- 1Program in Developmental & Stem Cell Biology, The Hospital for Sick Children, Toronto, ON, Canada
- 2Department of Molecular Genetics, University of Toronto, Toronto, ON, Canada
The hypothalamus is a brain region that exhibits highly conserved anatomy across vertebrate species and functions as a central regulatory hub for many physiological processes such as energy homeostasis and circadian rhythm. Neurons in the arcuate nucleus of the hypothalamus are largely responsible for sensing of peripheral signals such as leptin and insulin, and are critical for the regulation of food intake and energy expenditure. While these neurons are mainly born during embryogenesis, accumulating evidence have demonstrated that neurogenesis also occurs in postnatal-adult mouse hypothalamus, particularly in the first two postnatal weeks. This second wave of active neurogenesis contributes to the remodeling of hypothalamic neuronal populations and regulation of energy homeostasis including hypothalamic leptin sensing. Radial glia cell types, such as tanycytes, are known to act as neuronal progenitors in the postnatal mouse hypothalamus. Our recent study unveiled a previously unreported radial glia-like neural stem cell (RGL-NSC) population that actively contributes to neurogenesis in the postnatal mouse hypothalamus. We also identified Irx3 and Irx5, which encode Iroquois homeodomain-containing transcription factors, as genetic determinants regulating the neurogenic property of these RGL-NSCs. These findings are significant as IRX3 and IRX5 have been implicated in FTO-associated obesity in humans, illustrating the importance of postnatal hypothalamic neurogenesis in energy homeostasis and obesity. In this review, we summarize current knowledge regarding postnatal-adult hypothalamic neurogenesis and highlight recent findings on the radial glia-like cells that contribute to the remodeling of postnatal mouse hypothalamus. We will discuss characteristics of the RGL-NSCs and potential actions of Irx3 and Irx5 in the regulation of neural stem cells in the postnatal-adult mouse brain. Understanding the behavior and regulation of neural stem cells in the postnatal-adult hypothalamus will provide novel mechanistic insights in the control of hypothalamic remodeling and energy homeostasis.
Introduction
Obesity is a global health threat with increased risk for various chronic conditions including diabetes and cardiovascular diseases (Williams et al., 2015; Gonzalez-Muniesa et al., 2017). Although lifestyle changes have driven its prevalence to epidemic proportions, genetic factors play an important role in influencing which individuals within a population are more likely to develop obesity in response to a particular environment (Elks et al., 2012; van der Klaauw and Farooqi, 2015; Gonzalez-Muniesa et al., 2017). In the last few decades, tremendous efforts have been made to identify genetic factors involved in obesity. Among genetic variations in the human genome, single-nucleotide polymorphisms (SNPs) in the first intron of the Fat mass and obesity-associated gene (FTO) were identified to be most highly associated with obesity risk (Dina et al., 2007; Frayling et al., 2007; Cecil et al., 2008; Speakman et al., 2008; Tanofsky-Kraff et al., 2009). Recent studies further demonstrated that two homeobox genes, IRX3 and IRX5, in the vicinity of FTO directly mediate the effects of obesity-risk variants of FTO on body mass and composition regulation (Smemo et al., 2014; Claussnitzer et al., 2015; Laber et al., 2021; Sobreira et al., 2021).
The hypothalamus is a central regulatory hub for many physiological processes including energy homeostasis. Especially, the hypothalamic arcuate-median eminence (ARC-ME) is involved in the sensing of various signals such as the satiety hormone leptin (Dietrich and Horvath, 2013; Timper and Bruning, 2017; Friedman, 2019; Myers et al., 2021). It contains major classes of leptin-sensing neurons including those expressing orexigenic agouti-related peptide (AgRP) and anorexigenic pro-opiomelanocortin (POMC), radial glia-like cells (RGLs) (tanycytes, ependymocytes and neural stem cells) as well as NG2+ oligodendrocyte precursor cells (OPCs) (Dietrich and Horvath, 2013; Campbell et al., 2017; Rizzoti and Lovell-Badge, 2017; Timper and Bruning, 2017). Irx3 and Irx5 are expressed in multiple cell types of the ARC-ME, predominantly in a newly identified radial glia-like neural stem cell (RGL-NSC) population in the postnatal mouse hypothalamus (Smemo et al., 2014; Campbell et al., 2017; Son et al., 2021a). They are implicated in the regulation of energy homeostasis, particularly feeding regulation, and postnatal hypothalamic neurogenesis (Son et al., 2021a). The role of IRX3 and IRX5 in the regulation of energy homeostasis and development has been previously discussed in other reviews (Cavodeassi et al., 2001; Gomez-Skarmeta and Modolell, 2002; Kim et al., 2012; Tung et al., 2014; Herman and Rosen, 2015; de Araujo and Velloso, 2020). In this review, we aim to provide an update integrating our knowledge of their new functions in hypothalamic neurogenesis and discuss the intricacies and challenges in understanding their molecular actions.
IRX3 and IRX5 Homeobox Genes are Effectors of FTO Obesity-Risk Variants
Iroquois homeobox (Irx) genes encode a family of highly conserved TALE homeodomain-containing transcription factors (TFs). Iroquois genes were first discovered in the fruit fly Drosophila melanogaster. Mutations of these genes suppress bristle formation on the lateral notum, leaving only a wide band of bristles in the central part of the notum, reminiscent of the hairstyle of the Iroquois American Indians “Mohawk” - hence the name of the locus (Gomez-Skarmeta and Modolell, 1996; Gomez-Skarmeta et al., 1996; Leyns et al., 1996). Mammals have 6 Irx genes clustered in two 3-gene groups; the IrxA cluster on mouse chromosome 13 (human chromosome 5) consists of Irx1, Irx2, and Irx4, and the IrxB cluster on mouse chromosome 8 (human chromosome 16) contains Irx3, Irx5, and Irx6 (Peters et al., 2000; Cavodeassi et al., 2001; Kim et al., 2012). Among them, Irx3 and Irx5 of the IrxB cluster are involved in the development of many mammalian tissues including the heart, bone, limb bud, eye and ovary, with regulatory functions in embryonic patterning and specification as well as in tissue differentiation and specialization (Cheng et al., 2005; Costantini et al., 2005; Zhang et al., 2011; Gaborit et al., 2012; Kim et al., 2012; Li et al., 2014; Kim et al., 2016; Fu et al., 2018; Tan et al., 2020; Tao et al., 2020). Irx3 and Irx5 share similar expression patterns during embryonic development and their double mutant mice show more severe phenotypes than single mutants, suggesting that they possess overlapping function and act redundantly.
Besides their developmental functions, IRX3 and IRX5 have been highlighted as determinants of human obesity in connection with the intronic obesity risk variants of FTO, a gene in the vicinity of the IRXB cluster. Non-coding variations represented by SNPs in the first intron of FTO are the strongest genetic risk factors of polygenic obesity in humans (Dina et al., 2007; Frayling et al., 2007; Cecil et al., 2008; Speakman et al., 2008; Tanofsky-Kraff et al., 2009). Previous studies using chromatin conformation capture techniques (3C, 4C and Hi-C) have demonstrated that a genomic region including the intronic FTO SNPs directly loops into the promoter region of the neighboring IRX3 and IRX5, ∼ a half megabase downstream of FTO, in multiple tissues including adipose tissue and brain in humans (Figure 1A; Smemo et al., 2014; Claussnitzer et al., 2015; Sobreira et al., 2021). Furthermore, expression quantitative trait loci (eQTL) analysis revealed significantly higher expression levels of IRX3 and IRX5 in human brain, human hypothalamic arcuate-like neurons derived from human induced pluripotent stem cells (hiPSCs) and human adipocyte progenitor cells from risk-allele carriers, compared to non-risk allele carriers, whereas risk and non-risk carriers did not show significant difference of FTO expression (Figure 1A). Gene editing experiments in human adipocytes showed that an obesity-risk intronic variant of FTO results in higher expression of both IRX3 and IRX5 specifically in adipocyte progenitor cells (Claussnitzer et al., 2015), and deletion of a cis-regulatory module (CRM) harboring the FTO risk variant in mice results in lower Irx3 and Irx5 gene expression in adipocyte progenitors to roughly 70% of control levels (Laber et al., 2021). Furthermore, ∼20k-bp deletion spanning the orthologous obesity-associated interval of Fto in mice leads to down-regulation of both Irx3 and Irx5 in preadipocytes and developing hypothalamus (Sobreira et al., 2021). Collectively, these studies suggest that obesity-associated variants of FTO regulate the expression of IRX3 and IRX5 in the hypothalamus and adipose tissue contributing to metabolic changes.
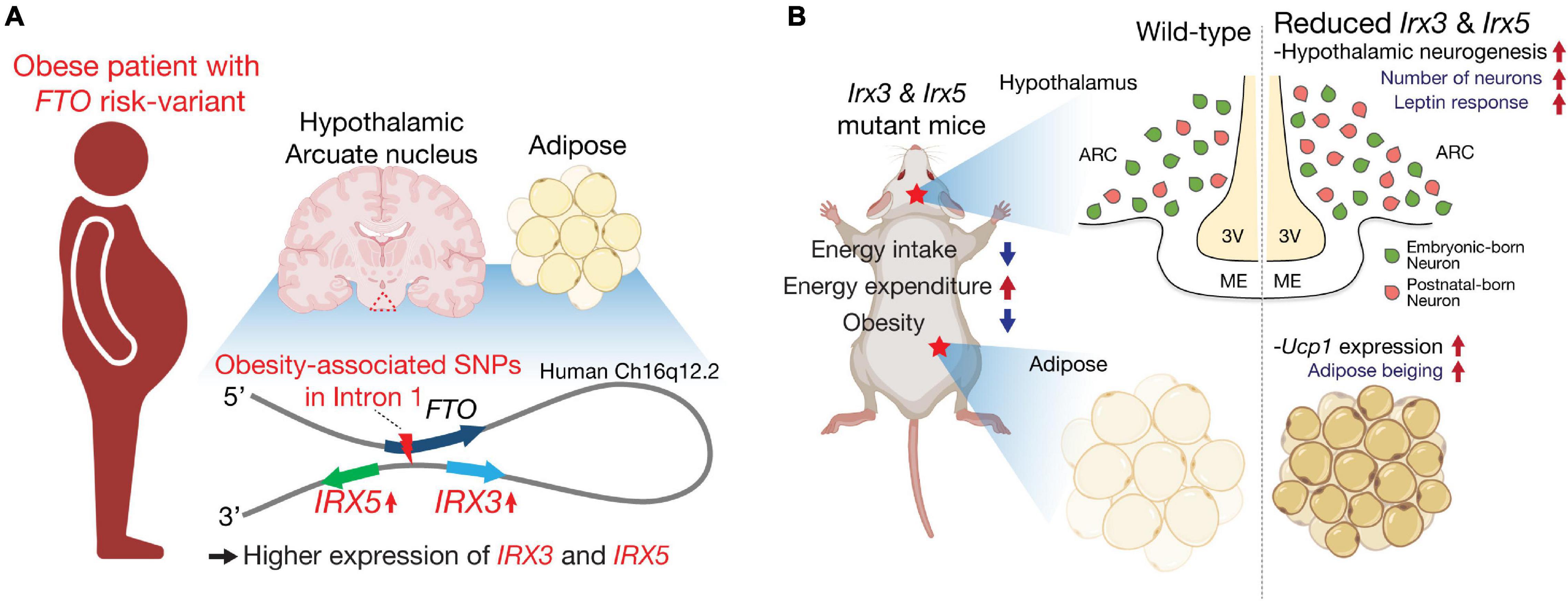
Figure 1. IRX3 and IRX5 are effectors of FTO obesity-risk variants and key determinants of energy homeostasis. (A) IRX3 and IRX5 are located about a half-megabase downstream of FTO. In human brain, particularly hypothalamic arcuate nucleus (triangle with dashed lines in red) and preadipocytes, they mediate the effects of obesity-associated variants located in the first intron of FTO through long-range interactions. (B) Mice with mutations in Irx3 and/or Irx5 exhibit an anti-obesity phenotype with increased energy expenditure and reduced food intake. These metabolic phenotypes are related to beiging of white adipose tissue and improved response to leptin as well as increased postnatal neurogenesis in the mediobasal hypothalamus. Schematics were created using illustrations from https://biorender.com.
The Importance of IRX3 and IRX5 in Metabolic Regulation
Studies of genetic mouse models have demonstrated that Irx3 and Irx5 are both involved in metabolic regulation. Although there are differences in the metabolic phenotypes depending on the models, Irx3- and Irx5-deficient mice are generally lean and display an anti-obesity phenotype with elevated energy expenditure due to adipose beiging (i.e., enhanced adipose thermogenesis) compared to wild-type controls (summarized in Table 1). Specifically, Irx3 knockout (Irx3tLz/tLz) and Irx5 knockout (Irx5eGFP/eGFP) mice generated by our group both exhibited lower body weight and fat composition (Smemo et al., 2014; Bjune et al., 2018), whereas their heterozygous counterparts Irx3tLZ/+ and Irx5eGFP/+ mice did not exhibit any changes in body weight and composition (Son et al., 2021a). In a recent report, another line of Irx3 knockout mice (Irx3Δ/Δ) generated by CRISPR editing was also shown to be lean and protected against diet-induced obesity (Sobreira et al., 2021). However, in the same study, mice homozygous for a new deletion mutation of Irx5 (Irx5Δ/Δ) exhibited early postnatal lethality, whereas heterozygous Irx5Δ/+ mutant mice were found to show an anti-obesity phenotype like Irx3 knockout mice. While this discrepancy of Irx5 mutant phenotypes remains to be investigated, genetic background and/or deletion of specific genomic sequences affecting the expression of neighboring genes could be contributing factors.
Due to the requirement of Irx3 and Irx5 in the development of many organs, including heart (Costantini et al., 2005; Zhang et al., 2011; Gaborit et al., 2012; Kim et al., 2016) and bone (Cain et al., 2016; Bai et al., 2019; Tan et al., 2020) which are also important for energy homeostasis, whole body knockout mice of Irx3 and Irx5 are both runty and thus not ideal for studying metabolic regulation. To circumvent this, a mouse line harboring cis-heterozygous mutant allele of Irx3 and Irx5 (Irx3+Irx5+/Irx3ΔIrx5eGFP; Irx3/5dHet) was employed to study the effects of half-reduction of the expression of Irx3 and Irx5 on energy homeostasis (Son et al., 2021a). As obesity-risk variants of FTO affect the expression levels of both IRX3 and IRX5 (Claussnitzer et al., 2015; Laber et al., 2021; Sobreira et al., 2021), Irx3/5dHet mice serve as a preclinical model mimicking lower IRX3 and IRX5 expression levels in humans. Unlike Irx3tLZ/tLZ and Irx5eGFP/eGFP mice, Irx3/5dHet mice do not show a runty phenotype and develop with a normal body length at weaning (Son et al., 2021a). Nonetheless, Irx3/5dHet mice display lower body weight and fat mass (Figure 1B), indicating that the dosage of Irx3 and Irx5 is critical for body mass regulation and suggesting that Irx3 and Irx5 play overlapping functions in energy homeostasis. Body weight and mass composition are determined by the balance of energy (food) intake and energy expenditure. Irx3/5dHet mice show reduced food intake as well as elevated energy homeostasis (Table 1). Specifically, they exhibit beiging of white adipose tissue (WAT) with upregulation of brown adipocyte markers including Ucp1 in WAT. In the hypothalamus, reduction of Irx3 and Irx5 dosage results in elevated neurogenesis during the early postnatal period leading to an increased number of orexigenic and anorexigenic arcuate neurons (Son et al., 2021a). Owing to the higher number of these leptin-sensing arcuate neurons, Irx3/5dHet mice display enhanced hypothalamic leptin response and reduced food intake. Indeed, reduced food intake is a key feature of the metabolic phenotypes of Irx3/5dHet mice as these mutant mice still exhibit a body weight significantly lower than their control counterparts at thermoneutrality, when the effects of adipose thermogenesis and difference in energy expenditure are minimized (Son et al., 2021a). These observations suggest that higher expression of IRX3 and IRX5 could lead to elevated food intake contributing to human obesity, which is mostly associated with excessive energy consumption.
The hypothalamic function of Irx3 in energy homeostasis has been examined in transgenic as well as conditional knockout models using Ins2-Cre, which targets specific hypothalamic cells with high Irx3 and Irx5 expression (see below). Ins2-Cre;Rosa26EnR–Irx3 mice with hypothalamic expression of a dominant-negative form of Irx3 (EnR-Irx3; full length IRX3 protein fused to the Engrailed transcriptional repressor domain) exhibit a strong metabolic phenotype with reduced food intake and elevated energy expenditure, similar to those of Irx3tLz/tLz and Irx5eGFP/eGFP mice (Table 1; Smemo et al., 2014). In contrast, Ins2-Cre;Irx3Flox/Flox mice with specific hypothalamic deletion of Irx3 display a less pronounced metabolic phenotype; they are not as lean as Ins2-Cre;Rosa26EnR–Irx3 mice and do not exhibit any adipose beiging/energy expenditure phenotypes (Table 1). Specifically, the phenotypes of Ins2-Cre;Irx3Flox/Flox mice include reduced food intake, enhanced hypothalamic leptin response, and elevated neurogenesis in the postnatal hypothalamus, which are also observed in Irx3/5dHet mice (Figure 1B; Son et al., 2021a). Together, these results suggest a hypothalamic function of Irx3 as well as Irx5 in feeding regulation through the control of postnatal neurogenesis. As most clinical data have illustrated that the risk alleles of FTO are associated with increased energy intake, our data highlight hypothalamic postnatal neurogenesis regulated by IRX3 and IRX5 as a potential mechanism affecting leptin response in human obesity.
Expression of IRX3 and IRX5 in Radial Glia-Like Cells of the Postnatal Mouse Hypothalamus
The hypothalamic ARC-ME contains diverse cell types involved in energy homeostasis (Timper and Bruning, 2017). de Araujo et al. (2019) reported that Irx3 is expressed in a subset of POMC neurons from the analysis of a public single-cell RNA-sequencing (scRNA-seq) dataset of ∼20,000 hypothalamic ARC-ME cells and suggested that POMC neuronal expression of Irx3 is important for the regulation of energy homeostasis (Campbell et al., 2017; de Araujo et al., 2019). On the contrary, neuronal expression of Irx3 and Irx5 is minimal in the postnatal mouse hypothalamus. Neither Irx3 nor Irx5 expression is detectable in the published transcriptional profiles of POMC neurons and AgRP neurons (Henry et al., 2015). Furthermore, in a recently published scRNA-seq dataset by Romanov et al., which profiled gene expression of the whole hypothalamus at diverse developmental timepoints from embryonic day (E) 15.5 to postnatal day (P) 23, Irx3 and Irx5 are almost exclusively expressed in radial glial cells at postnatal stages though their expression can be found in neurons of the posterior hypothalamus at embryonic timepoints (Romanov et al., 2020).
In our recent study, analysis of publicly available scRNA-seq datasets from adult hypothalamic ARC-ME revealed the expression of Irx3 and Irx5 mostly in RGLs including ependymocytes and tanycytes, as well as in some NG2+ OPCs. By RNA in situ hybridization, Irx3 and Irx5 expression are found along the wall of the third ventricle which harbors both ependymocytes and tanycytes (Figures 2A,B). Furthermore, by scRNA-seq analysis of Ins2-Cre;tdTomato+ cells from P10 ARC-ME, which include cells lining the third ventricle and their descendants in the ARC-ME, the expression of Irx3 and Irx5 is mainly detected in a novel RGL-NSC population in addition to tanycytes, ependymocytes, and NG2+ OPCs. Our data illustrate that Irx3 and Irx5 are predominantly expressed in the RGL-NSCs, which are distinct from tanycytes, and behave as neural progenitors in the postnatal mouse hypothalamus. Importantly, the function of Irx3 in postnatal neurogenesis in the hypothalamic ARC-ME and feeding regulation has been established by conditional deletion in these cells and their descendants in Ins2-Cre;Irx3Flox/Flox mice (Son et al., 2021a). Before discussing the potential functions of Irx3 and Irx5 in RGL-NSCs, we provide below a brief review of postnatal-adult neurogenesis and description of different RGL cell types residing in the hypothalamus.
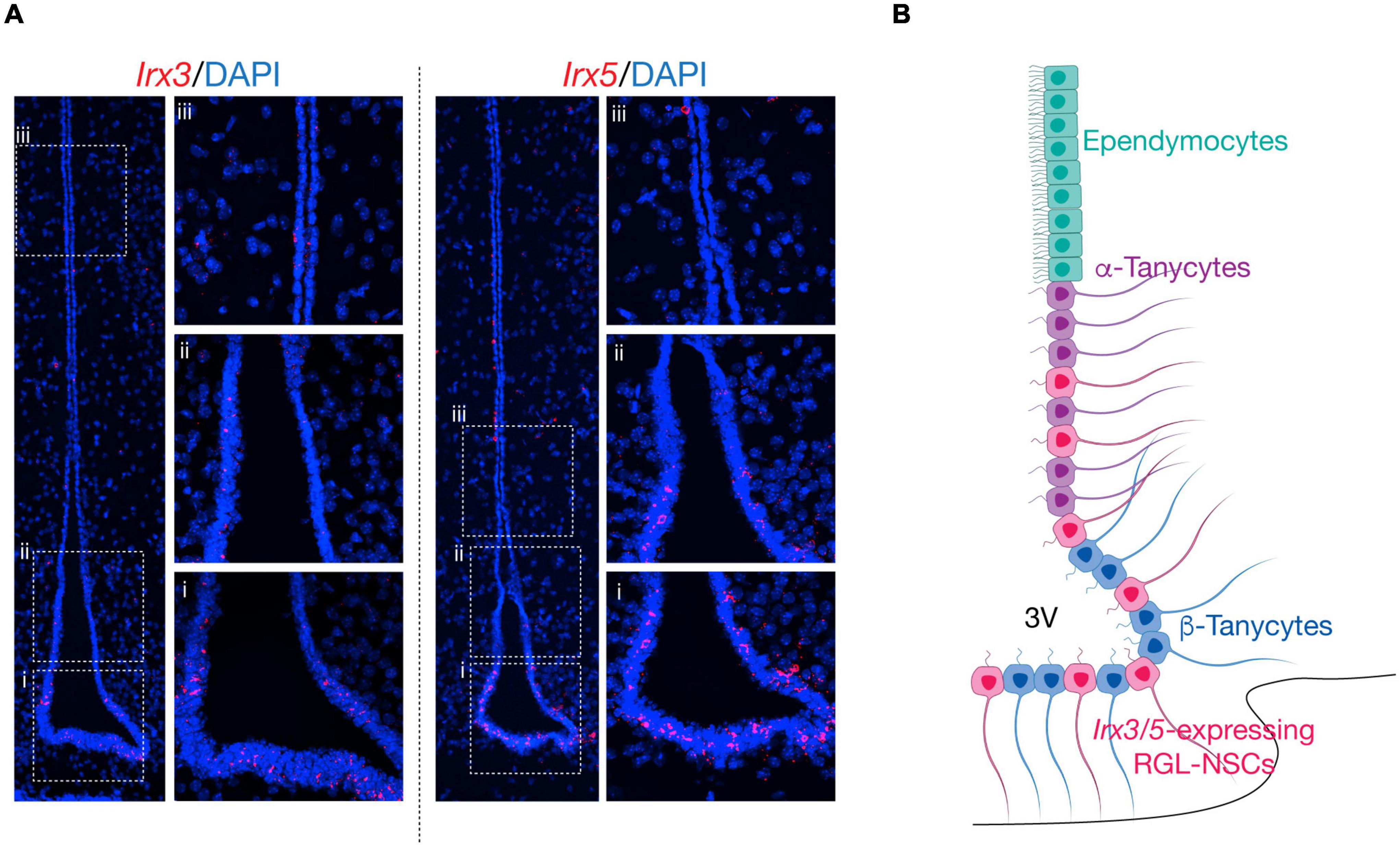
Figure 2. Schematic of Irx3 and Irx5 expression in the mediobasal hypothalamus. (A) RNA in situ hybridization of Irx3 and Irx5 the mediobasal hypothalamus at postnatal day (P) 14. Irx3 and Irx5 expression is mainly found in radial glia-like cells lining the third ventricle, including RGL-NSCs, tanycytes, and ependymocytes. Panels from bottom to top (ventral to dorsal) show representative regions from: β-tanycytes (i), α-tanycytes (ii) and ependymocytes (iii). (B) Schematic of the mediobasal hypothalamus. Along the third ventricle, ependymocytes are located at the dorsal ventricular walls, followed by α-tanycytes, and β-tanycytes. Irx3- and Irx5-expressing RGL-NSCs are interspersed among α-tanycytes and β-tanycytes in the dorsal ventricular wall and floor.
Postnatal-Adult Neurogenesis in the Hypothalamus
Neurogenesis is a complex and highly regulated process that results in the generation of new neurons. It occurs at high rates during the embryonic period when substantial quantities of new cells are generated by the proliferation/differentiation of neural precursor cells (NPCs, also known as radial glia cells (RGCs) as these NPCs possess astrocyte-like radial processes) (Gotz and Huttner, 2005; Taverna et al., 2014; Obernier and Alvarez-Buylla, 2019). Over the past decades, studies in multiple mammalian species have illustrated that substantial levels of neurogenesis persist in specific adult brain areas, known as neurogenic niches (Dimou and Gotz, 2014). Postnatal-adult neurogenesis corresponds to the series of events that lead to the production of new neurons in the postnatal-adult brain, from precursor cell division to the survival and functional integration of newly differentiated neurons (Lledo et al., 2006). Adult neurogenesis in the mouse brain starts around the second postnatal week (P14), when radial glia cells shift sharply from an embryonic to an adult state with distinct molecular identity from that of their embryonic progenitors, and continues throughout life (Hochgerner et al., 2018; Ghosh, 2019). In the adult mouse brain under normal conditions, well-characterized niches are mainly located in the subventricular zone (SVZ) of lateral ventricles (Doetsch et al., 1999; Magavi et al., 2000) and the hippocampal subgranular zone (SGZ) (Altman and Das, 1965). However, there are evidence suggesting constitutive neurogenesis also in the proximity of circumventricular organs including hypothalamus (Kokoeva et al., 2005, 2007; Hourai and Miyata, 2013).
Development of hypothalamic neuronal system starts during the embryonic period (Padilla et al., 2010; Ishii and Bouret, 2012; MacKay and Abizaid, 2014). In mice, hypothalamic neurons are mainly born during the embryonic period with a sharp peak of neurogenesis occurring around E12.5 (Padilla et al., 2010; Ishii and Bouret, 2012), followed by postnatal neurogenesis that actively contributes to the remodeling of hypothalamic neuronal population in juvenile (young adult; between P15 and P35) and adult mice (Lee et al., 2012; McNay et al., 2012; Haan et al., 2013; Yoo and Blackshaw, 2018; Figure 3). Although the basal (unstimulated) levels of neurogenesis in the adult hypothalamus are probably low and less than those seen in the well-established neurogenic niches, SVZ and SGZ (Lledo et al., 2006; Migaud et al., 2010), neurogenesis in the hypothalamus continues throughout adulthood and contributes to remodeling of the hypothalamic neuronal circuit. Early in vitro studies reported that cells dissociated from rodent hypothalamus, including the parenchymal regions and the ependymal layer of the third ventricle, proliferate, form neurospheres, and subsequently differentiate into both neural and glial lineages (Evans et al., 2002; Markakis et al., 2004; Xu et al., 2005), supporting the notion that third ventricle wall and its vicinity, particularly hypothalamic ARC-ME, are neurogenic niches in the adult mouse brain. These findings have been corroborated by studies using 5-bromo-2′-deoxyuridine (BrdU) or 5′-iodo-2′-deoxyuridine (IdU) labeling of proliferating cells as well as cell lineage analysis (Gotz and Huttner, 2005; Kokoeva et al., 2007; Lee et al., 2012; Li et al., 2012; McNay et al., 2012; Haan et al., 2013; Robins et al., 2013). ARC neurons exhibited substantial turnover in the adult mouse, with more than half of those neurons found at 4 weeks of age being replaced over the following 8 weeks (Pierce and Xu, 2010; Lee et al., 2012; Li et al., 2012; McNay et al., 2012). These adult-born hypothalamic neurons functionally integrate into the neuronal circuitry (Robins et al., 2013) and physiological responses including neuroendocrine regulation (e.g., release neurotransmitter/neuropeptides and leptin sensing) (Kokoeva et al., 2005; Pierce and Xu, 2010; Haan et al., 2013; Son et al., 2021a), suggesting adult neurogenesis likely contributes to other hypothalamic functions such as regulation of energy homeostasis.
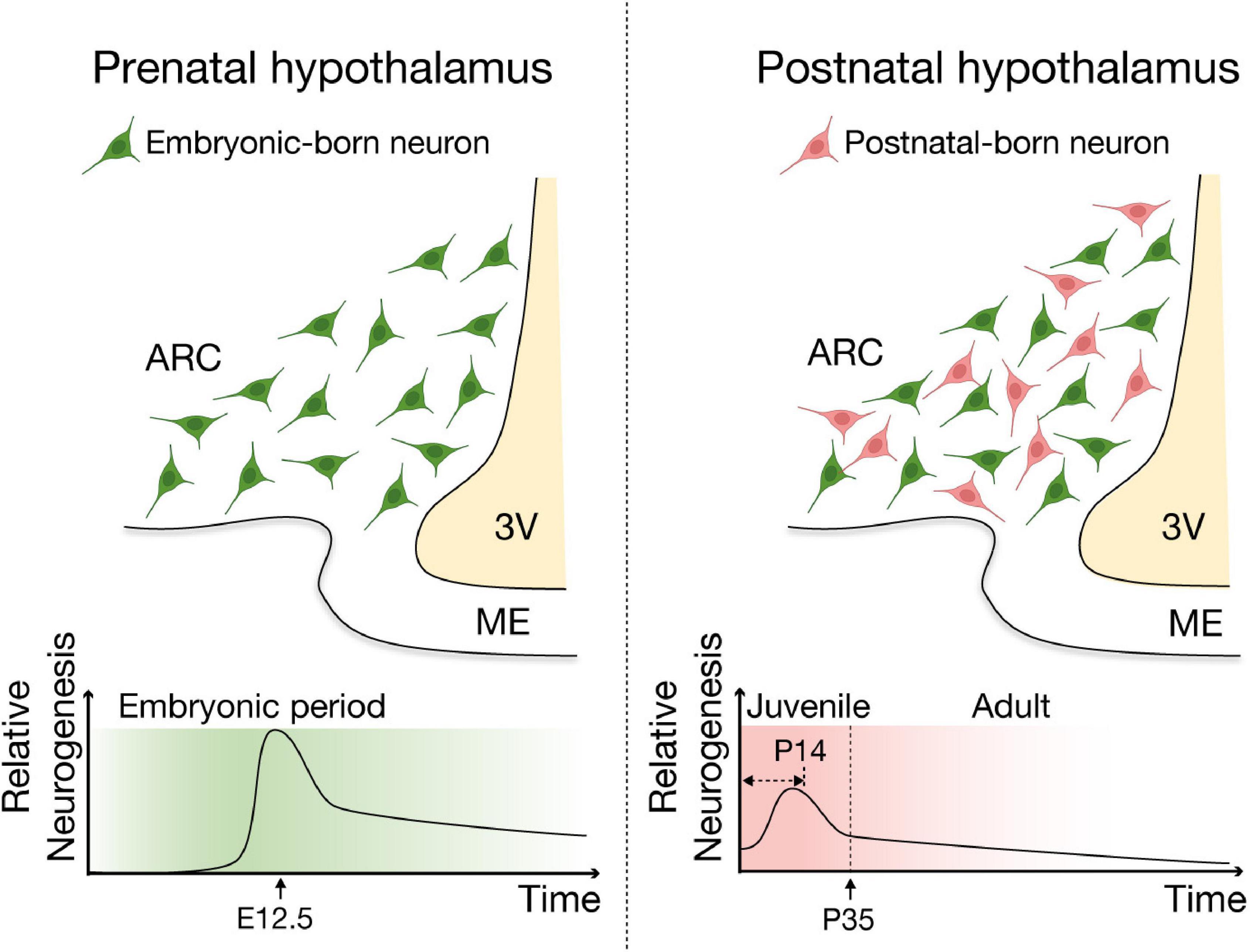
Figure 3. Schematic of embryonic and postnatal neurogenesis in the ARC-ME of the hypothalamus. Two major waves of neurogenesis have been reported in the hypothalamus. In mice, the neurogenic period of the developing hypothalamus encompasses embryonic day (E) 10 to E16, with a peak of neurogenesis at around E12.5. A second wave of neurogenesis is detected during the juvenile stages, especially within the first two postnatal weeks and continues throughout adulthood and contributes remodeling of the hypothalamic neuronal circuit. This figure was adapted from Son et al. (2021a) with modification.
Radial Glia-Like Cells in Postnatal Hypothalamic Neurogenesis
In the SVZ and SGZ, postnatal and adult NPCs are also known as RGLs, which share the characteristics of embryonic RGCs, including radial morphology, apical contact with the brain ventricles and expression of stemness markers (Urban and Guillemot, 2014; Morales and Mira, 2019). They are capable of undergoing both self-renewal through cell division and generating other neural cell types through differentiation. Besides these two neurogenic niches, RGLs also exist in other CNS regions, such as in the retina, where they are known as Muller glia (Goldman, 2014), and in some circumventricular organs, such as the median eminence of the hypothalamus (Bennett et al., 2009; Hourai and Miyata, 2013; Furube et al., 2020).
Tanycytes, Neural Progenitors in Postnatal Hypothalamic Neurogenesis
Tanycytes are specialized RGLs with a single elongated basal process that projects toward the hypothalamus parenchyma. They reside in the hypothalamus ventricular layer but are distinct from the cuboid and multi-ciliated ependymocytes. Tanycytes originate from the infundibulum of the mesencephalon, which is a local extension of the neuroepithelium located directly above the Rathke’s pouch (or future pituitary) (Pearson and Placzek, 2013; Rizzoti and Lovell-Badge, 2017). The LIM homeodomain transcription factor Lhx2 is critical for tanycyte specification and development, through activation and maintenance of the tanycyte-specific transcription factor Rax, and inhibition of the ependymocyte gene Rarres2 (Miranda-Angulo et al., 2014; Salvatierra et al., 2014). In rats, tanycytes arise perinatally and terminally differentiate during the first postnatal month (Rodriguez et al., 2005). In mice, the expression of tanycyte markers Rax and Gpr50 are first detected by RNA in situ hybridization around E14.5 in the ventricular zone of the mesencephalon, likely corresponding to “pre-tanycytes” (Shimogori et al., 2010). Recent scRNA-seq studies have identified a cell type closely resembling hypothalamic RGCs around E10 that has the potential to differentiate into multiple neural progenitors (Kim et al., 2020; Romanov et al., 2020; Zhang et al., 2021). Though tanycytes and ependymocytes are likely derived from these hypothalamic RGCs at late embryonic stages, their lineages appear to diverge as early as E13, as demonstrated by the expression of their respective cell type specific markers. Candidate factors, such as Wnt7b, Hopx, Ptch1, Nfib and Foxj1, are suggested to be implicated in this process (Kim et al., 2020). Along the same lines, Zhang et al. (2021) proposed a “state-switching” model, where a subpopulation of hypothalamic RGCs switch into tanycyte precursors at an early developmental stage (∼E11) and suggested that a substantial fraction of “pre-tanycytes” are generated in parallel to prenatal neurogenesis (Zhang et al., 2021).
Based on their dorsal-ventral location along the ventricular layer, marker gene expression and functions, tanycytes can be divided into four major subtypes, namely α1, α2, β1, and β2 (reviewed in Rodriguez et al., 2005; Goodman and Hajihosseini, 2015; Rizzoti and Lovell-Badge, 2017; Yoo and Blackshaw, 2018). Tanycytes not only play important regulatory roles in energy balance (Yoo et al., 2020), transport of nutrients and hormones between the blood-hypothalamus barrier (Mullier et al., 2010; Langlet et al., 2013; Balland et al., 2014; Miranda-Angulo et al., 2014; Duquenne et al., 2021), but also express canonical markers of adult NSCs in the SVZ and SGZ, including Nestin, Sox2, Vimentin, etc. (Rodriguez et al., 2005; Ming and Song, 2011; Lee et al., 2012; Bolborea and Dale, 2013; Goodman and Hajihosseini, 2015; Campbell et al., 2017; Yoo and Blackshaw, 2018).
Genetic lineage tracing has served as a powerful tool in uncovering the functional heterogeneity of neural precursor cells in postnatal and adult mouse hypothalamus as well as mapping their fate and differentiation potential. Table 2 summarizes various Cre mouse lines that have been used to target different hypothalamic neural precursors, where typically Cre recombinase is driven by a gene promoter of interest at a specific timepoint, allowing for the induction and expression of a fluorescent protein (or other markers) in the targeted neural stem/progenitor cells and its descendant progeny in the hypothalamus. In combination with BrdU labeling, these lineage tracing experiments highlight the proliferative ability of ventricular zone in the postnatal mouse hypothalamus. By using Rax-CreER (targeting all tanycytes), tanycytes were shown to give rise to both neurons and glia in the postnatal and juvenile mouse hypothalamus (Pak et al., 2014; Yoo et al., 2021). These findings were further supported by scRNA-seq analysis of Rax-CreER lineage cells (Yoo et al., 2021). However, it is worth noting that the neurogenic and gliogenic abilities of Rax+ tanycytes appear to be drastically reduced at adult stage, as tanycytes labeled by Rax-CreER at P60 generate very few neurons under physiological conditions (Pak et al., 2014).
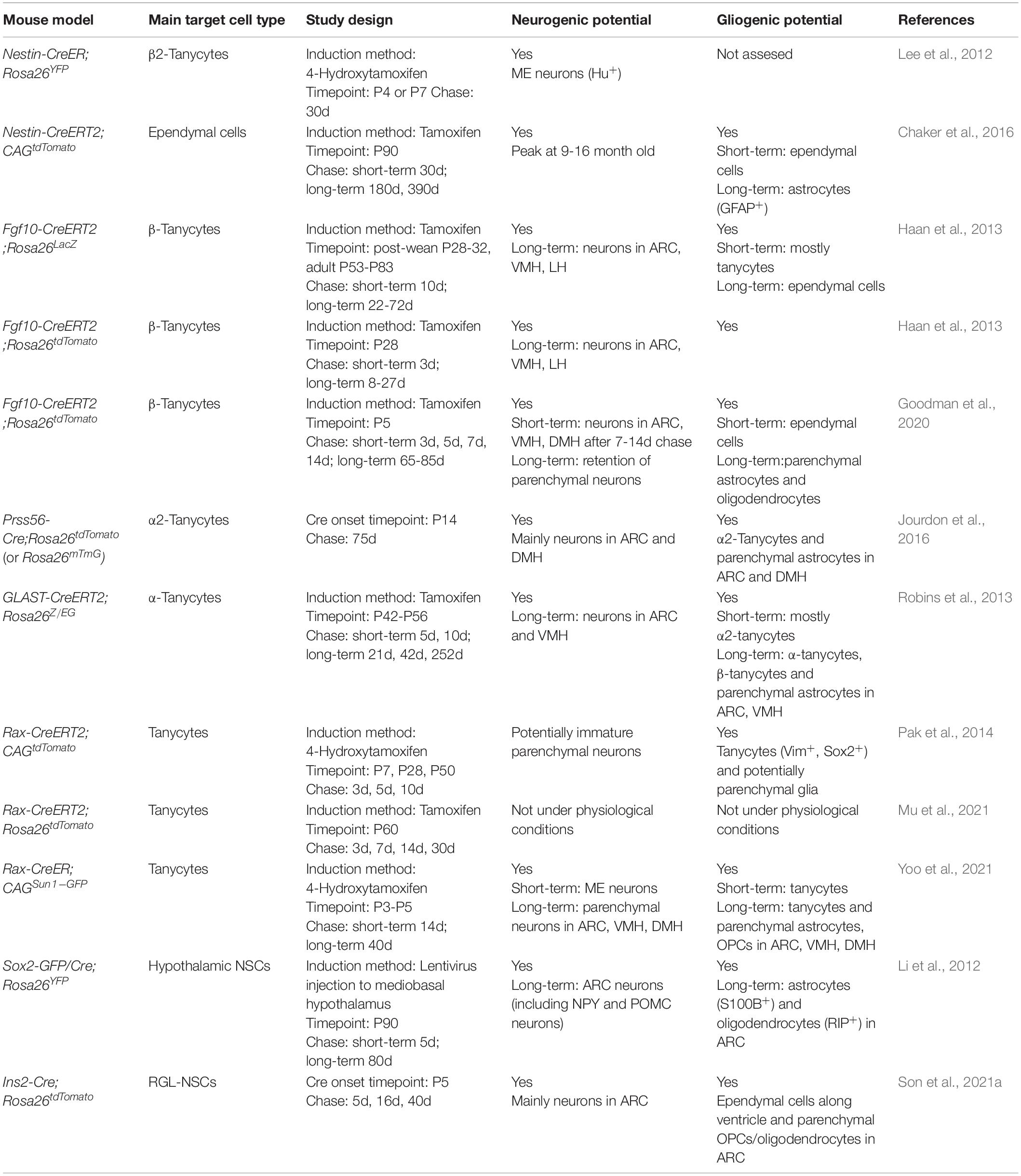
Table 2. Summary of lineage tracing studies of postnatal hypothalamic neurogenesis from radial glia-like cells.
These lineage tracing studies support the idea of RGL heterogeneity in the postnatal mouse hypothalamus and indicate that different RGL subtypes have distinct proliferation/differentiation abilities. Specifically, α-tanycytes, which are located at the lateral walls of the third ventricle, can be labeled by GLAST-Cre and appear to be mostly gliogenic with restricted neurogenic potential (Haan et al., 2013; Robins et al., 2013). They have limited self-renewal properties, generating new α- and β-tanycytes in the hypothalamic ventricular region (Robins et al., 2013). On the other hand, ventrally located β-tanycytes, such as Fgf10-labeled β-tanycytes and Nestin-labeled β2-tanycytes, show higher neurogenic potentials adding new neurons mainly to the VMH/ARC and ME, respectively (Lee et al., 2012; Haan et al., 2013; Goodman et al., 2020). Recently, Fgf10-labeled β-tanycytes have also been demonstrated to have the ability to give rise to a proliferating population of α-tanycytes that later undergoes both symmetric and asymmetric divisions to generate parenchymal daughter cells, suggesting that α-tanycytes can act as transient amplifying/intermediate cells during postnatal hypothalamic neurogenesis (Goodman et al., 2020).
Novel Radial Glia-Like Neural Stem Cells in the Postnatal ARC
Through lineage tracing and scRNA-seq analyses using Ins2-Cre, Son et al. (2021a) unveiled a previously unreported RGL cell type, termed as radial glia-like NSCs (RGL-NSCs), which line the hypothalamic third ventricle from around P5 (Son et al., 2021a). At P10, there is a rapid expansion of Ins2-Cre labeled cells in the parenchyma of the ARC, which gradually plateau after P21. Importantly, among the Ins2-Cre labeled cells in the parenchyma of adult ARC, over 85% of them are Hu+ neurons, and about one third of leptin-responsive AgRP/NPY and POMC/CART neurons in the adult ARC are derived from the Ins2-Cre lineage. These results suggest that the novel RGL-NSCs possess robust neurogenic capability in early postnatal mouse hypothalamus and corroborate with those of previous studies (Pierce and Xu, 2010; McNay et al., 2012) illustrating the presence of rapid and continuous neuronal turnover in the postnatal mouse hypothalamus.
Noticeably, when Cre activities are induced at a similar postnatal timepoint (∼P3-P5), while Rax-CreERT and other Cre mouse lines, such as Fgf10-Cre, efficiently label tanycytes and their descendant progenies in the VMH and DMH, Ins2-Cre appears to be more ideal for marking newly generated neurons residing in the ARC and ME regions of postnatal mouse hypothalamus. In addition, these RGL-NSCs are molecularly distinct from tanycytes of the mouse hypothalamus, as they do not express the canonical markers, such as Rax and Crym (Figure 4A). Indeed, comparison with Rax-CreERT marked cells (Yoo et al., 2021) at similar postnatal timepoints using a ‘reference-based integration’ analysis with the single-cell R toolkit Seurat (Stuart et al., 2019) reveals minimal overlap between the progeny of Rax-CreERT labeled tanycytes and those of RGL-NSCs (Figure 4B). By pseudo-temporal analysis (Wolf et al., 2019), RGL-NSCs are inferred to possess the potential of differentiating into both tanycyte and OPC lineages (Figure 4C). Interestingly, the expression of Irx3 and Irx5 appears to be downregulated upon differentiation of RGL-NSCs (Figure 4C). These RGL-NSCs display strong expression of stemness as well as astroglia markers including Sox2, Hes5, Agt, etc., which are features reminiscent of the adult NSCs in the SVZ and SGZ (Figure 4A). In particular, their transcriptome resembles those of the Sox2-eGFP NSCs from the SVZ of adult mouse brain (Shah et al., 2018). Furthermore, these hypothalamic RGL-NSCs also show high expression of neurogenic TFs including Pax6, Nkx2.2, and Olig2, which are consistent with their purported roles in neuron and oligodendrocyte differentiation.
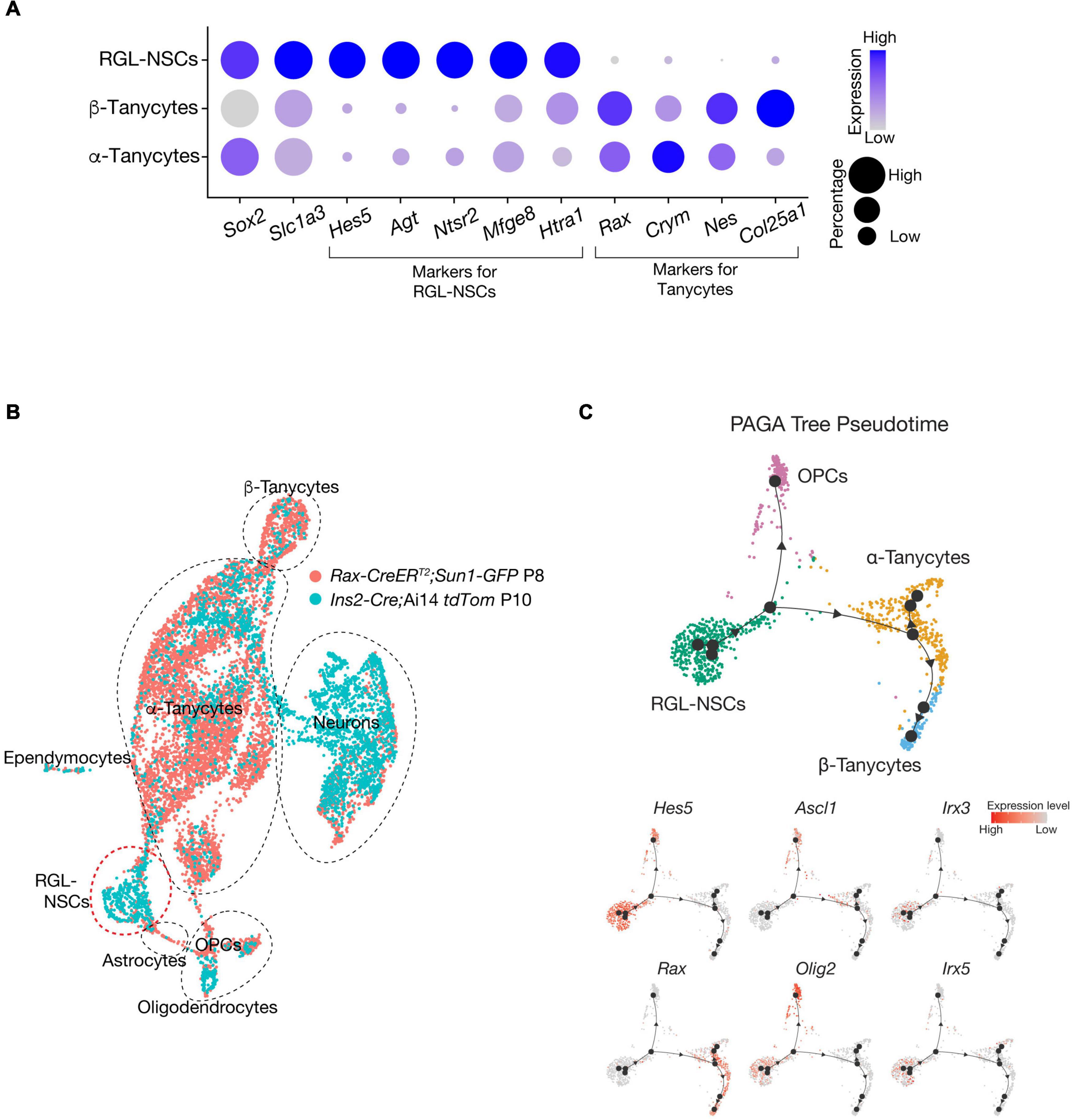
Figure 4. Irx3 and Irx5 expressing RGL-NSCs are distinct from tanycytes. (A) Expression of cell type-specific markers in RGL-NSCs, α-tanycytes, and β-tanycytes from the Ins2-Cre;Ai14 tdTomato scRNA-seq dataset. The size of the dot indicates the percentage of cells expressing a specific gene in each cluster, and the color represents the average expression level of the gene. RGL-NSCs and tanycytes share expression of the broad stemness marker Sox2 and glial marker Slc1a3 (also known as GLAST), while NSCs markers such as Hes5, Agt, Ntsr2 are almost exclusively found in RGL-NSCs, and canonical tanycytes markers such as Rax and Crym are absent in RGL-NSCs. (B) Integrated UMAP plot of Rax-CreERT2;Sun1-GFP at P8 (Cre recombinase activity induced between P3 and P5) (GSE160378) and Ins2-Cre;Ai14 tdTomato at P10 (GSE154969) scRNA-seq datasets through the reference-based integration feature of Seurat and UMAP visualization. The RGL-NSC cluster consists of cells originating from the Ins2-Cre;Ai14 tdTomato dataset, and does not overlap with tanycytes nor astrocytes. Also note that while a much larger amount of tanycytes are detected in the Rax-CreERT2;Sun1-GFP dataset, Ins2-Cre;Ai14 tdTomato is more robust at labeling neurons. (C) Pseudo-temporal analysis of Ins2-Cre labeled RGL-NSCs, tanycytes and OPCs using Partition-based graph abstraction (PAGA) Tree. The line with arrowhead indicates the inferred differentiation trajectory, which originates from Hes5-expressing RGL-NSCs, undergoes a bifurcated differentiation path to Olig2-expressing OPCs and Rax-expressing tanycytes. The NSC activation marker Ascl1 is detected in bridge cells but not in RGL-NSCs, suggesting their quiescence.
By profiling the transcriptome of the whole hypothalamus at multiple developmental timepoints from E15.5 to P23 using scRNA-seq, Romanov et al. (2020) have determined a comprehensive single cell map of the developing hypothalamus and identified a population of RGCs marked by Sox2 and Hes5 expression at both E15.5 and E17.5. These RGCs appear to be drastically diminished after birth and are apparently replaced by a postnatal type of RGL with elevated expression of several adult NSC markers including Agt, Htra1, Ntsr2 and Mfge8 (Yuzwa et al., 2017; Hochgerner et al., 2018; Shah et al., 2018; Zywitza et al., 2018). Intriguingly, the transcriptional profiles of the RGL-NSCs labeled by Ins2-Cre are highly similar to those of the postnatal RGLs identified by Romanov et al. (2020), but distinct from those of the embryonic RGCs. Together, these observations suggest that RGL-NSCs represent a postnatal type of RGL with robust neurogenic potential and Ins2-Cre can be used for enriching these postnatal hypothalamic NSCs in the mouse brain.
Regulatory Factors of Hypothalamic Postnatal Neurogenesis
Factors, such as hormonal signals, environment, and genetics, could affect postnatal neurogenesis in the hypothalamus. Specifically, remodeling of ARC through elevated neurogenesis by treatment with ciliary neurotrophic factor (CNTF) could lead to leptin-dependent weight loss and reduced food intake (Kokoeva et al., 2005). Secreted factors including epidermal growth factor (EGF), basic fibroblast growth factor (bFGF), insulin-like growth factor I (IGF-I) and brain-derived neurotrophic factor (BDNF) also could significantly stimulate proliferation, survival and development of newborn neurons in the adult stage (Pencea et al., 2001; Xu et al., 2005; Perez-Martin et al., 2010; Robins et al., 2013; Chaker et al., 2016).
Given the importance of the hypothalamus in controlling energy homeostasis, several studies using rodent models have evaluated the environmental effects on neurogenesis. In this aspect, perhaps the most widely studied factor is high fat diet (HFD), which has been reproducibly shown to impair survival of hypothalamic NSCs and inhibit neurogenesis in the hypothalamus (Lee et al., 2012, 2014; Li et al., 2012; McNay et al., 2012). For example, Li et al. (2012) reported that the neurogenic impairment of Sox2+ hypothalamic NSCs from chronic HFD treatment is associated with overactivation of the pro-inflammatory pathway involving IκB kinase β (IKKβ) and its downstream nuclear factor κB (NF-κB), as well as paracrine release of cytokines from neighboring microglia. By in vitro studies, they further suggested that IKKβ/NF-κB inhibit neuronal differentiation of these hypothalamic NSCs through direct activation of downstream Notch signaling (Li et al., 2012). Intriguingly, neurogenesis in the ME is increased in response to HFD in female mice illustrating that there is an apparent sex-difference in the control of hypothalamic neurogenesis (Lee et al., 2012, 2014). Although scRNA-seq analysis suggest that young male and female adult mice have a similar proportion of tanycytes and other neural cell types under normal diet (Campbell et al., 2017), their distinct responses to HFD could be attributed to multiple factors, such as sex hormone levels, pubertal neurogenesis in sexually dimorphic brain regions, penetrance of the blood-brain barrier, etc. (Ahmed et al., 2008; Lee et al., 2014). Furthermore, caloric restriction is associated with a tendency of reduced neurogenesis within the ME (Lee et al., 2014). On the other hand, heat exposure and physical activity facilitate proliferation of neuronal progenitor cells in the hypothalamus and promotes differentiation to neurons (Matsuzaki et al., 2009; Niwa et al., 2016).
In addition to extrinsic factors, genetic studies have begun to unveil the role of intrinsic factors in the control of postnatal ARC neurogenesis. For example, deletion of the nuclear factor I family of transcription factors (Nfia/b/x) in Rax+ tanycytes results in activation of Shh and Wnt signaling, which in turn stimulates proliferation of tanycytes and their robust neurogenic differentiation in postnatal mouse hypothalamus (Yoo et al., 2021). This study highlights the inhibitory functions of Nfia/b/x TFs in restricting the neurogenic differentiation of tanycytes and suggests the involvement of Shh and Wnt signaling in the control of hypothalamic postnatal neurogenesis. Goodman et al. (2020) reported that Fgf10 acts as a negative regulator of postnatal neurogenesis in mouse hypothalamus (Goodman et al., 2020). Conditional loss of Fgf10 in β-tanycytes retards their amplification, which is compensated by retention and delay of their transient intermediate progeny, eventually leading to increased number of parenchymal neurons in the VMH, DMH and ARC. Recently, Son et al. (2021a) demonstrated the regulatory functions of Irx3 and Irx5 in hypothalamic postnatal neurogenesis and ARC remodeling affecting leptin response. Specifically, Irx3/5dHet mice as well as Ins2-Cre;Irx3Flox/Flox mice with specific deletion of Irx3 in the RGL-NSCs both exhibit elevated neurogenesis in the postnatal ARC, leading to higher number of leptin-sensing neurons (Son et al., 2021a).
Activation of Neural Stem Cells in Postnatal Hypothalamus
In the SVZ and SGZ of adult mouse brain, the majority of RGLs are mitotically inactive and remain largely in G0 (out of the cell cycle), a fundamental characteristic that distinguishes them from embryonic RGCs (Morales and Mira, 2019). These quiescent NSCs (qNSCs) can be activated (active NSCs, or aNSCs) to re-enter the cell cycle, becoming either rapidly proliferating intermediate progenitor cells (IPC) that subsequently give rise to newborn neuronal cells, or returning to quiescence (Figure 5). Quiescence of NSCs is typically marked by astroglia features (Agt, ApoE, Aldh1h, etc.) with high Hes and Id gene expression, while factors associated with aNSCs include Fgfr3, Ascl1 and cell cycle genes (e.g., cyclinD) (Shin et al., 2015; Urban et al., 2016; Morales and Mira, 2019; Obernier and Alvarez-Buylla, 2019; Urban et al., 2019). Using a combination of fate mapping and scRNA-seq analyses, recent studies have further suggested the presence of additional states between quiescence and active, such as “dormant” (or deeply quiescent) and “primed” states for qNSCs, as well as early, mid or late stages for aNSCs, illustrating the functional and molecular heterogeneity of adult NSCs (Dulken et al., 2017; Basak et al., 2018; Hochgerner et al., 2018; Shah et al., 2018; Bottes et al., 2021).
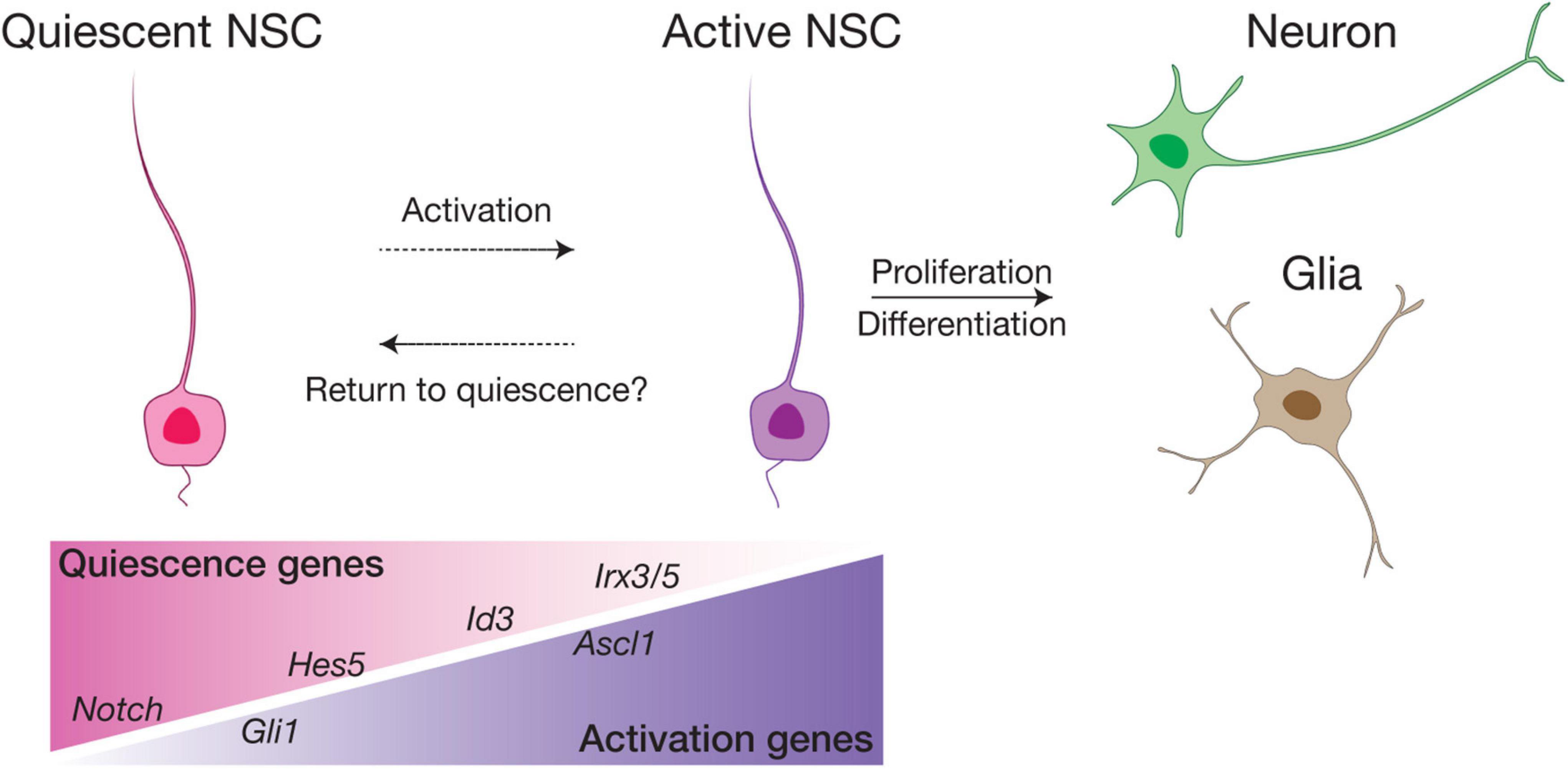
Figure 5. Schematic model of NSC quiescence and activation. In the SVZ and SGZ neurogenic niches, the majority of adult NSCs are quiescent and can be activated by various factors to proliferate and differentiate into neurons and glia. We postulate that in the ventral hypothalamus, RGL-NSCs are mostly in a quiescence state enriched in Notch ligand, Hes5 and Id3 expression. Irx3 and Irx5 are likely novel factors involved in maintaining the quiescence of RGL-NSCs in the postnatal hypothalamus. Sub-populations of primed RGL-NSCs expressing Gli1, and active NSCs marked by Ascl1 also exist in small proportions.
The balance between quiescence and activity is crucial for the long-term maintenance of adult NSC pools and prevention of tumors resulting from over-proliferation. Thus, this process is tightly regulated by multiple factors. Perhaps the most extensively studied signaling pathway in this context is Notch signaling, which plays a pivotal role in adult neurogenesis, promoting both quiescence and proliferation of NSCs. Deletion of the Notch effector gene Rbpj as well as inhibition of Notch signaling activate qNSCs, resulting in an immediate burst of neurogenesis, but ultimately lead to exhaustion of the NSC pool (Ehm et al., 2010; Imayoshi et al., 2010; Engler et al., 2018). On the other hand, activation of Notch signaling induces the expression of Hes and Hey families of TFs, which act together with Id factors, to maintain quiescence of NSCs through repression of the proneural TF Ascl1 (Bai et al., 2007; Urban et al., 2016; Boareto et al., 2017; Harris and Guillemot, 2019; Sueda et al., 2019). Shh signaling is another developmental pathway that has been implicated in postnatal and adult NSCs of the SVZ (Ahn and Joyner, 2005; Faigle and Song, 2013; Petrova et al., 2013; Daynac et al., 2016), where various components of the Shh signaling pathway are expressed. The use of Gli1-CreER mice illustrated that qNSCs and aNSCs of both SVZ and SGZ are Shh-responsive (Ahn and Joyner, 2005). Furthermore, a recent study using a combination of genetic fate mapping and transcriptome profiling studies revealed that Gli1+ qNSCs represent a long-term self-renewing qNSC subpopulation in the SGZ contributing to neurogenesis of the hippocampus (Bottes et al., 2021).
In our recent study, bioinformatics analysis has suggested that RGL-NSCs shift from quiescence to a more active state upon reduction of Irx3 and Irx5 dosage, which is consistent with the observation of elevated neurogenesis in Irx3/5dHet mice (Son et al., 2021a). These findings imply that Irx3 and Irx5 negatively regulate NSC activation and postnatal neurogenesis. Based on their transcriptomic profiles, most Ins2-Cre-labeled RGL-NSCs are quiescent with high expression of Hes and Id genes, and only very few cells show Ascl1 expression, representing a small population of aNSCs (Figure 6). Intriguingly, many Shh pathway genes are expressed in a subset of the RGL-NSCs indicating that Ins2-Cre-labeled RGL-NSCs might represent another Shh-responsive cell population in the postnatal brain; the membrane receptors (Ptch1 and Smo) as well as the intracellular mediators of Shh signaling (Sufu, Gli1, Gli2 and Gli3) are expressed in the RGL-NSC population, whereas the Shh ligand is expressed in neighboring tanycytes (Figure 6). Relevant to this, Irx3 and several neural progenitor genes, including Pax6, Nkx2.2 and Olig2, are known to be regulated by Shh signaling during ventral neural tube development (Briscoe et al., 2000; Qi et al., 2001; Zhou et al., 2001), and all these neural progenitor genes are also expressed in the RGL-NSCs. Furthermore, multiple studies have suggested that Irx genes are involved in early development of the central nervous system (Bellefroid et al., 1998; Gomez-Skarmeta et al., 1998; Cavodeassi et al., 2001). In the developing embryonic mouse brain, Irx3 and Irx5 might regulate the expression of its putative target Ascl1 (Cohen et al., 2000), which is a key regulator of embryonic neurogenesis in the hypothalamus (McNay et al., 2006; Alvarez-Bolado, 2019). Our recent study also suggests that Irx3 and Irx5 could affect postnatal hypothalamic neurogenesis through cell cycle regulation of RGL-NSCs (Son et al., 2021a). Consistent with this notion, reduction of Iro proteins promotes cell proliferation by accelerating G1 to S transition in Drosophila imaginal disks (Barrios et al., 2015) and loss of both Irx3 and Irx5 results in a higher proportion of G2/M cells with a reduction of G1 cells in the developing mouse limb bud (Tao et al., 2020).
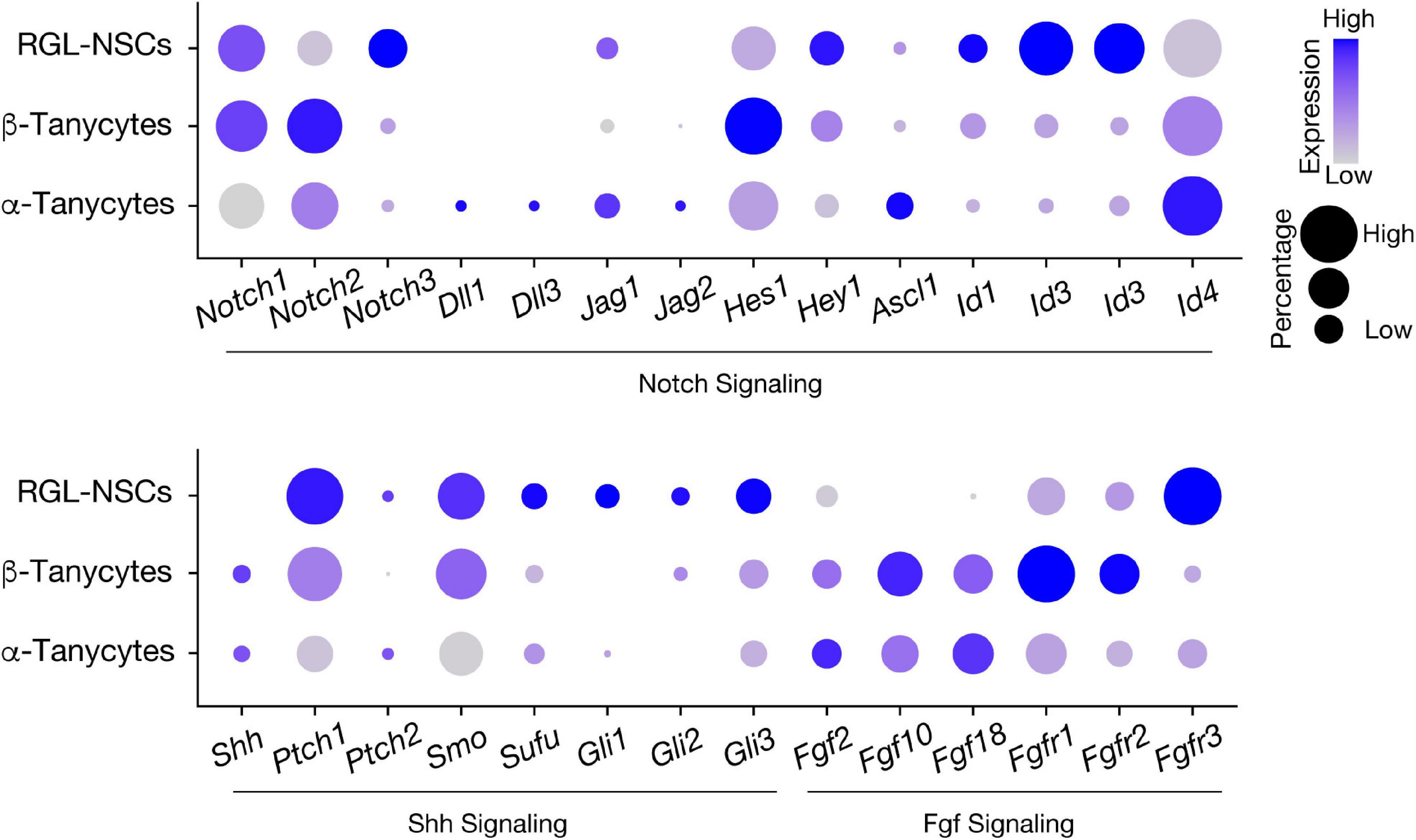
Figure 6. Expression of signaling pathway genes involved in neural stem cell activation in hypothalamic RGLs. Expression of Notch, Shh, and Fgf pathway-related genes in RGL-NSCs, α-tanycytes, and β-tanycytes from the Ins2-Cre;Ai14 tdTomato scRNA-seq dataset. The size of the dot indicates the percentage of cells expressing a specific gene in each cluster, and the color represents the average expression level of the gene.
Concluding Remarks and Future Directions
IRX3 and IRX5 have emerged as a strong link between the non-coding genetic variations of the FTO gene and obesity. Using genetic mouse models, work by us and others depicts their critical roles in metabolic regulation in the brain, as well as in peripheral organs such as adipose tissue (Smemo et al., 2014; Claussnitzer et al., 2015; Bjune et al., 2018; Sobreira et al., 2021; Son et al., 2021a,b). More recently, macrophage Irx3 has also been associated with metabolic inflammation and body weight control (Yao et al., 2021). In this review, we summarize the metabolic functions and regulatory mechanisms of Irx3 and Irx5 with a focus on the postnatal hypothalamus, where they are highly expressed, particularly in the newly identified RGL-NSCs giving rise to postnatal hypothalamic neurons, and regulate energy homeostasis. Recent technical advances such as chronic in vivo labeling, visualization tool, genetic lineage tracing, single-cell RNA sequencing and bioinformatic analysis have greatly contributed to our improved understanding of the hypothalamic neural precursor cells, and their contribution to hypothalamic neurogenesis. Using these approaches, we demonstrate that Ins2-Cre-labeled RGL-NSCs represent a bona fide population of NSCs in the postnatal mouse hypothalamus. Through differentiation into tanycytes and NG2+ OPCs, this novel NSC population likely contributes to both neuron and oligodendrocyte lineages in the hypothalamic ARC-ME, respectively.
Nonetheless, there are many remaining questions about RGL-NSCs and additional characterization is required. What factors regulate their stem cell behaviors and what are the molecular actions of Irx3 and Irx5 in these cells? Do they originate from a similar population of embryonic RGCs as tanycytes and ependymocytes, and what are the genes involved in their fate specification and divergence from other hypothalamic RGLs? Are they still present and functional in the adult and aging mouse hypothalamus?
While our current knowledge of hypothalamic RGL-NSCs is still limited, especially as their lineage relationship with other hypothalamic cell types is mainly based on computational analyses and lineage tracing experiments using the Ins2-Cre mouse line, additional in vivo fate mapping studies as well as clonal analysis using inducible labeling techniques specific to the RGL-NSC population are warranted. For example, the Hes5-CreERT2 mouse line, which has been previously used in mapping the NSC lineage of the hippocampal SGZ (Lugert et al., 2012), could be a powerful genetic tool to further investigate the lineage relationships between RGL-NSCs and other hypothalamic cell types such as tanycytes and NG2+ OPCs. In the hypothalamus, Hes5 is a marker of RGL-NSCs but is not found in other RGLs such as tanycytes, allowing for specific labeling of the RGL-NSC progeny (Figure 4A; Son et al., 2021a). In addition, the inducible nature of this mouse line, which is not available with the Ins2-Cre mouse line, will also enable targeting of RGL-NSCs at various timepoints during hypothalamus development, and investigating the functions of Irx3 and Irx5 as well as other regulatory factors in these RGL-NSCs in a spatiotemporal manner. At the same time, the lessons learnt from the neurogenic niches in SVZ and SGZ should provide us with many useful hints about potential genetic and environmental factors involved in the regulation of this novel hypothalamic NSC population. As RGL-NSCs and tanycytes display high expression of Notch, Shh and Fgf pathway genes (Figure 6), future studies focusing on these signaling pathways in the regulation of RGL-NSC behavior and hypothalamic neurogenesis in juvenile and adult mice will be informative. Furthermore, by defining the genes/pathways regulated by Irx3 and Irx5 in RGL-NSCs as well as their interplays with the Notch, Shh and Fgf pathways should unveil new knowledge about postnatal remodeling of the hypothalamus and provide mechanistic insights in NSC biology and obesity.
Author Contributions
ZD, JS, and C-CH conceived, designed, and wrote the manuscript. All authors contributed to the article and approved the submitted version.
Funding
This research was supported by Canadian Institutes of Health Research (PJT-178147) and the Canada Research Chairs program to C-CH and JS was supported by a postdoctoral fellowship award from Diabetes Canada.
Conflict of Interest
The authors declare that the research was conducted in the absence of any commercial or financial relationships that could be construed as a potential conflict of interest.
Publisher’s Note
All claims expressed in this article are solely those of the authors and do not necessarily represent those of their affiliated organizations, or those of the publisher, the editors and the reviewers. Any product that may be evaluated in this article, or claim that may be made by its manufacturer, is not guaranteed or endorsed by the publisher.
References
Ahmed, E. I., Zehr, J. L., Schulz, K. M., Lorenz, B. H., Doncarlos, L. L., and Sisk, C. L. (2008). Pubertal hormones modulate the addition of new cells to sexually dimorphic brain regions. Nat. Neurosci. 11, 995–997. doi: 10.1038/nn.2178
Ahn, S., and Joyner, A. L. (2005). In vivo analysis of quiescent adult neural stem cells responding to Sonic hedgehog. Nature 437, 894–897. doi: 10.1038/nature03994
Altman, J., and Das, G. D. (1965). Autoradiographic and histological evidence of postnatal hippocampal neurogenesis in rats. J. Comp. Neurol. 124, 319–335. doi: 10.1002/cne.901240303
Alvarez-Bolado, G. (2019). Development of neuroendocrine neurons in the mammalian hypothalamus. Cell Tissue Res. 375, 23–39. doi: 10.1007/s00441-018-2859-1
Bai, G., Sheng, N., Xie, Z., Bian, W., Yokota, Y., Benezra, R., et al. (2007). Id sustains Hes1 expression to inhibit precocious neurogenesis by releasing negative autoregulation of Hes1. Dev. Cell 13, 283–297. doi: 10.1016/j.devcel.2007.05.014
Bai, M., Han, Y., Wu, Y., Liao, J., Li, L., Wang, L., et al. (2019). Targeted genetic screening in mice through haploid embryonic stem cells identifies critical genes in bone development. PLoS Biol. 17:e3000350. doi: 10.1371/journal.pbio.3000350
Balland, E., Dam, J., Langlet, F., Caron, E., Steculorum, S., Messina, A., et al. (2014). Hypothalamic tanycytes are an ERK-gated conduit for leptin into the brain. Cell Metab. 19, 293–301. doi: 10.1016/j.cmet.2013.12.015
Barrios, N., Gonzalez-Perez, E., Hernandez, R., and Campuzano, S. (2015). The homeodomain iroquois proteins control cell cycle progression and regulate the size of developmental fields. PLoS Genet. 11:e1005463. doi: 10.1371/journal.pgen.1005463
Basak, O., Krieger, T. G., Muraro, M. J., Wiebrands, K., Stange, D. E., Frias-Aldeguer, J., et al. (2018). Troy+ brain stem cells cycle through quiescence and regulate their number by sensing niche occupancy. Proc. Natl. Acad. Sci. USA 115, E610–E619. doi: 10.1073/pnas.1715911114
Bellefroid, E. J., Kobbe, A., Gruss, P., Pieler, T., Gurdon, J. B., and Papalopulu, N. (1998). Xiro3 encodes a Xenopus homolog of the Drosophila Iroquois genes and functions in neural specification. EMBO J. 17, 191–203. doi: 10.1093/emboj/17.1.191
Bennett, L., Yang, M., Enikolopov, G., and Iacovitti, L. (2009). Circumventricular organs: a novel site of neural stem cells in the adult brain. Mol. Cell Neurosci. 41, 337–347. doi: 10.1016/j.mcn.2009.04.007
Bjune, J. I., Haugen, C., Gudbrandsen, O., Nordbo, O. P., Nielsen, H. J., Vage, V., et al. (2018). IRX5 regulates adipocyte amyloid precursor protein and mitochondrial respiration in obesity. Int J Obes 43, 2151–2162. doi: 10.1038/s41366-018-0275-y
Boareto, M., Iber, D., and Taylor, V. (2017). Differential interactions between Notch and ID factors control neurogenesis by modulating Hes factor autoregulation. Development 144, 3465–3474. doi: 10.1242/dev.152520
Bolborea, M., and Dale, N. (2013). Hypothalamic tanycytes: potential roles in the control of feeding and energy balance. Trends Neurosci. 36, 91–100. doi: 10.1016/j.tins.2012.12.008
Bottes, S., Jaeger, B. N., Pilz, G. A., Jorg, D. J., Cole, J. D., Kruse, M., et al. (2021). Long-term self-renewing stem cells in the adult mouse hippocampus identified by intravital imaging. Nat. Neurosci. 24, 225–233. doi: 10.1038/s41593-020-00759-4
Briscoe, J., Pierani, A., Jessell, T. M., and Ericson, J. (2000). A homeodomain protein code specifies progenitor cell identity and neuronal fate in the ventral neural tube. Cell 101, 435–445. doi: 10.1016/S0092-8674(00)80853-3
Cain, C. J., Gaborit, N., Lwin, W., Barruet, E., Ho, S., Bonnard, C., et al. (2016). Loss of Iroquois homeobox transcription factors 3 and 5 in osteoblasts disrupts cranial mineralization. Bone Rep. 5, 86–95. doi: 10.1016/j.bonr.2016.02.005
Campbell, J. N., Macosko, E. Z., Fenselau, H., Pers, T. H., Lyubetskaya, A., Tenen, D., et al. (2017). A molecular census of arcuate hypothalamus and median eminence cell types. Nat. Neurosci. 20, 484–496. doi: 10.1038/nn.4495
Cavodeassi, F., Modolell, J., and Gomez-Skarmeta, J. L. (2001). The Iroquois family of genes: from body building to neural patterning. Development 128, 2847–2855. doi: 10.1242/dev.128.15.2847
Cecil, J. E., Tavendale, R., Watt, P., Hetherington, M. M., and Palmer, C. N. (2008). An obesity-associated FTO gene variant and increased energy intake in children. N. Engl. J. Med. 359, 2558–2566. doi: 10.1056/NEJMoa0803839
Chaker, Z., George, C., Petrovska, M., Caron, J. B., Lacube, P., Caille, I., et al. (2016). Hypothalamic neurogenesis persists in the aging brain and is controlled by energy-sensing IGF-I pathway. Neurobiol. Aging 41, 64–72. doi: 10.1016/j.neurobiolaging.2016.02.008
Cheng, C. W., Chow, R. L., Lebel, M., Sakuma, R., Cheung, H. O., Thanabalasingham, V., et al. (2005). The Iroquois homeobox gene. Irx5, is required for retinal cone bipolar cell development. Dev. Biol. 287, 48–60. doi: 10.1016/j.ydbio.2005.08.029
Claussnitzer, M., Dankel, S. N., Kim, K. H., Quon, G., Meuleman, W., Haugen, C., et al. (2015). FTO obesity variant circuitry and adipocyte browning in humans. N. Engl. J. Med. 373, 895–907. doi: 10.1056/NEJMoa1502214
Cohen, D. R., Cheng, C. W., Cheng, S. H., and Hui, C. C. (2000). Expression of two novel mouse Iroquois homeobox genes during neurogenesis. Mech. Dev. 91, 317–321. doi: 10.1016/S0925-4773(99)00263-4
Costantini, D. L., Arruda, E. P., Agarwal, P., Kim, K. H., Zhu, Y., Zhu, W., et al. (2005). The homeodomain transcription factor Irx5 establishes the mouse cardiac ventricular repolarization gradient. Cell 123, 347–358. doi: 10.1016/j.cell.2005.08.004
Daynac, M., Tirou, L., Faure, H., Mouthon, M. A., Gauthier, L. R., Hahn, H., et al. (2016). Hedgehog controls quiescence and activation of neural stem cells in the adult ventricular-subventricular zone. Stem Cell Rep. 7, 735–748. doi: 10.1016/j.stemcr.2016.08.016
de Araujo, T. M., Razolli, D. S., Correa-Da-Silva, F., De Lima-Junior, J. C., Gaspar, R. S., Sidarta-Oliveira, D., et al. (2019). The partial inhibition of hypothalamic IRX3 exacerbates obesity. EBioMedicine 39, 448–460. doi: 10.1016/j.ebiom.2018.11.048
de Araujo, T. M., and Velloso, L. A. (2020). Hypothalamic IRX3: a new player in the development of obesity. Trends Endocrinol. Metab. 31, 368–377. doi: 10.1016/j.tem.2020.01.002
Dietrich, M. O., and Horvath, T. L. (2013). Hypothalamic control of energy balance: insights into the role of synaptic plasticity. Trends Neurosci. 36, 65–73. doi: 10.1016/j.tins.2012.12.005
Dimou, L., and Gotz, M. (2014). Glial cells as progenitors and stem cells: new roles in the healthy and diseased brain. Physiol. Rev. 94, 709–737. doi: 10.1152/physrev.00036.2013
Dina, C., Meyre, D., Gallina, S., Durand, E., Korner, A., Jacobson, P., et al. (2007). Variation in FTO contributes to childhood obesity and severe adult obesity. Nat. Genet. 39, 724–726. doi: 10.1038/ng2048
Doetsch, F., Caille, I., Lim, D. A., Garcia-Verdugo, J. M., and Alvarez-Buylla, A. (1999). Subventricular zone astrocytes are neural stem cells in the adult mammalian brain. Cell 97, 703–716. doi: 10.1016/S0092-8674(00)80783-7
Dulken, B. W., Leeman, D. S., Boutet, S. C., Hebestreit, K., and Brunet, A. (2017). Single-Cell transcriptomic analysis defines heterogeneity and transcriptional dynamics in the adult neural stem cell lineage. Cell Rep. 18, 777–790. doi: 10.1016/j.celrep.2016.12.060
Duquenne, M., Folgueira, C., Bourouh, C., Millet, M., Silva, A., Clasadonte, J., et al. (2021). Leptin brain entry via a tanycytic LepR-EGFR shuttle controls lipid metabolism and pancreas function. Nat. Metab. 3:4325. doi: 10.1038/s42255-021-00432-5
Ehm, O., Goritz, C., Covic, M., Schaffner, I., Schwarz, T. J., Karaca, E., et al. (2010). RBPJkappa-dependent signaling is essential for long-term maintenance of neural stem cells in the adult hippocampus. J. Neurosci. 30, 13794–13807. doi: 10.1523/JNEUROSCI.1567-10.2010
Elks, C. E., Den Hoed, M., Zhao, J. H., Sharp, S. J., Wareham, N. J., Loos, R. J., et al. (2012). Variability in the heritability of body mass index: a systematic review and meta-regression. Front. Endocrinol. 3:29. doi: 10.3389/fendo.2012.00029
Engler, A., Rolando, C., Giachino, C., Saotome, I., Erni, A., Brien, C., et al. (2018). Notch2 signaling maintains NSC quiescence in the murine ventricular-subventricular zone. Cell Rep. 22, 992–1002. doi: 10.1016/j.celrep.2017.12.094
Evans, J., Sumners, C., Moore, J., Huentelman, M. J., Deng, J., Gelband, C. H., et al. (2002). Characterization of mitotic neurons derived from adult rat hypothalamus and brain stem. J. Neurophysiol. 87, 1076–1085. doi: 10.1152/jn.00088.2001
Faigle, R., and Song, H. (2013). Signaling mechanisms regulating adult neural stem cells and neurogenesis. Biochim. Biophys. Acta 1830, 2435–2448. doi: 10.1016/j.bbagen.2012.09.002
Frayling, T. M., Timpson, N. J., Weedon, M. N., Zeggini, E., Freathy, R. M., Lindgren, C. M., et al. (2007). A common variant in the FTO gene is associated with body mass index and predisposes to childhood and adult obesity. Science 316, 889–894. doi: 10.1126/science.1141634
Friedman, J. M. (2019). Leptin and the endocrine control of energy balance. Nat. Metab. 1, 754–764. doi: 10.1038/s42255-019-0095-y
Fu, A., Oberholtzer, S. M., Bagheri-Fam, S., Rastetter, R. H., Holdreith, C., Caceres, V. L., et al. (2018). Dynamic expression patterns of Irx3 and Irx5 during germline nest breakdown and primordial follicle formation promote follicle survival in mouse ovaries. PLoS Genet. 14:e1007488. doi: 10.1371/journal.pgen.1007488
Furube, E., Ishii, H., Nambu, Y., Kurganov, E., Nagaoka, S., Morita, M., et al. (2020). Neural stem cell phenotype of tanycyte-like ependymal cells in the circumventricular organs and central canal of adult mouse brain. Sci. Rep. 10:2826. doi: 10.1038/s41598-020-59629-5
Gaborit, N., Sakuma, R., Wylie, J. N., Kim, K. H., Zhang, S. S., Hui, C. C., et al. (2012). Cooperative and antagonistic roles for Irx3 and Irx5 in cardiac morphogenesis and postnatal physiology. Development 139, 4007–4019. doi: 10.1242/dev.081703
Ghosh, H. S. (2019). Adult neurogenesis and the promise of adult neural stem cells. J. Exp. Neurosci. 13:1179069519856876. doi: 10.1177/1179069519856876
Goldman, D. (2014). Muller glial cell reprogramming and retina regeneration. Nat. Rev. Neurosci. 15, 431–442. doi: 10.1038/nrn3723
Gomez-Skarmeta, J. L., Diez Del, Corral, R., De La Calle-Mustienes, E., Ferre-Marco, D., and Modolell, J. (1996). Araucan and caupolican, two members of the novel iroquois complex, encode homeoproteins that control proneural and vein-forming genes. Cell 85, 95–105. doi: 10.1016/S0092-8674(00)81085-5
Gomez-Skarmeta, J. L., Glavic, A., De La Calle-Mustienes, E., Modolell, J., and Mayor, R. (1998). Xiro, a Xenopus homolog of the Drosophila Iroquois complex genes, controls development at the neural plate. EMBO J. 17, 181–190. doi: 10.1093/emboj/17.1.181
Gomez-Skarmeta, J. L., and Modolell, J. (1996). araucan and caupolican provide a link between compartment subdivisions and patterning of sensory organs and veins in the Drosophila wing. Genes Dev. 10, 2935–2945. doi: 10.1101/gad.10.22.2935
Gomez-Skarmeta, J. L., and Modolell, J. (2002). Iroquois genes: genomic organization and function in vertebrate neural development. Curr. Opin. Genet. Dev. 12, 403–408. doi: 10.1016/S0959-437X(02)00317-9
Gonzalez-Muniesa, P., Martinez-Gonzalez, M. A., Hu, F. B., Despres, J. P., Matsuzawa, Y., Loos, R. J. F., et al. (2017). Obesity. Nat. Rev. Dis. Primers 3:17034. doi: 10.1038/nrdp.2017.34
Goodman, T., and Hajihosseini, M. K. (2015). Hypothalamic tanycytes-masters and servants of metabolic, neuroendocrine, and neurogenic functions. Front. Neurosci. 9:387. doi: 10.3389/fnins.2015.00387
Goodman, T., Nayar, S. G., Clare, S., Mikolajczak, M., Rice, R., Mansour, S., et al. (2020). Fibroblast growth factor 10 is a negative regulator of postnatal neurogenesis in the mouse hypothalamus. Development 147:180950. doi: 10.1242/dev.180950
Gotz, M., and Huttner, W. B. (2005). The cell biology of neurogenesis. Nat. Rev. Mol. Cell Biol. 6, 777–788. doi: 10.1038/nrm1739
Haan, N., Goodman, T., Najdi-Samiei, A., Stratford, C. M., Rice, R., El Agha, E., et al. (2013). Fgf10-expressing tanycytes add new neurons to the appetite/energy-balance regulating centers of the postnatal and adult hypothalamus. J. Neurosci. 33, 6170–6180. doi: 10.1523/JNEUROSCI.2437-12.2013
Harris, L., and Guillemot, F. (2019). HES1, two programs: promoting the quiescence and proliferation of adult neural stem cells. Genes Dev. 33, 479–481. doi: 10.1101/gad.325761.119
Henry, F. E., Sugino, K., Tozer, A., Branco, T., and Sternson, S. M. (2015). Cell type-specific transcriptomics of hypothalamic energy-sensing neuron responses to weight-loss. Elife 4:98. doi: 10.7554/eLife.09800
Herman, M. A., and Rosen, E. D. (2015). Making Biological Sense of GWAS Data: Lessons from the FTO Locus. Cell Metab. 22, 538–539. doi: 10.1016/j.cmet.2015.09.018
Hochgerner, H., Zeisel, A., Lonnerberg, P., and Linnarsson, S. (2018). Conserved properties of dentate gyrus neurogenesis across postnatal development revealed by single-cell RNA sequencing. Nat. Neurosci. 21, 290–299. doi: 10.1038/s41593-017-0056-2
Hourai, A., and Miyata, S. (2013). Neurogenesis in the circumventricular organs of adult mouse brains. J. Neurosci. Res. 91, 757–770. doi: 10.1002/jnr.23206
Imayoshi, I., Sakamoto, M., Yamaguchi, M., Mori, K., and Kageyama, R. (2010). Essential roles of Notch signaling in maintenance of neural stem cells in developing and adult brains. J. Neurosci. 30, 3489–3498. doi: 10.1523/JNEUROSCI.4987-09.2010
Ishii, Y., and Bouret, S. G. (2012). Embryonic birthdate of hypothalamic leptin-activated neurons in mice. Endocrinology 153, 3657–3667. doi: 10.1210/en.2012-1328
Jourdon, A., Gresset, A., Spassky, N., Charnay, P., Topilko, P., and Santos, R. (2016). Prss56, a novel marker of adult neurogenesis in the mouse brain. Brain Struct. Funct. 221, 4411–4427. doi: 10.1007/s00429-015-1171-z
Kim, D. W., Washington, P. W., Wang, Z. Q., Lin, S. H., Sun, C., Ismail, B. T., et al. (2020). The cellular and molecular landscape of hypothalamic patterning and differentiation from embryonic to late postnatal development. Nat. Commun. 11:4360. doi: 10.1038/s41467-020-18231-z
Kim, K. H., Rosen, A., Bruneau, B. G., Hui, C. C., and Backx, P. H. (2012). Iroquois homeodomain transcription factors in heart development and function. Circ. Res. 110, 1513–1524. doi: 10.1161/CIRCRESAHA.112.265041
Kim, K. H., Rosen, A., Hussein, S. M., Puviindran, V., Korogyi, A. S., Chiarello, C., et al. (2016). Irx3 is required for postnatal maturation of the mouse ventricular conduction system. Sci. Rep. 6:19197. doi: 10.1038/srep19197
Kokoeva, M. V., Yin, H., and Flier, J. S. (2005). Neurogenesis in the hypothalamus of adult mice: potential role in energy balance. Science 310, 679–683. doi: 10.1126/science.1115360
Kokoeva, M. V., Yin, H., and Flier, J. S. (2007). Evidence for constitutive neural cell proliferation in the adult murine hypothalamus. J. Comp. Neurol. 505, 209–220. doi: 10.1002/cne.21492
Laber, S., Forcisi, S., Bentley, L., Petzold, J., Moritz, F., Smirnov, K. S., et al. (2021). Linking the FTO obesity rs1421085 variant circuitry to cellular, metabolic, and organismal phenotypes in vivo. Sci. Adv. 7:108. doi: 10.1126/sciadv.abg0108
Langlet, F., Levin, B. E., Luquet, S., Mazzone, M., Messina, A., Dunn-Meynell, A. A., et al. (2013). Tanycytic VEGF-A boosts blood-hypothalamus barrier plasticity and access of metabolic signals to the arcuate nucleus in response to fasting. Cell Metab. 17, 607–617. doi: 10.1016/j.cmet.2013.03.004
Lee, D. A., Bedont, J. L., Pak, T., Wang, H., Song, J., Miranda-Angulo, A., et al. (2012). Tanycytes of the hypothalamic median eminence form a diet-responsive neurogenic niche. Nat. Neurosci. 15, 700–702. doi: 10.1038/nn.3079
Lee, D. A., Yoo, S., Pak, T., Salvatierra, J., Velarde, E., Aja, S., et al. (2014). Dietary and sex-specific factors regulate hypothalamic neurogenesis in young adult mice. Front. Neurosci. 8:157. doi: 10.3389/fnins.2014.00157
Leyns, L., Gomez-Skarmeta, J. L., and Dambly-Chaudiere, C. (1996). iroquois: a prepattern gene that controls the formation of bristles on the thorax of Drosophila. Mech. Dev. 59, 63–72. doi: 10.1016/0925-4773(96)00577-1
Li, D., Sakuma, R., Vakili, N. A., Mo, R., Puviindran, V., Deimling, S., et al. (2014). Formation of proximal and anterior limb skeleton requires early function of Irx3 and Irx5 and is negatively regulated by Shh signaling. Dev. Cell 29, 233–240. doi: 10.1016/j.devcel.2014.03.001
Li, J., Tang, Y., and Cai, D. (2012). IKKbeta/NF-kappaB disrupts adult hypothalamic neural stem cells to mediate a neurodegenerative mechanism of dietary obesity and pre-diabetes. Nat. Cell Biol. 14, 999–1012. doi: 10.1038/ncb2562
Lledo, P. M., Alonso, M., and Grubb, M. S. (2006). Adult neurogenesis and functional plasticity in neuronal circuits. Nat. Rev. Neurosci. 7, 179–193. doi: 10.1038/nrn1867
Lugert, S., Vogt, M., Tchorz, J. S., Muller, M., Giachino, C., and Taylor, V. (2012). Homeostatic neurogenesis in the adult hippocampus does not involve amplification of Ascl1(high) intermediate progenitors. Nat. Commun. 3:670. doi: 10.1038/ncomms1670
MacKay, H., and Abizaid, A. (2014). Embryonic development of the hypothalamic feeding circuitry: Transcriptional, nutritional, and hormonal influences. Mol. Metab. 3, 813–822. doi: 10.1016/j.molmet.2014.09.004
Magavi, S. S., Leavitt, B. R., and Macklis, J. D. (2000). Induction of neurogenesis in the neocortex of adult mice. Nature 405, 951–955. doi: 10.1038/35016083
Markakis, E. A., Palmer, T. D., Randolph-Moore, L., Rakic, P., and Gage, F. H. (2004). Novel neuronal phenotypes from neural progenitor cells. J. Neurosci. 24, 2886–2897. doi: 10.1523/JNEUROSCI.4161-03.2004
Matsuzaki, K., Katakura, M., Hara, T., Li, G., Hashimoto, M., and Shido, O. (2009). Proliferation of neuronal progenitor cells and neuronal differentiation in the hypothalamus are enhanced in heat-acclimated rats. Pflugers Arch. 458, 661–673. doi: 10.1007/s00424-009-0654-2
McNay, D. E., Briancon, N., Kokoeva, M. V., Maratos-Flier, E., and Flier, J. S. (2012). Remodeling of the arcuate nucleus energy-balance circuit is inhibited in obese mice. J. Clin. Invest. 122, 142–152. doi: 10.1172/JCI43134
McNay, D. E., Pelling, M., Claxton, S., Guillemot, F., and Ang, S. L. (2006). Mash1 is required for generic and subtype differentiation of hypothalamic neuroendocrine cells. Mol. Endocrinol. 20, 1623–1632. doi: 10.1210/me.2005-0518
Migaud, M., Batailler, M., Segura, S., Duittoz, A., Franceschini, I., and Pillon, D. (2010). Emerging new sites for adult neurogenesis in the mammalian brain: a comparative study between the hypothalamus and the classical neurogenic zones. Eur. J. Neurosci. 32, 2042–2052. doi: 10.1111/j.1460-9568.2010.07521.x
Ming, G. L., and Song, H. (2011). Adult neurogenesis in the mammalian brain: significant answers and significant questions. Neuron 70, 687–702. doi: 10.1016/j.neuron.2011.05.001
Miranda-Angulo, A. L., Byerly, M. S., Mesa, J., Wang, H., and Blackshaw, S. (2014). Rax regulates hypothalamic tanycyte differentiation and barrier function in mice. J. Comp. Neurol. 522, 876–899. doi: 10.1002/cne.23451
Morales, A. V., and Mira, H. (2019). Adult Neural Stem Cells: Born to Last. Front. Cell Dev. Biol. 7:96. doi: 10.3389/fcell.2019.00096
Mu, W., Li, S., Xu, J., Guo, X., Wu, H., Chen, Z., et al. (2021). Hypothalamic Rax+ tanycytes contribute to tissue repair and tumorigenesis upon oncogene activation in mice. Nat. Commun. 12:2288. doi: 10.1038/s41467-021-22640-z
Mullier, A., Bouret, S. G., Prevot, V., and Dehouck, B. (2010). Differential distribution of tight junction proteins suggests a role for tanycytes in blood-hypothalamus barrier regulation in the adult mouse brain. J. Comp. Neurol. 518, 943–962. doi: 10.1002/cne.22273
Myers, M. G. Jr., Affinati, A. H., Richardson, N., and Schwartz, M. W. (2021). Central nervous system regulation of organismal energy and glucose homeostasis. Nat. Metab. 3, 737–750. doi: 10.1038/s42255-021-00408-5
Niwa, A., Nishibori, M., Hamasaki, S., Kobori, T., Liu, K., Wake, H., et al. (2016). Voluntary exercise induces neurogenesis in the hypothalamus and ependymal lining of the third ventricle. Brain Struct. Funct. 221, 1653–1666. doi: 10.1007/s00429-015-0995-x
Obernier, K., and Alvarez-Buylla, A. (2019). Neural stem cells: origin, heterogeneity and regulation in the adult mammalian brain. Development 146:59. doi: 10.1242/dev.156059
Padilla, S. L., Carmody, J. S., and Zeltser, L. M. (2010). Pomc-expressing progenitors give rise to antagonistic neuronal populations in hypothalamic feeding circuits. Nat. Med. 16, 403–405. doi: 10.1038/nm.2126
Pak, T., Yoo, S., Miranda-Angulo, A. L., Wang, H., and Blackshaw, S. (2014). Rax-CreERT2 knock-in mice: a tool for selective and conditional gene deletion in progenitor cells and radial glia of the retina and hypothalamus. PLoS One 9:e90381. doi: 10.1371/journal.pone.0090381
Pearson, C. A., and Placzek, M. (2013). Development of the medial hypothalamus: forming a functional hypothalamic-neurohypophyseal interface. Curr. Top Dev. Biol. 106, 49–88. doi: 10.1016/B978-0-12-416021-7.00002-X
Pencea, V., Bingaman, K. D., Wiegand, S. J., and Luskin, M. B. (2001). Infusion of brain-derived neurotrophic factor into the lateral ventricle of the adult rat leads to new neurons in the parenchyma of the striatum, septum, thalamus, and hypothalamus. J. Neurosci. 21, 6706–6717. doi: 10.1523/JNEUROSCI.21-17-06706.2001
Perez-Martin, M., Cifuentes, M., Grondona, J. M., Lopez-Avalos, M. D., Gomez-Pinedo, U., Garcia-Verdugo, J. M., et al. (2010). IGF-I stimulates neurogenesis in the hypothalamus of adult rats. Eur. J. Neurosci. 31, 1533–1548. doi: 10.1111/j.1460-9568.2010.07220.x
Peters, T., Dildrop, R., Ausmeier, K., and Ruther, U. (2000). Organization of mouse Iroquois homeobox genes in two clusters suggests a conserved regulation and function in vertebrate development. Genome Res. 10, 1453–1462. doi: 10.1101/gr.144100
Petrova, R., Garcia, A. D., and Joyner, A. L. (2013). Titration of GLI3 repressor activity by sonic hedgehog signaling is critical for maintaining multiple adult neural stem cell and astrocyte functions. J. Neurosci. 33, 17490–17505. doi: 10.1523/JNEUROSCI.2042-13.2013
Pierce, A. A., and Xu, A. W. (2010). De novo neurogenesis in adult hypothalamus as a compensatory mechanism to regulate energy balance. J. Neurosci. 30, 723–730. doi: 10.1523/JNEUROSCI.2479-09.2010
Qi, Y., Cai, J., Wu, Y., Wu, R., Lee, J., Fu, H., et al. (2001). Control of oligodendrocyte differentiation by the Nkx2.2 homeodomain transcription factor. Development 128, 2723–2733. doi: 10.1242/dev.128.14.2723
Rizzoti, K., and Lovell-Badge, R. (2017). Pivotal role of median eminence tanycytes for hypothalamic function and neurogenesis. Mol. Cell Endocrinol. 445, 7–13. doi: 10.1016/j.mce.2016.08.020
Robins, S. C., Stewart, I., Mcnay, D. E., Taylor, V., Giachino, C., Goetz, M., et al. (2013). alpha-Tanycytes of the adult hypothalamic third ventricle include distinct populations of FGF-responsive neural progenitors. Nat. Commun. 4:2049. doi: 10.1038/ncomms3049
Rodriguez, E. M., Blazquez, J. L., Pastor, F. E., Pelaez, B., Pena, P., Peruzzo, B., et al. (2005). Hypothalamic tanycytes: a key component of brain-endocrine interaction. Int. Rev. Cytol. 247, 89–164. doi: 10.1016/S0074-7696(05)47003-5
Romanov, R. A., Tretiakov, E. O., Kastriti, M. E., Zupancic, M., Haring, M., Korchynska, S., et al. (2020). Molecular design of hypothalamus development. Nature 582, 246–252. doi: 10.1038/s41586-020-2266-0
Salvatierra, J., Lee, D. A., Zibetti, C., Duran-Moreno, M., Yoo, S., Newman, E. A., et al. (2014). The LIM homeodomain factor Lhx2 is required for hypothalamic tanycyte specification and differentiation. J. Neurosci. 34, 16809–16820. doi: 10.1523/JNEUROSCI.1711-14.2014
Shah, P. T., Stratton, J. A., Stykel, M. G., Abbasi, S., Sharma, S., Mayr, K. A., et al. (2018). Single-Cell Transcriptomics and fate mapping of ependymal cells reveals an absence of neural stem cell function. Cell 173:e1049. doi: 10.1016/j.cell.2018.03.063
Shimogori, T., Lee, D. A., Miranda-Angulo, A., Yang, Y., Wang, H., Jiang, L., et al. (2010). A genomic atlas of mouse hypothalamic development. Nat. Neurosci. 13, 767–775. doi: 10.1038/nn.2545
Shin, J., Berg, D. A., Zhu, Y., Shin, J. Y., Song, J., Bonaguidi, M. A., et al. (2015). Single-Cell RNA-Seq with waterfall reveals molecular cascades underlying adult neurogenesis. Cell Stem Cell 17, 360–372. doi: 10.1016/j.stem.2015.07.013
Smemo, S., Tena, J. J., Kim, K. H., Gamazon, E. R., Sakabe, N. J., Gomez-Marin, C., et al. (2014). Obesity-associated variants within FTO form long-range functional connections with IRX3. Nature 507, 371–375. doi: 10.1038/nature13138
Sobreira, D. R., Joslin, A. C., Zhang, Q., Williamson, I., Hansen, G. T., Farris, K. M., et al. (2021). Extensive pleiotropism and allelic heterogeneity mediate metabolic effects of IRX3 and IRX5. Science 372, 1085–1091. doi: 10.1126/science.abf1008
Son, J. E., Dou, Z., Kim, K. H., Wanggou, S., Cha, V. S. B., Mo, R., et al. (2021a). Irx3 and Irx5 in Ins2-Cre(+) cells regulate hypothalamic postnatal neurogenesis and leptin response. Nat. Metab. 3, 701–713. doi: 10.1038/s42255-021-00382-y
Son, J. E., Kim, K.-H., and Hui, C.-C. (2021b). Deficiency of Irx5 protects mice from diet-induced obesity and associated metabolic abnormalities. BioRxiv 2021:462004. doi: 10.1101/2021.09.27.462004
Speakman, J. R., Rance, K. A., and Johnstone, A. M. (2008). Polymorphisms of the FTO gene are associated with variation in energy intake, but not energy expenditure. Obesity 16, 1961–1965. doi: 10.1038/oby.2008.318
Stuart, T., Butler, A., Hoffman, P., Hafemeister, C., Papalexi, E., Mauck, W. M. III, et al. (2019). Comprehensive integration of single-cell data. Cell 177:e1821. doi: 10.1016/j.cell.2019.05.031
Sueda, R., Imayoshi, I., Harima, Y., and Kageyama, R. (2019). High Hes1 expression and resultant Ascl1 suppression regulate quiescent vs. active neural stem cells in the adult mouse brain. Genes Dev. 33, 511–523. doi: 10.1101/gad.323196.118
Tan, Z., Kong, M., Wen, S., Tsang, K. Y., Niu, B., Hartmann, C., et al. (2020). IRX3 and IRX5 Inhibit Adipogenic differentiation of hypertrophic chondrocytes and promote osteogenesis. J. Bone Miner. Res. 35, 2444–2457. doi: 10.1002/jbmr.4132
Tanofsky-Kraff, M., Han, J. C., Anandalingam, K., Shomaker, L. B., Columbo, K. M., Wolkoff, L. E., et al. (2009). The FTO gene rs9939609 obesity-risk allele and loss of control over eating. Am. J. Clin. Nutr. 90, 1483–1488. doi: 10.3945/ajcn.2009.28439
Tao, H., Lambert, J. P., Yung, T. M., Zhu, M., Hahn, N. A., Li, D., et al. (2020). IRX3/5 regulate mitotic chromatid segregation and limb bud shape. Development 147:dev180042. doi: 10.1242/dev.180042
Taverna, E., Gotz, M., and Huttner, W. B. (2014). The cell biology of neurogenesis: toward an understanding of the development and evolution of the neocortex. Annu. Rev. Cell Dev. Biol. 30, 465–502. doi: 10.1146/annurev-cellbio-101011-155801
Timper, K., and Bruning, J. C. (2017). Hypothalamic circuits regulating appetite and energy homeostasis: pathways to obesity. Dis. Model Mech. 10, 679–689. doi: 10.1242/dmm.026609
Tung, Y. C., Yeo, G. S., O’rahilly, S., and Coll, A. P. (2014). Obesity and FTO: Changing Focus at a Complex Locus. Cell Metab. 20, 710–718. doi: 10.1016/j.cmet.2014.09.010
Urban, N., Blomfield, I. M., and Guillemot, F. (2019). Quiescence of adult mammalian neural stem cells: a highly regulated rest. Neuron 104, 834–848. doi: 10.1016/j.neuron.2019.09.026
Urban, N., and Guillemot, F. (2014). Neurogenesis in the embryonic and adult brain: same regulators, different roles. Front. Cell Neurosci. 8:396. doi: 10.3389/fncel.2014.00396
Urban, N., Van Den Berg, D. L., Forget, A., Andersen, J., Demmers, J. A., Hunt, C., et al. (2016). Return to quiescence of mouse neural stem cells by degradation of a proactivation protein. Science 353, 292–295. doi: 10.1126/science.aaf4802
van der Klaauw, A. A., and Farooqi, I. S. (2015). The hunger genes: pathways to obesity. Cell 161, 119–132. doi: 10.1016/j.cell.2015.03.008
Williams, E. P., Mesidor, M., Winters, K., Dubbert, P. M., and Wyatt, S. B. (2015). Overweight and Obesity: Prevalence. Consequences, and Causes of a Growing Public Health Problem. Curr. Obes. Rep. 4, 363–370. doi: 10.1007/s13679-015-0169-4
Wolf, F. A., Hamey, F. K., Plass, M., Solana, J., Dahlin, J. S., Gottgens, B., et al. (2019). PAGA: graph abstraction reconciles clustering with trajectory inference through a topology preserving map of single cells. Genome Biol. 20:59. doi: 10.1186/s13059-019-1663-x
Xu, Y., Tamamaki, N., Noda, T., Kimura, K., Itokazu, Y., Matsumoto, N., et al. (2005). Neurogenesis in the ependymal layer of the adult rat 3rd ventricle. Exp. Neurol. 192, 251–264. doi: 10.1016/j.expneurol.2004.12.021
Yao, J., Wu, D., Zhang, C., Yan, T., Zhao, Y., Shen, H., et al. (2021). Macrophage IRX3 promotes diet-induced obesity and metabolic inflammation. Nat. Immunol. 22:1268–1279 doi: 10.1038/s41590-021-01023-y
Yoo, S., and Blackshaw, S. (2018). Regulation and function of neurogenesis in the adult mammalian hypothalamus. Prog. Neurobiol. 170, 53–66. doi: 10.1016/j.pneurobio.2018.04.001
Yoo, S., Cha, D., Kim, S., Jiang, L., Cooke, P., Adebesin, M., et al. (2020). Tanycyte ablation in the arcuate nucleus and median eminence increases obesity susceptibility by increasing body fat content in male mice. Glia 68, 1987–2000. doi: 10.1002/glia.23817
Yoo, S., Kim, J., Lyu, P., Hoang, T. V., Ma, A., Trinh, V., et al. (2021). Control of neurogenic competence in mammalian hypothalamic tanycytes. Sci. Adv. 7:eabg3777. doi: 10.1126/sciadv.abg3777
Yuzwa, S. A., Borrett, M. J., Innes, B. T., Voronova, A., Ketela, T., Kaplan, D. R., et al. (2017). Developmental emergence of adult neural stem cells as revealed by single-cell transcriptional profiling. Cell Rep. 21, 3970–3986. doi: 10.1016/j.celrep.2017.12.017
Zhang, S. S., Kim, K. H., Rosen, A., Smyth, J. W., Sakuma, R., Delgado-Olguin, P., et al. (2011). Iroquois homeobox gene 3 establishes fast conduction in the cardiac His-Purkinje network. Proc. Natl. Acad. Sci. USA 108, 13576–13581. doi: 10.1073/pnas.1106911108
Zhang, Y. H., Xu, M., Shi, X., Sun, X. L., Mu, W., Wu, H., et al. (2021). Cascade diversification directs generation of neuronal diversity in the hypothalamus. Cell Stem Cell 28:e1488. doi: 10.1016/j.stem.2021.03.020
Zhou, Q., Choi, G., and Anderson, D. J. (2001). The bHLH transcription factor Olig2 promotes oligodendrocyte differentiation in collaboration with Nkx2.2. Neuron 31, 791–807. doi: 10.1016/S0896-6273(01)00414-7
Keywords: FTO (Fat Mass and Obesity-Associated) Gene, obesity, metabolic regulation, neural stem cell (NSC), tanycyte, neurogenesis, IRX3 gene, IRX5 gene
Citation: Dou Z, Son JE and Hui C-c (2021) Irx3 and Irx5 - Novel Regulatory Factors of Postnatal Hypothalamic Neurogenesis. Front. Neurosci. 15:763856. doi: 10.3389/fnins.2021.763856
Received: 24 August 2021; Accepted: 07 October 2021;
Published: 02 November 2021.
Edited by:
Qing-Feng Wu, Institute of Genetics and Developmental Biology, Chinese Academy of Sciences (CAS), ChinaReviewed by:
Daniel A. Berg, University of Aberdeen, United KingdomJong-Woo Sohn, Korea Advanced Institute of Science and Technology, South Korea
Copyright © 2021 Dou, Son and Hui. This is an open-access article distributed under the terms of the Creative Commons Attribution License (CC BY). The use, distribution or reproduction in other forums is permitted, provided the original author(s) and the copyright owner(s) are credited and that the original publication in this journal is cited, in accordance with accepted academic practice. No use, distribution or reproduction is permitted which does not comply with these terms.
*Correspondence: Joe Eun Son, am9lZXVuLnNvbkBzaWNra2lkcy5jYQ==; Chi-chung Hui, Y2NodWlAc2lja2tpZHMuY2E=