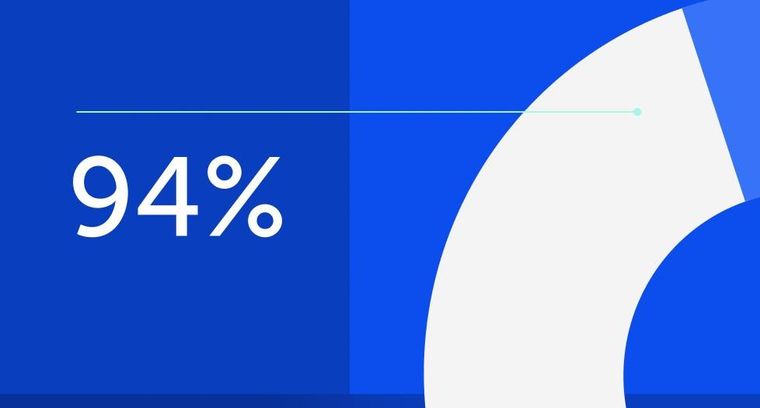
94% of researchers rate our articles as excellent or good
Learn more about the work of our research integrity team to safeguard the quality of each article we publish.
Find out more
REVIEW article
Front. Neurosci., 24 June 2022
Sec. Neurodegeneration
Volume 16 - 2022 | https://doi.org/10.3389/fnins.2022.882316
This article is part of the Research TopicGliopathies in Aging-Related Brain Diseases: from Understanding to TherapyView all 6 articles
Despite the discovery of numerous molecules and pathologies, the pathophysiology of various neurodegenerative diseases remains unknown. Genetics participates in the pathogenesis of neurodegeneration. Neural dysfunction, which is thought to be a cell-autonomous mechanism, is insufficient to explain the development of neurodegenerative disease, implying that other cells surrounding or related to neurons, such as glial cells, are involved in the pathogenesis. As the primary component of glial cells, astrocytes play a variety of roles in the maintenance of physiological functions in neurons and other glial cells. The pathophysiology of neurodegeneration is also influenced by reactive astrogliosis in response to central nervous system (CNS) injuries. Furthermore, those risk-gene variants identified in neurodegenerations are involved in astrocyte activation and senescence. In this review, we summarized the relationships between gene variants and astrocytes in four neurodegenerative diseases, including Alzheimer’s disease (AD), amyotrophic lateral sclerosis (ALS), frontotemporal dementia (FTD), and Parkinson’s disease (PD), and provided insights into the implications of astrocytes in the neurodegenerations.
Neurodegenerative diseases of the central nervous system (CNS) are a group of disorders characterized by chronic progressive damage of neurons with high heterogeneity. Because of the complex mechanisms of neurodegeneration, there are currently no effective treatments. Currently, environmental and genetic factors are both thought to be responsible for neural death. Several studies have confirmed that a large number of genes, such as the apolipoprotein E gene (APOE) in Alzheimer’s disease (AD), α-synuclein gene (SNCA) in Parkinson’s disease (PD), Cu/Zn-superoxide dismutase 1 gene (SOD1) in amyotrophic lateral sclerosis (ALS), and microtubule-associated protein tubulin-associated unit (Tau) gene (MAPT) in frontotemporal dementia (FTD), are pathogenic or risk to diseases. The discovery of genes in neurodegenerations have advanced the exploration of disease pathogenesis. Some gene–target therapies have been conducted in clinical research such as antisense oligonucleotide (ASO) silencing for SOD1 mutation, fused in sarcoma gene (FUS) mutation in ALS (Miller et al., 2020; Korobeynikov et al., 2022), as well as targeting protein apoE with antibodies to inhibit amyloid accumulation in AD (Liao et al., 2018). The majority of research on pathogenic or risk gene variants involved in neurodegenerative disorders has focused on neural changes and dysfunction. However, neural dysfunctions, such as oxidative stress damage, protein aggregation, and dysregulated RNA metabolism are insufficient to explain the development and heterogeneity of various diseases. Dysregulation of non-neural cells, such as glial cells, provides an opportunity to gain a better understanding of the pathogenic process of neural death, allowing for more effective target therapies in neurodegenerations.
Astrocytes are the most numerous subsets of glial cells, accounting for at least half of the cells in the brain and spinal cord. Astrocytes are highly differentiated cells that are connected by gap junctions (Liddelow et al., 2020). Astrocytes serve as a barrier, a source of nutrients for neurons, and assist in maintaining synaptic function by regulating ion homeostasis and neurotransmitters. Astrocytes dysfunction, such as reactive and senescent astrocytes that are influenced by both acute stress and chronic inflammatory or degenerative changes, causes the neuron to lose support accelerating neural death (Escartin et al., 2021). Therefore, exploring the relationship between astrocytes and neurodegenerations, particularly pathogenic or risk-gene variants in neurodegenerative diseases, provides a new perspective on disease mechanism.
In this review, we generalized the basic role of reactive astrocytes in neurodegeneration and described the relationships between astrocytes and pathogenic or risk genes in four neurodegenerative diseases (AD, ALS, FTD, and PD). We also discussed the potential roles of astrocytes in the diagnosis and treatment of neurodegeneration.
Astrocytes change morphology and functions in response to CNS injury or disease, known as reactive astrogliosis, and these cells are regarded as reactive astrocytes. Astrogliosis is defined as morphological hypertrophy, proliferation, and increased levels of specific protein markers in astrocytes, such as glial fibrillary acidic protein (GFAP), vimentin, and nestin (Strohm and Behrends, 2020). Reactive astrocytes are classified into two types: A1 for expressing neurotoxic and pro-inflammatory cytokines and A2 for producing neurotrophic factors and recovering synapses. This classification, however, does not cover all conditions and functions of reactive astrocytes. Neurotoxic factors and the loss of supportive functions from reactive astrocytes accelerate neural death via different mechanisms (Valori et al., 2014).
First, astrocytes and microglia collaborate in the immune response to stress in CNS. Astrocytes produce chemokines and cytokines that recruit leukocytes and boost local immune responses to protect the surrounding neurons (Castellani and Schwartz, 2020). In response to acute or chronic stress, nuclear translocation of NF-κB, which is activated by microgliosis, induces the inflammatory factors from reactive astrocytes. Meanwhile, reactive astrocytes produce inflammatory factors, such as tumor necrosis factor-alpha (TNF-α), interleukin-1 beta (IL-1β), and IL-17, which accelerates the activation of NF-κB and causes neurotoxicity (Kam et al., 2020; Linnerbauer et al., 2020; Strohm and Behrends, 2020).
Second, astrocytes aid in the maintenance of blood brain barrier (BBB) homeostasis. Astrocytes maintain a portion of their protective effect on BBB integrity by secreting anti-inflammatory factors such as sonic hedgehog, angiopoietin-1, retinoic acid, and insulin-like growth factor-1 to anti-inflammation, and prostaglandins, nitric oxide, or arachidonic acid to regulate cerebrovascular flow (Wei and Shetty, 2021; Zhao et al., 2021). Furthermore, in response to disease, reactive astrocytes play a role in repairing damaged BBB and promoting angiogenesis (Li et al., 2022). Dysregulated reactive astrocytes, on the other hand, destroy the structure of BBB by producing factors to decrease the permeability of BBB. Furthermore, the physical structure of BBB, which is composed of astrocyte end-feet and endothelial cells, is destroyed in reactive astrocytes (Mills et al., 2022). Glial cells-induced neuroinflammation exacerbates BBB damage and reduces its integrity.
Third, rather than neurons, astrocytes perform a large amount of glutamate reuptake via transporters such as excitatory amino acid transporter-1, -2 (EAAT-1 and EAAT-2), and glutamate is then metabolized into glutamine in astrocytes (Bantle et al., 2020). Astrocytes also increase glucose metabolism to assist glutamine release from neurons. Astrocytes absorb glucose from blood vessels and produce lactate (Strohm and Behrends, 2020). Mesencephalic astrocyte-derived neurotrophic factor (MANF) is involved in glucose homeostasis and energy metabolism (Wei and Shetty, 2021). Astrocytes protect neurons from fatty acid toxicity and provide energy to neurons via fatty acid metabolism (Bantle et al., 2020). Glutamate reuptake (Zhu et al., 2022), glucose (Calsolaro et al., 2021), and lipid metabolisms (Sierri et al., 2021) are all damaged in the active state of astrocytes, resulting in toxicity of excessive glutamate and lipid in neurons.
Fourth, astrocytes maintain synaptic transmission by regulating transmitters and cell excitability (Correa Bernardo et al., 2022). They recycle synaptic transmitters to prevent excitotoxicity to neurons (Strohm and Behrends, 2020). Astrocytes have long-term effects on synapses by releasing growth factors. However, toxicity to synapses was produced in reactive astrocytes via several pathways, including ATP deficiency, ion metabolisms dysfunction, and damaged ion channels, all of which degrade the synaptic integrity (Cervetto et al., 2021; Huiliang et al., 2021; Perez-Nievas et al., 2021).
Alzheimer’s disease is the most common neurodegenerative disease, characterized by progressive memory loss in clinical manifestation, with misfolding amyloid-beta (Aβ) protein aggregation and hyperphosphorylated tau protein (pTau). In an AD mouse model, pathological changes of microglia appeared before tau protein aggregation (Leng and Edison, 2021). Given that activated microglia influence astrogliosis, astrocytes may play a role in the progression of AD, even in the early and asymptomatic stages. Astrocytes appear neuroprotective in presymptomatic AD cases by internalizing Aβ, whereas astrocyte induced neurotoxicity subsequently due to excessive Aβ aggregation and other neurotoxic factors (de Majo et al., 2020). Biomarkers and pro-inflammatory factors secreted by reactive astrocytes were discovered in cerebrospinal fluid (CSF) of patients with early stages of AD (Janelidze et al., 2018). Astrocyte transcriptome analysis of AD brain tissue revealed gene changes associated with Aβ and pTau pathology in inflammation, protein regulation, oxidative stress, antioxidant function, lipid metabolism, and ion homeostasis (Viejo et al., 2022). Positron emission tomography (PET) and magnetic resonance imaging (MRI) (Choo et al., 2014; Vilemagne et al., 2022a) revealed dynamic changes in reactive astrocytes and Aβ due to mild cognition impairment. At preclinical stages, astrogliosis was induced by early Aβ deposition and loss of gray matter cells (Choo et al., 2014; Vilemagne et al., 2022a), whereas astrocyte atrophy appeared subsequently with greater Aβ deposition (Hsu et al., 2018). Astroglia tracer also revealed that reactive astrogliosis increased and reached a peak in preclinical stages of AD, followed by a decrease of astrogliosis and then rise again in dementia stages, exerting neurotoxic functions (Kumar et al., 2021). These findings suggest that astrogliosis occurs in the early stages of AD, with the formation and process of Aβ, and becomes neurotoxic to neurons as the disease progresses.
The apolipoprotein E (APOE) ε4 allele is the strongest risk gene variant of AD, especially late-onset AD, and it is widely expressed in astrocytes. Post-mortem studies discovered that APOE ε4 carriers had a higher Aβ plaque burden (Schmechel et al., 1993). Single-nucleus RNA sequencing in the frontal cortex of AD patients with APOE ε4 alleles showed astrocytes with highly expressed APOE ε4 and markers of reactive astrocytes (GFAP, HSP1B, IFITM3, TAPBP, CHI3L1, etc.) (Table 1; Griswold et al., 2021).
Astrocytes carrying APOE ε4 promote Aβ accumulation both in astrocytes and neurons. In vitro, astrocytes from hiPSC carrying APOE ε4 allele increased Aβ precursor protein (APP) levels, Aβ secretion in neurons, and decreased Aβ uptake in astrocytes (Lin et al., 2018; Lee S. I. et al., 2021; de Leeuw et al., 2022; Figure 1). Aβ was produced from APP, which was increased by the formation of lipid rafts induced by APOE ε4 astrocytes (Lee S. I. et al., 2021). Cholesterol signaling also participates in Aβ accumulation regulation (Wang H. et al., 2021). Astrocytes with APOE ε4 increased astrocyte-derived cholesterol (particularly lysosomal cholesterol), resulting in Aβ accumulation (Lin et al., 2018). In addition, astrocytes differentiated from hiPSC with APOE ε4 allele showed activation and released inflammatory cytokines, aggravating the pathological process and neuron death of AD (Table 1 and Figure 1; de Leeuw et al., 2022).
Figure 1. Summary of reactive astrocytes involved in pathogenic or risk gene variants of AD, ALS, and PD. Reactive astrocytes carrying APOE ε4 promote Aβ and pTau accumulation in both astrocytes and neurons. Reactive astrocytes with APOE ε4 allele secrete pro-inflammatory factors and damage BBB integrity by upregulating MMP9. Reactive astrocytes with SOD1 mutant increase glutamate secretion and hyperexcitability by dysregulated AMPAR, resulting in ER-mitochondrial impairment and oxidative stress in MNs. α-synuclein accumulation and oxidative stress increase in neurons through damaged ER-mitochondria in reactive astrocytes with PRKN, PINK1, DJ-1, SNCA mutant, and autophagy-lysosomal dysfunctions were found in reactive astrocytes with LRRK2, GBA mutant. AD, Alzheimer’s disease; ALS, amyotrophic lateral sclerosis; PD, Parkinson’s disease; APOE, apolipoprotein E; SOD1, Cu/Zn-superoxide dismutase 1 gene; LRRK2, Leucine-rich repeat kinase 2 gene; SNCA, α-synuclein gene; PRKN, parkin gene; PINK1, PTEN-induced putative kinase 1 gene; GBA, glucocerebrosidase gene; MMP9, matrix metalloproteinase 9; NF-κB, nuclear factor-kappa B; mTOR, mammalian target of rapamycin pathway; SG, stress granules; AMPAR, α-amino-3-hydroxy-5-methyl-4-isoxazole-propionic acid receptor; ER, endoplasmic reticulum; TLR, Toll-like receptor.
In the AD mouse model, removing APOE ε4 reduced microglial activation and alleviated Aβ deposition in the cortex (Mahan et al., 2022). Interestingly, knocking out APOE ε4 in microglia did not affect Aβ plaque or transcriptional expression compared to controls (Henningfield et al., 2022), indicating that other glial cells, such as astrocytes, play essential roles in Aβ production and accumulation, while microglia appears to maintain Aβ homeostasis. Moreover, APOE ε4 in astrocytes participated in pTau aggregation to accelerate AD progression (Wang C. et al., 2021). BBB destruction also hastens the progression of AD. In a mouse model with knockin human APOE ε4, the number of astrocyte end-feet covering blood vessels in the cortex decreased, and tight junctions of BBB were damaged by increased matrix metalloproteinase 9 (MMP9) (Jackson et al., 2021; Figure 1).
In conclusion, astrocytes carrying APOE ε4 lose their normal functions, resulting in compromised BBB integrity and difficulty in Aβ or pTau clearance, as well as neuroinflammation (Figure 2). With AD progression, reactive astrogliosis occurs in the early stages of AD and precedes the hallmarks of AD (Aβ and pTau deposition).
Figure 2. Reactive astrocytes in gene variants of AD and AD pathogenesis. Several mechanisms have changed in astrocytes with APOEε4 allele or PSEN/APP variant. Both APOE ε4 allele and PSEN/APP variant induce neuroinflammation by pro-inflammatory factors. Cholesterol dysfunction remains in reactive astrocytes with APOE ε4 allele. Besides, reactive astrocytes with APOE ε4 allele also accumulate Aβ and pTau protein aggregation, and BBB damage.
Presenilin genes (PSEN1 and PSEN2) and APP are responsible for the early onset autosomal dominant inheritance of AD. In the early stages of APP/PS1 transgenic mouse models, astrocytes activate and resist oxidative stress in cellular and extracellular circumstances (Table 1). Astrocytes surrounding Aβ plaque deposits were regulated in K+ concentration imbalance to maintain normal functions in neurons and synapses in the early stage (Huffels et al., 2022). PSEN/APP mutant in astrocytes disrupted amino acid transmission between astrocytes and neurons in response to a long-term detrimental stimulus. Active branched-chain amino acids (BCAA) metabolism, impaired leucine metabolism, and neurotransmitter dysfunction through gamma aminobutyric acid (GABA) uptake capacity were discovered in astrocytes carrying PSEN1 or APP pathogenic variants (Salcedo et al., 2021a,b). In the late stage of APP/PS1 mouse models, astrocytes also triggered immune signaling and lack of neuroprotection (Orre et al., 2014). Reactive astrocytes induced neuroinflammation and activated the transcriptional activity of NF-κB to induce inflammatory factors (Cao et al., 2021; Figure 2).
Amyotrophic lateral sclerosis is another neurodegeneration of great concern because of its rapid progression and high mortality. A variety of mechanisms are involved in the pathogenesis of ALS, such as mitochondrial damage, oxidative stress, amino acid toxicity, neuroinflammation, axon transport disorders, endoplasmic reticulum (ER) stress, abnormal protein clearance, abnormal RNA metabolism, etc. The pathological hallmark of the disease is abnormal TDP-43 protein inclusion in the cytoplasm. Familial ALS (fALS) accounts for approximately 5–10% of all cases. The discovery of ALS causative genes assists us to uncover the pathogenesis of ALS. FTD shares some common clinical features and genetics (such as C9orf72 and MAPT mutations). Pathological examination revealed that cortex astrogliosis is a feature of sporadic ALS (sALS) and fALS (Table 1; Kushner et al., 1991; Guttenplan et al., 2020). Astrogliosis detected by PET by measuring monoamine oxidase-B (MAO-B) activity remained in the motor cortex and temporal lobes of patients with ALS and associated with regions lacking cerebral blood flow (Kushner et al., 1991; Higashihara et al., 2021). In several models, astrocyte reaction appeared at the early stages of ALS and FTD and caused motor neurons (MNs) death (Sun et al., 2015; Vahsen et al., 2021). However, the dynamic changes in the reactive astrocytes in patients with ALS remain unclear due to a lack of in vivo research on neuroimaging and biomarkers. The mechanisms of ALS causative gene variants in astrocytes may provide insights into the progression of ALS or FTD.
The first causative gene for ALS, Cu/Zn-superoxide dismutase 1 (SOD1) mutation, was discovered in 1993. Protein aggregation and prion-like propagation of misfolded SOD1 protein are the main pathologies caused by SOD1 mutation (Canosa et al., 2022). SOD1 protein is a mitochondrial antioxidant enzyme, and SOD1 mutation increases cytoplasmic stress granules (SG) and ER stress in neurons (Rajpurohit et al., 2020; Figure 1). SOD1 gene loss of function contributes to the process of neuron death (Berdyński et al., 2022). A post-mortem study of SOD1-ALS patients discovered astrogliosis with a high level of C3 as a marker, as well as astrocyte hypertrophy in the motor cortex and spinal cord (Guttenplan et al., 2020).
Astrocytes derived from human mutant SOD1-overexpressing mice specifically damaged MNs, while other types of cells such as interneurons, GABAergic neurons, or dorsal root ganglion neurons were unaffected (Nagai et al., 2007; Bunton-Stasyshyn et al., 2015; Harlan et al., 2019). Meanwhile, in co-culture, microglia and fibroblasts with SOD1 mutant did not affect the MNs viability (Nagai et al., 2007). Astrocytes with SOD1 mutation reduced MNs viability in co-culture by inducing nitroxidative stress, transporting oxidative stress via secretomes (Rajpurohit et al., 2020), and producing hyperexcitability through dysregulated AMPA receptors and extracellular glutamate secretion to MNs (Van Damme et al., 2007; Rojas et al., 2014; Mohamed et al., 2019; Figure 1). Astrocytes derived from hiPSCs with SOD1 mutant showed astrocytes activation, increased levels of cytokines and the pro-inflammatory transcription factor NF-κB, and elevated SG. Moreover, astrocytes derived from SOD1-mutant hiPSCs stimulated mechanistic target of rapamycin (mTOR) activation induced by increased insulin-like growth factor 1 receptor (IGF1R) levels. IGF1R inhibition in astrocytes was found to be neuroprotective (Granatiero et al., 2021).
Animal models for studying astrocytes with SOD1 mutation investigated astrocytes changes at different stages. In the early and prodromal stages of ALS, astrocytes in the motor cortex and spinal cord of SOD1G93A mice demonstrated different vulnerability to oxidative stress as astrocytes in the motor cortex of the SOD1G93A mouse model had increased the oxidative stress, decreased the antioxidant capacity, and a relative mitochondria respiratory uncoupling, whereas the astrocytes in the spinal cord showed a higher endurance against oxidative damage through an increased antioxidant defense and a preserved mitochondrial respiratory function (Marini et al., 2021). The different responses of astrocytes from the motor cortex and spinal cords to oxidative stress indicate selective damage in ALS progression. Inflammation associated with astrocytes carrying SOD1 mutant also participates in the prodromal stage of ALS. The increasing level of astrocytic-specific TGFβ1 accelerated the disease progression in the SOD1G93A mouse model (Endo et al., 2015). The knockout inflammatory factors (IL-1α, TNFα, and C1q) slowed the progression along with reducing toxicity and activation of astrocytes in early mouse models (Guttenplan et al., 2020). Interestingly, decreasing NF-κB in astrocytes from the cortex of SOD1G93A mice in the prodromal stage inhibited cortical inflammation (Gomes et al., 2019). Despite the fact that astrocytes in the motor cortex and spinal cord had different endurance in the early stage of the disease, widespread and excessive oxidative stress in astrocytes was activated in the late stage of the SOD1G93A mouse model (López-Blanch et al., 2021). In the SOD1G93A mouse models, astrocytes from both the motor cortex and the spinal cords were characterized by ER-mitochondrial impairments, which were more pronounced in the mutated motor cortex than in the spinal cord cells (Marini et al., 2021). NF-κB increased in the symptomatic stage as well (Gomes et al., 2019), and astrocyte NF-κB-dependent activation accelerated the disease progression (Ouali Alami et al., 2018).
These findings suggest that SOD1 mutations participate in reactive astrogliosis in the early stage of ALS. Reactive astrocytes are primarily neurotoxic, causing oxidative stress, excessive excitability, and neuroinflammatory activation (Figure 3).
Figure 3. Reactive astrocytes in gene variants of ALS and ALS pathogenesis. There are several faces of astrocytes with gene variants of ALS. SOD1, TDP-43, FUS, MAPT variant, and C9orf72 expansion cause oxidative stress in both astrocytes and MNs. SOD1 variants also cause astrocytes to secret extracellular glutamates. Reactive astrocytes with SOD1 variants result in hyperexcitability to MNs. TDP-43 loss of function in astrocytes reduces metabolic support and glutamate uptake, while TDP-43 gain of function in astrocytes damages BBB integrity. Other processes, such as mitochondria impairments, neuroinflammation expansion, and disrupted autophagy and proteostasis, also contribute to MNs death.
TAR DNA-binding protein 43 (TDP-43) mutation results in abnormal TDP-43 inclusion in the cytoplasm of MNs and glial cells, which is a pathological hallmark of most sALS and fALS, as well as in FTD (Keating et al., 2022). TDP-43, as a DNA-/RNA-modulating protein, is involved in RNA splicing, transport, and translation, as well as cellular dysfunction and toxicity (Keating et al., 2022). Normal TDP-43 maintains the protective functions of MNs, loss of function of which causes hippocampal and cortical synaptic deficits, as well as dysfunction of RNA metabolisms (Ni et al., 2021). TDP-43 cytoplasmic aggregation in astrocytes is a key feature in fALS with TDP-43 mutant, sALS, and FTD. TDP-43 positive cytoplasmic inclusions were predominant in astrocytes of the anterior horn of the spinal cord, and quite rare in neurons in a patient with fALS who carried TDP-43 mutant (Takeda et al., 2019). RNA-seq transcriptomes on post-mortem frontal, temporal cortex, and cerebellum tissue from FTD patients with TDP-43 cytoplasmic aggregation discovered upregulated markers of astrocytes (GFAP) in the frontal cortex (Hasan et al., 2021).
TAR DNA-binding protein 43 mutation, like SOD1 mutation, is toxic to astrocytes. Astrocytes with TDP-43 mutant showed cytoplasmic mislocalization of TDP-43 protein and decreased astrocyte survival (Serio et al., 2013). When primary astrocytes with TDP-43 mutation were co-cultured with MNs, MNs death existed as a result of oxidative stress and dysregulated sodium channels (Rojas et al., 2014). On the other hand, normal astrocytes provide neuroprotective and metabolic support to neurons even when TDP-43 aggregation is mislocated. Astrocytes derived from sALS iPSCs were found to reduce cytoplasmic TDP-43 mislocalization from MNs to astrocytes (Smethurst et al., 2020). However, neuronal protection from astrocytes is limited. Primary astrocytes with TDP-43 inclusions accumulated more lipid droplets, activated aerobic glycolysis, and downregulated lactate transporters, resulting in decreased metabolic support for MNs and neurotoxicity (Velebit et al., 2020). TDP-43 inclusion in the cytoplasm also induced astrocyte inflammation and activation, secreting pro-inflammatory factors (IL-1β, IL-6, and TNFα) and causing neurodegeneration and neuroinflammation (Lee et al., 2020; Kim et al., 2021).
A mouse model with a human TDP-43 mutant that was restricted to astrocytes showed progressive loss of MNs, denervation of skeletal muscles, and consequent paralysis. TDP-43 mutant activated astrocytes, and glutamate transporters GLT-1 and GLAST in astrocytes were reduced, resulting in MNs neurotoxicity from high glutamate levels (Tong et al., 2013). Besides, astrogliosis and neuroinflammation were found in the majority of the spinal cord and cortex of TDP-43 transgenic mice (Yang et al., 2022). Levels of mutant TDP-43 expression in astrocytes determined the degree of injury to MNs (Yamanaka and Komine, 2018). In transgenic mice with TDP-43 mutant, reactive astrocytes secreted neurotoxic factors, lipocalin 2 (lcn2), to specifically cause neuron death, while other glial cells were unaffected (Table 1; Bi et al., 2013). Oxidative stress and abnormal ATP accumulation in astrocytes were also elevated in TDP-43 transgenic mice, along with glutathione and L-glutamate uptake deficits (Moujalled et al., 2017; Barton et al., 2020). TDP-43 overexpression may also activate astrocytes (Table 1). TDP-43 overexpression in astrocytes triggered inflammation in the brain, resulting in BBB permeability disruption (Zamudio et al., 2020).
These findings suggest that the balanced TDP-43 expression is essential to maintain astrocyte homeostasis. Astrocytes containing mutant TDP-43 destroy and transform the protective and supportive function of astrocytes into neurotoxicity through secreting pro-inflammatory factors, inducing oxidative stress and excitatory glutamate toxicity (Figure 3).
Hexanucleotide repeat expansions in C9orf72 comprise the most common causative gene of ALS and FTD in Caucasians. ER-mitochondrial signaling, protein homeostasis, and RNA metabolisms were found to be dysfunctional in cases with C9orf72 expansions (Gomez-Suaga et al., 2022). Dipeptide repeat (DPR) polypeptides translated by C9orf72 repeat expansions and deficiency of C9orf72 expression are detrimental to neurons and microglia (Lall et al., 2021). Post-mortem analysis of C9orf72-ALS patients revealed astrogliosis and astrocyte senescence (Table 1; Guttenplan et al., 2020). A group of enriched proteins specifically expressed from astrocytes was significantly increased when ALS patients with the C9orf72 expansion were compared to sALS patients (Umoh et al., 2018). GFAP expression was elevated in the motor cortex and hippocampus of transgenic mouse models expressing GFP-poly (GR)100 and poly (GA)50 (Table 1; Zhang et al., 2016; Zhang Y. J. et al., 2018), whereas GFAP level was not changed in the spinal cord (Schludi et al., 2017). C9orf72 BAC mouse models revealed that astrogliosis preferred to exist in the end-stage of the disease (Liu et al., 2016). However, there was no difference in plasma GFAP expression between the presymptomatic and symptomatic stages of C9orf72-FTD (Heller et al., 2020). Therefore, further research into the specific role of astrocytes with C9orf72 expansion is required.
Motor neurons undergo cell senescence due to oxidative stress and neurotoxicity when co-cultured with fibroblast-derived astrocytes from C9orf72-ALS patients (Birger et al., 2019; Zhao et al., 2020). Both astrocytes and neurons in cortical organoids derived from hiPSCs of C9orf72 cases existed in DPR polypeptides and expanded RNA foci via disrupted autophagy and proteostasis (Mizielinska et al., 2013; Conlon et al., 2016; Zhao et al., 2020; Szebényi et al., 2021). Astrocytes from hiPSCs with C9orf72 expansion caused voltage-activated currents and action potential output loss in MNs, resulting in disrupted action potentials (Zhao et al., 2020). There was a lack of energy production due to adenosine to inosine deamination defect, reduced glycogen metabolism, and mitochondrial respiration dysfunction in astrocytes in familial C9orf72 cases (Allen et al., 2019a,b). In addition, intracellular glutamate level was elevated in astrocytes of patients with C9orf72 expansion (Fomin et al., 2018). In contrast to SOD1-ALS astrocytes, glutamate secretion was not increased in C9orf72-ALS astrocytes in vitro (Mohamed et al., 2019). These results indicated that different glutamate secretion and uptake mechanisms exist in astrocytes with SOD1 mutant and C9orf72 expansion.
In conclusion, C9orf72 expansions are considered the primary cause of toxicity in astrocytes by disrupting energy supply and mitochondrial respiration. Astrocytes dysfunction results in neurotoxicity to MNs and, as a result, ALS pathogenesis (Figure 3).
Fused in sarcoma gene (FUS) mutation mainly exists in early onset ALS and FTD. FUS is a multifunctional DNA/RNA-binding protein involved in RNA processing and metabolism. Cytoplasmic FUS inclusion is another pathological hallmark of ALS. FUS protein mislocalization in mitochondria caused by FUS mutation causes mitochondrial death in neurons (Deng et al., 2015). FUS mutant also impaired neuromuscular junctions and caused mitochondrial dysfunction in neurons and skeletal muscle (Yu et al., 2022). An ALS patient carrying the FUS variant showed increased expression of FUS protein in reactive astrocytes and astrogliosis accumulated surrounding MNs with RNA foci (Wongworawat et al., 2020). Another patient with FTD carrying the FUS variant had FUS-positive cytoplasmic or intranuclear inclusion (Murakami et al., 2021). However, FUS inclusion remained mainly in oligodendrocytes rather than astrocytes in other ALS cases with the FUS variants (Mackenzie et al., 2011). These disparities were most likely caused by the small number of autopsies performed on ALS/FTD patients carrying FUS variants.
Silencing FUS expression in the brain by the AAV vector system provoked a proliferation of astrocytes and astrogliosis in marmosets (Table 1; Endo et al., 2018). FUS mutant astrocytes triggered MNs susceptible to excitotoxicity via AMPAR-mediated cell death (Kia et al., 2018). Clock and clock-controlled genes altered in FUS-ALS iPSC-derived astrocytes, contribute to metabolic and redox impairment (Killoy et al., 2021). In SOD1-mutant mouse models, misfolding cytoplasmic FUS accumulation in reactive astrocytes caused MN degenerative death via pro-inflammatory and neurotoxic pathways such as TNF-α with neutralizing antibodies (Li et al., 2016). These findings indicate that FUS deficiency and mislocalization are toxic to astrocytes. Meanwhile, astrocytes with FUS mutation reduced neuron viability through neuroinflammation and metabolic dysfunction (Figure 3).
The microtubule-associated protein tau (MAPT) is a causative gene in tauopathy diseases such as FTD, and progressive supranuclear palsy (PSP). MAPT encodes tau protein and produces six different isoforms of tau, primarily 3R and 4R in approximately 1:1 ratio in adult brains. Post-mortem neuropathological examination revealed that the presence of astrogliosis in patients with MAPT P301T variant, with these astrocytes retaining tau protein inclusion (Table 1; Erro et al., 2019).
Tau is expressed by astrocytes and oligodendrocytes, though at lower levels than in neurons (Chung et al., 2021). However, the high expression of phosphorylated tau in astrocytes in the pathological states suggests that astrocytes may also be involved in tauopathy. The overexpression of ptau in astrocytes was sufficient to cause cognitive decline in a mouse model (Richetin et al., 2020). Astrocytes derived from asymptomatic MAPT 10 + 16 intronic mutation iPSCs had a higher ratio of 4R:3R-tau transcript and protein than neurons (Setó-Salvia et al., 2021). Reactive astrocytes stimulated by tau express neurotoxic factors and respond to oxidative stress in vitro by producing ROS and membrane activation (Esteras et al., 2021; Ungerleider et al., 2021). Tau inhibited mitochondrial calcium efflux in both neurons and astrocytes derived from 10 + 16 MAPT hiPSC (Britti et al., 2020). In the presence of mitochondrial dysfunction, astrocytes and neurons derived from 10 + 16 MAPT hiPSC were more susceptible to calcium-induced caspase 3 activation and cell death (Britti et al., 2020).
Parkinson’s disease is the second most common neurodegeneration, characterized by dopaminergic neurons (DA neurons) death in the substantia nigra (SN) and dopamine (DA) deficiency in the striatum. Astrocyte response to acute and chronic stress earlier than neuron in PD. In contrast to the widespread astrogliosis seen in AD and ALS, the cortex of sporadic PD lacked or contained only mild GFAP-positive astrocytes in post-mortem examination (Tong et al., 2015; Cardinale et al., 2021). Patients with PD showed increased reactive astrocytes in the brainstem in the early stage of PD, while decreased reactive astrocytes in the cortex and brainstem in the middle and late stages of the disease, according to an in vivo PET imaging study (Wilson et al., 2019). These findings indicate different conditions of astrocytes during the PD process. Astrocytes provide neuroprotection via releasing antioxidants in response to oxidative stress in the early stages of PD (Takahashi and Mashima, 2022). However, pro-inflammatory factors, energy deficiency, and synapse dysfunction from reactive astrocytes accelerate neuron death in the late stages of PD (Liddelow et al., 2017; Kam et al., 2020). Pathogenic and risk genes associated with PD may assist in understanding the role of astrocytes in PD.
Leucine-rich repeat kinase 2 (LRRK2) is involved in sporadic and familial PD and is a link to lysosomal and mitochondrial functions. Excessive activation and phosphorylation of LRRK2 protein impaired autophagy in patients with PD (Di Maio et al., 2018; Pang et al., 2022). Astrogliosis and neural loss were discovered in the SNs of patients with the LRRK2 variant (Table 1; Takanashi et al., 2018).
Astrocyte atrophy was found in the derived astrocytes from PD patients with LRRK2G2019S mutation (Table 1; Ramos-Gonzalez et al., 2021). LRRK2G2019S mutated astrocyte from hiPSC appeared to cause nutritional damage to the DAergic neurons, damage the ATP supply, low-mitochondrial density, and ER disorder (de Rus Jacquet et al., 2021). Moreover, autophagy dysfunction was observed in astrocytes derived from LRRK2G2019S mutated iPSCs (di Domenico et al., 2019; Figure 1). LRRK2G2019S mutated astrocytes decreased the capacity to internalize and degrade fibrillar α-synuclein via the lysosomal pathway (Streubel-Gallasch et al., 2021). These disorders lead to a lack of α-synuclein internalization and clearance in astrocytes, as well as α-synuclein accumulation.
Primary cilia loss and astrocytic atrophy are the main characteristics of astrocytes in LRRK2 mutant models (Table 1; Khan et al., 2021), with astrocytes losing physical functions, such as ER stress, mitochondrial dysfunctions (Lee J. H. et al., 2021), etc. However, primary astrocytes transformed into reactive astrocytes in LRRK2 transgenic mice when exposed to additional oxidative stress, such as a toxic dose of MPTP (Arbez et al., 2020). In LRRK2 mutation mouse models, reactive astrocytes protect neurons through anti-inflammatory functions. Astrocyte activation of Nrf2 inhibited neural degeneration in transgenic mouse models by antagonizing LRRK2G2019S-induced Mad/Smad signaling (Lin et al., 2021).
These findings suggest that LRRK2 mutant trigger astrocyte senescence, resulting in neural death, while reactive astrocytes may also play anti-inflammation roles in PD patients with LRRK2 mutant (Figure 4).
Figure 4. Reactive astrocytes in gene variants of PD and PD pathogenesis. LRRK2, SNCA, and GBA variants impaired ER-mitochondria in astrocytes, accelerating α-synucleins aggregation in dopaminergic neurons. SNCA and GBA variants also cause neuroinflammation in astrocytes. PRKN, PINK1, and DJ-1 loss of functions induces oxidative stress in astrocytes and promote dopaminergic neuron death. Astrocytes lacking PRKN and PINK1 provide less metabolic support to neurons.
The α-synuclein gene (SNCA) was discovered in 1997 as the first familial PD gene. Point mutations (such as A53T, A30P, E46K, and H50Q) and copy number variation were common pathogenic variants in PD (Polymeropoulos et al., 1997). Insoluble α-synuclein accumulation is an important pathologic marker in PD. Astrocytes in post-mortems were found to internalize a significant amount of α-synuclein fibrils (Kovacs et al., 2014), and to participate in the spread of α-synuclein via extracellular vesicles or exosomes (Rostami et al., 2020).
Extensive reactive astrocytes were discovered in SNCA A53T transgenic mouse models (Table 1; Yang et al., 2015). In astrocytes, SNCA A53T and A30P variants remained in the impaired ER stress. Interestingly, rotenone-induced astrocyte senescence led to axonal degeneration of midbrain neurons with SNCA locus duplication (Simmnacher et al., 2020). Cytoplasmic α-synuclein in neurons recruits and activates astrocytes via a prion-like process, which is a common pathology in patients with PD (Sznejder-Pachołek et al., 2017; Rizor et al., 2019). Astrocytes reduced α-synuclein proteins misfolding, prevented neurotoxicity from α-synuclein aggregation, rescued damaged DA neurons, and then protected DA neurons at an early stage of PD (Jewett et al., 2018; Zhang Z. et al., 2018). Long-term α-synuclein stress, on the other hand, disrupts the physiological functions of astrocytes. Dysfunction of the ER-Golgi compartment α-synuclein overexpressing astrocytes may lead to a decrease in glial cell-derived neurotrophic factor (GDNF) level, which would suppress the neurite outgrowth (Liu et al., 2018). Besides, exogenous overexpression of α-synuclein proteins in astrocytes damaged the autophagy-lysosomal pathway and promoted astrocyte apoptosis (Erustes et al., 2018). Neuroinflammation induced by astrocytes with α-synuclein aggregation also hastens the progression of PD (Kim et al., 2018; Kwon et al., 2021).
In conclusion, SNCA variants cause astrocyte reaction and atrophy, as well as ER dysfunction. Aggregation of α-synuclein is toxic to astrocytes via multiple pathways, resulting in astrocyte dysfunction and accelerating α-synuclein accumulation (Figure 4).
PTEN-induced putative kinase 1 (PINK1), parkin (PRKN), and DJ-1 genes are all known to play a role in early onset PD (EOPD) (Bonifati et al., 2003; Dawson and Dawson, 2010; Kawajiri et al., 2011). Mitophagy is mediated by these genes via the PINK1/parkin pathway. PINK1 protein is a mitochondrial kinase, parkin is an E3 ubiquitin ligase, and DJ-1 protein participate in proteasome degradation (Panicker et al., 2017; Li et al., 2021).
Reactive astrocytes in iPSCs induced from patients with homozygous PRKN variants were reduced (Table 1; Kano et al., 2020). Deletion of the PRKN leads to abnormal astrocyte function, resulting in DA neurons being vulnerable to oxidative stress (Solano et al., 2008). Parkin controls neuronal homeostasis by regulating astrocyte ER stress and inflammation. In response to ER stress, parkin deficiency astrocytes increased ER stress and released cytokine, and decreased neural support (Singh et al., 2018).
Reactive astrocytes increase PINK1 protein expression (Table 1; Choi et al., 2016; Jarazo et al., 2022). Astrocytes with PINK1 loss of function increased neuroinflammation and lacked physiological support to neurons (Leites and Morais, 2021). PINK1 deficiency disrupted mitophagy, altered PINK1-dependent ubiquitin phosphorylation, and increased nitric oxide production in astrocytes (Sun et al., 2018; Barodia et al., 2019; Komilova et al., 2021).
DJ-1 is a neuroprotective protein in PD, which deficiency impaired glutamate uptake into astrocytes by altering EAAT2 expression in vitro (Kim et al., 2016). DJ-1 was found to be highly expressed in reactive astrocytes in vivo (Frøyset et al., 2018). DJ-1 is a positive regulator of STAT3 activation, the most important astrogliosis mediator (Choi et al., 2018b). DJ-1 overexpression in astrocytes protected neurons from multiple PD processes (de Miranda et al., 2018), and regulated several proteins that support physiological functions on astrocytes and neurons, including redox regulation, anti-inflammation, and mitochondrial respiration (Ashley et al., 2016; Frøyset et al., 2018). DJ-1 dysfunction resulted in the harmful inflammatory response in PD development (Choi et al., 2019). DJ-1 knockout astrocytes may provide less neuroprotection to surrounding neurons due to changes in pro-inflammatory mediator expression (Table 1; Ashley et al., 2016). DJ-1 knockout mice had defective astrogliosis caused by decreased CCL2, Sox9 expression, and reduced monocyte infiltration, which disrupted recovery from CNS injury and accelerated PD progression (Table 1; Choi et al., 2018a,2020).
Taken together, since PRKN/PINK1/DJ-1 genes are associated with mitophagy and ubiquitin functions, their loss of functions of these genes in astrocytes participates in excessive oxidative stress, neuroinflammation, and mitochondrial dysfunctions, all of which contribute to the PD process (Figure 4).
β-glucocerebrosidase gene (GBA), a pathogenic gene for Gaucher’s disease (GD) (Neumann et al., 2009), is the most common genetic risk factor of PD and is associated with an autophagic-lysosomal pathway (Senkevich and Gan-Or, 2020). GBA deficiency may cause α-synuclein aggregation and alter neuronal susceptibility to pathology (Henderson et al., 2020; Pang et al., 2022).
Extensively reactive astrocytes with GFAP, S100β, and severe cytoskeletal hypertrophy in astrocytes were discovered from iPSCs from GD patients (Table 1; Aflaki et al., 2020). Furthermore, residual GCase activity appeared to determine the degree of astrogliosis, inflammatory response, Ca+ dysfunction, and ability to process α-synuclein (Aflaki et al., 2020; Sonninen et al., 2020). Autophagy and lysosomal storage disorder in reactive astrocytes, combined with inflammation, disrupt mitochondrial homeostasis and cause α-synuclein aggregation in the cortex (Di Malta et al., 2012; Osellame et al., 2013; Booth et al., 2017; Sanyal et al., 2020). These findings suggest that GCase deficiency in GBA mutant astrocytes is likely a trigger of reactive astrogliosis through inflammation, impaired autophagy, etc., and as a result of PD progression (Figure 4).
As previously discussed, reactive astrogliosis is common in neurodegenerative diseases with pathogenic or risk gene variants, indicating that astrocytes activate in the early stages and precede hallmarks, and exert different effects throughout the disease progress. New technologies have emerged to assist researchers in directly observing the progression of reactive astrogliosis in patients. The detection of reactive astrocytes is gradually being implemented to detect the disease at its early stages and track its progression. A selective monoamine oxidase-B (MAO-B) tracer is also used to detect reactive astrogliosis since MAO-B is overexpressed in reactive astrocytes. The tracer identified the reactive astrogliosis in mild cognitive impairment (MCI) and AD (Vilemagne et al., 2022b) when compared to controls, and it was detectable at the preclinical stages of Aβ accumulation (Vilemagne et al., 2022a). Markers secreted by reactive astrocytes could also be implemented as a diagnostic tool in patients. Plasma GFAP differs in FTD and AD, which is useful to distinguish FTD and AD and predicting cognitive decline when combined with plasma Nfl detection (Zhu et al., 2021). Salivary GFAP is also considered a potential biomarker for the diagnosis of MCI and AD (Katsipis et al., 2021). These findings indicate that astrocytes are useful to recognize neurodegenerations, though the specificity and sensitivity of reactive astrocytes for diagnosis need to be improved since shared mechanisms induced by astrocytes exist in various neurodegenerative diseases. Moreover, given the neurotoxic roles of reactive astrocytes, regulating pro-inflammatory factors, synapse dysfunctions, high BBB permeability, etc. induced by reactive astrocytes probably reduce the neurotoxicity. Targeting molecular or pathways associated with astrocytes such as STAT, EAAT, GFAP, and connexin 43 via adeno-associated virus (AAV) reduced Aβ accumulation in mouse models (Price et al., 2021). Researchers also attempted to restore physiological capabilities in ALS patients by transplanting astrocytes derived from human embryonic stem cells (ClinicalTrials.gov Identifier: NCT03482050).
Astrocytes are crucial in the pathogenesis of neurodegenerations. Astrocytes maintain and support the physiological functions of neurons, synapses, and BBB. Pathological and related genes of neurodegenerations disrupt astrocyte homeostasis and cause astrocyte activation. Disease models involving several genes (APOE ε4 and SOD1) revealed that astrocytes activated adaptively to provide neuroprotection in the early stages or even prodromal stages of the disease, whereas reactive astrocytes gradually became neurotoxic as the disease progressed. Different causative genes lead to various pathological processes in astrocytes, including neuroinflammation, oxidative stress, and ER-mitochondria impairment, which eventually lead to misfolding protein aggregation in neurons and neural death. However, due to the heterogeneities of astrocytes in different stages of the disease, astrocytes treatments need to be more cautious. Better treatments based on dysregulated astrocytes require further research into astrocytes targeting neurodegeneration pathologies.
JH and CL selected studies and drafted the manuscript. HS made the study design and revised the manuscript. All authors contributed to the article and approved the submitted version.
This study was supported by the Sichuan Science and Technology Program (Grant No. 2022ZDZX0023), the National Natural Science Foundation of China (Grant No. 81871000), the National Key Research and Development Program of China (Grant No. 2021YFC2501203), and the 1.3.5 Project for Disciplines of Excellence, West China Hospital, Sichuan University (ZYJC18038).
The authors declare that the research was conducted in the absence of any commercial or financial relationships that could be construed as a potential conflict of interest.
All claims expressed in this article are solely those of the authors and do not necessarily represent those of their affiliated organizations, or those of the publisher, the editors and the reviewers. Any product that may be evaluated in this article, or claim that may be made by its manufacturer, is not guaranteed or endorsed by the publisher.
We would like to appreciate the hard work of all of the authors in the original manuscript. We created Figures 1–4 by Figdraw (www.figdraw.com).
Aflaki, E., Stubblefield, B. K., McGlinchey, R. P., McMahon, B., Ory, D. S., and Sidransky, E. (2020). A characterization of Gaucher iPS-derived astrocytes: potential implications for Parkinson’s disease. Neurobiol. Dis. 134:104647. doi: 10.1016/j.nbd.2019.104647
Allen, S. P., Hall, B., Castelli, L. M., Francis, L., Woof, R., Siskos, A. P., et al. (2019a). Astrocyte adenosine deaminase loss increases motor neuron toxicity in amyotrophic lateral sclerosis. Brain 142, 586–605. doi: 10.1093/brain/awy353
Allen, S. P., Hall, B., Woof, R., Francis, L., Gatto, N., Shaw, A. C., et al. (2019b). C9orf72 expansion within astrocytes reduces metabolic flexibility in amyotrophic lateral sclerosis. Brain 142, 3771–3790. doi: 10.1093/brain/awz302
Arbez, N., He, X., Huang, Y., Ren, M., Liang, Y., Nucifora, F. C., et al. (2020). G2019S-LRRK2 mutation enhances MPTP-linked Parkinsonism in mice. Hum. Mol. Genet. 29, 580–590. doi: 10.1093/hmg/ddz271
Ashley, A. K., Hinds, A. I., Hanneman, W. H., Tjalkens, R. B., and Legare, M. E. (2016). DJ-1 mutation decreases astroglial release of inflammatory mediators. Neurotoxicology 52, 198–203. doi: 10.1016/j.neuro.2015.12.007
Bantle, C. M., Hirst, W. D., Weihofen, A., and Shlevkov, E. (2020). Mitochondrial dysfunction in astrocytes: a role in Parkinson’s disease? Front. Cell Dev. Biol. 8:608026. doi: 10.3389/fcell.2020.608026
Barodia, S. K., Mcmeekin, L. J., Creed, R. B., Quinones, E. K., Cowell, R. M., and Goldberg, M. S. (2019). PINK1 phosphorylates ubiquitin predominantly in astrocytes. NPJ Parkinsons Dis. 5:29. doi: 10.1038/s41531-019-0101-9
Barton, S. K., Lau, C. L., Chiam, M. D. F., Tomas, D., Muyderman, H., Beart, P. M., et al. (2020). Mutant TDP-43 expression triggers TDP-43 pathology and cell autonomous effects on primary astrocytes: implications for non-cell autonomous pathology in ALS. Neurochem. Res. 45, 1451–1459. doi: 10.1007/s11064-020-03048-5
Berdyński, M., Miszta, P., Safranow, K., Andersen, P. M., Morita, M., Filipek, S., et al. (2022). SOD1 mutations associated with amyotrophic lateral sclerosis analysis of variant severity. Sci. Rep. 12:103. doi: 10.1038/s41598-021-03891-8
Bi, F., Huang, C., Tong, J., Qiu, G., Huang, B., Wu, Q., et al. (2013). Reactive astrocytes secrete lcn2 to promote neuron death. Proc. Natl. Acad. Sci. U.S.A. 110, 4069–4074. doi: 10.1073/pnas.1218497110
Birger, A., Ben-Dor, I., Ottolenghi, M., Turetsky, T., Gil, Y., Sweetat, S., et al. (2019). Human iPSC-derived astrocytes from ALS patients with mutated C9ORF72 show increased oxidative stress and neurotoxicity. EBioMedicine 50, 274–289. doi: 10.1016/j.ebiom.2019.11.026
Bonifati, V., Rizzu, P., Squitieri, F., Krieger, E., Vanacore, N., Van Swieten, J. C., et al. (2003). DJ-1(PARK7), a novel gene for autosomal recessive, early onset parkinsonism. Neurol. Sci. 24, 159–160. doi: 10.1007/s10072-003-0108-0
Booth, H. D. E., Hirst, W. D., and Wade-Martins, R. (2017). The role of astrocyte dysfunction in Parkinson’s disease pathogenesis. Trends Neurosci. 40, 358–370. doi: 10.1016/j.tins.2017.04.001
Britti, E., Ros, J., Esteras, N., and Abramov, A. Y. (2020). Tau inhibits mitochondrial calcium efflux and makes neurons vulnerable to calcium-induced cell death. Cell Calcium 86:102150. doi: 10.1016/j.ceca.2019.102150
Bunton-Stasyshyn, R. K., Saccon, R. A., Fratta, P., and Fisher, E. M. (2015). SOD1 function and its implications for amyotrophic lateral sclerosis pathology: new and renascent themes. Neuroscientist 21, 519–529. doi: 10.1177/1073858414561795
Calsolaro, V., Matthews, P. M., Donat, C. K., Livingston, N. R., Femminella, G. D., Guedes, S. S., et al. (2021). Astrocyte reactivity with late-onset cognitive impairment assessed in vivo using (11)C-BU99008 PET and its relationship with amyloid load. Mol. Psychiatry 26, 5848–5855. doi: 10.1038/s41380-021-01193-z
Canosa, A., Calvo, A., Moglia, C., Vasta, R., Palumbo, F., Solero, L., et al. (2022). Amyotrophic lateral sclerosis with SOD1 mutations shows distinct brain metabolic changes. Eur. J. Nucl. Med. Mol. Imaging 49, 2242–2250. doi: 10.1007/s00259-021-05668-7
Cao, L. L., Guan, P. P., Zhang, S. Q., Yang, Y., Huang, X. S., and Wang, P. (2021). Downregulating expression of OPTN elevates neuroinflammation via AIM2 inflammasome- and RIPK1-activating mechanisms in APP/PS1 transgenic mice. J. Neuroinflammation 18:281. doi: 10.1186/s12974-021-02327-4
Cardinale, A., Calabrese, V., De Iure, A., and Picconi, B. (2021). Alpha-synuclein as a prominent actor in the inflammatory synaptopathy of Parkinson’s disease. Int. J. Mol. Sci. 22:6517. doi: 10.3390/ijms22126517
Castellani, G., and Schwartz, M. (2020). Immunological features of non-neuronal brain cells: implications for Alzheimer’s disease immunotherapy. Trends Immunol. 41, 794–804. doi: 10.1016/j.it.2020.07.005
Cervetto, C., Averna, M., Vergani, L., Pedrazzi, M., Amato, S., Pelassa, S., et al. (2021). Reactive astrocytosis in a mouse model of chronic polyamine catabolism activation. Biomolecules 11:1274. doi: 10.3390/biom11091274
Choi, D. J., An, J., Jou, I., Park, S. M., and Joe, E. H. (2019). A Parkinson’s disease gene, DJ-1, regulates anti-inflammatory roles of astrocytes through prostaglandin D(2) synthase expression. Neurobiol. Dis. 127, 482–491. doi: 10.1016/j.nbd.2019.04.003
Choi, D. J., Eun, J. H., Kim, B. G., Jou, I., Park, S. M., and Joe, E. H. (2018a). A Parkinson’s disease gene, DJ-1, repairs brain injury through Sox9 stabilization and astrogliosis. Glia 66, 445–458. doi: 10.1002/glia.23258
Choi, D. J., Kwon, J. K., and Joe, E. H. (2018b). A Parkinson’s disease gene, DJ-1, regulates astrogliosis through STAT3. Neurosci. Lett. 685, 144–149. doi: 10.1016/j.neulet.2018.08.025
Choi, D. J., Yang, H., Gaire, S., Lee, K. A., An, J., Kim, B. G., et al. (2020). Critical roles of astrocytic-CCL2-dependent monocyte infiltration in a DJ-1 knockout mouse model of delayed brain repair. Glia 68, 2086–2101. doi: 10.1002/glia.23828
Choi, I., Choi, D. J., Yang, H., Woo, J. H., Chang, M. Y., Kim, J. Y., et al. (2016). PINK1 expression increases during brain development and stem cell differentiation, and affects the development of GFAP-positive astrocytes. Mol. Brain 9:5. doi: 10.1186/s13041-016-0186-6
Choo, I. L., Carter, S. F., Schöll, M. L., and Nordberg, A. (2014). Astrocytosis measured by 11C-deprenyl PET correlates with decrease in gray matter density in the parahippocampus of prodromal Alzheimer’s patients. Eur. J. Nucl. Med. Mol. Imaging 41, 2120–2126.
Chung, D. C., Roemer, S., Petrucelli, L., and Dickson, D. W. (2021). Cellular and pathological heterogeneity of primary tauopathies. Mol. Neurodegener. 16:57. doi: 10.1186/s13024-021-00476-x
Conlon, E. G., Lu, L., Sharma, A., Yamazaki, T., Tang, T., Shneider, N. A., et al. (2016). The C9ORF72 GGGGCC expansion forms RNA G-quadruplex inclusions and sequesters hnRNP H to disrupt splicing in ALS brains. eLife 5:e17820. doi: 10.7554/eLife.17820
Correa Bernardo, H. M., Moreira Carlos, R., Hildebrand Michael, E., and Vieira Luciene, B. (2022). The role of voltage gated calcium channels in basal ganglia neurodegenerative disorders. Curr. Neuropharmacol. 20, 1–1. doi: 10.2174/1570159X20666220327211156
Dawson, T. M., and Dawson, V. L. (2010). The role of parkin in familial and sporadic Parkinson’s disease. Mov. Disord. 25(Suppl. 1) S32–S39.
de Leeuw, S. M., Kirschner, A. W. T., Lindner, K., Rust, R., Budny, V., Wolski, W. E., et al. (2022). APOE2, E3, and E4 differentially modulate cellular homeostasis, cholesterol metabolism, and inflammatory response in isogenic iPSC-derived astrocytes. Stem Cell Rep. 17, 110–126.
de Majo, M., Koontz, M., Rowitch, D., and Ullian, E. M. (2020). An update on human astrocytes and their role in development and disease. Glia 68, 685–704. doi: 10.1002/glia.23771
de Miranda, B. R., Rocha, E. M., Bai, Q., El Ayadi, A., Hinkle, D., Burton, E. A., et al. (2018). Astrocyte-specific DJ-1 overexpression protects against rotenone-induced neurotoxicity in a rat model of Parkinson’s disease. Neurobiol. Dis. 115, 101–114. doi: 10.1016/j.nbd.2018.04.008
de Rus Jacquet, A., Tancredi, J. L., Lemire, A. L., Desantis, M. C., Li, W. P., and O’Shea, E. K. (2021). The LRRK2 G2019S mutation alters astrocyte-to-neuron communication via extracellular vesicles and induces neuron atrophy in a human iPSC-derived model of Parkinson’s disease. eLife 10:e73062. doi: 10.7554/eLife.73062
Deng, J., Yang, M., Chen, Y., Chen, X., Liu, J., Sun, S., et al. (2015). FUS interacts with HSP60 to promote mitochondrial damage. PLoS Genet. 11:e1005357. doi: 10.1371/journal.pgen.1005357
di Domenico, A., Carola, G., Calatayud, C., Pons-Espinal, M., Muñoz, J. P., Richaud-Patin, Y., et al. (2019). Patient-specific iPSC-derived astrocytes contribute to non-cell-autonomous neurodegeneration in Parkinson’s disease. Stem Cell Rep. 12, 213–229. doi: 10.1016/j.stemcr.2018.12.011
Di Maio, R., Hoffman, E. K., Rocha, E. M., Keeney, M. T., Sanders, L. H., De Miranda, B. R., et al. (2018). LRRK2 activation in idiopathic Parkinson’s disease. Sci. Transl. Med. 10:eaar5429. doi: 10.1126/scitranslmed.aar5429
Di Malta, C., Fryer, J. D., Settembre, C., and Ballabio, A. (2012). Astrocyte dysfunction triggers neurodegeneration in a lysosomal storage disorder. Proc. Natl. Acad. Sci. U.S.A. 109, E2334–E2342. doi: 10.1073/pnas.1209577109
Endo, F., Komine, O., Fujimori-Tonou, N., Katsuno, M., Jin, S., Watanabe, S., et al. (2015). Astrocyte-derived TGF-β1 accelerates disease progression in ALS mice by interfering with the neuroprotective functions of microglia and T cells. Cell Rep. 11, 592–604. doi: 10.1016/j.celrep.2015.03.053
Endo, K., Ishigaki, S., Masamizu, Y., Fujioka, Y., Watakabe, A., Yamamori, T., et al. (2018). Silencing of FUS in the common marmoset (Callithrix jacchus) brain via stereotaxic injection of an adeno-associated virus encoding shRNA. Neurosci. Res. 130, 56–64. doi: 10.1016/j.neures.2017.08.006
Erro, M. E., Zelaya, M. V., Mendioroz, M., Larumbe, R., Ortega-Cubero, S., Lanciego, J. L., et al. (2019). Globular glial tauopathy caused by MAPT P301T mutation: clinical and neuropathological findings. J. Neurol. 266, 2396–2405. doi: 10.1007/s00415-019-09414-w
Erustes, A. G., Stefani, F. Y., Terashima, J. Y., Stilhano, R. S., Monteforte, P. T., Da Silva Pereira, G. J., et al. (2018). Overexpression of α-synuclein in an astrocyte cell line promotes autophagy inhibition and apoptosis. J. Neurosci. Res. 96, 160–171. doi: 10.1002/jnr.24092
Escartin, C., Galea, E., Lakatos, A., O’Callaghan, J. P., Petzold, G. C., Serrano-Pozo, A., et al. (2021). Reactive astrocyte nomenclature, definitions, and future directions. Nat. Neurosci. 24, 312–325. doi: 10.1038/s41593-020-00783-4
Esteras, N., Kundel, F., Amodeo, G. F., Pavlov, E. V., Klenerman, D., and Abramov, A. Y. (2021). Insoluble tau aggregates induce neuronal death through modification of membrane ion conductance, activation of voltage-gated calcium channels and NADPH oxidase. FEBS J. 288, 127–141. doi: 10.1111/febs.15340
Fomin, V., Richard, P., Hoque, M., Li, C., Gu, Z., Fissore-O’Leary, M., et al. (2018). The C9ORF72 gene, implicated in amyotrophic lateral sclerosis and frontotemporal dementia, encodes a protein that functions in control of endothelin and glutamate signaling. Mol. Cell. Biol. 38:e00155-18. doi: 10.1128/MCB.00155-18
Frøyset, A. K., Edson, A. J., Gharbi, N., Khan, E. A., Dondorp, D., Bai, Q., et al. (2018). Astroglial DJ-1 over-expression up-regulates proteins involved in redox regulation and is neuroprotective in vivo. Redox Biol. 16, 237–247. doi: 10.1016/j.redox.2018.02.010
Gomes, C., Cunha, C., Nascimento, F., Ribeiro, J. A., Vaz, A. R., and Brites, D. (2019). Cortical neurotoxic astrocytes with early ALS pathology and miR-146a deficit replicate gliosis markers of symptomatic SOD1G93A mouse model. Mol. Neurobiol. 56, 2137–2158. doi: 10.1007/s12035-018-1220-8
Gomez-Suaga, P., Mórotz, G. M., Markovinovic, A., Martín-Guerrero, S. M., Preza, E., Arias, N., et al. (2022). Disruption of ER-mitochondria tethering and signalling in C9orf72-associated amyotrophic lateral sclerosis and frontotemporal dementia. Aging Cell 21:e13549. doi: 10.1111/acel.13549
Granatiero, V., Sayles, N. M., Savino, A. M., Konrad, C., Kharas, M. G., Kawamata, H., et al. (2021). Modulation of the IGF1R-MTOR pathway attenuates motor neuron toxicity of human ALS SOD1(G93A) astrocytes. Autophagy 17, 4029–4042. doi: 10.1080/15548627.2021.1899682
Griswold, A. J., Celis, K., Bussies, P. L., Rajabli, F., Whitehead, P. L., Hamilton-Nelson, K. L., et al. (2021). Increased APOE ε4 expression is associated with the difference in Alzheimer’s disease risk from diverse ancestral backgrounds. Alzheimers Dement. 17, 1179–1188. doi: 10.1002/alz.12287
Guttenplan, K. A., Weigel, M. K., Adler, D. I., Couthouis, J., Liddelow, S. A., Gitler, A. D., et al. (2020). Knockout of reactive astrocyte activating factors slows disease progression in an ALS mouse model. Nat. Commun. 11:3753. doi: 10.1038/s41467-020-17514-9
Harlan, B. A., Pehar, M., Killoy, K. M., and Vargas, M. R. (2019). Enhanced SIRT6 activity abrogates the neurotoxic phenotype of astrocytes expressing ALS-linked mutant SOD1. FASEB J. 33, 7084–7091. doi: 10.1096/fj.201802752R
Hasan, R., Humphrey, J., Bettencourt, C., Newcombe, J., Lashley, T., Fratta, P., et al. (2021). Transcriptomic analysis of frontotemporal lobar degeneration with TDP-43 pathology reveals cellular alterations across multiple brain regions. Acta Neuropathol. 143, 383–401. doi: 10.1007/s00401-021-02399-9
Heller, C., Foiani, M. S., Moore, K., Convery, R., Bocchetta, M., Neason, M., et al. (2020). Plasma glial fibrillary acidic protein is raised in progranulin-associated frontotemporal dementia. J. Neurol. Neurosurg. Psychiatry 91, 263–270. doi: 10.1136/jnnp-2019-321954
Henderson, M. X., Sedor, S., Mcgeary, I., Cornblath, E. J., Peng, C., Riddle, D. M., et al. (2020). Glucocerebrosidase activity modulates neuronal susceptibility to pathological α-synuclein insult. Neuron 105, 822–836.e7. doi: 10.1016/j.neuron.2019.12.004
Henningfield, C. M., Arreola, M. A., Soni, N., Spangenberg, E. E., and Green, K. N. (2022). Microglia-specific ApoE knock-out does not alter Alzheimer’s disease plaque pathogenesis or gene expression. Glia 70, 287–302. doi: 10.1002/glia.24105
Higashihara, M., Ishibashi, K., Tokumaru, A. M., Iwata, A., and Ishii, K. (2021). 18F-THK5351 PET can identify core lesions in different amyotrophic lateral sclerosis phenotypes. Clin. Nucl. Med. 46, e582–e583. doi: 10.1097/RLU.0000000000003755
Hsu, E. T., Gangolli, M., Su, S., Holleran, L., Stein, T. D., Alvarez, V. E., et al. (2018). Astrocytic degeneration in chronic traumatic encephalopathy. Acta Neuropathol. 136, 955–972. doi: 10.1007/s00401-018-1902-3
Huffels, C. F. M., Osborn, L. M., Hulshof, L. A., Kooijman, L., Henning, L., Steinhäuser, C., et al. (2022). Amyloid-β plaques affect astrocyte Kir4.1 protein expression but not function in the dentate gyrus of APP/PS1 mice. Glia 70, 748–767. doi: 10.1002/glia.24137
Huiliang, Z., Mengzhe, Y., Xiaochuan, W., Hui, W., Min, D., Mengqi, W., et al. (2021). Zinc induces reactive astrogliosis through ERK-dependent activation of Stat3 and promotes synaptic degeneration. J. Neurochem. 159, 1016–1027. doi: 10.1111/jnc.15531
Jackson, R. J., Meltzer, J. C., Nguyen, H., Commins, C., Bennett, R. E., Hudry, E., et al. (2021). APOE4 derived from astrocytes leads to blood-brain barrier impairment. Brain awab478. doi: 10.1093/brain/awab478 [Epub ahead of print].
Janelidze, S., Mattsson, N., Stomrud, E., Lindberg, O., Palmqvist, S., Zetterberg, H., et al. (2018). CSF biomarkers of neuroinflammation and cerebrovascular dysfunction in early Alzheimer disease. Neurology 91, e867–e877. doi: 10.1212/WNL.0000000000006082
Jarazo, J., Barmpa, K., Modamio, J., Saraiva, C., Sabaté-Soler, S., Rosety, I., et al. (2022). Parkinson’s disease phenotypes in patient neuronal cultures and brain organoids improved by 2-hydroxypropyl-β-cyclodextrin treatment. Mov. Disord. 37, 80–94. doi: 10.1002/mds.28810
Jewett, M., Dickson, E., Brolin, K., Negrini, M., Jimenez-Ferrer, I., and Swanberg, M. (2018). Glutathione S-transferase alpha 4 prevents dopamine neurodegeneration in a rat alpha-synuclein model of Parkinson’s disease. Front. Neurol. 9:222. doi: 10.3389/fneur.2018.00222
Kam, T. I., Hinkle, J. T., Dawson, T. M., and Dawson, V. L. (2020). Microglia and astrocyte dysfunction in Parkinson’s disease. Neurobiol. Dis. 144:105028. doi: 10.1016/j.nbd.2020.105028
Kano, M., Takanashi, M., Oyama, G., Yoritaka, A., Hatano, T., Shiba-Fukushima, K., et al. (2020). Reduced astrocytic reactivity in human brains and midbrain organoids with PRKN mutations. NPJ Parkinsons Dis. 6:33. doi: 10.1038/s41531-020-00137-8I
Katsipis, G., Tzekaki, E. E., Tsolaki, M., and Pantazaki, A. A. (2021). Salivary GFAP as a potential biomarker for diagnosis of mild cognitive impairment and Alzheimer’s disease and its correlation with neuroinflammation and apoptosis. J. Neuroimmunol. 361:577744. doi: 10.1016/j.jneuroim.2021.577744
Kawajiri, S., Saiki, S., Sato, S., and Hattori, N. (2011). Genetic mutations and functions of PINK1. Trends Pharmacol. Sci. 32, 573–580. doi: 10.1016/j.tips.2011.06.001
Keating, S. S., San Gil, R., Swanson, M. E. V., Scotter, E. L., and Walker, A. K. (2022). TDP-43 pathology: from noxious assembly to therapeutic removal. Prog. Neurobiol. 211:102229. doi: 10.1016/j.pneurobio.2022.102229
Khan, S. S., Sobu, Y., Dhekne, H. S., Tonelli, F., Berndsen, K., Alessi, D. R., et al. (2021). Pathogenic LRRK2 control of primary cilia and Hedgehog signaling in neurons and astrocytes of mouse brain. eLife 10:e67900. doi: 10.7554/eLife.67900
Kia, A., Mcavoy, K., Krishnamurthy, K., Trotti, D., and Pasinelli, P. (2018). Astrocytes expressing ALS-linked mutant FUS induce motor neuron death through release of tumor necrosis factor-alpha. Glia 66, 1016–1033. doi: 10.1002/glia.23298
Killoy, K. M., Pehar, M., Harlan, B. A., and Vargas, M. R. (2021). Altered expression of clock and clock-controlled genes in a hSOD1-linked amyotrophic lateral sclerosis mouse model. FASEB J. 35:e21343. doi: 10.1096/fj.202000386RR
Kim, C., Spencer, B., Rockenstein, E., Yamakado, H., Mante, M., Adame, A., et al. (2018). Immunotherapy targeting toll-like receptor 2 alleviates neurodegeneration in models of synucleinopathy by modulating α-synuclein transmission and neuroinflammation. Mol. Neurodegener. 13:43. doi: 10.1186/s13024-018-0276-2
Kim, J. H., Rahman, M. H., Park, D., Jo, M., Kim, H. J., and Suk, K. (2021). Identification of genetic modifiers of TDP-43: inflammatory activation of astrocytes for neuroinflammation. Cells 10:676. doi: 10.3390/cells10030676
Kim, J. M., Cha, S. H., Choi, Y. R., Jou, I., Joe, E. H., and Park, S. M. (2016). DJ-1 deficiency impairs glutamate uptake into astrocytes via the regulation of flotillin-1 and caveolin-1 expression. Sci. Rep. 6:28823. doi: 10.1038/srep28823
Komilova, N. R., Angelova, P. R., Berezhnov, A. V., Stelmashchuk, O. A., Mirkhodjaev, U. Z., Houlden, H., et al. (2021). Metabolically induced intracellular pH changes activate mitophagy, autophagy, and cell protection in familial forms of Parkinson’s disease. FEBS J. 289, 699–711. doi: 10.1111/febs.16198
Korobeynikov, V. A., Lyashchenko, A. K., Blanco-Redondo, B., Jafar-Nejad, P., and Shneider, N. A. (2022). Antisense oligonucleotide silencing of FUS expression as a therapeutic approach in amyotrophic lateral sclerosis. Nat. Med. 28, 104–116. doi: 10.1038/s41591-021-01615-z
Kovacs, G. G., Breydo, L., Green, R., Kis, V., Puska, G., Lőrincz, P., et al. (2014). Intracellular processing of disease-associated α-synuclein in the human brain suggests prion-like cell-to-cell spread. Neurobiol. Dis. 69, 76–92. doi: 10.1016/j.nbd.2014.05.020
Kumar, A., Fontana, I. C., and Nordberg, A. (2021). Reactive astrogliosis: a friend or foe in the pathogenesis of Alzheimer’s disease. J. Neurochem. 00, 1–16. doi: 10.1111/jnc.15565
Kushner, P. D., Stephenson, D. T., and Wright, S. (1991). Reactive astrogliosis is widespread in the subcortical white matter of amyotrophic lateral sclerosis brain. J. Neuropathol. Exp. Neurol. 50, 263–277. doi: 10.1097/00005072-199105000-00008
Kwon, O. C., Song, J. J., Yang, Y., Kim, S. H., Kim, J. Y., Seok, M. J., et al. (2021). SGK1 inhibition in glia ameliorates pathologies and symptoms in Parkinson disease animal models. EMBO Mol. Med. 13:e13076. doi: 10.15252/emmm.202013076
Lall, D., Lorenzini, I., Mota, T. A., Bell, S., Mahan, T. E., Ulrich, J. D., et al. (2021). C9orf72 deficiency promotes microglial-mediated synaptic loss in aging and amyloid accumulation. Neuron 109, 2275–2291.e8. doi: 10.1016/j.neuron.2021.05.020
LaRocca, T. J., Mariani, A., Watkins, L. R., and Link, C. D. (2019). TDP-43 knockdown causes innate immune activation via protein kinase R in astrocytes. Neurobiol. Dis. 132:104514. doi: 10.1016/j.nbd.2019.104514
Lee, J. H., Han, J. H., Joe, E. H., and Jou, I. (2021). Small heterodimer partner (SHP) aggravates ER stress in Parkinson’s disease-linked LRRK2 mutant astrocyte by regulating XBP1 SUMOylation. J. Biomed. Sci. 28:51. doi: 10.1186/s12929-021-00747-1
Lee, S., Kim, S., Kang, H. Y., Lim, H. R., Kwon, Y., Jo, M., et al. (2020). The overexpression of TDP-43 in astrocytes causes neurodegeneration via a PTP1B-mediated inflammatory response. J. Neuroinflammation 17:299. doi: 10.1186/s12974-020-01963-6
Lee, S. I., Jeong, W., Lim, H., Cho, S., Lee, H., Jang, Y., et al. (2021). APOE4-carrying human astrocytes oversupply cholesterol to promote neuronal lipid raft expansion and Aβ generation. Stem Cell Rep. 16, 2128–2137. doi: 10.1016/j.stemcr.2021.07.017
Leites, E. P., and Morais, V. A. (2021). The PINK1-mediated crosstalk between neural cells and the underlying link to Parkinson’s disease. Cells 10:1395. doi: 10.3390/cells10061395
Leng, F., and Edison, P. (2021). Neuroinflammation and microglial activation in Alzheimer disease: where do we go from here? Nat. Rev. Neurol. 17, 157–172. doi: 10.1038/s41582-020-00435-y
Li, J., Lu, Y., Liang, H., Tang, C., Zhu, L., Zhang, J., et al. (2016). Changes in the expression of FUS/TLS in spinal cords of SOD1 G93A transgenic mice and correlation with motor-neuron degeneration. Int. J. Biol. Sci. 12, 1181–1190. doi: 10.7150/ijbs.16158
Li, J. L., Lin, T. Y., Chen, P. L., Guo, T. N., Huang, S. Y., Chen, C. H., et al. (2021). Mitochondrial function and Parkinson’s disease: from the perspective of the electron transport chain. Front. Mol. Neurosci. 14:797833. doi: 10.3389/fnmol.2021.797833
Li, L., Zhou, J., Han, L., Wu, X., Shi, Y., Cui, W., et al. (2022). The specific role of reactive astrocytes in stroke. Front. Cell. Neurosci. 16:850866. doi: 10.3389/fncel.2022.850866
Liao, F., Li, A., Xiong, M., Bien-Ly, N., Jiang, H., Zhang, Y., et al. (2018). Targeting of nonlipidated, aggregated apoE with antibodies inhibits amyloid accumulation. J. Clin. Invest. 128, 2144–2155. doi: 10.1172/JCI96429
Liddelow, S. A., Guttenplan, K. A., Clarke, L. E., Bennett, F. C., Bohlen, C. J., Schirmer, L., et al. (2017). Neurotoxic reactive astrocytes are induced by activated microglia. Nature 541, 481–487.
Liddelow, S. A., Marsh, S. E., and Stevens, B. (2020). Microglia and astrocytes in disease: dynamic duo or partners in crime? Trends Immunol. 41, 820–835. doi: 10.1016/j.it.2020.07.006
Lin, Y. E., Lin, C. H., Ho, E. P., Ke, Y. C., Petridi, S., Elliott, C. J., et al. (2021). Glial Nrf2 signaling mediates the neuroprotection exerted by Gastrodia elata Blume in Lrrk2-G2019S Parkinson’s disease. eLife 10:e73753. doi: 10.7554/eLife.73753
Lin, Y. T., Seo, J., Gao, F., Feldman, H. M., Wen, H. L., Penney, J., et al. (2018). APOE4 causes widespread molecular and cellular alterations associated with Alzheimer’s disease phenotypes in human iPSC-derived brain cell types. Neuron 98, 1141–1154.e7.
Linnerbauer, M., Wheeler, M. A., and Quintana, F. J. (2020). Astrocyte crosstalk in CNS inflammation. Neuron 108, 608–622. doi: 10.1016/j.neuron.2020.08.012
Liu, M., Qin, L., Wang, L., Tan, J., Zhang, H., Tang, J., et al. (2018). α−synuclein induces apoptosis of astrocytes by causing dysfunction of the endoplasmic reticulum-Golgi compartment. Mol. Med. Rep. 18, 322–332. doi: 10.3892/mmr.2018.9002
Liu, Y., Pattamatta, A., Zu, T., Reid, T., Bardhi, O., Borchelt, D. R., et al. (2016). C9orf72 BAC mouse model with motor deficits and neurodegenerative features of ALS/FTD. Neuron 90, 521–534. doi: 10.1016/j.neuron.2016.04.005
López-Blanch, R., Salvador-Palmer, R., Estrela, J. M., and Obrador, E. (2021). An intercellular flow of glutathione regulated by interleukin 6 links astrocytes and the liver in the pathophysiology of amyotrophic lateral sclerosis. Antioxidants 10:2007. doi: 10.3390/antiox10122007
Mackenzie, I. R., Ansorge, O., Strong, M., Bilbao, J., Zinman, L., Ang, L. C., et al. (2011). Pathological heterogeneity in amyotrophic lateral sclerosis with FUS mutations: two distinct patterns correlating with disease severity and mutation. Acta Neuropathol. 122, 87–98. doi: 10.1007/s00401-011-0838-7
Mahan, T. E., Wang, C., Bao, X., Choudhury, A., Ulrich, J. D., and Holtzman, D. M. (2022). Selective reduction of astrocyte apoE3 and apoE4 strongly reduces Aβ accumulation and plaque-related pathology in a mouse model of amyloidosis. Mol. Neurodegener. 17:13. doi: 10.1186/s13024-022-00516-0
Marini, C., Cossu, V., Kumar, M., Milanese, M., Cortese, K., Bruno, S., et al. (2021). The role of endoplasmic reticulum in the differential endurance against redox stress in cortical and spinal astrocytes from the newborn SOD1(G93A) mouse model of amyotrophic lateral sclerosis. Antioxidants 10:1392. doi: 10.3390/antiox10091392
Miller, T., Cudkowicz, M., Shaw, P. J., Andersen, P. M., Atassi, N., Bucelli, R. C., et al. (2020). Phase 1-2 trial of antisense oligonucleotide tofersen for SOD1 ALS. N. Engl. J. Med. 383, 109–119. doi: 10.1056/NEJMoa2003715
Mills, W. A. III, Woo, A. M., Jiang, S., Martin, J., Surendran, D., Bergstresser, M., et al. (2022). Astrocyte plasticity in mice ensures continued endfoot coverage of cerebral blood vessels following injury and declines with age. Nat. Commun. 13:1794. doi: 10.1038/s41467-022-29475-2
Mizielinska, S., Lashley, T., Norona, F. E., Clayton, E. L., Ridler, C. E., Fratta, P., et al. (2013). C9orf72 frontotemporal lobar degeneration is characterised by frequent neuronal sense and antisense RNA foci. Acta Neuropathol. 126, 845–857. doi: 10.1007/s00401-013-1200-z
Mohamed, L. A., Markandaiah, S. S., Bonanno, S., Pasinelli, P., and Trotti, D. (2019). Excess glutamate secreted from astrocytes drives upregulation of P-glycoprotein in endothelial cells in amyotrophic lateral sclerosis. Exp. Neurol. 316, 27–38. doi: 10.1016/j.expneurol.2019.04.002
Moujalled, D., Grubman, A., Acevedo, K., Yang, S., Ke, Y. D., Moujalled, D. M., et al. (2017). TDP-43 mutations causing amyotrophic lateral sclerosis are associated with altered expression of RNA-binding protein hnRNP K and affect the Nrf2 antioxidant pathway. Hum. Mol. Genet. 26, 1732–1746. doi: 10.1093/hmg/ddx093
Murakami, A., Nakamura, M., Nakamura, Y., Kaneko, S., Yakushiji, Y., and Kusaka, H. (2021). An autopsy case report of neuronal intermediate filament inclusion disease presenting with predominantly upper motor neuron features. Neuropathology 41, 357–365. doi: 10.1111/neup.12741
Nagai, M., Re, D. B., Nagata, T., Chalazonitis, A., Jessell, T. M., Wichterle, H., et al. (2007). Astrocytes expressing ALS-linked mutated SOD1 release factors selectively toxic to motor neurons. Nat. Neurosci. 10, 615–622. doi: 10.1038/nn1876
Neumann, J., Bras, J., Deas, E., O’Sullivan, S. S., Parkkinen, L., Lachmann, R. H., et al. (2009). Glucocerebrosidase mutations in clinical and pathologically proven Parkinson’s disease. Brain 132, 1783–1794. doi: 10.1093/brain/awp044
Ni, J., Ren, Y., Su, T., Zhou, J., Fu, C., Lu, Y., et al. (2021). Loss of TDP-43 function underlies hippocampal and cortical synaptic deficits in TDP-43 proteinopathies. Mol. Psychiatry. 1–15. doi: 10.1038/s41380-021-01346-0
Orre, M., Kamphuis, W., Osborn, L. M., Melief, J., Kooijman, L., Huitinga, I., et al. (2014). Acute isolation and transcriptome characterization of cortical astrocytes and microglia from young and aged mice. Neurobiol. Aging 35, 1–14. doi: 10.1016/j.neurobiolaging.2013.07.008
Osellame, L. D., Rahim, A. A., Hargreaves, I. P., Gegg, M. E., Richard-Londt, A., Brandner, S., et al. (2013). Mitochondria and quality control defects in a mouse model of Gaucher disease–links to Parkinson’s disease. Cell Metab. 17, 941–953. doi: 10.1016/j.cmet.2013.04.014
Ouali Alami, N., Schurr, C., Olde Heuvel, F., Tang, L., Li, Q., Tasdogan, A., et al. (2018). NF-κB activation in astrocytes drives a stage-specific beneficial neuroimmunological response in ALS. EMBO J. 37:e98697. doi: 10.15252/embj.201798697
Pang, S. Y., Lo, R. C. N., Ho, P. W., Liu, H. F., Chang, E. E. S., Leung, C. T., et al. (2022). LRRK2, GBA and their interaction in the regulation of autophagy: implications on therapeutics in Parkinson’s disease. Transl. Neurodegener. 11:5. doi: 10.1186/s40035-022-00281-6
Panicker, N., Dawson, V. L., and Dawson, T. M. (2017). Activation mechanisms of the E3 ubiquitin ligase parkin. Biochem. J. 474, 3075–3086. doi: 10.1042/BCJ20170476
Perez-Nievas, B. G., Johnson, L., Beltran-Lobo, P., Hughes, M. M., Gammallieri, L., Tarsitano, F., et al. (2021). Astrocytic C-X-C motif chemokine ligand-1 mediates β-amyloid-induced synaptotoxicity. J. Neuroinflammation 18:306. doi: 10.1186/s12974-021-02371-0
Polymeropoulos, M. H., Lavedan, C., Leroy, E., Ide, S. E., Dehejia, A., Dutra, A., et al. (1997). Mutation in the alpha-synuclein gene identified in families with Parkinson’s disease. Science 276, 2045–2047. doi: 10.1126/science.276.5321.2045
Price, B. R., Johnson, L. A., and Norris, C. M. (2021). Reactive astrocytes: the nexus of pathological and clinical hallmarks of Alzheimer’s disease. Ageing Res. Rev. 68:101335. doi: 10.1016/j.arr.2021.101335
Rajpurohit, C. S., Kumar, V., Cheffer, A., Oliveira, D., Ulrich, H., Okamoto, O. K., et al. (2020). Mechanistic insights of astrocyte-mediated hyperactive autophagy and loss of motor neuron function in SOD1(L39R) linked amyotrophic lateral sclerosis. Mol. Neurobiol. 57, 4117–4133. doi: 10.1007/s12035-020-02006-0
Ramos-Gonzalez, P., Mato, S., Chara, J. C., Verkhratsky, A., Matute, C., and Cavaliere, F. (2021). Astrocytic atrophy as a pathological feature of Parkinson’s disease with LRRK2 mutation. NPJ Parkinsons Dis. 7:31. doi: 10.1038/s41531-021-00175-w
Richetin, K., Steullet, P., Pachoud, M., Perbet, R., Parietti, E., Maheswaran, M., et al. (2020). Tau accumulation in astrocytes of the dentate gyrus induces neuronal dysfunction and memory deficits in Alzheimer’s disease. Nat. Neurosci. 23, 1567–1579. doi: 10.1038/s41593-020-00728-x
Rizor, A., Pajarillo, E., Johnson, J., Aschner, M., and Lee, E. (2019). Astrocytic oxidative/nitrosative stress contributes to Parkinson’s disease pathogenesis: the dual role of reactive astrocytes. Antioxidants 8:265. doi: 10.3390/antiox8080265
Rojas, F., Cortes, N., Abarzua, S., Dyrda, A., and Van Zundert, B. (2014). Astrocytes expressing mutant SOD1 and TDP43 trigger motoneuron death that is mediated via sodium channels and nitroxidative stress. Front. Cell. Neurosci. 8:24. doi: 10.3389/fncel.2014.00024
Rostami, J., Fotaki, G., Sirois, J., Mzezewa, R., Bergström, J., Essand, M., et al. (2020). Astrocytes have the capacity to act as antigen-presenting cells in the Parkinson’s disease brain. J. Neuroinflammation 17:119. doi: 10.1186/s12974-020-01776-7
Salcedo, C., Andersen, J. V., Vinten, K. T., Pinborg, L. H., Waagepetersen, H. S., Freude, K. K., et al. (2021a). Functional metabolic mapping reveals highly active branched-chain amino acid metabolism in human astrocytes, which is impaired in iPSC-derived astrocytes in Alzheimer’s disease. Front. Aging Neurosci. 13:736580. doi: 10.3389/fnagi.2021.736580
Salcedo, C., Wagner, A., Andersen, J. V., Vinten, K. T., Waagepetersen, H. S., Schousboe, A., et al. (2021b). Downregulation of GABA transporter 3 (GAT3) is associated with deficient oxidative GABA metabolism in human induced pluripotent stem cell-derived astrocytes in Alzheimer’s disease. Neurochem. Res. 46, 2676–2686. doi: 10.1007/s11064-021-03276-3
Sanyal, A., Deandrade, M. P., Novis, H. S., Lin, S., Chang, J., Lengacher, N., et al. (2020). Lysosome and inflammatory defects in GBA1-mutant astrocytes are normalized by LRRK2 inhibition. Mov. Disord. 35, 760–773. doi: 10.1002/mds.27994
Schludi, M. H., Becker, L., Garrett, L., Gendron, T. F., Zhou, Q., Schreiber, F., et al. (2017). Spinal poly-GA inclusions in a C9orf72 mouse model trigger motor deficits and inflammation without neuron loss. Acta Neuropathol. 134, 241–254. doi: 10.1007/s00401-017-1711-0
Schmechel, D. E., Saunders, A. M., Strittmatter, W. J., Crain, B. J., Hulette, C. M., Joo, S. H., et al. (1993). Increased amyloid beta-peptide deposition in cerebral cortex as a consequence of apolipoprotein E genotype in late-onset Alzheimer disease. Proc. Natl. Acad. Sci. U.S.A. 90, 9649–9653. doi: 10.1073/pnas.90.20.9649
Senkevich, K., and Gan-Or, Z. (2020). Autophagy lysosomal pathway dysfunction in Parkinson’s disease; evidence from human genetics. Parkinsonism Relat. Disord. 73, 60–71. doi: 10.1016/j.parkreldis.2019.11.015
Serio, A., Bilican, B., Barmada, S. J., Ando, D. M., Zhao, C., Siller, R., et al. (2013). Astrocyte pathology and the absence of non-cell autonomy in an induced pluripotent stem cell model of TDP-43 proteinopathy. Proc. Natl. Acad. Sci. U.S.A. 110, 4697–4702. doi: 10.1073/pnas.1300398110
Setó-Salvia, N., Esteras, N., De Silva, R., De Pablo-Fernandez, E., Arber, C., Toomey, C. E., et al. (2021). Elevated 4R-tau in astrocytes from asymptomatic carriers of the MAPT 10+16 intronic mutation. J. Cell. Mol. Med. 26, 1327–1331. doi: 10.1111/jcmm.17136
Sierri, G., Dal Magro, R., Vergani, B., Leone, B. E., Formicola, B., Taiarol, L., et al. (2021). Reduced levels of ABCA1 transporter are responsible for the cholesterol efflux impairment in β-amyloid-induced reactive astrocytes: potential rescue from biomimetic HDLs. Int. J. Mol. Sci. 23:102. doi: 10.3390/ijms23010102
Simmnacher, K., Krach, F., Schneider, Y., Alecu, J. E., Mautner, L., Klein, P., et al. (2020). Unique signatures of stress-induced senescent human astrocytes. Exp. Neurol. 334:113466. doi: 10.1016/j.expneurol.2020.113466
Singh, K., Han, K., Tilve, S., Wu, K., Geller, H. M., and Sack, M. N. (2018). Parkin targets NOD2 to regulate astrocyte endoplasmic reticulum stress and inflammation. Glia 66, 2427–2437. doi: 10.1002/glia.23482
Smethurst, P., Risse, E., Tyzack, G. E., Mitchell, J. S., Taha, D. M., Chen, Y. R., et al. (2020). Distinct responses of neurons and astrocytes to TDP-43 proteinopathy in amyotrophic lateral sclerosis. Brain 143, 430–440. doi: 10.1093/brain/awz419
Solano, R. M., Casarejos, M. J., Menéndez-Cuervo, J., Rodriguez-Navarro, J. A., García De Yébenes, J., and Mena, M. A. (2008). Glial dysfunction in parkin null mice: effects of aging. J. Neurosci. 28, 598–611. doi: 10.1523/JNEUROSCI.4609-07.2008
Sonninen, T. M., Hämäläinen, R. H., Koskuvi, M., Oksanen, M., Shakirzyanova, A., Wojciechowski, S., et al. (2020). Metabolic alterations in Parkinson’s disease astrocytes. Sci. Rep. 10:14474. doi: 10.1038/s41598-020-71329-8
Streubel-Gallasch, L., Giusti, V., Sandre, M., Tessari, I., Plotegher, N., Giusto, E., et al. (2021). Parkinson’s disease-associated LRRK2 interferes with astrocyte-mediated alpha-synuclein clearance. Mol. Neurobiol. 58, 3119–3140. doi: 10.1007/s12035-021-02327-8
Strohm, L., and Behrends, C. (2020). Glia-specific autophagy dysfunction in ALS. Semin. Cell Dev. Biol. 99, 172–182. doi: 10.1016/j.semcdb.2019.05.024
Sun, L., Shen, R., Agnihotri, S. K., Chen, Y., Huang, Z., and Büeler, H. (2018). Lack of PINK1 alters glia innate immune responses and enhances inflammation-induced, nitric oxide-mediated neuron death. Sci. Rep. 8:383. doi: 10.1038/s41598-017-18786-w
Sun, S., Sun, Y., Ling, S. C., Ferraiuolo, L., Mcalonis-Downes, M., Zou, Y., et al. (2015). Translational profiling identifies a cascade of damage initiated in motor neurons and spreading to glia in mutant SOD1-mediated ALS. Proc. Natl. Acad. Sci. U.S.A. 112, E6993–E7002. doi: 10.1073/pnas.1520639112
Szebényi, K., Wenger, L. M. D., Sun, Y., Dunn, A. W. E., Limegrover, C. A., Gibbons, G. M., et al. (2021). Human ALS/FTD brain organoid slice cultures display distinct early astrocyte and targetable neuronal pathology. Nat. Neurosci. 24, 1542–1554. doi: 10.1038/s41593-021-00923-4
Sznejder-Pachołek, A., Joniec-Maciejak, I., Wawer, A., Ciesielska, A., and Mirowska-Guzel, D. (2017). The effect of α-synuclein on gliosis and IL-1α, TNFα, IFNγ, TGFβ expression in murine brain. Pharmacol. Rep. 69, 242–251.
Taha, D. M., Clarke, B. E., Hall, C. E., Tyzack, G. E., Ziff, O. J., Greensmith, L., et al. (2022). Astrocytes display cell autonomous and diverse early reactive states in familial amyotrophic lateral sclerosis. Brain 145, 481–489. doi: 10.1093/brain/awab328
Takahashi, S., and Mashima, K. (2022). Neuroprotection and disease modification by astrocytes and microglia in Parkinson disease. Antioxidants 11:170. doi: 10.3390/antiox11010170
Takanashi, M., Funayama, M., Matsuura, E., Yoshino, H., Li, Y., Tsuyama, S., et al. (2018). Isolated nigral degeneration without pathological protein aggregation in autopsied brains with LRRK2 p.R1441H homozygous and heterozygous mutations. Acta Neuropathol. Commun. 6:105. doi: 10.1186/s40478-018-0617-y
Takeda, T., Iijima, M., Shimizu, Y., Yoshizawa, H., Miyashiro, M., Onizuka, H., et al. (2019). p.N345K mutation in TARDBP in a patient with familial amyotrophic lateral sclerosis: an autopsy case. Neuropathology 39, 286–293. doi: 10.1111/neup.12559
Tong, J., Ang, L. C., Williams, B., Furukawa, Y., Fitzmaurice, P., Guttman, M., et al. (2015). Low levels of astroglial markers in Parkinson’s disease: relationship to α-synuclein accumulation. Neurobiol. Dis. 82, 243–253. doi: 10.1016/j.nbd.2015.06.010
Tong, J., Huang, C., Bi, F., Wu, Q., Huang, B., Liu, X., et al. (2013). Expression of ALS-linked TDP-43 mutant in astrocytes causes non-cell-autonomous motor neuron death in rats. EMBO J. 32, 1917–1926. doi: 10.1038/emboj.2013.122
Umoh, M. E., Dammer, E. B., Dai, J., Duong, D. M., Lah, J. J., Levey, A. I., et al. (2018). A proteomic network approach across the ALS-FTD disease spectrum resolves clinical phenotypes and genetic vulnerability in human brain. EMBO Mol. Med. 10, 48–62. doi: 10.15252/emmm.201708202
Ungerleider, K., Beck, J., Lissa, D., Turnquist, C., Horikawa, I., Harris, B. T., et al. (2021). Astrocyte senescence and SASP in neurodegeneration: tau joins the loop. Cell Cycle 20, 752–764. doi: 10.1080/15384101.2021.1909260
Vahsen, B. F., Gray, E., Thompson, A. G., Ansorge, O., Anthony, D. C., Cowley, S. A., et al. (2021). Non-neuronal cells in amyotrophic lateral sclerosis – from pathogenesis to biomarkers. Nat. Rev. Neurol. 17, 333–348. doi: 10.1038/s41582-021-00487-8
Valori, C. F., Brambilla, L., Martorana, F., and Rossi, D. (2014). The multifaceted role of glial cells in amyotrophic lateral sclerosis. Cell. Mol. Life Sci. 71, 287–297. doi: 10.1007/s00018-013-1429-7
Van Damme, P., Bogaert, E., Dewil, M., Hersmus, N., Kiraly, D., Scheveneels, W., et al. (2007). Astrocytes regulate GluR2 expression in motor neurons and their vulnerability to excitotoxicity. Proc. Natl. Acad. Sci. U.S.A. 104, 14825–14830. doi: 10.1073/pnas.0705046104
Velebit, J., Horvat, A., Smolič, T., Prpar Mihevc, S., Rogelj, B., Zorec, R., et al. (2020). Astrocytes with TDP-43 inclusions exhibit reduced noradrenergic cAMP and Ca2+ signaling and dysregulated cell metabolism. Sci. Rep. 10:6003. doi: 10.1038/s41598-020-62864-5
Viejo, L., Noori, A., Merrill, E., Das, S., Hyman, B. T., and Serrano-Pozo, A. (2022). Systematic review of human post-mortem immunohistochemical studies and bioinformatics analyses unveil the complexity of astrocyte reaction in Alzheimer’s disease. Neuropathol. Appl. Neurobiol. 48:e12753. doi: 10.1111/nan.12753
Vilemagne, V. L., Harada, R., Dore, V., Furumoto, S., Mulligan, R., Kudo, Y., et al. (2022a). Assessing reactive astrogliosis with (18)F-SMBT-1 across the Alzheimer’s disease spectrum. J. Nucl. Med. doi: 10.2967/jnumed.121.263255 [Epub ahead of print].
Vilemagne, V. L., Harada, R., Dore, V., Furumoto, S., Mulligan, R., Kudo, Y., et al. (2022b). First-in-human evaluation of (18)F-SMBT-1, a novel (18)F-labeled MAO-B PET tracer for imaging reactive astrogliosis. J. Nucl. Med. doi: 10.2967/jnumed.121.263254 [Epub ahead of print].
Wang, C., Xiong, M., Gratuze, M., Bao, X., Shi, Y., Andhey, P. S., et al. (2021). Selective removal of astrocytic APOE4 strongly protects against tau-mediated neurodegeneration and decreases synaptic phagocytosis by microglia. Neuron 109, 1657–1674.e7. doi: 10.1016/j.neuron.2021.03.024
Wang, H., Kulas, J. A., Wang, C., Holtzman, D. M., Ferris, H. A., and Hansen, S. B. (2021). Regulation of beta-amyloid production in neurons by astrocyte-derived cholesterol. Proc. Natl. Acad. Sci. U.S.A. 118:e2102191118. doi: 10.1073/pnas.2102191118
Wei, Z. D., and Shetty, A. K. (2021). Treating Parkinson’s disease by astrocyte reprogramming: progress and challenges. Sci. Adv. 7:eabg3198. doi: 10.1126/sciadv.abg3198
Wilson, H., Dervenoulas, G., Pagano, G., Tyacke, R. J., Polychronis, S., Myers, J., et al. (2019). Imidazoline 2 binding sites reflecting astroglia pathology in Parkinson’s disease: an in vivo11C-BU99008 PET study. Brain 142, 3116–3128. doi: 10.1093/brain/awz260
Wongworawat, Y. C., Liu, Y. A., Raghavan, R., White, C. L., Dietz, R., Zuppan, C., et al. (2020). Aggressive FUS-mutant motor neuron disease without profound spinal cord pathology. J. Neuropathol. Exp. Neurol. 79, 365–369. doi: 10.1093/jnen/nlaa011
Yamanaka, K., and Komine, O. (2018). The multi-dimensional roles of astrocytes in ALS. Neurosci. Res. 126, 31–38. doi: 10.1016/j.neures.2017.09.011
Yang, C., Qiao, T., Yu, J., Wang, H., Guo, Y., Salameh, J., et al. (2022). Low-level overexpression of wild type TDP-43 causes late-onset, progressive neurodegeneration and paralysis in mice. PLoS One 17:e0255710. doi: 10.1371/journal.pone.0255710
Yang, W., Wang, G., Wang, C. E., Guo, X., Yin, P., Gao, J., et al. (2015). Mutant alpha-synuclein causes age-dependent neuropathology in monkey brain. J. Neurosci. 35, 8345–8358. doi: 10.1523/JNEUROSCI.0772-15.2015
Yu, M., Zhao, X., Wu, W., Wang, Q., Liu, J., Zhang, W., et al. (2022). Widespread mislocalization of FUS is associated with mitochondrial abnormalities in skeletal muscle in amyotrophic lateral sclerosis with FUS mutations. J. Neuropathol. Exp. Neurol. 81, 172–181. doi: 10.1093/jnen/nlac004
Zamudio, F., Loon, A. R., Smeltzer, S., Benyamine, K., Navalpur Shanmugam, N. K., Stewart, N. J. F., et al. (2020). TDP-43 mediated blood-brain barrier permeability and leukocyte infiltration promote neurodegeneration in a low-grade systemic inflammation mouse model. J. Neuroinflammation 17:283. doi: 10.1186/s12974-020-01952-9
Zhang, Y. J., Gendron, T. F., Ebbert, M. T. W., O’Raw, A. D., Yue, M., Jansen-West, K., et al. (2018). Poly(GR) impairs protein translation and stress granule dynamics in C9orf72-associated frontotemporal dementia and amyotrophic lateral sclerosis. Nat. Med. 24, 1136–1142. doi: 10.1038/s41591-018-0071-1
Zhang, Y. J., Gendron, T. F., Grima, J. C., Sasaguri, H., Jansen-West, K., Xu, Y. F., et al. (2016). C9ORF72 poly(GA) aggregates sequester and impair HR23 and nucleocytoplasmic transport proteins. Nat. Neurosci. 19, 668–677. doi: 10.1038/nn.4272
Zhang, Z., Shen, Y., Luo, H., Zhang, F., Peng, D., Jing, L., et al. (2018). MANF protects dopamine neurons and locomotion defects from a human α-synuclein induced Parkinson’s disease model in C. elegans by regulating ER stress and autophagy pathways. Exp. Neurol. 308, 59–71. doi: 10.1016/j.expneurol.2018.06.016
Zhao, C., Devlin, A. C., Chouhan, A. K., Selvaraj, B. T., Stavrou, M., Burr, K., et al. (2020). Mutant C9orf72 human iPSC-derived astrocytes cause non-cell autonomous motor neuron pathophysiology. Glia 68, 1046–1064. doi: 10.1002/glia.23761
Zhao, M., Jiang, X. F., Zhang, H. Q., Sun, J. H., Pei, H., Ma, L. N., et al. (2021). Interactions between glial cells and the blood-brain barrier and their role in Alzheimer’s disease. Ageing Res. Rev. 72:101483. doi: 10.1016/j.arr.2021.101483
Zhu, J., Yang, Y., Ma, W., Wang, Y., Chen, L., Xiong, H., et al. (2022). Antiepilepticus effects of tetrahedral framework nucleic acid via inhibition of gliosis-induced downregulation of glutamine synthetase and increased AMPAR internalization in the postsynaptic membrane. Nano Lett. 22, 2381–2390. doi: 10.1021/acs.nanolett.2c00025
Zhu, N., Santos-Santos, M., Illán-Gala, I., Montal, V., Estellés, T., Barroeta, I., et al. (2021). Plasma glial fibrillary acidic protein and neurofilament light chain for the diagnostic and prognostic evaluation of frontotemporal dementia. Transl. Neurodegener. 10:50. doi: 10.1186/s40035-021-00275-w
Keywords: astrocyte, neurodegeneration, gene variant, AD, ALS, PD
Citation: Huang J, Li C and Shang H (2022) Astrocytes in Neurodegeneration: Inspiration From Genetics. Front. Neurosci. 16:882316. doi: 10.3389/fnins.2022.882316
Received: 23 February 2022; Accepted: 06 June 2022;
Published: 24 June 2022.
Edited by:
Tiziano Balzano, Centro Integral en Neurociencias A.C. HM CINAC, SpainReviewed by:
Ekaterina Fedotova, Research Center of Neurology, RussiaCopyright © 2022 Huang, Li and Shang. This is an open-access article distributed under the terms of the Creative Commons Attribution License (CC BY). The use, distribution or reproduction in other forums is permitted, provided the original author(s) and the copyright owner(s) are credited and that the original publication in this journal is cited, in accordance with accepted academic practice. No use, distribution or reproduction is permitted which does not comply with these terms.
*Correspondence: Huifang Shang, aGZzaGFuZzIwMDJAMTI2LmNvbQ==
Disclaimer: All claims expressed in this article are solely those of the authors and do not necessarily represent those of their affiliated organizations, or those of the publisher, the editors and the reviewers. Any product that may be evaluated in this article or claim that may be made by its manufacturer is not guaranteed or endorsed by the publisher.
Research integrity at Frontiers
Learn more about the work of our research integrity team to safeguard the quality of each article we publish.