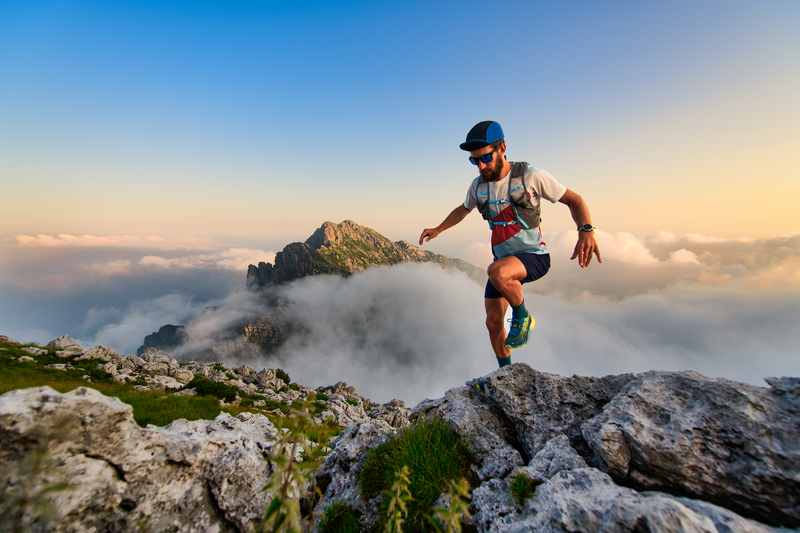
95% of researchers rate our articles as excellent or good
Learn more about the work of our research integrity team to safeguard the quality of each article we publish.
Find out more
REVIEW article
Front. Neurosci. , 24 May 2022
Sec. Neurodegeneration
Volume 16 - 2022 | https://doi.org/10.3389/fnins.2022.872509
This article is part of the Research Topic Translational Imaging in Neurodegenerative Proteinopathies View all 9 articles
The successful development and translation of PET imaging agents targeting β-amyloid plaques and hyperphosphorylated tau tangles have allowed for in vivo detection of these hallmarks of Alzheimer’s disease (AD) antemortem. Amyloid and tau PET have been incorporated into the A/T/N scheme for AD characterization and have become an integral part of ongoing clinical trials to screen patients for enrollment, prove drug action mechanisms, and monitor therapeutic effects. Meanwhile, preclinical PET imaging in animal models of AD can provide supportive information for mechanistic studies. With the recent advancement of gene editing technologies and AD animal model development, preclinical PET imaging in AD models will further facilitate our understanding of AD pathogenesis/progression and the development of novel treatments. In this study, we review the current state-of-the-art in preclinical PET imaging using animal models of AD and suggest future research directions.
Dementia is a category of neurodegenerative diseases that mainly affect the daily lives of older people and is characterized by progressive loss of memory, communication, problem-solving/thinking, and motorsensory abilities. The common types of dementia include vascular dementia, frontotemporal dementia, dementia with Lewy bodies, and Alzheimer’s disease (AD), which is the most common type and accounts for 60–80% of overall dementia cases (Alzheimer’s Association, 2021). Globally, there are 350,000 new cases of early onset dementia per year, and by 2050, 107 million people are predicted to be living with AD, among which 68% reside in the low- and middle-income countries (Global Burden of Disease Study).
The pathological hallmarks of AD are β-amyloid (Aβ)-containing extracellular plaques and oligomers and tau-containing intracellular neurofibrillary tangles (NFTs). The plaques and oligomers interfere with neuron-to-neuron communication at synapses, leading to neurodegeneration. Tau tangles block the transport of nutrients and other molecules inside the neurons, which contributes to neural death. In addition, the Aβ plaque and tau proteins can activate the microglia, which clears these toxic proteins and dead cells but may result in chronic inflammation (Long and Holtzman, 2019). Atrophy, a decrease in brain volume owing to the loss of synapses, dendrites, and neuronal cell bodies, is another biomarker for AD progression (Pini et al., 2016; Halliday, 2017). In addition, the decrease in glucose metabolism further compromises the brain’s function (Wang et al., 2016). Familial early-onset AD (FAD) is associated with mutated genes such as APP, PSEN1, PSEN2, and MAPT, which also significantly increase the risk for late-onset AD (LOAD) (Ryan and Rossor, 2010), while apolipoprotein E variant ε4 (APOEε4) (Kim et al., 2009) and triggering receptor expressed on myeloid cells 2 (TREM2) are associated with the highest risk of developing LOAD (Wolfe et al., 2018). These dominantly inherited Alzheimer’s disease (DIAD) caused by rare genetic mutations are associated with increased levels of Aβ and tau, decreased glucose metabolism, and brain atrophy 10–20 years before the symptoms set in. In addition, multiple enzymes are associated with AD, including the β-site APP cleaving enzyme 1 (BACE1) (Das and Yan, 2017), caspase 3 (Rohn, 2010), and aspartyl cathepsin (Haque et al., 2008), among others.
Animal models of AD have become essential for studying the pathogenesis and progression of AD pathology and for validating the mechanism of action of novel therapeutics before translation to human trials (Alzheimer’s Association, 2021). Many animal models have been developed to mimic the pathophysiological processes and progression mechanisms of AD and to preclinically test treatment methods (Figure 1). Both invertebrate and vertebrate animals have been used in modeling the aging and certain aspects of AD processes, as genetically modified animals that recapitulate certain traits of AD are needed to understand the pathological and biological mechanisms of AD. Because there has not been a rodent model that completely recapitulates human AD, rodent models have been mainly used for proving mechanisms of action for therapeutic interventions or testing the target binding specificity of imaging probes. A fully characterized AD animal model with stable phenotypes and a clear disease onset time can greatly help address the specific scientific questions.
Many neuroimaging methods, such as magnetic resonance imaging (MRI; structural and functional), computerized tomography (CT), and positron emission tomography (PET), have been increasingly employed to evaluate AD neurodegeneration. PET imaging uses radiolabeled tracers to detect and quantify cerebral and metabolic changes by targeting specific biomarkers that are associated with AD. Fluorodeoxyglucose (FDG) PET detects brain metabolism and amyloid PET that quantifies the amyloid deposit has been developed to understand AD pathogenesis and to monitor disease progression and therapeutic effects. Other neuro-specific, inflammation- and metabolic-associated radiotracers are under development for AD studies. In this study, we discuss the AD animal models that are relevant in AD PET imaging studies and summarize the PET tracers that have been tested in AD animal models and the findings from these studies.
In the field of neurodegenerative diseases, mice are the most commonly used animals for their biological features that are similar to those of humans, easily manipulated genetics to mimic human conditions and diseases, and a relatively short life span (1.5–2 years). Because mice do not develop AD naturally, transgenic mice are generated to recapitulate certain AD pathological features to fit the research needs (Table 1). The genes associated with the early onset of AD have been the main targets for transgenic manipulations.
The first Aβ plaque-developing mouse model is APP (V717F), which progressively develops extracellular thioflavin S-positive Aβ deposits, neuritic plaques, synaptic loss, astrocytosis, and microgliosis (Games et al., 1995). This model can be used for testing therapeutic drugs targeting amyloidosis. The commonly studied Swedish APP mutation (APPswe) K670N/M671L carries a transgene coding for the 695-amino acid isoform of human Aβ precursor protein bearing the Swedish mutation (Sturchler-Pierrat et al., 1997). This mouse model expresses high concentrations of the mutant Aβ, develops significant amyloid plaques, and displays memory deficits. It is useful for studying APP expression, amyloid plaque formation, neuronal decline, and memory loss associated with AD, as well as drug discoveries. Recently, Xu et al. (2015) achieved the amyloid deposition using murine genes carrying the APPswe mutation, indicating murine Aβ peptides can produce amyloid deposits that morphologically resemble those found in human AD.
The first NFT-developing mouse was achieved by expressing the familial FTLD MAPT mutation P301L under the control of the mouse prion promoter (Lewis et al., 2000). Allen et al. (2002) used mouse Thy1.2 promoter to reach a 2-fold increase in the expression of P301S mutant FTLD-tau compared with endogenous tau, with NFTs forming at 5 months of age. The first human MAPT transgenic model (ALZ7) with the human THY1.2 promoter expressed only a low level of the transgenic gene but achieved deposition of hyperphosphorylated tau in the somatodendritic domain (Gotz et al., 1995). The rTg4510 model uses a reversible binary transactivator system to achieve a high level of tau expression (13-fold) with P301L, NFT-like lesions, neuronal loss, cognitive impairment, and brain atrophy at an earlier time frame (Santacruz et al., 2005). This mouse develops progressive intracellular tau aggregations in the corticolimbic areas and forebrain atrophy. The human Tau (hTau) mice (Andorfer et al., 2003) were generated by crossing 8c mice expressing human 3R and 4R tau isoforms (Duff et al., 2000) with tau knockout (KO) mice generated by targeted disruption of exon one on the MAPT gene (Tucker et al., 2001). This mouse model expresses all six isoforms of hTau but lacks mouse tau. It develops age-associated tau pathology that appears most severe in the neocortex and hippocampus. No tau pathology was found in both 8c mice and tau KO mice. Recently, Saito et al. (2019) used a homologous recombination approach to replace the entire murine Mapt gene with the human ortholog to create a MAPT knock-in (KI) mouse model that expresses all six tau isoforms present in humans. They cross-bred the MAPT KI mice with single App KI mice to generate the APP/MAPT double knock-in (dKI) mice that exhibit higher tau phosphorylation than the single MAPT KI mice (Saito et al., 2019).
The APOE family consists of three isoforms: APOE2, APOE3, and APOE4, with APOE4 being the greatest genetic risk factor for AD (Kim et al., 2009). APOE-target replacement mice (APOE-TR Mice), in which the m-APOE coding sequence is replaced by that of an h-APOE allele, display alterations in synaptic number and structure, network connectivity, and behavior based on the specific APOE isoform expressed (Ji et al., 2003; Wang et al., 2005; Tai et al., 2011; Zhu et al., 2012; Dumanis et al., 2013; Koutseff et al., 2014; Neustadtl et al., 2017; Lewandowski et al., 2020). These mice exhibit isoform-specific differences in lipid physiology and synaptic function. Mice with h-APOE4 exhibit earlier and more severe AD pathology and memory decline (Bour et al., 2008; Sun et al., 2017; Lewandowski et al., 2020).
Triggering receptor expressed on myeloid cells 2 (TREM2) is expressed in microglia, and its genetic variants R47H and Y38C are linked to AD, frontotemporal dementia, and Nasu-Hakola disease, which is an early onset of dementia characterized by white matter pathology (Yaghmoor et al., 2014). While Trem2 variant R47H is largely associated with late-onset AD, Trem2 variant Y38C is associated with the development of early onset dementia (Jadhav et al., 2020). Both Trem2R47H and Trem2Y38C mice were generated using the CRISPR/Cas9 technique to introduce the point mutations of Trem2R47H and Trem2Y38C. TREM2 R47H homozygous mice exhibit a novel splice variant resulting in partial expression of mRNA and protein in the brain (Xiang et al., 2018). While mice harboring the Trem2 Y38C exhibited normal expression levels of TREM2, alterations were observed in the expression of neuronal and oligodendrocyte/myelin genes, along with regional decreases in synaptic protein levels, particularly in the hippocampus (Jadhav et al., 2020).
In addition to the “single-gene models” described above, combinations of AD-related genes have also been introduced in mice using transgenic technology. These combinatorial genetic models present greater phenotypical diversity, and thus offer more options for preclinical studies. The APP/PS1 mouse model was generated by administration of both APPswe mutant (K595N/M596L) and the ΔE9 mutant of presenilin 1 (PS1), which is an essential component of γ-secretase, the enzyme responsible for APP cleavage. Mutations in PS1 lead to dominant inheritance of early-onset FAD (Jankowsky et al., 2001). The mice develop Aβ deposits in the brain by 6–7 months of age, with 15-month-old females presenting a 5-fold (Aβ42) and 10-fold (Aβ40) increase in Aβ deposits in the cerebellum compared to males (Jankowsky et al., 2004; Ordonez-Gutierrez et al., 2016).
Although there are no reports that APP, PS1, and tau mutations occurring simultaneously in humans, the 3 × Tg strain is the most widely used model that presents aggregated Aβ and synaptic dysfunction. This model is created by co-injecting two constructs expressing APPswe and P301L mutant tau into oocytes obtained from PS1 M146V KI mice. These triple transgenic mice express mutant APP, PSEN2, and MAPT and show age-dependent accumulation of Aβ plaques and neurofibrillary tangle-like pathology, starting around 4 months of age (Grueninger et al., 2010).
The 5 × FAD strain combines the APPswe mutation with the Florida (I716V) and London (V717I) mutations of APP, as well as the M146L and L286V mutations of PSEN1. These mice show progressive cognitive deficits with several pathological hallmarks of AD, such as Aβ plaques, gliosis, synaptic degeneration, and neuronal loss, and develop tau pathology (Oakley et al., 2006).
This double mutant strain carries a humanized APOE4 knock-in mutation and a CRISPR/cas9-generated R47H point mutation of the Trem2 gene. This strain does not produce any severe phenotypes, even late in life, allowing a better understanding of the effect of AD risk factors in the context of aging (Kotredes et al., 2021).
Several AD-related KO mice are generated for understanding the pathophysiological role of AD-related proteins, including APP, MAPT, BACE1, APOE, PSEN1, PSEN2, and Trem E (Table 2).
APP KO mice display deficits in forelimb grip strength and locomotor activity and an age-related deficit in retention of memory for an aversive experience (Senechal et al., 2008). MAPT KO mice have been reported to show less evidence of brain dysfunction (Harada et al., 1994; Dawson et al., 2001; Morris et al., 2011). PSEN1 KO mice exhibit perinatal lethality in homozygous animals, which die shortly after birth (Shen et al., 1997). PSEN2 KO mice are viable and normal in growth and size and do not display any gross brain abnormalities, astrogliosis, or behavioral abnormalities by 12 months of age, and no deficit in APP processing (Herreman et al., 1999). APOE KO mice display poor lipoprotein clearance with subsequent accumulation of cholesterol-ester-enriched particles in the blood (Piedrahita et al., 1992). The systemic proinflammatory status of APOE KO mice also makes them good candidates for studying risk factors for AD (Lo Sasso et al., 2016). TREM KO mice show no behavioral and cognitive deficit (Kang et al., 2018). BACE1 KO mice do not display any gross physical or behavioral abnormalities (Cai et al., 2001).
Alzheimer’s disease models can also be generated by chemical induction. Synthetic Aβ and tau aggregates have been intraperitoneally injected to induce cerebral amyloids and intracerebral tauopathy (Gotz et al., 2001; Clavaguera et al., 2014). Intracranial injection of okadaic acid, a protein phosphatase inhibitor, increased tau phosphorylation and protein aggregation in distinct brain regions (Baker and Gotz, 2016). Intracranial injection of synthetic Aβ aggregates into P301L tau transgenic mice can accelerate NFT formation (Peeraer et al., 2015). Brain lysates from both transgenic mice and patients with AD also induce nucleation of protein aggregation along with neuronal projections in healthy mice or mice with preexisting AD pathology (Bolmont et al., 2007; Clavaguera et al., 2009; He et al., 2018). Lipopolysaccharide (LPS) acts as a Toll-like receptor 4 ligand to activate microglia to produce proinflammatory cytokines such as TNF-α, IL-1β, prostaglandin E2 (PGE2), and nitric oxide (NO) in the central nervous system (Heneka et al., 2015). The administration of LPS to animals induces cognitive impairment (Shaw et al., 2001; Choi et al., 2012) and high levels of Aβ1–42 (Zhao et al., 2019).
Other chemicals used for the induction of cognitive impairment include heavy metals (e.g., aluminum, cobalt, and cooper), scopolamine, ethanol, colchicine, an excitotoxin, streptozotocin, and sodium azide, among others, and have been nicely summarized in the review (More et al., 2016; Götz et al., 2018).
Brain injury is associated with elevated Aβ levels and tau phosphorylation (Yu et al., 2012), but not the formation of plaques and NFTs. In transgenic hTau and 3xTg mice, brain injury accentuates the development of tau pathology and Aβ accumulation (Tran et al., 2011; Ojo et al., 2013).
There is no AD mouse model that recapitulates all aspects of human AD. Even with the high levels of amyloid protein, the mice still do not display human-like cognitive deficits. The Aβ plaques in mice are often diffuse or exhibit fewer crosslinking fibrils even when they appear condensed. The tau pathology also shows a certain difference from humans, with a wide and uncontrollable range of expression levels in some AD model mice. Because there are dozens of different genes that are associated with AD, the different combinations of mutations in these genes, in conjunction with varying environmental stimulators, will contribute to each unique AD case (Naj and Schellenberg, 2017). Furthermore, the offspring of AD transgenic and wild mice are more likely to develop memory loss, indicating there are AD-associated genetics or environmental factors yet to be elucidated, which drives the continuing efforts for better mouse models. In 2016, the NIH started the MODEL-AD consortium to engineer mice with different genetic mutations associated with early- or late-onset AD (model-ad.org).
Compared to mice, rats are easier to handle and have larger brain sizes for easier surgical operation and imaging analysis (Ellenbroek and Youn, 2016). Both genetic and non-genetic rat AD models have been developed (Table 3). However, unlike transgenic AD mouse models, not as many AD rat models are available for scientific research and rats appear to be more resilient to AD pathology than mice (Charreau et al., 1996).
The APP transgenic rats appear to have lower expression levels of the APP transgene than the mouse AD model (Benedikz et al., 2009). TgAPPswe is the first APP transgenic rat that overexpresses human APP with Swedish mutation (K670N and M671L) (Ruiz-Opazo et al., 2004), with only a 56.8% increase in the expression level of APP mRNA, 21% increase for Aβ42, and 6% for Aβ40 in the brain. No AD-related pathology was found in these animals up to the age of 18 months. The Tg6590 rat generated by Fokesson et al. is another model that carries human APP with the Swedish mutation. The levels of both Aβ species are increased by 65% in the hippocampus and 40% in the cortex of 11-month-old animals. The rats display learning and memory deficits in the Morris water maze at 9 months and altered spontaneous behavior measured in open field (Kloskowska et al., 2010). The McGill-R-Thy1-APP rat model expressed hAPP751 bearing the Swedish and Indiana mutations, with intracellular Aβ inclusions detected as early as postnatal day 7 and Aβ plaques at 6–9 months of age (Leon et al., 2010). The Tg1116 rats express a human APP minigene containing both the Swedish and Indiana familial AD mutations (Flood et al., 2009). APP21 and APP31 express a human APP double mutant construct containing the Swedish and Indiana AD mutations driven by the ubiquitin-C promoter. The APP transgene is reported to be expressed in the brain, in neuronal but not glial cells (Agca et al., 2008). No pathological or behavioral studies have been published yet. The double homozygous Tg478/Tg1116 rats were generated by crossing Tg478 which expresses human APP with the Swedish mutation (Flood et al., 2009) and Tg1116. The rats produce sufficient levels of Aβ for amyloid deposition to occur by the age of 17–18 months (Flood et al., 2009).
TgF344-AD rats co-express APPswe and PS1ΔE9 transgenes and present with age-dependent cerebral amyloidosis that precedes tauopathy, gliosis, apoptotic loss of neurons in the cerebral cortex and hippocampus, and cognitive dysfunction (Cohen et al., 2013).
UKUR25 rats express human APP containing the Swedish and Indiana (V717F) mutations, and mutated PS1 (M146L). The main pathological feature was an intracellular accumulation of Aβ in neurons of the hippocampus and cortex without extracellular amyloid up to 24 months of age. Mild impairment in acquisition learning was found in 16-month-old male rats, with an increase in tau phosphorylation at S396 and S404 ERK2 sites (Echeverria et al., 2004a,b).
The PSAPP model rats express hAPP695 carrying the Swedish and London (K670N/M671L and V717I, respectively) mutations together with PSEN1 carrying the Finnish mutation (PS1, ΔE9) and develop Aβ deposition around 7 months of age (Flood et al., 2009). This strain was created by crossing double homozygous Tg478/Tg1116 rats with Tg11587 that carries a human PS-1 transgene with the familial AD mutation M146V. The homozygous rats produce sufficient levels of Aβ for amyloid deposition to occur by the age of 7 months. The triple homozygous transgenic rat, Tg478/Tg1116/Tg11587, has also been called the PSAPP rat. The compact amyloid deposits were found to be associated with activated microglia, reactive astrocytes, and phosphorylated tau immunoreactivity.
Overexpression of human non-mutated truncated tau encompassing 3R domains led to the first rat model of progressive cortical neurofibrillary degeneration (Filipcik et al., 2012). This transgenic rat expresses a truncated form of the human tau protein (truncated at amino acid positions 151–391), which is found in the brains of sporadic AD patients (Benedikz et al., 2009).
The aforementioned chemicals used to generate AD mouse models can also be employed in rats to create AD phenotypes (More et al., 2016; Götz et al., 2018).
Non-human primates such as rhesus macaques and marmosets are not known to develop AD but do accumulate Aβ deposits and show tauopathy in their aged brains (Paspalas et al., 2018; Haque and Levey, 2019; Arnsten et al., 2021b; Datta et al., 2021; Leslie et al., 2021). Intracranial injection of Aβ42 and thiorphan, an inhibitor of neprilysin that is responsible for Aβ clearance, has been employed to generate an AD model in middle-aged (16–17 years) rhesus monkeys (Li et al., 2010). Significant intracellular accumulation of Aβ was found in the neurons of the basal ganglia, cortex, and hippocampus, accompanied by neuronal atrophy and loss. Two injections of an adeno-associated virus expressing a double tau mutation (AAV-P301L/S320F) in the left hemisphere of rhesus monkeys result in misfolded tau propagation similar to that in humans. Tau spreading is accompanied by robust neuroinflammatory response driven by TREM2 + microglia, with biomarkers of inflammation and neuronal loss in cerebrospinal fluid and plasma (Beckman et al., 2021).
Other non-primate large animals used for AD modeling include domestic animals such as dogs and cats as well as farm animals including pigs, sheep, and cows. Aged dogs develop plaque pathology and cerebral amyloid angiopathy (Yu et al., 2011), as well as a dementia-like syndrome resembling human AD (Prpar Mihevc and Majdic, 2019; Abey et al., 2021). Tau dysfunction and tangles have been reported and associated with cognitive decline (Yu et al., 2011; Schmidt et al., 2015; Smolek et al., 2016). Cats also develop plaques, tangles, and brain atrophy along with cognitive decline as they age (Chambers et al., 2015; Fiock et al., 2020). Two transgenic pig models of AD have been reported using minipigs. The first one carries an hAPP transgene with the Swedish mutation driven by the human BDGFβ promoter, resulting in high levels of brain-specific Aβ expression (Kragh et al., 2009), and the second minipig model carries three copies of a transgene expressing the 695 variant of hAPP with the Swedish mutation and a human PSEN1 transgene with the M146L mutation (Jakobsen et al., 2016). Intraneuronal accumulation of Aβ1–42 was detected in two pigs: one at 10 months and one at 18 months. Plaque- and tangle-like pathologies have also been seen after traumatic brain injury (TBI) in pigs (Hoffe and Holahan, 2019). Tau pathology and Aβ plaques have been identified in aged sheep and goats as well (Braak et al., 1994).
The development and validation of the first-in-class Aβ PET radiotracer, the thioflavin T-derived Pittsburgh compound B ([11C]PIB or PIB), was a milestone in AD imaging. It not only allows the direct in vivo visualization and quantification of Aβ plaque in living subjects (Klunk et al., 2004) but also paves the road for the development and FDA approval of its 18F-labeled analog ([18F]flutemetamol), the stilbene derivative [18F]florbetaben, and the styrylpyridine derivative [18F]florbetapir, the use of which have become impactful in AD clinical trials and diagnosis. The intrinsic fluorescent characteristics of these imaging probes and their analogs allow for the microscopic assessment of their binding selectivity and binding preference to different forms of Aβ plaques and Aβ plaques at different locations (e.g., parenchymal and cerebral amyloid angiopathy, CAA) (Bacskai et al., 2003; Fodero-Tavoletti et al., 2012).
Many Aβ imaging tracers have been evaluated using multiple different AD animal models, mainly in AD mice. PIB has been tested in AD mice of APPswe, APP/PS1, 3 × Tg, 5 × FAD, Tg2576, and APP23 (Ni, 2021). Initial reports on PIB binding in Tg2576 and APP/PS1 mice at advanced ages were negative, even with abundant Aβ pathology (Klunk et al., 2005; Toyama et al., 2005); while PIB binding in APP23 mice was positive (Maeda S. et al., 2007). These data led to the hypothesis that the paucity of high-affinity binding sites for PIB in murine Aβ plaques requires very high molar activity PIB for successful imaging in murine AD models. Snellman et al. (2013) compared PIB uptake longitudinally in the brains of multiple AD mouse models and found higher PIB uptake in the cortex of APP23 mice compared with the wild-type controls and no difference in APP/PS1 and Tg2576 mice with their corresponding controls, consistent with previous results from other groups (Figure 2A). They also compared the thioflavin-T staining patterns and found that APP23 mice form large and compact human-like Aβ deposits, whereas Tg2576 mice and APP/PS1 mice form sparse fibrillar deposits. The results suggest that PIB binding is highly dependent on the AD model and the associated higher-order fibrillar structure rather than simple β sheets. At a microscopic level, the Aβ plaques formed in early onset autosomal dominant AD and sporadic AD brains have different levels of non-fibrillar Aβ species (Querol-Vilaseca et al., 2019), and the Aβ deposits in familial AD, sporadic AD, and cerebral amyloid angiopathy manifest different conformations (Condello et al., 2018). Further understanding of the interactions of the imaging probes with amyloid plaques of different forms will help with the development of probes targeting the various forms of misfolded Aβ proteins in the brain (Biancalana and Koide, 2010).
Figure 2. (A) [11C]PIB binding to Aβ deposits varies by mouse strains. APP23: Extensive Aβ deposits; Tg2576: Mild Aβ deposits; APP-swePS1dE9: Extensive Aβ deposits. This figure was adapted and modified from Snellman et al. J Nucl Med. 2013;54:1434-1441. (B) Uptake of the SV2A PET tracers [11C]UCB-J and [18F]SynVesT-1 in the brain of APP/PS1 and wild-type mice. The uptake of both tracers was lower in the hippocampus of APP/PS1 mice compared to wild-type controls.
One of the biggest advantages of small animal PET imaging is the longitudinal tracking of the pathogenesis and therapeutic effects of experimental drugs. This was demonstrated by the longitudinal PET imaging studies in AD animal models (Maeda J. et al., 2007; Deleye et al., 2017; Snellman et al., 2017). The challenges in imaging Aβ plaques in AD animal models are due to the different forms of plaques and disposition patterns in different animal models and at different ages of the same animals (Snellman et al., 2013). Other challenges are the quantification of the PET signals. For the quantitative analysis of human Aβ PET imaging data, the cerebellum was chosen as the reference region to generate distribution volume ratio (DVR) or standardized uptake value ratio (SUVR) because of the lack of specific binding of PIB in the human cerebellum (Lopresti et al., 2005; Price et al., 2005). However, there are emerging effective drugs targeting other pathological pathways and that do not alter Aβ plaque levels, e.g., Fyn inhibitor and mGluR5 silent allosteric modulator (SAM) (Kaufman et al., 2015; Haas et al., 2017). Thus, the objective assessment of their treatment effects needs different imaging biomarkers that are closely related to synaptic/functional recovery rather than Aβ plaque levels. The current consensus considers Aβ oligomers as the primary cause of the neurotoxicity derived from abnormal amyloidosis. Thus, the development of an imaging agent targeting Aβ oligomers is highly desirable, albeit challenging.
Large molecules such as antibodies have been developed for Aβ PET imaging. Sehlin et al. (2016) and Fang et al. (2019) engineered the 124I-labeled Aβ antibodies [124I]mAb158 and Di-scFv [124I]3D6-8D3, respectively, to detect the soluble Aβ in the tg-ArcSwe (AβPP E693G) and Swedish (AβPP KM670/671NL) mouse models with clearly visualized Aβ in the brain. The brain PET imaging shows a correlation between the PET signal and the levels of soluble Aβ aggregates. An increased SUVR of 2.2–3.5 in AD mice brains was reported compared to the wild-type brains. The evaluations of other amyloid imaging tracers in AD models have been summarized nicely in a recent review (Ni, 2021).
Targeting another hallmark of AD, tau neurofibrillary tangle is an extremely exciting area of PET tracer development. Tau is an axonally enriched microtubule-associated protein (MAP) that accumulates in the temporal and parietal neocortex in AD brains (Hung et al., 2016; DeTure and Dickson, 2019). Tau exists as six different isoforms, which contain either 3 or 4 microtubule-binding repeats (3R or 4R). The hyperphosphorylation and aggregation of tau with different repeats are involved in different neurodegenerative diseases, e.g., AD (3R/4R), Pick disease (3R), and progressive supranuclear palsy (4R). Postmortem histopathological studies demonstrated that NFTs are a better index of disease severity and progression than Aβ for patients with AD (Shoghi-Jadid et al., 2002). Tau pathology appears earlier than the Aβ plaque in human brains (Arnsten et al., 2021a). Tau-PET imaging allows the detection of tauopathy and highly predicts subsequent cognitive decline in both asymptomatic and symptomatic individuals (Leuzy et al., 2019; Wang and Edison, 2019; Beyer and Brendel, 2021).
[18F]FDDNP is the first PET tracer to visualize both amyloid plaques and tau tangles in living humans (Shin et al., 2011). Kuntner et al. (2009) compared 13–15-month-old age-matched wild-type litter mates with Tg2576 mice and found no difference in regional brain kinetics and DVR values. Later, the so-called first generation tau radioligand including [18F]THK523, the first tau selective tracer (Okamura et al., 2005), and other THK family tracers ([18F]THK5105, [18F]THK5117, [18F]THK5317, and [18F]THK5351) were developed and evaluated in human and AD mouse models. Fodero-Tavoletti et al. (2011) found higher retention of [18F]THK523 in the brains of rTg4510 mice compared with their wild-type littermates or 12-month-old APP/PS1 mice. Brendel et al. (2016) investigated [18F]THK5117 in Tau-P301S mice (PS19) and bigenic GSK-3β × Tau-P301L (biGT) mice and found increased SUVR in the brain stem of aged P301S mice and the entorhinal/amygdaloidal areas of biGT mice. In a separate study, the same group conducted a head-to-head comparison of [18F]T807 and [18F]THK5117 in Tau-P301S (P301S) mice (Brendel et al., 2018). Significantly elevated [18F]T807 than [18F]THK5117 uptake in the brainstem of P301S mice was evident at 6 months, and this increased further at 9 months. Thus, [18F]T807 appeared to be more sensitive than [18F]THK5117 to detect tau pathology in this model. Recently, [18F]THK5351 PET signal was found to correlate well with histological and biochemical tau changes, as well as motor, memory, and learning impairment, in P301S tau mice from 8 months over time (Moreno-Gonzalez et al., 2021).
Nevertheless, due to the off-target binding to monoamine oxidase-B (MAO-B), the THK family tracers are deemed to have limited utility in imaging tauopathies in AD (Ng et al., 2017; Murugan et al., 2019; Bao et al., 2021).
[11C]PBB3 is a pyrinated phenyl- and pyridinyl-butadienyl-benzothiazole and has been clinically used for in vivo detection of tauopathies in the human brain. [11C]PBB3 has been tested in the rTG4510 mouse (Ishikawa et al., 2018) and the PS19 transgenic mouse model (expressing 4R tau pathology) (Maruyama et al., 2013). Ni et al. (2018) compared [11C]PBB3 in PS19 and rTg4510 models and found increased binding in vivo in the neocortex and hippocampus of rTg4510 mice. In contrast, in vitro [11C]PBB3 binding was elevated in the brain stem but not in the hippocampus of PS19 mice. [18F]PM-PBB3, an 18F-labeled derivative of [11C]PBB3, has been demonstrated to detect significant tau deposits as measured by SUVR in the rTg4510 mice as early as 6 months of age (Weng et al., 2020). Recently, McMurray et al. reported the synthesis of [11C]LM229 based on the backbone of PBB3. [11C]LM229 showed high specificity for 4R tau aggregated in the brain sections of P301S tau mice and truncated human 151–351 3R (SHR24) and 4R (SHR72) tau aggregates in tau transgenic rat brain sections. Preliminary PET studies with [11C]LM229 in both WT and transgenic P310S tau mice confirmed BBB penetration by the radiotracer with maximum brain uptake (%ID/g max; WT = 1.56, P301S = 2.38) within the first minute, followed by washout during the 90-min scan (McMurray et al., 2021).
The most widely studied first-generation tau radioligand [18F]flortaucipir ([18F] T-807 and [18F]AV-1451) did not show any different retention in the cerebrum of the P301L tau transgenic mice compared to wild-type mice (Xia et al., 2013; Declercq et al., 2016), which was attributed to the use of transgenic mice expressing structurally different tau deposits in the animals than in humans (Duyckaerts et al., 2008).
Second-generation radiotracers with improved signal-to-noise ratio, less off-target, and lower non-specific binding are now available for tau imaging research. These tracers include [18F]PI2620, [18F]MK6240, [18F]GTP1, [18F]RO-948 (RO6958948), [18F]JNJ311 (JNJ64349311), and [18F]JNJ-067 (JNJ-64326067). Preliminary studies have been carried out in humans and healthy mice with promising results regarding the binding selectivity, affinity, and stability (Bao et al., 2021). So far, these tracers have not been tested in AD animal models.
Brain [18F]FDG PET primarily indicates synaptic activity. [18F]FDG uptake strongly correlates at autopsy with levels of the synaptic vesicle protein synaptophysin (Rocher et al., 2003). The degree and regional extent of hypometabolism measured by [18F]FDG-PET roughly correlate with the overall severity of cognitive impairment in AD. There is a close correlation between the regional accumulation of a tau-PET tracer ([18F]AV1451) and [18F]FDG hypometabolism (Rubinski et al., 2020). Along with amyloid imaging, [18F]FDG PET has been applied in multiple AD rodent models such as APPswe (Tg2576), 5 × FAD, APP/PS1, 3 × Tg, Tg4-42, TASTPM mice, and McGill-R-Thy1-APP rats (Waldron et al., 2015b; Bouter et al., 2018; Bouter and Bouter, 2019). Varying [18F]FDG PET results were found in Tg2576 mice. No differences in cerebral glucose metabolism were found in Tg2576 compared to WT mice in Kunter’s study (Kuntner et al., 2009), while Luo et al. (2012) found an increase in the FDG uptake in 7-month-old Tg2576, and Coleman et al. (2017) reported reduced FDG uptake in 18-month-old mice. Two separate [18F]FDG PET studies using 12-month-old APPPS1-21 mice reached the same conclusion that FDG uptake was reduced in the brain (Waldron et al., 2015a; Takkinen et al., 2017). Using APP/PS1 mice, both Poisnel et al. (2012) and Li et al. (2016) showed an age-dependent increase in glucose metabolism. In addition, PS2APP mice showed increased [18F]FDG uptake at 5 and 16 month (Brendel et al., 2016) and TASTPM mice were found to have decreased FDG uptake at 9 and 14 months of age (Waldron et al., 2015a,2017; Deleye et al., 2016). Contradictory results were reported in 5xFAD mice, with Rojas et al. (2013) reporting increased uptake of [18F]FDG in 11-month-old 5xFAD, and Macdonald et al. (2014) showing decreased uptake in 13-month-old mice. Sancheti et al. (2013) also reported decreased FDG uptake in 3xTg mice.
Clinical FDG PET imaging studies have shown promise in detecting early AD as neurodegeneration in certain brain regions (temporoparietal predominantly) is reflected by hypometabolism of FDG (Cohen and Klunk, 2014), and the hypometabolism pattern could serve as a predictive biomarker for conversion from MCI to AD dementia (Sala et al., 2020) earlier than MRI (Laforce<suffix>Jr.</suffix>, Soucy et al., 2018). However, there has been no suitable method to distinguish the FDG signal contributed by neuronal activity and immune cell activation, and thus the FDG PET signal could theoretically be influenced by two opposing forces, i.e., hypometabolism and neuroinflammation, at certain stages of AD pathogenesis and progression. With the recent development of PET imaging methods for synapse density (see section “PET Imaging of Synaptic Vesicle Glycoprotein 2A” for SV2A PET) and neuroinflammation (see section “PET Imaging of Neuroinflammation” for PET imaging of neuroinflammation), we are at a stage where we could potentially quantitatively attribute the FDG signals to synaptic and glial activities. This is of relevance in cases of MCI patients who show a positive correlation between Aβ PET and FDG PET.
Synaptic Vesicle Glycoprotein 2A is ubiquitously expressed in the neurons of the central nervous system and is widely used as one of the synaptic density biomarkers. Loss of synapses in the hippocampus and prefrontal cortex is implicated as an early pathological event in AD before the appearance of Aβ plaques and tau tangles and increasingly worsened during AD progression (Cai et al., 2019; Jackson et al., 2019).
[11C]Levetiracetam was first developed but was not pursued in further imaging study (Cai et al., 2014). Nevertheless, it encouraged the development of SV2A ligands with much higher affinities, including [11C]UCB-A (Estrada et al., 2016), [18F]UCB-H (Warnock et al., 2014; Bahri et al., 2017; Becker et al., 2017), and [11C]/[18F]UCB-J (Cai et al., 2019; Li et al., 2019b). Among these, [11C]UCB-J exhibited high brain uptake, fast and reversible tissue binding kinetics, and high specific binding signals in both non-human primates and humans (Finnema et al., 2016; Nabulsi et al., 2016). Most recently, [18F]SynVesT-1 (also known as [18F]SDM-8 (Li et al., 2019a) and [18F]MNI-1126 (Constantinescu et al., 2019) are developed and evaluated in non-human primates and humans (Li et al., 2021; Naganawa et al., 2021). Using APP/PS1 mice, Toyonaga et al. showed decreased [11C]UCB-J uptake as compared to the WT mice, and treatment with the tyrosine kinase Fyn inhibitor saracatinib reversed this effect (Toyonaga et al., 2019). Sadasivam et al. (2019, 2021) found a lower [18F]SynVesT-1 signal in the whole brain of APP/PS1 mice, compared with wild-type mice (Figure 2B). However, in a study using [11C]UCB-J in the tg-ArcSwe model and wild-type mice, a small but non-significant difference (∼5%) was found between the two groups, presumably due to large inter-animal variability (Xiong et al., 2021).
Microglia are macrophages in the brain that play an important role in neuroinflammation in AD. PET imaging of biomarkers of microglia provides insights into the time course of AD pathology. However, the diverse phenotypes of activated microglia and their different roles over the course of the AD trajectory make it challenging to develop radiotracers specific for neuroinflammation in AD.
The 18kDa translocator protein (TSPO) has been widely studied as a biomarker for microglial activation for over 20 years. Early radiotracers had disadvantages of low brain penetrability, low binding affinity for TSPO, the short half-life of the radioisotope, and sensitivity of binding affinity to gene polymorphisms (Zhou R. et al., 2021). [18F]DPA714 is one of the more recent radiotracers developed for TSPO. [18F]DPA714 was evaluated in APP/PS1 mice at different months to determine the role of microglia in the pathogenesis of AD neuroinflammation (Hu et al., 2020). Higher [18F]DPA714 uptake was noted in the cortex and hippocampus of 12–13 and 15–16-months-old but not younger AD mice compared with control mice. Another longitudinal PET study in APP23 mice used [18F]GE180 for TSPO imaging and [11C]PIB for assessing amyloid deposition in ex vivo autoradiography experiments (Lopez-Picon et al., 2018). The APP23 model was chosen because of high [11C]PIB binding in the brain of model mice compared with other AD models such as APP/PS1. AD mice were imaged with [18F]GE-180 at 17, 20, and 26 months of age. The binding of [18F]GE-180 plateaued in the frontal cortex and hippocampus regions in the early stage of AD, but amyloidosis increased throughout the later stages of AD (17–26 months of age). Thus, [18F]GE-180 appeared to be useful for tracking TSPO/neuroinflammation in early-stage AD but not for monitoring disease progression.
Compared with TSPO, colony-stimulating factor 1 receptor (CSF1R) expression in the brain is predominantly localized to microglia and low in other cell types. [11C]CPPC was developed from a potent CSF1R inhibitor with an IC50 of 0.8 nM (Horti et al., 2019) and evaluated in a mouse model of AD-related amyloidosis-overexpressing APP with Swedish and Indiana mutations (Melnikova et al., 2013). [11C]CPPC had about 30% higher uptake in the cortex of AD mice compared with control mice at 40 min post injection. Significantly higher uptake in the hippocampus and cerebellum was also observed in the AD mice. Additionally, increased expression of CSF1R after LPS treatment and about 50% specific binding of [11C]CPPC in LPS-treated mice were observed relative to sham controls. Autoradiography studies with [3H]CPPC demonstrated the lack of specificity of [3H]CPPC in brain tissues of LPS-treated Sprague-Dawley rats (Knight et al., 2021). In another study, the imaging performance of [11C]CPPC was compared with that of [11C]GW2580 in mouse models of acute and chronic neuroinflammation and a rhesus monkey (Zhou X. et al., 2021). In WT vs. APP-KI mice, [11C]GW2580 demonstrated higher sensitivity than [11C]CPPC, shown by a greater increase in [11C]GW2580 uptake in the neocortex, forebrain, and striatum of APP-KI mice compared with that in WT based on SUVR measurements at 60–90 min. Blocking studies in the monkey showed higher specificity for [11C]GW2580 over [11C]CPPC.
TREM2 is a relatively new biomarker for microglial activation. Bispecific antibody scaffolds that bind to transferrin to enter the brain and to TREM2 were chemically conjugated and radiolabeled with relatively longer-lived radioisotopes. One example is 124I-mAb1729-scFv8D3CL, which was evaluated in Arc-Swe transgenic AD mice (Meier et al., 2021). While areas under the curve (AUC) for 124I-mAb1729-scFv8D3CL at 24–72 h post injection were higher in caudate, cortex, thalamus, and hippocampus of AD mice compared with control mice, significant differences in SUVs were not observed for the individual imaging timepoints. However, ex vivo binding studies through autoradiography with the radiotracer showed significant differences between the animal models. The lack of a significant difference in vivo was then attributed to the increased blood residence time of 124I-mAb1729-scFv8D3CL. Thus, radiolabeling of smaller antibody fragments is desirable to address the slow pharmacokinetic issue.
Another new biomarker for AD is the purinergic P2X ligand-gated ion channel type 7 receptor (P2X7R), which is involved in triggering parts of the AD neurodegenerative processes. P2X7R activates microglia in acute AD models (Sanz et al., 2009) and increases the production of chemokines mediated by Aβ peptide in chronic AD models (Martin et al., 2019). [18F]JNJ-64413739 was evaluated in a rat model of acute neuroinflammation. The uptake of [18F]JNJ-64413739 was found to be elevated in the LPS-treated site of the rat brain compared with the contralateral hemisphere of the brain treated with PBS (Berdyyeva et al., 2019). Biomarkers for neuroinflammation, such as higher mRNA levels of P2X7R, TSPO, and Aif1, were associated with the LPS-treated site. It was acknowledged that LPS treatment as a model of neuroinflammation is considered extreme and that this novel tracer warrants evaluation in rodent models of AD and other neurodegenerative diseases. Other PET tracers for P2X7R and other biomarkers of neuroinflammation are reviewed by Zhou R. et al. (2021). So far, the development of neuroinflammation imaging agents has been focused on targeting microglial activation, largely ignoring the other glial cell types. It would be instrumental to be able to distinguish the protective microglial activation in early AD from the later destructive phenotype to guide the proper timing of anti-inflammatory treatments.
Glutamate is the major excitatory neurotransmitter in the brain and acts on the ionotropic glutamate receptors (iGluRs) and metabotropic glutamate receptors (mGluRs) to regulate synaptic plasticity. iGluRs comprise three subfamilies: α-amino-3- hydroxy-5-methyl-4-isoxasolepropionic acid (AMPA) receptors, kainate receptors, and NMDARs (Traynelis et al., 2010). mGluRs are a family of G-protein-coupled receptors with 8 subtypes, mGluR1-8. Both iGluRs and mGluRs are found to be involved in synaptic malfunctions in AD (Avila et al., 2017; Foster et al., 2017; Wang and Reddy, 2017; Liu et al., 2019; Srivastava et al., 2020).
Several imaging tracers for glutamate receptors have been developed. So far, only the mGluR5 radiotracer [18F]FPEB has been evaluated in 5 × FAD mice (Lee et al., 2019), APP/PS1 mice (Varlow et al., 2020), and Tg-ArcSwe mice (Fang et al., 2017), with conflicting results: compared to wild-type animals, uptake of [18F]FPEB was found to be lower in 5 × FAD mice, with no difference in Tg-ArcSwe mice, and increased in APP/PS1 mice. Shimojo et al. (2020) observed that radioactivity signals derived from the other mGluR5 tracer (E)-[11C]ABP688 were unaltered relative to controls at 2 months of age in rTg4510 mice but then gradually declined with aging in parallel with progressive brain atrophy.
The deficit in cholinergic neurotransmission is a prominent pathophysiological feature in AD. Dramatic loss of cholinergic neurons located in the basal forebrain increased levels of α7 nicotinic acetylcholine receptor (α7 nAChR) (Ikonomovic et al., 2009; Marutle et al., 2013) and decreased levels of M1 muscarinic acetylcholine receptor (M1 mAChR) (Yi et al., 2020) were found in the cortical regions of human AD brains (Ferreira-Vieira et al., 2016). The following PET imaging agents for cholinergic targets have been developed: 1) [11C]NS14492 (Ettrup et al., 2011), [11C](R)-MeQAA (Nishiyama et al., 2015), and [18F]ASEM (Gao et al., 2013) for α7 nAChR; 2) [11C](+)3-MPB (Yamamoto et al., 2011) and [18F]fluorobenzyl-dexetimide (Rowe et al., 2021) for mAChR; 3) [11C]LSN3172176 for M1 mAChR (Nabulsi et al., 2019); and 4) [11C]MK-6884 M4 mAChR (Tong et al., 2020). Uptake of the α7 nAChR tracer [11C](R)-MeQAA was found to be increased in aged monkeys (Nishiyama et al., 2015), and lower uptake of the other α7 nAChR tracer [18F]ASEM was seen in aged TgF334 rats compared with wild-type rats (Chaney et al., 2021). No difference was noted in the brain uptake of the acetylcholine esterase tracer [11C]MP4A between the APP23 and wild-type mice at 10–13 months of age (Heneka et al., 2006).
Altered expression of endogenous cannabinoid receptor 2 (CB2), histaminergic receptors, sigma receptors, adenosine receptors (A1A and A2A receptors), and enzymes (BACE1, caspase 3, aspartryl cathepsin, and TrkB/C), as well as abnormalities in dopamine and serotonin neurotransmission, have been noted in AD. These provide additional targets for PET radiotracer development for AD imaging. The CB2 radiotracers [11C]A-836339 have been tested in the LPS-induced neuroinflammation mouse model and the Appswe/PS1/dE9 mouse model (Horti et al., 2010), while [18F]JHU94620 has been tested in the LPS-induced neuroinflammation mouse model, which shows high-affinity binding to CB2R and sufficient selectivity over CB1R. A few tracers targeting caspase 3 and aspartryl capthepsin have been tested in AD model mice, with the majority of the tracers mainly tested in human subjects.
There has been great interest in imaging the compromised BBB in animal models of amyloidosis and patients with AD, as there is evidence of damaged BBB at the early stages of AD in patients and animal models. PET imaging of specific transporters and receptors expressed at the BBB was recently reviewed thoroughly by Ni98.
Alzheimer’s disease animal models have played essential roles in the development of PET radiotracers for imaging a diverse set of biological and pathological biomarkers in AD (Table 4). In turn, with well-validated PET tracers, PET imaging allows for longitudinal tracking of pathological phenotypes of AD in the same animals, boosting the statistical power in mechanistic studies of AD-related phenotypical and functional changes and facilitating the development of novel interventions through treatment effects monitoring. Currently, there is no perfect AD animal model that can fully recapitulate all features of human AD. However, with the rapid development in molecular biological technologies and our improved understanding of human AD etiology factors, we envision that the generation of more refined animal models with closer proximity to human AD pathogenesis will deepen our understanding of this devastating degenerative disease, further the development of biomarkers for preclinical diagnosis, and open new avenues for early and effective interventions.
Currently, PET radiotracers targeting Aβ plaque, tau pathology, synaptic density, and neuroinflammation have been tested on several major AD models for their relatively prominent and consistent pathological phenotypes. To retrieve the most approximate characteristics of the tracer in humans, the proper selection of AD models is key to the success of tracer development, as the neuropathological features vary based on different AD animal models. The selection of a proper AD model is also pivotal to the longitudinal and mechanistic studies of AD and anti-amyloid treatments (Manook et al., 2012; Snellman et al., 2013, 2017). One important point to bear in mind is that when choosing AD animals for PET imaging, correlation with behavioral measures, not just the AD pathologies, should be presented, and the time frame for imaging should also match those for the appearance of the biological phenotypes and related behavioral alterations.
The major advantage of using rat models of AD pathologies is their relatively large brain size, which reduces the partial volume effects in quantitative PET imaging analysis (Toyonaga et al., 2022). Rats are easier to handle than mice, less readily stressed by humans, and produce more robust behavioral testing results (Long and Holtzman, 2019). In addition, the APP/PS1 rats develop tau pathology in the brain, while the APP/PS1 mice with the same promoter lack tau pathology, indicating the APP/PS1 transgene in rats produces closer neuropathology to humans than in mice (Pini et al., 2016).
The translation and clinical Aβ, tau, and FDG PET imaging have transformed our understanding of AD (Scheltens et al., 2021), generated new insights (Aschenbrenner et al., 2018), and opened an avenue for the early detection of AD (Frisoni et al., 2017). With the development of new PET imaging tracers, we expect to gain a deeper understanding of AD at the systemic level and hopefully discover and validate new treatment targets beyond Aβ and tau.
BC, ZC, YH, and BM-N contributed to the conception and design of the review. BC wrote the first draft of the manuscript. BC, BM-N, and ZC wrote sections of the manuscript. ZC, BC, and YH revised the manuscript and approved the final version. BC, EB, TT, and JT prepared the figure and table. All authors contributed to manuscript revision, read, and approved the submitted version.
ZC was supported by grants from the National Institutes of Health (NIH) R01AG058773, R01AG069921, and the Archer Foundation.
The contents are solely the responsibility of the authors and do not necessarily represent the official view of the funding agencies.
The authors declare that the research was conducted in the absence of any commercial or financial relationships that could be construed as a potential conflict of interest.
All claims expressed in this article are solely those of the authors and do not necessarily represent those of their affiliated organizations, or those of the publisher, the editors and the reviewers. Any product that may be evaluated in this article, or claim that may be made by its manufacturer, is not guaranteed or endorsed by the publisher.
Abey, A., Davies, D., Goldsbury, C., Buckland, M., Valenzuela, M., and Duncan, T. (2021). Distribution of tau hyperphosphorylation in canine dementia resembles early Alzheimer’s disease and other tauopathies. Brain Pathol. 31, 144–162. doi: 10.1111/bpa.12893
Agca, C., Fritz, J. J., Walker, L. C., Levey, A. I., Chan, A. W., Lah, J. J., et al. (2008). Development of transgenic rats producing human beta-amyloid precursor protein as a model for Alzheimer’s disease: transgene and endogenous APP genes are regulated tissue-specifically. BMC Neurosci. 9:28. doi: 10.1186/1471-2202-9-28
Allen, B., Ingram, E., Takao, M., Smith, M. J., Jakes, R., Virdee, K., et al. (2002). Abundant tau filaments and nonapoptotic neurodegeneration in transgenic mice expressing human P301S tau protein. J. Neurosci. 22, 9340–9351. doi: 10.1523/JNEUROSCI.22-21-09340.2002
Alzghool, O. M., Rokka, J., López-Picón, F. R., Snellman, A., Helin, J. S., Okamura, N., et al. (2021). (S)-[18F]THK5117 brain uptake is associated with Aβ plaques and MAO-B enzyme in a mouse model of Alzheimer’s disease. Neuropharmacology 196:108676. doi: 10.1016/j.neuropharm.2021.108676
Alzheimer’s Association (2021). 2021 Alzheimer’s disease facts and figures. Alzheimers Dement. 17, 327–406. doi: 10.1002/alz.12328
Andorfer, C., Kress, Y., Espinoza, M., de Silva, R., Tucker, K. L., Barde, Y. A., et al. (2003). Hyperphosphorylation and aggregation of tau in mice expressing normal human tau isoforms. J. Neurochem. 86, 582–590. doi: 10.1046/j.1471-4159.2003.01879.x
Arnsten, A. F. T., Datta, D., Del Tredici, K., and Braak, H. (2021a). Hypothesis: tau pathology is an initiating factor in sporadic Alzheimer’s disease. Alzheimers Dement. 17, 115–124. doi: 10.1002/alz.12192
Arnsten, A. F. T., Datta, D., and Preuss, T. M. (2021b). Studies of aging nonhuman primates illuminate the etiology of early-stage Alzheimer’s-like neuropathology: an evolutionary perspective. Am. J. Primatol. 83:e23254. doi: 10.1002/ajp.23254
Aschenbrenner, A. J., Gordon, B. A., Benzinger, T. L. S., Morris, J. C., and Hassenstab, J. J. (2018). Influence of tau PET, amyloid PET, and hippocampal volume on cognition in Alzheimer disease. Neurology 91, e859–e866. doi: 10.1212/WNL.0000000000006075
Avila, J., Llorens-Martin, M., Pallas-Bazarra, N., Bolos, M., Perea, J. R., Rodriguez-Matellan, A., et al. (2017). Cognitive decline in neuronal aging and Alzheimer’s disease: role of NMDA receptors and associated proteins. Front. Neurosci. 11:626. doi: 10.3389/fnins.2017.00626
Bacskai, B. J., Hickey, G. A., Skoch, J., Kajdasz, S. T., Wang, Y., Huang, G. F., et al. (2003). Four-dimensional multiphoton imaging of brain entry, amyloid binding, and clearance of an amyloid-beta ligand in transgenic mice. Proc. Natl. Acad. Sci. U.S.A. 100, 12462–12467. doi: 10.1073/pnas.2034101100
Bahri, M. A., Plenevaux, A., Aerts, J., Bastin, C., Becker, G., Mercier, J., et al. (2017). Measuring brain synaptic vesicle protein 2A with positron emission tomography and [(18)F]UCB-H. Alzheimers Dement. 3, 481–486. doi: 10.1016/j.trci.2017.08.004
Baker, S., and Gotz, J. (2016). A local insult of okadaic acid in wild-type mice induces tau phosphorylation and protein aggregation in anatomically distinct brain regions. Acta Neuropathol. Commun. 4:32. doi: 10.1186/s40478-016-0300-0
Baker, S. L., Provost, K., Thomas, W., Whitman, A. J., Janabi, M., Schmidt, M. E., et al. (2021). Evaluation of [18F]-JNJ-64326067-AAA tau PET tracer in humans. J. Cereb. Blood Flow Metab. 41, 3302–3313. doi: 10.1177/0271678X211031035
Bao, W., Xie, F., Zuo, C., Guan, Y., and Huang, Y. H. P. E. T. (2021). Neuroimaging of Alzheimer’s disease: radiotracers and their utility in clinical research. Front. Aging Neurosci. 13:624330. doi: 10.3389/fnagi.2021.624330
Becker, G., Warnier, C., Serrano, M. E., Bahri, M. A., Mercier, J., Lemaire, C., et al. (2017). Pharmacokinetic characterization of [(18)F]UCB-H PET radiopharmaceutical in the rat brain. Mol. Pharm. 14, 2719–2725. doi: 10.1021/acs.molpharmaceut.7b00235
Beckman, D., Chakrabarty, P., Ott, S., Dao, A., Zhou, E., Janssen, W. G., et al. (2021). A novel tau-based rhesus monkey model of Alzheimer’s pathogenesis. Alzheimers Dement. 17, 933–945. doi: 10.1002/alz.12318
Benedikz, E., Kloskowska, E., and Winblad, B. (2009). The rat as an animal model of Alzheimer’s disease. J. Cell Mol. Med. 13, 1034–1042.
Berdyyeva, T., Xia, C., Taylor, N., He, Y., Chen, G., Huang, C., et al. (2019). PET imaging of the P2X7 ion channel with a novel tracer. Mol. Imaging Biol. 21, 871–878. doi: 10.1007/s11307-018-01313-2
Beyer, L., and Brendel, M. (2021). Imaging of tau pathology in neurodegenerative diseases: an update. Semin. Nucl. Med. 51, 253–263. doi: 10.1053/j.semnuclmed.2020.12.004
Biancalana, M., and Koide, S. (2010). Molecular mechanism of Thioflavin-T binding to amyloid fibrils. Biochim. Biophys. Acta 1804, 1405–1412. doi: 10.1016/j.bbapap.2010.04.001
Bolmont, T., Clavaguera, F., Meyer-Luehmann, M., Herzig, M. C., Radde, R., Staufenbiel, M., et al. (2007). Induction of tau pathology by intracerebral infusion of amyloid-beta -containing brain extract and by amyloid-beta deposition in APP x Tau transgenic mice. Am. J. Pathol. 171, 2012–2020. doi: 10.2353/ajpath.2007.070403
Bour, A., Grootendorst, J., Vogel, E., Kelche, C., Dodart, J. C., Bales, K., et al. (2008). Middle-aged human apoE4 targeted-replacement mice show retention deficits on a wide range of spatial memory tasks. Behav. Brain Res. 193, 174–182. doi: 10.1016/j.bbr.2008.05.008
Bouter, C., and Bouter, Y. (2019). (18)F-FDG-PET in mouse models of Alzheimer’s disease. Front. Med. 6:71. doi: 10.3389/fmed.2019.00071
Bouter, C., Henniges, P., Franke, T. N., Irwin, C., Sahlmann, C. O., Sichler, M. E., et al. (2018). (18)F-FDG-PET detects drastic changes in brain metabolism in the Tg4-42 model of Alzheimer’s disease. Front. Aging Neurosci. 10:425. doi: 10.3389/fnagi.2018.00425
Braak, H., Braak, E., and Strothjohann, M. (1994). Abnormally phosphorylated tau protein related to the formation of neurofibrillary tangles and neuropil threads in the cerebral cortex of sheep and goat. Neurosci. Lett. 171, 1–4. doi: 10.1016/0304-3940(94)90589-4
Brendel, M., Jaworska, A., Probst, F., Overhoff, F., Korzhova, V., Lindner, S., et al. (2016). Small-animal PET Imaging of tau pathology with 18F-THK5117 in 2 transgenic mouse models. J. Nucl. Med. 57, 792–798. doi: 10.2967/jnumed.115.163493
Brendel, M., Yousefi, B. H., Blume, T., Herz, M., Focke, C., Deussing, M., et al. (2018). Comparison of (18)F-T807 and (18)F-THK5117 PET in a mouse model of tau pathology. Front. Aging Neurosci. 10:174. doi: 10.3389/fnagi.2018.00174
Cai, H., Wang, Y., McCarthy, D., Wen, H., Borchelt, D. R., Price, D. L., et al. (2001). BACE1 is the major beta-secretase for generation of Abeta peptides by neurons. Nat. Neurosci. 4, 233–234. doi: 10.1038/85064
Cai, H. C., Mangner, T., Muzik, O., and Chugani, H. (2014). Radiosynthesis and preliminary evaluation of 11C-levetiracetam for PET imaging of SV2A expression. J. Nucl. Med. 55, 1152–1155. doi: 10.1021/ml500285t
Cai, Z., Li, S., Matuskey, D., Nabulsi, N., and Huang, Y. (2019). PET imaging of synaptic density: a new tool for investigation of neuropsychiatric diseases. Neurosci. Lett. 691, 44–50. doi: 10.1016/j.neulet.2018.07.038
Chambers, J. K., Tokuda, T., Uchida, K., Ishii, R., Tatebe, H., Takahashi, E., et al. (2015). The domestic cat as a natural animal model of Alzheimer’s disease. Acta Neuropathol. Commun. 3:78. doi: 10.1186/s40478-015-0258-3
Chaney, A. M., Lopez-Picon, F. R., Serriere, S., Wang, R., Bochicchio, D., Webb, S. D., et al. (2021). Prodromal neuroinflammatory, cholinergic and metabolite dysfunction detected by PET and MRS in the TgF344-AD transgenic rat model of AD: a collaborative multi-modal study. Theranostics 11, 6644–6667. doi: 10.7150/thno.56059
Charreau, B., Tesson, L., Soulillou, J. P., Pourcel, C., and Anegon, I. (1996). Transgenesis in rats: technical aspects and models. Transgen. Res. 5, 223–234. doi: 10.1007/BF01972876
Chiaravalloti, A., Castellano, A. E., Ricci, M., Barbagallo, G., Sannino, P., Ursini, F., et al. (2018). Coupled Imaging with [18F]FBB and [18F]FDG in AD subjects show a selective association between amyloid burden and cortical dysfunction in the brain. Mol. Imaging Biol. 20, 659–666. doi: 10.1007/s11307-018-1167-1
Chiotis, K., Stenkrona, P., Almkvist, O., Stepanov, V., Ferreira, D., Arakawa, R., et al. (2018). Dual tracer tau PET imaging reveals different molecular targets for 11C-THK5351 and 11C-PBB3 in the Alzheimer brain. Eur. J. Nucl. Med. Mol. Imaging 45, 1605–1617. doi: 10.1007/s00259-018-4012-5
Choi, D. Y., Lee, J. W., Lin, G., Lee, Y. K., Lee, Y. H., Choi, I. S., et al. (2012). Obovatol attenuates LPS-induced memory impairments in mice via inhibition of NF-kappaB signaling pathway. Neurochem. Int. 60, 68–77. doi: 10.1016/j.neuint.2011.11.005
Chotipanich, C., Nivorn, M., Kunawudhi, A., Promteangtrong, C., Boonkawin, N., and Jantarato, A. (2020). Evaluation of imaging windows for Tau PET imaging using 18F-PI2620 in cognitively normal individuals, mild cognitive impairment, and Alzheimer’s disease patients. Mol. Imaging 19:1536012120947582. doi: 10.1177/1536012120947582
Clavaguera, F., Bolmont, T., Crowther, R. A., Abramowski, D., Frank, S., Probst, A., et al. (2009). Transmission and spreading of tauopathy in transgenic mouse brain. Nat. Cell Biol. 11, 909–913. doi: 10.1038/ncb1901
Clavaguera, F., Hench, J., Lavenir, I., Schweighauser, G., Frank, S., Goedert, M., et al. (2014). Peripheral administration of tau aggregates triggers intracerebral tauopathy in transgenic mice. Acta Neuropathol. 127, 299–301. doi: 10.1007/s00401-013-1231-5
Cohen, A. D., and Klunk, W. E. (2014). Early detection of Alzheimer’s disease using PiB and FDG PET. Neurobiol. Dis. 72(Pt A), 117–122. doi: 10.1016/j.nbd.2014.05.001
Cohen, R. M., Rezai-Zadeh, K., Weitz, T. M., Rentsendorj, A., Gate, D., Spivak, I., et al. (2013). A transgenic Alzheimer rat with plaques, tau pathology, behavioral impairment, oligomeric aβ, and frank neuronal loss. J. Neurosci. 33, 6245–6256. doi: 10.1523/JNEUROSCI.3672-12.2013
Coleman, R. A., Liang, C., Patel, R., Ali, S., and Mukherjee, J. (2017). Brain and brown adipose tissue metabolism in transgenic Tg2576 mice models of Alzheimer disease assessed using (18)F-FDG PET imaging. Mol. Imaging 16:1536012117704557. doi: 10.1177/1536012117704557
Condello, C., Lemmin, T., Stohr, J., Nick, M., Wu, Y., Maxwell, A. M., et al. (2018). Structural heterogeneity and intersubject variability of Abeta in familial and sporadic Alzheimer’s disease. Proc. Natl. Acad. Sci. U.S.A. 115, E782–E791. doi: 10.1073/pnas.1714966115
Constantinescu, C. C., Tresse, C., Zheng, M., Gouasmat, A., Carroll, V. M., Mistico, L., et al. (2019). Development and in vivo preclinical imaging of fluorine-18-labeled synaptic vesicle protein 2A (SV2A) PET tracers. Mol. Imaging Biol. 21, 509–518. doi: 10.1007/s11307-018-1260-5
Das, B., and Yan, R. (2017). Role of BACE1 in Alzheimer’s synaptic function. Transl. Neurodegener. 6:23. doi: 10.1186/s40035-017-0093-5
Datta, D., Leslie, S. N., Wang, M., Morozov, Y. M., Yang, S., Mentone, S., et al. (2021). Age-related calcium dysregulation linked with tau pathology and impaired cognition in non-human primates. Alzheimers Dement. 17, 920–932. doi: 10.1002/alz.12325
Dawson, H. N., Ferreira, A., Eyster, M. V., Ghoshal, N., Binder, L. I, and Vitek, M. P. (2001). Inhibition of neuronal maturation in primary hippocampal neurons from tau deficient mice. J. Cell Sci. 114(Pt 6), 1179–1187. doi: 10.1242/jcs.114.6.1179
Declercq, L., Celen, S., Lecina, J., Ahamed, M., Tousseyn, T., Moechars, D., et al. (2016). Comparison of New Tau PET-Tracer Candidates With [18F]T808 and [18F]T807. Mol. Imaging 15:1536012115624920. doi: 10.1177/1536012115624920
Deleye, S., Waldron, A. M., Richardson, J. C., Schmidt, M., Langlois, X., Stroobants, S., et al. (2016). The effects of physiological and methodological determinants on 18F-FDG mouse brain imaging exemplified in a double transgenic alzheimer model. Mol. Imaging 15:1536012115624919. doi: 10.1177/1536012115624919
Deleye, S., Waldron, A. M., Verhaeghe, J., Bottelbergs, A., Wyffels, L., Van Broeck, B., et al. (2017). Evaluation of small-animal PET outcome measures to detect disease modification induced by BACE inhibition in a transgenic mouse model of Alzheimer disease. J. Nucl. Med. 58, 1977–1983. doi: 10.2967/jnumed.116.187625
DeTure, M. A., and Dickson, D. W. (2019). The neuropathological diagnosis of Alzheimer’s disease. Mol. Neurodegener. 14:32.
Duff, K., Knight, H., Refolo, L. M., Sanders, S., Yu, X., Picciano, M., et al. (2000). Characterization of pathology in transgenic mice over-expressing human genomic and cDNA tau transgenes. Neurobiol. Dis. 7, 87–98. doi: 10.1006/nbdi.1999.0279
Dumanis, S. B., DiBattista, A. M., Miessau, M., Moussa, C. E., and Rebeck, G. W. (2013). APOE genotype affects the pre-synaptic compartment of glutamatergic nerve terminals. J. Neurochem. 124, 4–14. doi: 10.1111/j.1471-4159.2012.07908.x
Duyckaerts, C., Potier, M. C., and Delatour, B. (2008). Alzheimer disease models and human neuropathology: similarities and differences. Acta Neuropathol. 115, 5–38. doi: 10.1007/s00401-007-0312-8
Echeverria, V., Ducatenzeiler, A., Alhonen, L., Janne, J., Grant, S. M., Wandosell, F., et al. (2004a). Rat transgenic models with a phenotype of intracellular Abeta accumulation in hippocampus and cortex. J. Alzheimers Dis. 6, 209–219. doi: 10.3233/jad-2004-6301
Echeverria, V., Ducatenzeiler, A., Dowd, E., Janne, J., Grant, S. M., Szyf, M., et al. (2004b). Altered mitogen-activated protein kinase signaling, tau hyperphosphorylation and mild spatial learning dysfunction in transgenic rats expressing the beta-amyloid peptide intracellularly in hippocampal and cortical neurons. Neuroscience 129, 583–592. doi: 10.1016/j.neuroscience.2004.07.036
Ellenbroek, B., and Youn, J. (2016). Rodent models in neuroscience research: is it a rat race? Dis. Model. Mech. 9, 1079–1087. doi: 10.1242/dmm.026120
Estrada, S., Lubberink, M., Thibblin, A., Sprycha, M., Buchanan, T., Mestdagh, N., et al. (2016). [(11)C]UCB-A, a novel PET tracer for synaptic vesicle protein 2A. Nucl. Med. Biol. 43, 325–332. doi: 10.1016/j.nucmedbio.2016.03.004
Ettrup, A., Mikkelsen, J. D., Lehel, S., Madsen, J., Nielsen, E. O., Palner, M., et al. (2011). 11C-NS14492 as a novel PET radioligand for imaging cerebral alpha7 nicotinic acetylcholine receptors: in vivo evaluation and drug occupancy measurements. J. Nucl. Med. 52, 1449–1456. doi: 10.2967/jnumed.111.088815
Fan, Z., Calsolaro, V., Atkinson, R. A., Femminella, G. D., Waldman, A., Buckley, C., et al. (2016). Flutriciclamide (18F-GE180) PET: first-in-human PET study of novel third-generation in vivo marker of human translocator protein. J. Nucl. Med. 57, 1753–1759. doi: 10.2967/jnumed.115.169078
Fang, X. T., Eriksson, J., Antoni, G., Yngve, U., Cato, L., Lannfelt, L., et al. (2017). Brain mGluR5 in mice with amyloid beta pathology studied with in vivo [(11)C]ABP688 PET imaging and ex vivo immunoblotting. Neuropharmacology 113(Pt A), 293–300. doi: 10.1016/j.neuropharm.2016.10.009
Fang, X. T., Hultqvist, G., Meier, S. R., Antoni, G., Sehlin, D., and Syvanen, S. (2019). High detection sensitivity with antibody-based PET radioligand for amyloid beta in brain. Neuroimage 184, 881–888. doi: 10.1016/j.neuroimage.2018.10.011
Ferreira-Vieira, T. H., Guimaraes, I. M., Silva, F. R., and Ribeiro, F. M. (2016). Alzheimer’s disease: targeting the cholinergic system. Curr. Neuropharmacol. 14, 101–115.
Filipcik, P., Zilka, N., Bugos, O., Kucerak, J., Koson, P., Novak, P., et al. (2012). First transgenic rat model developing progressive cortical neurofibrillary tangles. Neurobiol. Aging 33, 1448–1456. doi: 10.1016/j.neurobiolaging.2010.10.015
Finnema, S. J., Nabulsi, N. B., Eid, T., Detyniecki, K., Lin, S. F., Chen, M. K., et al. (2016). Imaging synaptic density in the living human brain. Sci. Transl. Med. 8:348ra96. doi: 10.1126/scitranslmed.aaf6667
Fiock, K. L., Smith, J. D., Crary, J. F., and Hefti, M. M. (2020). beta-amyloid and tau pathology in the aging feline brain. J. Comp. Neurol. 528, 108–113. doi: 10.1002/cne.24741
Flood, D. G., Lin, Y. G., Lang, D. M., Trusko, S. P., Hirsch, J. D., Savage, M. J., et al. (2009). A transgenic rat model of Alzheimer’s disease with extracellular Abeta deposition. Neurobiol. Aging 30, 1078–1090. doi: 10.1016/j.neurobiolaging.2007.10.006
Fodero-Tavoletti, M. T., Brockschnieder, D., Villemagne, V. L., Martin, L., Connor, A. R., Thiele, A., et al. (2012). In vitro characterization of [18F]-florbetaben, an Abeta imaging radiotracer. Nucl. Med. Biol. 39, 1042–1048. doi: 10.1016/j.nucmedbio.2012.03.001
Fodero-Tavoletti, M. T., Okamura, N., Furumoto, S., Mulligan, R. S., Connor, A. R., McLean, C. A., et al. (2011). 18F-THK523: a novel in vivo tau imaging ligand for Alzheimer’s disease. Brain 134(Pt 4), 1089–1100. doi: 10.1093/brain/awr038
Foster, T. C., Kyritsopoulos, C., and Kumar, A. (2017). Central role for NMDA receptors in redox mediated impairment of synaptic function during aging and Alzheimer’s disease. Behav. Brain Res. 322(Pt B), 223–232. doi: 10.1016/j.bbr.2016.05.012
Frisoni, G. B., Boccardi, M., Barkhof, F., Blennow, K., Cappa, S., Chiotis, K., et al. (2017). Strategic roadmap for an early diagnosis of Alzheimer’s disease based on biomarkers. Lancet Neurol. 16, 661–676. doi: 10.1016/S1474-4422(17)30159-X
Games, D., Adams, D., Alessandrini, R., Barbour, R., Berthelette, P., Blackwell, C., et al. (1995). Alzheimer-type neuropathology in transgenic mice overexpressing V717F beta-amyloid precursor protein. Nature 373, 523–527. doi: 10.1038/373523a0
Gao, Y., Kellar, K. J., Yasuda, R. P., Tran, T., Xiao, Y., Dannals, R. F., et al. (2013). Derivatives of dibenzothiophene for positron emission tomography imaging of alpha7-nicotinic acetylcholine receptors. J. Med. Chem. 56, 7574–7589. doi: 10.1021/jm401184f
Götz, J., Bodea, L. G., and Goedert, M. (2018). Rodent models for Alzheimer disease. Nat. Rev. Neurosci. 19, 583–598. doi: 10.1038/s41583-018-0054-8
Gotz, J., Chen, F., van Dorpe, J., and Nitsch, R. M. (2001). Formation of neurofibrillary tangles in P301l tau transgenic mice induced by Abeta 42 fibrils. Science 293, 1491–1495. doi: 10.1126/science.1062097
Gotz, J., Probst, A., Spillantini, M. G., Schafer, T., Jakes, R., Burki, K., et al. (1995). Somatodendritic localization and hyperphosphorylation of tau protein in transgenic mice expressing the longest human brain tau isoform. EMBO J. 14, 1304–1313. doi: 10.1002/j.1460-2075.1995.tb07116.x
Grueninger, F., Bohrmann, B., Czech, C., Ballard, T. M., Frey, J. R., Weidensteiner, C., et al. (2010). Phosphorylation of Tau at S422 is enhanced by Abeta in TauPS2APP triple transgenic mice. Neurobiol. Dis. 37, 294–306. doi: 10.1016/j.nbd.2009.09.004
Guehl, N. J., Wooten, D. W., Yokell, D. L., Moon, S. H., Dhaynaut, M., Katz, S., et al. (2019). Evaluation of pharmacokinetic modeling strategies for in-vivo quantification of tau with the radiotracer [18F]MK6240 in human subjects. Eur. J. Nucl. Med. Mol. Imaging 46, 2099–2111. doi: 10.1007/s00259-019-04419-z
Haas, L. T., Salazar, S. V., Smith, L. M., Zhao, H. R., Cox, T. O., Herber, C. S., et al. (2017). Silent allosteric modulation of mGluR5 maintains glutamate signaling while rescuing Alzheimer’s mouse phenotypes. Cell Rep. 20, 76–88. doi: 10.1016/j.celrep.2017.06.023
Halliday, G. (2017). Pathology and hippocampal atrophy in Alzheimer’s disease. Lancet Neurol. 16, 862–864. doi: 10.1016/S1474-4422(17)30343-5
Haque, A., Banik, N. L., and Ray, S. K. (2008). New insights into the roles of endolysosomal cathepsins in the pathogenesis of Alzheimer’s disease: cathepsin inhibitors as potential therapeutics. CNS Neurol. Disord. Drug Targets 7, 270–277. doi: 10.2174/187152708784936653
Haque, R. U., and Levey, A. I. (2019). Alzheimer’s disease: a clinical perspective and future nonhuman primate research opportunities. Proc. Natl. Acad. Sci. U.S.A. 116, 26224–26229. doi: 10.1073/pnas.1912954116
Harada, A., Oguchi, K., Okabe, S., Kuno, J., Terada, S., Ohshima, T., et al. (1994). Altered microtubule organization in small-calibre axons of mice lacking tau protein. Nature 369, 488–491. doi: 10.1038/369488a0
He, Z., Guo, J. L., McBride, J. D., Narasimhan, S., Kim, H., Changolkar, L., et al. (2018). Amyloid-beta plaques enhance Alzheimer’s brain tau-seeded pathologies by facilitating neuritic plaque tau aggregation. Nat. Med. 24, 29–38. doi: 10.1038/nm.4443
Heneka, M. T., Carson, M. J., El Khoury, J., Landreth, G. E., Brosseron, F., Feinstein, D. L., et al. (2015). Neuroinflammation in Alzheimer’s disease. Lancet Neurol. 14, 388–405.
Heneka, M. T., Ramanathan, M., Jacobs, A. H., Dumitrescu-Ozimek, L., Bilkei-Gorzo, A., Debeir, T., et al. (2006). Locus ceruleus degeneration promotes Alzheimer pathogenesis in amyloid precursor protein 23 transgenic mice. J. Neurosci. 26, 1343–1354. doi: 10.1523/JNEUROSCI.4236-05.2006
Herreman, A., Hartmann, D., Annaert, W., Saftig, P., Craessaerts, K., Serneels, L., et al. (1999). Presenilin 2 deficiency causes a mild pulmonary phenotype and no changes in amyloid precursor protein processing but enhances the embryonic lethal phenotype of presenilin 1 deficiency. Proc. Natl. Acad. Sci. U.S.A. 96, 11872–11877. doi: 10.1073/pnas.96.21.11872
Hoffe, B., and Holahan, M. R. (2019). The use of pigs as a translational model for studying neurodegenerative diseases. Front. Physiol. 10:838. doi: 10.3389/fphys.2019.00838
Horti, A. G., Gao, Y., Ravert, H. T., Finley, P., Valentine, H., Wong, D. F., et al. (2010). Synthesis and biodistribution of [11C]A-836339, a new potential radioligand for PET imaging of cannabinoid type 2 receptors (CB2). Bioorg. Med. Chem. 18, 5202–5207. doi: 10.1016/j.bmc.2010.05.058
Horti, A. G., Gao, Y., Kuwabara, H., Wang, Y., Abazyan, S., Yasuda, R. P., et al. (2014). 18F-ASEM, a radiolabeled antagonist for imaging the α7-nicotinic acetylcholine receptor with PET. J. Nucl. Med. 55, 672–677. doi: 10.2967/jnumed.113.132068
Horti, A. G., Naik, R., Foss, C. A., Minn, I., Misheneva, V., Du, Y., et al. (2019). PET imaging of microglia by targeting macrophage colony-stimulating factor 1 receptor (CSF1R). Proc. Natl. Acad. Sci. U.S.A. 116, 1686–1691. doi: 10.1073/pnas.1812155116
Hu, W., Pan, D., Wang, Y., Bao, W., Zuo, C., Guan, Y., et al. (2020). PET imaging for dynamically monitoring neuroinflammation in APP/PS1 mouse model using. Front. Neurosci. 14:810. doi: 10.3389/fnins.2020.00810
Hung, A. S., Liang, Y., Chow, T. C., Tang, H. C., Wu, S. L., Wai, M. S., et al. (2016). Mutated tau, amyloid and neuroinflammation in Alzheimer disease-A brief review. Prog. Histochem. Cytochem. 51, 1–8. doi: 10.1016/j.proghi.2016.01.001
Ikonomovic, M. D., Wecker, L., Abrahamson, E. E., Wuu, J., Counts, S. E., Ginsberg, S. D., et al. (2009). Cortical alpha7 nicotinic acetylcholine receptor and beta-amyloid levels in early Alzheimer disease. Arch. Neurol. 66, 646–651. doi: 10.1001/archneurol.2009.46
Ishikawa, A., Tokunaga, M., Maeda, J., Minamihisamatsu, T., Shimojo, M., Takuwa, H., et al. (2018). In vivo visualization of tau accumulation, microglial activation, and brain atrophy in a mouse model of tauopathy rTg4510. J. Alzheimers Dis. 61, 1037–1052. doi: 10.3233/JAD-170509
Jackson, J., Jambrina, E., Li, J., Marston, H., Menzies, F., Phillips, K., et al. (2019). Targeting the synapse in Alzheimer’s disease. Front. Neurosci. 13:735. doi: 10.3389/fnins.2019.00735
Jadhav, V. S., Lin, P. B. C., Pennington, T., Di Prisco, G. V., Jannu, A. J., Xu, G., et al. (2020). Trem2 Y38C mutation and loss of Trem2 impairs neuronal synapses in adult mice. Mol. Neurodegener. 15:62. doi: 10.1186/s13024-020-00409-0
Jakobsen, J. E., Johansen, M. G., Schmidt, M., Liu, Y., Li, R., Callesen, H., et al. (2016). Expression of the Alzheimer’s disease mutations AbetaPP695sw and PSEN1M146I in double-transgenic gottingen minipigs. J. Alzheimers Dis. 53, 1617–1630. doi: 10.3233/JAD-160408
Jankowsky, J. L., Fadale, D. J., Anderson, J., Xu, G. M., Gonzales, V., Jenkins, N. A., et al. (2004). Mutant presenilins specifically elevate the levels of the 42 residue beta-amyloid peptide in vivo: evidence for augmentation of a 42-specific gamma secretase. Hum. Mol. Genet. 13, 159–170. doi: 10.1093/hmg/ddh019
Jankowsky, J. L., Slunt, H. H., Ratovitski, T., Jenkins, N. A., Copeland, N. G., and Borchelt, D. R. (2001). Co-expression of multiple transgenes in mouse CNS: a comparison of strategies. Biomol. Eng. 17, 157–165. doi: 10.1016/s1389-0344(01)00067-3
Ji, Y., Gong, Y., Gan, W., Beach, T., Holtzman, D. M., and Wisniewski, T. (2003). Apolipoprotein E isoform-specific regulation of dendritic spine morphology in apolipoprotein E transgenic mice and Alzheimer’s disease patients. Neuroscience 122, 305–315. doi: 10.1016/j.neuroscience.2003.08.007
Kang, S. S., Kurti, A., Baker, K. E., Liu, C. C., Colonna, M., Ulrich, J. D., et al. (2018). Behavioral and transcriptomic analysis of Trem2-null mice: not all knockout mice are created equal. Hum. Mol. Genet. 27, 211–223. doi: 10.1093/hmg/ddx366
Kaufman, A. C., Salazar, S. V., Haas, L. T., Yang, J., Kostylev, M. A., Jeng, A. T., et al. (2015). Fyn inhibition rescues established memory and synapse loss in Alzheimer mice. Ann. Neurol. 77, 953–971. doi: 10.1002/ana.24394
Kim, J., Basak, J. M., and Holtzman, D. M. (2009). The role of apolipoprotein E in Alzheimer’s disease. Neuron 63, 287–303.
Kloskowska, E., Pham, T. M., Nilsson, T., Zhu, S., Oberg, J., Codita, A., et al. (2010). Cognitive impairment in the Tg6590 transgenic rat model of Alzheimer’s disease. J. Cell Mol. Med. 14, 1816–1823. doi: 10.1111/j.1582-4934.2009.00809.x
Klunk, W. E., Engler, H., Nordberg, A., Wang, Y., Blomqvist, G., Holt, D. P., et al. (2004). Imaging brain amyloid in Alzheimer’s disease with Pittsburgh Compound-B. Ann. Neurol. 55, 306–319. doi: 10.1002/ana.20009
Klunk, W. E., Lopresti, B. J., Ikonomovic, M. D., Lefterov, I. M., Koldamova, R. P., Abrahamson, E. E., et al. (2005). Binding of the positron emission tomography tracer Pittsburgh compound-B reflects the amount of amyloid-beta in Alzheimer’s disease brain but not in transgenic mouse brain. J. Neurosci. 25, 10598–10606. doi: 10.1523/JNEUROSCI.2990-05.2005
Knight, A. C., Varlow, C., Zi, T., Liang, S. H., Josephson, L., Schmidt, K., et al. (2021). In vitro evaluation of [3 H]CPPC as a tool radioligand for CSF-1R. ACS Chem. Neurosci. 12, 998–1006. doi: 10.1021/acschemneuro.0c00802
Kotredes, K. P., Oblak, A., Pandey, R. S., Lin, P. B., Garceau, D., Williams, H., et al. (2021). Uncovering disease mechanisms in a novel mouse model expressing humanized APOEepsilon4 and Trem2*R47H. Front. Aging Neurosci. 13:735524. doi: 10.3389/fnagi.2021.735524
Koutseff, A., Mittelhaeuser, C., Essabri, K., Auwerx, J., and Meziane, H. (2014). Impact of the apolipoprotein E polymorphism, age and sex on neurogenesis in mice: pathophysiological relevance for Alzheimer’s disease? Brain Res. 1542, 32–40. doi: 10.1016/j.brainres.2013.10.003
Kragh, P. M., Nielsen, A. L., Li, J., Du, Y., Lin, L., Schmidt, M., et al. (2009). Hemizygous minipigs produced by random gene insertion and handmade cloning express the Alzheimer’s disease-causing dominant mutation APPsw. Transgenic Res. 18, 545–558. doi: 10.1007/s11248-009-9245-4
Kuntner, C., Kesner, A. L., Bauer, M., Kremslehner, R., Wanek, T., Mandler, M., et al. (2009). Limitations of small animal PET imaging with [18F]FDDNP and FDG for quantitative studies in a transgenic mouse model of Alzheimer’s disease. Mol. Imaging Biol. 11, 236–240. doi: 10.1007/s11307-009-0198-z
Laforce, R. Jr., Soucy, J. P., Sellami, L., Dallaire-Théroux, C., Brunet, F., Bergeron, D., et al. (2018). Molecular imaging in dementia: past, present, and future. Alzheimers Dement. 14, 1522–1552. doi: 10.1016/j.jalz.2018.06.2855
Lee, M., Lee, H. J., Jeong, Y. J., Oh, S. J., Kang, K. J., Han, S. J., et al. (2019). Age dependency of mGluR5 availability in 5xFAD mice measured by PET. Neurobiol. Aging 84, 208–216. doi: 10.1016/j.neurobiolaging.2019.08.006
Leon, W. C., Canneva, F., Partridge, V., Allard, S., Ferretti, M. T., DeWilde, A., et al. (2010). A novel transgenic rat model with a full Alzheimer’s-like amyloid pathology displays pre-plaque intracellular amyloid-beta-associated cognitive impairment. J. Alzheimers Dis. 20, 113–126. doi: 10.3233/JAD-2010-1349
Leslie, S. N., Kanyo, J., Datta, D., Wilson, R. S., Zeiss, C., Duque, A., et al. (2021). Simple, single-shot phosphoproteomic analysis of heat-stable tau identifies age-related changes in pS235- and pS396-tau levels in non-human primates. Front. Aging Neurosci. 13:767322. doi: 10.3389/fnagi.2021.767322
Leuzy, A., Chiotis, K., Lemoine, L., Gillberg, P. G., Almkvist, O., Rodriguez-Vieitez, E., et al. (2019). Tau PET imaging in neurodegenerative tauopathies-still a challenge. Mol. Psychiatry 24, 1112–1134. doi: 10.1038/s41380-018-0342-8
Lewandowski, C. T., Weng, J. M., and LaDu, M. J. (2020). Alzheimer’s disease pathology in APOE transgenic mouse models: the who, what, when, where, why, and how. Neurobiol. Dis. 139:104811. doi: 10.1016/j.nbd.2020.104811
Lewis, J., McGowan, E., Rockwood, J., Melrose, H., Nacharaju, P., Van Slegtenhorst, M., et al. (2000). Neurofibrillary tangles, amyotrophy and progressive motor disturbance in mice expressing mutant (P301L) tau protein. Nat. Genet. 25, 402–405. doi: 10.1038/78078
Li, S., Naganawa, M., Pracitto, R., Najafzadeh, S., Holden, D., Henry, S., et al. (2021). Assessment of test-retest reproducibility of [(18)F]SynVesT-1, a novel radiotracer for PET imaging of synaptic vesicle glycoprotein 2A. Eur. J. Nucl. Med. Mol. Imaging 48, 1327–1338. doi: 10.1007/s00259-020-05149-3
Li, S. Y., Cai, Z. X., Wu, X. A., Holden, D., Pracitto, R., Kapinos, M., et al. (2019a). Synthesis and in vivo evaluation of a novel PET radiotracer for imaging of synaptic vesicle glycoprotein 2A (SV2A) in nonhuman primates. ACS Chem. Neurosci. 10, 1544–1554. doi: 10.1021/acschemneuro.8b00526
Li, S. Y., Cai, Z. X., Zhang, W. J., Holden, D., Lin, S. F., Finnema, S. J., et al. (2019b). Synthesis and in vivo evaluation of [F-18]UCB-J for PET imaging of synaptic vesicle glycoprotein 2A (SV2A). Eur. J. Nucl. Med. Mol. I 46, 1952–1965. doi: 10.1007/s00259-019-04357-w
Li, W., Wu, Y., Min, F., Li, Z., Huang, J., and Huang, R. (2010). A nonhuman primate model of Alzheimer’s disease generated by intracranial injection of amyloid-beta42 and thiorphan. Metab. Brain Dis. 25, 277–284. doi: 10.1007/s11011-010-9207-9
Li, X. Y., Men, W. W., Zhu, H., Lei, J. F., Zuo, F. X., Wang, Z. J., et al. (2016). Age- and brain region-specific changes of glucose metabolic disorder, learning, and memory dysfunction in early Alzheimer’s disease assessed in APP/PS1 transgenic mice using (18)F-FDG-PET. Int. J. Mol. Sci. 17:1707. doi: 10.3390/ijms17101707
Liu, J., Chang, L., Song, Y., Li, H., and Wu, Y. (2019). The role of NMDA receptors in Alzheimer’s disease. Front. Neurosci. 13:43. doi: 10.3389/fnins.2019.00043
Lo Sasso, G., Schlage, W. K., Boue, S., Veljkovic, E., Peitsch, M. C., and Hoeng, J. (2016). The Apoe(-/-) mouse model: a suitable model to study cardiovascular and respiratory diseases in the context of cigarette smoke exposure and harm reduction. J. Transl. Med. 14:146. doi: 10.1186/s12967-016-0901-1
Long, J. M., and Holtzman, D. M. (2019). Alzheimer disease: an update on pathobiology and treatment strategies. Cell 179, 312–339. doi: 10.1016/j.cell.2019.09.001
Lopez-Picon, F. R., Snellman, A., Eskola, O., Helin, S., Solin, O., Haaparanta-Solin, M., et al. (2018). Neuroinflammation appears early on PET imaging and then plateaus in a mouse model of alzheimer disease. J. Nucl. Med. 59, 509–515. doi: 10.2967/jnumed.117.197608
Lopresti, B. J., Klunk, W. E., Mathis, C. A., Hoge, J. A., Ziolko, S. K., Lu, X., et al. (2005). Simplified quantification of Pittsburgh Compound B amyloid imaging PET studies: a comparative analysis. J. Nucl. Med. 46, 1959–1972.
Luo, F., Rustay, N. R., Ebert, U., Hradil, V. P., Cole, T. B., Llano, D. A., et al. (2012). Characterization of 7- and 19-month-old Tg2576 mice using multimodal in vivo imaging: limitations as a translatable model of Alzheimer’s disease. Neurobiol. Aging 33, 933–944. doi: 10.1016/j.neurobiolaging.2010.08.005
Macdonald, I. R., DeBay, D. R., Reid, G. A., O’Leary, T. P., Jollymore, C. T., Mawko, G., et al. (2014). Early detection of cerebral glucose uptake changes in the 5XFAD mouse. Curr. Alzheimer Res. 11, 450–460. doi: 10.2174/1567205011666140505111354
Maeda, J., Ji, B., Irie, T., Tomiyama, T., Maruyama, M., Okauchi, T., et al. (2007). Longitudinal, quantitative assessment of amyloid, neuroinflammation, and anti-amyloid treatment in a living mouse model of Alzheimer’s disease enabled by positron emission tomography. J. Neurosci. 27, 10957–10968. doi: 10.1523/JNEUROSCI.0673-07.2007
Maeda, S., Sahara, N., Saito, Y., Murayama, M., Yoshiike, Y., Kim, H., et al. (2007). Granular tau oligomers as intermediates of tau filaments. Biochemistry 46, 3856–3861. doi: 10.1021/bi061359o
Manook, A., Yousefi, B. H., Willuweit, A., Platzer, S., Reder, S., Voss, A., et al. (2012). Small-animal PET imaging of amyloid-beta plaques with [11C]PiB and its multi-modal validation in an APP/PS1 mouse model of Alzheimer’s disease. PLoS One 7:e31310. doi: 10.1371/journal.pone.0031310
Martin, E., Amar, M., Dalle, C., Youssef, I., Boucher, C., Le Duigou, C., et al. (2019). New role of P2X7 receptor in an Alzheimer’s disease mouse model. Mol. Psychiatry 24, 108–125. doi: 10.1038/s41380-018-0108-3
Marutle, A., Gillberg, P. G., Bergfors, A., Yu, W., Ni, R., Nennesmo, I., et al. (2013). (3)H-deprenyl and (3)H-PIB autoradiography show different laminar distributions of astroglia and fibrillar beta-amyloid in Alzheimer brain. J. Neuroinflamm. 10:90. doi: 10.1186/1742-2094-10-90
Maruyama, M., Shimada, H., Suhara, T., Shinotoh, H., Ji, B., Maeda, J., et al. (2013). Imaging of tau pathology in a tauopathy mouse model and in Alzheimer patients compared to normal controls. Neuron 79, 1094–1108. doi: 10.1016/j.neuron.2013.07.037
McMurray, L., Macdonald, J. A., Ramakrishnan, N. K., Zhao, Y., Williamson, D. W., Tietz, O., et al. (2021). Synthesis and assessment of novel probes for imaging tau pathology in transgenic mouse and rat models. ACS Chem. Neurosci. 12, 1885–1893. doi: 10.1021/acschemneuro.0c00790
Meier, S. R., Sehlin, D., Hultqvist, G., and Syvänen, S. (2021). Pinpointing brain TREM2 levels in two mouse models of Alzheimer’s disease. Mol. Imaging Biol. 23, 665–675. doi: 10.1007/s11307-021-01591-3
Melnikova, T., Fromholt, S., Kim, H., Lee, D., Xu, G., Price, A., et al. (2013). Reversible pathologic and cognitive phenotypes in an inducible model of Alzheimer-amyloidosis. J. Neurosci. 33, 3765–3779. doi: 10.1523/JNEUROSCI.4251-12.2013
More, S. V., Kumar, H., Cho, D. Y., Yun, Y. S., and Choi, D. K. (2016). Toxin-induced experimental models of learning and memory impairment. Int. J. Mol. Sci. 17:1447. doi: 10.3390/ijms17091447
Moreno-Gonzalez, I., Edwards, G. A. III, Hasan, O., Gamez, N., Schulz, J. E., Fernandez-Valenzuela, J. J., et al. (2021). Longitudinal assessment of tau-associated pathology by (18)F-THK5351 PET imaging: a histological, biochemical, and behavioral Study. Diagnostics 11:1874. doi: 10.3390/diagnostics11101874
Morris, M., Maeda, S., Vossel, K., and Mucke, L. (2011). The many faces of tau. Neuron 70, 410–426. doi: 10.1016/j.neuron.2011.04.009
Murugan, N. A., Chiotis, K., Rodriguez-Vieitez, E., Lemoine, L., Agren, H., and Nordberg, A. (2019). Cross-interaction of tau PET tracers with monoamine oxidase B: evidence from in silico modelling and in vivo imaging. Eur. J. Nucl. Med. Mol. Imaging 46, 1369–1382. doi: 10.1007/s00259-019-04305-8
Nabulsi, N. B., Holden, D., Zheng, M. Q., Bois, F., Lin, S. F., Najafzadeh, S., et al. (2019). Evaluation of (11)C-LSN3172176 as a novel PET tracer for imaging M1 muscarinic acetylcholine receptors in nonhuman primates. J. Nucl. Med. 60, 1147–1153. doi: 10.2967/jnumed.118.222034
Nabulsi, N. B., Mercier, J., Holden, D., Carre, S., Najafzadeh, S., Vandergeten, M. C., et al. (2016). Synthesis and preclinical evaluation of 11C-UCB-J as a PET tracer for imaging the synaptic vesicle glycoprotein 2A in the brain. J. Nucl. Med. 57, 777–784. doi: 10.2967/jnumed.115.168179
Naganawa, M., Li, S., Nabulsi, N., Henry, S., Zheng, M. Q., Pracitto, R., et al. (2021). First-in-human evaluation of (18)F-SynVesT-1, a radioligand for PET imaging of synaptic vesicle glycoprotein 2A. J. Nucl. Med. 62, 561–567. doi: 10.2967/jnumed.120.249144
Naj, A. C., and Schellenberg, G. D. (2017). Alzheimer’s disease genetics, C. genomic variants, genes, and pathways of Alzheimer’s disease: an overview. Am. J. Med. Genet. B Neuropsychiatr. Genet. 174, 5–26. doi: 10.1002/ajmg.b.32499
Nakaizumi, K., Ouchi, Y., Terada, T., Yoshikawa, E., Kakimoto, A., Isobe, T., et al. (2018). In vivo depiction of α7 nicotinic receptor loss for cognitive decline in Alzheimer’s disease. J. Alzheimers Dis. 61, 1355–1365. doi: 10.3233/JAD-170591
Neustadtl, A. L., Winston, C. N., Parsadanian, M., Main, B. S., Villapol, S., and Burns, M. P. (2017). Reduced cortical excitatory synapse number in APOE4 mice is associated with increased calcineurin activity. Neuroreport 28, 618–624. doi: 10.1097/WNR.0000000000000811
Ng, K. P., Pascoal, T. A., Mathotaarachchi, S., Therriault, J., Kang, M. S., Shin, M., et al. (2017). Monoamine oxidase B inhibitor, selegiline, reduces (18)F-THK5351 uptake in the human brain. Alzheimers Res. Ther. 9:25. doi: 10.1186/s13195-017-0253-y
Ni, R. (2021). Positron emission tomography in animal models of Alzheimer’s disease amyloidosis: translational implications. Pharmaceuticals 14:1179. doi: 10.3390/ph14111179
Ni, R., Ji, B., Ono, M., Sahara, N., Zhang, M. R., Aoki, I., et al. (2018). Comparative in vitro and in vivo quantifications of pathologic tau deposits and their association with neurodegeneration in tauopathy mouse models. J. Nucl. Med. 59, 960–966. doi: 10.2967/jnumed.117.201632
Nishiyama, S., Ohba, H., Kanazawa, M., Kakiuchi, T., and Tsukada, H. (2015). Comparing alpha7 nicotinic acetylcholine receptor binding, amyloid-beta deposition, and mitochondria complex-I function in living brain: a PET study in aged monkeys. Synapse 69, 475–483. doi: 10.1002/syn.21842
Oakley, H., Cole, S. L., Logan, S., Maus, E., Shao, P., Craft, J., et al. (2006). Intraneuronal beta-amyloid aggregates, neurodegeneration, and neuron loss in transgenic mice with five familial Alzheimer’s disease mutations: potential factors in amyloid plaque formation. J. Neurosci. 26, 10129–10140. doi: 10.1523/JNEUROSCI.1202-06.2006
Ojo, J. O., Mouzon, B., Greenberg, M. B., Bachmeier, C., Mullan, M., and Crawford, F. (2013). Repetitive mild traumatic brain injury augments tau pathology and glial activation in aged hTau mice. J. Neuropathol. Exp. Neurol. 72, 137–151. doi: 10.1097/NEN.0b013e3182814cdf
Okamura, N., Furumoto, S., Fodero-Tavoletti, M. T., Mulligan, R. S., Harada, R., Yates, P., et al. (2014). Non-invasive assessment of Alzheimer’s disease neurofibrillary pathology using 18F-THK5105 PET. QARain 137, 1762–1771. doi: 10.1093/brain/awu064
Okamura, N., Suemoto, T., Furumoto, S., Suzuki, M., Shimadzu, H., Akatsu, H., et al. (2005). Quinoline and benzimidazole derivatives: candidate probes for in vivo imaging of tau pathology in Alzheimer’s disease. J. Neurosci. 25, 10857–10862. doi: 10.1523/JNEUROSCI.1738-05.2005
Ordonez-Gutierrez, L., Fernandez-Perez, I., Herrera, J. L., Anton, M., Benito-Cuesta, I., and Wandosell, F. (2016). AbetaPP/PS1 transgenic mice show sex differences in the cerebellum associated with aging. J. Alzheimers Dis. 54, 645–656. doi: 10.3233/JAD-160572
Paspalas, C. D., Carlyle, B. C., Leslie, S., Preuss, T. M., Crimins, J. L., Huttner, A. J., et al. (2018). The aged rhesus macaque manifests Braak stage III/IV Alzheimer’s-like pathology. Alzheimers Dement. 14, 680–691. doi: 10.1016/j.jalz.2017.11.005
Peeraer, E., Bottelbergs, A., Van Kolen, K., Stancu, I. C., Vasconcelos, B., Mahieu, M., et al. (2015). Intracerebral injection of preformed synthetic tau fibrils initiates widespread tauopathy and neuronal loss in the brains of tau transgenic mice. Neurobiol. Dis. 73, 83–95. doi: 10.1016/j.nbd.2014.08.032
Piedrahita, J. A., Zhang, S. H., Hagaman, J. R., Oliver, P. M., and Maeda, N. (1992). Generation of mice carrying a mutant apolipoprotein E gene inactivated by gene targeting in embryonic stem cells. Proc. Natl. Acad. Sci. U.S.A. 89, 4471–4475. doi: 10.1073/pnas.89.10.4471
Pini, L., Pievani, M., Bocchetta, M., Altomare, D., Bosco, P., Cavedo, E., et al. (2016). Brain atrophy in Alzheimer’s disease and aging. Ageing Res. Rev. 30, 25–48. doi: 10.1016/j.arr.2016.01.002
Poisnel, G., Herard, A. S., El Tannir El Tayara, N., Bourrin, E., Volk, A., Kober, F., et al. (2012). Increased regional cerebral glucose uptake in an APP/PS1 model of Alzheimer’s disease. Neurobiol. Aging 33, 1995–2005. doi: 10.1016/j.neurobiolaging.2011.09.026
Price, J. C., Klunk, W. E., Lopresti, B. J., Lu, X., Hoge, J. A., Ziolko, S. K., et al. (2005). Kinetic modeling of amyloid binding in humans using PET imaging and Pittsburgh compound-B. J. Cereb. Blood Flow Metab. 25, 1528–1547. doi: 10.1038/sj.jcbfm.9600146
Prpar Mihevc, S., and Majdic, G. (2019). Canine cognitive dysfunction and Alzheimer’s disease - two facets of the same disease? Front. Neurosci. 13:604. doi: 10.3389/fnins.2019.00604
Querol-Vilaseca, M., Colom-Cadena, M., Pegueroles, J., Nuñez-Llaves, R., Luque-Cabecerans, J., Muñoz-Llahuna, L., et al. (2019). Nanoscale structure of amyloid-β plaques in Alzheimer’s disease. Sci. Rep. 9:5181.
Rabinovici, G. D., Furst, A. J., O’Neil, J. P., Racine, C. A., Mormino, E. C., Baker, S. L., et al. (2007). 11C-PIB PET imaging in Alzheimer disease and frontotemporal lobar degeneration. Neurology 68, 1205–1212. doi: 10.1212/01.wnl.0000259035.98480.ed
Rocher, A. B., Chapon, F., Blaizot, X., Baron, J. C., and Chavoix, C. (2003). Resting-state brain glucose utilization as measured by PET is directly related to regional synaptophysin levels: a study in baboons. Neuroimage 20, 1894–1898. doi: 10.1016/j.neuroimage.2003.07.002
Rodriguez-Vieitez, E., Leuzy, A., Chiotis, K., Saint-Aubert, L., Wall, A., and Nordberg, A. (2017). Comparability of [18F]THK5317 and [11C]PIB blood flow proxy images with [18F]FDG positron emission tomography in Alzheimer’s disease. J. Cereb. Blood Flow Metab. 37, 740–749. doi: 10.1177/0271678X16645593
Rohn, T. T. (2010). The role of caspases in Alzheimer’s disease; potential novel therapeutic opportunities. Apoptosis 15, 1403–1409. doi: 10.1007/s10495-010-0463-2
Rojas, S., Herance, J. R., Gispert, J. D., Abad, S., Torrent, E., Jimenez, X., et al. (2013). In vivo evaluation of amyloid deposition and brain glucose metabolism of 5XFAD mice using positron emission tomography. Neurobiol. Aging 34, 1790–1798. doi: 10.1016/j.neurobiolaging.2012.12.027
Rowe, C. C., Krishnadas, N., Ackermann, U., Dore, V., Goh, R. Y. W., Guzman, R., et al. (2021). Imaging of brain muscarinic receptors with (18)F-Fluorobenzyl-Dexetimide: a first in human study. Psychiatry Res. Neuroimaging 316, 111354. doi: 10.1016/j.pscychresns.2021.111354
Rubinski, A., Franzmeier, N., Neitzel, J., and Ewers, M. (2020). Alzheimer’s Disease Neuroimaging, I. FDG-PET hypermetabolism is associated with higher tau-PET in mild cognitive impairment at low amyloid-PET levels. Alzheimers Res. Ther. 12:133. doi: 10.1186/s13195-020-00702-6
Ruiz-Opazo, N., Kosik, K. S., Lopez, L. V., Bagamasbad, P., Ponce, L. R., and Herrera, V. L. (2004). Attenuated hippocampus-dependent learning and memory decline in transgenic TgAPPswe Fischer-344 rats. Mol. Med. 10, 36–44. doi: 10.2119/2003-00044.herrera
Ryan, N. S., and Rossor, M. N. (2010). Correlating familial Alzheimer’s disease gene mutations with clinical phenotype. Biomark Med. 4, 99–112. doi: 10.2217/bmm.09.92
Sadasivam, P., Fang, X. T., Toyonaga, T., Lee, S., Xu, Y., Zheng, M. Q., et al. (2021). Quantification of SV2A Binding in Rodent Brain Using [(18)F]SynVesT-1 and PET Imaging. Mol. Imaging Biol. 23, 372–381. doi: 10.1007/s11307-020-01567-9
Sadasivam, P., Smith, L., Spurrier, J., Lee, S., Lindemann, M., Toyonaga, T., et al. (2019). The new PET tracer F-18-SDM-8 effectively detects reduced SV2a binding in a rodent model of Alzheimer’s disease. J. Cerebr. Blood F Met. 39, 97–97.
Saito, T., Mihira, N., Matsuba, Y., Sasaguri, H., Hashimoto, S., Narasimhan, S., et al. (2019). Humanization of the entire murine Mapt gene provides a murine model of pathological human tau propagation. J. Biol. Chem. 294, 12754–12765. doi: 10.1074/jbc.RA119.009487
Sala, A., Caprioglio, C., Santangelo, R., Vanoli, E. G., Iannaccone, S., Magnani, G., et al. (2020). Brain metabolic signatures across the Alzheimer’s disease spectrum. Eur. J. Nucl. Med. Mol. Imaging 47, 256–269. doi: 10.1007/s00259-019-04559-2
Sanabria Bohórquez, S. S., Maøıìk, J., Ogasawara, A., Tinianow, J., Gill, H. S., Barret, O., et al. (2019). [18F]GTP1 (Genentech Tau Probe 1), a radioligand for detecting neurofibrillary tangle tau pathology in Alzheimer’s disease. Eur. J. Nucl. Med. Mol. Imaging 46, 2077–2089. doi: 10.1007/s00259-019-04399-0
Sancheti, H., Akopian, G., Yin, F., Brinton, R. D., Walsh, J. P., and Cadenas, E. (2013). Age-dependent modulation of synaptic plasticity and insulin mimetic effect of lipoic acid on a mouse model of Alzheimer’s disease. PLoS One 8:e69830. doi: 10.1371/journal.pone.0069830
Santacruz, K., Lewis, J., Spires, T., Paulson, J., Kotilinek, L., Ingelsson, M., et al. (2005). Tau suppression in a neurodegenerative mouse model improves memory function. Science 309, 476–481. doi: 10.1126/science.1113694
Sanz, J. M., Chiozzi, P., Ferrari, D., Colaianna, M., Idzko, M., Falzoni, S., et al. (2009). Activation of microglia by amyloid {beta} requires P2X7 receptor expression. J. Immunol. 182, 4378–4385. doi: 10.4049/jimmunol.0803612
Scheltens, P., De Strooper, B., Kivipelto, M., Holstege, H., Chételat, G., Teunissen, C. E., et al. (2021). Alzheimer’s disease. Lancet 397, 1577–1590.
Schmidt, F., Boltze, J., Jager, C., Hofmann, S., Willems, N., Seeger, J., et al. (2015). Detection and quantification of beta-amyloid, pyroglutamyl abeta, and tau in aged canines. J. Neuropathol. Exp. Neurol. 74, 912–923. doi: 10.1097/NEN.0000000000000230
Sehlin, D., Fang, X. T., Cato, L., Antoni, G., Lannfelt, L., and Syvanen, S. (2016). Antibody-based PET imaging of amyloid beta in mouse models of Alzheimer’s disease. Nat. Commun. 7:10759. doi: 10.1038/ncomms10759
Senechal, Y., Kelly, P. H., and Dev, K. K. (2008). Amyloid precursor protein knockout mice show age-dependent deficits in passive avoidance learning. Behav. Brain Res. 186, 126–132. doi: 10.1016/j.bbr.2007.08.003
Shaw, K. N., Commins, S., and O’Mara, S. M. (2001). Lipopolysaccharide causes deficits in spatial learning in the watermaze but not in BDNF expression in the rat dentate gyrus. Behav. Brain Res. 124, 47–54. doi: 10.1016/s0166-4328(01)00232-7
Shen, J., Bronson, R. T., Chen, D. F., Xia, W., Selkoe, D. J., and Tonegawa, S. (1997). Skeletal and CNS defects in Presenilin-1-deficient mice. Cell 89, 629–639. doi: 10.1016/s0092-8674(00)80244-5
Shimojo, M., Takuwa, H., Takado, Y., Tokunaga, M., Tsukamoto, S., Minatohara, K., et al. (2020). Selective disruption of inhibitory synapses leading to neuronal hyperexcitability at an early stage of tau pathogenesis in a mouse model. J. Neurosci. 40, 3491–3501. doi: 10.1523/JNEUROSCI.2880-19.2020
Shin, J., Kepe, V., Barrio, J. R., and Small, G. W. (2011). The merits of FDDNP-PET imaging in Alzheimer’s disease. J. Alzheimers Dis. 26, (Suppl. 3), 135–145. doi: 10.3233/JAD-2011-0008
Shoghi-Jadid, K., Small, G. W., Agdeppa, E. D., Kepe, V., Ercoli, L. M., Siddarth, P., et al. (2002). Localization of neurofibrillary tangles and beta-amyloid plaques in the brains of living patients with Alzheimer disease. Am. J. Geriatr. Psychiatry 10, 24–35. doi: 10.1097/00019442-200201000-00004
Smolek, T., Madari, A., Farbakova, J., Kandrac, O., Jadhav, S., Cente, M., et al. (2016). Tau hyperphosphorylation in synaptosomes and neuroinflammation are associated with canine cognitive impairment. J. Comp. Neurol. 524, 874–895. doi: 10.1002/cne.23877
Snellman, A., Lopez-Picon, F. R., Rokka, J., Salmona, M., Forloni, G., Scheinin, M., et al. (2013). Longitudinal amyloid imaging in mouse brain with 11C-PIB: comparison of APP23, Tg2576, and APPswe-PS1dE9 mouse models of Alzheimer disease. J. Nucl. Med. 54, 1434–1441. doi: 10.2967/jnumed.112.110163
Snellman, A., Rokka, J., Lopez-Picon, F. R., Helin, S., Re, F., Loyttyniemi, E., et al. (2017). Applicability of [(11)C]PIB micro-PET imaging for in vivo follow-up of anti-amyloid treatment effects in APP23 mouse model. Neurobiol. Aging 57, 84–94. doi: 10.1016/j.neurobiolaging.2017.05.008
Srivastava, A., Das, B., Yao, A. N. Y., and Yan, R. Q. (2020). Metabotropic glutamate receptors in alzheimer’s disease synaptic dysfunction: therapeutic opportunities and hope for the future. J. Alzheimers Dis. 78, 1345–1361. doi: 10.3233/JAD-201146
Sturchler-Pierrat, C., Abramowski, D., Duke, M., Wiederhold, K. H., Mistl, C., Rothacher, S., et al. (1997). Two amyloid precursor protein transgenic mouse models with Alzheimer disease-like pathology. Proc. Natl. Acad. Sci. U.S.A. 94, 13287–13292. doi: 10.1073/pnas.94.24.13287
Su, Y., Fu, J., Yu, J., Zhao, Q., Guan, Y., Zuo, C., et al. (2020). Tau PET imaging with [18F]PM-PBB3 in frontotemporal dementia with MAPT mutation. J. Alzheimers. Dis. 76, 149–157. doi: 10.3233/JAD-200287
Sun, G. Z., He, Y. C., Ma, X. K., Li, S. T., Chen, D. J., Gao, M., et al. (2017). Hippocampal synaptic and neural network deficits in young mice carrying the human APOE4 gene. CNS Neurosci. Ther. 23, 748–758. doi: 10.1111/cns.12720
Tai, L. M., Youmans, K. L., Jungbauer, L., Yu, C., and Ladu, M. J. (2011). Introducing human APOE into abeta transgenic mouse models. Int. J. Alzheimers Dis. 2011:810981. doi: 10.4061/2011/810981
Takkinen, J. S., Lopez-Picon, F. R., Al Majidi, R., Eskola, O., Krzyczmonik, A., Keller, T., et al. (2017). Brain energy metabolism and neuroinflammation in ageing APP/PS1-21 mice using longitudinal (18)F-FDG and (18)F-DPA-714 PET imaging. J. Cereb. Blood Flow Metab. 37, 2870–2882. doi: 10.1177/0271678X16677990
Tauber, C., Beaufils, É, Hommet, C., Ribeiro, M., Vercouillie, J., Vierron, E., et al. (2013). QARain [18F]FDDNP binding and glucose metabolism in advanced elderly healthy subjects and Alzheimer’s disease patients. J. Alzheimers Dis. 36, 311–320. doi: 10.3233/JAD-122068
Tong, L., Li, W., Lo, M. M., Gao, X., Wai, J. M., Rudd, M., et al. (2020). Discovery of [(11)C]MK-6884: a positron emission tomography (PET) imaging agent for the study of M4Muscarinic receptor positive allosteric modulators (PAMs) in neurodegenerative diseases. J. Med. Chem. 63, 2411–2425. doi: 10.1021/acs.jmedchem.9b01406
Toyama, H., Ye, D., Ichise, M., Liow, J. S., Cai, L., Jacobowitz, D., et al. (2005). PET imaging of brain with the beta-amyloid probe, [11C]6-OH-BTA-1, in a transgenic mouse model of Alzheimer’s disease. Eur. J. Nucl. Med. Mol. Imaging 32, 593–600. doi: 10.1007/s00259-005-1780-5
Toyonaga, T., Fesharaki-Zadeh, A., Strittmatter, S. M., Carson, R. E., and Cai, Z. (2022). PET imaging of synaptic density: challenges and opportunities of synaptic vesicle glycoprotein 2A PET in small animal imaging. Front. Neurosci. 16:787404. doi: 10.3389/fnins.2022.787404
Toyonaga, T., Smith, L. M., Finnema, S. J., Gallezot, J. D., Naganawa, M., Bini, J., et al. (2019). In vivo synaptic density imaging with (11)C-UCB-J detects treatment effects of saracatinib in a mouse model of alzheimer disease. J. Nucl. Med. 60, 1780–1786. doi: 10.2967/jnumed.118.223867
Tran, H. T., LaFerla, F. M., Holtzman, D. M., and Brody, D. L. (2011). Controlled cortical impact traumatic brain injury in 3xTg-AD mice causes acute intra-axonal amyloid-beta accumulation and independently accelerates the development of tau abnormalities. J. Neurosci. 31, 9513–9525. doi: 10.1523/JNEUROSCI.0858-11.2011
Traynelis, S. F., Wollmuth, L. P., McBain, C. J., Menniti, F. S., Vance, K. M., Ogden, K. K., et al. (2010). Glutamate receptor ion channels: structure, regulation, and function. Pharmacol. Rev. 62, 405–496. doi: 10.1124/pr.109.002451
Tucker, K. L., Meyer, M., and Barde, Y. A. (2001). Neurotrophins are required for nerve growth during development. Nat. Neurosci. 4, 29–37. doi: 10.1038/82868
Varlow, C., Murrell, E., Holland, J. P., Kassenbrock, A., Shannon, W., Liang, S. H., et al. (2020). Revisiting the radiosynthesis of [(18)F]FPEB and preliminary PET imaging in a mouse model of Alzheimer’s disease. Molecules 25:982. doi: 10.3390/molecules25040982
Waldron, A. M., Verhaeghe, J., Wyffels, L., Schmidt, M., Langlois, X., Van Der Linden, A., et al. (2015a). Preclinical comparison of the amyloid-beta radioligands [(11)C]Pittsburgh compound B and [(18)F]florbetaben in aged APPPS1-21 and BRI1-42 mouse models of cerebral amyloidosis. Mol. Imaging Biol. 17, 688–696. doi: 10.1007/s11307-015-0833-9
Waldron, A. M., Wintmolders, C., Bottelbergs, A., Kelley, J. B., Schmidt, M. E., Stroobants, S., et al. (2015b). In vivo molecular neuroimaging of glucose utilization and its association with fibrillar amyloid-beta load in aged APPPS1-21 mice. Alzheimers Res. Ther. 7:76. doi: 10.1186/s13195-015-0158-6
Waldron, A. M., Wyffels, L., Verhaeghe, J., Richardson, J. C., Schmidt, M., Stroobants, S., et al. (2017). Longitudinal characterization of [18F]-FDG and [18F]-AV45 uptake in the double transgenic TASTPM mouse model. J. Alzheimers Dis. 55, 1537–1548. doi: 10.3233/JAD-160760
Wang, C., Wilson, W. A., Moore, S. D., Mace, B. E., Maeda, N., Schmechel, D. E., et al. (2005). Human apoE4-targeted replacement mice display synaptic deficits in the absence of neuropathology. Neurobiol. Dis. 18, 390–398. doi: 10.1016/j.nbd.2004.10.013
Wang, H. F., Tan, L., Cao, L., Zhu, X. C., Jiang, T., Tan, M. S., et al. (2016). Alzheimer’s disease neuroimaging, I. Application of the IWG-2 diagnostic criteria for Alzheimer’s disease to the ADNI. J. Alzheimers Dis. 51, 227–236. doi: 10.3233/JAD-150824
Wang, R., and Reddy, P. H. (2017). Role of glutamate and NMDA receptors in Alzheimer’s disease. J. Alzheimers Dis. 57, 1041–1048. doi: 10.3233/JAD-160763
Wang, Y. T., and Edison, P. (2019). Tau imaging in neurodegenerative diseases using positron emission tomography. Curr. Neurol. Neurosci. Rep. 19:45. doi: 10.1007/s11910-019-0962-7
Warnock, G. I., Aerts, J., Bahri, M. A., Bretin, F., Lemaire, C., Giacomelli, F., et al. (2014). Evaluation of 18F-UCB-H as a novel PET tracer for synaptic vesicle protein 2A in the brain. J. Nucl. Med. 55, 1336–1341. doi: 10.2967/jnumed.113.136143
Weng, C. C., Hsiao, I. T., Yang, Q. F., Yao, C. H., Tai, C. Y., Wu, M. F., et al. (2020). Characterization of (18)F-PM-PBB3 ((18)F-APN-1607) uptake in the rTg4510 mouse model of tauopathy. Molecules 25:1750. doi: 10.3390/molecules25071750
Wolfe, C. M., Fitz, N. F., Nam, K. N., Lefterov, I., and Koldamova, R. (2018). The role of APOE and TREM2 in Alzheimer’s disease-current understanding and perspectives. Int. J. Mol. Sci. 20:81. doi: 10.3390/ijms20010081
Wong, D. F., Comley, R. A., Kuwabara, H., Rosenberg, P. B., Resnick, S. M., Ostrowitzki, S., et al. (2018). Characterization of 3 novel tau radiopharmaceuticals, 11C-RO-963, 11C-RO-643, and 18F-RO-948, in healthy controls and in alzheimer subjects. J. Nucl. Med. 59, 1869–1876. doi: 10.2967/jnumed.118.209916
Xia, C. F., Arteaga, J., Chen, G., Gangadharmath, U., Gomez, L. F., Kasi, D., et al. (2013). [(18)F]T807, a novel tau positron emission tomography imaging agent for Alzheimer’s disease. Alzheimers Dement. 9, 666–676. doi: 10.1016/j.jalz.2012.11.008
Xiang, X., Piers, T. M., Wefers, B., Zhu, K., Mallach, A., Brunner, B., et al. (2018). The Trem2 R47H Alzheimer’s risk variant impairs splicing and reduces Trem2 mRNA and protein in mice but not in humans. Mol. Neurodegener. 13:49. doi: 10.1186/s13024-018-0280-6
Xiong, M., Roshanbin, S., Rokka, J., Schlein, E., Ingelsson, M., Sehlin, D., et al. (2021). In vivo imaging of synaptic density with [(11)C]UCB-J PET in two mouse models of neurodegenerative disease. Neuroimage 239:118302. doi: 10.1016/j.neuroimage.2021.118302
Xu, G., Ran, Y., Fromholt, S. E., Fu, C., Yachnis, A. T., Golde, T. E., et al. (2015). Murine Abeta over-production produces diffuse and compact Alzheimer-type amyloid deposits. Acta Neuropathol. Commun. 3:72. doi: 10.1186/s40478-015-0252-9
Yaghmoor, F., Noorsaeed, A., Alsaggaf, S., Aljohani, W., Scholtzova, H., Boutajangout, A., et al. (2014). The role of TREM2 in Alzheimer’s disease and other neurological disorders. J. Alzheimers Dis. Parkinson. 4:160. doi: 10.4172/2161-0460.1000160
Yamamoto, S., Nishiyama, S., Kawamata, M., Ohba, H., Wakuda, T., Takei, N., et al. (2011). Muscarinic receptor occupancy and cognitive impairment: a PET study with [11C](+)3-MPB and scopolamine in conscious monkeys. Neuropsychopharmacology 36, 1455–1465. doi: 10.1038/npp.2011.31
Yi, J. H., Whitcomb, D. J., Park, S. J., Martinez-Perez, C., Barbati, S. A., Mitchell, S. J., et al. (2020). M1 muscarinic acetylcholine receptor dysfunction in moderate Alzheimer’s disease pathology. Brain Commun. 2:fcaa058. doi: 10.1093/braincomms/fcaa058
Yu, C. H., Song, G. S., Yhee, J. Y., Kim, J. H., Im, K. S., Nho, W. G., et al. (2011). Histopathological and immunohistochemical comparison of the brain of human patients with Alzheimer’s disease and the brain of aged dogs with cognitive dysfunction. J. Comp. Pathol. 145, 45–58. doi: 10.1016/j.jcpa.2010.11.004
Yu, F., Zhang, Y., and Chuang, D. M. (2012). Lithium reduces BACE1 overexpression, beta amyloid accumulation, and spatial learning deficits in mice with traumatic brain injury. J. Neurotrauma 29, 2342–2351. doi: 10.1089/neu.2012.2449
Zeydan, B., Schwarz, C. G., Przybelski, S. A., Lesnick, T. G., Kremers, W. K., Senjem, M. L., et al. (2021). Comparison of 11 C-Pittsburgh compound-B and 18 F-flutemetamol white matter binding in PET. J. Nucl. Med. doi: 10.2967/jnumed.121.263281 [Epub ahead of print].
Zhao, J., Bi, W., Xiao, S., Lan, X., Cheng, X., Zhang, J., et al. (2019). Neuroinflammation induced by lipopolysaccharide causes cognitive impairment in mice. Sci. Rep. 9:5790. doi: 10.1038/s41598-019-42286-8
Zheng, C., Holden, D., Zheng, M. Q., Pracitto, R., Wilcox, K. C., Lindemann, M., et al. (2022). A metabolically stable PET tracer for imaging synaptic vesicle protein 2A: synthesis and preclinical characterization of [18F]SDM-16. Eur. J. Nucl. Med. Mol. Imaging 49, 1482–1496. doi: 10.1007/s00259-021-05597-5
Zhou, R., Ji, B., Kong, Y., Qin, L., Ren, W., Guan, Y., et al. (2021). PET imaging of neuroinflammation in Alzheimer’s disease. Front. Immunol. 12:739130. doi: 10.3389/fimmu.2021.739130
Zhou, X., Ji, B., Seki, C., Nagai, Y., Minamimoto, T., Fujinaga, M., et al. (2021). PET imaging of colony-stimulating factor 1 receptor: a head-to-head comparison of a novel radioligand. J. Cereb. Blood Flow Metab. 41, 2410–2422. doi: 10.1177/0271678X211004146
Keywords: positron emission tomography, Alzheimer’s disease, β-amyloid (Aβ), tau, neurodegeneration, SV2A, neuroinflamamation, animal model
Citation: Chen B, Marquez-Nostra B, Belitzky E, Toyonaga T, Tong J, Huang Y and Cai Z (2022) PET Imaging in Animal Models of Alzheimer’s Disease. Front. Neurosci. 16:872509. doi: 10.3389/fnins.2022.872509
Received: 09 February 2022; Accepted: 25 April 2022;
Published: 24 May 2022.
Edited by:
Ruiqing Ni, ETH Zürich, SwitzerlandReviewed by:
Nicolas Tournier, Commissariat à l’Energie Atomique et aux Energies Alternatives (CEA), FranceCopyright © 2022 Chen, Marquez-Nostra, Belitzky, Toyonaga, Tong, Huang and Cai. This is an open-access article distributed under the terms of the Creative Commons Attribution License (CC BY). The use, distribution or reproduction in other forums is permitted, provided the original author(s) and the copyright owner(s) are credited and that the original publication in this journal is cited, in accordance with accepted academic practice. No use, distribution or reproduction is permitted which does not comply with these terms.
*Correspondence: Zhengxin Cai, SmFzb24uY2FpQHlhbGUuZWR1
Disclaimer: All claims expressed in this article are solely those of the authors and do not necessarily represent those of their affiliated organizations, or those of the publisher, the editors and the reviewers. Any product that may be evaluated in this article or claim that may be made by its manufacturer is not guaranteed or endorsed by the publisher.
Research integrity at Frontiers
Learn more about the work of our research integrity team to safeguard the quality of each article we publish.