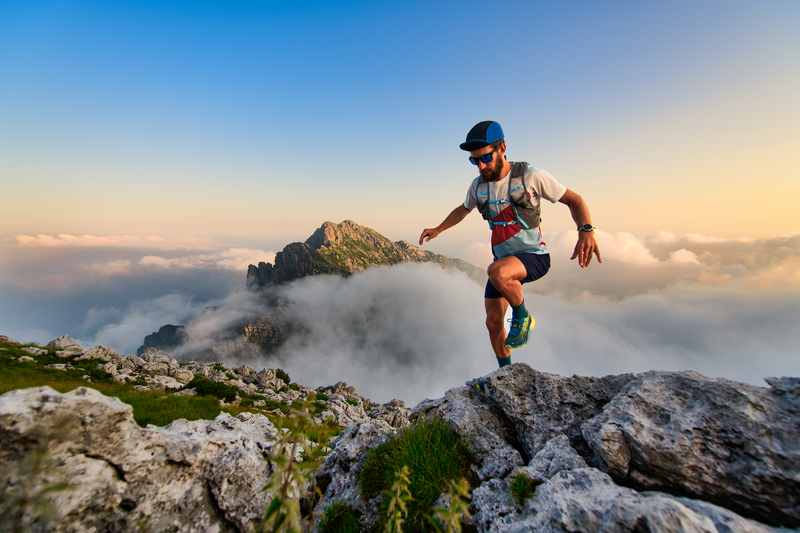
94% of researchers rate our articles as excellent or good
Learn more about the work of our research integrity team to safeguard the quality of each article we publish.
Find out more
REVIEW article
Front. Neurosci. , 31 March 2022
Sec. Neurodegeneration
Volume 16 - 2022 | https://doi.org/10.3389/fnins.2022.807473
This article is part of the Research Topic Advances in the Imaging Techniques of Radiologically subtle CNS Disorders View all 13 articles
Since 1995, more than 100 transgenic (Tg) mouse models of Alzheimer’s disease (AD) have been generated in which mutant amyloid precursor protein (APP) or APP/presenilin 1 (PS1) cDNA is overexpressed (1st generation models). Although many of these models successfully recapitulate major pathological hallmarks of the disease such as amyloid β peptide (Aβ) deposition and neuroinflammation, they have suffered from artificial phenotypes in the form of overproduced or mislocalized APP/PS1 and their functional fragments, as well as calpastatin deficiency-induced early lethality, calpain activation, neuronal cell death without tau pathology, endoplasmic reticulum stresses, and inflammasome involvement. Such artifacts bring two important uncertainties into play, these being (1) why the artifacts arise, and (2) how they affect the interpretation of experimental results. In addition, destruction of endogenous gene loci in some Tg lines by transgenes has been reported. To overcome these concerns, single App knock-in mouse models harboring the Swedish and Beyreuther/Iberian mutations with or without the Arctic mutation (AppNL–G–F and AppNL–F mice) were developed (2nd generation models). While these models are interesting given that they exhibit Aβ pathology, neuroinflammation, and cognitive impairment in an age-dependent manner, the model with the Artic mutation, which exhibits an extensive pathology as early as 6 months of age, is not suitable for investigating Aβ metabolism and clearance because the Aβ in this model is resistant to proteolytic degradation and is therefore prone to aggregation. Moreover, it cannot be used for preclinical immunotherapy studies owing to the discrete affinity it shows for anti-Aβ antibodies. The weakness of the latter model (without the Arctic mutation) is that the pathology may require up to 18 months before it becomes sufficiently apparent for experimental investigation. Nevertheless, this model was successfully applied to modulating Aβ pathology by genome editing, to revealing the differential roles of neprilysin and insulin-degrading enzyme in Aβ metabolism, and to identifying somatostatin receptor subtypes involved in Aβ degradation by neprilysin. In addition to discussing these issues, we also provide here a technical guide for the application of App knock-in mice to AD research. Subsequently, a new double knock-in line carrying the AppNL–F and Psen1P117L/WT mutations was generated, the pathogenic effect of which was found to be synergistic. A characteristic of this 3rd generation model is that it exhibits more cored plaque pathology and neuroinflammation than the AppNL–G–F line, and thus is more suitable for preclinical studies of disease-modifying medications targeting Aβ. Furthermore, a derivative AppG–F line devoid of Swedish mutations which can be utilized for preclinical studies of β-secretase modifier(s) was recently created. In addition, we introduce a new model of cerebral amyloid angiopathy that may be useful for analyzing amyloid-related imaging abnormalities that can be caused by anti-Aβ immunotherapy. Use of the App knock-in mice also led to identification of the α-endosulfine-KATP channel pathway as components of the somatostatin-evoked physiological mechanisms that reduce Aβ deposition via the activation of neprilysin. Such advances have provided new insights for the prevention and treatment of preclinical AD. Because tau pathology plays an essential role in AD pathogenesis, knock-in mice with human tau wherein the entire murine Mapt gene has been humanized were generated. Using these mice, the carboxy-terminal PDZ ligand of neuronal nitric oxide synthase (CAPON) was discovered as a mediator linking tau pathology to neurodegeneration and showed that tau humanization promoted pathological tau propagation. Finally, we describe and discuss the current status of mutant human tau knock-in mice and a non-human primate model of AD that we have successfully created.
The deposition of amyloid β peptide (Aβ) in the brain is the major pathological hallmark of Alzheimer’s disease (AD), which is considered the most common type of dementia in the world (Karran and De Strooper, 2016; Selkoe and Hardy, 2016). To date, disease-associated mutations in the presenilin 1 (PSEN1) and presenilin 2 (PSEN2) genes number more than 300, while more than 50 mutations have been reported in the amyloid precursor protein (APP) gene (Alzforum1). In response to these findings, many transgenic mouse models overexpressing mutant APP or APP/PSEN1 cDNAs have been developed (1st generation models) (Sasaguri et al., 2017), however they often suffer from experimental limitations resulting from the mislocalization of APP (Figure 1) and by the overproduction of APP fragments such as the C-terminal fragment of APP generated by β-secretase (CTF-β) and APP intracellular domain (AICD). Neither of these fragments appears to accumulate in AD brains, meaning that artificial endosomal abnormalities (Kwart et al., 2019) and transcriptional malfunctions (Nalivaeva et al., 2014), respectively, may be induced. Other overexpression artifacts include calpain activation (Saito et al., 2016), calpastatin deficiency-induced early lethality (Higuchi et al., 2012), and endoplasmic reticulum stresses (Hashimoto et al., 2018). Furthermore, it was demonstrated that the random insertion of transgene(s) resulted in the destruction of unexpectedly large regions of endogenous gene loci in the host animal (Gamache et al., 2019). We suggest that all transgenic mouse models being used in research in which APP or APP/PSEN1 are overexpressed should undergo whole genome sequencing (WGS) so that destroyed loci that possibly affect their phenotypes can be identified (Sasaguri et al., 2017).
Figure 1. Mislocalization of APP in APP-overexpressing mice. App KO mice, WT mice, APP23 (APP-overexpressing mice) and App KI mice (AppNL–F/NL–F) were subjected to immunohistochemistry using antibodies to APP, 22C11 (upper panels) and synaptophysin, a synaptic vesicle marker (lower panels) as indicated. App KO mice were used as negative controls for APP staining. While APP is selectively expressed in the axons of WT and KI mice, APP23 expresses unphysiologically high levels of APP not only in the axons but also in the soma and dendrites. The scale bar indicates 2 mm.
To overcome these drawbacks, single App knock-in mice, i.e., AppNL–G–F/NLG–F knock-in (AppNL–G–F) and AppNL–F/NL–F knock-in (AppNL–F) lines, were generated that harbor the Swedish (KM670/671NL) (Citron et al., 1992; Mullan et al., 1992) and Beyreuther/Iberian (I716F) (Lichtenthaler et al., 1999) mutations with or without the Arctic (E693G) (Nilsberth et al., 2001) mutation (2nd generation models) (Figure 2) (Saito et al., 2014; Sasaguri et al., 2017). These mice, which exhibit typical Aβ pathology, neuroinflammation and memory impairment (Saito et al., 2014; Masuda et al., 2016), are being used in more than 500 research laboratories world-wide. At present, the AppNL–G–F line is being used more frequently than the AppNL–F line given that it develops Aβ pathology approximately three times faster (Saito et al., 2014) and can be used to analyze downstream events such as neuroinflammation (Shirotani et al., 2019; Chen et al., 2020; Sobue et al., 2021), pericyte signaling (Nortley et al., 2019), oxidative stress (Hashimoto et al., 2019; Hongo et al., 2020; Uruno et al., 2020), tau propagation (Saito et al., 2019), and spatial memory impairment (Masuda et al., 2016; Jun et al., 2020; Sutoko et al., 2021; Table 1). Human Arctic mutation carriers are indistinguishable from other familial and sporadic AD patients in pathological and neurological terms except for low retention of 11C-labeled Pittsburgh compound B (PiB) in PET study (Basun et al., 2008), indicating that the mutant mice are relevant models for studying AD in general.
In addition to AppNL–F and AppNL–G–F models, App knock-in mice devoid of the Swedish mutations (AppG–F mice) have been recently developed, in which the Swedish mutations (NL) were replaced by a wild-type sequence (KM) (Figure 3 and Table 2). The AppG–F mice are more suitable for preclinical studies of β-secretase inhibition given that the Swedish mutation affects the reactivity of APP to β-site amyloid precursor protein cleaving enzyme 1 (BACE1) and most AD patients do not carry Swedish mutations (Watamura et al., 2021b).
Figure 3. AppG–F mice suitable for studies of BACE1 inhibitors. The AppG–F line is devoid of the Swedish mutation that influences the β-secretase activity and elevates the quantity of CTFβ. (The AppG–F line instead carries a wild-type sequence: KM.) The AppG–F model would be appropriate for use in preclinical studies of β-secretase inhibitors without the interference of the Swedish mutation.
Despite the advantages mentioned above, the AppNL–G–F line is not suitable for investigating the metabolism, clearance or deposition of Aβ because the Arctic mutation present in the middle of the Aβ sequence results in an Aβ that is resistant to proteolytic degradation (Tsubuki et al., 2003) and susceptible to aggregation (Nilsberth et al., 2001). Moreover, this model is not suitable for use in preclinical immunotherapy studies due to its affinity for anti-Aβ antibodies, even in the presence of guanidine hydrochloride (GuHCl) (Saito et al., 2014). The Arctic mutation may also directly or indirectly interfere with interactions between Aβ deposition and the apolipoprotein E genotype (Morishima-Kawashima et al., 2000), although there is no experimental evidence for this. In contrast, the AppNL–F line accumulates wild-type human Aβ, but it may take up to 18 months for the pathology to become sufficiently evident for investigational studies to be carried out (Saito et al., 2014), which is too long for researchers to wait in a practical sense. Therefore, a new mouse model that accumulates wild-type human Aβ as quickly as the AppNL–G–F model, but did not depend on the presence of the Arctic mutation was desired.
To achieve this, the heterozygous Psen1P117L/WT mutant line (Psen1P117L) which, of the several Psen1 mutants, exhibits the largest increase in Aβ42/Aβ40 ratio in the brain (Sasaguri et al., 2018) was utilized. The Psen1P117L line was generated by base editing technology (Komor et al., 2016). The AppNL–F mice were crossed with Psen1P117L mice, despite it being unclear whether their pathogenic effects, both of which act on the γ-cleavage of CTF-β, would be additive or not in vivo (Figure 4). The pathological phenotypes of AppNL–F mice were markedly enhanced in a synergistic manner with the Psen1P117L mutation (Sato et al., 2021), with AppNL–F X Psen1P117L/WT mice showing a more aggressive cored plaque pathology and neuroinflammation than the AppNL–G–F mice (Figure 5). These double mutant mice (3rd generation model) will likely become highly relevant tools for examining the pathologic mechanisms upstream of Aβ deposition. Moreover, these mice can be highly useful for the preclinical screening of disease-modifying therapy candidates promoting Aβ degradation or disaggregation, without the added concern associated with artificial effects caused by the Arctic mutation. We expect the double homozygous line, AppNL–F X Psen1P117L/P117L, to exhibit an even more-aggressive pathology. In any case, the AppNL–F X Psen1P117L mice are superior to the AppNL–G–F mice or the AppNL–G–I mice (Xia et al., 2021) for universal and unbiased drug screening particularly because the Aβ-degrading enzyme, neprilysin (NEP: Neutral endopeptidase), has become a therapeutic target. The AppNL–G–I mice are similarly designed as the AppNL–G–F mice, in which the Beyreuther/Iberian mutation was replaced by the Austrian mutation (Kumar-Singh et al., 2000). The characteristics of the App knock-in mouse lines are listed in Table 3.
Figure 4. Scheme of AppNL–F × Psen1P117L double-mutant mice. For the generation of the double-mutant mice, the AppNL–F line was crossbred with the Psen1P117L line whose mutation was introduced in the endogenous Psen1 gene utilizing base editing technology. The synergistic effects of the pathogenic mutations in the App and Psen1 genes strongly accelerates the deposition of wild-type human Aβ in mouse brains.
Figure 5. AD pathology in the hippocampus of a 3rd generation model mouse. A 12-month-old AppNL–F X Psen1P117L/WT mouse was analyzed by immunohistochemistry. Blue: Aβ plaques; red: microglia; green: astrocytes. The bar indicates 25 μm.
There are several precautions to be aware of to make the best use of the App knock-in mice.
A number of the App knock-in mouse users use incorrect nomenclature such as APP-NLF, APPNLF and APPNL–F instead of the AppNL–F mice, which accords with international rules of standard genomic nomenclature. Genetic names always need to be italicized.
Approximately 20 lines of mutant mice, published or unpublished, can currently or in the very near future be provided to academic and not-for-profit researchers for non-commercial research in a timely fashion with minimum restrictions (Table 2; Saito et al., 2014; Sasaguri et al., 2018). It is also recommended that scientists use optimized protocols for isolating Aβ from animal brain as previously described (Iwata et al., 2005; Figure 6). The method allows the most sensitive quantification of both soluble and insoluble Aβ with the smallest protocol deviations.
Figure 6. Outlined protocols for extraction and quantification of Aβ from tissues. See text for detailed explanation.
In most cases, the knock-in mice are used in a homozygous state to accelerate the generation of pathological and behavioral phenotypes. However, the number of recessive mutations increases over time if the breeding of mice is maintained in this way. It is necessary therefore to back-cross heterozygous mutant mice with the wild-type C57B6/J mice to remove these extraneous mutations, preferentially for 5–10 generations at an interval of 5–10 generations. Those groups dealing with poor reproductive output of mice due to their extremely inbred nature can contact RIKEN BioResource Research Center (email: YW5pbWFsLmJyY0ByaWtlbi5qcA==), a national mouse repository of Japan (Mizuno-Iijima et al., 2021), to renew their strains.
It was previously indicated that some anti-Aβ antibodies are inappropriate for biochemically and pathologically detecting the Arctic Aβ produced by AppNL–G–F mice (Saito et al., 2014; Figure 7). Note that the AppNL–G–F is the most frequently used model because it recapitulates Aβ pathology and neuroinflammation much faster than other lines. This applies to all other transgenic and knock-in mice that carry intra-Aβ mutations, including the Arctic and Dutch mutations (Van Broeckhoven et al., 1990; Li et al., 2014). In contrast, there is no restriction regarding use of antibodies for the AppNL–F X Psen1P117L mice.
Figure 7. Reactivity of different antibodies to Arctic Aβ in AppNL–G–F mice. (A) Epitope map of anti-Aβ antibodies. (B,C) Quantification of Arctic Aβ species using BNT77 as a capture antibody. BNT77 binds to the mid-portion of Aβ [see epitope map (A)]. A sandwich ELISA kit (Wako, Japan) was used to quantify Aβx-40 (C) and Aβx-42 (D), respectively. (D,E) Quantification of Arctic Aβ species using BAN50 as a capture antibody. BAN50 binds to the N-terminal region of Aβ [see epitope map (A)]. A sandwich ELISA kit (Wako, Japan) was used to quantify Aβx-40 (D) and Aβx-42 (E), respectively. BNT77 and BAN50 captured Arctic Aβ more weakly than wild-type Aβ. (F) Immunohistochemistry using various anti-Aβ antibodies. Brain sections derived from AppNL–F mice (24 months old) were immunostained using antibodies with different epitopes after antigen retrieval as indicated (upper panels); those of AppNL–G–F mice (9 months old) were similarly immunostained (lower panels). Scale bars represent 500 μm.
In our experience, the most sensitive and reproducible test involves contextual fear conditioning, although its irreversible nature can be problematic. In a more complex sense, the application of a multi-task paradigm such as IntelliCage (NewBehavior AG, Zurich, Switzerland) would be more informative (Codita et al., 2010; Masuda et al., 2016). We recently showed that the mouse genotypes can be predicted from their behavioral parameters by machine learning (Sutoko et al., 2021). It should be emphasized that the App knock-in mice are models of preclinical AD because the App knock-in mice, like all the APP and APP/PS1 transgenic mice, do not recapitulate tau pathology alone (Sasaguri et al., 2017). Consistently, we observe only mild cognitive decline in these mice. In contrast, the App knock-in mice crossbred with mutant MAPT knock-in mice exhibited accelerated tau pathology (Table 2).
Most AD patients exhibit parenchymal and vascular Aβ deposition in the brains, and both pathologies seem to be driven by impaired Aβ clearance within the interstitial fluid and perivascular drainage pathways (Greenberg et al., 2020). Iliff et al. (2012) injected fluorescent tracers into Tie2-GFP:NG2-DsRed double reporter mice, which express GFP in all cerebral blood vessels and DsRed in perivascular cells, and successfully observed glymphatic pathway; subarachnoid CSF influx into the brain parenchyma and bulk ISF solute clearance from the parenchyma within the perivascular spaces. Importantly, in AD model mice, glymphatic CSF influx is reduced and the clearance of Aβ is severely impaired (Peng et al., 2016). Impaired glymphatic pathway may contribute to the deposition of Aβ in the blood vessels of the brain, cerebral amyloid angiopathy (CAA). Although CAA is profoundly observed in most AD patients (Brenowitz et al., 2015), limited model mice, such as those with Dutch/Iowa mutation, exhibit apparent CAA, thus making it difficult to determine how CAA contributes to the pathogenesis of sporadic AD. Notably, human vascular endothelial cells express significant level of APP770 and human plasma contains ∼100 ng/ml of sAPP770 (Kitazume et al., 2010). Since peripheral blood cells other than platelet do not express APP, and platelets release sAPP770 upon their activation (Miura et al., 2020), it is considered that plasma sAPP770 is mostly derived from endothelial APP770. Because in rodents plasma sAPP is a markedly lower (∼100 pg/ml) than that of humans (Kitazume et al., 2012), it’s possible that low level of endothelial APP expression in mice could be one of the reasons that App knock-in mice exhibit mild CAA pathology. To overcome this, a mouse line that specifically expresses human APP770 in endothelial cells has just been generated (unpublished). In brief, floxed hAPP770NL mice under the CMV early enhancer/chicken β-actin promoter were first generated. These mice were then crossed with Tie2-Cre mice, in which the Tie2 promoter directs the expression of Cre recombinase in the endothelial cells to obtain double transgenic (Tg) mice.
App knock-in mouse models were previously produced by Li et al. (2014) who used multiple pathogenic mutations. These mice carry the Swedish (K670N/M671L), Dutch (E693Q), and London (V717I) mutations with the humanized Aβ sequence. The Dutch mutation results in an intensive CAA pathology in humans, thereby causing brain hemorrhage and early mortality (Levy et al., 1990; Van Broeckhoven et al., 1990). This mutation is therefore not specifically responsible for causing FAD. These mice did not develop prominent Aβ deposits over their lifespan, but when they were crossbred with Psen1M146V knock-in mice, an age-dependent deposition of Aβ was seen in the resultant double knock-in mice. The deposition of Aβ was detected not only in the parenchyma of the cerebral cortex but also in the cerebral vasculature, similar to that seen in CAA in humans. Double knock-in mice that did not have the Dutch mutation exhibited virtually no vascular pathology. In this way, if the authors had used the Beyreuther/Iberian or Austrian mutation instead of the London mutation in the mouse App gene then they probably would not have had to introduce the Psen1 knock-in mice. Knock-in mice harboring the Dutch mutation can still serve as relevant models for CAA; however they may not be appropriate for examining the effect of immunotherapy on CAA because the Dutch mutation is present in the middle of the Aβ sequence.
NEP and insulin-degrading enzyme (IDE) are considered the two major catabolic enzymes that degrade Aβ (Qiu et al., 1998; Iwata et al., 2000, 2001; Farris et al., 2003; Leissring et al., 2003). NEP is capable of degrading both soluble and insoluble Aβ (Iwata et al., 2000, 2001; Huang et al., 2006), but it is not clearly shown whether endogenous IDE could degrade insoluble Aβ in the mouse brains (Farris et al., 2003), rather IDE appears to be involved in metabolism of AICD. Unfortunately, their roles in Aβ metabolism in vivo have never been compared in an impartial and side-by-side manner. Once double mutants crossbred single App knock-in mice with NEP (Mme) KO mice and with IDE (Ide) KO mice were analyzed in detail for their biochemical properties and Aβ pathology properties, it would be clear their distinct roles in APP metabolism and the AD pathogenesis.
Further to the above, a deficiency of NEP had no significant impact on the levels of various neuropeptides (Sasaguri et al., 2018) as well as enkephalins (Saria et al., 1997) that are well known to be in vitro substrates for NEP (Turner et al., 1996, 2000, 2001; Turner and Nalivaeva, 2006) in the cerebral cortex and hippocampal formation of mice, presumably because NEP is mainly expressed in secretory vesicles and on the presynaptic membranes of excitatory neurons (Iwata et al., 2002, 2004, 2013), while most if not all neuropeptides are secreted from inhibitory neurons. This argues against the concern that NEP up-regulation for the treatment of preclinical AD would reduce the levels of these neuropeptides. These findings also indicate that NEP relatively selectively degrades Aβ in the brain. Whereas familial AD (FAD) is unambiguously caused by an increased anabolism of Aβ, and of Aβ42 and Aβ43 in particular (Selkoe and Hardy, 2016), the anabolism of Aβ appears unaffected prior to its deposition in the brain that subsequently leads to the onset of sporadic AD (SAD). These observations suggest that NEP-sensitive amyloidogenic Aβ likely plays a primary pathogenic role in the etiology of SAD. Our findings are consistent with the aging-dependent decline of NEP expression in the human brain and with recent genome-wide association studies (GWAS) indicating that variants of the gene encoding NEP (MME) are associated with the risk of SAD development (Bellenguez et al., 2020). Taken together, our results imply that the aging-associated decrease in NEP expression is a primary cause of SAD and could thus be a target for the treatment of preclinical AD once other factors such as apolipoprotein E genotypes have also been considered.
Since NEP is a major Aβ-degrading enzyme and it is downregulated upon aging, its decreased levels in the brain will most likely lead to increased Aβ levels (Yasojima et al., 2001; Carpentier et al., 2002; Iwata et al., 2002; Maruyama et al., 2005; Hellström-Lindahl et al., 2008). NEP is regulated by the neuropeptide somatostatin (Saito et al., 2005). Somatostatin, which binds to somatostatin receptors, is also decreased upon aging and in AD possibly due to loss of somatostatin-positive interneurons (Davies et al., 1980; Beal et al., 1985; Bergström et al., 1991; Hayashi et al., 1997; van de Nes et al., 2002; Lu et al., 2004; Gahete et al., 2010). Somatostatin, which was first identified to regulate secretion of growth hormone from pituitary, has been traditionally abbreviated as SRIF (somatotropin release-inhibiting factor) (Møller et al., 2003), so we will keep to this nomenclature in this review. SST1, SST2, SST3, SST4, and SST5 are used to express somatostatin receptor subtypes 1–5. Interestingly, mutations in SRIF are linked to AD (Vepsäläinen et al., 2007). By using a combination of in vitro and in vivo approaches to identify the subtype specificity of the five SSTs expressed in the brain and considered to be involved in the regulation of NEP. We would like to emphasize that it is necessary to use a co-culture system of primary neurons from the cortex, hippocampus, and striatum for in vitro experiments (Kakiya et al., 2012; Nilsson et al., 2020; Watamura et al., 2021a).
Using a battery of Sst double knockout (dKO) mice, we found that NEP is regulated by SST1 and SST4 in a redundant manner. Sst1 and Sst4 dKO mice exhibit a specific decrease of presynaptic NEP in the Lacunosum molecular layer. Moreover, a genetic deficiency of Sst1 and Sst4 in the App knock-in mice aggravated the Aβ pathology in the hippocampus. As a first proof of concept towards an Aβ-lowering strategy involving NEP, a treatment with an agonist selective for SST1 and SST4 could ameliorates the Aβ pathology and improves cognitive outcomes in the App knock-in AD mouse model as schematized in Figure 8 (Nilsson et al., 2020). These results indicate that a combination of SST1 and SST4 homodimers or the SST1 and SST4 heterodimer may become a target for pharmaceutical intervention to treat preclinical AD. Of note, the expression of SRIF in human brain declines with aging and in AD (Davies et al., 1980; Lu et al., 2004) and may causally contribute to AD pathogenesis via reduction of NEP activity/expression.
Figure 8. Somatostatin receptor subtypes 1 and 4 (SST1/4) regulate the Aβ-degrading enzyme NEP. The neuropeptide somatostatin (SRIF) was identified as a regulator of NEP activity through in vitro screening. Subsequent analysis of the effect of genetic deletion of somatostatin receptor (SST) subtypes in mice revealed that SST1 and SST4 regulate NEP in a redundant manner. This was further confirmed by concurrently deleting SST1 and SST4 in App KI mice, which aggravated the Aβ pathology. SST1/4 can be either a combination of SST1 and SST4 homodimers or an SST1/SST4 heterodimer.
Although SRIF is known to regulate Aβ catabolism by enhancing NEP-catalyzed proteolytic degradation, the mechanism by which SRIF actually regulates NEP activity is yet to be fully elucidated. Proteomic analyses enabled α-endosulfine (ENSA), an endogenous ligand of the ATP-sensitive potassium (KATP) channel, to be identified as a negative regulator of NEP downstream of SRIF signaling (Watamura et al., 2021a). The expression of ENSA is significantly increased in AD mouse models and in patients with AD. In addition, NEP directly contributes to the degradation of ENSA, suggesting a substrate-dependent feedback loop regulating NEP activity.
It was also discovered the specific KATP channel subtype [sulfonylurea receptor subunit 1 (SUR1) and inwardly rectifying K+ channel 6.2 (Kir6.2)] that modulates NEP activity, resulting in altered Aβ levels in the brain. Pharmacological intervention targeting this particular KATP channel by diazoxide attenuated Aβ deposition, with impaired memory function rescued via the NEP activation in our AD mouse model. These findings provide a mechanism explaining the molecular link between KATP channels and NEP activation. They also provide new insights into how ENSA and the KATP channel could profile as a new therapeutic target for lowering Aβ and thus provide an alternative strategy to prevent AD. Figure 9 summarizes the NEP activation mechanism that involves SRIF receptor subtypes, ENSA and KATP channel involvement.
Figure 9. Role of ENSA in the regulation of NEP activity. Schematic illustration of the mechanism describing NEP activity in the brain. ENSA, a downstream protein of SST-SST1/4 signaling, plays a role as a ligand of the KATP channel composed of sulfonylurea receptor subunit 1 (SUR1) and inwardly rectifying K+ channel 6.2 (Kir6.2), resulting in the activation of NEP. SST1/4 can be either a combination of SST1 and SST4 homodimers or an SST1/SST4 heterodimer.
To date, most if not all, mouse models of tauopathy have been unable to recapitulate the tau pathology without overexpressing mutant human tau protein. As a novel in vivo platform for studying human tauopathy, human MAPT knock-in mice have been developed in which the entire Mapt gene including all exons and introns are humanized (Hashimoto et al., 2019). In each strain, the MAPT and Mapt genes encoded human and murine tau proteins, respectively. This was done by crossing MAPT knock-in mice with single App knock-in mice in order to study the role of the Aβ-tau axis in the etiology of AD. The double knock-in mice exhibited a more pronounced tau phosphorylation status than single MAPT knock-in mice but lacked evidence of tau pathology and neurodegeneration (in a manner similar to that of single App knock-in mice) even after waiting until the mice were 24 months old.
In both the absence and presence of Aβ amyloidosis, the tau humanization has been found to significantly accelerate the propagation of AD brain-derived pathological tau (Figure 10; Saito et al., 2019). Tau accumulation was intensified in the latter case and closely associated with dystrophic neurites, consistently showing that Aβ amyloidosis affects tau pathology. These results indicated that pathological human tau interacted better with human tau than with murine tau, and suggest the presence of a species-defined preference between the pathogenic proteins. The MAPT knock-in mice also facilitate the investigation of behavioral properties and of human tau characteristics in living animal models. In addition, mutant MAPT knock-in mice carrying various pathogenic mutations have been generated (Table 2). These mice exhibit aging-dependent tau aggregation and cognitive impairment in a manner accelerated by Aβ pathology and are being provided to the research community upon request.
Figure 10. Propagation of AD-tau in mouse brains. Propagation of tau in each mouse line was observed 3 months after AD-tau injection. Brain sections were immunostained with AT8 (red). Humanization of the host animal tau affects the transmission of the pathogenic agents. AppNL–G–F/MAPT dKI mice exhibited greater pathological propagation than AppNL–G–F KI mice.
Pathological tau causes synaptic dysfunction and loss of synapses. One of promising molecules that mediates tau pathology-induced neurotoxicity is N-methyl-D-aspartate receptor (NMDAR). For example, tau accumulation disturbs synaptic plasticity through JAK2/STAT1-mediated suppression of NMDAR (Li et al., 2019). Phosphorylation of tau at Tyr18, which is mediated by the tyrosine kinase Fyn, enhances NMDAR-dependent excitotoxicity (Guo et al., 2020). Several reports indicated that glutamate-induced excitotoxicity was prevented by downmodulation of tau (Roberson et al., 2007) (Ittner et al., 2010). We also previously identified a NMDAR-related molecule as a tau binding protein which is involved in tau pathology- induced neurodegeneration.
To elucidate key molecules underlying tau accumulation-induced neurodegeneration, a comprehensive screening of tau-interacting proteins (tau interactome) was constructed. Tau-binding proteins were isolated by immunoprecipitation-LC-MS/MS (IP-MS) using a Flag-tag antibody and wild-type tau Tg (wtau-Tg) mice, which is expressing human tau tagged with a flag epitope (Kimura et al., 2007). Considering that tau is a microtubule-binding protein, we validated the methods used to generate the tau interactome by identifying the tubulin beta-4A chain as one of the tau-binding proteins.
Of the many proteins identified in the tau interactome, we focused on carboxy-terminal PDZ ligand of neuronal nitric oxide synthase (CAPON) (Hashimoto et al., 2019), which is an adaptor protein of neuronal nitric oxide synthase (nNOS). CAPON acts as an enzyme for the production of nitric oxide (NO) and is involved in NMDAR-mediated excitotoxicity (Jaffrey et al., 1998). It is thought to recruit substrates to nNOS and facilitate their NO-mediated modification through protein-protein interactions (Jaffrey et al., 1998). The presence of CAPON polymorphisms associated with schizophrenia and other psychiatric disorders has been reported in several studies (Brzustowicz, 2008; Freudenberg et al., 2015). Moreover, CAPON was shown to positively regulate spine density (Richier et al., 2010) and to regulate neuronal cell death downstream of the NMDAR (Li et al., 2013). These findings suggest that CAPON contributes to neurotransmission and neuronal excitotoxicity. In addition, one report showed that CAPON is upregulated in CA1 pyramidal cells in the AD brain (Hashimoto et al., 2012), implying that CAPON may play an important role in the pathogenesis of AD. The mechanism(s) underlying these effects nevertheless remain(s) unknown.
To further elucidate the effects of CAPON on AD pathology, we introduced CAPON cDNA into the brains of AppNL–G–F and AppNL–G–F X MAPT (hTau) double-KI mice using a newly developed adeno-associated virus (AAV)-mediated approach. We analyzed the effects of human tau protein as it is known that the hTau-KI mouse expresses an endogenous level of WT human tau. These experiments revealed that CAPON expression facilitates hippocampal atrophy in conjunction with neuronal cell death, and that a deficiency of CAPON in the P301S-Tau-Tg tauopathy mouse model suppressed tau pathology and neurodegeneration (Figure 11). From our results, an intervention in the interaction between CAPON-tau or CAPON-nNOS could be a new approach for the treatment of AD.
Figure 11. Functions of CAPON in neurodegeneration. (A) Brain sections from WT, P301S-Tau-Tg or nos1ap-/-/P301S-Tau-Tg mice stained by the conventional method using hematoxylin and eosin (H&E). A CAPON (Nos1ap) deficiency restores AD-related pathological phenotypes in P301S-Tau-Tg mice. (B) Scheme of CAPON action. Aβ pathology elevates the level and localization of CAPON in hippocampal pyramidal cells. CAPON-induced neuronal cell death is closely associated with the pathological tau protein, although there appears to be a tau-independent mechanism in play as well.
Common marmosets (marmosets, Callithrix jacchus) are small non-human primates that belong to the New World Primates (Figure 12; Mansfield, 2003). They have been increasingly utilized in neuroscience because of advantages that were observed over other research primates (Okano, 2021; Park and Sasaki, 2021). Marmosets possess physiological functions, brain structures and complex cognitive/social behaviors similar to those of humans; they communicate mainly via visual and auditory measures. In association with AD research, the amino acid sequence of Aβ in marmosets is identical to that of humans, with aged wild-type marmosets starting to accumulate Aβ from 7 years of age or even earlier (Geula et al., 2002; Rodriguez-Callejas et al., 2016). In addition, adolescent marmosets exhibit tau hyperphosphorylation, but not neurofibrillary tangle formation, in the brain that increases with aging (Rodriguez-Callejas et al., 2016). Their life spans in captivity are as long as 10–15 years, making them suitable for age-related research (Tardif et al., 2011). Their immune systems and metabolic functions resemble those of humans (t Hart and Massacesi, 2009; Tardif et al., 2011) and thus may affect the pathogenic processes related to AD (Ennerfelt and Lukens, 2020; Kellar and Craft, 2020; Rosario et al., 2020). Because sleep disorder is an early clinical symptom of AD (Pyun et al., 2019), it is noteworthy that marmosets share with humans the sleep phases composed of rapid eye movement (REM) and non-REM cycles (Crofts et al., 2001). Among various non-human primate species, the marmoset seems most applicable to genetic manipulation, i.e., generation of designed mutants, for which their high reproductive efficacy is advantageous (Sasaki et al., 2009; Sato et al., 2016; Park and Sasaki, 2021). Furthermore, fecundity characteristics of marmosets, such as a short period of sexual maturity, multiple births, and short gestation interval, are suitable for producing genetically modified disease models (Tardif et al., 2003).
Figure 12. Photograph of common marmosets (Callithrix jacchus). The photo shows members of captive common marmoset family. Their small body size, fecundity, and high cognitive functions are a suitable model for neuroscience. The photograph of marmosets was taken by WK at CIEA.
Majority of FAD-causing mutations reside in the PSEN1 gene (Scearce-Levie et al., 2020). Typically, deletion mutations in exon 9 (Crook et al., 1998; Prihar et al., 1999; Smith et al., 2001; Dumanchin et al., 2006; Le Guennec et al., 2017) or point mutations at the 3′ splice site (acceptor site) of exon 9 in the PSEN1 gene cause dominantly inherited FAD. The point mutations instigate exon 9 elimination and S290C modification in the corresponding mRNA at the junction sites of exons 8 and 10 via the conversion of alternative splicing (Hutton et al., 1996; Kwok et al., 1997; Steiner et al., 1999; Brooks et al., 2003; Blauwendraat et al., 2016). Thus, generation of a marmoset model of AD is set out in which exon 9 of the PSEN1 gene product is deleted using gene-editing technologies to produce AD marmoset models. Since TALEN exhibited high genome-editing efficacy, generates few off-target effects, and produces little mosaicism, the TALEN would be a suitable tool for producing exon 9 deletion in the PSEN1 gene (Sato et al., 2016; Zhang et al., 2019). Although it is a non-peer review data, the exon 9 deletion in the PSEN1 gene that is an AD causing mutation has been successfully introduced into non-human primates by TALEN (Sato et al., 2020).
All authors listed have made a substantial, direct, and intellectual contribution to the work, and approved it for publication.
Part of the work described was supported by the following research grants: JSPS KAKENHI Grant Number JP18K07402 (HS); JSPS KAKENHI Grant Numbers JP15K19036 and JP19K16271 and RIKEN Special Postdoctoral Research program (SH); JSPS KAKENHI Grant Number JP21K15378 (NW); AMED Grant Number JP19dm0207065 (ES), AMED Grant Number JP18am0101036 (SK); The Swedish Research Council 2018-02843 (BW); Hållsten Research Foundation (PN); JSPS KAKENHI Grant Number JP20H03564/AMED Grant Numbers JP21gm1210010s0102 and JP21dk0207050h001/JST [Moonshot R&D] Grant Number JPMJMS2024/Grant-in-aid for Research in Nagoya City University Grant Number 2021101/the Hori Sciences and Arts Foundation, and Toyoaki Scholarship Foundation (TS); AMED Grant Numbers JP16dm0107068h, JP17dm0107068h, JP18dm0107068h (NI and TS); AMED Grant Number JP15dm0207001 and RIKEN Aging Project (TCS).
The authors declare that the research was conducted in the absence of any commercial or financial relationships that could be construed as a potential conflict of interest.
All claims expressed in this article are solely those of the authors and do not necessarily represent those of their affiliated organizations, or those of the publisher, the editors and the reviewers. Any product that may be evaluated in this article, or claim that may be made by its manufacturer, is not guaranteed or endorsed by the publisher.
We thank Yukiko Nagai-Watanabe for secretarial assistance.
Barrett, T., Stangis, K. A., Saito, T., Saido, T., and Park, K. H. J. (2021). Neuronal cell cycle re-entry enhances neuropathological features in AppNLF knock-in mice. J. Alzheimers Dis. 82, 1683–1702. doi: 10.3233/jad-210091
Basun, H., Bogdanovic, N., Ingelsson, M., Almkvist, O., Naslund, J., Axelman, K., et al. (2008). Clinical and neuropathological features of the arctic APP gene mutation causing early-onset Alzheimer disease. Arch. Neurol. 65, 499–505. doi: 10.1001/archneur.65.4.499
Beal, M. F., Mazurek, M. F., Tran, V. T., Chattha, G., Bird, E. D., and Martin, J. B. (1985). Reduced numbers of somatostatin receptors in the cerebral cortex in Alzheimer’s disease. Science 229, 289–291. doi: 10.1126/science.2861661
Bellenguez, C., Küçükali, F., Jansen, I., Andrade, V., Moreno-Grau, S., Amin, N., et al. (2020). New insights on the genetic etiology of Alzheimer’s and related dementia. medRxiv [preprint] doi: 10.1101/2020.10.01.20200659
Bergström, L., Garlind, A., Nilsson, L., Alafuzoff, I., Fowler, C. J., Winblad, B., et al. (1991). Regional distribution of somatostatin receptor binding and modulation of adenylyl cyclase activity in Alzheimer’s disease brain. J. Neurol. Sci. 105, 225–233. doi: 10.1016/0022-510x(91)90149-2
Blauwendraat, C., Wilke, C., Jansen, I. E., Schulte, C., Simon-Sanchez, J., Metzger, F. G., et al. (2016). Pilot whole-exome sequencing of a German early-onset Alzheimer’s disease cohort reveals a substantial frequency of PSEN2 variants. Neurobiol. Aging 37, 208.e11–208.e17. doi: 10.1016/j.neurobiolaging.2015.09.016.
Brenowitz, W. D., Nelson, P. T., Besser, L. M., Heller, K. B., and Kukull, W. A. (2015). Cerebral amyloid angiopathy and its co-occurrence with Alzheimer’s disease and other cerebrovascular neuropathologic changes. Neurobiol. Aging 36, 2702–2708. doi: 10.1016/j.neurobiolaging.2015.06.028
Brooks, W. S., Kwok, J. B., Kril, J. J., Broe, G. A., Blumbergs, P. C., Tannenberg, A. E., et al. (2003). Alzheimer’s disease with spastic paraparesis and ‘cotton wool’ plaques: two pedigrees with PS-1 exon 9 deletions. Brain 126, 783–791. doi: 10.1093/brain/awg084
Carpentier, M., Robitaille, Y., DesGroseillers, L., Boileau, G., and Marcinkiewicz, M. (2002). Declining expression of neprilysin in Alzheimer disease vasculature: possible involvement in cerebral amyloid angiopathy. J. Neuropathol. Exp. Neurol. 61, 849–856. doi: 10.1093/jnen/61.10.849
Chen, W. T., Lu, A., Craessaerts, K., Pavie, B., Sala Frigerio, C., Corthout, N., et al. (2020). Spatial transcriptomics and in situ sequencing to study alzheimer’s disease. Cell 182, 976-991.e19. doi: 10.1016/j.cell.2020.06.038.
Chiasseu, M., Fesharaki-Zadeh, A., Saito, T., Saido, T. C., and Strittmatter, S. M. (2020). Gene-environment interaction promotes Alzheimer’s risk as revealed by synergy of repeated mild traumatic brain injury and mouse App knock-in. Neurobiol. Dis. 145:105059. doi: 10.1016/j.nbd.2020.105059
Citron, M., Oltersdorf, T., Haass, C., McConlogue, L., Hung, A. Y., Seubert, P., et al. (1992). Mutation of the beta-amyloid precursor protein in familial Alzheimer’s disease increases beta-protein production. Nature 360, 672–674. doi: 10.1038/360672a0
Codita, A., Gumucio, A., Lannfelt, L., Gellerfors, P., Winblad, B., Mohammed, A. H., et al. (2010). Impaired behavior of female tg-ArcSwe APP mice in the IntelliCage: a longitudinal study. Behav. Brain Res. 215, 83–94. doi: 10.1016/j.bbr.2010.06.034
Crofts, H. S., Wilson, S., Muggleton, N. G., Nutt, D. J., Scott, E. A., and Pearce, P. C. (2001). Investigation of the sleep electrocorticogram of the common marmoset (Callithrix jacchus) using radiotelemetry. Clin. Neurophysiol. 112, 2265–2273. doi: 10.1016/s1388-2457(01)00699-x
Crook, R., Verkkoniemi, A., Perez-Tur, J., Mehta, N., Baker, M., Houlden, H., et al. (1998). A variant of Alzheimer’s disease with spastic paraparesis and unusual plaques due to deletion of exon 9 of presenilin 1. Nat. Med. 4, 452–455. doi: 10.1038/nm0498-452
Davies, P., Katzman, R., and Terry, R. D. (1980). Reduced somatostatin-like immunoreactivity in cerebral cortex from cases of Alzheimer disease and Alzheimer senile dementa. Nature 288, 279–280. doi: 10.1038/288279a0
Dumanchin, C., Tournier, I., Martin, C., Didic, M., Belliard, S., Carlander, B., et al. (2006). Biological effects of four PSEN1 gene mutations causing Alzheimer disease with spastic paraparesis and cotton wool plaques. Hum. Mutat. 27:1063. doi: 10.1002/humu.9458
Ennerfelt, H. E., and Lukens, J. R. (2020). The role of innate immunity in Alzheimer’s disease. Immunol Rev. 297, 225–246. doi: 10.1111/imr.12896
Farris, W., Mansourian, S., Chang, Y., Lindsley, L., Eckman, E. A., Frosch, M. P., et al. (2003). Insulin-degrading enzyme regulates the levels of insulin, amyloid beta-protein, and the beta-amyloid precursor protein intracellular domain in vivo. Proc. Natl. Acad. Sci. U S A. 100, 4162–4167. doi: 10.1073/pnas.0230450100
Freudenberg, F., Alttoa, A., and Reif, A. (2015). Neuronal nitric oxide synthase (NOS1) and its adaptor, NOS1AP, as a genetic risk factors for psychiatric disorders. Genes Brain Behav. 14, 46–63. doi: 10.1111/gbb.12193
Gahete, M. D., Rubio, A., Durán-Prado, M., Avila, J., Luque, R. M., and Castaño, J. P. (2010). Expression of Somatostatin, cortistatin, and their receptors, as well as dopamine receptors, but not of neprilysin, are reduced in the temporal lobe of Alzheimer’s disease patients. J. Alzheimers. Dis. 20, 465–475. doi: 10.3233/jad-2010-1385
Gamache, J., Benzow, K., Forster, C., Kemper, L., Hlynialuk, C., Furrow, E., et al. (2019). Factors other than hTau overexpression that contribute to tauopathy-like phenotype in rTg4510 mice. Nat. Commun. 10:2479. doi: 10.1038/s41467-019-10428-1
Geula, C., Nagykery, N., and Wu, C. K. (2002). Amyloid-beta deposits in the cerebral cortex of the aged common marmoset (Callithrix jacchus): incidence and chemical composition. Acta Neuropathol. 103, 48–58. doi: 10.1007/s004010100429
Greenberg, S. M., Bacskai, B. J., Hernandez-Guillamon, M., Pruzin, J., Sperling, R., and van Veluw, S. J. (2020). Cerebral amyloid angiopathy and Alzheimer disease - one peptide, two pathways. Nat. Rev. Neurol. 16, 30–42. doi: 10.1038/s41582-019-0281-2
Guo, T., Zhang, D., Zeng, Y., Huang, T. Y., Xu, H., and Zhao, Y. (2020). Molecular and cellular mechanisms underlying the pathogenesis of Alzheimer’s disease. Mol. Neurodegener. 15:40. doi: 10.1186/s13024-020-00391-7
Hama, H., Hioki, H., Namiki, K., Hoshida, T., Kurokawa, H., Ishidate, F., et al. (2015). ScaleS: an optical clearing palette for biological imaging. Nat. Neurosci. 18, 1518–1529. doi: 10.1038/nn.4107
Hashimoto, M., Bogdanovic, N., Nakagawa, H., Volkmann, I., Aoki, M., Winblad, B., et al. (2012). Analysis of microdissected neurons by 18O mass spectrometry reveals altered protein expression in Alzheimer’s disease. J. Cell Mol. Med. 16, 1686–1700. doi: 10.1111/j.1582-4934.2011.01441.x
Hashimoto, S., Ishii, A., Kamano, N., Watamura, N., Saito, T., Ohshima, T., et al. (2018). Endoplasmic reticulum stress responses in mouse models of Alzheimer’s disease: overexpression paradigm versus knockin paradigm. J. Biol. Chem. 293, 3118–3125. doi: 10.1074/jbc.M117.811315
Hashimoto, S., Matsuba, Y., Kamano, N., Mihira, N., Sahara, N., Takano, J., et al. (2019). Tau binding protein CAPON induces tau aggregation and neurodegeneration. Nat. Commun. 10:2394. doi: 10.1038/s41467-019-10278-x
Hayashi, M., Yamashita, A., and Shimizu, K. (1997). Somatostatin and brain-derived neurotrophic factor mRNA expression in the primate brain: decreased levels of mRNAs during aging. Brain Res. 749, 283–289. doi: 10.1016/s0006-8993(96)01317-0
Hellström-Lindahl, E., Ravid, R., and Nordberg, A. (2008). Age-dependent decline of neprilysin in Alzheimer’s disease and normal brain: inverse correlation with a beta levels. Neurobiol. Aging 29, 210–221. doi: 10.1016/j.neurobiolaging.2006.10.010
Higuchi, M., Iwata, N., Matsuba, Y., Takano, J., Suemoto, T., Maeda, J., et al. (2012). Mechanistic involvement of the calpain-calpastatin system in Alzheimer neuropathology. FASEB J. 26, 1204–1217. doi: 10.1096/fj.11-187740
Hongo, N., Takamura, Y., Nishimaru, H., Matsumoto, J., Tobe, K., Saito, T., et al. (2020). Astaxanthin ameliorated parvalbumin-positive neuron deficits and Alzheimer’s disease-related pathological progression in the hippocampus of App(NL-G-F/NL-G-F) Mice. Front. Pharmacol. 11:307. doi: 10.3389/fphar.2020.00307
Huang, S. M., Mouri, A., Kokubo, H., Nakajima, R., Suemoto, T., Higuchi, M., et al. (2006). Neprilysin-sensitive synapse-associated amyloid-beta peptide oligomers impair neuronal plasticity and cognitive function. J. Biol. Chem. 281, 17941–17951. doi: 10.1074/jbc.M601372200
Hutton, M., Busfield, F., Wragg, M., Crook, R., Perez-Tur, J., Clark, R. F., et al. (1996). Complete analysis of the presenilin 1 gene in early onset Alzheimer’s disease. Neuroreport 7, 801–805. doi: 10.1097/00001756-199602290-00029
Iliff, J. J., Wang, M., Liao, Y., Plogg, B. A., Peng, W., Gundersen, G. A., et al. (2012). A paravascular pathway facilitates CSF flow through the brain parenchyma and the clearance of interstitial solutes, including amyloid β. Sci. Transl. Med. 4:147ra111. doi: 10.1126/scitranslmed.3003748
Ittner, L. M., Ke, Y. D., Delerue, F., Bi, M., Gladbach, A., van Eersel, J., et al. (2010). Dendritic function of tau mediates amyloid-beta toxicity in Alzheimer’s disease mouse models. Cell 142, 387–397. doi: 10.1016/j.cell.2010.06.036
Iwata, N., Higuchi, M., and Saido, T. C. (2005). Metabolism of amyloid-beta peptide and Alzheimer’s disease. Pharmacol. Ther. 108, 129–148. doi: 10.1016/j.pharmthera.2005.03.010
Iwata, N., Mizukami, H., Shirotani, K., Takaki, Y., Muramatsu, S., Lu, B., et al. (2004). Presynaptic localization of neprilysin contributes to efficient clearance of amyloid-beta peptide in mouse brain. J. Neurosci. 24, 991–998. doi: 10.1523/jneurosci.4792-03.2004
Iwata, N., Sekiguchi, M., Hattori, Y., Takahashi, A., Asai, M., Ji, B., et al. (2013). Global brain delivery of neprilysin gene by intravascular administration of AAV vector in mice. Sci. Rep. 3:1472. doi: 10.1038/srep01472
Iwata, N., Takaki, Y., Fukami, S., Tsubuki, S., and Saido, T. C. (2002). Region-specific reduction of a beta-degrading endopeptidase, neprilysin, in mouse hippocampus upon aging. J. Neurosci. Res. 70, 493–500. doi: 10.1002/jnr.10390
Iwata, N., Tsubuki, S., Takaki, Y., Shirotani, K., Lu, B., Gerard, N. P., et al. (2001). Metabolic regulation of brain Abeta by neprilysin. Science 292, 1550–1552. doi: 10.1126/science.1059946
Iwata, N., Tsubuki, S., Takaki, Y., Watanabe, K., Sekiguchi, M., Hosoki, E., et al. (2000). Identification of the major Abeta1-42-degrading catabolic pathway in brain parenchyma: suppression leads to biochemical and pathological deposition. Nat. Med. 6, 143–150. doi: 10.1038/72237
Jaffrey, S. R., Snowman, A. M., Eliasson, M. J., Cohen, N. A., and Snyder, S. H. (1998). CAPON: a protein associated with neuronal nitric oxide synthase that regulates its interactions with PSD95. Neuron 20, 115–124. doi: 10.1016/s0896-6273(00)80439-0
Jun, H., Bramian, A., Soma, S., Saito, T., Saido, T. C., and Igarashi, K. M. (2020). Disrupted place cell remapping and impaired grid cells in a knockin model of Alzheimer’s disease. Neuron 107, 1095-1112.e6. doi: 10.1016/j.neuron.2020.06.023.
Kakiya, N., Saito, T., Nilsson, P., Matsuba, Y., Tsubuki, S., Takei, N., et al. (2012). Cell surface expression of the major amyloid-beta peptide (A beta)-degrading enzyme, neprilysin, depends on phosphorylation by mitogen-activated protein kinase/extracellular signal-regulated kinase kinase (MEK) and dephosphorylation by protein phosphatase 1a. J. Biol. Chem. 287, 29362–29372. doi: 10.1074/jbc.M112.340372
Karran, E., and De Strooper, B. (2016). The amyloid cascade hypothesis: are we poised for success or failure? J. Neurochem. 139(Suppl. 2), 237–252. doi: 10.1111/jnc.13632
Kellar, D., and Craft, S. (2020). Brain insulin resistance in Alzheimer’s disease and related disorders: mechanisms and therapeutic approaches. Lancet Neurol. 19, 758–766. doi: 10.1016/s1474-4422(20)30231-3
Kimura, T., Yamashita, S., Fukuda, T., Park, J. M., Murayama, M., Mizoroki, T., et al. (2007). Hyperphosphorylated tau in parahippocampal cortex impairs place learning in aged mice expressing wild-type human tau. EMBO J. 26, 5143–5152. doi: 10.1038/sj.emboj.7601917
Kitazume, S., Tachida, Y., Kato, M., Yamaguchi, Y., Honda, T., Hashimoto, Y., et al. (2010). Brain endothelial cells produce amyloid {beta} from amyloid precursor protein 770 and preferentially secrete the O-glycosylated form. J. Biol. Chem. 285, 40097–40103. doi: 10.1074/jbc.M110.144626
Kitazume, S., Yoshihisa, A., Yamaki, T., Oikawa, M., Tachida, Y., Ogawa, K., et al. (2012). Soluble amyloid precursor protein 770 is released from inflamed endothelial cells and activated platelets: a novel biomarker for acute coronary syndrome. J. Biol. Chem. 287, 40817–40825. doi: 10.1074/jbc.M112.398578
Komor, A. C., Kim, Y. B., Packer, M. S., Zuris, J. A., and Liu, D. R. (2016). Programmable editing of a target base in genomic DNA without double-stranded DNA cleavage. Nature 533, 420–424. doi: 10.1038/nature17946
Kumar-Singh, S., De Jonghe, C., Cruts, M., Kleinert, R., Wang, R., Mercken, M., et al. (2000). Nonfibrillar diffuse amyloid deposition due to a gamma(42)-secretase site mutation points to an essential role for N-truncated a beta(42) in Alzheimer’s disease. Hum. Mol. Genet. 9, 2589–2598. doi: 10.1093/hmg/9.18.2589
Kwart, D., Gregg, A., Scheckel, C., Murphy, E. A., Paquet, D., Duffield, M., et al. (2019). A large panel of isogenic APP and PSEN1 mutant human iPSC neurons reveals shared endosomal abnormalities mediated by APP β-CTFs, Not Aβ. Neuron 104, 256–270.e5. doi: 10.1016/j.neuron.2019.07.010.
Kwok, J. B., Taddei, K., Hallupp, M., Fisher, C., Brooks, W. S., Broe, G. A., et al. (1997). Two novel (M233T and R278T) presenilin-1 mutations in early-onset Alzheimer’s disease pedigrees and preliminary evidence for association of presenilin-1 mutations with a novel phenotype. Neuroreport 8, 1537–1542. doi: 10.1097/00001756-199704140-00043
Le Guennec, K., Veugelen, S., Quenez, O., Szaruga, M., Rousseau, S., Nicolas, G., et al. (2017). Deletion of exons 9 and 10 of the presenilin 1 gene in a patient with early-onset Alzheimer disease generates longer amyloid seeds. Neurobiol. Dis. 104, 97–103. doi: 10.1016/j.nbd.2017.04.020
Leissring, M. A., Farris, W., Chang, A. Y., Walsh, D. M., Wu, X., Sun, X., et al. (2003). Enhanced proteolysis of beta-amyloid in APP transgenic mice prevents plaque formation, secondary pathology, and premature death. Neuron 40, 1087–1093. doi: 10.1016/s0896-6273(03)00787-6
Levy, E., Carman, M. D., Fernandez-Madrid, I. J., Power, M. D., Lieberburg, I., van Duinen, S. G., et al. (1990). Mutation of the Alzheimer’s disease amyloid gene in hereditary cerebral hemorrhage, Dutch type. Science 248, 1124–1126. doi: 10.1126/science.2111584
Li, H., Guo, Q., Inoue, T., Polito, V. A., Tabuchi, K., Hammer, R. E., et al. (2014). Vascular and parenchymal amyloid pathology in an Alzheimer disease knock-in mouse model: interplay with cerebral blood flow. Mol. Neurodegener. 9:28. doi: 10.1186/1750-1326-9-28
Li, L. L., Ginet, V., Liu, X., Vergun, O., Tuittila, M., Mathieu, M., et al. (2013). The nNOS-p38MAPK pathway is mediated by NOS1AP during neuronal death. J. Neurosci. 33, 8185–8201. doi: 10.1523/JNEUROSCI.4578-12.2013
Li, X. G., Hong, X. Y., Wang, Y. L., Zhang, S. J., Zhang, J. F., Li, X. C., et al. (2019). Tau accumulation triggers STAT1-dependent memory deficits by suppressing NMDA receptor expression. EMBO Rep. 20:e47202. doi: 10.15252/embr.201847202
Lichtenthaler, S. F., Wang, R., Grimm, H., Uljon, S. N., Masters, C. L., and Beyreuther, K. (1999). Mechanism of the cleavage specificity of Alzheimer’s disease gamma-secretase identified by phenylalanine-scanning mutagenesis of the transmembrane domain of the amyloid precursor protein. Proc. Natl. Acad. Sci. U S A. 96, 3053–3058. doi: 10.1073/pnas.96.6.3053
Lu, T., Pan, Y., Kao, S. Y., Li, C., Kohane, I., Chan, J., et al. (2004). Gene regulation and DNA damage in the ageing human brain. Nature 429, 883–891. doi: 10.1038/nature02661
Maezono, S. E. B., Kanuka, M., Tatsuzawa, C., Morita, M., Kawano, T., Kashiwagi, M., et al. (2020). Progressive changes in sleep and its relations to Amyloid-β distribution and learning in single app knock-in mice. eNeuro 7:ENEURO.0093-20.2020. doi: 10.1523/ENEURO.0093-20.2020
Maruyama, M., Higuchi, M., Takaki, Y., Matsuba, Y., Tanji, H., Nemoto, M., et al. (2005). Cerebrospinal fluid neprilysin is reduced in prodromal Alzheimer’s disease. Ann. Neurol. 57, 832–842. doi: 10.1002/ana.20494
Masuda, A., Kobayashi, Y., Kogo, N., Saito, T., Saido, T. C., and Itohara, S. (2016). Cognitive deficits in single app knock-in mouse models. Neurobiol. Learn. Mem. 135, 73–82. doi: 10.1016/j.nlm.2016.07.001
Miura, S., Yoshihisa, A., Misaka, T., Yamaki, T., Kojima, T., Toyokawa, M., et al. (2020). Amyloid precursor protein 770 is specifically expressed and released from platelets. J. Biol. Chem. 295, 13194–13201. doi: 10.1074/jbc.RA120.012904
Mizuno-Iijima, S., Nakashiba, T., Ayabe, S., Nakata, H., Ike, F., Hiraiwa, N., et al. (2021). Mouse resources at the RIKEN bioresource research center and the national bioresource project core facility in Japan. Mamm. Genome Online ahead of print. doi: 10.1007/s00335-021-09916-x
Møller, L. N., Stidsen, C. E., Hartmann, B., and Holst, J. J. (2003). Somatostatin receptors. Biochim. Biophys. Acta 1616, 1–84. doi: 10.1016/s0005-2736(03)00235-9
Morishima-Kawashima, M., Oshima, N., Ogata, H., Yamaguchi, H., Yoshimura, M., Sugihara, S., et al. (2000). Effect of apolipoprotein E allele epsilon4 on the initial phase of amyloid beta-protein accumulation in the human brain. Am. J. Pathol. 157, 2093–2099. doi: 10.1016/s0002-9440(10)64847-x
Mullan, M., Crawford, F., Axelman, K., Houlden, H., Lilius, L., Winblad, B., et al. (1992). A pathogenic mutation for probable Alzheimer’s disease in the APP gene at the N-terminus of beta-amyloid. Nat. Genet. 1, 345–347. doi: 10.1038/ng0892-345
Nagata, K., Takahashi, M., Matsuba, Y., Okuyama-Uchimura, F., Sato, K., Hashimoto, S., et al. (2018). Generation of app knock-in mice reveals deletion mutations protective against Alzheimer’s disease-like pathology. Nat. Commun. 9:1800. doi: 10.1038/s41467-018-04238-0
Nakazono, T., Lam, T. N., Patel, A. Y., Kitazawa, M., Saito, T., Saido, T. C., et al. (2017). Impaired in vivo gamma oscillations in the medial entorhinal cortex of knock-in Alzheimer model. Front. Syst. Neurosci. 11:48. doi: 10.3389/fnsys.2017.00048
Nalivaeva, N. N., Belyaev, N. D., Kerridge, C., and Turner, A. J. (2014). Amyloid-clearing proteins and their epigenetic regulation as a therapeutic target in Alzheimer’s disease. Front. Aging Neurosci. 6:235. doi: 10.3389/fnagi.2014.00235
Nilsberth, C., Westlind-Danielsson, A., Eckman, C. B., Condron, M. M., Axelman, K., Forsell, C., et al. (2001). The ‘Arctic’ APP mutation (E693G) causes Alzheimer’s disease by enhanced Abeta protofibril formation. Nat. Neurosci. 4, 887–893. doi: 10.1038/nn0901-887
Nilsson, P., Sörgjerd, K., Kakiya, N., Sasaguri, H., Watamura, N., Shimozawa, M., et al. (2020). Somatostatin receptor subtypes 1 and 4 redundantly regulate neprilysin, the major amyloid-beta degrading enzyme in brain. bioRxiv [preprint] doi: 10.1101/2020.05.09.085795
Nortley, R., Korte, N., Izquierdo, P., Hirunpattarasilp, C., Mishra, A., Jaunmuktane, Z., et al. (2019). Amyloid β oligomers constrict human capillaries in Alzheimer’s disease via signaling to pericytes. Science 365:eaav9518. doi: 10.1126/science.aav9518
Okano, H. (2021). Current status of and perspectives on the application of marmosets in neurobiology. Annu. Rev. Neurosci. 44, 27–48. doi: 10.1146/annurev-neuro-030520-101844
Park, J. E., and Sasaki, E. (2021). Assisted reproductive techniques and genetic manipulation in the common marmoset. Ilar J. Online ahead of print. doi: 10.1093/ilar/ilab002
Peng, W., Achariyar, T. M., Li, B., Liao, Y., Mestre, H., Hitomi, E., et al. (2016). Suppression of glymphatic fluid transport in a mouse model of Alzheimer’s disease. Neurobiol. Dis. 93, 215–225. doi: 10.1016/j.nbd.2016.05.015
Prihar, G., Verkkoniem, A., Perez-Tur, J., Crook, R., Lincoln, S., Houlden, H., et al. (1999). Alzheimer disease PS-1 exon 9 deletion defined. Nat. Med. 5:1090. doi: 10.1038/13383
Pyun, J. M., Kang, M. J., Yun, Y., Park, Y. H., and Kim, S. (2019). APOE ε4 and REM sleep behavior disorder as risk factors for sundown syndrome in Alzheimer’s disease. J. Alzheimers. Dis. 69, 521–528. doi: 10.3233/jad-190032
Qiu, W. Q., Walsh, D. M., Ye, Z., Vekrellis, K., Zhang, J., Podlisny, M. B., et al. (1998). Insulin-degrading enzyme regulates extracellular levels of amyloid beta-protein by degradation. J. Biol. Chem. 273, 32730–32738. doi: 10.1074/jbc.273.49.32730
Richier, L., Williton, K., Clattenburg, L., Colwill, K., O’Brien, M., Tsang, C., et al. (2010). NOS1AP associates with scribble and regulates dendritic spine development. J. Neurosci. 30, 4796–4805. doi: 10.1523/JNEUROSCI.3726-09.2010
Roberson, E. D., Scearce-Levie, K., Palop, J. J., Yan, F., Cheng, I. H., Wu, T., et al. (2007). Reducing endogenous tau ameliorates amyloid beta-induced deficits in an Alzheimer’s disease mouse model. Science 316, 750–754. doi: 10.1126/science.1141736
Rodriguez-Callejas, J. D., Fuchs, E., and Perez-Cruz, C. (2016). Evidence of tau hyperphosphorylation and dystrophic microglia in the common marmoset. Front. Aging Neurosci. 8:315. doi: 10.3389/fnagi.2016.00315
Rosario, D., Boren, J., Uhlen, M., Proctor, G., Aarsland, D., Mardinoglu, A., et al. (2020). Systems biology approaches to understand the host-microbiome interactions in neurodegenerative diseases. Front. Neurosci. 14:716. doi: 10.3389/fnins.2020.00716
Saito, T., Iwata, N., Tsubuki, S., Takaki, Y., Takano, J., Huang, S. M., et al. (2005). Somatostatin regulates brain amyloid beta peptide Abeta42 through modulation of proteolytic degradation. Nat. Med. 11, 434–439. doi: 10.1038/nm1206
Saito, T., Matsuba, Y., Mihira, N., Takano, J., Nilsson, P., Itohara, S., et al. (2014). Single App knock-in mouse models of Alzheimer’s disease. Nat. Neurosci. 17, 661–663. doi: 10.1038/nn.3697
Saito, T., Matsuba, Y., Yamazaki, N., Hashimoto, S., and Saido, T. C. (2016). Calpain activation in Alzheimer’s model mice is an artifact of APP and presenilin overexpression. J. Neurosci. 36, 9933–9936. doi: 10.1523/jneurosci.1907-16.2016
Saito, T., Mihira, N., Matsuba, Y., Sasaguri, H., Hashimoto, S., Narasimhan, S., et al. (2019). Humanization of the entire murine Mapt gene provides a murine model of pathological human tau propagation. J. Biol. Chem. 294, 12754–12765. doi: 10.1074/jbc.RA119.009487
Salobrar-García, E., López-Cuenca, I., Sánchez-Puebla, L., de Hoz, R., Fernández-Albarral, J. A., Ramírez, A. I., et al. (2020). Retinal thickness changes over time in a murine AD model APP (NL-F/NL-F). Front. Aging Neurosci. 12:625642. doi: 10.3389/fnagi.2020.625642
Saria, A., Hauser, K. F., Traurig, H. H., Turbek, C. S., Hersh, L., and Gerard, C. (1997). Opioid-related changes in nociceptive threshold and in tissue levels of enkephalins after target disruption of the gene for neutral endopeptidase (EC 3.4.24.11) in mice. Neurosci. Lett. 234, 27–30. doi: 10.1016/s0304-3940(97)00660-5
Sasaguri, H., Nagata, K., Sekiguchi, M., Fujioka, R., Matsuba, Y., Hashimoto, S., et al. (2018). Introduction of pathogenic mutations into the mouse Psen1 gene by base editor and Target-AID. Nat. Commun. 9:2892. doi: 10.1038/s41467-018-05262-w
Sasaguri, H., Nilsson, P., Hashimoto, S., Nagata, K., Saito, T., De Strooper, B., et al. (2017). APP mouse models for Alzheimer’s disease preclinical studies. EMBO J. 36, 2473–2487. doi: 10.15252/embj.201797397
Sasaki, E., Suemizu, H., Shimada, A., Hanazawa, K., Oiwa, R., Kamioka, M., et al. (2009). Generation of transgenic non-human primates with germline transmission. Nature 459, 523–527. doi: 10.1038/nature08090
Sato, K., Oiwa, R., Kumita, W., Henry, R., Sakuma, T., Ito, R., et al. (2016). Generation of a nonhuman primate model of severe combined immunodeficiency using highly efficient genome editing. Cell Stem Cell 19, 127–138. doi: 10.1016/j.stem.2016.06.003
Sato, K., Sasaguri, H., Kumita, W., Inoue, T., Kurotaki, Y., Nagata, K., et al. (2020). A non-human primate model of familial Alzheimer’s disease. bioRxiv [preprint] doi: 10.1101/2020.08.24.264259
Sato, K., Watamura, N., Fujioka, R., Mihira, N., Sekiguchi, M., Nagata, K., et al. (2021). A 3(rd) generation mouse model of Alzheimer’s disease shows early and increased cored plaque pathology composed of wild-type human amyloid β peptide. J. Biol. Chem. 297:101004. doi: 10.1016/j.jbc.2021.101004
Scearce-Levie, K., Sanchez, P. E., and Lewcock, J. W. (2020). Leveraging preclinical models for the development of Alzheimer disease therapeutics. Nat. Rev. Drug Discov. 19, 447–462. doi: 10.1038/s41573-020-0065-9
Selkoe, D. J., and Hardy, J. (2016). The amyloid hypothesis of Alzheimer’s disease at 25 years. EMBO Mol. Med. 8, 595–608. doi: 10.15252/emmm.201606210
Shirotani, K., Hori, Y., Yoshizaki, R., Higuchi, E., Colonna, M., Saito, T., et al. (2019). Aminophospholipids are signal-transducing TREM2 ligands on apoptotic cells. Sci. Rep. 9:7508. doi: 10.1038/s41598-019-43535-6
Smith, M. J., Kwok, J. B., McLean, C. A., Kril, J. J., Broe, G. A., Nicholson, G. A., et al. (2001). Variable phenotype of Alzheimer’s disease with spastic paraparesis. Ann. Neurol. 49, 125–129.
Sobue, A., Komine, O., Hara, Y., Endo, F., Mizoguchi, H., Watanabe, S., et al. (2021). Microglial gene signature reveals loss of homeostatic microglia associated with neurodegeneration of Alzheimer’s disease. Acta Neuropathol. Commun. 9:1. doi: 10.1186/s40478-020-01099-x
Steiner, H., Romig, H., Grim, M. G., Philipp, U., Pesold, B., Citron, M., et al. (1999). The biological and pathological function of the presenilin-1 Deltaexon 9 mutation is independent of its defect to undergo proteolytic processing. J. Biol. Chem. 274, 7615–7618. doi: 10.1074/jbc.274.12.7615
Susaki, E. A., Shimizu, C., Kuno, A., Tainaka, K., Li, X., Nishi, K., et al. (2020). Versatile whole-organ/body staining and imaging based on electrolyte-gel properties of biological tissues. Nat. Commun. 11:1982. doi: 10.1038/s41467-020-15906-5
Sutoko, S., Masuda, A., Kandori, A., Sasaguri, H., Saito, T., Saido, T. C., et al. (2021). Early identification of Alzheimer’s disease in mouse models: application of deep neural network algorithm to cognitive behavioral parameters. iScience 24:102198. doi: 10.1016/j.isci.2021.102198
t Hart, B. A., and Massacesi, L. (2009). Clinical, pathological, and immunologic aspects of the multiple sclerosis model in common marmosets (Callithrix jacchus). J. Neuropathol. Exp. Neurol. 68, 341–355. doi: 10.1097/NEN.0b013e31819f1d24
Tachida, Y., Miura, S., Imamaki, R., Ogasawara, N., Takuwa, H., Sahara, N., et al. (2020). Endothelial expression of human APP leads to cerebral amyloid angiopathy in mice. bioRxiv [preprint] doi: 10.1101/2020.07.22.215418
Takamura, R., Mizuta, K., Sekine, Y., Islam, T., Saito, T., Sato, M., et al. (2021). Modality-Specific impairment of hippocampal CA1 neurons of Alzheimer’s disease model mice. J. Neurosci. 41, 5315–5329. doi: 10.1523/jneurosci.0208-21.2021
Tardif, S. D., Mansfield, K. G., Ratnam, R., Ross, C. N., and Ziegler, T. E. (2011). The marmoset as a model of aging and age-related diseases. ILAR J. 52, 54–65. doi: 10.1093/ilar.52.1.54
Tardif, S. D., Smucny, D. A., Abbott, D. H., Mansfield, K., Schultz-Darken, N., and Yamamoto, M. E. (2003). Reproduction in captive common marmosets (Callithrix jacchus). Comp. Med. 53, 364–368.
Tsubuki, S., Takaki, Y., and Saido, T. C. (2003). Dutch, Flemish, Italian, and Arctic mutations of APP and resistance of Abeta to physiologically relevant proteolytic degradation. Lancet 361, 1957–1958. doi: 10.1016/s0140-6736(03)13555-6
Turner, A. J., and Nalivaeva, N. N. (2006). Proteinase dysbalance in pathology: the neprilysin (NEP) and angiotensin-converting enzyme (ACE) families. Cell Mol. Biol. (Noisy-le-grand) 52, 40–48.
Turner, A. J., Brown, C. D., Carson, J. A., and Barnes, K. (2000). The neprilysin family in health and disease. Adv. Exp. Med. Biol. 477, 229–240. doi: 10.1007/0-306-46826-3_25
Turner, A. J., Isaac, R. E., and Coates, D. (2001). The neprilysin (NEP) family of zinc metalloendopeptidases: genomics and function. Bioessays 23, 261–269. doi: 10.1002/1521-1878(200103)23:3<261::AID-BIES1036>3.0.CO;2-K
Turner, A. J., Murphy, L. J., Medeiros, M. S., and Barnes, K. (1996). Endopeptidase-24.11 (neprilysin) and relatives: twenty years on. Adv. Exp. Med. Biol. 389, 141–148. doi: 10.1007/978-1-4613-0335-0_17
Uruno, A., Matsumaru, D., Ryoke, R., Saito, R., Kadoguchi, S., Saigusa, D., et al. (2020). Nrf2 suppresses oxidative stress and inflammation in app knock-in Alzheimer’s disease model mice. Mol. Cell Biol. 40:e00467-19. doi: 10.1128/mcb.00467-19
Van Broeckhoven, C., Haan, J., Bakker, E., Hardy, J. A., Vanhul, W., Wehnert, A., et al. (1990). Amyloid-Beta protein-precursor gene and hereditary cerebral-hemorrhage with amyloidosis (DUTCH). Science 248, 1120–1122. doi: 10.1126/science.1971458
van de Nes, J. A., Sandmann-Keil, D., and Braak, H. (2002). Interstitial cells subjacent to the entorhinal region expressing somatostatin-28 immunoreactivity are susceptible to development of Alzheimer’s disease-related cytoskeletal changes. Acta Neuropathol. 104, 351–356. doi: 10.1007/s00401-002-0551-7
Vepsäläinen, S., Helisalmi, S., Koivisto, A. M., Tapaninen, T., Hiltunen, M., and Soininen, H. (2007). Somatostatin genetic variants modify the risk for Alzheimer’s disease among Finnish patients. J. Neurol. 254, 1504–1508. doi: 10.1007/s00415-007-0539-2
Watamura, N., Kakiya, N., Nilsson, P., Tsubuki, S., Kamano, N., Takahashi, M., et al. (2021a). Somatostatin-evoked Aβ catabolism in the brain: mechanistic involvement of α-endosulfine-KATP channel pathway. Mol. Psychiatry Online ahead of print. doi: 10.1038/s41380-021-01368-8
Watamura, N., Sato, K., Shiihashi, G., Iwasaki, A., Kamano, N., Takahashi, M., et al. (2021b). An isogenic panel of single app knock-in mouse models of Alzheimer’s disease confers differential profiles of β-secretase inhibition and endosomal abnormalities. bioRxiv [preprint] doi: 10.1101/2021.08.22.457278
Xia, D., Lianoglou, S., Sandmann, T., Calvert, M., Suh, J. H., Thomsen, E., et al. (2021). Fibrillar Aβ causes profound microglial metabolic perturbations in a novel APP knock-in mouse model. bioRxiv [preprint] doi: 10.1101/2021.01.19.426731
Yasojima, K., Akiyama, H., McGeer, E. G., and McGeer, P. L. (2001). Reduced neprilysin in high plaque areas of Alzheimer brain: a possible relationship to deficient degradation of beta-amyloid peptide. Neurosci. Lett. 297, 97–100. doi: 10.1016/s0304-3940(00)01675-x
Keywords: Alzheimer’s disease, amyloid – beta, amyloidosis, tau propagation, somatostatin, mouse model, non-human primate (NHP)
Citation: Sasaguri H, Hashimoto S, Watamura N, Sato K, Takamura R, Nagata K, Tsubuki S, Ohshima T, Yoshiki A, Sato K, Kumita W, Sasaki E, Kitazume S, Nilsson P, Winblad B, Saito T, Iwata N and Saido TC (2022) Recent Advances in the Modeling of Alzheimer’s Disease. Front. Neurosci. 16:807473. doi: 10.3389/fnins.2022.807473
Received: 02 November 2021; Accepted: 22 February 2022;
Published: 31 March 2022.
Edited by:
Brian Hansen, Aarhus University, DenmarkReviewed by:
Eugenio Gutiérrez Jiménez, Aarhus University, DenmarkCopyright © 2022 Sasaguri, Hashimoto, Watamura, Sato, Takamura, Nagata, Tsubuki, Ohshima, Yoshiki, Sato, Kumita, Sasaki, Kitazume, Nilsson, Winblad, Saito, Iwata and Saido. This is an open-access article distributed under the terms of the Creative Commons Attribution License (CC BY). The use, distribution or reproduction in other forums is permitted, provided the original author(s) and the copyright owner(s) are credited and that the original publication in this journal is cited, in accordance with accepted academic practice. No use, distribution or reproduction is permitted which does not comply with these terms.
*Correspondence: Takashi Saito, c2FpdG8tdEBtZWQubmFnb3lhLWN1LmFjLmpw; Nobuhisa Iwata, aXdhdGEtbkBuYWdhc2FraS11LmFjLmpw; Takaomi C. Saido, dGFrYW9taS5zYWlkb0ByaWtlbi5qcA==
Disclaimer: All claims expressed in this article are solely those of the authors and do not necessarily represent those of their affiliated organizations, or those of the publisher, the editors and the reviewers. Any product that may be evaluated in this article or claim that may be made by its manufacturer is not guaranteed or endorsed by the publisher.
Research integrity at Frontiers
Learn more about the work of our research integrity team to safeguard the quality of each article we publish.