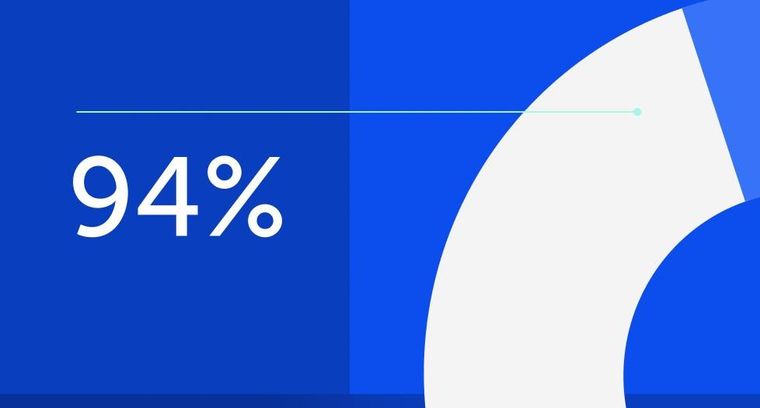
94% of researchers rate our articles as excellent or good
Learn more about the work of our research integrity team to safeguard the quality of each article we publish.
Find out more
REVIEW article
Front. Neurosci., 02 May 2022
Sec. Neurodegeneration
Volume 16 - 2022 | https://doi.org/10.3389/fnins.2022.899887
This article is part of the Research TopicBrain Hypoxia and Ischemia: New Insights into Neurodegeneration and Neuroprotection, Volume IIView all 9 articles
Ischemic stroke is defined as an infarction in the brain, caused by impaired cerebral blood supply, leading to local brain tissue ischemia, hypoxic necrosis, and corresponding neurological deficits. At present, revascularization strategies in patients with acute ischemic stroke include intravenous thrombolysis and mechanical endovascular treatment. However, due to the short treatment time window (<4.5 h) and method restrictions, clinical research is focused on new methods to treat ischemic stroke. Exosomes are nano-sized biovesicles produced in the endosomal compartment of most eukaryotic cells, containing DNA, complex RNA, and protein (30–150 nm). They are released into surrounding extracellular fluid upon fusion between multivesicular bodies and the plasma membrane. Exosomes have the characteristics of low immunogenicity, good innate stability, high transmission efficiency, and the ability to cross the blood–brain barrier, making them potential therapeutic modalities for the treatment of ischemic stroke. The seed sequence of miRNA secreted by exosomes is base-paired with complementary mRNA to improve the microenvironment of ischemic tissue, thereby regulating downstream signal transduction activities. With exosome research still in the theoretical and experimental stages, this review aims to shed light on the potential of exosomes derived from mesenchymal stem cells in the treatment of ischemic stroke.
Ischemic stroke (IS) is characterized by the sudden loss of blood circulation to an area of the brain, resulting in a corresponding loss of neurologic function. Acute IS is caused by thrombotic or embolic occlusion of a cerebral artery and is the leading cause of death and acquired disability worldwide (Ai et al., 2021). Cerebral artery thrombosis accounts for > 80% of all strokes (Liu et al., 2021). Cerebral ischemia can lead to various secondary harmful effects, including reperfusion injury, ischemia hypoxia, increased intracellular calcium levels, blood–brain barrier (BBB) damage, glial cell activation, oxidative stress, ion imbalance, apoptosis, and inflammation, ultimately causing neuronal damage and death. These pathological reactions eventually lead to corresponding neurologic symptoms, such as cognitive, motor, and sensory dysfunction, which seriously affect the physical health and quality of life of patients with IS. The 30-day mortality rate is 5–15%, and the disability rate exceeds 50% (Strain et al., 2021). Without effective treatment within 4.5 h after onset, the mortality and disability rates of IS are even higher (Liu et al., 2021). Rapid reperfusion with intravenous thrombolysis and endovascular thrombectomy represent the mainstays of treatment. However, due to the strict inclusion criteria and the many contraindications for thrombolysis, including the risk of hemorrhagic transformation, the clinical applications of endovascular therapy remain limited (Ai et al., 2021). Therefore, developing new treatment methods is necessary.
Exosomes are membrane vesicles secreted by any cell type and are generally released into the intercellular space after binding to the plasma membrane (Cai et al., 2021). They contain several biologically active genomic and non-genomic molecules, including DNA, miRNAs, mRNA, lncRNA, various proteins, lipids, and enzymes, which can change the biological behavior of cells through paracrine or autocrine mechanisms (Baruah and Wary, 2019). The special phospholipid bilayer of the exosomal membrane can prevent the decomposition of biologically active substances under harsh conditions and facilitate the storage of these exosomes in vitro (Quaglia et al., 2022). These biological properties provide the basis for potentially new treatment modalities. In recent years, many studies have focused on the different functions of exosomes in the treatment of various diseases. Exosomes have been reported to promote neurological recovery and angiogenesis after IS (Xin et al., 2013, 2021; Yang et al., 2017; Baruah and Wary, 2019). Exosomes derived from mesenchymal stem cells (MSCs) are considered to have the greatest potential in the treatment of stroke; the secretion of exosomes by MSCs is considered a major mechanism by which the latter promotes neurovascular remodeling and the recovery of neurological function after stroke (Hong et al., 2019).
Here, we review studies on exosomes derived from MSCs, which demonstrate that in various ischemic diseases, exosomes released by MSCs affect most damaged tissues (Anderson et al., 2016). Among them, bone marrow-derived MSCs send signals to endogenous cell groups, including immune cells and endothelial cells, to trigger their tissue healing ability (Shang et al., 2022). Animal experiments have shown that transplantation of bone-marrow MSCs (BMSCs) can significantly improve the neurological deficit and quality of life in the middle cerebral artery occlusion (MACO) rat model (Zhang Z. et al., 2021), and this effect may likely be due to exosomes secreted by stem cells (Chen H. et al., 2022). Whether other types of MSCs have the ability to improve the microenvironment, reduce inflammatory response, and promote microvascular response in IS is still unclear.
IS is a cascade of damage responses secondary to neurovascular injury during cerebral cell ischemia and hypoxia, mainly caused by cerebral artery occlusion of thrombotic or embolic origin.
Reperfusion is the action by which blood supply is restored to the brain (Barzegar et al., 2021). Reperfusion of the ischemic area is the most effective method to protect brain structure and function, though it can also cause reperfusion injury (Castelli et al., 2021). Reperfusion injury after IS, defined as the rapid increase in tissue damage after the blood supply to ischemic brain tissue cells is restored, may occur after thrombolysis with tissue plasminogen activator (t-PA), after mechanical thrombectomy, or as a result of spontaneous reperfusion (Lin et al., 2016). Reperfusion, although theoretically beneficial to hypoxic tissues, can produce paradoxical tissue responses such as calcium overload, promotion of reactive oxygen species (ROS), deposition of pro-inflammatory immune cells in ischemic tissue, activation of glial cells, and damage of the blood–brain barrier (Kalogeris et al., 2016).
When an IS occurs, brain tissue ischemia and hypoxia will interfere with the oxidative phosphorylation of mitochondria, leading to disorders of energy metabolism and reduced ATP production. Tissue ATP is rapidly depleted, and calcium ions (Ca2+) are released from the mitochondria and endoplasmic reticulum (Shi et al., 2021). If the depleted ATP pump function does not recover after reperfusion, significant extracellular fluid Ca2+ will flow into the intracellular fluid. This process not only produces free radicals but also makes large blood vessels contract, thereby aggravating ischemia and hypoxia and leading to the formation of a vicious circle. This is the main pathogenesis of extensive tissue cell injury (Liu et al., 2018) (Figure 1).
Figure 1. Pathological process of ischemic stroke: (1) Arterial thrombosis or arterial embolism will lead to ischemia and hypoxia of nerve cells, leading to damage of mitochondrial structure and function, decrease of ATP generation, increase of intracellular calcium overload and free radical generation, and aggravation of ischemia and hypoxia. (2) In addition, this process can damage endothelial cells and increase inflammatory response. (3) Mitochondrial structure damage can increase the concentration of OH–, causing oxidative stress. (4) Reperfusion injury can increase mitochondrial membrane potential (MMP), resulting in increased OH– production and increased oxidative stress.
After ischemia and reperfusion, the function and structure of the mitochondria are impaired, leading to an increase of the oxygen atom tetravalent, leakage, and the generation of hydroxyl free radicals. Another important source of ROS is reduced nicotinamide adenine dinucleotide phosphate oxidase (NOX). Studies have revealed that ischemia–reperfusion can increase the mitochondrial membrane potential, leading to excessive production of ROS (Lin et al., 2016). When ROS exceeds the antioxidant capacity, oxidative stress will occur, which directly damages all cellular components, including protein, DNA, RNA, and lipids.
After endothelial cell (EC) injury, leukocyte infiltration and the subsequent inflammatory response play a crucial role. Activated leukocytes adhere to the EC, infiltrate brain tissue, destroy the BBB, and release pro-inflammatory factors (TNF-α, IL-1, IL-6, MCP1) and inflammatory mediators (ROS, NOS), leading to the deterioration of the ischemic penumbra (Cai et al., 2021). IL-1 can induce the release of arachidonic acid, promote the production of TNF-α and IL-1β, lead to the deposition of inflammatory cells, and aggravate tissue damage (Zhang et al., 2018).
Microglia are the main innate immune cells in the central nervous system; they play an important role in regulating neuroinflammation (Heneka et al., 2014). Both IS and reperfusion injury can increase the morphological changes and activity of the microglia (Surinkaew et al., 2018). Once cerebral ischemia/reperfusion (I/R) injury occurs, the resting microglia enter the active state (Hu et al., 2012). The activated microglia will be recruited to the ischemic and penumbra areas to play either neuroprotective or inflammatory roles (Xiong et al., 2016). Brain damage caused by stroke activates and differentiates microglia into M1 or M2 phenotypes (Song et al., 2019). M1 phenotypes have been confirmed to express CD86+, CD206–, and CD16/32+ and produce high levels of pro-inflammatory cytokines, such as INF-γ, IL-6, TNF-α, IL1-β, and KC/GRO/cytokine-induced neutrophil chemoattractant (CINC) (Spellicy and Stice, 2021). The differentiation of microglia strengthens the deterioration of the local inflammatory environment, promotes the progress of acute brain injury, and hinders nerve regeneration after injury and subsequent long-term functional recovery (Zhang Z. et al., 2021). In contrast to the M1 type, the M2 type is the “good” phenotype, which can protect the nerves from ischemia and hypoxia, and promote the long-term recovery after a stroke (Song et al., 2019). Studies have revealed that the M2 phenotype (CD86–, CD206+) increased the gene expression of Arg-1, IL-10, STAT6; released CXCL1, GROα, neutrophil activating protein alpha, CINC; and induced a more restorative local environment (Spellicy and Stice, 2021). Based on their ability to produce IL-4 and IL-10, adjacent cells can be protected by removing cell debris and releasing nutrients Moreover, M2 microglia can also perform necroptosis and produce many protective and nutritional factors that promote neurogenesis (Zhang Z. et al., 2021; Figure 2).
Figure 2. Ischemia and hypoxia can lead to the polarization of microglia into M1 microglia and M2 microglia. The surface antigens of the two microglias are different. M1 microglia expresses CD16, CD32, and CD86, while M2 microglia expresses CD206. MiRNA in exosomes derived from different types of mesenchymal stem cells can regulate some inflammatory pathways or mechanisms to up-regulate or down-regulate the production of M1 and M2, thereby changing the ratio of M1/M2 in the brain and reducing the inflammatory response and secondary tissue damage involved in M1.
The key points of IS treatment are recanalization, saving ischemic penumbra, and reducing infarct size. The vascular reconstruction strategies for acute IS include intravenous thrombolysis and mechanical recanalization (Minnerup et al., 2016). Although these two treatment methods have excellent results in specific patient groups, they have strict selection criteria, which are related mostly but not exclusively to the narrow therapeutic time-window, thus limiting their clinical application (Chen et al., 2016). The efficacy of intravenous thrombolysis treating stroke is highly dependent on the treatment time (Ai et al., 2021). Initially, rt-PA was proven to be safe within 3 h after stroke; however, recent studies have extended this time window to 4.5 h (Bsat et al., 2021). Most patients present outside this time-window and are not eligible for thrombolysis. Moreover, due to the low recanalization rate, especially in treating severe stroke caused by occlusion of large intracranial vessels, the efficacy of drug thrombolysis is limited (Minnerup et al., 2016). Adjuvant drugs such as anticoagulants, antiplatelet agents, and anti-inflammatory drugs may have a certain role in extending the therapeutic window (Mahjoubin-Tehran et al., 2021). A new (“Lean Principles”) approach for optimizing the treatment process has also been reported, that allows patients with stroke to receive thrombolytic therapy within 60 min and improves their survival rate (Souza et al., 2021). Nevertheless, this approach does not directly address the problem of vascular stenosis caused by thromboembolism or atherosclerotic plaque. Thrombectomy is only suitable for patients with large vessel occlusion (Minnerup et al., 2016). Experiments suggest that mechanical thrombectomy combined with intravenous thrombolysis can significantly improve the neurological prognosis after 3 months and reduce mortality (fewer patients met inclusion criteria for both mechanical thrombectomy and intravenous thrombolysis) (Minnerup et al., 2016). Additionally, clinical randomized controlled trials have revealed that in patients with acute IS from large-vessel occlusion, endovascular thrombectomy alone was non-inferior in terms of functional outcomes, within a 20% margin of confidence, to endovascular thrombectomy preceded by intravenous alteplase administered within 4.5 h after symptom onset (Yang et al., 2020). Therefore, the majority of patients with IS still lack a treatment option that is both effective and safe, highlighting the need for an alternative treatment approach.
Increasing amount of data indicate that stem cell therapy is a promising option for many diseases that cannot be effectively treated by existing treatment strategies. Recently, stem cell therapy has demonstrated potential as a treatment option for neurological diseases such as Parkinson’s, amyotrophic lateral sclerosis, and Huntington’s disease (Chen et al., 2014, 2020; Minnerup et al., 2016; Tang et al., 2022). In addition to replace lost cells during ischemia–reperfusion (the replacement mechanism) (Hurd et al., 2021), stem cells also secrete various cytokines and growth factors to induce diverse beneficial effects, such as anti-inflammatory nerve cell protection and induction of the endogenic recovery system (Yoo et al., 2013), which can provide a good environment for recovery (paracrine mechanism), which is taking the lead (Salikhova et al., 2021). Exosomes are extracellular vesicles that are released by cells after the fusion of polycystic body and plasma membrane (Mahjoubin-Tehran et al., 2021). They are 50–100 nm in diameter and can be secreted by various types of cells, including bone marrow-derived mesenchymal stem cells (BMSCs), adipose-derived mesenchymal stem cells (ADMSCs), and human umbilical cord-derived mesenchymal stem cells (HUCMSCs) (Wang et al., 2018). Although different cells release exosomes with different molecules, exosomes contain traditional markers that make them significantly different from other extracellular vesicles (Hassanpour et al., 2020; Rezaie et al., 2021). CD9, CD63, and CD81 are the most commonly used markers of exosomes. As a conservative family of tetraspanin proteins, CD9, CD63, and CD81 are also associated with many different functions, including cell adhesion, motility, and proliferation, and are involved in exosome cargo selection and trafficking. They are often used as markers for identification, quantification, or purification of exosomes (Garcia-Martin et al., 2022). Several miRNAs are loaded in the exosomes of different cell-types, and they play an important role in the recovery of damaged neurons (Deng et al., 2019). MSCs are the most effective at producing exosomes (Cosenza et al., 2018). MSCs have the ability to self-renew, regenerate, and differentiate in damaged tissues; regulate inflammation; and activate microglia (Castro-Manrreza and Montesinos, 2015; Li et al., 2015). Moreover, some of the benefits of MSCs include their relative abundance (in human’s bone marrow), avoidance of ethical problems (not from fetuses such as neural stem cells and embryonic stem cells), and a lack of tumorigenicity compared to induced pluripotent stem cells (Stonesifer et al., 2017). This allows MSCs to provide the basis for effective new therapies for various human diseases. Although information on the exact mechanism of MSC-mediated restorative effect is limited, with < 1% of transplanted MSCs reaching and colonizing the target site (most of them are embedded in the pulmonary capillary bed due to their large size), the therapeutic effects observed may be mainly attributed to the production of paracrine factors from transplanted MSC-derived exosomes (Chen H. et al., 2022). Since the potency of exosomes in tissue regeneration and repair is greater than those of stem cells themselves, stem-cell derived exosomes have become the focus of recent studies (Kalani and Tyagi, 2015).
Exosomes derived from MSCs can pass through the BBB and can also regulate the gene expression of recipient cells by delivering functional substances. Because of their small size, exosomes can escape the phagocytosis of macrophages. Compared with polymer nanoparticles and liposomes, they can escape naturally and stably from lysosome degradation. Generally speaking, because exosomes are not immunogenic, they are good candidates for gene drug delivery (Yang et al., 2017). They can also mediate intercellular communication. Recent studies have confirmed that exosomes can affect angiogenesis and glial involvement by regulating the production of nucleotides and proteins, and participate in the remodeling process after cerebral infarction (Luo et al., 2019; Kim et al., 2020). It has been reported that > 20% of miRNAs in human body changes (increases or decreases) after IS, indicating that miRNA is an important mediator of IS (Mahjoubin-Tehran et al., 2021). MiRNAs are small, highly conserved, 20–25 nt non-coding RNAs in eukaryotes (Kabekkodu et al., 2018; Huuskonen et al., 2022). They target approximately 60% of mammalian genes after transcription and participate in most biological and pathophysiological processes (Xin et al., 2014). By pairing with the 3′UTR of target mRNA molecules, miRNA regulates the expression of target genes and downstream signaling pathways at the post-transcriptional level, resulting in degradation or inhibition of target mRNA translation (Soifer et al., 2007; Baruah and Wary, 2019).
Ischemic injury in other organs reportedly can be improved by using exosomes derived from MSCs (Table 1). For example, Mathew et al. (2019) discovered that MSC-extracellular vesicles (MSC-EV) can be used to treat retinal I/R injury. Experimental data support that MSC-EV significantly enhances functional recovery, and reduces neuroinflammation and apoptosis. Yan et al. (2020) discovered that in the exosomes derived from HUCMSCs, the mir-421/circhipk3/foxo3a pathway can be used to prevent pyrolysis and repair ischemic muscle damage. Similarly, Zhang et al. (2019) have reported experimental data supporting fracture repair and angiogenesis potential through hypoxia-inducible factor-1α (HIF1α). Hu et al. (2018) have reported that exosomes rich in miR-21-3p secreted by human umbilical cord blood cells can accelerate wound healing and promote angiogenesis. In recent years, the application of exosomes in the treatment of cardiovascular diseases has been commonly reported. Research has increasingly focused on myocardial ischemia injury, which is directly linked to the increase of the elderly population and subsequently to the increase of cardiovascular diseases. For instance, Wei et al. (2019) discovered that overexpression of miRNA-181a in MSC-derived exosomes can inhibit the inflammatory response after myocardial I/R injury. Khan et al. (2015) and Sun et al. (2020) have revealed that miR-221-3p and miR-294 from MSCs could promote the repair of damaged cardiomyocytes. Shi et al. (2018) identified that miR-21, an exosome derived from MSCs, down-regulated the expression of PTEN by activating the PI3K/AKT signaling pathway, thereby protecting cardiomyocytes from death induced by ischemia and oxidative stress. In addition, the latest study found that miRNA-21 can also improve myocardial remodeling after myocardial ischemia-reperfusion injury by reprogramming macrophages (Tan et al., 2021). Reprogrammed macrophages coordinate the remodeling of the myocardial microenvironment in the anti-inflammatory treatment of ischemia reperfusion (IR) injury (Tan et al., 2021). MiR-30c-5p can down-regulate BCL2-like 11 (BCL2L11) to improve myocardial injury, histopathological changes and apoptosis in rat I/R model (Meng et al., 2021). Meanwhile, MiR-29a-3p can attenuate ischemia reperfusion by up-regulating nuclear factor erythroid-2 related factor 2 (NRF2) and inhibiting Cyclin T2 (CCNT2) (Tian et al., 2021). MSCs/Exosomes/miRNAs are widely used in cardiac ischemic diseases, which may provide new guidance for the treatment of ischemic stroke. Xu F. et al. (2019) first demonstrated that miR-423-5p derived from ADMSCs is a key active molecule in exosome-induced angiogenesis. Additionally, in recent years, miR-423-5p has also been intensively researched in the diagnosis and treatment setting of various tumors (Shan et al., 2020; Jiang et al., 2021; Xue et al., 2022). Additionally, various studies have focused on exosome intercellular communication, storage of biological information, and the use of biomarkers, especially in the potential applications of regeneration and nerve protection (Kalani and Tyagi, 2015; Doyle and Wang, 2019; Gurunathan et al., 2019). Among them, miRNA can affect the various stages of cerebral ischemia injury and can worsen or reduce brain damage through various ways.
As mentioned before, the pathological response caused by IS is a complex process closely related to inflammation, calcium overload, and oxidative stress (Kalogeris et al., 2016; Enzmann et al., 2018; Castelli et al., 2021). This part of the article will elaborate on the different functions of miRNAs in different stroke-related pathological processes (Figure 3).
Figure 3. The source and function of stem cells: exosomes derived from various mesenchymal stem cells (bone marrow-, adipose-tissue and umbilical cord mesenchymal stem cells) secrete miRNA that can participate in many pathological or physiological processes of ischemic stroke. These miRNAs can reduce inflammation, oxidative stress, cell death/apoptosis, or promote the formation of blood vessels, cells and structures.
During IS, reperfusion causes an inflammatory cascade, a key mechanism of secondary neuronal damage and death after the initial ischemic attack. As already discussed, changes in the brain tissue microenvironment affect the resident microglia transformation to M1 or M2 phenotypes (polarization) (Spellicy and Stice, 2021). Balancing the two polarizations of microglia is a promising method for the treatment of stroke. Experimental results reveal that BMSCs-exosomes (BMSCs-Exos) can reverse the CysLT2R-ERK1/2-mediated microglial M1 polarization, and thus inhibit microglial injury (Zhao et al., 2020b). Cysteinyl leukotrienes (CysLTs) are mainly produced by the decomposition of necrotic cells, acting as an effective inflammatory medium that aggravates the development of ischemic penumbra. BMSCs-Exos significantly inhibited the expression of CysLT2R in microglia after IS, reduced the secretion of pro-inflammatory cytokines, and increased the production of anti-inflammatory and neurotrophic factors (Zhao et al., 2020b). In vitro data suggest that miR-223-3p from BMSCs-Exos reduces inflammatory damage by inhibiting the polarization of microglia M1, and this is closely related to the down-regulation of the transcription and expression of cyyslt2r (Zhao et al., 2020a). MiR-223 is highly encapsulated in the exosomes released by MSCs and thus it is the most abundant miRNA in MSCs (Morales et al., 2022). Exosomal miR-223 can effectively promote the transformation of harmful M1 microglia induced by NMLTC4/ltd4 to beneficial M2 phenotypes, thereby increasing the expression of anti-inflammatory cytokines (Morales et al., 2022). Further experimental data suggest that miRNA-26b-5p derived from HUCMSCs exosomes target CH25H to inactivate TLR pathway, thereby inhibiting M1 polarization and at the same time attenuate autophagy-induced brain injury by promoting M2 microglia polarization (Jiang et al., 2018; Li G. et al., 2020). The results also revealed that the expression of miR-30d-5p could significantly inhibit the expression of Beclin-1 and Atg5- by targeting the 3′UTR levels of Beclin-1 and Atg5- and inhibit the ischemia-induced polarization of microglia to M1 (Jiang et al., 2018). All accumulating data suggest that the increase of the M2/M1 ratio in the brain has beneficial anti-inflammatory properties and neuroprotective effects (Gao et al., 2016; Kumar et al., 2016). In addition to changing the polarization of microglia in the brain, many other anti-inflammatory mechanisms have been discovered in recent years. Mechanism studies have shown that miR-146a-5p in exosomes derived from HUCMSCs alleviate neuroinflammation and neurological deficits caused by microglia after IS through IRAK1/TRAF6 (Zhang Z. et al., 2021). Overexpression of IRAK1 and TRAF6 is an important factor in the expression of pro-inflammatory genes and can activate the NFκB-a transcription factor. MiR-146a-5p down-regulates inflammation by binding to the 3′UTR of mRNA encoding the receptor proteins RAK1 and TRAF6. These (IRAK1 and TRAF6) are mainly involved in the toll-like receptor (TLR)-activated pathway, resulting in the expression of pro-inflammatory mediators (Wang et al., 2020). Exosomes-miR-542-3p also attenuates IS-induced apoptosis and ROS and inflammatory response by regulating TLR4 in glial cells (Cai et al., 2021). TLR4 is an important natural immune receptor, which can regulate the inflammatory response of multiple organs (Bruning et al., 2021). MiR-22-3p derived from exosomes of ADMSCs can inhibit inflammatory response and reduce cerebral ischemia injury by promoting macrophage M2 polarization and inhibit inflammation (Fang et al., 2021); inhibiting the activity of caspase-3, increasing the expression of Bcl-2 in neurons (Yu et al., 2015); reducing the expression of NF-κB co-activator NCOA1, and significantly inhibiting the activity of NF-κB (Yu et al., 2015); and reducing the apoptosis rate of cortical neurons (Yu et al., 2015). Exosomes from BMSCs with overexpression of miR-138-5p delay inflammation by inhibiting LCN2, an iron transporter involved in the response to brain injury (Deng et al., 2022). The miR-221-3p targeting ATF3 can significantly decrease the expression of pro-inflammatory cytokines (TNF-α, MCP-1, VCAM-1, IL-6) and chemokines (CCL2, CCL3) and block macrophage infiltration and microglia activation, thus potentially alleviating ischemia induced neuronal injury (Shan et al., 2021). The effects of the various exosome-derived miRNAs are summarized in Table 2.
Brain cells will produce a large amount of ROS during ischemia and hypoxia. Oxidative stress is the sum of many pathological processes, such as mitochondrial damage, calcium overload and complement activation, which is an interactive cycle (Lin et al., 2016; Yin et al., 2016). Therefore, blocking one of the links could reduce neuronal death and neurological dysfunction caused by ischemia and hypoxia. MSCs can transfer its mitochondria to damaged endothelial cells, promote angiogenesis, reduce infarct volume, and improve functional recovery (Liu et al., 2019). In an in vitro model of cerebral ischemia, MSC-exosome-mediated-miR-92b-3p inhibited oxidative stress-induced neuronal damage, although the exact mechanism of action remains unclear (Xu L. et al., 2019). Recent studies have identified that receptor for advanced glycation end products (RAGE) is excessive in hypoxic cells of ischemic brain, exosomes can downregulate RAGE and miR-181a, and significantly reduce cerebral infarction area in an IS model (Kim et al., 2021). Meanwhile, recent studies have also reported that miR-451a can reduce ROS production by inhibiting the total expression of Rac1 and the formation of NOX (Li H. et al., 2021). Exosomes derived from human umbilical vein endothelial cells can reduce oxidative stress by inhibiting mitochondrial RNA-processing endoribonuclease in neurons through distance transfer of miR-206 and miR-1-3p (Zhong and Luo, 2021). However, studies on the potential of MSC-exosomes to block the generation of mitochondrial ROS alone are few. This may be a new research direction for the treatment of IS in the future.
After IS, several brain cells die due to various pathological reactions. MiR-29b-3p in BMSCs-Exos inhibits cell apoptosis by reducing the expression of Bax, cutting caspase3 and up-regulating Bcl-2 (Hou et al., 2020), and promotes neuronal survival by promoting the anti-apoptotic signal cascades (Zhang Y. et al., 2021). MiR-26a-5p in MSC-exosomes inhibits microglial apoptosis by targeting CDK6 (Cheng et al., 2021). Similar to neurons, oligodendrocytes are highly sensitive to hypoxia, oxidative stress, inflammatory mediators, injuries, or injuries induced by infection. MiR-134 in the exosomes derived from BMSCs inhibits the apoptosis of oligodendrocytes by negative regulation of the caspase-8-dependent apoptosis pathway. Caspase8 and camp responsive element binding protein are specific targets of miR134 (Xiao et al., 2018). In addition to inhibiting the traditional apoptotic pathway, the latest research has revealed that circ_0000647 was elevated in the animal model of cerebral ischemia, and miR-126-5p was weakly expressed in cerebral cells under ischemia and hypoxia (Dai et al., 2022). Exosomes-circ_0000647 promotes apoptosis by targeting miR-126-5p to reduce TRAF3 (TRAF3 is the target gene of miR-126-5p) (Dai et al., 2022). The discovery of these new biomarkers may provide a new therapeutic target for IS in addition to contributing to a more detailed understanding of the pathogenesis of IS.
The repair mechanism of damaged tissue requires effective EC regeneration and reinstatement of blood flow in damaged ischemic tissue. In order to improve the repair process, the EC in the innermost layer of the blood vessels will regenerate and proliferate to maintain blood supply in the tissues and tissue homeostasis (Baruah and Wary, 2019) and promote angiogenesis in ischemic areas (Figure 4). Data have revealed that within 21 days after ischemia, new blood vessels form at the edge of the ischemic area and gradually extend to the ischemic center through budding (Li X. et al., 2020). Consequently, enhancing vascular proliferation in ischemic brain tissue has potential therapeutic prospects. Proteomics analysis indicated that exosomes released from MSCs have angiogenic paracrine effects (Ghafouri-Fard et al., 2020). The collateral circulation can protect ischemic brain tissue by increasing the blood supply of ischemic penumbra (Chen W. et al., 2022). Exosomes-CXC receptor type 4 can enhance the regeneration and tube-like structure formation of microvessel endothelial cells (MECs) (Li X. et al., 2020). CXC motif CR4 belongs to the G protein-coupled receptor superfamily and participates in homing of many cells (Shichinohe et al., 2007). CXCL12 promotes angiogenesis in vivo. CXCL12 can bind to CXCR4 on cells expressing CXCR4, including brain MECs. This promotes the migration of brain MECs to ischemic tissues and improves the repair of brain tissue (Liu Q. W. et al., 2022). MiR-29b-3p in exosomes derived from BMSC targets PTEN and activates Akt-signaling pathway to accelerate angiogenesis in IS (Hou et al., 2020). The gene for PTEN, located on human chromosome 19, encodes a double phosphatase that dephosphorylates protein and lipid substrates (Garcia-Junco-Clemente and Golshani, 2014). Moreover, PTEN negatively regulates the phosphatidylinositol 3-kinase/protein kinase B (PI3K/Akt) signaling pathway, thereby accelerating angiogenesis after IS (Qu et al., 2016). MiR-29b-3p negatively regulates PTEN, activates Akt-signaling pathway, and accelerates angiogenesis (Hou et al., 2020). ADMSCs-exosomes (AMSCs-Exos) promote the angiogenesis of BMSCs after IS through the miR-181b-5p/TRPM7 axis. The Mg2+ transmembrane transport channel (TRPM7) is the direct target of miR-181b-5p. MiR-181b-5p up-regulates the expression of HIF-1α and VEGF by targeting TRPM7, and down-regulates the expression of TIMP3 (Yang et al., 2018). Moreover, miR-210 can also promote microvascular angiogenesis, which is consistent with the up-regulation of the vascular endothelial growth factor (VEGF) protein. The miR-210 is the main miRNA induced by hypoxia, which promotes angiogenesis mediated by the VEGF signaling pathway (Lou et al., 2012; Meng et al., 2018).
Figure 4. MiRNAs in exosomes secreted by mesenchymal stem cells promote angiogenesis in ischemic tissue. Meanwhile, CXCL12 secreted by exosomes can bind to CRCX4 and promote the migration of cerebrovascular endothelial cells to ischemic tissue. In addition, miRNAs in exosomes activate PTEN-PIK3-Akt or TRPM7-TIPM3/HIF-1α/VEGF pathways to promote angiogenesis. CXCL12 = SDF-1, Stromal cell derived factor 1; CRCX4, CXC receptor type 4; PTEN, Phosphatase and tensin homolog; PIK3, Phosphatidylinositol 3-kinase; Akt, Protein kinase B; TRPM7, Mg2+ transmembrane transport channel; TIPM3, Tissue inhibitor of metalloproteinase 3; HIF-1α, Hypoxia induce factor-1α; VEGF, Vascular endothelial growth factor.
In the injured central nervous system, glial scars are the main obstacle to axon regeneration, and they consist of extracellular matrix deposition and proliferation of reactive astrocytes (Figure 5). The latter significantly express connective tissue growth factor, which is down-regulated by miR-133 (Xin et al., 2013). The overexpression of miR-133b in exosomes secreted by BMSCs can stimulate the secondary secretion of exosomes from astrocytes and reduce the thickness of glial scars, thus promoting the recovery of neurological function and increasing neural plasticity (Vizoso et al., 2017). MiR-17-92 from BMSCs-Exos enhances axonal myelin remodeling and motor electrophysiological recovery after stroke by activating the PI3K/Akt/mTOR/GSK-3β signaling pathway. The overexpression of miR-17-92 promotes the axon growth of primary cortical neurons by down-regulating PTEN and subsequently activating the PI3K/Akt/mTOR signaling pathway (Xin et al., 2017). MiR-17-92 promotes oligodendrogenesis during neurodevelopment and enhances stroke-induced neurogenesis (Xin et al., 2021). Axonal regeneration after spinal cord injury can also be regulated by the PTEN/PI3K/mTOR signaling pathway (Hu et al., 2022). GSK-3β plays a key role in axon regeneration. Inactivation of GSK-3β promotes axonal growth and neurological recovery in the central nervous system (Arciniegas Ruiz and Eldar-Finkelman, 2021; Rodriguez-Jimenez et al., 2021). MiR-22-3p derived from adipose-tissue MSC exosomes can alleviate cerebral ischemia injury by inhibiting the BMP2/BMF axis mediated by kdm6b. KBMP2 is a neurotrophic factor that can induce the growth of dopaminergic neurons in the midbrain in vivo and in vitro and play a neurotrophic role (Zhang Y. et al., 2021).
Figure 5. MiRNAs in exosomes secreted by mesenchymal stem cells stimulate cell and structure growth. miRNA-133b can down-regulate the expression of CTGF in astrocytes, reduce the formation of glial scar and promote the remodeling of myelin sheath. miRNA-17-92 can down-regulate the expression of PTEN, thereby activating the PIK3-Akt pathway and inactivating GSK-3β, promoting the growth of neuronal axons. miRNA-124 promotes neurogenesis in SVZ and striatum regions. miRNA-22-3p can inhibit KDM6B-mediated KBMP2/BMP pathway and play a neurotrophic role. CTGF, Connective tissue growth factor; PTEN, Phosphatase and tensin homolog; PIK3, Phosphatidylinositol 3-kinase; Akt, Protein kinase B; GSK-3β, Glucogen synthase kinase-3β; SVZ, Subventricular zone; KDM6B, Lysine(K)-specific demethylase 6B; KBMP2, Neurotrophic factor; BMP, Bone morphogenetic protein.
After cerebral ischemia, the expression of miR-124 in the ischemic penumbra increases (Yang et al., 2017). MiR-124 is the most abundant miRNA in the brain and a determinant of the fate of subventricular zone (SVZ) neurons. It mediates stroke-induced neurogenesis in adult SVZ and striatum. The ectopic expression of miR-124 in the cortex promotes the differentiation of neural progenitor cells into neuronal lines, and further improves the anti-ischemic ability through cortical neurogenesis (Zhou and Qi, 2021). Recent studies have reported that CircOGDH (cyclic RNA derived from ketoglutarate dehydrogenase) is highly expressed in plasma exosomes of patients with IS, and CircOGDH interacts with miR-5112 in primary cortical neurons to increase neuronal damage (Liu Y. et al., 2022). By contrast, knockdown of CircOGDH significantly enhanced neuronal viability under ischemic conditions. Therefore, CircOGDH and miR-5112 may be potential therapeutic targets for regulating the viability of ischemic neurons (Liu Y. et al., 2022).
In summary, MSC-exosomes are mostly derived from BMSCs and ADMSCs, while HUCMSCs are used less frequently. However, the experimental results revealed that HUCMSCs were easier to obtain than BMSCs, demonstrated higher viability, were more easily accepted by patients, and were less susceptible to immune-mediated graft rejection (Shang et al., 2021). Additionally, although HUCMSCs are considered more primitive than BMSCs, they have not demonstrated teratoma induction, although they demonstrated immunoregulatory ability. In the clinical research setting, HUCMSCs have the advantage of preventing pain during bone marrow extraction, thus improving volunteer compliance (Eiro et al., 2021). The increasing number of umbilical cord blood preserved in new-borns also suggests an increased possibility for future clinical implementation of this method.
MSCs have paracrine and immune regulation functions, which can change the microenvironment of damaged brain tissue and transforms it to a more regenerative and less inflammatory environment. MSCs can promote cell migration, angiogenesis, immune regulation, nerve protection, and nerve circuit reconstruction (Law et al., 2021). Moreover, many preclinical research evidences have demonstrated that MSCs promote the clinical transformation of MSCs in the treatment of IS. Recent randomized controlled trials (RCTs) and meta-analysis have suggested that stem cell-based therapy can improve neurological deficits and activities of daily living in patients with IS, although its benefits are still limited (Li Z. et al., 2020). Moreover, intravenous injection of MSCs has been proven to be safe, well tolerated, and relatively feasible. However, several common limitations exist for current RCTs, such as small sample size (Law et al., 2021), long-term waiting for MSC culture, delayed assessment of the treatment group, age of participants, randomization time, heterogeneity of ischemic brain injury site, and severity (Chung et al., 2021). Therefore, further randomized, double-blind, large-scale clinical trials are necessary to evaluate the long-term efficacy and safety of this treatment method.
IS is the leading cause of global morbidity and mortality. Exosomes act as natural carriers of biologically active molecules such as bioactive molecules, various proteins, and coding and non-coding RNAs and can transmit information between cells and tissues while lacking immunogenicity (Zhou et al., 2022). Exosome-MiRNA regulates transcriptional and post-transcriptional gene expression and modulates various tissue repair, inflammation, hypoxia, and angiogenesis pathways (Lee et al., 2021). The most important effect is on the tissue microenvironment by mediating various physiological/pathological pathways. A typical example is the repair of ischemic brain injury, which is the focus of this paper. We briefly introduced the potential mechanisms of MSC-exosome-miRNA-mediated nerve function recovery, such as anti-apoptosis, anti-inflammation, nerve plasticity, and angiogenesis. However, the research of MSCs and exosomes in the treatment of IS is still in its infancy in clinical practice. Future clinical trials should be conducted to answer how several MSC-exosomes are obtained, how the survival of most transplanted cells can be increased, how the infarction of most MSC-exosomes can be guided, and how the distribution of MSC-exosomes without any tissue damage is detected (Jiang et al., 2022). Additionally, almost all experiments were conducted in healthy animals. Some highly related complications, such as diabetes, hypertension, hyperlipidemia, and heart disease, may need to be considered because they will affect the formation and treatment of stroke (Li J. Y. et al., 2021). Finally, the extension of exosome half-life and the improvement of the targeting ability should be considered in its application in medicine. At the same time, overcoming drug resistance and expanding the clinical application of conventional drugs are also crucial (Yang et al., 2021). Therefore, stem cells and exosomes may be a promising new treatment in clinical practice, which needs further research.
XH queried the research background. ZP and JS participated in the understanding of exosome development. WH carried out literature reading on the clinical manifestations of ischemic stroke and the advantages and disadvantages of existing treatment. RG and LS carried out language grammar and logic examination of manuscripts. FZ and PS participated in the review of medical professional knowledge of manuscripts. LS and YX conceived the study, participated in its design and coordination and helped draft the manuscript. All authors read and approved the final manuscript.
This work was supported by the Science and Technology Bureau of Quanzhou (grant no. 2020CT003).
The authors declare that the research was conducted in the absence of any commercial or financial relationships that could be construed as a potential conflict of interest.
All claims expressed in this article are solely those of the authors and do not necessarily represent those of their affiliated organizations, or those of the publisher, the editors and the reviewers. Any product that may be evaluated in this article, or claim that may be made by its manufacturer, is not guaranteed or endorsed by the publisher.
We would like to thank Editage (www.editage.cn) for English language editing.
AMSCs, adipose-derived mesenchymal stem cells; Arg-1, arginase-1; Atg5, autophagy-related gene 5; Bax, bcl-associated X protein; BBB, blood brain barrier; Beclin-1, autophagy gene beclin 1; BMF, bone marrowfacror; BMP2, bone morphogenic protein 2; BMSCs, bone marrow-derived mesenchymal stem cells; CCL2/CCL3, chemotactic factor; CH25H, cholesterol-25-hydroxylase; CINC, cytokine-induced neutrophil chemoattractant; CircHIPK3, circular RNA HIPK3; CXCL1/CXCL12, chemotactic factor; CXCR4, CXC motif chemokine receptor type 4; CysLT2R, cysteinyl leukotriene receptor 2; CysLTs, cysteinyl leukotrienes; EC, endothelial cell; ERK1/2, extracellular signal-regulated kinase 1/2; Exos, exosomes; Foxo3a, forkhead box o3a; GRO, growth-related oncogene; GRO-α, growth-related oncogene α; GSK-3β, glucogen synthase kinase-3β; HIF-1α, hypoxia induce factor-1α; HUCMSCs, human umbilical cord-derived mesenchymal stem cells; I/R, ischemia and reperfusion; IL-1, interleukins-1; IL-10, interleukins-10; IL-1β, interleukins-1β; IL-4, interleukins-4; IL-6, interleukins-6; INF-γ, interferon-y; IP, ischemic penumbra; IRAK1, interleukin receptor associated kinase 1; IS, ischemic stroke; KC, keratinocyte-derived chemokine; LTC4/LTD4, leukotriene C4/D4; MCP1, mononuclear chemotaxis protein-1; MSC-EV, mesenchymal stem cells-external vesicles; MSCs, mesenchymal stem cells; mTOR, mammalian target of rapamycin; NFκ B-a, nuclear factor kappa B-a; NOS, nitric oxide synthase; NOX, reduced nicotinamide adenine dinucleotide phosphate oxidase; PETN, phosphatase and tensin homolog; PI3K, phosphatidylinositol 3-kinase; PKB/AKT, protein kinase B; PTEN, phosphatase and tensin homolog; ROS, reactive oxygen species; rt-PA, recombinant tissue plasminogen activator; STAT6, signal transducers and activators of transcription 6; SVZ, subventricular zone; TIMP3, tissue inhibitor of metalloproteinase 3; TLR, toll-like receptor; TLR4, toll-like receptor 4; TNFα, tumor necrosis factor α; TRAF6, tumor necrosis factor receptor-associated factor 6; TRPM7, Mg2+ transmembrane transport channel; VCAM-1, vascular cell adhesion molecule-1; VEGF, vascular endothelial growth factor.
Ai, Z., Cheng, C., Zhou, L., Yin, S., Wang, L., and Liu, Y. (2021). Bone marrow mesenchymal stem cells-derived extracellular vesicles carrying microRNA-221-3p protect against ischemic stroke via ATF3. Brain Res. Bull. 172, 220–228. doi: 10.1016/j.brainresbull.2021.04.022
Anderson, J. D., Johansson, H. J., Graham, C. S., Vesterlund, M., Pham, M. T., Bramlett, C. S., et al. (2016). Comprehensive proteomic analysis of mesenchymal stem cell exosomes reveals modulation of angiogenesis via nuclear factor-kappaB signaling. Stem Cells 34, 601–613. doi: 10.1002/stem.2298
Arciniegas Ruiz, S. M., and Eldar-Finkelman, H. (2021). Glycogen synthase kinase-3 inhibitors: preclinical and clinical focus on CNS-A decade onward. Front. Mol. Neurosci. 14:792364. doi: 10.3389/fnmol.2021.792364
Baruah, J., and Wary, K. K. (2019). Exosomes in the regulation of vascular endothelial cell regeneration. Front. Cell Dev. Biol. 7:353. doi: 10.3389/fcell.2019.00353
Barzegar, M., Stokes, K. Y., Chernyshev, O., Kelley, R. E., and Alexander, J. S. (2021). The Role of the ACE2/MasR axis in ischemic stroke: new insights for therapy. Biomedicines 9:1667. doi: 10.3390/biomedicines9111667
Bruning, E. E., Coller, J. K., Wardill, H. R., and Bowen, J. M. (2021). Site-specific contribution of Toll-like receptor 4 to intestinal homeostasis and inflammatory disease. J. Cell Physiol. 236, 877–888. doi: 10.1002/jcp.29976
Bsat, S., Halaoui, A., Kobeissy, F., Moussalem, C., El Houshiemy, M. N., Kawtharani, S., et al. (2021). Acute ischemic stroke biomarkers: a new era with diagnostic promise? Acute Med. Surg. 8:e696. doi: 10.1002/ams2.696
Cai, G., Cai, G., Zhou, H., Zhuang, Z., Liu, K., Pei, S., et al. (2021). Mesenchymal stem cell-derived exosome miR-542-3p suppresses inflammation and prevents cerebral infarction. Stem Cell Res. Ther. 12:2. doi: 10.1186/s13287-020-02030-w
Castelli, V., Antonucci, I., d’Angelo, M., Tessitore, A., Zelli, V., Benedetti, E., et al. (2021). Neuroprotective effects of human amniotic fluid stem cells-derived secretome in an ischemia/reperfusion model. Stem Cells Transl. Med. 10, 251–266. doi: 10.1002/sctm.20-0268
Castro-Manrreza, M. E., and Montesinos, J. J. (2015). Immunoregulation by mesenchymal stem cells: biological aspects and clinical applications. J. Immunol. Res. 2015, 394917. doi: 10.1155/2015/394917
Chen, H., Xue, R., Huang, P., Wu, Y., Fan, W., He, X., et al. (2022). Modified Exosomes: a Good Transporter for miRNAs within Stem Cells to Treat Ischemic Heart Disease. J. Cardiovasc. Transl. Res. [Epub ahead of print]. doi: 10.1007/s12265-022-10216-1
Chen, W., Huang, W., Yang, Y., and Li, K. (2022). Methylglyoxal Scavengers Attenuate Angiogenesis Dysfunction Induced by Methylglyoxal and Oxygen-Glucose Deprivation. Oxid. Med. Cell. Longev. 2022:8854457. doi: 10.1155/2022/8854457
Chen, K. H., Chen, C. H., Wallace, C. G., Yuen, C. M., Kao, G. S., Chen, Y. L., et al. (2016). Intravenous administration of xenogenic adipose-derived mesenchymal stem cells (ADMSC) and ADMSC-derived exosomes markedly reduced brain infarct volume and preserved neurological function in rat after acute ischemic stroke. Oncotarget 7, 74537–74556. doi: 10.18632/oncotarget.12902
Chen, K. H., Shao, P. L., Li, Y. C., Chiang, J. Y., Sung, P. H., Chien, H. W., et al. (2020). Human umbilical cord-derived mesenchymal stem cell therapy effectively protected the brain architecture and neurological function in rat after acute traumatic brain injury. Cell Transplant. 29:963689720929313. doi: 10.1177/0963689720929313
Chen, Y. T., Chiang, H. J., Chen, C. H., Sung, P. H., Lee, F. Y., Tsai, T. H., et al. (2014). Melatonin treatment further improves adipose-derived mesenchymal stem cell therapy for acute interstitial cystitis in rat. J. Pineal Res. 57, 248–261. doi: 10.1111/jpi.12164
Cheng, C., Chen, X., Wang, Y., Cheng, W., Zuo, X., Tang, W., et al. (2021). MSCsderived exosomes attenuate ischemia-reperfusion brain injury and inhibit microglia apoptosis might via exosomal miR-26a-5p mediated suppression of CDK6. Mol. Med. 27:67. doi: 10.1186/s10020-021-00324-0
Chung, J. W., Chang, W. H., Bang, O. Y., Moon, G. J., Kim, S. J., Kim, S. K., et al. (2021). Efficacy and Safety of Intravenous Mesenchymal Stem Cells for Ischemic Stroke. Neurology 96, e1012–e1023. doi: 10.1212/WNL.0000000000011440
Cosenza, S., Toupet, K., Maumus, M., Luz-Crawford, P., Blanc-Brude, O., Jorgensen, C., et al. (2018). Mesenchymal stem cells-derived exosomes are more immunosuppressive than microparticles in inflammatory arthritis. Theranostics 8, 1399–1410. doi: 10.7150/thno.21072
Coskunpinar, E., Cakmak, H. A., Kalkan, A. K., Tiryakioglu, N. O., Erturk, M., and Ongen, Z. (2016). Circulating miR-221-3p as a novel marker for early prediction of acute myocardial infarction. Gene 591, 90–96. doi: 10.1016/j.gene.2016.06.059
Dai, Y., Sheng, Y., Deng, Y., Wang, H., Zhao, Z., Yu, X., et al. (2022). Circ_0000647 promotes cell injury by modulating miR-126-5p/TRAF3 axis in oxygen-glucose deprivation and reperfusion-induced SK-N-SH cell model. Int. Immunopharmacol. 104:108464. doi: 10.1016/j.intimp.2021.108464
de Freitas, R. C. C., Bortolin, R. H., Lopes, M. B., Tamborlin, L., Meneguello, L., Silbiger, V. N., et al. (2017). Modulation of miR-26a-5p and miR-15b-5p Exosomal Expression Associated with Clopidogrel-Induced Hepatotoxicity in HepG2 Cells. Front. Pharmacol. 8:906. doi: 10.3389/fphar.2017.00906
Deng, Y., Chen, D., Gao, F., Lv, H., Zhang, G., Sun, X., et al. (2019). Exosomes derived from microRNA-138-5p-overexpressing bone marrow-derived mesenchymal stem cells confer neuroprotection to astrocytes following ischemic stroke via inhibition of LCN2. J. Biol. Eng. 13:71. doi: 10.1186/s13036-019-0193-0
Deng, Y., Chen, D., Gao, F., Lv, H., Zhang, G., Sun, X., et al. (2022). Correction to: exosomes derived from microRNA-138-5poverexpressing bone marrow-derived mesenchymal stem cells confer neuroprotection to astrocytes following ischemic stroke via inhibition of LCN2. J. Biol. Eng. 16:8. doi: 10.1186/s13036-022-00285-w
Doyle, L. M., and Wang, M. Z. (2019). Overview of extracellular vesicles, their origin, composition, purpose, and methods for exosome isolation and analysis. Cells 8:727. doi: 10.3390/cells8070727
Eiro, N., Fraile, M., Fernandez-Francos, S., Sanchez, R., Costa, L. A., and Vizoso, F. J. (2021). Importance of the origin of mesenchymal (stem) stromal cells in cancer biology: “alliance” or “war” in intercellular signals. Cell Biosci. 11:109. doi: 10.1186/s13578-021-00620-6
Enzmann, G., Kargaran, S., and Engelhardt, B. (2018). Ischemia-reperfusion injury in stroke: impact of the brain barriers and brain immune privilege on neutrophil function. Ther. Adv. Neurol. Disord. 11:1756286418794184. doi: 10.1177/1756286418794184
Fang, H., Yang, M., Pan, Q., Jin, H. L., Li, H. F., Wang, R. R., et al. (2021). MicroRNA-22-3p alleviates spinal cord ischemia/reperfusion injury by modulating M2 macrophage polarization via IRF5. J. Neurochem. 156, 106–120. doi: 10.1111/jnc.15042
Gao, J., Grill, R. J., Dunn, T. J., Bedi, S., Labastida, J. A., Hetz, R. A., et al. (2016). Human Neural Stem Cell Transplantation-Mediated Alteration of Microglial/Macrophage Phenotypes after Traumatic Brain Injury. Cell Transplant. 25, 1863–1877. doi: 10.3727/096368916X691150
Garcia-Junco-Clemente, P., and Golshani, P. P. T. E. N. (2014). A master regulator of neuronal structure, function, and plasticity. Commun. Integr. Biol. 7:e28358. doi: 10.4161/cib.28358
Garcia-Martin, R., Brandao, B. B., Thomou, T., Altindis, E., and Kahn, C. R. (2022). Tissue differences in the exosomal/small extracellular vesicle proteome and their potential as indicators of altered tissue metabolism. Cell Rep. 38:110277. doi: 10.1016/j.celrep.2021.110277
Ghafouri-Fard, S., Shoorei, H., and Taheri, M. (2020). Non-coding RNAs participate in the ischemia-reperfusion injury. Biomed. Pharmacother. 129:110419. doi: 10.1016/j.biopha.2020.110419
Gurunathan, S., Kang, M. H., Jeyaraj, M., Qasim, M., and Kim, J. H. (2019). Review of the isolation, characterization, biological function, and multifarious therapeutic approaches of exosomes. Cells 8:307. doi: 10.3390/cells8040307
Hassanpour, M., Rezabakhsh, A., Rezaie, J., Nouri, M., and Rahbarghazi, R. (2020). Exosomal cargos modulate autophagy in recipient cells via different signaling pathways. Cell Biosci. 10:92. doi: 10.1186/s13578-020-00455-7
Heneka, M. T., Kummer, M. P., and Latz, E. (2014). Innate immune activation in neurodegenerative disease. Nat. Rev. Immunol. 14, 463–477. doi: 10.1038/nri3705
Hong, S. B., Yang, H., Manaenko, A., Lu, J., Mei, Q., and Hu, Q. (2019). Potential of Exosomes for the Treatment of Stroke. Cell Transplant. 28, 662–670. doi: 10.1177/0963689718816990
Hou, K., Li, G., Zhao, J., Xu, B., Zhang, Y., Yu, J., et al. (2020). Bone mesenchymal stem cell-derived exosomal microRNA-29b-3p prevents hypoxic-ischemic injury in rat brain by activating the PTEN-mediated Akt signaling pathway. J. Neuroinflammation 17:46.
Hu, X., Li, P., Guo, Y., Wang, H., Leak, R. K., Chen, S., et al. (2012). Microglia/macrophage polarization dynamics reveal novel mechanism of injury expansion after focal cerebral ischemia. Stroke 43, 3063–3070. doi: 10.1161/STROKEAHA.112.659656
Hu, X., Pan, J., Li, Y., Jiang, Y., Zheng, H., Shi, R., et al. (2022). Extracellular vesicles from adipose-derived stem cells promote microglia M2 polarization and neurological recovery in a mouse model of transient middle cerebral artery occlusion. Stem Cell Res. Ther. 13:21. doi: 10.1186/s13287-021-02668-0
Hu, Y., Rao, S. S., Wang, Z. X., Cao, J., Tan, Y. J., Luo, J., et al. (2018). Exosomes from human umbilical cord blood accelerate cutaneous wound healing through miR-21-3p-mediated promotion of angiogenesis and fibroblast function. Theranostics 8, 169–184. doi: 10.7150/thno.21234
Hurd, M. D., Goel, I., Sakai, Y., and Teramura, Y. (2021). Current status of ischemic stroke treatment: from thrombolysis to potential regenerative medicine. Regen. Ther. 18, 408–417. doi: 10.1016/j.reth.2021.09.009
Huuskonen, M. T., Wang, Y., Nikolakopoulou, A. M., Montagne, A., Dai, Z., Lazic, D., et al. (2022). Protection of ischemic white matter and oligodendrocytes in mice by 3K3A-activated protein C. J. Exp. Med. 219:e20211372. doi: 10.1084/jem.20211372
Jiang, L., Chen, W., Ye, J., and Wang, Y. (2022). Potential role of exosomes in ischemic stroke treatment. Biomolecules 12:115. doi: 10.3390/biom12010115
Jiang, M., Wang, H., Jin, M., Yang, X., Ji, H., Jiang, Y., et al. (2018). Exosomes from MiR-30d-5p-ADSCs reverse acute ischemic stroke-induced, autophagy-mediated brain injury by promoting M2 microglial/macrophage polarization. Cell Physiol. Biochem. 47, 864–878. doi: 10.1159/000490078
Jiang, Y., Zhang, H., Li, W., Yan, Y., Yao, X., and Gu, W. (2021). LINC01426 contributes to clear cell renal cell carcinoma progression by modulating CTBP1/miR-423-5p/FOXM1 axis via interacting with IGF2BP1. J. Cell Physiol. 236, 427–439. doi: 10.1002/jcp.29871
Kabekkodu, S. P., Shukla, V., Varghese, V. K., D’ Souza, J., Chakrabarty, S., and Satyamoorthy, K. (2018). Clustered miRNAs and their role in biological functions and diseases. Biol. Rev. Camb. Philos. Soc. 93, 1955–1986. doi: 10.1111/brv.12428
Kalani, A., and Tyagi, N. (2015). Exosomes in neurological disease, neuroprotection, repair and therapeutics: problems and perspectives. Neural Regen. Res. 10, 1565–1567. doi: 10.4103/1673-5374.165305
Kalogeris, T., Baines, C. P., Krenz, M., and Korthuis, R. J. (2016). Ischemia/Reperfusion. Compr. Physiol. 7, 113–170.
Khan, M., Nickoloff, E., Abramova, T., Johnson, J., Verma, S. K., Krishnamurthy, P., et al. (2015). Embryonic stem cell-derived exosomes promote endogenous repair mechanisms and enhance cardiac function following myocardial infarction. Circ. Res. 117, 52–64. doi: 10.1161/CIRCRESAHA.117.305990
Kim, H. Y., Kim, T. J., Kang, L., Kim, Y. J., Kang, M. K., Kim, J., et al. (2020). Mesenchymal stem cell-derived magnetic extracellular nanovesicles for targeting and treatment of ischemic stroke. Biomaterials 243:119942. doi: 10.1016/j.biomaterials.2020.119942
Kim, M., Lee, Y., and Lee, M. (2021). Hypoxia-specific anti-RAGE exosomes for nose-to-brain delivery of anti-miR-181a oligonucleotide in an ischemic stroke model. Nanoscale 13, 14166–14178. doi: 10.1039/d0nr07516g
Kumar, A., Barrett, J. P., Alvarez-Croda, D. M., Stoica, B. A., Faden, A. I., and Loane, D. J. (2016). NOX2 drives M1-like microglial/macrophage activation and neurodegeneration following experimental traumatic brain injury. Brain Behav. Immun. 58, 291–309. doi: 10.1016/j.bbi.2016.07.158
Law, Z. K., Tan, H. J., Chin, S. P., Wong, C. Y., Wan Yahya, W. N. N., Muda, A. S., et al. (2021). The effects of intravenous infusion of autologous mesenchymal stromal cells in patients with subacute middle cerebral artery infarct: a phase 2 randomized controlled trial on safety, tolerability and efficacy. Cytotherapy 23, 833–840. doi: 10.1016/j.jcyt.2021.03.005
Lee, J. Y., Chung, J., Byun, Y., Kim, K. H., An, S. H., and Kwon, K. (2021). Mesenchymal stem cell-derived small extracellular vesicles protect cardiomyocytes from doxorubicin-induced cardiomyopathy by upregulating survivin expression via the miR-199a-3p-Akt-Sp1/p53 signaling pathway. Int. J. Mol. Sci. 22:7102. doi: 10.3390/ijms22137102
Li, C. Y., Wu, X. Y., Tong, J. B., Yang, X. X., Zhao, J. L., Zheng, Q. F., et al. (2015). Comparative analysis of human mesenchymal stem cells from bone marrow and adipose tissue under xeno-free conditions for cell therapy. Stem Cell Res. Ther. 6:55. doi: 10.1186/s13287-015-0066-5
Li, G., Xiao, L., Qin, H., Zhuang, Q., Zhang, W., Liu, L., et al. (2020). Exosomes-carried microRNA-26b-5p regulates microglia M1 polarization after cerebral ischemia/reperfusion. Cell Cycle 19, 1022–1035. doi: 10.1080/15384101.2020.1743912
Li, H., Luo, Y., Liu, P., Liu, P., Hua, W., Zhang, Y., et al. (2021). Exosomes containing miR-451a is involved in the protective effect of cerebral ischemic preconditioning against cerebral ischemia and reperfusion injury. CNS Neurosci. Ther. 27, 564–576. doi: 10.1111/cns.13612
Li, J. Y., Li, Q. Q., and Sheng, R. (2021). The role and therapeutic potential of exosomes in ischemic stroke. Neurochem. Int. 151:105194. doi: 10.1016/j.neuint.2021.105194
Lin, L., Wang, X., and Yu, Z. (2016). Ischemia-reperfusion injury in the brain: mechanisms and potential therapeutic strategies. Biochem. Pharmacol. 5:213. doi: 10.4172/2167-0501.1000213
Liu, F., Lu, J., Manaenko, A., Tang, J., and Hu, Q. (2018). Mitochondria in ischemic stroke: new insight and implications. Aging Dis. 9, 924–937. doi: 10.14336/AD.2017.1126
Liu, K., Guo, L., Zhou, Z., Pan, M., and Yan, C. (2019). Mesenchymal stem cells transfer mitochondria into cerebral microvasculature and promote recovery from ischemic stroke. Microvasc. Res. 123, 74–80. doi: 10.1016/j.mvr.2019.01.001
Li, Z., Dong, X., Tian, M., Liu, C., Wang, K., Li, L., et al. (2020). Stem cell-based therapies for ischemic stroke: a systematic review and meta-analysis of clinical trials. Stem Cell Res. Ther. 11:252. doi: 10.1186/s13287-020-01762-z
Liu, Q. W., He, Y., and Xu, W. W. (2022). Molecular functions and therapeutic applications of exosomal noncoding RNAs in cancer. Exp. Mol. Med. 54, 216–225. doi: 10.1038/s12276-022-00744-w
Liu, Y., Li, Y., Zang, J., Zhang, T., Li, Y., Tan, Z., et al. (2022). CircOGDH Is a Penumbra biomarker and therapeutic target in acute ischemic stroke. Circ. Res. 130, 907–924. doi: 10.1161/CIRCRESAHA.121.319412
Liu, Y., Yeh, P. K., Lin, Y. P., and Sung, Y. F. (2021). Steroid-Responsive gradenigo’s syndrome mimicking subdural hematoma. Cureus 13:e19547. doi: 10.7759/cureus.19547
Lou, Y. L., Guo, F., Liu, F., Gao, F. L., Zhang, P. Q., Niu, X., et al. (2012). miR-210 activates notch signaling pathway in angiogenesis induced by cerebral ischemia. Mol. Cell Biochem. 370, 45–51. doi: 10.1007/s11010-012-1396-6
Luo, X., Wang, W., Li, D., Xu, C., Liao, B., Li, F., et al. (2019). Plasma Exosomal miR-450b-5p as a possible biomarker and therapeutic target for transient ischaemic attacks in rats. J. Mol. Neurosci. 69, 516–526. doi: 10.1007/s12031-019-01341-9
Mahjoubin-Tehran, M., Rezaei, S., Jesmani, A., Birang, N., Morshedi, K., Khanbabaei, H., et al. (2021). New epigenetic players in stroke pathogenesis: from non-coding RNAs to exosomal non-coding RNAs. Biomed. Pharmacother. 140:111753. doi: 10.1016/j.biopha.2021.111753
Mathew, B., Ravindran, S., Liu, X., Torres, L., Chennakesavalu, M., Huang, C. C., et al. (2019). Mesenchymal stem cell-derived extracellular vesicles and retinal ischemia-reperfusion. Biomaterials 197, 146–160. doi: 10.1016/j.biomaterials.2019.01.016
Meng, S., Hu, Y., Zhu, J., Feng, T., and Quan, X. (2021). miR-30c-5p acts as a therapeutic target for ameliorating myocardial ischemia-reperfusion injury. Am. J. Transl. Res. 13, 2198–2212.
Meng, Z. Y., Kang, H. L., Duan, W., Zheng, J., Li, Q. N., and Zhou, Z. J. (2018). MicroRNA-210 promotes accumulation of neural precursor cells around ischemic foci after cerebral ischemia by regulating the SOCS1-STAT3-VEGF-C Pathway. J. Am. Heart Assoc. 7:e005052. doi: 10.1161/JAHA.116.005052
Minnerup, J., Wersching, H., Teuber, A., Wellmann, J., Eyding, J., Weber, R., et al. (2016). Outcome after thrombectomy and intravenous thrombolysis in patients with acute ischemic stroke: a prospective observational study. Stroke 47, 1584–1592. doi: 10.1161/STROKEAHA.116.012619
Morales, L., Oliveros, J. C., Enjuanes, L., and Sola, I. (2022). Contribution of Host miRNA-223-3p to SARS-CoV-Induced Lung Inflammatory Pathology. mBio 13:e0313521. doi: 10.1128/mbio.03135-21
Qu, L., Gao, Y., Sun, H., Wang, H., Liu, X., and Sun, D. (2016). Role of PTEN-Akt-CREB signaling pathway in nervous system impairment of rats with chronic arsenite exposure. Biol. Trace Elem. Res. 170, 366–372. doi: 10.1007/s12011-015-0478-1
Quaglia, M., Merlotti, G., Colombatto, A., Bruno, S., Stasi, A., Franzin, R., et al. (2022). Stem cell-derived extracellular vesicles as potential therapeutic approach for acute kidney injury. Front. Immunol. 13:849891. doi: 10.3389/fimmu.2022.849891
Rezaie, J., Aslan, C., Ahmadi, M., Zolbanin, N. M., Kashanchi, F., and Jafari, R. (2021). The versatile role of exosomes in human retroviral infections: from immunopathogenesis to clinical application. Cell Biosci. 11:19. doi: 10.1186/s13578-021-00537-0
Rodriguez-Jimenez, F. J., Vilches, A., Perez-Arago, M. A., Clemente, E., Roman, R., Leal, J., et al. (2021). Activation of Neurogenesis in Multipotent Stem Cells Cultured In Vitro and in the Spinal Cord Tissue After Severe Injury by Inhibition of Glycogen Synthase Kinase-3. Neurotherapeutics 18, 515–533. doi: 10.1007/s13311-020-00928-0
Salikhova, D., Bukharova, T., Cherkashova, E., Namestnikova, D., Leonov, G., Nikitina, M., et al. (2021). Therapeutic Effects of hiPSC-Derived glial and neuronal progenitor cells-conditioned medium in experimental ischemic stroke in rats. Int. J. Mol. Sci. 22:4694. doi: 10.3390/ijms22094694
Shan, G., Gu, J., Zhou, D., Li, L., Cheng, W., Wang, Y., et al. (2020). Cancer-associated fibroblast-secreted exosomal miR-423-5p promotes chemotherapy resistance in prostate cancer by targeting GREM2 through the TGF-beta signaling pathway. Exp. Mol. Med. 52, 1809–1822. doi: 10.1038/s12276-020-0431-z
Shan, Y., Hu, J., Lv, H., Cui, X., and Di, W. (2021). miR-221 exerts neuroprotective effects in ischemic stroke by inhibiting the proinflammatory response. J. Stroke Cerebrovasc. Dis. 30:105489. doi: 10.1016/j.jstrokecerebrovasdis.2020.105489
Shang, Y., Guan, H., and Zhou, F. (2021). Biological Characteristics of Umbilical Cord Mesenchymal Stem Cells and Its Therapeutic Potential for Hematological Disorders. Front. Cell Dev. Biol. 9:570179. doi: 10.3389/fcell.2021.570179
Shang, Z., Wang, R., Li, D., Chen, J., Zhang, B., Wang, M., et al. (2022). Spinal Cord Injury: A Systematic Review and Network Meta-Analysis of Therapeutic Strategies Based on 15 Types of Stem Cells in Animal Models. Front. Pharmacol. 13:819861. doi: 10.3389/fphar.2022.819861
Shi, B., Wang, Y., Zhao, R., Long, X., Deng, W., and Wang, Z. (2018). Bone marrow mesenchymal stem cell-derived exosomal miR-21 protects C-kit+ cardiac stem cells from oxidative injury through the PTEN/PI3K/Akt axis. PLoS One 13:e0191616. doi: 10.1371/journal.pone.0191616
Shi, H., Tang, H., Ai, W., Zeng, Q., Yang, H., Zhu, F., et al. (2021). Schisandrin B Antagonizes Cardiotoxicity Induced by Pirarubicin by Inhibiting Mitochondrial Permeability Transition Pore (mPTP) Opening and Decreasing Cardiomyocyte Apoptosis. Front. Pharmacol. 12:733805. doi: 10.3389/fphar.2021.733805
Shichinohe, H., Kuroda, S., Yano, S., Hida, K., and Iwasaki, Y. (2007). Role of SDF-1/CXCR4 system in survival and migration of bone marrow stromal cells after transplantation into mice cerebral infarct. Brain Res. 1183, 138–147. doi: 10.1016/j.brainres.2007.08.091
Soifer, H. S., Rossi, J. J., and Saetrom, P. (2007). MicroRNAs in disease and potential therapeutic applications. Mol. Ther. 15, 2070–2079. doi: 10.1038/sj.mt.6300311
Song, Y., Li, Z., He, T., Qu, M., Jiang, L., Li, W., et al. (2019). M2 microglia-derived exosomes protect the mouse brain from ischemia-reperfusion injury via exosomal miR-124. Theranostics 9, 2910–2923. doi: 10.7150/thno.30879
Souza, D. L., Korzenowski, A. L., Alvarado, M. M., Sperafico, J. H., Ackermann, A. E. F., Mareth, T., et al. (2021). A Systematic Review on Lean Applications’ in Emergency Departments. Healthcare 9:763. doi: 10.3390/healthcare9060763
Spellicy, S. E., and Stice, S. L. (2021). Tissue and Stem Cell Sourced Extracellular Vesicle Communications with Microglia. Stem Cell Rev. Rep. 17, 357–368. doi: 10.1007/s12015-020-10011-y
Stonesifer, C., Corey, S., Ghanekar, S., Diamandis, Z., Acosta, S. A., and Borlongan, C. V. (2017). Stem cell therapy for abrogating stroke-induced neuroinflammation and relevant secondary cell death mechanisms. Prog. Neurobiol. 158, 94–131. doi: 10.1016/j.pneurobio.2017.07.004
Strain, W. D., Elyas, S., Wedge, N., Mounce, L., Henley, W., James, M., et al. (2021). Evaluation of microalbuminuria as a prognostic indicator after a TIA or minor stroke in an outpatient setting: the prognostic role of microalbuminuria in TIA evolution (ProMOTE) study. BMJ Open 11:e043253. doi: 10.1136/bmjopen-2020-043253
Sun, L., Zhu, W., Zhao, P., Zhang, J., Lu, Y., Zhu, Y., et al. (2020). Down-Regulated Exosomal MicroRNA-221 - 3p Derived From Senescent Mesenchymal Stem Cells Impairs Heart Repair. Front. Cell Dev. Biol. 8:263. doi: 10.3389/fcell.2020.00263
Sun, Y., Gui, H., Li, Q., Luo, Z. M., Zheng, M. J., Duan, J. L., et al. (2013). MicroRNA-124 protects neurons against apoptosis in cerebral ischemic stroke. CNS Neurosci. Ther. 19, 813–819. doi: 10.1111/cns.12142
Surinkaew, P., Sawaddiruk, P., Apaijai, N., Chattipakorn, N., and Chattipakorn, S. C. (2018). Role of microglia under cardiac and cerebral ischemia/reperfusion (I/R) injury. Metab. Brain Dis. 33, 1019–1030. doi: 10.1007/s11011-018-0232-4
Tan, H., Song, Y., Chen, J., Zhang, N., Wang, Q., Li, Q., et al. (2021). Platelet-Like Fusogenic Liposome-Mediated Targeting Delivery of miR-21 Improves Myocardial Remodeling by Reprogramming Macrophages Post Myocardial Ischemia-Reperfusion Injury. Adv. Sci. 8:e2100787. doi: 10.1002/advs.202100787
Tang, X. L., Wysoczynski, M., Gumpert, A. M., Li, Y., Wu, W. J., Li, H., et al. (2022). Effect of intravenous cell therapy in rats with old myocardial infarction. Mol. Cell Biochem. 477, 431–444. doi: 10.1007/s11010-021-04283-2
Tian, R., Guan, X., Qian, H., Wang, L., Shen, Z., Fang, L., et al. (2021). Restoration of NRF2 attenuates myocardial ischemia reperfusion injury through mediating microRNA-29a-3p/CCNT2 axis. Biofactors 47, 414–426. doi: 10.1002/biof.1712
Vizoso, F. J., Eiro, N., Cid, S., Schneider, J., and Perez-Fernandez, R. (2017). Mesenchymal Stem Cell Secretome: Toward Cell-Free Therapeutic Strategies in Regenerative Medicine. Int. J. Mol. Sci. 18:1852. doi: 10.3390/ijms18091852
Wang, Y., Zhang, S., Li, H., Wang, H., Zhang, T., Hutchinson, M. R., et al. (2020). Small-Molecule modulators of toll-like receptors. Acc. Chem. Res. 53, 1046–1055. doi: 10.1021/acs.accounts.9b00631
Wang, Y., Zhao, R., Liu, D., Deng, W., Xu, G., Liu, W., et al. (2018). Exosomes Derived from miR-214-Enriched Bone Marrow-derived mesenchymal stem cells regulate oxidative damage in cardiac stem cells by targeting CaMKII. Oxid. Med. Cell Longev. 2018:4971261. doi: 10.1155/2018/4971261
Wei, Z., Qiao, S., Zhao, J., Liu, Y., Li, Q., Wei, Z., et al. (2019). miRNA-181a over-expression in mesenchymal stem cell-derived exosomes influenced inflammatory response after myocardial ischemia-reperfusion injury. Life Sci. 232:116632. doi: 10.1016/j.lfs.2019.116632
Xiao, Y., Geng, F., Wang, G., Li, X., Zhu, J., and Zhu, W. (2018). Bone marrow-derived mesenchymal stem cells-derived exosomes prevent oligodendrocyte apoptosis through exosomal miR-134 by targeting caspase-8. J. Cell Biochem. [Epub ahead of print]. doi: 10.1002/jcb.27519
Xin, H., Katakowski, M., Wang, F., Qian, J. Y., Liu, X. S., Ali, M. M., et al. (2017). MicroRNA cluster miR-17-92 Cluster in Exosomes Enhance Neuroplasticity and Functional Recovery After Stroke in Rats. Stroke 48, 747–753. doi: 10.1161/STROKEAHA.116.015204
Xin, H., Li, Y., and Chopp, M. (2014). Exosomes/miRNAs as mediating cell-based therapy of stroke. Front. Cell Neurosci. 8:377. doi: 10.3389/fncel.2014.00377
Xin, H., Li, Y., Liu, Z., Wang, X., Shang, X., Cui, Y., et al. (2013). MiR-133b promotes neural plasticity and functional recovery after treatment of stroke with multipotent mesenchymal stromal cells in rats via transfer of exosome-enriched extracellular particles. Stem Cells 31, 2737–2746. doi: 10.1002/stem.1409
Xin, H., Liu, Z., Buller, B., Li, Y., Golembieski, W., Gan, X., et al. (2021). MiR-17-92 enriched exosomes derived from multipotent mesenchymal stromal cells enhance axon-myelin remodeling and motor electrophysiological recovery after stroke. J. Cereb. Blood Flow Metab. 41, 1131–1144. doi: 10.1177/0271678X20950489
Xiong, X. Y., Liu, L., and Yang, Q. W. (2016). Functions and mechanisms of microglia/macrophages in neuroinflammation and neurogenesis after stroke. Prog. Neurobiol. 142, 23–44. doi: 10.1016/j.pneurobio.2016.05.001
Xu, F., Xiang, Q., Huang, J., Chen, Q., Yu, N., Long, X., et al. (2019). Exosomal miR-423-5p mediates the proangiogenic activity of human adipose-derived stem cells by targeting Sufu. Stem Cell Res. Ther. 10:106. doi: 10.1186/s13287-019-1196-y
Xu, L., Cao, H., Xie, Y., Zhang, Y., Du, M., Xu, X., et al. (2019). Exosome-shuttled miR-92b-3p from ischemic preconditioned astrocytes protects neurons against oxygen and glucose deprivation. Brain Res. 1717, 66–73. doi: 10.1016/j.brainres.2019.04.009
Li, X., Zhang, Y., Wang, Y., Zhao, D., Sun, C., Zhou, S., et al. (2020). Exosomes derived from CXCR4-Overexpressing BMSC promoted activation of microvascular endothelial cells in cerebral ischemia/reperfusion injury. Neural Plast. 2020:8814239. doi: 10.1155/2020/8814239
Xue, P., Huang, S., Han, X., Zhang, C., Yang, L., Xiao, W., et al. (2022). Exosomal miR-101-3p and miR-423-5p inhibit medulloblastoma tumorigenesis through targeting FOXP4 and EZH2. Cell Death Differ. 29, 82–95. doi: 10.1038/s41418-021-00838-4
Yan, B., Zhang, Y., Liang, C., Liu, B., Ding, F., Wang, Y., et al. (2020). Stem cell-derived exosomes prevent pyroptosis and repair ischemic muscle injury through a novel exosome/circHIPK3/FOXO3a pathway. Theranostics 10, 6728–6742. doi: 10.7150/thno.42259
Yang, J., Zhang, X., Chen, X., Wang, L., and Yang, G. (2017). Exosome Mediated Delivery of miR-124 Promotes Neurogenesis after Ischemia. Mol. Ther. Nucleic Acids 7, 278–287. doi: 10.1016/j.omtn.2017.04.010
Yang, L., Qian, J., Yang, B., He, Q., Wang, J., and Weng, Q. (2021). Challenges and Improvements of Novel Therapies for Ischemic Stroke. Front. Pharmacol. 12:721156. doi: 10.3389/fphar.2021.721156
Yang, P., Zhang, Y., Zhang, L., Zhang, Y., Treurniet, K. M., Chen, W., et al. (2020). Endovascular Thrombectomy with or without Intravenous Alteplase in Acute Stroke. N. Engl. J. Med. 382, 1981–1993.
Yang, Y., Cai, Y., Zhang, Y., Liu, J., and Xu, Z. (2018). Exosomes secreted by adipose-derived stem cells contribute to angiogenesis of brain microvascular endothelial cells following oxygen-glucose deprivation in vitro through MicroRNA-181b/TRPM7 Axis. J. Mol. Neurosci. 65, 74–83. doi: 10.1007/s12031-018-1071-9
Yin, C., Liang, Y., Zhang, J., Ruan, G., Li, Z., Pang, R., et al. (2016). Umbilical cord-derived mesenchymal stem cells relieve hindlimb ischemia through enhancing angiogenesis in tree shrews. Stem Cells Int. 2016:9742034. doi: 10.1155/2016/9742034
Yoo, J., Kim, H. S., and Hwang, D. Y. (2013). Stem cells as promising therapeutic options for neurological disorders. J. Cell Biochem. 114, 743–753. doi: 10.1002/jcb.24427
Yu, H., Wu, M., Zhao, P., Huang, Y., Wang, W., and Yin, W. (2015). Neuroprotective effects of viral overexpression of microRNA-22 in rat and cell models of cerebral ischemia-reperfusion injury. J. Cell Biochem. 116, 233–241. doi: 10.1002/jcb.24960
Zhang, L., Xia, R., Jia, J., Wang, L., Li, K., Li, Y., et al. (2018). Oleanolic acid protects against cognitive decline and neuroinflammation-mediated neurotoxicity by blocking secretory phospholipase A2 IIA-activated calcium signals. Mol. Immunol. 99, 95–103. doi: 10.1016/j.molimm.2018.04.015
Zhang, Y., Hao, Z., Wang, P., Xia, Y., Wu, J., Xia, D., et al. (2019). Exosomes from human umbilical cord mesenchymal stem cells enhance fracture healing through HIF-1alpha-mediated promotion of angiogenesis in a rat model of stabilized fracture. Cell Prolif. 52:e12570. doi: 10.1111/cpr.12570
Zhang, Y., Liu, J., Su, M., Wang, X., and Xie, C. (2021). Exosomal microRNA-22-3p alleviates cerebral ischemic injury by modulating KDM6B/BMP2/BMF axis. Stem Cell Res. Ther. 12:111. doi: 10.1186/s13287-020-02091-x
Zhang, Z., Zou, X., Zhang, R., Xie, Y., Feng, Z., Li, F., et al. (2021). Human umbilical cord mesenchymal stem cell-derived exosomal miR-146a-5p reduces microglial-mediated neuroinflammation via suppression of the IRAK1/TRAF6 signaling pathway after ischemic stroke. Aging 13, 3060–3079. doi: 10.18632/aging.202466
Zhao, Y., Gan, Y., Xu, G., Yin, G., and Liu, D. (2020b). MSCs-Derived Exosomes Attenuate Acute Brain Injury and Inhibit Microglial Inflammation by Reversing CysLT2R-ERK1/2 Mediated Microglia M1 Polarization. Neurochem. Res. 45, 1180–1190. doi: 10.1007/s11064-020-02998-0
Zhao, Y., Gan, Y., Xu, G., Hua, K., and Liu, D. (2020a). Exosomes from MSCs overexpressing microRNA-223-3p attenuate cerebral ischemia through inhibiting microglial M1 polarization mediated inflammation. Life Sci. 260:118403. doi: 10.1016/j.lfs.2020.118403
Zhong, Y., and Luo, L. (2021). Exosomes from Human Umbilical Vein Endothelial Cells Ameliorate Ischemic Injuries by Suppressing the RNA Component of Mitochondrial RNA-processing Endoribonuclease via the Induction of miR-206/miR-1-3p Levels. Neuroscience 476, 34–44.
Zhou, L., Kodidela, S., Godse, S., Thomas-Gooch, S., Kumar, A., Raji, B., et al. (2022). Targeted drug delivery to the central nervous system using extracellular vesicles. Pharmaceuticals 15:358. doi: 10.3390/ph15030358
Keywords: exosomes, ischemic stroke, miRNAs, mesenchymal stem cells, treatment
Citation: Xiong Y, Song J, Huang X, Pan Z, Goldbrunner R, Stavrinou L, Lin S, Hu W, Zheng F and Stavrinou P (2022) Exosomes Derived From Mesenchymal Stem Cells: Novel Effects in the Treatment of Ischemic Stroke. Front. Neurosci. 16:899887. doi: 10.3389/fnins.2022.899887
Received: 19 March 2022; Accepted: 13 April 2022;
Published: 02 May 2022.
Edited by:
Elena Rybnikova, Pavlov Institute of Physiology (RAS), RussiaReviewed by:
Jafar Rezaie, Urmia University of Medical Sciences, IranCopyright © 2022 Xiong, Song, Huang, Pan, Goldbrunner, Stavrinou, Lin, Hu, Zheng and Stavrinou. This is an open-access article distributed under the terms of the Creative Commons Attribution License (CC BY). The use, distribution or reproduction in other forums is permitted, provided the original author(s) and the copyright owner(s) are credited and that the original publication in this journal is cited, in accordance with accepted academic practice. No use, distribution or reproduction is permitted which does not comply with these terms.
*Correspondence: Shu Lin, c2h1bGluMTk1NkAxMjYuY29t; Weipeng Hu, bmV1cm9zdXJnZXJ5X2Z5ZXlAMTYzLmNvbQ==; Feng Zheng, ZHIuZmVuZy56aGVuZ0BnbWFpbC5jb20=
Disclaimer: All claims expressed in this article are solely those of the authors and do not necessarily represent those of their affiliated organizations, or those of the publisher, the editors and the reviewers. Any product that may be evaluated in this article or claim that may be made by its manufacturer is not guaranteed or endorsed by the publisher.
Research integrity at Frontiers
Learn more about the work of our research integrity team to safeguard the quality of each article we publish.