- 1Department of Systems Pharmacology and Translational Therapeutics, Perelman School of Medicine, University of Pennsylvania, Philadelphia, PA, United States
- 2Huck Institute for Life Sciences, Pennsylvania State University, University Park, PA, United States
- 3Department of Medicine, Center for Sleep and Circadian Neurobiology, Perelman School of Medicine, University of Pennsylvania, Philadelphia, PA, United States
The opioid epidemic remains a significant healthcare problem and is attributable to over 100,000 deaths per year. Poor sleep increases sensitivity to pain, impulsivity, inattention, and negative affect, all of which might perpetuate drug use. Opioid users have disrupted sleep during drug use and withdrawal and report poor sleep as a reason for relapse. However, preclinical studies investigating the relationship between sleep loss and substance use and the associated underlying neurobiological mechanisms of potential interactions are lacking. One of the most common forms of sleep loss in modern society is chronic short sleep (CSS) (<7 h/nightly for adults). Here, we used an established model of CSS to investigate the influence of disrupted sleep on opioid reward in male mice. The CSS paradigm did not increase corticosterone levels or depressive-like behavior after a single sleep deprivation session but did increase expression of Iba1, which typically reflects microglial activation, in the hypothalamus after 4 weeks of CSS. Rested control mice developed a morphine preference in a 2-bottle choice test, while mice exposed to CSS did not develop a morphine preference. Both groups demonstrated morphine conditioned place preference (mCPP), but there were no differences in conditioned preference between rested and CSS mice. Taken together, our results show that recovery sleep after chronic sleep disruption lessens voluntary opioid intake, without impacting conditioned reward associated with morphine.
Introduction
Sleep is an essential biological function that has many roles in maintaining the health of an organism, and better sleep quality is beginning to be emphasized in education and medicine (Eban-Rothschild et al., 2017; Ramar et al., 2021). The amount of time adults sleep has decreased since the 1940s, leading the CDC to classify insufficient sleep as a public health epidemic (CDC, 2015). A significant fraction (∼35%) of the US population does not get enough sleep (Liu et al., 2016). Millions of hours and over $400 billion dollars of productivity are lost each year due to the consequences of poor sleep in the United States (Hafner et al., 2017). Beyond economic consequences, the epidemic of poor sleep is concerning as there is an association between sleep duration and risk of death, with those that do not get the recommended 7–9 h of sleep per night at higher mortality risk (Vgontzas et al., 2010; Watson et al., 2015). In addition to risk of death, poor sleep has a plethora of adverse effects on social and emotional behavior (Walker and van der Helm, 2009; Van Der Helm et al., 2010; Gujar et al., 2011; Ben Simon and Walker, 2018), cognitive performance (Dinges, 2005; Walker, 2008), cardiovascular function (Tall and Jelic, 2019; Ahmad and Didia, 2020), as well as analgesic response (Roehrs et al., 2006; Krause et al., 2019) among others.
One adverse consequence of poor sleep is its association with substance use disorders. Specifically, sleep deprivation can increase the vulnerability for drug use (Brower and Perron, 2010). Adolescents are particularly vulnerable to short sleep influencing later drug use (Roane and Taylor, 2008; Sivertsen et al., 2015; Logan et al., 2018). Sleep problems are a predictor of relapse to opioid use in opioid dependent patients in the month after detoxification (Dijkstra et al., 2008). Poor sleep quality is associated with opioid craving in patients going through treatment for opioid dependence in a residential setting (Lydon-Staley et al., 2017). Subjects from inpatient and outpatient settings report poor sleep as a reason for relapse to opioid use (Maulik et al., 2002). These clinical studies highlight the importance of sleep quality as it relates to future opioid use. However, to our knowledge, the impact of chronic sleep disruption on opioid use and reward has not been tested in a controlled preclinical experiment.
Opioid signaling in the brain is influenced by sleep deprivation. Early studies on 72 h REM sleep deprivation generated a short wakefulness period when the rat is placed back in its home cage. The opioid receptor antagonist naloxone decreased the latency to sleep during this period while morphine extended the wake period (Fratta et al., 1987). Sleep deprived rats show decreased mu opioid receptor densities in the limbic system compared to controls (Fadda et al., 1991). Ten days of sleep deprivation in rats increases gene expression of the endogenous opioids proenkephalin and prodynorphin in the striatum (Baldo et al., 2013). Acute sleep deprivation in mice decreased the analgesic properties of morphine in the hotplate test (Alexandre et al., 2017). In humans, worse quality sleep and decreased time sleeping are associated with increased binding potential (BPnd) from [11C] Carfentanil at the mu opioid receptor (Campbell et al., 2013). Two nights of sleep disruption (20 min awakening per hour and one random 60 min awakening) decreased the analgesic effect of morphine in healthy adult subjects (Smith et al., 2020). Taken together, these studies show a central interaction between sleep deprivation and opioid signaling.
Consequences of sleep deprivation also include neuroinflammation. Sleep deprivation for both 1 and 7 days increased the expression of Iba-1, a microglial marker, in the mouse brain (Zhu et al., 2012). Chronic short sleep (CSS), 8 h/day, 3x/week for 4 weeks increased Iba-1, GFAP, and CD68 in the CA1 region of the hippocampus (Zhu et al., 2018). 48 and 72 h of sleep deprivation produced a range of proinflammatory cytokines in the brain and blood of rats (Wadhwa et al., 2017). Sleep disruption-induced neuroinflammation may provide a convergent mechanism leading to opioid use (Cahill and Taylor, 2017).
The effects of sleep deprivation on drug use are mixed, and most evidence comes from experiments conducting acute but not chronic sleep deprivation. Six hours of sleep deprivation after conditioning and before testing did not affect morphine conditioned place preference (mCPP), but sleep deprivation after the test and before a priming session did disrupt morphine reward-related memory consolidation in CPP (Shi et al., 2011). 4 h of sleep deprivation during conditioning enhanced cocaine CPP at 3 and 8 mg/kg in mice, while 4 h sleep deprivation after conditioning enhanced CPP only at 8 mg/kg (Bjorness and Greene, 2020). Photoperiod shifting, which disrupts the normal sleep/wake cycle, blocked preference for food CPP in rats (McDonald et al., 2013). Acute sleep deprivation increased self-administration of sucrose in mice, dependent on basolateral amygdala to nucleus accumbens signaling (Wang et al., 2020). One month of constant light increased morphine preference in a two-bottle choice test compared to rats maintained on a standard light-dark schedule (Garmabi et al., 2016). Acute sleep deprivation increased the rate of lever pressing and hastened response for cocaine during progressive-ratio self-administration in low drug-taking rats (Puhl et al., 2009). 8 days of sleep restriction via the disk treadmill method did not affect low drug taking rats but high drug takers showed greater responding in fixed ratio and higher breakpoints in progressive ratio self-administration for cocaine (Puhl et al., 2013). Sleep restriction during the active/dark period, which condenses REM during the inactive/light phase decreased incubation of craving for cocaine, while increasing sleep fragmentation sped up the incubation of craving for cocaine (Chen et al., 2015). Overall, the effect of sleep deprivation on reward is mixed depending on the drug and on the type and length of sleep deprivation. More research in this area will help elucidate a better understanding of exactly how sleep deprivation contributes to drug reward.
Most individuals that have abused opioids report oral administration (McCabe et al., 2007; Katz et al., 2008). Therefore, we used a two-bottle choice method for administering morphine. Our method used a 0.2% saccharin solution in both the morphine and vehicle bottles, which generally produces a morphine preference in C57Bl6/J mice (Belknap et al., 1993), and avoids the confound of quinine influencing choice consumption more than morphine (Grim et al., 2018). We used an established model of CSS (Zhu et al., 2018) with non-invasive sleep (behavioral quiescence) recording and automated drinking sensors to assess the effect of prolonged sleep disturbance on both voluntary consumption and associative rewarding properties of morphine in male mice. This sleep deprivation did not affect corticosterone levels or affective behavior, but did increase Iba1 expression, in the hypothalamus. To confirm the impact of this sleep deprivation, we analyzed Iba1 expression, a marker of microglia, in the hypothalamus due to its role in mediating sleep/wake behavior (Saper et al., 2005), stress and endocrine response (Winsky-Sommerer, 2004), and morphine reward (Harris et al., 2007). We hypothesized that CSS would increase both voluntary consumption of and conditioned place preference for morphine. In contrast to our hypothesis, rested mice displayed morphine preference while CSS mice did not. There was no difference in morphine CPP between rested and CSS mice. These data represent, to the best of our knowledge, the first investigation of extended sleep deprivation as it relates to morphine consumption and reward in mice.
Materials and Methods
Mice
Experiments were performed on male C57BL/6NTac mice and were 7 weeks old at the start of the experiment. Mice were housed individually for the sleep analysis and the following 2-bottle choice task (Figure 1A). A separate group of mice was housed in groups of 4 for the CPP experiment. A total of 20 animals (n = 10/group) were used for the 2-bottle choice experiments. Of the mice in the 2-bottle choice, 10 were housed in the sleep sensors, (n = 5 rested/5 CSS). In the CPP experiment, a separate cohort of 24 mice were used (n = 12 per group, rested vs. CSS), which all received morphine injections during CPP. Lights were maintained on a standard 12 h/12 h light cycle with lights on at 0700 h and off at 1900 h. CPP experiments occurred between 0900 and 1400 h. Animals were provided with ad libitum food and water access. All experiments were conducted in accordance with the NIH Guide for the Care and Use of Laboratory Animals and were approved by the University of Pennsylvania’s Institutional Animal Care and Use Committee (IACUC).
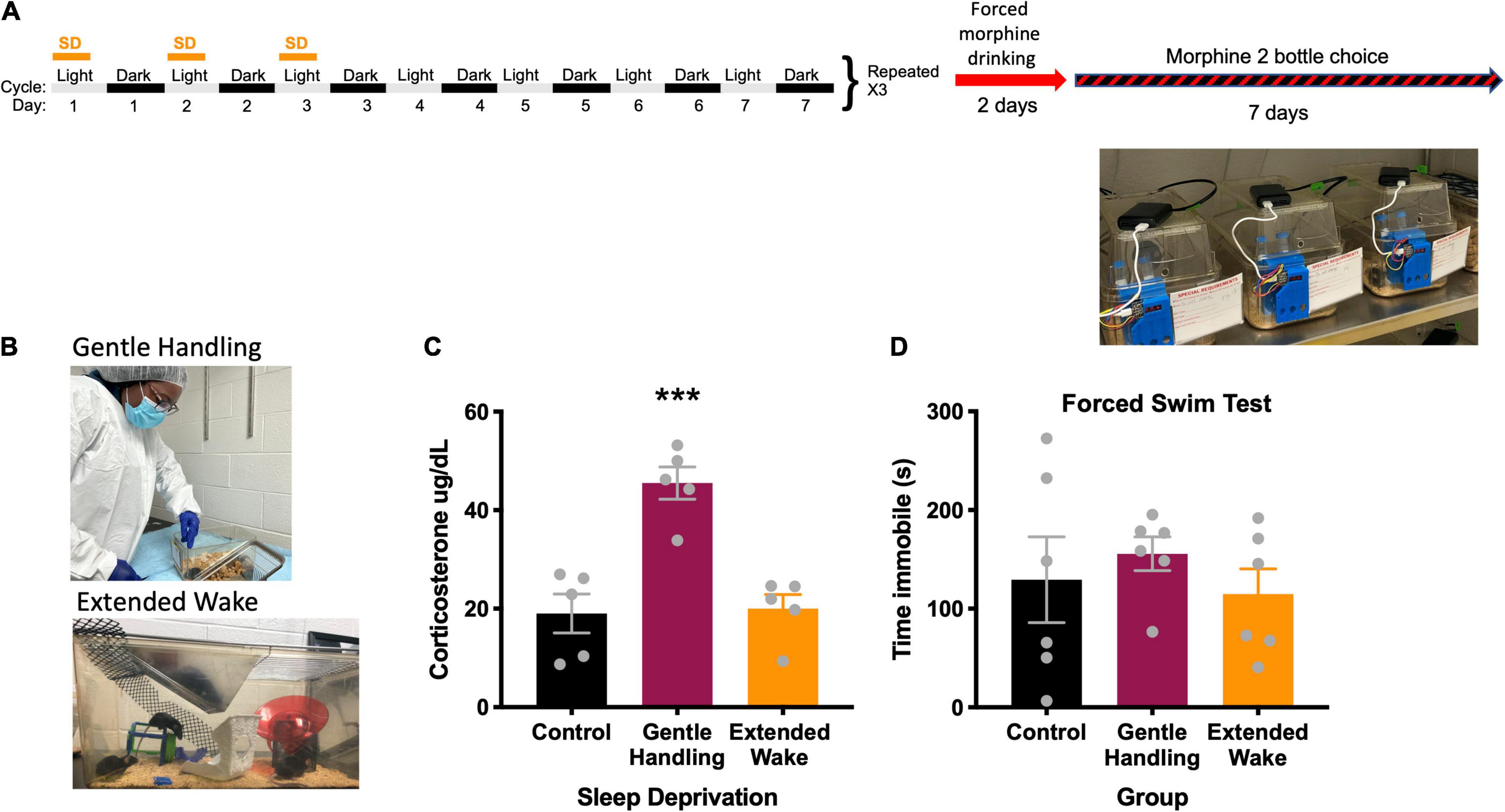
Figure 1. (A) Experimental design, (B) depiction of types of sleep deprivation, (C) radioimmunoassay of corticosterone comparing types of sleep deprivation, *** indicates p < 0.001. (D) Forced swim test comparing types of sleep deprivation.
Sleep Deprivation
We first evaluated two well-established methods of sleep deprivation, gentle-handling by direct touch and novel environment/extended wake (Figure 1B) in order to model CSS. To determine which sleep deprivation method would result in less stress, we measured serum corticosterone. In the gentle handling by direct touch method, mice were kept awake by an investigator constantly monitoring the state of the animal. When the animal appeared to be close to sleep, the investigator would open the cage and move the mouse around with a hand or paintbrush if necessary, which suppresses nearly all sleep (Franken et al., 1991), and increases corticosterone in an acute sleep deprivation (Kopp et al., 2006). Control mice in the gentle handling by direct touch experiment were in the same room but had no intervention from the experimenter. Extended wake was conducted by placing objects in a cage at different periods before the mouse falls asleep (Zhu et al., 2018). Objects included climbing wire, upside-down pencil holders, toys, and running wheels (Figure 1B). The extended wake paradigm was used for mice undergoing the 2-bottle choice and conditioned place preference studies. Here, mice were sleep deprived with the extended wake method for the first 8 h of the light cycle (0700–1500 h) on the first 3 days of 4 consecutive weeks. This method of long-term sleep deprivation via extended wake is referred to as CSS (See timeline in Figure 1A). Mice in the CSS group had standard bedding, water, and chow, with novel objects placed throughout the 8 h extended wake period. Rested control mice were placed in the same room for the first hour of the lights on period (0700–0800 h), when mice are typically awake, in order to expose them to the same room and stimuli without excessive sleep deprivation.
Corticosterone
After a single acute sleep deprivation period of either gentle handling by direct touch or extended wake, trunk blood was collected for corticosterone assessment. Control mice were in the same room as the sleep deprivation mice but were left alone experimentally. Corticosterone was collected immediately at the end of the single acute sleep deprivation session at 1,500 h. All samples were collected from all groups at the same time on the same day. Corticosterone was not collected immediately after the 4 weeks of CSS because we did not want to interfere with subsequent behavioral experiments. Blood was collected in heparin coated tubes (Sarstedt Inc., prod. No. 16.443.100, Newton, NC) and was spun at 3000 RPM for 20 min. Corticosterone concentrations in plasma samples were measured using a commercial radioimmunoassay (MP Biomedicals, Solon OH; cat. no. 07120103). Standards and samples were analyzed in singlet by an experienced researcher, blind to treatment conditions, that produces low CVs with duplicates. Assay manufacturer guidelines were followed with the exception that volumes were halved for all reagents and samples; this method produces control sample values within the expected range and intra-assay coefficients of variation below 10%. Corticosterone concentrations are expressed as μg/dl.
Forced Swim
To determine whether CSS resulted in depressive-like behaviors, we performed the forced swim test in rested and sleep deprived mice. The forced swim test was conducted on a separate group of mice that did not undergo CPP or 2-bottle choice. The FST was conducted after a single sleep deprivation session to determine the impact of sleep disruption on affective behavior that could confound interpretation of subsequent behavior experiments. Control mice were exposed to the same room as sleep deprived mice for the forced swim test but had no experimental manipulation. Plastic cylinders (23 cm × 14 cm diameter) were filled to 10 cm with 25°C water. Mice were placed in the cylinder and left for 6 min. Total immobility time for the entire 6 min period was scored by a trained observer blind to treatment conditions (Gundersen et al., 2013).
Sleep Analysis
Sleep (behavioral quiescence) was monitored non-invasively using the COMPASS system (Continuous Open Mouse Phenotyping of Activity and Sleep Status) (Brown et al., 2017) in which homecages are fixed with infrared sensors on the center of the top of the cage (Figure 1A). Activity is generated continuously (100 ms increments 100 times for a 10s bin) and results in data binned every 10s with a score between 0 and 100 depicting quantification of movement in each of the 100 increments in the 10s bin. Continuous inactivity of more than 40 s in this system correlates with EEG measures of sleep at Pearson coefficients of 0.95 or greater (Brown et al., 2017). Periods of immobility (0 activity for more than 40 s) were converted to time sleeping in python and time sleeping was plotted and analyzed for the dark cycle and light cycle separately in GraphPad Prism.
Two-Bottle Choice Test
Following the last day of the 4 weeks of CSS, mice underwent a forced administration of morphine in the drinking water (0.5 mg/mL morphine dissolved in 0.2% saccharin to neutralize the bitter taste of morphine) (Eacret et al., 2021). Pilot experiments showed that mice did not develop a preference to morphine without this 2-day morphine acclimation period (data not shown). After this 2-day forced exposure, mice were exposed to one bottle of 0.5 mg/ml morphine in 0.2% saccharin and one bottle of 0.2% saccharin alone. These liquids were in 15 mL conical tubes placed in a 3D printed lickometer with sensors under the sipper tubes to continuously monitor intake from each bottle (Godynyuk et al., 2019; Figure 1A). Mice were exposed to both morphine in saccharin and saccharin bottles for 7 days. Volume was measured manually every 24 h as well as continuously from the lickometer system and binned into average content preference every 12 h. Plots from the lickometer data were generated in SipperViz.py.1
Conditioned Place Preference
Morphine conditioned place preference (mCPP), following 4 weeks of CSS was conducted on a separate cohort of mice in order to assess context-related associations of the rewarding properties of morphine (for review, see McKendrick and Graziane, 2020). Fifteen minute pre- and post-conditioning test sessions and eight daily 30-min conditioning sessions consisting of alternating side-pairings of morphine (10 mg/kg, s.c.) and saline (0.9% saline, s.c.) were used to determine conditioned place preference (Walters et al., 2005). Mice were acclimated to the mCPP behavioral room for 1 h on two separate days prior to the CPP pre-test following the end of the 4 weeks of sleep deprivation. During the pre-test session, mice were placed in a two-chambered place preference chamber (Med Associates, inc., St. Albans, VT) inside a sound-attenuated chamber (Med Associates, inc., St. Albans, VT) and allowed to explore both sides for 15 min (900 s). The amount of time spent on each side was recorded, and data were used to divide the animals into groups with similar biases on each side. Mice were conditioned daily for 8 days after the pre-test, with one side of the box exposed on odd pairing days and the other side exposed on even pairing days. Each group received morphine (10 mg/kg: morphine sulfate, NIDA Drug Supply, Research Triangle Park, NC) on one side and saline (0.9% sodium chloride) on the other. Drug-paired sides were randomized among groups. Locomotor activities for each conditioning session were measured using two consecutive beam breaks. One day after the last conditioning session, animals were allowed to explore freely between the two sides for 15 min (900 s), and time spent on each side was recorded. The preference score (time spent on the drug-paired side minus time in the saline-paired side on the postconditioning day minus the pre-conditioning day) was calculated for each mouse.
Statistics
The statistical test was chosen based on the number and type of comparisons. For comparisons between 2 groups, t-tests were used, while a one-way ANOVA was used for groups of more than 2. For sleep studies, body weight, volume consumed, and locomotor activity over time, a two-way ANOVA with repeated measures by day was used. If a main or interaction effect occurred, Tukey’s post-hoc test was used for multiple comparisons. For continuous data, area under the curve with 95% confidence intervals (CI) were reported. F-tests were used to detect possible differences in variance between groups. The alpha value was set to 0.05 to denote statistical significance.
Results
Corticosterone, Sleep, and Body Weight Responses to Sleep Deprivation
We found that the extended wake method to prevent sleep was less stressful to mice compared to the gentle handling by direct touch method (Figure 1C). In the corticosterone assessment following both deprivation methods, there was a main effect of sleep deprivation [F(2, 12) = 19.67, p = 0.0002] (Figure 1C). Tukey’s post-hoc test revealed the gentle handling by direct touch sleep deprivation increased corticosterone compared to extended wake (p = 0.0005) and rested controls (p = 0.0004). There was no significant difference in immobility in the forced swim test following sleep deprivation [F(2, 15) = 0.4516, p = 0.6450] (Figure 1D).
During the light cycle, the sleep deprived mice got less sleep over the duration of the study [F(1, 8) = 145.7, p < 0.0001] (Figure 2A), and had significantly decreased total sleep (t = 12.07, df = 8, p < 0.0001) (Figure 2C). Tukey’s post-hoc test showed that there were no differences in sleep time during the light cycle on non-sleep deprivation days (p > 0.96). During the dark cycle, there was no main effect of sleep deprivation [F(1, 8) = 1.467, p = 0.2604), but there was a Day × CSS interaction [F(25, 200) = 5.696, p < 0.0001) (Figure 2B). Tukey’s post-hoc test revealed significant increases in recovery sleep on day 18 (p = 0.0260) and day 20 (p = 0.0165) (Figure 2B). There was no difference in total sleep time between rested mice and CSS mice in the dark cycle (t = 1.211, df = 8, p = 0.2604) (Figure 2D). There were no differences in the average bout length of sleep between rested and CSS mice throughout the study (t = 0.8246, df = 8, p = 0.4335) (Figure 2E).
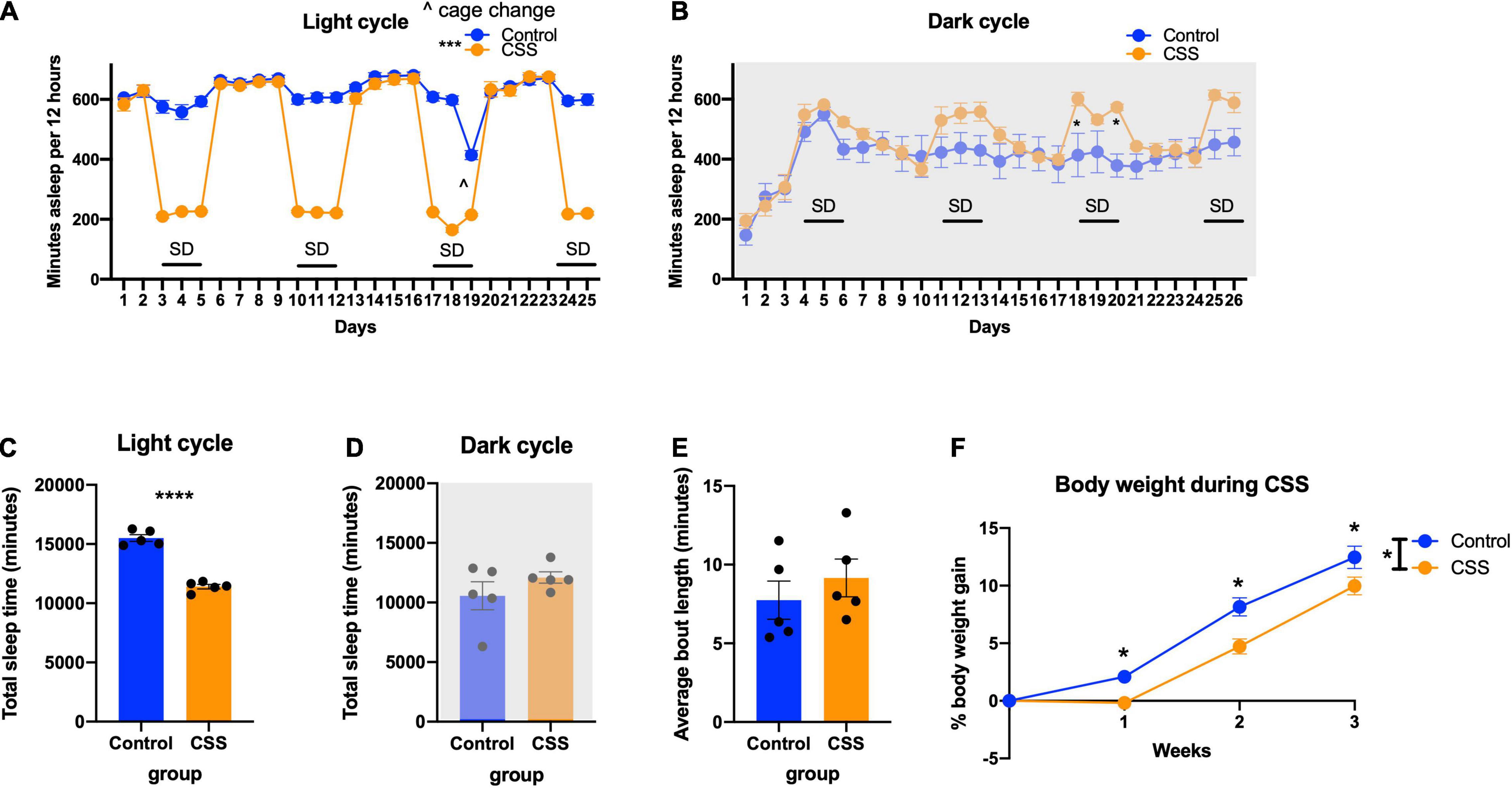
Figure 2. (A) Time sleeping per 12 h during the light (inactive) cycle each day during sleep deprivation. *** indicates p < 0.001. (B) Time sleeping per 12 h during the dark (active) cycle. * indicates p < 0.05 in Tukey’s post-hoc test. (C) Total time sleeping across the duration of the experiment in the light cycle. **** indicates p < 0.0001. (D) Total time sleeping across the duration of the experiment in the dark cycle. (E) Average bout length of sleep during the 4 weeks of sleep deprivation. (F) Body weight during the weeks of sleep deprivation. * indicates p < 0.05, ∧ indicates cage change.
CSS mice in the 2-bottle choice experiment gained less weight than rested control mice [F(1, 38) = 10.47, p = 0.0025] and showed a time x sleep interaction [F(3, 114) = 4.451, p = 0.0054] (Figure 2F). Tukey’s post-hoc test showed a significant difference between rested and CSS mice at weeks 1 (p = 0.0388), 2 (p = 0.0005), and 3 (p = 0.0191).
Microglial Responses to Chronic Short Sleep
CSS mice showed increased Iba1 expression (positive cell counts per mm2) in the hypothalamus compared to rested control mice (t = 2.182, df = 16, p = 0.0443) (Supplementary Figures 1A–C).
2-Bottle Choice
Rested control mice during the 7 days of 2-bottle choice had increased area under the curve (total area 15,203, 95%CI 13,013–17,394) for preference percentage compared to CSS mice (total area 9,554, 95%CI 6,864–12,244) (Figure 3A) indicating a greater preference for morphine in non-sleep deprived mice. Of interest, rested and CSS mice drank similar total volumes [F(1, 19, = 1.907), p = 0.1834] (Figure 3E). The preference pattern and volume measurements were manually verified every 24 h. The average grouped chronogram shows that over the 7-day experiment, the rested mice drank more morphine during the late part of the dark/active period into the early part of the light/inactive period (Figure 3B). Graphs of drink counts over 7 days further display circadian information and show less light cycle morphine drinking in control compared to CSS (Supplementary Figures 2A,B). Rested and CSS mice have a different pattern of inter-drink intervals with respect to morphine (Figures 3C,D), interpreted as rested controls drink more actively from the morphine bottle, thereby showing a higher frequency of drinks with lower time between drinks.
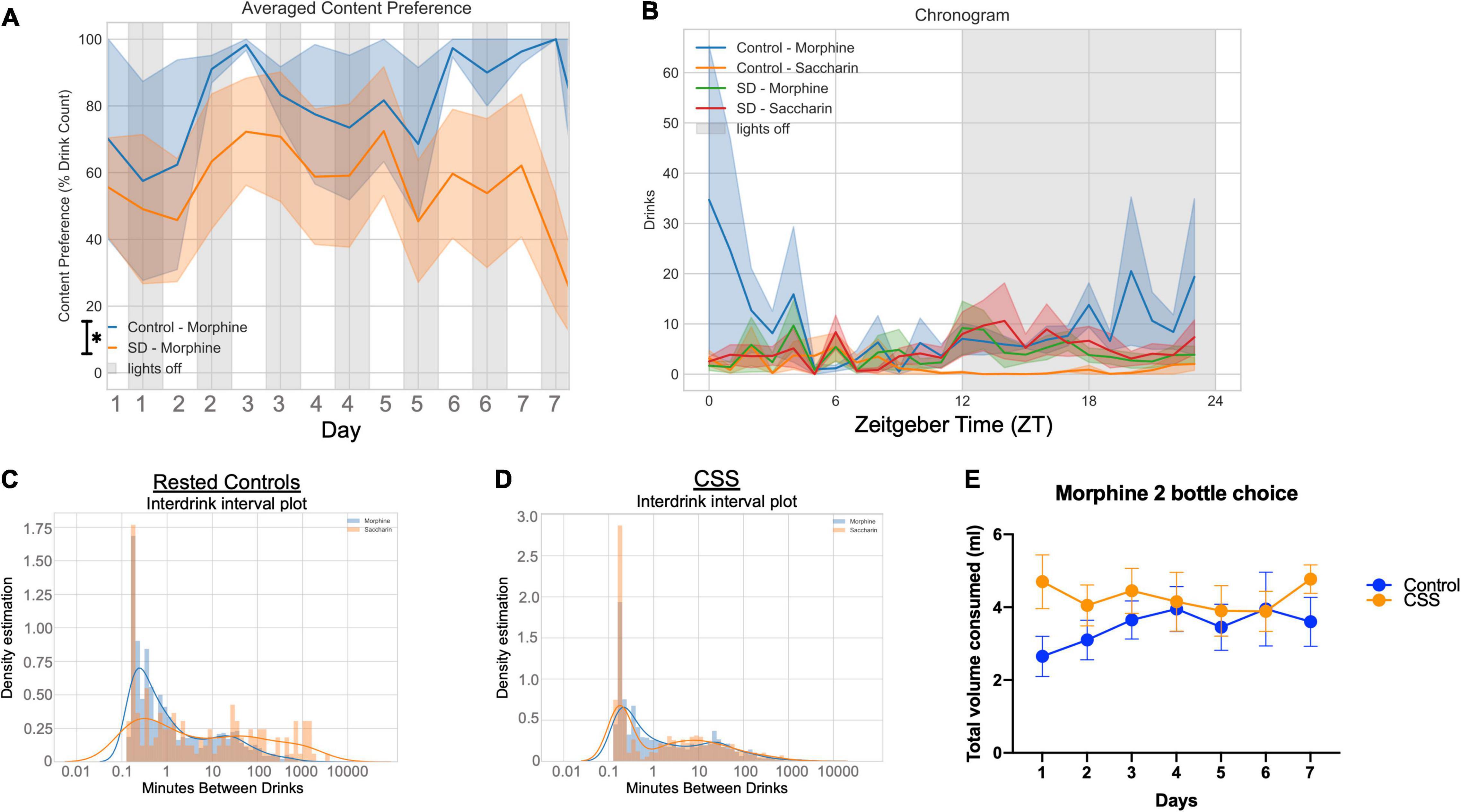
Figure 3. (A) Average content preference over 7 days between rested and chronic short sleep (CSS) groups, plotted in 12-h bins. (B) Averaged chronogram showing drinks per hour of circadian time for all 7 days. (C) Interdrink interval between morphine and saccharin for the rested control group. (D) Interdrink interval between morphine and saccharin for the chronic short sleep group. (E) Total volume consumed, measured manually, of both morphine and saccharin combined for both the rested and sleep deprived groups.
Sleep during the 2-bottle choice test was analyzed, and during the light cycle there was a trend toward increased sleep during the light cycle in CSS mice [F(1, 8) = 5.180, p = 0.0524], and a significant day x sleep interaction [F(7, 56) = 2.544, p = 0.0240] (Figure 4A). In the dark cycle during the 2-bottle choice there was no difference between rested and CSS mice [F(1, 8) = 1.402, p = 0.2704] and no day x sleep interaction [F(8, 64) = 0.6251, p = 0.7537] (Figure 4B). There was no significant difference in average sleep bout length between rested and CSS mice during the 2-bottle choice period (t = 1.690, df = 8, p = 0.1294) (Figure 4C). There was no significant difference in maximum bout length (t = 1.587, df = 8, p = 0.1511) (Figure 4D) or total bouts (t = 0.9921, df = 8, p = 0.3502) (Figure 4E).
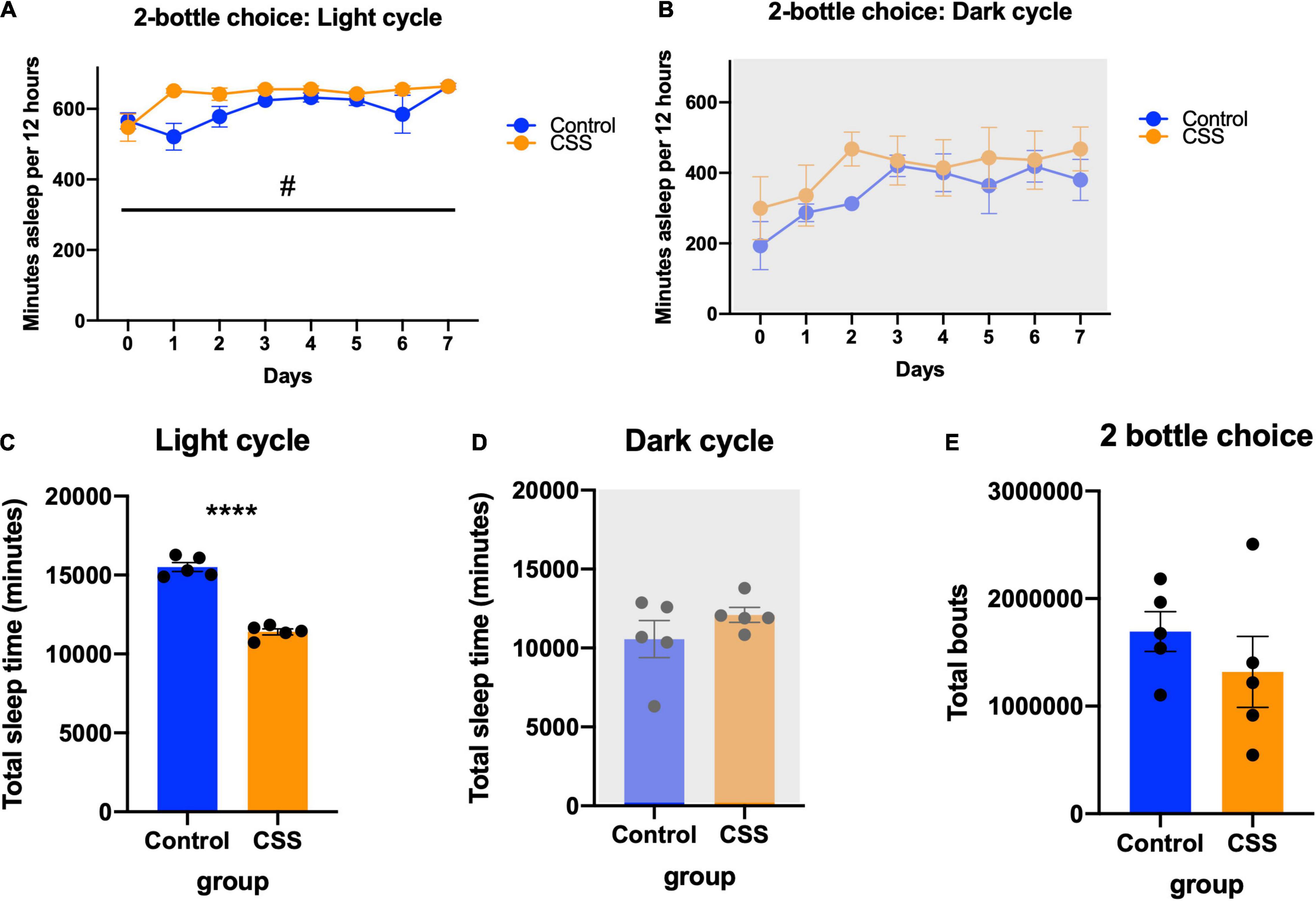
Figure 4. (A) Minutes asleep per 12 h during the light (inactive) cycle during the 2-bottle choice experiment. #Denotes significant main Day × CSS interaction effect (B) minutes asleep per 12 h during the dark (active) cycle during the 2-bottle choice experiment. (C) Average bout length compared between rested and sleep deprived mice during the 2-bottle choice experiment. (D) Maximum bout length compared between control and CSS during the 2-bottle choice experiment. (E) Total bouts of sleep during the 2-bottle choice experiment. **** indicates p < 0.0001.
Conditioned Place Preference
Mice in the sleep deprivation-CPP study, which was conducted after 4 weeks of CSS, had a non-significant trend toward decreased body weight [F(1, 22) = 3.743, p = 0.0660] (Figure 5A). In this experiment there was a week x CSS interaction effect [F(3, 66) = 4.637, p = 0.0053], and Tukey’s post-hoc test revealed a significant difference in body weight between rested and CSS at week 2 (p = 0.0065). Both groups displayed a preference for the morphine paired side of the chamber [F(1, 22) = 6.452, p = 0.0187] (Figure 5C), with no significant differences in preference score (t = 0.5258, df = 22, p = 0.6043) (Figure 5B). A 2-way repeated measures ANOVA revealed a morphine preference between pretest and test [F(1, 22) = 13.80, p = 0.0012] and an effect of subject [F(22, 22) = 2.247, p = 0.0320] (Figure 5D). Both groups showed a locomotor sensitization to morphine, with ambulation increasing over the 4 conditioning days [F(2.421, 50.83) = 29.43, p < 0.0001] (Figure 5E). There was no effect of CSS on locomotion during the conditioning days [F(1, 21) = 0.6605, p = 0.4255] (Figure 5E).
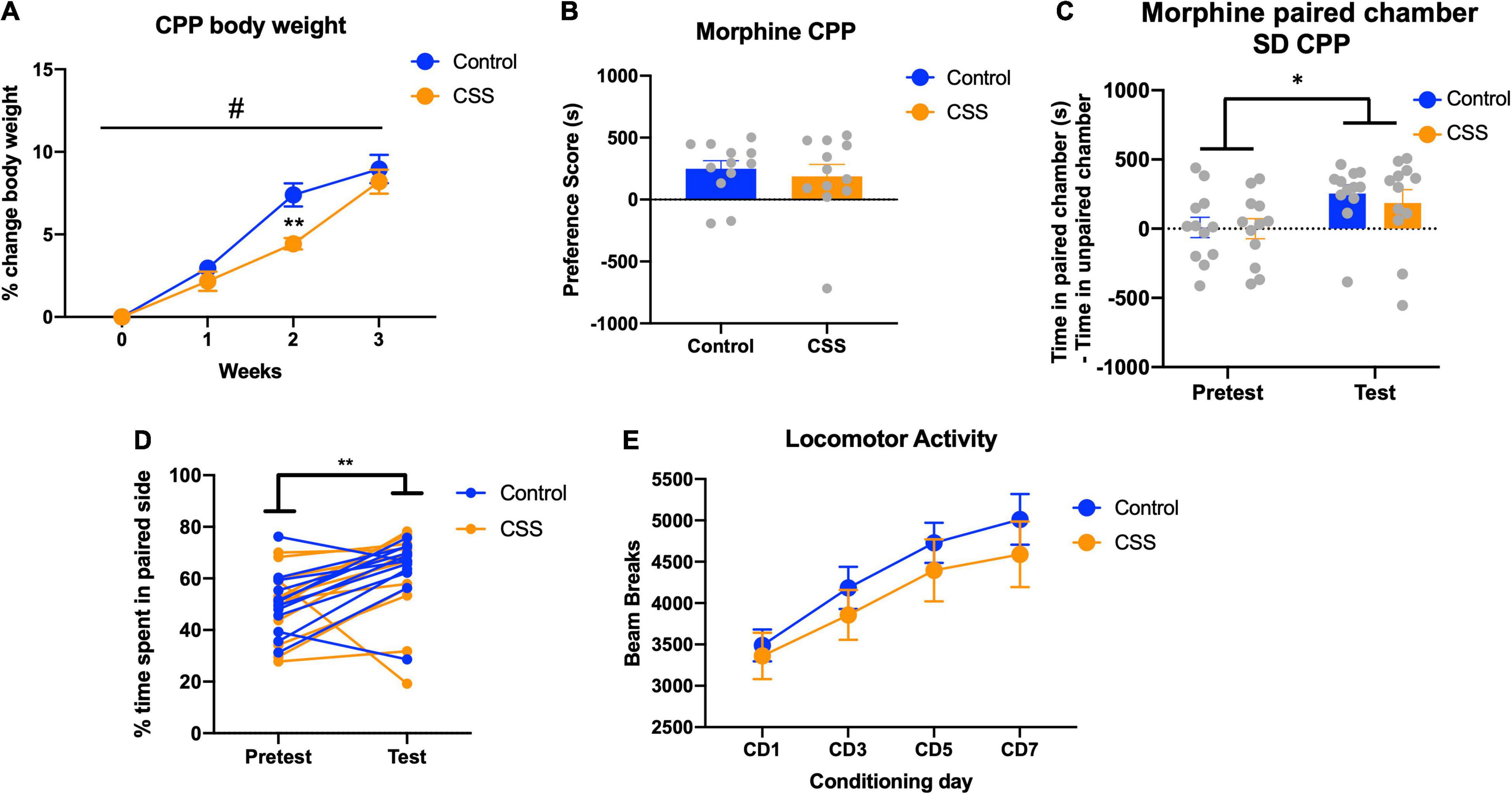
Figure 5. (A) Body weight for the animals in the CPP cohort during the 4 weeks of sleep deprivation. ** indicates p < 0.01. (B) Preference score for CPP, defined by time in the morphine-paired chamber on the post-conditioning test day of CPP minus time in the morphine-paired chamber on the pretest. (C) Time in the morphine paired chamber minus time in the saline paired chamber, for both pretest and test. (D)% of time spent on the morphine-paired side of the CPP chamber on both the pretest and posttest, repeated measures by animal. (E) Locomotor activity from the 4 days of conditioning with morphine. * indicates p < 0.05, # indicates significant main week × CSS interaction effect.
Discussion
Here, we provide the first study of long-term sleep disruption on morphine intake and reward in mice. Our long-term CSS paradigm does not increase corticosterone levels and does not change immobility in the forced swim test. There does appear to be somewhat of a bimodal distribution in the FST, highlighting individual differences in response to sleep deprivation, however the group size is not sufficient to reliably generate two distinct subpopulations. Individual differences with respect to sleep deprivation could affect recovery sleep as well as preference in the 2-bottle choice and CPP experiments. Our sleep deprivation period restricts sleep by 25% during the light cycle over the total time course of the experiment, and as NREM and REM total sleep time are associated with corticosterone regulation more strongly in the light cycle than the dark cycle (Nollet et al., 2019), sleep disruption during the normal inactive period of the animal would be more robust than sleep disruption during the active period. The lack of stress response and no changes in the FST allows us to isolate the effects of lack of sleep vs. confounds such as increased corticosterone and altered affective behaviors, which could themselves influence morphine consumption and/or conditioned reward, as stress changes the rewarding properties of drugs of abuse and causes changes in reinstatement (Will et al., 1998; Mantsch et al., 2016). That CSS mice had increased recovery sleep in the subsequent dark period after sleep loss supports effective sleep deprivation in this study, as does increased Iba1 expression in the hypothalamus.
Following exposure to CSS, we assayed two different methods of morphine reward, voluntary intake and paired association. Based on the clinical data, we hypothesized that CSS would increase vulnerability to opioid use- in our model reflected by increased morphine preference in the 2-bottle choice and increased time in the morphine-paired chamber in the CPP test. However, we found that chronic sleep disruption prevents morphine preference and does not affect conditioned reward. One interpretation of lower morphine preference in the sleep deprivation group is that less morphine is required for reward. Another is that morphine itself is less rewarding, or even becomes aversive. The CPP test was conducted to determine whether the latter interpretation is valid, yet we found that the rewarding associative properties of morphine are the same between rested and CSS mice. Of note, the dose of morphine tested in the CPP paradigm (10 mg/kg) is comparable to the dose of morphine ultimately consumed in the 2-bottle choice test; mice drank 4 mL total per day, 60% of which is morphine, which calculates to approximately 15 mg/kg/day based on a 25 g mouse. While this study examined the impact of extended sleep disruption on morphine reward, future studies should examine the impact of opioid use on sleep quality.
Advantages of the oral self-administration paradigm are that it allows us to examine how morphine exposure affects sleep without any injections or surgery, typically required in intravenous-self administration models. This is particularly important as acute opioids administered via injection can disrupt sleep but long term studies of the effects of opioids on sleep are rare (Coffey et al., 2016; Eacret et al., 2020). Further, the lickometer system used in combination with the COMPASS system allowed us to observe morphine intake throughout the circadian time course. These automated, continuous data-generating apparatuses provide for a non-biased, non-invasive, and more ethologically relevant analysis than traditional methods, which is actively promoted in the field of sleep and addiction research (Depoy et al., 2017).
Limitations of this study are that memory was not assayed in response to sleep deprivation. The CSS paradigm has been shown to decrease spatial object preference in B6 mice (Owen et al., 2021), thus, from this assay alone we cannot exclude that the animals’ ability to discern left from right and therefore morphine from saccharin, could have been influenced by sleep loss. The morphine and saccharin bottles remained on the same side during the 2-bottle choice experiment to decrease the requirement of memory in the task. Morphine has a ∼3 h half-life, so had we switched the sides of morphine and saccharin bottles it could have disrupted the animal’s true preference. In pilot experiments we found that rested mice do not develop a preference without the initial 2 days of forced morphine exposure and switching sides of the morphine or saccharin bottles disrupts preference for 1–2 days. The CSS mice did, however, show place preference for morphine and thus do demonstrate intact spatial memory at least for this assay. Another limitation is that these studies were conducted in male mice. Future studies should determine whether disrupted sleep affects vulnerability to opioid use differentially according to sex. Further, enriched environment and exercise have been shown to be neurogenic and antidepressant (Hattori et al., 2007; Kobilo et al., 2011; Nicolas et al., 2015), and our extended wake paradigm does provide an enriched environment of sorts. There was no difference in the forced swim test after extended wake, a behavioral test previously used as a screen for antidepressant efficacy (Porsolt et al., 1978; Abelaira et al., 2013), however, as with corticosterone measurements, this was conducted after a single sleep deprivation session, not following the full 4 weeks of CSS. This provided evidence that the method of sleep deprivation was not acutely stressful. Given that we do not have FST or corticosterone data after CSS, we cannot rule out that the continued and extended sleep disruption is stressful in these particular mice. However, the same paradigm of 4 weeks of CSS does not produce changes in corticosterone (Zhang et al., 2014). Sleep in this study was defined by behavioral quiescence from the non-invasive COMPASS system. While this system can interpret sleep with an accurate correlation to EEG measures of sleep (Brown et al., 2017), given that behavioral quiescence is defined as 40s + of immobility and we show ∼600 m sleep in the light cycle and ∼400 m sleep in the dark cycle, it is possible that behavioral quiescence overestimates sleep as the animal could be awake but immobile. This system was sensitive enough to show increases in recovery sleep in the dark cycle following the daytime sleep deprivation, though it lacks the ability to capture spectral power to interpret sleep intensity. Sleep deprivation can increase sleep intensity and EEG methods would be necessary to interpret sleep differences in this way (Borbély et al., 1984).
Overall, we show that recovery sleep after CSS blocks morphine preference in a voluntary intake context but not in a paired association context. This was a surprising result to us, as in humans it appears that short sleep promotes vulnerability for drug use. For that reason, we conducted an additional experiment (CPP) to either verify or inform this phenotype. We found that while CSS blocks morphine preference in a voluntary intake model, there was no difference in mCPP after CSS. It is possible that our preclinical model does not accurately reflect the human condition, in which case it is still important to know how CSS affects opioid intake in mice. It is also possible that sleep disruption is dose-dependent, and different durations of sleep disruption than that used in this experiment could provide different results. While these results are preliminary and more work needs to be done in order to elucidate the neurobiological mechanisms underlying sleep disruption-induced changes in opioid intake, this study marks a step toward creating a foundation for this realm of research. Despite correlational epidemiological findings which have been used to link sleep dysfunction and opioid use, our study found no detrimental impact of CSS on opioid reward. Future studies should focus on elucidating how to use sleep as a symptom or phenotypic marker of opioid use disorder or withdrawal. There could exist the possibility to target sleep disruption in order to ameliorate opioid use and symptoms.
Data Availability Statement
The raw data supporting the conclusions of this article will be made available by the authors, without undue reservation.
Ethics Statement
The animal study was reviewed and approved by the University of Pennsylvania Institutional Animal Care and Use Committee.
Author Contributions
DE and JB conceptualized the experimental design, with input on sleep deprivation from SV. DE and CL performed the behavioral methodology, investigated, validated the data, and carried out the analysis. JC and SC performed the radioimmunoassay. JB provided the funding acquisition and resources. DE wrote the initial draft of the manuscript. SV and JB reviewed and edited the manuscript. All authors contributed to the article and approved the submitted version.
Funding
This work was supported by the National Institutes of Health grants NIDA T32 DA028874 (to DE) and R01 DA047743 (to JB).
Conflict of Interest
The authors declare that the research was conducted in the absence of any commercial or financial relationships that could be construed as a potential conflict of interest.
Publisher’s Note
All claims expressed in this article are solely those of the authors and do not necessarily represent those of their affiliated organizations, or those of the publisher, the editors and the reviewers. Any product that may be evaluated in this article, or claim that may be made by its manufacturer, is not guaranteed or endorsed by the publisher.
Acknowledgments
We would like to thank Juliet Mengaziol, Julia Noreck, and Julia Ferrante for help with sleep deprivation and Dr. Amelia Dunn for help with programming. We would like to thank Jonathan Nofziger and Dr. Zhaolan Zhou for help with the COMPASS activity boxes and Drs. Blake Kimmey and Gregory Corder for help with 3D printing. A prototype of the 3D printed lickometer was printed courtesy of the University of Pennsylvania Libraries’ Biotech Commons.
Supplementary Material
The Supplementary Material for this article can be found online at: https://www.frontiersin.org/articles/10.3389/fnins.2022.836693/full#supplementary-material
Footnotes
References
Abelaira, H. M., Reus, G. Z., and Quevedo, J. (2013). Animal models as tools to study the pathophysiology of depression. Rev. Bras. Psiquiatr. 35, S112–S120. doi: 10.1590/1516-4446-2013-1098
Ahmad, A., and Didia, S. C. (2020). Effects of Sleep Duration on Cardiovascular Events. Curr. Cardiol. Rep. 22:18. doi: 10.1007/s11886-020-1271-0
Alexandre, C., Latremoliere, A., Ferreira, A., Miracca, G., Yamamoto, M., Scammell, T. E., et al. (2017). Decreased alertness due to sleep loss increases pain sensitivity in mice. Nat. Med. 23, 768–774. doi: 10.1038/nm.4329
Baldo, B. A., Hanlon, E. C., Obermeyer, W., Bremer, Q., Paletz, E., and Benca, R. M. (2013). Upregulation of Gene Expression in Reward-Modulatory Striatal Opioid Systems by Sleep Loss. Neuropsychopharmacology 38, 2578–2587. doi: 10.1038/npp.2013.174
Belknap, J. K., Crabbe, J. C., Riggan, J., and O’Toole, L. A. (1993). Voluntary consumption of morphine in 15 inbred mouse strains. Psychopharmacology 112, 352–358. doi: 10.1007/BF02244932
Ben Simon, E., and Walker, M. P. (2018). Sleep loss causes social withdrawal and loneliness. Nat. Commun. 9:3146. doi: 10.1038/s41467-018-05377-0
Bjorness, T. E., and Greene, R. W. (2020). Sleep deprivation enhances cocaine conditioned place preference in an orexin receptor-modulated manner. eNeuro 7:ENEURO.0283-20.2020. doi: 10.1523/ENEURO.0283-20.2020
Borbély, A. A., Tobler, I., and Hanagasioglu, M. (1984). Effect of sleep deprivation on sleep and EEG power spectra in the rat. Behav. Brain Res. 14, 171–182. doi: 10.1016/0166-4328(84)90186-4
Brower, K. J., and Perron, B. E. (2010). Sleep disturbance as a universal risk factor for relapse in addictions to psychoactive substances. Med. Hypotheses 74, 928–933. doi: 10.1016/j.mehy.2009.10.020
Brown, L. A., Hasan, S., Foster, R. G., and Peirson, S. N. (2017). COMPASS: continuous open mouse phenotyping of activity and sleep status. Wellcome Open Res. 1:2. doi: 10.12688/wellcomeopenres.9892.1
Cahill, C. M., and Taylor, A. M. (2017). Neuroinflammation—a co-occurring phenomenon linking chronic pain and opioid dependence. Curr. Opin. Behav. Sci. 13, 171–177. doi: 10.1016/j.cobeha.2016.12.003
Campbell, C. M., Bounds, S. C., Kuwabara, H., Edwards, R. R., Campbell, J. N., Haythornthwaite, J. A., et al. (2013). Individual variation in sleep quality and duration is related to cerebral Mu opioid receptor binding potential during tonic laboratory pain in healthy subjects. Pain Med. 14, 1882–1892. doi: 10.1111/pme.12231
CDC (2015). Insufficient Sleep Is a Public Health Problem. cdc.gov. Atlanta: Centers for Disease Control and Prevention.
Chen, B., Wang, Y., Liu, X., Liu, Z., Dong, Y., and Huang, Y. H. (2015). Sleep regulates incubation of cocaine craving. J. Neurosci. 35, 13300–13310. doi: 10.1523/JNEUROSCI.1065-15.2015
Coffey, A. A., Guan, Z., Grigson, P. S., and Fang, J. (2016). Reversal of the sleep–wake cycle by heroin self-administration in rats. Brain Res. Bull. 123, 33–46. doi: 10.1016/J.BRAINRESBULL.2015.09.008
Depoy, L. M., Mcclung, C. A., and Logan, R. W. (2017). Neural Mechanisms of Circadian Regulation of Natural and Drug Reward. Neural Plast. 2017:5720842. doi: 10.1155/2017/5720842
Dijkstra, B. A. G., De Jong, C. A. J., Krabbe, P. F. M., and Van Der Staak, C. P. F. (2008). Prediction of abstinence in opioid-dependent patients. J. Addict. Med. 2, 194–201. doi: 10.1097/ADM.0b013e31818a6596
Dinges, D. (2005). Neurocognitive Consequences of Sleep Deprivation Neurocognitive Consequences of Sleep Deprivation. Semin. Neurol. 25, 117–129. doi: 10.1055/s-0029-1237117
Eacret, D., Noreck, J., and Blendy, J. A. (2021). Adenosine Monophosphate-activated Protein Kinase (AMPK) in serotonin neurons mediates select behaviors during protracted withdrawal from morphine in mice. Behav. Brain Res. 419:113688. doi: 10.1016/j.bbr.2021.113688
Eacret, D., Veasey, S. C., and Blendy, J. A. (2020). Bidirectional relationship between opioids and disrupted sleep: putative mechanisms. Mol. Pharmacol. 98, 445–453. doi: 10.1124/mol.119.119107
Eban-Rothschild, A., Giardino, W. J., and De Lecea, L. (2017). To sleep or not to sleep: neuronal and ecological insights. Curr. Opin. Neurobiol. 44, 132–138. doi: 10.1016/j.conb.2017.04.010
Fadda, P., Tortorella, A., and Fratta, W. (1991). Sleep deprivation decreases μ and δ opioid receptor binding in the rat limbic system. Neurosci. Lett. 129, 315–317. doi: 10.1016/0304-3940(91)90489-G
Franken, P., Tobler, I., and Borbély, A. A. (1991). Sleep homeostasis in the rat: simulation of the time course of EEG slow-wave activity. Neurosci. Lett. 130, 141–144. doi: 10.1016/0304-3940(91)90382-4
Fratta, W., Collu, M., Martellotta, M. C., Pichiri, M., Muntoni, F., and Gessa, G. L. (1987). Stress-induced insomnia: opioid-dopamine interactions. Eur. J. Pharmacol. 142, 437–440. doi: 10.1016/0014-2999(87)90084-7
Garmabi, B., Vousooghi, N., Vosough, M., Yoonessi, A., Bakhtazad, A., and Zarrindast, M. R. (2016). Effect of circadian rhythm disturbance on morphine preference and addiction in male rats: involvement of period genes and dopamine D1 receptor. Neuroscience 322, 104–114. doi: 10.1016/j.neuroscience.2016.02.019
Godynyuk, E., Bluitt, M. N., Tooley, J. R., Kravitz, A. V., and Creed, M. C. (2019). An open-source, automated home-cage sipper device for monitoring liquid ingestive behavior in rodents. eNeuro 6:ENEURO.0292-19.2019. doi: 10.1523/ENEURO.0292-19.2019
Grim, T. W., Park, S. J., Schmid, C. L., Laprairie, R. B., Cameron, M., and Bohn, L. M. (2018). The effect of quinine in two bottle choice procedures in C57BL6 mice: opioid preference, somatic withdrawal, and pharmacokinetic outcomes. Drug Alcohol Depend. 191, 195–202. doi: 10.1016/j.drugalcdep.2018.05.034
Gujar, N., Yoo, S. S., Hu, P., and Walker, M. P. (2011). Sleep deprivation amplifies reactivity of brain reward networks, biasing the appraisal of positive emotional experiences. J. Neurosci. 31, 4466–4474. doi: 10.1523/JNEUROSCI.3220-10.2011
Gundersen, B. B., Briand, L. A., Onksen, J. L., LeLay, J., Kaestner, K. H., and Blendy, J. A. (2013). Increased Hippocampal Neurogenesis and Accelerated Response to Antidepressants in Mice with Specific Deletion of CREB in the Hippocampus: role of cAMP Response-Element Modulator. J. Neurosci. 33, 13673–13685. doi: 10.1523/JNEUROSCI.1669-13.2013
Hafner, M., Stepanek, M., Taylor, J., Troxel, W., and Stolk, C. (2017). Why sleep matters – the economic costs of insufficient sleep: a cross-country comparative analysis. Rand Health Q. 6:11. doi: 10.7249/rr1791
Harris, G. C., Wimmer, M., Randall-Thompson, J. F., and Aston-Jones, G. (2007). Lateral hypothalamic orexin neurons are critically involved in learning to associate an environment with morphine reward. Behav. Brain Res. 183, 43–51. doi: 10.1016/j.bbr.2007.05.025
Hattori, S., Hashimoto, R., Miyakawa, T., Yamanaka, H., Maeno, H., Wada, K., et al. (2007). Enriched environments influence depression-related behavior in adult mice and the survival of newborn cells in their hippocampi. Behav. Brain Res. 180, 69–76. doi: 10.1016/j.bbr.2007.02.036
Katz, N., Fernandez, K., Chang, A., Benoit, C., and Butler, S. F. (2008). Internet-based survey of nonmedical prescription opioid use in the United States. Clin. J. Pain 24, 528–535. doi: 10.1097/AJP.0b013e318167a087
Kobilo, T., Yuan, C., and van Praag, H. (2011). Endurance factors improve hippocampal neurogenesis and spatial memory in mice. Learn. Mem. 18, 103–107. doi: 10.1101/lm.2001611
Kopp, C., Longordo, F., Nicholson, J. R., and Lüthi, A. (2006). Insufficient sleep reversibly alters bidirectional synaptic plasticity and NMDA receptor function. J. Neurosci. 26, 12456–12465. doi: 10.1523/JNEUROSCI.2702-06.2006
Krause, A. J., Prather, A. A., Wager, T. D., Lindquist, M. A., and Walker, M. P. (2019). The pain of sleep loss: a brain characterization in humans. J. Neurosci. 39, 2291–2300. doi: 10.1523/JNEUROSCI.2408-18.2018
Liu, Y., Wheaton, A. G., Chapman, D. P., Cunningham, T. J., Lu, H., and Croft, J. B. (2016). Prevalence of Healthy Sleep Duration among Adults — United States, 2014. MMWR Morb. Mortal. Wkly. Rep. 65, 137–141. doi: 10.15585/mmwr.mm6506a1
Logan, R. W., Hasler, B. P., Forbes, E. E., Franzen, P. L., Torregrossa, M. M., Huang, Y. H., et al. (2018). Impact of Sleep and Circadian Rhythms on Addiction Vulnerability in Adolescents. Biol. Psychiatry 83, 987–996. doi: 10.1016/j.biopsych.2017.11.035
Lydon-Staley, D. M., Cleveland, H. H., Huhn, A. S., Cleveland, M. J., Harris, J., Stankoski, D., et al. (2017). Daily sleep quality affects drug craving, partially through indirect associations with positive affect, in patients in treatment for nonmedical use of prescription drugs. Addict. Behav. 65, 275–282. doi: 10.1016/j.addbeh.2016.08.026
Mantsch, J. R., Baker, D. A., Funk, D., Lê, A. D., and Shaham, Y. (2016). Stress-induced reinstatement of drug seeking: 20 years of progress. Neuropsychopharmacology 41, 335–356. doi: 10.1038/npp.2015.142
Maulik, P. K., Tripathi, B. M., and Pal, H. R. (2002). Coping behaviors and relapse precipitants in opioid dependence: a study from North India. J. Subst. Abuse Treat. 22, 135–140. doi: 10.1016/S0740-5472(02)00225-8
McCabe, S. E., Cranford, J. A., Boyd, C. J., and Teter, C. J. (2007). Motives, diversion and routes of administration associated with nonmedical use of prescription opioids. Addict. Behav. 32, 562–575. doi: 10.1016/j.addbeh.2006.05.022
McDonald, R. J., Zelinski, E. L., Keeley, R. J., Sutherland, D., Fehr, L., and Hong, N. S. (2013). Multiple effects of circadian dysfunction induced by photoperiod shifts: alterations in context memory and food metabolism in the same subjects. Physiol. Behav. 118, 14–24. doi: 10.1016/j.physbeh.2013.04.010
McKendrick, G., and Graziane, N. M. (2020). Drug-Induced Conditioned Place Preference and Its Practical Use in Substance Use Disorder Research. Front. Behav. Neurosci. 14:582147. doi: 10.3389/fnbeh.2020.582147
Nicolas, S., Veyssière, J., Gandin, C., Zsürger, N., Pietri, M., Heurteaux, C., et al. (2015). Neurogenesis-independent antidepressant-like effects of enriched environment is dependent on adiponectin. Psychoneuroendocrinology 57, 72–83. doi: 10.1016/j.psyneuen.2015.03.017
Nollet, M., Hicks, H., McCarthy, A. P., Wu, H., Möller-Levet, C. S., Laing, E. E., et al. (2019). REM sleep’s unique associations with corticosterone regulation, apoptotic pathways, and behavior in chronic stress in mice. Proc. Natl. Acad. Sci. U. S. A. 116, 2733–2742. doi: 10.1073/pnas.1816456116
Owen, J. E., Zhu, Y., Fenik, P., Zhan, G., Bell, P., Liu, C., et al. (2021). Late-in-life neurodegeneration after chronic sleep loss in young adult mice. Sleep 44:zsab057. doi: 10.1093/sleep/zsab057
Porsolt, R. D., Anton, G., Blavet, N., and Jalfre, M. (1978). Behavioural despair in rats: a new model sensitive to antidepressant treatments. Eur. J. Pharmacol. 47, 379–391. doi: 10.1016/0014-2999(78)90118-8
Puhl, M. D., Boisvert, M., Guan, Z., Fang, J., and Grigson, P. S. (2013). A novel model of chronic sleep restriction reveals an increase in the perceived incentive reward value of cocaine in high drug-taking rats. Pharmacol. Biochem. Behav. 109, 8–15. doi: 10.1016/j.pbb.2013.04.010
Puhl, M. D., Fang, J., and Grigson, P. S. (2009). Acute sleep deprivation increases the rate and efficiency of cocaine self-administration, but not the perceived value of cocaine reward in rats. Pharmacol. Biochem. Behav. 94, 262–270. doi: 10.1016/j.pbb.2009.09.005
Ramar, K., Malhotra, R. K., Carden, K. A., Martin, J. L., Abbasi-Feinberg, F., Aurora, R. N., et al. (2021). Sleep is essential to health: an American Academy of Sleep Medicine position statement. J. Clin. Sleep Med. 17, 2115–2119. doi: 10.5664/jcsm.9476
Roane, B. M., and Taylor, D. J. (2008). Adolescent insomnia as a risk factor for early adult depression and substance abuse. Sleep 31, 1351–1356. doi: 10.5665/sleep/31.10.1351
Roehrs, T., Hyde, M., Blaisdell, B., Greenwald, M., and Roth, T. (2006). Sleep loss and REM sleep loss are hyperalgesic. Sleep 29, 145–151. doi: 10.1093/sleep/29.2.145
Saper, C. B., Scammell, T. E., and Lu, J. (2005). Hypothalamic regulation of sleep and circadian rhythms. Nature 437, 1257–1263. doi: 10.1038/nature04284
Shi, H. S., Luo, Y. X., Xue, Y. X., Wu, P., Zhu, W. L., Ding, Z. B., et al. (2011). Effects of sleep deprivation on retrieval and reconsolidation of morphine reward memory in rats. Pharmacol. Biochem. Behav. 98, 299–303. doi: 10.1016/j.pbb.2011.01.006
Sivertsen, B., Skogen, J. C., Jakobsen, R., and Hysing, M. (2015). Sleep and use of alcohol and drug in adolescence. A large population-based study of Norwegian adolescents aged 16 to 19 years. Drug Alcohol Depend. 149, 180–186. doi: 10.1016/j.drugalcdep.2015.01.045
Smith, M. T., Mun, C. J., Remeniuk, B., Finan, P. H., Campbell, C. M., Buenaver, L. F., et al. (2020). Experimental sleep disruption attenuates morphine analgesia: findings from a randomized trial and implications for the opioid abuse epidemic. Sci. Rep. 10:20121. doi: 10.1038/s41598-020-76934-1
Tall, A. R., and Jelic, S. (2019). How broken sleep promotes cardiovascular disease. Nature 566, 329–330. doi: 10.1038/d41586-019-00393-6
Van Der Helm, E., Gujar, N., and Walker, M. P. (2010). Sleep deprivation impairs the accurate recognition of human emotions. Sleep 33, 335–342. doi: 10.1093/sleep/33.3.335
Vgontzas, A. N., Liao, D., Pejovic, S., Calhoun, S., Karataraki, M., Basta, M., et al. (2010). Insomnia with short sleep duration and mortality: the Penn State cohort. Sleep 33, 1159–1164. doi: 10.1093/sleep/33.9.1159
Wadhwa, M., Kumari, P., Chauhan, G., Roy, K., Alam, S., Kishore, K., et al. (2017). Sleep deprivation induces spatial memory impairment by altered hippocampus neuroinflammatory responses and glial cells activation in rats. J. Neuroimmunol. 312, 38–48. doi: 10.1016/j.jneuroim.2017.09.003
Walker, M. P. (2008). Cognitive consequences of sleep and sleep loss. Sleep Med. 9, S29–S34. doi: 10.1016/S1389-9457(08)70014-5
Walker, M. P., and van der Helm, E. (2009). Overnight Therapy? The Role of Sleep in Emotional Brain Processing. Psychol. Bull. 135, 731–748. doi: 10.1037/a0016570
Walters, C. L., Godfrey, M., Li, X., and Blendy, J. A. (2005). Alterations in morphine-induced reward, locomotor activity, and thermoregulation in CREB-deficient mice. Brain Res. 1032, 193–199. doi: 10.1016/j.brainres.2004.11.013
Wang, Y., Liu, Z., Cai, L., Guo, R., Dong, Y., and Huang, Y. H. (2020). A Critical Role of Basolateral Amygdala–to–Nucleus Accumbens Projection in Sleep Regulation of Reward Seeking. Biol. Psychiatry 87, 954–966. doi: 10.1016/j.biopsych.2019.10.027
Watson, N. F., Badr, M. S., Belenky, G., Bliwise, D. L., Buxton, O. M., Buysse, D., et al. (2015). Recommended amount of sleep for a healthy adult: a joint consensus statement of the American Academy of Sleep Medicine and Sleep Research Society. Sleep 38, 843–844. doi: 10.5665/sleep.4716
Will, M. J., Watkins, L. R., and Maier, S. F. (1998). Uncontrollable stress potentiates morphine’s rewarding properties. Pharmacol. Biochem. Behav. 60, 655–664. doi: 10.1016/S0091-3057(98)00027-6
Winsky-Sommerer, R. (2004). Interaction between the Corticotropin-Releasing Factor System and Hypocretins (Orexins): a Novel Circuit Mediating Stress Response. J. Neurosci. 24, 11439–11448. doi: 10.1523/JNEUROSCI.3459-04.2004
Zhang, J., Zhu, Y., Zhan, G., Fenik, P., Panossian, L., Wang, M. M., et al. (2014). Extended wakefulness: compromised metabolics in and degeneration of locus ceruleus neurons. J. Neurosci. 34, 4418–4431. doi: 10.1523/JNEUROSCI.5025-12.2014
Zhu, B., Dong, Y., Xu, Z., Gompf, H. S., Ward, S. A. P., Xue, Z., et al. (2012). Sleep disturbance induces neuroinflammation and impairment of learning and memory. Neurobiol. Dis. 48, 348–355. doi: 10.1016/j.nbd.2012.06.022
Keywords: sleep, reward, morphine, hypothalamus, preference
Citation: Eacret D, Lemchi C, Caulfield JI, Cavigelli SA, Veasey SC and Blendy JA (2022) Chronic Sleep Deprivation Blocks Voluntary Morphine Consumption but Not Conditioned Place Preference in Mice. Front. Neurosci. 16:836693. doi: 10.3389/fnins.2022.836693
Received: 15 December 2021; Accepted: 24 January 2022;
Published: 17 February 2022.
Edited by:
Rodrigo España, Drexel University, United StatesReviewed by:
Brooke Schmeichel, East Tennessee State University, United StatesTheresa Bjorness, University of Texas Southwestern Medical Center, United States
Copyright © 2022 Eacret, Lemchi, Caulfield, Cavigelli, Veasey and Blendy. This is an open-access article distributed under the terms of the Creative Commons Attribution License (CC BY). The use, distribution or reproduction in other forums is permitted, provided the original author(s) and the copyright owner(s) are credited and that the original publication in this journal is cited, in accordance with accepted academic practice. No use, distribution or reproduction is permitted which does not comply with these terms.
*Correspondence: Julie A. Blendy, YmxlbmR5QHBlbm5tZWRpY2luZS51cGVubi5lZHU=