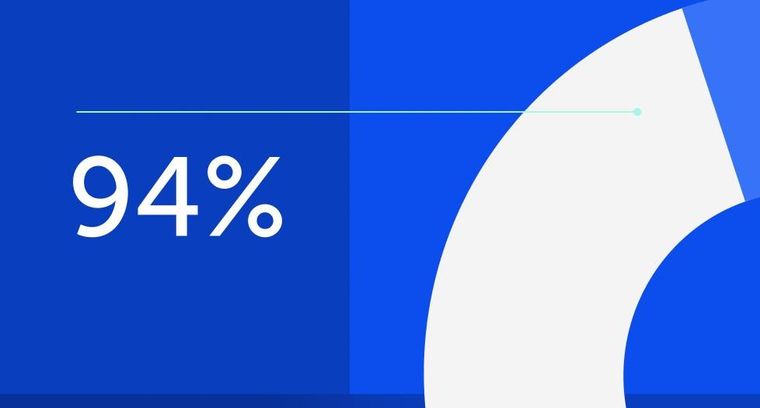
94% of researchers rate our articles as excellent or good
Learn more about the work of our research integrity team to safeguard the quality of each article we publish.
Find out more
REVIEW article
Front. Neurosci., 28 April 2022
Sec. Neurodevelopment
Volume 16 - 2022 | https://doi.org/10.3389/fnins.2022.889155
This article is part of the Research TopicCell adhesion molecules in neural development and diseaseView all 10 articles
Precise wiring of neural circuits is essential for brain connectivity and function. During development, axons respond to diverse cues present in the extracellular matrix or at the surface of other cells to navigate to specific targets, where they establish precise connections with post-synaptic partners. Cell adhesion molecules (CAMs) represent a large group of structurally diverse proteins well known to mediate adhesion for neural circuit assembly. Through their adhesive properties, CAMs act as major regulators of axon navigation, fasciculation, and synapse formation. While the adhesive functions of CAMs have been known for decades, more recent studies have unraveled essential, non-adhesive functions as well. CAMs notably act as guidance cues and modulate guidance signaling pathways for axon pathfinding, initiate contact-mediated repulsion for spatial organization of axonal arbors, and refine neuronal projections during circuit maturation. In this review, we summarize the classical adhesive functions of CAMs in axonal development and further discuss the increasing number of other non-adhesive functions CAMs play in neural circuit assembly.
Forming precise neural circuits is critical for nervous system function. Defects in neuronal connectivity have notably been observed in multiple neurodevelopmental disorders including fragile X syndrome (Swanson et al., 2018), autism spectrum disorders (McFadden and Minshew, 2013; Di Martino et al., 2014; Avital et al., 2015), tuberous sclerosis complex (Widjaja et al., 2010; Baumer et al., 2015; Im et al., 2016) and others, making the wiring of axonal connections a subject of intense research.
Developing axons navigate along precise paths toward their target by responding to attractive and repulsive guidance cues present in their environment. Navigation is ensured by highly motile structures at the leading end of axons, the growth cones, which harbor a unique repertoire of receptors at their surface that allow them to interpret the various extracellular signals they encounter. Many secreted and membrane-anchored factors including cell adhesion molecules (CAMs) (Tessier-Lavigne and Goodman, 1996), the canonical guidance cues Ephrins, Netrins, Semaphorins, and Slits (Dickson, 2002), neurotrophic and growth factors (Charoy et al., 2012), and morphogens (Sánchez-Camacho and Bovolenta, 2009; Yam and Charron, 2013), provide long-range or contact-mediated signals. Growth cones integrate the guidance information they receive from these signals and in turn, transduce the mechanical forces required for axon extension and turning (Kerstein et al., 2015). After reaching their final destination, axons stop elongating, branch extensively to form a terminal arbor and establish specific synaptic connections with appropriate partners. Patterns of connectivity are subsequently remodeled and refined in an activity-dependent manner, leading to the establishment of precise local circuits for an efficient transfer of information (Kutsarova et al., 2017). Axon pathfinding, selective target innervation and specificity of synapse formation are central aspects of circuit wiring that all critically rely on long-range as well as contact-mediated signaling between axons and their substrate or surrounding cells.
CAMs form a very large group of transmembrane or glycophosphatidylinositol (GPI)-anchored proteins that mediate contacts between cells or cells and a substrate via homophilic or heterophilic interactions. First identified in the mid-70s as molecules mediating cell-cell adhesion (Rutishauser et al., 1976), CAMs have historically been classified into four main families including integrins, CAMs of the immunoglobulin superfamily (IgSF), cadherins, and selectins based on the structural composition of their extracellular domain (Bock, 1991). However, this classification omits molecules discovered afterward that also act as cell adhesion proteins such as neuroligins, neurexins, teneurins, and synaptic proteins containing extracellular leucine-rich repeats (LRRs) (Figure 1). Thanks to their diverse extracellular domains conferring distinct adhesion modalities and engaging in unique protein interactions, CAMs regulate many aspects of neural development ranging from neurogenesis (Homan et al., 2018; Huang et al., 2020) and neuronal migration (Schmid and Maness, 2008; Solecki, 2012; Chen et al., 2018) to neurite development (Pollerberg et al., 2013; Missaire and Hindges, 2015), synaptogenesis (Gerrow and El-Husseini, 2006; Yogev and Shen, 2014) and myelination (Rasband and Peles, 2021). Not surprisingly, many mutations in CAMs have been linked to neurodevelopmental and psychiatric disorders (Sakurai, 2017; Cuttler et al., 2021; Jaudon et al., 2021). Interestingly, whereas CAMs have long been known to regulate axonal development and synaptogenesis through their adhesive properties, other non-adhesive and perhaps counterintuitive functions of CAMs have more recently emerged. In this review, we summarize the classical adhesive functions of CAMs in axonal and synaptic development and further discuss the increasing number of other non-adhesive roles CAMs play in neural circuit assembly and maturation.
Figure 1. Main families of CAMs expressed in the nervous system. CAMs have historically been classified into families based on the structural composition of their extracellular domain. Integrins form obligate heterodimers composed of α and β integrin subunits that cluster at the plasma membrane to mediate adhesion to the extracellular matrix. CAMs of the Immunoglobulin Superfamily (IgSF) are characterized by the presence of one or more Ig-like domains that can be followed by Fibronectin type III domain (Fn3) repeats. IgSF CAMs mediate adhesion by engaging in homophilic or heterophilic interactions. Most of them include a transmembrane and intracellular domains, but some like Contactins are GPI-anchored. CAMs of the Cadherin Superfamily mostly engage in homophilic interactions and are characterized by the presence of one or more calcium-binding cadherin repeats. The Leucine-rich repeat (LRR) Superfamily includes adhesion molecules that are characterized by the presence or LRRs and can include Ig or Fn3 domains in their extracellular domain. LRR CAMs are often found at synapses and engage in both homophilic or heterophilic trans-interactions. Neurexins and Neuroligins engage in heterophilic interactions in trans at nascent synapses to promote synaptic differentiation and stabilization. Teneurins are type II single-pass transmembrane proteins that interact homophilically or engage in trans-interactions with Latrophilins, a class of adhesion G-protein coupled receptors (not shown).
Through their adhesive properties, CAMs mediate stabilizing contacts and attachment between axons and their surrounding environment that are critical for axon navigation, fasciculation, target selection, and synaptogenesis.
Proper axon pathfinding requires a tightly controlled adhesion of growth cones to their substrate. The assembly and detachment of adhesions to the extracellular matrix (ECM) is especially critical for the advance of pioneer axons that are the first to extend in a particular region. Like non-neuronal cells that form integrin-mediated focal adhesions at their leading edge during migration (Mishra and Manavathi, 2021), growth cones assemble similar integrin-dependent adhesions named point contacts with the ECM (Gomez et al., 1996; Woo and Gomez, 2006). Point contacts form after integrins at the surface of growth cones bind ECM ligands, leading to the clustering of integrins and subsequent recruitment of adaptor proteins linking integrins to the actin cytoskeleton (Figure 2A). By stabilizing filopodial protrusions and restraining the retrograde flow of actin at the growth cone periphery, point contacts promote the advance of the growth cone and axon extension (Woo and Gomez, 2006; Myers and Gomez, 2011; Nichol et al., 2016; Kershner and Welshhans, 2017). Interestingly, many extracellular factors regulate axon elongation and cell migration by modulating integrin-mediated adhesions (Nakamoto et al., 2004; Bechara et al., 2008). Substrates or guidance cues that promote or inhibit the localized assembly and turnover of point contacts lead to growth cone turning in vitro (Hines et al., 2010; Myers and Gomez, 2011; Nichol et al., 2016; Kerstein et al., 2017), further suggesting a direct role for integrin-mediated attachment in axon pathfinding. In mammals, 18 α and 8 β integrins can assemble into 24 heterodimers that bind with different affinities to a large diversity of ECM ligands (Hynes, 2002). Inhibiting integrin β1 that forms the majority of integrin heterodimers reduces retinal axon elongation in zebrafish (Lilienbaum et al., 1995). It also prevents ipsilateral retinal projections from innervating a specific sublamina of the superior colliculus that expresses the ECM glycoprotein Nephronectin in mouse (Su et al., 2021; Figure 2B). Thus, integrin-mediated interactions between growth cones and the ECM not only regulate the rate of axon elongation but also directly specify target selection for circuit wiring. To what extent a cell-specific integrin code generated by the different combinations of α and β integrins contributes to the specificity of network assembly remains to be elucidated.
Figure 2. Adhesive functions of CAMs in circuit wiring. (A) Integrins mediate adhesion to the ECM for growth cone advance. Binding of integrins to ECM ligands leads to the clustering of integrins and the recruitment of adaptor proteins linking integrins to the actin cytoskeleton. The point contacts hence formed promote growth cone advance by stabilizing filopodial protrusions and restraining the retrograde flow of actin at the growth cone periphery. (B) Integrins mediate adhesion to the ECM for target selection. In the visual system, integrin α8β1 is selectively expressed by retinal ganglion cells projecting ipsilaterally. Its ligand, the ECM glycoprotein Nephronectin, is restricted to a sublamina at the target. Interaction between integrin α8β1 and Nephronectin is necessary for the laminar targeting of ipsilateral axons to the rostral stratum opticum (SO). Deleting integrin α8β1 or Nephronectin causes a dramatic loss of ipsilateral projections while contralateral projections remain unaffected in the stratum griseum superficial (SGS). Adapted from Su et al. (2021). (C,D) Protocadherin-17 (Pcdh17) mediates trans-axonal interactions for proper tract formation. (C) Pcdh17 accumulates at homotypic contacts between growth cones and axons from amygdala neurons, where it recruits the WAVE complex, Lamellipodin, and Ena/VASP proteins that remodel the actin cytoskeleton and promote membrane protrusion. Pcdh17-mediated adhesion enhances growth cone motility and enable growth cone advance along homotypic axons. (D) Pcdh17 is required for the extension of amygdala axons through the stria terminalis toward the hypothalamus. Adapted from Hayashi et al. (2014). (E) A CAM code specifies laminar targeting and synaptic specificity in the retina. Sdk1, Sdk2, Dscam, DscamL, and Cntns (not all a represented here) are expressed in non-overlapping subsets of bipolar (green), amacrine (orange), and retinal ganglion cells (purple) and engage in homophilic trans-interactions to direct synapse formation between matching partners in specific laminae (S1–S5) of the inner plexiform layer (IPL). Classical cadherins (Cdh) also contribute to the molecular code specifying connections. INL, inner nuclear layer; GCL, ganglion cell layer. Adapted from Sanes and Zipursky (2020).
In addition to adhering to the ECM, pioneer growth cones directly interact with surrounding glial cells such as neuroepithelial cells, radial glial cells, and astrocytes during their navigation (Rigby et al., 2020). Glial cells often localize at intermediate choice points along migratory routes where they act as guideposts providing contact-mediated spatial information or acting as a permissive substrate for growth. For instance, radial glia create a palisade at the optic chiasm where they directly contact and guide retinal axons (Marcus et al., 1995). More recently, neural stem cells residing in the ventricular zone of the forebrain medial ganglionic eminence have been shown to use their radial fiber scaffold to direct corticospinal axons at the junction between the striatum and globus pallidus (Kaur et al., 2020). Most adhesive contacts between navigating growth cones and glial cells appear to be mediated by CAMs of the Immunoglobulin and Cadherin superfamilies. N-cadherin and NCAM, for instance, promote retinal axon outgrowth over astrocytes in vitro (Neugebauer et al., 1988). They also mediate strong interactions between growth cones of olfactory axons and ensheathing cells in vivo, enabling olfactory axons to ride along ensheathing cell bodies as they pioneer the path toward the olfactory bulb (Su and He, 2010). In the spinal cord, NrCAM expressed by floor plate cells guides commissural axons across the midline by, in part, interacting with Contactin-2 (Cntn2, also known as TAG-1 or axonin-1) at the axonal surface (Stoeckli et al., 1997; Fitzli et al., 2000). NrCAM is also expressed by radial glia at the optic chiasm and promotes the crossing of NrCAM-positive retinal axons projecting contralaterally (Williams et al., 2006; Kuwajima et al., 2012). Along the optic tract, NF-protocadherin, a member of the Cadherin superfamily, mediates interactions between retinal axons and their neuroepithelial substrate for proper pathfinding (Leung et al., 2013). Retinal axons expressing L1CAM are then guided in the superior colliculus by collicular cells expressing ALCAM (also called BEN, DM-GRASP, SC1, or Neurolin) (Buhusi et al., 2009). In the absence of ALCAM, axonal branches fail to extend mediolaterally, leading to defects in retinotopic map formation. Outside the visual system, recent work has revealed that Celsr3, a member of the Flamingo group within the Cadherin superfamily, is present at the surface of commissural growth cones and promotes axon pathfinding across the floor plate by binding in trans to Dystroglycan, a transmembrane protein at the surface of neurepithelial cells (Lindenmaier et al., 2019). Interestingly, other families of CAMs such as teneurins and neuroligins have been found to accumulate in growth cones during axon elongation (Suzuki et al., 2014; Gatford et al., 2021). Determining whether they also mediate adhesion between pioneer growth cones and glial cells will be important to gain a more comprehensive view of the inter-cellular interactions that govern neural circuit assembly during early development.
Pioneer axons play a critical role in neural circuit assembly, not only by defining first itineraries toward appropriate targets, but also by acting as guides and providing a scaffold for later-born axons that follow them (Pike et al., 1992; Hidalgo and Brand, 1997; Rash and Richards, 2001). In the olfactory and retinotectal systems, for instance, ablation of early-born pioneer neurons causes follower axons to misroute and fail to build proper connections (Whitlock and Westerfield, 1998; Pittman et al., 2008; Okumura et al., 2016). The formation of axon tracts en route to a target involves homotypic or heterotypic fasciculation between axons, which are usually initiated after a growth cone contacts the shaft of a neighboring axon and moves along it. Alternatively, axon shafts can dynamically interact, leading to a zippering behavior triggering their fasciculation (Šmít et al., 2017). Extensive literature has demonstrated a major role for IgSF CAMs in regulating homotypic axon-axon interactions (Spead and Poulain, 2021). CAMs engaged in homophilic (between same CAMs) or heterophilic (between different CAMs) trans-interactions mediate the selective recognition between elongating growth cones and pre-existing axon shafts, thereby dictating the specificity of axonal bundling for tract formation. They also provide the adhesive force required for growth cone advance through their coupling to the actin cytoskeleton (Pollerberg et al., 2013; Abe et al., 2018). Not surprisingly, the loss of specific CAMs leads to disorganized tracts in many circuits. Blocking L1CAM in the chick hindlimb, for instance, causes a defasciculation of both motor and sensory axons that fail to project to their respective targets (Landmesser et al., 1988; Honig and Rutishauser, 1996; Honig et al., 2002). L1CAM is also required for the fasciculation between axons innervating the peduncle of the mushroom bodies in Drosophila (Siegenthaler et al., 2015). Likewise, ALCAM and DSCAM both regulate the fasciculation of retinal axons in the visual system (Pollerberg and Mack, 1994; Ott et al., 1998; Weiner et al., 2004; Bruce et al., 2017). Continuous synthesis of ALCAM in growth cones is notably required for the preferential growth of retinal axons on ALCAM substrates and maintained by local mRNA translation (Thelen et al., 2012). In the mouse motor system, Cntn2 accumulates in the distal segment of motor axons extending in the periphery and controls their fasciculation (Suter et al., 2020). Conversely in the peripheral system, SynCAM2 and SynCAM3 were found to regulate contacts between sensory afferents as they enter the dorsal root entry zone of the spinal cord (Frei et al., 2014). Similarly to IgSF CAMs, members of the Cadherin superfamily have also emerged as important regulators of tract organization. Tectofugal projections innervating different parts of the brain elongate along pre-existing axonal pathways expressing the same cadherin, demonstrating that cadherins mediate selective axon fasciculation through homotypic trans-interactions (Treubert-Zimmermann et al., 2002). As such, cadherins organize axonal tracts depending on their selective expression in the nervous system. N-cadherin and Cadherin-8, for instance, are both required for the fasciculation of mossy fibers in the hippocampus (Bekirov et al., 2008), while Cadherin-11 promotes the bundling of motor axons (Marthiens et al., 2005). More recently, Protocadherin-17 (Pcdh17) has been found to regulate the formation of homotypic contacts between amygdala axons elongating toward the hypothalamus and ventral striatum (Hayashi et al., 2014; Figures 2C,D). Growth cones lacking Pdch17 no longer migrate along Pdch17-positive axons, whereas axons ectopically expressing Pdch17 intermingle with axons expressing endogenous Pdch17.
Guidance cues can regulate axon fasciculation and pathfinding by modulating the levels of CAMs at the axonal surface. Semaphorin3D, for instance, promotes the bundling of medial longitudinal fascicle axons in zebrafish by increasing L1CAM protein levels (Wolman et al., 2007). In Drosophila, Semaphorin-1a reverse signaling promotes the fasciculation of photoreceptor axons by inhibiting Rho1, a small GTPase known to mediate the degradation of the NCAM ortholog Fasciclin 2 (Hsieh et al., 2014). Conversely in the Xenopus visual system, Semaphorin-3A (Sema3A) prevents retinal axons from exiting their normal trajectories by inducing the local translation of NF-protocadherin in retinal growth cones (Leung et al., 2013). Alternatively, extracellular factors can regulate the strength of growth cone adhesion by modulating the coupling of CAMs to the actin cytoskeleton. Netrin-1, for instance, promotes traction force for growth cone migration by enhancing the coupling of L1CAM to F-actin via the adaptor Shootin1a (Kubo et al., 2015; Baba et al., 2018). Whether other guidance cues such as Ephrins (Luxey et al., 2013) or Slits (Jaworski and Tessier-Lavigne, 2012) regulate axon fasciculation by modulating CAMs and their adhesive properties remains to be determined.
CAMs not only regulate axon growth, fasciculation and guidance toward a main target but also dictate the specificity of synapse formed between axons and dendrites. After reaching their main target area, axons must establish synapses with appropriate partners while avoiding unsuitable ones. Synaptic specificity is achieved by both laminar targeting, during which axon terminals and dendrites of post-synaptic neurons sharing similar functional properties assemble into local layers within the main target, and specific cellular and sub-cellular synapse assembly.
Studies on the mechanisms governing laminar targeting in the visual system have demonstrated critical roles for IgSF CAMs and cadherins in mediating trans-cellular recognition between correct synaptic partners (Huberman et al., 2010; Sanes and Zipursky, 2020). In the chick retina, Sidekick 1 (Sdk1), Sdk2, Dscam, DscamL, and Cntns were found to be uniquely expressed in non-overlapping subsets of two classes of interneurons, the bipolar and amacrine cells, as well as in their post-synaptic partners, the retinal ganglion cells (RGCs) (Figure 2E). Interestingly, RGCs and interneurons with matching expression of these IgSF CAMs form synapses in specific sublaminae of the inner plexiform layer (Yamagata et al., 2002; Yamagata and Sanes, 2008, 2012). Modifying the “CAM code” a neuron expresses by depleting or overexpressing any of these CAMs diverts axonal arbors to sublaminae expressing the matching set of CAMs, indicating an essential role for homophilic trans-interactions in laminar targeting. Similar laminar targeting defects were observed in mice lacking Sdks, DSCAM, or Cntn5, suggesting conserved roles for these CAMs across vertebrates (Fuerst et al., 2010; Krishnaswamy et al., 2015; Peng et al., 2017; Yamagata and Sanes, 2019). However, whereas Sdks and Cntn5 regulate laminar targeting by homophilic interactions in both mouse and chick, DSCAM appears to exert its function differently in mouse by masking signaling from cadherins (Simmons et al., 2017; Garrett et al., 2018). Indeed, cadherins also contribute significantly to the coding of laminar targeting through their homophilic interactions. Among the 15 different classical cadherins detected in the direction-selective circuits in the retina, six are expressed in combination or individually in populations of functionally distinct interneurons and RGCs and control their laminar connectivity (Duan et al., 2014, 2018). Cadherin-8 (Cdh8) and Cdh9, for instance, direct axons of a subset of bipolar cells to RGCs responding to bright or dark moving objects, whereas Cdh7 and Cdh18 specify synapses formed between amacrine cells and RGCs responding to nasal motion. Cdh6, on the other hand, targets not only amacrine cell axons to RGCs responding to ventral motion in the retina, but also RGC axons to their specific visual targets in the brain (Osterhout et al., 2011). A similar coding principle for axon-target matching has been observed in other circuits. In the hippocampus, for instance, Cdh9 specifically regulates the formation of mossy fiber synapses between dentate gyrus and CA3 neurons (Williams et al., 2011). Likewise in the cerebellum, Cdh7 mediates synapse formation between pontine axons and granule neurons (Kuwako et al., 2014). More recently, IgSF11 has been identified as the homophilic adhesion molecule controlling synapse formation between inhibitory chandelier cells and pyramidal neurons in the cortex (Hayano et al., 2021).
Other CAMs besides IgSF CAMs and cadherins regulate synaptic specificity. Teneurins, for instance, are expressed in several inter-connected regions in the nervous system and form trans-synaptic interactions by binding homophilically or heterophilically to Latrophilins, a class of adhesion G-protein coupled receptors (Boucard et al., 2014; Araç and Li, 2019). The role of teneurins in synaptic partner matching was first established in Drosophila, in which Ten-a and Ten-m instruct target selection in the motor and olfactory systems (Hong et al., 2012; Mosca et al., 2012). Teneurins were found afterward to also specify connectivity in the hippocampus and the visual system of vertebrates. Teneurin-2 (Tenm2) promotes synapse formation between CA3 Schaffer collaterals and CA1 pyramidal hippocampal neurons by forming a trans-synaptic complex with Latrophilin-3 (Lphn3) and FLRT3 (Sando et al., 2019). In contrast, Tenm3 homophilic interactions are required for the precise targeting of proximal CA1 axons to the distal subiculum (Berns et al., 2018). Tenm3 also regulates the connectivity of orientation-selective RGCs in the zebrafish retina and optic tectum (Antinucci et al., 2013, 2016).
Thus, homophilic and heterophilic trans-interactions between specific CAMs generate a combinatorial recognition code for synaptic matching in addition to signaling for axon growth termination and synapse formation. This code not only specifies connectivity between distinct sets of neurons but can also direct synaptic specificity at a sub-cellular level through the accumulation of CAMs at defined sites. In the cerebellum, for instance, the IgSF CAM Neurofascin-185 accumulates in the axon initial segment (AIS) of Purkinje cells and directs the formation of pinceau synapses by basket interneurons (Ango et al., 2004). Similarly in the cortex, anchoring of L1CAM to the AIS of pyramidal neurons is required for selective innervation by Chandelier cells (Tai et al., 2019). The code specifying cellular or sub-cellular synaptic interactions is most often generated by the differential expression of CAMs among neurons but can also be achieved by the temporal regulation of CAM expression. In Drosophila, for instance, N-Cadherin is expressed by both R7 and R8 photoreceptors, albeit at different levels and moments. N-Cadherin expression peaks in R8 cells at the time R8 axons arrive at their target layer in the medulla, leading to axonal innervation of that layer. In contrast, R7 axons that express high levels of N-cadherin at a later timepoint bypass the R8 target and terminate in a more distant layer (Petrovic and Hummel, 2008). Alternatively, CAM trans-interactions can be modulated by alternative splicing. Splicing of Tenm2, for instance, changes the structure of Tenm2’s extracellular β-propeller domain and determines which trans-synaptic partner Tenm2 interacts with (Li et al., 2018, 2020). Tenm2 lacking the splice insert interacts with Latrophilins to promote excitatory synapse formation, whereas Tenm2 containing the insert cannot and specifies inhibitory synapses instead. Contact-mediated signaling between matching trans-synaptic partners eventually initiates synapse formation and stabilization by recruiting additional CAMs such as neurexins, neuroligins and LRR-containing adhesion molecules. As for synaptic matching, the assembly of synapses with specific properties is governed by the type of trans-synaptic molecules engaged. This vast field of research falls outside the scope of this review, but we refer interested readers to excellent recent articles on that topic (Schroeder and de Wit, 2018; Gomez et al., 2021; Südhof, 2021).
Although the adhesive properties of CAMs play critical roles at all stages of circuit wiring, CAM functions go far beyond adhesion and mechanical stabilization. Through their intracellular domains and interactions with other receptors at the plasma membrane, CAMs can activate or modulate a panoply of intracellular signaling pathways leading to morphological or transcriptional changes. They can also be cleaved from the plasma membrane and act as bona fide signaling ligands in the extracellular environment. CAMs have thus emerged as major signaling orchestrators of nervous system assembly.
Axons elongating over long distances require the synthesis of new raw materials to sustain the assembly of cytoskeletal structures and membrane components. While local mRNA translation can be activated in growth cones to induce rapid changes in response to local cues (Dalla Costa et al., 2020; Agrawal and Welshhans, 2021), a constant communication between the growth cone and the nucleus regulates nuclear transcription to adjust gene expression to axonal needs. An increasing body of evidence indicates that CAMs regulate transcription in developing neurons, both indirectly by regulating the activation or trafficking of transcription factors, and directly, by acting in the nucleus themselves.
Several CAMs stimulate gene transcription by activating intracellular signaling pathways, either through the activity of their intracellular domain or by interacting with signaling receptors (Figure 3A). L1CAM and NCAM, for instance, were both reported to activate mitogen-activated protein kinase (MAPK) pathways to promote neurite outgrowth (Kolkova et al., 2000; Schmid et al., 2000; Poplawski et al., 2012). NCAM interacts with the fibroblast growth factor receptor (FGFR) to activate MAPK and, in turn, the transcription factors CREB and c-Fos (Jessen et al., 2001; Niethammer et al., 2002). Activation of MAPK by L1CAM, on the other hand, requires L1CAM internalization (Schaefer et al., 1999; Schmid et al., 2000). Alternatively, CAMs might regulate transcription by directly modulating the nuclear trafficking of proteins with transcriptional activity. The intracellular domain of NF-protocadherin, for instance, directly binds TAF1, a component of the basal transcription factor complex TFIID (Heggem and Bradley, 2003). Inhibiting either NF-protocadherin or TAF1 severely impairs retinal axon initiation and elongation, suggesting that NF-protocadherin might regulate TAF1-dependent transcriptional programs to promote axonal growth (Piper et al., 2008). Conversely, classical cadherins constitutively retain the transcriptional coactivators β-catenin and p120 at the plasma membrane, thereby preventing them from translocating to the nucleus (Nelson and Nusse, 2004). Whether such a sequestration contributes to the transcriptional regulation of axonal development remains, however, unclear.
Figure 3. Non-adhesive functions of CAMs in circuit assembly. (A) CAMs regulate transcription for axon growth by activating intracellular signaling pathways from the plasma membrane (1), acting directly in the nucleus after proteolytic cleavage (2), or regulating the transport of molecules with transcriptional activity (3). NCAM, for instance, interacts with FGFR to activate the MAPK pathway and in turn, transcription (1). Alternatively, proteolytic processing of NCAM releases a fragment that is trafficked through endosomes and the endoplasmic reticulum (ER), released in the cytoplasm, and finally translocated into the nucleus where it regulates transcription (2). NF-protocadherin (NF-Pcdh) directly interacts with TAF1, a component of the basal transcription factor complex TFIID, suggesting that NF-Pcdh might regulate axon elongation through TAF1-mediated transcriptional control. (B) CAMs instruct axon repulsion by modulating signaling pathways. Both L1CAM and Cntn2 form a complex with Nrp1, the receptor to the repulsive guidance cue Sema3A, at the plasma membrane. Cntn2 modulates axon response to Sema3A by regulating the endocytosis of the Nrp1/L1CAM/Sema3A complex. After internalization, L1CAM and Nrp1 become segregated by Cntn2 into two distinct trafficking pathways. Nrp1 is routed to endocytic compartments where its increased association with PlexinA4 signals for collapse. Adapted from Law et al. (2008) and Dang et al. (2012). (C) CAMs instruct repulsion by acting as guidance cues. In the hippocampus, reciprocal repulsions mediated by Tenm3 and Lphn2 ensure proper target selection. Axons originating from proximal CA1 (pCA1) neurons (green) express Tenm3 and project to the distal subiculum (dSub) after being repelled by Lphn2 (pink) present in the proximal subiculum (pSub). Conversely, distal CA1 (dCA1) axons expressing Lphn2 (pink) are repelled by Tenm3 in dSub (green) and project to pSub. Adapted from Pederick et al. (2021). (D) Clustered Pcdhs regulate self-avoidance. Pcdh genes are organized into three adjacent clusters that include several variable exons. Each variable exon codes for an extracellular and transmembrane domains and is preceded by a promoter randomly activated in individual neurons to drive transcription. Stochastic promoter choice leads to the production of different Pcdh isoforms from each of the three clusters in a cell-specific manner, thereby generating a unique combination of Pcdh α, β, and γ expression in each neuron. Sister branches from the same terminal arbor express the same code of Pcdhs at their surface and repel each other after Pcdhs interact homophilically in trans. (E) CAMs ensure tiling of terminal arbors. In the Drosophila visual system, DSCAM2, Turtle (Tutl), and Flamingo (Fmi) together with Gogo engage in homophilic trans-interactions to activate repulsion, thereby control the proper spacing of L1-L5, R8, and R7 terminal arbors, respectively, in the medulla. Adapted from Spead and Poulain (2021).
In addition to activating transcriptional pathways from the plasma membrane, a number of CAMs have recently emerged as transcriptional activators or repressors acting directly in the nucleus for controlling axon elongation (Figure 3A). Indeed, several proteases including caspases, matrix metalloproteases, and members of the ADAM family, can cleave the intracellular domains of transmembrane CAMs, which then translocate to the nucleus to regulate transcription. Activation of NCAM, for instance, leads to its cleavage by a serine protease and the subsequent nuclear import of a C-terminal fragment that is necessary for NCAM-induced axon growth (Kleene et al., 2010; Homrich et al., 2018). Interestingly, the fragment generated after cleavage includes not only the intracellular domain of NCAM but also its transmembrane domain and a stub of its extracellular domain, indicating that NCAM is proteolytic processed by an extracellular enzyme. Modification of the extracellular stub by polysialic acid, a glycan known to modulate NCAM function, does not prevent its nuclear import but leads to the transcription of a distinct set of genes, demonstrating a unique role for NCAM glycosylation in transcriptional regulation (Westphal et al., 2016, 2017a,b). L1CAM, DSCAM and DSCAML1 also regulate transcription after cleavage. Like NCAM, activated L1CAM undergoes a serine protease-dependent cleavage at the plasma membrane that generates a fragment containing the intracellular, transmembrane and part of the extracellular domains (Lutz et al., 2012, 2014). The sumoylated L1CAM fragment hence generated traffics to endosomes and the cytoplasm before translocating in a sumoylation-dependent manner to the nucleus where it interacts with multiple nuclear proteins (Girbes Minguez et al., 2020). Interaction with heterochromatin protein 1 is notably required for L1CAM-mediated neurite outgrowth in cultured cortical neurons (Kleene et al., 2022). Conversely, the intracellular domains generated after cleavage of DSCAM and DSCAML1 by γ-secretase alter the transcription of genes regulating circuit formation and inhibit axon growth when overexpressed in cortical neurons (Sachse et al., 2019). Other CAMs besides IgSF CAMs appear to directly regulate transcription in the nucleus. The intracellular domain of Tenm2, for instance, is released after homophilic interaction and represses the activity of Zic1, a transcription factor known to regulate the targeting of mossy fibers in the cerebellum (Bagutti et al., 2003; Dipietrantonio and Dymecki, 2009). Conversely, Tenm3’s intracellular domain interacts with Zic2, another member of the Zic family that specifies binocular vision by regulating the guidance of retinal axons projecting ipsilaterally in the visual system (Herrera et al., 2003; Glendining et al., 2017). Both Zic2 and its transcriptional target EphB1 are upregulated in Tenm3 mutants, which likely explains the strong ipsilateral targeting defects observed in these mice (Leamey et al., 2007; Dharmaratne et al., 2012). As for Tenm1, its intracellular domain has been shown to regulate transcription by binding to the transcriptional repressors MBD1 and HINT1 (Nunes et al., 2005; Schöler et al., 2015). Defining the mechanisms controlling the release and transport of CAM intracellular domains, and identifying the cell-specific nuclear partners they interact with, remain important questions to address for better understanding how long distance communication between axons and soma modulate axon guidance at choice points and target innervation.
Although CAMs were first shown to promote axon growth through their adhesive properties, many of them have since emerged as signaling receptors or ligands instructing growth cone guidance independently of adhesion. Perhaps unexpectedly, CAMs were found to actively participate in the control of axon repulsion by dictating the axon’s sensitivity to repulsive signals. Many IgSF CAMs, for instance, form signaling complexes with receptors to repulsive guidance cues. L1CAM directly binds in cis to Neuropilin-1 (Nrp1), the receptor to Sema3A, and is required for Sema3A-induced growth cone collapse (Castellani et al., 2000, 2002). L1CAM mediates both signaling downstream of Nrp1 and Nrp1 internalization upon Sema3A binding, thus coordinating signaling cascades instructing growth cone collapse with a decreased adhesiveness (Castellani et al., 2004; Bechara et al., 2008). Interestingly, Cntn2 also forms a complex with Nrp1 and L1CAM at the plasma membrane and regulates axon response to Sema3A by modulating the endocytosis of the Nrp1/L1CAM/Sema3A complex (Law et al., 2008; Figure 3B). L1CAM and Nrp1 are endocytosed together but become segregated by Cntn2 into two distinct trafficking pathways (Dang et al., 2012). Nrp1 is notably routed to endocytic compartments where it increases its association with PlexinA4, which in turn signals for collapse. In the absence of Cntn2, Nrp1, and L1CAM are no longer separated intracellularly and signaling is reduced. Similarly to L1CAM that associates with Nrp1, NrCAM forms a complex with Nrp2 and PlexinA3 (Falk et al., 2005; Demyanenko et al., 2011). In response to Sema3F, NrCAM clusters Nrp2 and PlexinA3 at the plasma membrane, which activates signaling for growth cone collapse. Thalamocortical axons lacking NrCAM are no longer sensitive to Sema3F in vivo and misproject caudally in the ventral telencephalon (Demyanenko et al., 2011). Other guidance pathways besides Semaphorin signaling are controlled by IgSF CAMs. NCAM, for instance, clusters and activates EphA3 in response to ephrin-A5, thereby eliciting RhoA-dependent growth cone collapse in GABAergic interneurons (Sullivan et al., 2016). Similarly, DSCAM interacts with Unc5 to trigger the repulsion of cerebellar axons away from Netrin-1 (Purohit et al., 2012). More recently, the LRR-containing adhesion molecule FLRT3 was shown to directly interact with Robo1, a receptor to Slits (Leyva-Díaz et al., 2014). FLRT3 not only modulates the repulsion of rostral thalamocortical axons in response to Slit1 in vitro, but also their attraction toward Netrin-1 in a Robo1-dependent manner in vivo.
In addition to forming signaling complexes with guidance receptors, CAMs can act directly as instructive cues for axon repulsion. In Drosophila, for instance, Integrins α1 and α2 mediate trans-axonal repulsive signaling between motor axons to induce their defasciculation and target them to proper targets (Huang et al., 2007). Mutants lacking either of these integrins have increased axon fasciculation that causes a lack of muscle innervation. Likewise in vitro, the close homolog of L1CAM, Chl1, engages in homophilic interactions to repel ventral midbrain dopaminergic axons (Alsanie et al., 2017). Very recently, repulsive interactions between Tenm3 and Lphn2 were shown to topographically direct CA1 axons to the subiculum in the hippocampus (Pederick et al., 2021; Figure 3C). CAMs can instruct axon repulsion not only locally by signaling from the surface of cells or others axons, but also distally by acting as a gradient after shedding of their extracellular domain. The ectodomains of FLRT2 and FLRT3, for instance, are released after cleavage by metalloproteases and act as repulsive guidance cues for hippocampal axons expressing Unc5 (Yamagishi et al., 2011). While many CAMs are processed by metalloproteases (Saftig and Lichtenthaler, 2015), the functions of their ectodomains in circuit assembly remain surprisingly unknown. We can anticipate, though, that ectodomain release would increase the functional diversity of CAMs and provide an additional level of spatiotemporal regulation for signaling. The ectodomain of Tenm2, for instance, is proteolytically cleaved during development and was recently shown to attract hippocampal axons in vitro by binding to Lphn1 (Vysokov et al., 2016, 2018).
Neuronal connectivity and function rely on the precise innervation of targets by axons. After reaching their final destination, axons branch extensively to form elaborate terminal arbors within specific territories. Branching patterns form dynamically and become spatially organized to maximize the coverage of an area while minimizing redundancy of targeting. Optimal coverage is achieved through two main mechanisms (Grueber and Sagasti, 2010). Isoneural spacing or self-avoidance refers to the repulsion between axonal branches of a same neuron, so that branches avoid overlapping with each other. Likewise, arbors from distinct neurons that share the same function do not overlap, a phenomenon referred to as heteroneural avoidance or tiling. Both self-avoidance and tiling pertain to axons as well as dendrites and are achieved by contact-mediated repulsion (Sagasti et al., 2005).
First described in studies analyzing the receptor fields of sensory neurons in the leech (Nicholls and Baylor, 1968), self-avoidance relies on the ability of sister branches from the same terminal arbor to discriminate “self” from “non-self” before repelling each other. This selective recognition is achieved by the expression of a cell-surface molecular code that is common to sister branches but distinct among branches from different neurons. In Drosophila, DSCAM1 generates such a code. Alternative splicing of DSCAM1 pre-mRNA produces 38,016 distinct isoforms that differ in their ectodomain and are expressed in a probabilistic way (Schmucker et al., 2000). Consequently, each isoform gives an individual neuron a unique molecular identity. As only identical ectodomains can engage in homophilic trans-interactions due to conformational constraints, only sister branches sharing the same isoform will recognize each other (Wojtowicz et al., 2004, 2007). DSCAM1-mediated homophilic interactions initiate repulsion rather than adhesion in this context, which contrasts with the known function of DSCAM in promoting axon fasciculation (Bruce et al., 2017). DSCAM1-mediated self-avoidance enables the proper spatial organization of both axonal and dendritic arbors in diverse neuronal populations (Wang J. et al., 2002; Zhan et al., 2004; Hattori et al., 2007; Matthews et al., 2007; Soba et al., 2007). In the absence of DSCAM1, branches fail to separate and overlap or fasciculate instead. Interestingly, although the vertebrate ortholog DSCAM and its homolog DSCAML mediate self-avoidance in the mouse retina (Fuerst et al., 2008, 2009), they are not highly spliced and thus do not generate a molecular code like DSCAM1. Instead, DSCAM appears to mask adhesion-promoting signaling from other CAMs like cadherins (Garrett et al., 2018). If not DSCAM, which cell-surface molecules generate a recognition code in vertebrates? Like DSCAM1, clustered Protocadherins (Pcdhs) exist in a multitude of isoforms and as such, represent the largest subgroup in the Cadherin superfamily. About 60 Pcdh genes are organized into three adjacent clusters designed as Pcdh α, β, and γ (Wu and Maniatis, 1999; Figure 3D). Each cluster includes several variable exons that each codes for an extracellular and transmembrane domains. Each variable exon is further preceded by a promoter that is randomly activated in individual neurons to drive transcription (Tasic et al., 2002; Wang X. et al., 2002). Thus, stochastic promoter choice leads to the production of different Pcdh isoforms from each of the three clusters in a cell-specific manner, thereby generating a unique combination of Pcdh α, β, and γ expression in each neuron (Esumi et al., 2005; Kaneko et al., 2006; Thu et al., 2014; Mountoufaris et al., 2017). Clusters α and γ further include constant exons coding for a common intracellular domain that are combined to each variable exon by alternative splicing. Like DSCAM1, Pcdhs engage in strict homophilic trans-interactions and as such, provide a recognition code for sister branches of the same neuron (Hasegawa et al., 2012; Thu et al., 2014; Mountoufaris et al., 2017). They also form multimers in cis at the plasma membrane and signal through the intracellular domain of Pcdhs α and γ to initiate repulsion. Thanks to their diversity, Pcdhs have emerged as the main mediators of axonal and dendritic self-avoidance in vertebrates (Lefebvre et al., 2012). Deleting all three Pcdh clusters in mice, for instance, causes a loss of self-avoidance and severe arborization defects in olfactory axons (Mountoufaris et al., 2017). Conversely, “erasing” the Pcdh code by overexpressing a single-tricluster gene repertoire prevents olfactory axons from converging into stereotypically positioned glomeruli in the olfactory bulb.
Protocadherins also appear to regulate axonal tiling in addition to self-avoidance. In the basal ganglia and hippocampus, for instance, serotonergic axon terminals are evenly spaced in their target fields but become clumped together in mice lacking Pcdhαc2, the only Pcdhα isoform expressed in serotonergic neurons (Chen et al., 2017). Whether tiling of other axonal populations is similarly regulated by the expression of unique Pcdhs remains to be determined. Other CAMs, however, have been identified as tiling regulators. DSCAM is notably required for proper axonal and dendritic tiling of bipolar cells in the retina (Simmons et al., 2017). Likewise in the Drosophila visual system, DSCAM2, the atypical cadherin Flamingo (Fmi), and the IgSF CAM Turtle (Tutl) enable proper spacing of distinct classes of axons in spatially restricted columns in the medulla (Millard et al., 2007; Tomasi et al., 2008; Ferguson et al., 2009; Hakeda-Suzuki et al., 2011; Figure 3E). Tutl engages in homophilic trans-interactions to prevent adjacent R7 photoreceptor cell terminals from overlapping (Ferguson et al., 2009). Similarly, Fmi mediates repulsive trans-interactions between R8 photoreceptors axons. Fmi interacts in cis with Gogo, another transmembrane receptor eliciting repulsive axon-axon interactions, suggesting that both proteins might act as a complex at the surface of branches to ensure tiling (Tomasi et al., 2008; Hakeda-Suzuki et al., 2011). Lastly, DSCAM2 ensures proper spacing of L1 axonal arbors, thereby restricting them to specific columns. In dscam2 mutants, L1 axons still innervate the correct layer of the medulla but are no longer restricted to a single column (Millard et al., 2007). Altogether, these studies indicate that the selective expression of individual or combined CAMs generates a cell-surface recognition code that ensures the segregation of terminal arbors into non-overlapping domains while instructing laminar targeting and synaptic specificity.
In conclusion, a large body of literature now demonstrates that CAMs orchestrate a striking number of developmental processes that are critical for neural circuit wiring. By engaging in homophilic and heterophilic trans-interactions, CAMs not only provide the adhesion and mechanical stabilization required for proper axon guidance and fasciculation, but also generate a cell-surface recognition code essential for synaptic specificity and contact-mediated self-avoidance and tiling. CAMs further act as bona fide signaling factors instructing growth cone behavior, modulating the activities of guidance receptors, and controlling transcriptional programs for axonal development.
Surprisingly, whether CAMs also contribute to the regressive events that refine neural circuits remains poorly known. Selective axon degeneration, for instance, is used to remodel axonal projections during metamorphosis in insects or prune exuberant axons or axonal branches in vertebrates (Riccomagno and Kolodkin, 2015; Schuldiner and Yaron, 2015). In the visual system, retinal axons that missort along the optic tract selectively degenerate, leading to proper pre-target topographic ordering of retinal projections (Poulain and Chien, 2013). Refinement of terminal retinal arbors also occurs at the target in an activity-dependent manner, leading to the sharpening of retinotopic maps and the segregation of ipsi- and contralateral axons into eye-specific territories in animals with binocular vision (Stellwagen and Shatz, 2002; Chandrasekaran et al., 2005; Ben Fredj et al., 2010). Interestingly, Cntn2 was recently identified as a key regulator of retinotectal map sharpening in zebrafish (Spead et al., 2021). Nasal retinal axons progressively refine their projection domain to the posterior tectum in wild-type but not in the absence of Cntn2, causing a lack of retinotopic map refinement along the antero-posterior axis. How Cntn2 remodels axon terminal arbors remains to be determined but might involve an interplay between Cntn2 signaling and neuronal activity. Indeed, neuronal activity might modulate the targeting of Cntn2 to the plasma membrane, just as it regulates that of Cntn1 in hypothalamic axons (Pierre et al., 2001). Conversely, Cntn2 could modulate neuronal activity by regulating sodium or potassium channels. Cntn1, for instance, interacts with several voltage-gated sodium channels and increases their density at the plasma membrane (Kazarinova-Noyes et al., 2001; Liu et al., 2001). Dissecting the cross-talk between CAMs and other signaling pathways will be critical to fully comprehend the multiple roles CAMs play at various stages of circuit assembly. It might also provide new strategies for correcting mechanisms that get dysregulated in the context of neurodevelopmental disorders.
Both authors listed have made a substantial, direct, and intellectual contribution to the work, and approved it for publication.
This study was supported by the National Institutes of Health/National Institute of Neurological Disorders and Stroke (R01NS109197 and R21NS124542 to FP).
The authors declare that the research was conducted in the absence of any commercial or financial relationships that could be construed as a potential conflict of interest.
All claims expressed in this article are solely those of the authors and do not necessarily represent those of their affiliated organizations, or those of the publisher, the editors and the reviewers. Any product that may be evaluated in this article, or claim that may be made by its manufacturer, is not guaranteed or endorsed by the publisher.
We thank all referees for their constructive feedback and apologize for omitting any references owing to space restrictions.
Abe, K., Katsuno, H., Toriyama, M., Baba, K., Mori, T., Hakoshima, T., et al. (2018). Grip and slip of L1-CAM on adhesive substrates direct growth cone haptotaxis. Proc. Natl. Acad. Sci. USA 115, 2764–2769. doi: 10.1073/pnas.1711667115
Agrawal, M., and Welshhans, K. (2021). Local translation across neural development: a focus on radial glial cells, axons, and synaptogenesis. Front. Mol. Neurosci. 14:717170. doi: 10.3389/fnmol.2021.717170
Alsanie, W. F., Penna, V., Schachner, M., Thompson, L. H., and Parish, C. L. (2017). Homophilic binding of the neural cell adhesion molecule CHL1 regulates development of ventral midbrain dopaminergic pathways. Sci. Rep. 7:9368. doi: 10.1038/s41598-017-09599-y
Ango, F., di Cristo, G., Higashiyama, H., Bennett, V., Wu, P., and Huang, Z. J. (2004). Ankyrin-based subcellular gradient of neurofascin, an immunoglobulin family protein, directs GABAergic innervation at purkinje axon initial segment. Cell 119, 257–272. doi: 10.1016/j.cell.2004.10.004
Antinucci, P., Nikolaou, N., Meyer, M. P., and Hindges, R. (2013). Teneurin-3 specifies morphological and functional connectivity of retinal ganglion cells in the vertebrate visual system. Cell Rep. 5, 582–592. doi: 10.1016/j.celrep.2013.09.045
Antinucci, P., Suleyman, O., Monfries, C., and Hindges, R. (2016). Neural mechanisms generating orientation selectivity in the retina. Curr. Biol. 26, 1802–1815. doi: 10.1016/j.cub.2016.05.035
Araç, D., and Li, J. (2019). Teneurins and latrophilins: two giants meet at the synapse. Curr. Opin. Struct. Biol. 54, 141–151. doi: 10.1016/j.sbi.2019.01.028
Avital, H., Behrman, M., and Malach, R. (2015). The idiosyncratic brain: distortion of spontaneous connectivity patterns in autism spectrum disorder. Nat. Neurosci. 18, 302–309. doi: 10.1038/nn.3919
Baba, K., Yoshida, W., Toriyama, M., Shimada, T., Manning, C. F., Saito, M., et al. (2018). Gradient-reading and mechano-effector machinery for netrin-1-induced axon guidance. Elife 7:e34593. doi: 10.7554/eLife.34593
Bagutti, C., Forro, G., Ferralli, J., Rubin, B., and Chiquet-Ehrismann, R. (2003). The intracellular domain of teneurin-2 has a nuclear function and represses zic-1-mediated transcription. J. Cell. Sci. 116, 2957–2966. doi: 10.1242/jcs.00603
Baumer, F. M., Song, J. W., Mitchell, P. D., Pienaar, R., Sahin, M., Grant, P. E., et al. (2015). Longitudinal changes in diffusion properties in white matter pathways of children with tuberous sclerosis complex. Pediatr. Neurol. 52, 615–623. doi: 10.1016/j.pediatrneurol.2015.02.004
Bechara, A., Nawabi, H., Moret, F., Yaron, A., Weaver, E., Bozon, M., et al. (2008). FAK-MAPK-dependent adhesion disassembly downstream of L1 contributes to semaphorin3A-induced collapse. EMBO. J. 27, 1549–1562. doi: 10.1038/emboj.2008.86
Bekirov, I. H., Nagy, V., Svoronos, A., Huntley, G. W., and Benson, D. L. (2008). Cadherin-8 and N-cadherin differentially regulate pre- and postsynaptic development of the hippocampal mossy fiber pathway. Hippocampus 18, 349–363. doi: 10.1002/hipo.20395
Ben Fredj, N., Hammond, S., Otsuna, H., Chien, C. B., Burrone, J., and Meyer, M. P. (2010). Synaptic activity and activity-dependent competition regulates axon arbor maturation, growth arrest, and territory in the retinotectal projection. J. Neurosci. 30, 10939–10951. doi: 10.1523/JNEUROSCI.1556-10.2010
Berns, D. S., DeNardo, L. A., Pederick, D. T., and Luo, L. (2018). Teneurin-3 controls topographic circuit assembly in the hippocampus. Nature 554, 328–333. doi: 10.1038/nature25463
Bock, E. (1991). Cell-cell adhesion molecules. Biochem. Soc. Trans. 19, 1076–1080. doi: 10.1042/bst0191076
Boucard, A. A., Maxeiner, S., and Südhof, T. C. (2014). Latrophilins function as heterophilic cell-adhesion molecules by binding to teneurins: regulation by alternative splicing. J. Biol. Chem. 289, 387–402. doi: 10.1074/jbc.M113.504779
Bruce, F. M., Brown, S., Smith, J. N., Fuerst, P. G., and Erskine, L. (2017). DSCAM promotes axon fasciculation and growth in the developing optic pathway. Proc. Natl. Acad. Sci. USA 114, 1702–1707. doi: 10.1073/pnas.1618606114
Buhusi, M., Demyanenko, G. P., Jannie, K. M., Dalal, J., Darnell, E. P., Weiner, J. A., et al. (2009). ALCAM regulates mediolateral retinotopic mapping in the superior colliculus. J. Neurosci. 50, 15630–15641. doi: 10.1523/JNEUROSCI.2215-09.2009
Castellani, V., Chédotal, A., Schachner, M., Faivre-Sarrailh, C., and Rougon, G. (2000). Analysis of the L1-deficient mouse phenotype reveals cross-talk between Sema3A and L1 signaling pathways in axonal guidance. Neuron 27, 237–249. doi: 10.1016/s0896-6273(00)00033-7
Castellani, V., De Angelis, E., Kenwrick, S., and Rougon, G. (2002). Cis and trans interactions of L1 with neuropilin-1 control axonal responses to semaphorin 3A. EMBO. J. 23, 6348–6357. doi: 10.1093/emboj/cdf645
Castellani, V., Falk, J., and Rougon, G. (2004). Semaphorin3A-induced receptor endocytosis during axon guidance responses is mediated by L1 CAM. Mol. Cell Neurosci. 26, 89–100. doi: 10.1016/j.mcn.2004.01.010
Chandrasekaran, A. R., Plas, D. T., Gonzalez, E., and Crair, M. C. (2005). Evidence for an instructive role of retinal activity in retinotopic map refinement in the superior colliculus of the mouse. J. Neurosci. 25, 6929–6938. doi: 10.1523/JNEUROSCI.1470-05.2005
Charoy, C., Nawabi, H., Reynaud, F., Derrington, E., Bozon, M., Wright, K., et al. (2012). gdnf activates midline repulsion by Semaphorin3B via NCAM during commissural axon guidance. Neuron 75, 1051–1066. doi: 10.1016/j.neuron.2012.08.02
Chen, W. V., Nwakeze, C. L., Denny, C. A., O’Keeffe, S., Rieger, M. A., Mountoufaris, G., et al. (2017). Pcdhαc2 is required for axonal tiling and assembly of serotonergic circuitries in mice. Science 356, 406–411. doi: 10.1126/science.aal3231
Chen, Y. A., Lu, I. L., and Tsai, J. W. (2018). Contactin-1/F3 regulates neuronal migration and morphogenesis through modulating RhoA Activity. Front. Mol. Neurosci. 11:422. doi: 10.3389/fnmol.2018.00422
Cuttler, K., Hassan, M., Carr, J., Cloete, R., and Bardien, S. (2021). Emerging evidence implicating a role for neurexins in neurodegenerative and neuropsychiatric disorders. Open Biol. 11:210091. doi: 10.1098/rsob.210091
Dalla Costa, I., Buchanan, C. N., Zdradzinski, M. D., Sahoo, P. K., Smith, T. P., et al. (2020). The functional organization of axonal mRNA transport and translation. Nat. Rev. Neurosci. 22, 77–91. doi: 10.1038/s41583-020-00407-7
Dang, P., Smythe, E., and Furley, A. J. (2012). TAG1 regulates the endocytic trafficking and signaling of the semaphorin3A receptor complex. J. Neurosci. 32, 10370–10382. doi: 10.1523/JNEUROSCI.5874-11.2012
Demyanenko, G. P., Riday, T. T., Tran, T. S., Dalal, J., Darnell, E. P., Brennaman, L. H., et al. (2011). NrCAM deletion causes topographic mistargeting of thalamocortical axons to the visual cortex and disrupts visual acuity. J. Neurosci. 31, 1545–1558. doi: 10.1523/JNEUROSCI.4467-10.2011
Dharmaratne, N., Glendining, K. A., Young, T. R., Tran, H., Sawatari, A., and Leamey, C. A. (2012). Ten-m3 is required for the development of topography in the ipsilateral retinocollicular pathway. PLoS One 7:e43083. doi: 10.1371/journal.pone.0043083
Di Martino, A., Yan, C. G., Li, Q., Denio, E., Castellanos, F. X., and Alaerts, K. (2014). The autism brain imaging data exchange: towards a large-scale evaluation of the intrinsic brain architecture in autism. Mol. Psychiatry 19, 659–667. doi: 10.1038/mp.2013.78
Dickson, B. J. (2002). Molecular mechanisms of axon guidance. Science 298, 1959–1964. doi: 10.1126/science.1072165
Dipietrantonio, H. J., and Dymecki, S. M. (2009). Zic1 levels regulate mossy fiber neuron position and axon laterality choice in the ventral brain stem. Neuroscience 162, 560–573. doi: 10.1016/j.neuroscience.2009.02.082
Duan, X., Krishnaswamy, A., De la Huerta, I., and Sanes, J. R. (2014). Type II cadherins guide assembly of a direction-selective retinal circuit. Cell 158, 793–807. doi: 10.1016/j.cell.2014.06.047
Duan, X., Krishnaswamy, A., Laboulaye, M. A., Liu, J., Peng, Y. R., Yamagata, M., et al. (2018). Cadherin combinations recruit dendrites of distinct retinal neurons to a shared interneuronal scaffold. Neuron 99, 1145–1154. doi: 10.1016/j.neuron.2018.08.019
Esumi, S., Kakazu, N., Taguchi, Y., Hirayama, T., Sasaki, A., Hirabayashi, T., et al. (2005). Monoallelic yet combinatorial expression of variable exons of the protocadherin-alpha gene cluster in single neurons. Nat. Genet. 37, 171–176. doi: 10.1038/ng1500
Falk, J., Bechara, A., Fiore, R., Nawabi, H., Zhou, H., Hoyo-Becerra, C., et al. (2005). Dual functional activity of semaphorin 3B is required for positioning the anterior commissure. Neuron 48, 63–75. doi: 10.1016/j.neuron.2005.08.033
Ferguson, K., Long, H., Cameron, S., Chang, W. T., and Rao, Y. (2009). The conserved Ig superfamily member Turtle mediates axonal tiling in Drosophila. J. Neurosci. 29, 14151–14159. doi: 10.1523/JNEUROSCI.2497-09.2009
Fitzli, D., Stoeckli, E. T., Kunz, S., Siribour, K., Rader, C., Kunz, B., et al. (2000). A direct interaction of axonin-1 with NgCAM-related cell adhesion molecule (NrCAM) results in guidance, but not growth of commissural axons. J. Cell Biol. 149, 951–968. doi: 10.1083/jcb.149.4.951
Frei, J. A., Andermatt, I., Gesemann, M., and Stoeckli, E. T. (2014). The SynCAM synaptic cell adhesion molecules are involved in sensory axon pathfinding by regulating axon-axon contacts. J. Cell Sci. 127, 5288–5302. doi: 10.1242/jcs.157032
Fuerst, P. G., Bruce, F., Tian, M., Wei, W., Elstrott, J., Feller, M. B., et al. (2009). DSCAM and DSCAML1 function in self-avoidance in multiple cell types in the developing mouse retina. Neuron 64, 484–497. doi: 10.1016/j.neuron.2009.09.027
Fuerst, P. G., Harris, B. S., Johnson, K. R., and Burgess, R. W. (2010). A novel null allele of mouse DSCAM survives to adulthood on an inbred C3H background with reduced phenotypic variability. Genesis 48, 578–584. doi: 10.1002/dvg.20662
Fuerst, P. G., Koizumi, A., Masland, R. H., and Burgess, R. W. (2008). Neurite arborization and mosaic spacing in the mouse retina require DSCAM. Nature 2008, 470–474. doi: 10.1038/nature06514
Garrett, A. M., Khalil, A., Walton, D. O., and Burgess, R. W. (2018). DSCAM promotes self-avoidance in the developing mouse retina by masking the functions of cadherin superfamily members. Proc. Natl. Acad. Sci. USA 115, E10216–E10224. doi: 10.1073/pnas.1809430115
Gatford, N. J. F., Deans, P. J. M., Duarte, R. R. R., Chennell, G., Sellers, K. J., et al. (2021). Neuroligin-3 and Neuroligin-4X form Nanoscopic clusters and regulate growth cone organization and size. Hum. Mol. Genet. 20:ddab277. doi: 10.1093/hmg/ddab277
Gerrow, K., and El-Husseini, A. (2006). Cell adhesion molecules at the synapse. Front. Biosci. 11:2400–2419. doi: 10.2741/1978
Girbes Minguez, M., Wolters-Eisfeld, G., Lutz, D., Buck, F., Schachner, M., and Kleene, R. (2020). The cell adhesion molecule L1 interacts with nuclear proteins via its intracellular domain. FASEB. J. 34, 9869–9883. doi: 10.1096/fj.201902242R
Glendining, K. A., Liu, S. C., Nguyen, M., Dharmaratne, N., Nagarajah, R., Iglesias, M. A., et al. (2017). Downstream mediators of Ten-m3 signalling in the developing visual pathway. BMC Neurosci. 18:78. doi: 10.1186/s12868-017-0397-5
Gomez, A. M., Traunmüller, L., and Scheiffele, P. (2021). Neurexins: molecular codes for shaping neuronal synapses. Nat. Rev. Neurosci. 22, 137–151. doi: 10.1038/s41583-020-00415-7
Gomez, T. M., Roche, F. K., and Letourneau, P. C. (1996). Chick sensory neuronal growth cones distinguish fibronectin from laminin by making substratum contacts that resemble focal contacts. J. Neurobiol. 29, 18–34. doi: 10.1002/(SICI)1097-4695(199601)29:1<18::AID-NEU2>3.0.CO;2-A
Grueber, W., and Sagasti, A. (2010). Self-avoidance and tiling: Mechanisms of dendrite and axon spacing. Cold Spring Harb. Perspect. Biol. 2:a001750. doi: 10.1101/cshperspect.a001750
Hakeda-Suzuki, S., Berger-Müller, S., Tomasi, T., Usui, T., Horiuchi, S. Y., Uemura, T., et al. (2011). Golden Goal collaborates with Flamingo in conferring synaptic-layer specificity in the visual system. Nat. Neurosci. 14, 314–323. doi: 10.1038/nn.2756
Hasegawa, S., Hirabayashi, T., Kondo, T., Inoue, K., Esumi, S., Okayama, A., et al. (2012). Constitutively expressed Protocadherin-α regulates the coalescence and elimination of homotypic olfactory axons through its cytoplasmic region. Front. Mol. Neurosci. 5:97. doi: 10.3389/fnmol.2012.00097
Hattori, D., Demir, E., Kim, H. W., Viragh, E., Zipursky, S. L., and Dickson, B. J. (2007). Dscam diversity is essential for neuronal wiring and self-recognition. Nature 449, 223–227. doi: 10.1038/nature06099
Hayano, Y., Ishino, Y., Hyun, J. H., Orozco, C. G., Steinecke, A., Potts, E., et al. (2021). IgSF11 homophilic adhesion proteins promote layer-specific synaptic assembly of the cortical interneuron subtype. Sci. Adv. 7:eabf1600. doi: 10.1126/sciadv.abf1600
Hayashi, S., Inoue, Y., Kiyonari, H., Abe, T., Misaki, K., Moriguchi, H., et al. (2014). Protocadherin-17 mediates collective axon extension by recruiting actin regulator complexes to interaxonal contacts. Dev. Cell 30, 673–687. doi: 10.1016/j.devcel.2014.07.015
Heggem, M. A., and Bradley, R. S. (2003). The cytoplasmic domain of Xenopus NF-protocadherin interacts with TAF1/set. Dev. Cell 2003, 419–429. doi: 10.1016/s1534-5807(03)00036-4
Herrera, E., Brown, L., Aruga, J., Rachel, R. A., Dolen, G., Mikoshiba, K., et al. (2003). Zic2 patterns binocular vision by specifying the uncrossed retinal projection. Cell 114, 545–557. doi: 10.1016/s0092-8674(03)00684-6
Hidalgo, A., and Brand, A. H. (1997). Targeted neuronal ablation: The role of pioneer neurons in guidance and fasciculation in the CNS of Drosophila. Development 124, 3253–3262. doi: 10.1242/dev.124.17.3253
Hines, J. H., Abu-Rub, M., and Henley, J. R. (2010). Asymmetric endocytosis and remodeling of beta1-integrin adhesions during growth cone chemorepulsion by MAG. Nat. Neurosci. 13, 829–837. doi: 10.1038/nn.2554
Homan, C. C., Pederson, S., To, T. H., Tan, C., Piltz, S., Corbett, M. A., et al. (2018). PCDH19 regulation of neural progenitor cell differentiation suggests asynchrony of neurogenesis as a mechanism contributing to PCDH19 Girls Clustering Epilepsy. Neurobiol. Dis. 116, 106–119. doi: 10.1016/j.nbd.2018.05.004
Homrich, M., Es-Saddiki, F., Gotthard, I., Laurini, C., Stein, E., Wobst, H., et al. (2018). NCAM140 is translocated into the nucleus by an importin-β1-dependent mechanism. Exp. Cell Res. 371, 372–378.
Hong, W., Mosca, T. J., and Luo, L. (2012). Teneurins instruct synaptic partner matching in an olfactory map. Nature 484, 201–207. doi: 10.1038/nature10926
Honig, M. G., and Rutishauser, U. S. (1996). Changes in the segmental pattern of sensory neuron projections in the chick hindlimb under conditions of altered cell adhesion molecule function. Dev. Biol. 175, 325–337. doi: 10.1006/dbio.1996.0118
Honig, M. G., Camilli, S. J., and Xue, Q. S. (2002). Effects of L1 blockade on sensory axon outgrowth and pathfinding in the chick hindlimb. Dev. Biol. 243, 137–154. doi: 10.1006/dbio.2001.0556
Hsieh, H. H., Chang, W. T., Yu, L., and Rao, Y. (2014). Control of axon-axon attraction by Semaphorin reverse signaling. Proc. Natl. Acad. Sci. USA 111, 11383–11388. doi: 10.1073/pnas.1321433111
Huang, R., Yuan, D. J., Li, S., Liang, X. S., Gao, Y., et al. (2020). NCAM regulates temporal specification of neural progenitor cells via profilin2 during corticogenesis. J. Cell Biol. 219:e201902164. doi: 10.1083/jcb.201902164
Huang, Z., Yazdani, U., Thompson-Peer, K. L., Kolodkin, A. L., and Terman, J. R. (2007). Crk-associated substrate (Cas) signaling protein functions with integrins to specify axon guidance during development. Development 134, 2337–2347. doi: 10.1242/dev.004242
Huberman, A. D., Clandinin, T. R., and Baier, H. (2010). Molecular and cellular mechanisms of lamina-specific axon targeting. Cold Spring Harb. Perspect. Biol. 2:a001743. doi: 10.1101/cshperspect.a001743
Hynes, R. O. (2002). Integrins: bidirectional, allosteric signaling machines. Cell 110, 673–687. doi: 10.1016/s0092-8674(02)00971-6
Im, K., Ahtam, B., Haehn, D., Peters, J. M., Warfield, S. K., Sahin, M., et al. (2016). Altered structural brain networks in tuberous sclerosis complex. Cereb. Cortex. 26, 2046–2058. doi: 10.1093/cercor/bhv026
Jaudon, F., Thalhammer, A., and Cingolani, L. A. (2021). Integrin adhesion in brain assembly: from molecular structure to neuropsychiatric disorders. Eur. J. Neurosci. 53, 3831–3850. doi: 10.1111/ejn.14859
Jaworski, A., and Tessier-Lavigne, M. (2012). Autocrine/juxtaparacrine regulation of axon fasciculation by Slit-Robo signaling. Nat. Neurosci. 15, 367–369. doi: 10.1038/nn.3037
Jessen, U., Novitskaya, V., Pedersen, N., Serup, P., Berezin, V., and Bock, E. (2001). The transcription factors CREB and c-Fos play key roles in NCAM-mediated neuritogenesis in PC12-E2 cells. J. Neurochem. 79, 1149–1160. doi: 10.1046/j.1471-4159.2001.00636.x
Kaneko, R., Kato, H., Kawamura, Y., Esumi, S., Hirayama, T., Hirabayashi, T., et al. (2006). Allelic gene regulation of Pcdh-alpha and Pcdh-gamma clusters involving both monoallelic and biallelic expression in single Purkinje cells. J. Biol. Chem. 281, 30551–30560. doi: 10.1074/jbc.M605677200
Kaur, N., Han, W., Li, Z., Madrigal, M. P., Shim, S., Pochareddy, S., et al. (2020). Neural stem cells direct axon guidance via their radial fiber scaffold. Neuron 107, 1197–1211. doi: 10.1016/j.neuron.2020.06.035
Kazarinova-Noyes, K., Malhotra, J. D., McEwen, D. P., Mattei, L. N., Berglund, E. O., Ranscht, B., et al. (2001). Contactin associates with Na+ channels and increases their functional expression. J. Neurosci. 21, 7517–7525. doi: 10.1523/JNEUROSCI.21-19-07517.2001
Kershner, L., and Welshhans, K. (2017). RACK1 is necessary for the formation of point contacts and regulates axon growth. Dev. Neurobiol. 77, 1038–1056. doi: 10.1002/dneu.22491
Kerstein, P. C., Nichol, R. H. I. V., and Gomez, T. M. (2015). Mechanochemical regulation of growth cone motility. Front. Cell Neurosci. 9:244. doi: 10.3389/fncel.2015.00244
Kerstein, P. C., Patel, K. M., and Gomez, T. M. (2017). Calpain-mediated proteolysis of talin and FAK regulates adhesion dynamics necessary for axon guidance. J. Neurosci. 6, 1568–1580. doi: 10.1523/JNEUROSCI.2769-16.2016
Kleene, R., Loers, G., Castillo, G., and Schachner, M. (2022). Cell adhesion molecule L1 interacts with the chromo shadow domain of heterochromatin protein 1 isoforms α, β, and γ via its intracellular domain. FASEB. J. 36:e22074. doi: 10.1096/fj.202100816R
Kleene, R., Mzoughi, M., Joshi, G., Kalus, I., Bormann, U., Schulze, C., et al. (2010). NCAM-induced neurite outgrowth depends on binding of calmodulin to NCAM and on nuclear import of NCAM and fak fragments. J. Neurosci. 30, 10784–10798. doi: 10.1523/JNEUROSCI.0297-10.2010
Kolkova, K., Novitskaya, V., Pedersen, N., Berezin, V., and Bock, E. (2000). Neural cell adhesion molecule-stimulated neurite outgrowth depends on activation of protein kinase C and the Ras-mitogen-activated protein kinase pathway. J. Neurosci. 20, 2238–2246. doi: 10.1523/JNEUROSCI.20-06-02238.2000
Krishnaswamy, A., Yamagata, M., Duan, X., Hong, Y. K., and Sanes, J. R. (2015). Sidekick 2 directs formation of a retinal circuit that detects differential motion. Nature 524, 466–470. doi: 10.1038/nature14682
Kubo, Y., Baba, K., Toriyama, M., Minegishi, T., Sugiura, T., Kozawa, S., et al. (2015). Shootin1-cortactin interaction mediates signal-force transduction for axon outgrowth. J. Cell. Biol. 210, 663–676. doi: 10.1083/jcb.201505011
Kutsarova, E., Munz, M., and Ruthazer, E. S. (2017). Rules for Shaping Neural Connections in the Developing Brain. Front. Neural. Circuits. 10:111. doi: 10.3389/fncir.2016.00111
Kuwajima, T., Yoshida, Y., Takegahara, N., Petros, T. J., Kumanogoh, A., Jessell, T. M., et al. (2012). Optic chiasm presentation of Semaphorin6D in the context of Plexin-A1 and Nr-CAM promotes retinal axon midline crossing. Neuron 74, 676–690. doi: 10.1016/j.neuron.2012.03.025
Kuwako, K. I., Nishimoto, Y., Kawase, S., Okano, H. J., and Okano, H. (2014). Cadherin-7 regulates mossy fiber connectivity in the cerebellum. Cell Rep. 9, 311–323. doi: 10.1016/j.celrep.2014.08.063
Landmesser, L., Dahm, L., Schultz, K., and Rutishauser, U. (1988). Distinct roles for adhesion molecules during innervation of embryonic chick muscle. Dev. Biol. 130, 645–670. doi: 10.1016/0012-1606(88)90358-2
Law, C. O., Kirby, R. J., Aghamohammadzadeh, S., and Furley, A. J. W. (2008). The neural adhesion molecule TAG-1 modulates responses of sensory axons to diffusible guidance signals. Development 135, 2361–2371. doi: 10.1242/dev.009019
Leamey, C. A., Merlin, S., Lattouf, P., Sawatari, A., Zhou, X., Demel, N., et al. (2007). Ten_m3 regulates eye-specific patterning in the mammalian visual pathway and is required for binocular vision. PLoS. Biol. 5:e241. doi: 10.1371/journal.pbio.0050241
Lefebvre, J. L., Kostadinov, D., Chen, W. V., Maniatis, T., and Sanes, J. R. (2012). Protocadherins mediate dendritic self-avoidance in the mammalian nervous system. Nature 488, 517–521. doi: 10.1038/nature11305
Leung, L. C., Urbančič, V., Baudet, M. L., Dwivedy, A., Bayley, T. G., Lee, A. C., et al. (2013). Coupling of NF-protocadherin signaling to axon guidance by cue-induced translation. Nat. Neurosci. 16, 166–173. doi: 10.1038/nn.3290
Leyva-Díaz, E., del Toro, D., Menal, M. J., Cambray, S., Susín, R., Tessier-Lavigne, M., et al. (2014). FLRT3 is a Robo1-interacting protein that determines Netrin-1 attraction in developing axons. Curr. Biol. 24, 494–508. doi: 10.1016/j.cub.2014.01.042
Li, J., Shalev-Benami, M., Sando, R., Jiang, X., Kibrom, A., Wang, J., et al. (2018). Structural basis for teneurin function in circuit-wiring: a toxin motif at the synapse. Cell 173, 735–748. doi: 10.1016/j.cell.2018.03.036
Li, J., Xie, Y., Cornelius, S., Jiang, X., Sando, R., Kordon, S. P., et al. (2020). Alternative splicing controls teneurin-latrophilin interaction and synapse specificity by a shape-shifting mechanism. Nat. Commun. 11:2140. doi: 10.1038/s41467-020-16029-7
Lilienbaum, A., Reszka, A. A., Horwitz, A. F., and Holt, C. E. (1995). Chimeric integrins expressed in retinal ganglion cells impair process outgrowth in vivo. Mol. Cell. Neurosci. 6, 139–152. doi: 10.1006/mcne.1995.1013
Lindenmaier, L. B., Parmentier, N., Guo, C., Tissir, F., and Wright, K. M. (2019). Dystroglycan is a scaffold for extracellular axon guidance decisions. Elife 8:e42143. doi: 10.7554/eLife.42143
Liu, C. J., Dib-Hajj, S. D., Black, J. A., Greenwood, J., Lian, Z., and Waxman, S. G. (2001). Direct interaction with contactin targets voltage-gated sodium channel Na(v)1.9/NaN to the cell membrane. J. Biol. Chem. 276, 46553–46561. doi: 10.1074/jbc.M108699200
Lutz, D., Wolters-Eisfeld, G., Joshi, G., Djogo, N., Jakovcevski, I., Schachner, M., et al. (2012). Generation and nuclear translocation of sumoylated transmembrane fragment of cell adhesion molecule L1. J. Biol. Chem. 287, 17161–17175. doi: 10.1074/jbc.M112.346759
Lutz, D., Wolters-Eisfeld, G., Schachner, M., and Kleene, R. (2014). Cathepsin E generates a sumoylated intracellular fragment of the cell adhesion molecule L1 to promote neuronal and Schwann cell migration as well as myelination. J. Neurochem. 128, 713–724. doi: 10.1111/jnc.12473
Luxey, M., Jungas, T., Laussu, J., Audouard, C., Garces, A., and Davy, A. (2013). Eph:ephrin-B1 forward signaling controls fasciculation of sensory and motor axons. Dev. Biol. 383, 264–274. doi: 10.1016/j.ydbio.2013.09.010
Marcus, R. C., Blazeski, R., Godement, P., and Mason, C. A. (1995). Retinal axon divergence in the optic chiasm: uncrossed axons diverge from crossed axons within a midline glial specialization. J. Neurosci. 5, 3716–3729. doi: 10.1523/JNEUROSCI.15-05-03716.1995
Marthiens, V., Gavard, J., Padilla, F., Monnet, C., Castellani, V., Lambert, M., et al. (2005). A novel function for cadherin-11 in the regulation of motor axon elongation and fasciculation. Mol. Cell. Neurosci. 28, 715–726. doi: 10.1016/j.mcn.2004.12.001
Matthews, B. J., Kim, M. E., Flanagan, J. J., Hattori, D., Clemens, J. C., Zipursky, S. L., et al. (2007). Dendrite self-avoidance is controlled by Dscam. Cell 129, 593–604. doi: 10.1016/j.cell.2007.04.013
McFadden, K., and Minshew, N. J. (2013). Evidence for dysregulation of axonal growth and guidance in the etiology of ASD. Front. Hum. Neurosci. 7:671. doi: 10.3389/fnhum.2013.00671
Millard, S. S., Flanagan, J. J., Pappu, K. S., Wu, W., and Zipursky, S. L. (2007). Dscam2 mediates axonal tiling in the Drosophila visual system. Nature 447, 720–724. doi: 10.1038/nature05855
Mishra, Y. G., and Manavathi, B. (2021). Focal adhesion dynamics in cellular function and disease. Cell Signal. 85:110046. doi: 10.1016/j.cellsig.2021.110046
Missaire, M., and Hindges, R. (2015). The role of cell adhesion molecules in visual circuit formation: from neurite outgrowth to maps and synaptic specificity. Dev. Neurobiol. 75, 569–583. doi: 10.1002/dneu.22267
Mosca, T. J., Hong, W., Dani, V. S., Favaloro, V., and Luo, L. (2012). Trans-synaptic Teneurin signalling in neuromuscular synapse organization and target choice. Nature 484, 237–241. doi: 10.1038/nature10923
Mountoufaris, G., Chen, W. V., Hirabayashi, Y., O’Keeffe, S., Chevee, M., Nwakeze, C. L., et al. (2017). Multicluster Pcdh diversity is required for mouse olfactory neural circuit assembly. Science 356, 411–414. doi: 10.1126/science.aai8801
Myers, J. P., and Gomez, T. M. (2011). Focal adhesion kinase promotes integrin adhesion dynamics necessary for chemotropic turning of nerve growth cones. J. Neurosci. 31, 13585–13595. doi: 10.1523/JNEUROSCI.2381-11.2011
Nakamoto, T., Kain, K. H., and Ginsberg, M. H. (2004). Neurobiology: New connections between integrins and axon guidance. Curr. Biol. 14, R121–R123. doi: 10.1016/j.cub.2004.01.020
Nelson, W. J., and Nusse, R. (2004). Convergence of Wnt, beta-catenin, and cadherin pathways. Science 303, 1483–1487. doi: 10.1126/science.1094291
Neugebauer, K. M., Tomaselli, K. J., Lilien, J., and Reichardt, L. F. (1988). N-cadherin, NCAM, and integrins promote retinal neurite outgrowth on astrocytes in vitro. J. Cell Biol. 107, 1177–1187. doi: 10.1083/jcb.107.3.1177
Nichol, R. H., Hagen, K. M., Lumbard, D. C., Dent, E. W., and Gómez, T. M. (2016). Guidance of axons by local coupling of retrograde flow to point contact adhesions. J. Neurosci. 36, 2267–2282. doi: 10.1523/JNEUROSCI.2645-15.2016
Nicholls, J. G., and Baylor, D. A. (1968). Specific modalities and receptive fields of sensory neurons in CNS of the leech. J. Neurophysiol. 31, 740–756. doi: 10.1152/jn.1968.31.5.740
Niethammer, P., Delling, M., Sytnyk, V., Dityatev, A., Fukami, K., and Schachner, M. (2002). Cosignaling of NCAM via lipid rafts and the FGF receptor is required for neuritogenesis. J. Cell Biol. 157, 521–532. doi: 10.1083/jcb.200109059
Nunes, S. M., Ferralli, J., Choi, K., Brown-Luedi, M., Minet, A. D., and Chiquet-Ehrismann, R. (2005). The intracellular domain of teneurin-1 interacts with MBD1 and CAP/ponsin resulting in subcellular codistribution and translocation to the nuclear matrix. Exp. Cell Res. 305, 122–132. doi: 10.1016/j.yexcr.2004.12.020
Okumura, M., Kato, T., Miura, M., and Chihara, T. (2016). Hierarchical axon targeting of Drosophila olfactory receptor neurons specified by the proneural transcription factors Atonal and Amos. Genes Cell. Devot. Mol. Cell Mech. 21, 53–64. doi: 10.1111/gtc.12321
Osterhout, J. A., Josten, N., Yamada, J., Pan, F., Wu, S. W., Nguyen, P. L., et al. (2011). Cadherin-6 mediates axon-target matching in a non-image-forming visual circuit. Neuron 71, 632–639. doi: 10.1016/j.neuron.2011.07.006
Ott, H., Bastmeyer, M., and Stuermer, C. A. (1998). Neurolin, the goldfish homolog of DM-GRASP, is involved in retinal axon pathfinding to the optic disk. J. Neurosci. 18, 3363–3372. doi: 10.1523/jneurosci.18-09-03363.1998
Pederick, D. T., Lui, J. H., Gingrich, E. C., Xu, C., Wagner, M. J., Liu, Y., et al. (2021). Reciprocal repulsions instruct the precise assembly of parallel hippocampal networks. Science 372, 1068–1073. doi: 10.1126/science.abg1774
Peng, Y. R., Tran, N. M., Krishnaswamy, A., Kostadinov, D., Martersteck, E. M., and Sanes, J. R. (2017). Satb1 regulates contactin 5 to pattern dendrites of a mammalian retinal ganglion cell. Neuron 95, 869–883. doi: 10.1016/j.neuron.2017.07.019
Petrovic, M., and Hummel, T. (2008). Temporal identity in axonal target layer recognition. Nature 456, 800–803. doi: 10.1038/nature07407
Pierre, K., Dupouy, B., Allard, M., Poulain, D. A., and Theodosis, D. T. (2001). Mobilization of the cell adhesion glycoprotein F3/contactin to axonal surfaces is activity dependent. Eur. J. Neurosci. 14, 645–656. doi: 10.1046/j.0953-816x.2001.01682.x
Pike, S. H., Melancon, E. F., and Eisen, J. S. (1992). Pathfinding by zebrafish motoneurons in the absence of normal pioneer axons. Development 114, 825–831. doi: 10.1242/dev.114.4.825
Piper, M., Dwivedy, A., Leung, L., Bradley, R. S., and Holt, C. E. (2008). NF-protocadherin and TAF1 regulate retinal axon initiation and elongation in vivo. J. Neurosci. 28, 100–105. doi: 10.1523/JNEUROSCI.4490-07.2008
Pittman, A. J., Law, M. Y., and Chien, C. B. (2008). Pathfinding in a large vertebrate axon tract: Isotypic interactions guide retinotectal axons at multiple choice points. Development 135, 2865–2871. doi: 10.1242/dev.025049
Pollerberg, G. E., and Mack, T. G. (1994). Cell adhesion molecule SC1/DMGRASP is expressed on growing axons of retina ganglion cells and is involved in mediating their extension on axons. Dev. Biol. 165, 670–687. doi: 10.1006/dbio.1994.1284
Pollerberg, G. E., Thelen, K., Theiss, M. O., and Hochlehnert, B. C. (2013). The role of cell adhesion molecules for navigating axons: density matters. Mech. Dev. 130, 359–372. doi: 10.1016/j.mod.2012.11.002
Poplawski, G. H., Tranziska, A. K., Leshchyns’ka, I., Meier, I. D., Streichert, T., et al. (2012). L1CAM increases MAP2 expression via the MAPK pathway to promote neurite outgrowth. Mol. Cell Neurosci. 50, 169–178. doi: 10.1016/j.mcn.2012.03.010
Poulain, F. E., and Chien, C. B. (2013). Proteoglycan-mediated axon degeneration corrects pretarget topographic sorting errors. Neuron 78, 49–56. doi: 10.1016/j.neuron.2013.02.005
Purohit, A. A., Li, W., Qu, C., Dwyer, T., Shao, Q., Guan, K. L., et al. (2012). Down syndrome cell adhesion molecule (DSCAM) associates with uncoordinated-5C (UNC5C) in netrin-1-mediated growth cone collapse. J. Biol. Chem. 287, 27126–27138. doi: 10.1074/jbc.M112.340174
Rasband, M. N., and Peles, E. (2021). Mechanisms of node of Ranvier assembly. Nat. Rev. Neurosci. 22, 7–20. doi: 10.1038/s41583-020-00406-8
Rash, B. G., and Richards, L. J. (2001). A role for cingulate pioneering axons in the development of the corpus callosum. J. Comp. Neurol. 434, 147–157. doi: 10.1002/cne.1170
Riccomagno, M. M., and Kolodkin, A. L. (2015). Sculpting neural circuits by axon and dendrite pruning. Annu. Rev. Cell Dev. Biol. 31, 779–805. doi: 10.1146/annurev-cellbio-100913-013038
Rigby, M. J., Gomez, T. M., and Puglielli, L. (2020). Glial cell-axonal growth cone interactions in neurodevelopment and regeneration. Front. Neurosci. 14:203. doi: 10.3389/fnins.2020.00203
Rutishauser, U., Thiery, J. P., Brackenbury, R., Sela, B. A., and Edelman, G. M. (1976). Mechanisms of adhesion among cells from neural tissues of the chick embryo. Proc. Natl. Acad. Sci. USA 73, 577–581. doi: 10.1073/pnas.73.2.577
Sachse, S. M., Lievens, S., Ribeiro, L. F., Dascenco, D., Masschaele, D., et al. (2019). Nuclear import of the DSCAM-cytoplasmic domain drives signaling capable of inhibiting synapse formation. EMBO. J. 38:e99669. doi: 10.15252/embj.201899669
Saftig, P., and Lichtenthaler, S. F. (2015). The alpha secretase ADAM10: A metalloprotease with multiple functions in the brain. Prog. Neurobiol. 135, 1–20. doi: 10.1016/j.pneurobio.2015.10.003
Sagasti, A., Guido, M. R., Raible, D. W., and Schier, A. F. (2005). Repulsive interactions shape the morphologies and functional arrangement of zebrafish peripheral sensory arbors. Curr. Biol. 15, 804–814. doi: 10.1016/j.cub.2005.03.048
Sakurai, T. (2017). The role of cell adhesion molecules in brain wiring and neuropsychiatric disorders. Mol. Cell Neurosci. 81, 4–11. doi: 10.1016/j.mcn.2016.08.005
Sánchez-Camacho, C., and Bovolenta, P. (2009). Emerging mechanisms in morphogen-mediated axon guidance. Bioessays 31, 1013–1025. doi: 10.1002/bies.200900063
Sando, R., Jiang, X., and Südhof, T. C. (2019). Latrophilin GPCRs direct synapse specificity by coincident binding of FLRTs and teneurins. Science 363:eaav7969. doi: 10.1126/science.aav7969
Sanes, J. R., and Zipursky, S. L. (2020). Synaptic specificity, recognition molecules, and assembly of neural circuits. Cell 181, 536–556. doi: 10.1016/j.cell.2020.04.008
Schaefer, A. W., Kamiguchi, H., Wong, E. V., Beach, C. M., Landreth, G., and Lemmon, V. (1999). Activation of the MAPK signal cascade by the neural cell adhesion molecule L1 requires L1 internalization. J. Biol. Chem. 274, 37965–37973. doi: 10.1074/jbc.274.53.37965
Schmid, R. S., and Maness, P. F. (2008). L1 and NCAM adhesion molecules as signaling coreceptors in neuronal migration and process outgrowth. Curr. Opin. Neurobiol. 18, 245–250. doi: 10.1016/j.conb.2008.07.015
Schmid, R. S., Pruitt, W. M., and Maness, P. F. (2000). A MAP kinase-signaling pathway mediates neurite outgrowth on L1 and requires Src-dependent endocytosis. J. Neurosci. 20, 4177–4188. doi: 10.1523/JNEUROSCI.20-11-04177.2000
Schmucker, D., Clemens, J. C., Shu, H., Worby, C. A., Xiao, J., Muda, M., et al. (2000). Drosophila Dscam is an axon guidance receptor exhibiting extraordinary molecular diversity. Cell 101, 671–684. doi: 10.1016/s0092-8674(00)80878-8
Schöler, J., Ferralli, J., Thiry, S., and Chiquet-Ehrismann, R. (2015). The intracellular domain of teneurin-1 induces the activity of microphthalmia-associated transcription factor (MITF) by binding to transcriptional repressor HINT1. J. Biol. Chem. 290, 8154–8165. doi: 10.1074/jbc.M114.615922
Schroeder, A., and de Wit, J. (2018). Leucine-rich repeat-containing synaptic adhesion molecules as organizers of synaptic specificity and diversity. Exp. Mol. Med. 50, 1–9. doi: 10.1038/s12276-017-0023-8
Schuldiner, O., and Yaron, A. (2015). Mechanisms of developmental neurite pruning. Cell Mol. Life Sci. 72, 101–119. doi: 10.1007/s00018-014-1729-6
Siegenthaler, D., Enneking, E. M., Moreno, E., and Pielage, J. (2015). L1CAM/Neuroglian controls the axon-axon interactions establishing layered and lobular mushroom body architecture. J. Cell Biol. 208, 1003–1018.
Simmons, A. B., Bloomsburg, S. J., Sukeena, J. M., Miller, C. J., Ortega-Burgos, Y., Borghuis, B. G., et al. (2017). DSCAM-mediated control of dendritic and axonal arbor outgrowth enforces tiling and inhibits synaptic plasticity. Proc. Natl. Acad. Sci. USA 114, E10224–E10233. doi: 10.1073/pnas.1713548114
Šmít, D., Fouquet, C., Pincet, F., Zapotocky, M., and Trembleau, A. (2017). Axon tension regulates fasciculation/defasciculation through the control of axon shaft zippering. Elife 6:e19907. doi: 10.7554/eLife.19907
Soba, P., Zhu, S., Emoto, K., Younger, S., Yang, S. J., Yu, H. H., et al. (2007). Drosophila sensory neurons require Dscam for dendritic self-avoidance and proper dendritic field organization. Neuron 54, 403–416. doi: 10.1016/j.neuron.2007.03.029
Solecki, D. J. (2012). Sticky situations: recent advances in control of cell adhesion during neuronal migration. Curr. Opin. Neurobiol. 5, 791–798. doi: 10.1016/j.conb.2012.04.010
Spead, O., and Poulain, F. E. (2021). Trans-axonal signaling in neural circuit wiring. Int. J. Mol. Sci. 21:5170. doi: 10.3390/ijms21145170
Spead, O., Weaver, C. J., Moreland, T., and Poulain, F. E. (2021). Live imaging of retinotectal mapping reveals topographic map dynamics and a previously undescribed role for Contactin 2 in map sharpening. Development 148:dev199584. doi: 10.1242/dev.199584
Stellwagen, D., and Shatz, C. J. (2002). An instructive role for retinal waves in the development of retinogeniculate connectivity. Neuron 33, 357–367. doi: 10.1016/s0896-6273(02)00577-9
Stoeckli, E. T., Sonderegger, P., Pollerberg, G. E., and Landmesser, L. T. (1997). Interference with axonin-1 and NrCAM interactions unmasks a floor-plate activity inhibitory for commissural axons. Neuron 18, 209–221. doi: 10.1016/s0896-6273(00)80262-7
Su, J., Sabbagh, U., Liang, Y., Olejníková, L., Dixon, K. G., Russell, A. L., et al. (2021). A cell-ECM mechanism for connecting the ipsilateral eye to the brain. Proc. Natl. Acad. Sci. USA 118:e2104343118. doi: 10.1073/pnas.2104343118
Su, Z., and He, C. (2010). Olfactory ensheathing cells: biology in neural development and regeneration. Prog. Neurobiol. 9, 517–532. doi: 10.1016/j.pneurobio.2010.08.008
Südhof, T. C. (2021). The cell biology of synapse formation. J. Cell Biol. 220:e202103052. doi: 10.1083/jcb.202103052
Sullivan, C. S., Kümper, M., Temple, B. S., and Maness, P. F. (2016). The neural cell adhesion molecule (NCAM) promotes clustering and activation of EphA3 Receptors in GABAergic interneurons to induce ras homolog gene family, member A (RhoA)/Rho-associated protein kinase (ROCK)-mediated Growth Cone Collapse. J. Biol. Chem. 51, 26262–26272. doi: 10.1074/jbc.M116.760017
Suter, T., Blagburn, S. V., Fisher, S. E., Anderson-Keightly, H. M., D’Elia, K. P., and Jaworski, A. (2020). TAG-1 multifunctionality coordinates neuronal migration, axon guidance, and fasciculation. Cell Rep. 30, 1164–1177. doi: 10.1016/j.celrep.2019.12.085
Suzuki, N., Numakawa, T., Chou, J., de Vega, S., Mizuniwa, C., and Sekimoto, K. (2014). Teneurin-4 promotes cellular protrusion formation and neurite outgrowth through focal adhesion kinase signaling. FASEB J. 28, 1386–9137. doi: 10.1096/fj.13-241034
Swanson, M. R., Wolff, J. J., Shen, M. D., Styner, M., Estes, A., Gerig, G., et al. (2018). Development of white matter circuitry in infants with fragile X Syndrome. JAMA Psychiatry 75, 505–513. doi: 10.1001/jamapsychiatry.2018.0180
Tai, Y., Gallo, N. B., Wang, M., Yu, J. R., and Van Aelst, L. (2019). Axo-axonic innervation of neocortical pyramidal neurons by GABAergic chandelier cells requires ankyring-associated L1CAM. Neuron 102, 358–372. doi: 10.1016/j.neuron.2019.02.009
Tasic, B., Nabholz, C. E., Baldwin, K. K., Kim, Y., Rueckert, E. H., Ribich, S. A., et al. (2002). Promoter choice determines splice site selection in protocadherin alpha and gamma pre-mRNA splicing. Mol. Cell. 10, 21–33. doi: 10.1016/s1097-2765(02)00578-6
Tessier-Lavigne, M., and Goodman, C. S. (1996). The molecular biology of axon guidance. Science 274, 1123–1133. doi: 10.1126/science.274.5290.1123
Thelen, K., Maier, B., Faber, M., Albrecht, C., Fischer, P., and Pollerberg, G. E. (2012). Translation of the cell adhesion molecule ALCAM in axonal growth cones-Regulation and functional importance. J. Cell Sci. 125, 1003–1014. doi: 10.1242/jcs.096149
Thu, C. A., Chen, W. V., Rubinstein, R., Chevee, M., Wolcott, H. N., Felsovalyi, K. O., et al. (2014). Single-cell identity generated by combinatorial homophilic interactions between α, β, and γ protocadherins. Cell 158, 1045–1059. doi: 10.1016/j.cell.2014.07.012
Tomasi, T., Hakeda-Suzuki, S., Ohler, S., Schleiffer, A., and Suzuki, T. (2008). The transmembrane protein Golden goal regulates R8 photoreceptor axon-axon and axon-target interactions. Neuron 57, 691–704. doi: 10.1016/j.neuron.2008.01.012
Treubert-Zimmermann, U., Heyers, D., and Redies, C. (2002). Targeting axons to specific fiber tracts in vivo by altering cadherin expression. J. Neurosci. 22, 7617–7626. doi: 10.1523/JNEUROSCI.22-17-07617.2002
Vysokov, N. V., Silva, J. P., Lelianova, V. G., Ho, C., Djamgoz, M. B., Tonevitsky, A. G., et al. (2016). The mechanism of regulated release of lasso/Teneurin-2. Front. Mol. Neurosci. 9:59. doi: 10.3389/fnmol.2016.00059
Vysokov, N. V., Silva, J. P., Lelianova, V. G., Suckling, J., Cassidy, J., Blackburn, J. K., et al. (2018). Proteolytically released Lasso/teneurin-2 induces axonal attraction by interacting with latrophilin-1 on axonal growth cones. Elife 7:e37935. doi: 10.7554/eLife.37935
Wang, J., Zugates, C. T., Liang, I. H., Lee, C. H., and Lee, T. (2002). Drosophila Dscam is required for divergent segregation of sister branches and suppresses ectopic bifurcation of axons. Neuron 33, 559–571. doi: 10.1016/s0896-6273(02)00570-6
Wang, X., Su, H., and Bradley, A. (2002). Molecular mechanisms governing Pcdh-gamma gene expression: evidence for a multiple promoter and cis-alternative splicing model. Genes Dev. 16, 1890–1905. doi: 10.1101/gad.1004802
Weiner, J. A., Koo, S. J., Nicolas, S., Fraboulet, S., Pfaff, S. L., Pourquié, O., et al. (2004). Axon fasciculation defects and retinal dysplasias in mice lacking the immunoglobulin superfamily adhesion molecule BEN/ALCAM/SC1. Mol. Cell. Neurosci. 27, 59–69. doi: 10.1016/j.mcn.2004.06.005
Westphal, N., Kleene, R., Lutz, D., Theis, T., and Schachner, M. (2016). Polysialic acid enters the cell nucleus attached to a fragment of the neural cell adhesion molecule NCAM to regulate the circadian rhythm in mouse brain. Mol. Cell Neurosci. 74, 114–127. doi: 10.1016/j.mcn.2016.05.003
Westphal, N., Loers, G., Lutz, D., Theis, T., Kleene, R., and Schachner, M. (2017a). Generation and intracellular trafficking of a polysialic acid-carrying fragment of the neural cell adhesion molecule NCAM to the cell nucleus. Sci. Rep. 7:8622. doi: 10.1038/s41598-017-09468-8
Westphal, N., Theis, T., Loers, G., Schachner, M., and Kleene, R. (2017b). Nuclear fragments of the neural cell adhesion molecule NCAM with or without polysialic acid differentially regulate gene expression. Sci. Rep. 7:13631. doi: 10.1038/s41598-017-14056-x
Whitlock, K. E., and Westerfield, M. A. (1998). transient population of neurons pioneers the olfactory pathway in the zebrafish. J. Neurosci. 18, 8919–8927. doi: 10.1523/jneurosci.18-21-08919.1998
Widjaja, E., Simao, G., Mahmoodabadi, S. Z., Ochi, A., Snead, O. C., Rutka, J., et al. (2010). Diffusion tensor imaging identifies changes in normal-appearing white matter within the epileptogenic zone in tuberous sclerosis complex. Epilepsy Res. 89, 246–253. doi: 10.1016/j.eplepsyres.2010.01.008
Williams, M. E., Wilke, S. A., Daggett, A., Davis, E., Otto, S., Ravi, D., et al. (2011). Cadherin-9 regulates synapse-specific differentiation in the developing hippocampus. Neuron 71, 640–655. doi: 10.1016/j.neuron.2011.06.019
Williams, S. E., Grumet, M., Colman, D. R., Henkemeyer, M., Mason, C. A., and Sakurai, T. (2006). A role for Nr-CAM in the patterning of binocular visual pathways. Neuron 50, 535–547. doi: 10.1016/j.neuron.2006.03.037
Wojtowicz, W. M., Flanagan, J. J., Millard, S. S., Zipursky, S. L., and Clemens, J. C. (2004). Alternative splicing of Drosophila Dscam generates axon guidance receptors that exhibit isoform-specific homophilic binding. Cell 118, 619–633. doi: 10.1016/j.cell.2004.08.021
Wojtowicz, W. M., Wu, W., Andre, I., Qian, B., Baker, D., and Zipursky, S. L. (2007). A vast repertoire of Dscam binding specificities arises from modular interactions of variable Ig domains. Cell 130, 1134–1145. doi: 10.1016/j.cell.2007.08.026
Wolman, M. A., Regnery, A. M., Becker, T., Becker, C. G., and Halloran, M. C. (2007). Semaphorin3D regulates axon axon interactions by modulating levels of L1 cell adhesion molecule. J. Neurosci. 27, 9653–9663. doi: 10.1523/jneurosci.1741-07.2007
Woo, S., and Gomez, T. M. (2006). Rac1 and RhoA promote neurite outgrowth through formation and stabilization of growth cone point contacts. J. Neurosci. 26, 1418–1428. doi: 10.1523/JNEUROSCI.4209-05.2006
Wu, Q., and Maniatis, T. (1999). A striking organization of a large family of human neural cadherin-like cell adhesion genes. Cell 97, 779–790. doi: 10.1016/s0092-8674(00)80789-8
Yam, P. T., and Charron, F. (2013). Signaling mechanisms of non-conventional axon guidance cues: the Shh, BMP and Wnt morphogens. Curr. Opin. Neurobiol. 23, 965–973. doi: 10.1016/j.conb.2013.09.002
Yamagata, M., and Sanes, J. R. (2008). Dscam and Sidekick proteins direct lamina-specific synaptic connections in vertebrate retina. Nature 451, 465–469. doi: 10.1038/nature06469
Yamagata, M., and Sanes, J. R. (2012). Expanding the Ig superfamily code for laminar specificity in retina: expression and role of contactins. J. Neurosci. 32, 14402–14414. doi: 10.1523/JNEUROSCI.3193-12.2012
Yamagata, M., and Sanes, J. R. (2019). Expression and roles of the immunoglobulin superfamily recognition molecule sidekick1 in mouse retina. Front. Mol. Neurosci. 11:485. doi: 10.3389/fnmol.2018.00485
Yamagata, M., Weiner, J. A., and Sanes, J. R. (2002). Sidekicks: synaptic adhesion molecules that promote lamina-specific connectivity in the retina. Cell 110, 649–660. doi: 10.1016/s0092-8674(02)00910-8
Yamagishi, S., Hampel, F., Hata, K., Del Toro, D., Schwark, M., Kvachnina, E., et al. (2011). FLRT2 and FLRT3 act as repulsive guidance cues for Unc5-positive neurons. EMBO. J. 30, 2920–2933. doi: 10.1038/emboj.2011.189
Yogev, S., and Shen, K. (2014). Cellular and molecular mechanisms of synaptic specificity. Annu. Rev. Cell. Dev. Biol. 30, 417–437. doi: 10.1146/annurev-cellbio-100913-012953
Keywords: growth cone, axon targeting, pathfinding, synaptic specificity, signaling, contact
Citation: Moreland T and Poulain FE (2022) To Stick or Not to Stick: The Multiple Roles of Cell Adhesion Molecules in Neural Circuit Assembly. Front. Neurosci. 16:889155. doi: 10.3389/fnins.2022.889155
Received: 03 March 2022; Accepted: 28 March 2022;
Published: 28 April 2022.
Edited by:
Robert Hindges, King’s College London, United KingdomReviewed by:
Esther Stoeckli, University of Zurich, SwitzerlandCopyright © 2022 Moreland and Poulain. This is an open-access article distributed under the terms of the Creative Commons Attribution License (CC BY). The use, distribution or reproduction in other forums is permitted, provided the original author(s) and the copyright owner(s) are credited and that the original publication in this journal is cited, in accordance with accepted academic practice. No use, distribution or reproduction is permitted which does not comply with these terms.
*Correspondence: Fabienne E. Poulain, ZnBvdWxhaW5AbWFpbGJveC5zYy5lZHU=
Disclaimer: All claims expressed in this article are solely those of the authors and do not necessarily represent those of their affiliated organizations, or those of the publisher, the editors and the reviewers. Any product that may be evaluated in this article or claim that may be made by its manufacturer is not guaranteed or endorsed by the publisher.
Research integrity at Frontiers
Learn more about the work of our research integrity team to safeguard the quality of each article we publish.