- 1Department of Neurology, Affiliated Hospital of Zunyi Medical University, Zunyi, China
- 2The Collaborative Innovation Center of Tissue Damage Repair and Regeneration Medicine of Zunyi Medical University, Zunyi, China
Epilepsy is a common chronic brain disease. There are many clinical methods to control epileptic seizures, such as anti-seizure medications (ASMs) or surgical removal of epileptogenic lesions. However, the pathophysiology of epilepsy is still unknown, making it difficult to control or prevent it. The host’s immune system monitors gut microbes, interacts with microbes through pattern recognition receptors such as Toll-like receptors (TLRs) and NOD-like receptors (NLRs) expressed by innate immune cells, and activates immune responses in the body to kill pathogens and balance the relationship between microbes and host. In addition, inflammatory responses induced by the innate immune system are seen in animal models of epilepsy and temporal lobe epilepsy brain tissue to combat pathogens or injuries. This review summarizes the potential relationship between gut microbes, innate immunity, and epilepsy based on recent research to provide more hints for researchers to explore this field further.
Introduction
As a common chronic neurological disorder, epilepsy is characterized by susceptibility to epileptic seizures and associated cognitive and psychological impairments (Devinsky et al., 2018). According to a meta-analysis, the annual incidence of epilepsy is approximately67.77 per 100,000 persons; age and gender have less effect on the incidence; the incidence is quite high in low- and middle-income nations (Fiest et al., 2017). Recently, intestinal microbial composition, metabolites, and synthetic functions in various neurological diseases have been gradually understood. The regulatory role of intestinal microbes has been found in neuropsychiatric diseases such as Alzheimer’s disease (AD), Parkinson’s disease (PD), and autism spectrum disorder (ASD) (Sharon et al., 2016). Epilepsy is a disabling neurological disorder, and its specific pathogenesis is still unclear. Research into the regulation of brain function by gut signals may help elucidate epilepsy pathogenesis, drug resistance, or potential therapeutic targets. Innate immunity is an important bridge linking vertebrate and invertebrate immune recognition in the long evolution of organisms (Litman and Cooper, 2007). Cellular pattern recognition receptors recognize highly conserved pathogen-associated molecular patterns (PAMPs) produced only by microorganisms to distinguish foreign tissues (Kaur and Secord, 2019). Some studies have pointed out that intestinal dysbiosis can induce peripheral inflammation and central nervous system (CNS) inflammation (or sterile inflammation) by inducing the innate immune system to produce cytokines (Levy et al., 2017). Although the brain is considered an immune-privileged site, there has been a steady stream of research on neuroinflammation and epilepsy (Vezzani et al., 2011). Neuroinflammation is often triggered by danger signals, including endogenous injury or infection, that rapidly activate pattern recognition receptors (PRRs) expressed by innate immune cells, altering seizure thresholds (Vezzani et al., 2016). This review summarizes the potential relationship between gut microbes, innate immunity, and epilepsy to provide more hints for future studies.
Possible Mechanisms by Which Intestinal Microbes Are Involved in Epilepsy
Intercommunication Between Intestinal Microbes and the Central Nervous System
There are rich connections between the CNS and intestinal microbes. Research has shown that intestinal microorganisms can modify the CNS, affecting behavior, mood, cognition, and even causing anxiety and depression. The gut–brain axis refers to the interaction between the gut microbes and the CNS, involving multiple systems such as nerves and endocrine (Collins et al., 2012; Holmes et al., 2012, 2020; Nicholson et al., 2012; Montiel-Castro et al., 2013). Intercommunication between intestinal microbes and the CNS involves two neuroanatomical pathways, and one is the direct exchange of information between the brain and the gut, including the vagus nerve in the spinal cord and the autonomic nervous system. The other is a bidirectional communication in the spinal cord through the enteric nervous system, autonomic nervous system, and vagus nerve of the gut (Wang and Wang, 2016).
Although the structure of the microorganism is relatively stable, factors including infection, diet, or genetics can also affect the intestinal flora, resulting in the growth of pathogenic bacteria, the loss of normal commensal bacteria, and the decline in diversity (Levy et al., 2017), which is called dysbiosis, and can further lead to diseases such as obesity (Gérard, 2016), autoimmune disease (Knip and Siljander, 2016), neurological disorders (Tremlett et al., 2017), and inflammatory bowel disease (Wlodarska et al., 2015). A study showed that using mass spectrometry to detect chemicals in the peripheral circulation in germ-free (GF) mice found that the synthesis of most substances depends on the gut microbiome (Wikoff et al., 2009). Substances produced by intestinal microbial metabolism can act on the CNS. For example, short-chain fatty acids (SCFAs) are the final product produced by microorganisms in the lower intestinal tract through fermentation, including acetic acid, propionic acid, and butyric acid (Martin-Gallausiaux et al., 2021), are important substrates for keeping the integrity of the epithelial barrier (Morrison and Preston, 2016), and are also involved in regulating human immune function and exerting anti-inflammatory effects (Martin-Gallausiaux et al., 2021). SCFAs can also penetrate the gut–blood barrier and the blood–brain barrier (BBB) (Macfabe, 2012), alter neurotransmitter and hormone concentrations (Alexander et al., 2019), reduce the permeability of the BBB (Braniste et al., 2014), and regulate the formation and function of microglia (Hu et al., 2020).
In addition, entheogenic GABA, 5-HT, can affect microglial activation in the brain (Abdel-Haq et al., 2019). Diaz Heijtz et al. (2011) showed that GF mice exhibited less anxiety-like behavior than conventional mice fed in a specific pathogen-free (SPF) environment. However, after the adult GF mice were transferred to the SPF environment, there was no significant increase or decrease in anxiety-like behavior, but the anxiety-like behavior of their offspring returned to normal (Diaz Heijtz et al., 2011). It has also been shown that ACTH and corticosterone levels under restraint stress are different in GF mice and SPF mice (Sudo et al., 2004). All of these studies illustrate the ability of gut microbes to regulate behavior.
Although the BBB separates the brain and peripheral tissues, gut microbiota can influence CNS function (Bercik et al., 2011; Diaz Heijtz et al., 2011). By comparing GF and SPF rats, Erny et al. (2015) showed that gut microbiota could affect microglial function, including reducing cell-activated gene transcription and increasing transcriptional repressor expression, and Mosher and Wyss-Coray (2015) observed that entheogenic SCFAs can promote microglial maturation and related gene expression in SPF mice. Microglia are located in the CNS and are the primary immune cells in the brain, but they are closely related to the mononuclear macrophage system (Erny et al., 2015) and can generate immune responses to peripheral inflammation. Combrinck et al. (2002) used an intraperitoneal injection of lipopolysaccharide (LPS) to induce peripheral systemic inflammation and observed further activation of activated microglia in the brain in animals with chronic inflammation, suggesting that there is an interaction between peripheral systemic inflammation and the CNS.
Gut microbial surface substances and their metabolites can positively or negatively affect the CNS through the peripheral circulation or enteric nervous system, and microbial-derived neurotransmitters have regulatory effects on neurons or nerve cells after entering the brain. The metabolites can not only play an anti-inflammatory effect and protect the BBB but also play a pro-inflammatory effect, activate microglia to secrete inflammatory mediators, and then damage the BBB, leading to leakage.
General Characteristics of the Gut Microbiota in Epileptic and Non-epileptic Patients
Gut microbes are a microbial ecosystem that exists in the human gastrointestinal tract. The average adult’s gut microbes weigh as much as the human brain, and these microbes encode genes that are more than 100 times more abundant than the human genome (Dinan et al., 2015), and there are more than 1014 kinds of microorganisms. In addition to bacteria, archaea, viruses, bacteriophages, yeasts, and fungi coexist in the gut (Cani, 2018). This complex microbial population is increasingly becoming an important role in affecting human health and is closely related to the stability of the human body’s internal environment. For example, (1) the formation of a bacterial barrier in the gut prevents pathogenic bacteria from penetrating the intestinal mucosal barrier and invading the host (Chopyk and Grakoui, 2020), (2) the synthesis of nutrients and supplements, such as SCFAs and vitamins (Bishehsari et al., 2018; Li et al., 2020), (3) detoxification of ingested dietary toxicants (Letertre et al., 2020), (4) modulation of host immune system function (Takiishi et al., 2017), and (5) gut–brain communication (Morais et al., 2021).
The characteristics of a healthy gut microbiome can be summed up in four words, namely, diversity, stability, resistance, and resilience. These four words correspond to the richness within the microbiota, the ability to adapt to environmental changes, and the ability of the microbiota to recover (Lozupone et al., 2012). To investigate the impact of gut microbes on humans, methods such as 16S ribosomal RNA gene sequencing (Johnson et al., 2019), metagenomic sequencing (Riesenfeld et al., 2004), and Illumina genome analyzers (Qin et al., 2010) are used to explore the composition of the microbiota. Alpha diversity (single site, such as the human gut) and beta diversity (multiple sites, such as different parts of the human body) were applied to evaluate sequencing data, and the human gut microbiota could be roughly divided into four phyla, namely, Bacteroidetes, Firmicutes, Proteobacteria, and Actinobacteria (Arumugam et al., 2011; Greenhalgh et al., 2016; Binda et al., 2018). Firmicutes and Bacteroidetes accounted for 90% of the intestinal flora abundance, and Proteobacteria and Actinobacteria accounted for 10%. The abundance of Bacteroidetes is associated with a high-fat, high-protein diet, and malnutrition (Johnson et al., 2017), while Firmicutes is increased in obese mice (Turnbaugh et al., 2006).
In healthy people, the composition of the gut microbiome is all about the same, but there is a marked difference in the microbial structure compared with epilepsy patients. According to Peng et al. (2018), an abnormally increased abundance of rare flora was observed in the patients with drug-resistant epilepsy, while patients with drug-sensitive epilepsy have an intestinal flora composition similar to healthy controls. Recently, a 22-year-old patient with drug-resistant epilepsy who received gut microbiota transplantation from a healthy individual remained seizure-free for 20 months despite discontinuation of anti-seizure medications (ASMs) (He et al., 2017). Therefore, it is inferred that normal gut microbiota can control epileptic seizures.
Effects of Modulating Gut Microbiota on Epileptic Seizures
Diet is the most important factor in modifying the structure and function of intestinal flora (Zmora et al., 2019). The ketogenic diet (KD) and the modified Atkins diet are commonly used for epilepsy control. They are characterized by high fat and low carbohydrates, making the body switch from consuming glucose to consuming ketone bodies produced by fat metabolism as an energy source (Ułamek-Kozioł et al., 2019). A study shows that children with drug-resistant epilepsy treated with a KD for seizures had lower gut microbial diversity than healthy infants (Xie et al., 2017). The KD also had different effects on the intestinal microbiota composition of healthy infants and children with drug-resistant epilepsy. Firmicutes did not change significantly in healthy infants before and after KD treatment, but the proportion of Bacteroidetes increased. In addition, Proteobacteria was more enriched in children with drug-resistant epilepsy, and the proportion decreased after KD treatment (Xie et al., 2017). In the study by Wells et al. (2020), KD had a good effect on seizure control. This study has shown that elevated levels of ketone bodies (through a KD, exogenous ketonic supplements, or weight loss) can reduce airway hyperresponsiveness in obese asthmatic rats, which may be related to the inhibition of inflammatory responses (Mank et al., 2022).
The possible mechanism of the KD for epilepsy is not fully understood, but it may exert antiepileptic effects by increasing endogenous adenosine (Masino et al., 2009), opening ATP-sensitive potassium channels (Juge et al., 2010), acting on adenosine A1 receptors (Kadowaki et al., 2017), and inhibiting lactate dehydrogenase (Sada et al., 2015). Furthermore, according to Braakman and van Ingen (2018), six drug-resistant epilepsy patients acquired seizure-freedom during antibiotic use, suggesting that antibiotics may be a potential treatment for epilepsy.
Recently, probiotics represented by Bifidobacteria and Lactobacilli exist in our daily diets, such as yogurt, nutritional supplements, and fermented biscuits. One study showed that after oral administration of 8 mixed probiotics (mainly Lactobacillus) as a supplement to antiepileptic drugs in epilepsy patients, 28.9% of patients had more than 50% reduction in seizures, which was similar to other new ASMs; meanwhile, the quality of life (QoL) in the effective group of probiotics was significantly improved (Gómez-Eguílaz et al., 2018), and it is suggested that probiotic supplementation therapy may be a new method to control epilepsy.
Possible Mechanisms by Which Innate Immunity Is Involved in Epileptic Seizures
The innate immune system is an evolutionarily ancient part consisting of barriers, small molecules, and cellular components. In 1885, Paul Ehrlich found that intravenous acid dyes could not stain brain tissue, and Emil Goldmann also found that brain tissue failed to stain after intravenous trypan blue in 1908 (Pachter et al., 2003), leading to the concept of BBB. The innate immune system protects organs and tissues from pathogenic damage without the need for additional measures (McComb et al., 2019). Innate immunity in the CNS includes the BBB, glial cells, and various cytokines.
Blood–Brain Barrier
The BBB acts as the dividing line between the central and peripheral parts, preventing blood cells, pathogens, and poisons from entering the brain (Montagne et al., 2017). The phenomenon that neurons regulate the BBB function puts forward the neurovascular unit (NVU) concept. NVU is formed by the mutual coupling of vascular-related cells, glial cells, and neurons (Zlokovic, 2011), which regulates not only cerebral blood flow (Lecrux and Hamel, 2011) but also the tight junction (TJ) protein between endothelium can limit the paracellular permeability of the BBB, reducing the substance transport between cerebrospinal fluid and blood (Zlokovic, 2008), thereby maintaining central environmental homeostasis. The basis of this barrier function depends on the structure of TJs between endothelial cells, which are mainly composed of claudin, occludin, and ZO proteins (Berndt et al., 2019; Lochhead et al., 2020; Yuan et al., 2020). Among them, claudin-5 is the main protein constituting TJs (Greene et al., 2019). Research shows that the CNS of claudin-5 knockout mice displays higher permeability to macromolecules (Lochhead et al., 2020). At the same time, in cultured cerebral vascular endothelial cells, claudin-5 overexpression showed decreased paracellular permeability and increased tightness (Ohtsuki and Terasaki, 2007). The study by Li et al. (2016) showed that sodium butyrate could improve the neurological deficit after traumatic brain injury, upregulate the expression of TJs, and reduce the permeability of the BBB. Some studies have also pointed out that the local leakage of plasma proteins caused by the increased permeability of the BBB may play a critical role in epileptogenesis (Bankstahl et al., 2018).
Gliacytes
In the CNS, astrocytes are one of the most abundant glial cells. They have an important role in the CNS and actively participate in the composition of the BBB or promote or limit the development of diseases (Linnerbauer et al., 2020). Furthermore, the role of astrocytes in epileptogenesis is increasingly well understood (Ivens et al., 2007; Seifert et al., 2010; Heinemann et al., 2012).
Glutamine synthase is an enzyme specifically expressed in astrocytes in the CNS, which can catalyze the synthesis of glutamine from ammonia and glutamate (Rose et al., 2013), thus maintaining CNS homeostasis, but decreased or lost glutamine synthase expression was found in patients with temporal lobe epilepsy (Sandhu et al., 2021), and this may be one of the possible mechanisms for inducing seizures. In addition, astrocytes also express a variety of potassium ion channels on the surface of astrocytes (Seifert et al., 2018) to maintain intracellular and extracellular potassium balance through potassium ion buffering and potassium ion uptake (Seifert and Steinhäuser, 2013). During the neuronal activity, extracellular potassium concentrations can rapidly fluctuate to upper levels, sufficient to induce seizures if the hyperkalemic environment is not properly corrected. Niday and Tzingounis (2018) suggested that extracellular potassium rises to depolarize neurons in the absence of Kir 4.1 channels, leading to the inactivation of sodium channels, thereby prolonging neuronal firing time or increasing the frequency, which causes neuronal damage hyperexcitability. Furthermore, in the research of Snowball et al. (2019), using a lentiviral vector packaging the engineered potassium channel (EKC) gene significantly reduced the number of seizures in focal neocortical epilepsy.
Microglia are macrophages in the brain parenchyma that derive from primitive hematopoiesis in the yolk sac (Aguzzi et al., 2013) and play an important role in neuronal health, apoptosis, and synapse formation (Nayak et al., 2014). Many studies show that microglia are closely related to neurological diseases (Hansen et al., 2018; Ho, 2019; Jia et al., 2021). The possible mechanisms include inflammation, gliosis, and stress. Microglia-derived inflammation is important in epileptogenesis, and ASMs with antiglial inflammatory properties benefit seizure control in a previous study (Dambach et al., 2014). Studies have also focused on the connection between microglia and neurons as the main factor for microglia activation, and the inflammatory proteins released by glial activation can increase excitability and contribute to epilepsy (Alyu and Dikmen, 2017).
Cytokines
As part of innate immunity, cytokines are also closely related to the BBB. For example, in Qin et al.’s (2019) study, it was found that interleukin-1β can induce the pericyte NF-κB/p65 pathway leading to upregulation of matrix metalloproteinase-9 expression to the destruction of vascular endothelial TJs, thereby increasing the permeability of the BBB. TGF-β plays a crucial role in human development. When TGF-β binds to TGF-βR2, downstream SMAD proteins are phosphorylated and translocated to the nucleus, ultimately regulating the transcription of target genes of TGF-β (Vander Ark et al., 2018). Albumin present in brain tissue is translocated into cells by astrocytes through binding to TGF-β receptors on astrocytes and by downregulating the Kir 4.1 potassium channel on the surface of astrocytes, and it leads to the decrease of extracellular buffer potassium, increasing N-methyl-D-aspartate (NMDA) receptor-mediated neuronal excitability (Ivens et al., 2007).
The IL-1 family consists of pro- and anti-inflammatory cytokines (Yazdi and Ghoreschi, 2016). Among them, interleukin-1β is the most characteristic pro-inflammatory interleukin. Description of IL-1β was initially thought to be an endogenous pyrogen that induces the expression of COX-2, iNOS, TNF-α, IL-6, chemokines, adhesion molecules, and matrix metalloproteinases (Dinarello, 2005).
Studies have shown that the genes for IL-1β, IL-1R1, and IL-1RA are overexpressed in rodent models of epilepsy (Mukhtar, 2020). IL-1R1 is a receptor for IL-1β, belonging to the Toll-like/IL-1 receptor, activating prostaglandins (PGs) and NF-κB through MyD88 protein, thereby inducing inflammation (Yazdi and Ghoreschi, 2016). IL-1RA is an endogenous inhibitor of IL-1R1 (Ferrara-Bowens et al., 2017). Vezzani et al. (2008) suggested that IL-1β activates IL-1R1 on neurons and induces tyrosine phosphorylation of the NR2B subunit of NMDA receptor through Src kinase, leading to increased NMDA receptor-mediated calcium influx, thereby enhancing neuronal excitability. IL-1β also promotes glutamate release and inhibits glutamate reuptake by astrocytes through TNF-α, thereby inducing epileptiform events (Vezzani et al., 2008). IL-6 is a cytokine with dual effects, and proper IL-6 expression is very important for host defense function. After infection or injury, IL-6 is rapidly secreted and produced by monocytes/macrophages (Tanaka et al., 2016). In the healthy CNS, IL-6 is lowly expressed, but astrocytes and microglia become important sources of IL-6 in the CNS (Gruol, 2015). When TGF-beta on astrocytes can transmit information to the cells, upregulation of IL-6 leads to increased cortical excitability and finally induces epileptiform discharges in vitro (Levy et al., 2015).
Cyclooxygenase (COX) is an enzyme present on the cell membrane that catalyzes arachidonic acid to PGs (Clària, 2003). COX has three isoenzymes, of which COX-2 is an inducible enzyme associated with inflammation (Zhu et al., 2020), and found the induction of COX-2 in a hippocampal kindled rat model, suggesting that COX-2 is a key factor in epileptogenesis (Rawat et al., 2019). Rojas et al., 2014. found that celecoxib has an antiepileptic effect on acute seizures, but the antiepileptic effect of NSAIDs appears to be related to the time of administration (Rojas et al., 2014). Besides, a previous study by Kovács et al. (2011) showed that intraperitoneal injection of LPS in WAG/Rij rats enhanced their spike-wave discharge (Coenen and Van Luijtelaar, 2003), they subsequently found that the number and duration of spike-wave discharge increased after intraventricular injection of LPS in WAG/Rij rats, NSAIDs (indomethacin) could eliminate this phenomenon, and this strongly validates the role of COX in epilepsy.
HMGB1 is a highly conserved and proteinaceous structure in cells and acts as a classic alarm protein due to its ability to activate DAMP receptors of the innate immune system when present extracellularly (Yang et al., 2020). There are many reported receptors of HMGB1, such as RAGE, TLR9, TLR4, CD24, and CXCR4 (Paudel et al., 2018), but only RAGE and TLR4 are not controversial (Andersson et al., 2018). Recently, the role of HMGB1 in epilepsy has gradually attracted researchers’ attention. Zhao et al. (2017) showed that anti-HMGB1 mAbs could antagonize seizures in various epilepsy models and TLR4 knockout mice. This antiepileptic effect was absent (Zhao et al., 2017). Fu et al. (2017) also found that anti-HMGB1 monoclonal antibodies can delay HMGB1 translocation and downregulate the expression of inflammation-related inflammation-related factors.
Lipopolysaccharide, albumin, and SCFAs in the peripheral circulation can act on various receptors, resulting in increased calcium conductance, downregulated potassium channels, promotion of intracellular transcription, and release of immune molecules to cause BBB damage, which in turn lowers the seizure threshold or promotes epileptiform discharges, which eventually lead to seizures.
The Possible Mechanism of Gut Microbiota Regulating Innate Immunity and Participating in Epilepsy
For the study of epileptogenesis, Devinsky et al. (2018) suggested that the spontaneous epileptic rat, which mimics the characteristics of human epileptic seizures, could be used as a research model for acquired epilepsy models, postnatal brain injury, or infection. In contrast, genetic models have spontaneous or induced genetic modifications that induce seizures. Epileptogenesis is caused by epileptogenic events (or risk factors) or genetic alterations that can persist long before the first clinical seizure and increase susceptibility to epilepsy, leading to spontaneous recurrent seizures (Pitkänen et al., 2015). Since the last century, the role of inflammation in epileptogenesis has been paid more and more attention. Inflammation is a defense mechanism of the body against damaging factors, and although the brain is considered an immune-privileged area, both innate and acquired immune responses can be rapidly induced in the CNS (Mukhtar, 2020).
As mentioned earlier, gut microbial structural stability plays an important role in metabolism, immunity, and homeostasis. Disturbances in the microbial structure are associated with a variety of neurological diseases, such as autism (Saurman et al., 2020), PD (Elfil et al., 2020), AD (Sochocka et al., 2019), and even affect mental behavior (Lach et al., 2018; Peirce and Alvi na, 2019). More and more studies have linked epilepsy susceptibility to changes in gut microbiota structure. For example, probiotic supplementation can reduce seizures by more than 50% in patients with epilepsy (Gómez-Eguílaz et al., 2018), antibiotics can affect seizures (Ghanizadeh and Berk, 2015; Lum et al., 2020), and dietary therapy can alter the structure of intestinal microbes to reduce seizures (Zhang et al., 2018). Another study found that mice transplanted with gut microbiota from depressed patients developed depression-like behaviors, while mice transplanted with gut microbiota from healthy individuals did not develop depression-like behaviors (Zheng et al., 2016).
Gut microbes can also affect CNS function, and Goehler et al. (2005) also found in mice fed specific strains that gut microbes can transmit pathogen signals to the CNS via the vagus nerve. Some pathogens in the gut can produce toxins (such as LPS) that cross the intestinal mucosal barrier and then enter the circulation, and finally, cross the BBB to induce neuroinflammation (Alexandrov et al., 2019). Neuroinflammation is well documented in AD and PD (Heneka et al., 2015; Rocha et al., 2018; Megur et al., 2020; Leng and Edison, 2021). Of course, it also contributes to epileptogenesis (Paudel et al., 2018; Sharma et al., 2019; Hodges and Lugo, 2020). In the experiments of Hu et al. (2020), the alteration of gut microbiota induced by a high-salt diet led to a decrease in the production of gut-derived SCFA and induced BBB dysfunction and microglial activation in mice, as well as the expression of cortical IL-1β, IL-6, and TNF-α.
Lipopolysaccharide is present on the surface of many bacterial membranes, and the host’s immune system can trigger the immune response by recognizing the conserved structures of microbial species in a manner called PAMPs (Takeuchi and Akira, 2010; Bertani and Ruiz, 2018). Gao et al. (2014) found elevated concentrations of TNF-α and IL-1β in hippocampal slices exposed to LPS early and that LPS could increase the frequency of epileptiform discharges and neuronal excitability. Györffy et al. (2014) suggested that in a genetic absence epilepsy model, WAG/Rij rat, peripheral injection of LPS can promote spike-wave discharge and found a variety of differentially expressed proteins in the brain, most of which are associated with epilepsy, LPS-related inflammation, and sleep. The experiments of Brunner et al. (2021) confirmed that exogenous supplementation of ketogenic supplement in WAG/Rij rats can reduce LPS-induced spike-wave discharge by inhibiting the inflammatory response.
As a significant neurotoxin and pro-inflammatory substance, LPS is a typical ligand of TLR4, which can alter synaptic transmission and affect long-term potentiation (Vezzani et al., 2013). In addition, the intracerebral injection of LPS lowered the seizure threshold (Matin et al., 2015). The use of LPS in the rat cerebral cortex can induce increased neuronal excitability and produce epileptiform discharges, and this effect can be antagonized by IL-1RA, suggesting that LPS may exert such effects through IL-1R (Vezzani et al., 2013). Studies have also shown that intestinal inflammation can increase susceptibility to epilepsy and reduce the efficacy of ASMs (De Caro et al., 2019). Riazi et al. (2010) found that intestinal inflammation exacerbates seizures, and inducing peripheral inflammation using LPS increases epilepsy susceptibility. Endogenous ligands of TLR4, including HMGB1 and IL-1β, are produced by glial cells after brain injury, thereby mimicking LPS to exert pro-inflammatory effects (Devinsky et al., 2013).
The BBB is the major regulator of various molecules and cells into or out of the CNS, including microglia, endothelial cells, astrocytes, pericytes, and basement membranes (Sharif et al., 2018). TJs between endothelium are a major factor in determining their permeability, and a lack of TJ protein expression shows increased permeability to small molecules (Sweeney et al., 2019). LPS downregulates the expression of TJs, thereby increasing the permeability of the BBB. The possible mechanisms include the destruction of the BBB caused by PGs or nitric oxide (Varatharaj and Galea, 2017). Other possible factors include matrix metalloproteinases (Qin et al., 2015) and reactive oxygen species (Yu et al., 2015). The permeability of the BBB can also be modulated by intestinal flora, and microbial-derived metabolites can promote the integrity of the BBB (Parker et al., 2020), which controls the flow of circulating substances into and out of the brain. Braniste et al. (2014) suggested that intestinal flora disturbance is beneficial to increasing BBB permeability. The most pronounced change following increased BBB permeability is albumin penetration (van Vliet et al., 2015), which is involved in epileptogenicity by inducing excitatory synaptogenesis by binding to TGF-β receptors on astrocytes (Weissberg et al., 2015). In addition, albumin binding to TGF-β induces seizures by mediating impaired extracellular potassium buffering and increased neuronal excitability (Ivens et al., 2007).
Furthermore, LPS-induced systemic inflammation can lead to transcriptional activation of microglia inflammatory genes throughout the brain (Soulet and Rivest, 2008). After the IL-1R1 and TLR4 expressed on its surface are activated by endogenous ligands, the transcription of inflammatory genes mediated by NF-κB and activator protein-1 (AP-1) is formed, thereby continuing the inflammatory event (Vezzani et al., 2016). Microglia, as the primary immune cells in the brain, participate in the formation of NVU (Abbott et al., 2010) and can respond rapidly to any damage to the CNS (Liu et al., 2020), and their pro-inflammatory phenotype (M1) has demonstrated a damaging effect on the NVU (Boche et al., 2013). In the study by Ronaldson and Davis (2020), after 7 days of in vivo injection of LPS to induce inflammation, it was found that activated microglia can damage the integrity of the BBB and cause BBB leakage, to understand the effect of circulating cytokines on the BBB; Nzou et al. (2020) co-incubated tissue sections with exogenous cytokines and found that IL-6 and TNF-α can also affect the distribution of TJs, leading to leakage of the BBB. This evidence suggests that systemic inflammation has a role in regulating the permeability of the BBB. Mazarati et al. (2017) also suggested that inflammatory cytokines produced by peripheral inflammation activate microglia through peripheral afferent nerves or the BBB, thereby synthesizing cytokines to induce inflammation.
Conclusion
The relationship between epilepsy and gut microbes has received more and more attention, and related research is also in the ascendant. The intestine is the largest gathering place of microorganisms in the human body. A reasonable microbial population can promote the formation of the intestinal immune system and become the immune barrier of the human body. The disorder of the intestinal flora structure weakens the function of the intestinal immune barrier. The various innate immune molecules produced by it can induce local or systemic inflammation and even cause damage to the distant BBB structure through its surface molecules or metabolites (see Figure 1). The destruction of the BBB exposes the brain tissue to the attack of peripheral inflammatory factors or immune cells, which in turn induces glial cells in the brain to produce inflammatory factors, lowers the seizure threshold or induces epileptiform discharges, and even directly leads to epileptic seizures (see Figure 2), all of these have positive effects on the occurrence and development of epilepsy. This study reviews the possible mechanisms by which gut microbes regulate innate immune function and participate in the development of epilepsy, providing a better direction for further research in this field.
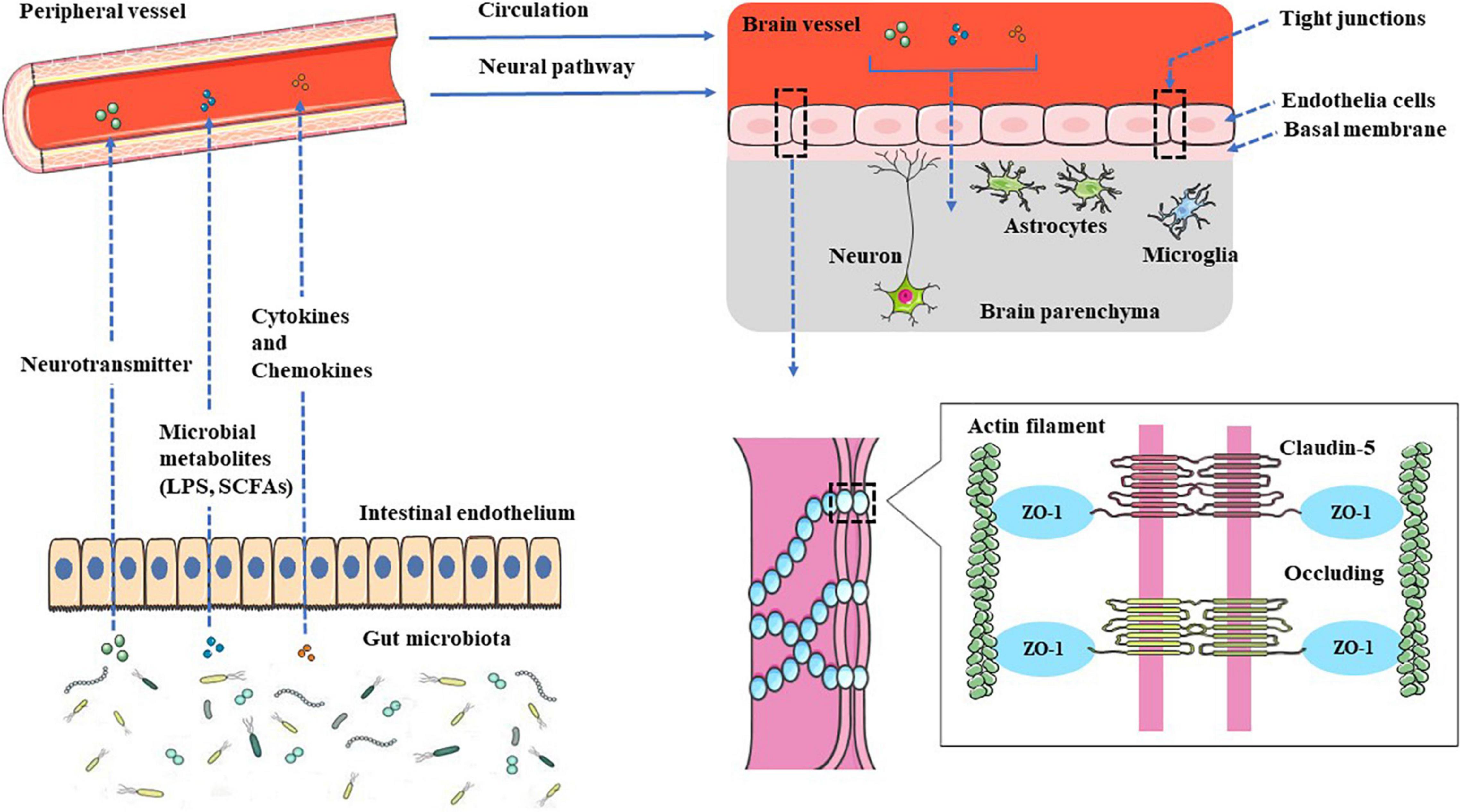
Figure 1. Gut–brain communication. Gut microbial surface substances and their metabolites can positively or negatively affect the CNS through the peripheral circulation or enteric nervous system, and microbial-derived neurotransmitters have regulatory effects on neurons or nerve cells after entering the brain. The metabolites can not only play an anti-inflammatory effect, protect the BBB but also play a pro-inflammatory effect, activate microglia to secrete inflammatory mediators, and then damage the BBB, leading to leakage.
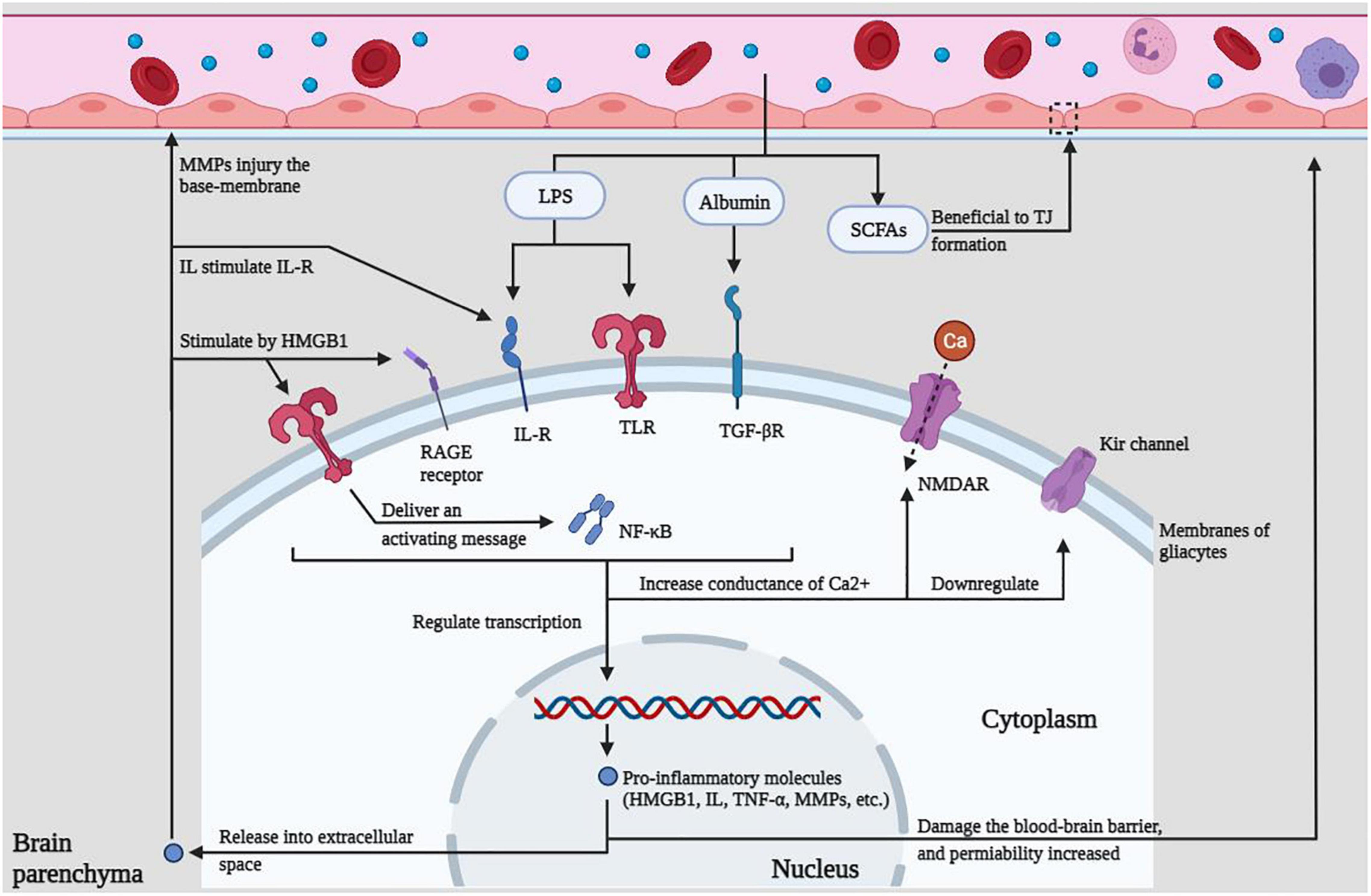
Figure 2. Innate immune regulation within the CNS. LPS, albumin, and SCFAs in the peripheral circulation can act on various receptors, resulting in increased calcium conductance, down-regulated potassium channels, promotion of intracellular transcription, and release of immune molecules to cause BBB damage, which in turn lowers the seizure threshold or promotes epileptiform discharges, which eventually lead to seizures.
Author Contributions
LZ, SL, and ZT designed and wrote the manuscript. ZX and CY helped with proofreading and revision. All authors contributed to the article and approved the final version.
Funding
Expenses related to the manuscript publication were supported by the funding of the Collaborative Innovation Center of Chinese Ministry of Education (2020-39).
Conflict of Interest
The authors declare that the research was conducted in the absence of any commercial or financial relationships that could be construed as a potential conflict of interest.
Publisher’s Note
All claims expressed in this article are solely those of the authors and do not necessarily represent those of their affiliated organizations, or those of the publisher, the editors and the reviewers. Any product that may be evaluated in this article, or claim that may be made by its manufacturer, is not guaranteed or endorsed by the publisher.
References
Abbott, N. J., Patabendige, A. A., Dolman, D. E., Yusof, S. R., and Begley, D. J. (2010). Structure and function of the blood-brain barrier. Neurobiol. Dis. 37, 13–25. doi: 10.1016/j.nbd.2009.07.030
Abdel-Haq, R., Schlachetzki, J., Glass, C. K., and Mazmanian, S. K. (2019). Microbiome-microglia connections via the gut-brain axis. J. Exp. Med. 216, 41–59. doi: 10.1084/jem.20180794
Aguzzi, A., Barres, B. A., and Bennett, M. L. (2013). Microglia: scapegoat, saboteur, or something else. Science 339, 156–161. doi: 10.1126/science.1227901
Alexander, C., Swanson, K. S., Fahey, G. C., and Garleb, K. A. (2019). Perspective: physiologic importance of short-chain fatty acids from nondigestible carbohydrate fermentation. Adv. Nutr. 10, 576–589. doi: 10.1093/advances/nmz004
Alexandrov, P., Zhai, Y., Li, W., and Lukiw, W. (2019). Lipopolysaccharide-stimulated, NF- kB-, miRNA-146a- and miRNA-155-mediated molecular-genetic communication between the human gastrointestinal tract microbiome and the brain. Folia Neuropathol. 57, 211–219. doi: 10.5114/fn.2019.88449
Alyu, F., and Dikmen, M. (2017). Inflammatory aspects of epileptogenesis: contribution of molecular inflammatory mechanisms. Acta Neuropsychiatr. 29, 1–16. doi: 10.1017/neu.2016.47
Andersson, U., Yang, H., and Harris, H. (2018). Extracellular HMGB1 as a therapeutic target in inflammatory diseases. Expert Opin. Ther. Targets 22, 263–277. doi: 10.1080/14728222.2018.1439924
Arumugam, M., Raes, J., Pelletier, E., Le Paslier, D., Yamada, T., Mende, D. R., et al. (2011). Enterotypes of the human gut microbiome. Nature 473, 174–180. doi: 10.1038/nature09944
Bankstahl, M., Breuer, H., Leiter, I., Märkel, M., Bascu nana, P., Michalski, D., et al. (2018). Blood-brain barrier leakage during early epileptogenesis is associated with rapid remodeling of the neurovascular unit. eNeuro 5:ENEURO.0123-18.2018. doi: 10.1523/ENEURO.0123-18.2018
Bercik, P., Denou, E., Collins, J., Jackson, W., Lu, J., Jury, J., et al. (2011). The intestinal microbiota affect central levels of brain-derived neurotropic factor and behavior in mice. Gastroenterology 141, 599–609, 609.e1–3. doi: 10.1053/j.gastro.2011.04.052
Berndt, P., Winkler, L., Cording, J., Breitkreuz-Korff, O., Rex, A., Dithmer, S., et al. (2019). Tight junction proteins at the blood-brain barrier: far more than claudin-5. Cell. Mol. Life Sci. 76, 1987–2002. doi: 10.1007/s00018-019-03030-7
Bertani, B., and Ruiz, N. (2018). Function and biogenesis of lipopolysaccharides. EcoSal Plus 8. doi: 10.1128/ecosalplus.ESP-0001-2018
Binda, C., Lopetuso, L. R., Rizzatti, G., Gibiino, G., Cennamo, V., and Gasbarrini, A. (2018). Actinobacteria: a relevant minority for the maintenance of gut homeostasis. Dig. Liver Dis. 50, 421–428. doi: 10.1016/j.dld.2018.02.012
Bishehsari, F., Engen, P. A., Preite, N. Z., Tuncil, Y. E., Naqib, A., Shaikh, M., et al. (2018). Dietary fiber treatment corrects the composition of gut microbiota, promotes SCFA Production, and suppresses colon carcinogenesis. Genes (Basel) 9:102. doi: 10.3390/genes9020102
Boche, D., Perry, V. H., and Nicoll, J. A. (2013). Review: activation patterns of microglia and their identification in the human brain. Neuropathol. Appl. Neurobiol. 39, 3–18. doi: 10.1111/nan.12011
Braakman, H., and van Ingen, J. (2018). Can epilepsy be treated by antibiotics. J. Neurol. 265, 1934–1936. doi: 10.1007/s00415-018-8943-3
Braniste, V., Al-Asmakh, M., Kowal, C., Anuar, F., Abbaspour, A., Tóth, M., et al. (2014). The gut microbiota influences blood-brain barrier permeability in mice. Sci. Transl. Med. 6:263ra158. doi: 10.1126/scitranslmed.3009759
Brunner, B., Ari, C., D’Agostino, D. P., and Kovács, Z. (2021). Adenosine receptors modulate the exogenous ketogenic supplement-evoked alleviating effect on lipopolysaccharide-generated increase in absence epileptic activity in WAG/Rij Rats. Nutrients 13:4082. doi: 10.3390/nu13114082
Cani, P. D. (2018). Human gut microbiome: hopes, threats and promises. Gut 67, 1716–1725. doi: 10.1136/gutjnl-2018-316723
Chopyk, D. M., and Grakoui, A. (2020). Contribution of the intestinal microbiome and gut barrier to hepatic disorders. Gastroenterology 159, 849–863. doi: 10.1053/j.gastro.2020.04.077
Clària, J. (2003). Cyclooxygenase-2 biology. Curr. Pharm. Des. 9, 2177–2190. doi: 10.2174/1381612033454054
Coenen, A. M., and Van Luijtelaar, E. L. (2003). Genetic animal models for absence epilepsy: a review of the WAG/Rij strain of rats. Behav. Genet. 33, 635–655. doi: 10.1023/a:1026179013847
Collins, S. M., Surette, M., and Bercik, P. (2012). The interplay between the intestinal microbiota and the brain. Nat. Rev. Microbiol. 10, 735–742. doi: 10.1038/nrmicro2876
Combrinck, M. I., Perry, V. H., and Cunningham, C. (2002). Peripheral infection evokes exaggerated sickness behaviour in pre-clinical murine prion disease. Neuroscience 112, 7–11. doi: 10.1016/s0306-4522(02)00030-1
Dambach, H., Hinkerohe, D., Prochnow, N., Stienen, M. N., Moinfar, Z., Haase, C. G., et al. (2014). Glia and epilepsy: experimental investigation of antiepileptic drugs in an astroglia/microglia co-culture model of inflammation. Epilepsia 55, 184–192. doi: 10.1111/epi.12473
De Caro, C., Leo, A., Nesci, V., Ghelardini, C., di Cesare Mannelli, L., Striano, P., et al. (2019). Intestinal inflammation increases convulsant activity and reduces antiepileptic drug efficacy in a mouse model of epilepsy. Sci. Rep. 9:13983. doi: 10.1038/s41598-019-50542-0
Devinsky, O., Vezzani, A., Najjar, S., De Lanerolle, N. C., and Rogawski, M. A. (2013). Glia and epilepsy: excitability and inflammation. Trends Neurosci. 36, 174–184. doi: 10.1016/j.tins.2012.11.008
Devinsky, O., Vezzani, A., O’Brien, T. J., Jette, N., Scheffer, I. E., de Curtis, M., et al. (2018). Epilepsy. Nat. Rev. Dis. Primers 4:18024. doi: 10.1038/nrdp.2018.24
Diaz Heijtz, R., Wang, S., Anuar, F., Qian, Y., Björkholm, B., Samuelsson, A., et al. (2011). Normal gut microbiota modulates brain development and behavior. Proc. Natl. Acad. Sci. U.S.A. 108, 3047–3052. doi: 10.1073/pnas.1010529108
Dinan, T. G., Stilling, R. M., Stanton, C., and Cryan, J. F. (2015). Collective unconscious: how gut microbes shape human behavior. J. Psychiatr. Res. 63, 1–9. doi: 10.1016/j.jpsychires.2015.02.021
Dinarello, C. A. (2005). Interleukin-1beta. Crit. Care Med. 33, S460–S462. doi: 10.1097/01.ccm.0000185500.11080.91
Elfil, M., Kamel, S., Kandil, M., Koo, B. B., and Schaefer, S. M. (2020). Implications of the gut microbiome in Parkinson’s disease. Mov. Disord. 35, 921–933. doi: 10.1002/mds.28004
Erny, D., Hrabì de Angelis, A. L., Jaitin, D., Wieghofer, P., Staszewski, O., David, E., et al. (2015). Host microbiota constantly control maturation and function of microglia in the CNS. Nat. Neurosci. 18, 965–977. doi: 10.1038/nn.4030
Ferrara-Bowens, T. M., Chandler, J. K., Guignet, M. A., Irwin, J. F., Laitipaya, K., Palmer, D. D., et al. (2017). Neuropathological and behavioral sequelae in IL-1R1 and IL-1Ra gene knockout mice after soman (GD) exposure. Neurotoxicology 63, 43–56. doi: 10.1016/j.neuro.2017.08.010
Fiest, K. M., Sauro, K. M., Wiebe, S., Patten, S. B., Kwon, C. S., Dykeman, J., et al. (2017). Prevalence and incidence of epilepsy: a systematic review and meta-analysis of international studies. Neurology 88, 296–303. doi: 10.1212/WNL.0000000000003509
Fu, L., Liu, K., Wake, H., Teshigawara, K., Yoshino, T., Takahashi, H., et al. (2017). Therapeutic effects of anti-HMGB1 monoclonal antibody on pilocarpine-induced status epilepticus in mice. Sci. Rep. 7:1179. doi: 10.1038/s41598-017-01325-y
Gao, F., Liu, Z., Ren, W., and Jiang, W. (2014). Acute lipopolysaccharide exposure facilitates epileptiform activity via enhanced excitatory synaptic transmission and neuronal excitability in vitro. Neuropsychiatr. Dis. Treat. 10, 1489–1495. doi: 10.2147/NDT.S65695
Gérard, P. (2016). Gut microbiota and obesity. Cell. Mol. Life Sci. 73, 147–162. doi: 10.1007/s00018-015-2061-5
Ghanizadeh, A., and Berk, M. (2015). Beta-lactam antibiotics as a possible novel therapy for managing epilepsy and autism, a case report and review of literature. Iran. J. Child Neurol. 9, 99–102.
Goehler, L. E., Gaykema, R. P., Opitz, N., Reddaway, R., Badr, N., and Lyte, M. (2005). Activation in vagal afferents and central autonomic pathways: early responses to intestinal infection with Campylobacter jejuni. Brain Behav. Immun. 19, 334–344. doi: 10.1016/j.bbi.2004.09.002
Gómez-Eguílaz, M., Ramón-Trapero, J. L., Pérez-Martínez, L., and Blanco, J. R. (2018). The beneficial effect of probiotics as a supplementary treatment in drug-resistant epilepsy: a pilot study. Benef. Microbes 9, 875–881. doi: 10.3920/BM2018.0018
Greene, C., Hanley, N., and Campbell, M. (2019). Claudin-5: gatekeeper of neurological function. Fluids Barriers CNS 16:3. doi: 10.1186/s12987-019-0123-z
Greenhalgh, K., Meyer, K. M., Aagaard, K. M., and Wilmes, P. (2016). The human gut microbiome in health: establishment and resilience of microbiota over a lifetime. Environ. Microbiol. 18, 2103–2116. doi: 10.1111/1462-2920.13318
Gruol, D. L. (2015). IL-6 regulation of synaptic function in the CNS. Neuropharmacology 96, 42–54. doi: 10.1016/j.neuropharm.2014.10.023
Györffy, B., Kovács, Z., Gulyássy, P., Simor, A., Völgyi, K., Orbán, G., et al. (2014). Brain protein expression changes in WAG/Rij rats, a genetic rat model of absence epilepsy after peripheral lipopolysaccharide treatment. Brain Behav. Immun. 35, 86–95. doi: 10.1016/j.bbi.2013.09.001
Hansen, D. V., Hanson, J. E., and Sheng, M. (2018). Microglia in Alzheimer’s disease. J. Cell Biol. 217, 459–472. doi: 10.1083/jcb.201709069
He, Z., Cui, B. T., Zhang, T., Li, P., Long, C. Y., Ji, G. Z., et al. (2017). Fecal microbiota transplantation cured epilepsy in a case with Crohn’s disease: the first report. World J. Gastroenterol. 23, 3565–3568. doi: 10.3748/wjg.v23.i19.3565
Heinemann, U., Kaufer, D., and Friedman, A. (2012). Blood-brain barrier dysfunction, TGFβ signaling, and astrocyte dysfunction in epilepsy. Glia 60, 1251–1257. doi: 10.1002/glia.22311
Heneka, M. T., Carson, M. J., El Khoury, J., Landreth, G. E., Brosseron, F., Feinstein, D. L., et al. (2015). Neuroinflammation in Alzheimer’s disease. Lancet Neurol. 14, 388–405. doi: 10.1016/S1474-4422(15)70016-5
Ho, M. S. (2019). Microglia in Parkinson’s Disease. Adv. Exp. Med. Biol. 1175, 335–353. doi: 10.1007/978-981-13-9913-8_13
Hodges, S. L., and Lugo, J. N. (2020). Therapeutic role of targeting mTOR signaling and neuroinflammation in epilepsy. Epilepsy Res. 161:106282. doi: 10.1016/j.eplepsyres.2020.106282
Holmes, E., Kinross, J., Gibson, G. R., Burcelin, R., Jia, W., Pettersson, S., et al. (2012). Therapeutic modulation of microbiota-host metabolic interactions. Sci. Transl. Med. 4:137rv6. doi: 10.1126/scitranslmed.3004244
Holmes, M., Flaminio, Z., Vardhan, M., Xu, F., Li, X., Devinsky, O., et al. (2020). Cross talk between drug-resistant epilepsy and the gut microbiome. Epilepsia 61, 2619–2628. doi: 10.1111/epi.16744
Hu, L., Zhu, S., Peng, X., Li, K., Peng, W., Zhong, Y., et al. (2020). High salt elicits brain inflammation and cognitive dysfunction, accompanied by alternations in the gut microbiota and decreased SCFA production. J. Alzheimers Dis. 77, 629–640. doi: 10.3233/JAD-200035
Ivens, S., Kaufer, D., Flores, L. P., Bechmann, I., Zumsteg, D., Tomkins, O., et al. (2007). TGF-beta receptor-mediated albumin uptake into astrocytes is involved in neocortical epileptogenesis. Brain 130, 535–547. doi: 10.1093/brain/awl317
Jia, X., Gao, Z., and Hu, H. (2021). Microglia in depression: current perspectives. Sci. China Life Sci. 64, 911–925. doi: 10.1007/s11427-020-1815-6
Johnson, E. L., Heaver, S. L., Walters, W. A., and Ley, R. E. (2017). Microbiome and metabolic disease: revisiting the bacterial phylum Bacteroidetes. J. Mol. Med. (Berl.) 95, 1–8. doi: 10.1007/s00109-016-1492-2
Johnson, J. S., Spakowicz, D. J., Hong, B. Y., Petersen, L. M., Demkowicz, P., Chen, L., et al. (2019). Evaluation of 16S rRNA gene sequencing for species and strain-level microbiome analysis. Nat. Commun. 10:5029. doi: 10.1038/s41467-019-13036-1
Juge, N., Gray, J. A., Omote, H., Miyaji, T., Inoue, T., Hara, C., et al. (2010). Metabolic control of vesicular glutamate transport and release. Neuron 68, 99–112. doi: 10.1016/j.neuron.2010.09.002
Kadowaki, A., Sada, N., Juge, N., Wakasa, A., Moriyama, Y., and Inoue, T. (2017). Neuronal inhibition and seizure suppression by acetoacetate and its analog, 2-phenylbutyrate. Epilepsia 58, 845–857. doi: 10.1111/epi.13718
Kaur, B. P., and Secord, E. (2019). Innate Immunity. Pediatr. Clin. North Am. 66, 905–911. doi: 10.1016/j.pcl.2019.06.011
Knip, M., and Siljander, H. (2016). The role of the intestinal microbiota in type 1 diabetes mellitus. Nat. Rev. Endocrinol. 12, 154–167. doi: 10.1038/nrendo.2015.218
Kovács, Z., Czurkó, A., Kékesi, K. A., and Juhász, G. (2011). Intracerebroventricularly administered lipopolysaccharide enhances spike-wave discharges in freely moving WAG/Rij rats. Brain. Res. Bull. 85, 410–416. doi: 10.1016/j.brainresbull.2011.05.003
Lach, G., Schellekens, H., Dinan, T. G., and Cryan, J. F. (2018). Anxiety, depression, and the microbiome: a role for gut peptides. Neurotherapeutics 15, 36–59. doi: 10.1007/s13311-017-0585-0
Lecrux, C., and Hamel, E. (2011). The neurovascular unit in brain function and disease. Acta Physiol. (Oxf.) 203, 47–59. doi: 10.1111/j.1748-1716.2011.02256.x
Leng, F., and Edison, P. (2021). Neuroinflammation and microglial activation in Alzheimer disease: where do we go from here. Nat. Rev. Neurol. 17, 157–172. doi: 10.1038/s41582-020-00435-y
Letertre, M., Munjoma, N., Wolfer, K., Pechlivanis, A., McDonald, J., Hardwick, R. N., et al. (2020). A two-way interaction between methotrexate and the gut microbiota of male sprague-dawley rats. J. Proteome Res. 19, 3326–3339. doi: 10.1021/acs.jproteome.0c00230
Levy, M., Kolodziejczyk, A. A., Thaiss, C. A., and Elinav, E. (2017). Dysbiosis and the immune system. Nat. Rev. Immunol. 17, 219–232. doi: 10.1038/nri.2017.7
Levy, N., Milikovsky, D. Z., Baranauskas, G., Vinogradov, E., David, Y., Ketzef, M., et al. (2015). Differential TGF-β signaling in glial subsets underlies IL-6-mediated epileptogenesis in mice. J. Immunol. 195, 1713–1722. doi: 10.4049/jimmunol.1401446
Li, H., Sun, J., Wang, F., Ding, G., Chen, W., Fang, R., et al. (2016). Sodium butyrate exerts neuroprotective effects by restoring the blood-brain barrier in traumatic brain injury mice. Brain Res. 1642, 70–78. doi: 10.1016/j.brainres.2016.03.031
Li, Y., Luo, Z. Y., Hu, Y. Y., Bi, Y. W., Yang, J. M., Zou, W. J., et al. (2020). The gut microbiota regulates autism-like behavior by mediating vitamin B(6) homeostasis in EphB6-deficient mice. Microbiome 8:120. doi: 10.1186/s40168-020-00884-z
Linnerbauer, M., Wheeler, M. A., and Quintana, F. J. (2020). Astrocyte Crosstalk in CNS Inflammation. Neuron 108, 608–622. doi: 10.1016/j.neuron.2020.08.012
Litman, G. W., and Cooper, M. D. (2007). Why study the evolution of immunity. Nat. Immunol. 8, 547–548. doi: 10.1038/ni0607-547
Liu, L. R., Liu, J. C., Bao, J. S., Bai, Q. Q., and Wang, G. Q. (2020). Interaction of microglia and astrocytes in the neurovascular unit. Front. Immunol. 11:1024. doi: 10.3389/fimmu.2020.01024
Lochhead, J. J., Yang, J., Ronaldson, P. T., and Davis, T. P. (2020). Structure, function, and regulation of the blood-brain barrier tight junction in central nervous system disorders. Front Physiol. 11:914. doi: 10.3389/fphys.2020.00914
Lozupone, C. A., Stombaugh, J. I., Gordon, J. I., Jansson, J. K., and Knight, R. (2012). Diversity, stability and resilience of the human gut microbiota. Nature 489, 220–230. doi: 10.1038/nature11550
Lum, G. R., Olson, C. A., and Hsiao, E. Y. (2020). Emerging roles for the intestinal microbiome in epilepsy. Neurobiol. Dis. 135:104576. doi: 10.1016/j.nbd.2019.104576
Macfabe, D. F. (2012). Short-chain fatty acid fermentation products of the gut microbiome: implications in autism spectrum disorders. Microb. Ecol. Health Dis. 23. doi: 10.3402/mehd.v23i0.19260
Mank, M. M., Reed, L. F., Walton, C. J., Barup, M., Ather, J. L., and Poynter, M. E. (2022). Therapeutic ketosis decreases methacholine hyperresponsiveness in mouse models of inherent obese asthma. Am. J. Physiol. Lung Cell. Mol. Physiol. 322, 243–L257. doi: 10.1152/ajplung.00309.2021
Martin-Gallausiaux, C., Marinelli, L., Blottière, H. M., Larraufie, P., and Lapaque, N. (2021). SCFA: mechanisms and functional importance in the gut. Proc. Nutr. Soc. 80, 37–49. doi: 10.1017/S0029665120006916
Masino, S. A., Kawamura, M., Wasser, C. D., Pomeroy, L. T., and Ruskin, D. N. (2009). Adenosine, ketogenic diet and epilepsy: the emerging therapeutic relationship between metabolism and brain activity. Curr. Neuropharmacol. 7, 257–268. doi: 10.2174/157015909789152164
Matin, N., Tabatabaie, O., Falsaperla, R., Lubrano, R., Pavone, P., Mahmood, F., et al. (2015). Epilepsy and innate immune system: a possible immunogenic predisposition and related therapeutic implications. Hum. Vaccin. Immunother. 11, 2021–2029. doi: 10.1080/21645515.2015.1034921
Mazarati, A. M., Lewis, M. L., and Pittman, Q. J. (2017). Neurobehavioral comorbidities of epilepsy: role of inflammation. Epilepsia 58(Suppl. 3) 48–56. doi: 10.1111/epi.13786
McComb, S., Thiriot, A., Akache, B., Krishnan, L., and Stark, F. (2019). Introduction to the Immune System. Methods Mol. Biol. 2024, 1–24. doi: 10.1007/978-1-4939-9597-4_1
Megur, A., Baltriukienë, D., Bukelskienë, V., and Burokas, A. (2020). The microbiota-gut-brain axis and Alzheimer’s disease: neuroinflammation is to blame. Nutrients 13:37. doi: 10.3390/nu13010037
Montagne, A., Zhao, Z., and Zlokovic, B. V. (2017). Alzheimer’s disease: a matter of blood-brain barrier dysfunction. J. Exp. Med. 214, 3151–3169. doi: 10.1084/jem.20171406
Montiel-Castro, A. J., González-Cervantes, R. M., Bravo-Ruiseco, G., and Pacheco-López, G. (2013). The microbiota-gut-brain axis: neurobehavioral correlates, health and sociality. Front. Integr. Neurosci. 7:70. doi: 10.3389/fnint.2013.00070
Morais, L. H., Schreiber, H. L. IV, and Mazmanian, S. K. (2021). The gut microbiota-brain axis in behaviour and brain disorders. Nat. Rev. Microbiol. 19, 241–255. doi: 10.1038/s41579-020-00460-0
Morrison, D. J., and Preston, T. (2016). Formation of short chain fatty acids by the gut microbiota and their impact on human metabolism. Gut Microbes 7, 189–200. doi: 10.1080/19490976.2015.1134082
Mosher, K. I., and Wyss-Coray, T. (2015). Go with your gut: microbiota meet microglia. Nat. Neurosci. 18, 930–931. doi: 10.1038/nn.4051
Mukhtar, I. (2020). Inflammatory and immune mechanisms underlying epileptogenesis and epilepsy: from pathogenesis to treatment target. Seizure 82, 65–79. doi: 10.1016/j.seizure.2020.09.015
Nayak, D., Roth, T. L., and McGavern, D. B. (2014). Microglia development and function. Annu. Rev. Immunol. 32, 367–402. doi: 10.1146/annurev-immunol-032713-120240
Nicholson, J. K., Holmes, E., Kinross, J., Burcelin, R., Gibson, G., Jia, W., et al. (2012). Host-gut microbiota metabolic interactions. Science 336, 1262–1267. doi: 10.1126/science.1223813
Niday, Z., and Tzingounis, A. V. (2018). Potassium channel gain of function in epilepsy: an unresolved paradox. Neuroscientist 24, 368–380. doi: 10.1177/1073858418763752
Nzou, G., Wicks, R. T., VanOstrand, N. R., Mekky, G. A., Seale, S. A., El-Taibany, A., et al. (2020). Multicellular 3D neurovascular unit model for assessing hypoxia and neuroinflammation induced blood-brain barrier dysfunction. Sci. Rep. 10:9766. doi: 10.1038/s41598-020-66487-8
Ohtsuki, S., and Terasaki, T. (2007). Contribution of carrier-mediated transport systems to the blood-brain barrier as a supporting and protecting interface for the brain; importance for CNS drug discovery and development. Pharm. Res. 24, 1745–1758. doi: 10.1007/s11095-007-9374-5
Pachter, J. S., de Vries, H. E., and Fabry, Z. (2003). The blood-brain barrier and its role in immune privilege in the central nervous system. J. Neuropathol. Exp. Neurol. 62, 593–604. doi: 10.1093/jnen/62.6.593
Parker, A., Fonseca, S., and Carding, S. R. (2020). Gut microbes and metabolites as modulators of blood-brain barrier integrity and brain health. Gut Microbes 11, 135–157. doi: 10.1080/19490976.2019.1638722
Paudel, Y. N., Shaikh, M. F., Chakraborti, A., Kumari, Y., Aledo-Serrano, Á, Aleksovska, K., et al. (2018). HMGB1: a common biomarker and potential target for TBI, neuroinflammation, epilepsy, and cognitive dysfunction. Front. Neurosci. 12:628. doi: 10.3389/fnins.2018.00628
Peirce, J. M., and Alvi na, K. (2019). The role of inflammation and the gut microbiome in depression and anxiety. J. Neurosci. Res. 97, 1223–1241. doi: 10.1002/jnr.24476
Peng, A., Qiu, X., Lai, W., Li, W., Zhang, L., Zhu, X., et al. (2018). Altered composition of the gut microbiome in patients with drug-resistant epilepsy. Epilepsy Res. 147, 102–107. doi: 10.1016/j.eplepsyres.2018.09.013
Pitkänen, A., Lukasiuk, K., Dudek, F. E., and Staley, K. J. (2015). Epileptogenesis. Cold Spring Harb. Perspect. Med. 5:a022822. doi: 10.1101/cshperspect.a022822
Qin, J., Li, R., Raes, J., Arumugam, M., Burgdorf, K. S., Manichanh, C., et al. (2010). A human gut microbial gene catalogue established by metagenomic sequencing. Nature 464, 59–65. doi: 10.1038/nature08821
Qin, L. H., Huang, W., Mo, X. A., Chen, Y. L., and Wu, X. H. (2015). LPS Induces occludin dysregulation in cerebral microvascular endothelial cells via MAPK signaling and augmenting MMP-2 levels. Oxid. Med. Cell. Longev. 2015:120641. doi: 10.1155/2015/120641
Qin, W., Li, J., Zhu, R., Gao, S., Fan, J., Xia, M., et al. (2019). Melatonin protects blood-brain barrier integrity and permeability by inhibiting matrix metalloproteinase-9 via the NOTCH3/NF-κB pathway. Aging (Albany NY) 11, 11391–11415. doi: 10.18632/aging.102537
Rawat, C., Kukal, S., Dahiya, U. R., and Kukreti, R. (2019). Cyclooxygenase-2 (COX-2) inhibitors: future therapeutic strategies for epilepsy management. J. Neuroinflammation 16:197. doi: 10.1186/s12974-019-1592-3
Riazi, K., Galic, M. A., and Pittman, Q. J. (2010). Contributions of peripheral inflammation to seizure susceptibility: cytokines and brain excitability. Epilepsy Res. 89, 34–42. doi: 10.1016/j.eplepsyres.2009.09.004
Riesenfeld, C. S., Schloss, P. D., and Handelsman, J. (2004). Metagenomics: genomic analysis of microbial communities. Annu. Rev. Genet. 38, 525–552. doi: 10.1146/annurev.genet.38.072902.091216
Rocha, E. M., De Miranda, B., and Sanders, L. H. (2018). Alpha-synuclein: pathology, mitochondrial dysfunction and neuroinflammation in Parkinson’s disease. Neurobiol. Dis. 109, 249–257. doi: 10.1016/j.nbd.2017.04.004
Rojas, A., Jiang, J., Ganesh, T., Yang, M. S., Lelutiu, N., Gueorguieva, P., et al. (2014). Cyclooxygenase-2 in epilepsy. Epilepsia 55, 17–25. doi: 10.1111/epi.12461
Ronaldson, P. T., and Davis, T. P. (2020). Regulation of blood-brain barrier integrity by microglia in health and disease: a therapeutic opportunity. J. Cereb. Blood Flow Metab. 40, S6–S24. doi: 10.1177/0271678X20951995
Rose, C. F., Verkhratsky, A., and Parpura, V. (2013). Astrocyte glutamine synthetase: pivotal in health and disease. Biochem. Soc. Trans. 41, 1518–1524. doi: 10.1042/BST20130237
Sada, N., Lee, S., Katsu, T., Otsuki, T., and Inoue, T. (2015). Epilepsy treatment. Targeting LDH enzymes with a stiripentol analog to treat epilepsy. Science 347, 1362–1367. doi: 10.1126/science.aaa1299
Sandhu, M., Gruenbaum, B. F., Gruenbaum, S. E., Dhaher, R., Deshpande, K., Funaro, M. C., et al. (2021). Astroglial glutamine synthetase and the pathogenesis of mesial temporal lobe epilepsy. Front. Neurol. 12:665334. doi: 10.3389/fneur.2021.665334
Saurman, V., Margolis, K. G., and Luna, R. A. (2020). Autism spectrum disorder as a brain-gut-microbiome axis disorder. Dig. Dis. Sci. 65, 818–828. doi: 10.1007/s10620-020-06133-5
Seifert, G., and Steinhäuser, C. (2013). Neuron-astrocyte signaling and epilepsy. Exp. Neurol. 244, 4–10. doi: 10.1016/j.expneurol.2011.08.024
Seifert, G., Carmignoto, G., and Steinhäuser, C. (2010). Astrocyte dysfunction in epilepsy. Brain Res. Rev. 63, 212–221. doi: 10.1016/j.brainresrev.2009.10.004
Seifert, G., Henneberger, C., and Steinhäuser, C. (2018). Diversity of astrocyte potassium channels: an update. Brain Res. Bull. 136, 26–36. doi: 10.1016/j.brainresbull.2016.12.002
Sharif, Y., Jumah, F., Coplan, L., Krosser, A., Sharif, K., and Tubbs, R. S. (2018). Blood brain barrier: a review of its anatomy and physiology in health and disease. Clin. Anat. 31, 812–823. doi: 10.1002/ca.23083
Sharma, R., Leung, W. L., Zamani, A., O’Brien, T. J., Casillas Espinosa, P. M., and Semple, B. D. (2019). Neuroinflammation in post-traumatic epilepsy: pathophysiology and tractable therapeutic targets. Brain Sci. 9:318. doi: 10.3390/brainsci9110318
Sharon, G., Sampson, T. R., Geschwind, D. H., and Mazmanian, S. K. (2016). The central nervous system and the gut microbiome. Cell 167, 915–932. doi: 10.1016/j.cell.2016.10.027
Snowball, A., Chabrol, E., Wykes, R. C., Shekh-Ahmad, T., Cornford, J. H., Lieb, A., et al. (2019). Epilepsy gene therapy using an engineered potassium channel. J. Neurosci. 39, 3159–3169. doi: 10.1523/JNEUROSCI.1143-18.2019
Sochocka, M., Donskow-Łysoniewska, K., Diniz, B. S., Kurpas, D., Brzozowska, E., and Leszek, J. (2019). the gut microbiome alterations and inflammation-driven pathogenesis of Alzheimer’s disease-a critical review. Mol. Neurobiol. 56, 1841–1851. doi: 10.1007/s12035-018-1188-4
Soulet, D., and Rivest, S. (2008). Microglia. Curr. Biol. 18, R506–R508. doi: 10.1016/j.cub.2008.04.047
Sudo, N., Chida, Y., Aiba, Y., Sonoda, J., Oyama, N., Yu, X. N., et al. (2004). Postnatal microbial colonization programs the hypothalamic-pituitary-adrenal system for stress response in mice. J. Physiol. 558, 263–275. doi: 10.1113/jphysiol.2004.063388
Sweeney, M. D., Zhao, Z., Montagne, A., Nelson, A. R., and Zlokovic, B. V. (2019). Blood-brain barrier: from physiology to disease and back. Physiol. Rev. 99, 21–78. doi: 10.1152/physrev.00050.2017
Takeuchi, O., and Akira, S. (2010). Pattern recognition receptors and inflammation. Cell 140, 805–820. doi: 10.1016/j.cell.2010.01.022
Takiishi, T., Fenero, C., and Câmara, N. (2017). Intestinal barrier and gut microbiota: shaping our immune responses throughout life. Tissue Barriers 5:e1373208. doi: 10.1080/21688370.2017.1373208
Tanaka, T., Narazaki, M., Masuda, K., and Kishimoto, T. (2016). Regulation of IL-6 in Immunity and Diseases. Adv. Exp. Med. Biol. 941, 79–88. doi: 10.1007/978-94-024-0921-5_4
Tremlett, H., Bauer, K. C., Appel-Cresswell, S., Finlay, B. B., and Waubant, E. (2017). The gut microbiome in human neurological disease: a review. Ann. Neurol. 81, 369–382. doi: 10.1002/ana.24901
Turnbaugh, P. J., Ley, R. E., Mahowald, M. A., Magrini, V., Mardis, E. R., and Gordon, J. I. (2006). An obesity-associated gut microbiome with increased capacity for energy harvest. Nature 444, 1027–1031. doi: 10.1038/nature05414
Ułamek-Kozioł, M., Czuczwar, S. J., Januszewski, S., and Pluta, R. (2019). Ketogenic diet and epilepsy. Nutrients 11, 2510. doi: 10.3390/nu11102510
van Vliet, E. A., Aronica, E., and Gorter, J. A. (2015). Blood-brain barrier dysfunction, seizures and epilepsy. Semin. Cell Dev. Biol. 38, 26–34. doi: 10.1016/j.semcdb.2014.10.003
Vander Ark, A., Cao, J., and Li, X. (2018). TGF-β receptors: in and beyond TGF-β signaling. Cell Signal. 52, 112–120. doi: 10.1016/j.cellsig.2018.09.002
Varatharaj, A., and Galea, I. (2017). The blood-brain barrier in systemic inflammation. Brain Behav. Immun. 60, 1–12. doi: 10.1016/j.bbi.2016.03.010
Vezzani, A., Balosso, S., and Ravizza, T. (2008). The role of cytokines in the pathophysiology of epilepsy. Brain Behav. Immun. 22, 797–803. doi: 10.1016/j.bbi.2008.03.009
Vezzani, A., French, J., Bartfai, T., and Baram, T. Z. (2011). The role of inflammation in epilepsy. Nat. Rev. Neurol. 7, 31–40. doi: 10.1038/nrneurol.2010.178
Vezzani, A., Friedman, A., and Dingledine, R. J. (2013). The role of inflammation in epileptogenesis. Neuropharmacology 69, 16–24. doi: 10.1016/j.neuropharm.2012.04.004
Vezzani, A., Fujinami, R. S., White, H. S., Preux, P. M., Blümcke, I., Sander, J. W., et al. (2016). Infections, inflammation and epilepsy. Acta Neuropathol. 131, 211–234. doi: 10.1007/s00401-015-1481-5
Wang, H. X., and Wang, Y. P. (2016). Gut microbiota-brain axis. Chin. Med. J. (Engl.) 129, 2373–2380. doi: 10.4103/0366-6999.190667
Weissberg, I., Wood, L., Kamintsky, L., Vazquez, O., Milikovsky, D. Z., Alexander, A., et al. (2015). Albumin induces excitatory synaptogenesis through astrocytic TGF-β/ALK5 signaling in a model of acquired epilepsy following blood-brain barrier dysfunction. Neurobiol. Dis. 78, 115–125. doi: 10.1016/j.nbd.2015.02.029
Wells, J., Swaminathan, A., Paseka, J., and Hanson, C. (2020). Efficacy and safety of a ketogenic diet in children and adolescents with refractory epilepsy-a review. Nutrients 12:1809. doi: 10.3390/nu12061809
Wikoff, W. R., Anfora, A. T., Liu, J., Schultz, P. G., Lesley, S. A., Peters, E. C., et al. (2009). Metabolomics analysis reveals large effects of gut microflora on mammalian blood metabolites. Proc. Natl. Acad. Sci. U.S.A. 106, 3698–3703. doi: 10.1073/pnas.0812874106
Wlodarska, M., Kostic, A. D., and Xavier, R. J. (2015). An integrative view of microbiome-host interactions in inflammatory bowel diseases. Cell Host Microbe 17, 577–591. doi: 10.1016/j.chom.2015.04.008
Xie, G., Zhou, Q., Qiu, C. Z., Dai, W. K., Wang, H. P., Li, Y. H., et al. (2017). Ketogenic diet poses a significant effect on imbalanced gut microbiota in infants with refractory epilepsy. World J. Gastroenterol. 23, 6164–6171. doi: 10.3748/wjg.v23.i33.6164
Yang, H., Wang, H., and Andersson, U. (2020). Targeting inflammation driven by HMGB1. Front. Immunol. 11:484. doi: 10.3389/fimmu.2020.00484
Yazdi, A. S., and Ghoreschi, K. (2016). The Interleukin-1 family. Adv. Exp. Med. Biol. 941, 21–29. doi: 10.1007/978-94-024-0921-5_2
Yu, H. Y., Cai, Y. B., and Liu, Z. (2015). Activation of AMPK improves lipopolysaccharide-induced dysfunction of the blood-brain barrier in mice. Brain Inj. 29, 777–784. doi: 10.3109/02699052.2015.1004746
Yuan, S., Liu, K. J., and Qi, Z. (2020). Occludin regulation of blood-brain barrier and potential therapeutic target in ischemic stroke. Brain Circ. 6, 152–162. doi: 10.4103/bc.bc_29_20
Zhang, Y., Zhou, S., Zhou, Y., Yu, L., Zhang, L., and Wang, Y. (2018). Altered gut microbiome composition in children with refractory epilepsy after ketogenic diet. Epilepsy Res. 145, 163–168. doi: 10.1016/j.eplepsyres.2018.06.015
Zhao, J., Wang, Y., Xu, C., Liu, K., Wang, Y., Chen, L., et al. (2017). Therapeutic potential of an anti-high mobility group box-1 monoclonal antibody in epilepsy. Brain Behav. Immun. 64, 308–319. doi: 10.1016/j.bbi.2017.02.002
Zheng, P., Zeng, B., Zhou, C., Liu, M., Fang, Z., Xu, X., et al. (2016). Gut microbiome remodeling induces depressive-like behaviors through a pathway mediated by the host’s metabolism. Mol. Psychiatry. 21, 786–796. doi: 10.1038/mp.2016.44
Zhu, X., Yao, Y., Yang, J., Zhengxie, J., Li, X., Hu, S., et al. (2020). COX-2-PGE(2) signaling pathway contributes to hippocampal neuronal injury and cognitive impairment in PTZ-kindled epilepsy mice. Int. Immunopharmacol. 87:106801. doi: 10.1016/j.intimp.2020.106801
Zlokovic, B. V. (2008). The blood-brain barrier in health and chronic neurodegenerative disorders. Neuron 57, 178–201. doi: 10.1016/j.neuron.2008.01.003
Zlokovic, B. V. (2011). Neurovascular pathways to neurodegeneration in Alzheimer’s disease and other disorders. Nat. Rev. Neurosci. 12, 723–738. doi: 10.1038/nrn3114
Keywords: epilepsy, innate immunity, central nervous system, gut–brain axis, microorganisms
Citation: Zhang L, Li S, Tai Z, Yu C and Xu Z (2022) Gut Microbes Regulate Innate Immunity and Epilepsy. Front. Neurosci. 16:870197. doi: 10.3389/fnins.2022.870197
Received: 06 February 2022; Accepted: 13 April 2022;
Published: 01 June 2022.
Edited by:
Shaohua Hu, Zhejiang University, ChinaReviewed by:
Zsolt Kovacs, Eötvös Loránd University, HungaryChunfu Zheng, University of Calgary, Canada
Copyright © 2022 Zhang, Li, Tai, Yu and Xu. This is an open-access article distributed under the terms of the Creative Commons Attribution License (CC BY). The use, distribution or reproduction in other forums is permitted, provided the original author(s) and the copyright owner(s) are credited and that the original publication in this journal is cited, in accordance with accepted academic practice. No use, distribution or reproduction is permitted which does not comply with these terms.
*Correspondence: Changyin Yu, eXVjaGFuZ3lpbjY4QDE2My5jb20=; Zucai Xu, ZG9jeHpjQDEyNi5jb20=