- 1Henan Key Laboratory of Neural Regeneration and Repairment, Life Science Research Center, The First Affiliated Hospital of Xinxiang Medical University, Weihui, China
- 2Department of Thoracic Surgery, The First Affiliated Hospital of Xinxiang Medical University, Weihui, China
Background: The carotid body (CB) plays a critical role in oxygen sensing; however, the role of glutamatergic signaling in the CB response to hypoxia remains uncertain. We previously found that functional multiple glutamate transporters and inotropic glutamate receptors (iGluRs) are expressed in the CB. The aim of this present research is to investigate the expression of group I metabotropic glutamate receptors (mGluRs) (mGluR1 and 5) in the CB and its physiological function in rat CB response to acute hypoxia.
Methods: RT-PCR and immunostaining were conducted to examine the mRNA and protein expression of group I mGluRs in the human and rat CB. Immunofluorescence staining was performed to examine the cellular localization of mGluR1 in the rat CB. In vitro carotid sinus nerve (CSN) discharge recording was performed to detect the physiological function of mGluR1 in CB response to acute hypoxia.
Results: We found that (1) mRNAs of mGluR1 and 5 were both expressed in the human and rat CB. (2) mGluR1 protein rather than mGluR5 protein was present in rat CB. (3) mGluR1 was distributed in type I cells of rat CB. (4) Activation of mGluR1 inhibited the hypoxia-induced enhancement of CSN activity (CSNA), as well as prolonged the latency time of CB response to hypoxia. (5) The inhibitory effect of mGluR1 activation on rat CB response to hypoxia could be blocked by GABAB receptor antagonist.
Conclusion: Our findings reveal that mGluR1 in CB plays a presynaptic feedback inhibition on rat CB response to hypoxia.
Introduction
Oxygen sensing mediated by central and peripheral chemoreceptors is essential for survival of multicellular organisms. Carotid body (CB), a highly vascular tissue, is mainly a peripheral chemoreceptor located in the adventitia of the bifurcation of the common carotid artery. CB is usually organized in glomeruli clusters, which are composed of four to eight neuron-like glomus cells (type I cells) and one to two glia-like sustentacular cells (type II cells) (Ortega-Sáenz and López-Barneo, 2020). Type I glomus cells are tyrosine hydroxylase (TH)-positive O2 sensing cells, and their cytoplasm is rich in abundant synaptic vesicles that contain neurotransmitters and neuromodulators. Type II sustentacular cells that encase clusters of type I cells are glial fibrillary acidic protein (GFAP) positive cells and can be transformed into type I glomus cells under certain conditions (Platero-Luengo et al., 2014).
Evidence supports that the type I glomus cells, as presynaptic neuroendocrine cells, establish synapses with afferent sensory fibers of carotid sinus nerve (CSN) (as postsynaptic nerve endings) (Ortega-Sáenz and López-Barneo, 2020). There is general acceptance that transduction of the hypoxic stimulus in the CB involves closure of O2-sensitive K+ channels, which leads to membrane depolarization of type I cells. This process induces Ca2+-dependent release of neurotransmitters from type I cells; subsequently, the neurotransmitters act on specific postsynaptic receptors on CSN afferent fibers (Chang et al., 2015). It is established that these neurotransmitters [such as ATP, GABA, and acetylcholine (ACh)] in type I cells regulate CB oxygen sensing not only by acting on the corresponding ionotropic receptors of chemosensory nerve endings (Zhang et al., 2000, 2009; Rong et al., 2003; Varas et al., 2003; Alcayaga et al., 2007; Sacramento et al., 2019) but also by acting on non-ionotropic receptors of chemosensory nerve endings (such as ATP and ACh) (Alcayaga et al., 2000; Shirahata et al., 2004) or non-ionotropic receptors of type I cells themselves (such as GABA and ATP) (Fearon et al., 2003; Tse et al., 2012). Studies show that neurotransmitters acting on non-ionotropic receptors of type I cells provide feedback mechanisms to regulate the CB hypoxia response. Xu et al. (2005) found that ATP exert strong negative feedback regulation on CB hypoxia response by restricting the increase in [Ca2+]i during hypoxia or acting on P2Y receptors on type I cells to close the background ion channels. GABA was also demonstrated to play a negative feedback role by acting on GABAB receptors located on type I cells to inhibit the hypoxia-induced release of ATP and ACh (Fearon et al., 2003). Although evidence supports numerous mechanisms by which hypoxia-triggered depolarization of type I cells leads to excitation of the CSN, the glutamatergic neurotransmitter system its expression in CB as well as its effect on the hypoxia-triggered excitation of the CSN has not been systematically explored.
In the central nervous system, glutamate is the major neurotransmitter. In the process of glutamatergic synaptic transmission, once an action potential depolarizes the presynaptic membrane, glutamate accumulated in presynaptic transmitter vesicles by the vesicular glutamate transporters (VGluTs) is released into the synaptic cleft by exocytosis and then acts on the corresponding postsynaptic receptors to transmit neuronal signaling. Excitatory amino acid transporters (EAATs) on adjacent glial cells scavenge glutamate from the synaptic cleft to terminate glutamate’s functions (Zhou and Danbolt, 2014; Reiner and Levitz, 2018). Glutamate receptors are divided into two families: ionotropic glutamate receptors (iGluRs) and metabotropic glutamate receptors (mGluRs) (Reiner and Levitz, 2018). Playing an important role in synaptic transmission/plasticity, iGluRs are ligand-gated ion channels, which once activated leads to a rapid ion flux, and are further divided into three main subfamilies: alpha-amino-3-hydroxy-5-methyl-4-isoxazole propionic acid receptors (AMPARs), N-methyl-D-aspartate receptors (NMDARs), and kainate receptors (KARs) (Traynelis et al., 2010; Reiner and Levitz, 2018). mGluRs belong to the G protein-coupled receptor family and are divided into group I mGluRs (GluR1/5), group II mGluRs (mGluR2/3) and group III mGluRs (mGluR4, mGluR6–8) (Niswender and Conn, 2010; Reiner and Levitz, 2018). mGluRs activate the G protein-coupled signal cascades leading to changes in synaptic function/excitability by inhibiting the presynaptic transmitter release (Sladeczek et al., 1993; Gereau and Conn, 1995) or by regulating the postsynaptic response (Sheng et al., 2017; Jin et al., 2018).
It is established that glutamatergic signaling functions not only in the central nervous system but also in the peripheral nervous system (Tremolizzo et al., 2012; Chen and Kukley, 2020). Multiple VGluTs, EAATs (Li et al., 2020), and iGluRs (Liu et al., 2009, 2018) have been demonstrated to exist in the rat CB. We found that exposure of rats to cyclic intermittent hypoxia (CIH) alters the expression of some of these molecules. iGluRs in the CB are functional because blockading with the NMDAR antagonist MK801 reduces endothelin-induced CSN activity (CSNA) (Liu et al., 2018). These data suggested that iGluR-mediated glutamatergic signaling might occur in the CB, but the information regarding the mGluRs expression in the CB and its physiological function in the CB reponse to hypoxia is unknown.
We previously demonstrated that group I mGluRs exist in human and rat adrenal glands and that mGluR1 is involved in hypoxia-induced upregulation of TH in cultured rat adrenal medulla (Fan et al., 2020). Both adrenal medulla and CB originate from the neural crest and function as peripheral chemoreceptors in acute oxygen sensing (Gao et al., 2019). Taken together, these observations led us to speculate that group I mGluRs might be present in the CB and are involved in the carotid chemoreflex. In this study, we examined the expression of group I mGluRs in the human and rat CB and its cellular localization in the rat CB by RT-PCR and immunostaining. Moreover, we investigated the physiological function of mGluR1 in rat CB response to acute hypoxia.
Materials and Methods
Human Carotid Body
Human surgical specimens were obtained from The First Affiliated Hospital of Xinxiang Medical University with consent from patients (grant approval number: 2016008). Human CB specimen was obtained from a patient with left CB paraganglioma, and human cerebral cortex tissue was obtained from a patient with craniocerebral trauma. The protocol related to human subjects was conducted in accordance with the declaration of Helsinki and approved by the Human Ethics Committee of The First Affiliated Hospital of Xinxiang Medical University.
Animals and Rat Carotid Body Harvest
Adult male Sprague-Dawley (SD) rats were ordered from Beijing Vital River Laboratory Animal Technology Co., Ltd. (Beijing, China). The age of the rats was 8 weeks, and the body weight was 240–250 g at entry into the protocol. Rats were housed under room temperature and standard humidity (50 ± 5%) with a 12-h day/night cycle with laboratory chow and water ad libitum. All procedures performed in this study were in accordance with national animal research regulations, and all animal experimental protocols were approved by the Institutional Animal Ethics Committee at The First Affiliated Hospital of Xinxiang Medical University.
After the SD rat was anesthetized by inhalation of 2% isoflurane (RWD Life Science, Shenzhen, China), the rat was decapitated and the bilateral carotid bifurcations were rapidly removed and placed into 95% O2–5% CO2 saturated ice-cold phosphate-buffered saline (PBS). The CBs were dissected (within 4 min) and immediately soaked in RNAlater (Qiagen, Valencia, CA, United States), then stored at −80°C until analyzed.
RNA Extraction and Reverse Transcription-PCR
Total RNA was extracted from human CB specimens and rat CBs (total 16 CBs pooled from 8 rats) using TRIzol reagent (Thermo Fisher Scientific, Waltham, MA, United States) according to the manufacturer’s protocol. After removal of gDNA, 500 ng of total RNA was reversely transcribed into cDNA by using QuantiTect Reverse Transcription Kit (Qiagen, Valencia, CA, United States), in accordance with the manufacturer’s protocol. The RNA without reverse transcriptase was used in reverse transcription (RT) reaction to obtain a negative cDNA control. The mRNA expression level was detected by semi-quantitative PCR in a Veriti® 96-well Thermal Cycler (Applied Biosystems, Foster City, CA, United States) using HotStarTaq® Master Mix Kit (Qiagen, Valencia, CA, United States). Two microliters of cDNA samples was mixed with 10 μl of HotStarTaq Master Mix and 1 μl of gene-specific primer pairs in a final reaction volume of 20 μl to amplify the target gene. The PCR reactive conditions included an initial step at 95°C for 10 min, followed by 40 cycles at 94°C for 50 s, proper annealing temperature for 50 s, extension at 72°C for 1 min, and then ending at 72°C for 10 min. An equal volume of the negative cDNA control was used in the PCR reaction as negative PCR control. The PCR product was loaded into 1.2% agarose gel. A total of three technical replicates were conducted for each target gene. All primers were exon spanning and designed using Primer-BLAST.1 Details of all primers are listed in Tables 1, 2.
Immuno-Staining
After the rats were fixed with 4% neutral buffered formalin by cardiac perfusion, the carotid bifurcations containing the CB were removed and embedded in paraffin. The paraffin-embedded carotid bifurcations were cut by ShandonTM FinesseTM 325 Microtomes (Thermo Fisher Scientific, Waltham, MA, United States) to prepare CB sections (3 μm).
For immunohistochemistry staining, after being deparaffinized, hydrated, and antigen retrieved, the CB sections were blocked with 10% goat serum at room temperature for 1 h, then incubated at 4°C overnight with the following primary antibodies: rabbit anti-mGluR1 (1:300, Abcam, Cambridge, United Kingdom, Cat No. ab82211) and anti-mGluR5 (1:100, Abcam, Cambridge, United Kingdom, Cat No. ab76316). After being washed three times in PBS, sections were incubated with GTVisionTM III Detection System Mouse & Rabbit Kit (HRP/DAB) (GeneTech, Shanghai, China), according to the manufacturer’s instruction, to yield a brown crystalline antigen–antibody complex product. The paraffin-embedded rat brain sections were incubated with primary antibodies as the positive control. The negative staining control was prepared by replacing the primary antibody to PBS containing 5% normal goat serum. The staining was examined through the Nikon H600L microscope and photographed with Nikon digital camera DS-Fi1c (Nikon, Tokyo, Japan).
For double immunofluorescence staining, after blocking with 10% goat serum, the CB sections were exposed to a mixture of two primary antibodies as follows: rabbit anti-mGluR1 (1:100, CST, Danvers, MA, United States, Cat No. 12551) with mouse anti-TH (1:2,000, Sigma, St. Louis, MO, United States, Cat No. T2928); rabbit anti-mGluR1 (1:100, CST, Danvers, MA, United States, Cat No. 12551) with mouse anti-GFAP (1:200, CST, Danvers, MA, United States, Cat No. 3670). After washing with PBS, the sections were incubated with a mixture of second antibody of Alexa Fluor 488 goat anti-rabbit IgG (1:400, CST, Danvers, MA, United States, Cat No. 4412) with Alexa Fluor 555 goat anti-mouse IgG (1:400, CST, Danvers, MA, United States, Cat No. 4409). The negative staining control was prepared by omitting the primary antibody. The staining was examined and photographed with the Nikon C2 confocal microscope (Nikon, Tokyo, Japan).
In vitro Carotid Sinus Nerve Discharge Recording
The CB response to hypoxia was recorded by in vitro CSN discharge recording similar to the method described in a previous report (Rong et al., 2003). Briefly, SD rat was anesthetized by inhalation of 2% isoflurane, and then decapitated. The bilateral carotid bifurcations containing the CB were then rapidly removed and placed into 95% O2–5% CO2 saturated ice-cold Kreb’s solution (in mM: NaCl 113, KCl 5.9, NaH2PO4 1.2, MgSO4 1.2, NaHCO3 25, glucose 11.5, CaCl2 2, and pH 7.4). The sinus nerve attached to the CB was dissected under a microscope, and the bifurcation was placed into a recording chamber (3 ml) perfused with 95% O2–5% CO2 saturated warm Kreb’s solution (15 ml/min) with the chamber temperature being kept at 33°C. The CSN discharge was recorded using a suction electrode (Warner Instruments, Hamden, CT, United States). The signaling was amplified (1,000×) and filtered (100–1,000 Hz) by a DP-311 differential amplifier (Warner Instruments, Hamden, CT, United States), then collected by PowerLab 8/35 (AD Instruments, Bella Vista, NSW, Australia) and analyzed and integrated by LabChart 8 software (AD Instruments, Bella Vista, NSW, Australia). To detect the CB response to hypoxia, the CB was exposed to 5% O2–5% CO2–90% N2 saturated Kreb’s solution for a period of 90 s at an interval of 15 min. Hypoxia-induced CSNA was normalized by integrated CSNA under hypoxia divided by the integrated baseline CSNA under 95% O2–5% CO2 condition. Group I receptor agonist (S)-3,5-dihydroxyphenylglycine (DHPG, 40 μM) (Sigma, St. Louis, MO, United States), mGluR1 antagonist (3,4-dihydro-2H-pyrano[2,3-b]quinolin-7-yl)-(cis-4-methoxycyclohexyl)-methanone (JNJ16259685, 20 μM) (Santa Cruz Biotechnology, Dallas, TX, United States), and DHPG (40 μM) combined with JNJ or DHPG combined with GABAB receptor antagonist CGP52432 (10 μM) (Selleck Chemicals, Houston, TX, United States) were applied by switching the basal perfusate to 95% O2–5% CO2 saturated Kreb’s solution containing the compound for 10 min before switching to 5% O2–5% CO2–90% N2 saturated Kreb’s solution containing the compound.
Statistical Analysis
The Shapiro–Wilk normality tests of all data could be conducted with the SPSS statistical software. All data were normally distributed, and the normality test results were shown in the online supplementary tables. The data were presented as mean ± SEM. Statistical evaluation was conducted by one-way ANOVA with repeated measures. p < 0.05 was considered significant.
Results
mRNA Expression of Group I Metabotropic Glutamate Receptors in Human and Rat Carotid Body
Reverse transcription-PCR was performed to detect the mRNA expression of group I mGluRs (GRM1 and GRM5) in human and rat CB. As shown in Figure 1, PCR-amplified transcripts corresponding to the predicted sizes of GRM1 and GRM5 were detected in human (Figure 1A) and rat CB (Figure 1B) as well as brain cerebral cortex, which was used as positive control. These results indicate that both human and rat CB express all group I mGluR mRNAs.
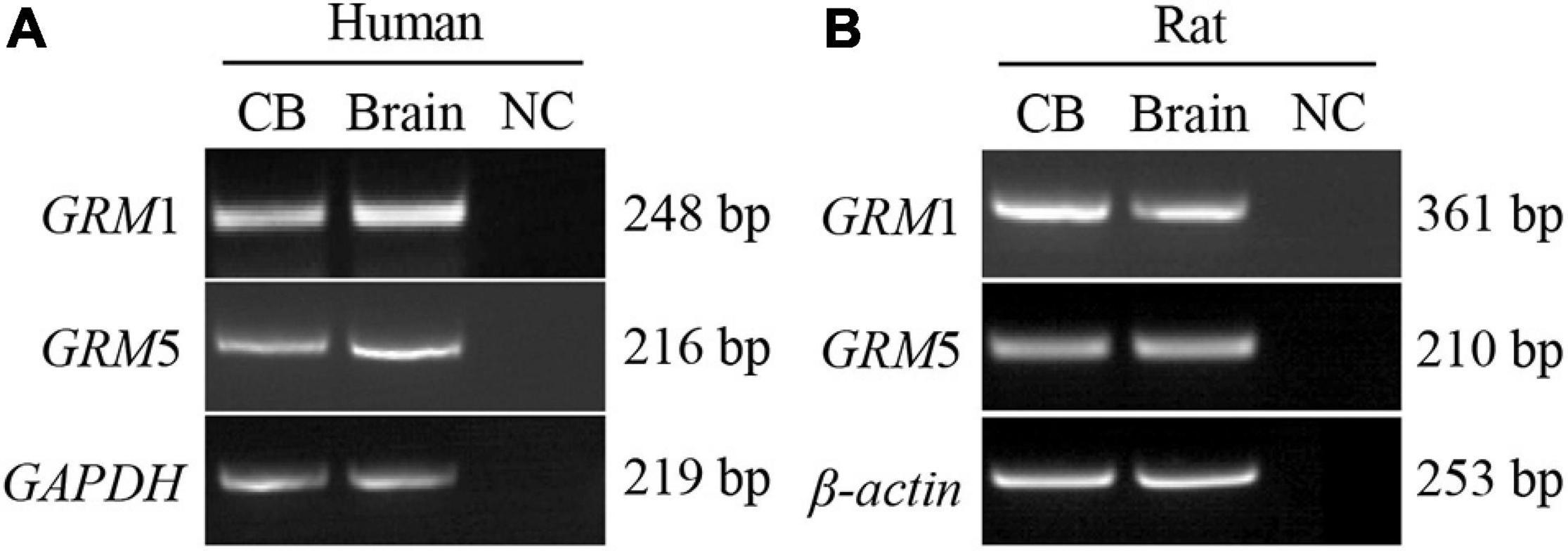
Figure 1. mRNA expression of group I mGluRs in human and rat CB. (A,B) Representative agarose gel images show the RT-PCR results of group I mGluR mRNA expression in human and rat CB. CB, left lanes in (A,B). RNA extracted from the human brain cerebral cortex was used as positive control (brain, middle lanes in A,B). An equal volume of the negative cDNA control was used in the PCR as PCR negative control (NC; right lanes in A,B). GRM, glutamate metabotropic receptor.
Protein Expression of Group I Metabotropic Glutamate Receptors in Rat Carotid Body
To detect the protein expression of group I mGluRs (mGluR1 and mGluR5) in rat CB, immunohistochemistry was performed. Representative photomicrographs in Figures 2A1,A2 showed that mGluR1-positive immunoreaction was ubiquitously detected in the clustering glomeruli of rat CB. However, mGluR5-specific immunostaining was not observed in rat CB (Figures 2B1,B2). The representative negative controls (NCs) in the absence of the anti-mGluR1 or mGluR5 antibody did not yield specific staining in rat CB (Figures 2C1,C2). Rat brain sections with intense immunoreactive mGluR1 (Figure 2D) or mGluR5 (Figure 2E) were used as positive controls. These results indicate that mGluR1 protein rather than mGluR5 protein is present in rat CB.
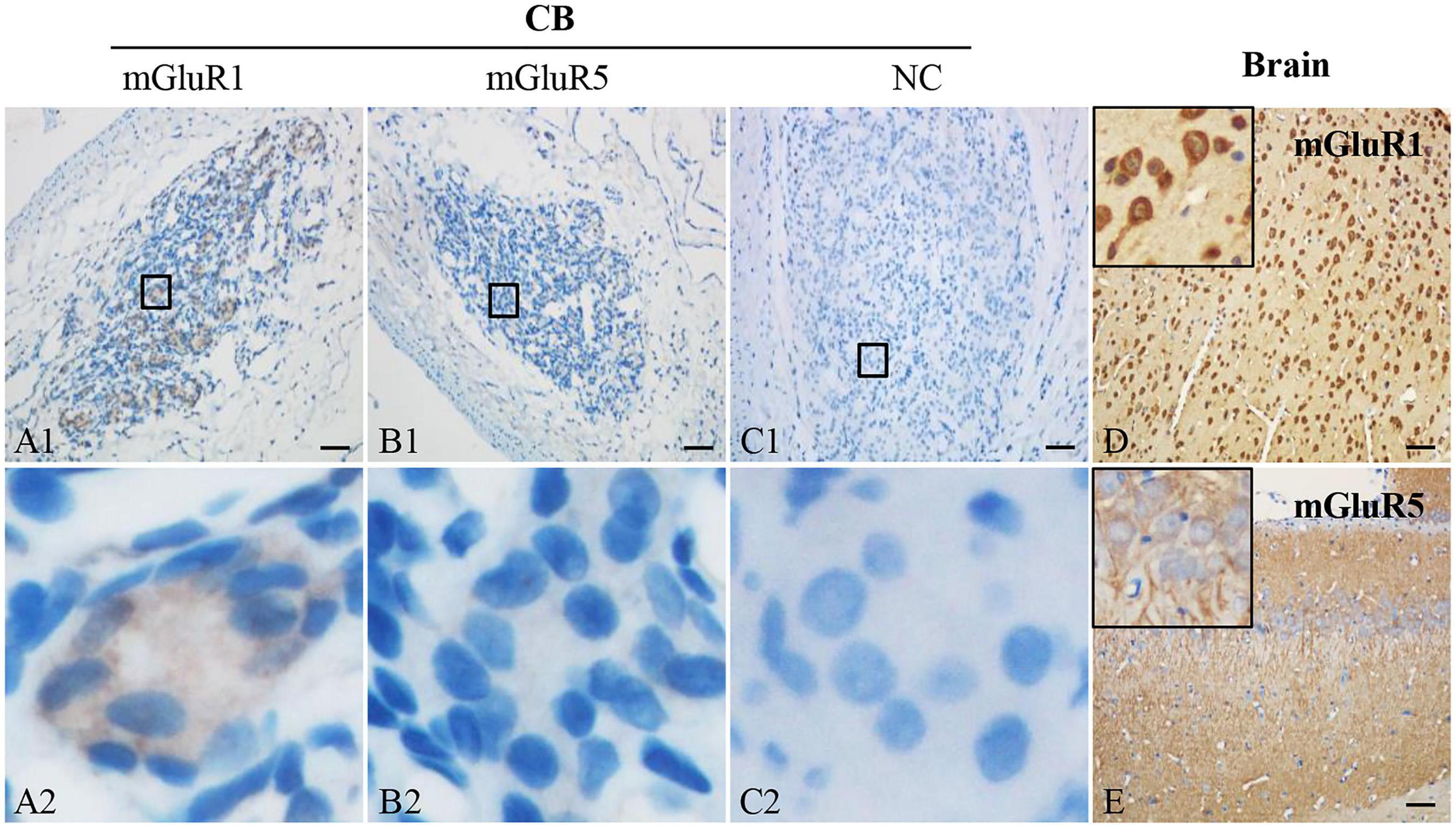
Figure 2. Immunohistochemical staining of mGluR1 and mGluR5 in rat CB. Representative images of rat CB and brain sections were immunostained with mGluR1 and mGluR5. Panels (C1,C2) are negative staining controls obtained by omitting the primary antibody. mGluR1 (D) or mGluR5 (E) staining of rat brain sections were used as positive controls. Panels (A2–C2) are higher-magnification images of framed areas in (A1–C1), respectively. The brown staining represents the mGluR1 or mGluR5, while the blue staining represents hematoxylin-stained cell nuclei. mGluR, glutamate metabotropic receptor; NC, negative control. Scale bar, 50 μm for all images.
Cellular Localization of Metabotropic Glutamate Receptors 1 in Rat Carotid Body
The CB is composed of type I glomus cells and type II sustentacular cells. To further ascertain the localization of mGluR1 in rat CB, double immunofluorescence was performed. TH was used as the type I glomus cell marker and GFAP was used as the type II cell marker. As shown in Figure 3, the mGluR1 immunoreactive signal was diffusely present in the cytoplasm of round-shaped cells which are clustered in rat CB and co-localized with TH-positive immunoreactivity (Figures 3A–C), but not detected with GFAP-positive signals (Figures 3D–F), thus indicating that mGluR1 distributes in type I cells rather in type II cells in rat CB.
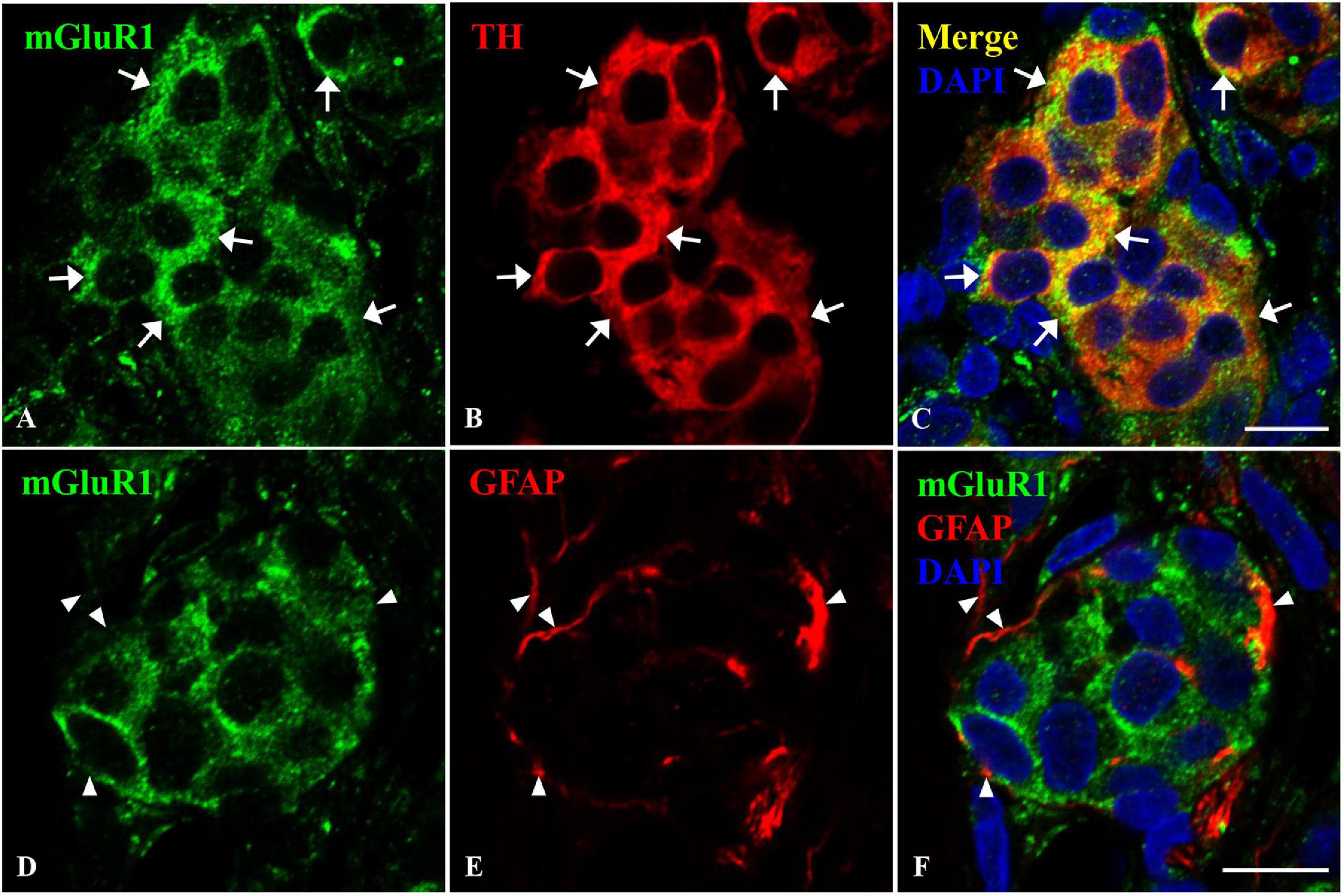
Figure 3. Distribution of mGluR1 in rat CB. Panels (A–C) are double immunofluorescence staining of mGluR1 (green) with TH (red) in rat CB. Panels (D–F) are double immunofluorescence staining of mGluR1 (green) and GFAP (red) in rat CB. Some TH-immunoreactive type I cells are immunoreactive for mGluR1 (arrows), whereas the GFAP-immunoreactive type II cells are not immunoreactive for mGluR1 (arrowheads). TH, tyrosine hydroxylase, the CB type I cells marker; GFAP, glial fibrillary acidic protein, the CB type II cells marker; mGluR, glutamate metabotropic receptor. Scale bar, 10 μm for all images.
Activation of Group I Metabotropic Glutamate Receptors Attenuates Carotid Body Response to Hypoxia
As a chemoreceptor, the CB senses the reduction of arterial PO2 during hypoxia, resulting in afferent activation of the CSN, which is also known as the CB response to hypoxia. To investigate the effect of mGluR1 on the CB response to hypoxia, CSN discharge was recorded from CB-sinus nerve preparations ex vivo. A representative trace of CSNA is shown in Figure 4A. Similar to a previous study (Rong et al., 2003), the baseline CSNA under 95% O2 was increased sharply after exposure to 5% O2 hypoxia. The time from exposure to hypoxia to the onset of CSNA increase is referred to as latency time (t; Figure 4C) of CB response to hypoxia. After application of selective group I mGluR agonist DHPG into basal perfusate (95% O2–5% CO2 saturated Kreb’s solution) for 10 min, DHPG (40 μM) significantly inhibited the CSNA under hypoxia conditions (normalized integrated CSNA before treatment with DHPG vs. normalized integrated CSNA while treatment with DHPG: 248.80 ± 26.17 vs. 175.03 ± 19.23%; n = 9, p < 0.005) (Figures 4A,B). The latency time of CB response to hypoxia was 47.78 ± 2.52 s before treatment with DHPG, 83.89 ± 1.82 s during treatment with DHPG, and 45.56 ± 2.36 s after treatment with DHPG (Figures 4C,D; n = 9, p < 0.01). These results indicate that group I mGluR activation inhibits CSNA under hypoxia and increases the latency time of CB response to hypoxia; that is, activation of group I mGluRs attenuates the CB response to hypoxia.
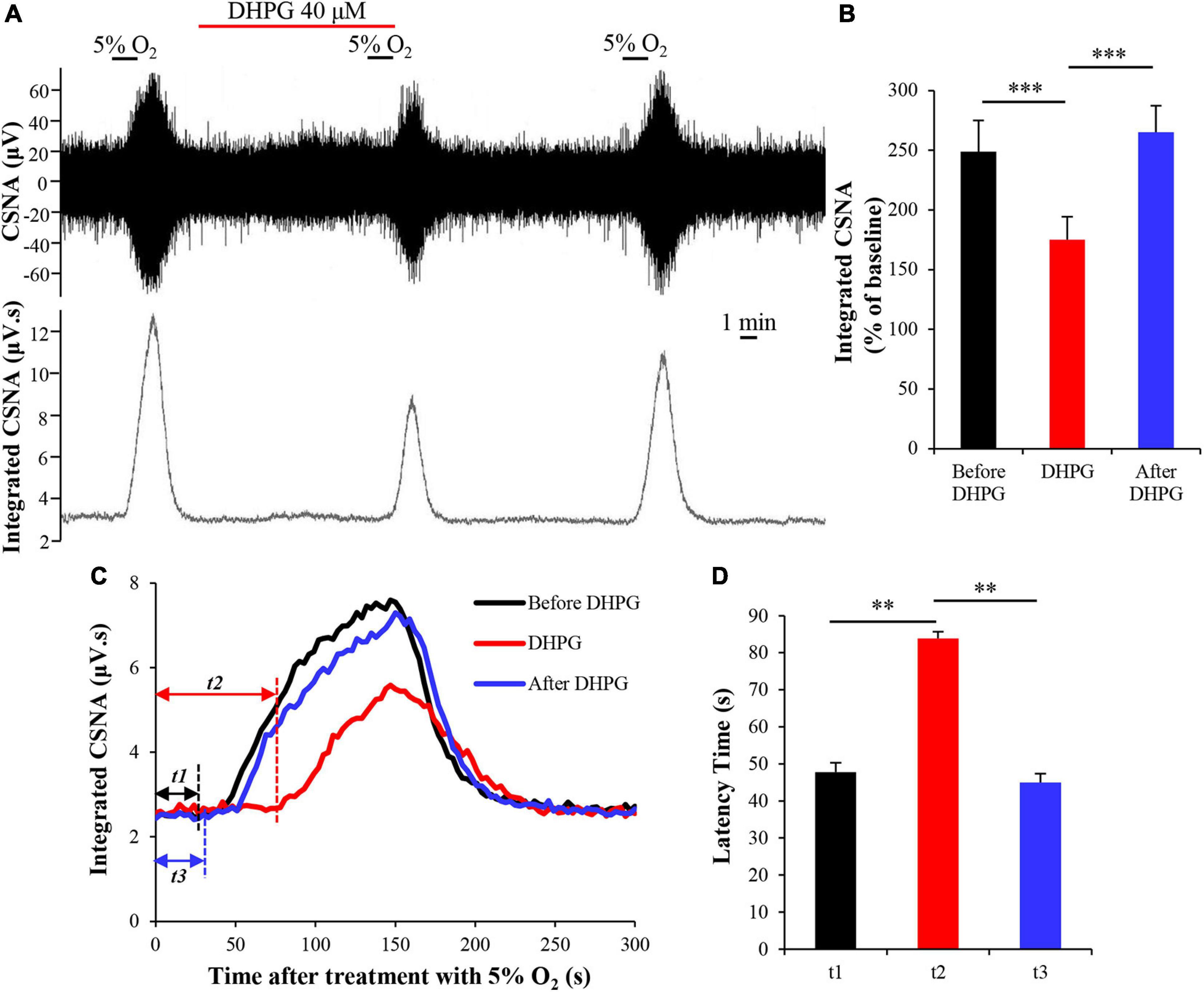
Figure 4. DHPG attenuates CB response to hypoxia. (A) Effect of DHPG (40 μM) on the CSNA under hypoxia conditions. Note that DHPG reversibly reduces the CSNA under hypoxia condition. (B) DHPG (40 μM) reduces the integrated CSNA during hypoxia. (C) Changes in integrated CSNA within 5 min of switching to 5% O2–5% CO2–90% N2 saturated Kreb’s solution. t1, latency time of CB response to hypoxia before treatment with DHPG (40 μM); t2, latency time of CB response to hypoxia while treatment with DHPG; t3, latency time of CB response to hypoxia after treatment with DHPG. (D) DHPG (40 μM) reversibly increases the latency time of CB response to hypoxia. DHPG, group I mGluRs agonist; CSNA, carotid sinus nerve activity; t, time. The data were presented as mean ± SEM. n = 9, ∗∗p < 0.01 and ∗∗∗p < 0.005 indicate significant difference (one-way ANOVA with repeated measures).
Activation of Metabotropic Glutamate Receptors 1 Attenuates Carotid Body Response to Hypoxia
To assess the role of mGluR1 in DHPG-attenuated CB response to hypoxia, we first investigated the effects of mGluR1 antagonist JNJ16259685 (JNJ) on CB response to hypoxia by applying JNJ (20 μM) in basal perfusate for 10 min before exposing it to hypoxia. We found that JNJ enhanced the CSNA under hypoxia conditions, as indicated by an increase in normalized integrated CSNA from 186.72 ± 13.72 to 232.77 ± 17.61% (n = 7, p < 0.005) (Figures 5A,B). The inhibitory effect of DHPG on CSNA under hypoxia conditions was also blocked by pre-perfusion with JNJ for 5 min, as the normalized integrated CSNA increased from 144.60 ± 8.05 (treatment with DHPG only) to 200.87 ± 15.77% (treatment with DHPG and JNJ) (n = 4, p < 0.05) (Figures 5C,D). These results indicate that DHPG attenuates CB response to hypoxia by activating mGluR1; that is, mGluR1 in CB may act as an inhibitory feedback regulator to modulate CB chemoreflex.
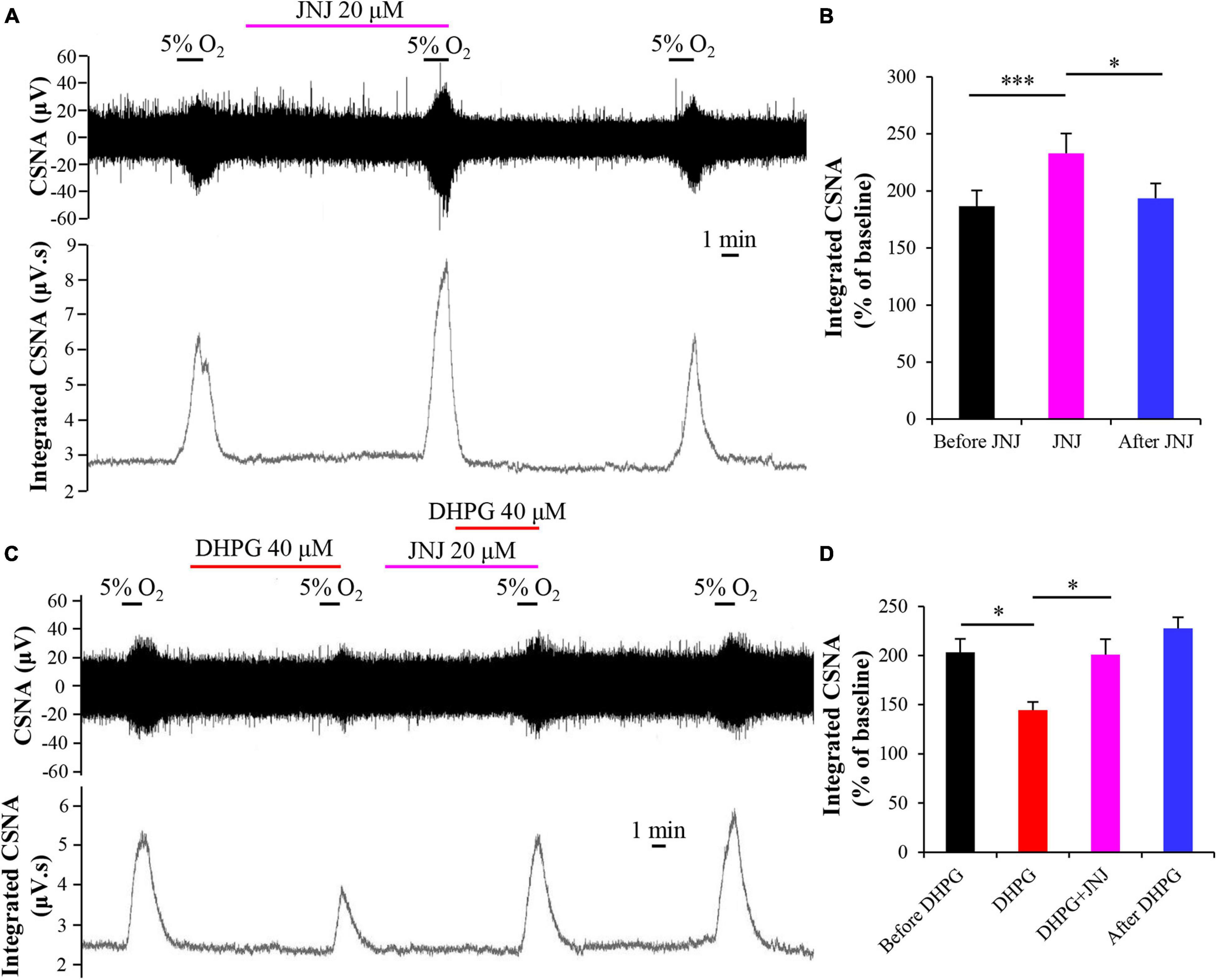
Figure 5. mGluR1 antagonist JNJ blocks the inhibitory effect of DHPG on CB response to hypoxia. (A) Effect of JNJ (20 μM) on the CSNA under hypoxia conditions. Note that DHPG reversibly enhances the CSNA under hypoxia conditions. (B) JNJ (20 μM) increases the normalized integrated CSNA during hypoxia. n = 7. (C,D) Pretreatment with JNJ prevents the inhibitory effect of DHPG on CB response to hypoxia. The data were presented as mean ± SEM. n = 4. ∗p < 0.05 and ∗∗∗p < 0.05 indicate significant difference (one-way ANOVA with repeated measures). CSNA, carotid sinus nerve activity; JNJ, JNJ16259685, mGluR1 antagonist.
Inhibitory Effect of (S)-3,5-Dihydroxyphenylglycine on Carotid Body Response to Hypoxia Involves the GABAB Receptor
Previous studies found that GABA inhibits hypoxia-induced postsynaptic sensory responses in coculture of rat CB type I cells with petrosal neurons by acting on GABAA receptors (Zhang et al., 2009). We also found that GABA inhibits the rat CB hypoxia response in vitro (unpublished data). GABA also plays an autoreceptor feedback inhibition in CB response to hypoxia via acting on the GABAB receptor in CB type I cells (Fearon et al., 2003), and the cross talk has been demonstrated between the GABAB receptor and other receptors (such as GABAA receptor, mGluR1, and NMDA receptor) (Xu et al., 2014). Therefore, we speculate that the GABAB receptor may be involved in the DHPG-induced inhibitory effect on CB response to hypoxia by activating mGluR1. To determine whether activated mGluR1 exerts its inhibitory effect on CB response to hypoxia through GABAB receptor, we applied the GABAB receptor antagonist CGP52432 (10 μM) for 5 min before DHPG treatment. As shown in Figure 6, compared with normalized integrated CSNA during treatment with DHPG (148.96 ± 21.28%), the normalized integrated CSNA was increased to 208.00 ± 36.18% (n = 5, p < 0.05) after pre-perfusion with CGP52432. That is, CGP52432 could prevent the inhibitory effect of DHPG on CB response to hypoxia. This result indicates that the GABAB receptor in CB may be involved in the mGluR1-induced feedback inhibition in CB response to hypoxia.
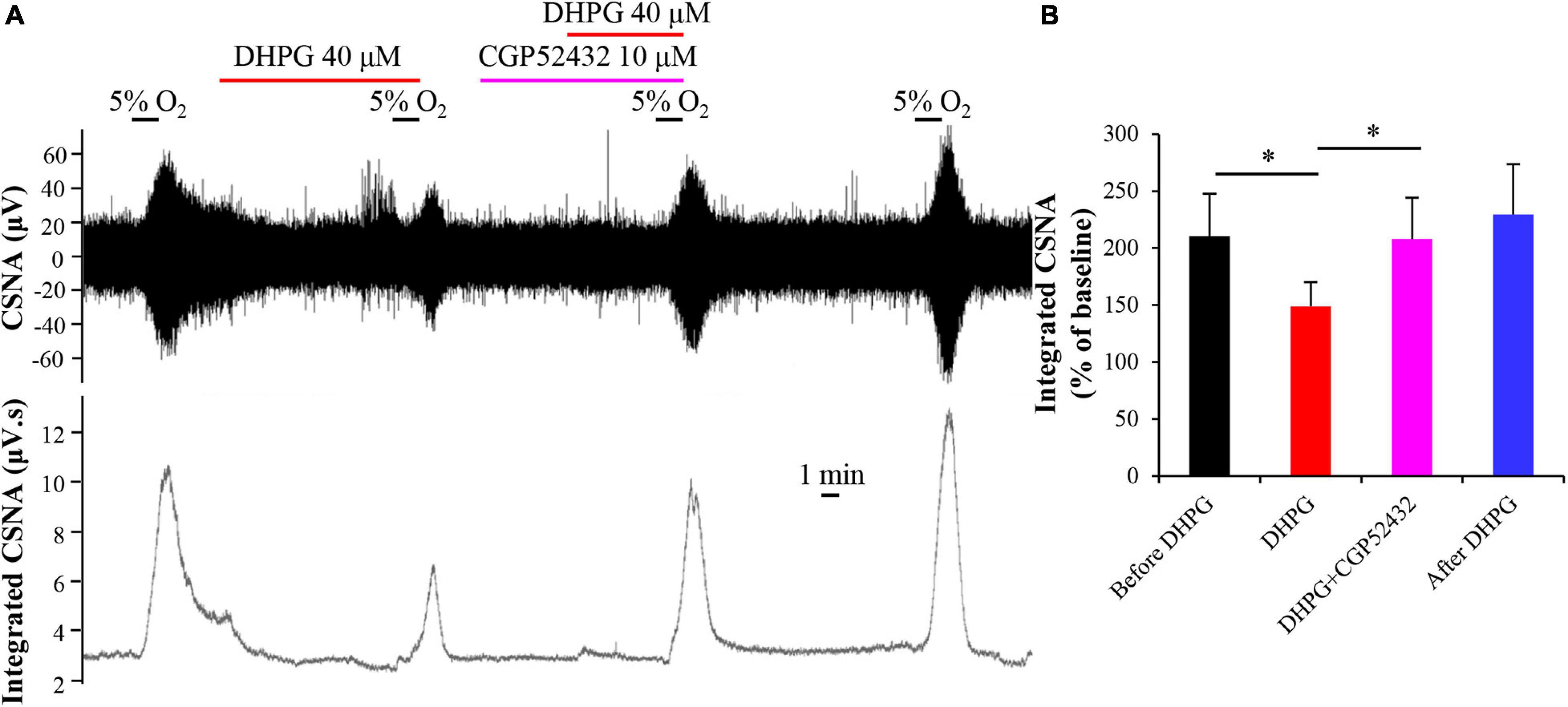
Figure 6. GABAB receptor antagonist CGP52432 blocks inhibitory effect of DHPG on CB response to hypoxia. (A) Representative traces of changes in raw CSNA and integrated CSNA recordings. Note that pretreatment with CGP52432 prevents the inhibitory effect of DHPG on CSNA under hypoxia conditions. (B) Statistical analysis of normalized integrated CSNA after difference treatment. The data were presented as mean ± SEM. n = 5, ∗p < 0.05 indicates significant difference (one-way ANOVA with repeated measures). CSNA, carotid sinus nerve activity; CGP52432, GABAB receptor antagonist; DHPG, group I mGluRs agonist.
Discussion
In this study, we found for the first time that mRNAs of group I mGluRs (mGluR1 and mGluR5) were expressed in human and rat CB, whereas the protein of mGluR1 instead of mGluR5 was detected in rat CB and scattered throughout the CB. Moreover, we determined that mGluR1 was distributed in type I glomus cells. Our investigation indicates that group I mGluR activation by DHPG inhibited CB response to hypoxia, while mGluR1 antagonist JNJ16259685 enhanced CB response to hypoxia and blocked the inhibitory effect of DHPG on CB response to hypoxia. We also found that the inhibitory effect of DHPG on CB response to hypoxia might be related to the GABAB receptor in CB type I cells, as the inhibitory effect could be blocked by inhibition of the GABAB receptor. Taken together, these results indicate that, rather than mGluR5, functional mGluR1 exists in the CB and may play a role in presynaptic feedback inhibition in rat CB hypoxia sensitivity.
The CB transmits the changes of oxygen levels in arterial blood through chemosensory synapses formed by type I cells (as presynaptic neuroendocrine cells) with CSN afferent fibers (as postsynaptic nerve endings). Hypoxia-induced depolarization of type I cells releases a variety of neurotransmitters, which are the material basis of CB oxygen sensory transmission (Ortega-Sáenz and López-Barneo, 2020), acting on the corresponding receptors of CSN afferent fibers. Studies have confirmed that neurotransmitters in CB type I cells such as ATP, ACh, 5-HT, and GABA regulate CB response to hypoxia by acting on P2X receptors (Zhang et al., 2000; Prasad et al., 2001; Iturriaga et al., 2007), nicotinic ACh receptors (nAChR; Zhang et al., 2000; Varas et al., 2003; Iturriaga et al., 2007), 5-HT2 receptors (Jacono et al., 2005), and GABAA receptors (Zhang et al., 2009) on postsynaptic CSN afferent fibers, respectively. Nonetheless, the role of glutamate and its receptors, the most important neurotransmitter in the central nervous system, in CB response to hypoxia is unclear.
Torrealba et al. (1996) report that type I cells in the cat CB had high concentrations of glutamate, but glutamate release from in vitro cat CB superfusion with high potassium within 2 min was not observed. The perfusate used in this study was 50 or 70 mM potassium Krebs–Ringer–HEPES (KRH) solution. Accordingly, they speculated that glutamate could not be released as a neurotransmitter from type I cells. Interestingly, when Kim et al. (2004) measured the ACh release from rabbit CB, they found that 30 or 60 mM KCl stimulation for 15 min had no effect on ACh release, but increasing the KCl concentration to 100 mM significantly induced the ACh release from the CB, indicating whether or not a transmitter release from the CB triggered by high potassium in vitro might be dependent on potassium concentration and duration time. Hence, it cannot entirely exclude the possibility that the superfusion condition in the study by Torrealba was not optimum to induce glutamate release from the CB stimulated with high-potassium solution in vitro. Interestingly, Yokoyama et al. (2014) found that VGluT2 is localized in the carotid sinus afferent nerve terminals. Recently, we have demonstrated multiple subtypes of VGluTs (VGluT3) and EAATs (EAAT2 and 3) being present in the CB (Li et al., 2020). These data suggested that glutamate might be releasing from CB itself or carotid sinus afferent nerve terminals, and then acting as a neurotransmitter. We also found that functional NMDAR1 and AMPAR1 in rat CB might regulate the CB chemoreflex plasticity (Liu et al., 2009, 2018). In this study, we further discovered that mRNAs of group I mGluRs (mGluR1 and 5) were also expressed in human and rat CB (Figure 1); this finding is consistent with our previous report; i.e., the adrenal medulla, another oxygen sensor originating similarly with the CB, expresses both mGluR1 and 5 (Fan et al., 2020). Among group I mGluRs, only mGluR1 protein was detected in rat CB by immunostaining (Figure 2). Awobuluyi et al. (2003) reported that glutamate inotropic receptor NMDAR1 (NR1) mRNA was present in PC12 cells but not its protein, and the underlying mechanism might be from the 3′ untranslated region of NR1 blocking the translation of NR1 mRNA at initiation in PC12 cells. Therefore, rat CB expressing mGluR5 mRNA but lacking obviously expression of mGluR5 protein implies translational or posttranscriptional modification. Additional work will be necessary to characterize the discrepancy expression between mGluR5 mRNA and protein in rat CB.
As members of the G protein-coupled receptor family, mGluRs are divided into three subtypes, groups I, II, and III, based on its receptor structure and physiological activity (Ohashi et al., 2002). In the central nervous system, the three groups of mGluRs have different precise synaptic site locations. Generally, group I mGluRs are located primarily on the postsynaptic membrane, and groups II and III mGluRs are primarily on the presynaptic membrane (Shigemoto et al., 1997). However, some studies have shown presynaptic group I mGluRs. Romano et al. (1995) reported that some mGluR5 were located in the presynaptic axon terminal. Wittmann et al. (2001) also found that mGluR1 was localized on presynaptic elements of the excitatory synapses of substantia nigra pars reticulata by immunohistochemistry at the electron microscope level. In this study, mGluR1 immunoreactive signals were co-localized with TH immunoreactives, and moreover, both signals were diffusely present in the cytoplasm of clustered round-shaped cells (Figure 3), demonstrating the ubiquitous presence of mGluR1 in type I cells of rat CB. However, we cannot rule out that mGluR1 is also present in TH-positive nerve terminals projected from petrosal ganglion neurons, which has been shown to express TH (Czyzyk-Krzeska et al., 1991; Finley et al., 1992). Additional co-staining of mGluR1 with a specific marker of postsynaptic nerve ending, such as the P2X2 receptor, will help answer this question. Due to type I cells being presynaptic neuroendocrine cells to the afferent nerve terminal of CSN, we thus speculate that mGluR1 in the carotid chemoreflex might regulate the synaptic transmission via a presynaptic mechanism.
Group I mGluRs play an important role in synaptic transmission and synaptic plasticity through G protein coupling (Anwyl, 2009; Reiner and Levitz, 2018) or through non-canonical effector pathways (Eng et al., 2016). Previous studies demonstrated that group I mGluRs participated in pathogenic and adaptogenic response to hypoxia in rat brain development (Simonyi et al., 1999; Vetrovoy et al., 2021) and mGluR1/5 was involved in the enhancement of respiratory rhythm during early hypoxia by concomitant activation of L-type Ca(2+) channels in inspiratory brainstem neurons (Mironov and Richter, 2000). Our previous study also found that inhibition of mGluR1 blocked the hypoxia-induced activation of ERK1/2 in rat adrenal medulla (Fan et al., 2020). These data suggested that group I mGluRs are likely to be involved in hypoxic modification of channel activity or regulation of hypoxia response molecules in some conditions. In this study, we observed that superfusion of rat CB in vitro with DHPG, an agonist of group I mGluRs, decreased the acute hypoxia-evoked discharge activity of CSN (Figure 4), and this effect was blocked by mGluR1 antagonist JNJ16259685 (Figure 5), indicating an inhibitory effect of mGluR1 on CB response to acute hypoxia. There is evidence shown that, via activation of the G protein-coupled receptor downstream protein kinase C, postsynaptic mGluR1 mediates the potentiation of NMDAR activity in hippocampus glutamatergic synapses (Skeberdis et al., 2001) or the hyper-excitability of layer 5 pyramidal neurons in the rat anterior cingulate cortex (Gao et al., 2016). In contrast, some evidence showed that mGluR1 or 5 inhibits excitatory or inhibitory synaptic transmission in the hippocampus CA1 (Gereau and Conn, 1995; Manzoni and Bockaert, 1995) and the superior colliculus (White et al., 2003) via presynaptic mechanism. Studies also found that activation of group I mGluRs by DHPG induces a long-term depression of excitatory synaptic transmission in the hippocampus (Fitzjohn et al., 2001; Faas et al., 2002) or hippocampal interneurons via presynaptic P/Q-type Ca2+ channels (Le Duigou et al., 2011). Thus, activation of group I mGluRs results in different functions in the central and peripheral nervous systems, which depends on its localization on the synaptic site. Based on these above studies and our results in this study, we speculate that activation of mGluR1 likely reduces hypoxia-induced CB response by regulating the release of other neurotransmitters in presynaptic type I cells or by regulating P/Q-type Ca2+ channels in type I cells.
We also found that activation of mGluR1 by DHPG prolonged the latency time of CB response to hypoxia (Figures 4C,D). Hypoxia induces Ca2+-dependent release of a variety of excitatory (such as ATP and ACh) (Zhang et al., 2000) and inhibitory (such as GABA and dopamine) (Zhang et al., 2009, 2018) transmitters in rat CB. These transmitters, which released from rat CB, act on postsynaptic P2X2/3 receptors and nAChR to produce excitatory effects or on GABAA receptors and D2 receptors to produce inhibitory effects on CSNA to regulate the final CSN afferent signal. Jacono et al. (2005) detected the release of neurotransmitters in the perfusion solution after treatment with acute hypoxia by using high-performance liquid chromatography coupled with an electrochemical detection system. They found that hypoxia induced the release of different neurotransmitters from type I cells in a time-dependent manner, i.e., different neurotransmitters released at different times following hypoxia. Therefore, we speculated that DHPG may inhibit the release of some neurotransmitters in CB type I cells under early hypoxia, subsequently leading to the prolonged latency of CB response to hypoxia.
Carotid body has a complex regulatory network. A variety of neurotransmitters or neuromodulators act on the corresponding receptors in type I cells or CSN afferent fibers to produce excitatory or inhibitory effects and ultimately regulate the input of CB type I cells’ oxygen-sensitive signals (Aldossary et al., 2020; Ortega-Sáenz and López-Barneo, 2020). Many of these receptors are G protein-coupled receptors located not only in CSN afferent fibers but also in type I cells and type II cells (Guidolin et al., 2018). For example, GABA inhibits hypoxia-induced release of ATP and ACh by acting on presynaptic GABAB receptor-mediated activation of TWIK-related acid-sensitive potassium channel 1 (TASK-1) (Fearon et al., 2003). Evidence showed that G protein-coupled receptors may interact with each other under various physiological and pathological conditions (Guidolin et al., 2018, 2019). Based on a varietyof G protein-coupled receptors located in CB, the researchers suggested that there may be receptor–receptor interactions in CB (Porzionato et al., 2018). Previous studies found that there is also an interaction between GABAB and mGluR1, which is not a direct physical interaction between both receptors but rather a synergistic enhancement effect between G protein-coupled receptors (Rives et al., 2009; Xu et al., 2014). In this study, our results showed that pre-blocking GABAB receptor activity by superfusion with its antagonist CGP52432 at least partially blocked the inhibitory effect of DHPG on acute hypoxia-evoked discharge of CSN (Figure 6). This suggests that the inhibitory effect of mGluR1 activation on CB hypoxia response is at least partially related to the GABAB receptor. Future studies will determine how mGluR1 activates GABAB directly or indirectly.
Taken together, our results in this study provide a novel candidate molecule that is involved in regulating CB response to hypoxia. We speculate that hypoxia not only stimulates the release of ATP, ACh, and GABA from CB type I cells but also stimulates the release of glutamate, which activates mGluR1 receptors on the surface of type I cells. Subsequently, mGluR1 may enhance the effect of GABAB receptors by directly interacting with GABAB receptors or indirectly modulating the release of GABA, and ultimately attenuates hypoxia-evoked enhancement of CSN afferent discharges (Figure 7).
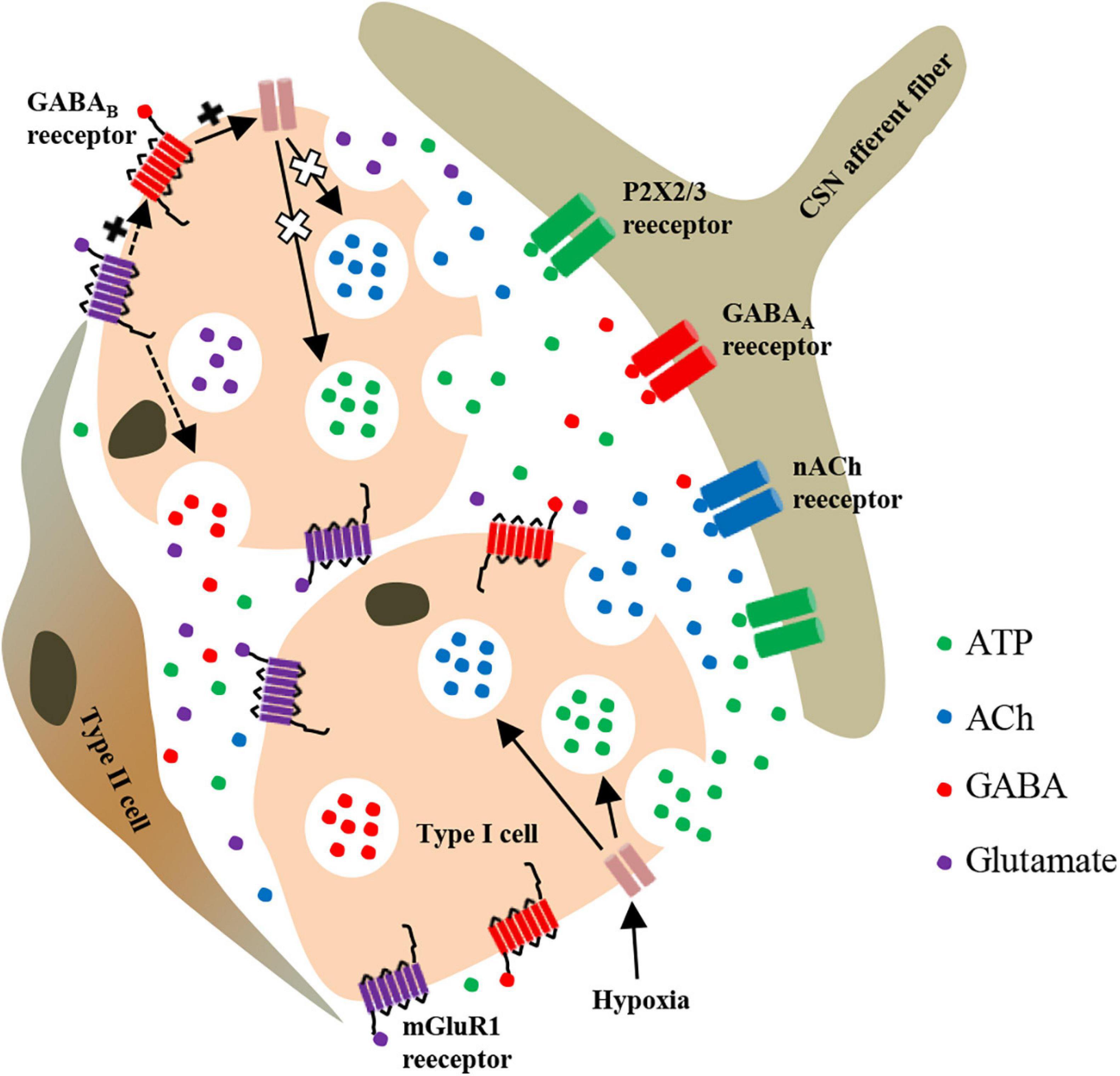
Figure 7. Schematic representation of mGluR1-mediated regulation of neurotransmitter release from rat CB type I cells during hypoxia. Inhibition of TASK-1 in type I cells by hypoxia leads to membrane depolarization and release of various neurotransmitters. Among these neurotransmitters, glutamate and GABA act on presynaptic mGluR1 and GABAB receptors in themselves or in adjacent type I cells. GABAB activation further activates TASK-1, thus leading to an increase of potassium outflow, then limiting the degree of depolarization of type I cells in order to inhibit the further release of ATP and ACh induced by hypoxia. Additionally, mGluR1 activation may enhance the activation effect of GABAB receptor on TASK-1 through synergistic enhancement or modulate the release of GABA. In other words, both mGluR1 and GABAB receptors on type I cells regulate the CSN afferent signals of CB response to hypoxia through a presynaptic feedback mechanism. P2X2/3, purinergic P2X2/3 receptor; nACh, nicotinic ACh receptor.
Although this study found that GABAB receptors may be involved in the inhibitory effect of mGluR1 on CB response to hypoxia, we cannot rule out other presynaptic mechanisms involved in this inhibitory effect. Further studies via single unit electrophysiological recording, along with primary coculture of type I cells with petrosal ganglion cells, will hopefully clarify the specific mechanism of mGluR1 on CB response to hypoxia. In addition, we cannot rule out that glutamate, like 5-HT (Jacono et al., 2005), participates in CB response to hypoxia as a modulator rather than an initiator. Therefore, further work on detecting glutamate release from CB after hypoxia treatment is necessary.
Conclusion
In summary, our study found that the protein of mGluR1, rather than mGluR5, is mainly expressed in the CB and plays a presynaptic feedback inhibition on CB response to hypoxia.
Data Availability Statement
The original contributions presented in the study are included in the article/Supplementary Material, further inquiries can be directed to the corresponding author.
Ethics Statement
The studies involving human participants were reviewed and approved by the Ethics Committee of The First Affiliated Hospital of Xinxiang Medical University. The patients/participants provided their written informed consent to participate in this study. The animal study was reviewed and approved by the Institutional Animal Ethics Committee at The First Affiliated Hospital of Xinxiang Medical University.
Author Contributions
YL designed the study. YL and BZ supervised the study execution. CL, CZ, and LH performed the experiments and analyzed the data. CL and YL wrote the manuscript. CL, BZ, and YL reviewed the manuscript. All authors read and approved the final manuscript.
Funding
This work was supported by the National Natural Science Foundation of China to YL (Grant No. 81973988).
Conflict of Interest
The authors declare that the research was conducted in the absence of any commercial or financial relationships that could be construed as a potential conflict of interest.
Publisher’s Note
All claims expressed in this article are solely those of the authors and do not necessarily represent those of their affiliated organizations, or those of the publisher, the editors and the reviewers. Any product that may be evaluated in this article, or claim that may be made by its manufacturer, is not guaranteed or endorsed by the publisher.
Acknowledgments
We thank Weifang Rong (Department of Anatomy and Physiology, Shanghai Jiao Tong University School of Medicine, Shanghai, China) for the technical guidance of in vitro carotid sinus nerve recording. We thank Chenfang Qi (Intensive Care Unit, Lowell General Hospital, Lowell, United States) for providing language editorial assistance.
Supplementary Material
The Supplementary Material for this article can be found online at: https://www.frontiersin.org/articles/10.3389/fnins.2021.741214/full#supplementary-material
Abbreviations
ACh, acetylcholine; AMPARs, alpha-amino-3-hydroxy-5-methyl-4-isoxazole propionic acid receptors; CB, carotid body; CIH, cyclic intermittent hypoxia; CSN, carotid sinus nerve; CSNA, carotid sinus nerve activity; DHPG, (S)-3,5-dihydroxyphenylglycine; EAAT, excitatory amino acid transporter; GFAP, glial fibrillary acidic protein; iGluRs, ionotropic glutamate receptors; JNJ, JNJ16258685, (3,4-dihydro-2H-pyrano[2,3-b]quinolin-7-yl)-(cis-4-methoxycyclohexyl)-methanone; KARs, kainite receptors; GRM, mGluRs, metabotropic glutamate receptors; NC, negative control; NMDARs, N-methyl-D-aspartate receptors; nAChR, nicotinic ACh receptors; VGluT, vesicular glutamate transporter; PBS, phosphate-buffered saline; RT, reverse transcription; SD, Sprague-Dawley; TH, tyrosine hydroxylase; TASK-1, TWIK-related acid-sensitive potassium channel 1.
Footnotes
References
Alcayaga, J., Cerpa, V., Retamal, M., Arroyo, J., Iturriaga, R., and Zapata, P. (2000). Adenosine triphosphate-induced peripheral nerve discharges generated from the cat petrosal ganglion in vitro. Neurosci. Lett. 282, 185–188. doi: 10.1016/s0304-3940(00)00896-x
Alcayaga, C., Varas, R., Valdés, V., Cerpa, V., Arroyo, J., Iturriaga, R., et al. (2007). ATP- and ACh-induced responses in isolated cat petrosal ganglion neurons. Brain Res. 1131, 60–67. doi: 10.1016/j.brainres.2006.11.012
Aldossary, H. S., Alzahrani, A. A., Nathanael, D., Alhuthail, E. A., Ray, C. J., Batis, N., et al. (2020). G-protein-coupled receptor (GPCR) signaling in the carotid body: roles in hypoxia and cardiovascular and respiratory disease. Int. J. Mol. Sci. 21:6012. doi: 10.3390/ijms21176012
Anwyl, R. (2009). Metabotropic glutamate receptor-dependent long-term potentiation. Neuropharmacology 56, 735–740. doi: 10.1016/j.neuropharm.2009.01.002
Awobuluyi, M., Vazhappilly, R., and Sucher, N. J. (2003). Translational activity of N-methyl-D-aspartate receptor subunit NR1 mRNA in PC12 cells. Neurosignals 12, 283–291. doi: 10.1159/000075310
Chang, A. J., Ortega, F. E., Riegler, J., Madison, D. V., and Krasnow, M. A. (2015). Oxygen regulation of breathing through an olfactory receptor activated by lactate. Nature 527, 240–244. doi: 10.1038/nature15721
Chen, T. J., and Kukley, M. (2020). Glutamate receptors and glutamatergic signalling in the peripheral nerves. Neural Regen. Res. 15, 438–447. doi: 10.4103/1673-5374.266047
Czyzyk-Krzeska, M. F., Bayliss, D. A., Lawson, E. E., and Millhorn, D. E. (1991). Expression of messenger RNAs for peptides and tyrosine hydroxylase in primary sensory neurons that innervate arterial baroreceptors and chemoreceptors. Neurosci. Lett. 129, 98–102. doi: 10.1016/0304-3940(91)90729-d
Eng, A. G., Kelver, D. A., Hedrick, T. P., and Swanson, G. T. (2016). Transduction of group I mGluR-mediated synaptic plasticity by beta-arrestin2 signalling. Nat. Commun. 7:13571. doi: 10.1038/ncomms13571
Faas, G. C., Adwanikar, H., Gereau, R. W. IV, and Saggau, P. (2002). Modulation of presynaptic calcium transients by metabotropic glutamate receptor activation: a differential role in acute depression of synaptic transmission and long-term depression. J. Neurosci. 22, 6885–6890. doi: 10.1523/JNEUROSCI.22-16-06885.2002
Fan, Y. N., Li, C., Huang, L., Chen, L., Tang, Z., Han, G., et al. (2020). Characterization of group I metabotropic glutamate receptors in rat and human adrenal glands. Front. Physiol. 11:401. doi: 10.3389/fphys.2020.00401
Fearon, I. M., Zhang, M., Vollmer, C., and Nurse, C. A. (2003). GABA mediates autoreceptor feedback inhibition in the rat carotid body via presynaptic GABAB receptors and TASK-1. J. Physiol. 553, 83–94. doi: 10.1113/jphysiol.2003.048298
Finley, J. C., Polak, J., and Katz, D. M. (1992). Transmitter diversity in carotid body afferent neurons: dopaminergic and peptidergic phenotypes. Neuroscience 51, 973–987. doi: 10.1016/0306-4522(92)90534-9
Fitzjohn, S. M., Palmer, M. J., May, J. E., Neeson, A., Morris, S. A., and Collingridge, G. L. (2001). A characterisation of long-term depression induced by metabotropic glutamate receptor activation in the rat hippocampus in vitro. J. Physiol. 537, 421–430. doi: 10.1111/j.1469-7793.2001.00421.x
Gao, L., Ortega-Sáenz, P., and López-Barneo, J. (2019). Acute oxygen sensing-role of metabolic specifications in peripheral chemoreceptor cells. Respir. Physiol. Neurobiol. 265, 100–111. doi: 10.1016/j.resp.2018.08.007
Gao, S. H., Wen, H. Z., Shen, L. L., Zhao, Y. D., and Ruan, H. Z. (2016). Activation of mGluR1 contributes to neuronal hyperexcitability in the rat anterior cingulate cortex via inhibition of HCN channels. Neuropharmacology 105, 361–377. doi: 10.1016/j.neuropharm.2016.01.036
Gereau, R. W. IV, and Conn, P. J. (1995). Multiple presynaptic metabotropic glutamate receptors modulate excitatory and inhibitory synaptic transmission in hippocampal area CA1. J. Neurosci. 15, 6879–6889. doi: 10.1523/JNEUROSCI.15-10-06879.1995
Guidolin, D., Marcoli, M., Tortorella, C., Maura, G., and Agnati, L. F. (2018). G protein-coupled receptor-receptor interactions give integrative dynamics to intercellular communication. Rev. Neurosci. 29, 703–726. doi: 10.1515/revneuro-2017-0087
Guidolin, D., Marcoli, M., Tortorella, C., Maura, G., and Agnati, L. F. (2019). Receptor-receptor interactions as a widespread phenomenon: novel targets for drug development? Front. Endocrinol. (Lausanne) 10:53. doi: 10.3389/fendo.2019.00053
Iturriaga, R., Varas, R., and Alcayaga, J. (2007). Electrical and pharmacological properties of petrosal ganglion neurons that innervate the carotid body. Respir. Physiol. Neurobiol. 157, 130–139. doi: 10.1016/j.resp.2006.12.006
Jacono, F. J., Peng, Y. J., Kumar, G. K., and Prabhakar, N. R. (2005). Modulation of the hypoxic sensory response of the carotid body by 5-hydroxytryptamine: role of the 5-HT2 receptor. Respir. Physiol. Neurobiol. 145, 135–142. doi: 10.1016/j.resp.2004.10.002
Jin, L. E., Wang, M., Galvin, V. C., Lightbourne, T. C., Conn, P. J., Arnsten, A. F. T., et al. (2018). mGluR2 versus mGluR3 metabotropic glutamate receptors in primate dorsolateral prefrontal cortex: postsynaptic mGluR3 strengthen working memory networks. Cereb. Cortex 28, 974–987. doi: 10.1093/cercor/bhx005
Kim, D. K., Prabhakar, N. R., and Kumar, G. K. (2004). Acetylcholine release from the carotid body by hypoxia: evidence for the involvement of autoinhibitory receptors. J. Appl. Physiol. (1985) 96, 376–383. doi: 10.1152/japplphysiol.00726.2003
Le Duigou, C., Holden, T., and Kullmann, D. M. (2011). Short- and long-term depression at glutamatergic synapses on hippocampal interneurons by group I mGluR activation. Neuropharmacology 60, 748–756. doi: 10.1016/j.neuropharm.2010.12.015
Li, C., Huang, L., Jia, X., Zhao, B., Chen, L., and Liu, Y. (2020). Functional glutamate transporters are expressed in the carotid chemoreceptor. Respir. Res. 21:208. doi: 10.1186/s12931-020-01468-z
Liu, Y., Ji, E. S., Xiang, S., Tamisier, R., Tong, J., Huang, J., et al. (2009). Exposure to cyclic intermittent hypoxia increases expression of functional NMDA receptors in the rat carotid body. J. Appl. Physiol. (1985) 106, 259–267. doi: 10.1152/japplphysiol.90626.2008
Liu, Y., Li, C., Jia, X., Huang, L., and Weiss, J. W. (2018). AMPA receptor-dependent glutamatergic signaling is present in the carotid chemoreceptor. Neuroscience 382, 59–68. doi: 10.1016/j.neuroscience.2018.04.032
Manzoni, O., and Bockaert, J. (1995). Metabotropic glutamate receptors inhibiting excitatory synapses in the CA1 area of rat hippocampus. Eur. J. Neurosci. 7, 2518–2523. doi: 10.1111/j.1460-9568.1995.tb01051.x
Mironov, S. L., and Richter, D. W. (2000). Hypoxic modulation of L-type Ca(2+) channels in inspiratory brainstem neurones: intracellular signalling pathways and metabotropic glutamate receptors. Brain Res. 869, 166–177. doi: 10.1016/s0006-8993(00)02396-9
Niswender, C. M., and Conn, P. J. (2010). Metabotropic glutamate receptors: physiology, pharmacology, and disease. Annu. Rev. Pharmacol. Toxicol. 50, 295–322. doi: 10.1146/annurev.pharmtox.011008.145533
Ohashi, H., Maruyama, T., Higashi-Matsumoto, H., Nomoto, T., Nishimura, S., and Takeuchi, Y. (2002). A novel binding assay for metabotropic glutamate receptors using [3H] L-quisqualic acid and recombinant receptors. Z. Naturforsch. C J. Biosci. 57, 348–355. doi: 10.1515/znc-2002-3-425
Ortega-Sáenz, P., and López-Barneo, J. (2020). Physiology of the carotid body: from molecules to disease. Annu. Rev. Physiol. 82, 127–149. doi: 10.1146/annurev-physiol-020518-114427
Platero-Luengo, A., González-Granero, S., Durán, R., Díaz-Castro, B., Piruat, J. I., García-Verdugo, J. M., et al. (2014). An O2-sensitive glomus cell-stem cell synapse induces carotid body growth in chronic hypoxia. Cell 156, 291–303. doi: 10.1016/j.cell.2013.12.013
Porzionato, A., Stocco, E., Guidolin, D., Agnati, L., Macchi, V., and De Caro, R. (2018). Receptor–receptor interactions of G protein-coupled receptors in the carotid body: a working hypothesis. Front. Physiol. 9:697. doi: 10.3389/fphys.2018.00697
Prasad, M., Fearon, I. M., Zhang, M., Laing, M., Vollmer, C., and Nurse, C. A. (2001). Expression of P2X2 and P2X3 receptor subunits in rat carotid body afferent neurones: role in chemosensory signalling. J. Physiol. 537, 667–677. doi: 10.1111/j.1469-7793.2001.00667.x
Reiner, A., and Levitz, J. (2018). Glutamatergic signaling in the central nervous system: ionotropic and metabotropic receptors in concert. Neuron 98, 1080–1098. doi: 10.1016/j.neuron.2018.05.018
Rives, M. L., Vol, C., Fukazawa, Y., Tinel, N., Trinquet, E., Ayoub, M. A., et al. (2009). Crosstalk between GABAB and mGlu1a receptors reveals new insight into GPCR signal integration. EMBO J. 28, 2195–2208. doi: 10.1038/emboj.2009.177
Romano, C., Sesma, M. A., McDonald, C. T., O’Malley, K., Van den Pol, A. N., and Olney, J. W. (1995). Distribution of metabotropic glutamate receptor mGluR5 immunoreactivity in rat brain. J. Comp. Neurol. 355, 455–469. doi: 10.1002/cne.903550310
Rong, W., Gourine, A. V., Cockayne, D. A., Xiang, Z., Ford, A. P., Spyer, K. M., et al. (2003). Pivotal role of nucleotide P2X2 receptor subunit of the ATP-gated ion channel mediating ventilatory responses to hypoxia. J. Neurosci. 23, 11315–11321. doi: 10.1523/JNEUROSCI.23-36-11315.2003
Sacramento, J. F., Olea, E., Ribeiro, M. J., Prieto-Lloret, J., Melo, B. F., Gonzalez, C., et al. (2019). Contribution of adenosine and ATP to the carotid body chemosensory activity in ageing. J. Physiol. 597, 4991–5008. doi: 10.1113/JP274179
Sheng, N., Yang, J., Silm, K., Edwards, R. H., and Nicoll, R. A. (2017). A slow excitatory postsynaptic current mediated by a novel metabotropic glutamate receptor in CA1 pyramidal neurons. Neuropharmacology 115, 4–9. doi: 10.1016/j.neuropharm.2016.08.028
Shigemoto, R., Kinoshita, A., Wada, E., Nomura, S., Ohishi, H., Takada, M., et al. (1997). Differential presynaptic localization of metabotropic glutamate receptor subtypes in the rat hippocampus. J. Neurosci. 17, 7503–7522. doi: 10.1523/JNEUROSCI.17-19-07503.1997
Shirahata, M., Hirasawa, S., Okumura, M., Mendoza, J. A., Okumura, A., Balbir, A., et al. (2004). Identification of M1 and M2 muscarinic acetylcholine receptors in the cat carotid body chemosensory system. Neuroscience 128, 635–644. doi: 10.1016/j.neuroscience.2004.06.068
Simonyi, A., Zhang, J. P., and Sun, G. Y. (1999). Changes in mRNA levels for group I metabotropic glutamate receptors following in utero hypoxia-ischemia. Brain Res. Dev. Brain Res. 112, 31–37. doi: 10.1016/s0165-3806(98)00152-7
Skeberdis, V. A., Lan, J., Opitz, T., Zheng, X., Bennett, M. V., and Zukin, R. S. (2001). mGluR1-mediated potentiation of NMDA receptors involves a rise in intracellular calcium and activation of protein kinase C. Neuropharmacology 40, 856–865. doi: 10.1016/s0028-3908(01)00005-3
Sladeczek, F., Momiyama, A., and Takahashi, T. (1993). Presynaptic inhibitory action of a metabotropic glutamate receptor agonist on excitatory transmission in visual cortical neurons. Proc. Biol. Sci. 253, 297–303. doi: 10.1098/rspb.1993.0117
Torrealba, F., Bustos, G., and Montero, V. M. (1996). Glutamate in the glomus cells of the cat carotid body: immunocytochemistry and in vitro release. Neurochem. Int. 28, 625–631. doi: 10.1016/0197-0186(95)00130-1
Traynelis, S. F., Wollmuth, L. P., McBain, C. J., Menniti, F. S., Vance, K. M., Ogden, K. K., et al. (2010). Glutamate receptor ion channels: structure, regulation, and function. Pharmacol. Rev. 62, 405–496. doi: 10.1124/pr.109.002451
Tremolizzo, L., Sala, G., Zoia, C. P., and Ferrarese, C. (2012). Assessing glutamatergic function and dysfunction in peripheral tissues. Curr. Med. Chem. 19, 1310–1315. doi: 10.2174/092986712799462702
Tse, A., Yan, L., Lee, A. K., and Tse, F. W. (2012). Autocrine and paracrine actions of ATP in rat carotid body. Can. J. Physiol. Pharmacol. 90, 705–711. doi: 10.1139/y2012-054
Varas, R., Alcayaga, J., and Iturriaga, R. (2003). ACh and ATP mediate excitatory transmission in cat carotid identified chemoreceptor units in vitro. Brain Res. 988, 154–163. doi: 10.1016/s0006-8993(03)03366-3
Vetrovoy, O., Stratilov, V., Nimiritsky, P., Makarevich, P., and Tyulkova, E. (2021). Prenatal hypoxia induces premature aging accompanied by impaired function of the glutamatergic system in rat hippocampus. Neurochem. Res. 46, 550–563. doi: 10.1007/s11064-020-03191-z
White, A. M., Kylänpää, R. A., Christie, L. A., McIntosh, S. J., Irving, A. J., and Platt, B. (2003). Presynaptic group I metabotropic glutamate receptors modulate synaptic transmission in the rat superior colliculus via 4-AP sensitive K(+) channels. Br. J. Pharmacol. 140, 1421–1433. doi: 10.1038/sj.bjp.0705570
Wittmann, M., Hubert, G. W., Smith, Y., and Conn, P. J. (2001). Activation of metabotropic glutamate receptor 1 inhibits glutamatergic transmission in the substantia nigra pars reticulata. Neuroscience 105, 881–889. doi: 10.1016/s0306-4522(01)00254-8
Xu, C., Zhang, W., Rondard, P., Pin, J. P., and Liu, J. (2014). Complex GABAB receptor complexes: how to generate multiple functionally distinct units from a single receptor. Front. Pharmacol. 5:12. doi: 10.3389/fphar.2014.00012
Xu, J., Xu, F., Tse, F. W., and Tse, A. (2005). ATP inhibits the hypoxia response in type I cells of rat carotid bodies. J. Neurochem. 92, 1419–1430. doi: 10.1111/j.1471-4159.2004.02978.x
Yokoyama, T., Nakamuta, N., Kusakabe, T., and Yamamoto, Y. (2014). Vesicular glutamate transporter 2-immunoreactive afferent nerve terminals in the carotid body of the rat. Cell Tissue Res. 358, 271–275. doi: 10.1007/s00441-014-1921-x
Zhang, M., Clarke, K., Zhong, H., Vollmer, C., and Nurse, C. A. (2009). Postsynaptic action of GABA in modulating sensory transmission in co-cultures of rat carotid body via GABA(A) receptors. J. Physiol. 587, 329–344. doi: 10.1113/jphysiol.2008.165035
Zhang, M., Vollmer, C., and Nurse, C. A. (2018). Adenosine and dopamine oppositely modulate a hyperpolarization-activated current I(h) in chemosensory neurons of the rat carotid body in co-culture. J. Physiol. 596, 3101–3117. doi: 10.1113/JP274743
Zhang, M., Zhong, H., Vollmer, C., and Nurse, C. A. (2000). Co-release of ATP and ACh mediates hypoxic signalling at rat carotid body chemoreceptors. J. Physiol. 525(Pt 1), 143–158. doi: 10.1111/j.1469-7793.2000.t01-1-00143.x
Keywords: carotid body, hypoxia, glutamate, group I metabotropic glutamate receptors, DHPG
Citation: Li C, Zhao B, Zhao C, Huang L and Liu Y (2021) Metabotropic Glutamate Receptors 1 Regulates Rat Carotid Body Response to Acute Hypoxia via Presynaptic Mechanism. Front. Neurosci. 15:741214. doi: 10.3389/fnins.2021.741214
Received: 21 July 2021; Accepted: 07 September 2021;
Published: 05 October 2021.
Edited by:
Rodrigo Del Rio, Pontifical Catholic University of Chile, ChileReviewed by:
Julio Alcayaga, University of Chile, ChileRobert Timothy Richard Huckstepp, University of Warwick, United Kingdom
Copyright © 2021 Li, Zhao, Zhao, Huang and Liu. This is an open-access article distributed under the terms of the Creative Commons Attribution License (CC BY). The use, distribution or reproduction in other forums is permitted, provided the original author(s) and the copyright owner(s) are credited and that the original publication in this journal is cited, in accordance with accepted academic practice. No use, distribution or reproduction is permitted which does not comply with these terms.
*Correspondence: Yuzhen Liu, eXV6aGVubGl1QHh4bXUuZWR1LmNu