- 1Department of Biological Sciences, Western Michigan University, Kalamazoo, MI, United States
- 2Department of Biology, University of Iowa, Iowa City, IA, United States
- 3Department of Biomedical Sciences, Western Michigan University Homer Stryker MD School of Medicine, Kalamazoo, MI, United States
During development the afferent neurons of the inner ear make precise wiring decisions in the hindbrain reflective of their topographic distribution in the periphery. This is critical for the formation of sensory maps capable of faithfully processing both auditory and vestibular input. Disorganized central projections of inner ear afferents in Fzd3 null mice indicate Wnt/PCP signaling is involved in this process and ear transplantation in Xenopus indicates that Fzd3 is necessary in the ear but not the hindbrain for proper afferent navigation. However, it remains unclear in which cell type of the inner ear Fzd3 expression is influencing the guidance of inner ear afferents to their proper synaptic targets in the hindbrain. We utilized Atoh1-cre and Neurod1-cre mouse lines to conditionally knockout Fzd3 within the mechanosensory hair cells of the organ of Corti and within the inner ear afferents, respectively. Following conditional deletion of Fzd3 within the hair cells, the central topographic distribution of inner ear afferents was maintained with no gross morphological defects. In contrast, conditional deletion of Fzd3 within inner ear afferents leads to central pathfinding defects of both cochlear and vestibular afferents. Here, we show that Fzd3 is acting in a cell autonomous manner within inner ear afferents to regulate central pathfinding within the hindbrain.
Introduction
External information is relayed to the brain through precisely organized neural circuits that allow for sensory perception of the immediate environment (Cang and Feldheim, 2013; Fritzsch et al., 2019). The development of this neural wiring involves the growth and navigation of axons to their proper synaptic targets, often over large distances. Navigating axons integrate attractive and repulsive guidance information from the environment for directed extension (Kolodkin and Tessier-Lavigne, 2011). This guidance information comes in the form of both secreted and extracellular matrix-associated molecular cues of varying spatial distribution. Interpretation of these signals by growing axons into an appropriate chemotropic response allows for navigation and the formation of functional synapses with their targets (Maler et al., 1974; Chilton, 2006; Chai et al., 2015; Russell and Bashaw, 2017). This synaptic connection between sensory neurons and their centrally located targets generates a sensory map capable of faithfully transmitting information from the afferent divisions of sensory organs to their respective nuclei (Fritzsch et al., 2019).
The afferent neurons of the inner ear make precise wiring decisions in the hindbrain reflective of the topographic distribution of hair cells in the periphery (Maklad and Fritzsch, 2003; Appler and Goodrich, 2011). These specific decisions are essential for assembling the neuronal circuits dedicated for processing auditory or vestibular input. Auditory and vestibular signals are relayed from the periphery to the hindbrain by spiral ganglion neurons (SGNs) and vestibular afferents, respectively. SGNs send peripheral projections into the cochlea, where they innervate tonotopically organized hair cells. Within the cochlea, frequencies are encoded across a gradient with higher frequencies detected by hair cells in the base and lower frequencies detected by hair cells in the apex (Warr and Guinan, 1979; Bourk et al., 1981; Luo et al., 2009; Meyer et al., 2009). SGNs send their central processes to the cochlear nucleus (CN) located within the hindbrain, maintaining their tonotopic organization. Upon entering the hindbrain, SGNs will bifurcate, sending descending processes toward the posteroventral cochlear nucleus (PVCN) and dorsal cochlear nucleus (DCN), as well as ascending processes toward the anteroventral cochlear nucleus (AVCN), where they synapse on second order neurons within these nuclei (Wickesberg and Oertel, 1988; Ryugo and Parks, 2003). Vestibular afferents innervate the various peripheral sensory organs of the vestibular system and send central projections to specific areas of the vestibular nucleus (VN) and cerebellum (Dickman and Fang, 1996; Maklad and Fritzsch, 2003). While vestibular afferents and SGNs project together toward the hindbrain as the vestibulocochlear nerve, within the nerve, these two populations of neurons remain segregated, maintaining topographic fidelity to their respective peripheral sensory organs (Fritzsch, 1981).
The mechanisms that guide inner ear afferents to their peripheral hair cell targets has been a long-standing question (Fekete and Campero, 2007; Coate and Kelley, 2013; Coate et al., 2015). However, relatively little is known about the molecular guidance associated with central pathfinding. Remarkably, across all vertebrate species, auditory and vestibular afferents target the same respective dorsoventral columns of the hindbrain, indicating that central pathfinding may be occurring through a set of conserved guidance mechanisms (Duncan and Fritzsch, 2012). In support of this, transplantation studies in Xenopus and chick have suggested that inner ear afferents respond to conserved guidance cues during central circuit assembly (Elliott et al., 2013, 2015; Elliott and Fritzsch, 2018; Gordy et al., 2018). Furthermore, mouse SGNs project to the CN even in the absence of a majority of their second order synaptic targets (Maricich et al., 2009; Elliott et al., 2017), implying that the target cells themselves are not necessary for proper guidance. Taken together, these studies indicate that inner ear afferents may be responding to conserved spatiotemporal gradients of diffusible guidance cues for proper central pathfinding. Wnt/PCP signaling in the CNS is conserved across vertebrates and may be a promising avenue (Goodrich, 2008). Two Wnt/PCP signaling components, Prickle1 (Yang et al., 2017) and Frizzled3 (Fzd3) (Wang et al., 2002; Duncan et al., 2019), have been shown to be involved in central circuit assembly. In Prickle1 mutants, apical SGNs aberrantly cover a more expansive area of the CN and overlap with basal fibers (Yang et al., 2017). A similar phenotype is observed in Fzd3 null mutants, in which apical SGNs project in a disorganized manner within the CN (Duncan et al., 2019). Additionally, in Fzd3 null mutants, some vestibular afferents will project out of the VN and into the CN in mice or into the lateral line nucleus in frogs, indicating a reliance on Fzd3 within vestibular afferents as well (Duncan et al., 2019). Transplantation of ears between control and Fzd3 knockdown Xenopus embryos demonstrated that a loss of Fzd3 within the hindbrain did not result in aberrant vestibular afferent projections, whereas afferent projections were aberrant when Fzd3 expression was downregulated within the inner ear (Duncan et al., 2019). This suggests that it is Fzd3 expression within the ear and not in the hindbrain that is necessary for central guidance of auditory and vestibular afferents. Fzd3 is expressed throughout the inner ear, including inner ear afferents (Lu et al., 2011; Ghimire et al., 2018) and hair cells. Within the inner ear afferents, Fzd3 is expressed in a gradient, with a higher expression in the apex compared to the base (Duncan et al., 2019). This distinctive expression pattern suggests that the differential level of Fzd3 expression may contribute to the proper segregation of SGNs to their respective regions of the CN; however, it remains unclear if Fzd3 is acting in a cell autonomous manner within inner ear afferents or if Fzd3 expression within their peripheral hair cell targets is the root of the central defects observed in mutants (Duncan et al., 2019). Either scenario seems likely as Fzd3 has been shown to have a cell autonomous role in some instances (Wang et al., 2002; Chai et al., 2014), while in others, it is the expression of Fzd3 within other cell types that is critical for proper axon pathfinding (Qu et al., 2014).
In the present work, we utilized two Fzd3 conditional knockout models to selectively eliminate Fzd3 from individual cell populations within the inner ear. We used Neurod1-cre (Li et al., 2012) to recombine floxed Fzd3 (Hua et al., 2013) within inner ear afferents and Atoh1-cre (Matei et al., 2005) to recombine Fzd3 within inner ear hair cells (Wang et al., 2005; Fujiyama et al., 2009; Yang et al., 2010). Our results show that Fzd3-mediated regulation of inner ear afferent central pathfinding relies on the expression of Fzd3 within inner ear afferents.
Materials and Methods
Ethics Statement
All animal protocols used in these studies were approved by the Institutional Animal Care and Use Committee at Western Michigan University (20-11-01).
Mice
Atoh1-cre:Fzd3f/f mice were produced by breeding Atoh1-cre:Fzd3f/+ males with Fzd3f/f or Fzd3f/+ females (Matei et al., 2005; Hua et al., 2013). Neurod1-cre:Fzd3f/f mice were produced by breeding Neurod1-cre:Fzdf/+ males with Fzd3f/f or Fzd3f/+ females (Li et al., 2012; Hua et al., 2013). All experiments were performed at postnatal day 0 (P0). Neonates were anesthetized by intraperitoneal injection of a lethal dose of Avertin (1.25% of 2.2.2-tribromoethanol) at a dose of 480 mg/kg and then perfused with 4% PFA using a peristaltic pump. Samples were stored in 4% PFA at 4°C until time of experiment. At least three biological replicates were used for each genotype in all experiments.
Immunohistochemistry
To characterize the specific cell types in which Cre recombination would occur in both the Atoh1-cre and Neurod1-cre transgenic mouse lines, immunohistochemistry was performed on the cochleae of P0 mice. After fixation, ears were dissected to expose the cochleae. Samples were left in 1X PBS for 1 h. Samples were then washed in 0.05% Tween-20/PBS. Blocking was performed using 5% donkey serum, 0.5% Triton-X, and 1% bovine serum albumin in 1X PBS at room temperature on a rocker. After a quick rinse using 0.05% Tween-20/PBS, primary antibodies were added to the samples. Primary antibodies include Myo7a rabbit (1:500, Catalog #25-6790, Proteus Biosciences, Inc.) and Neurofilament Heavy Chain 200 kDa (NF200) chick (1:200, Catalog ID NFH, Aves), diluted in blocking solution. Samples were left in primary antibody solution at 4°C on a rocker for 2 days. Samples were then rinsed four times with 0.05% Tween-20/PBS, with each rinse being 30 min in duration. A fluorescent secondary (anti-rabbit, 1:1,000, Catalog #A31573 Invitrogen, or anti-chick, 1:1,000, Catalog #A11039, Invitrogen) was diluted in blocking solution and then added to each sample. Samples were left overnight at 4°C on a rocker. Samples were then washed three times with 0.05% Tween-20/PBS, with each wash being 30 min in duration. Samples were then mounted on glass slides using glycerol and imaged with a Nikon C2 Confocal Microscope.
RNAscope
To confirm the loss of Fzd3 in the conditional knockout mouse models, RNAscope® was used to label individual Fzd3 mRNA in the inner ears from P0 Atoh1-cre:Fzd3f/f, Neurod1-cre:Fzd3f/f, and respective control littermate mice. Three ears from each genotype were removed from the skull, post-fixed in 4% PFA for an hour, and stored in 70% EtOH until processing. Inner ears were then dissected in 70% EtOH to remove the cartilage around the entire cochlea and the ventral most part of the vestibular portion. Dissected inner ears were processed as previously described for whole-mount RNAscope® (Kersigo et al., 2018). Ears were transferred to 70% MeOH, dehydrated to 100% MeOH, and rehydrated through a series of MeOH/0.1% Tween 20 in RNase-free phosphate-buffered saline (PBT) dilutions. Ears were treated with Protease III solution (Advanced Cell Diagnostics 322337, 1x) for 18 min and washed three times in PBT for 5 min each. Ears were incubated in probe solution for Fzd3 (Advanced Cell Diagnostics, 404891, C1) and Ubiquitin C (Advanced Cell Diagnostics, 310771, C3) for ears from Atoh1-cre:Fzd3f/f and control littermates and in probe solution for Fzd3 (Advanced Cell Diagnostics, 404891, C1) and TrkC/Ntrk3 to label inner ear neurons (Fariñas et al., 2001; Green et al., 2012; Kersigo et al., 2018; Duncan et al., 2019) (Advanced Cell Diagnostics, 423621, C2) for ears from Neurod1-cre:Fzd3f/f and control littermates. Ears were incubated in their respective probe solutions overnight at 40°C with gentle agitation following manufacturer’s instructions. After overnight incubation, the probe solution was removed, and ears were washed in RNase-free 0.2x saline sodium citrate (SSC) three times for 15 min each. Ears were incubated in AMP1 solution (Advanced Cell Diagnostics, 1x) at 40°C for 35 min and washed three times in SSC. Ears were then incubated in AMP2 solution (Advanced Cell Diagnostics, 1x) at 40°C for 20 min and washed three times in SSC. Ears were incubated in AMP3 solution (Advanced Cell Diagnostics, 1x) at 40°C for 35 min and washed three times in SSC. Ears were incubated in AMP4 solution (Advanced Cell Diagnostics, Alt C, 1x) at 40°C for 20 min and washed three times in SSC.
Ears from Atoh1-cre:Fzd3f/f and control littermates were transferred to PBS, blocked for 1 h in 5% normal goat serum (NGS), and incubated with antibody against Myo7a (1:400; Proteus Biosciences, Inc.) in PBS overnight at 37°C to label hair cells. Ears were washed three times in PBS for 1 h each before blocking in 5% NGS/0.1% Triton X-100 in PBS. Ears were incubated in goat-anti-rabbit 647 secondary antibody (1:500; Alexa) and Hoechst stain (1:1,000) in PBS overnight at 4°C. Ears were washed three times in PBS for 1 h each. Ears from Neurod1-cre:Fzd3f/f and control littermates were incubated in a DAPI solution (Advanced Cell Diagnostics, 1x) at 4°C overnight and washed three times in PBS. All ears were mounted on a slide in Vectashield® (Vector Laboratories, Inc.). Images were acquired using a Leica SP8 confocal microscope with LAS X software.
Lipophilic Dye Tracing
To label specific inner ear afferents, tissue on the exterior of the skull was dissected to reveal the lateral half of the inner ear. For cochlear labeling, pieces of lipophilic dye-soaked filter paper were placed in the base of the cochlea (NeuroVue® Red) and in the apex of the cochlea (NeuroVue® Jade). For vestibular labeling, lipophilic dye-soaked filter paper was placed at the junction of the anterior cristae and utricle (NeuroVue® Maroon) (Tonniges et al., 2010; Duncan et al., 2011). Placement was consistent for both control and mutant samples. Heads were then placed in vials containing 4% PFA and incubated at 37°C for 3 days to allow for dye diffusion. Following incubation, heads were hemisected and the brain was removed. The brain was flat-mounted with lateral side up on a slide in glycerol and imaged within 1 h of preparation. All imaging was performed using a Nikon Confocal C2 Microscope with NIS software.
Afferent Reconstruction
To reconstruct individual afferents, Z-stack images were loaded into Fiji-ImageJ software. Ten individual apical afferents per sample were manually traced in the AVCN using the Simple Neurite Tracer plugin under the Segmentation tab by selecting points along a single fiber. Selected afferents were approximately 10 μm apart throughout the entire AVCN. The tracing started after the bifurcation of the neurite. If an axon could not be traced at the exact 10 μm distance, the next closest axon was selected. Images were imported into CorelDraw software and individual tracings were relabeled with different colors denoting their position within the AVCN. Neurons from three mutant and three littermate control mice were analyzed.
Quantification of Axon Crossings
The reconstructed axons were examined for the number of times that an axon crossed another axon when projecting. The number of times that axons crossed one another was averaged and standard deviation was calculated in both control and mutant samples. A Student’s t-test was performed to assess significance of the data. Significance was determined at p < 0.05.
Results
Central Tonotopic Organization of Spiral Ganglion Neurons Is Unaffected by Loss of Fzd3 Within Peripheral Hair Cells
Since Fzd3 has been shown to be expressed within the cochlear hair cells (Wang et al., 2006; Stuebner et al., 2010; Qu et al., 2014; Duncan et al., 2019), we assessed whether loss of Fzd3 in this cellular population affected central pathfinding of SGNs through a non-cell autonomous manner. The basic helix-loop-helix (bHLH) transcription factor Atoh1 is expressed in hair cells and has been shown to play a critical role in their fate determination and differentiation (Bermingham et al., 1999). Thus, we used an Atoh1-cre line that has been shown by several studies to drive Cre expression within inner ear hair cells (Supplementary Figure 1; Matei et al., 2005; Perrin et al., 2010, 2013; Ueyama et al., 2014; Tarchini et al., 2016; Kannan-Sundhari et al., 2020) to conditionally delete Fzd3 (Supplementary Figure 1). Although we found variability in Atoh1-cre driving tdTomato expression in supporting cells, all samples expressed tdTomato within hair cells and there was no evidence of tdTomato in inner ear afferents. Application of lipophilic dyes into the base (red) and apex (green) of control cochleae showed that SGNs project in an organized manner toward and within the CN, with basal and apical fibers remaining segregated based on their tonotopic distribution in the periphery (Figures 1A,C), consistent with previous morphological reports (Wickesberg and Oertel, 1988; Ryugo and Parks, 2003). Similarly in Atoh1-cre:Fzd3f/f mutants, tonotopic organization is maintained with basal fibers projecting more dorsally than apical fibers (Figures 1B,D). Overall, there appears to be no difference in tonotopic distribution of SGNs within the CN between control (Figures 1A,B) and Atoh1-cre:Fzd3f/f mutants (Figures 1B,D). This indicates that Fzd3 expression within hair cells is not necessary for proper central pathfinding of SGNs.
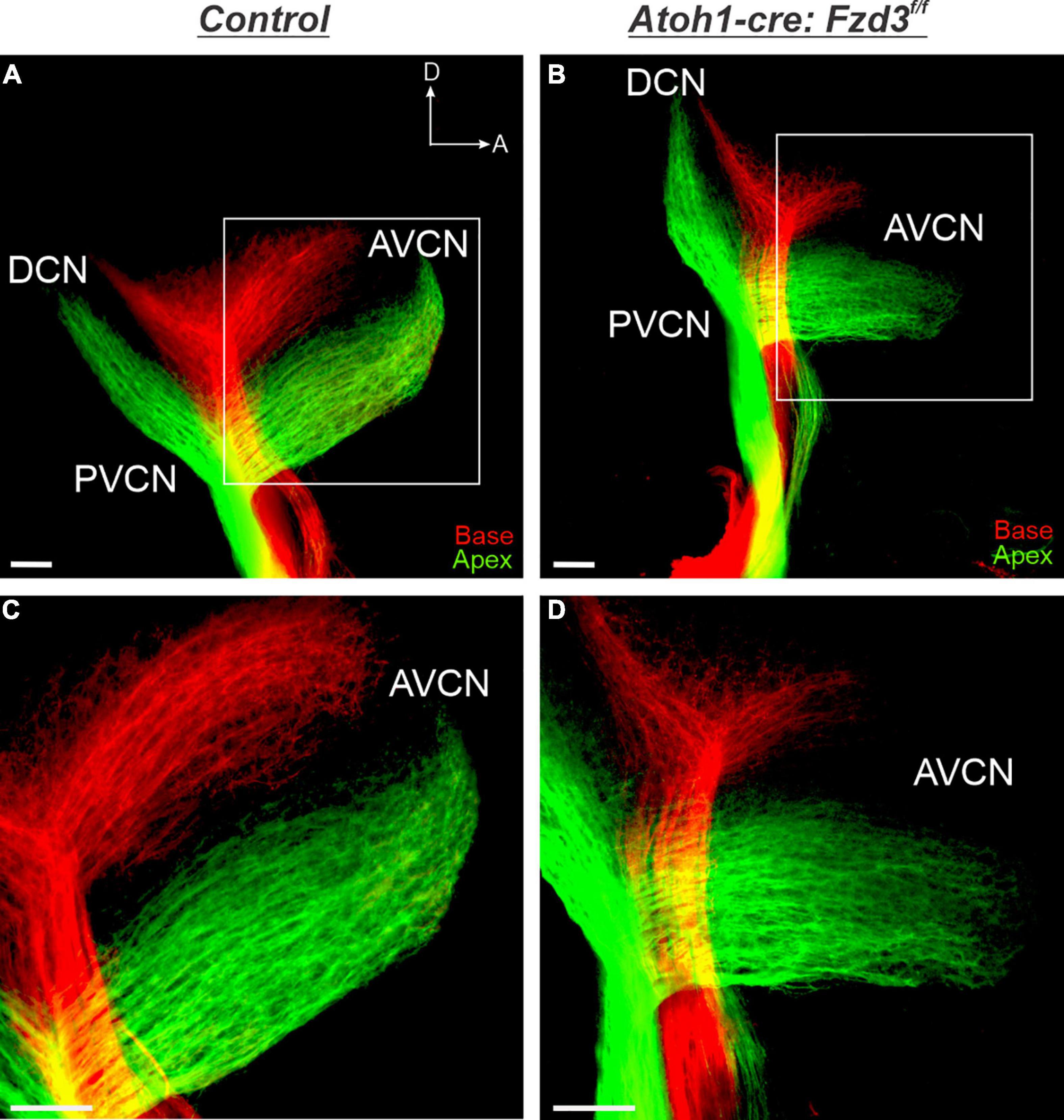
Figure 1. Cochlear afferent central pathfinding unaffected by loss of Fzd3 within hair cells. Lipophilic dyes were applied to the base (red) and apex (green) of control (A,C) and Atoh1-cre:Fzd3f/f mutant (B,D) cochleae at P0 and their central projections were analyzed (A–D). In the control, afferent neurites from the base and apex remain segregated and bifurcate sending projections toward the DCN and AVCN (A,C). In the Atoh1-cre:Fzd3f/f mutant, afferent neurites from the base and apex also remain segregated and bifurcate sending projections toward the DCN and AVCN (B,D). Higher magnification of the AVCN (C,D) showing the segregation of basal and apical afferents in both control (C) and Atoh1-cre:Fzd3f/f mutant (D) central projections. CN, cochlear nucleus; AVCN, anteroventral cochlear nucleus; DCN, dorsal cochlear nucleus; PVCN, posteroventral cochlear nucleus; A, anterior; D, dorsal. Orientation for all panels the same as (A). Scale bars, 100 μm. Three mutant animals and three littermate controls were analyzed.
To confirm that there was no difference between control and Atoh1-cre:Fzd3f/f mice central projections, we investigated the trajectories of afferents as they projected within the AVCN. To this end, ten apical SGNs were traced per animal for each of three control and three Atoh1-cre:Fzd3f/f mutants. In both control and Atoh1-cre:Fzd3f/f mice, adjacent fibers preserved relative separation between each other with infrequent path crossing events (Figures 2A,B). The number of times that individual axons crossed one another within the AVCN did not statistically differ between control and Atoh1-cre:Fzd3f/f mutants (Student’s t-test, p > 0.05) (Figure 2D). These findings indicate that Fzd3 expression within inner ear hair cells does not influence the tonotopic distribution of SGNs within the hindbrain.
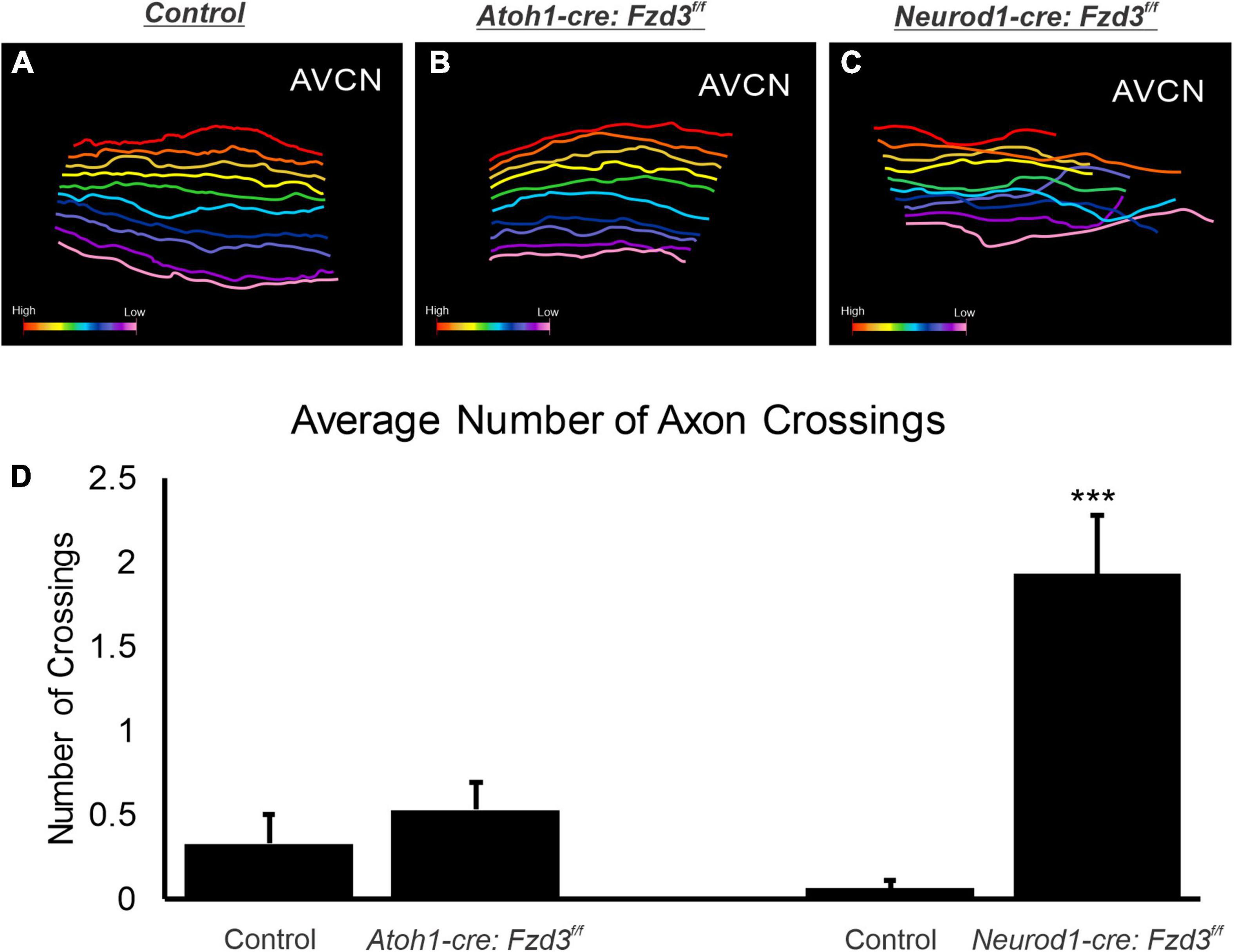
Figure 2. Analysis of cochlear afferent central projections following conditional deletion of Fzd3. Reconstruction of 10 evenly spaced apical afferents in the AVCN of control (A), Atoh1-cre:Fzd3f/f mutant (B), and Neurod1-cre:Fzd3f/f mutant (C). Colored gradient bar represents the transition from higher frequency reconstructed fibers (red) to lower frequency reconstructed fibers (pink) (A–C). The mean number of times an axon crossed another axon in littermate control and mutant samples (D). Error bars represent standard error of the mean. ***p < 0.001.
Loss of Fzd3 in Spiral Ganglion Neurons Results in Aberrant Tonotopic Organization Within the Cochlear Nucleus
Since Fzd3 has also been shown to be expressed in cochlear afferents (Supplementary Figure 1; Lu et al., 2011; Ghimire et al., 2018; Duncan et al., 2019), we next investigated if expression specifically within SGNs was influencing proper central pathfinding within the CN. The proneural bHLH transcription factor Neurod1 has been shown to play a role in inner ear afferents early during differentiation (Appler and Goodrich, 2011; Macova et al., 2019). We characterized a Neurod1-cre mouse line (Li et al., 2012) by combining it with a tdTomato reporter (Neurod1-cre:tdTomato+) to verify that Cre expression was specifically driven within inner ear afferent neurons (Supplementary Figure 1). Neurod1 is expressed in SGNs beginning at E8.75 (Liu et al., 2000) and therefore eliminates Fzd3 before inner ear afferents delaminate from the otocyst (Ruben, 1967; Kim et al., 2001). As before, control littermates showed the stereotypical projections of apical and basal SGNs as visualized by application of lipophilic dyes into the base (red) and apex (green) (Figures 3A,C). In contrast, in Neurod1-cre:Fzd3f/f mice, SGNs did not maintain the same degree of segregation between basal and apical fibers. Frequently, apical fibers would project dorsally into regions typically innervated by basal fibers. This dorsal projection of apical fibers occurred in both the AVCN and PVCN of Neurod1-cre:Fzdf/f mutant mice (Figures 3B,D). Even within the apical SGNs alone, many projections in the AVCN of Neurod1-cre:Fzd3f/f mutant mice did not maintain the same degree of tonotopic organization (Figure 3F) when compared to control mice (Figure 3E). To better visualize this divergence from controls, 10 apical SGNs were traced for both 3 control and 3 Neurod1-cre:Fzd3f/f mutants within the AVCN. In control mice, adjacent fibers maintained parallel separation between each other with infrequent path crossing events (Figure 2A). In contrast, apical fibers from Neurod1-cre:Fzd3f/f mice, frequently intersected with adjacent fibers as they projected (Figure 2C). The number of times that individual axons crossed one another within the AVCN was significantly greater in Neurod1-cre:Fzd3f/f mice (Figure 2D) than in controls (Figure 2D) (Student’s t-test, p < 0.001). Overall, these findings suggest that Fzd3 is acting in a cell autonomous manner within SGNs to influence central pathfinding within the CN.
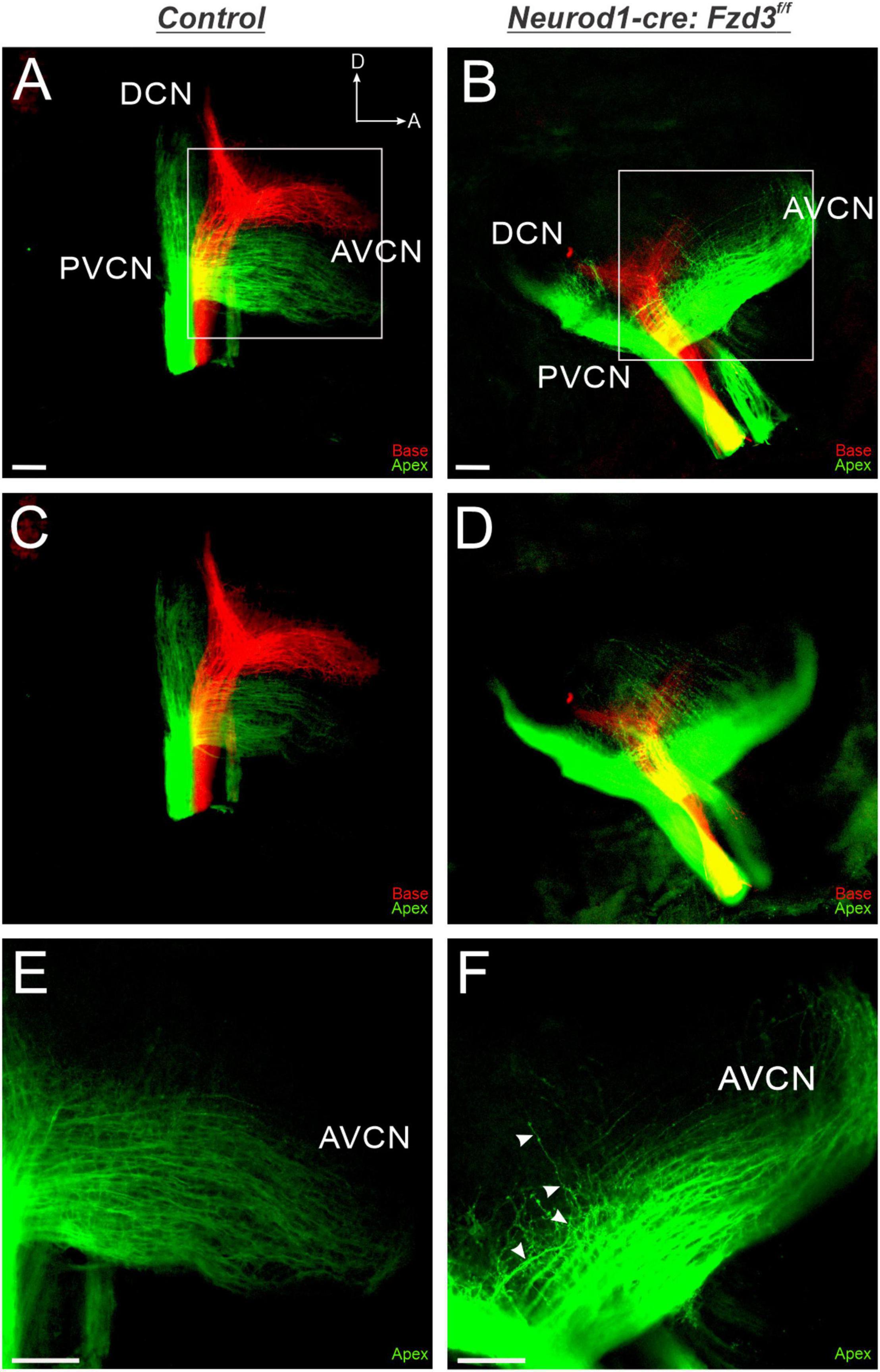
Figure 3. Loss of Fzd3 in cochlear afferents results in central pathfinding defects. Lipophilic dyes were applied to the base (red) and apex (green) of control (A,C,E) and Neurod1-cre:Fzd3f/f mutant (B,D,F) cochleae at P0 and their central projections were analyzed (A–F). In the control, afferent neurites from the base and apex remain segregated and bifurcate, sending projections toward the DCN and AVCN (A,C). In contrast some apical afferents in Neurod1-cre:Fzd3f/f mutants project more dorsally, resulting in a loss of segregation between basal and apical fibers (B,D). Stack images rendered at different depths in the CN for control (C) and Neurod1-cre:Fzd3f/f mutant (D) central projections. Higher magnification of the AVCN (E,F) showing apical afferents projecting parallel to each other in the control (D). Some apical afferents in the Neurod1-cre:Fzd3f/f mutant (F) do not maintain separation from other fibers as they project toward the AVCN resulting in frequent crossover events. The white arrows show the trajectory of a single fiber as it projects in the AVCN of the Neurod1-cre:Fzd3f/f mutant (F). CN, cochlear nucleus; AVCN, anteroventral cochlear nucleus; DCN, dorsal cochlear nucleus; PVCN, posteroventral cochlear nucleus; A, anterior; D, dorsal. Orientation for all panels the same as (A). Scale bars, 100 μm. Three mutant animals and three littermate controls were analyzed.
Loss of Fzd3 in Vestibular Afferents Results in Central Pathfinding Defects
Given that Fzd3 is acting in a cell autonomous manner within SGNs for proper central pathfinding (Figures 1–3), we next investigated whether Fzd3 plays a similar functional role within vestibular afferents. Since Fzd3 is expressed in vestibular afferents (Lu et al., 2011; Ghimire et al., 2018) as well as their peripheral hair cell targets (Supplementary Figure 1; Wang et al., 2006), we evaluated in which of these cell types Fzd3 expression contributes to proper vestibular afferent central pathfinding. To achieve this, we utilized Atoh1-cre and Neurod1-cre lines to conditionally delete Fzd3 from the vestibular hair cells and the vestibular afferents, respectively. Application of lipophilic dyes into the utricle/anterior cristae (cyan) and apex (yellow outline) of control mice revealed normal projection of vestibular afferents toward the VN, with stereotyped segregation from SGNs (Figures 4A,D). Similarly, in Atoh1-cre:Fzd3f/f mutants, SGNs and vestibular afferents remain segregated (Figures 4B,E). In contrast, vestibular afferents in Neurod1-cre:Fzd3f/f mutants, often project in a disorganized manner to the VN and as well as to the AVCN (Figures 4C,F). In these mice, some fibers are observed projecting first to the VN and from there to the AVCN. Additionally, many fibers appear to exit the vestibular nerve prematurely and project into areas typically innervated by SGNs. Altogether, these findings suggest that Fzd3 is acting in a cell autonomous manner within vestibular afferents as well to facilitate proper central pathfinding to the VN.
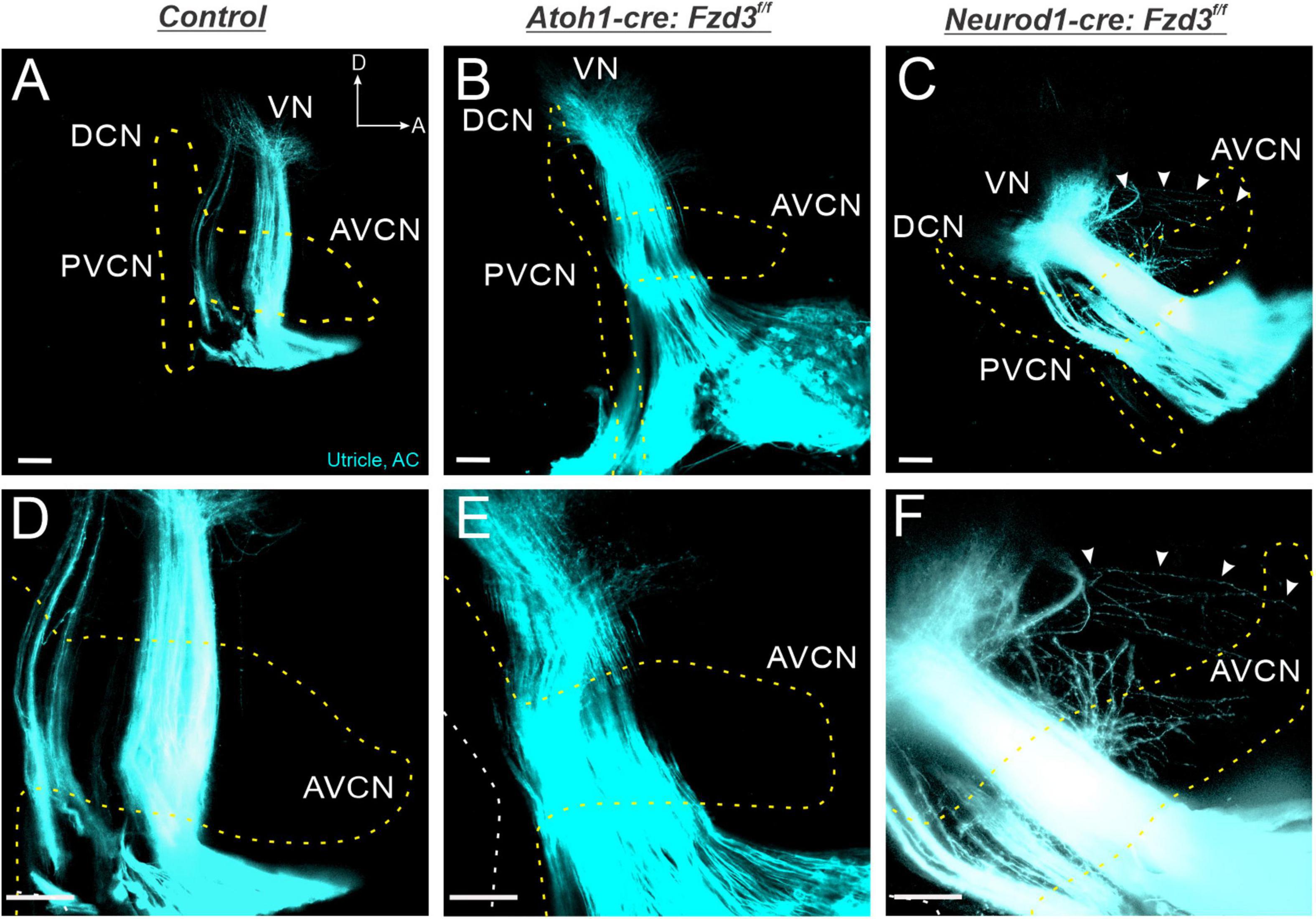
Figure 4. Vestibular afferents project out of the VN following the loss of Fzd3. Lipophilic dyes were applied to the apex (projection boundary outlined by dotted yellow line) and the utricle/anterior crista (cyan) of control (A,D), Atoh1-cre:Fzd3f/f (B,E), and Neurod1-cre:Fzd3f/f mutant (C,F) cochleae at P0 and their central projections were analyzed (A–F). In the control, vestibular afferents project toward the VN and remain segregated from cochlear afferents (A). In the Atoh1-cre:Fzd3f/f mutant, vestibular afferents also remain segregated from cochlear afferents and send projections toward the VN (B). In contrast, vestibular afferents in the Neurod1-cre:Fzd3f/f mutant project out of the VN into the AVCN resulting in a loss of segregation between cochlear and vestibular afferents (C). Higher magnification of the VN and AVCN (D–F) showing the projection of vestibular and apical afferents in the control (D), Atoh1-cre:Fzd3f/f (E) and Neurod1-cre:Fzd3f/f mutant (D). The white arrows show the projection of a single vestibular afferent out of the VN, into the AVCN of the Neurod1-cre:Fzd3f/f mutant (C,F). AC, anterior cristae; VN, vestibular nucleus; AVCN, anteroventral cochlear nucleus; DCN, dorsal cochlear nucleus; PVCN, posteroventral cochlear nucleus; A, anterior; D, dorsal. Orientation for all panels the same as (A). Scale bars, 100 μm. Three mutant animals and three littermate controls were analyzed.
Discussion
The results presented here expand upon our previous study implicating a possible conserved role of Fzd3 in inner ear afferent targeting of the correct dorsoventral column in the hindbrain (Duncan et al., 2019). Furthermore, using unique ear transplantation experiments in Xenopus, this previous study determined that Fzd3 was dispensable within the hindbrain but was required by cells originating from the ear for proper afferent guidance centrally. Consequently, our present study was designed to determine which cell type(s) within the inner ear required Fzd3 expression for appropriate targeting of inner ear afferent central projections. By utilizing conditional knockout mouse lines of Fzd3 in hair cells (Atoh1-cre, Figures 1, 4) and in inner ear afferents themselves (Neurod1-cre, Figures 3, 4), we demonstrate that Fzd3 acts in a cell autonomous manner within the inner ear afferents to influence proper central pathfinding of both SGNs and vestibular afferents (Figure 5). Conditionally knocking out Fzd3 from the hair cells (Figures 1, 4) has no apparent effect on the central pathfinding of either inner ear afferent populations. Conditional deletion of Fzd3 from the inner ear afferents leads to central pathfinding defects in both SGNs and vestibular afferents (Figures 3, 4), comparable to the central phenotype observed in Fzd3 null mutants (Duncan et al., 2019). Consistent with the null mice, Neurod1-cre:Fzd3f/f mice display aberrant SGN pathfinding, especially by apical SGNs, as well as complete mistargeting of some vestibular afferents to the cochlear nucleus. We cannot rule out a minor non-autonomous effect from another cell type not examined in the current study.
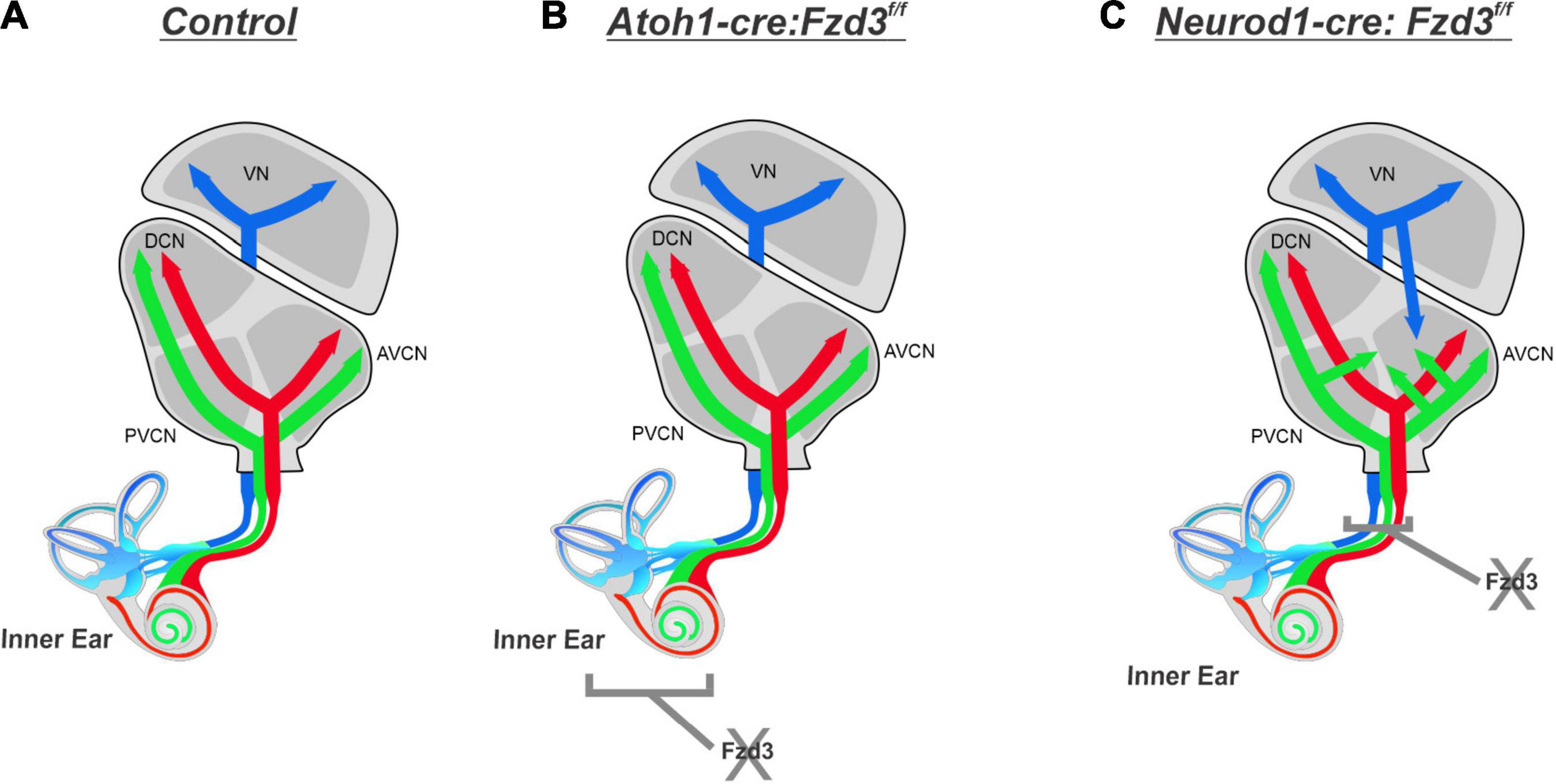
Figure 5. Proposed effect of Fzd3 in inner ear afferent central pathfinding. Cochlear afferents from the base (red) and apex (green) project into the CN and bifurcate sending projections toward the DCN and AVCN while maintaining the tonotopic map established in the periphery (A). Vestibular afferents (blue) project toward the VN and remain segregated from cochlear afferents (A). Inner ear afferent central pathfinding is unaffected by loss of Fzd3 within the hair cells in Atoh1-cre:Fzd3f/f mutants (B). Loss of Fzd3 within inner ear afferents in Neurod1-cre:Fzd3f/f mutants disrupts central pathfinding, resulting in aberrant projection of both apical SGNs and vestibular afferents (C). VN, vestibular nucleus; CN, cochlear nucleus; AVCN, anteroventral cochlear nucleus; DCN, dorsal cochlear nucleus; PVCN, posteroventral cochlear nucleus.
Despite multiple studies looking into cell-specific requirements for Fzd3 in axon guidance, variation exists between different cell types, with Fzd3 having a cell autonomous role in some instances of neuronal circuit assembly but a non-cell autonomous role in others. While our data supports that Fzd3 has a cell autonomous role in inner ear afferent central pathfinding, it is important to note that Fzd3 does not always have a cell autonomous role in axon guidance within the central nervous system. A study examining the role of Wnt/PCP pathways in development of facial branchiomotor neurons suggested that Fzd3 may have both cell autonomous and non-cell autonomous roles, but that they do not have to be considered separately (Vivancos et al., 2009). Their rationale, supported by the differing roles of Fzd3 determined in other studies (Wang et al., 2002; Qu et al., 2010, 2014; Chai et al., 2014), proposes that Fzd3 may function in the response of developing axons to chemoattraction cues (cell autonomous), but that Fzd3 may act in surrounding cell types to influence axon guidance. The inner ear afferent neurons have been shown to have defects in their peripheral projections due to loss of Fzd3 in a non-cell autonomous manner (Ghimire et al., 2018; Ghimire and Deans, 2019). Thus, the loss of Fzd3 has a non-autonomous effect on the peripheral projections and our study shows an autonomous effect on the central projection of inner ear afferents. The cell autonomous nature of Fzd3 in controlling the central pathfinding of inner ear afferent neurons is similar to that of 5-HT and mdDA neurons of the hindbrain (Fenstermaker et al., 2010), and commissural axons in the neural tube (Lyuksyutova et al., 2003; Onishi et al., 2013; Onishi and Zou, 2017). In both of these locations it is thought that Wnts are the ligand binding FZD3.
Some studies suggest that the role of Fzd3, at least in some instances, may be to establish pioneer neurons or to organize guidepost cells (Qin et al., 2020). Therefore, it may be the loss of these critical guides in absence of Fzd3 that leads to disruption of normal projection patterns for developing axons. It is plausible that without Fzd3, an interaction that would normally occur between basal and apical fibers is missing, or a new interaction has been introduced, that is leading to the misprojections observed when Fzd3 is conditionally deleted from inner ear afferent neurons. Conversely, there may be missing or newly present cues between SGN fibers and vestibular afferents, contributing to the presence of vestibular afferents in the CN. It is unclear if the defects seen by eliminating Fzd3 using Neurod1-Cre is due to the loss from all neurons, or if the effects seen are due to elimination of Fzd3 in subpopulations of neurons. This subpopulation effect of Fzd3 will need to be clarified in future studies.
The graded expression of Fzd3 across SGNs (Duncan et al., 2019) may explain why we observed greater central pathfinding defects in apical SGNs in the Fzd3 mutants. Since Fzd3 is expressed at higher levels in apical SGNs as compared to basal SGNs (Duncan et al., 2019), loss of Fzd3 may have a more severe impact on the ability of apical afferents to properly navigate within the CN. This suggests that gradients of Wnt/PCP signaling components may be restricting the projection of a given SGN to a specific region of the CN, possibly with additional repulsive/attractive ques in the extracellular environment, as has previously been shown for the visual system. In the visual system, a gradient of another Wnt/PCP signaling component, Ryk, expressed across retinal ganglion cells, mediates repulsion from the graded expression of Wnt3 ligand in the tectum. Fzd expression in the retinal ganglion cells mediates attraction that, together with Ryk repulsion, helps establish the dorsoventral retinal mapping to the tectum (Schmitt et al., 2006). Whether SGNs are directed to a particular dorsoventral region of the CN based on the FZD3 receptor concentration and the concentration of Wnt ligands released from the dorsal hindbrain remains to be explored. Furthermore, the extent that additional Wnt/PCP components, such as RYK or other FZD receptors, are involved in inner ear pathfinding is not yet known. Fzd3 has been shown to work together with Celsr3, another PCP protein, in development of both the peripheral and central nervous system (Wang et al., 2002; Zhou et al., 2008; Vivancos et al., 2009; Qu et al., 2010, 2014; Chai et al., 2014). Wnt-mediated axon guidance has been well-established in other major neuronal tracts (Lyuksyutova et al., 2003; Liu et al., 2005; Keeble and Cooper, 2006; Fenstermaker et al., 2010) beyond the ear and eye and the expression of Wnts in the dorsal hindbrain is conserved across vertebrate species (Hernandez-Miranda et al., 2017; Elliott and Fritzsch, 2018). However, the issue of which Wnt ligand(s) play a role in central pathfinding of SGNs is complicated by the existence of 19 different Wnts in mammals that can bind to 10 different FZD receptors (Wang et al., 2012), as well as ROR (Minami et al., 2010) and RYK (Yoshikawa et al., 2003) receptors. Some promising candidates are Wnt3a and Wnt5a, based on their expression in hindbrain regions that the inner ear afferents are targeting (Louvi et al., 2007; Di Bonito and Studer, 2017) and their ability to bind to FZD3 (Dijksterhuis et al., 2014). Wnt1 is also expressed in the same hindbrain region; however, it has not been shown to bind to FZD3 (Dijksterhuis et al., 2014). Identification of Wnt ligands/receptors involved in inner ear afferent central pathfinding will be critical in beginning to unravel the role Wnt/PCP signaling plays in this process.
Data Availability Statement
The raw data supporting the conclusions of this article will be made available by the authors, without undue reservation.
Ethics Statement
The animal study was reviewed and approved by the IACUC Western Michigan University.
Author Contributions
ZS and EK carried out lipophilic dye and tdTomato experiments, data collection, and wrote the initial manuscript. KE carried out the RNAscope experiments. SS-K and PB aided in tissue collection and performed experiments. All authors conceived and designed the experiments, and edited the final manuscript.
Funding
This work was supported by grants from NIH (R21 DC017589 to JD and R03 DC015333 to KE) and funds from WMU Office of the Dean for the College of Arts and Sciences to JD.
Conflict of Interest
The authors declare that the research was conducted in the absence of any commercial or financial relationships that could be construed as a potential conflict of interest.
Publisher’s Note
All claims expressed in this article are solely those of the authors and do not necessarily represent those of their affiliated organizations, or those of the publisher, the editors and the reviewers. Any product that may be evaluated in this article, or claim that may be made by its manufacturer, is not guaranteed or endorsed by the publisher.
Acknowledgments
We would like to thank Andrew Leiter for mice. The use of the Leica TCS SP8 confocal microscope was made possible by a grant from the Roy. J. Carver Charitable Trust. We also thank the Office of the Vice President for Research and Innovation (OVPRI) of Western Michigan University and the Office of the Vice President for Research (OVPR) of the University of Iowa for support.
Supplementary Material
The Supplementary Material for this article can be found online at: https://www.frontiersin.org/articles/10.3389/fnins.2021.779871/full#supplementary-material
References
Appler, J. M., and Goodrich, L. V. (2011). Connecting the ear to the brain: molecular mechanisms of auditory circuit assembly. Prog. Neurobiol. 93, 488–508. doi: 10.1016/j.pneurobio.2011.01.004
Bermingham, N. A., Hassan, B. A., Price, S. D., Vollrath, M. A., Ben-Arie, N., Eatock, R. A., et al. (1999). Math1: an essential gene for the generation of inner ear hair cells. Science 284, 1837–1841. doi: 10.1126/science.284.5421.1837
Bourk, T. R., Mielcarz, J. P., and Norris, B. E. (1981). Tonotopic organization of the anteroventral cochlear nucleus of the cat. Hear. Res. 4, 215–241. doi: 10.1016/0378-5955(81)90008-3
Cang, J., and Feldheim, D. A. (2013). Developmental mechanisms of topographic map formation and alignment. Annu. Rev. Neurosci. 36, 51–77. doi: 10.1146/annurev-neuro-062012-170341
Chai, G., Goffinet, A. M., and Tissir, F. (2015). Celsr3 and Fzd3 in axon guidance. Int. J. Biochem. Cell Biol. 64, 11–14. doi: 10.1016/j.biocel.2015.03.013
Chai, G., Zhou, L., Manto, M., Helmbacher, F., Clotman, F., Goffinet, A. M., et al. (2014). Celsr3 is required in motor neurons to steer their axons in the hindlimb. Nat. Neurosci. 17, 1171–1179. doi: 10.1038/nn.3784
Chilton, J. K. (2006). Molecular mechanisms of axon guidance. Dev. Biol. 292, 13–24. doi: 10.1016/j.ydbio.2005.12.048
Coate, T. M., and Kelley, M. W. (2013). Making connections in the inner ear: recent insights into the development of spiral ganglion neurons and their connectivity with sensory hair cells. Semin. Cell Dev. Biol. 24, 460–469. doi: 10.1016/j.semcdb.2013.04.003
Coate, T. M., Spita, N. A., Zhang, K. D., Isgrig, K. T., and Kelley, M. W. (2015). Neuropilin-2/Semaphorin-3F-mediated repulsion promotes inner hair cell innervation by spiral ganglion neurons. Elife 4:e07830. doi: 10.7554/eLife.07830
Di Bonito, M., and Studer, M. (2017). Cellular and Molecular Underpinnings of Neuronal Assembly in the Central Auditory System during Mouse Development. Front. Neural Circuits 11:18. doi: 10.3389/fncir.2017.00018
Dickman, J. D., and Fang, Q. (1996). Differential central projections of vestibular afferents in pigeons. J. Comp. Neurol. 367, 110–131. doi: 10.1002/(SICI)1096-9861(19960325)367:1<110::AID-CNE8>3.0.CO;2-6
Dijksterhuis, J., Petersen, J., and Schulte, G. (2014). WNT/F rizzled signalling: receptor–ligand selectivity with focus on FZD-G protein signalling and its physiological relevance: IUPHAR Review 3. Br. J. Pharmacol. 171, 1195–1209. doi: 10.1111/bph.12364
Duncan, J., Kersigo, J., Gray, B., and Fritzsch, B. (2011). Combining Lipophilic dye, in situ Hybridization, Immunohistochemistry, and Histology. J. Vis. Exp. 49:e2451. doi: 10.3791/2451
Duncan, J. S., and Fritzsch, B. (2012). Evolution of sound and balance perception: innovations that aggregate single hair cells into the ear and transform a gravistatic sensor into the organ of corti. Anat. Rec. 295, 1760–1774.
Duncan, J. S., Fritzsch, B., Houston, D. W., Ketchum, E. M., Kersigo, J., Deans, M. R., et al. (2019). Topologically correct central projections of tetrapod inner ear afferents require Fzd3. Sci. Rep. 9:10298. doi: 10.1038/s41598-019-46553-6
Elliott, K. L., and Fritzsch, B. (2018). Ear transplantations reveal conservation of inner ear afferent pathfinding cues. Sci. Rep. 8:13819. doi: 10.1038/s41598-018-31952-y
Elliott, K. L., Houston, D. W., Decook, R., and Fritzsch, B. (2015). Ear manipulations reveal a critical period for survival and dendritic development at the single-cell level in Mauthner neurons. Dev. Neurobiol. 75, 1339–1351. doi: 10.1002/dneu.22287
Elliott, K. L., Houston, D. W., and Fritzsch, B. (2013). Transplantation of Xenopus laevis tissues to determine the ability of motor neurons to acquire a novel target. PLoS One 8:e55541. doi: 10.1371/journal.pone.0055541
Elliott, K. L., Kersigo, J., Pan, N., Jahan, I., and Fritzsch, B. (2017). Spiral Ganglion Neuron Projection Development to the Hindbrain in Mice Lacking Peripheral and/or Central Target Differentiation. Front. Neural Circuits 11:25. doi: 10.3389/fncir.2017.00025
Fariñas, I., Jones, K. R., Tessarollo, L., Vigers, A. J., Huang, E., Kirstein, M., et al. (2001). Spatial shaping of cochlear innervation by temporally regulated neurotrophin expression. J. Neurosci. 21, 6170–6180. doi: 10.1523/JNEUROSCI.21-16-06170.2001
Fekete, D. M., and Campero, A. M. (2007). Axon guidance in the inner ear. Int. J. Dev. Biol. 51, 549–556. doi: 10.1387/ijdb.072341df
Fenstermaker, A. G., Prasad, A. A., Bechara, A., Adolfs, Y., Tissir, F., Goffinet, A., et al. (2010). Wnt/planar cell polarity signaling controls the anterior-posterior organization of monoaminergic axons in the brainstem. J. Neurosci. 30, 16053–16064. doi: 10.1523/JNEUROSCI.4508-10.2010
Fritzsch, B. (1981). The pattern of lateral-line afferents in urodeles. A horseradish- peroxidase study. Cell Tissue Res. 218, 581–594. doi: 10.1007/BF00210117
Fritzsch, B., Elliott, K. L., and Pavlinkova, G. (2019). Primary sensory map formations reflect unique needs and molecular cues specific to each sensory system. F1000Res. 8:345. doi: 10.12688/f1000research.17717.1
Fujiyama, T., Yamada, M., Terao, M., Terashima, T., Hioki, H., Inoue, Y. U., et al. (2009). Inhibitory and excitatory subtypes of cochlear nucleus neurons are defined by distinct bHLH transcription factors, Ptf1a and Atoh1. Development 136, 2049–2058. doi: 10.1242/dev.033480
Ghimire, S. R., and Deans, M. R. (2019). Frizzled3 and Frizzled6 Cooperate with Vangl2 to Direct Cochlear Innervation by Type II Spiral Ganglion Neurons. J. Neurosci. 39, 8013–8023. doi: 10.1523/JNEUROSCI.1740-19.2019
Ghimire, S. R., Ratzan, E. M., and Deans, M. R. (2018). A non-autonomous function of the core PCP protein VANGL2 directs peripheral axon turning in the developing cochlea. Development 145:dev159012. doi: 10.1242/dev.159012
Goodrich, L. V. (2008). The plane facts of PCP in the CNS. Neuron 60, 9–16. doi: 10.1016/j.neuron.2008.09.003
Gordy, C., Straka, H., Houston, D. W., Fritzsch, B., and Elliott, K. L. (2018). Transplantation of Ears Provides Insights into Inner Ear Afferent Pathfinding Properties. Dev. Neurobiol. 78, 1064–1080. doi: 10.1002/dneu.22629
Green, S. H., Bailey, E., Wang, Q., and Davis, R. L. (2012). The Trk A, B, C’s of neurotrophins in the cochlea. Anat. Rec. 295, 1877–1895. doi: 10.1002/ar.22587
Hernandez-Miranda, L. R., Muller, T., and Birchmeier, C. (2017). The dorsal spinal cord and hindbrain: from developmental mechanisms to functional circuits. Dev. Biol. 432, 34–42. doi: 10.1016/j.ydbio.2016.10.008
Hua, Z. L., Smallwood, P. M., and Nathans, J. (2013). Frizzled3 controls axonal development in distinct populations of cranial and spinal motor neurons. Elife 2:e01482. doi: 10.7554/eLife.01482
Kannan-Sundhari, A., Abad, C., Maloof, M. E., Ayad, N. G., Young, J. I., Liu, X. Z., et al. (2020). Bromodomain Protein BRD4 Is Essential for Hair Cell Function and Survival. Front. Cell Dev. Biol. 8:576654. doi: 10.3389/fcell.2020.576654
Keeble, T. R., and Cooper, H. M. (2006). Ryk: a novel Wnt receptor regulating axon pathfinding. Int. J. Biochem. Cell Biol. 38, 2011–2017. doi: 10.1016/j.biocel.2006.07.005
Kersigo, J., Pan, N., Lederman, J. D., Chatterjee, S., Abel, T., Pavlinkova, G., et al. (2018). A RNAscope whole mount approach that can be combined with immunofluorescence to quantify differential distribution of mRNA. Cell Tissue Res. 374, 251–262. doi: 10.1007/s00441-018-2864-4
Kim, W. Y., Fritzsch, B., Serls, A., Bakel, L. A., Huang, E. J., Reichardt, L. F., et al. (2001). NeuroD-null mice are deaf due to a severe loss of the inner ear sensory neurons during development. Development 128, 417–426. doi: 10.1242/dev.128.3.417
Kolodkin, A. L., and Tessier-Lavigne, M. (2011). Mechanisms and molecules of neuronal wiring: a primer. Cold Spring Harb. Perspect. Biol. 3:a001727. doi: 10.1101/cshperspect.a001727
Li, H. J., Kapoor, A., Giel-Moloney, M., Rindi, G., and Leiter, A. B. (2012). Notch signaling differentially regulates the cell fate of early endocrine precursor cells and their maturing descendants in the mouse pancreas and intestine. Dev. Biol. 371, 156–169. doi: 10.1016/j.ydbio.2012.08.023
Liu, M., Pereira, F. A., Price, S. D., Chu, M. J., Shope, C., Himes, D., et al. (2000). Essential role of BETA2/NeuroD1 in development of the vestibular and auditory systems. Genes Dev. 14, 2839–2854. doi: 10.1101/gad.840500
Liu, Y., Shi, J., Lu, C. C., Wang, Z. B., Lyuksyutova, A. I., Song, X. J., et al. (2005). Ryk-mediated Wnt repulsion regulates posterior-directed growth of corticospinal tract. Nat. Neurosci. 8, 1151–1159. doi: 10.1038/nn1520
Louvi, A., Yoshida, M., and Grove, E. A. (2007). The derivatives of the Wnt3a lineage in the central nervous system. J. Comp. Neurol. 504, 550–569. doi: 10.1002/cne.21461
Lu, C. C., Appler, J. M., Houseman, E. A., and Goodrich, L. V. (2011). Developmental profiling of spiral ganglion neurons reveals insights into auditory circuit assembly. J. Neurosci. 31, 10903–10918. doi: 10.1523/JNEUROSCI.2358-11.2011
Luo, F., Wang, Q., Farid, N., Liu, X., and Yan, J. (2009). Three-dimensional tonotopic organization of the C57 mouse cochlear nucleus. Hear. Res. 257, 75–82. doi: 10.1016/j.heares.2009.08.002
Lyuksyutova, A. I., Lu, C.-C., Milanesio, N., King, L. A., Guo, N., Wang, Y., et al. (2003). Anterior-Posterior Guidance of Commissural Axons by Wnt-Frizzled Signaling. Science 302, 1984–1988. doi: 10.1126/science.1089610
Macova, I., Pysanenko, K., Chumak, T., Dvorakova, M., Bohuslavova, R., Syka, J., et al. (2019). Neurod1 Is Essential for the Primary Tonotopic Organization and Related Auditory Information Processing in the Midbrain. J. Neurosci. 39, 984–1004. doi: 10.1523/JNEUROSCI.2557-18.2018
Maklad, A., and Fritzsch, B. (2003). Development of vestibular afferent projections into the hindbrain and their central targets. Brain Res. Bull. 60, 497–510. doi: 10.1016/s0361-9230(03)00054-6
Maler, L., Finger, T., and Karten, H. J. (1974). Differential projections of ordinary lateral line receptors and electroreceptors in the gymnotid fish, Apteronotus (Sternarchus) albifrons. J. Comp. Neurol. 158, 363–382. doi: 10.1002/cne.901580402
Maricich, S. M., Xia, A., Mathes, E. L., Wang, V. Y., Oghalai, J. S., Fritzsch, B., et al. (2009). Atoh1-lineal neurons are required for hearing and for the survival of neurons in the spiral ganglion and brainstem accessory auditory nuclei. J. Neurosci. 29, 11123–11133. doi: 10.1523/JNEUROSCI.2232-09.2009
Matei, V., Pauley, S., Kaing, S., Rowitch, D., Beisel, K. W., Morris, K., et al. (2005). Smaller inner ear sensory epithelia in Neurog 1 null mice are related to earlier hair cell cycle exit. Dev. Dyn. 234, 633–650. doi: 10.1002/dvdy.20551
Meyer, A. C., Frank, T., Khimich, D., Hoch, G., Riedel, D., Chapochnikov, N. M., et al. (2009). Tuning of synapse number, structure and function in the cochlea. Nat. Neurosci. 12, 444–453. doi: 10.1038/nn.2293
Minami, Y., Oishi, I., Endo, M., and Nishita, M. (2010). Ror-family receptor tyrosine kinases in noncanonical Wnt signaling: their implications in developmental morphogenesis and human diseases. Dev. Dyn. 239, 1–15. doi: 10.1002/dvdy.21991
Onishi, K., Shafer, B., Lo, C., Tissir, F., Goffinet, A. M., and Zou, Y. (2013). Antagonistic Functions of Dishevelleds Regulate Frizzled3 Endocytosis via Filopodia Tips in Wnt-Mediated Growth Cone Guidance. J. Neurosci. 33, 19071–19085. doi: 10.1523/JNEUROSCI.2800-13.2013
Onishi, K., and Zou, Y. (2017). Sonic Hedgehog switches on Wnt/planar cell polarity signaling in commissural axon growth cones by reducing levels of Shisa2. eLife 6:e25269. doi: 10.7554/eLife.25269
Perrin, B. J., Sonnemann, K. J., and Ervasti, J. M. (2010). β-Actin and γ-Actin Are Each Dispensable for Auditory Hair Cell Development But Required for Stereocilia Maintenance. PLoS Genet. 6:e1001158. doi: 10.1371/journal.pgen.1001158
Perrin, B. J., Strandjord, D. M., Narayanan, P., Henderson, D. M., Johnson, K. R., and Ervasti, J. M. (2013). β-Actin and Fascin-2 Cooperate to Maintain Stereocilia Length. J. Neurosci. 33, 8114–8121. doi: 10.1523/JNEUROSCI.0238-13.2013
Qin, J., Wang, M., Zhao, T., Xiao, X., Li, X., Yang, J., et al. (2020). Early forebrain neurons and scaffold fibers in human embryos. Cereb. Cortex 30, 913–928. doi: 10.1093/cercor/bhz136
Qu, Y., Glasco, D. M., Zhou, L., Sawant, A., Ravni, A., Fritzsch, B., et al. (2010). Atypical cadherins Celsr1-3 differentially regulate migration of facial branchiomotor neurons in mice. J. Neurosci. 30, 9392–9401. doi: 10.1523/JNEUROSCI.0124-10.2010
Qu, Y., Huang, Y., Feng, J., Alvarez-Bolado, G., Grove, E. A., Yang, Y., et al. (2014). Genetic evidence that Celsr3 and Celsr2, together with Fzd3, regulate forebrain wiring in a Vangl-independent manner. Proc. Natl. Acad. Sci. U. S. A. 111, E2996–E3004. doi: 10.1073/pnas.1402105111
Ruben, R. J. (1967). Development of the inner ear of the mouse : a radioautographic study of terminal mitoses. Acta Otolaryngol. 220, 1–44.
Russell, S. A., and Bashaw, G. J. (2017). Axon Guidance Pathways and the Control of Gene Expression. Dev. Dyn. 247, 571–580. doi: 10.1002/dvdy.24609
Ryugo, D. K., and Parks, T. N. (2003). Primary innervation of the avian and mammalian cochlear nucleus. Brain Res. Bull. 60, 435–456. doi: 10.1016/s0361-9230(03)00049-2
Schmitt, A. M., Shi, J., Wolf, A. M., Lu, C.-C., King, L. A., and Zou, Y. (2006). Wnt–Ryk signalling mediates medial–lateral retinotectal topographic mapping. Nature 439, 31–37. doi: 10.1038/nature04334
Stuebner, S., Faus-Kessler, T., Fischer, T., Wurst, W., and Prakash, N. (2010). Fzd3 and Fzd6 deficiency results in a severe midbrain morphogenesis defect. Dev. Dyn. 239, 246–260. doi: 10.1002/dvdy.22127
Tarchini, B., Tadenev, A. L. D., Devanney, N., and Cayouette, M. (2016). A link between planar polarity and staircase-like bundle architecture in hair cells. Development 143, 3926–3932. doi: 10.1242/dev.139089
Tonniges, J., Hansen, M., Duncan, J., Bassett, M. J., Fritzsch, B., Gray, B. D., et al. (2010). Photo- and bio-physical characterization of novel violet and near-infrared lipophilic fluorophores for neuronal tracing. J. Microsc. 239, 117–134. doi: 10.1111/j.1365-2818.2009.03363.x
Ueyama, T., Sakaguchi, H., Nakamura, T., Goto, A., Morioka, S., Shimizu, A., et al. (2014). Maintenance of stereocilia and apical junctional complexes by Cdc42 in cochlear hair cells. J. Cell Sci. 127, 2040–2052. doi: 10.1242/jcs.143602
Vivancos, V., Chen, P., Spassky, N., Qian, D., Dabdoub, A., Kelley, M., et al. (2009). Wnt activity guides facial branchiomotor neuron migration, and involves the PCP pathway and JNK and ROCK kinases. Neural Dev. 4:7. doi: 10.1186/1749-8104-4-7
Wang, J., Sinha, T., and Wynshaw-Boris, A. (2012). Wnt signaling in mammalian development: lessons from mouse genetics. Cold Spring Harb. Perspect. Biol. 4:a007963. doi: 10.1101/cshperspect.a007963
Wang, V. Y., Rose, M. F., and Zoghbi, H. Y. (2005). Math1 expression redefines the rhombic lip derivatives and reveals novel lineages within the brainstem and cerebellum. Neuron 48, 31–43. doi: 10.1016/j.neuron.2005.08.024
Wang, Y., Guo, N., and Nathans, J. (2006). The role of Frizzled3 and Frizzled6 in neural tube closure and in the planar polarity of inner-ear sensory hair cells. J. Neurosci. 26, 2147–2156. doi: 10.1523/JNEUROSCI.4698-05.2005
Wang, Y., Thekdi, N., Smallwood, P. M., Macke, J. P., and Nathans, J. (2002). Frizzled-3 is required for the development of major fiber tracts in the rostral CNS. J. Neurosci. 22, 8563–8573. doi: 10.1523/JNEUROSCI.22-19-08563.2002
Warr, W. B., and Guinan, J. J. Jr. (1979). Efferent innervation of the organ of Corti: two separate systems. Brain Res. 173, 152–155. doi: 10.1016/0006-8993(79)91104-1
Wickesberg, R. E., and Oertel, D. (1988). Tonotopic projection from the dorsal to the anteroventral cochlear nucleus of mice. J. Comp. Neurol. 268, 389–399. doi: 10.1002/cne.902680308
Yang, H., Xie, X., Deng, M., Chen, X., and Gan, L. (2010). Generation and characterization of Atoh1-Cre knock-in mouse line. Genesis 48, 407–413. doi: 10.1002/dvg.20633
Yang, T., Kersigo, J., Wu, S., Fritzsch, B., and Bassuk, A. G. (2017). Prickle1 regulates neurite outgrowth of apical spiral ganglion neurons but not hair cell polarity in the murine cochlea. PLoS One 12:e0183773. doi: 10.1371/journal.pone.0183773
Yoshikawa, S., Mckinnon, R. D., Kokel, M., and Thomas, J. B. (2003). Wnt-mediated axon guidance via the Drosophila Derailed receptor. Nature 422, 583–588. doi: 10.1038/nature01522
Keywords: inner ear afferent, spiral ganglion neurons (SGN), Frizzled3, neuronal projections, Wnt/PCP
Citation: Stoner ZA, Ketchum EM, Sheltz-Kempf S, Blinkiewicz PV, Elliott KL and Duncan JS (2022) Fzd3 Expression Within Inner Ear Afferent Neurons Is Necessary for Central Pathfinding. Front. Neurosci. 15:779871. doi: 10.3389/fnins.2021.779871
Received: 20 September 2021; Accepted: 29 December 2021;
Published: 27 January 2022.
Edited by:
Gwenaelle S. G. Geleoc, Boston Children’s Hospital and Harvard Medical School, United StatesReviewed by:
Fabienne E. Poulain, University of South Carolina, United StatesAndre Goffinet, Catholic University of Louvain, Belgium
Copyright © 2022 Stoner, Ketchum, Sheltz-Kempf, Blinkiewicz, Elliott and Duncan. This is an open-access article distributed under the terms of the Creative Commons Attribution License (CC BY). The use, distribution or reproduction in other forums is permitted, provided the original author(s) and the copyright owner(s) are credited and that the original publication in this journal is cited, in accordance with accepted academic practice. No use, distribution or reproduction is permitted which does not comply with these terms.
*Correspondence: Karen L. Elliott, a2FyZW4tZWxsaW90dEB1aW93YS5lZHU=; Jeremy S. Duncan, amVyZW15LmR1bmNhbkB3bWljaC5lZHU=
†These authors have contributed equally to this work and share first authorship