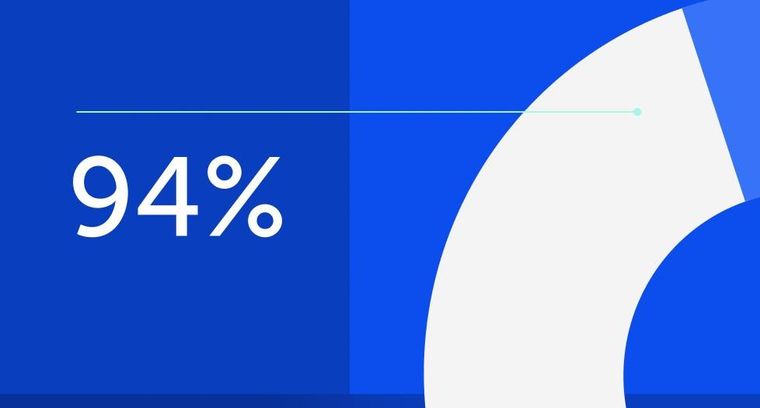
94% of researchers rate our articles as excellent or good
Learn more about the work of our research integrity team to safeguard the quality of each article we publish.
Find out more
REVIEW article
Front. Neurosci., 09 January 2023
Sec. Neuropharmacology
Volume 16 - 2022 | https://doi.org/10.3389/fnins.2022.1084493
This article is part of the Research TopicRestoring endoplasmic reticulum proteostasis to treat neurological disordersView all 5 articles
Neurological motor disorders (NMDs) such as Parkinson's disease and Huntington's disease are characterized by the accumulation and aggregation of misfolded proteins that trigger cell death of specific neuronal populations in the central nervous system. Differential neuronal loss initiates the impaired motor control and cognitive function in the affected patients. Although major advances have been carried out to understand the molecular basis of these diseases, to date there are no treatments that can prevent, cure, or significantly delay the progression of the disease. In this context, strategies such as gene editing, cellular therapy, among others, have gained attention as they effectively reduce the load of toxic protein aggregates in different models of neurodegeneration. Nevertheless, these strategies are expensive and difficult to deliver into the patients' nervous system. Thus, small molecules and natural products that reduce protein aggregation levels are highly sought after. Numerous drug discovery efforts have analyzed large libraries of synthetic compounds for the treatment of different NMDs, with a few candidates reaching clinical trials. Moreover, the recognition of new druggable targets for NMDs has allowed the discovery of new small molecules that have demonstrated their efficacy in pre-clinical studies. It is also important to recognize the contribution of natural products to the discovery of new candidates that can prevent or cure NMDs. Additionally, the repurposing of drugs for the treatment of NMDs has gained huge attention as they have already been through clinical trials confirming their safety in humans, which can accelerate the development of new treatment. In this review, we will focus on the new advances in the discovery of small molecules for the treatment of Parkinson's and Huntington's disease. We will begin by discussing the available pharmacological treatments to modulate the progression of neurodegeneration and to alleviate the motor symptoms in these diseases. Then, we will analyze those small molecules that have reached or are currently under clinical trials, including natural products and repurposed drugs.
As life expectancy has increased substantially during the past decades, the global prevalence of neurodegenerative disease has grown rapidly, as aging is one of the main factors involved in neurodegeneration (Hou et al., 2019; Azam et al., 2021). Among the wide range of neurodegenerative diseases, neurological motor disorders (NMDs) such as Parkinson's disease (PD) and Huntington's disease (HD) have increased their prevalence, having a negative impact on public health as the associated financial burden will continue to increase (Ou et al., 2021). Moreover, NMDs and other neurodegenerative diseases are not only a freight for the patients and public health systems, but also negatively impact the quality of life of caregivers, which are usually patient's family members (Achenbach and Saft, 2021; Rosqvist et al., 2022).
Even though patients from each NMD develop specific combination of motor symptoms, there are some common pathophysiological mechanisms in the development and progression of these diseases. The motor symptoms described in PD are triggered by the neurodegeneration of dopaminergic neurons, partly due to an accumulation of intraneuronal abnormal alpha-synuclein (α-syn) protein aggregates (Jankovic and Tan, 2020). The loss of dopaminergic neurons causes the decrease of an essential neurotransmitter: dopamine. Dopamine is produced in different areas of the nervous system, especially in the Substantia Nigra Pars Compacta (SNpc), and it is involved in multiple brain functions, such as behavior, cognition, motor activity, motivation, and reward (Schapira et al., 2017; Jankovic and Tan, 2020). Dopamine deficiency leads to a dysfunction of motor coordination in PD patients, among other problems.
On the other hand, Huntington's disease (HD) is an autosomal–dominant inherited neurological disorder caused by an unstable trinucleotide CAG repeat expansion at the N-terminus of the IT-15 gene, encoding the ~350 kDa huntingtin protein (Htt) (Jimenez-Sanchez et al., 2017; Tabrizi et al., 2020). This mutation causes Htt to contain an abnormally long polyglutamine (polyQ) tract, which confers Htt toxic properties (Tabrizi et al., 2020). HD prevalence was estimated at 2.7 per 100,000 inhabitants, with differences between regions of the world (McColgan and Tabrizi, 2018). A higher prevalence rate of HD has been reported in populations of Caucasian origin, not registering a difference between men and women (McColgan and Tabrizi, 2018). The clinical manifestation of HD is characterized by the loss of motor functions in the initial stages of the disease. Motor dysfunction continues throughout the progression of the disease, to what is added the appearance of psychiatric disorders and dementia (Tabrizi et al., 2020). Patients follow a relentless course of cognitive and motor impairment, which ultimately leads to death between 12 and 15 years from the onset of the symptoms (Tabrizi et al., 2020). Recent evidence indicates that mutant huntingtin (mHtt) aggregation in susceptible neurons may be responsible for the onset and progression of HD phenotypes, and death of affected neurons are associated with the accumulation of mutant proteins in insoluble aggregates (Tabrizi et al., 2020).
Despite numerous efforts being made to better understand the cellular mechanisms underlying the development of these disorders, to date there are no treatments that can prevent, cure, or significantly delay the progression of the disease (Troncoso-Escudero et al., 2020). Moreover, the current pharmacological strategies are only palliative and do not slow down or reverse the progression of the disease. As an example, pharmacological approaches for PD treatment aim to correct the loss of the fine motor control using drugs that (1) increase dopamine availability with dopamine precursors (Levodopa) or dopaminergic agonists (Pramipexole), and that (2) inhibit dopamine degradation by the monoamine-oxidase (Selegeline and Rasagiline) or catechol-o-metyltransferase (Entocapone and Tolcapone) (Van der Schyf, 2015; Carrera and Cacabelos, 2019). The chronic administration of antiparkinsonian drugs results in a “wearing-off phenomenon,” which produces additional psychomotor and autonomic complications, like dyskinesia (Ammal Kaidery et al., 2013; Cacabelos, 2017). Moreover, L-dopa pharmacokinetics are unpredictable and commonly lead to increased administration, complex regimens, and poor patient compliance. Nevertheless, L-dopa treatment remains as the gold standard treatment for movement disorder in PD patients (Oertel and Schulz, 2016; Fox et al., 2018).
For HD, only one approved pharmacological therapy for the treatment of motor symptoms has been approved: tetrabenazine (Yero and Rey, 2008). This is a small molecule that reduces dopamine neurotransmission via its depletion from presynaptic vesicles by inhibition of the vesicular monoamine transporter 2 (VMAT-2) (Yero and Rey, 2008). Tetrabenazine treatment results in a reduction of the chorea symptom in HD patients (Rodrigues and Wild, 2020). Nevertheless, sedation, anxiety, depression, and suicidality, have been observed as side effects of its administrations, and its use is limited in HD patients (Group et al., 2016; Rodrigues and Wild, 2020). On 2017, the FDA approved a deuterated derivative of tetrabenazine, deutetrabenazine, which has an improved pharmacokinetic profile that enables a less frequent daily dosage with comparable systemic exposure of the drug. The improved pharmacokinetics results in a better safety profile of the drug (Group et al., 2016).
Given that the current FDA-approved drugs for the treatment of PD and HD have several drawbacks, different therapeutic alternatives have been explored for the development of new treatments. In this context, novel therapeutic strategies such as cellular replacement therapies and gene editing, among others, have gained attention as they have shown to effectively reduce the load of toxic protein aggregates in different models of neurodegeneration, and thus, hold the potential to have disease modifying effects (Troncoso-Escudero et al., 2020). Cellular replacement therapies were among the first treatments used for PD and HD, which looked to restore the neuronal populations lost in the brain of the affected patients. Despite some functional recovery being observed in treated patients, important religious and ethical concerns regarding the use of fetuses arose (Troncoso-Escudero et al., 2020). Because of this, strategies to obtain dopaminergic or GABAergic neurons from embryonic stem cells and induced pluripotent stem cells emerged, with promising results in animal models of PD and HD (Troncoso-Escudero et al., 2020). Interestingly, an ongoing clinical trial will evaluate if dopamine progenitor cells derived from pluripotent stem cells injected into the putamen of 10 PD patients is safe and well tolerable, and if patients present potential side effects (NCT: 04802733). In addition to cell replacement, other strategies have looked to correct the mutated genes found in PD and HD patients. Several gene silencing/editing technologies have been applied for this, such as RNA interference, antisense oligonucleotides and CRISPR/cas9. Many clinical trials using gene therapy for PD have been completed, showing modest improvements in motor function (Troncoso-Escudero et al., 2020). Two promising clinical trials were evaluating the use of antisense oligonucleotides to reduce the levels of HTT (IONIS-HTTRx; NCT03342053, NCT03761849) and mHTT (PRECISION HD1/2; NCT03225833, NCT03225846) in the brains of HD patients. Unfortunately, both studies have been terminated due to lack of efficacy of the treatments.
Considering all the above, the lack of consistent and clinically relevant results for gene and cellular therapy, together with the cost and difficulties for delivery, highlights the urgent need to investigate other therapeutic approaches. In this critical context and considering the numerous efforts that have helped to define and/or re-define the pathophysiological mechanisms underlying NMDs, there is an interesting avenue for the rational design and development of new small molecules that target those mechanisms to halt and/or revert the progression of NMDs. Moreover, we can also explore the chemical space of natural products to discover new therapies for NMDs, an strategy that is supported by several epidemiological studies (Sanadgol et al., 2017; Polito et al., 2018; Rahman et al., 2021; Uddin et al., 2021) and the ceaseless relevance of natural products as drug leads (Newman and Cragg, 2020). Furthermore, “old drugs” can still be useful in drug discovery efforts through drug repurposing, as these compounds have already been proved safe and thus, the development costs would be potentially lower, and the development timelines should be shorter (Pushpakom et al., 2019). In this review, we discuss recent advances in the discovery of new small molecules, natural products or natural extracts, and drug repurposing for the treatment NMDs. Given the extensive amount of literature regarding small molecules under investigation for the treatment of NMDs (Elkouzi et al., 2019; Dash et al., 2021; Devadiga and Bharate, 2022; Liu et al., 2022), this review focuses primarily on those strategies that have reached clinical trials and thus, have potential to reach approval for the treatment of NMDs.
In this section, we will focus on those synthetic small molecules that have reached clinical trials for the treatment of PD and HD. Nevertheless, we also considered synthetic small molecules that are still under pre-clinical investigation given that they represent novel and promising strategies for the treatment of PD and HD (Figure 1A).
Figure 1. Targets of small molecules in neurodegenerative motor disorders. Synthetic small molecules (A), small molecules from natural sources (B) or Repurposing FDA-approved drugs (C) used to alleviate clinical symptoms of Parkinson's disease (blue box) or Huntington's disease (orange box). These molecules participate in modified specific cellular pathways related to alleviating or delaying the neurodegenerative process, as protein aggregation, inflammatory response (inflammosome), cellular differentiation, G protein coupled receptor, calcium channel activity or reactive oxygen species (ROS) production.
Although several strategies have been explored for PD treatment (Troncoso-Escudero et al., 2020), the identification of α-syn accumulation as one of the key pathological features of this disease has led to the development of pharmacological strategies that directly or indirectly target α-syn misfolded to prevent its aggregation and all the toxic events associated to aggregate formation (Savitt and Jankovic, 2019; Teil et al., 2020). One example of small molecules that directly modulates protein aggregation is NPT200-11, a totally synthetic small molecule derivative of NPT100-18A, a de novo synthetized molecule modeled to target specific regions of the α-syn protein that are key players in oligomer formation (Wrasidlo et al., 2016). Although treatment with NPT100-18A showed the effect of decreasing the α-syn accumulation in vitro and in vivo models of PD (Wrasidlo et al., 2016), its poor bioavailability led to the later development of NPT200-11, which retained its effects over α-syn aggregation with improved physicochemical and pharmacokinetic properties (Price et al., 2018). Recently, oral treatment with NPT200-11 showed the ability to reach the brain and to exert positive effects over neuropathological and behavioral endpoints related to its ability to reduce α-syn aggregation in a transgenic mouse model of PD (Price et al., 2018). The safety and tolerability of NPT200-11 have already been analyzed in a clinical trial where the drug was administered to healthy subjects (NCT02606682, Table 1), but its safety and efficacy in PD patients remains to be elucidated. Interestingly, UCB0599, the R-enantiomer of NPT200-11, has shown an acceptable safety and tolerability profile (Smit et al., 2022) and is currently under a phase 2 clinical trial to determine its effects over clinical symptoms of PD (NCT04658186, Table 1).
Table 1. Description of synthetic small molecules used in Parkinson's disease or Huntington's disease.
The recognition of the role in the loss of dopaminergic neurons of the activation of the NLR family pyrin domain containing 3 (NLRP3) inflammasome by α-syn aggregates in PD pathology accounts for another pharmacological target that can be exploited for PD treatment (Gordon et al., 2018; Wang et al., 2019; Haque et al., 2020). The NLRP3 inflammasome is a key component of the innate immune system involved in the activation of caspase-1 and the secretion of pro-inflammatory cytokines such as IL-1beta and IL-18 (Kelley et al., 2019) and, thus, it plays an important role in the pathogenesis of inflammation-related diseases. Also, extracellular α-syn acts as a pro-inflammatory molecule, which can trigger the activation of NLRP3, contributing to the inflammatory state in the PD brain (Otani and Shichita, 2020). In a proof-of-concept experiment, the oral treatment with NLRP3 inhibitor MCC950 was able protect dopaminergic neurons from α-syn aggregates-induced activation of the inflammasome, improving the motor performance in a preclinical mouse model of PD, but due to patent problems MCC950 cannot be commercialized (Gordon et al., 2018). IZD174 is a small molecule inhibitor of NLRP3 that will be part of a phase 1 clinical trial with PD patients that is currently recruiting subjects (NCT04338997, Table 1) and which will evaluate its pharmacokinetics and pharmacodynamics after oral treatment. Although targeting the NLRP3 inflammasome seems like a promising strategy for PD treatment, the lack of information on how the activation of the NLRP3 inflammasome can directly affect the survival of dopaminergic neurons has slowed down the progress in this direction (Haque et al., 2020).
Another target with pharmacological potential for the treatment of PD is the NURR1 transcription factor. This transcription factor is involved in the development and differentiation of dopaminergic neurons in the midbrain and has an important function in the continuing maintenance of these types of cells under both physiological conditions and stress (Decressac et al., 2013). NURR1 can form heterodimers with the retinoid X receptor (RXR) and, consequently, RXR ligands can modulate the functions of NURR1 (Wang et al., 2016). BRF110 is a synthetic small molecule designed to specifically activate the NURR1-RXR heterodimer, whose oral treatment has shown to protect dopaminergic neurons from α-syn and to restore dopamine synthesis in preclinical models of PD (Spathis et al., 2017). Nevertheless, to our knowledge, no clinical trials related to BRF110 have been posted to date. IRX4204 is another synthetic RXR ligand that can cross the BBB to specifically activate the NURR1-RXR heterodimer (Wang et al., 2016). The oral treatment with this drug significatively reduced PD motor symptoms, restored the dopaminergic neuron loss, and increased the expression of molecules related to dopamine synthesis (Wang et al., 2016). A phase 1 clinical trial of IRX4204 in patients with early-stage PD has already been completed (NCT02438215, Table 1), showed that the drug was safe and well tolerated, but no changes in dopamine transporter binding was observed (Sanders et al., 2016). Interestingly, patients presented a trend toward improvement in motor symptoms, opening the window for further analysis of IRX4204 (Sanders et al., 2016).
Cu (II) ATSM is a cupper-containing radiolabeled imaging agent that has shown to inhibit α-syn aggregation in vitro, which mediated cell toxicity, and dopaminergic neuron loss in preclinical models of PD (Hung et al., 2012). In a study aiming to analyze other pathways involved in the neuroprotective activities of Cu (II) ATSM, a RNAseq whole transcriptome sequencing approach was utilized to evaluate the changes in the SNpc upon treatment with Cu (II) ATSM in a mouse model of PD (Cheng et al., 2016). Results showed a set of genes related to brain and cognitive development, neuroplasticity, regulation and cellular response, whose expression was recovered upon oral treatment with Cu (II) ATSM in PD animals (Cheng et al., 2016), thus the beneficial effects of Cu (II) ATSM treatment are not only related to its indirect effects over protein aggregation but might also be related to its ability to restore gene expression. The safety and tolerability of Cu (II) ATSM treatment in PD patients has recently been analyzed in a multicenter phase 1 clinical trial (NCT03204929, Table 1), although results haven't been posted yet.
Although it was not developed as a disease-modifying treatment, IRL790 (Mesdopetam) is a novel compound that has already been tested in clinical trials to analyze its safety and tolerability in PD patients (Svenningsson et al., 2018) and, recently, its efficacy in the treatment of PD-related dyskinesia has also been evaluated (NCT03368170, Table 1). IRL790 is a dopamine antagonist that was rationally developed to antagonize the D3/D2 receptors (Waters et al., 2020). Upon oral treatment, IRL790 was able to exert anti-dyskinesic and anti-psychotic effects in preclinical models without having a negative impact on the normal dopamine transmission and, in consequence, in the normal motor function (Waters et al., 2020). Hence, IRL790 treatment might have a positive impact on the quality of life of PD patients suffering from dyskinesia and psychosis.
NYX-458 is another example of a non-disease-modifier drug being developed for the treatment of PD. Specifically, NYX-458 is a modulator of the NMDA receptor that has shown the effect of facilitating synaptic plasticity (Khan et al., 2018) and chronic low-dose oral treatment with this drug resulted in an improvement of the cognitive performance in a primate model of PD (Barth et al., 2020). The safety and tolerability of NYX-458 is being tested in a phase 2 clinical trial on PD patients with mild cognitive impairment, where the drug's effects on cognitive performance are also being explored (NCT04148391, Table 1). This clinical trial is expected to be completed by the end of 2022.
Strategies that directly or indirectly target mHTT aggregation have also been under development for HD treatment (Tabrizi et al., 2019). As in PD, the rationale behind targeting mHTT aggregation is in line with its pathogenic role in the progression of the disease. PBT2 is an orally available metal chelator with the ability to prevent protein aggregation associated with metal-mediated oxidative stress (Cherny et al., 2012). The oral treatment with PBT2 has shown to reduce neuronal damage, reverse motor dysfunction and extend lifespan in preclinical models of HD which express polyQ extended proteins that are able to form toxic aggregates (Cherny et al., 2012). The safety, tolerability, and efficacy of PBT2 has already been tested in a phase 2 clinical trial including patients with early to mid-stage HD (NCT01590888, Table 1). The results of this clinical trial showed that oral treatment with PBT2 is generally well tolerated and safe, as the incidence of serious adverse effects in all treated patients was low (Huntington Study Group Reach2HD Investigators, 2015). Furthermore, treatment with PBT2 also showed to improve the cognitive performance of treated patients, but a larger study is needed in order to confirm its beneficial effects in HD patients (Huntington Study Group Reach2HD Investigators, 2015).
Selisistat is a small molecule inhibitor of the Sirtuin 1 deacetylase (SirT1), whose use as treatment has shown to protect against mHTT toxicity in cellular and preclinical models of HD (Smith et al., 2014). Its significant effects over mHTT aggregation produced an improvement in the motor function and lifespan of treated animals (Smith et al., 2014). The effects of selisistat and other SirT1 inhibitors over protein aggregation can be explained by the requirement of post-translational acetylation for the clearance of mHTT through the autophagy machinery (Jeong et al., 2009). Four clinical trials of selisistat treatment in HD patients have already been performed (NCT01485965; NCT01485952; NCT01521585; NCT01521832, Table 1), from where its safety and tolerability have been confirmed (Süssmuth et al., 2015). Although selisistat treatment did not show adverse effects on the motor and cognitive performance of HD patients (Süssmuth et al., 2015), its efficacy as a disease-modifying treatment of HD remains to be evaluated.
As previously explained, the activation of NLRP3 inflammasome by α-syn aggregates has been related to neuronal death in PD (Gordon et al., 2018; Wang et al., 2019; Haque et al., 2020). Recently, Paladino et al showed that the expression of NLRP3 was significantly higher in R6/2 mice compared to control mice (Paldino et al., 2020). Moreover, oral treatment of R6/2 animals with Olarapib, an FDA approved drug commonly used for the treatment of ovarian cancer, significantly reduced the expression of NLRP3, the levels of neuroinflammation, and promoted neuroprotection in treated animals (Paldino et al., 2020). More recently, Chen et al. (2022), showed that oral treatment with the highly selective NLRP3 inhibitor MCC950 suppressed NLRP3 inflammasome, reduced levels of pro-inflammatory cytokines and neuronal toxicity in the R6/2 preclinical model and, more importantly, improved motor dysfunction and extended lifespan in treated animals compared to non-treated mice.
Pridopidine is a dopamine D2 receptor ligand that upon interaction destabilize the active conformation of the receptor and, consequently, acts as a dopamine antagonist increasing synthesis, release, and metabolism of dopamine in subcortical areas (Waters et al., 2018). Preclinical data shows that treatment with pridopidine has a higher affinity for the sigma 1 receptor, which is in the endoplasmic reticulum (ER), and upon interaction with pridopidine promotes neuroprotection in mouse primary cortical neurons and patient-derived induced pluripotent stem cells (Eddings et al., 2019). Furthermore, pridopidine treatment has also shown to improve behavioral symptoms and reverse the changes in gene transcription related to HD in a preclinical model of the disease (Garcia-Miralles et al., 2017; Kusko et al., 2018). The safety, tolerability, and efficacy as a symptomatic treatment of this drug has already been tested in phase 1 (NCT03019289, Table 1), phase 2 (NCT02006472, NCT01306929, Table 1) and phase 3 (NCT00724048, NCT00665223, Table 1) clinical trials. These studies demonstrated that 90 mg of pridopidine acts as selective S1R ligand (Grachev et al., 2021) and that treatment of HD patients with pridopidine results in significant reduction in the decline in Total Functional Capacity, a scale used to assess independence, perform domestic work, among other tasks (McGarry et al., 2020a,b). Further studies are needed to determine its properties as a disease modifying treatment for HD.
Although it's been a long time since the golden era of natural products drug discovery, small molecules from natural sources still play an important role in drug development (Newman and Cragg, 2020). Among the most distributed molecules in the plant kingdom are polyphenolic compounds, with more than 10,000 different molecules described to date (Figueira et al., 2017). The consumption of phenolic-rich foods has been identified by several epidemiologic studies as beneficial for cognitive function (Commenges et al., 2000; Devore et al., 2010; Lefèvre-Arbogast et al., 2018), although there have been some controversies (Crichton et al., 2013; Reale et al., 2020). The accumulation of evidence has led to the preclinical analysis of the potential role of polyphenolic compounds in the prevention and treatment of neurodegenerative diseases by different studies (revised in Maher, 2019). In this section we will focus on in vivo and clinical evidence for the use of polyphenolic compounds and polyphenolic-rich plant extracts for prevention or treatment of PD and HD. The neuroprotective properties of other types of phytochemicals and plant extracts can be found in Figure 1B (Choudhary et al., 2013; Zhang et al., 2017; Javed et al., 2018).
Previous reviews have analyzed the potential neuroprotective capacity of polyphenolic compounds, showing that these types of compounds could be acting through their antioxidant, anti-inflammatory, and anti-aggregation activities (Zhang et al., 2017; Javed et al., 2018; Kujawska and Jodynis-Liebert, 2018; Maher, 2019). One of the most studied polyphenolic compounds is resveratrol. Resveratrol is a stilbene most notably found in grapes and blueberries, whose antioxidant and anti-inflammatory activities have been extensively studied (revised in Diaz-Gerevini et al., 2016; Repossi et al., 2020). Its neuroprotective properties have also been analyzed and several mechanisms of action of this molecule have been described (Bastianetto et al., 2015; Kou and Chen, 2017; Gomes et al., 2018; Lin et al., 2018). In different in vivo PD models, resveratrol has shown the ability to delay the progression of the disease and improve motor function through the rescue of dopaminergic neurons (Huang et al., 2019), induction of α-syn degradation by autophagy (Guo et al., 2016), inhibition of α-syn oligomerization and reduction of neuroinflammation and oxidative stress (Zhang et al., 2018). Also, the concomitant use of resveratrol and L-dopa in a mouse model of PD has been effective in reducing the dopaminergic neuron loss and motor dysfunction, with the advantage of a lower administration of L-dopa, and therefore, less side effects manifestation (Liu et al., 2019). The potential applications of resveratrol in the treatment of PD and other diseases are limited by its poor solubility and extensive hepatic metabolisms which lowers its bioavailability (Salehi et al., 2018; Arbo et al., 2020). This issue has led to the development of resveratrol derivatives with improved pharmacokinetics and similar biological effects, whose potential uses in PD treatment are reviewed in (Arbo et al., 2020). BIA6-512 is a resveratrol derivative (trans-resveratrol) whose pharmacokinetics have been analyzed in clinical trials as monotherapy (NCT03095105 and NCT03093389, Table 2) and in combination with L-dopa (NCT03091543, Table 2), but no clinical trials studying the efficacy of BIA6-512 treatment, alone or in combination, have been performed to date.
Table 2. Description of small molecules from natural sources used in Parkinson's disease or Huntington's disease.
Flavonoids are one of the biggest family of polyphenolic compounds found in plants with around 5000 molecules described, classified by the degree of oxidation of their central ring, their hydroxylation pattern, and the presence of substitution in C3 (Petrussa et al., 2013; Maher, 2019). These compounds can be found in a variety of foods and food products such as grapes, berries, tea and cocoa (Terahara, 2015) and their neuroprotective effects have been extensively analyzed in preclinical models (revised in Vauzour et al., 2008; Spencer, 2009; Vauzour, 2014; Flanagan et al., 2018; Gildawie et al., 2018; Bakoyiannis et al., 2019; Maher, 2019; Di Meo et al., 2020). Nevertheless, our search for clinical trials of flavonoids related to PD revealed that not many of these plant metabolites reach the clinical stage for the treatment of neurodegenerative diseases. In this context, one clinical trial in phase 2 of green tea polyphenols was found. In this clinical trial performed in China (NCT00461942, Table 2), the safety and efficacy of the treatment with a green tea extract was analyzed in PD patients not yet taking prescribed PD-specific medications, but results are not posted. The rationale behind the use of a green tea extract is the presence of catechins, specifically EGCG (epigallocatechin-3-gallate), a polyphenolic compound of the flavonoid family (Nagle et al., 2006). EGCG treatment has shown the ability to inhibit α-syn in in vitro and human postmortem tissue models of PD by directly interacting with specific amino acid sequences in the protein and blocking aggregation-prone regions by intermolecular hydrophobic interactions (Xu et al., 2016). Furthermore, EGCG oral treatment rescued striatal dopaminergic neurons by protecting them against oxidative stress related to iron in a MPTP-induced PD mouse model (Xu et al., 2016). EGCG treatment has also shown to modulate autophagy and extend cell survival (Holczer et al., 2018), a mechanism that could also be involved in its neuroprotective properties of EGCG (Limanaqi et al., 2019). The effects of the treatment with a standardized green tea extract have also been analyzed in a preclinical model of PD (Pinto et al., 2015). In this study, long-term treatment with green tea extract resulted in positive effects on motor and behavioral performance of treated animals with respect to control, and in a reversal of DA levels in the striatum and protection against oxidative stress (Pinto et al., 2015). More details on the neuroprotective mechanisms of EGCG can be found elsewhere (Wang et al., 2022). Considering the accumulated pre-clinical evidence, the disease-modifying properties of EGCG should be further analyzed in clinical trials.
Cannabinoids are a class of secondary metabolites found in Cannabis sativa, a plant whose leaves and flowers have been used since ancient times for different purposes. Cannabidiol (CBD) is the second most abundant component of the plant, behind delta9-tetrahydrocannabinol (Δ9-THC). The endocannabinoid system, mainly composed of CB1 and CB2 receptors, and their endogenous ligands, plays a key role in the regulation of physiological processes and its alteration has been observed in several pathological conditions, such as movement disorders (Bilkei-Gorzo, 2012; Buhmann et al., 2017, 2019). For this reason, many efforts to develop cannabinoid-based therapeutics to target the endocannabinoid system for the treatment of disease have been made, but these efforts have been challenged by the complexity and promiscuity of the cannabinoids actions (Morales and Jagerovic, 2020). In the context of PD, the role of the endocannabinoid system is still unclear (Cristino et al., 2020; Junior et al., 2020; Morales and Jagerovic, 2020). Nevertheless, treatment with different phytocannabinoids has shown neuroprotective effects in preclinical animal models of PD (García et al., 2011; Ojha et al., 2016; Espadas et al., 2020). Moreover, Nabilone, a synthetic analog of tetrahydrocannabinol, was included in 2 clinical trials (NCT03769896; NCT03773796, Table 2) to assess its efficacy and safety for the treatment of non-motor symptoms in patients with PD (Peball et al., 2019). Results from these studies indicate that Nabilone treatment has beneficial effects on sleep outcomes in PD patients that have been experiencing sleep problems at baseline (Peball et al., 2022). Whether or not phytocannabinoids or their synthetic derivatives can have a significant impact on motor performance of PD patients remains to be elucidated (Buhmann et al., 2017).
Oral treatment with resveratrol extended lifespan in both models and reversed motor impairment related to mHTT expression in treated mice compared to control, effects that can be explained by the activation of the ERK signaling pathway (Maher et al., 2011). ERK signaling alterations have been related to the development of movement disorders (Hutton et al., 2017) and, in turn, mHTT has shown to alter ERK signaling in HD models (Apostol et al., 2006), which might explain the neuroprotective effects of resveratrol treatment. Resveratrol has also shown to protect against dopamine and mHTT toxicity in a neuronal cell model of HD by preventing oxidative stress and promoting autophagy-degradation of mHTT (Vidoni et al., 2018). Also, resveratrol treatment has shown to improve mitochondrial activity in a transgenic mouse model of HD, an effect that correlated with a significant improvement in motor function of treated animals compared to control (Naia et al., 2017). The therapeutic potential of resveratrol was analyzed in a clinical trial comprising 102 participants with HD (NCT023366339, Table 2), where the primary output was to assess the rate of caudate atrophy after 1 year of treatment. This clinical trial was recently completed, but no results have been posted yet.
EGCG treatment inhibits early events of mHTT aggregation in vitro, reduces the toxicity of mHTT in a yeast model of HD, and improves motor performance in a transgenic fly model of HD (Ehrnhoefer et al., 2006). Also, treatment with a green tea infusion has shown to modestly reduce neurodegeneration related to mHTT aggregation in a fly model of HD (Varga et al., 2020). A phase 2 multicenter clinical trial of EGCG, denominated the ETON-Study (NCT01357681, Table 2), aimed to analyze the effects on cognitive function of EGCG treatment for 1 year in patients with HD and the safety and tolerability of the treatment were also studied. This study was completed in 2015, but results were not posted.
Curcumin is one of the most active polyphenolic compounds from Curcuma, has shown to possess anti-oxidant and anti-inflammatory potential in the treatment of several disorders including diabetes, cardiovascular, neurologic, metabolic, inflammatory, and skin disorders, hepatotoxicity, respiratory tract infections, and diseases of infectious origin (Zhou et al., 2011; Khan et al., 2019). Curcumin treatment has been analyzed in pre-clinical models of Alzheimer's disease (AD) and amyotrophic lateral sclerosis (ALS), which have shown promising results (Chico et al., 2018; Voulgaropoulou et al., 2019; Mohseni et al., 2021; Lv et al., 2022). In these studies, curcumin treatment has shown to inhibit protein aggregation and decrease neuroinflammation, mechanisms that could also be promissory for PD and HD treatment. Moreover, curcumin treatment has also been analyzed in clinical trials of AD and ALS (Ringman et al., 2012; Chico et al., 2018; Voulgaropoulou et al., 2019; Mohseni et al., 2021; Lv et al., 2022). Furthermore, a chronic administration of curcumin in preclinical models of Huntington's disease has been shown to alleviate both the brain pathophysiology associated with reduced levels of huntingtin protein aggregates and to alleviate the motor symptoms described in R6/2 mice, along with reduced inflammation and intestinal damage associated with the progression of Huntington's disease (Elifani et al., 2019). Thus, curcumin treatment is a potential candidate for clinical trials in PD and HD patients.
The evidence for the use of cannabinoids as treatment for HD has been somewhat mixed given the high heterogeneity of different motor and cognitive symptoms of HD patients. Dronabinol is a synthetic form of delta-9-tetrahydrocannabinol (Δ9-THC), the primary psychoactive component of cannabis. This natural product is a partial agonist at Cannabinoid-1 receptor (CB1R) and Cannabinoid-2 receptor (CB2R). In a clinical trial (NCT01502046), treatment with dronabinol showed beneficial symptomatic motor effects in HD patients with dystonia as a primary motor syndrome (rigidity or muscle contracture). It was also observed an improvement in weight and food intake in patients with more advanced disease stages, but dronabinol treatment has failed to demonstrate positive effects in the management of chorea (Saft et al., 2018).
Considering that the translation from the accumulated knowledge on human disease to successful therapeutics has been slower than expected, repurposing drugs has become an attractive strategy for the identification of new therapeutic uses of already approved drugs (Oprea and Mestres, 2012; Chen et al., 2019; Pushpakom et al., 2019; Roessler et al., 2021; Koponen et al., 2022). This has become especially important for those diseases that, like neurodegenerative diseases, have an unmet clinical need. The advantages of drug repurposing are diverse: the failure risk is lower, the timeframe for development is reduced, and less investment is needed, as the candidate drugs have already been developed and their safety and tolerability has been assessed in clinical trials (Pushpakom et al., 2019). Drug repurposing strategies and examples of successful drug repurposing can be revised on (Pushpakom et al., 2019). In this section we will discuss examples of drugs that have been repurposed or whose repurposing is being studied for the treatment of PD and HD (Figure 1C).
As mentioned before, the gold standard pharmacological intervention for PD is L-dopa, a dopamine agonist, which can be combined with MAO-B and COMT inhibitors to improve its efficacy and lower its side effects. Since its discovery and initial use for PD treatment, just a few drugs have accomplished to be approved by the FDA, and most of them share its mechanism of action. The imperative need to expand the catalog of drugs available for patients has opened the opportunity for the study of previous FDA-approved drugs. This was the case back in the 1970s for amantadine, a NMDA receptor antagonist drug that was originally developed as an influenza prophylactic and later, after appropriate clinical trials, repurposed as treatment for motor complications in PD treatment (Athauda and Foltynie, 2018). Thereafter, several drugs have been tested for its positive effects in PD patients. Ambroxol is an expectorant drug that has been available worldwide for more than 30 years. After a high-throughput screen of an FDA-approved drugs library comprising 1,040 different compounds, ambroxol was identified and found to be a pH-dependent, mixed-type inhibitor of glucocerebrosidase (GCase), interacting with both active and non-active site residues of GCase. Its inhibitory activity was maximal at neutral pH in endoplasmic reticulum (ER), and the activity was undetectable at the acidic pH of lysosome (Maegawa et al., 2009). Ambroxol acts as a pharmacological chaperone with the ability to stabilize the GCase (Maegawa et al., 2009). GCase has been identified as a potential pharmacological target for the treatment of PD as mutations in its gene is one of the strongest genetic factors for PD (Avenali et al., 2020), and thus, ambroxol properties have been analyzed in different models of PD (Athauda and Foltynie, 2018). In vivo data indicated that ambroxol could cross the blood-brain barrier and reduce the levels of α-syn and p-α-syn in brain mice (Migdalska-Richards et al., 2016). Also, ambroxol treatment has recently demonstrated its safety and tolerability in PD patients with and without GBA1 gene mutations (Mullin et al., 2020), and is currently under clinical trial to demonstrate its disease-modifying properties in PD patients by the evaluation of cognitive and motor test, also the detection of neurodegeneration markers by MRI, the determination of pharmacokinetics and pharmacodynamics of ambroxol in plasma and the evaluation of GCase activity in lymphocytes (Silveira et al., 2019) (NCT052875, Table 3).
Table 3. Description of repurposed FDA-approved drugs for Parkinson's disease of Huntington's disease.
An epidemiological study showed that long term treatment with calcium channel blocker antihypertensives was related with a lower risk of developing PD (Becker et al., 2008) and, due to its affinity for calcium channels and brain bioavailability, isradipine was considered as a promissory candidate for PD treatment (Athauda and Foltynie, 2018). The safety and tolerability of isradipine treatment have already been analyzed in clinical trials (NCT00909545, NCT00753636, Table 3) but recently, the STEADY-PD III trial which studied the efficacy of isradipine treatment in early PD, concluded that this intervention did not slow the progression of the disease (NCT02168842, Table 3) (Parkinson Study Group STEADY-PD III Investigators., 2020).
Another drug that is being studied to be repurposed for PD treatment is deferiprone, an iron chelator that can cross the blood brain barrier (BBB) and remove the excess of iron in the brain (Dexter et al., 2011; Martin-Bastida et al., 2017). This drug was initially designed in the 1980s for the treatment of iron overload related to blood transfusions (Hider and Hoffbrand, 2018). More recently, it has been observed that iron can accumulate in the brain during aging, mediating ROS production and facilitating protein aggregation and neuronal death (Guo and Scarlata, 2013; Joppe et al., 2019; Lévy et al., 2019). Hence deferiprone might have effects over α-syn aggregation. Furthermore, as iron is required for dopamine synthesis, its concentrations are higher in substantia nigra pars compacta (SNpc) dopaminergic neurons and, as these neurons are more susceptible to the higher production of ROS associated to aging (Choi et al., 2012; Trist et al., 2019), the beneficial effects of deferiprone in the treatment of PD might also be directly related to its iron chelation activity (Devos et al., 2020). Thus, deferiprone treatment has been analyzed in order to assess its neuroprotective properties in preclinical models of PD, observing that the subcutaneous administration of deferiprone can rescue dopaminergic neuron from degeneration (Dexter et al., 2011) and that its oral administration can improve motor and cognitive performance in treated animals, without having a significative effect over α-syn aggregation (Carboni et al., 2017). Considering the preclinical data, deferiprone treatment has also been analyzed in clinical trials including PD patients. In a phase 2 clinical trial (NCT02655315, Table 3), the short-term treatment with deferiprone in patients with early-stage PD showed to be safe, well tolerated, and able to reduce iron accumulation in specific brain regions (Martin-Bastida et al., 2017). The evidence accumulated through the years led to the development of a large multicenter clinical trial which is currently evaluating the disease-modifying properties of deferiprone on 372 subjects (NCT02655315, Table 3). To date, no results regarding this trial have been published.
An important line of investigation respecting drug repurposing for PD is related to inhibiting or decreasing the α-syn aggregation. In 2017, Mittal et al., uncovered a novel function of a group of drugs previously approved by the FDA. Under the premise of finding drugs that can reduce the levels of the SNCA gene, and hence, the α-syn levels, a selective β2-adrenoreceptor (β2AR) agonists reduced the expression levels of SNCA (Mittal et al., 2017). The decrease in the expression of SNCA and the α-syn levels using β2AR agonists was confirmed using cell lines, primary neurons, and animal models (Mittal et al., 2017). Interestingly, using mice treated with MPTP, a chemical used to model PD, the investigators found that treatment of these mice with clenbuterol, a β2AR agonist, abrogated the neurodegeneration observed under MPTP administration (Mittal et al., 2017). Moreover, these findings were confirmed using iPSCs-derived neuronal cultures from a PD patient (Mittal et al., 2017). Interestingly, the use of salbutamol, a β2AR agonist and a drug typically prescribed for asthma, was associated with a reduced risk of PD in the Norwegian population (Mittal et al., 2017). The later finding was also confirmed in a study using medical records of a large Israeli cohort (Gronich et al., 2018). Salbutamol has also been linked in the promotion of the expression of fibroblast growth factor 20 (FGF20) in the striatum of naïve rats (Fletcher et al., 2019). FGF20 is a brain-specific factor that plays a crucial role in the development, maintenance, and survival of dopaminergic neurons. Interestingly, salbutamol increased dopaminergic neuron survival in 6-OHDA-lesioned rats (Fletcher et al., 2019), supporting the results of Mittal et al. (2017). Despite these interesting results, other have found no association between the use of β2AR agonists and PD risk (Searles Nielsen et al., 2018), so further studies are needed to rather confirm or disprove these findings. Moreover, to date no clinical trial for the study of the association of salbutamol use and PD risk is under way.
Several approved drugs have been analyzed for the treatment of HD and some of them have been included in clinical trials, but just a few currently remain under active development (Devadiga and Bharate, 2022). Fenofibrate is an FDA-approved drug that is commonly used for the treatment of severe hypertriglyceridemia and mixed dyslipidemia. Fenofibrate can promote the activation of peroxisome proliferator-activated receptor-α (PPAR-α), a subtype of PPAR receptors expressed in brown adipose tissue, liver, kidney, heart, skeletal muscle, CNS, and T-cells, that acts as a lipid sensor performing essential metabolic functions (McKeage and Keating, 2011; Bhateja et al., 2012; Dickey et al., 2016). PPAR-α activation has been related to anti-inflammatory and antioxidant effects, and thus, the neuroprotective effects of PPAR-α activators such as fenofibrate have been investigated (Bordet et al., 2006; Bhateja et al., 2012). In this context, it has been observed that the intraperitoneal administration of fenofibrate significantly improved motor deficits and cognitive function, reduced oxidative stress, and restored antioxidant mechanisms in a pharmacologically-induced model of HD (Bordet et al., 2006). A phase II clinical trial evaluating the safety and efficacy of fenofibrate treatment was initiated in 2017 and remains active (NCT03515213, Table 3). Results were expected to be posted on august 2021 but, to date, they have not been reported. It has also been reported that HTT can directly interact with PPAR-δ, the most abundant PPAR in the CNS, altering its function as a transcriptional factor and, thus, contributing to mitochondrial dysfunction and neurotoxicity in pre-clinical models of HD (Dickey et al., 2016). Furthermore, altering the activation of PPAR-δ through the expression of a transgenic construct in mice induces HD-like phenotypes, confirming the crucial role of this receptor in maintaining neuronal health (Dickey et al., 2016). Consequently, intraperitoneal administration of the selective and potent PPAR-δ agonist KD3010 attenuated neurological dysfunction and improved motor function in HD mice without any notable side effects (Dickey et al., 2016). Thus, PPAR activators hold potential for the development of new therapeutics for NMDs and other neurological diseases either through repurposing or discovery of new molecules.
Metformin is another FDA approved drug that is used worldwide for the treatment of type 2 diabetes mellitus. In 2017, results from an observational study on HD revealed that metformin intake is associated with a better cognitive function in HD patients (Hervás et al., 2017). These observations were in line with previous reports that indicated that metformin treatment had protective effects in diverse pre-clinical models of HD (Ma et al., 2007; Jin et al., 2016; Vázquez-Manrique et al., 2016). The underlying mechanisms of the neuroprotective effects of metformin in HD might be related to its effects on the AMP-activated protein kinase (AMPK) but also other mechanisms might be involved (Ma et al., 2007; Jin et al., 2016; Vázquez-Manrique et al., 2016; Sanchis et al., 2019; Río et al., 2022). AMPK plays a key role in the response to metabolic changes, targeting proteins in diverse catabolic pathways to inactivate them and activating anabolic pathways to restore homeostasis (Vázquez-Manrique et al., 2016). Using a transgenic mouse model of HD, Ma et al. (2007) showed that the oral administration of metformin was associated to a significant increment in AMPK activation in the brain of treated animals and this, in turn, was related to increased survival and decreased hind limb clasping time in male mice, which is representative of an improvement in disease progression compared to control. Furthermore, it has been observed that AMPK activation by metformin treatment protects against neuronal dysfunction induced by overexpressing mHTT in Caenorhabditis elegans and mice (Ma et al., 2007). Moreover, Sanchis et al. (2019) demonstrated that metformin treatment reduced neuronal toxicity and improved neuropsychiatric and motor behavior in a transgenic mouse model of HD that overexpresses mHTT. Importantly, they also observed that metformin could cross the blood-brain barrier, reducing mHTT aggregation, preventing inflammation, and decreasing the levels of PERK, a biomarker of cellular stress (Sanchis et al., 2019). Other mechanisms involved in the neuroprotective effects of metformin can be revised elsewhere (Río et al., 2022). Considering this evidence, a clinical trial to analyze the safety and efficacy of metformin in adults with HD was initiated by the end of 2021 (NCT04826692). Results from this interventional study are expected to be posted by august 2024.
The most studied motor disorders are PD and HD. These NMDs are characterized by different etiological factors, differential vulnerability, the development of motor symptoms, and specific clinical manifestations. Interestingly, the neuronal circuits affected in these pathologies are common and thus, this knowledge could drive the recognition of the potential molecular pathways to develop future therapeutic treatments. We explored this hypothesis in this review, and, through extensive literature research, we noticed that common molecular pathways came up as interesting targets to evaluate and develop new small molecules that modulate specific molecular processes, such as protein aggregation, inflammation, and G protein-coupled receptor signaling. Nevertheless, current clinical trials in NMDs have a high failure rate. This might be due to patients presenting a high diversity in both genetic backgrounds and exposure of patients to environmental factors, which might influence the beginning of symptoms manifestation. For this reason, it is necessary to search for new biomarkers that could help us to discriminate between different subpopulations of patients, to reach a more personalized and precise medicine. This could allow for a better selection of participants included in clinical trials, considering the effect of the small molecules being studied on specific molecular processes.
In the context of natural products, a limitation in the development of these molecules as therapeutic agents for PD, HD, and other diseases, appears to be their lack of specificity. As stated before, compounds like resveratrol, EGCG and curcumin have been extensively investigated and they are normally recognized as hits in multiple assays. This has led to the recognition of molecular targets for these compounds, some of which have been discussed here. Compounds with this promiscuous nature have been denominated Pan Assay Interference compounds (PAINS) and are recognized as undesirable compounds in drug discovery efforts (Baell and Holloway, 2010; Baell, 2016). Some structural features on different natural products have been identified as PAINS motifs, like the catechol groups in EGCG and the quinone in the oxidized form of resveratrol (Baell, 2016), which might explain the diversity of mechanisms related to their pharmacological effects. Furthermore, although natural products are considered safe for consuming, these molecules can produce adverse effects by, for example, pharmacological interactions with other drugs (Wang et al., 2020). Despite these limitations, natural products are still a valuable starting point for drug development, as they can be used as scaffolds for the rational design of new drugs with the aid of high-content screening and computational tools (Kurita et al., 2015; Rodrigues et al., 2016; Davison and Brimble, 2019).
An important limitation for small molecules, either synthetic or from natural origin, in the context of NMD treatment, is the ability of these compounds to reach the central nervous system. Considering the molecular pathways involved in the development of NMDs, the bioavailability of the drug in the brain is a key factor to consider when developing an effective therapy for these pathologies. This limitation might be already accounted for when screening large libraries of synthetic molecules, as the physicochemical characteristics of the compounds can be previously optimized to assure their distribution to the brain (Wan et al., 2007). The application of recent advances in molecular docking and computer-aided design combined with cell-based platforms are helping to advance in the discovery of already clinically applicable molecules (Aldewachi et al., 2021). In the context of natural products, brain accessibility is also an issue. As an example, the oral bioavailability, of polyphenolic compounds has been pointed out by researchers as a limitation for the use of these molecules as pharmacological products (D'Archivio et al., 2010; Bohn, 2014; Kawabata et al., 2019). Nevertheless, evidence for the accumulation of these molecules in the brain can be found elsewhere (Ehrnhoefer et al., 2006; Figueira et al., 2017; Maher, 2019). Furthermore, advances in pharmaceutical technology, such as biomaterial and nanotechnology applications, have also been applied by researchers to overcome the physiological barriers that limit accessibility of drugs to the brain (Saeedi et al., 2019, 2022; Teleanu et al., 2019).
It is important to also recognize the importance of biotechnological products in drug discovery. Biotechnological products have also emerged as a promising class of drugs for the treatment of neurodegenerative diseases. An example of this is the engineered fusion protein NPT088. NPT088 targets amyloid in vitro and in animal models of Alzheimer's disease (AD), reducing β-amyloid plaque and tau aggregate loads in a mouse disease model (Levenson et al., 2016). In a clinical trial, patients with mild to moderate AD, were treated with NTP088. At the endpoint (6 months), NTP088 treatment was generally safe and well-tolerated (Michelson et al., 2019) but no significant effects over brain plaques, tau aggregates or AD symptoms were observed. However, it is important to notice that NPT088 more specifically targets aggregates of misfolded proteins rather than monomer subunits and consequently, it might be interesting to evaluate this type of drugs in the context of other misfolded protein-related neurodegenerative disorders.
Thus, there is hope for the discovery of new or old molecules that can be used in the treatment of NMDs and for the development of drugs that can be easily and effectively administered to patients.
Although we still have no cure for most NMDs, current research involving new molecules with novel mechanisms of action is promising, but not free of limitations. Considering the broad spectrum of research aiming to decipher the molecular mechanisms underlying NMDs, the number of new or repurposed molecules that reach clinical trials is still low. This might be explained, to some extent, by the intrinsic limitations of animal models for preclinical studies, the difficulty of accessing the brain, and the lack of human biomarkers that strongly correlate to the progress of the disease, and the difficulty of accessing the brain. Nonetheless, recent advances in strategies to accelerate the discovery or repurposing of drugs, on top of the innovations on delivery systems to the brain, restore the confidence that small molecules can play big roles in the treatment of NMDs.
RP-A, DS, PT-E, MC-O, and RV wrote and edited the manuscript and planned the manuscript. RV prepared the figures. All authors contributed equally to the critical reading of the final manuscript, including figures.
This work was directly funded by FONDAP program 15150012, Millennium Institute P09-015-F, and FONDECYT 1191003 (RV).
The authors declare that the research was conducted in the absence of any commercial or financial relationships that could be construed as a potential conflict of interest.
All claims expressed in this article are solely those of the authors and do not necessarily represent those of their affiliated organizations, or those of the publisher, the editors and the reviewers. Any product that may be evaluated in this article, or claim that may be made by its manufacturer, is not guaranteed or endorsed by the publisher.
Achenbach, J., and Saft, C. (2021). Another perspective on Huntington's disease: disease burden in family members and pre-manifest HD when compared to genotype-negative participants from ENROLL-HD. Brain Sci. 11, 1621. doi: 10.3390/brainsci11121621
Aldewachi, H., Al-Zidan, R. N., Conner, M. T., and Salman, M. M. (2021). High-throughput screening platforms in the discovery of novel drugs for neurodegenerative diseases. Bioengineering 8, 30. doi: 10.3390/bioengineering8020030
Ammal Kaidery, N., Tarannum, S., and Thomas, B. (2013). Epigenetic landscape of Parkinson's disease: emerging role in disease mechanisms and therapeutic modalities. Neurotherapeutics 10, 698–708. doi: 10.1007/s13311-013-0211-8
Apostol, B. L., Illes, K., Pallos, J., Bodai, L., Wu, J., Strand, A., et al. (2006). Mutant huntingtin alters MAPK signaling pathways in PC12 and striatal cells: ERK1/2 protects against mutant huntingtin-associated toxicity. Hum. Mol. Genet. 15, 273–285. doi: 10.1093/hmg/ddi443
Arbo, B. D., André-Miral, C., Nasre-Nasser, R. G., Schimith, L. E., Santos, M. G., Costa-Silva, D., et al. (2020). Resveratrol derivatives as potential treatments for Alzheimer's and Parkinson's disease. Front. Aging Neurosci. 12, 103. doi: 10.3389/fnagi.2020.00103
Athauda, D., and Foltynie, T. (2018). Drug repurposing in Parkinson's disease. CNS Drugs. 32, 747–761. doi: 10.1007/s40263-018-0548-y
Avenali, M., Blandini, F., and Cerri, S. (2020). Glucocerebrosidase defects as a major risk factor for Parkinson's disease. Front. Aging Neurosci. 12, 97. doi: 10.3389/fnagi.2020.00097
Azam, S., Haque, M. E., Balakrishnan, R., Kim, I.-S., and Choi, D.-K. (2021). The ageing brain: molecular and cellular basis of neurodegeneration. Front. Cell Dev. Biol. 9, 683459. doi: 10.3389/fcell.2021.683459
Baell, J. B. (2016). Feeling nature's PAINS: natural products, natural product drugs, and pan assay interference compounds (PAINS). J. Nat. Prod. 79, 616–628. doi: 10.1021/acs.jnatprod.5b00947
Baell, J. B., and Holloway, G. A. (2010). New substructure filters for removal of pan assay interference compounds (PAINS) from screening libraries and for their exclusion in bioassays. J. Med. Chem. 53, 2719–2740. doi: 10.1021/jm901137j
Bakoyiannis, I., Daskalopoulou, A., Pergialiotis, V., and Perrea, D. (2019). Phytochemicals and cognitive health: are flavonoids doing the trick? Biomed. Pharmacother. 109, 1488–1497. doi: 10.1016/j.biopha.2018.10.086
Barth, A. L., Schneider, J. S., Johnston, T. H., Hill, M. P., Brotchie, J. M., Moskal, J. R., et al. (2020). NYX-458 improves cognitive performance in a primate Parkinson's disease model. Mov. Disord. 35, 640–649. doi: 10.1002/mds.27962
Bastianetto, S., Menard, C., and Quirion, R. (2015). Neuroprotective action of resveratrol. Biochim. Biophys. Acta 1852, 1195–1201. doi: 10.1016/j.bbadis.2014.09.011
Becker, C., Jick, S. S., and Meier, C. R. (2008). Use of antihypertensives and the risk of Parkinson disease. Neurology 70, 1438–1444. doi: 10.1212/01.wnl.0000303818.38960.44
Bhateja, D. K., Dhull, D. K., Gill, A., Sidhu, A., Sharma, S., Reddy, B. V. K., et al. (2012). Peroxisome proliferator-activated receptor-alpha activation attenuates 3-nitropropionic acid induced behavioral and biochemical alterations in rats: possible neuroprotective mechanisms. Eur. J. Pharmacol. 674, 33–43. doi: 10.1016/j.ejphar.2011.10.029
Bilkei-Gorzo, A. (2012). The endocannabinoid system in normal and pathological brain ageing. Philos. Trans. R. Soc. Lond. B. Biol. Sci. 367, 3326–3341. doi: 10.1098/rstb.2011.0388
Bohn, T. (2014). Dietary factors affecting polyphenol bioavailability. Nutr. Rev. 72, 429–452. doi: 10.1111/nure.12114
Bordet, R., Gel,é, P., Duriez, P., and Fruchart, J.-C. (2006). PPARs: a new target for neuroprotection. J. Neurol. Neurosurg. Psychiatry 77, 285–287. doi: 10.1136/jnnp.2005.077495
Buhmann, C., Mainka, T., Ebersbach, G., and Gandor, F. (2017). Cannabinoids in Parkinson's disease. Cannabis Cannabinoid Res. 2, 21–29. doi: 10.1089/can.2017.0002
Buhmann, C., Mainka, T., Ebersbach, G., and Gandor, F. (2019). Evidence for the use of cannabinoids in Parkinson's disease. J. Neural Transm. 126, 913–924. doi: 10.1007/s00702-019-02018-8
Cacabelos, R. (2017). Parkinson's disease: from pathogenesis to pharmacogenomics. Int. J. Mol. Sci. 18, 551. doi: 10.3390/ijms18030551
Carboni, E., Tatenhorst, L., Tönges, L., Barski, E., Dambeck, V., Bähr, M., et al. (2017). Deferiprone rescues behavioral deficits induced by mild iron exposure in a mouse model of alpha-synuclein aggregation. Neuromolecular Med. 19, 309–321. doi: 10.1007/s12017-017-8447-9
Carrera, I., and Cacabelos, R. (2019). Current drugs and potential future neuroprotective compounds for Parkinson's disease. Curr. Neuropharmacol. 17, 295–306. doi: 10.2174/1570159X17666181127125704
Chen, K.-P., Hua, K.-F., Tsai, F.-T., Lin, T.-Y., Cheng, C.-Y., Yang, D.-I., et al. (2022). A selective inhibitor of the NLRP3 inflammasome as a potential therapeutic approach for neuroprotection in a transgenic mouse model of Huntington's disease. J. Neuroinflammation 19, 56. doi: 10.1186/s12974-022-02419-9
Chen, X., Gumina, G., and Virga, K. G. (2019). Recent advances in drug repurposing for Parkinson's disease. Curr. Med. Chem. 26, 5340–5362. doi: 10.2174/0929867325666180719144850
Cheng, L., Quek, C. Y. J., Hung, L. W., Sharples, R. A., Sherratt, N. A., Barnham, K. J., et al. (2016). Gene dysregulation is restored in the Parkinson's disease MPTP neurotoxic mice model upon treatment of the therapeutic drug Cu(II)(atsm). Sci. Rep. 6, 22398. doi: 10.1038/srep22398
Cherny, R. A., Ayton, S., Finkelstein, D. I., Bush, A. I., McColl, G., Massa, S. M., et al. (2012). PBT2 Reduces toxicity in a C. elegans model of polyQ aggregation and extends lifespan reduces striatal atrophy and improves motor performance in the R6/2 mouse model of Huntington's disease. J. Huntingtons. Dis. 1, 211–219. doi: 10.3233/JHD-120029
Chico, L., Ienco, E. C., Bisordi, C., Gerfo, A. L., Petrozzi, L., Petrucci, A., et al. (2018). Amyotrophic lateral sclerosis and oxidative stress: a double-blind therapeutic trial after curcumin supplementation. CNS Neurol. Disord. Drug Targets 17, 767–779. doi: 10.2174/1871527317666180720162029
Choi, D.-H., Cristóvão, A. C., Guhathakurta, S., Lee, J., Joh, T. H., Beal, M. F., et al. (2012). NADPH oxidase 1-mediated oxidative stress leads to dopamine neuron death in Parkinson's disease. Antioxid. Redox Signal. 16, 1033–1045. doi: 10.1089/ars.2011.3960
Choudhary, S., Kumar, P., and Malik, J. (2013). Plants and phytochemicals for Huntington's disease. Pharmacogn. Rev. 7, 81–91. doi: 10.4103/0973-7847.120505
Commenges, D., Scotet, V., Renaud, S., Jacqmin-Gadda, H., Barberger-Gateau, P., Dartigues, J. F., et al. (2000). Intake of flavonoids and risk of dementia. Eur. J. Epidemiol. 16, 357–363. doi: 10.1023/A:1007614613771
Crichton, G. E., Bryan, J., and Murphy, K. J. (2013). Dietary antioxidants, cognitive function and dementia–a systematic review. Plant Foods Hum. Nutr. 68, 279–292. doi: 10.1007/s11130-013-0370-0
Cristino, L., Bisogno, T., and Di Marzo, V. (2020). Cannabinoids and the expanded endocannabinoid system in neurological disorders. Nat. Rev. Neurol. 16, 9–29. doi: 10.1038/s41582-019-0284-z
D'Archivio, M., Filesi, C., Varì, R., Scazzocchio, B., and Masella, R. (2010). Bioavailability of the polyphenols: status and controversies. Int. J. Mol. Sci. 11, 1321–1342. doi: 10.3390/ijms11041321
Dash, R., Jahan, I., Ali, M. C., Mitra, S., Munni, Y. A., Timalsina, B., et al. (2021). Potential roles of natural products in the targeting of proteinopathic neurodegenerative diseases. Neurochem. Int. 145, 105011. doi: 10.1016/j.neuint.2021.105011
Davison, E. K., and Brimble, M. A. (2019). Natural product derived privileged scaffolds in drug discovery. Curr. Opin. Chem. Biol. 52, 1–8. doi: 10.1016/j.cbpa.2018.12.007
Decressac, M., Volakakis, N., Björklund, A., and Perlmann, T. (2013). NURR1 in Parkinson disease–from pathogenesis to therapeutic potential. Nat. Rev. Neurol. 9, 629–636. doi: 10.1038/nrneurol.2013.209
Devadiga, S. J., and Bharate, S. S. (2022). Recent developments in the management of Huntington's disease. Bioorg. Chem. 120, 105642. doi: 10.1016/j.bioorg.2022.105642
Devore, E. E., Grodstein, F., van Rooij, F. J. A., Hofman, A., Stampfer, M. J., Witteman, J. C. M., et al. (2010). Dietary antioxidants and long-term risk of dementia. Arch. Neurol. 67, 819–825. doi: 10.1001/archneurol.2010.144
Devos, D., Cabantchik, Z. I., Moreau, C., Danel, V., Mahoney-Sanchez, L., Bouchaoui, H., et al. (2020). Conservative iron chelation for neurodegenerative diseases such as Parkinson's disease and amyotrophic lateral sclerosis. J. Neural Transm. 127, 189–203. doi: 10.1007/s00702-019-02138-1
Dexter, D. T., Statton, S. A., Whitmore, C., Freinbichler, W., Weinberger, P., Tipton, K. F., et al. (2011). Clinically available iron chelators induce neuroprotection in the 6-OHDA model of Parkinson's disease after peripheral administration. J. Neural Transm. 118, 223–231. doi: 10.1007/s00702-010-0531-3
Di Meo, F., Valentino, A., Petillo, O., Peluso, G., Filosa, S., Crispi, S., et al. (2020). Bioactive polyphenols and neuromodulation: molecular mechanisms in neurodegeneration. Int. J. Mol. Sci. 21, 2564. doi: 10.3390/ijms21072564
Diaz-Gerevini, G. T., Repossi, G., Dain, A., Tarres, M. C., Das, U. N., Eynard, A. R., et al. (2016). Beneficial action of resveratrol: how and why? Nutrition 32, 174–178. doi: 10.1016/j.nut.2015.08.017
Dickey, A. S., Pineda, V. V., Tsunemi, T., Liu, P. P., Miranda, H. C., Gilmore-Hall, S. K., et al. (2016). PPAR-delta is repressed in Huntington's disease, is required for normal neuronal function and can be targeted therapeutically. Nat. Med. 22, 37–45. doi: 10.1038/nm.4003
Eddings, C. R., Arbez, N., Akimov, S., Geva, M., Hayden, M. R., Ross, C. A., et al. (2019). Pridopidine protects neurons from mutant-huntingtin toxicity via the sigma-1 receptor. Neurobiol. Dis. 129, 118–129. doi: 10.1016/j.nbd.2019.05.009
Ehrnhoefer, D. E., Duennwald, M., Markovic, P., Wacker, J. L., Engemann, S., Roark, M., et al. (2006). Green tea (-)-epigallocatechin-gallate modulates early events in huntingtin misfolding and reduces toxicity in Huntington's disease models. Hum. Mol. Genet. 15, 2743–2751. doi: 10.1093/hmg/ddl210
Elifani, F., Amico, E., Pepe, G., Capocci, L., Castaldo, S., Rosa, P., et al. (2019). Curcumin dietary supplementation ameliorates disease phenotype in an animal model of Huntington's disease. Hum. Mol. Genet. 28, 4012–4021. doi: 10.1093/hmg/ddz247
Elkouzi, A., Vedam-Mai, V., Eisinger, R. S., and Okun, M. S. (2019). Emerging therapies in Parkinson disease - repurposed drugs and new approaches. Nat. Rev. Neurol. 15, 204–223. doi: 10.1038/s41582-019-0155-7
Espadas, I., Keifman, E., Palomo-Garo, C., Burgaz, S., García, C., Fernández-Ruiz, J., et al. (2020). Beneficial effects of the phytocannabinoid Delta(9)-THCV in L-DOPA-induced dyskinesia in Parkinson's disease. Neurobiol. Dis. 141, 104892. doi: 10.1016/j.nbd.2020.104892
Figueira, I., Menezes, R., Macedo, D., Costa, I., and Dos Santos, C. N. (2017). Polyphenols beyond barriers: a glimpse into the brain. Curr. Neuropharmacol. 15, 562–594. doi: 10.2174/1570159X14666161026151545
Flanagan, E., Müller, M., Hornberger, M., and Vauzour, D. (2018). Impact of flavonoids on cellular and molecular mechanisms underlying age-related cognitive decline and neurodegeneration. Curr. Nutr. Rep. 7, 49–57. doi: 10.1007/s13668-018-0226-1
Fletcher, E. J. R., Jamieson, A. D., Williams, G., Doherty, P., and Duty, S. (2019). Targeted repositioning identifies drugs that increase fibroblast growth factor 20 production and protect against 6-hydroxydopamine-induced nigral cell loss in rats. Sci. Rep. 9, 8336. doi: 10.1038/s41598-019-44803-1
Fox, S. H., Katzenschlager, R., Lim, S.-Y., Barton, B., de Bie, R. M. A., Seppi, K., et al. (2018). International Parkinson and movement disorder society evidence-based medicine review: update on treatments for the motor symptoms of Parkinson's disease. Mov. Disord. 33, 1248–1266. doi: 10.1002/mds.27372
García, C., Palomo-Garo, C., García-Arencibia, M., Ramos, J., Pertwee, R., Fernández-Ruiz, J., et al. (2011). Symptom-relieving and neuroprotective effects of the phytocannabinoid Delta(9)-THCV in animal models of Parkinson's disease. Br. J. Pharmacol. 163, 1495–1506. doi: 10.1111/j.1476-5381.2011.01278.x
Garcia-Miralles, M., Geva, M., Tan, J. Y., Yusof, N. A. B. M., Cha, Y., Kusko, R., et al. (2017). Early pridopidine treatment improves behavioral and transcriptional deficits in YAC128 Huntington disease mice. JCI Insight 2, e95665. doi: 10.1172/jci.insight.95665
Gildawie, K. R., Galli, R. L., Shukitt-Hale, B., and Carey, A. N. (2018). Protective effects of foods containing flavonoids on age-related cognitive decline. Curr. Nutr. Rep. 7, 39–48. doi: 10.1007/s13668-018-0227-0
Gomes, B. A. Q., Silva, J. P. B., Romeiro, C. F. R., Dos Santos, S. M., Rodrigues, C. A., Gonçalves, P. R., et al. (2018). Neuroprotective mechanisms of resveratrol in Alzheimer's disease: role of SIRT1. Oxid. Med. Cell. Longev. 2018, 8152373. doi: 10.1155/2018/8152373
Gordon, R., Albornoz, E. A., Christie, D. C., Langley, M. R., Kumar, V., Mantovani, S., et al. (2018). Inflammasome inhibition prevents alpha-synuclein pathology and dopaminergic neurodegeneration in mice. Sci. Transl. Med. 10, eaah4066. doi: 10.1126/scitranslmed.aah4066
Grachev, I. D., Meyer, P. M., Becker, G. A., Bronzel, M., Marsteller, D., Pastino, G., et al. (2021). Sigma-1 and dopamine D2/D3 receptor occupancy of pridopidine in healthy volunteers and patients with Huntington disease: a [(18)F] fluspidine and [(18)F] fallypride PET study. Eur. J. Nucl. Med. Mol. Imaging 48, 1103–1115. doi: 10.1007/s00259-020-05030-3
Gronich, N., Abernethy, D. R., Auriel, E., Lavi, I., Rennert, G., Saliba, W., et al. (2018). beta2-adrenoceptor agonists and antagonists and risk of Parkinson's disease. Mov. Disord. 33, 1465–1471. doi: 10.1002/mds.108
Group, H. S., Frank, S., Testa, C. M., Stamler, D., Kayson, E., Davis, C., et al. (2016). Effect of deutetrabenazine on chorea among patients with Huntington disease: a randomized clinical trial. JAMA. 316, 40–50. doi: 10.1001/jama.2016.8655
Guo, Y., and Scarlata, S. A. (2013). loss in cellular protein partners promotes alpha-synuclein aggregation in cells resulting from oxidative stress. Biochemistry 52, 3913–3920. doi: 10.1021/bi4002425
Guo, Y.-J., Dong, S.-Y., Cui, X.-X., Feng, Y., Liu, T., Yin, M., et al. (2016). Resveratrol alleviates MPTP-induced motor impairments and pathological changes by autophagic degradation of alpha-synuclein via SIRT1-deacetylated LC3. Mol. Nutr. Food Res. 60, 2161–2175. doi: 10.1002/mnfr.201600111
Haque, M. E., Akther, M., Jakaria, M., Kim, I.-S., Azam, S., Choi, D.-K., et al. (2020). Targeting the microglial NLRP3 inflammasome and its role in Parkinson's disease. Mov. Disord. 35, 20–33. doi: 10.1002/mds.27874
Hervás, D., Fornés-Ferrer, V., Gómez-Escribano, A. P., Sequedo, M. D., Peir,ó, C., Millán, J. M., et al. (2017). Metformin intake associates with better cognitive function in patients with Huntington's disease. PLoS ONE. 12, e0179283. doi: 10.1371/journal.pone.0179283
Hider, R. C., and Hoffbrand, A. V. (2018). The role of deferiprone in iron chelation. N. Engl. J. Med. 379, 2140–2150. doi: 10.1056/NEJMra1800219
Holczer, M., Besze, B., Zámb,ó, V., Csala, M., Bánhegyi, G., Kapuy, O., et al. (2018). Epigallocatechin-3-gallate (EGCG) promotes autophagy-dependent survival via influencing the balance of mTOR-AMPK pathways upon endoplasmic reticulum stress. Oxid. Med. Cell. Longev. 2018, 6721530. doi: 10.1155/2018/6721530
Hou, Y., Dan, X., Babbar, M., Wei, Y., Hasselbalch, S. G., Croteau, D. L., et al. (2019). Ageing as a risk factor for neurodegenerative disease. Nat. Rev. Neurol. 15, 565–581. doi: 10.1038/s41582-019-0244-7
Huang, N., Zhang, Y., Chen, M., Jin, H., Nie, J., Luo, Y., et al. (2019). Resveratrol delays 6-hydroxydopamine-induced apoptosis by activating the PI3K/Akt signaling pathway. Exp. Gerontol. 124, 110653. doi: 10.1016/j.exger.2019.110653
Hung, L. W., Villemagne, V. L., Cheng, L., Sherratt, N. A., Ayton, S., White, A. R., et al. (2012). The hypoxia imaging agent CuII(atsm) is neuroprotective and improves motor and cognitive functions in multiple animal models of Parkinson's disease. J. Exp. Med. 209, 837–854. doi: 10.1084/jem.20112285
Huntington Study Group Reach2HD Investigators. (2015). Safety, tolerability, and efficacy of PBT2 in Huntington's disease: a phase 2, randomised, double-blind, placebo-controlled trial. Lancet Neurol. 14, 39–47. doi: 10.1016/S1474-4422(14)70262-5
Hutton, S. R., Otis, J. M., Kim, E. M., Lamsal, Y., Stuber, G. D., Snider, W. D., et al. (2017). ERK/MAPK signaling is required for pathway-specific striatal motor functions. J. Neurosci. 37, 8102–8115. doi: 10.1523/JNEUROSCI.0473-17.2017
Jankovic, J., and Tan, E. K. (2020). Parkinson's disease: etiopathogenesis and treatment. J. Neurol. Neurosurg. Psychiatry 91, 795–808. doi: 10.1136/jnnp-2019-322338
Javed, H., Nagoor Meeran, M. F., Azimullah, S., Adem, A., Sadek, B., et al. (2018). Plant extracts and phytochemicals targeting alpha-synuclein aggregation in Parkinson's disease models. Front. Pharmacol. 9, 1555. doi: 10.3389/fphar.2018.01555
Jeong, H., Then, F. Jr, T. J. M., Mazzulli, J. R., Cui, L., Savas, J. N., et al. (2009). Acetylation targets mutant huntingtin to autophagosomes for degradation. Cell 137, 60–72. doi: 10.1016/j.cell.2009.03.018
Jimenez-Sanchez, M., Licitra, F., Underwood, B. R., and Rubinsztein, D. C. (2017). Huntington's disease: mechanisms of pathogenesis and therapeutic strategies. Cold Spring Harb. Perspect. Med. 7, a024240. doi: 10.1101/cshperspect.a024240
Jin, J., Gu, H., Anders, N. M., Ren, T., Jiang, M., Tao, M., et al. (2016). Metformin protects cells from mutant huntingtin toxicity through activation of AMPK and modulation of mitochondrial dynamics. Neuromolecular Med. 18, 581–592. doi: 10.1007/s12017-016-8412-z
Joppe, K., Roser, A.-E., Maass, F., and Lingor, P. (2019). The contribution of iron to protein aggregation disorders in the central nervous system. Front. Neurosci. 13, 15. doi: 10.3389/fnins.2019.00015
Junior, N. C. F., Dos-Santos-Pereira, M., Guimarães, F. S., and Bel, E. D. (2020). Cannabidiol and cannabinoid compounds as potential strategies for treating Parkinson's disease and L-DOPA-induced dyskinesia. Neurotox. Res. 37, 12–29. doi: 10.1007/s12640-019-00109-8
Kawabata, K., Yoshioka, Y., and Terao, J. (2019). Role of intestinal microbiota in the bioavailability and physiological functions of dietary polyphenols. Molecules 24, 370. doi: 10.3390/molecules24020370
Kelley, N., Jeltema, D., Duan, Y., and He, Y. (2019). The NLRP3 inflammasome: an overview of mechanisms of activation and regulation. Int. J. Mol. Sci. 20, 3328. doi: 10.3390/ijms20133328
Khan, H., Ullah, H., and Nabavi, S. M. (2019). Mechanistic insights of hepatoprotective effects of curcumin: therapeutic updates and future prospects. Food Chem. Toxicol. 124, 182–191. doi: 10.1016/j.fct.2018.12.002
Khan, M. A., Houck, D. R., Gross, A. L., Zhang, X.-L., Cearley, C., Madsen, T. M., et al. (2018). NYX-2925 is a novel NMDA receptor-specific spirocyclic-beta-lactam that modulates synaptic plasticity processes associated with learning and memory. Int. J. Neuropsychopharmacol. 21, 242–254. doi: 10.1093/ijnp/pyx096
Koponen, M., Paakinaho, A., Lin, J., Hartikainen, S., and Tolppanen, A.-M. (2022). Identification of drugs associated with lower risk of parkinson's disease using a systematic screening approach in a nationwide nested case-control study. Clin. Epidemiol. 14, 1217–1227. doi: 10.2147/CLEP.S381289
Kou, X., and Chen, N. (2017). Resveratrol as a natural autophagy regulator for prevention and treatment of Alzheimer's disease. Nutrients 9, 927. doi: 10.3390/nu9090927
Kujawska, M., and Jodynis-Liebert, J. (2018). Polyphenols in Parkinson's disease: a systematic review of in vivo studies. Nutrients 10, 642. doi: 10.3390/nu10050642
Kurita, K. L., Glassey, E., and Linington, R. G. (2015). Integration of high-content screening and untargeted metabolomics for comprehensive functional annotation of natural product libraries. Proc. Natl. Acad. Sci. USA. 112, 11999–12004. doi: 10.1073/pnas.1507743112
Kusko, R., Dreymann, J., Ross, J., Cha, Y., Escalante-Chong, R., Garcia-Miralles, M., et al. (2018). Large-scale transcriptomic analysis reveals that pridopidine reverses aberrant gene expression and activates neuroprotective pathways in the YAC128 HD mouse. Mol. Neurodegener. 13, 25. doi: 10.1186/s13024-018-0259-3
Lefèvre-Arbogast, S., Gaudout, D., Bensalem, J., Letenneur, L., Dartigues, J. F., Hejblum, B. P., et al. (2018). Pattern of polyphenol intake and the long-term risk of dementia in older persons. Neurology 90, e1979–e1988. doi: 10.1212/WNL.0000000000005607
Levenson, J. M., Schroeter, S., Carroll, J. C., Cullen, V., Asp, E., Proschitsky, M., et al. (2016). NPT088 reduces both amyloid-beta and tau pathologies in transgenic mice. Alzheimers. Dement. 2, 141–155. doi: 10.1016/j.trci.2016.06.004
Lévy, E., Banna, N. E., Baïlle, D., Heneman-Masurel, A., Truchet, S., Rezaei, H., et al. (2019). Causative links between protein aggregation and oxidative stress: a review. Int. J. Mol. Sci. 20, 3896. doi: 10.3390/ijms20163896
Limanaqi, F., Biagioni, F., Busceti, C. L., Ryskalin, L., Polzella, M., Frati, A., et al. (2019). Phytochemicals bridging autophagy induction and alpha-synuclein degradation in Parkinsonism. Int. J. Mol. Sci. 20, 3274. doi: 10.3390/ijms20133274
Lin, K. L., Lin, K. J., Wang, P. W., Chuang, J. H., Lin, H. Y., Chen, S. D., et al. (2018). Resveratrol provides neuroprotective effects through modulation of mitochondrial dynamics and ERK1/2 regulated autophagy. Free Radic. Res. 52, 1371–1386. doi: 10.1080/10715762.2018.1489128
Liu, Q., Zhu, D., Jiang, P., Tang, X., Lang, Q., Yu, Q., et al. (2019). Resveratrol synergizes with low doses of L-DOPA to improve MPTP-induced Parkinson disease in mice. Behav. Brain Res. 367, 10–18. doi: 10.1016/j.bbr.2019.03.043
Liu, W., Wang, G., Wang, Z., Wang, G., Huang, J., Liu, B., et al. (2022). Repurposing small-molecule drugs for modulating toxic protein aggregates in neurodegenerative diseases. Drug Discov. Today. 27, 1994–2007. doi: 10.1016/j.drudis.2022.04.003
Lv, H., Wang, Y., Yang, X., Ling, G., and Zhang, P. (2022). Application of curcumin nanoformulations in Alzheimer's disease: prevention, diagnosis and treatment. Nutr. Neurosci. 1−16. doi: 10.1080/1028415X.2022.2084550
Ma, T. C., Buescher, J. L., Oatis, B., Funk, J. A., Nash, A. J., Carrier, R. L., et al. (2007). Metformin therapy in a transgenic mouse model of Huntington's disease. Neurosci. Lett. 411, 98–103. doi: 10.1016/j.neulet.2006.10.039
Maegawa, G. H. B., Tropak, M. B., Buttner, J. D., Rigat, B. A., Fuller, M., Pandit, D., et al. (2009). Identification and characterization of ambroxol as an enzyme enhancement agent for Gaucher disease. J. Biol. Chem. 284, 23502–23516. doi: 10.1074/jbc.M109.012393
Maher, P. (2019). The potential of flavonoids for the treatment of neurodegenerative diseases. Int. J. Mol. Sci. 20, 3056. doi: 10.3390/ijms20123056
Maher, P., Dargusch, R., Bodai, L., Gerard, P. E., Purcell, J. M., and Marsh, J. L. (2011). ERK activation by the polyphenols fisetin and resveratrol provides neuroprotection in multiple models of Huntington's disease. Hum. Mol. Genet. 20, 261–270. doi: 10.1093/hmg/ddq460
Martin-Bastida, A., Ward, R. J., Newbould, R., Piccini, P., Sharp, D., Kabba, C., et al. (2017). Brain iron chelation by deferiprone in a phase 2 randomised double-blinded placebo controlled clinical trial in Parkinson's disease. Sci. Rep. 7, 1398. doi: 10.1038/s41598-017-01402-2
McColgan, P., and Tabrizi, S. J. (2018). Huntington's disease: a clinical review. Eur. J. Neurol. 25, 24–34. doi: 10.1111/ene.13413
McGarry, A., Auinger, P., Kieburtz, K., Geva, M., Mehra, M., Abler, V., et al. (2020a). Additional safety and exploratory efficacy data at 48 and 60 months from open-hart, an open-label extension study of pridopidine in Huntington disease. J. Huntingtons. Dis. 9, 173–184. doi: 10.3233/JHD-190393
McGarry, A., Leinonen, M., Kieburtz, K., Geva, M., Olanow, C. W., Hayden, M., et al. (2020b). Effects of pridopidine on functional capacity in early-stage participants from the PRIDE-HD study. J. Huntingtons. Dis. 9, 371–380. doi: 10.3233/JHD-200440
McKeage, K., and Keating, G. M. (2011). Fenofibrate: a review of its use in dyslipidaemia. Drugs 71, 1917–1946. doi: 10.2165/11208090-000000000-00000
Michelson, D., Grundman, M., Magnuson, K., Fisher, R., Levenson, J. M., Aisen, P., et al. (2019). Randomized, placebo controlled trial of NPT088, a phage-derived, amyloid-targeted treatment for Alzheimer's disease. J Prev Alzheimers Dis. 6, 228–231. doi: 10.14283/jpad.2019.37
Migdalska-Richards, A., Daly, L., Bezard, E., and Schapira, A. H. V. (2016). Ambroxol effects in glucocerebrosidase and alpha-synuclein transgenic mice. Ann. Neurol. 80, 766–775. doi: 10.1002/ana.24790
Mittal, S., Bjørnevik, K., Im, D. S., Flierl, A., Dong, X., Locascio, J. J., et al. (2017). beta2-Adrenoreceptor is a regulator of the alpha-synuclein gene driving risk of Parkinson's disease. Science 357, 891–898. doi: 10.1126/science.aaf3934
Mohseni, M., Sahebkar, A., Askari, G., Johnston, T. P., Alikiaii, B., Bagherniya, M., et al. (2021). The clinical use of curcumin on neurological disorders: an updated systematic review of clinical trials. Phytother. Res. 35, 6862–6882. doi: 10.1002/ptr.7273
Morales, P., and Jagerovic, N. (2020). Novel approaches and current challenges with targeting the endocannabinoid system. Expert Opin. Drug Discov. 15, 917–930. doi: 10.1080/17460441.2020.1752178
Mullin, S., Smith, L., Lee, K., D'Souza, G., Woodgate, P., Elflein, J., et al. (2020). Ambroxol for the treatment of patients with parkinson disease with and without glucocerebrosidase gene mutations: a nonrandomized, noncontrolled trial. JAMA Neurol. 77, 427–434. doi: 10.1001/jamaneurol.2019.4611
Nagle, D. G., Ferreira, D., and Zhou, Y. D. (2006). Epigallocatechin-3-gallate (EGCG): chemical and biomedical perspectives. Phytochemistry 67, 1849–1855. doi: 10.1016/j.phytochem.2006.06.020
Naia, L., Rosenstock, T. R., Oliveira, A. M., Oliveira-Sousa, S. I., Caldeira, G. L., Carmo, C., et al. (2017). Comparative mitochondrial-based protective effects of resveratrol and nicotinamide in Huntington's disease models. Mol. Neurobiol. 54, 5385–5399. doi: 10.1007/s12035-016-0048-3
Newman, D. J., and Cragg, G. M. (2020). Natural products as sources of new drugs over the nearly four decades from 01/1981 to 09/2019. J. Nat. Prod. 83, 770–803. doi: 10.1021/acs.jnatprod.9b01285
Oertel, W., and Schulz, J. B. (2016). Current and experimental treatments of Parkinson disease: a guide for neuroscientists. J. Neurochem. 139, 325–337. doi: 10.1111/jnc.13750
Ojha, S., Javed, H., Azimullah, S., and Haque, M. E. (2016). beta-Caryophyllene, a phytocannabinoid attenuates oxidative stress, neuroinflammation, glial activation, and salvages dopaminergic neurons in a rat model of Parkinson disease. Mol. Cell. Biochem. 418, 59–70. doi: 10.1007/s11010-016-2733-y
Oprea, T. I., and Mestres, J. (2012). Drug repurposing: far beyond new targets for old drugs. AAPS J. 14, 759–763. doi: 10.1208/s12248-012-9390-1
Otani, K., and Shichita, T. (2020). Cerebral sterile inflammation in neurodegenerative diseases. Inflamm. Regen. 40, 28. doi: 10.1186/s41232-020-00137-4
Ou, Z., Pan, J., Tang, S., Duan, D., Yu, D., Nong, H., et al. (2021). Global trends in the incidence, prevalence, and years lived with disability of Parkinson's disease in 204 countries/territories from 1990 to 2019. Front Public Health 9, 776847. doi: 10.3389/fpubh.2021.776847
Paldino, E., D'Angelo, V., Laurenti, D., Angeloni, C., Sancesario, G., Fusco, F. R., et al. (2020). Modulation of inflammasome and pyroptosis by olaparib, a PARP-1 inhibitor, in the R6/2 mouse model of Huntington's disease. Cells 9, 2286. doi: 10.3390/cells9102286
Parkinson Study Group STEADY-PD III Investigators. (2020). Isradipine versus placebo in early parkinson disease: a randomized trial. Ann. Intern. Med. 172, 591–598. doi: 10.7326/M19-2534
Peball, M., Seppi, K., Krismer, F., Knaus, H.-G., Spielberger, S., Heim, B., et al. (2022). Effects of nabilone on sleep outcomes in patients with Parkinson's disease: a post-hoc analysis of NMS-Nab study. Mov Disord Clin Pract. 9, 751–758. doi: 10.1002/mdc3.13471
Peball, M., Werkmann, M., Ellmerer, P., Stolz, R., Valent, D., Knaus, H.-G., et al. (2019). Nabilone for non-motor symptoms of Parkinson's disease: a randomized placebo-controlled, double-blind, parallel-group, enriched enrolment randomized withdrawal study (The NMS-Nab Study). J. Neural Transm. 126, 1061–1072. doi: 10.1007/s00702-019-02021-z
Petrussa, E., Braidot, E., Zancani, M., Peresson, C., Bertolini, A., Patui, S., et al. (2013). Plant flavonoids–biosynthesis, transport and involvement in stress responses. Int. J. Mol. Sci. 14, 14950–14973. doi: 10.3390/ijms140714950
Pinto, N. B., Alexandre, B. D., Neves, K. R. T., Silva, A. H., Leal, L. K. A. M., Viana, G. S. B., et al. (2015). Neuroprotective properties of the standardized extract from Camellia sinensis (Green Tea) and its main bioactive components, epicatechin and epigallocatechin gallate, in the 6-OHDA model of Parkinson's disease. Evid. Based Complement. Alternat. Med. 2015, 161092. doi: 10.1155/2015/161092
Polito, C. A., Cai, Z.-Y., Shi, Y.-L., Li, X.-M., Yang, R., Shi, M., et al. (2018). Association of tea consumption with risk of Alzheimer's disease and anti-beta-amyloid effects of tea. Nutrients 10, 655. doi: 10.3390/nu10050655
Price, D. L., Koike, M. A., Khan, A., Wrasidlo, W., Rockenstein, E., Masliah, E., et al. (2018). The small molecule alpha-synuclein misfolding inhibitor, NPT200-11, produces multiple benefits in an animal model of Parkinson's disease. Sci. Rep. 8, 16165. doi: 10.1038/s41598-018-34490-9
Pushpakom, S., Iorio, F., Eyers, P. A., Escott, K. J., Hopper, S., Wells, A., et al. (2019). Drug repurposing: progress, challenges and recommendations. Nat. Rev. Drug Discov. 18, 41–58. doi: 10.1038/nrd.2018.168
Rahman, M. H., Akter, R., and Kamal, M. A. (2021). Prospective function of different antioxidant containing natural products in the treatment of neurodegenerative diseases. CNS Neurol. Disord. Drug Targets 20, 694–703. doi: 10.2174/19963181MTA4gNDED1
Reale, M., Costantini, E., Jagarlapoodi, S., Khan, H., Belwal, T., Cichelli, A., et al. (2020). Relationship of wine consumption with Alzheimer's disease. Nutrients 12, 206. doi: 10.3390/nu12010206
Repossi, G., Das, U. N., and Eynard, A. R. (2020). Molecular basis of the beneficial actions of resveratrol. Arch. Med. Res. 51, 105–114. doi: 10.1016/j.arcmed.2020.01.010
Ringman, J. M., Frautschy, S. A., Teng, E., Begum, A. N., Bardens, J., Beigi, M., et al. (2012). Oral curcumin for Alzheimer's disease: tolerability and efficacy in a 24-week randomized, double blind, placebo-controlled study. Alzheimers. Res. Ther. 4, 43. doi: 10.1186/alzrt146
Río, C. T.-D., Tortajada-Pérez, J., Gómez-Escribano, A. P., Caster,á, F., Peir,ó, C., Millán, J. M., et al. (2022). Metformin to treat Huntington disease: a pleiotropic drug against a multi-system disorder. Mech. Ageing Dev. 204, 111670. doi: 10.1016/j.mad.2022.111670
Rodrigues, F. B., and Wild, E. J. (2020). Huntington's disease clinical trials corner: April 2020. J. Huntingtons. Dis. 9, 185–197. doi: 10.3233/JHD-200002
Rodrigues, T., Reker, D., Schneider, P., and Schneider, G. (2016). Counting on natural products for drug design. Nat. Chem. 8, 531–541. doi: 10.1038/nchem.2479
Roessler, H. I., Knoers, N. V. A. M., van Haelst, M. M., and van Haaften, G. (2021). Drug repurposing for rare diseases. Trends Pharmacol. Sci. 42, 255–267. doi: 10.1016/j.tips.2021.01.003
Rosqvist, K., Schrag, A., Odin, P., and Consortium, T. C. (2022). Caregiver burden and quality of life in late stage Parkinson's disease. Brain Sci. 12, 111. doi: 10.3390/brainsci12010111
Saeedi, M., Eslamifar, M., Khezri, K., and Dizaj, S. M. (2019). Applications of nanotechnology in drug delivery to the central nervous system. Biomed. Pharmacother. 111, 666–675. doi: 10.1016/j.biopha.2018.12.133
Saeedi, M., Eslamifar, M., Khezri, K., and Dizaj, S. M. (2022). Drug delivery to the central nervous system. Nat Rev Mater. 7, 314–331. doi: 10.1038/s41578-021-00394-w
Saft, C., von Hein, S. M., Lücke, T., Thiels, C., Peball, M., Djamshidian, A., et al. (2018). Cannabinoids for treatment of dystonia in Huntington's disease. J. Huntingtons. Dis. 7, 167–173. doi: 10.3233/JHD-170283
Salehi, B., Mishra, A. P., Nigam, M., Sener, B., Kilic, M., Sharifi-Rad, M., et al. (2018). Resveratrol: a double-edged sword in health benefits. Biomedicines 6, 91. doi: 10.3390/biomedicines6030091
Sanadgol, N., Zahedani, S. S., Sharifzadeh, M., Khalseh, R., Barbari, G. R., Abdollahi, M., et al. (2017). Recent updates in imperative natural compounds for healthy brain and nerve function: a systematic review of implications for multiple sclerosis. Curr. Drug Targets 18, 1499–1517. doi: 10.2174/1389450118666161108124414
Sanchis, A., García-Gimeno, M. A., Cañada-Martínez, A. J., Sequedo, M. D., Millán, J. M., Sanz, P., et al. (2019). Metformin treatment reduces motor and neuropsychiatric phenotypes in the zQ175 mouse model of Huntington disease. Exp. Mol. Med. 51, 1–16. doi: 10.1038/s12276-019-0264-9
Sanders, M., Chandraratna, R., Marek, K., and Jennings, D. (2016). A phase 1 clinical study of the retinoid X receptor (RXR) selective agonist IRX4204 in patients with early Parkinson's disease (PD) (P2, 342.). Neurology. 86.
Savitt, D., and Jankovic, J. (2019). Targeting alpha-synuclein in Parkinson's disease: progress towards the development of disease-modifying therapeutics. Drugs 79, 797–810. doi: 10.1007/s40265-019-01104-1
Schapira, A. H. V., Chaudhuri, K. R., and Jenner, P. (2017). Non-motor features of Parkinson disease. Nat. Rev. Neurosci. 18, 435–450. doi: 10.1038/nrn.2017.62
Searles Nielsen, S., Gross, A., Camacho-Soto, A., Willis, A. W., and Racette, B. A. (2018). beta2-adrenoreceptor medications and risk of Parkinson disease. Ann. Neurol. 84, 683–693. doi: 10.1002/ana.25341
Silveira, C. R. A., MacKinley, J., Coleman, K., Li, Z., Finger, E., Bartha, R., et al. (2019). Ambroxol as a novel disease-modifying treatment for Parkinson's disease dementia: protocol for a single-centre, randomized, double-blind, placebo-controlled trial. BMC Neurol. 19, 20. doi: 10.1186/s12883-019-1252-3
Smit, J. W., Basile, P., Prato, M. K., Detalle, L., Mathy, F.-X., Schmidt, A., et al. (2022). Phase 1/1b studies of UCB0599, an Oral inhibitor of alpha-synuclein misfolding. Including a randomized study in Parkinson's disease. Mov. Disord. 37, 2045–2056. doi: 10.1002/mds.29170
Smith, M. R., Syed, A., Lukacsovich, T., Purcell, J., Barbaro, B. A., Worthge, S. A., et al. (2014). A potent and selective Sirtuin 1 inhibitor alleviates pathology in multiple animal and cell models of Huntington's disease. Hum. Mol. Genet. 23, 2995–3007. doi: 10.1093/hmg/ddu010
Spathis, A. D., Asvos, X., Ziavra, D., Karampelas, T., Topouzis, S., Cournia, Z., et al. (2017). Nurr1:RXRalpha heterodimer activation as monotherapy for Parkinson's disease. Proc. Natl. Acad. Sci. USA. 114, 3999–4004. doi: 10.1073/pnas.1616874114
Spencer, J. P. (2009). Flavonoids and brain health: multiple effects underpinned by common mechanisms. Genes Nutr. 4, 243–250. doi: 10.1007/s12263-009-0136-3
Süssmuth, S. D., Haider, S., Landwehrmeyer, G. B., Farmer, R., Frost, C., Tripepi, G., et al. (2015). An exploratory double-blind, randomized clinical trial with selisistat, a SirT1 inhibitor, in patients with Huntington's disease. Br. J. Clin. Pharmacol. 79, 465–476. doi: 10.1111/bcp.12512
Svenningsson, P., Johansson, A., Nyholm, D., Tsitsi, P., Hansson, F., Sonesson, C., et al. (2018). Safety and tolerability of IRL790 in Parkinson's disease with levodopa-induced dyskinesia-a phase 1b trial. NPJ Parkinsons Dis. 4, 35. doi: 10.1038/s41531-018-0071-3
Tabrizi, S. J., Flower, M. D., Ross, C. A., and Wild, E. J. (2020). Huntington disease: new insights into molecular pathogenesis and therapeutic opportunities. Nat. Rev. Neurol. 16, 529–546. doi: 10.1038/s41582-020-0389-4
Tabrizi, S. J., Ghosh, R., and Leavitt, B. R. (2019). Huntingtin lowering strategies for disease modification in Huntington's disease. Neuron 101, 801–819. doi: 10.1016/j.neuron.2019.01.039
Teil, M., Arotcarena, M.-L., Faggiani, E., Laferriere, F., Bezard, E., Dehay, B., et al. (2020). Targeting alpha-synuclein for PD Therapeutics: a pursuit on all fronts. Biomolecules 10, 391. doi: 10.3390/biom10030391
Teleanu, D. M., Negut, I., Grumezescu, V., Grumezescu, A. M., and Teleanu, R. I. (2019). Nanomaterials for drug delivery to the central nervous system. Nanomaterials 9, 371. doi: 10.3390/nano9030371
Terahara, N. (2015). Flavonoids in foods: a review. Nat. Prod. Commun. 10, 521–528. doi: 10.1177/1934578X1501000334
Trist, B. G., Hare, D. J., and Double, K. L. (2019). Oxidative stress in the aging substantia nigra and the etiology of Parkinson's disease. Aging Cell 18, e13031. doi: 10.1111/acel.13031
Troncoso-Escudero, P., Sepulveda, D., Pérez-Arancibia, R., Parra, A. V., Arcos, J., Grunenwald, F., et al. (2020). On the right track to treat movement disorders: promising therapeutic approaches for Parkinson's and Huntington's DISEASE. Front. Aging Neurosci. 12, 571185. doi: 10.3389/fnagi.2020.571185
Uddin, M. S., Mamun, A. A., Rahman, M. M., Jeandet, P., Alexiou, A., Behl, T., et al. (2021). Natural products for neurodegeneration: regulating neurotrophic signals. Oxid. Med. Cell. Longev. 2021, 8820406. doi: 10.1155/2021/8820406
Van der Schyf, C. J. (2015). Rational drug discovery design approaches for treating Parkinson's disease. Expert Opin. Drug Discov. 10, 713–741. doi: 10.1517/17460441.2015.1041495
Varga, J., Dér, N. P., Zsindely, N., and Bodai, L. (2020). Green tea infusion alleviates neurodegeneration induced by mutant Huntingtin in Drosophila. Nutr. Neurosci. 23, 183–189. doi: 10.1080/1028415X.2018.1484021
Vauzour, D. (2014). Effect of flavonoids on learning, memory and neurocognitive performance: relevance and potential implications for Alzheimer's disease pathophysiology. J. Sci. Food Agric. 94, 1042–1056. doi: 10.1002/jsfa.6473
Vauzour, D., Vafeiadou, K., Rodriguez-Mateos, A., Rendeiro, C., and Spencer, J. P. E. (2008). The neuroprotective potential of flavonoids: a multiplicity of effects. Genes Nutr. 3, 115–126. doi: 10.1007/s12263-008-0091-4
Vázquez-Manrique, R. P., Farina, F., Cambon, K., Sequedo, M. D., Parker, A. J., Millán, J. M., et al. (2016). AMPK activation protects from neuronal dysfunction and vulnerability across nematode, cellular and mouse models of Huntington's disease. Hum. Mol. Genet. 25, 1043–1058. doi: 10.1093/hmg/ddv513
Vidoni, C., Secomandi, E., Castiglioni, A., Melone, M. A. B., and Isidoro, C. (2018). Resveratrol protects neuronal-like cells expressing mutant Huntingtin from dopamine toxicity by rescuing ATG4-mediated autophagosome formation. Neurochem. Int. 117, 174–187. doi: 10.1016/j.neuint.2017.05.013
Voulgaropoulou, S. D., van Amelsvoort, T. A. M. J., Prickaerts, J., and Vingerhoets, C. (2019). The effect of curcumin on cognition in Alzheimer's disease and healthy aging: a systematic review of pre-clinical and clinical studies. Brain Res. 1725, 146476. doi: 10.1016/j.brainres.2019.146476
Wan, H., Rehngren, M., Giordanetto, F., Bergström, F., and Tunek, A. (2007). High-throughput screening of drug-brain tissue binding and in silico prediction for assessment of central nervous system drug delivery. J. Med. Chem. 50, 4606–4615. doi: 10.1021/jm070375w
Wang, J., Bi, W., Zhao, W., Varghese, M., Koch, R. J., Walker, R. H., et al. (2016). Selective brain penetrable Nurr1 transactivator for treating Parkinson's disease. Oncotarget 7, 7469–7479. doi: 10.18632/oncotarget.7191
Wang, K., Gao, Q., Zhang, T., Rao, J., Ding, L., Qiu, F., et al. (2020). Inhibition of CYP2C9 by natural products: insight into the potential risk of herb-drug interactions. Drug Metab. Rev. 52, 35–257. doi: 10.1080/03602532.2020.1758714
Wang, S., Yuan, Y.-H., Chen, N.-H., and Wang, H.-B. (2019). The mechanisms of NLRP3 inflammasome/pyroptosis activation and their role in Parkinson's disease. Int. Immunopharmacol. 67, 458–464. doi: 10.1016/j.intimp.2018.12.019
Wang, Y., Wu, S., Li, Q., Lang, W., Li, W., Jiang, X., et al. (2022). Epigallocatechin-3-gallate: a phytochemical as a promising drug candidate for the treatment of Parkinson's disease. Front. Pharmacol. 13, 977521. doi: 10.3389/fphar.2022.977521
Waters, S., Sonesson, C., Svensson, P., Tedroff, J., Carta, M., Ljung, E., et al. (2020). Preclinical pharmacology of [2-(3-fluoro-5-methanesulfonylphenoxy)ethyl](propyl)amine (IRL790), a novel dopamine transmission modulator for the treatment of motor and psychiatric complications in Parkinson's disease. J. Pharmacol. Exp. Ther. 374, 113–125. doi: 10.1124/jpet.119.264226
Waters, S., Tedroff, J., Ponten, H., Klamer, D., Sonesson, C., Waters, N., et al. (2018). Pridopidine: overview of pharmacology and rationale for its use in Huntington's disease. J. Huntingtons. Dis. 7, 1–16. doi: 10.3233/JHD-170267
Wrasidlo, W., Tsigelny, I. F., Price, D. L., Dutta, G., Rockenstein, E., Schwarz, T. C., et al. (2016). A de novo compound targeting alpha-synuclein improves deficits in models of Parkinson's disease. Brain 139(Pt 12), 3217–36. doi: 10.1093/brain/aww238
Xu, Y., Zhang, Y., Quan, Z., Wong, W., Guo, J., Zhang, R., et al. (2016). Epigallocatechin gallate (EGCG) inhibits alpha-synuclein aggregation: a potential agent for Parkinson's disease. Neurochem. Res. 41, 2788–2796. doi: 10.1007/s11064-016-1995-9
Yero, T., and Rey, J. A. (2008). Tetrabenazine (xenazine), an FDA-approved treatment option for Huntington's disease-related chorea. P T. 33, 690–694.
Zhang, H., Bai, L., He, J., Zhong, L., Duan, X., Ouyang, L., et al. (2017). Recent advances in discovery and development of natural products as source for anti-Parkinson's disease lead compounds. Eur. J. Med. Chem. 141, 257–272. doi: 10.1016/j.ejmech.2017.09.068
Zhang, L.-F., Yu, X.-L., Ji, M., Liu, S.-Y., Wu, X.-L., Wang, Y.-J., et al. (2018). Resveratrol alleviates motor and cognitive deficits and neuropathology in the A53T alpha-synuclein mouse model of Parkinson's disease. Food Funct. 9, 6414–6426. doi: 10.1039/C8FO00964C
Keywords: Parkinson's disease, Huntington's disease, drug repurposing, pharmacological therapy, small molecules, natural products
Citation: Pérez-Arancibia R, Cisternas-Olmedo M, Sepúlveda D, Troncoso-Escudero P and Vidal RL (2023) Small molecules to perform big roles: The search for Parkinson's and Huntington's disease therapeutics. Front. Neurosci. 16:1084493. doi: 10.3389/fnins.2022.1084493
Received: 30 October 2022; Accepted: 20 December 2022;
Published: 09 January 2023.
Edited by:
Vanessa Olzon Zambelli, Butantan Institute, BrazilReviewed by:
Ana Carolina Pinheiro Campos, Hospital Sirio Libanes, BrazilCopyright © 2023 Pérez-Arancibia, Cisternas-Olmedo, Sepúlveda, Troncoso-Escudero and Vidal. This is an open-access article distributed under the terms of the Creative Commons Attribution License (CC BY). The use, distribution or reproduction in other forums is permitted, provided the original author(s) and the copyright owner(s) are credited and that the original publication in this journal is cited, in accordance with accepted academic practice. No use, distribution or reproduction is permitted which does not comply with these terms.
*Correspondence: Rene L. Vidal, cmVuZS52aWRhbEB1bWF5b3IuY2w=
Disclaimer: All claims expressed in this article are solely those of the authors and do not necessarily represent those of their affiliated organizations, or those of the publisher, the editors and the reviewers. Any product that may be evaluated in this article or claim that may be made by its manufacturer is not guaranteed or endorsed by the publisher.
Research integrity at Frontiers
Learn more about the work of our research integrity team to safeguard the quality of each article we publish.