- 1Vagal Afferent Research Group, Adelaide Medical School, The University of Adelaide, Adelaide, SA, Australia
- 2Nutrition, Diabetes and Gut Health, Lifelong Health Theme, South Australian Health and Medical Research Institute, Adelaide, SA, Australia
The vagus nerve is crucial in the bidirectional communication between the gut and the brain. It is involved in the modulation of a variety of gut and brain functions. Human studies indicate that the descending vagal signaling from the brain is impaired in functional dyspepsia. Growing evidence indicate that the vagal signaling from gut to brain may also be altered, due to the alteration of a variety of gut signals identified in this disorder. The pathophysiological roles of vagal signaling in functional dyspepsia is still largely unknown, although some studies suggested it may contribute to reduced food intake and gastric motility, increased psychological disorders and pain sensation, nausea and vomiting. Understanding the alteration in vagal signaling and its pathophysiological roles in functional dyspepsia may provide information for new potential therapeutic treatments of this disorder. In this review, we summarize and speculate possible alterations in vagal gut-to-brain and brain-to-gut signaling and the potential pathophysiological roles in functional dyspepsia.
Introduction
Functional dyspepsia is a common upper gastrointestinal disorder affecting approximately 20% of the population. It is classified by Rome IV into three subtypes, PDS (accounting to 38% of functional dyspepsia patients), characterize by bothersome postprandial fullness and early satiation; EPS (27% of patients), characterized by gastric pain or burning; and a mixture of both (35% of patients) (Talley and Ford, 2015). Functional dyspepsia is a disorder of the gut–brain axis, with heterogeneous pathophysiological changes observed in both the upper gastrointestinal tract and the brain. The changes include gastroduodenal hypersensitivity (observed in 34–66% of patients), delayed gastric emptying (20–50% of patients), abnormal gastric accommodation (∼40% of patients), increased gastroduodenal inflammation and permeability (up to 40% of patients), and abnormal brain functions including abnormal psychological processes (Vanheel and Farre, 2013; Ford et al., 2020; Wauters et al., 2020b). The etiology of functional dyspepsia may arise either from brain to gut pathways, with psychological disorders occurring before gastric disorders, or from gut to brain pathways, with gastric disorders preceding the onset of psychological disorders (Talley, 2020). This bidirectional etiology suggests the gut–brain communication plays a critical role in the development of functional dyspepsia.
The vagus nerve is an important signaling pathway enabling communication between the gut and brain. The ascending vagal nerves (i.e., vagal afferents) sense local mechanical and chemical signals within the gut and send these signals to the brain. This is crucial for several principal brain functions, including the regulation of food intake and mood. The descending vagal nerves (i.e., vagal efferents) transfer brain signals to the gut, including brain signals triggered by stress or ascending gut signals (e.g., vagovagal reflex). These descending vagal efferent signals modulate gut functions including gastrointestinal sensation, gut motility, hormone secretion, acid secretion, and inflammation. Therefore, any alterations in vagal ascending signaling can have an impact on brain function and, further, on gut function, and any alterations in vagal descending signaling can have an impact on gut function and subsequently on brain function.
It is likely the vagus nerve is involved in the development of functional dyspepsia, given that the physiological functions of the vagus nerve largely overlap with the pathophysiological changes observed in functional dyspepsia. In addition, the bidirectional vagal signaling pathway is in accordance with the bidirectional etiological features of functional dyspepsia. Abundant evidence indicates a subset (∼30%) of functional dyspepsia patients have impaired vagal efferent activity (Hausken et al., 1993; Hveem et al., 1998; Lorena et al., 2002; Dal et al., 2014; Guo et al., 2018). In addition, recent findings, using animal models of functional dyspepsia, suggest vagal afferent signaling in response to gastric distension may be increased in functional dyspepsia (Li et al., 2019; Cordner et al., 2021). Furthermore, vagal nerve stimulation has been shown to reverse some of the pathophysiological changes (Hou et al., 2020; Zhu et al., 2021). This evidence suggests the vagus nerve could be a pathological component and a therapeutic target for functional dyspepsia, however, the role of vagal nerves are still largely unknown.
There is increasing knowledge of altered gut and brain signals in functional dyspepsia, providing potential implications for altered vagal signaling in the pathophysiology of this disorder. In this review, we summarize and speculate possible alterations in vagal gut-to-brain and brain-to-gut signaling and the potential pathophysiological implications in functional dyspepsia. We will particularly focus on the vagal innervation of the stomach and duodenum, the major gut regions associated with functional dyspepsia. It should be noted that there are parallel pathways in the communication between the gut and brain, e.g., via the blood circulation or sympathetic pathways or indirectly via vagal innervation of other organs, which should be recognized although beyond the scope of this review.
Neuroanatomy of Vagal Neurocircuitry
The vagal neurocircuitry provides the structural foundations for the vagal mediated gut–brain communication. It is composed of vagal afferents, vagal efferents and the dorsal vagal complex in the brainstem, where vagal associated signals are integrated and coordinated centrally (Berthoud and Neuhuber, 2000).
Vagal Afferent Nerves
The vagal afferent nerves constitute approximately 80-90% of the fibers in the vagus (Foley and DuBois, 1937; Asala and Bower, 1986). They have cell bodies located in the nodose ganglia and jugular ganglia, with nerve endings projecting both centrally and peripherally. In terms of vagal innervation of the gastrointestinal tract, there is a high density of peripheral endings in the gastroduodenal region, with a gradual reduction in density further down the gastrointestinal tract (Wang and Powley, 2000). Vagal afferent gastroduodenal terminals can be functionally classified into mechanosensitive and chemosensitive afferents. Mechanosensitive vagal afferents sense mechanical stimuli including food related mucosal stroking, distension and contraction. It includes mechanosensitive mucosal endings, IGLEs located in the myenteric plexus between the muscular layers, and IMAs, distributed within the muscular layers (Clarke and Davison, 1978; Zagorodnyuk et al., 2001). Chemosensitive vagal afferents have peripheral endings which sense chemical stimuli including nutrients, hormones, pH, and immune stimuli (pathogen, food allergies, and microbiota). The central endings of gastroduodenal vagal afferents terminate in the dorsal vagal complex in the brainstem, mostly in the NTS, but also in the area postrema, the DMV and the trigeminal island (Kalia and Sullivan, 1982).
Dorsal Vagal Complex
The dorsal vagal complex consists of the NTS, DMV, area postrema, and the trigeminal island. It receives ascending gastroduodenal vagal afferent input. The neurons in the NTS have ascending axons projecting to a large number of brain areas, including brain areas involved in food intake regulation and mood, such as the hypothalamus and amygdala (Ricardo and Koh, 1978; Rinaman, 2007, 2010). The NTS neurons also have nerve fibers projecting directly or indirectly to vagal efferent neurons in the DMV, constituting a pathway for reflex feedback to the gastrointestinal tract, via the vagal efferent pathway, to modulate gut functions. In addition, the dorsal vagal complex receives descending projections from higher brain regions, including the cortex, hypothalamus and amygdala (van der Kooy et al., 1984). The dorsal vagal complex can also be readily modulated by circulating factors, including hormones and inflammatory factors (Blevins et al., 2000), as it lies outside the blood–brain barrier.
Vagal Efferent Nerves
The vagal efferent nerves account for approximately 10–20% of vagal fibers (Foley and DuBois, 1937; Asala and Bower, 1986). The cell bodies of vagal efferent nerves innervating the stomach and duodenum are located within the DMV, descending mainly via the gastric and hepatic branches of the vagus nerve (Berthoud et al., 1991). Their axons project to gastroduodenal myenteric neurons in the myenteric plexus of the enteric nerve system (Berthoud et al., 1991). Vagal efferent mediated activation of myenteric neurons leads to the regulation of gastrointestinal functions, such as motility, secretion, and immune responses (Chang et al., 2003).
Vagal Afferent Sensation of Gastroduodenal Mechanical Stimuli in Functional Dyspepsia
There is a recent review on the neural signaling of gut mechanosensation in ingestive and digestive process which provides a comprehensive review of gut mechanosensation (Kim et al., 2022). In the gastroduodenal region, mechanosensitive vagal afferents sense gastroduodenal distension (Zagorodnyuk and Brookes, 2000) and generate signals in linear relation to the levels of distension, i.e., the degree of filling of the gastroduodenal lumen (McCann and Rogers, 1992). Besides distension, muscle contraction can also activate tension sensitive vagal afferents (Iggo, 1955, 1957), and contraction can further enhance the response of vagal afferents to distension (Iggo, 1955). Evidence indicates the distension and contraction signals may be sensed by IGLE terminals in the gastroduodenal region. More specifically, studies indicate distinct populations of glucagon-like peptide 1 receptor (GLP-1R) expressing IGLEs in the stomach and oxytocin receptor (Oxtr) expressing IGLEs in the duodenum are the major tension sensitive vagal afferents (Williams et al., 2016; Bai et al., 2019). These signals are conveyed to the brain to regulate food intake, mood and indirectly pain sensation, or transferred via the vagal reflex pathway to modulate gastric motility and secretion. In functional dyspepsia, gastroduodenal mechanical stimuli are altered (Troncon et al., 1994) and the sensitivity of vagal afferents to mechanical stimuli is increased (Li et al., 2019; Cordner et al., 2021), which likely leads to altered vagal afferent signaling and corresponding gut and brain malfunctions.
Altered Gastroduodenal Mechanical Stimuli in Functional Dyspepsia
Evidence indicates food induced gastroduodenal distension may be altered in functional dyspepsia. A subset of functional dyspepsia patients have abnormal intragastric distribution of food, with reduced food accommodation in the proximal stomach, increased antral area (Hausken and Berstad, 1992; Pilichiewicz et al., 2008) and food retention in the antrum (Troncon et al., 1994; Urbain et al., 1995). Therefore, in these patients, the level of food induced distension may be reduced in the proximal stomach and enhanced in the gastric antrum, hence reducing or increasing the corresponding ascending distension sensitive vagal afferent signals. Furthermore, functional dyspepsia is associated with an increase in gastric antral contractions (antral motor activity indices) after food intake (Urbain et al., 1995), ultimately leading to increased activation of mechanosensitive vagal afferents.
Increased Vagal Afferent Sensitivity to Gastric Distension in Functional Dyspepsia
Recent animal studies indicate the sensitivity of gastric vagal afferents to gastric distension is increased in functional dyspepsia (Li et al., 2019; Cordner et al., 2021), consistent with the increased sensitivity to gastric distension in functional dyspepsia patients (Coffin et al., 1994; Barbera et al., 1995a; Caldarella et al., 2003). It should be noted that this vagal afferent hypersensitivity has been consistently observed in two different animal models of functional dyspepsia, namely an unpredictable chronic mild stress mouse model (Li et al., 2019), which has a central origin, and a gastric irritation (0.1% iodoacetamide) induced rat model, which has a peripheral origin within the gut (Cordner et al., 2021). This suggests vagal afferent hypersensitivity to gastric distension may be a crucial pathological component of functional dyspepsia with both etiologic origins. Human studies have also shown that functional dyspepsia patients have increased perception to balloon-induced duodenal distension (Holtmann et al., 1996). Whether this involves hypersensitivity of duodenal vagal afferents to duodenal distension requires further investigation.
The sensitivity of mechanosensitive vagal afferents can be modulated by a variety of circulating or locally released mediators, including hormones, neurotransmitters, inflammatory factors, endotoxins and pH. Therefore, altered levels of these mediators or altered modulation by these mediators may lead to vagal afferent hypersensitivity. For example, the gut hormone CCK, released from the duodenum, has been shown to increase the sensitivity of gastric and duodenal vagal afferents to distension (Davison and Clarke, 1988; Blackshaw and Grundy, 1990; Schwartz et al., 1995). In functional dyspepsia, diet induced CCK release is increased (Pilichiewicz et al., 2008), and the CCK-A receptor antagonist dexloxiglumide reduced gastric distension-induced symptoms in functional dyspepsia patients (Feinle et al., 2001). Therefore, it is possible that vagal afferent distension signals are enhanced due to the increase in CCK levels, leading to functional dyspepsia symptoms. Further, in functional dyspepsia, specifically patients with PDS, circulating acylated ghrelin levels are reduced (Shindo et al., 2009; Choi et al., 2016). Ghrelin is known to inhibit vagal afferent responses to gastric distension (Page et al., 2007) and, therefore, a reduction in ghrelin levels is likely to lead to an increase in sensitivity of gastric vagal afferents. As these afferents play an important role in satiety signaling (Date et al., 2002), it is conceivable that this pathway could contribute to the symptoms of early satiety and/or bloating observed in PDS, however, there is a lack of direct evidence. Furthermore, chemical stimuli such as hydrochloric acid also increase the response of gastric vagal afferents to distension (Kang et al., 2004). In functional dyspepsia although the rate of gastric acid secretion was within the normal range (Collen and Loebenberg, 1989), duodenal acid exposure was increased in a subgroup (64%) of patients (Lee et al., 2004), likely as a consequence of the reduced duodenal acid clearance rate (Samsom et al., 1999). This increase in luminal acid exposure may contribute to increased vagal afferent mechanosensitivity in a subgroup of functional dyspepsia patients.
Evidence indicates inflammation, specifically mast cell infiltration, may play a role in vagal afferent hypersensitivity. Mast cell infiltration has been observed in the stomach and duodenum of functional dyspepsia patients (Du et al., 2018) and can be triggered by both stress and gastric irritation in animal models (Cordner et al., 2021). In a gastric irritation-induced rat model of functional dyspepsia, the mast cell stabilizer ketotifen reduced vagal afferent hypersensitivity as well as the associated pain response and psychological disorders (Cordner et al., 2021). A variety of molecular mediators are released by mast cells in response to environmental changes, including reactive oxygen species, histamine, serotonin, protease, and cytokines (Traina, 2019), however, their effects on mechanosensitive vagal afferent are unknown and require investigation. In addition, whether other immune cells, such as eosinophils, are also involved in this vagal afferent hypersensitivity is not known. Identification of the molecular factors contributing to vagal afferent hypersensitivity to food related stimuli (e.g., stretch) may provide novel new therapeutic targets to improve the symptoms associated with functional dyspepsia.
Pathophysiological Roles of Enhanced Vagal Signaling in Response to Distension in Functional Dyspepsia
Increased vagal signaling in response to distension may be associated with multiple pathophysiological changes and symptoms in functional dyspepsia, including altered food intake, mood, pain perception, gastric accommodation and emptying and duodenal motility, which are summarized in Figure 1.
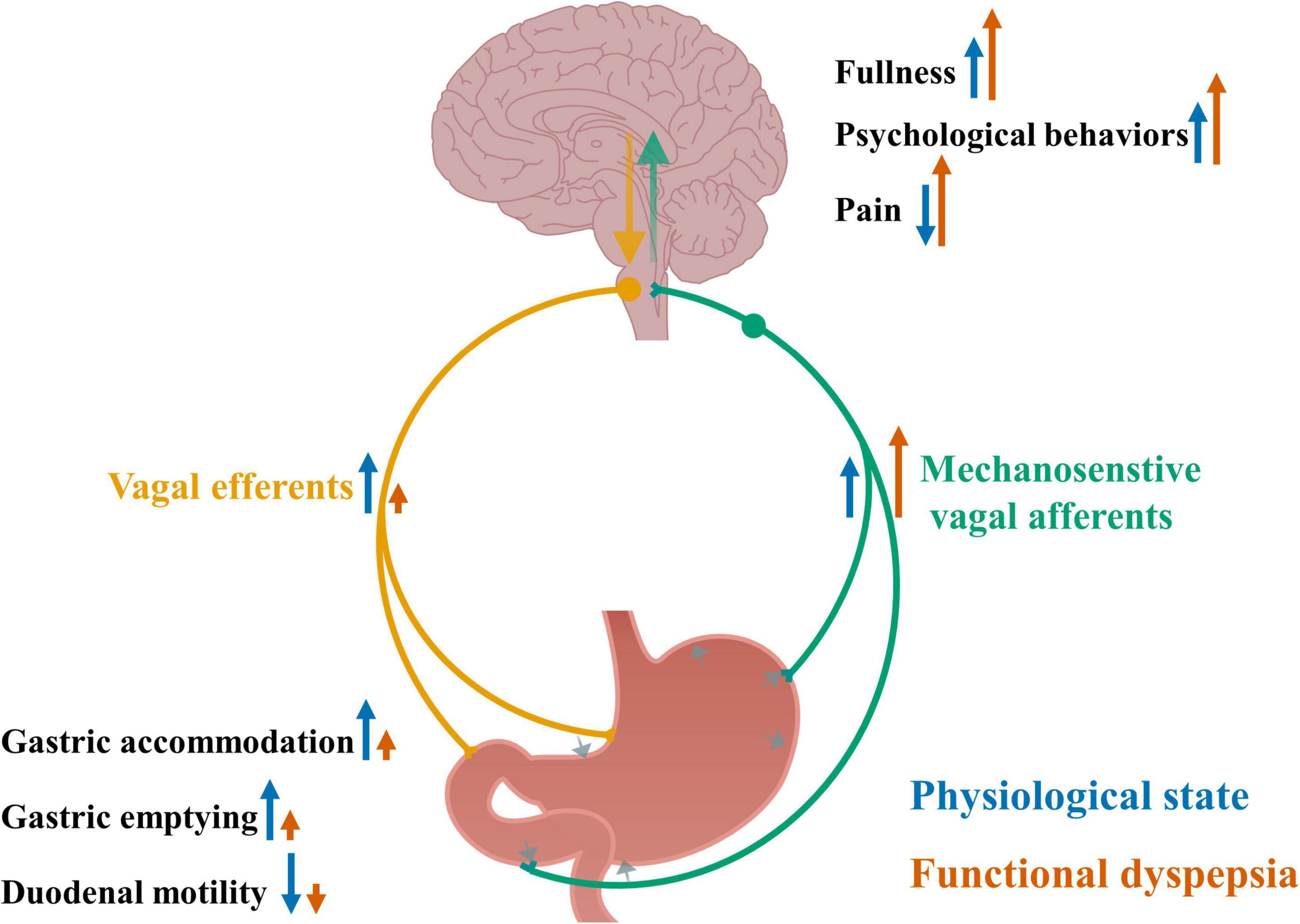
Figure 1. Altered vagal signaling in response to gastric distension and its associated pathophysiological changes in functional dyspepsia. Gastric distension and contraction activate mechanosensitive vagal afferents and send signals to the brain, leading to increased feeling of fullness, increased psychological behaviors and reduced pain sensation, and further via vagal efferents send signals to the gut, leading to increased gastric accommodation and emptying, and reduced duodenal motility. In functional dyspepsia, the sensitivity of vagal afferents to gastric distension is increased, accompanying increased feeling of fullness, psychological behaviors and pain, and impaired gastric accommodation and emptying, and duodenal motility. Blue arrows indicate an effect to increase or decrease nerve activity or physiological outcomes under normal physiological conditions. Orange arrows indicate an effect to increase or decrease nerve activity or physiological outcomes in functional dyspepsia and the size of the arrows indicate the levels of the effects.
In the Modulation of Food Intake
Detection of gastric distension by gastric vagal afferents is a well-appreciated physiological mechanism for the initiation of postprandial satiation and termination of food intake. The level of antral distension is positively correlated with postprandial fullness in healthy subjects (Jones et al., 1997). A recent study found that duodenal vagal afferent responses to duodenal distension also contribute to the postprandial inhibition of food intake, providing a more sustained inhibition of food intake compared to gastric afferents (Bai et al., 2019). The inhibition of food intake in response to vagal afferent distension signals may involve multiple regions in the hypothalamus, the central center for appetite regulation. For example, activation of gastric and duodenal tension sensitive vagal afferents led to inhibition of AgRP neurons in the hypothalamus and reduced food intake in mice (Bai et al., 2019). Furthermore, vagal afferent responses to gastric distension activate oxytocin secreting neurons in the PVN to release oxytocin in rats (Ueta et al., 1991), which is known to inhibit food intake via multiple central pathways (Onaka and Takayanagi, 2019).
Functional dyspepsia, specifically PDS, is characterized by bothersome postprandial fullness and early satiety. In functional dyspepsia patients, delayed gastric emptying, resulting in increased gastric distension, was found to be associated with symptom severity of early satiety and postprandial fullness (Stanghellini et al., 1996; Cuomo et al., 2001). In addition, impaired gastric accommodation in functional dyspepsia, likely increasing the response of mechanosensitive vagal afferents to the same amount of food, was also associated with early satiety and weight loss in functional dyspepsia, with normalization of gastric accommodation improving meal-induced early satiety (Tack et al., 1998). Therefore, it is possible that mechanosensitive vagal afferents are involved in the impaired gastric emptying and impaired gastric accommodation induced postprandial fullness and early satiety in functional dyspepsia. Furthermore, there is also direct evidence to indicate vagal afferents are hypersensitive to gastric distension, in an unpredictable chronic mild stress mouse model of functional dyspepsia, which is accompanied by reduced food intake and body weight (Li et al., 2019). This further supports the possibility that vagal afferent hypersensitivity may lead to reduced food intake. Therefore, there are likely a number of additive factors that contribute to the hypersensitivity observed in functional dyspepsia, with any combination of these factors leading to similar symptoms, reinforcing the idea of personalized treatment. The factors contributing to hypersensitivity of the gut–brain axis requires further investigation before we can consider this type of targeted therapy. Despite the importance of distension sensitive duodenal vagal afferent in initiating satiation, the role of duodenal afferents in functional dyspepsia has not been investigated.
In the Modulation of Mood
Evidence indicates vagal sensation of gut signals is involved in the modulation of mood and emotion. In healthy rats, complete and selective subdiaphragmatic vagal deafferentation, which block vagal sensation of abdominal signals including gut signals, reduced innate anxiety-like behavior and learned fear (Klarer et al., 2014). This is associated with altered neurotransmitter levels, i.e., increased GABA and decreased noradrenaline in specific regions of the limbic area, the critical area regulating both innate anxiety and conditioned fear (Klarer et al., 2014). Furthermore, optical activation of the gut-innervating vagal sensory neurons in the right ganglion of mice stimulated reward behaviors and induced dopamine release from the substantia nigra (Han et al., 2018). This suggests vagal sensation of gut signals may play a role in the modulation of emotional states.
Functional dyspepsia is highly associated with psychological disorders, including anxiety and depression. In some patients, the psychological disorders occur after the gastric symptoms, suggesting altered gut signals may contribute to the development of these psychological disorders (Talley, 2020). In gastric irritation-induced functional dyspepsia rats, vagal afferent hypersensitivity to gastric distension accompanied increased anxiety- and depressive-like behaviors (Cordner et al., 2021). Furthermore, subdiaphragmatic vagotomy or reversing vagal afferent hypersensitivity, using the mast cell stabilizer ketotifen, normalized these psychological behaviors (Cordner et al., 2021). This suggests the vagal afferent hypersensitivity to gastric distension may lead to psychological disorders. Consistent with this, rat vagal afferent deafferentation reduced innate anxiety-like behavior and learned fear in physiological conditions (Klarer et al., 2014). Furthermore, in a rat model of functional dyspepsia vagal afferent hypersensitivity was accompanied by an increase and decrease in gene expression of CRF and BDNF respectively in the amygdala, with the altered expression partially normalized by subdiaphragmatic vagotomy (Cordner et al., 2021). Since increased CRF and decreased BDNF have been observed in increased anxiety- and depressive-like behavior (Liu et al., 2011; Sagarkar et al., 2017), the altered gene expression may contribute to the central mechanisms responsible for vagal afferent induced psychological disorders in functional dyspepsia.
In the Modulation of Pain Perception
There is growing evidence to indicate vagal afferent signaling may indirectly modulate pain sensation. For example, activation of gastric vagal afferents through non-painful gastric distension reduced somatic pain perception in healthy human (Sedan et al., 2005). In addition, vagal nerve stimulation has been shown to relieve pain in both human and animal studies, as summarized in a review paper by Chakravarthy et al. (2015), possibly via a mechanism involving activation of vagal afferents. The anti-nociceptive effects of vagal nerve stimulation may be via different pathways, including inhibition of nociceptive neurons in the spinal cord (Ammons et al., 1983; Chandler et al., 1991), activation of the vagal anti-inflammatory pathway which inhibits inflammation associated pain (Hosoi et al., 2000; Khasar et al., 2003), modulation of attention and mood pathways which interact with the pain sensation pathway(George et al., 2000), or modulating other brain areas that modulate pain (Randich and Aicher, 1988; Nishikawa et al., 1999).
Functional dyspepsia, specifically EPS is characterized by epigastric pain or burning. Hypersensitivity to gastroduodenal distension is well described to induce pain in functional dyspepsia (Holtmann et al., 1996; Tack et al., 2001) and evidence suggests this may involve a vagal afferent pathway. In a rat model of functional dyspepsia, gastric pain accompanied vagal afferent hypersensitivity to gastric distension (Cordner et al., 2021). This pain behavior was partially improved by vagotomy or when vagal afferent hypersensitivity was reversed (Cordner et al., 2021). This suggests a role of vagal distension signaling in the increased pain perception observed in functional dyspepsia, which is in contrast to the anti-nociceptive effects observed in physiological conditions. Indeed, the gastric distension-induced pain behavior in rats with functional dyspepsia was attenuated by blocking ventrolateral PAG activity, the central descending pathway through which vagal afferents can convey information to the spinal cord (Craig, 2003), suggesting vagal hypersensitivity may increase gastric pain through the activation of brain descending signals to the spinal cord (Cordner et al., 2021). In addition, in functional dyspepsia patients, gastric distension failed to activate the brain area pregenual anterior cingulate cortex (pACC) and brain signals known to inhibit anxiety (Van Oudenhove et al., 2010). Anxiety is known to exacerbate pain sensation, therefore, the impaired modulation of gastric distension on anxiety may also lead to increased pain sensation.
In the Modulation of Gastroduodenal Motility
The response of gastroduodenal vagal afferents to distension is crucial for the regulation of gastroduodenal motility, i.e., relaxation and contraction, via a vagovagal reflex. The vagal reflex pathway includes: (1) gastric distension (corpus and antrum)-induced relaxation of the proximal stomach (Abrahamsson, 1973; Abrahamsson and Jansson, 1973), which allows accommodation of ingested food without significantly increasing intragastric pressure; (2) antral distension-induced relaxation of the pylorus and inhibition of pyloric contractions, which enables the pylorus to receive food from the antrum and facilitate gastric emptying into the duodenum (Ishiguchi et al., 2001); and (3) duodenal distension-induced relaxation of the gastric corpus and antrum and contraction of the pylorus (De Ponti et al., 1987; Holzer and Raybould, 1992; Coffin et al., 1994; Shafik, 1998), which prolongs food digestion in the stomach and delays gastric emptying.
Population studies indicate gastric distension-induced gastric relaxation, duodenal distension-induced gastric relaxation (Coffin et al., 1994), and duodenal distension-induced inhibition of small intestinal motility are impaired in functional dyspepsia (Holtmann et al., 1996), suggesting an impaired vagovagal reflex likely contributes to the impaired gastric accommodation, delayed gastric emptying and reduced duodenal motility in functional dyspepsia (Ford et al., 2020). The direct role of vagal afferents in these pathophysiological changes has not been reported, however, the increased vagal afferent signals in response to gastric distension [i.e., vagal afferent hypersensitivity, observed in mouse models of functional dyspepsia (Li et al., 2019; Cordner et al., 2021)], should amplify the vagovagal reflex and, therefore, it is unlikely abnormal vagal afferent signaling contributes to the impaired vagovagal reflex in functional dyspepsia. Other mechanisms, such as altered central processing of vagal afferent signals, and/or descending vagal efferent signaling, may be involved, which requires further investigation.
Vagal Afferent Sensation of Gastroduodenal Nutrients, Hormones and pH Levels in Functional Dyspepsia
Gastroduodenal chemosensitive vagal afferents can sense chemical stimuli including nutrients, gut hormones and pH levels. Chemosensitive vagal afferents, mainly in the duodenum, have been found to detect and respond to a wide range of nutrients, including amino acids (Jeanningros, 1982), short-chain fatty acids (Lal et al., 2001), and glucose (Mei, 1978). The nutrient-induced activation of vagal afferents is mainly secondary to nutrient-induced gut hormone release, such as CCK activation of duodenal vagal afferents (Feinle et al., 1996; Richards et al., 1996; Reidelberger et al., 2004), serotonin activation of duodenal vagal afferents (Zhu et al., 2001), GLP-1 activation of gastric vagal afferents (Bucinskaite et al., 2009) and PYY activation of gastric vagal afferents (Abbott et al., 2005; Koda et al., 2005), although direct nutrient-induced activation has also been observed (Grabauskas et al., 2010). Capsaicin, a chemical compound found in some foods, has been shown to activate gastroduodenal chemosensitive vagal afferents (Blackshaw et al., 2000). These vagal signals are conveyed to the brain, involved in the modulation of food intake, nociception, or gastric motility and secretion. Luminal acid has been found to activate gastric vagal afferents (Richards et al., 1996). The activation of vagal afferents, by noxious levels of acid, leads to activation of several brain areas, including amygdala, hypothalamic PVN, supraoptic nucleus, habenula, involved in the regulation of mood, nociception, emotion, and food intake (Michl et al., 2001). Further, there is evidence to indicate that vagal afferent responses to acid contribute to acid-induced pain sensation (Lamb et al., 2003).
Increased Gastroduodenal Food Chemicals and Hormones, and Reduced pH Levels in Functional Dyspepsia
Functional dyspepsia patients may have increased exposure of gastroduodenal chemical stimuli. Firstly, functional dyspepsia is associated with increased small intestinal mucosal permeability (Vanheel et al., 2014). This ‘leaky’ gut may increase the passive diffusion of luminal content into the mucosa, such as the food chemical capsaicin and luminal acid, therefore increasing their direct contact with chemosensitive vagal afferents in the mucosa. In addition, the production of gut hormones could be altered in functional dyspepsia, contributing to their altered exposure to vagal afferents. For example, lipid-induced release of CCK is upregulated in functional dyspepsia (Pilichiewicz et al., 2008). Furthermore, despite normal gastric fluid acid levels (Collen and Loebenberg, 1989), functional dyspepsia patients have increased exposure of acid in the duodenum (Lee et al., 2004), probably due to delayed clearance of acid from the duodenum (Samsom et al., 1999). This, in combination with a ‘leaky’ gut, may increase the exposure of acid to vagal afferent endings. The impact of altered chemical stimuli within the gut on chemosensitive vagal afferents in functional dyspepsia and the potential pathophysiological changes are illustrated in Figure 2.
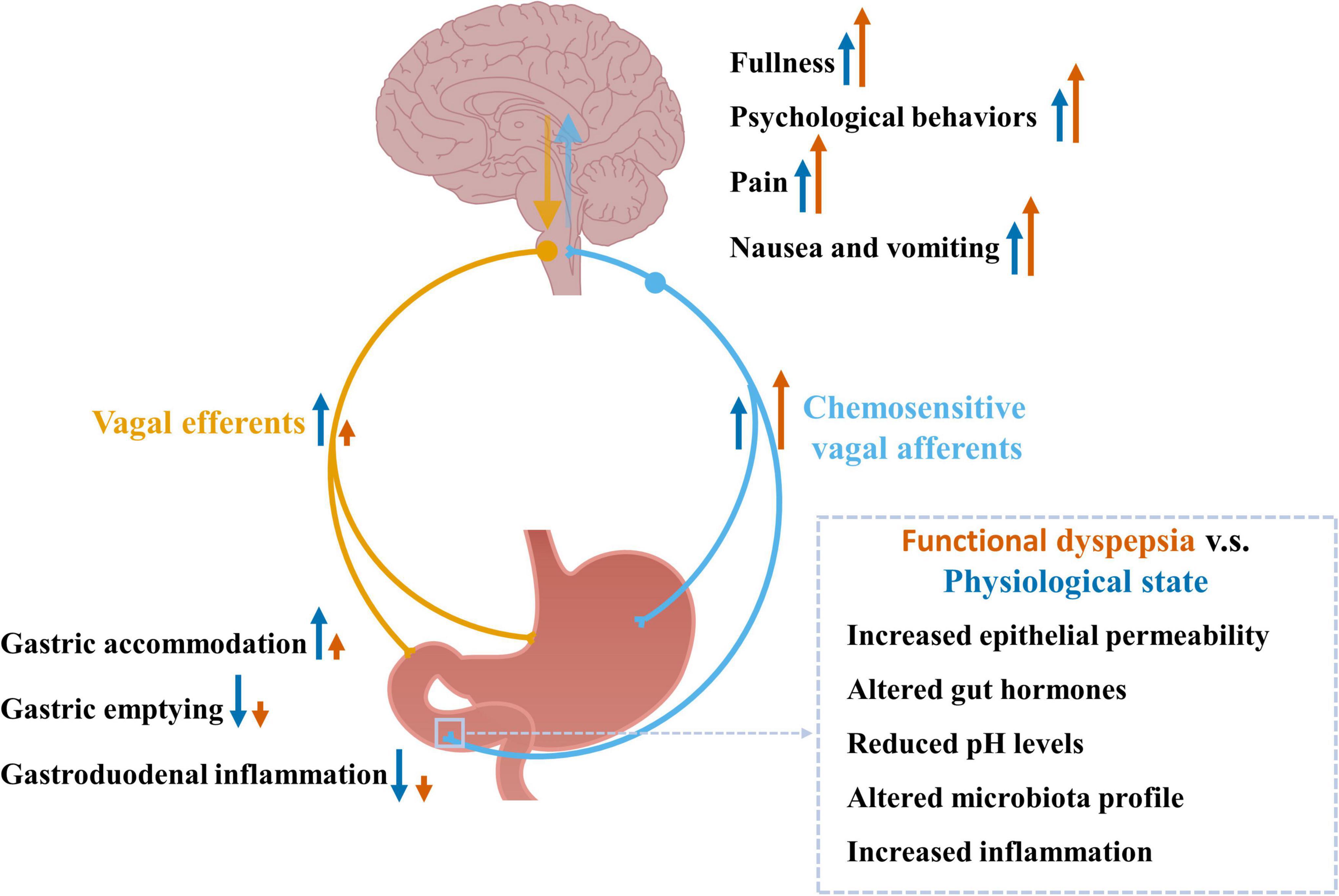
Figure 2. Chemosensitive vagal afferent signaling in response to altered chemical stimuli observed in functional dyspepsia and the associated pathophysiological changes. Chemosensitive vagal afferents can sense chemical stimuli including nutrients, gut hormones, pH levels, and inflammation-associated factors. These signals are sent to the brain via vagal afferents where the information is processed leading to increased fullness, psychological behaviors, pain sensation, nausea, and vomiting, as well as reflex signals back to the gut, via vagal efferents, leading to increased gastric accommodation and reduced gastric emptying. Functional dyspepsia is associated with increased gastroduodenal permeability, infiltration of eosinophil and mast cells, altered microbiota profile and increased chemical stimuli, which may enhance vagal afferent signals to the brain contributing to the increased feelings of fullness, psychological behaviors, pain sensation, nausea and vomiting observed in functional dyspepsia. The chemical stimuli induced vagovagal reflex is impaired in functional dyspepsia (Feinle et al., 2001; Schwartz et al., 2001), which may lead to impaired gastric accommodation, and impaired inhibition on gastric emptying and gastroduodenal inflammation.
Hypersensitivity of Chemosensitive Vagal Afferents in Functional Dyspepsia
There is no direct evidence on whether the sensitivity of vagal afferents to chemical stimuli is altered in functional dyspepsia. However, there is abundant evidence that functional dyspepsia patients have increased symptoms in response to exposure of several chemical stimuli. In functional dyspepsia patients, intraduodenal lipid infusion induces symptoms, including bloating, fullness, discomfort, nausea, and vomiting (Barbera et al., 1995a,b). A subgroup of functional dyspepsia patients demonstrate hypersensitivity to capsaicin ingestion, with significantly higher gastrointestinal symptoms compared to healthy controls (Hammer et al., 2008; Fuhrer et al., 2011; Hammer and Fuhrer, 2017). In addition, CCK administration triggers symptoms, including pain, early satiety, abdominal bloating, nausea, and vomiting, in functional dyspepsia patients (Chua et al., 1994). Furthermore, functional dyspepsia patients have increased nausea in response to duodenal acid infusion compared with healthy subjects (Samsom et al., 1999; Lee et al., 2004). Overall, this evidence suggests that hypersensitivity to chemical stimuli exists in functional dyspepsia, which may involve the hypersensitivity of chemosensitive vagal afferents, however, this requires further investigation.
Pathophysiological Roles of Altered Vagal Signaling in Response to Chemical Stimuli in Functional Dyspepsia
In the Modulation of Food Intake
Functional dyspepsia patients have heightened feelings of fullness in response to intraduodenal infusion of lipid compared to healthy controls (Barbera et al., 1995a,b). The lipid-induced early satiety could be due to an increase in lipid-induced CCK release (Feinle et al., 1996). CCK is known to inhibit food intake via the activation of chemosensitive vagal afferents and, in functional dyspepsia, high fat diet-induced CCK release is increased (Pilichiewicz et al., 2008). Further, CCK administration enhanced feelings of fullness and abdominal bloating (Chua et al., 1994) and infusion of CCK antagonists reduced lipid-induced feelings of fullness (Feinle et al., 2001). This suggests increased CCK signaling, via chemosensitive vagal afferents, may contribute to the early satiety observed in functional dyspepsia, although other pathways such as the modulatory effect of CCK on mechanosensitive vagal afferents could also be altered in functional dyspepsia. PYY is another anorexigenic hormone which can inhibit food intake via the activation of vagal afferents. Although postprandial PYY levels are reported to be reduced (Pilichiewicz et al., 2008) or unchanged (Witte et al., 2016) compared to healthy control, there is a correlation between postprandial PYY levels and feelings of fullness, suggesting a potential role of this hormone in early satiety in functional dyspepsia (Witte et al., 2016). In functional dyspepsia, postprandial GLP-1 levels are not changed and not associated with sensation of fullness (Witte et al., 2016). In addition, although serotonin inhibits food intake via vagal afferents, it is reported plasma serotonin levels are reduced (Cheung et al., 2013) or not changed (Faure et al., 2010) in functional dyspepsia, suggesting it may not contribute to the increased perception of fullness in functional dyspepsia, however, direct evidence is required.
In the Modulation of Pain Perception, Nausea, and Vomiting
Most functional dyspepsia patients have increased sensitivity to duodenal lipid infusion, which induces discomfort, pain, nausea, and vomit (Barbera et al., 1995a; Feinle-Bisset et al., 2003). These nutrient-induced symptoms are specific to fat, and not observed with glucose (Barbera et al., 1995b; Pilichiewicz et al., 2008). These lipid-induced symptoms may, at least in part, be mediated via lipid-induced CCK release. In functional dyspepsia patients, plasma CCK concentrations are positively correlated with the scores for nausea and pain (Pilichiewicz et al., 2008). Furthermore, in functional dyspepsia, intravenous administration of CCK increases abdominal pain, nausea, and vomiting (Chua et al., 1994). Further, the discomfort and nausea induced by lipid infusion are alleviated by treatment with the CCK-1 receptor antagonist dexloxiglumide (Feinle et al., 2001). Although serotonin induces nausea and vomiting via vagal afferents, in functional dyspepsia patients plasma serotonin levels are reduced (Cheung et al., 2013), suggesting it may not play an important role in nausea and vomiting in functional dyspepsia. In functional dyspepsia patients, postprandial GLP-1 levels are not changed, however, a positive correlation of GLP-1 levels with nausea was observed, suggesting a potential role of GLP-1 in nausea in functional dyspepsia (Witte et al., 2016). Evidence indicates duodenal acid plays a more significant role in inducing nausea in functional dyspepsia patients, compared to lipid and carbohydrate (Schwartz et al., 2001; Lee et al., 2004). In addition, intragastric acid administration also triggered the pain response, via vagal afferents, in rats (Lamb et al., 2003). In functional dyspepsia, duodenal acid exposure is increased (Samsom et al., 1999), which may trigger nausea and pain via a vagal afferent pathway.
In the Modulation of Gastroduodenal Motility
A subgroup of functional dyspepsia patients have reduced gastric compliance and/or delayed gastric emptying (Ford et al., 2020). Evidence indicates this may, at least in part, be due to impaired modulation of nutrients on gastric relaxation and motility. In functional dyspepsia patients, lipid-induced gastric compliance was lower compared to the healthy control group and, furthermore, the CCK-1 receptor antagonist dexloxiglumide blocked lipid-induced gastric compliance (Feinle et al., 2001). Therefore, it is possible that the lipid–CCK–vagal pathway may be altered in functional dyspepsia, contributing to impaired gastric compliance and delayed gastric emptying. In addition, functional dyspepsia is also associated with impaired duodenal motility (Sha et al., 2009). Evidence indicates this could be due to a reduction in acid-induced duodenal motility in functional dyspepsia patients (Schwartz et al., 2001). Whether this involves an impaired acid-vagal pathway requires investigation.
Vagal Afferent Sensation of Gastroduodenal Immune Stimuli in Functional Dyspepsia
The lumen of the gastrointestinal tract can be exposed to external harmful stimuli, which is defensed by mucosal structural barriers, as well as a local immune system including immune cells. This local immune defense system is stimulated by luminal antigens, pathogens, microbiota, and lipid (known as postprandial low-grade inflammation). Vagal afferents are important immunosensory nerves in the gastrointestinal tract, which can be directly activated by immune stimuli, such as LPS (Hosoi et al., 2005), or indirectly through immune stimuli associated release of: (1) microbiota products, such as short chain fatty acids; (2) hormones [e.g., serotonin released in response to LPS or short chain fatty acids (Dalile et al., 2019) and CCK released in response to long chain fatty acids (Lal et al., 2001), or IL-1β (Kurosawa et al., 1997)]; or (3) inflammatory mediators, such as cytokines released from immune cells (Steinberg et al., 2016; Zanos et al., 2018).
Immune cells can be activated by antigens and release a variety of mediators. For example, mast cells have been shown to release histamine, serotonin, tryptase, and cytokines (TNF, IL-4, basic fibroblast growth factor, stem cell factor) (Moon et al., 2014), and eosinophils release IL-12, IL-4, transforming growth factor-β, chemokines, and growth factors (Travers and Rothenberg, 2015) in response to immune stimuli. Vagal afferent endings are in close contact with mast cells in the small intestinal mucosa (Williams et al., 1997) and receptors for some of these mediators, such as 5-HT3, TNF and IL-1β, have been shown to be expressed in vagal afferent neurons (Steinberg et al., 2016). Further, these mediators have been shown to activate vagal afferents (Hillsley and Grundy, 1998; Steinberg et al., 2016; Zanos et al., 2018). These vagal mediated immune signals contribute to multiple functions, such as peripheral IL-1 and LPS-induced depression (Bluthe et al., 1994, 1996; Luheshi et al., 2000), IL-1β and LPS-induced inhibition of food-motivated behavior (Bret-Dibat et al., 1995), endotoxin, IL-1 and TNF-induced hyperalgesia (Watkins et al., 1994, 1995), and bacterial endotoxin, TNF and IL-1β-induced activation of the HPA axis (Gaykema et al., 1995; Fleshner et al., 1998).
Enhanced Gastroduodenal Immune Signals in Functional Dyspepsia
A subset of functional dyspepsia patients is associated with increased gastroduodenal permeability, which can increase the passive diffusion of luminal immune stimuli into the gut (Vanheel et al., 2014). Several studies have demonstrated that the gastroduodenal microbiota composition and amount are different between functional dyspepsia patients and healthy subjects, and the microbiota dysbiosis may be associated with the severity of functional dyspepsia symptoms, which have been well summarized in recent reviews (Tziatzios et al., 2020; Wauters et al., 2020a; Gurusamy et al., 2021). In addition, it is reported that ∼17% of functional dyspepsia patients have symptoms initiated after acute gastroenteritis (Tack et al., 2002). Several pathogens, including Salmonella spp., Escherichia coli O157, C. jejuni, Giardia lamblia, and Norovirus are associated with the post-infectious functional dyspepsia symptoms (Futagami et al., 2015). A subgroup of functional dyspepsia patients have gastroduodenal low-grade inflammation, with increased mast cell and eosinophil infiltration and degranulation. In H. pylori negative functional dyspepsia patients, plasma cytokines IL-1β, IL-10, and TNF-α levels are increased compared to healthy subjects (Liebregts et al., 2011). Furthermore, duodenal transcript expression of IL-1β, but not TNF-α is increased in functional dyspepsia (Komori et al., 2019). Indeed, plasma cytokine IL-1β, IL-10, and TNF-α levels are correlated with symptom intensity of pain, cramps, nausea, and vomiting, and associated with delayed gastric emptying, in H. pylori negative functional dyspepsia patients (Liebregts et al., 2011). Therefore, immune signals sensed by vagal afferents may be amplified, which may be involved in the corresponding symptoms, as illustrated in Figure 2.
Pathophysiological Roles of Altered Vagal Signaling in Response to Gastroduodenal Inflammation in Functional Dyspepsia
In the Modulation of Mood
Vagal signaling to the brain in response to immune stimuli contributes to the modulation of mood. Oral administration of the common bacterial enteropathogen C. jejuni in mice led to activation of the NTS, the central vagal afferent terminals, as well as brain regions regulating affective behaviors, such as the hypothalamic PVN, amygdala, and stria terminali (Gaykema et al., 2004). Furthermore, oral challenge with C. jejuni or C. rodentium induced anxiety-like behavior via activation of vagal pathways and brain areas, including PVN, amygdala and stria terminalis (Lyte et al., 2006; Goehler et al., 2008). This bacterial activation of vagal afferents may be via LPS and cytokines, as LPS and IL-1 have been shown to depress social exploration behavior, i.e., increase anxiety, via a vagal afferent pathway.
In functional dyspepsia, increased degranulation of duodenal mast cells are associated with anxiety and depression (Yuan et al., 2015). Furthermore, a 10-year follow-up study found that duodenal eosinophilia is associated with anxiety at follow-up (Ronkainen et al., 2021), suggesting gastroduodenal inflammation may contribute to psychological disorders in those patients who have gut symptoms preceding psychological disorders. Therefore, it is possible that inflammation and the increased vagal signaling in response to the inflammation contributes to the increase in psychological disorders. However, direct evidence is required to confirm this.
In the Modulation of Gastroduodenal Inflammation
The vagal afferent sensing of gastroduodenal immune signals leads to an anti-inflammatory effect. Indeed, stimulation of the vagus nerve, and specifically vagal afferent nerve, attenuated the systemic inflammatory response to endotoxin (Borovikova et al., 2000; Murray et al., 2021b) and, conversely, vagotomy sensitized animals to inflammation in a colitis animal model (Ghia et al., 2006). This anti-inflammatory effect is mediated by two pathways, the HPA axis and the vagal efferent pathway (Bonaz et al., 2016). Vagal afferents send inflammatory signals to the NTS. The NTS has projections to CRF containing neurons in the hypothalamus (Bonaz et al., 2016). This activates the HPA axis, the classic hormonal anti-inflammatory pathway, and the release of glucocorticoids to inhibit peripheral inflammation (Gaykema et al., 1995). The vagal efferent pathway is via a vagovagal inflammatory reflex, where vagal afferent mediated inflammatory signals can be centrally integrated and sent back via a descending vagal efferent pathway. The vagal efferent released neurotransmitter acetylcholine has been shown to attenuate the release of proinflammatory cytokines therefore inhibiting peripheral inflammation (Borovikova et al., 2000). This is in line with the increased hypothalamic CRF levels and plasma corticosterone levels in gastric irritation-induced functional dyspepsia rats (Liu et al., 2011), suggesting the gut inflammation-induced anti-inflammatory HPA axis pathway may be increased in functional dyspepsia. Whether the vagovagal inflammatory reflex is altered due to increased vagal afferent immune signals is not known. However, considering the generally reduced vagal efferent activity in functional dyspepsia, it is possible the vagovagal inflammatory reflex is impaired. This requires further investigation.
In the Modulation of Pain and Food Intake
Immune factors LPS, IL-1β, and TNF-α have been found to induce pain sensation and inhibit food intake via a vagal afferent pathway (Watkins et al., 1994, 1995; Schwartz et al., 1997). In H. pylori negative functional dyspepsia patients, peripheral blood mononuclear cell mediated release of cytokines IL-1β, IL-10, IL-6, and TNF-α were correlated with intensity of pain and cramps, and also nausea, vomiting and delayed gastric emptying, which can lead to reduced food intake (Liebregts et al., 2011). This suggests enhanced vagal signaling in response to inflammation may contribute to increased pain sensation and inhibition of food intake in functional dyspepsia.
Vagal Efferent Signaling From the Brain to the Gut in Functional Dyspepsia
Vagal efferents transfer brain signals to the gut, including brain signals triggered by vagal afferent gut signals, circulating hormonal signals, or descending brain signals from other brain regions. These efferents, play an important role in the modulation of gastroduodenal motility, secretion and inflammation.
Reduced Vagal Efferent Signaling in Functional Dyspepsia
Although there is no direct assessment of gastroduodenal vagal efferent activity in functional dyspepsia, a reduced cardiac vagal tone has been consistently observed in functional dyspepsia patients at basal level, after mental stress or after food intake (Hausken et al., 1993; Hveem et al., 1998; Lorena et al., 2002; Dal et al., 2014; Guo et al., 2018). The cardiac vagal tone was assessed based on variability in heart rate, including the respiratory sinus arrhythmia, monitoring the peak-to trough changes of heart rate within successive respiratory cycles, or 24-hour heart rate variability, monitoring the variation in the time interval between successive heartbeats over 24-hour period. Gastroduodenal vagal efferents share the same central origin with the cardiac vagal efferents (Bieger and Hopkins, 1987), and therefore the reduced cardiac vagal tone may more generally apply to the gastrointestinal tract. This is supported by evidence that reduced cardiac vagal tone is associated with impaired gastric relaxation and contraction in functional dyspepsia. In addition, increasing vagal tone with the use of breathing exercises reverses the impaired gastroduodenal functions in functional dyspepsia (Hjelland et al., 2007).
The reduced vagal efferent activity in functional dyspepsia could be due to the altered brain signals generated by stress. In functional dyspepsia patients, vagal tone is reduced after mental stress (Hausken et al., 1993; Hveem et al., 1998), and psychological factors explain a substantial amount of the reduction in vagal tone (Haug et al., 1994). CRF released from the hypothalamus is an important stress mediator and CRF in the brain inhibits vagal efferent activity (Broccardo and Improta, 1990; Kosoyan et al., 1999). Functional dyspepsia is associated with increased hypothalamic CRF levels (Zhu et al., 2020), which may contribute to the reduced vagal efferent activity. Furthermore, hypothalamic CRF levels can also be increased by gastric inflammation (Liu et al., 2011), suggesting reduced vagal efferent activity in functional dyspepsia could be mediated by gut inflammatory signals as well.
Functional dyspepsia is associated with an impaired vagovagal reflex in response to gut mechanical and chemical signals, as described above. Therefore, the reduced vagal efferent activity in functional dyspepsia could also be due to the impaired vagovagal reflex. The underlying mechanisms of the impaired vagovagal reflex are not known. However, in functional dyspepsia patients, reduced vagal efferent activity is correlated with increased sensation to small intestinal distension (Holtmann et al., 1998), therefore, the reduced vagal efferent activity is possibly due to a desensitization in response to the heightened vagal afferent signals. Furthermore, it is not known whether the vagal efferent anatomical innervation of the gut is altered in functional dyspepsia, which may also affect the vagal efferent function.
Pathophysiological Roles of Reduced Vagal Efferent Signaling in Functional Dyspepsia
In the Modulation of Gastrointestinal Motility
Vagal efferents coordinate with the motor neurons of the enteric nerve system, continuously adjusting gastric smooth muscle relaxation and contraction to finely control gastric motility, which is essential for normal gastric accommodation, grinding, digestion and emptying. Two parallel vagal efferent pathways are involved in this function, namely the inhibitory and excitatory vagal motor pathways. Activation of the inhibitory vagal motor pathway, or inhibition of the excitatory vagal motor pathway (tonic contraction) results in a decrease in gastric contractile tone and intragastric pressure, gastric relaxation and an increase in the gastric reservoir. In contrast, activation of the excitatory vagal motor pathway, results in an increase in gastric contractile tone and gastric motility, a reduction in the gastric reservoir, and an increase in intragastric pressure. Therefore, inhibition of the inhibitory vagal motor pathway may contribute to the resting tonic contraction.
In functional dyspepsia patients, reduced vagal efferent activity is associated with delayed gastric emptying and antral hypomotility (Hausken et al., 1993; Haug et al., 1994; Guo et al., 2018). In addition, vagal activation by sham feeding and breath exercises improves antral motility, gastric emptying and gastric accommodation in functional dyspepsia patients (Hjelland et al., 2007; Lunding et al., 2008; Frokjaer et al., 2016). Therefore, vagal efferent activity could be an important mechanism and therapeutic target for altered gastroduodenal motility in functional dyspepsia. The main neurotransmitters used by the NTS neurons are acetylcholine, γ-aminobutyric acid (GABA), glutamate and noradrenaline, among which, GABA plays a major role in the DMV, where vagal efferent neurons are located (Travagli and Anselmi, 2016). However, evidence indicates GABA concentrations in multiple brain regions including DMV are not altered (Mak et al., 2020). However, it is unknown whether the functional effect of GABA on vagal efferent signaling is altered. In functional dyspepsia patients, abnormal brain signaling in response to gastric distension has been observed, including impaired pACC activation, and impaired deactivation of dorsal pons and amygdala (Van Oudenhove et al., 2010). The dorsal vagal complex receives projections from many brain regions, including cortex, hypothalamus, and amygdala (van der Kooy et al., 1984). Therefore, it is possible the brain signals received in the dorsal vagal complex are altered, leading to the impaired vagal efferent activity in functional dyspepsia, however, this requires further investigation. In functional dyspepsia patients, reduced vagal efferent activity is correlated with increased sensation to small intestinal distension, and partial vagotomy further increases the small intestinal sensation compared to patients without vagotomy, suggesting a role of impaired vagal efferent function in the development of gastrointestinal hypersensitivity (Holtmann et al., 1998). This could be an indirect effect due to the impact of impaired vagal efferent function on gastroduodenal motility, however, this requires further investigation.
In the Modulation of Gastrointestinal Secretion
Vagal efferents play an important role in the regulation of gastroduodenal secretions. Activation of vagal efferents result in the gastric secretion of acid from parietal cells, histamine from EC-like cells and gastrin from antral gastrin cells, as well as inhibition of somatostatin release from delta cells (Chang et al., 2003). In addition, vagal efferents stimulate bicarbonate secretion from the gastric and duodenal mucosa, which is important for neutralization of acid in the stomach and duodenum, protecting the mucosa from gastric acid injury (Fandriks and Jonson, 1990).
In functional dyspepsia, gastric acid levels are not different compared to healthy control (Collen and Loebenberg, 1989), and the mean plasma gastrin levels (at baseline, after exposing to acute stress and after food stimulation) are comparable to healthy control (Jonsson et al., 1998). However, histamine levels are higher in duodenal tissue of functional dyspepsia patients compared to healthy control (Zhu et al., 2020). Stress upregulated plasma somatostatin levels is earlier in functional dyspepsia patients compared to healthy controls, and the mean plasma somatostatin levels (at baseline, after exposing to acute stress and after food stimulation) are higher in patients with a higher degree of dyspeptic symptoms compared with patients with a lower degree of symptoms (Jonsson et al., 1998). Whether vagal efferents play a role in altering gastrointestinal secretions is not known and requires further investigation.
In the Modulation of Gastrointestinal Inflammation
Vagal efferents play a crucial anti-inflammatory role by transferring descending brain signals to modulate peripheral inflammatory responses. Activation of vagal efferents leads to release of acetylcholine from vagal efferent endings. Evidence indicates different types of immune cells may be sensitive to acetylcholine and modulated by vagal efferents, including macrophages, T-cells, and mast cells. In vitro, acetylcholine significantly inhibits the release of proinflammatory cytokines (e.g., TNF, IL-1β, IL-6, and IL-18) from endotoxin LPS stimulated macrophages, attenuating the peripheral inflammatory response to endotoxin (Borovikova et al., 2000). The acetylcholine inhibited TNF release from macrophages is mediated via activation of α-7 nicotinic Ach receptors (α7nAChRs) on macrophages (Wang et al., 2003). Anterograde tracing studies indicate vagal efferent fibers projecting to the small intestine are confined within the myenteric plexus regions, specifically around nNOS, VIP, and ChAT positive enteric neurons, which are in close proximity to α7nAChR of macrophages (Cailotto et al., 2014). Therefore, vagal efferents may modulate macrophages indirectly via the myenteric plexus. Recent findings indicate this activation of macrophages relies on acetylcholine released from T-cells activated by vagal efferent signaling (Murray et al., 2021a). Mast cells also express α7nAChRs (Sudheer et al., 2006). Acetylcholine stimulates rat mast cell degranulation and release of histamine (Masini et al., 1985a). The response of mast cells to acetylcholine is correlated with the sensitization of host and mast cells (Masini et al., 1985a,b). Furthermore, cervical vagal nerve stimulation was shown to increase histamine content in intestinal mucosal mast cells (Gottwald et al., 1995), and truncal vagotomy reduced the number of intestinal mucosal mast cells (Gottwald et al., 1997). This evidence suggests intestinal mast cells can be modulated by vagal efferent signaling from the brain, however, the role of mast cells in the anti-inflammatory role of vagal efferents needs further investigation.
In functional dyspepsia, reduced vagal efferent activity may lead to an impaired vagal efferent anti-inflammatory response, which may partly contribute to the increased inflammation in functional dyspepsia. Furthermore, in functional dyspepsia patients, vagal nerve stimulation increased vagal efferent activity and reduced plasma cytokine levels (Hou et al., 2020), suggesting that upregulating vagal efferent activity in functional dyspepsia could be a therapeutic target to improve inflammation.
Therapeutic Implications of Vagus Nerve for Functional Dyspepsia
Considering the potential pathophysiological roles of increased vagal afferent signaling and reduced vagal efferent signaling in functional dyspepsia, restoring normal vagal signaling could be an important therapeutic target for the subgroup of functional dyspepsia patients with altered vagal signaling. The approaches could target any components in the bidirectional signaling loop, including altered gut signals, vagal afferent hypersensitivity, altered brain signals and the reduced vagal efferent activity, as illustrated in Figure 3. Among them, increasing vagal efferent activity has been studied for the treatment of functional dyspepsia (Hjelland et al., 2007; Lunding et al., 2008; Zhu et al., 2021). Many approaches have been found to improve vagal efferent activity, including vagal nerve stimulation, gum chewing, meditation, deep breathing, physical exercise, and drugs targeting the cholinergic system. Vagal nerve stimulation has been shown to prevent an increase in intestinal permeability (Costantini et al., 2010; Zhou et al., 2013), promote gastric emptying by increasing pyloric sphincter relaxation, increase antral contraction amplitude and peristaltic velocity (Lu et al., 2018), and inhibit inflammation (Murray et al., 2021b). In functional dyspepsia patients, vagal nerve stimulation increased gastric accommodation and motility, reduced feeling of fullness, reduced bloating and pain, and reduced anxiety and depression scores via enhanced vagal efferent activity (Zhu et al., 2021). Similarly, sham feeding and deep breath exercises, known to increase vagal efferent activity, improved gastric accommodation and increased gastric motility, as well as increased the quality of life of functional dyspepsia patients (Hjelland et al., 2007; Lunding et al., 2008).
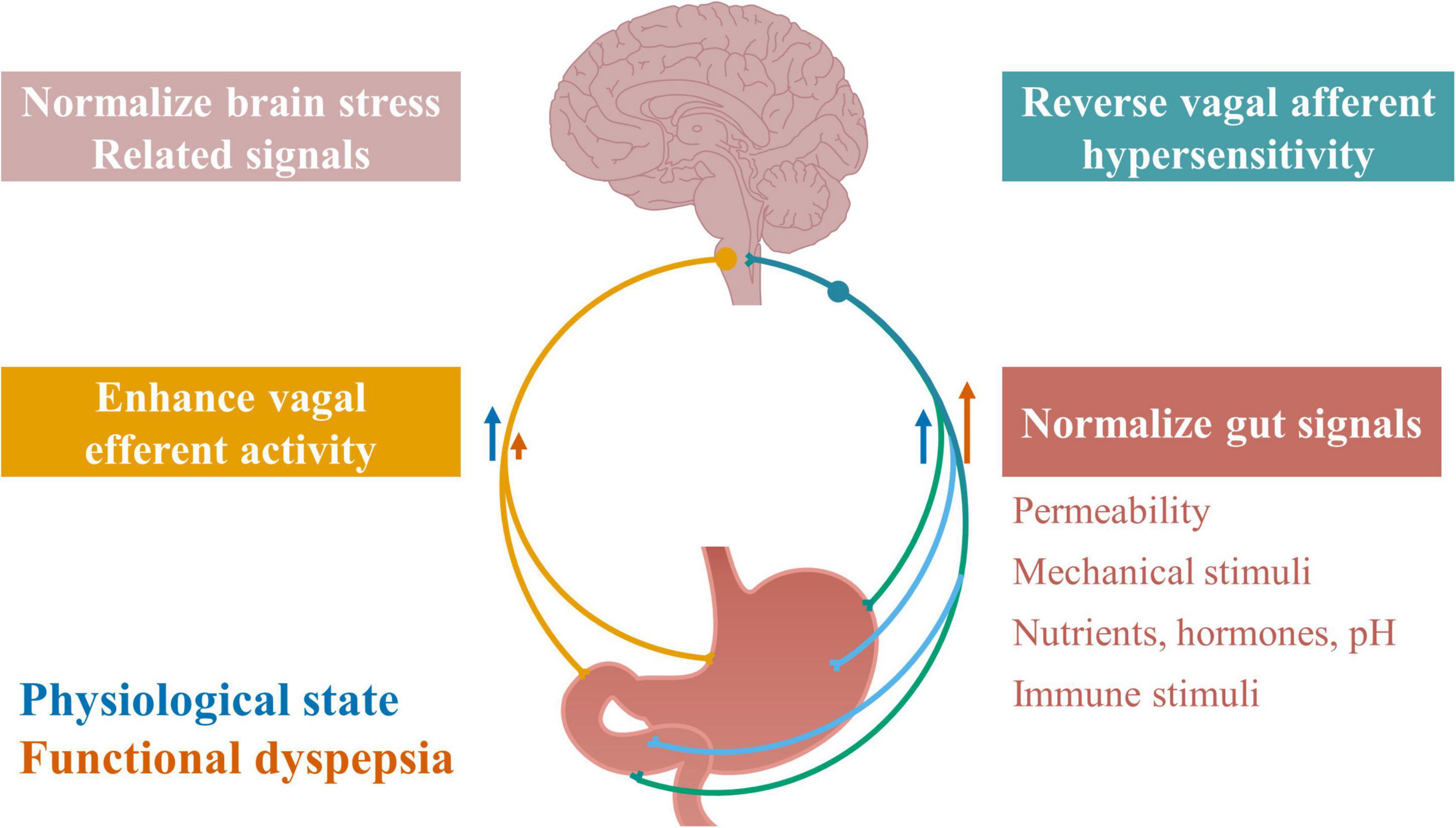
Figure 3. Therapeutic targets along vagal signaling pathways in functional dyspepsia. Restoring normal vagal signaling could be an important therapeutic target for functional dyspepsia, by normalizing any component in the vagal signaling loop, including normalizing gut signals, reversing vagal afferent hypersensitivity, normalizing brain stress related signals, and enhancing vagal efferent activity. Normalizing gut signals may target gastroduodenal permeability, mechanical stimuli, and chemical stimuli including nutrients, hormones, pH levels, and immune stimuli including microbiota.
Alterations in vagal signaling might be part of the mechanisms of existing clinical approaches for the treatment of functional dyspepsia. Some prokinetic agents, such as acotiamide, have shown beneficial effects on improving gastric emptying and gastric accommodation in functional dyspepsia patients (Nakamura et al., 2017). These changes in gastrointestinal motility are likely to impact on the mechanical stimuli applied to the vagal afferents and thus their activity. Acid suppressive therapy is effective to improve heart burn and epigastric pain in a subset of functional dyspepsia patients with EPS (Moayyedi et al., 2004), likely via a reduction in acid-induced activation of vagal pain signals. H. pylori eradication in functional dyspepsia led to long-term relief of symptoms in functional dyspepsia patients with H. pylori infection (Malfertheiner et al., 2003), and is likely through elimination of infection associated mediators. Psychological therapy has also been shown to reduce symptoms, for up to a year, in a subset of functional dyspepsia patients and likely works by reducing stress related signals from modulating the vagal efferent pathway (Van Oudenhove and Aziz, 2013). Functional dyspepsia is a heterogeneous disorder with diverse symptoms and pathophysiological changes. No existing therapy is effective to the majority of patients. It is suggested that future therapies, for functional dyspepsia patients need to be personalized and based on the individual pathological subtypes (Talley, 2020). Altered vagal signaling may be a pathophysiological change in a subset of functional dyspepsia patients and, therefore, the vagus nerve could be a potential therapeutic target for this subset of patients. Targeting ascending vagal signals might be appropriate for pathologies associated with early satiety and pain, while targeting descending vagal signals might be more appropriate for pathologies such as inflammation and abnormal gastroduodenal motility. Further understanding of the underlying mechanisms responsible for altered vagal signaling in functional dyspepsia is needed to provide insight for the development of new therapeutics for the treatment of functional dyspepsia.
Discussion
The vagus nerve plays a crucial role in the physiological modulation of brain and gut functions. In functional dyspepsia, vagal signaling between gut and brain may be disrupted in a subset of patients, contributing to a disrupted gut–brain axis and subsequent pathophysiological changes. However, the existing knowledge of this role is still very limited. A thorough understanding on the role of the vagus nerve in functional dyspepsia may provide potential clinical pathophysiological targets and eventually therapeutic targets for this disorder. This requires further studies into the mechanisms driving disordered vagal signaling including the: (1) molecular mechanisms driving the altered vagal signaling in functional dyspepsia (impaired vagal efferent signaling and heightened mechanosensitivity of vagal afferents); (2) role of chemosensitive vagal afferents in functional dyspepsia (response to local gastroduodenal chemical changes, including pH, immune associated factors, nutrients and hormones); (3) anatomical changes of vagal nerve in functional dyspepsia; (4) association of vagal signaling pathways to functional dyspepsia symptoms and pathophysiologies; and (5) effectiveness of reversing altered vagal signaling in improving functional dyspepsia symptoms and associated pathophysiological changes, etc. These potential studies will rely on clinical research and, importantly, the animal models of functional dyspepsia. Over the last decade, many animal models of functional dyspepsia have been developed, including stress-related models and gastrointestinal irritation-induced animal models, which aim to mimic the bidirectional origin of this disorder. However, none of these animal models are widely used and accepted, and the validity of these models is still not clear, as discussed in recent reviews (Ye et al., 2018; Accarie and Vanuytsel, 2020). Therefore, further understanding of the animal models is essential when choosing the appropriate animal model for studying the role of the vagus nerve, as well as other mechanisms of functional dyspepsia.
Author Contributions
HL wrote the review under the supervision of AP. AP edited the manuscript and provided assistance with the structure of the review. Both authors contributed to the manuscript and approved the submitted version.
Conflict of Interest
The authors declare that the research was conducted in the absence of any commercial or financial relationships that could be construed as a potential conflict of interest.
Publisher’s Note
All claims expressed in this article are solely those of the authors and do not necessarily represent those of their affiliated organizations, or those of the publisher, the editors and the reviewers. Any product that may be evaluated in this article, or claim that may be made by its manufacturer, is not guaranteed or endorsed by the publisher.
Abbreviations
IGLEs, intraganglionic laminar endings; IMAs, intramuscular arrays; NTS, nucleus of solitary tract; DMV, dorsal motor nucleus of the vagus; CCK, cholecystokinin; LPS, lipopolysaccharide; AgRP, agouti-related protein; PVN, paraventricular nucleus; PDS, postprandial distress syndrome; CRF, corticotrophin-releasing factor; BDNF, brain-derived neurotrophic factor; EPS, epigastric pain syndrome; PAG, periaqueductal gray; GLP-1, glucagon-like peptide-1; PYY, peptide YY; EC, enterochromaffin; TNF, tumor necrosis factor; IL-1β, interleukin-1β; HPA axis, hypothalamic–pituitary–adrenal axis; H. pylori, Helicobacter pylori; C. jejuni, Campylobacter jejuni; C. rodentium, Citrobacter rodentium; α7nAChRs, α-7 nicotinic Ach receptors.
References
Abbott, C. R., Monteiro, M., Small, C. J., Sajedi, A., Smith, K. L., Parkinson, J. R., et al. (2005). The inhibitory effects of peripheral administration of peptide YY(3-36) and glucagon-like peptide-1 on food intake are attenuated by ablation of the vagal-brainstem-hypothalamic pathway. Brain Res. 1044, 127–131. doi: 10.1016/j.brainres.2005.03.011
Abrahamsson, H. (1973). Vagal relaxation of the stomach induced from the gastric antrum. Acta Physiol. Scand. 89, 406–414. doi: 10.1111/j.1748-1716.1973.tb05535.x
Abrahamsson, H., and Jansson, G. (1973). Vago-vagal gastro-gastric relaxation in the cat. Acta Physiol. Scand. 88, 289–295. doi: 10.1111/j.1748-1716.1973.tb05457.x
Accarie, A., and Vanuytsel, T. (2020). Animal models for functional gastrointestinal disorders. Front. Psychiatry 11:509681.
Ammons, W. S., Blair, R. W., and Foreman, R. D. (1983). Vagal afferent inhibition of primate thoracic spinothalamic neurons. J. Neurophysiol. 50, 926–940. doi: 10.1152/jn.1983.50.4.926
Asala, S. A., and Bower, A. J. (1986). An electron microscope study of vagus nerve composition in the ferret. Anat. Embryol. 175, 247–253. doi: 10.1007/BF00389602
Bai, L., Mesgarzadeh, S., Ramesh, K. S., Huey, E. L., Liu, Y., Gray, L. A., et al. (2019). Genetic identification of vagal sensory neurons that control feeding. Cell 179:e1123. doi: 10.1016/j.cell.2019.10.031
Barbera, R., Feinle, C., and Read, N. W. (1995a). Abnormal sensitivity to duodenal lipid infusion in patients with functional dyspepsia. Eur. J. Gastroenterol. Hepatol. 7, 1051–1057. doi: 10.1097/00042737-199511000-00007
Barbera, R., Feinle, C., and Read, N. W. (1995b). Nutrient-specific modulation of gastric mechanosensitivity in patients with functional dyspepsia. Dig. Dis. Sci. 40, 1636–1641. doi: 10.1007/BF02212683
Berthoud, H. R., and Neuhuber, W. L. (2000). Functional and chemical anatomy of the afferent vagal system. Auton. Neurosci. 85, 1–17. doi: 10.1016/S1566-0702(00)00215-0
Berthoud, H. R., Carlson, N. R., and Powley, T. L. (1991). Topography of efferent vagal innervation of the rat gastrointestinal tract. Am. J. Physiol. 260, R200–R207. doi: 10.1152/ajpregu.1991.260.1.R200
Bieger, D., and Hopkins, D. A. (1987). Viscerotopic representation of the upper alimentary tract in the medulla oblongata in the rat: the nucleus ambiguus. J. Comp. Neurol. 262, 546–562. doi: 10.1002/cne.902620408
Blackshaw, L. A., and Grundy, D. (1990). Effects of cholecystokinin (CCK-8) on two classes of gastroduodenal vagal afferent fibre. J. Auton. Nerv. Syst. 31, 191–201. doi: 10.1016/0165-1838(90)90185-l
Blackshaw, L. A., Page, A. J., and Partosoedarso, E. R. (2000). Acute effects of capsaicin on gastrointestinal vagal afferents. Neuroscience 96, 407–416. doi: 10.1016/s0306-4522(99)00547-3
Blevins, J. E., Stanley, B. G., and Reidelberger, R. D. (2000). Brain regions where cholecystokinin suppresses feeding in rats. Brain Res. 860, 1–10. doi: 10.1016/s0006-8993(99)02477-4
Bluthe, R. M., Michaud, B., Kelley, K. W., and Dantzer, R. (1996). Vagotomy attenuates behavioural effects of interleukin-1 injected peripherally but not centrally. Neuroreport 7, 1485–1488. doi: 10.1097/00001756-199606170-00008
Bluthe, R. M., Walter, V., Parnet, P., Laye, S., Lestage, J., Verrier, D., et al. (1994). Lipopolysaccharide induces sickness behaviour in rats by a vagal mediated mechanism. C R Acad. Sci. III 317, 499–503.
Bonaz, B., Sinniger, V., and Pellissier, S. (2016). Anti-inflammatory properties of the vagus nerve: potential therapeutic implications of vagus nerve stimulation. J. Physiol. 594, 5781–5790. doi: 10.1113/JP271539
Borovikova, L. V., Ivanova, S., Zhang, M., Yang, H., Botchkina, G. I., Watkins, L. R., et al. (2000). Vagus nerve stimulation attenuates the systemic inflammatory response to endotoxin. Nature 405, 458–462. doi: 10.1038/35013070
Bret-Dibat, J. L., Bluthe, R. M., Kent, S., Kelley, K. W., and Dantzer, R. (1995). Lipopolysaccharide and interleukin-1 depress food-motivated behavior in mice by a vagal-mediated mechanism. Brain Behav. Immun. 9, 242–246. doi: 10.1006/brbi.1995.1023
Broccardo, M., and Improta, G. (1990). Pituitary-adrenal and vagus modulation of sauvagine- and CRF-induced inhibition of gastric emptying in rats. Eur. J. Pharmacol. 182, 357–362. doi: 10.1016/0014-2999(90)90294-g
Bucinskaite, V., Tolessa, T., Pedersen, J., Rydqvist, B., Zerihun, L., Holst, J. J., et al. (2009). Receptor-mediated activation of gastric vagal afferents by glucagon-like peptide-1 in the rat. Neurogastroenterol. Motil. 21, 978–e978. doi: 10.1111/j.1365-2982.2009.01317.x
Cailotto, C., Gomez-Pinilla, P. J., Costes, L. M., Van Der Vliet, J., Di Giovangiulio, M., Nemethova, A., et al. (2014). Neuro-anatomical evidence indicating indirect modulation of macrophages by vagal efferents in the intestine but not in the spleen. PLoS One 9:e87785. doi: 10.1371/journal.pone.0087785
Caldarella, M. P., Azpiroz, F., and Malagelada, J. R. (2003). Antro-fundic dysfunctions in functional dyspepsia. Gastroenterology 124, 1220–1229. doi: 10.1016/s0016-5085(03)00287-7
Chakravarthy, K., Chaudhry, H., Williams, K., and Christo, P. J. (2015). Review of the uses of vagal nerve stimulation in chronic pain management. Curr. Pain Headache Rep. 19:54. doi: 10.1007/s11916-015-0528-6
Chandler, M. J., Hobbs, S. F., Bolser, D. C., and Foreman, R. D. (1991). Effects of vagal afferent stimulation on cervical spinothalamic tract neurons in monkeys. Pain 44, 81–87. doi: 10.1016/0304-3959(91)90152-N
Chang, H. Y., Mashimo, H., and Goyal, R. K. (2003). Musings on the wanderer: what’s new in our understanding of vago-vagal reflex? IV. Current concepts of vagal efferent projections to the gut. Am. J. Physiol. Gastrointest Liver Physiol. 284, G357–G366. doi: 10.1152/ajpgi.00478.2002
Cheung, C. K., Lee, Y. Y., Chan, Y., Cheong, P. K., Law, W. T., Lee, S. F., et al. (2013). Decreased Basal and postprandial plasma serotonin levels in patients with functional dyspepsia. Clin. Gastroenterol. Hepatol. 11, 1125–1129. doi: 10.1016/j.cgh.2013.03.026
Choi, Y. J., Kim, N., Yoon, H., Shin, C. M., Park, Y. S., Park, J. H., et al. (2016). Increase in plasma acyl ghrelin levels is associated with abatement of dyspepsia following Helicobacter pylori eradication. J. Gastroenterol. 51, 548–559. doi: 10.1007/s00535-015-1124-6
Chua, A. S., Dinan, T. G., Rovati, L. C., and Keeling, P. W. (1994). Cholecystokinin hyperresponsiveness in dysmotility-type nonulcer dyspepsia. Ann. N Y Acad. Sci. 713, 298–299. doi: 10.1111/j.1749-6632.1994.tb44077.x
Clarke, G. D., and Davison, J. S. (1978). Mucosal receptors in the gastric antrum and small intestine of the rat with afferent fibres in the cervical vagus. J. Physiol. 284, 55–67. doi: 10.1113/jphysiol.1978.sp012527
Coffin, B., Azpiroz, F., Guarner, F., and Malagelada, J. R. (1994). Selective gastric hypersensitivity and reflex hyporeactivity in functional dyspepsia. Gastroenterology 107, 1345–1351. doi: 10.1016/0016-5085(94)90536-3
Collen, M. J., and Loebenberg, M. J. (1989). Basal gastric acid secretion in nonulcer dyspepsia with or without duodenitis. Dig. Dis. Sci. 34, 246–250. doi: 10.1007/BF01536059
Cordner, Z. A., Li, Q., Liu, L., Tamashiro, K. L., Bhargava, A., Moran, T. H., et al. (2021). Vagal gut-brain signaling mediates amygdaloid plasticity, affect and pain in a functional dyspepsia model. JCI Insight. 6:e144046. doi: 10.1172/jci.insight.144046
Costantini, T. W., Bansal, V., Peterson, C. Y., Loomis, W. H., Putnam, J. G., Rankin, F., et al. (2010). Efferent vagal nerve stimulation attenuates gut barrier injury after burn: modulation of intestinal occludin expression. J. Trauma 68, 1349–1354. doi: 10.1097/TA.0b013e3181dccea0
Craig, A. D. (2003). A new view of pain as a homeostatic emotion. Trends Neurosci. 26, 303–307. doi: 10.1016/s0166-2236(03)00123-1
Cuomo, R., Sarnelli, G., Grasso, R., Bruzzese, D., Pumpo, R., Salomone, M., et al. (2001). Functional dyspepsia symptoms, gastric emptying and satiety provocative test: analysis of relationships. Scand. J. Gastroenterol. 36, 1030–1036. doi: 10.1080/003655201750422611
Dal, K., Deveci, O. S., Kucukazman, M., Ata, N., Sen, O., Ozkan, S., et al. (2014). Decreased parasympathetic activity in patients with functional dyspepsia. Eur. J. Gastroenterol. Hepatol. 26, 748–752. doi: 10.1097/MEG.0000000000000111
Dalile, B., Van Oudenhove, L., Vervliet, B., and Verbeke, K. (2019). The role of short-chain fatty acids in microbiota-gut-brain communication. Nat. Rev. Gastroenterol. Hepatol. 16, 461–478. doi: 10.1038/s41575-019-0157-3
Date, Y., Murakami, N., Toshinai, K., Matsukura, S., Niijima, A., Matsuo, H., et al. (2002). The role of the gastric afferent vagal nerve in ghrelin-induced feeding and growth hormone secretion in rats. Gastroenterology 123, 1120–1128. doi: 10.1053/gast.2002.35954
Davison, J. S., and Clarke, G. D. (1988). Mechanical properties and sensitivity to CCK of vagal gastric slowly adapting mechanoreceptors. Am. J. Physiol. 255, G55–G61. doi: 10.1152/ajpgi.1988.255.1.G55
De Ponti, F., Azpiroz, F., and Malagelada, J. R. (1987). Reflex gastric relaxation in response to distention of the duodenum. Am. J. Physiol. 252, G595–G601. doi: 10.1152/ajpgi.1987.252.5.G595
Du, L., Chen, B., Kim, J. J., Chen, X., and Dai, N. (2018). Micro-inflammation in functional dyspepsia: a systematic review and meta-analysis. Neurogastroenterol. Motil. 30:e13304. doi: 10.1111/nmo.13304
Fandriks, L., and Jonson, C. (1990). Vagal and sympathetic control of gastric and duodenal bicarbonate secretion. J. Intern. Med. Suppl. 732, 103–107. doi: 10.1111/j.1365-2796.1990.tb01480.x
Faure, C., Patey, N., Gauthier, C., Brooks, E. M., and Mawe, G. M. (2010). Serotonin signaling is altered in irritable bowel syndrome with diarrhea but not in functional dyspepsia in pediatric age patients. Gastroenterology 139, 249–258. doi: 10.1053/j.gastro.2010.03.032
Feinle, C., D’amato, M., and Read, N. W. (1996). Cholecystokinin-A receptors modulate gastric sensory and motor responses to gastric distension and duodenal lipid. Gastroenterology 110, 1379–1385. doi: 10.1053/gast.1996.v110.pm8613041
Feinle, C., Meier, O., Otto, B., D’amato, M., and Fried, M. (2001). Role of duodenal lipid and cholecystokinin A receptors in the pathophysiology of functional dyspepsia. Gut 48, 347–355. doi: 10.1136/gut.48.3.347
Feinle-Bisset, C., Meier, B., Fried, M., and Beglinger, C. (2003). Role of cognitive factors in symptom induction following high and low fat meals in patients with functional dyspepsia. Gut 52, 1414–1418. doi: 10.1136/gut.52.10.1414
Fleshner, M., Goehler, L. E., Schwartz, B. A., Mcgorry, M., Martin, D., Maier, S. F., et al. (1998). Thermogenic and corticosterone responses to intravenous cytokines (IL-1beta and TNF-alpha) are attenuated by subdiaphragmatic vagotomy. J. Neuroimmunol. 86, 134–141. doi: 10.1016/s0165-5728(98)00026-5
Foley, J. O., and DuBois, F. S. (1937). Quantitative studies of the vagus nerve in the cat I. The ratio of sensory to motor fibers. J. Nerv. Ment. Dis. 86:587. doi: 10.1097/00005053-193711000-00019
Ford, A. C., Mahadeva, S., Carbone, M. F., Lacy, B. E., and Talley, N. J. (2020). Functional dyspepsia. Lancet 396, 1689–1702.
Frokjaer, J. B., Bergmann, S., Brock, C., Madzak, A., Farmer, A. D., Ellrich, J., et al. (2016). Modulation of vagal tone enhances gastroduodenal motility and reduces somatic pain sensitivity. Neurogastroenterol. Motil. 28, 592–598. doi: 10.1111/nmo.12760
Fuhrer, M., Vogelsang, H., and Hammer, J. (2011). A placebo-controlled trial of an oral capsaicin load in patients with functional dyspepsia. Neurogastroenterol. Motil. 23:918. doi: 10.1111/j.1365-2982.2011.01766.x
Futagami, S., Itoh, T., and Sakamoto, C. (2015). Systematic review with meta-analysis: post-infectious functional dyspepsia. Alim. Pharmacol. Ther. 41, 177–188. doi: 10.1111/apt.13006
Gaykema, R. P., Dijkstra, I., and Tilders, F. J. (1995). Subdiaphragmatic vagotomy suppresses endotoxin-induced activation of hypothalamic corticotropin-releasing hormone neurons and ACTH secretion. Endocrinology 136, 4717–4720. doi: 10.1210/endo.136.10.7664696
Gaykema, R. P., Goehler, L. E., and Lyte, M. (2004). Brain response to cecal infection with Campylobacter jejuni: analysis with Fos immunohistochemistry. Brain Behav. Immun. 18, 238–245. doi: 10.1016/j.bbi.2003.08.002
George, M. S., Sackeim, H. A., Rush, A. J., Marangell, L. B., Nahas, Z., Husain, M. M., et al. (2000). Vagus nerve stimulation: a new tool for brain research and therapy. Biol. Psychiatry 47, 287–295. doi: 10.1016/s0006-3223(99)00308-x
Ghia, J. E., Blennerhassett, P., Kumar-Ondiveeran, H., Verdu, E. F., and Collins, S. M. (2006). The vagus nerve: a tonic inhibitory influence associated with inflammatory bowel disease in a murine model. Gastroenterology 131, 1122–1130. doi: 10.1053/j.gastro.2006.08.016
Goehler, L. E., Park, S. M., Opitz, N., Lyte, M., and Gaykema, R. P. (2008). Campylobacter jejuni infection increases anxiety-like behavior in the holeboard: possible anatomical substrates for viscerosensory modulation of exploratory behavior. Brain Behav. Immun. 22, 354–366. doi: 10.1016/j.bbi.2007.08.009
Gottwald, T. P., Hewlett, B. R., Lhotak, S., and Stead, R. H. (1995). Electrical stimulation of the vagus nerve modulates the histamine content of mast cells in the rat jejunal mucosa. Neuroreport 7, 313–317. doi: 10.1097/00001756-199512290-00075
Gottwald, T., Lhotak, S., and Stead, R. H. (1997). Effect of truncal vagotomy and capsaicin on mast cells and IgA-positive plasma cells in rat jejunal mucosa. Neurogastroenterol. Motil. 9, 25–32. doi: 10.1046/j.1365-2982.1997.d01-4.x
Grabauskas, G., Song, I., Zhou, S., and Owyang, C. (2010). Electrophysiological identification of glucose-sensing neurons in rat nodose ganglia. J. Physiol. 588, 617–632. doi: 10.1113/jphysiol.2009.182147
Guo, W. J., Yao, S. K., Zhang, Y. L., Du, S. Y., Wang, H. F., Yin, L. J., et al. (2018). Impaired vagal activity to meal in patients with functional dyspepsia and delayed gastric emptying. J. Int. Med. Res. 46, 792–801. doi: 10.1177/0300060517726442
Gurusamy, S. R., Shah, A., Talley, N. J., Koloski, N., Jones, M. P., Walker, M. M., et al. (2021). Small intestinal bacterial overgrowth in functional dyspepsia: a systematic review and meta-analysis. Am. J. Gastroenterol. 116, 935–942. doi: 10.14309/ajg.0000000000001197
Hammer, J., and Fuhrer, M. (2017). Clinical characteristics of functional dyspepsia depending on chemosensitivity to capsaicin. Neurogastroenterol. Motil. 29, 1–12. doi: 10.1111/nmo.13103
Hammer, J., Fuhrer, M., Pipal, L., and Matiasek, J. (2008). Hypersensitivity for capsaicin in patients with functional dyspepsia. Neurogastroenterol. Motil. 20, 125–133. doi: 10.1111/j.1365-2982.2007.00997.x
Han, W., Tellez, L. A., Perkins, M. H., Perez, I. O., Qu, T., Ferreira, J., et al. (2018). A neural circuit for gut-induced reward. Cell 175, 665–678. doi: 10.1016/j.cell.2018.08.049
Haug, T. T., Svebak, S., Hausken, T., Wilhelmsen, I., Berstad, A., and Ursin, H. (1994). Low vagal activity as mediating mechanism for the relationship between personality factors and gastric symptoms in functional dyspepsia. Psychosom. Med. 56, 181–186. doi: 10.1097/00006842-199405000-00001
Hausken, T., and Berstad, A. (1992). Wide gastric antrum in patients with non-ulcer dyspepsia. Effect of cisapride. Scand. J. Gastroenterol. 27, 427–432. doi: 10.3109/00365529209000100
Hausken, T., Svebak, S., Wilhelmsen, I., Haug, T. T., Olafsen, K., Pettersson, E., et al. (1993). Low vagal tone and antral dysmotility in patients with functional dyspepsia. Psychosom. Med. 55, 12–22. doi: 10.1097/00006842-199301000-00004
Hillsley, K., and Grundy, D. (1998). Serotonin and cholecystokinin activate different populations of rat mesenteric vagal afferents. Neurosci. Lett. 255, 63–66. doi: 10.1016/s0304-3940(98)00690-9
Hjelland, I. E., Svebak, S., Berstad, A., Flatabo, G., and Hausken, T. (2007). Breathing exercises with vagal biofeedback may benefit patients with functional dyspepsia. Scand. J. Gastroenterol. 42, 1054–1062. doi: 10.1080/00365520701259208
Holtmann, G., Goebell, H., and Talley, J. (1996). Impaired small intestinal peristaltic reflexes and sensory thresholds are independent functional disturbances in patients with chronic unexplained dyspepsia. Am. J. Gastroenterol. 91, 485–491.
Holtmann, G., Goebell, H., Jockenhoevel, F., and Talley, N. J. (1998). Altered vagal and intestinal mechanosensory function in chronic unexplained dyspepsia. Gut 42, 501–506. doi: 10.1136/gut.42.4.501
Holzer, H. H., and Raybould, H. E. (1992). Vagal and splanchnic sensory pathways mediate inhibition of gastric motility induced by duodenal distension. Am. J. Physiol. 262, G603–G608. doi: 10.1152/ajpgi.1992.262.4.G603
Hosoi, T., Okuma, Y., and Nomura, Y. (2000). Electrical stimulation of afferent vagus nerve induces IL-1beta expression in the brain and activates HPA axis. Am. J. Physiol. Regul. Integr. Comp. Physiol. 279, R141–R147. doi: 10.1152/ajpregu.2000.279.1.R141
Hosoi, T., Okuma, Y., Matsuda, T., and Nomura, Y. (2005). Novel pathway for LPS-induced afferent vagus nerve activation: possible role of nodose ganglion. Auton. Neurosci. 120, 104–107. doi: 10.1016/j.autneu.2004.11.012
Hou, L., Rong, P., Wei, W., Fang, J., Wang, D., Zhai, W., et al. (2020). Effect and mechanism study on transcutaneous auricular vagus nerve stimulation for functional dyspepsia model rats. World J. Acupun. Moxibust. 30, 49–56. doi: 10.1016/j.wjam.2020.02.007
Hveem, K., Svebak, S., Hausken, T., and Berstad, A. (1998). Effect of mental stress and cisapride on autonomic nerve functions in functional dyspepsia. Scand. J. Gastroenterol. 33, 123–127. doi: 10.1080/00365529850166824
Iggo, A. (1955). Tension receptors in the stomach and the urinary bladder. J. Physiol. 128, 593–607. doi: 10.1113/jphysiol.1955.sp005327
Iggo, A. (1957). Gastro-intestinal tension receptors with unmyelinated afferent fibres in the vagus of the cat. Q. J. Exp. Physiol. Cogn. Med. Sci. 42, 130–143. doi: 10.1113/expphysiol.1957.sp001228
Ishiguchi, T., Nakajima, M., Sone, H., Tada, H., Kumagai, A. K., and Takahashi, T. (2001). Gastric distension-induced pyloric relaxation: central nervous system regulation and effects of acute hyperglycaemia in the rat. J. Physiol. 533, 801–813. doi: 10.1111/j.1469-7793.2001.t01-1-00801.x
Jeanningros, R. (1982). Vagal unitary responses to intestinal amino acid infusions in the anesthetized cat: a putative signal for protein induced satiety. Physiol. Behav. 28, 9–21. doi: 10.1016/0031-9384(82)90094-4
Jones, K. L., Doran, S. M., Hveem, K., Bartholomeusz, F. D., Morley, J. E., Sun, W. M., et al. (1997). Relation between postprandial satiation and antral area in normal subjects. Am. J. Clin. Nutr. 66, 127–132. doi: 10.1093/ajcn/66.1.127
Jonsson, B. H., Uvnas-Moberg, K., Theorell, T., and Gotthard, R. (1998). Gastrin, cholecystokinin, and somatostatin in a laboratory experiment of patients with functional dyspepsia. Psychosom. Med. 60, 331–337. doi: 10.1097/00006842-199805000-00020
Kalia, M., and Sullivan, J. M. (1982). Brainstem projections of sensory and motor components of the vagus nerve in the rat. J. Comp. Neurol. 211, 248–265. doi: 10.1002/cne.902110304
Kang, Y. M., Bielefeldt, K., and Gebhart, G. F. (2004). Sensitization of mechanosensitive gastric vagal afferent fibers in the rat by thermal and chemical stimuli and gastric ulcers. J. Neurophysiol. 91, 1981–1989. doi: 10.1152/jn.01097.2003
Khasar, S. G., Green, P. G., Miao, F. J., and Levine, J. D. (2003). Vagal modulation of nociception is mediated by adrenomedullary epinephrine in the rat. Eur. J. Neurosci. 17, 909–915. doi: 10.1046/j.1460-9568.2003.02503.x
Kim, M., Heo, G., and Kim, S. Y. (2022). Neural signalling of gut mechanosensation in ingestive and digestive processes. Nat. Rev. Neurosci. 23, 135–156. doi: 10.1038/s41583-021-00544-7
Klarer, M., Arnold, M., Gunther, L., Winter, C., Langhans, W., and Meyer, U. (2014). Gut vagal afferents differentially modulate innate anxiety and learned fear. J. Neurosci. 34, 7067–7076. doi: 10.1523/JNEUROSCI.0252-14.2014
Koda, S., Date, Y., Murakami, N., Shimbara, T., Hanada, T., Toshinai, K., et al. (2005). The role of the vagal nerve in peripheral PYY3-36-induced feeding reduction in rats. Endocrinology 146, 2369–2375. doi: 10.1210/en.2004-1266
Komori, K., Ihara, E., Minoda, Y., Ogino, H., Sasaki, T., Fujiwara, M., et al. (2019). The altered mucosal barrier function in the duodenum plays a role in the pathogenesis of functional dyspepsia. Dig. Dis. Sci. 64, 3228–3239. doi: 10.1007/s10620-019-5470-8
Kosoyan, H. P., Wei, J. Y., and Tache, Y. (1999). Intracisternal sauvagine is more potent than corticotropin-releasing factor to decrease gastric vagal efferent activity in rats. Peptides 20, 851–858. doi: 10.1016/s0196-9781(99)00072-8
Kurosawa, M., Uvnas-Moberg, K., Miyasaka, K., and Lundeberg, T. (1997). Interleukin-1 increases activity of the gastric vagal afferent nerve partly via stimulation of type A CCK receptor in anesthetized rats. J. Auton. Nerv. Syst. 62, 72–78. doi: 10.1016/s0165-1838(96)00111-7
Lal, S., Kirkup, A. J., Brunsden, A. M., Thompson, D. G., and Grundy, D. (2001). Vagal afferent responses to fatty acids of different chain length in the rat. Am. J. Physiol. Gastroint. Liver Physiol. 281, G907–G915. doi: 10.1152/ajpgi.2001.281.4.G907
Lamb, K., Kang, Y. M., Gebhart, G. F., and Bielefeldt, K. (2003). Gastric inflammation triggers hypersensitivity to acid in awake rats. Gastroenterology 125, 1410–1418. doi: 10.1016/j.gastro.2003.07.010
Lee, K. J., Demarchi, B., Demedts, I., Sifrim, D., Raeymaekers, P., and Tack, J. (2004). A pilot study on duodenal acid exposure and its relationship to symptoms in functional dyspepsia with prominent nausea. Am. J. Gastroenterol. 99, 1765–1773. doi: 10.1111/j.1572-0241.2004.30822.x
Li, H., Buisman-Pijlman, F. T. A., Nunez-Salces, M., Christie, S., Frisby, C. L., Inserra, A., et al. (2019). Chronic stress induces hypersensitivity of murine gastric vagal afferents. Neurogastroenterol. Motil. 31:e13669. doi: 10.1111/nmo.13669
Liebregts, T., Adam, B., Bredack, C., Gururatsakul, M., Pilkington, K. R., Brierley, S. M., et al. (2011). Small bowel homing T cells are associated with symptoms and delayed gastric emptying in functional dyspepsia. Am. J. Gastroenterol. 106, 1089–1098. doi: 10.1038/ajg.2010.512
Liu, L., Li, Q., Sapolsky, R., Liao, M., Mehta, K., Bhargava, A., et al. (2011). Transient gastric irritation in the neonatal rats leads to changes in hypothalamic CRF expression, depression- and anxiety-like behavior as adults. PLoS One 6:e19498. doi: 10.1371/journal.pone.0019498
Lorena, S. L., Figueiredo, M. J., Almeida, J. R., and Mesquita, M. A. (2002). Autonomic function in patients with functional dyspepsia assessed by 24-hour heart rate variability. Dig. Dis. Sci. 47, 27–31. doi: 10.1023/a:1013246900041
Lu, K. H., Cao, J., Oleson, S., Ward, M. P., Phillips, R. J., Powley, T. L., et al. (2018). Vagus nerve stimulation promotes gastric emptying by increasing pyloric opening measured with magnetic resonance imaging. Neurogastroenterol. Motil. 30:e13380. doi: 10.1111/nmo.13380
Luheshi, G. N., Bluthe, R. M., Rushforth, D., Mulcahy, N., Konsman, J. P., Goldbach, M., et al. (2000). Vagotomy attenuates the behavioural but not the pyrogenic effects of interleukin-1 in rats. Auton. Neurosci. 85, 127–132. doi: 10.1016/S1566-0702(00)00231-9
Lunding, J. A., Nordstrom, L. M., Haukelid, A. O., Gilja, O. H., Berstad, A., and Hausken, T. (2008). Vagal activation by sham feeding improves gastric motility in functional dyspepsia. Neurogastroenterol. Motil. 20, 618–624. doi: 10.1111/j.1365-2982.2007.01076.x
Lyte, M., Li, W., Opitz, N., Gaykema, R. P., and Goehler, L. E. (2006). Induction of anxiety-like behavior in mice during the initial stages of infection with the agent of murine colonic hyperplasia Citrobacter rodentium. Physiol. Behav. 89, 350–357. doi: 10.1016/j.physbeh.2006.06.019
Mak, A. D. P., Ho, Y. M., Leung, O. N. W., Chou, I. W. Y., Lui, R., Wong, S., et al. (2020). Unaltered Brain GABA Concentrations and Resting fMRI activity in functional dyspepsia with and without comorbid depression. Front. Psychiatry 11:549749. doi: 10.3389/fpsyt.2020.549749
Malfertheiner, P., Fischbach, W., Layer, P., Leodolter, A., Stolte, M., Demleitner, K., et al. (2003). Helicobacter pylori eradication is beneficial in the treatment of functional dyspepsia. Alim. Pharmacol. Ther. 18, 615–625. doi: 10.1046/j.1365-2036.2003.01695.x
Masini, E., Fantozzi, R., Conti, A., Blandina, P., Brunelleschi, S., and Mannaioni, P. F. (1985a). Immunological modulation of cholinergic histamine release in isolated rat mast cells. Agents Act. 16, 152–154. doi: 10.1007/BF01983125
Masini, E., Fantozzi, R., Conti, A., Blandina, P., Brunelleschi, S., and Mannaioni, P. F. (1985b). Mast cell heterogeneity in response to cholinergic stimulation. Int. Arch. Allergy Appl. Immunol. 77, 184–185. doi: 10.1159/000233780
McCann, M. J., and Rogers, R. C. (1992). Impact of antral mechanoreceptor activation on the vago-vagal reflex in the rat: functional zonation of responses. J. Physiol. 453, 401–411. doi: 10.1113/jphysiol.1992.sp019235
Mei, N. (1978). Vagal glucoreceptors in the small intestine of the cat. J. Physiol. 282, 485–506. doi: 10.1113/jphysiol.1978.sp012477
Michl, T., Jocic, M., Heinemann, A., Schuligoi, R., and Holzer, P. (2001). Vagal afferent signaling of a gastric mucosal acid insult to medullary, pontine, thalamic, hypothalamic and limbic, but not cortical, nuclei of the rat brain. Pain 92, 19–27. doi: 10.1016/s0304-3959(00)00467-x
Moayyedi, P., Delaney, B. C., Vakil, N., Forman, D., and Talley, N. J. (2004). The efficacy of proton pump inhibitors in nonulcer dyspepsia: a systematic review and economic analysis. Gastroenterology 127, 1329–1337. doi: 10.1053/j.gastro.2004.08.026
Moon, T. C., Befus, A. D., and Kulka, M. (2014). Mast cell mediators: their differential release and the secretory pathways involved. Front. Immunol. 5:569. doi: 10.3389/fimmu.2014.00569
Murray, K., Rude, K. M., Sladek, J., and Reardon, C. (2021a). Divergence of neuroimmune circuits activated by afferent and efferent vagal nerve stimulation in the regulation of inflammation. J. Physiol. 599, 2075–2084. doi: 10.1113/JP281189
Murray, K., Rude, K. M., Sladek, J., and Reardon, C. (2021b). Electrical activation of vagal efferent or afferent neurons elicits two distinct mechanisms of anti-inflammatory actions. J. Immunol. 206:13.
Nakamura, K., Tomita, T., Oshima, T., Asano, H., Yamasaki, T., Okugawa, T., et al. (2017). A double-blind placebo controlled study of acotiamide hydrochloride for efficacy on gastrointestinal motility of patients with functional dyspepsia. J. Gastroenterol. 52, 602–610. doi: 10.1007/s00535-016-1260-7
Nishikawa, Y., Koyama, N., Yoshida, Y., and Yokota, T. (1999). Activation of ascending antinociceptive system by vagal afferent input as revealed in the nucleus ventralis posteromedialis. Brain Res. 833, 108–111. doi: 10.1016/s0006-8993(99)01521-8
Onaka, T., and Takayanagi, Y. (2019). Role of oxytocin in the control of stress and food intake. J. Neuroendocrinol. 31:e12700. doi: 10.1111/jne.12700
Page, A. J., Slattery, J. A., Milte, C., Laker, R., O’donnell, T., Dorian, C., et al. (2007). Ghrelin selectively reduces mechanosensitivity of upper gastrointestinal vagal afferents. Am. J. Physiol. Gastroint. Liver Physiol. 292, G1376–G1384. doi: 10.1152/ajpgi.00536.2006
Pilichiewicz, A. N., Feltrin, K. L., Horowitz, M., Holtmann, G., Wishart, J. M., Jones, K. L., et al. (2008). Functional dyspepsia is associated with a greater symptomatic response to fat but not carbohydrate, increased fasting and postprandial CCK, and diminished PYY. Am. J. Gastroenterol. 103, 2613–2623. doi: 10.1111/j.1572-0241.2008.02041.x
Randich, A., and Aicher, S. A. (1988). Medullary substrates mediating antinociception produced by electrical stimulation of the vagus. Brain Res. 445, 68–76. doi: 10.1016/0006-8993(88)91075-x
Reidelberger, R. D., Hernandez, J., Fritzsch, B., and Hulce, M. (2004). Abdominal vagal mediation of the satiety effects of CCK in rats. Am. J. Physiol. Regul. Integr. Comp. Physiol. 286, R1005–R1012. doi: 10.1152/ajpregu.00646.2003
Ricardo, J. A., and Koh, E. T. (1978). Anatomical evidence of direct projections from the nucleus of the solitary tract to the hypothalamus, amygdala, and other forebrain structures in the rat. Brain Res. 153, 1–26. doi: 10.1016/0006-8993(78)91125-3
Richards, W., Hillsley, K., Eastwood, C., and Grundy, D. (1996). Sensitivity of vagal mucosal afferents to cholecystokinin and its role in afferent signal transduction in the rat. J. Physiol. 497, 473–481. doi: 10.1113/jphysiol.1996.sp021781
Rinaman, L. (2007). Visceral sensory inputs to the endocrine hypothalamus. Front. Neuroendocrinol. 28:50–60. doi: 10.1016/j.yfrne.2007.02.002
Rinaman, L. (2010). Ascending projections from the caudal visceral nucleus of the solitary tract to brain regions involved in food intake and energy expenditure. Brain Res. 1350, 18–34. doi: 10.1016/j.brainres.2010.03.059
Ronkainen, J., Aro, P., Jones, M., Walker, M. M., Agreus, L., Andreasson, A., et al. (2021). Duodenal eosinophilia and the link to anxiety: A population-based endoscopic study. Neurogastroenterol. Motil. 33:e14109. doi: 10.1111/nmo.14109
Sagarkar, S., Bhamburkar, T., Shelkar, G., Choudhary, A., Kokare, D. M., and Sakharkar, A. J. (2017). Minimal traumatic brain injury causes persistent changes in DNA methylation at BDNF gene promoters in rat amygdala: A possible role in anxiety-like behaviors. Neurobiol. Dis. 106, 101–109. doi: 10.1016/j.nbd.2017.06.016
Samsom, M., Verhagen, M. A., Vanberge Henegouwen, G. P., and Smout, A. J. (1999). Abnormal clearance of exogenous acid and increased acid sensitivity of the proximal duodenum in dyspeptic patients. Gastroenterology 116, 515–520. doi: 10.1016/s0016-5085(99)70171-x
Schwartz, G. J., Plata-Salaman, C. R., and Langhans, W. (1997). Subdiaphragmatic vagal deafferentation fails to block feeding-suppressive effects of LPS and IL-1 beta in rats. Am. J. Physiol. 273, R1193–R1198. doi: 10.1152/ajpregu.1997.273.3.R1193
Schwartz, G. J., Tougas, G., and Moran, T. H. (1995). Integration of vagal afferent responses to duodenal loads and exogenous CCK in rats. Peptides 16, 707–711. doi: 10.1016/0196-9781(95)00033-g
Schwartz, M. P., Samsom, M., and Smout, A. J. (2001). Chemospecific alterations in duodenal perception and motor response in functional dyspepsia. Am. J. Gastroenterol. 96, 2596–2602. doi: 10.1111/j.1572-0241.2001.04103.x
Sedan, O., Sprecher, E., and Yarnitsky, D. (2005). Vagal stomach afferents inhibit somatic pain perception. Pain 113, 354–359. doi: 10.1016/j.pain.2004.11.012
Sha, W., Pasricha, P. J., and Chen, J. D. (2009). Correlations among electrogastrogram, gastric dysmotility, and duodenal dysmotility in patients with functional dyspepsia. J. Clin. Gastroenterol. 43, 716–722. doi: 10.1097/MCG.0b013e31818b8ed9
Shafik, A. (1998). Effect of duodenal distension on the pyloric sphincter and antrum and the gastric corpus: duodenopyloric reflex. World J. Surg. 22, 1061–1064. doi: 10.1007/s002689900517
Shindo, T., Futagami, S., Hiratsuka, T., Horie, A., Hamamoto, T., Ueki, N., et al. (2009). Comparison of gastric emptying and plasma ghrelin levels in patients with functional dyspepsia and non-erosive reflux disease. Digestion 79, 65–72. doi: 10.1159/000205740
Stanghellini, V., Tosetti, C., Paternico, A., Barbara, G., Morselli-Labate, A. M., Monetti, N., et al. (1996). Risk indicators of delayed gastric emptying of solids in patients with functional dyspepsia. Gastroenterology 110, 1036–1042. doi: 10.1053/gast.1996.v110.pm8612991
Steinberg, B. E., Silverman, H. A., Robbiati, S., Gunasekaran, M. K., Tsaava, T., Battinelli, E., et al. (2016). Cytokine-specific neurograms in the sensory vagus nerve. Bioelect. Med. 3, 7–17. doi: 10.15424/bioelectronmed.2016.00007
Sudheer, P. S., Hall, J. E., Donev, R., Read, G., Rowbottom, A., and Williams, P. E. (2006). Nicotinic acetylcholine receptors on basophils and mast cells. Anaesthesia 61, 1170–1174. doi: 10.1111/j.1365-2044.2006.04870.x
Tack, J., Caenepeel, P., Fischler, B., Piessevaux, H., and Janssens, J. (2001). Symptoms associated with hypersensitivity to gastric distention in functional dyspepsia. Gastroenterology 121, 526–535. doi: 10.1053/gast.2001.27180
Tack, J., Demedts, I., Dehondt, G., Caenepeel, P., Fischler, B., Zandecki, M., et al. (2002). Clinical and pathophysiological characteristics of acute-onset functional dyspepsia. Gastroenterology 122, 1738–1747. doi: 10.1053/gast.2002.33663
Tack, J., Piessevaux, H., Coulie, B., Caenepeel, P., and Janssens, J. (1998). Role of impaired gastric accommodation to a meal in functional dyspepsia. Gastroenterology 115, 1346–1352. doi: 10.1016/s0016-5085(98)70012-5
Talley, N. J. (2020). What causes functional gastrointestinal disorders? a proposed disease model. Am. J. Gastroenterol. 115, 41–48. doi: 10.14309/ajg.0000000000000485
Traina, G. (2019). Mast cells in gut and brain and their potential role as an emerging therapeutic target for neural diseases. Front. Cell Neurosci. 13:345. doi: 10.3389/fncel.2019.00345
Travagli, R. A., and Anselmi, L. (2016). Vagal neurocircuitry and its influence on gastric motility. Nat. Rev. Gastroenterol. Hepatol. 13, 389–401. doi: 10.1038/nrgastro.2016.76
Travers, J., and Rothenberg, M. E. (2015). Eosinophils in mucosal immune responses. Mucosal. Immunol. 8, 464–475. doi: 10.1038/mi.2015.2
Troncon, L. E., Bennett, R. J., Ahluwalia, N. K., and Thompson, D. G. (1994). Abnormal intragastric distribution of food during gastric emptying in functional dyspepsia patients. Gut 35, 327–332. doi: 10.1136/gut.35.3.327
Tziatzios, G., Gkolfakis, P., Papanikolaou, I. S., Mathur, R., Pimentel, M., Giamarellos-Bourboulis, E. J., et al. (2020). Gut microbiota dysbiosis in functional dyspepsia. Microorganisms 8:691. doi: 10.3390/microorganisms8050691
Ueta, Y., Kannan, H., and Yamashita, H. (1991). Gastric afferents to the paraventricular nucleus in the rat. Exp. Brain Res. 84, 487–494. doi: 10.1007/BF00230960
Urbain, J. L., Vekemans, M. C., Parkman, H., Van Cauteren, J., Mayeur, S. M., and Van Den Maegdenbergh, V. (1995). Dynamic antral scintigraphy to characterize gastric antral motility in functional dyspepsia. J. Nucl. Med. 36, 1579–1586.
van der Kooy, D., Koda, L. Y., Mcginty, J. F., Gerfen, C. R., and Bloom, F. E. (1984). The organization of projections from the cortex, amygdala, and hypothalamus to the nucleus of the solitary tract in rat. J. Comp. Neurol. 224, 1–24. doi: 10.1002/cne.902240102
Van Oudenhove, L., and Aziz, Q. (2013). The role of psychosocial factors and psychiatric disorders in functional dyspepsia. Nat. Rev. Gastroenterol. Hepatol. 10, 158–167. doi: 10.1038/nrgastro.2013.10
Van Oudenhove, L., Vandenberghe, J., Dupont, P., Geeraerts, B., Vos, R., Dirix, S., et al. (2010). Abnormal regional brain activity during rest and (anticipated) gastric distension in functional dyspepsia and the role of anxiety: a H(2)(15)O-PET study. Am. J. Gastroenterol. 105, 913–924. doi: 10.1038/ajg.2010.39
Vanheel, H., and Farre, R. (2013). Changes in gastrointestinal tract function and structure in functional dyspepsia. Nat. Rev. Gastroenterol. Hepatol. 10, 142–149. doi: 10.1038/nrgastro.2012.255
Vanheel, H., Vicario, M., Vanuytsel, T., Van Oudenhove, L., Martinez, C., Keita, A. V., et al. (2014). Impaired duodenal mucosal integrity and low-grade inflammation in functional dyspepsia. Gut 63, 262–271. doi: 10.1136/gutjnl-2012-303857
Wang, F. B., and Powley, T. L. (2000). Topographic inventories of vagal afferents in gastrointestinal muscle. J. Comp. Neurol. 421, 302–324. doi: 10.1002/(sici)1096-9861(20000605)421:3<302::aid-cne2>3.0.co;2-n
Wang, H., Yu, M., Ochani, M., Amella, C. A., Tanovic, M., Susarla, S., et al. (2003). Nicotinic acetylcholine receptor alpha7 subunit is an essential regulator of inflammation. Nature 421, 384–388. doi: 10.1038/nature01339
Watkins, L. R., Goehler, L. E., Relton, J., Brewer, M. T., and Maier, S. F. (1995). Mechanisms of tumor necrosis factor-alpha (TNF-alpha) hyperalgesia. Brain Res. 692, 244–250. doi: 10.1016/0006-8993(95)00715-3
Watkins, L. R., Wiertelak, E. P., Goehler, L. E., Smith, K. P., Martin, D., and Maier, S. F. (1994). Characterization of cytokine-induced hyperalgesia. Brain Res. 654, 15–26. doi: 10.1016/0006-8993(94)91566-0
Wauters, L., Burns, G., Ceulemans, M., Walker, M. M., Vanuytsel, T., Keely, S., et al. (2020a). Duodenal inflammation: an emerging target for functional dyspepsia? Expert. Opin. Ther. Targets 24, 511–523. doi: 10.1080/14728222.2020.1752181
Wauters, L., Talley, N. J., Walker, M. M., Tack, J., and Vanuytsel, T. (2020b). Novel concepts in the pathophysiology and treatment of functional dyspepsia. Gut 69, 591–600. doi: 10.1136/gutjnl-2019-318536
Williams, E. K., Chang, R. B., Strochlic, D. E., Umans, B. D., Lowell, B. B., and Liberles, S. D. (2016). Sensory neurons that detect stretch and nutrients in the digestive system. Cell 166, 209–221. doi: 10.1016/j.cell.2016.05.011
Williams, R. M., Berthoud, H. R., and Stead, R. H. (1997). Vagal afferent nerve fibres contact mast cells in rat small intestinal mucosa. Neuroimmunomodulation 4, 266–270. doi: 10.1159/000097346
Witte, A. B., Hilsted, L., Holst, J. J., and Schmidt, P. T. (2016). Peptide YY3-36 and glucagon-like peptide-1 in functional dyspepsia. Secretion and role in symptom generation. Scand. J. Gastroenterol. 51, 400–409. doi: 10.3109/00365521.2015.1101780
Ye, Y., Wang, X. R., Zheng, Y., Yang, J. W., Yang, N. N., Shi, G. X., et al. (2018). Choosing an animal model for the study of functional dyspepsia. Can. J. Gastroenterol. Hepatol. 2018:1531958. doi: 10.1155/2018/1531958
Yuan, H. P., Li, Z., Zhang, Y., Li, X. P., Li, F. K., and Li, Y. Q. (2015). Anxiety and depression are associated with increased counts and degranulation of duodenal mast cells in functional dyspepsia. Int. J. Clin. Exp. Med. 8, 8010–8014.
Zagorodnyuk, V. P., and Brookes, S. J. (2000). Transduction sites of vagal mechanoreceptors in the guinea pig esophagus. J. Neurosci. 20, 6249–6255. doi: 10.1523/JNEUROSCI.20-16-06249.2000
Zagorodnyuk, V. P., Chen, B. N., and Brookes, S. J. (2001). Intraganglionic laminar endings are mechano-transduction sites of vagal tension receptors in the guinea-pig stomach. J. Physiol. 534, 255–268. doi: 10.1111/j.1469-7793.2001.00255.x
Zanos, T. P., Silverman, H. A., Levy, T., Tsaava, T., Battinelli, E., Lorraine, P. W., et al. (2018). Identification of cytokine-specific sensory neural signals by decoding murine vagus nerve activity. Proc. Natl. Acad. Sci. USA 115, E4843–E4852. doi: 10.1073/pnas.1719083115
Zhou, H., Liang, H., Li, Z. F., Xiang, H., Liu, W., and Li, J. G. (2013). Vagus nerve stimulation attenuates intestinal epithelial tight junctions disruption in endotoxemic mice through alpha7 nicotinic acetylcholine receptors. Shock 40, 144–151. doi: 10.1097/SHK.0b013e318299e9c0
Zhu, C., Zhao, L., Zhao, J., and Zhang, S. (2020). Sini San ameliorates duodenal mucosal barrier injury and lowgrade inflammation via the CRF pathway in a rat model of functional dyspepsia. Int. J. Mol. Med. 45, 53–60. doi: 10.3892/ijmm.2019.4394
Zhu, J. X., Zhu, X. Y., Owyang, C., and Li, Y. (2001). Intestinal serotonin acts as a paracrine substance to mediate vagal signal transmission evoked by luminal factors in the rat. J. Physiol. 530, 431–442. doi: 10.1111/j.1469-7793.2001.0431k.x
Keywords: functional dyspepsia, vagus nerve, gut–brain axis, vagal afferent, vagal efferent
Citation: Li H and Page AJ (2022) Altered Vagal Signaling and Its Pathophysiological Roles in Functional Dyspepsia. Front. Neurosci. 16:858612. doi: 10.3389/fnins.2022.858612
Received: 20 January 2022; Accepted: 28 March 2022;
Published: 22 April 2022.
Edited by:
Jan D. Huizinga, McMaster University, CanadaReviewed by:
Paul Forsythe, University of Alberta, CanadaZachary Cordner, Johns Hopkins Medicine, United States
Ji-Hong Chen, McMaster University, Canada
Gregory M. Holmes, The Pennsylvania State University (PSU), United States
Copyright © 2022 Li and Page. This is an open-access article distributed under the terms of the Creative Commons Attribution License (CC BY). The use, distribution or reproduction in other forums is permitted, provided the original author(s) and the copyright owner(s) are credited and that the original publication in this journal is cited, in accordance with accepted academic practice. No use, distribution or reproduction is permitted which does not comply with these terms.
*Correspondence: Hui Li, aHVpLmxpMDFAYWRlbGFpZGUuZWR1LmF1