- 1Department of Child Health Care, Children’s Hospital of Fudan University, National Children’s Medical Center, Shanghai, China
- 2Department of Neuroscience, University of Virginia, Charlottesville, VA, United States
Neuroimmune interactions have been studied for decades. Several neurodevelopmental disorders have been associated with immune dysfunction. However, the effects of immune system on neuronal function remain unknown. Herein, based on c-Fos protein expression, we characterized the brain areas that are activated after contextual fear conditioning (CFC) training or retrieval in severe combined immune deficiency (SCID) and wild-type mice. Further, we analyzed the interregional correlations of c-Fos activity that are affected by deficiency in adaptive immunity. Results showed significantly lower c-Fos density in learning and memory-associated brain regions of SCID mice after memory retrieval, but not during the CFC training. Moreover, SCID mice exhibited remarkably discordant interregional neuronal activities of learning neuron circuits after CFC training, which could be the cause of inefficient activation of the memory circuit after retrieval. These results provide a new perspective on how adaptive immunity affects neuronal function. Adaptive immune deficiency impairs the coordination of neural activity after training and retrieval, which might be a potential therapeutic target for neurodevelopmental disorders.
Introduction
A wide range of neurodevelopmental disorders, including autism spectrum disorder (Hughes et al., 2018) and neurodevelopmental delay (Bodnar et al., 2020), have been associated with immune system dysregulation. Advances in our understanding of neuroimmune interactions have led to fundamental changes in the concepts of immunology and neuroscience. The immune system is not self-regulated, but functions in close association with the nervous system (Dantzer, 2018). The nervous system regulates immune factors through neuroendocrine mediators and peripheral nerve endings innervating immune organs. Reciprocally, immune cells and mediators play a regulatory role in the nervous system and participate in the elimination and plasticity of synapses during development, as well as in synaptic plasticity during adulthood (Tanabe and Yamashita, 2018; Morimoto and Nakajima, 2019).
Studies have demonstrated that adaptive immunity is implicated in brain functions, including learning and memory retrieval (Brynskikh et al., 2008; Baruch and Schwartz, 2013; Radjavi et al., 2014). The first evidence came from experiments that examined the cognitive ability of wild-type (WT) and severe combined immune deficiency (SCID, deficient in both T cell and B cell responses) mice, by using the Morris water maze, a hippocampal-dependent visuospatial learning/memory task (Kipnis et al., 2004). SCID mice manifested significant impairment of spatial memory compared to their WT counterparts, and the brain function in SCID mice was restored when adaptive immunity was enhanced. Subsequently, researchers found that depletion of adaptive immune cells in naive mice also impairs spatial memory, supporting the notion that immunity plays a lifelong role in maintaining brain functions (Wolf et al., 2009; Derecki et al., 2010). However, the mechanism by which adaptive immunity affects memory is poorly understood.
Memory is a complex process through which information acquired during learning is stored. Synaptic and system changes are involved in the memory processes. The synaptic change is completed within hours of training and involves the stabilization of synaptic connectivity in localized circuits (Takeuchi et al., 2014). System changes are a more prolonged process that involves gradual reorganization of the brain regions that support memory (Frankland and Bontempi, 2005). Contextual fear conditioning (CFC) behavioral tests have been well studied as hippocampus-dependent spatial and associative learning tasks that activate hippocampal–cortical memory networks (Cowansage et al., 2014). Episodic memories initially require rapid synaptic plasticity within the hippocampus for their formation and are gradually consolidated in neocortical networks for permanent storage (Kitamura et al., 2017). Evidence shows that the hippocampus, amygdala, retrosplenial cortex (RSP), and prefrontal cortex are key regions supporting memory consolidation and retrieval processes (Tonegawa et al., 2018; de Sousa et al., 2019).
c-Fos is one of the first transcription factors whose induction was shown to be activity-dependent and is routinely used as an indicator of neuronal activation (Gallo et al., 2018; Silva et al., 2019). Fear memory paradigms have been shown to produce changes in c-Fos expression in different structures (Maviel et al., 2004; Lopez et al., 2012; Pignatelli et al., 2019). In this study, using c-Fos protein expression pattern, we characterized the brain areas that are activated in WT and SCID mice after CFC training or retrieval and evaluated the interregional correlations of c-Fos activity affected by adaptive immunity deficiency. Our results helped to uncover the immunity-neuron interaction and the potential therapy for neurodevelopmental disorders with immune dysfunction.
Materials and Methods
Mouse Strains and Housing
B6.CB17-Prkdcscid/SzJ (SCID, JAX 001913) and WT mice (C57BL/6J background) purchased from the Jackson Laboratory were maintained in the animal facility for at least 1 week prior to the start of the experiments. Mice were maintained and bred in-house under standard 12-h light–dark cycle conditions. They were given standard rodent chow and sterilized tap water ad libitum, unless stated otherwise. Male mice at 10–20 weeks of age were used for the behavioral experiments. Experimenters were blinded to the experimental groups during scoring and quantification. All experiments were approved by the Institutional Animal Care and Use Committee of the University of Virginia.
Contextual Fear Conditioning Training and Retrieval
The equipment used was purchased from Coulbourn Instruments (United States). Figure 1A illustrates the experimental design. During training, mice were introduced to a new context (test chamber), following which a stimulus was delivered in the form of a foot shock. The walls of the test chamber were painted white, and the chamber was scented with 0.25% benzaldehyde. Each mouse was placed in the test chamber for 3 min. After 3 min, electric foot shock (2 s, 0.50 mA) was delivered thrice during 198–200, 258–260, and 318–320 s. The mice were left in the test chamber for an additional 30 s before they were returned to their home cages.
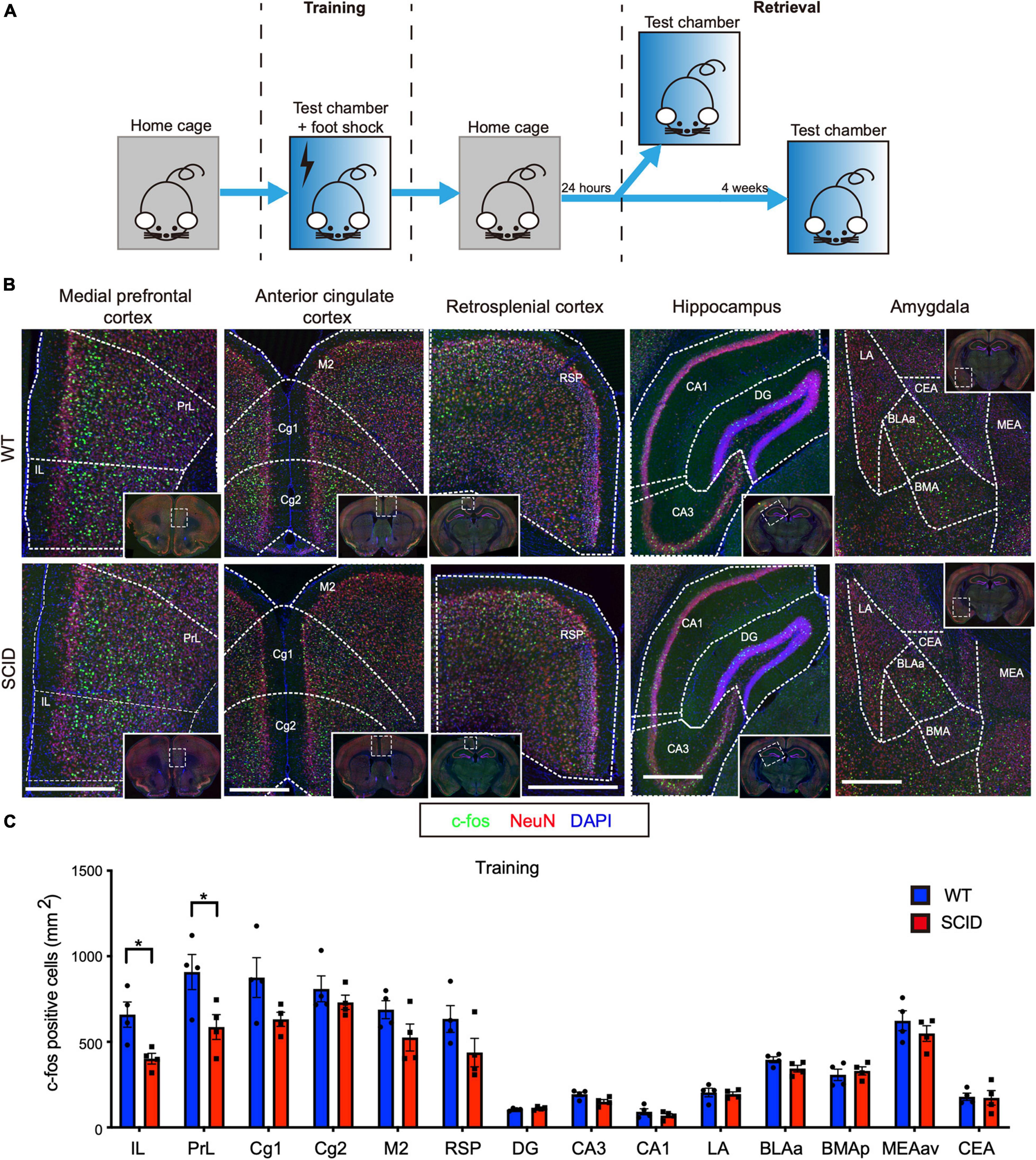
Figure 1. Limited differences in c-Fos expression between SCID and WT mice brain after contextual fear conditioning training. (A) General scheme of contextual fear conditioning training and retrieval. (B) Representative immunofluorescence images of the brain sections from WT (top) and SCID (bottom) mice 90 min after CFC training. c-Fos is in green, NeuN in red, and DAPI in blue. Brain regions: medial prefrontal cortex, anterior cingulate cortex, retrosplenial cortex, hippocampus, and amygdala. Scale bar, 500 μm. Low-power images were presented in the white solid line frames, with white dotted frames that showed the regions zoomed in. White dotted line outlined most of the brain regions for quantification. (C) c-Fos–positive cell density in different brain regions of WT and SCID mice (n = 4, data represent mean ± SEM, *p < 0.05).
At 1 day or 4 weeks posttraining, CFC-trained mice were returned to the test chamber for memory retrieval. The test at 1 day posttraining was referred to as recent retrieval. The test at 4 weeks posttraining was called remote retrieval. Mice were kept in the test cage for 3 min and returned back to their home cage without delivering the electric foot shock.
Immunohistochemistry
Mice were euthanized by Euthasol overdose (10% vol/vol in saline, intraperitoneally) at 90 min (for c-Fos) and 60 min (for Arc) after training or retrieval. The animals were transcardially perfused with ice-cold phosphate-buffered saline (PBS) containing heparin (10 U mL–1) followed by 4% paraformaldehyde (PFA). Brains were dissected out and kept in 4% PFA overnight at 4°C. The fixed brains were washed with PBS and cryoprotected by immersing them in 30% sucrose solution for 48 h at 4°C. The fixed brain tissue was frozen in Tissue-Plus OCT compound (Thermo Scientific) and sliced into 40-μm-thick free-floating coronal sections using a cryostat (Leica). Brain sections were stored in PBS containing 0.02% sodium azide at 4°C until further use.
Brain sections were blocked with 1% bovine serum albumin (BSA), 2% normal serum (either goat or chicken), 0.2% Triton X-100, and 0.1% Tween 20 in PBS for 1 h at room temperature (RT). Sections were then incubated with appropriate primary antibodies in PBS containing 5% BSA and 0.2% Triton X-100 overnight at 4°C. The primary antibodies used were as follows: rabbit anti-c-Fos (1:1,000, Synaptic Systems, 226003), mouse anti-NeuN (1:300; Millipore, MAB377, clone A60), or rabbit anti-Arc (1:100, Santa Cruz, sc-15325). Brain sections were then washed three times for 15 min at RT with PBS containing 0.2% Triton X-100 and 0.1% Tween 20. This was followed by incubation at RT for 1 or 1.5 h with species-matched fluorescently conjugated secondary antibodies (1:1,000, Invitrogen) diluted in PBS containing 0.2% Triton X-100. Nuclei were stained with 4′,6-diamidino-2-phenylindole (DAPI, 1:10,000, Sigma-Aldrich) at RT for 15 min. The sections were washed with PBS three times for 15 min and mounted with Aqua-Mount (Thermo Scientific TA-125-AM) under coverslips.
Image Acquisition and Analysis
For detecting c-Fos–positive cells, samples from the same experiment were stained and analyzed simultaneously using same settings on the Olympus FV1200 laser scanning confocal microscope with a 10× objective and 0.40 NA. Quantification of c-Fos expression was performed using the Fiji software (NIH). The following brain regions were analyzed using specific coordinates: infralimbic cortex (IL) and prelimbic cortex (PrL) at +1.98 to +1.54 mm from bregma; cingulate cortex (Cg1, Cg2, and M2) at +1.10 to +0.26 mm from bregma; hippocampus and RSP at −1.58 to −2.06 mm from bregma; and amygdala at −1.46 to −2.06 mm from bregma. Brain areas were manually outlined based on the DAPI and NeuN signals following the Allen Brain Reference Atlas and measured automatically by Fiji. The c-Fos–positive nuclei for each brain region were counted using the “Analyze Particles” tool in Fiji. Only the c-Fos–positive nuclei within a specific size range were counted, and a constant threshold level of staining was used to distinguish c-Fos–positive cells as follows: a fluorescence area between 18.07 and 117.78 μm2 and mean intensity greater than 60 (arbitrary units) were counted. This method has been described previously (Lopatina et al., 2014; Zhong et al., 2014). The density of c-Fos–positive cells (c-Fos per mm2) in each brain region was averaged from three sections per animal and at least four mice for each group.
Statistical Analysis
Statistical analysis was performed using Prism 8.0 (GraphPad Software, Inc.). Comparison between two groups was performed using a two-tailed unpaired Student t-test. Pearson correlation coefficients were calculated for pairwise comparisons of c-Fos density among all 14 brain regions analyzed. Correlations were displayed as a color-coded correlation matrix generated using a custom R-code (R version 3.6.3).
Results
c-Fos Expression in Severe Combined Immune Deficiency and Wild-Type Mice After Contextual Fear Conditioning Training
Contextual fear conditioning behavioral test, a hippocampus-dependent spatial and associative learning/memory task, was used to compare the spatial learning/memory of SCID and WT mice. The CFC training was performed by transferring each mouse from the home cage to the test chamber where the animals received an electric foot shock (Figure 1A). SCID and WT mice were euthanized 90 min after CFC training. To investigate the brain regions that are activated during the training, brain slices were stained for c-Fos and NeuN. We quantified the density of c-Fos–positive cells in 14 brain regions that are known to play a crucial role in contextual fear learning and memory (Tovote et al., 2015). Figure 1B shows the density of c-Fos–positive cells in the IL, PrL, anterior cingulate cortex (Cg1, Cg2, and M2), RSP, hippocampus (DG, CA1, and CA3), and amygdala.
Although strong c-Fos expression was observed in these 14 brain regions of SCID mice, c-Fos expression was slightly higher in the WT mice brain. However, significant differences were observed only in the IL and PrL (p < 0.05, Figure 1C). IL and PrL regions have well-established roles in the fear memory circuit (Frankland and Bontempi, 2005).
Inefficient c-Fos Expression in Severe Combined Immune Deficiency Mice Following Memory Retrieval
To assess brain activity during recent memory retrieval, CFC-trained SCID and WT mice were transferred to the test cage at 1 day post-CFC training (Figure 1A). In the retrieval experiment, no electric shock was given to the animals. The animals were euthanized 90 min later, and c-Fos expression in the brain was evaluated (Figure 2A). Significant differences were observed in the hippocampus (DG, CA1, and CA3; p < 0.001, p < 0.05, and p < 0.05, respectively) and RSP (p < 0.01) (Figure 2B). Furthermore, the density of Acr (another immediate early gene)–positive cells at RSP and DG regions was lower in SCID mice than WT, which was consistent with c-Fos expression (p < 0.05) (Figures 2C,D).
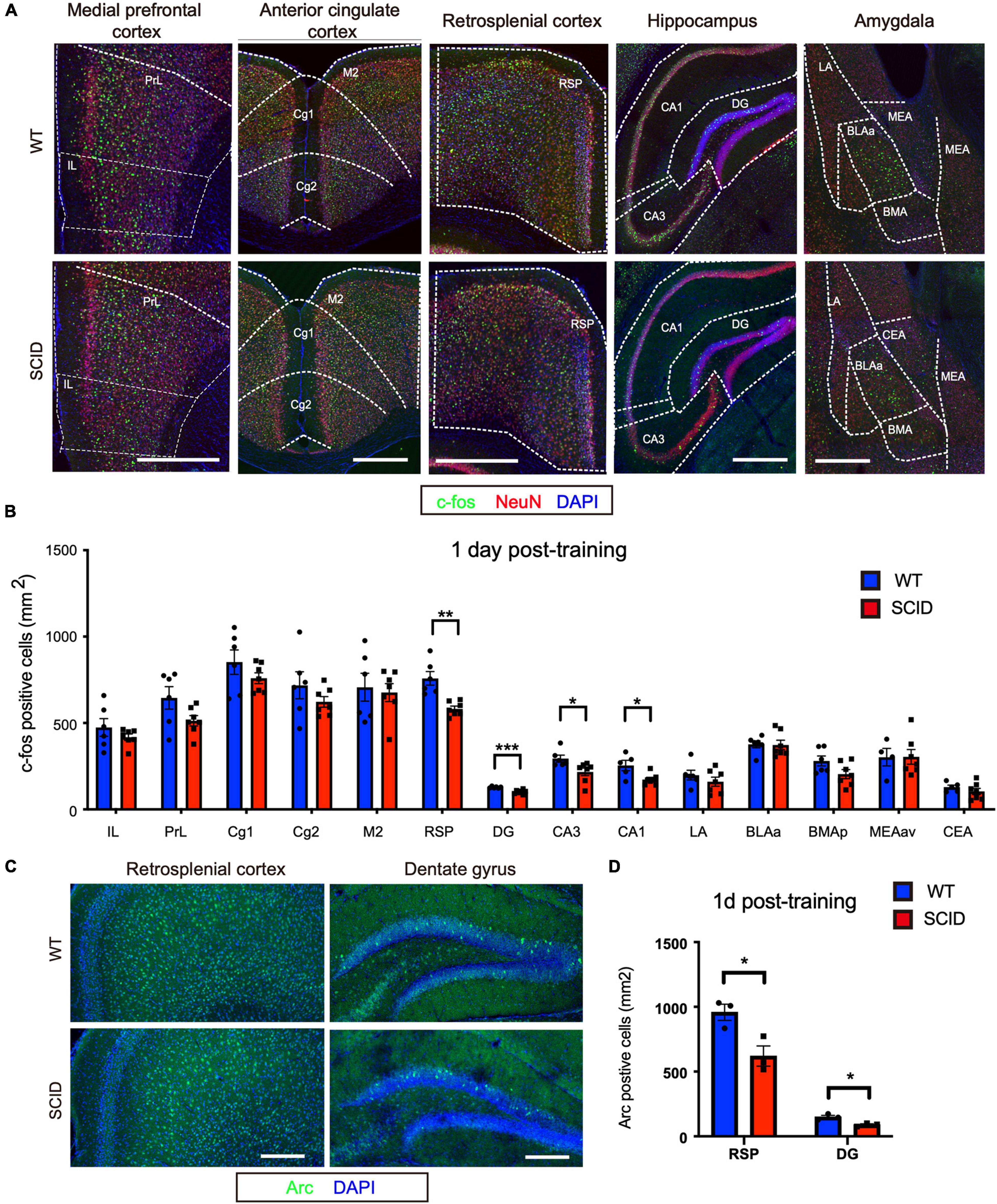
Figure 2. Inefficient neuronal activity in the hippocampus and retrosplenial cortex of SCID mice after recent memory retrieval. (A) Representative immunofluorescence images of five brain regions from WT and SCID mice after recent memory retrieval (1 day after CFC training). c-Fos is in green, NeuN in red, and DAPI in blue. Scale bar, 500 μm. (B) c-Fos–positive cell density in different brain regions of WT and SCID mice after recent memory retrieval (n = 6–7 per group, data represent mean ± SEM, *p < 0.05). (C) Representative immunofluorescence images of retrosplenial cortex and dentate gyrus from WT and SCID mice 60 min after recent memory retrieval. Arc is in green, and DAPI in blue. Scale bar, 200 μm. (D) Quantified Acr-positive cells at RSP and DG regions after recent memory retrieval (n = 3 per group; data represent mean ± SEM, *p < 0.05, **p < 0.01, ***p < 0.001).
Remote memory retrieval was performed at 4 weeks post-CFC training (Figure 1A). CFC-trained SCID and WT mice were placed in the test cage without giving the electric shock. Results showed significantly lower c-Fos levels in SCID mice, especially in the medial PrL, RSP, and CA1 (Figures 3A,B).
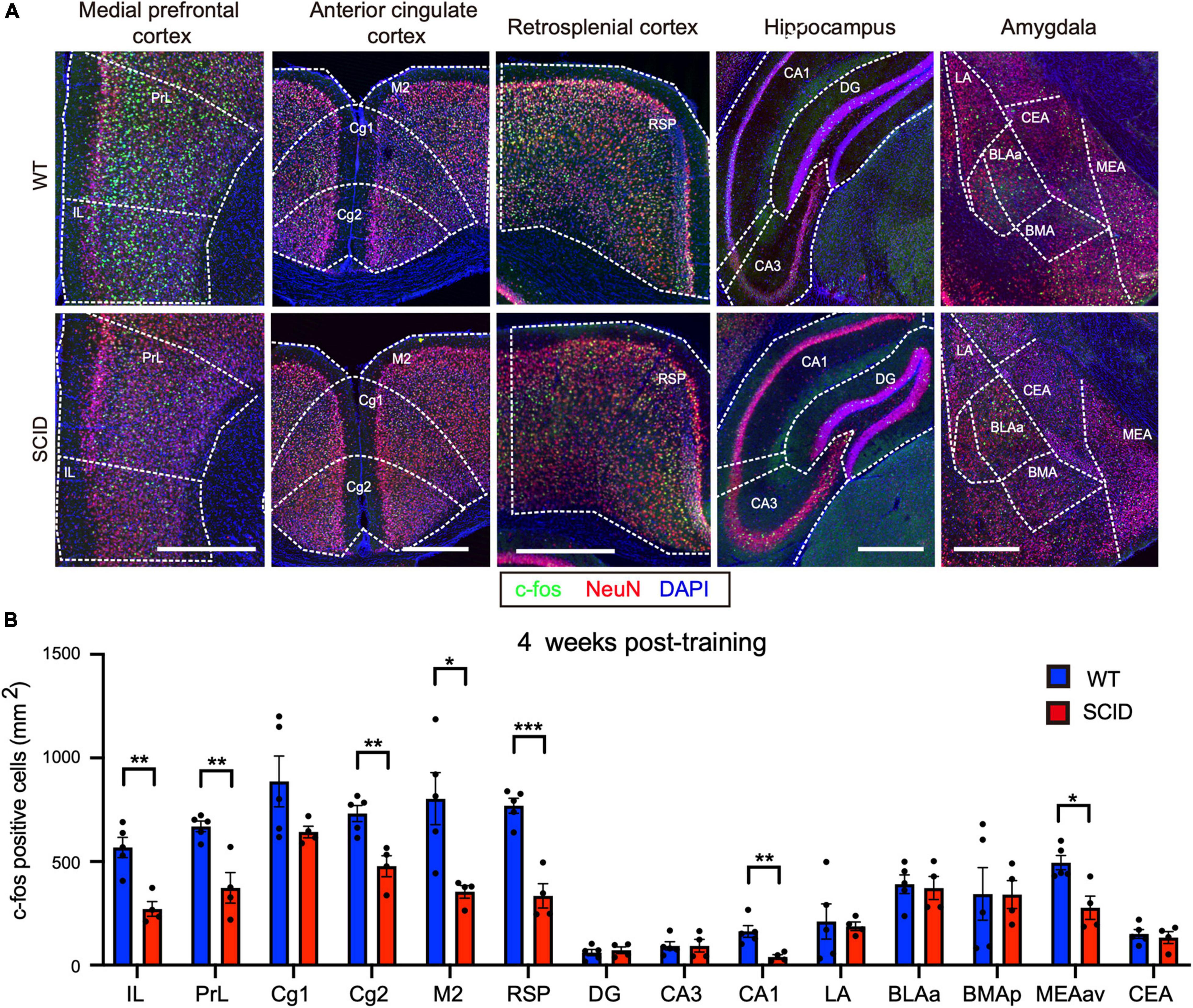
Figure 3. Significantly lower neuronal activity in memory-associated brain regions of SCID mice after remote memory retrieval. (A) Representative immunofluorescence images of five different brain regions from WT and SCID mice after remote memory retrieval (4 weeks after CFC training). c-Fos is in green, NeuN in red, and DAPI in blue. Scale bar, 500 μm. (B) c-Fos–positive cell density in the brain regions of WT and SCID mice after remote memory retrieval (n = 4–5 per group; data represent mean ± SEM, *p < 0.05, **p < 0.01, ***p < 0.001).
Lack of Adaptive Immunity Impairs the Interregional Activation of Learning and Memory Neuron Circuits
To gain insight into the functional connections within the learning and memory neuron circuits, we computed the correlation between each pair of brain regions in each group. The interregional correlation matrix for each experimental group led to the identification of sets of regions whose c-Fos density covaried across mice.
Results showed that in WT mice after CFC training, there was a strong connection between the brain regions that are important for learning and memory (Figure 4A, left). However, SCID mice showed remarkably discordant activation between the hippocampus–prefrontal cortex, hippocampus–amygdala, and prefrontal cortex–amygdala (Figure 4A, right). With the withdrawal of DG activation in memory retrieval, the prefrontal cortex to process memories might mirror that of the hippocampus to process recent memories. Inefficient activation of the cortex regions during the CFC training affected the memory retrieval, especially remote memory retrieval (Figures 4B,C).
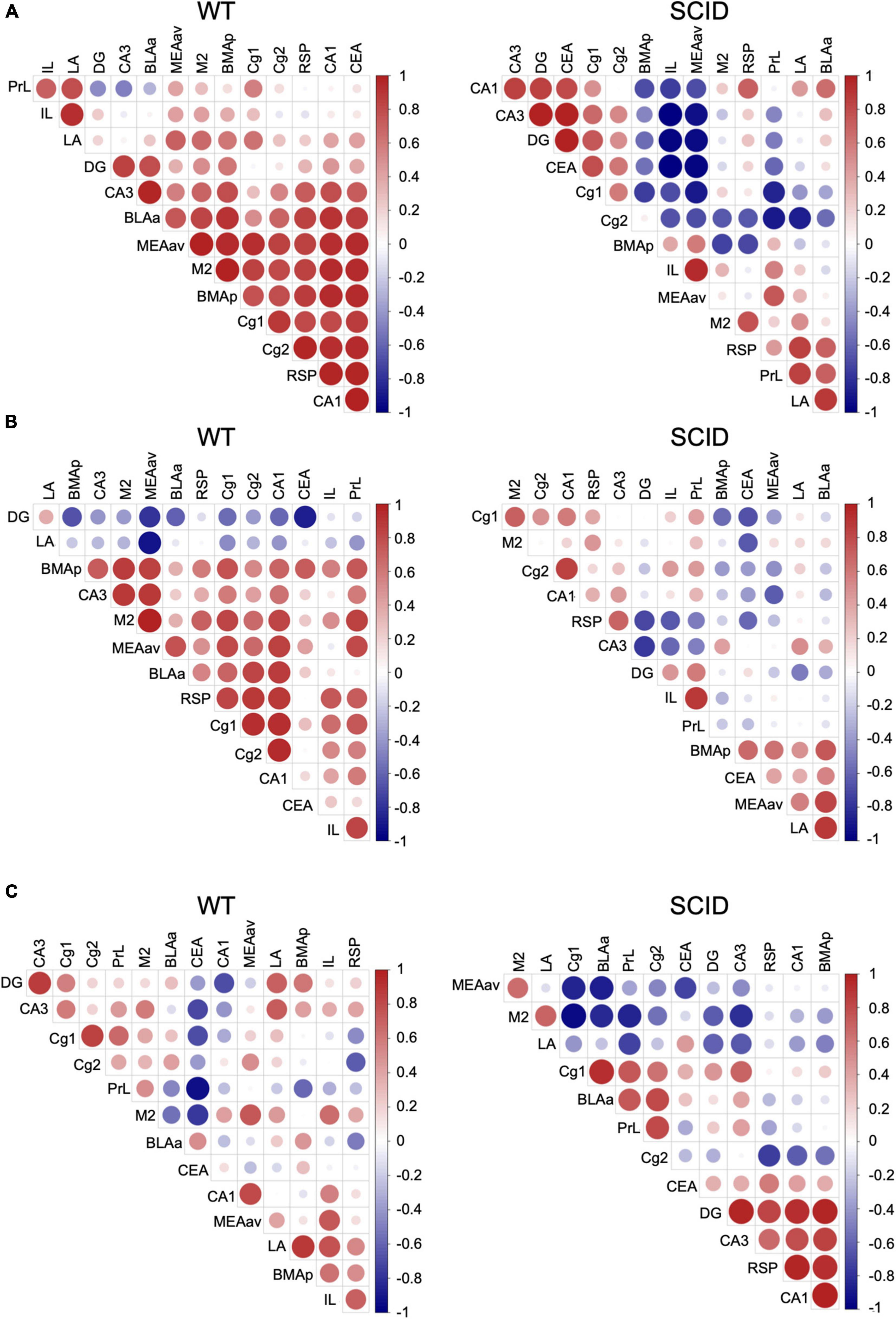
Figure 4. Variations in interregional correlation of c-Fos activation between WT and SCID mice. Pearson correlation matrices showing interregional correlations of c-Fos activation density. Axes represent the brain regions. The different colors represent Pearson correlation coefficients (scale, right). Panel (A) is for WT and SCID mice after the CFC training. Panel (B) represents WT and SCID mice after recent retrieval. Panel (C) represents WT and SCID mice after remote retrieval.
Discussion
To assess brain function and memory processes in mice lacking adaptive immunity, we compared c-Fos expression in 14 brain regions of SCID and WT mice that are known to be involved in the acquisition of contextual memories. IL and PrL showed remarkably lower c-Fos expression during learning in SCID mice. In recent memory retrieval test at 1 day post-CFC training, remarkable differences in c-Fos density in the RSP and hippocampus were observed between SCID and WT mice. In the remote memory retrieval test at 4 weeks post-CFC training, the prefrontal cortex, RSP, CA1, and medial nuclei of the amygdala of the SCID mice showed significantly lower c-Fos expression than WT mice.
Memories are thought to be initially stored within the hippocampal–amygdala network (recent memory) and, over time, slowly consolidate within the neocortex for permanent storage (remote memory) (Frankland and Bontempi, 2005; Lopez et al., 2012; Tonegawa et al., 2018; Todd et al., 2019). Consistent with previous studies, our study found that in WT mice, the DG-CA3 hippocampal network was active during learning and recent memory retrieval (1 day posttraining), whereas it was not activated by remote memory retrieval (4 weeks posttraining). Hippocampal CA1 activity was involved in both recent and remote memory retrieval. In our study, significantly lower neuronal activity was observed in the hippocampus and neocortex of the SCID mice during memory retrieval.
Furthermore, interregional activation patterns showed that SCID mice have abnormalities in neural circuits, which might be the cause of memory dysfunctions. The low neuronal activity in SCID mice was limited to IL and PrL regions during the learning stage. However, remarkable discordant activation was observed between the hippocampus–prefrontal cortex, hippocampus–amygdala, and prefrontal cortex–amygdala. IL and PrL, as part of the prefrontal cortex, are highly interconnected with the hippocampus, sensory cortex, motor cortex, and limbic cortex; therefore, they are ideally suited to integrate and synthesize information from a large number of different sources (Euston et al., 2012). Kitamura et al. (2017) found that neocortical prefrontal memory engram cells are rapidly generated during initial learning through inputs from both the hippocampus and the basolateral amygdala and became functionally mature with time, which is critical for remote contextual fear memory. The insufficient activation of IL and PrL by the hippocampus–prefrontal cortex circuitry does not affect the learning ability of SCID mice, but could have a potential effect on memory retrieval. The importance of interregional coactivation in neuron circuits has been demonstrated using chemogenetic neuronal inhibition and computational modeling (Kitamura et al., 2017; Roy et al., 2017; Pignatelli et al., 2019).
The findings of this study have to be seen in light of some limitations. In the CFC behavior test, SCID mice did not show significant difference for freezing time between genders. Considering stable behavior phenotype, we used only male mice in the study. However, immune deficiency and neurodevelopmental disorders affect both male and female. It is better to include female mice in a future study. The second limitation concerns that the study did not provide mechanistic insights into the impairments of neural activity in SCID mice after training and retrieval. Further studies will be aimed at understanding the molecular basis that underlies how adaptive immunity impairs neural activity.
Overall, our results provide new details about how adaptive immunity affects neuronal function. Mice lacking components of adaptive immunity have problems with neuronal activation and interregional coordination of neuron circuits during training and memory retrieval. Our results uncover the neural–immune interactions and will help in developing novel therapies for neurodevelopmental disorders due to immune dysfunction.
Data Availability Statement
The raw data supporting the conclusions of this article will be made available by the authors, without undue reservation.
Ethics Statement
The animal study was reviewed and approved by the Institutional Animal Care and Use Committee of the University of Virginia.
Author Contributions
HL performed and analyzed IHC experiments and took the lead in writing the manuscript. ZF performed the behavioral tests. MH took part in c-Fos data analysis. XX conceived the project and provided advice. All authors provided feedback and contribution to the final manuscript.
Funding
This work was supported by the National Key Research and Development Program of China (No. 2016YFC1306205) and Key Subject Construction Project of Shanghai Municipal Health Commission (No. shslczdzk02903).
Conflict of Interest
The authors declare that the research was conducted in the absence of any commercial or financial relationships that could be construed as a potential conflict of interest.
Publisher’s Note
All claims expressed in this article are solely those of the authors and do not necessarily represent those of their affiliated organizations, or those of the publisher, the editors and the reviewers. Any product that may be evaluated in this article, or claim that may be made by its manufacturer, is not guaranteed or endorsed by the publisher.
Acknowledgments
We thank Jiawei Lu and Yunzhou Huang (Lu Ming Biotech Co., Ltd., Shanghai, China) for assistance with the analysis of the Pearson correlation coefficients. We would like to thank Editage (www.editage.com) for English language editing.
References
Baruch, K., and Schwartz, M. (2013). CNS-specific T cells shape brain function via the choroid plexus. Brain Behav. Immun. 34, 11–16. doi: 10.1016/j.bbi.2013.04.002
Bodnar, T. S., Raineki, C., Wertelecki, W., Yevtushok, L., Plotka, L., Granovska, I., et al. (2020). Immune network dysregulation associated with child neurodevelopmental delay: modulatory role of prenatal alcohol exposure. J. Neuroinflammation 17:39. doi: 10.1186/s12974-020-1717-8
Brynskikh, A., Warren, T., Zhu, J., and Kipnis, J. (2008). Adaptive immunity affects learning behavior in mice. Brain Behav. Immun. 22, 861–869. doi: 10.1016/j.bbi.2007.12.008
Cowansage, K. K., Shuman, T., Dillingham, B. C., Chang, A., Golshani, P., and Mayford, M. (2014). Direct reactivation of a coherent neocortical memory of context. Neuron 84, 432–441. doi: 10.1016/j.neuron.2014.09.022
Dantzer, R. (2018). Neuroimmune interactions: from the brain to the immune system and vice versa. Physiol. Rev. 98, 477–504. doi: 10.1152/physrev.00039.2016
de Sousa, A. F., Cowansage, K. K., Zutshi, I., Cardozo, L. M., Yoo, E. J., Leutgeb, S., et al. (2019). Optogenetic reactivation of memory ensembles in the retrosplenial cortex induces systems consolidation. Proc. Natl. Acad. Sci. U.S.A. 116, 8576–8581. doi: 10.1073/pnas.1818432116
Derecki, N. C., Cardani, A. N., Yang, C. H., Quinnies, K. M., Crihfield, A., Lynch, K. R., et al. (2010). Regulation of learning and memory by meningeal immunity: a key role for IL-4. J. Exp. Med. 207, 1067–1080. doi: 10.1084/jem.20091419
Euston, D. R., Gruber, A. J., and McNaughton, B. L. (2012). The role of medial prefrontal cortex in memory and decision making. Neuron 76, 1057–1070. doi: 10.1016/j.neuron.2012.12.002
Frankland, P. W., and Bontempi, B. (2005). The organization of recent and remote memories. Nat. Rev. Neurosci. 6, 119–130. doi: 10.1038/nrn1607
Gallo, F. T., Katche, C., Morici, J. F., Medina, J. H., and Weisstaub, N. V. (2018). Immediate early genes, memory and psychiatric disorders: focus on c-Fos, Egr1 and Arc. Front. Behav. Neurosci. 12:79. doi: 10.3389/fnbeh.2018.00079
Hughes, H. K., Mills Ko, E., Rose, D., and Ashwood, P. (2018). Immune dysfunction and autoimmunity as pathological mechanisms in autism spectrum disorders. Front. Cell Neurosci. 12:405. doi: 10.3389/fncel.2018.00405
Kipnis, J., Cohen, H., Cardon, M., Ziv, Y., and Schwartz, M. (2004). T cell deficiency leads to cognitive dysfunction: implications for therapeutic vaccination for schizophrenia and other psychiatric conditions. Proc. Natl. Acad. Sci. U.S.A. 101, 8180–8185. doi: 10.1073/pnas.0402268101
Kitamura, T., Ogawa, S. K., Roy, D. S., Okuyama, T., Morrissey, M. D., Smith, L. M., et al. (2017). Engrams and circuits crucial for systems consolidation of a memory. Science 356, 73–78. doi: 10.1126/science.aam6808
Lopatina, O., Yoshihara, T., Nishimura, T., Zhong, J., Akther, S., Fakhrul, A. A., et al. (2014). Anxiety- and depression-like behavior in mice lacking the CD157/BST1 gene, a risk factor for Parkinson’s disease. Front. Behav. Neurosci. 8:133. doi: 10.3389/fnbeh.2014.00133
Lopez, J., Herbeaux, K., Cosquer, B., Engeln, M., Muller, C., Lazarus, C., et al. (2012). Context-dependent modulation of hippocampal and cortical recruitment during remote spatial memory retrieval. Hippocampus 22, 827–841. doi: 10.1002/hipo.20943
Maviel, T., Durkin, T. P., Menzaghi, F., and Bontempi, B. (2004). Sites of neocortical reorganization critical for remote spatial memory. Science 305, 96–99. doi: 10.1126/science.1098180
Morimoto, K., and Nakajima, K. (2019). Role of the immune system in the development of the central nervous system. Front. Neurosci. 13:916. doi: 10.3389/fnins.2019.00916
Pignatelli, M., Ryan, T. J., Roy, D. S., Lovett, C., Smith, L. M., Muralidhar, S., et al. (2019). Engram cell excitability state determines the efficacy of memory retrieval. Neuron 101, 274.e275–284.e275. doi: 10.1016/j.neuron.2018.11.029
Radjavi, A., Smirnov, I., and Kipnis, J. (2014). Brain antigen-reactive CD4+ T cells are sufficient to support learning behavior in mice with limited T cell repertoire. Brain Behav. Immun. 35, 58–63. doi: 10.1016/j.bbi.2013.08.013
Roy, D. S., Kitamura, T., Okuyama, T., Ogawa, S. K., Sun, C., Obata, Y., et al. (2017). Distinct neural circuits for the formation and retrieval of episodic memories. Cell 170, 1000.e1019–1012.e1019. doi: 10.1016/j.cell.2017.07.013
Silva, B. A., Burns, A. M., and Graff, J. (2019). A cFos activation map of remote fear memory attenuation. Psychopharmacology 236, 369–381. doi: 10.1007/s00213-018-5000-y
Takeuchi, T., Duszkiewicz, A. J., and Morris, R. G. (2014). The synaptic plasticity and memory hypothesis: encoding, storage and persistence. Philos. Trans. R. Soc. Lond. B Biol. Sci. 369:20130288. doi: 10.1098/rstb.2013.0288
Tanabe, S., and Yamashita, T. (2018). The role of immune cells in brain development and neurodevelopmental diseases. Int. Immunol. 30, 437–444. doi: 10.1093/intimm/dxy041
Todd, T. P., Fournier, D. I., and Bucci, D. J. (2019). Retrosplenial cortex and its role in cue-specific learning and memory. Neurosci. Biobehav. Rev. 107, 713–728. doi: 10.1016/j.neubiorev.2019.04.016
Tonegawa, S., Morrissey, M. D., and Kitamura, T. (2018). The role of engram cells in the systems consolidation of memory. Nat. Rev. Neurosci. 19, 485–498. doi: 10.1038/s41583-018-0031-2
Tovote, P., Fadok, J. P., and Luthi, A. (2015). Neuronal circuits for fear and anxiety. Nat. Rev. Neurosci. 16, 317–331. doi: 10.1038/nrn3945
Wolf, S. A., Steiner, B., Akpinarli, A., Kammertoens, T., Nassenstein, C., Braun, A., et al. (2009). CD4-positive T lymphocytes provide a neuroimmunological link in the control of adult hippocampal neurogenesis. J. Immunol. 182, 3979–3984. doi: 10.4049/jimmunol.0801218
Keywords: adaptive immune, c-Fos, neural activity, contextual fear conditioning, neurodevelopmental disorders
Citation: Li H, Fu Z, Hu M and Xu X (2021) Adaptive Immune Deficiency Impairs Neural Activity After Training and Retrieval. Front. Neurosci. 15:739580. doi: 10.3389/fnins.2021.739580
Received: 11 July 2021; Accepted: 18 October 2021;
Published: 15 November 2021.
Edited by:
Xiaoyan Ke, Nanjing Brain Hospital Affiliated to Nanjing Medical University, ChinaReviewed by:
Cesar Canales, University of California, Davis, United StatesEmanuela Zuccaro, University of Padua, Italy
Copyright © 2021 Li, Fu, Hu and Xu. This is an open-access article distributed under the terms of the Creative Commons Attribution License (CC BY). The use, distribution or reproduction in other forums is permitted, provided the original author(s) and the copyright owner(s) are credited and that the original publication in this journal is cited, in accordance with accepted academic practice. No use, distribution or reproduction is permitted which does not comply with these terms.
*Correspondence: Huiping Li, bGlodWlwaW5nQGZ1ZGFuLmVkdS5jbg==; Xiu Xu, eHV4aXVAZnVkYW4uZWR1LmNu