permanent disabilities in approximately 80% of post-stroke patients (Moskowitz et al., 2010; Lallukka et al., 2018). Metabolic disruption of neurons activates immune responses, resulting in a complex chain of molecular events, which further promote progressive cellular damage and irretrievable neuronal death (Moskowitz et al., 2010; Khoshnam et al., 2017).
The ischemic/reperfusion (I/R) injury is caused by a sudden restriction of blood supply and oxygen, followed by subsequent restoration of blood flow and reoxygenation, contributing supplementary to the global oxidative stress (Eltzschig and Eckle, 2011). The I/R injury is the main actor in the neuroinflammatory repertoire, triggering different cell death provoking events, which include apoptosis, blood-brain barrier (BBB) disruption and mitochondrial dysfunction (Eltzschig and Eckle, 2011; Khoshnam et al., 2017).
The neuroprotective agents under current research address either the ischemic core, or the viable penumbra region, with the aim of reestablishing the collateral blow flow and ameliorating the microenvironment damaged tissue (Eltzschig and Eckle, 2011; He et al., 2021). The standard therapeutic strategy for ischemic stroke remains thrombolytic reperfusion therapy provided by intravenous tissue plasminogen activator that is, however, limited by a short therapeutic window of 3-4,5 hours (Del Zoppo et al., 2009; IST-3 collaborative group et al., 2012; Fonarow et al., 2014).
Preclinical translation of neuroprotective drugs into clinical settings is failing. Even with advancing experimental studies on animal models, with excellent human reproducibility provided by thromboembolic stroke models, i.e., reproducible infarct size, and penumbra zone, there are still many promising neuroprotective agents in preclinical studies that fail to show a significant effect on patients (Dirnagl, 2006; Canazza et al., 2014; Luo et al., 2019). Dirnag et al. attributed this limited clinical potential of experimental drugs to statistical errors, lack of blinding and randomization of the animals, and negative publication bias (Dirnagl, 2006). Unexplored impediments steam from the limited ability of drugs to penetrate the BBB and target the ischemic neuronal tissue, resulting in decreased efficient concentration of the neuroprotective agents (Saugstad, 2010; Ponnusamy and Yip, 2019). In this context, selective drug delivery systems such as stroke tissue-related homing peptides and nanoparticles-mediated agents are emerging (Hong et al., 2008; He et al., 2021).
Micro RNAs (miRNAs) are small, non-coding RNA molecules, containing around 18–25 nucleotides, which pose a post-transcriptional regulatory role by down-regulating messenger RNAs (mRNAs) (Jonas and Izaurralde, 2015). Binding to the target mRNAs by base pairing, miRNAs negatively regulate gene expression of mRNAs via cleavage of mRNA, translation repression or destabilization of mRNA structure (Bartel, 2009; MacFarlane and Murphy, 2010).
The first pathological condition described, related to miRNAs was chronic lymphocytic leukemia (Calin et al., 2004). Since then, multiple studies outline the potential of miRNAs to mediate several pathological mechanisms of human diseases—i.e., cancer, neurological disorders, immune system disorders, acting as signaling molecules and mediators of cell-cell communication in different cellular processes such as proliferation, differentiation, and apoptosis (Smirnova et al., 2005; Garofalo et al., 2010; Tüfekci et al., 2014). MicroRNAs are key master regulators of gene expression in the brain, in processes related to brain development and its normal functioning, i.e., synaptogenesis, myelination, cerebral vasculogenesis and angiogenesis, but also in different brain disorders: ischemic stroke, neurodegenerative disease, traumatic brain injury, spinal cord injury, hypoxic-ischemic encephalopathy (Saugstad, 2010; Ponnusamy and Yip, 2019).
MicroRNAs also play a pivotal role in I/R injury, the main contributor to reactive oxygen species (ROS) production, cellular metabolic disfunctions associated with/underlying ischemic stroke (Ouyang et al., 2015; Cao et al., 2021). Recent studies have shown that I/R-related miRNAs alter the mitochondrial response and mediate multiple pathways that further promote neuronal survival and apoptosis (Jeyaseelan et al., 2008; Di et al., 2014; Hu et al., 2015; Ouyang et al., 2015). Min et al. highlighted the altered expression profile of miRNAs in brain I/R injury, which consisted of 15 miRNAs upregulated and 44 miRNAs downregulated (Min et al., 2015). MiRNAs modulate critical signaling pathways in I/R injury, associated with fibrosis, neoangiogenesis, necrosis, apoptosis and inflammation (Ghafouri-Fard et al., 2020).
However, miRNAs have also been reported in promoting the pathogenesis of ischemic stroke—i.e., atherosclerosis, hypertension, hyperlipidemia, plaque rupture, bidirectionally influencing the pathological chain of ischemic events, both pathogenesis and pathways (Rink and Khanna, 2011). In this direction, advancing the knowledge in gene functions using agomirs or antagomirs—double stranded miRNA agents, chemically modified at antisense strand that act as miRNA mimickers or inhibitors—could provide potential neuroprotective effects in modulating pathological processes in ischemic injuries (Kadir et al., 2020).
Neuroscience confronts limited therapeutic strategies aimed at protecting ischemic tissue, for which there is a critical and urgent need for advancing our knowledge. A depth overview of the molecular pathways involved in ischemic stroke, which are targeted by specific miRNAs, could provide future perspectives in the development of neuroprotective miRNA agents. This review aims to summarize the recent literature evidence of the miRNAs involved in signaling and modulating cerebral ischemia-reperfusion injuries, thus pointing their potential in limiting neuronal damage and repair mechanisms.
Development of the adult brain and its functions are a highly studied subject in today’s literature. Normal brain development proceeds via complex multistep processes, which involves early embryonic stage- neurogenesis, consisting in proliferation and differentiation of precursor neuronal cells, continuing to myelination and synaptogenesis in the childhood and adulthood period, which contributes to synaptic plasticity and memory (Semple et al., 2013). MiRNAs play essential roles in controlling neurodevelopment processes and normal brain functions, and dysregulation of miRNA expression profiling has been related to perinatal brain injury (Cho et al., 2019). Ponnusamy and Yip (2019) deciphered the role of miRNA involved in normal brain development’ processes under normoxic and hypoxic conditions, consisting in myelination, axonal outgrowth, dendric outgrowth, synaptogenesis, neuronal differentiation, neuronal migration, angiogenesis.
Neurodegenerative diseases, which are mainly characterized by intracellular or extracellular protein aggregate formation, resulting to neuron dysfunction in certain brain areas, includes Alzheimer’s disease (AD), Parkinson’s disease (PD), Huntington’s disease and multiple sclerosis (MS) (Quinlan et al., 2017).
Mounting evidence suggested the role of miRNAs-based therapeutics in modulating the prognosis of neurodegenerative diseases, emerging new miRNAs biomarkers for a better disease control (Quinlan et al., 2017). Thus, Juźwik et al. (2019) in a systematic review of 12 neurodegenerative disease identified 10 miRNAs frequently dysregulated, including miR-9-5p, miR-21-5p, miR-29a-3p, miR-29b-3p, miR-29c-3p, miR-124-3p, miR-132-3p, miR146a-5p, miR-155-5p, and miR-223-3p. Notably, a different expression level of miRNAs, miR-9-5p, miR-21-5p, the miR-29, miR-124-3p, and miR-132-3p have been revealed, suggesting the mixed expression levels of miRNAs.
PD is characterized by dopaminergic neuron loss from the substantia nigra, with dysregulated level of miRNAs expression in the striatal brain areas and dopaminergic neurons (Nies et al., 2021). Prefrontal cortex of post-mortem PD patients exhibited 125 dysregulated miRNAs, of which miR-10b-5p levels being associated with clinical onset in both PD and Huntington’s Disease (Hoss et al., 2016). The pathogenesis of PD related to miRNAs have been explained by modulation of PD-associated genes and protein expression related to α-synuclein-induced neuroinflammation, and degeneration of dopaminergic neurons (Nies et al., 2021). Down regulation of miR-425 in MPTP injected mouse PD model contributes to necroptosis and apoptosis activation, disintegration of mitochondrial membrane, ultimately leading to neuron loss and dopamine depletion. Moreover, miR-103a-3p, miR-30b-5p, and miR-29a-3p exhibited high levels of expression after Levodopa treatment, suggesting the role of miRNAs as disease modifier agents in PD (Serafin et al., 2015). Recent studies have shown that suppressing miR-34a can improve neuronal loss related to PD (Chua and Tang, 2019).
Sun et al. (2021) using bioinformatic analysis, reviewed the dysregulated miRNAs expression profiling in tissues of AD patients’ brain, blood and CSF, correlated with pathological processes. Therefore, 27 dysregulated miRNAs identified have been related to neuroinflammation, amyloidogenesis, tau phosphorylation, synaptogenesis, apoptosis, and neuron degradation (Sun et al., 2021).
Multiple in vivo and in vitro animal models revealed the potential of miRNAs to counteracting beta-amyloid or tau reduction, inhibiting of apoptosis, and synaptic protection. In APP/PS1 transgenic mice, miR-137 exhibited reduced levels in the cerebral cortex, hippocampus, and serum, suggesting the neuroprotective potential of miR-137 to suppress p-tau overexpression (Jiang et al., 2018b). Moreover, inhibition of miR-98 in N2a/APP cells suppressed Aβ production by upregulating insulin-like growth factor 1 pathway (Hu et al., 2013, 1).
Neuroinflammation plays critical roles in MS pathogenesis consisting in dysregulation of inflammatory cell events in the brain, resulting in BBB disruption, damage of myelin and oligodendrocytes, neuro-axonal damage and inflammation (Haase and Linker, 2021).
MiR-155 which exhibited upregulated levels in MS, poses important role in BBB disruption under inflammatory conditions, which drives to demyelination processes, i.e., microglial activation, polarization of astrocyte. In 58 MS patients with adult onset, miR-320a, miR-125a-5p, miR-652-3p, miR-185-5p, miR-942-5p, miR-25-3p were significantly upregulated in peripheral blood samples, controlling transcription factors of SP1, NF-κB, TP53, HDAC1, and STAT3 (Nuzziello et al., 2018).
Unbalance of inflammatory reactions including dysfunction of memory T-cells and Treg cells contributed to continuous and progression inflammatory demyelinating of CNS. For instance, in MS patients, miR-19a, miR-19b, miR-25, and miR-106 elicited significantly upregulated levels in Treg cells compared with healthy controls (Gao et al., 2021). Targeting dysregulated miRNAs represents a therapeutic strategy. Thus, inhibiting let-7e decrease the differentiation of Th1 and Th17 cells, reducing the severity of MS in experimental autoimmune encephalomyelitis (Angelou et al., 2019). Increasing evidence ascertained the involvement of miRNAs in the initiation and progression of multifold types of cancer. Petrescu et al. (2019) reviewed the main dysregulated miRNAs related to brain tumors pathogenesis in glioma, meningioma, pituitary adenoma, and astrocytoma.
Multiple pathological processes associated with gliomagenesis were controlled by miRNAs. From disrupting BBB by targeting junctional proteins, zonula occludens-1 (ZO-1), occludin and β-catenin, to angiogenic, infiltration and migration of glioma cells by downregulating MMP2, MMP9, VEGF, all these tumor promoting processes are modulated by several miRNAs (Petrescu et al., 2019).
MiRNAs could be also used as clinical prognosis biomarkers. In 90 serum astrocytoma patients, miR-15b-5p, -16-5p, -19a-3p, -19b-3, 20a-5p, 106a-5p, 130a-3p, 181b-5p and 208a-3p exhibited upregulation levels, with miR-19a-3p, -106a-5p, and -181b-5p being associated with lower survival rate (Zhi et al., 2015).
Hypoxic or ischemic brain injury give rise to a heterogeneity of histological findings, in which the neurons, the glial cells, the neuropile and the brain microvasculature are affected. These alterations in brain histological structures occur in chronological order and depends on the magnitude and duration of ischemia, and the extension of tissue damage. Two areas are examined: the ‘’ischemic core” or the irreversibly damaged area, and the ‘’ischemic penumbra,” the hypoperfused area, which still contains viable cells.
The earliest change which occurs in the ischemic core is represented by neuronal swelling, because of the cytotoxic edema caused by ion alteration. The damaged neurons are large, with pale staining cytoplasm and pyknotic nucleus in hematoxylin and eosin (H&E) staining. After hours, in the ischemic core appear the red, eosinophilic, or ischemic neurons, characterized on routine histological sections by cell shrinkage, a pyknotic nucleus without nucleolus, and a highly eosinophilic cytoplasm, devoided of Nissle bodies. These neurons may be found also in the penumbra area for 1 or 2 days. Another aspect of advanced neuronal degeneration is represented by ‘ghost neurons’, found in the ischemic core and in the ischemic penumbra zone, which exhibits an irregular and very ill-defined cell border, pale staining cytoplasm in H&E staining and pyknotic, dark nucleus. The disintegration of dead neurons leads to parenchymal necrosis and release of cellular debris, which later will be engulfed by macrophages (Mărgăritescu et al., 2009; Rahaman and Del Bigio, 2018).
Activation and proliferation of microglia, the resident macrophages in the central nervous system, occurs in the ischemic core in the first hours after ischemic injury, being involved in removing the necrotic tissue. During activation, microglia undergo morphological changes, with increase in cell body size and retraction of cytoplasmatic processes, acquiring an amoeboid phenotype in the ischemic core. In the ischemic penumbra and in the marginal zone we can find numerous highly ramified microglia (reactive microglia), which can migrate to the ischemic core, suggesting the fact that microglia may exhibit different morphological patterns, according to degree of ischemia and the time interval after ischemia (Zhang, 2019). After about 3 days, a lot of bone marrow-derived macrophages infiltrated the ischemic core and the ischemic penumbra (mostly), where they phagocytose the cellular and myelin debris, having a foamy appearance on histological sections. Activated microglia express high levels of immunomarker Iba1 +, while bone marrow-derived macrophages are highly positive for CD45 (Mărgăritescu et al., 2009; Li et al., 2014b; Magaki et al., 2018; Washida et al., 2019; Zhang, 2019).
In the ischemic core, swelling or edematous astrocytes may be found in the early phase, with a pale staining cytoplasm and disrupted cytoplasmatic processes; eventually, these cells will die. In the ischemic penumbra, the surviving astrocyte proliferate and undergo hypertrophy (reactive astrogliosis), expressing high amounts of glial fibrillary acidic protein. In routine histological sections, reactive astrocytes are large, star-shaped cells, having a coarse nuclear chromatin, glassy eosinophilic cytoplasm and long, branching cytoplasmatic processes; they are also called gemistocytic astrocytes. Astrogliosis represents a hallmark of nervous tissue injury after ischemia, and always follows the microglial activation and blood-derived macrophages invasion. After several days, the astrocytes and microglial cells from the ischemic penumbra surround the ischemic core and the cells will fill the necrotic areas, forming the glial scar tissue, an eosinophilic zone in H&E staining, with neuron loss and numerous glial cells, mainly reactive astrocytes (Mărgăritescu et al., 2009; Li et al., 2014b; Magaki et al., 2018).
In the first hours after ischemic injury, oligodendrocytes damage may cause axonal degeneration and demyelination, leading to rarefaction of the white matter (Mărgăritescu et al., 2009; Washida et al., 2019).
In the ischemic core, structural changes of the small blood vessels are observed, such as: endothelial cell (ECs) swelling, pericyte and ECs detachment from the basement membrane, narrowing of the lumen, hyalinization and vascular wall thickening and sclerosis, with increase amount of collagen fibers and disintegration of vascular smooth muscle cells. These vascular modifications, in addition to morphological changes of astrocyte foot processes, lead to alteration of the BBB, which cause the vasogenic edema in the neuropil. Disruption of BBB or disintegration of capillaries in the necrotic areas, induce the appearance of microhemorrhages, extravasated and lysed erythrocytes releasing hemosiderin pigment, which is phagocytized by macrophages (siderophages) (Mărgăritescu et al., 2009; Rahaman and Del Bigio, 2018; Liu et al., 2019a).
The ischemic penumbra contains congested blood vessels, surrounded by perivascular edema. After 3 days, neovascularization occurs within the ischemic penumbra, but the newly formed blood vessels are abnormal, thin, highly permeable, thus increasing the pre-existing brain edema (Rahaman and Del Bigio, 2018; Liu et al., 2019a).
Polymorphonuclear leukocytes (PMNs) and macrophages play a key role in early inflammatory reaction after brain ischemia, while lymphocytes (mostly T lymphocytes), are involved in the delayed phases of ischemia. An acute inflammatory reaction appears within the first 4-6 hours after ischemic injury, with PMNs infiltration in the necrotic tissue. Within the first 3 days, activated microglia and blood-derived macrophages invade the necrotic area, engulfing the cellular and myelin debris (lipid-laden macrophages) (Kawabori and Yenari, 2015; Anrather and Iadecola, 2016).
Neuronal damage after recanalization has long been known to occur following ischemic stroke through a unique type of injury that is not expressed during the hypoxic period (S.M. Humphrey et al., 1973; Baird et al., 1994). As ischemic events are responsible for stroke in almost 80% of cases, even with the achievement of reperfusion via thrombolysis, stent retrievers or spontaneous reperfusion, I/R injuries have been shown to have deleterious and noteworthy effects of brain function and ischemic area after artery occlusion (Zhang et al., 1994). Animal studies have shown that the area damaged by the initial ischemic event can increase in size after repermeabilization of the affected artery, compared to continuous occlusion (Zhang et al., 1994). As pathophysiological mechanism may be possible targets for therapy and prevention of reperfusion injury, altering the BBB has been thought as the main mechanism involved. New evidence suggests multiple damage mechanism that can alter neuronal function in I/R injury such as the activation of the complement system (inhibition of which may yield less ischemia-reperfusion cardiac injury), the increase in leukocyte taxis to the affected area (the depletion of which can be a target in limiting reperfusion damage), cellular component damage, the stress caused by ROS and the activation of platelets can cause reperfusion damage and cerebral edema (Lin et al., 2016; Wu et al., 2018). Another molecular mechanism for brain damage after I/R concerns matrix metalloproteinases (MMPs) and their ability to interrupt endothelial junctions after restoration of blood flow (Candelario-Jalil et al., 2009). The vasogenic edema is caused by a biphasic “opening” of the BBB, with the early phase occurring several hours after reperfusion and being related to the activation of gelatinase A (MMP-2) and the second, 1 to 2 days after restoration of blood flow, associated with the expression and activation of gelatinase B (MMP-9) and stromelysin-1 (MMP3) (Rosenberg and Yang, 2007).
ROS are responsible for the damage to cellular components, such as mitochondria, nucleic acids and proteins (Brieger et al., 2012). Their role in reperfusion injury has long been presumed and recent data confirm that superoxide molecules can be produced after reperfusion following brain ischemia and molecules such as NADPH oxidase (NOX) can be involved in I/R injury in the brain and altering the BBB through their ability to transfer electrons to molecular oxygen (Kim et al., 2017b; Yang, 2019). The latter can be considered a way through which the mechanisms involved in I/R injury link to each other, especially when referring to the first phase of I/R brain injury related to the BBB in case of ischemic brain injury.
An important pathway that can lead to aggravating I/R injury is related to cellular component damage. ROS are causing damage to nucleic acids and macromolecules, as stated above, but also to mitochondria leading to ATP depletion, anaerobic metabolism and malfunctioning of ion pumps (Sanderson et al., 2013). The ischemia-reperfusion model in mitochondrial injury consists of calcium overload due to the altered function of the endoplasmic reticulum, which can generate ROS that may hyperpolarize the mitochondria membrane and surpass the antioxidants present in the cell (Wu et al., 2018). Excess reactive oxygen may escape from the electron transport chain and activate mechanisms that interfere with apoptosis and necrosis, while mitochondrial disfunction regarding fission and fusion becomes impaired during IR injury (Turrens, 2003; Andreyev et al., 2005). Besides an excess in ROS, reperfusion-induced inflammation also causes the release of cytokines, causing cytokine storm that ultimately injures the surrounding tissue (Eltzschig and Eckle, 2011).
Oxidative stress during I/R injury is thought to be caused by three different systems: xanthine oxidase system, NADPH oxidase (NOX) system and nitric oxide synthase (NOS) system (Cantu-Medellin and Kelley, 2013; Ma et al., 2017b). NOX-derived free oxygen radicals are known to cause the increase in local inflammatory cell presence and may lead to impaired perfusion of multiple organs (Sedeek et al., 2009; Meza et al., 2019). Even though the NOS system has a well-established role in providing nitric oxide as an antioxidant protective agent against I/R injury, it is also known that this type of injury can transform NOS into a superoxide generating system, with a resulting decrease in cellular NO and increase in ROS (Forstermann and Munzel, 2006). The free oxygen radicals can promote inflammation in the affected cells and can lead to cellular death (Lisa and Bernardi, 2006).
Inflammation represents a mechanism that has important implications in determining the amount of damage during reperfusion injury. This mechanism can yield effects through the cytokines, and molecules produced by the endothelium and parenchymal cells during I/R injury, but also by the number of leukocytes attracted to the damaged area. Oxidative stress, as mentioned above, can also be a means of aggravating ROS induced inflammation by increasing the expression of pro-inflammatory factors such as TNF-α and interleukin (IL)-1β (Turovsky et al., 2021). The adhesion of white blood cells to the endothelium, slow-rolling and trans-endothelial migration are augmented by flow restoration after ischemia, together with increased oxygen content. As more free oxygen radicals are produced, and leukocyte activation is ongoing due to danger signals, NADPH oxidase produces more ROS, neutrophils are able to release different cell damaging hydrolytic enzymes and generate hypochlorous acid via the activity of myeloperoxidase, pore-forming molecules being produced in the detriment of the vascular and parenchymal cells (Granger et al., 1993; Frangogiannis, 2015). Oxidative stress and NO depletion are also responsible for triggering humoral response to I/R injury as molecules such as TNF-α, IL-1, ANG II, LTB4 and PAF (linking the activation of platelets to neutrophil I/R damage) (García-Culebras et al., 2019). In addition to inflammation, complement system activation (C’) has been associated to I/R injury, both by increasing chemotaxis and activation in damage area leukocytes and activating the membrane attack complex to induce cellular damage (Gorsuch et al., 2012). Inhibiting the C5a fragment has also been shown to decrease neutrophile tissue infiltration (Wood et al., 2020). As inflammation is strongly linked to multiple types of cell death, nuclear factors that stimulate the expression of genes related to inflammation have been seen as a mechanism and also as a potential target during I/R injury. Different studies have supported this view, as strategies such as ulinastatin administration to mice undergoing temporary middle cerebral artery occlusion, which downregulates TLR4 and NF-kB expression, sodium butyrate administered during I/R injury of the lung and inhibiting NF-κB and JAK2/STAT3 signaling pathways or combination of octreotide and melatonin to alleviate the inflammasome-induced pyroptosis through the inhibition of TLR4-NF-κB-NLRP3 pathway in liver I/R injury, have clearly showed that NF-kB plays an important role in reperfusion injury (Li et al., 2017b; El-Sisi et al., 2021; Ying et al., 2021).
Neutrophils can adhere to the endothelial wall where necrosis factors expressed by injured cells are exhibited on the luminal surface and contact the leukocytes (such as P-selectin). After flow reestablishment, the cells are able to cytoskeletal shape-shift and adapt to linear flow, moving through an inter-endothelial pattern and eventually localizing points of entry by mechanism of actin polymerization and matrix metalloproteinase activity and gaps between pericytes (Nourshargh and Alon, 2014). Other immune cells such as lymphocytes, thrombocytes, mast cells or macrophages are also believed to play a role in I/R injury by increasing the presence of tissue neutrophils (Rodrigues and Granger, 2010). Platelets are also involved in attracting leukocytes and inducing I/R damage by their activation in the presence of inflammatory cytokines including PAF, due to the damage of endothelial cells, lack of NO, prostacyclin, and abundance of ROS (Esch et al., 2010; Franks et al., 2010).
In response to brain hypoxia/ischemia, miRNAs modulate a complex network of gene expression, for which they were proposed as potential and reproducible biomarkers in ischemic stroke due to a consistent correlation with neuropathological changes and prognosis of stroke (Vijayan and Reddy, 2016; Condrat et al., 2020). Several types of hypoxia/ischemia-sensitive miRNAs, whose blood levels are correlated with their brain circulating levels, were identified as potential clinical biomarkers in stroke: miR-210, miR-125a-5p, miR-125b-5p, and miR-143-3p (Zeng et al., 2011; Tiedt et al., 2017). MiRNAs influence gene expression in response to hypoxic/ischemic injury, and in turn the inflammatory responses triggered by ischemia-hypoxia dysregulate miRNA expression (Chen et al., 2020b). In the complex array of neuroinflammatory events, microRNAs are at the center of target gene regulation and modulation, microglia activation, cytokine production, cell apoptosis, mitochondrial disfunction and immune cell development, maintaining the vicious processes that lead to the progression and extension of neuronal damage (Chen et al., 2020b).
The most important of these processes are displayed in Figure 1.
Figure 1. General mechanisms in ischemia/reperfusion injury. Abbreviations: Akt, Protein kinase B; BBB, blood-brain barrier; C5, complement fraction 5; CXCL, C-X-C Motif Chemokine Ligand; DAMP, damage-associated molecular pattern; GPX4, glutathione peroxidase 4; IL, interleukin; LOOH, lipid alcohol; LOX, lipid peroxide; MAC, membrane attack complex; MMP, matrix metalloproteinase; NAD, nicotinamide adenine dinucleotide; NF-kB, nuclear factor kappa-light-chain-enhancer of activated B cells; NOS, nitric oxide synthase; NOX, NADPH oxidase; PI3K, phosphoinositide 3-kinases; ROS, reactive oxygen species; TLR, Toll-like receptor; TNF-α, tumoral necrosis factor α.
The inflammatory response is one of the major consequences of cerebral ischemia and miRNAs play an important role in its regulation. The involvement of several miRNAs in these pathways is presented in Tables 1, 2. Changes in the expression of inflammatory cytokines may occur after cerebral I/R injury (Wu et al., 2020). In lesions caused by I/R, inflammation is initiated by stagnant blood flow (vessel occlusion) and is then maintained by leukocytes activation and release of pro-inflammatory cytokines. Reducing or stopping the blood flow causes changes in the coagulation cascade, activates NF-kB and increases the expression of adhesion molecules on endothelial cells (Jurcau and Simion, 2021). Decreasing the amount of oxygen in the tissue causes varying degrees of damage. The first innate immune mechanism that is involved in this mechanism is the activation of toll-like receptors (TLRs). Activation of these receptors determines the activation of NF-kB, recognized as a pathway with a major role in the inflammatory response and with the ability to modulate several cytokines (TNF-α, IL-1β, and IL-6) and other mediators (iNOS, PGE2) (Shi et al., 2018; Yang et al., 2020). Microglia is the main factor involved in neuroinflammation. Its function and morphology are altered after ischemia. Activation of the microglia leads to its migration in and around the affected area (Hao et al., 2020). Together with the microglia, macrophages accumulate in the lesion (Islam et al., 2018). Following this activation process, the microglia release large amounts of pro-inflammatory cytokines (TNF-α, IL-6, IL-1β) that are considered to be the main factors involved in acute inflammation in ischemic stroke (Hao et al., 2020; Wang et al., 2020c).
I/R damage can be ameliorated by transforming growth factor β1 (TGF-β1), a cytokine with anti-inflammatory effects (Yang et al., 2020). TGF-β1 is a factor produced in large amounts in the lesion, starting on day 5 after reperfusion or later. A source of TGF-β1 may be the microglia and macrophages. The anti-inflammatory effect of TGF-β1 is thought to be a consequence of phosphorylation of the Smad protein by binding of this ligand to TGF-β receptors (Islam et al., 2018). Another member of the TGF family, TGF-β2, has a neuroprotective effect, being considered a neuroprotective factor. The expression of this protein is increased in animals with transient cerebral ischemia. Activation of the TGF-β2/Smad3 signaling pathway is essential for neuroprotection in ischemic brain injury (Peng et al., 2019).
The inflammatory response can be initiated by inflammasomes, complex molecular protein structures that are sensitive to cellular changes when homeostasis is lost (Franke et al., 2021). The main components of an inflammasome are a NLR sensor molecule, a pro-inflammatory caspase, and an adaptor protein (apoptosis-associated speck-like protein (ASC)) with a role in transmitting cellular signals (Hong et al., 2019; Caseley et al., 2020). Currently, the most studied inflammasome is nod-like receptor protein 3 (NLRP3). It plays an important role in various diseases with inflammatory components. Activation of NLRP3 leads to cerebral ischemia by releasing proinflammatory cytokines, such as IL-1β and IL-18. In the first stage after cerebral I/R injury, microglia become the main reservoir for activated NLRP3 inflammasome. In the following stages, NLRP3 are activated in both neurons and endothelial cells (Gao et al., 2017; Gong et al., 2018). The interaction between inflammasomes and TXNIP (thioredoxin interacting protein) leads to the activation of inflammation. In a normal, stress-free state, TXNIP is linked to Trx1 (thioredoxin1). Thus, NLRP3 is in inactive form. In stroke, a state with high oxidative stress, TXNIP and Trx1 dissociate and thus NLRP3 is activated. Nuclear factor erythroid 2-related factor 2 (Nrf2) is involved in the oxidative process and can interfere with processes that are consequences of oxidative stress. Trx1 has a neuroprotective effect against I/R and Nrf2 lesions by regulating the Trx1/TXNIP interaction negatively regulates NLRP3 inflammasome (Hou et al., 2018).
In I/R injuries, the first pathological event is represented by hypoxia due to ischemia. This causes cell death by mitochondrial damage and ROS formation. In the following phases, several inflammatory pathways are activated, besides the initial ROS events, all of which contribute to neuronal damage and loss of function (Jurcau and Simion, 2021).
Necrosis is the main form of cell death present in the hypoxic regions closest to the ischemic core. It is characterized by plasma membrane permeation and cell and organelle swelling (D’Arcy, 2019). It is caused by the intense stress caused by the lack of oxygen and nutrients in the ischemic areas. Necroptosis shares similar death-pattern characteristics to necrosis, but it is controlled by death signals and therefore, it is considered a form of programmed cell death (Wu et al., 2018). Necroptosis requires the presence of death signals, such as tumoral necrosis factor (TNF) receptor and the activity of receptor-interacting protein 1 (RIP1 or RIPK1) (Festjens et al., 2007; Vandenabeele et al., 2010). In cerebral I/R injuries, inhibiting RIP1 reduces the neuronal damage (Degterev et al., 2008; Kim et al., 2017a). Several other therapeutic approaches have been tested in murine models for reducing necroptosis, however, the data regarding miRNAs is scarce (Liao et al., 2020). Among the studies miRNAs, miR-497 and miR-369 seem to have a role in necroptosis by influencing the cellular response to TNF-α (Hsu et al., 2020; Yin et al., 2022).
Compared to necrosis, apoptosis is a coordinated formed of programmed cell death. It involves the activation of a complex cascade of processes and the activation of caspases, cysteine proteases with a pivotal role in this process (Elmore, 2007). In I/R injuries, it is present both in the initial hypoxic phase, as well as in the reperfusion state, but activated via different pathways (Wu et al., 2018). In the hypoxic phase, the intrinsic pathway plays a more important role, caused by the hypoxia-induces mitochondrial damage, which leads to the formation of apoptosomes and the activation of caspase 9, which leads to the activation of caspase 3 and the execution pathway. In the reperfusion state, the inflammatory mediators present in large amounts are responsible for the activation of the extrinsic pathway, where caspase 8 activation leads to caspase 3 activation and the execution pathway that includes DNA degradation, cytoskeletal reorganization and in the end, the formation of apoptotic bodies and cell death (Radak et al., 2017).
Apoptosis inhibition strategies were found to be effective in cerebral ischemia-reperfusion injury models, by reducing the extent of the infarct volume and improving the neurological score (Gong et al., 2017; Tang et al., 2020; Wang et al., 2021a). Biochanin A, an O-methylated isoflavone, reduced the expression of pro-apoptotic proteins Bax, Bcl-2, caspase-3 and caspase-12 in a model of middle cerebral artery occlusion and reperfusion (MCAO) (Guo et al., 2021b). Also, astragalin, another flavonoid reduced the expression of Bax and caspase-3, while upregulating the expression of Bcl-Xl (Chen et al., 2020a). Among these strategies, miRNA-based therapeutic approaches are presenting promising experimental results (Sun et al., 2018a; Liu et al., 2019b).
One of the most studied miRNAs in I/R pathologies is miR-124 (Liu et al., 2019b). In a rat model of MCAO, miR-124 presented as a promising biomarker for cerebral stroke injuries (Weng et al., 2011). Also, in patients with ischemic stroke, miR-124 as well as miR-9 were significantly elevated, supporting the idea of using miRNAs as biomarkers in I/R injuries (Ji et al., 2016). Another study in stroke patients showed the utility of miR-124-3p and miR-16 as biomarkers (Rainer et al., 2016).
In an experimental study, miR-211 downregulation increased the neurological damage and infarct volume of the mouse brain via a loss of Bcl-2-binding component 3 (BBC3) inhibition (Liu et al., 2020). BBC3 is also known as p53-up-regulated modulator of apoptosis and is part of the Bcl-2 protein family. Its main mechanism of action is interacting with other Bcl-2 family members proteins and promoting apoptosis (Nakano and Vousden, 2001). By upregulating miR-211, BBC3 was inhibited and the infarct size, neurological score and apoptosis were decreased. Another miRNA that acts by inhibiting BBC3 is miR-29a. In transient forebrain ischemia, miR-29a levels were decreased in the ischemic areas and its upregulation provided a protective effect in I/R injury (Ouyang et al., 2013). MiR-7-5p was upregulated in I/R injury models, degrading Sirtuin 1, a protein which alleviates I/R injuries, and therefore increasing neuronal apoptosis (Zhao and Wang, 2020; Diwan et al., 2021). In another study, miR-7-5p expression was reduced in MCAO rat models and its increase reduced the formation of ROS and inflammatory molecules and reduced the associated neuronal apoptosis (Xu et al., 2019). Similar results were found by Kim et al. in a rat model of I/R, where miR-7-5p levels were downregulated and pre-ischemic administration of miR-7 reduced I/R associated apoptosis and neuronal injury (Kim et al., 2018). The regulation of several other miRNAs has been studied in correlation with pro-apoptotic proteins or apoptosis, which are presented in Tables 1, 2.
Pyroptosis is considered a gasdermin (GSDM)-mediated programmed cell death (Shi et al., 2015). Compared to apoptosis, pyroptosis includes in its characteristics inflammation, as well as pore formation and cell swelling, with loss of cell membrane integrity. It includes the activation of caspases, however, these are different than in apoptosis, pyroptosis being activated by caspases 1, 4, 5, and 11 (Yu et al., 2021a). The canonical pathway in pyroptosis is characterized by cleaved-caspase 1 inflammasome formation, GSDM cleavage and release of IL-1β and IL-18 (Nunes and de Souza, 2013). The process by which pyroptosis is activated has been reviewed in detail by Yu et al. (2021a).
In cerebral I/R injuries, pyroptosis inhibition through the NF-kB pathway reduced the infarct volume and improved the neurological recovery. Also, inhibition of inflammasome formation via NLRP3 and NLRP1 regulation proved successful in improving neuronal survival and diminishing the impact of I/R injuries (Chen et al., 2020a; Sun et al., 2020b; Huang et al., 2021a). In this process, several miRNAs have been profiled to be activated and possible therapeutical targets for pyroptosis inhibition (Wang et al., 2020a). Gastrodin regulated the miR-22/NEAT1 axis and reduced the pro-inflammatory cytokines, reducing pyroptosis and attenuating the I/R injuries both in vivo and in vitro (Zhang et al., 2021a). MiR-124, which was previously discussed for apoptosis and was described as a marker of I/R injury, inhibits STAT3 expression and thereby reduces pyroptosis and improves the neurological outcome (Sun et al., 2020a). Overall, more studies are needed in order to fully elucidate how miRNAs regulation is related to pyroptosis and how these could potentially be used as therapeutic targets.
Ferroptosis is a recently described form of iron dependent cell death (Zhang et al., 2021c). Intracellular iron accumulation leads through the Fenton reaction to the formation of hydroxyl radicals that are ROS. ROS formation leads to lipid peroxidation (mainly phosphatidylethanolamine polyunsaturated fatty acids) that are destroying the lipid membranes, causing cell death. Ferroptosis is involved in several pathologies, including inflammatory pathologies, neurodegenerative diseases, cancers and I/R injuries (Liang et al., 2019; Capelletti et al., 2020; Li et al., 2020; Reichert et al., 2020; Sun et al., 2020c; Mitre et al., 2022). In mice experimental models of I/R injury, ferroptosis inhibition reduces the intestinal ischemic area and also protects the lungs and liver against ischemia-induced remote injuries (Li et al., 2019b,2020; Qiang et al., 2020; Deng et al., 2021). In acute myocardial infarction, ferroptosis inhibition by liproxstatin-1 presented promising results by reducing the infarct size in experimental studies (Lillo-Moya et al., 2021). More studies are needed to determine the clinical efficiency of ferroptosis-inhibiting strategies in I/R injuries.
In cerebral I/R injury, tau-mediated iron accumulation can trigger ferroptosis (Tuo et al., 2017). Ferroptosis activation increases the neuronal damage and the ischemic area (Zhao et al., 2022). Inhibiting this process by enhancing the expression of GPX4, the main regulatory enzyme of ferroptosis, leads to reduced neuronal deficit after ischemia and reduced neuronal death (Guan et al., 2019, 2021). These results are similar with other experimental studies, where ferroptosis inhibition by inhibiting its various pathways improved the neurological outcome and reduced the affected area in I/R injuries (Chen et al., 2021; Guo et al., 2021a; Wang et al., 2021b; Tuo et al., 2022; Xu et al., 2022).
In patients with acute ischemic stroke, miR-214 levels were downregulated. In mice, upregulating the levels of miR-214 reduced the infarct size and improved the neurological scores (Lu et al., 2020a). In oxygen-glucose deprivation, miR-194 upregulation improved cell survival and viability, as well as reduced the expression of ACSL4, while upregulating GPX4. These results indicate that miR-194 could potentially reduce ferroptosis and thus improve neuronal survival in vivo (Li et al., 2021d).
In I/R injuries, the reperfusion process provides a large amount of oxygen carried by the red blood cells to the ischemic site. At the same time, the rapid alterations in oxygen flow allows the generation of ROS. Ischemia also modifies the concentration of antioxidative agents, which leads to greater damage caused by the generated ROS. In the ischemia stage, ATP production is reduced. Consecutively, the function of ion-exchange channels and enzymes is altered, leading to mitochondrial dysfunction and electrolytes imbalance. In these circumstances, the oxidative stress pathways are further activated: the NADPH oxidase (NOX) complex, the inducible nitric oxide (iNOS) complex and the xanthine oxidase complex (Wu et al., 2018).
Mitochondria is the main source for ROS synthesis due to the electron chains from the mitochondrial inner membrane, NOXs and mitochondrial redox carriers complexes I and III. In physiological states, the generation of ROS, like superoxide anion, hydrogen peroxide and hydroxide radical, is at a low level and antioxidants, like superoxide dismutase (SOD), catalases, glutathione peroxidase (GSHPx) and glutathione, control any excess of ROS (Hu et al., 2015). The excessive production or delayed elimination of ROS is often a starting point for CIRI. An excessive amount of ROS in the brain interacts with structural molecules, such as proteins, lipids, carbohydrates and nucleic acids, affecting the neuronal biochemical processes and promoting neuronal death. The main mechanisms involved in ROS toxicity are: mitochondrial membrane lipid peroxidation, cross-linking of molecules, like nucleic acids, proteins and carbohydrates that alter their function in biochemical processes, endothelial damage of the BBB and consecutively increased permeability, activation of inflammatory key factors, like cytokines and adhesion molecules, and increased synthesis of excitatory amino acids (EAA), involved in delayed neuron death (Wu et al., 2020).
Oxidative stress is involved in DNA damage, local inflammation and endothelial dysfunction. Nuclear factor (erythroid-derived 2) -related factor 2 (Nrf2) is an antioxidant regulator activated in oxidative stress conditions that upregulate the expression of antioxidant genes, like superoxide dismutase (SOD), heme-oxygenase-1 (HO-1), NADPH- quinone oxidoreductase 1 (NQO1) and glutathione S transferase (GST) (Chen et al., 2015).
Li et al. (2019a) showed that theaflavin has an antioxidant and neuroprotective effect in a rat model of I/R injury and in neural stem cells subjected to oxygen-glucose deprivation and reoxygenation (OGD/R), increasing the expression of Nrf2 by downregulating miRNA-128-3p. The study confirmed that the miRNA-128-3p level of expression is increased in CIRI, and it is responsible for ROS generation.
Zhao et al. (2014) demonstrated that miR-23a-3p is increased in a CIRI mice model, a protective trial mechanism activated to increase the antioxidant ability of the neurons and to suppress oxidative stress. MiR-23a-3p agomir decreased the synthesis of nitric oxide (NO), 3-nitrotyrosine and hydrogen peroxide-induced lactate dehydrogenase release and increased the expression of manganese superoxide dismutase, an enzyme that protects the mitochondrial energy network from oxidative stress damage. Another similar study found out that miR-424 levels increased at 1 and 4 h and decreased at 24 h after reperfusion in an I/R mice model. MiR-424 agomir decreased the level of excessive ROS and lipid peroxidation product malondialdehyde (MDA) generated after reperfusion and increased the expression of SOD and Nrf2. The study concluded that miR-424 activates an antioxidant mechanism in CIRI to limit further damage (Liu et al., 2015).
Huang R and the collaborators suggested that the reduced level of miR-34b expression in focal cerebral I/R is associated with oxidative stress parameters and decreased antioxidant ability. They showed that overexpression of miR-34b ameliorates CIRI through suppression of Keap1 and increase of Nrf2 and heme oxygenase (HO-1). Kelch-like ECH-associated protein 1 (Keap1)/Nrf2/ARE signaling pathway has been proved to be an important antioxidant mechanism and a potential target for miR-34b (Huang et al., 2019). Nrf2/ARE inhibition and excessive ROS production are common mechanisms that involve other miRNAs downregulation, such as miR-98-5p or miR-135b-5p (Duan et al., 2018; Sun et al., 2018b).
Wei et al. (2015) concluded that the miR-200 family increases ROS production, reduces mitochondrial membrane potential and modulates apoptosis network during CIRI, especially miR-200a-3p, miR-200b-3p and miR-429. The imbalance between ROS excessive production (MDA) and reduced antioxidant (SOD) ability causing oxidative stress damage is also determined by miR-106b-5p upregulation. MiR-106b-5p accentuates neurons death by involving the Bcl-2 family proteins, with the pro-apoptotic protein Bax and antiapoptotic protein B cell lymphoma-2 balance dysregulation (Bcl-2). Li et al. (2017a) reported that miR-106b-5p antagomir ameliorates the oxidative stress imbalance and activates antiapoptotic proteins, like Bcl-2 and myeloid cell leukemia-1 (Mcl-1). MiR-421 is also upregulated in CIRI and seems to activate the same pathological mechanisms (Yue et al., 2020). Nrf2/ARE mediated antioxidant pathways inhibition and ROS excessive production were described in a large number of studies referring to miRNAs upregulation: miR-153 (Ji et al., 2017), miR-93 (Wang et al., 2016), miR-142-5p (Wang et al., 2017) and miR-302b-3p that also targets fibroblast growth factor 15 (FGF15) (Zhang et al., 2019b).
Mitochondrial pathways involved in the survival of the cell are ATP production and synthesis of different molecules used in signaling networks. Mitochondria environment is also a place for miRNAs mediated posttranscriptional regulation, affecting energy metabolism, biochemical homeostasis and the activity of enzymes related to oxidative stress pathways. In CIRI, mitochondrial damage is involved in pathophysiological processes, such as ROS excessive production, reduced antioxidant activity, energy metabolism dysregulation and neuronal apoptosis (Hu et al., 2015).
To establish a possible interaction between miRNAs and mitochondrial damage, Xia et al. (2020) designed a model of OGD/R in primary cortical neuron culture. They proved that the decreased expression of miR-142-3p is involved in mitochondrial dysfunction and suggested that miR-142-3p regulates enzymes involved in mitochondrial biogenesis and function, such as electron transfer chain complexes I-III, peroxisome proliferator-activated receptor- γ coactivator-1α (PGC1α), mitochondrial transcription factor A (TFAM), and nuclear respiratory factor 1 (NRF1). Moreover, miR-142-3p overexpression improves mitochondrial function by decreasing the ROS toxic effects due to inhibition of NOX2/Rac Family Small GTPase 1 (Rac1)/ROS signaling pathway (Xia et al., 2020).
NADPH oxidase (NOX) is a family of 7 enzymes, NOX1 to NOX5 and dual oxidase (Duox-1 and Duox-2). NOX2 and NOX4 have been described as important enzymes that coordinate neuronal apoptosis and ROS generation in CIRI (Liang et al., 2018; Zuo et al., 2020).
Protein kinase CK2 (casein kinase 2) is a kinase that phosphorylates a large number of different substrates; therefore, it is involved in different cellular processes. It has been outlined that CK2 has a neuroprotective effect in CIRI by downregulating NADPH oxidases NOX2 and NOX4. Both in vivo and in vitro studies concluded that miR-125b is upregulated in I/R injury, while CK2α is decreased and proved that mi-R-125b binds with 3′UTR of CK2α and directly suppresses CK2 levels, resulting in NOX2 and NOX4 activation and ROS overproduction and neuronal apoptosis (Liang et al., 2018). Zuo et al. (2020) showed that miR-652 is significantly decreased, while the expression of NOX2 is increased in a CIRI rat model and in a cell hypoxia/reoxygenation (H/R) model. Overexpression of miR-652 in H/R cells reduced NOX2 expression and ROS production and ameliorated brain tissue CIRI (Zuo et al., 2020). A similar study that used both in vitro and in vivo CIRI models found out that miR-532-3p level of expression is reduced and NOX2 level is increased and suggested that miR-532-3p downregulation may be a part of CIRI through the NOX2 pathway (Mao et al., 2020).
The downregulation of several miRNAs in the ischemic brain tissue in hyperglycemic rats has been associated with NOX2 and NOX4 genes: miRNA-29a-5p, miRNA-29c-3p, miRNA-126a-5p, miRNA-132-3p, miRNA-136-3p, miRNA-138-5p, miRNA-139-5p, miRNA-153-5p, miRNA-337-3p, and miRNA-376a-5p. NOX2 was identified as the target gene of miR-126a-5p whereas NOX4 was the target gene of miR-29a-5p, miR-29c-3p and miR-132-3p (Liu et al., 2017). NOX4 was also studied as a target for miR-25, miR-92a and miR-146a. In an experimental study of CIRI, the expression levels of miR-25, miR-92a and miR-146a were decreased, but the NOX4 protein expression was increased in the interventional group. Treatment with isoflavones resulted in decreased ROS generation and neuronal cell death related to the inhibition of NOX4 via the induction of NOX4-related miRNAs (Hong et al., 2018).
Alongside with oxidative stress, apoptosis and inflammation, disruption of BBB and subsequent increased permeability of BBB, results in myelin sheath damage and brain edema, leading to neuronal dysfunction (Haley and Lawrence, 2017; Jiang et al., 2018a; Ma et al., 2020). BBB dysfunction has been ascertained in multiple brain disorders, including stroke, traumatic brain injury (TBI), MS, epilepsy, AD, amyotrophic lateral sclerosis and PD (Daneman, 2012; Kamphuis et al., 2015). The main pathways activated upon BBB disruption consists of tight junction protein degradation, microvascular endothelial cells (ECs) damage, immune cell infiltration and activation of cytokine expression (Shen and Ma, 2020). MiRNAs have been shown to modulate BBB function under various pathological conditions, from: ischemic brain injury, TBI, spinal cord injury to neurodegenerative diseases (AD, Vascular dementia), brain tumors and cerebral infections (Ma et al., 2020).
In MCAO-induced CRTC1 knockout mice model, reduced levels of miRNA-132/212 have been correlated with aggravated BBB permeability and increased infarct volume. Moreover, miRNA-132 promotes BBB integrity expression, by binding to 3-UTR regions of the target genes of tight junction-associated protein-1 (TJAP-1), claudin-1, thus repressing junction protein’s expression (Yan et al., 2021). Peripheral blood samples of 48 cerebrovascular patients revealed decreased levels of miR-539, which was related to impaired BBB. By binding to SNAI2, miR-539 has been shown to restore endothelial cell permeability by repressing MMP9 signaling pathway (Li et al., 2021a).
The expression of intercellular junctions could also be regulated by miR-27a-3p mimics via upregulating the protein expression of claudin-5 and occludin, thus impairing BBB permeability in CMEC/D3cells model (Harati et al., 2022). In MCAO-induced miR-182 KD (knockout) mice, the integrity of BBB was restored, with increased expression of tight junction proteins (Zhang et al., 2020b).
The cellular components of BBB have also been regulated by miRNAs upon ischemic insult. In ischemic rat brain and cultured pericytes, miR-149-5 expression was decreased. Downregulation of miR-149-5p expression enhances S1PR2 in pericytes, which was associated with decreased N-cadherin expression and increased pericyte migration, thus aggravating BBB integrity. Intracerebroventricular injection of agomir-149-5p has been shown to increase the level of N-cadherin and decrease pericyte migration, ameliorating BBB dysfunction (Wan et al., 2018).
Vascular endothelium poses important roles in BBB homeostasis and integrity (Hawkins and Davis, 2005). The integrity of BBB depends on the ‘injury’ status of brain microvascular endothelial cells (BMECs), suggesting that protecting BMECs represents a therapeutic strategy against ischemic stroke. CI/R injury induces autophagy in BMECs, and in turn autophagy further protects BMECs upon CI/R injury, suggesting the protective mechanism of autophagy on BMECs exposed to OGD/R injury (Li et al., 2014a). Ln RNA Malat1 promotes down-regulation of miR-26b to promote neuroprotective effects in CI/R injury by stimulating autophagy of BMECs (Li et al., 2017c).
Multiple studies evidenced that JAK2/STAT3 signaling pathways have been activated after ischemic stroke, posing neuropathogenic roles in I/R injury (Liang et al., 2016). Interestingly, silencing JAK2/STAT3 pathway has been associated with up-regulation expression levels of miRNAs in various pathological settings, including hepatopulmonary syndrome rat model, pancreatic cancer cells (Wang et al., 2015a; Yin et al., 2022).
In MCAO mice model and OGD-induced neuronal cells dysfunction, miR-216a was down-regulated. Overexpression of miR-216a exhibited neuroprotective effects against I/R injury by negatively regulating JAK2/STAT3 signaling pathway (Tian et al., 2018).
Mitogen-activated protein kinases pathway (MAPKs) participate in signal transduction, exerting regulatory roles on cell death and survival, being involved in different biological processes, including differentiation, cell proliferation and apoptosis (Nozaki et al., 2001; Imajo et al., 2006). Under ischemic conditions, MAPK activated inflammatory processes and promoted neuronal cell death, the expression level of MAPK being highly expressed in the cerebral macrophages from the ischemic core after stroke (Madhyastha et al., 2012; Wang et al., 2019; Xie et al., 2019; Zeng et al., 2019).
MiR-22 ameliorates the neuroinflammatory responses in vivo and in vitro animal models of I/R injury, by suppressing p38 MAPK/NF-κB pathways (Dong et al., 2019). In ischemic rat model, miR-145 exhibited low expression levels, which was associated with suppressing the MAPK pathways. Interestingly, in rat neuronal stem cells (NSCs), miR-145, p38 and ERK increased in a cultured time-dependent manner, suggesting the neuroprotective mechanisms promoted with growth of the NSCs. miR-145 promoted NSCs proliferation and inhibited apoptosis, whereas MAPK’s inhibitor (SB203580) enhanced apoptosis and inhibited NSCs proliferation. After cerebral injection of NSCs in the ischemic rat cortex, the walking ability and neurological impairment of ischemic stroke rats improved over time, miR-145 playing critical roles in NSCs-promoted recovery of ischemic rat cortex, by targeting MAPK pathway (Xue et al., 2019). Moreover, miR-339 accelerated the progression of I/R injury in MCAO-rat model and PC12 cells exposed to OGD/R treatment, by stimulating proliferation and apoptosis of neuronal cells. The deleterious effects of miR-339 on neuronal injury proceed via inhibiting FGF9/CACNG2 axis, thus activating MAPK signaling pathway in ischemic stroke (Gao et al., 2020, 2). MiR-410 exhibited low levels in I/R mouse model and miR-410 mimic transfection reversed neuron apoptosis and enhanced hippocampal neuron survival via suppressing TIMP2-dependent MAPK pathway (Liu et al., 2018). Moreover, miR-410 overexpression decreased expression levels of TIMP2, p38, JNK and ERK proteins (Liu et al., 2018).
Hypoxia-inducible factor-1 (HIF-1), transcription factor, activated in response to oxygen levels fluctuations, modulates gene expression aimed at facilitating cell adaptation in hypoxic conditions (Sharp and Bernaudin, 2004; Shi, 2009). Noteworthy, hypoxic/pharmacological induction of HIF-1 in vivo and in vitro ischemic stroke models elicited neuroprotection against ischemic insult by promoting antiapoptotic mechanisms and contributing to the neuronal cell’s survival (Siddiq et al., 2005; Baranova et al., 2007). However, depending on the intensity of the injurious stimulus and duration of ischemia, HIF-1 might promote both cell survival in mild hypoxic conditions or neuron apoptosis in long-term hypoxia (Helton et al., 2005; Baranova et al., 2007). Serum samples of 52 ischemic stroke patients showed a lower miR-210 expression level, with a variable mean of miR-210 between different time points (time of admission and 3 months after stroke) and a higher HIF-1α levels, which does not change in a time-dependent manner. Increased expression levels of miR-210 and decreased HIF-1α levels exhibited a better survival rate in these patients (Rahmati et al., 2021). In OGD/R induced neuroblastoma cells microRNA-186 elicited antiapoptotic effects, by downregulating HIF-1α (Li et al., 2021b). PC12 cells exposed to OGD/R injury exhibited elevated miR-134 and HIF-1α expression levels. HIF-1α overexpression may alleviate OGD/R-induced injury, by suppressing miR-134 expression (Zhang et al., 2020a). Moreover, by inhibiting miR-134 expression, HIF-1α induces the activation of ERK1/2 and JAK1/STAT3 pathways (Zhang et al., 2020a).
Vascular endothelial growth factor (VEGF), a pro-angiogenic factor which modulates vasculogenesis and neoangiogenesis, presents essential properties in both physiological and pathological conditions, such as wound healing and repair, pregnancy, diabetic retinopathy, tumor growth and metastasis, and ischemic processes, myocardial infarction, and ischemic stroke (Melincovici et al., 2018; Shim and Madsen, 2018). VEGF regulates cerebral angiogenesis after stroke, promoting either restoration of blood supply after ischemic injury, or promoting BBB disruption by increasing vascular permeability (Zhang et al., 2017; Geiseler and Morland, 2018). The beneficial or deleterious effects promoted by VEGF depends on the level of expression of VEGF. For instance, an elevated VEGF expression leads to neurological deterioration, whereas an appropriate level of VEGF sustains the recovery process of brain in response to hypoxia (Zhang et al., 2021b).
Brain Microvascular Endothelial Cells (BMVEC) exposed to OGD elicited increased level of VEGF and reduced miR-150 expression. In OGD-induced BMVEC cells, downregulation of miR-150 and upregulated its predicted target, MYB induced VEGF expression, thus regulating cerebral angiogenesis after ischemic stroke (Zhang et al., 2021b). Serum samples from 78 diabetic and non-diabetic patients with ischemic stroke (acute ischemic stroke or transient ischemic attack) revealed a high level of miRNA-195-5p and miRNA-451a at 0, 24, and 72 hours after the stroke event, with low levels of BDNF and VEGF-A at the same time-points (Giordano et al., 2020).
The brain derived neurotrophic factor (BDNF), crucial neurotrophic factor involved in the regulation process of synaptic transmission and brain plasticity activity, promotes neuroprotective effects in hypoxic and excitotoxic-induced neuron cell death (Degos et al., 2013; Miranda et al., 2019).
Besides transcriptional and translational regulation, BDNF expression might be regulated upon post-transcriptional level, by epigenetic mechanisms, including neuronal activity, hormones environmental factors such as exercise and stress (Metsis et al., 1993; Lubin et al., 2008; Miranda et al., 2019). The expression levels of BDNF have a high reach in hippocampus, being also detected in the cerebellum, cerebral cortex and amygdala (Hofer et al., 1990). In MCAO mice model, upregulated level of miR-191-5p was associated with disturbed angiogenesis, by inhibiting BDNF, suggesting the neuroprotective mechanisms promoted by miR-191-5p inhibition (Wu et al., 2021). In OGD-induced mouse neurons and astrocytes, inhibiting miR-128 by treatment with ARPP21 antagonistic intron exhibited up-regulation of BDNF and CREB1, therefore inhibiting apoptosis and promoting neurological recovery against ischemic stroke (Chai et al., 2021).
Mounting evidence revealed the involvement of PI3K/Akt signaling pathway in cerebral ischemic/hypoxic injury, emerging new promising strategy for ischemic stroke (Zhang et al., 2018). By phosphorylating the inositol group in the plasma membrane phospholipids, PI3K/Akt pathway acts as a critical regulator of multifold cell processes, including cell growth, proliferation, coagulation, inflammation under different physiological and pathological settings (Fruman et al., 1998; Li et al., 2008).
Activation of the PI3K/Akt pathway by increasing miR-18b exhibited decreased apoptosis rate and reduced neuroinflammation in OGDR induced SH-SY 5Y cell dysfunction and MCAO mice model (Min et al., 2020). MiR-22 exhibited low expression level in cerebral I/R injury. Treatment with miR-22 mimic in MCAO rat model revealed increased levels of serum VEGF and Ang-1 and the levels of p-PI3K/PI3K and p-Akt/Akt proteins. Thus, miR-22 promoted angiogenic and neuroprotective effects in ischemia/reperfusion injury by activating PI3K/Akt signaling pathway (Wang et al., 2020b).
Aquaporin (AQP)-4, the active regulator of water flux, poses critical role in edema formation, emerging new therapeutic targets for counteracting vascular edema in ischemic stroke (Zador et al., 2009). In this context, miR-29b, 130a and -32 were shown to repress AQP-4 (Sepramaniam et al., 2010, 2012; Wang et al., 2015b). MiR-29b overexpression promoted neuroprotection in ischemic stroke, by ameliorating BBB disruption upon ischemic stroke. Moreover, AQP-4 expression significantly decreased after miR-29b overexpression (Wang et al., 2015b, 4). Treatment of OGD-induced human astrocytoma cells injury and MCAO rat model with anti-miR-320a exhibited decreased infarct volume of cerebral ischemia, via upregulation of AQP1 and 4 (Sepramaniam et al., 2010).
All these mechanisms are simultaneously present during I/R injury and it is hard to separate these events from each other. MiRNAs are interlinked with oxidative stress damage, inflammatory mediators production, inflammation and cell death. As a general rule, “reversing” the expression of the miRNAs involved in cerebral I/R injuries (inhibiting an over-expressed miRNA or mimicking the effect of a down-regulated miRNA) improved the outcome and studied parameters. This holds true for the majority of studies and could mean that a miRNA-centered therapeutic approach could be beneficial. Although experimental in vivo and in vitro models showed outcome improvements when analyzing one pathway and miRNA, it is very likely that in a clinical setting these strategies to be insufficient. It could be that by inhibiting one pathway, another one to over-express or that the benefit of such therapies to be clinically insignificant. Further research is needed to determine the exact roles of miRNAs and of miRNAs stimulation or inhibition in I/R injuries and to determine the most favorable candidates as treatment options.
M-AN, A-OM, C-CB, A-II, CM, MB, and C-SM were involved in the literature search and writing of the manuscript. A-OM, C-CB, and MB prepared the figure and tables. M-AN, A-OM, and A-DB performed the critical reading of the manuscript. All authors contributed to manuscript preparation and revision and reviewed the final version making the necessary changes and approved the submitted version.
The authors declare that the research was conducted in the absence of any commercial or financial relationships that could be construed as a potential conflict of interest.
All claims expressed in this article are solely those of the authors and do not necessarily represent those of their affiliated organizations, or those of the publisher, the editors and the reviewers. Any product that may be evaluated in this article, or claim that may be made by its manufacturer, is not guaranteed or endorsed by the publisher.
Andreyev, A. Y., Kushnareva, Y. E., and Starkov, A. A. (2005). Mitochondrial metabolism of reactive oxygen species. Biochem. Biokhimiia 70, 200–214. doi: 10.1007/s10541-005-0102-7
Angelou, C. C., Wells, A. C., Vijayaraghavan, J., Dougan, C. E., Lawlor, R., Iverson, E., et al. (2019). Differentiation of pathogenic Th17 cells is negatively regulated by let-7 micrornas in a mouse model of multiple sclerosis. Front. Immunol. 10:3125. doi: 10.3389/fimmu.2019.03125
Anrather, J., and Iadecola, C. (2016). Inflammation and stroke: an overview. neurother. J. Am. Soc. Exp. Neurother. 13, 661–670. doi: 10.1007/s13311-016-0483-x
Baird, A. E., Donnan, G. A., Austin, M. C., Fitt, G. J., Davis, S. M., and McKay, W. J. (1994). Reperfusion after thrombolytic therapy in ischemic stroke measured by single-photon emission computed tomography. Stroke 25, 79–85. doi: 10.1161/01.STR.25.1.79
Baranova, O., Miranda, L. F., Pichiule, P., Dragatsis, I., Johnson, R. S., and Chavez, J. C. (2007). Neuron-specific inactivation of the hypoxia inducible factor 1 alpha increases brain injury in a mouse model of transient focal cerebral ischemia. J. Neurosci. Off. J. Soc. Neurosci. 27, 6320–6332. doi: 10.1523/JNEUROSCI.0449-07.2007
Bartel, D. P. (2009). MicroRNAs: target recognition and regulatory functions. Cell 136, 215–233. doi: 10.1016/j.cell.2009.01.002
Bernstein, D. L., and Rom, S. (2020). Let-7g* and miR-98 reduce stroke-induced production of proinflammatory cytokines in mouse brain. Front. Cell Dev. Biol. 8:632. doi: 10.3389/fcell.2020.00632
Bernstein, D. L., Zuluaga-Ramirez, V., Gajghate, S., Reichenbach, N. L., Polyak, B., Persidsky, Y., et al. (2020). Mir-98 reduces endothelial dysfunction by protecting blood-brain barrier (BBB) and improves neurological outcomes in mouse ischemia/reperfusion stroke model. J. Cereb. Blood Flow Metab. Off. J. Int. Soc. Cereb. Blood Flow Metab. 40, 1953–1965. doi: 10.1177/0271678X19882264
Brieger, K., Schiavone, S., Miller, F. J., and Krause, K.-H. (2012). Reactive oxygen species: from health to disease. Swiss Med. Wkly. 142:w13659. doi: 10.4414/smw.2012.13659
Calin, G. A., Liu, C.-G., Sevignani, C., Ferracin, M., Felli, N., Dumitru, C. D., et al. (2004). MicroRNA profiling reveals distinct signatures in B cell chronic lymphocytic leukemias. Proc. Natl. Acad. Sci. U. S. A. 101, 11755–11760. doi: 10.1073/pnas.0404432101
Canazza, A., Minati, L., Boffano, C., Parati, E., and Binks, S. (2014). Experimental models of brain ischemia: a review of techniques, magnetic resonance imaging, and investigational cell-based therapies. Front. Neurol. 5:19. doi: 10.3389/fneur.2014.00019
Candelario-Jalil, E., Yang, Y., and Rosenberg, G. A. (2009). Diverse roles of matrix metalloproteinases and tissue inhibitors of metalloproteinases in neuroinflammation and cerebral ischemia. Neuroscience 158, 983–994. doi: 10.1016/j.neuroscience.2008.06.025
Cantu-Medellin, N., and Kelley, E. E. (2013). Xanthine oxidoreductase-catalyzed reactive species generation: a process in critical need of reevaluation. Redox. Biol. 1, 353–358. doi: 10.1016/j.redox.2013.05.002
Cao, M., Song, W., Liang, R., Teng, L., Zhang, M., Zhang, J., et al. (2021). MicroRNA as a potential biomarker and treatment strategy for ischemia-reperfusion injury. Int. J. Genomics 2021:e9098145. doi: 10.1155/2021/9098145
Capelletti, M. M., Manceau, H., Puy, H., and Peoc’h, K. (2020). Ferroptosis in liver diseases: an overview. Int. J. Mol. Sci. 21:E4908. doi: 10.3390/ijms21144908
Caseley, E. A., Poulter, J. A., Rodrigues, F., and McDermott, M. F. (2020). Inflammasome inhibition under physiological and pharmacological conditions. Genes Immun. 21, 211–223. doi: 10.1038/s41435-020-0104-x
Chai, Z., Gong, J., Zheng, P., and Zheng, J. (2020). Inhibition of miR-19a-3p decreases cerebral ischemia/reperfusion injury by targeting IGFBP3 in vivo and in vitro. Biol. Res. 53:17. doi: 10.1186/s40659-020-00280-9
Chai, Z., Zheng, P., and Zheng, J. (2021). Mechanism of ARPP21 antagonistic intron miR-128 on neurological function repair after stroke. Ann. Clin. Transl. Neurol. 8, 1408–1421. doi: 10.1002/acn3.51379
Chen, B., Lu, Y., Chen, Y., and Cheng, J. (2015). The role of Nrf2 in oxidative stress-induced endothelial injuries. J. Endocrinol. 225, R83–R99. doi: 10.1530/JOE-14-0662
Chen, W., Jiang, L., Hu, Y., Tang, N., Liang, N., Li, X.-F., et al. (2021). Ferritin reduction is essential for cerebral ischemia-induced hippocampal neuronal death through p53/SLC7A11-mediated ferroptosis. Brain Res. 1752:147216. doi: 10.1016/j.brainres.2020.147216
Chen, X., Cheng, C., Zuo, X., and Huang, W. (2020a). Astragalin alleviates cerebral ischemia-reperfusion injury by improving anti-oxidant and anti-inflammatory activities and inhibiting apoptosis pathway in rats. BMC Complement. Med. Ther. 20:120. doi: 10.1186/s12906-020-02902-x
Chen, Y.-M., He, X.-Z., Wang, S.-M., and Xia, Y. (2020b). δ-opioid receptors, microRNAs, and neuroinflammation in cerebral ischemia/hypoxia. Front. Immunol. 11:421. doi: 10.3389/fimmu.2020.00421
Cho, K. H. T., Xu, B., Blenkiron, C., and Fraser, M. (2019). Emerging roles of miRNAs in brain development and perinatal brain injury. Front. Physiol. 10:227. doi: 10.3389/fphys.2019.00227
Chua, C. E. L., and Tang, B. L. (2019). miR-34a in neurophysiology and neuropathology. J. Mol. Neurosci. MN 67, 235–246. doi: 10.1007/s12031-018-1231-y
Condrat, C. E., Thompson, D. C., Barbu, M. G., Bugnar, O. L., Boboc, A., Cretoiu, D., et al. (2020). miRNAs as biomarkers in disease: latest findings regarding their role in diagnosis and prognosis. Cells 9:276. doi: 10.3390/cells9020276
Daneman, R. (2012). The blood-brain barrier in health and disease. Ann. Neurol. 72, 648–672. doi: 10.1002/ana.23648
D’Arcy, M. S. (2019). Cell death: a review of the major forms of apoptosis, necrosis and autophagy. Cell Biol. Int. 43, 582–592. doi: 10.1002/cbin.11137
Degos, V., charpentier, T. L., Chhor, V., Brissaud, O., Lebon, S., Schwendimann, L., et al. (2013). Neuroprotective effects of dexmedetomidine against glutamate agonist-induced neuronal cell death are related to increased astrocyte brain-derived neurotrophic factor expression. Anesthesiology 118, 1123–1132. doi: 10.1097/ALN.0b013e318286cf36
Degterev, A., Hitomi, J., Germscheid, M., Ch’en, I. L., Korkina, O., Teng, X., et al. (2008). Identification of RIP1 kinase as a specific cellular target of necrostatins. Nat. Chem. Biol. 4, 313–321. doi: 10.1038/nchembio.83
Del Zoppo, G. J., Saver, J. L., Jauch, E. C., Adams, H. P., and American Heart Association Stroke Council (2009). Expansion of the time window for treatment of acute ischemic stroke with intravenous tissue plasminogen activator: a science advisory from the American Heart Association/American Stroke Association. Stroke 40, 2945–2948. doi: 10.1161/STROKEAHA.109.192535
Deng, F., Zhao, B.-C., Yang, X., Lin, Z.-B., Sun, Q.-S., Wang, Y.-F., et al. (2021). The gut microbiota metabolite capsiate promotes Gpx4 expression by activating TRPV1 to inhibit intestinal ischemia reperfusion-induced ferroptosis. Gut Microbes 13, 1–21. doi: 10.1080/19490976.2021.1902719
Deng, Y., Ma, G., Dong, Q., Sun, X., Liu, L., Miao, Z., et al. (2019). Overexpression of miR-224-3p alleviates apoptosis from cerebral ischemia reperfusion injury by targeting FIP200. J. Cell. Biochem. 120, 17151–17158. doi: 10.1002/jcb.28975
Di, Y., Lei, Y., Yu, F., Changfeng, F., Song, W., and Xuming, M. (2014). MicroRNAs expression and function in cerebral ischemia reperfusion injury. J. Mol. Neurosci. MN 53, 242–250. doi: 10.1007/s12031-014-0293-8
Dirnagl, U. (2006). Bench to bedside: the quest for quality in experimental stroke research. J. Cereb. Blood Flow Metab. Off. J. Int. Soc. Cereb. Blood Flow Metab. 26, 1465–1478. doi: 10.1038/sj.jcbfm.9600298
Diwan, D., Vellimana, A. K., Aum, D. J., Clarke, J., Nelson, J. W., Lawrence, M., et al. (2021). Sirtuin 1 mediates protection against delayed cerebral ischemia in subarachnoid hemorrhage in response to hypoxic postconditioning. J. Am. Heart Assoc. 10:e021113. doi: 10.1161/JAHA.121.021113
Dong, H., Cui, B., and Hao, X. (2019). MicroRNA-22 alleviates inflammation in ischemic stroke via p38 MAPK pathways. Mol. Med. Rep. 20, 735–744. doi: 10.3892/mmr.2019.10269
Duan, Q., Sun, W., Yuan, H., and Mu, X. (2018). MicroRNA-135b-5p prevents oxygen-glucose deprivation and reoxygenation-induced neuronal injury through regulation of the GSK-3β/Nrf2/ARE signaling pathway. Arch. Med. Sci. 14, 735–744. doi: 10.5114/aoms.2017.71076
Elmore, S. (2007). Apoptosis: a review of programmed cell death. Toxicol. Pathol. 35:495. doi: 10.1080/01926230701320337
El-Sisi, A. E.-D. E.-S., Sokar, S. S., Shebl, A. M., Mohamed, D. Z., and Abu-Risha, S. E.-S. (2021). Octreotide and melatonin alleviate inflammasome-induced pyroptosis through inhibition of TLR4-NF-κB-NLRP3 pathway in hepatic ischemia/reperfusion injury. Toxicol. Appl. Pharmacol. 410:115340. doi: 10.1016/j.taap.2020.115340
Eltzschig, H. K., and Eckle, T. (2011). Ischemia and reperfusion–from mechanism to translation. Nat. Med. 17, 1391–1401. doi: 10.1038/nm.2507
Esch, J. S. A., Jurk, K., Knoefel, W. T., Roeder, G., Voss, H., Tustas, R. Y., et al. (2010). Platelet activation and increased tissue factor expression on monocytes in reperfusion injury following orthotopic liver transplantation. Platelets 21, 348–359. doi: 10.3109/09537101003739897
Fang, H., Li, H.-F., He, M.-H., Yang, M., and Zhang, J.-P. (2021). HDAC3 Downregulation improves cerebral ischemic injury via regulation of the SDC1-Dependent JAK1/STAT3 signaling pathway through miR-19a upregulation. Mol. Neurobiol. 58, 3158–3174. doi: 10.1007/s12035-021-02325-w
Fang, Z., He, Q.-W., Li, Q., Chen, X.-L., Baral, S., Jin, H.-J., et al. (2016). MicroRNA-150 regulates blood-brain barrier permeability via Tie-2 after permanent middle cerebral artery occlusion in rats. FASEB J. Off. Publ. Fed. Am. Soc. Exp. Biol. 30, 2097–2107. doi: 10.1096/fj.201500126
Festjens, N., Vanden Berghe, T., Cornelis, S., and Vandenabeele, P. (2007). RIP1, a kinase on the crossroads of a cell’s decision to live or die. Cell Death Differ. 14, 400–410. doi: 10.1038/sj.cdd.4402085
Fonarow, G. C., Zhao, X., Smith, E. E., Saver, J. L., Reeves, M. J., Bhatt, D. L., et al. (2014). Door-to-needle times for tissue plasminogen activator administration and clinical outcomes in acute ischemic stroke before and after a quality improvement initiative. JAMA 311, 1632–1640. doi: 10.1001/jama.2014.3203
Forstermann, U., and Munzel, T. (2006). Endothelial nitric oxide synthase in vascular disease: from marvel to menace. Circulation 113, 1708–1714. doi: 10.1161/CIRCULATIONAHA.105.602532
Frangogiannis, N. G. (2015). Inflammation in cardiac injury, repair and regeneration. Curr. Opin. Cardiol. 30, 240–245. doi: 10.1097/HCO.0000000000000158
Franke, M., Bieber, M., Kraft, P., Weber, A. N. R., Stoll, G., and Schuhmann, M. K. (2021). The NLRP3 inflammasome drives inflammation in ischemia/reperfusion injury after transient middle cerebral artery occlusion in mice. Brain. Behav. Immun. 92, 221–231. doi: 10.1016/j.bbi.2020.12.009
Franks, Z. G., Campbell, R. A., Weyrich, A. S., and Rondina, M. T. (2010). Platelet-leukocyte interactions link inflammatory and thromboembolic events in ischemic stroke. Ann. N. Y. Acad. Sci. 1207, 11–17. doi: 10.1111/j.1749-6632.2010.05733.x
Fruman, D. A., Meyers, R. E., and Cantley, L. C. (1998). Phosphoinositide kinases. Annu. Rev. Biochem. 67, 481–507. doi: 10.1146/annurev.biochem.67.1.481
Gao, L., Dong, Q., Song, Z., Shen, F., Shi, J., and Li, Y. (2017). NLRP3 inflammasome: a promising target in ischemic stroke. Inflamm. Res. Off. J. Eur. Histamine Res. Soc. Al 66, 17–24. doi: 10.1007/s00011-016-0981-7
Gao, X.-Z., Ma, R.-H., and Zhang, Z.-X. (2020). miR-339 promotes hypoxia-induced neuronal apoptosis and impairs cell viability by targeting FGF9/CACNG2 and mediating MAPK pathway in ischemic stroke. Front. Neurol. 11:436. doi: 10.3389/fneur.2020.00436
Gao, Y., Han, D., and Feng, J. (2021). MicroRNA in multiple sclerosis. Clin. Chim. Acta Int. J. Clin. Chem. 516, 92–99. doi: 10.1016/j.cca.2021.01.020
García-Culebras, A., Durán-Laforet, V., Peña-Martínez, C., Moraga, A., Ballesteros, I., Cuartero, M. I., et al. (2019). Role of TLR4 (Toll-Like Receptor 4) in N1/N2 neutrophil programming after stroke. Stroke 50, 2922–2932. doi: 10.1161/STROKEAHA.119.025085
Garofalo, M., Condorelli, G. L., Croce, C. M., and Condorelli, G. (2010). MicroRNAs as regulators of death receptors signaling. Cell Death Differ. 17, 200–208. doi: 10.1038/cdd.2009.105
Geiseler, S. J., and Morland, C. (2018). The janus face of VEGF in stroke. Int. J. Mol. Sci. 19:E1362. doi: 10.3390/ijms19051362
Ghafouri-Fard, S., Shoorei, H., and Taheri, M. (2020). Non-coding RNAs participate in the ischemia-reperfusion injury. Biomed. Pharmacother. Biomedecine Pharmacother. 129:110419. doi: 10.1016/j.biopha.2020.110419
Giordano, M., Trotta, M. C., Ciarambino, T., D’Amico, M., Galdiero, M., Schettini, F., et al. (2020). Circulating MiRNA-195-5p and -451a in diabetic patients with transient and acute ischemic stroke in the emergency department. Int. J. Mol. Sci. 21:E7615. doi: 10.3390/ijms21207615
Gong, L., Tang, Y., An, R., Lin, M., Chen, L., and Du, J. (2017). RTN1-C mediates cerebral ischemia/reperfusion injury via ER stress and mitochondria-associated apoptosis pathways. Cell Death Dis. 8:e3080. doi: 10.1038/cddis.2017.465
Gong, Z., Pan, J., Shen, Q., Li, M., and Peng, Y. (2018). Mitochondrial dysfunction induces NLRP3 inflammasome activation during cerebral ischemia/reperfusion injury. J. Neuroinflammation 15:242. doi: 10.1186/s12974-018-1282-6
Gorsuch, W. B., Chrysanthou, E., Schwaeble, W. J., and Stahl, G. L. (2012). The complement system in ischemia-reperfusion injuries. Immunobiology 217, 1026–1033. doi: 10.1016/j.imbio.2012.07.024
Granger, D. N., Kvietys, P. R., and Perry, M. A. (1993). Leukocyte–endothelial cell adhesion induced by ischemia and reperfusion. Can. J. Physiol. Pharmacol. 71, 67–75. doi: 10.1139/y93-011
Guan, X., Li, X., Yang, X., Yan, J., Shi, P., Ba, L., et al. (2019). The neuroprotective effects of carvacrol on ischemia/reperfusion-induced hippocampal neuronal impairment by ferroptosis mitigation. Life Sci. 235:116795. doi: 10.1016/j.lfs.2019.116795
Guan, X., Li, Z., Zhu, S., Cheng, M., Ju, Y., Ren, L., et al. (2021). Galangin attenuated cerebral ischemia-reperfusion injury by inhibition of ferroptosis through activating the SLC7A11/GPX4 axis in gerbils. Life Sci. 264:118660. doi: 10.1016/j.lfs.2020.118660
Guo, H., Zhu, L., Tang, P., Chen, D., Li, Y., Li, J., et al. (2021a). Carthamin yellow improves cerebral ischemia-reperfusion injury by attenuating inflammation and ferroptosis in rats. Int. J. Mol. Med. 47:52. doi: 10.3892/ijmm.2021.4885
Guo, M.-M., Qu, S.-B., Lu, H.-L., Wang, W.-B., He, M.-L., Su, J.-L., et al. (2021b). biochanin a alleviates cerebral ischemia/reperfusion injury by suppressing endoplasmic reticulum stress-induced apoptosis and p38MAPK signaling pathway in vivo and in vitro. Front. Endocrinol. 12:646720. doi: 10.3389/fendo.2021.646720
Haase, S., and Linker, R. A. (2021). Inflammation in multiple sclerosis. Ther. Adv. Neurol. Disord. 14:17562864211007688. doi: 10.1177/17562864211007687
Haley, M. J., and Lawrence, C. B. (2017). The blood-brain barrier after stroke: structural studies and the role of transcytotic vesicles. J. Cereb. Blood Flow Metab. Off. J. Int. Soc. Cereb. Blood Flow Metab. 37, 456–470. doi: 10.1177/0271678X16629976
Hao, T., Yang, Y., Li, N., Mi, Y., Zhang, G., Song, J., et al. (2020). Inflammatory mechanism of cerebral ischemia-reperfusion injury with treatment of stepharine in rats. Phytomedicine 79:153353. doi: 10.1016/j.phymed.2020.153353
Harati, R., Hammad, S., Tlili, A., Mahfood, M., Mabondzo, A., and Hamoudi, R. (2022). miR-27a-3p regulates expression of intercellular junctions at the brain endothelium and controls the endothelial barrier permeability. PLoS ONE 17:e0262152. doi: 10.1371/journal.pone.0262152
Hawkins, B. T., and Davis, T. P. (2005). The blood-brain barrier/neurovascular unit in health and disease. Pharmacol. Rev. 57, 173–185. doi: 10.1124/pr.57.2.4
He, W., Zhang, Z., and Sha, X. (2021). Nanoparticles-mediated emerging approaches for effective treatment of ischemic stroke. Biomaterials 277:121111. doi: 10.1016/j.biomaterials.2021.121111
Helton, R., Cui, J., Scheel, J. R., Ellison, J. A., Ames, C., Gibson, C., et al. (2005). Brain-specific knock-out of hypoxia-inducible factor-1alpha reduces rather than increases hypoxic-ischemic damage. J. Neurosci. Off. J. Soc. Neurosci. 25, 4099–4107. doi: 10.1523/JNEUROSCI.4555-04.2005
Hong, H.-Y., Choi, J. S., Kim, Y. J., Lee, H. Y., Kwak, W., Yoo, J., et al. (2008). Detection of apoptosis in a rat model of focal cerebral ischemia using a homing peptide selected from in vivo phage display. J. Control. Release Off. J. Control. Release Soc. 131, 167–172. doi: 10.1016/j.jconrel.2008.07.020
Hong, P., Gu, R.-N., Li, F.-X., Xiong, X.-X., Liang, W.-B., You, Z.-J., et al. (2019). NLRP3 inflammasome as a potential treatment in ischemic stroke concomitant with diabetes. J. Neuroinflammation 16:121. doi: 10.1186/s12974-019-1498-0
Hong, S., Kwon, J., Hiep, N. T., Sim, S. J., Kim, N., Kim, K. H., et al. (2018). The isoflavones and extracts from maclura tricuspidata fruit protect against neuronal cell death in ischemic injury via induction of Nox4-targeting miRNA-25, miRNA-92a, and miRNA-146a. J. Funct. Foods 40, 785–797. doi: 10.1016/j.jff.2017.12.011
Hoss, A. G., Labadorf, A., Beach, T. G., Latourelle, J. C., and Myers, R. H. (2016). microRNA profiles in parkinson’s disease prefrontal cortex. Front. Aging Neurosci. 8:36. doi: 10.3389/fnagi.2016.00036
Hou, Y., Wang, Y., He, Q., Li, L., Xie, H., Zhao, Y., et al. (2018). Nrf2 inhibits NLRP3 inflammasome activation through regulating Trx1/TXNIP complex in cerebral ischemia reperfusion injury. Behav. Brain Res. 336, 32–39. doi: 10.1016/j.bbr.2017.06.027
Hsu, C.-C., Huang, C.-C., Chien, L.-H., Lin, M.-T., Chang, C.-P., Lin, H.-J., et al. (2020). Ischemia/reperfusion injured intestinal epithelial cells cause cortical neuron death by releasing exosomal microRNAs associated with apoptosis, necroptosis, and pyroptosis. Sci. Rep. 10:14409. doi: 10.1038/s41598-020-71310-5
Hu, Y., Deng, H., Xu, S., and Zhang, J. (2015). MicroRNAs regulate mitochondrial function in cerebral ischemia-reperfusion injury. Int. J. Mol. Sci. 16, 24895–24917. doi: 10.3390/ijms161024895
Hu, Y.-K., Wang, X., Li, L., Du, Y.-H., Ye, H.-T., and Li, C.-Y. (2013). MicroRNA-98 induces an Alzheimer’s disease-like disturbance by targeting insulin-like growth factor 1. Neurosci. Bull. 29, 745–751. doi: 10.1007/s12264-013-1348-5
Huang, L., Li, X., Liu, Y., Liang, X., Ye, H., Yang, C., et al. (2021a). Curcumin alleviates cerebral ischemia-reperfusion injury by inhibiting NLRP1-dependent neuronal pyroptosis. Curr. Neurovasc. Res. 18, 189–196. doi: 10.2174/1567202618666210607150140
Huang, R., Ma, J., Niu, B., Li, J., Chang, J., Zhang, Y., et al. (2019). MiR-34b protects against focal cerebral ischemia-reperfusion (I/R) injury in rat by targeting keap1. J. Stroke Cerebrovasc. Dis. 28, 1–9. doi: 10.1016/j.jstrokecerebrovasdis.2018.08.023
Huang, Y., Wang, Y., Duan, Z., Liang, J., Xu, Y., Zhang, S., et al. (2021b). Restored microRNA-326-5p Inhibits neuronal apoptosis and attenuates mitochondrial damage via suppressing STAT3 in cerebral ischemia/reperfusion injury. Nanoscale Res. Lett. 16:63. doi: 10.1186/s11671-021-03520-3
Huang, Z., Lu, L., Jiang, T., Zhang, S., Shen, Y., Zheng, Z., et al. (2018). miR-29b affects neurocyte apoptosis by targeting MCL-1 during cerebral ischemia/reperfusion injury. Exp. Ther. Med. 16, 3399–3404. doi: 10.3892/etm.2018.6622
Imajo, M., Tsuchiya, Y., and Nishida, E. (2006). Regulatory mechanisms and functions of MAP kinase signaling pathways. IUBMB Life 58, 312–317. doi: 10.1080/15216540600746393
Islam, A., Choudhury, M. E., Kigami, Y., Utsunomiya, R., Matsumoto, S., Watanabe, H., et al. (2018). Sustained anti-inflammatory effects of TGF-β1 on microglia/macrophages. Biochim. Biophys. Acta BBA - Mol. Basis Dis. 1864, 721–734. doi: 10.1016/j.bbadis.2017.12.022
IST-3 collaborative group, Sandercock, P., Wardlaw, J. M., Lindley, R. I, Dennis, M., Cohen, G., et al. (2012). The benefits and harms of intravenous thrombolysis with recombinant tissue plasminogen activator within 6 h of acute ischaemic stroke (the third international stroke trial [IST-3]): a randomised controlled trial. Lancet Lond. Engl. 379, 2352–2363. doi: 10.1016/S0140-6736(12)60768-5
Jeyaseelan, K., Lim, K. Y., and Armugam, A. (2008). MicroRNA expression in the blood and brain of rats subjected to transient focal ischemia by middle cerebral artery occlusion. Stroke 39, 959–966. doi: 10.1161/STROKEAHA.107.500736
Ji, Q., Gao, J., Zheng, Y., Liu, X., Zhou, Q., Shi, C., et al. (2017). Inhibition of microRNA-153 protects neurons against ischemia/reperfusion injury in an oxygen–glucose deprivation and reoxygenation cellular model by regulating Nrf2/HO-1 signaling. J. Biochem. Mol. Toxicol. 31, 1–8. doi: 10.1002/jbt.21905
Ji, Q., Ji, Y., Peng, J., Zhou, X., Chen, X., Zhao, H., et al. (2016). Increased brain-specific MiR-9 and MiR-124 in the serum exosomes of acute ischemic stroke patients. PLoS ONE 11:e0163645. doi: 10.1371/journal.pone.0163645
Jia, T., Wang, M., Yan, W., Wu, W., and Shen, R. (2022). Upregulation of miR-489-3p attenuates cerebral ischemia/reperfusion injury by targeting histone deacetylase 2 (HDAC2). Neuroscience 484, 16–25. doi: 10.1016/j.neuroscience.2021.12.009
Jiang, D., Sun, X., Wang, S., and Man, H. (2019). Upregulation of miR-874-3p decreases cerebral ischemia/reperfusion injury by directly targeting BMF and BCL2L13. Biomed. Pharmacother. 117:108941. doi: 10.1016/j.biopha.2019.108941
Jiang, X., Andjelkovic, A. V., Zhu, L., Yang, T., Bennett, M. V. L., Chen, J., et al. (2018a). Blood-brain barrier dysfunction and recovery after ischemic stroke. Prog. Neurobiol. 163–164, 144–171. doi: 10.1016/j.pneurobio.2017.10.001
Jiang, Y., Xu, B., Chen, J., Sui, Y., Ren, L., Li, J., et al. (2018b). Micro-RNA-137 inhibits tau hyperphosphorylation in alzheimer’s disease and targets the CACNA1C gene in transgenic mice and human neuroblastoma SH-SY5Y cells. Med. Sci. Monit. Int. Med. J. Exp. Clin. Res. 24:5635. doi: 10.12659/MSM.908765
Jonas, S., and Izaurralde, E. (2015). Towards a molecular understanding of microRNA-mediated gene silencing. Nat. Rev. Genet. 16, 421–433. doi: 10.1038/nrg3965
Jurcau, A., and Simion, A. (2021). Neuroinflammation in cerebral ischemia and ischemia/reperfusion injuries: from pathophysiology to therapeutic strategies. Int. J. Mol. Sci. 23:14. doi: 10.3390/ijms23010014
Juźwik, C. A., Drake, S., Zhang, Y., Paradis-Isler, N., Sylvester, A., Amar-Zifkin, A., et al. (2019). microRNA dysregulation in neurodegenerative diseases: a systematic review. Prog. Neurobiol. 182:101664. doi: 10.1016/j.pneurobio.2019.101664
Kadir, R. R. A., Alwjwaj, M., and Bayraktutan, U. (2020). MicroRNA: an emerging predictive, diagnostic, prognostic and therapeutic strategy in ischaemic stroke. Cell. Mol. Neurobiol. 42, 1301–1319. doi: 10.1007/s10571-020-01028-5
Kamphuis, W. W., Derada Troletti, C., Reijerkerk, A., Romero, I. A., and de Vries, H. E. (2015). The blood-brain barrier in multiple sclerosis: microRNAs as key regulators. CNS Neurol. Disord. Drug Targets 14, 157–167. doi: 10.2174/1871527314666150116125246
Kawabori, M., and Yenari, M. A. (2015). Inflammatory responses in brain ischemia. Curr. Med. Chem. 22:1258.
Khoshnam, S. E., Winlow, W., and Farzaneh, M. (2017). The interplay of micrornas in the inflammatory mechanisms following ischemic stroke. J. Neuropathol. Exp. Neurol. 76, 548–561. doi: 10.1093/jnen/nlx036
Kim, C. R., Kim, J. H., Park, H.-Y. L., and Park, C. K. (2017a). Ischemia reperfusion injury triggers TNFα induced-necroptosis in rat retina. Curr. Eye Res. 42, 771–779. doi: 10.1080/02713683.2016.1227449
Kim, J. Y., Park, J., Lee, J. E., and Yenari, M. A. (2017b). NOX inhibitors - a promising avenue for ischemic stroke. Exp. Neurobiol. 26, 195–205. doi: 10.5607/en.2017.26.4.195
Kim, T., Mehta, S. L., Morris-Blanco, K. C., Chokkalla, A. K., Chelluboina, B., Lopez, M., et al. (2018). The microRNA miR-7a-5p ameliorates ischemic brain damage by repressing α-synuclein. Sci. Signal. 11:eaat4285. doi: 10.1126/scisignal.aat4285
Lallukka, T., Ervasti, J., Lundström, E., Mittendorfer-Rutz, E., Friberg, E., Virtanen, M., et al. (2018). Trends in diagnosis-specific work disability before and after stroke: a longitudinal population-based study in Sweden. J. Am. Heart Assoc. 7:e006991. doi: 10.1161/JAHA.117.006991
Li, H., Gao, A., Feng, D., Wang, Y., Zhang, L., Cui, Y., et al. (2014a). Evaluation of the protective potential of brain microvascular endothelial cell autophagy on blood-brain barrier integrity during experimental cerebral ischemia-reperfusion injury. Transl. Stroke Res. 5, 618–626. doi: 10.1007/s12975-014-0354-x
Li, H., Han, G., He, D., Wang, Y., Lin, Y., Zhang, T., et al. (2021a). miR-539 targeting SNAI2 regulates MMP9 signaling pathway and affects blood-brain barrier permeability in cerebrovascular occlusive diseases: a study based on head and neck ultrasound and CTA. J. Healthc. Eng. 2021:5699025. doi: 10.1155/2021/5699025
Li, H., Zhang, N., Lin, H.-Y., Yu, Y., Cai, Q.-Y., Ma, L., et al. (2014b). Histological, cellular and behavioral assessments of stroke outcomes after photothrombosis-induced ischemia in adult mice. BMC Neurosci. 15:58. doi: 10.1186/1471-2202-15-58
Li, L., Qu, Y., Mao, M., Xiong, Y., and Mu, D. (2008). The involvement of phosphoinositid 3-kinase/Akt pathway in the activation of hypoxia-inducible factor-1alpha in the developing rat brain after hypoxia-ischemia. Brain Res. 1197, 152–158. doi: 10.1016/j.brainres.2007.12.059
Li, P., Shen, M., Gao, F., Wu, J., Zhang, J., Teng, F., et al. (2017a). An antagomir to MicroRNA-106b-5p ameliorates cerebral ischemia and reperfusion injury in rats via inhibiting apoptosis and oxidative stress. Mol. Neurobiol. 54, 2901–2921. doi: 10.1007/s12035-016-9842-1
Li, R., Li, X., Wu, H., Yang, Z., Li, F. E. I., and Jianhong, Z. H. U. (2019a). Theaflavin attenuates cerebral ischemia/reperfusion injury by abolishing miRNA-128-3p-mediated Nrf2 inhibition and reducing oxidative stress. Mol. Med. Rep. 20, 4893–9404. doi: 10.3892/mmr.2019.10755
Li, S., Wang, Y., Wang, M., Chen, L., Chen, S., Deng, F., et al. (2021b). microRNA-186 alleviates oxygen-glucose deprivation/reoxygenation-induced injury by directly targeting hypoxia-inducible factor-1α. J. Biochem. Mol. Toxicol. 35, 1–11. doi: 10.1002/jbt.22752
Li, W., Zhu, Q., Xu, X., and Hu, X. (2021c). MiR-27a-3p suppresses cerebral ischemia-reperfusion injury by targeting FOXO1. Aging 13, 11727–11737. doi: 10.18632/aging.202866
Li, X., Su, L., Zhang, X., Zhang, C., Wang, L., Li, Y., et al. (2017b). Ulinastatin downregulates TLR4 and NF-kB expression and protects mouse brains against ischemia/reperfusion injury. Neurol. Res. 39, 367–373. doi: 10.1080/01616412.2017.1286541
Li, X., Zhang, X., Liu, Y., Pan, R., Liang, X., Huang, L., et al. (2021d). Exosomes derived from mesenchyml stem cells ameliorate oxygen-glucose deprivation/reoxygenation-induced neuronal injury via transferring MicroRNA-194 and targeting Bach1. Tissue Cell 73:101651. doi: 10.1016/j.tice.2021.101651
Li, Y., Cao, Y., Xiao, J., Shang, J., Tan, Q., Ping, F., et al. (2020). Inhibitor of apoptosis-stimulating protein of p53 inhibits ferroptosis and alleviates intestinal ischemia/reperfusion-induced acute lung injury. Cell Death Differ. 27, 2635–2650. doi: 10.1038/s41418-020-0528-x
Li, Y., Feng, D., Wang, Z., Zhao, Y., Sun, R., Tian, D., et al. (2019b). Ischemia-induced ACSL4 activation contributes to ferroptosis-mediated tissue injury in intestinal ischemia/reperfusion. Cell Death Differ. 26, 2284–2299. doi: 10.1038/s41418-019-0299-4
Li, Z., Li, J., and Tang, N. (2017c). Long noncoding RNA Malat1 is a potent autophagy inducer protecting brain microvascular endothelial cells against oxygen-glucose deprivation/reoxygenation-induced injury by sponging miR-26b and upregulating ULK2 expression. Neuroscience 354, 1–10. doi: 10.1016/j.neuroscience.2017.04.017
Liang, C., Zhang, X., Yang, M., and Dong, X. (2019). Recent progress in ferroptosis inducers for cancer therapy. Adv. Mater. Deerfield Beach Fla 31:e1904197. doi: 10.1002/adma.201904197
Liang, J., Wang, Q., Li, J.-Q., Guo, T., and Yu, D. (2020). Long non-coding RNA MEG3 promotes cerebral ischemia-reperfusion injury through increasing pyroptosis by targeting miR-485/AIM2 axis. Exp. Neurol. 325:113139. doi: 10.1016/j.expneurol.2019.113139
Liang, Y., Xu, J., Wang, Y., Tang, J. Y., Yang, S. L., Xiang, H. G., et al. (2018). Inhibition of MiRNA-125b decreases cerebral ischemia/reperfusion injury by targeting CK2α/NADPH oxidase signaling. Cell. Physiol. Biochem. 45, 1818–1826. doi: 10.1159/000487873
Liao, S., Apaijai, N., Chattipakorn, N., and Chattipakorn, S. C. (2020). The possible roles of necroptosis during cerebral ischemia and ischemia/reperfusion injury. Arch. Biochem. Biophys. 695:108629. doi: 10.1016/j.abb.2020.108629
Lillo-Moya, J., Rojas-Solé, C., Muñoz-Salamanca, D., Panieri, E., Saso, L., and Rodrigo, R. (2021). Targeting ferroptosis against ischemia/reperfusion cardiac injury. Antioxidants 10:667. doi: 10.3390/antiox10050667
Lin, L., Wang, X., and Yu, Z. (2016). Ischemia-reperfusion injury in the brain: mechanisms and potential therapeutic strategies. Biochem. Pharmacol. Open Access 5:213. doi: 10.4172/2167-0501.1000213
Lisa, F. D., and Bernardi, P. (2006). Mitochondria and ischemia-reperfusion injury of the heart: fixing a hole. Cardiovasc. Res. 70, 191–199. doi: 10.1016/j.cardiores.2006.01.016
Liu, N.-N., Dong, Z.-L., and Han, L.-L. (2018). MicroRNA-410 inhibition of the TIMP2-dependent MAPK pathway confers neuroprotection against oxidative stress-induced apoptosis after ischemic stroke in mice. Brain Res. Bull. 143, 45–57. doi: 10.1016/j.brainresbull.2018.09.009
Liu, P., Zhao, H., Wang, R., Wang, P., Tao, Z., Gao, L., et al. (2015). MicroRNA-424 protects against focal cerebral ischemia and reperfusion injury in mice by suppressing oxidative stress. Stroke 46, 513–519. doi: 10.1161/STROKEAHA.114.007482
Liu, Q., Radwanski, R., Babadjouni, R., Patel, A., Hodis, D. M., Baumbacher, P., et al. (2019a). Experimental chronic cerebral hypoperfusion results in decreased pericyte coverage and increased blood-brain barrier permeability in the corpus callosum. J. Cereb. Blood Flow Metab. Off. J. Int. Soc. Cereb. Blood Flow Metab. 39, 240–250. doi: 10.1177/0271678X17743670
Liu, W., Miao, Y., Zhang, L., Xu, X., and Luan, Q. (2020). MiR-211 protects cerebral ischemia/reperfusion injury by inhibiting cell apoptosis. Bioengineered 11:189. doi: 10.1080/21655979.2020.1729322
Liu, X., Feng, Z., Du, L., Huang, Y., Ge, J., Deng, Y., et al. (2019b). The potential role of MicroRNA-124 in cerebral ischemia injury. Int. J. Mol. Sci. 21:E120. doi: 10.3390/ijms21010120
Liu, X., Wang, X., Zhang, L., Zhou, Y., Yang, L., and Yang, M. (2021). By targeting apoptosis facilitator BCL2L13, microRNA miR-484 alleviates cerebral ischemia/reperfusion injury-induced neuronal apoptosis in mice. Bioengineered 12, 948–959. doi: 10.1080/21655979.2021.1898134
Liu, Z., Tuo, Y. H., Chen, J. W., Wang, Q. Y., Li, S., Li, M. C., et al. (2017). NADPH oxidase inhibitor regulates microRNAs with improved outcome after mechanical reperfusion. J. NeuroInterventional Surg. 9, 702–706. doi: 10.1136/neurintsurg-2016-012463
Lu, J., Xu, F., and Lu, H. (2020a). LncRNA PVT1 regulates ferroptosis through miR-214-mediated TFR1 and p53. Life Sci. 260:118305. doi: 10.1016/j.lfs.2020.118305
Lu, M.-Y., Wu, J.-R., Liang, R.-B., Wang, Y.-P., Zhu, Y.-C., Ma, Z.-T., et al. (2020b). Upregulation of miR-219a-5p decreases cerebral ischemia/reperfusion injury in vitro by targeting Pde4d. J. Stroke Cerebrovasc. Dis. 29:104801. doi: 10.1016/j.jstrokecerebrovasdis.2020.104801
Lubin, F. D., Roth, T. L., and Sweatt, J. D. (2008). Epigenetic regulation of bdnf gene transcription in the consolidation of fear memory. J. Neurosci. 28, 10576–10586. doi: 10.1523/JNEUROSCI.1786-08.2008
Luo, Y., Tang, H., Li, H., Zhao, R., Huang, Q., and Liu, J. (2019). Recent advances in the development of neuroprotective agents and therapeutic targets in the treatment of cerebral ischemia. Eur. J. Med. Chem. 162, 132–146. doi: 10.1016/j.ejmech.2018.11.014
Ma, F., Zhang, X., and Yin, K.-J. (2020). MicroRNAs in central nervous system diseases: a prospective role in regulating blood-brain barrier integrity. Exp. Neurol. 323:113094. doi: 10.1016/j.expneurol.2019.113094
Ma, J., Shui, S., Han, X., Guo, D., Li, T., and Yan, L. (2017a). microRNA-200a silencing protects neural stem cells against cerebral ischemia/reperfusion injury. PLoS One 12:e0172178. doi: 10.1371/journal.pone.0172178
Ma, M. W., Wang, J., Zhang, Q., Wang, R., Dhandapani, K. M., Vadlamudi, R. K., et al. (2017b). NADPH oxidase in brain injury and neurodegenerative disorders. Mol. Neurodegener. 12:7. doi: 10.1186/s13024-017-0150-7
MacFarlane, L.-A., and Murphy, P. R. (2010). MicroRNA: biogenesis. Funct. Role Cancer. Curr. Genomics 11, 537–561. doi: 10.2174/138920210793175895
Madhyastha, R., Madhyastha, H., Nakajima, Y., Omura, S., and Maruyama, M. (2012). MicroRNA signature in diabetic wound healing: promotive role of miR-21 in fibroblast migration. Int. Wound J. 9, 355–361. doi: 10.1111/j.1742-481X.2011.00890.x
Magaki, S. D., Williams, C. K., and Vinters, H. V. (2018). Glial function (and dysfunction) in the normal & ischemic brain. Neuropharmacology 134, 218–225. doi: 10.1016/j.neuropharm.2017.11.009
Mao, L., Zuo, M. L., Wang, A. P., Tian, Y., Dong, L. C., Li, T. M., et al. (2020). Low expression of miR-532-3p contributes to cerebral ischemia/reperfusion oxidative stress injury by directly targeting NOX2. Mol. Med. Rep. 22, 2415–2423. doi: 10.3892/mmr.2020.11325
Mărgăritescu, O., Mogoantă, L., Pirici, I., Pirici, D., Cernea, D., and Mărgăritescu, C. (2009). Histopathological changes in acute ischemic stroke. Romanian J. Morphol. Embryol. Rev. Roum. Morphol. Embryol. 50, 327–339.
Melincovici, C. S., Boşca, A. B., Şuşman, S., Mărginean, M., Mihu, C., Istrate, M., et al. (2018). Vascular endothelial growth factor (VEGF) - key factor in normal and pathological angiogenesis. Romanian J. Morphol. Embryol. Rev. Roum. Morphol. Embryol. 59, 455–467.
Metsis, M., Timmusk, T., Arenas, E., and Persson, H. (1993). Differential usage of multiple brain-derived neurotrophic factor promoters in the rat brain following neuronal activation. Proc. Natl. Acad. Sci. U.S.A 90, 8802–8806. doi: 10.1073/pnas.90.19.8802
Meza, C. A., La Favor, J. D., Kim, D.-H., and Hickner, R. C. (2019). Endothelial dysfunction: is there a hyperglycemia-induced imbalance of NOX and NOS? Int. J. Mol. Sci. 20:E3775. doi: 10.3390/ijms20153775
Min, X., Wang, T., Cao, Y., Liu, J., Li, J., and Wang, T. (2015). MicroRNAs: a novel promising therapeutic target for cerebral ischemia/reperfusion injury? Neural Regen. Res. 10, 1799–1808. doi: 10.4103/1673-5374.170302
Min, X.-L., He, M., Shi, Y., Xie, L., Ma, X.-J., and Cao, Y. (2020). miR-18b attenuates cerebral ischemia/reperfusion injury through regulation of ANXA3 and PI3K/Akt signaling pathway. Brain Res. Bull. 161, 55–64. doi: 10.1016/j.brainresbull.2020.04.021
Yin, K. J., Deng, Z., Huang, H., Hamblin, M., Xie, C., Zhang, J., et al. (2022). miR-497 regulates neuronal death in mouse brain after transient focal cerebral ischemia. Neurobiol. Dis. 38, 17–26. doi: 10.1016/j.nbd.2009.12.021
Miranda, M., Morici, J. F., Zanoni, M. B., and Bekinschtein, P. (2019). Brain-derived neurotrophic factor: a key molecule for memory in the healthy and the pathological brain. Front. Cell. Neurosci. 13:363. doi: 10.3389/fncel.2019.00363
Mitre, A.-O., Florian, A. I., Buruiana, A., Boer, A., Moldovan, I., Soritau, O., et al. (2022). Ferroptosis involvement in glioblastoma treatment. Medicina 58:319. doi: 10.3390/medicina58020319
Moskowitz, M. A., Lo, E. H., and Iadecola, C. (2010). The science of stroke: mechanisms in search of treatments. Neuron 67, 181–198. doi: 10.1016/j.neuron.2010.07.002
Nakano, K., and Vousden, K. H. (2001). PUMA, a novel proapoptotic gene, is induced by p53. Mol. Cell 7, 683–694. doi: 10.1016/S1097-2765(01)00214-3
Nies, Y. H., Mohamad Najib, N. H., Lim, W. L., Kamaruzzaman, M. A., Yahaya, M. F., and Teoh, S. L. (2021). MicroRNA dysregulation in parkinson’s disease: a narrative review. Front. Neurosci. 15:660379. doi: 10.3389/fnins.2021.660379
Nourshargh, S., and Alon, R. (2014). Leukocyte migration into inflamed tissues. Immunity 41, 694–707. doi: 10.1016/j.immuni.2014.10.008
Nozaki, K., Nishimura, M., and Hashimoto, N. (2001). Mitogen-activated protein kinases and cerebral ischemia. Mol. Neurobiol 23, 1–19. doi: 10.1385/MN:23:1:01
Nunes, T., and de Souza, H. S. (2013). Inflammasome in intestinal inflammation and cancer. Mediators Inflamm. 2013:654963. doi: 10.1155/2013/654963
Nuzziello, N., Vilardo, L., Pelucchi, P., Consiglio, A., Liuni, S., Trojano, M., et al. (2018). Investigating the role of MicroRNA and transcription factor co-regulatory networks in multiple sclerosis pathogenesis. Int. J. Mol. Sci. 19:3652. doi: 10.3390/ijms19113652
Ouyang, Y.-B., Stary, C. M., White, R. E., and Giffard, R. G. (2015). The use of microRNAs to modulate redox and immune response to stroke. Antioxid. Redox Signal. 22, 187–202. doi: 10.1089/ars.2013.5757
Ouyang, Y.-B., Xu, L., Lu, Y., Sun, X., Yue, S., Xiong, X.-X., et al. (2013). Astrocyte-enriched miR-29a targets PUMA and reduces neuronal vulnerability to forebrain ischemia. Glia 61, 1784–1794. doi: 10.1002/glia.22556
Peng, L., Yin, J., Wang, S., Ge, M., Han, Z., Wang, Y., et al. (2019). TGF-β2/Smad3 signaling pathway activation through enhancing VEGF and CD34 Ameliorates cerebral ischemia/reperfusion injury after isoflurane post-conditioning in rats. Neurochem. Res. 44, 2606–2618. doi: 10.1007/s11064-019-02880-8
Petrescu, G. E. D., Sabo, A. A., Torsin, L. I., Calin, G. A., and Dragomir, M. P. (2019). MicroRNA based theranostics for brain cancer: basic principles. J. Exp. Clin. Cancer Res. CR 38:231. doi: 10.1186/s13046-019-1180-5
Ponnusamy, V., and Yip, P. K. (2019). The role of microRNAs in newborn brain development and hypoxic ischaemic encephalopathy. Neuropharmacology 149, 55–65. doi: 10.1016/j.neuropharm.2018.11.041
Qiang, Z., Dong, H., Xia, Y., Chai, D., Hu, R., and Jiang, H. (2020). Nrf2 and STAT3 alleviates ferroptosis-mediated IIR-ALI by regulating SLC7A11. Oxid. Med. Cell. Longev. 2020:5146982. doi: 10.1155/2020/5146982
Quinlan, S., Kenny, A., Medina, M., Engel, T., and Jimenez-Mateos, E. M. (2017). MicroRNAs in neurodegenerative diseases. Int. Rev. Cell Mol. Biol. 334, 309–343. doi: 10.1016/bs.ircmb.2017.04.002
Radak, D., Katsiki, N., Resanovic, I., Jovanovic, A., Sudar-Milovanovic, E., Zafirovic, S., et al. (2017). Apoptosis and acute brain ischemia in ischemic stroke. Curr. Vasc. Pharmacol. 15, 115–122. doi: 10.2174/1570161115666161104095522
Rahaman, P., and Del Bigio, M. R. (2018). Histology of brain trauma and hypoxia-ischemia. Acad. Forensic Pathol. 8, 539–554. doi: 10.1177/1925362118797728
Rahmati, M., Ferns, G. A., and Mobarra, N. (2021). The lower expression of circulating miR-210 and elevated serum levels of HIF-1α in ischemic stroke; possible markers for diagnosis and disease prediction. J. Clin. Lab. Anal. 35:e24073. doi: 10.1002/jcla.24073
Rainer, T. H., Leung, L. Y., Chan, C. P. Y., Leung, Y. K., Abrigo, J. M., Wang, D., et al. (2016). Plasma miR-124-3p and miR-16 concentrations as prognostic markers in acute stroke. Clin. Biochem. 49, 663–668. doi: 10.1016/j.clinbiochem.2016.02.016
Hofer, M., Pagliusi, S. R., Hohn, A., Leibrock, J., and Barde, Y. A. (1990). Regional distribution of brain-derived neurotrophic factor mRNA in the adult mouse brain. EMBO J. 9, 2459–2464. doi: 10.1002/j.1460-2075.1990.tb07423.x
Reichert, C. O., de Freitas, F. A., Sampaio-Silva, J., Rokita-Rosa, L., Barros, P., de, L., et al. (2020). Ferroptosis mechanisms involved in neurodegenerative diseases. Int. J. Mol. Sci. 21:E8765. doi: 10.3390/ijms21228765
Ren, Z., Xie, P., Lv, J., Hu, Y., Guan, Z., Chen, L., et al. (2020). miR-187-3p inhibitor attenuates cerebral ischemia/reperfusion injury by regulating Seipin-mediated autophagic flux. Int. J. Mol. Med. 46, 1051–1062. doi: 10.3892/ijmm.2020.4642
Rink, C., and Khanna, S. (2011). MicroRNA in ischemic stroke etiology and pathology. Physiol. Genomics 43, 521–528. doi: 10.1152/physiolgenomics.00158.2010
Rodrigues, S. F., and Granger, D. N. (2010). Role of blood cells in ischaemia-reperfusion induced endothelial barrier failure. Cardiovasc. Res. 87, 291–299. doi: 10.1093/cvr/cvq090
Rosenberg, G. A., and Yang, Y. (2007). Vasogenic edema due to tight junction disruption by matrix metalloproteinases in cerebral ischemia. Neurosurg. Focus 22 5, 1–9. doi: 10.3171/foc.2007.22.5.5
Sanderson, T. H., Reynolds, C. A., Kumar, R., Przyklenk, K., and Hüttemann, M. (2013). Molecular mechanisms of ischemia–reperfusion injury in brain: pivotal role of the mitochondrial membrane potential in reactive oxygen species generation. Mol. Neurobiol. 47, 9–23. doi: 10.1007/s12035-012-8344-z
Saugstad, J. A. (2010). MicroRNAs as effectors of brain function with roles in ischemia and injury, neuroprotection, and neurodegeneration. J. Cereb. Blood Flow Metab. Off. J. Int. Soc. Cereb. Blood Flow Metab. 30, 1564–1576. doi: 10.1038/jcbfm.2010.101
Sedeek, M., Hébert, R. L., Kennedy, C. R., Burns, K. D., and Touyz, R. M. (2009). Molecular mechanisms of hypertension: role of nox family NADPH oxidases. Curr. Opin. Nephrol. Hypertens. 18, 122–127. doi: 10.1097/MNH.0b013e32832923c3
Semple, B. D., Blomgren, K., Gimlin, K., Ferriero, D. M., and Noble-Haeusslein, L. J. (2013). Brain development in rodents and humans: identifying benchmarks of maturation and vulnerability to injury across species. Prog. Neurobiol. 106–107, 1–16. doi: 10.1016/j.pneurobio.2013.04.001
Sepramaniam, S., Armugam, A., Lim, K. Y., Karolina, D. S., Swaminathan, P., Tan, J. R., et al. (2010). MicroRNA 320a functions as a novel endogenous modulator of aquaporins 1 and 4 as well as a potential therapeutic target in cerebral ischemia. J. Biol. Chem. 285, 29223–29230. doi: 10.1074/jbc.M110.144576
Sepramaniam, S., Ying, L. K., Armugam, A., Wintour, E. M., and Jeyaseelan, K. (2012). MicroRNA-130a represses transcriptional activity of aquaporin 4 M1 promoter. J. Biol. Chem. 287, 12006–12015. doi: 10.1074/jbc.M111.280701
Serafin, A., Foco, L., Zanigni, S., Blankenburg, H., Picard, A., Zanon, A., et al. (2015). Overexpression of blood microRNAs 103a, 30b, and 29a in L-dopa-treated patients with PD. Neurology 84, 645–653. doi: 10.1212/WNL.0000000000001258
Sharp, F. R., and Bernaudin, M. (2004). HIF1 and oxygen sensing in the brain. Nat. Rev. Neurosci. 5, 437–448. doi: 10.1038/nrn1408
Shen, G., and Ma, Q. (2020). MicroRNAs in the blood-brain barrier in hypoxic-ischemic brain injury. Curr. Neuropharmacol. 18, 1180–1186. doi: 10.2174/1570159X18666200429004242
Shen, J., Li, G., Zhu, Y., Xu, Q., Zhou, H., Xu, K., et al. (2021). Foxo1-induced miR-92b down-regulation promotes blood-brain barrier damage after ischaemic stroke by targeting NOX4. J. Cell. Mol. Med. 25, 5269–5282. doi: 10.1111/jcmm.16537
Shi, C.-X., Ding, Y.-B., Jin, F. Y. J., Li, T., Ma, J.-H., Qiao, L.-Y., et al. (2018). Effects of sevoflurane post-conditioning in cerebral ischemia-reperfusion injury via TLR4/NF-κB pathway in rats. Eur. Rev. Med. Pharmacol. Sci. 22, 1770–1775. doi: 10.26355/eurrev_201803_14595
Shi, H. (2009). Hypoxia inducible Factor 1 as a therapeutic target in ischemic stroke. Curr. Med. Chem. 16:4593. doi: 10.2174/092986709789760779
Shi, J., Zhao, Y., Wang, K., Shi, X., Wang, Y., Huang, H., et al. (2015). Cleavage of GSDMD by inflammatory caspases determines pyroptotic cell death. Nature 526, 660–665. doi: 10.1038/nature15514
Shi, Y., Yi, Z., Zhao, P., Xu, Y., and Pan, P. (2021). MicroRNA-532-5p protects against cerebral ischemia-reperfusion injury by directly targeting CXCL1. Aging 13, 11528–11541. doi: 10.18632/aging.202846
Shim, J. W., and Madsen, J. R. (2018). VEGF signaling in neurological disorders. Int. J. Mol. Sci. 19:E275. doi: 10.3390/ijms19010275
Siddiq, A., Ayoub, I. A., Chavez, J. C., Aminova, L., Shah, S., LaManna, J. C., et al. (2005). Hypoxia-inducible factor prolyl 4-hydroxylase inhibition. a target for neuroprotection in the central nervous system. J. Biol. Chem. 280, 41732–41743. doi: 10.1074/jbc.M504963200
Humphrey, S. M., Hearse, D. J., and Chain, E. B. (1973). Abrupt reoxygenation of the anoxic potassium-arrested perfused rat heart: a study of myocardial enzyme release. J Mol Cell Cardiol 5, 395–407. doi: 10.1016/0022-2828(73)90030-8
Smirnova, L., Gräfe, A., Seiler, A., Schumacher, S., Nitsch, R., and Wulczyn, F. G. (2005). Regulation of miRNA expression during neural cell specification. Eur. J. Neurosci. 21, 1469–1477. doi: 10.1111/j.1460-9568.2005.03978.x
Sun, C., Liu, J., Duan, F., Cong, L., and Qi, X. (2021). The role of the microRNA regulatory network in Alzheimer’s disease: a bioinformatics analysis. Arch. Med. Sci. 18, 206–222. doi: 10.5114/aoms/80619
Sun, H., Li, J.-J., Feng, Z.-R., Liu, H.-Y., and Meng, A.-G. (2020a). MicroRNA-124 regulates cell pyroptosis during cerebral ischemia-reperfusion injury by regulating STAT3. Exp. Ther. Med. 20:227. doi: 10.3892/etm.2020.9357
Sun, J.-J., Zhang, X.-Y., Qin, X.-D., Zhang, J., Wang, M.-X., and Yang, J.-B. (2019). MiRNA-210 induces the apoptosis of neuronal cells of rats with cerebral ischemia through activating HIF-1α-VEGF pathway. Eur. Rev. Med. Pharmacol. Sci. 23, 2548–2554. doi: 10.26355/eurrev_201903_17403
Sun, P., Liu, D. Z., Jickling, G. C., Sharp, F. R., and Yin, K.-J. (2018a). MicroRNA-based therapeutics in central nervous system injuries. J. Cereb. Blood Flow Metab. Off. J. Int. Soc. Cereb. Blood Flow Metab. 38, 1125–1148. doi: 10.1177/0271678X18773871
Sun, R., Peng, M., Xu, P., Huang, F., Xie, Y., Li, J., et al. (2020b). Low-density lipoprotein receptor (LDLR) regulates NLRP3-mediated neuronal pyroptosis following cerebral ischemia/reperfusion injury. J. Neuroinflammation 17:330. doi: 10.1186/s12974-020-01988-x
Sun, X., Li, X., Ma, S., Guo, Y., and Li, Y. (2018b). MicroRNA-98-5p ameliorates oxygen-glucose deprivation/reoxygenation (OGD/R)-induced neuronal injury by inhibiting Bach1 and promoting Nrf2/ARE signaling. Biochem. Biophys. Res. Commun. 507, 114–121. doi: 10.1016/j.bbrc.2018.10.182
Sun, Y., Chen, P., Zhai, B., Zhang, M., Xiang, Y., Fang, J., et al. (2020c). The emerging role of ferroptosis in inflammation. Biomed. Pharmacother. Biomedecine Pharmacother. 127:110108. doi: 10.1016/j.biopha.2020.110108
Tang, H., Gamdzyk, M., Huang, L., Gao, L., Lenahan, C., Kang, R., et al. (2020). Delayed recanalization after MCAO ameliorates ischemic stroke by inhibiting apoptosis via HGF/c-Met/STAT3/Bcl-2 pathway in rats. Exp. Neurol. 330:113359. doi: 10.1016/j.expneurol.2020.113359
Tao, Z., Zhao, H., Wang, R., Liu, P., Yan, F., Zhang, C., et al. (2015). Neuroprotective effect of microRNA-99a against focal cerebral ischemia–reperfusion injury in mice. J. Neurol. Sci. 355, 113–119. doi: 10.1016/j.jns.2015.05.036
Liang, Z., Wu, G., Fan, C., Xu, J., Jiang, S., Yan, X., et al. (2016). The emerging role of signal transducer and activator of transcription 3 in cerebral ischemic and hemorrhagic stroke. Prog. Neurobiol. 137, 1–16. doi: 10.1016/j.pneurobio.2015.11.001
Tian, Y. S., Zhong, D., Liu, Q. Q., Zhao, X. L., Sun, H. X., Jin, J., et al. (2018). Upregulation of miR-216a exerts neuroprotective effects against ischemic injury through negatively regulating JAK2/STAT3-involved apoptosis and inflammatory pathways. J. Neurosurg. 130, 977–988. doi: 10.3171/2017.5.JNS163165
Tiedt, S., Prestel, M., Malik, R., Schieferdecker, N., Duering, M., Kautzky, V., et al. (2017). RNA-seq identifies circulating miR-125a-5p, miR-125b-5p, and miR-143-3p as potential biomarkers for acute ischemic stroke. Circ. Res. 121, 970–980. doi: 10.1161/CIRCRESAHA.117.311572
Tu, Y., and Hu, Y. (2021). MiRNA-34c-5p protects against cerebral ischemia/reperfusion injury: involvement of anti-apoptotic and anti-inflammatory activities. Metab. Brain Dis. 36, 1341–1351. doi: 10.1007/s11011-021-00724-5
Tüfekci, K. U., Oner, M. G., Meuwissen, R. L. J., and Genç, S. (2014). The role of microRNAs in human diseases. Methods Mol. Biol. Clifton NJ 1107, 33–50. doi: 10.1007/978-1-62703-748-8_3
Tuo, Q.-Z., Lei, P., Jackman, K. A., Li, X.-L., Xiong, H., Li, X.-L., et al. (2017). Tau-mediated iron export prevents ferroptotic damage after ischemic stroke. Mol. Psychiatry 22, 1520–1530. doi: 10.1038/mp.2017.171
Tuo, Q.-Z., Liu, Y., Xiang, Z., Yan, H.-F., Zou, T., Shu, Y., et al. (2022). Thrombin induces ACSL4-dependent ferroptosis during cerebral ischemia/reperfusion. Signal Transduct. Target. Ther. 7:59. doi: 10.1038/s41392-022-00917-z
Turovsky, E. A., Varlamova, E. G., and Plotnikov, E. Y. (2021). Mechanisms underlying the protective effect of the peroxiredoxin-6 are mediated via the protection of astrocytes during ischemia/reoxygenation. Int J Mol Sci 22:8805. doi: 10.3390/ijms22168805
Turrens, J. F. (2003). Mitochondrial formation of reactive oxygen species. J. Physiol. 552, 335–344. doi: 10.1113/jphysiol.2003.049478
Vandenabeele, P., Galluzzi, L., Vanden Berghe, T., and Kroemer, G. (2010). Molecular mechanisms of necroptosis: an ordered cellular explosion. Nat. Rev. Mol. Cell Biol. 11, 700–714. doi: 10.1038/nrm2970
Vijayan, M., and Reddy, P. H. (2016). Peripheral biomarkers of stroke: focus on circulatory microRNAs. Biochim. Biophys. Acta 1862, 1984–1993. doi: 10.1016/j.bbadis.2016.08.003
Wan, Y., Jin, H.-J., Zhu, Y.-Y., Fang, Z., Mao, L., He, Q., et al. (2018). MicroRNA-149-5p regulates blood-brain barrier permeability after transient middle cerebral artery occlusion in rats by targeting S1PR2 of pericytes. FASEB J. Off. Publ. Fed. Am. Soc. Exp. Biol. 32, 3133–3148. doi: 10.1096/fj.201701121R
Wang, J., Xu, Z., Chen, X., Li, Y., Chen, C., Wang, C., et al. (2018). MicroRNA-182-5p attenuates cerebral ischemia-reperfusion injury by targeting toll-like receptor 4. Biochem. Biophys. Res. Commun. 505, 677–684. doi: 10.1016/j.bbrc.2018.09.165
Wang, K., Ru, J., Zhang, H., Chen, J., Lin, X., Lin, Z., et al. (2020a). Melatonin enhances the therapeutic effect of plasma exosomes against cerebral ischemia-induced pyroptosis through the TLR4/NF-κB pathway. Front. Neurosci. 14:848. doi: 10.3389/fnins.2020.00848
Wang, L., Zhuang, L., Rong, H., Guo, Y., Ling, X., Wang, R., et al. (2015a). MicroRNA-101 inhibits proliferation of pulmonary microvascular endothelial cells in a rat model of hepatopulmonary syndrome by targeting the JAK2/STAT3 signaling pathway. Mol. Med. Rep. 12, 8261–8267. doi: 10.3892/mmr.2015.4471
Wang, M., Chen, Z., Yang, L., and Ding, L. (2021a). Sappanone a protects against inflammation, oxidative stress and apoptosis in cerebral ischemia-reperfusion injury by alleviating endoplasmic reticulum stress. Inflammation 44, 934–945. doi: 10.1007/s10753-020-01388-6
Wang, N., Zhang, L., Lu, Y., Zhang, M., Zhang, Z., Wang, K., et al. (2017). Down-regulation of microRNA-142-5p attenuates oxygen-glucose deprivation and reoxygenation-induced neuron injury through up-regulating Nrf2/ARE signaling pathway. Biomed. Pharmacother. 89, 1187–1195. doi: 10.1016/j.biopha.2017.03.011
Wang, P., Cui, Y., Ren, Q., Yan, B., Zhao, Y., Yu, P., et al. (2021b). Mitochondrial ferritin attenuates cerebral ischaemia/reperfusion injury by inhibiting ferroptosis. Cell Death Dis. 12:447. doi: 10.1038/s41419-021-03725-5
Wang, P., Liang, X., Lu, Y., Zhao, X., and Liang, J. (2016). MicroRNA-93 downregulation ameliorates cerebral ischemic injury through the Nrf2/HO-1 defense pathway. Neurochem. Res. 41, 2627–2635. doi: 10.1007/s11064-016-1975-0
Wang, P., Pan, R., Weaver, J., Jia, M., Yang, X., Yang, T., et al. (2021c). MicroRNA-30a regulates acute cerebral ischemia-induced blood–brain barrier damage through ZnT4/zinc pathway. J. Cereb. Blood Flow Metab. 41, 641–655. doi: 10.1177/0271678X20926787
Wang, Q.-C., Lu, L., and Zhou, H.-J. (2019). Relationship between the MAPK/ERK pathway and neurocyte apoptosis after cerebral infarction in rats. Eur. Rev. Med. Pharmacol. Sci. 23, 5374–5381. doi: 10.26355/eurrev_201906_18206
Wang, S., and Liu, Z. (2021). Inhibition of microRNA-143-3p Attenuates cerebral ischemia/reperfusion injury by targeting FSTL1. Neuromolecular Med. 23, 500–510. doi: 10.1007/s12017-021-08650-6
Wang, X., Shi, C., Pan, H., Meng, X., and Ji, F. (2020b). MicroRNA-22 exerts its neuroprotective and angiogenic functions via regulating PI3K/Akt signaling pathway in cerebral ischemia-reperfusion rats. J. Neural Transm. Vienna Austria 1996, 35–44. doi: 10.1007/s00702-019-02124-7
Wang, Y., Huang, J., Ma, Y., Tang, G., Liu, Y., Chen, X., et al. (2015b). MicroRNA-29b is a therapeutic target in cerebral ischemia associated with aquaporin 4. J. Cereb. Blood Flow Metab. Off. J. Int. Soc. Cereb. Blood Flow Metab. 35, 1977–1984. doi: 10.1038/jcbfm.2015.156
Wang, Y., Xiao, G., He, S., Liu, X., Zhu, L., Yang, X., et al. (2020c). Protection against acute cerebral ischemia/reperfusion injury by QiShenYiQi via neuroinflammatory network mobilization. Biomed. Pharmacother. 125:109945. doi: 10.1016/j.biopha.2020.109945
Washida, K., Hattori, Y., and Ihara, M. (2019). Animal models of chronic cerebral hypoperfusion: from mouse to primate. Int. J. Mol. Sci. 20:E6176. doi: 10.3390/ijms20246176
Wei, R., Zhang, R., Xie, Y., Shen, L., and Chen, F. (2015). Hydrogen suppresses hypoxia/reoxygenation-induced cell death in hippocampal neurons through reducing oxidative stress. Cell. Physiol. Biochem. 36, 585–598. doi: 10.1159/000430122
Weng, H., Shen, C., Hirokawa, G., Ji, X., Takahashi, R., Shimada, K., et al. (2011). Plasma miR-124 as a biomarker for cerebral infarction. Biomed. Res. Tokyo Jpn. 32, 135–141. doi: 10.2220/biomedres.32.135
Wood, A. J., Vassallo, A. M., Ruchaud-Sparagano, M.-H., Scott, J., Zinnato, C., Gonzalez-Tejedo, C., et al. (2020). C5a impairs phagosomal maturation in the neutrophil through phosphoproteomic remodeling. JCI Insight 5:137029. doi: 10.1172/jci.insight.137029
Wu, L., Xiong, X., Wu, X., Ye, Y., Jian, Z., Zhi, Z., et al. (2020). Targeting oxidative stress and inflammation to prevent ischemia-reperfusion injury. Front. Mol. Neurosci. 13:28. doi: 10.3389/fnmol.2020.00028
Wu, M. Y., Yiang, G. T., Liao, W. T., Tsai, A. P. Y., Cheng, Y. L., Cheng, P. W., et al. (2018). Current mechanistic concepts in ischemia and reperfusion injury. Cell. Physiol. Biochem. 46, 1650–1667. doi: 10.1159/000489241
Wu, Y., Yang, S., Zheng, Z., Pan, H., Jiang, Y., Bai, X., et al. (2021). MiR-191-5p disturbed the angiogenesis in a mice model of cerebral infarction by targeting inhibition of bdnF. Neurol. India 69, 1601–1607. doi: 10.4103/0028-3886.333459
Wu, Y., Yao, J., and Feng, K. (2020c). miR-124-5p/NOX2 axis modulates the ROS production and the inflammatory microenvironment to protect against the cerebral I/R injury. Neurochem. Res. 45, 404–417. doi: 10.1007/s11064-019-02931-0
Xia, P. P., Zhang, F., Chen, C., Wang, Z. H., Wang, N., Li, L. Y., et al. (2020). Rac1 relieves neuronal injury induced by oxygenglucose deprivation and re-oxygenation via regulation of mitochondrial biogenesis and function. Neural Regen. Res. 15, 1937–1946. doi: 10.4103/1673-5374.280325
Xie, W., Zhu, T., Dong, X., Nan, F., Meng, X., Zhou, P., et al. (2019). HMGB1-triggered inflammation inhibition of notoginseng leaf triterpenes against cerebral ischemia and reperfusion injury via MAPK and NF-κB signaling pathways. Biomolecules 9:512. doi: 10.3390/biom9100512
Xie, Y.-L., Zhang, B., and Jing, L. (2018). MiR-125b blocks Bax/Cytochrome C/Caspase-3 apoptotic signaling pathway in rat models of cerebral ischemia-reperfusion injury by targeting p53. Neurol. Res. 40, 828–837. doi: 10.1080/01616412.2018.1488654
Xing, F., Liu, Y., Dong, R., and Cheng, Y. (2021). miR-374 improves cerebral ischemia reperfusion injury by targeting Wnt5a. Exp. Anim. 70, 126–136. doi: 10.1538/expanim.20-0034
Xu, H., Nie, B., Liu, L., Zhang, C., Zhang, Z., Xu, M., et al. (2019). Curcumin prevents brain damage and cognitive dysfunction during ischemic-reperfusion through the regulation of miR-7-5p. Curr. Neurovasc. Res. 16, 441–454. doi: 10.2174/1567202616666191029113633
Xu, Y., Liu, Y., Li, K., Yuan, D., Yang, S., Zhou, L., et al. (2022). COX-2/PGE2 pathway inhibits the ferroptosis induced by cerebral ischemia reperfusion. Mol. Neurobiol. 59, 1619–1631. doi: 10.1007/s12035-021-02706-1
Xue, W.-S., Wang, N., Wang, N.-Y., Ying, Y.-F., and Xu, G.-H. (2019). miR-145 protects the function of neuronal stem cells through targeting MAPK pathway in the treatment of cerebral ischemic stroke rat. Brain Res. Bull. 144, 28–38. doi: 10.1016/j.brainresbull.2018.08.023
Yan, H., Kanki, H., Matsumura, S., Kawano, T., Nishiyama, K., Sugiyama, S., et al. (2021). MiRNA-132/212 regulates tight junction stabilization in blood-brain barrier after stroke. Cell Death Discov. 7:380. doi: 10.1038/s41420-021-00773-w
Yang, C., Wei, X., Fu, X., Qian, L., Xie, L., Liu, H., et al. (2021). Down-regulating microRNA-20a regulates CDH1 to protect against cerebral ischemia/reperfusion injury in rats. Cell Cycle 20, 54–64. doi: 10.1080/15384101.2020.1856498
Yang, J. (2019). The role of reactive oxygen species in angiogenesis and preventing tissue injury after brain ischemia. Microvasc. Res. 123, 62–67. doi: 10.1016/j.mvr.2018.12.005
Yang, T., Feng, C., Wang, D., Qu, Y., Yang, Y., Wang, Y., et al. (2020). Neuroprotective and anti-inflammatory effect of tangeretin against cerebral ischemia-reperfusion injury in rats. Inflammation 43, 2332–2343. doi: 10.1007/s10753-020-01303-z
Yao, X., Wang, Y., and Zhang, D. (2018). microRNA-21 Confers neuroprotection against cerebral ischemia-reperfusion injury and alleviates blood-brain barrier disruption in rats via the MAPK signaling pathway. J. Mol. Neurosci. 65, 43–53. doi: 10.1007/s12031-018-1067-5
Yao, X., Yao, R., Yi, J., and Huang, F. (2019). Upregulation of miR-496 decreases cerebral ischemia/reperfusion injury by negatively regulating BCL2L14. Neurosci. Lett. 696, 197–205. doi: 10.1016/j.neulet.2018.12.039
Ying, X.-D., Wei, G., and An, H. (2021). Sodium butyrate relieves lung ischemia-reperfusion injury by inhibiting NF-κB and JAK2/STAT3 signaling pathways. Eur. Rev. Med. Pharmacol. Sci. 25, 413–422. doi: 10.26355/eurrev_202101_24409
Yu, H., Wu, M., Zhao, P., Huang, Y., Wang, W., and Yin, W. (2015). Neuroprotective effects of viral overexpression of microRNA-22 in rat and cell models of cerebral ischemia-reperfusion injury. J. Cell. Biochem. 116, 233–241. doi: 10.1002/jcb.24960
Yu, P., Zhang, X., Liu, N., Tang, L., Peng, C., and Chen, X. (2021a). Pyroptosis: mechanisms and diseases. Signal Transduct. Target. Ther. 6, 1–21. doi: 10.1038/s41392-021-00507-5
Yu, S., Zhai, J., Yu, J., Yang, Q., and Yang, J. (2021b). miR-98-5p protects against cerebral ischemia/reperfusion injury through anti-apoptosis and anti-oxidative stress in mice. J. Biochem. 169, 195–206. doi: 10.1093/jb/mvaa099
Yu, S.-J., Yu, M.-J., Bu, Z.-Q., He, P.-P., and Feng, J. (2020). MicroRNA-670 aggravates cerebral ischemia/reperfusion injury via the Yap pathway. Neural Regen. Res. 16, 1024–1030. doi: 10.4103/1673-5374.300455
Yu, S.-J., Yu, M.-J., Bu, Z.-Q., He, P.-P., and Feng, J. (2021c). MicroRNA-670 aggravates cerebral ischemia/reperfusion injury via the yap pathway. Neural Regen. Res. 16, 1024–1030. doi: 10.4103/1673-5374.300455
Yue, Y., Zhao, H., Yue, Y., Zhang, Y., and Wei, W. (2020). Downregulation of microrna-421 relieves cerebral ischemia/reperfusion injuries: involvement of anti-apoptotic and antioxidant activities. NeuroMolecular Med. 22, 411–419. doi: 10.1007/s12017-020-08600-8
Zador, Z., Stiver, S., Wang, V., and Manley, G. T. (2009). Role of aquaporin-4 in cerebral edema and stroke. Handb. Exp. Pharmacol. 190, 159–170. doi: 10.1007/978-3-540-79885-9_7
Zeng, L., Liu, J., Wang, Y., Wang, L., Weng, S., Tang, Y., et al. (2011). MicroRNA-210 as a novel blood biomarker in acute cerebral ischemia. Front. Biosci. Elite Ed. 3:1265–1272. doi: 10.2741/e330
Zeng, Z., Zhang, Y., Liang, X., Wang, F., Zhao, J., Xu, Z., et al. (2019). Qingnao dripping pills mediate immune-inflammatory response and MAPK signaling pathway after acute ischemic stroke in rats. J. Pharmacol. Sci. 139, 143–150. doi: 10.1016/j.jphs.2018.12.009
Zhang, H., Liu, X., Yang, F., Cheng, D., and Liu, W. (2020a). Overexpression of HIF-1α protects PC12 cells against OGD/R-evoked injury by reducing miR-134 expression. Cell Cycle Georget. Tex 19, 990–999. doi: 10.1080/15384101.2020.1743903
Zhang, H.-S., Ouyang, B., Ji, X.-Y., and Liu, M.-F. (2021a). Gastrodin alleviates cerebral ischaemia/reperfusion injury by inhibiting pyroptosis by regulating the lncRNA NEAT1/miR-22-3p axis. Neurochem. Res. 46, 1747–1758. doi: 10.1007/s11064-021-03285-2
Zhang, H.-T., Zhang, P., Gao, Y., Li, C.-L., Wang, H.-J., Chen, L.-C., et al. (2017). Early VEGF inhibition attenuates blood-brain barrier disruption in ischemic rat brains by regulating the expression of MMPs. Mol. Med. Rep. 15, 57–64. doi: 10.3892/mmr.2016.5974
Zhang, J., Shi, L., Zhang, L., Zhao, Z., Liang, F., Xu, X., et al. (2016). MicroRNA-25 negatively regulates cerebral ischemia/reperfusion injury-induced cell apoptosis through Fas/FasL pathway. J. Mol. Neurosci. 58, 507–516. doi: 10.1007/s12031-016-0712-0
Zhang, R. L., Chopp, M., Chen, H., and Garcia, J. H. (1994). Temporal profile of ischemic tissue damage, neutrophil response, and vascular plugging following permanent and transient (2H) middle cerebral artery occlusion in the rat. J. Neurol. Sci. 125, 3–10. doi: 10.1016/0022-510x(94)90234-8
Zhang, S. (2019). Microglial activation after ischaemic stroke. Stroke Vasc. Neurol. 4, 71–74. doi: 10.1136/svn-2018-000196
Zhang, S., Chen, A., and Chen, X. (2021b). A feedback loop involving MicroRNA-150 and MYB regulates VEGF expression in brain microvascular endothelial cells after oxygen glucose deprivation. Front. Physiol. 12:619904. doi: 10.3389/fphys.2021.619904
Zhang, T., Tian, C., Wu, J., Zhang, Y., Wang, J., Kong, Q., et al. (2020b). MicroRNA-182 exacerbates blood-brain barrier (BBB) disruption by downregulating the mTOR/FOXO1 pathway in cerebral ischemia. FASEB J. Off. Publ. Fed. Am. Soc. Exp. Biol. 34, 13762–13775. doi: 10.1096/fj.201903092R
Zhang, Y., Lu, X., Tai, B., Li, W., and Li, T. (2021c). Ferroptosis and Its multifaceted roles in cerebral stroke. Front. Cell. Neurosci. 15:615372. doi: 10.3389/fncel.2021.615372
Zhang, Y., Shan, Z., Zhao, Y., and Ai, Y. (2019a). Sevoflurane prevents miR-181a-induced cerebral ischemia/reperfusion injury. Chem. Biol. Interact. 308, 332–338. doi: 10.1016/j.cbi.2019.06.008
Zhang, Z., Wang, N., Zhang, Y., Zhao, J., and Lv, J. (2019b). Downregulation of microRNA-302b-3p relieves oxygen-glucose deprivation/re-oxygenation induced injury in murine hippocampal neurons through up-regulating Nrf2 signaling by targeting fibroblast growth factor 15/19. Chem. Biol. Interact. 309:108705. doi: 10.1016/j.cbi.2019.06.018
Zhang, Z., Yao, L., Yang, J., Wang, Z., and Du, G. (2018). PI3K/Akt and HIF-1 signaling pathway in hypoxia-ischemia (Review). Mol. Med. Rep. 18, 3547–3554. doi: 10.3892/mmr.2018.9375
Zhao, B., Wang, P., Yu, J., and Zhang, Y. (2021). MicroRNA-376b-5p targets SOX7 to alleviate ischemic brain injury in a mouse model through activating Wnt/β-catenin signaling pathway. Life Sci. 270:119072. doi: 10.1016/j.lfs.2021.119072
Zhao, H., Tao, Z., Wang, R., Liu, P., Yan, F., Li, J., et al. (2014). MicroRNA-23a-3p attenuates oxidative stress injury in a mouse model of focal cerebral ischemia-reperfusion. Brain Res. 1592, 65–72. doi: 10.1016/j.brainres.2014.09.055
Zhao, J., and Wang, B. (2020). MiR-7-5p Enhances cerebral ischemia-reperfusion injury by degrading sirt1 mRNA. J. Cardiovasc. Pharmacol. 76, 227–236. doi: 10.1097/FJC.0000000000000852
Zhao, J., Wu, Y., Liang, S., and Piao, X. (2022). Activation of SSAT1/ALOX15 axis aggravates cerebral ischemia/reperfusion injury via triggering neuronal ferroptosis. Neuroscience 485, 78–90. doi: 10.1016/j.neuroscience.2022.01.017
Zheng, T., Shi, Y., Zhang, J., Peng, J., Zhang, X., Chen, K., et al. (2019). MiR-130a exerts neuroprotective effects against ischemic stroke through PTEN/PI3K/AKT pathway. Biomed. Pharmacother. 117:109117. doi: 10.1016/j.biopha.2019.109117
Zhi, F., Shao, N., Wang, R., Deng, D., Xue, L., Wang, Q., et al. (2015). Identification of 9 serum microRNAs as potential noninvasive biomarkers of human astrocytoma. Neuro-Oncol. 17, 383–391. doi: 10.1093/neuonc/nou169
Keywords: miRNAs, ischemia reperfusion, cell death, inflammation, oxidative stress
Citation: Neag M-A, Mitre A-O, Burlacu C-C, Inceu A-I, Mihu C, Melincovici C-S, Bichescu M and Buzoianu A-D (2022) miRNA Involvement in Cerebral Ischemia-Reperfusion Injury. Front. Neurosci. 16:901360. doi: 10.3389/fnins.2022.901360
Received: 21 March 2022; Accepted: 23 May 2022;
Published: 10 June 2022.
Edited by:
Yongjun Sun, Hebei University of Science and Technology, ChinaCopyright © 2022 Neag, Mitre, Burlacu, Inceu, Mihu, Melincovici, Bichescu and Buzoianu. This is an open-access article distributed under the terms of the Creative Commons Attribution License (CC BY). The use, distribution or reproduction in other forums is permitted, provided the original author(s) and the copyright owner(s) are credited and that the original publication in this journal is cited, in accordance with accepted academic practice. No use, distribution or reproduction is permitted which does not comply with these terms.
*Correspondence: Andrei-Otto Mitre, YW5kcmVpLm1pdHJlOTdAZ21haWwuY29t
Disclaimer: All claims expressed in this article are solely those of the authors and do not necessarily represent those of their affiliated organizations, or those of the publisher, the editors and the reviewers. Any product that may be evaluated in this article or claim that may be made by its manufacturer is not guaranteed or endorsed by the publisher.
Research integrity at Frontiers
Learn more about the work of our research integrity team to safeguard the quality of each article we publish.