- 1Department of Neurosurgery, Maxine Dunitz Neurosurgical Research Institute, Cedars-Sinai Medical Center, Los Angeles, CA, United States
- 2Department of Biomedical Sciences, Cedars-Sinai Medical Center, Los Angeles, CA, United States
The retina has been increasingly investigated as a site of Alzheimer’s disease (AD) manifestation for over a decade. Early reports documented degeneration of retinal ganglion cells and their axonal projections. Our group provided the first evidence of the key pathological hallmarks of AD, amyloid β-protein (Aβ) plaques including vascular Aβ deposits, in the retina of AD and mild cognitively impaired (MCI) patients. Subsequent studies validated these findings and further identified electroretinography and vision deficits, retinal (p)tau and inflammation, intracellular Aβ accumulation, and retinal ganglion cell-subtype degeneration surrounding Aβ plaques in these patients. Our data suggest that the brain and retina follow a similar trajectory during AD progression, probably due to their common embryonic origin and anatomical proximity. However, the retina is the only CNS organ feasible for direct, repeated, and non-invasive ophthalmic examination with ultra-high spatial resolution and sensitivity. Neurovascular unit integrity is key to maintaining normal CNS function and cerebral vascular abnormalities are increasingly recognized as early and pivotal factors driving cognitive impairment in AD. Likewise, retinal vascular abnormalities such as changes in vessel density and fractal dimensions, blood flow, foveal avascular zone, curvature tortuosity, and arteriole-to-venule ratio were described in AD patients including early-stage cases. A rapidly growing number of reports have suggested that cerebral and retinal vasculopathy are tightly associated with cognitive deficits in AD patients and animal models. Importantly, we recently identified early and progressive deficiency in retinal vascular platelet-derived growth factor receptor-β (PDGFRβ) expression and pericyte loss that were associated with retinal vascular amyloidosis and cerebral amyloid angiopathy in MCI and AD patients. Other studies utilizing optical coherence tomography (OCT), retinal amyloid-fluorescence imaging and retinal hyperspectral imaging have made significant progress in visualizing and quantifying AD pathology through the retina. With new advances in OCT angiography, OCT leakage, scanning laser microscopy, fluorescein angiography and adaptive optics imaging, future studies focusing on retinal vascular AD pathologies could transform non-invasive pre-clinical AD diagnosis and monitoring.
Introduction
Alzheimer’s disease (AD) is the leading cause of senile dementia, accounting for 60–80% of total cases (Alzheimer’s Association, 2020). By 2050, over six million Americans are projected to live with AD, which could lead to a staggering $355 billion national financial burden (National Institue on Aging, 2019; Alzheimer’s Association, 2020). AD patients progressively develop irreversible cognitive loss due to neurodegeneration in the brain and other direct or indirect factors such as accumulation of toxic molecules, neuroinflammation, and vascular damage. The main pathological hallmarks of AD are amyloid β-protein (Aβ) accumulation and neurofibrillary tangles, mainly composed of hyperphosphorylated (p)tau deposits, that may exist inside or outside of neurons and in blood vessels (Bloom, 2014; Cisternas et al., 2019). Our group identified these hallmarks in the retina of postmortem and living AD and mild cognitively impaired (MCI) patients (Koronyo-Hamaoui et al., 2011; La Morgia et al., 2016; Koronyo et al., 2017). Investigation of CNS and fluid biomarkers has become an essential part of AD research. In 2018, the National Institute on Aging and Alzheimer’s Association (NIA-AA) created an updated research framework for classifying pathological phases of AD based on detection of abnormal levels of molecular biomarkers Aβ (A), tau (T), and neurodegeneration [AT(N)], regardless of cognitive status in living patients (Jack et al., 2018). The ATN framework was also proposed to be expandable to include new AD biomarkers such as vascular biomarkers (ATNV) (Jack et al., 2018).
Vascular pathology in AD is an expanding subject and a growing number of studies show that vascular-related damage in the brain and retina can predict cognitive decline (Vidal and Mavet, 1989; Baker et al., 2007; Gharbiya et al., 2014; Boyle et al., 2015; Bulut et al., 2016, 2018; Cunha et al., 2017; McGrory et al., 2017; Planton et al., 2017; Cabrera DeBuc et al., 2018; Deal et al., 2018; Jiang et al., 2018; O’Bryhim et al., 2018; van der Flier et al., 2018; Iadecola et al., 2019; Jung et al., 2019; Montagne et al., 2020; Shi et al., 2020a; Li et al., 2021). Cerebral vascular damage such as ischemia leads to disturbed nutrient supply, induces oxidative stress and inflammatory activities, impedes Aβ clearance and/or alters amyloid-processing enzymes (Marchesi, 2011), all of which can contribute to neurodegeneration and cognitive decline. Studies have also proposed that the onset of clinical dementia may be preceded by reduced cerebral blood flow associated with insufficient Aβ clearance (Wolters et al., 2017; Govindpani et al., 2019). With new disease-modifying therapies on the horizon and emphasizing the need for early intervention (Tonda-Turo et al., 2018), the current challenge is to diagnose AD early and accurately in the clinical setting to allow for an effective outcome that could limit the damage and prevent further disease progression.
Vascular Damage in AD Brain
The brain is nourished by one of the human body’s richest networks of blood vessels (Prensa, 2014), rendering its vascular network highly susceptible to aging and AD-related cerebral damage. Studies indicate that AD pathology is associated with severe effects on cerebral blood vessels, potentially by a wide range of complications (Govindpani et al., 2019). These include cerebral amyloid angiopathy (CAA) (Ellis et al., 1996; Arvanitakis et al., 2011; Viswanathan and Greenberg, 2011), vascular non-perfusion (Bonte et al., 1986; Hirsch et al., 1997; Binnewijzend et al., 2016), neurovascular unit (NVU) uncoupling and degeneration (Higuchi et al., 1987; Vinters et al., 1994; Claudio, 1996), angiogenesis (Desai et al., 2009; Biron et al., 2011), small blood vessel distortions (Hassler, 1965; Beskow et al., 1971; Fischer et al., 1990; Kalaria and Kroon, 1992), blood–brain barrier (BBB) breakdown and damage (Slemmon et al., 1994; Zipser et al., 2007; Bell and Zlokovic, 2009; Ryu and McLarnon, 2009; Sengillo et al., 2013; van de Haar et al., 2016a, b), vascular tau accumulation (Williams et al., 2005; Castillo-Carranza et al., 2017), dysregulated glucose metabolism (Kalaria and Harik, 1989; Harik, 1992), inflammation (Grammas and Ovase, 2001; Tripathy et al., 2007), hypertension (Launer, 2002; Gabin et al., 2017), hypercholesterolemia (Matsuzaki et al., 2011), and atherosclerosis (Alzheimer, 1911; Yarchoan et al., 2012).
Amyloid plaques are the most considerable hallmarks of AD, with 42 and 40 amino acid-long Aβ alloforms tightly associated with AD pathogenesis and vascular pathology (Blennow et al., 2015; Selkoe and Hardy, 2016). Nearly 85% of AD patients develop varying degrees of CAA complications (Arvanitakis et al., 2011; Viswanathan and Greenberg, 2011), defined by Aβ deposits inside walls of arteries, arterioles and capillaries (DeSimone et al., 2017). Accumulation of Aβ within blood vessels is associated with damage to muscular and elastic tissue, possibly replaced by Aβ fibrils, leading to lobar cerebral hemorrhage (ICH) or vascular non-perfusion (Mehndiratta et al., 2012; Keable et al., 2016). CAA can also trigger other pathogenic pathways, such as inflammation and oxidative stress, further leading to cerebral tissue damage (Ghiso et al., 2010).
Alzheimer’s Retinopathy
Over the past decade, the retina has been extensively investigated as a top candidate site of AD manifestation beyond the brain, as it shares many structural, cellular, molecular, and functional similarities with the brain (Hinton et al., 1986; Purves, 2001; Patton et al., 2005; Koronyo-Hamaoui et al., 2011; Koronyo et al., 2012, 2017; Schon et al., 2012; Erskine and Herrera, 2014; Crair and Mason, 2016; Hart et al., 2016; La Morgia et al., 2016; den Haan et al., 2018a; Asanad et al., 2019; Grimaldi et al., 2019; Lee S. et al., 2020; Mirzaei et al., 2020; Schultz et al., 2020; Snyder et al., 2021). Given the parallel pathology in the brain and retina, the retina has the potential to become a non-invasive diagnostic window since it is not shielded by bone and is easily accessible by ophthalmic exams such as optical coherence tomography (OCT) and fundoscopy (including scanning laser ophthalmoscopy) with subcellular resolution. The retina is directly and indirectly connected to the brain through bundles of neuronal axons forming the optic nerve, and by retinal and cerebral blood vessels, which may facilitate transportation of abnormal Aβ and tau species and further lead to the spread of AD pathology throughout the CNS (Morin et al., 1993). In addition, the discovery of dysfunctional lymphatic vessels within the brain of rodent models of AD implicates this CNS-specific lymphatic network, referred to as the glymphatic system (Jessen et al., 2015), as a culprit of insufficient cerebral amyloid clearance in AD (Louveau et al., 2015; Da Mesquita et al., 2018; Ahn et al., 2019). Recently, an ocular lymphatic drainage system was also identified in rodent models, which relies on an aquaporin-4-dependent pathway to clear fluid and metabolites (Wang et al., 2020). The roles of such lymphatic systems in retinal diseases and AD remain to be explored in future studies.
Studies conducted by OCT, electroretinogram (ERG), and histological examinations on cognitively impaired patients and laboratory animals have extensively described various retinal pathological and functional changes associated with AD development. In fact, the retina is heavily affected by AD pathology and displays a wide spectrum of retinopathy (reviewed in Mirzaei et al., 2020). This includes optic nerve degeneration and retinal neuronal and ganglion cell (RGC) loss (Hinton et al., 1986; Blanks et al., 1989, 1996; La Morgia et al., 2016; Koronyo et al., 2017; Asanad et al., 2019), retinal nerve fiber layer (NFL) thinning (Kergoat et al., 2001; Parisi et al., 2001; Berisha et al., 2007; Paquet et al., 2007; Moschos et al., 2012; Kirbas et al., 2013; Marziani et al., 2013; Moreno-Ramos et al., 2013; Kromer et al., 2014; Shi et al., 2014; Bayhan et al., 2015; Coppola et al., 2015; Gao et al., 2015; Liu et al., 2015; La Morgia et al., 2016), gliosis (Hinton et al., 1986; Curcio and Drucker, 1993; Blanks et al., 1996; Guo et al., 2010; Grimaldi et al., 2019), and vascular degeneration and injury (Patton et al., 2005; Frost et al., 2013; Cheung et al., 2014; Feke et al., 2015; Williams et al., 2015; Kapasi and Schneider, 2016; Shi et al., 2020b). This retinal damage can explain, at least in part, the visual dysfunctions (Sadun and Bassi, 1990; Armstrong and Syed, 1996; Risacher et al., 2020), sleep disturbances (La Morgia et al., 2016; Wang and Holtzman, 2020), and ERG abnormalities (Trick et al., 1989; Parisi et al., 2001; Moschos et al., 2012) documented in AD patients. Such findings have largely encouraged basic research in the AD retina and exploration of retinal imaging techniques for AD diagnosis.
Our group was the first to demonstrate the existence of Aβ accumulation, the hallmark AD pathology, in the retina of AD patients, including early-stage cases. In a study published in mid-2010, we revealed the aggregation of Aβ deposits in retinal flat-mounts isolated from 13 out of 13 neuropathologically confirmed AD and mild cognitively impaired (MCI) patients, which was minimally or undetected in 5 cognitively normal (CN) subjects negative for brain amyloid (Koronyo-Hamaoui et al., 2011). Further, this pioneer study demonstrated for the first time the ability to non-invasively detect curcumin-labeled Aβ deposits in live murine models of AD (Koronyo-Hamaoui et al., 2011). Importantly, similar reductions in retinal and brain Aβ plaques were detected ex vivo and in vivo in AD-model mice (Koronyo-Hamaoui et al., 2011; Koronyo et al., 2012) in response to immunomodulation therapies (Butovsky et al., 2006; Koronyo-Hamaoui et al., 2009; Bakalash et al., 2011; Koronyo et al., 2015; Rentsendorj et al., 2018; Doustar et al., 2020). Although a few studies failed to detect Aβ and/or (p)tau in the retina of AD patients, these reports included low case numbers (Schon et al., 2012; Ho et al., 2014; Williams et al., 2017) and only examined limited retinal regions in cross sections, focusing on less affected regions in these patients (La Morgia et al., 2016; Koronyo et al., 2017; Asanad et al., 2019; Shi et al., 2020b). It is possible this discrepancy in findings could also be due to differences in retinal tissue preservation, processing, and/or immunostaining protocols.
Subsequent studies by La Morgia et al. (2016), Lee S. et al. (2020), and others also demonstrated Aβ plaques and vascular-associated deposits in postmortem retinas of AD patient cohorts. Retinal amyloidosis in AD patients was in stark contrast to minimal pathology observed in the retinas of CN individuals (Tsai et al., 2014; La Morgia et al., 2016; den Haan et al., 2018a; Grimaldi et al., 2019; Lee S. et al., 2020; Qiu et al., 2020; Shi et al., 2020a; Cao et al., 2021). In 2017, Koronyo et al. (2017) published the development of more advanced human retinal extraction and histological techniques. Authors utilized immunofluorescence, anti-Aβ compound labeling, non-fluorescence immunostaining, and transmission electron microscopy (TEM) to measure Aβ42 plaque burden, characterize retinal Aβ plaque subtypes and morphology including identifying retinal Aβ fibrils and protofibrils, and describe Aβ plaque topographical and layer distribution in a larger cohort of 23 AD patients vs. 14 age- and sex-matched CN patients (Koronyo et al., 2017). In this study, several Aβ-epitope labeling techniques including Gallyas silver stain, curcumin, thioflavin-S, congo red, as well as a combination of monoclonal antibodies against various N’-, C’- and center Aβ sequences were used to describe amyloidosis in the human AD retina. Hence, together with post-mortem detection by immunofluorescence staining, peroxidase-based staining, and TEM analysis on retinal flat-mounts and cross-sections, this study profoundly validated Aβ accumulation in the AD retina in comparison to CN controls. We also demonstrated a significant correlation between retinal and brain plaque burdens, and more importantly, provided the first proof-of-concept trial using curcumin labeling and a scanning laser ophthalmoscope to detect and quantify retinal Aβ plaques in living patients, (Koronyo et al., 2017).
Indeed, multiple biochemical and histological studies corroborated these findings of Aβ deposits in the human AD retina (den Haan et al., 2018a; Grimaldi et al., 2019; Lee S. et al., 2020; Qiu et al., 2020) and further described retinal pTau, Aβ40 and Aβ42 accumulation, inflammation, and correlations between retinal and cerebral Aβ levels in AD patients (Alexandrov et al., 2011; Schon et al., 2012; den Haan et al., 2018b; Grimaldi et al., 2019; Lee S. et al., 2020; Qiu et al., 2020; Schultz et al., 2020; Shi et al., 2020b). More recently, in vivo retinal amyloid imaging in living MCI and AD patients was achieved via either retinal curcumin-enhanced fluorescence and SLO imaging or hyperspectral imaging (Hadoux et al., 2019; More et al., 2019; Dumitrascu et al., 2020; Lemmens et al., 2020; Ngolab et al., 2021).
Recent studies by Chibhabha et al. (2020); Sidiqi et al. (2020), and Barton et al. (2021) in the APPSWE/PS1ΔE9 transgenic mouse model further corroborated these findings via Aβ retinal curcumin imaging. In fact, numerous studies in AD rodent models have detected Aβ and its alloforms such as Aβ40 and Aβ42 in the AD retina (Inestrosa et al., 2005; Dutescu et al., 2009; Liu et al., 2009; Alexandrov et al., 2011; Ardiles et al., 2012; Schon et al., 2012; Williams et al., 2013; Yang et al., 2013; Zhao et al., 2013; Park et al., 2014; Tsai et al., 2014; Du et al., 2015; Parthasarathy et al., 2015; Chiasseu et al., 2017; Grimaldi et al., 2018; Harrison et al., 2019).
Retinal Vascular Aβ Deposits in AD Patients and Animal Models
An early study by Liu et al. (2009) in the Tg2576 transgenic murine model describes Aβ deposits within retinal microvessels by immunostaining against various Aβ epitopes, using mAbs clones 6E10, 12F4 and 5C3, in retinal cross-sections. Histological examinations by La Morgia et al. (2016) and Koronyo et al. (2017) of retinas from AD patients and age- and sex-matched cognitively normal controls provided evidence for retinal Aβ deposits inside blood vessel walls, perivascular and along blood vessels by immunostaining for 12F4-positive Aβ42 in retinal flat-mounts and cross-sections. In the Koronyo et al. (2017) study, retinal vascular Aβ accumulation in retinal flat-mounts and cross-sections of AD patients was also validated by other techniques including congo red, Gallyas silver stain, curcumin, 11A50-B10-positive Aβ40 immunostaining, as well as TEM analysis (Koronyo et al., 2017). In murine models of AD, a study by the same team demonstrated that following systemic administration of curcumin to APPSWEPS1ΔE9 model mice, ex vivo examination of retinal flatmounts revealed double-labeling of curcumin with 4G8 for Aβ deposits inside retinal blood vessels (Koronyo-Hamaoui et al., 2011).
Amyloidosis in cerebral blood vessels predominately consists of Aβ40 alloforms (Gravina et al., 1995). Accordingly, Shi et al. (2020b) conducted the first stereological quantification and mapping of Aβ40 in retinal blood vessels by immunostaining of 11A50-B10 and JRF/cAβ 40/28—specific monoclonal antibodies detecting the Aβ40 alloform—in retinal cross-sections and isolated retinal blood vessels from MCI and AD patients (see Figures 1A–E,G,K,L for retinal vascular amyloidosis). The pattern that was revealed by Aβ40 immunoreactivity covered most vascular compartments including tunica media, adventitia, and intima, indicating retinal blood vessels may also be thoroughly affected by Aβ deposition (Figure 1B). Increased levels of Aβ1–40 peptides in the retina of AD patients as compared with age- and sex-matched cognitively normal controls was further validated by a sandwich enzyme-linked immunosorbent (ELISA) analytical biochemistry assay (Shi et al., 2020b). When correlated with cerebral pathologies, levels of retinal Aβ40 significantly associated with entorhinal cortex plaque load and had a trend of predicting cognitive decline and CAA. Retinal vascular Aβ40 tightly associated with neuritic plaques in the entorhinal cortex and combined cerebral regions including hippocampus, frontal cortex, temporal cortex, and parietal cortex. A study by Schultz et al. (2020) also successfully correlated levels of retinal high molecular weight Aβ42 and Aβ40 with neurofibrillary tangles (NFT) and Aβ scores in the hippocampus of AD patients. Another notable finding was the downregulation of low-density lipoprotein receptor-related protein 1 (LRP1) in AD retina, suggesting compromised Aβ clearance (Shi et al., 2020b).
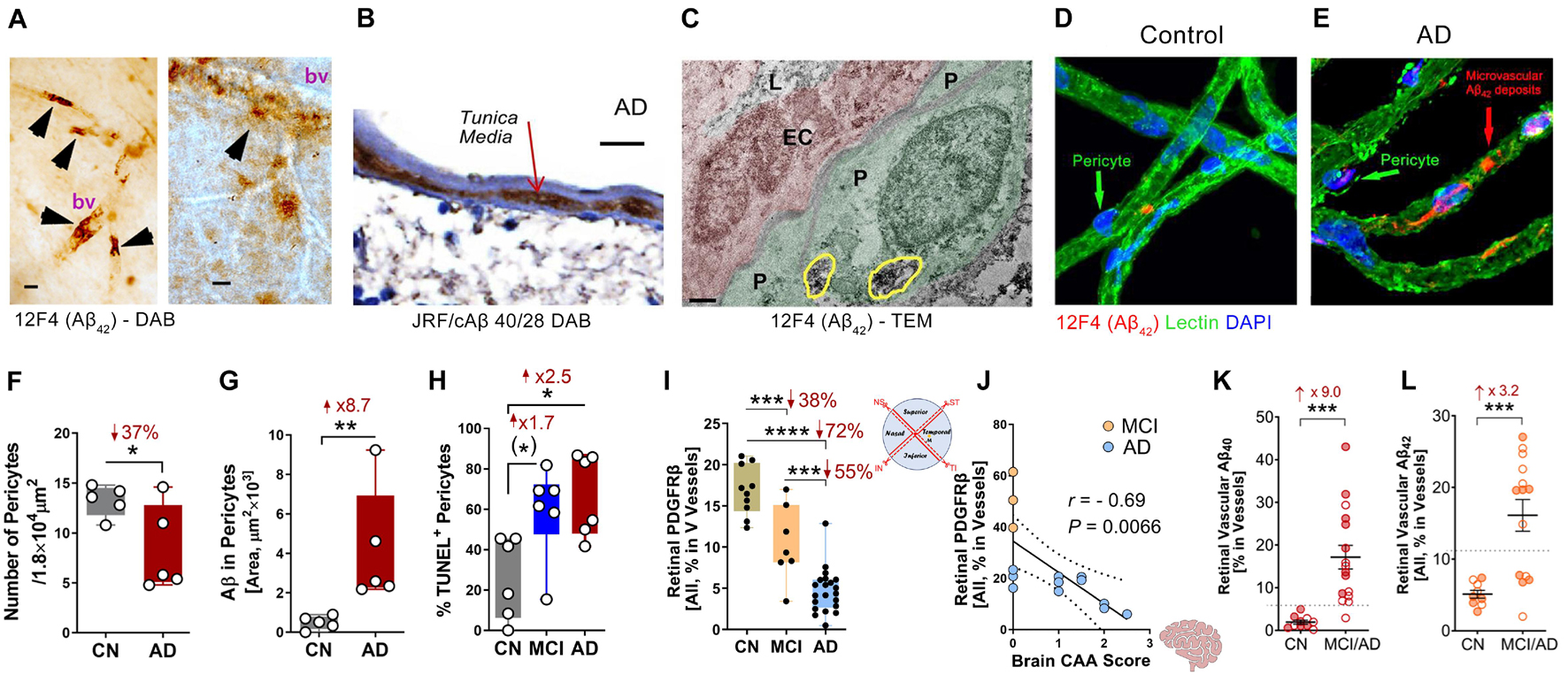
Figure 1. Retinal vascular amyloidosis and pericyte loss in the retina of MCI and AD patients. (A) 3,3′-Diaminobenzidine (DAB) staining of Aβ42 by 12F4 antibody in retinal blood vessels from flat-mount retina in an AD patient. Scale bar = 20 μm. (B) DAB staining of Aβ40 by JRF/cAβ 40/28 antibody on a retinal cross-section sample from an AD patient. Scale bar = 20 μm. (C) Transmission electron microscopy (TEM) for Aβ42 by 12F4 antibody staining in retinal blood vessels and pericytes. P, pericyte; EC, endothelial cell; L, lumen. Yellow circles indicate Aβ42 staining. Scale bar = 0.5 μm. (D,E) Immunostaining of Aβ42 by 12F4 antibody on retinal blood vessels isolated from an AD patient and control. Scale bars = 20 μm. (F) Quantification of pericytes in AD patients and cognitively normal (CN) controls based on isolated blood vessels. (G) Stereological quantification of Aβ in pericytes in AD patients and CN controls based on isolated blood vessels. (H) Quantification of terminal deoxynucleotidyl transferase-mediated dUTP nick-end labeling (TUNEL) positive pericytes on retinal cross-sections from CN, mild cognitively impaired (MCI), and AD patients. (I) Stereological quantification of PDGFRβ on retinal cross-sections from CN, MCI, and AD patients. (J) Pearson’s (r) correlation between cerebral amyloidosis angiopathy (CAA) and retinal PDGFRβ from MCI and AD patients. (K,L) Stereological quantification of panel (K). Aβ40 and (L) Aβ42 in CN versus MCI/AD patients. Filled circles represent males and clear circles represent females. Data from individual human donor as well as groups are shown as mean ± SEM. *p < 0.05, **p < 0.01, ***p < 0.001, ****p < 0.0001, by one-way ANOVA with Sidak’s post hoc multiple comparison test (more than 2 groups) or unpaired 2-tailed Student’s t test (2 groups). Fold and percentage changes are shown in red. Panel A reproduced from Koronyo et al. (2017) with permission of ASCI via Copyright Clearance Center. Panels B–L reproduced from Shi et al. (2020b) under terms of the Creative Commons Attribution 4.0 International License (http://creativecommons.org/licenses/by/4.0/).
In a subsequent report, Shi et al. (2020a) detected Aβ40 accumulation in retinal blood vessels of 8-month-old APPSWEPS1ΔE9 mice. Another recent study by Habiba et al. (2021) revealed detectable levels of Aβ40 and Aβ42 oligomers in the retina and blood as early as in 3-month-old APP/PS1 mice, prior to their detection in the respective brain. It is important to note that the transgenic APP/PS1 mouse model is driven by increased production of human amyloidogenic Aβ peptides, and therefore does not fully represent the human disease. Nevertheless, this mouse model is known to develop Aβ plaques and intracellular soluble Aβ oligomers, (p)tau, pronounced micro- and astrogliosis, synaptic loss, as well as cognitive and visual decline (Jankowsky et al., 2003; Butovsky et al., 2006; Koronyo-Hamaoui et al., 2009; Bakalash et al., 2011; Koronyo et al., 2015; Rentsendorj et al., 2018; Doustar et al., 2020; Vit et al., 2021). Intriguingly, a recent study by Chintapaludi et al. (2020) detected early onset alterations of retinal inflammatory genes before cerebral amyloidosis. Nevertheless, more supporting evidence and validation is needed to further evaluate the feasibility to diagnose AD by retinal vascular amyloid imaging.
AD-Related Retinal Vasculopathy
Mounting evidence has demonstrated a wide range of retinal vascular abnormalities in both AD patients and animals, such as reduced macular microvascular density (O’Bryhim et al., 2018), decreased blood flow (Berisha et al., 2007; Feke et al., 2015; Einarsdottir et al., 2016), compromised microvascular network (Frost et al., 2013; Cheung et al., 2014; Williams et al., 2015; Einarsdottir et al., 2016; Cabrera DeBuc et al., 2018), damaged vascular branching complexity (Frost et al., 2013; Cheung et al., 2014), vein narrowing (Berisha et al., 2007; Frost et al., 2013; Cheung et al., 2014; Feke et al., 2015; Cabrera DeBuc et al., 2018), and increased vascular tortuosity (Cheung et al., 2014). Among these findings, several studies showed significant correlations between retinal vascular impairment and AD susceptibility, while others did not. Nevertheless, these discoveries have provided numerous potential retinal vascular targets for AD monitoring and diagnosis. Compared to the brain, a distinct feature of the retina is the existence of Müller glial cells, which are the principal retinal glial cell type that maintain neuronal activity by regulating extracellular concentration of neurotransmitters and neuroactive ions (Newman and Reichenbach, 1996). Indeed, a previously published report suggested that retinal Aβ is engulfed by these specialized Muller glial cells (den Haan et al., 2018b), warranting further research on the potential role of these retina-specific glial cells in AD pathogenesis. It is important to note that most investigations are still limited to cross-sectional observations. Future studies should seek to apply standardized protocols and design with longitudinal study methods.
Another similarity between the retina and brain is the blood-organ barrier: the blood–retinal barrier (BRB) is highly comparable to the BBB, both structurally and functionally (Campbell and Humphries, 2012; Zenaro et al., 2017; Cai et al., 2018). The BBB is composed of cerebral vascular endothelial cells with tight junctions (TJ), astrocyte end-feet and supporting pericytes, while the BRB is made of an inner barrier of retinal vascular endothelial cells and an outer barrier of retinal epithelial cells, both with TJ and supporting pericytes (Campbell and Humphries, 2012; Zenaro et al., 2017; Cai et al., 2018). The main functions of these barriers are to modulate the influx of ions, proteins and water, as well as curb the infiltration of circulating immune cells (Cunha-Vaz et al., 2011). In AD, a compromised BBB is viewed as one of the principal causes for cerebral amyloidosis due to its essential role in clearing abundant cerebral Aβ to the circulating blood via the vascular network (Zlokovic et al., 1993; DeMattos et al., 2002; Banks et al., 2003; Do et al., 2015; Zhao et al., 2015; Sweeney et al., 2018). Recently, the Zlokovic group has successfully connected the BBB-associated pericyte injury biomarker, soluble PDGFRβ, in cerebrospinal fluid (CSF) to cognitive decline in apolipoprotein E (APOE4) carriers even after controlling for Aβ and tau status (Montagne et al., 2020). These findings suggest that BBB biomarkers might be an option for next-generation AD diagnostics and therapeutics.
Recent investigation of BRB in MCI and AD patients by Shi et al. (2020b) has revealed early and progressive retinal vascular PDGFRβ deficiency and pericyte loss associated with retinal vascular Aβ40 and Aβ42 deposition in postmortem tissues from MCI and AD patients (Figures 1D–J). In a subset of patients with neuropathological reports, retinal vascular PDGFRβ expression significantly correlated with CAA and cognitive decline assessed by the Mini-Mental State Examination (MMSE). These data suggest that pericyte loss or PDGFRβ downregulation may precede AD progression. The retinal pericytes in cognitively impaired patients were found to accumulate Aβ40 and Aβ42 and undergo apoptosis, demonstrated by terminal deoxynucleotidyl transferase dUTP nick end labeling (TUNEL) assay and cleaved caspase-3 nuclear staining. Interestingly, a previous study detected increased neuronal apoptosis in the rat retina induced by intra-vitreous injection of Aβ1–42 oligomers (Fisichella et al., 2016). In a subsequent study, the Koronyo-Hamaoui group further discovered significantly augmented capillary degeneration in 8-month-old APPSWEPS1ΔE9 mice compared to wild type littermates that was further exacerbated in 12-month-old mice (Figures 2A,B; Shi et al., 2020a). Retinal capillary loss was associated with increased retinal vascular amyloidosis, indicating more BRB damage may be driven by vascular Aβ deposition and implicated in AD pathology (Shi et al., 2020a). Western blot analysis of whole retinal lysates revealed altered expression of key TJ molecules of the BRB, including claudin-1 and zonula occuludens-1 (ZO-1) (Figures 2C,D). These changes were also accompanied by elevated NF-κB p65 phosphorylation in retinas of 12-month-old ADtg mice, implicating upregulated inflammation in the retina with increased vascular amyloidosis burden. Having found these changes in retinal blood vessels and capillaries of AD-model mice, the authors sought to explore how these vascular pathologies may have affected BRB permeability. In vivo fluorescein (332 Da) imaging of APPSWEPS1ΔE9 mice showed live retinal vascular leakage in 12-month-old but not in 8-month-old mouse models of AD (Figure 2E). Intriguingly, intravenous injection of larger FITC-dextran (1,000 kDa) and Texas-Red-dextran (3 kDa) molecules in 6-month-old APPSWEPS1ΔE9 mice followed by ex vivo postmortem retinal imaging and quantification of the fluorescent signal indicated a dramatic increase in retinal vascular leakage of both molecules (Figures 2F,G). These BRB permeability changes in transgenic AD mice occur even earlier than the respective cerebral leakage measured by the same molecules (Lahiri et al., 2019). The difference between in vivo and ex vivo observations is suggestive of a shift in molecular size-dependent transporting mechanisms through the BRB in the AD transgenic mice model. Accordingly, a recent study utilizing the C57BL/6 mouse revealed a decrease in plasma protein transport activity through the BBB in the aged brain, driven by transport shifting from ligand-specific receptor-mediated to non-specific caveolar transcytosis (Yang et al., 2020). Whether this also occurs in AD patients’ BRB needs further validation. Overall, such discoveries have suggested that several BRB compartments are affected in AD disease progression that should be further evaluated as biomarkers for AD diagnosis.
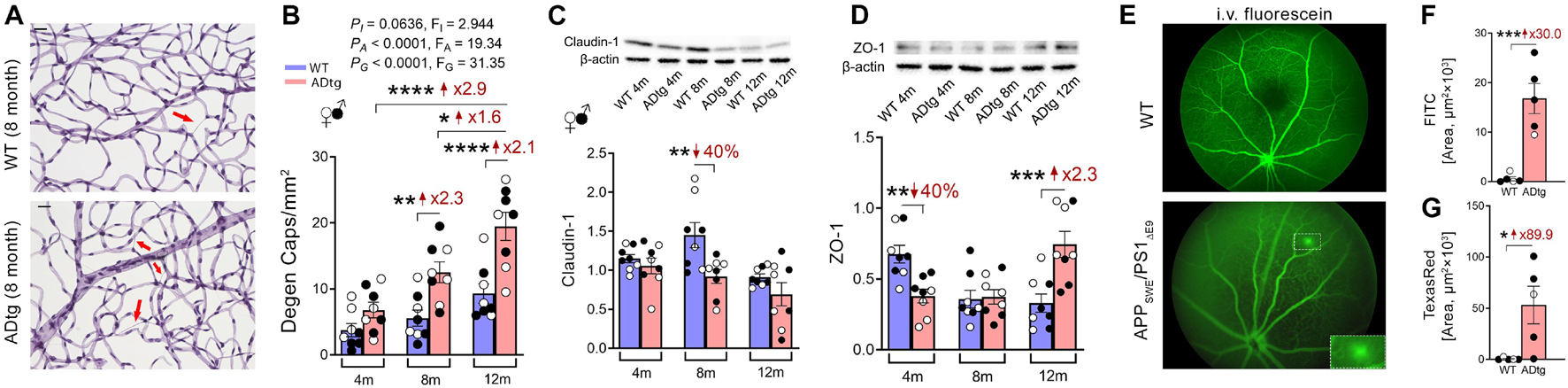
Figure 2. Retinal vasculopathy in APPSWEPS1ΔE9 (ADtg) mice. (A,B) Representative images of periodic acid-Schiff (PAS)-stained, hematoxylin-counterstained isolated retinal microvasculature from ADtg and matched wild type (WT) littermates. Acellular degenerated retinal capillaries are indicated by red arrows. (B) Numbers of degenerated retinal capillaries when mice are stratified by mouse genotypes, WT or ADtg, by age groups of 4, 8, and 12 months. (C,D) Western-Blot analysis of panel (C) claudin-1 and (D) ZO-1 in retinal lysates from 4, 8, and 12-month-old APPSWEPS1Δ E9 mice and WT controls. (E) Images showing in vivo retinal microvascular imaging for leakage after intraperitoneal fluorescein injection in 12-month-old WT and ADtg mice. (F,G) Quantitative analysis of the panel (D) FITC (1,000 kDa) or (E) Texas Red (3 kDa)-stained area in retinal flat-mounts from WT or ADtg mice. Black-filled circles represent males and clear circles represent females. Data from individual mouse as well as groups are shown as mean ± SEM. *p < 0.05, **p < 0.01, ***p < 0.001, ****p < 0.0001, by 2-way ANOVA with Sidak’s post hoc multiple comparison test (more than 2 groups) or unpaired 2-tailed Student’s t test (2 groups). Fold and percentage changes are shown in red. Reproduced from Shi et al. (2020a) under terms of the Creative Commons Attribution 4.0 International License (http://creativecommons.org/licenses/by/4.0/).
Cerebral Imaging for AD
Recent developments in brain imaging modalities have significantly improved the ability to rule-in AD related cerebral pathologies in at-risk populations (Johnson et al., 2012). These include MRI (fMRI) (Smith et al., 1999; Machulda et al., 2003; Dickerson et al., 2004; Johnson et al., 2006, 2012), fluorodeoxyglucose (FDG) positron emission tomography (PET) (Foster et al., 1983; Hoffman et al., 2000; Engler et al., 2006), amyloid PET imaging (Drzezga et al., 2008; Ikonomovic et al., 2008), PET imaging of copper trafficking (Torres et al., 2016; Andreozzi et al., 2017), and transcranial Doppler (TCD) ultrasound (Roher et al., 2011). However, these techniques are still subject to a variety of limitations such as high cost, low availability, low spatial resolution, low specificity, or involving the use of unsafe radio isotopes (Johnson et al., 2012). Nevertheless, current imaging techniques do not provide a solution for large scale screening of pre-symptomatic at-risk populations, which is the main goal of current efforts to develop more sensitive ocular examination techniques for AD diagnosis.
Retinal OCT and OCT-A Imaging in MCI and AD Patients
Optical coherence tomography has been a pioneer technology in capturing retinal structural changes in living AD patients. This technology utilizes low-coherence light to acquire two- and three-dimensional images of retinal cross-sectional anatomy with micrometer resolution (Frohman et al., 2008; Popescu et al., 2011; Aumann et al., 2019). It provides non-invasive live measurements of retinal layer structure and is widely used in ophthalmic exanimations for diagnosis of glaucoma, age-related macular degeneration (AMD), diabetic retinopathy (DR), as well as other ocular diseases (Lang, 2007; Medical Advisory, 2009; Sathyan et al., 2012). Parisi et al. (2001) utilized this technology for the first time in AD patients, demonstrating a significant reduction in retinal nerve fiber layer (NFL) thickness as compared to healthy control individuals. Paquet et al. (2007) further described a significant reduction of retinal NFL thickness in MCI, mild AD, moderate AD, and severe AD patients compared to healthy controls. Subsequently, numerous studies verified these early studies and reported decreases in NFL, ganglion cell layer (GCL), and macula thickness correlating with cognitive decline (Kromer et al., 2014; Cunha et al., 2016; Doustar et al., 2017; Ferrari et al., 2017; Polans et al., 2017; Polo et al., 2017; Bulut et al., 2018; Janez-Escalada et al., 2019; Salobrar-Garcia et al., 2019; Czako et al., 2020; Dumitrascu and Koronyo-Hamaoui, 2020; Mejia-Vergara et al., 2021; Yan et al., 2021). OCT-adaptive optics is a relatively newer advancement of this technology which provides ultra-high-resolution images, including of blood vessel walls, that warrants further testing in the AD retina (Snyder et al., 2021).
Among the many advances in OCT technology, OCT-angiography (OCTA) has been specifically developed for the investigation of retinal blood vessels, revolutionizing the diagnosis of retinal vascular-related disorders (de Carlo et al., 2015; Chalam and Sambhav, 2016; Hagag et al., 2017). It provides high-resolution motion-contrast images based on backscattered light from neuronal and vascular tissues in the retina (Kashani et al., 2017). This enables visualization of various retinal vascular abnormalities such as microaneurysms, neovascularization, retinal vascular non-perfusion, reduced vascular density, and modified foveal avascular zone (FAZ) (Kashani et al., 2017). OCT-A received FDA approval in 2016 and has been rigorously used in diagnosis of retinal vascular diseases including DR, uveitis, AMD, and others (Pichi et al., 2017; Khadamy et al., 2018; Schneider and Fowler, 2018; Tey et al., 2019). The significant potential of this technology has recently led to a surge of research activity related to its utility in exploring retinal biomarkers in AD. An early case-control study by Bulut et al. (2018) on a total of 52 AD patients and healthy controls described a significant decrease in retinal vascular density, reduced retinal and choroidal thickness, as well as enlarged FAZ area in the patients. Shortly after, Jiang et al. (2018) based on 52 participants demonstrated lower densities of retinal vascular network, superficial vascular plexus (SVP), and deep vascular plexus (DCP) in MCI and AD patients, while O’Bryhim et al. (2018) with 32 participants validated increased FAZ area in AD patients. To date, such OCTA case-controlled studies seem to be largely consistent in demonstrating retinal vascular density loss and increased FAZ area in AD patients but differ in identifying vascular areas affected, the superficial vs. deep, or parafoveal vs. perifoveal vessels (Lahme et al., 2018; Sadda et al., 2019; Yoon et al., 2019; Zabel et al., 2019; Zhang et al., 2019; Czako et al., 2020; Lee J.Y. et al., 2020; Wu et al., 2020; Rifai et al., 2021). Overall, these are indeed breakthrough findings that warrant further investigation, considering OCTA is a relatively new technology. It is also important to note that sample sizes in most of these studies are relatively small. To better evaluate OCTA as a diagnostic tool for AD, longitudinal studies with a standardized consistent protocol and large case numbers are needed.
Blood–retinal barrier permeability in laboratory animals is usually measured by injecting fluorescent dyes such as fluorescein (Do carmo et al., 1998) or Evans blue (Xu et al., 2001), followed by in vivo or ex vivo imaging for retinal vascular leakage. Fundus fluorescein angiography (FFA) was developed based on visualizing fluorescent dye by fundus camera that has been widely used to evaluate retinal vascular circulation and BRB integrity (Marmor and Ravin, 2011). Another modified OCT method, OCT-leakage, was recently developed to monitor retinal edema, thus evaluating BRB damage (Cunha-Vaz et al., 2016; Cunha-Vaz, 2017). This method applies a proprietary algorithm to identify sites of decreased optical reflectivity, then the system quantifies and detects the correlation of retinal extracellular space. The developer tested OCT-leakage on 28 patients and provided consistent output between FFA and OCT-leakage for BRB damage in diabetic retinopathy (Cunha-Vaz et al., 2017). Both FFA and OCT-leakage can potentially be tested in cognitively impaired patients to investigate the potential of BRB permeability monitoring for AD diagnosis.
Conclusion
In summary, recent advancements in retinal vascular research in AD patients and animal models have provided many potential candidate targets for non-invasive diagnosis by retinal vascular imaging. These include but are not limited to retinal vascular amyloidosis, FAZ area, vascular leakage, vascular blood flow and perfusion, TJ alteration, vascular density, pericyte and PDGFRβ loss, vascular branching complexity and others. Reports suggest that certain vascular abnormalities occur very early during AD progression and may predict cognitive decline in patients; thus, their detection may be critical for early diagnosis and prognosis prediction. However, since some of these vascular findings are commonly observed in retinal degenerative and inflammatory diseases, it is important to also consider AD-specific hallmark biomarkers such as Aβ and (p)tau for accurate diagnosis. Finally, with the recent development of retinal amyloid imaging (Koronyo et al., 2017; Dumitrascu et al., 2020; Ngolab et al., 2021), pericyte imaging (Schallek et al., 2013), OCTA and OCT-leakage (Cunha-Vaz et al., 2016; Cunha-Vaz, 2017), hyperspectral imaging (Hadoux et al., 2019; More et al., 2019; Lemmens et al., 2020), and FFA (Marmor and Ravin, 2011), future studies may pave a the way for next-generation non-invasive ophthalmic imaging technologies to facilitate AD monitoring and diagnosis.
Author Contributions
HS and MK-H: draft manuscript and figures preparation. MK-H, HS, YK, AR, D-TF, NM, JS, and KB: manuscript editing. MK-H: study supervision. All authors read and approved the submitted version.
Funding
The work of MK-H was supported by the National Institute of Health (NIH)/NIA Grant Numbers: R01AG056478, R01 AG056478-04S1 and R01AG055865, as well as by the Haim Saban and Tom Gordon Private Foundations.
Conflict of Interest
YK, MK-H, and KB are co-founders and stockholders of NeuroVision Imaging, Inc., Sacramento, CA, United States. MK-H, HS, YK, and KB are inventors on Patent Application No. 62/970,083 filed February 4, 2020 entitled “Method of Detecting Cognitive Impairment.”
The remaining authors declare that the research was conducted in the absence of any commercial or financial relationships that could be construed as a potential conflict of interest.
Publisher’s Note
All claims expressed in this article are solely those of the authors and do not necessarily represent those of their affiliated organizations, or those of the publisher, the editors and the reviewers. Any product that may be evaluated in this article, or claim that may be made by its manufacturer, is not guaranteed or endorsed by the publisher.
Acknowledgments
We thank Mia Oviatt for help with manuscript editing. The authors dedicate the manuscript to the memory of Dr. Salomon Moni Hamaoui and Lillian Jones Black, who died of Alzheimer’s disease.
References
Ahn, J. H., Cho, H., Kim, J.-H., Kim, S. H., Ham, J.-S., Park, I., et al. (2019). Meningeal lymphatic vessels at the skull base drain cerebrospinal fluid. Nature 572, 62–66. doi: 10.1038/s41586-019-1419-5
Alexandrov, P. N., Pogue, A., Bhattacharjee, S., and Lukiw, W. J. (2011). Retinal amyloid peptides and complement factor H in transgenic models of Alzheimer’s disease. Neuroreport 22, 623–627. doi: 10.1097/wnr.0b013e3283497334
Alzheimer, A. (1911). Über eigenartige Krankheitsfälle des späteren Alters. Z. f. d. g. Neur. u. Psych. 4:356. doi: 10.1007/bf02866241
Alzheimer’s Association (2020). What is Alzheimer’s Disease? [Online]. Available online at: https://www.alz.org/alzheimers-dementia/what-is-alzheimers (Accessed June 1, 2021).∗
Andreozzi, E. M., Torres, J. B., Sunassee, K., Dunn, J., Walker-Samuel, S., Szanda, I., et al. (2017). Studies of copper trafficking in a mouse model of Alzheimer’s disease by positron emission tomography: comparison of (64)Cu acetate and (64)CuGTSM. Metallomics 9, 1622–1633. doi: 10.1039/c7mt00227k
Ardiles, A. O., Tapia-Rojas, C. C., Mandal, M., Alexandre, F., Kirkwood, A., Inestrosa, N. C., et al. (2012). Postsynaptic dysfunction is associated with spatial and object recognition memory loss in a natural model of Alzheimer’s disease. Proc. Natl. Acad. Sci. U.S.A. 109, 13835–13840. doi: 10.1073/pnas.1201209109
Armstrong, R. A., and Syed, A. B. (1996). Alzheimer’s disease and the eye. Ophthalmic Physiol. Opt. 16(Suppl. 1), S2–S8.
Arvanitakis, Z., Leurgans, S. E., Wang, Z., Wilson, R. S., Bennett, D. A., and Schneider, J. A. (2011). Cerebral amyloid angiopathy pathology and cognitive domains in older persons. Ann. Neurol. 69, 320–327. doi: 10.1002/ana.22112
Asanad, S., Ross-Cisneros, F. N., Nassisi, M., Barron, E., Karanjia, R., and Sadun, A. A. (2019). The retina in alzheimer’s disease: histomorphometric analysis of an ophthalmologic biomarker. Invest. Ophthalmol. Vis. Sci. 60, 1491–1500. doi: 10.1167/iovs.18-25966
Aumann, S., Donner, S., Fischer, J., and Muller, F. (2019). “Optical coherence tomography (OCT): principle and technical realization,” in High Resolution Imaging in Microscopy and Ophthalmology: New Frontiers in Biomedical Optics, ed. J. F. Bille (Cham: Springer), 59–85. doi: 10.1007/978-3-030-16638-0_3
Bakalash, S., Pham, M., Koronyo, Y., Salumbides, B. C., Kramerov, A., Seidenberg, H., et al. (2011). Egr1 expression is induced following glatiramer acetate immunotherapy in rodent models of glaucoma and Alzheimer’s disease. Invest. Ophthalmol. Vis. Sci. 52, 9033–9046. doi: 10.1167/iovs.11-7498
Baker, M. L., Marino Larsen, E. K., Kuller, L. H., Klein, R., Klein, B. E., Siscovick, D. S., et al. (2007). Retinal microvascular signs, cognitive function, and dementia in older persons: the cardiovascular health study. Stroke 38, 2041–2047. doi: 10.1161/strokeaha.107.483586
Banks, W. A., Robinson, S. M., Verma, S., and Morley, J. E. (2003). Efflux of human and mouse amyloid beta proteins 1-40 and 1-42 from brain: impairment in a mouse model of Alzheimer’s disease. Neuroscience 121, 487–492. doi: 10.1016/s0306-4522(03)00474-3
Barton, S. M., To, E., Rogers, B. P., Whitmore, C., Uppal, M., Matsubara, J. A., et al. (2021). Inhalable Thioflavin S for the detection of amyloid beta deposits in the retina. Molecules 26:835. doi: 10.3390/molecules26040835
Bayhan, H. A., Aslan Bayhan, S., Celikbilek, A., Tanik, N., and Gurdal, C. (2015). Evaluation of the chorioretinal thickness changes in Alzheimer’s disease using spectral-domain optical coherence tomography. Clin. Exp. Ophthalmol. 43, 145–151.
Bell, R. D., and Zlokovic, B. V. (2009). Neurovascular mechanisms and blood-brain barrier disorder in Alzheimer’s disease. Acta Neuropathol. 118, 103–113. doi: 10.1007/s00401-009-0522-3
Berisha, F., Feke, G. T., Trempe, C. L., Mcmeel, J. W., and Schepens, C. L. (2007). Retinal abnormalities in early Alzheimer’s disease. Invest. Ophthalmol. Vis. Sci. 48, 2285–2289.
Beskow, J., Hassler, O., and Ottosson, J. O. (1971). Cerebral arterial deformities in relation to senile deterioration. Acta Psychiatr. Scand. Suppl. 221, 111–119. doi: 10.1111/j.1600-0447.1971.tb02143.x
Binnewijzend, M. A., Benedictus, M. R., Kuijer, J. P., Van Der Flier, W. M., Teunissen, C. E., Prins, N. D., et al. (2016). Cerebral perfusion in the predementia stages of Alzheimer’s disease. Eur. Radiol. 26, 506–514. doi: 10.1007/s00330-015-3834-9
Biron, K. E., Dickstein, D. L., Gopaul, R., and Jefferies, W. A. (2011). Amyloid triggers extensive cerebral angiogenesis causing blood brain barrier permeability and hypervascularity in Alzheimer’s disease. PLoS One 6:e23789. doi: 10.1371/journal.pone.0023789
Blanks, J. C., Hinton, D. R., Sadun, A. A., and Miller, C. A. (1989). Retinal ganglion cell degeneration in Alzheimer’s disease. Brain Res. 501, 364–372.
Blanks, J. C., Schmidt, S. Y., Torigoe, Y., Porrello, K. V., Hinton, D. R., and Blanks, R. H. (1996). Retinal pathology in Alzheimer’s disease, II. Regional neuron loss and glial changes in GCL. Neurobiol. Aging 17, 385–395. doi: 10.1016/0197-4580(96)00009-7
Blennow, K., Mattsson, N., Scholl, M., Hansson, O., and Zetterberg, H. (2015). Amyloid biomarkers in Alzheimer’s disease. Trends Pharmacol. Sci. 36, 297–309.
Bloom, G. S. (2014). Amyloid-beta and tau: the trigger and bullet in Alzheimer disease pathogenesis. JAMA Neurol. 71, 505–508. doi: 10.1001/jamaneurol.2013.5847
Bonte, F. J., Ross, E. D., Chehabi, H. H., and Devous, M. D. Sr. (1986). SPECT study of regional cerebral blood flow in Alzheimer disease. J. Comput. Assist. Tomogr. 10, 579–583. doi: 10.1097/00004728-198607000-00005
Boyle, P. A., Yu, L., Nag, S., Leurgans, S., Wilson, R. S., Bennett, D. A., et al. (2015). Cerebral amyloid angiopathy and cognitive outcomes in community-based older persons. Neurology 85, 1930–1936. doi: 10.1212/wnl.0000000000002175
Bulut, M., Kurtulus, F., Gozkaya, O., Erol, M. K., Cengiz, A., Akidan, M., et al. (2018). Evaluation of optical coherence tomography angiographic findings in Alzheimer’s type dementia. Br. J. Ophthalmol. 102, 233–237. doi: 10.1136/bjophthalmol-2017-310476
Bulut, M., Yaman, A., Erol, M. K., Kurtuluş, F., Toslak, D., Doğan, B., et al. (2016). Choroidal thickness in patients with mild cognitive impairment and Alzheimer’s type dementia. J. Ophthalmol. 2016:7291257.
Butovsky, O., Koronyo-Hamaoui, M., Kunis, G., Ophir, E., Landa, G., Cohen, H., et al. (2006). Glatiramer acetate fights against Alzheimer’s disease by inducing dendritic-like microglia expressing insulin-like growth factor 1. Proc. Natl. Acad. Sci. U.S.A. 103, 11784–11789. doi: 10.1073/pnas.0604681103
Cabrera DeBuc, D., Somfai, G. M., Arthur, E., Kostic, M., Oropesa, S., and Mendoza Santiesteban, C. (2018). Investigating multimodal diagnostic eye biomarkers of cognitive impairment by measuring vascular and neurogenic changes in the retina. Front. Physiol. 9:1721. doi: 10.3389/fphys.2018.01721
Cai, Z., Qiao, P. F., Wan, C. Q., Cai, M., Zhou, N. K., and Li, Q. (2018). Role of blood-brain barrier in Alzheimer’s Disease. J. Alzheimers Dis. 63, 1223–1234.
Campbell, M., and Humphries, P. (2012). The blood-retina barrier: tight junctions and barrier modulation. Adv. Exp. Med. Biol. 763, 70–84. doi: 10.1007/978-1-4614-4711-5_3
Cao, K. J., Kim, J. H., Kroeger, H., Gaffney, P. M., Lin, J. H., Sigurdson, C. J., et al. (2021). ARCAM-1 facilitates fluorescence detection of amyloid-containing deposits in the retina. Transl. Vis. Sci. Technol. 10:5. doi: 10.1167/tvst.10.7.5
Castillo-Carranza, D. L., Nilson, A. N., Van Skike, C. E., Jahrling, J. B., Patel, K., Garach, P., et al. (2017). Cerebral microvascular accumulation of tau oligomers in Alzheimer’s Disease and related tauopathies. Aging Dis. 8, 257–266. doi: 10.14336/ad.2017.0112
Chalam, K. V., and Sambhav, K. (2016). Optical coherence tomography angiography in retinal diseases. J. Ophthalmic. Vis. Res. 11, 84–92.
Cheung, C. Y., Ong, Y. T., Ikram, M. K., Ong, S. Y., Li, X., Hilal, S., et al. (2014). Microvascular network alterations in the retina of patients with Alzheimer’s disease. Alzheimers Demen. 10, 135–142. doi: 10.1016/j.jalz.2013.06.009
Chiasseu, M., Alarcon-Martinez, L., Belforte, N., Quintero, H., Dotigny, F., Destroismaisons, L., et al. (2017). Tau accumulation in the retina promotes early neuronal dysfunction and precedes brain pathology in a mouse model of Alzheimer’s disease. Mol. Neurodegener. 12:58.
Chibhabha, F., Yang, Y., Ying, K., Jia, F., Zhang, Q., Ullah, S., et al. (2020). Non-invasive optical imaging of retinal Aβ plaques using curcumin loaded polymeric micelles in APP(swe)/PS1(ΔE9) transgenic mice for the diagnosis of Alzheimer’s disease. J. Mater Chem. B 8, 7438–7452. doi: 10.1039/d0tb01101k
Chintapaludi, S. R., Uyar, A., Jackson, H. M., Acklin, C. J., Wang, X., Sasner, M., et al. (2020). Staging Alzheimer’s Disease in the brain and retina of B6.APP/PS1 mice by transcriptional profiling. J. Alzheimers Dis. 73, 1421–1434. doi: 10.3233/jad-190793
Cisternas, P., Taylor, X., and Lasagna-Reeves, C. A. (2019). The Amyloid-Tau-Neuroinflammation axis in the context of cerebral amyloid angiopathy. Int. J. Mol. Sci. 20:6319. doi: 10.3390/ijms20246319
Claudio, L. (1996). Ultrastructural features of the blood-brain barrier in biopsy tissue from Alzheimer’s disease patients. Acta Neuropathol. 91, 6–14. doi: 10.1007/s004010050386
Coppola, G., Di Renzo, A., Ziccardi, L., Martelli, F., Fadda, A., Manni, G., et al. (2015). Optical coherence tomography in Alzheimer’s Disease: a meta-analysis. PLoS One 10:e0134750. doi: 10.1371/journal.pone.0134750
Crair, M. C., and Mason, C. A. (2016). Reconnecting eye to brain. J. Neurosci. 36, 10707–10722. doi: 10.1523/jneurosci.1711-16.2016
Cunha, J. P., Proença, R., Dias-Santos, A., Melancia, D., Almeida, R., Águas, H., et al. (2017). Choroidal thinning: Alzheimer’s disease and aging. Alzheimers Demen. (Amst.) 8, 11–17.
Cunha, L. P., Almeida, A. L., Costa-Cunha, L. V., Costa, C. F., and Monteiro, M. L. (2016). The role of optical coherence tomography in Alzheimer’s disease. Int. J. Retina Vitreous 2:24.
Cunha-Vaz, J. (2017). The blood-retinal barrier in the management of retinal disease: EURETINA award lecture. Ophthalmologica 237, 1–10. doi: 10.1159/000455809
Cunha-Vaz, J., Bernardes, R., and Lobo, C. (2011). Blood-retinal barrier. Eur. J. Ophthalmol. 21(Suppl. 6), S3–S9.
Cunha-Vaz, J., Santos, T., Alves, D., Marques, I., Neves, C., Soares, M., et al. (2017). Agreement between OCT leakage and fluorescein angiography to identify sites of alteration of the blood-retinal barrier in diabetes. Ophthalmol. Retina 1, 395–403. doi: 10.1016/j.oret.2017.02.002
Cunha-Vaz, J., Santos, T., Ribeiro, L., Alves, D., Marques, I., and Goldberg, M. (2016). OCT-Leakage: a new method to identify and locate abnormal fluid accumulation in diabetic retinal edema. Invest. Ophthalmol. Vis. Sci. 57, 6776–6783. doi: 10.1167/iovs.16-19999
Curcio, C. A., and Drucker, D. N. (1993). Retinal ganglion cells in Alzheimer’s disease and aging. Ann. Neurol. 33, 248–257. doi: 10.1002/ana.410330305
Czako, C., Kovacs, T., Ungvari, Z., Csiszar, A., Yabluchanskiy, A., Conley, S., et al. (2020). Retinal biomarkers for Alzheimer’s disease and vascular cognitive impairment and dementia (VCID): implication for early diagnosis and prognosis. Geroscience 42, 1499–1525. doi: 10.1007/s11357-020-00252-7
Da Mesquita, S., Louveau, A., Vaccari, A., Smirnov, I., Cornelison, R. C., Kingsmore, K. M., et al. (2018). Publisher correction: functional aspects of meningeal lymphatics in ageing and Alzheimer’s disease. Nature 564:E7.
de Carlo, T. E., Romano, A., Waheed, N. K., and Duker, J. S. (2015). A review of optical coherence tomography angiography (OCTA). Int. J. Retina Vitreous 1:5.
Deal, J. A., Sharrett, A. R., Rawlings, A. M., Gottesman, R. F., Bandeen-Roche, K., Albert, M., et al. (2018). Retinal signs and 20-year cognitive decline in the Atherosclerosis Risk in Communities Study. Neurology 90, e1158–e1166.
DeMattos, R. B., Bales, K. R., Cummins, D. J., Paul, S. M., and Holtzman, D. M. (2002). Brain to plasma amyloid-beta efflux: a measure of brain amyloid burden in a mouse model of Alzheimer’s disease. Science 295, 2264–2267. doi: 10.1126/science.1067568
den Haan, J., Morrema, T. H. J., Rozemuller, A. J., Bouwman, F. H., and Hoozemans, J. J. M. (2018a). Different curcumin forms selectively bind fibrillar amyloid beta in post mortem Alzheimer’s disease brains: implications for in-vivo diagnostics. Acta Neuropathol. Commun. 6:75.
den Haan, J., Morrema, T. H. J., Verbraak, F. D., De Boer, J. F., Scheltens, P., Rozemuller, A. J., et al. (2018b). Amyloid-beta and phosphorylated tau in post-mortem Alzheimer’s disease retinas. Acta Neuropathol. Commun. 6:147.
Desai, B. S., Schneider, J. A., Li, J. L., Carvey, P. M., and Hendey, B. (2009). Evidence of angiogenic vessels in Alzheimer’s disease. J. Neural. Transm. (Vienna) 116, 587–597. doi: 10.1007/s00702-009-0226-9
DeSimone, C. V., Graff-Radford, J., El-Harasis, M. A., Rabinstein, A. A., Asirvatham, S. J., and Holmes, D. R. Jr. (2017). Cerebral amyloid angiopathy: diagnosis. clinical implications, and management strategies in atrial fibrillation. J. Am. Coll. Cardiol. 70, 1173–1182.
Dickerson, B. C., Salat, D. H., Bates, J. F., Atiya, M., Killiany, R. J., Greve, D. N., et al. (2004). Medial temporal lobe function and structure in mild cognitive impairment. Ann. Neurol. 56, 27–35. doi: 10.1002/ana.20163
Do carmo, A., Ramos, P., Reis, A., Proenca, R., and Cunha-Vaz, J. G. (1998). Breakdown of the inner and outer blood retinal barrier in streptozotocin-induced diabetes. Exp. Eye Res. 67, 569–575. doi: 10.1006/exer.1998.0546
Do, T. M., Dodacki, A., Alata, W., Calon, F., Nicolic, S., Scherrmann, J. M., et al. (2015). Age-Dependent regulation of the blood-brain barrier influx/efflux equilibrium of amyloid-beta peptide in a mouse model of Alzheimer’s Disease (3xTg-AD). J. Alzheimers Dis. 49, 287–300. doi: 10.3233/jad-150350
Doustar, J., Rentsendorj, A., Torbati, T., Regis, G. C., Fuchs, D. T., Sheyn, J., et al. (2020). Parallels between retinal and brain pathology and response to immunotherapy in old, late-stage Alzheimer’s disease mouse models. Aging Cell 19:e13246.
Doustar, J., Torbati, T., Black, K. L., Koronyo, Y., and Koronyo-Hamaoui, M. (2017). Optical coherence tomography in Alzheimer’s Disease and other neurodegenerative diseases. Front. Neurol. 8:701. doi: 10.3389/fneur.2017.00701
Drzezga, A., Grimmer, T., Henriksen, G., Stangier, I., Perneczky, R., Diehl-Schmid, J., et al. (2008). Imaging of amyloid plaques and cerebral glucose metabolism in semantic dementia and Alzheimer’s disease. Neuroimage 39, 619–633. doi: 10.1016/j.neuroimage.2007.09.020
Du, L. Y., Chang, L. Y., Ardiles, A. O., Tapia-Rojas, C., Araya, J., Inestrosa, N. C., et al. (2015). Alzheimer’s Disease-related protein expression in the retina of octodon degus. PLoS One 10:e0135499. doi: 10.1371/journal.pone.0135499
Dumitrascu, O. M., and Koronyo-Hamaoui, M. (2020). Retinal vessel changes in cerebrovascular disease. Curr. Opin. Neurol. 33, 87–92. doi: 10.1097/wco.0000000000000779
Dumitrascu, O. M., Lyden, P. D., Torbati, T., Sheyn, J., Sherzai, A., Sherzai, D., et al. (2020). Sectoral segmentation of retinal amyloid imaging in subjects with cognitive decline. Alzheimers Demen. (Amst.) 12:e12109.
Dutescu, R. M., Li, Q. X., Crowston, J., Masters, C. L., Baird, P. N., and Culvenor, J. G. (2009). Amyloid precursor protein processing and retinal pathology in mouse models of Alzheimer’s disease. Graefes Arch. Clin. Exp. Ophthalmol. 247, 1213–1221. doi: 10.1007/s00417-009-1060-3
Einarsdottir, A. B., Hardarson, S. H., Kristjansdottir, J. V., Bragason, D. T., Snaedal, J., and Stefansson, E. (2016). Retinal oximetry imaging in Alzheimer’s disease. J. Alzheimers Dis. 49, 79–83. doi: 10.3233/jad-150457
Ellis, R. J., Olichney, J. M., Thal, L. J., Mirra, S. S., Morris, J. C., Beekly, D., et al. (1996). Cerebral amyloid angiopathy in the brains of patients with Alzheimer’s disease: the CERAD experience, Part XV. Neurology 46, 1592–1596. doi: 10.1212/wnl.46.6.1592
Engler, H., Forsberg, A., Almkvist, O., Blomquist, G., Larsson, E., Savitcheva, I., et al. (2006). Two-year follow-up of amyloid deposition in patients with Alzheimer’s disease. Brain 129, 2856–2866. doi: 10.1093/brain/awl178
Erskine, L., and Herrera, E. (2014). Connecting the retina to the brain. ASN Neuro 6:1759091414562107.
Feke, G. T., Hyman, B. T., Stern, R. A., and Pasquale, L. R. (2015). Retinal blood flow in mild cognitive impairment and Alzheimer’s disease. Alzheimers Demen. (Amst.) 1, 144–151.
Ferrari, L., Huang, S. C., Magnani, G., Ambrosi, A., Comi, G., and Leocani, L. (2017). Optical coherence tomography reveals retinal neuroaxonal thinning in frontotemporal dementia as in Alzheimer’s Disease. J. Alzheimers Dis. 56, 1101–1107. doi: 10.3233/jad-160886
Fischer, V. W., Siddiqi, A., and Yusufaly, Y. (1990). Altered angioarchitecture in selected areas of brains with Alzheimer’s disease. Acta Neuropathol. 79, 672–679. doi: 10.1007/bf00294246
Fisichella, V., Giurdanella, G., Platania, C. B., Romano, G. L., Leggio, G. M., Salomone, S., et al. (2016). TGF-beta1 prevents rat retinal insult induced by amyloid-beta (1-42) oligomers. Eur. J. Pharmacol. 787, 72–77. doi: 10.1016/j.ejphar.2016.02.002
Foster, N. L., Chase, T. N., Fedio, P., Patronas, N. J., Brooks, R. A., and Di Chiro, G. (1983). Alzheimer’s disease: focal cortical changes shown by positron emission tomography. Neurology 33, 961–965. doi: 10.1212/wnl.33.8.961
Frohman, E. M., Fujimoto, J. G., Frohman, T. C., Calabresi, P. A., Cutter, G., and Balcer, L. J. (2008). Optical coherence tomography: a window into the mechanisms of multiple sclerosis. Nat. Clin. Pract. Neurol. 4, 664–675.
Frost, S., Kanagasingam, Y., Sohrabi, H., Vignarajan, J., Bourgeat, P., Salvado, O., et al. (2013). Retinal vascular biomarkers for early detection and monitoring of Alzheimer’s disease. Transl. Psychiatry 3:e233. doi: 10.1038/tp.2012.150
Gabin, J. M., Tambs, K., Saltvedt, I., Sund, E., and Holmen, J. (2017). Association between blood pressure and Alzheimer disease measured up to 27 years prior to diagnosis: the HUNT Study. Alzheimers Res. Ther. 9:37.
Gao, L., Liu, Y., Li, X., Bai, Q., and Liu, P. (2015). Abnormal retinal nerve fiber layer thickness and macula lutea in patients with mild cognitive impairment and Alzheimer’s disease. Arch. Gerontol. Geriatr. 60, 162–167. doi: 10.1016/j.archger.2014.10.011
Gharbiya, M., Trebbastoni, A., Parisi, F., Manganiello, S., Cruciani, F., D’antonio, F., et al. (2014). Choroidal thinning as a new finding in Alzheimer’s disease: evidence from enhanced depth imaging spectral domain optical coherence tomography. J. Alzheimers Dis. 40, 907–917. doi: 10.3233/jad-132039
Ghiso, J., Tomidokoro, Y., Revesz, T., Frangione, B., and Rostagno, A. (2010). Cerebral Amyloid Angiopathy And Alzheimer’s Disease. Hirosaki Igaku 61, S111–S124.
Govindpani, K., Mcnamara, L. G., Smith, N. R., Vinnakota, C., Waldvogel, H. J., Faull, R. L., et al. (2019). Vascular dysfunction in Alzheimer’s Disease: a prelude to the pathological process or a consequence of it? J. Clin. Med. 8:651. doi: 10.3390/jcm8050651
Grammas, P., and Ovase, R. (2001). Inflammatory factors are elevated in brain microvessels in Alzheimer’s disease. Neurobiol. Aging 22, 837–842. doi: 10.1016/s0197-4580(01)00276-7
Gravina, S. A., Ho, L., Eckman, C. B., Long, K. E., Otvos, L. Jr., Younkin, L. H., et al. (1995). Amyloid beta protein (A beta) in Alzheimer’s disease brain. Biochemical and immunocytochemical analysis with antibodies specific for forms ending at A beta 40 or A beta 42(43). J. Biol. Chem. 270, 7013–7016.
Grimaldi, A., Brighi, C., Peruzzi, G., Ragozzino, D., Bonanni, V., Limatola, C., et al. (2018). Inflammation, neurodegeneration and protein aggregation in the retina as ocular biomarkers for Alzheimer’s disease in the 3xTg-AD mouse model. Cell Death Dis. 9:685.
Grimaldi, A., Pediconi, N., Oieni, F., Pizzarelli, R., Rosito, M., Giubettini, M., et al. (2019). Neuroinflammatory Processes. A1 astrocyte activation and protein aggregation in the retina of alzheimer’s disease patients, possible biomarkers for early diagnosis. Front Neurosci 13:925. doi: 10.3389/fnins.2019.00925
Guo, L., Duggan, J., and Cordeiro, M. F. (2010). Alzheimer’s disease and retinal neurodegeneration. Curr. Alzheimer Res. 7, 3–14. doi: 10.2174/156720510790274491
Habiba, U., Descallar, J., Kreilaus, F., Adhikari, U. K., Kumar, S., Morley, J. W., et al. (2021). Detection of retinal and blood Abeta oligomers with nanobodies. Alzheimers Demen. (Amst.) 13:e12193.
Hadoux, X., Hui, F., Lim, J. K. H., Masters, C. L., Pebay, A., Chevalier, S., et al. (2019). Non-invasive in vivo hyperspectral imaging of the retina for potential biomarker use in Alzheimer’s disease. Nat. Commun. 10:4227.
Hagag, A. M., Gao, S. S., Jia, Y., and Huang, D. (2017). Optical coherence tomography angiography: technical principles and clinical applications in ophthalmology. Taiwan J. Ophthalmol. 7, 115–129. doi: 10.4103/tjo.tjo_31_17
Harik, S. I. (1992). Changes in the glucose transporter of brain capillaries. Can. J. Physiol. Pharmacol. 70(Suppl.), S113–S117.
Harrison, I. F., Whitaker, R., Bertelli, P. M., O’callaghan, J. M., Csincsik, L., Bocchetta, M., et al. (2019). Optic nerve thinning and neurosensory retinal degeneration in the rTg4510 mouse model of frontotemporal dementia. Acta Neuropathol. Commun. 7:4.
Hart, N. J., Koronyo, Y., Black, K. L., and Koronyo-Hamaoui, M. (2016). Ocular indicators of Alzheimer’s: exploring disease in the retina. Acta Neuropathol. 132, 767–787. doi: 10.1007/s00401-016-1613-6
Hassler, O. (1965). Vascular changes in senile brains. A micro-angiographic study. Acta Neuropathol. 5, 40–53. doi: 10.1007/bf00689161
Higuchi, Y., Miyakawa, T., Shimoji, A., and Katsuragi, S. (1987). Ultrastructural changes of blood vessels in the cerebral cortex in Alzheimer’s disease. Jpn. J. Psychiatry Neurol. 41, 283–290. doi: 10.1111/j.1440-1819.1987.tb00414.x
Hinton, D. R., Sadun, A. A., Blanks, J. C., and Miller, C. A. (1986). Optic-nerve degeneration in Alzheimer’s disease. N. Engl. J. Med. 315, 485–487.
Hirsch, C., Bartenstein, P., Minoshima, S., Mosch, D., Willoch, F., Buch, K., et al. (1997). Reduction of regional cerebral blood flow and cognitive impairment in patients with Alzheimer’s disease: evaluation of an observer-independent analytic approach. Demen. Geriatr. Cogn. Disord. 8, 98–104. doi: 10.1159/000106613
Ho, C. Y., Troncoso, J. C., Knox, D., Stark, W., and Eberhart, C. G. (2014). Beta-amyloid, phospho-tau and alpha-synuclein deposits similar to those in the brain are not identified in the eyes of Alzheimer’s and Parkinson’s disease patients. Brain Pathol. 24, 25–32. doi: 10.1111/bpa.12070
Hoffman, J. M., Welsh-Bohmer, K. A., Hanson, M., Crain, B., Hulette, C., Earl, N., et al. (2000). FDG PET imaging in patients with pathologically verified dementia. J. Nucl. Med. 41, 1920–1928.
Iadecola, C., Duering, M., Hachinski, V., Joutel, A., Pendlebury, S. T., Schneider, J. A., et al. (2019). Vascular cognitive impairment and dementia: JACC scientific expert panel. J. Am. Coll. Cardiol. 73, 3326–3344.
Ikonomovic, M. D., Klunk, W. E., Abrahamson, E. E., Mathis, C. A., Price, J. C., Tsopelas, N. D., et al. (2008). Post-mortem correlates of in vivo PiB-PET amyloid imaging in a typical case of Alzheimer’s disease. Brain 131, 1630–1645. doi: 10.1093/brain/awn016
Inestrosa, N. C., Reyes, A. E., Chacon, M. A., Cerpa, W., Villalon, A., Montiel, J., et al. (2005). Human-like rodent amyloid-beta-peptide determines Alzheimer pathology in aged wild-type Octodon degu. Neurobiol. Aging 26, 1023–1028. doi: 10.1016/j.neurobiolaging.2004.09.016
Jack, C.R., Jr, Bennett, D. A., Blennow, K., Carrillo, M. C., Dunn, B., Haeberlein, S. B., et al. (2018). NIA-AA research framework: toward a biological definition of Alzheimer’s disease. Alzheimers Demen. 14, 535–562. doi: 10.1016/j.jalz.2018.02.018
Janez-Escalada, L., Janez-Garcia, L., Salobrar-Garcia, E., Santos-Mayo, A., De Hoz, R., Yubero, R., et al. (2019). Spatial analysis of thickness changes in ten retinal layers of Alzheimer’s disease patients based on optical coherence tomography. Sci. Rep. 9:13000.
Jankowsky, J. L., Xu, G., Fromholt, D., Gonzales, V., and Borchelt, D. R. (2003). Environmental enrichment exacerbates amyloid plaque formation in a transgenic mouse model of Alzheimer disease. J. Neuropathol. Exp. Neurol. 62, 1220–1227. doi: 10.1093/jnen/62.12.1220
Jessen, N. A., Munk, A. S., Lundgaard, I., and Nedergaard, M. (2015). The glymphatic system: a beginner’s guide. Neurochem. Res. 40, 2583–2599.
Jiang, H., Wei, Y., Shi, Y., Wright, C. B., Sun, X., Gregori, G., et al. (2018). Altered macular microvasculature in mild cognitive impairment and Alzheimer Disease. J. Neuroophthalmol. 38, 292–298. doi: 10.1097/wno.0000000000000580
Johnson, K. A., Fox, N. C., Sperling, R. A., and Klunk, W. E. (2012). Brain imaging in Alzheimer disease. Cold Spring Harb. Perspect. Med. 2:a006213.
Johnson, S. C., Schmitz, T. W., Moritz, C. H., Meyerand, M. E., Rowley, H. A., Alexander, A. L., et al. (2006). Activation of brain regions vulnerable to Alzheimer’s disease: the effect of mild cognitive impairment. Neurobiol. Aging 27, 1604–1612. doi: 10.1016/j.neurobiolaging.2005.09.017
Jung, N. Y., Han, J. C., Ong, Y. T., Cheung, C. Y., Chen, C. P., Wong, T. Y., et al. (2019). Retinal microvasculature changes in amyloid-negative subcortical vascular cognitive impairment compared to amyloid-positive Alzheimer’s disease. J. Neurol. Sci. 396, 94–101. doi: 10.1016/j.jns.2018.10.025
Kalaria, R. N., and Harik, S. I. (1989). Reduced glucose transporter at the blood-brain barrier and in cerebral cortex in Alzheimer disease. J. Neurochem. 53, 1083–1088. doi: 10.1111/j.1471-4159.1989.tb07399.x
Kalaria, R. N., and Kroon, S. N. (1992). Expression of leukocyte antigen CD34 by brain capillaries in Alzheimer’s disease and neurologically normal subjects. Acta Neuropathol. 84, 606–612.
Kapasi, A., and Schneider, J. A. (2016). Vascular contributions to cognitive impairment, clinical Alzheimer’s disease, and dementia in older persons. Biochim. Biophys. Acta 1862, 878–886. doi: 10.1016/j.bbadis.2015.12.023
Kashani, A. H., Chen, C. L., Gahm, J. K., Zheng, F., Richter, G. M., Rosenfeld, P. J., et al. (2017). Optical coherence tomography angiography: a comprehensive review of current methods and clinical applications. Prog. Retin. Eye Res. 60, 66–100.
Keable, A., Fenna, K., Yuen, H. M., Johnston, D. A., Smyth, N. R., Smith, C., et al. (2016). Deposition of amyloid β in the walls of human leptomeningeal arteries in relation to perivascular drainage pathways in cerebral amyloid angiopathy. Biochim. Biophys. Acta 1862, 1037–1046. doi: 10.1016/j.bbadis.2015.08.024
Kergoat, H., Kergoat, M. J., Justino, L., Chertkow, H., Robillard, A., and Bergman, H. (2001). An evaluation of the retinal nerve fiber layer thickness by scanning laser polarimetry in individuals with dementia of the Alzheimer type. Acta Ophthalmol. Scand. 79, 187–191. doi: 10.1034/j.1600-0420.2001.079002187.x
Khadamy, J., Abri Aghdam, K., and Falavarjani, K. G. (2018). An update on optical coherence tomography angiography in diabetic retinopathy. J. Ophthalmic. Vis. Res. 13, 487–497.
Kirbas, S., Turkyilmaz, K., Anlar, O., Tufekci, A., and Durmus, M. (2013). Retinal nerve fiber layer thickness in patients with Alzheimer disease. J. Neuroophthalmol. 33, 58–61. doi: 10.1097/wno.0b013e318267fd5f
Koronyo, Y., Biggs, D., Barron, E., Boyer, D. S., Pearlman, J. A., Au, W. J., et al. (2017). Retinal amyloid pathology and proof-of-concept imaging trial in Alzheimer’s disease. JCI Insight 2:e93621.
Koronyo, Y., Salumbides, B. C., Black, K. L., and Koronyo-Hamaoui, M. (2012). Alzheimer’s disease in the retina: imaging retinal abeta plaques for early diagnosis and therapy assessment. Neurodegener. Dis. 10, 285–293. doi: 10.1159/000335154
Koronyo, Y., Salumbides, B. C., Sheyn, J., Pelissier, L., Li, S., Ljubimov, V., et al. (2015). Therapeutic effects of glatiramer acetate and grafted CD115(+) monocytes in a mouse model of Alzheimer’s disease. Brain 138, 2399–2422. doi: 10.1093/brain/awv150
Koronyo-Hamaoui, M., Ko, M. K., Koronyo, Y., Azoulay, D., Seksenyan, A., Kunis, G., et al. (2009). Attenuation of AD-like neuropathology by harnessing peripheral immune cells: local elevation of IL-10 and MMP-9. J. Neurochem. 111, 1409–1424. doi: 10.1111/j.1471-4159.2009.06402.x
Koronyo-Hamaoui, M., Koronyo, Y., Ljubimov, A. V., Miller, C. A., Ko, M. K., Black, K. L., et al. (2011). Identification of amyloid plaques in retinas from Alzheimer’s patients and noninvasive in vivo optical imaging of retinal plaques in a mouse model. Neuroimage 54(Suppl. 1), S204–S217.
Kromer, R., Serbecic, N., Hausner, L., Froelich, L., Aboul-Enein, F., and Beutelspacher, S. C. (2014). Detection of retinal nerve fiber layer defects in Alzheimer’s Disease using SD-OCT. Front Psychiatry 5:22. doi: 10.3389/fpsyt.2014.00022
La Morgia, C., Ross-Cisneros, F. N., Koronyo, Y., Hannibal, J., Gallassi, R., Cantalupo, G., et al. (2016). Melanopsin retinal ganglion cell loss in Alzheimer disease. Ann. Neurol. 79, 90–109.
Lahiri, S., Regis, G. C., Koronyo, Y., Fuchs, D. T., Sheyn, J., Kim, E. H., et al. (2019). Acute neuropathological consequences of short-term mechanical ventilation in wild-type and Alzheimer’s disease mice. Crit. Care 23:63.
Lahme, L., Esser, E. L., Mihailovic, N., Schubert, F., Lauermann, J., Johnen, A., et al. (2018). Evaluation of ocular perfusion in Alzheimer’s Disease using optical coherence tomography angiography. J. Alzheimers Dis. 66, 1745–1752. doi: 10.3233/jad-180738
Lang, G. E. (2007). Optical coherence tomography findings in diabetic retinopathy. Dev. Ophthalmol. 39, 31–47. doi: 10.1159/000098498
Launer, L. J. (2002). Demonstrating the case that AD is a vascular disease: epidemiologic evidence. Ageing Res. Rev. 1, 61–77. doi: 10.1016/s0047-6374(01)00364-5
Lee, J. Y., Kim, J. P., Jang, H., Kim, J., Kang, S. H., Kim, J. S., et al. (2020). Optical coherence tomography angiography as a potential screening tool for cerebral small vessel diseases. Alzheimers Res. Ther. 12:73.
Lee, S., Jiang, K., Mcilmoyle, B., To, E., Xu, Q. A., Hirsch-Reinshagen, V., et al. (2020). Amyloid beta immunoreactivity in the retinal ganglion cell layer of the Alzheimer’s Eye. Front. Neurosci. 14:758. doi: 10.3389/fnins.2020.00758
Lemmens, S., Van Craenendonck, T., Van Eijgen, J., De Groef, L., Bruffaerts, R., De Jesus, D. A., et al. (2020). Combination of snapshot hyperspectral retinal imaging and optical coherence tomography to identify Alzheimer’s disease patients. Alzheimers Res. Ther. 12:144.
Li, M., Li, Y., Zuo, L., Hu, W., and Jiang, T. (2021). Increase of blood-brain barrier leakage is related to cognitive decline in vascular mild cognitive impairment. BMC Neurol. 21:159. doi: 10.1186/s12883-021-02189-6
Liu, B., Rasool, S., Yang, Z., Glabe, C. G., Schreiber, S. S., Ge, J., et al. (2009). Amyloid-peptide vaccinations reduce {beta}-amyloid plaques but exacerbate vascular deposition and inflammation in the retina of Alzheimer’s transgenic mice. Am. J. Pathol. 175, 2099–2110. doi: 10.2353/ajpath.2009.090159
Liu, D., Zhang, L., Li, Z., Zhang, X., Wu, Y., Yang, H., et al. (2015). Thinner changes of the retinal nerve fiber layer in patients with mild cognitive impairment and Alzheimer’s disease. BMC Neurol. 15:14. doi: 10.1186/s12883-015-0268-6
Louveau, A., Smirnov, I., Keyes, T. J., Eccles, J. D., Rouhani, S. J., Peske, J. D., et al. (2015). Structural and functional features of central nervous system lymphatic vessels. Nature 523, 337–341. doi: 10.1038/nature14432
Machulda, M. M., Ward, H. A., Borowski, B., Gunter, J. L., Cha, R. H., O’brien, P. C., et al. (2003). Comparison of memory fMRI response among normal, MCI, and Alzheimer’s patients. Neurology 61, 500–506. doi: 10.1212/01.wnl.0000079052.01016.78
Marchesi, V. T. (2011). Alzheimer’s dementia begins as a disease of small blood vessels, damaged by oxidative-induced inflammation and dysregulated amyloid metabolism: implications for early detection and therapy. FASEB J. 25, 5–13. doi: 10.1096/fj.11-0102ufm
Marmor, M. F., and Ravin, J. G. (2011). Fluorescein angiography: insight and serendipity a half century ago. Arch. Ophthalmol. 129, 943–948. doi: 10.1001/archophthalmol.2011.160
Marziani, E., Pomati, S., Ramolfo, P., Cigada, M., Giani, A., Mariani, C., et al. (2013). Evaluation of retinal nerve fiber layer and ganglion cell layer thickness in Alzheimer’s disease using spectral-domain optical coherence tomography. Invest. Ophthalmol. Vis. Sci. 54, 5953–5958. doi: 10.1167/iovs.13-12046
Matsuzaki, T., Sasaki, K., Hata, J., Hirakawa, Y., Fujimi, K., Ninomiya, T., et al. (2011). Association of Alzheimer disease pathology with abnormal lipid metabolism: the Hisayama Study. Neurology 77, 1068–1075. doi: 10.1212/wnl.0b013e31822e145d
McGrory, S., Cameron, J. R., Pellegrini, E., Warren, C., Doubal, F. N., Deary, I. J., et al. (2017). The application of retinal fundus camera imaging in dementia: a systematic review. Alzheimers Demen. (Amst.) 6, 91–107. doi: 10.1016/j.dadm.2016.11.001
Medical Advisory, S. (2009). Optical coherence tomography for age-related macular degeneration and diabetic macular edema: an evidence-based analysis. Ont. Health Technol. Assess. Ser. 9, 1–22. doi: 10.1007/978-3-642-01467-3_1
Mehndiratta, P., Manjila, S., Ostergard, T., Eisele, S., Cohen, M. L., Sila, C., et al. (2012). Cerebral amyloid angiopathy-associated intracerebral hemorrhage: pathology and management. Neurosurg. Focus 32:E7.
Mejia-Vergara, A. J., Karanjia, R., and Sadun, A. A. (2021). OCT parameters of the optic nerve head and the retina as surrogate markers of brain volume in a normal population, a pilot study. J. Neurol. Sci. 420:117213. doi: 10.1016/j.jns.2020.117213
Mirzaei, N., Shi, H., Oviatt, M., Doustar, J., Rentsendorj, A., Fuchs, D.-T., et al. (2020). Alzheimer’s retinopathy: seeing disease in the eyes. Front. Neurosci. 14:921. doi: 10.3389/fnins.2020.00921
Montagne, A., Nation, D. A., Sagare, A. P., Barisano, G., Sweeney, M. D., Chakhoyan, A., et al. (2020). APOE4 leads to blood-brain barrier dysfunction predicting cognitive decline. Nature 581, 71–76. doi: 10.1038/s41586-020-2247-3
More, S. S., Beach, J. M., Mcclelland, C., Mokhtarzadeh, A., and Vince, R. (2019). In vivo assessment of retinal biomarkers by hyperspectral imaging: early detection of Alzheimer’s Disease. ACS Chem. Neurosci. 10, 4492–4501. doi: 10.1021/acschemneuro.9b00331
Moreno-Ramos, T., Benito-Leon, J., Villarejo, A., and Bermejo-Pareja, F. (2013). Retinal nerve fiber layer thinning in dementia associated with Parkinson’s disease, dementia with Lewy bodies, and Alzheimer’s disease. J. Alzheimers Dis. 34, 659–664. doi: 10.3233/jad-121975
Morin, P. J., Abraham, C. R., Amaratunga, A., Johnson, R. J., Huber, G., Sandell, J. H., et al. (1993). Amyloid precursor protein is synthesized by retinal ganglion cells, rapidly transported to the optic nerve plasma membrane and nerve terminals, and metabolized. J. Neurochem. 61, 464–473. doi: 10.1111/j.1471-4159.1993.tb02147.x
Moschos, M. M., Markopoulos, I., Chatziralli, I., Rouvas, A., Papageorgiou, S. G., Ladas, I., et al. (2012). Structural and functional impairment of the retina and optic nerve in Alzheimer’s disease. Curr. Alzheimer Res. 9, 782–788. doi: 10.2174/156720512802455340
National Institue on Aging (2019). Alzheimer’s Disease Fact Sheet. [Online]. Available online at: https://www.nia.nih.gov/health/alzheimers-disease-fact-sheet (accessed May 22, 2019).
Newman, E., and Reichenbach, A. (1996). The Muller cell: a functional element of the retina. Trends Neurosci. 19, 307–312.
Ngolab, J., Donohue, M., Belsha, A., Salazar, J., Cohen, P., Jaiswal, S., et al. (2021). Feasibility study for detection of retinal amyloid in clinical trials: the Anti-Amyloid Treatment in Asymptomatic Alzheimer’s Disease (A4) trial. Alzheimers Dement (Amst). 13:e12199. doi: 10.1002/dad2.12199
O’Bryhim, B. E., Apte, R. S., Kung, N., Coble, D., and Van Stavern, G. P. (2018). Association of preclinical Alzheimer Disease with optical coherence tomographic angiography findings. JAMA Ophthalmol. 136, 1242–1248. doi: 10.1001/jamaophthalmol.2018.3556
Paquet, C., Boissonnot, M., Roger, F., Dighiero, P., Gil, R., and Hugon, J. (2007). Abnormal retinal thickness in patients with mild cognitive impairment and Alzheimer’s disease. Neurosci. Lett. 420, 97–99. doi: 10.1016/j.neulet.2007.02.090
Parisi, V., Restuccia, R., Fattapposta, F., Mina, C., Bucci, M. G., and Pierelli, F. (2001). Morphological and functional retinal impairment in Alzheimer’s disease patients. Clin. Neurophysiol. 112, 1860–1867.
Park, S. W., Kim, J. H., Mook-Jung, I., Kim, K. W., Park, W. J., and Park, K. H. (2014). Intracellular amyloid beta alters the tight junction of retinal pigment epithelium in 5XFAD mice. Neurobiol. Aging 35, 2013–2020. doi: 10.1016/j.neurobiolaging.2014.03.008
Parthasarathy, R., Chow, K. M., Derafshi, Z., Fautsch, M. P., Hetling, J. R., Rodgers, D. W., et al. (2015). Reduction of amyloid-beta levels in mouse eye tissues by intra-vitreally delivered neprilysin. Exp. Eye Res. 138, 134–144. doi: 10.1016/j.exer.2015.06.027
Patton, N., Aslam, T., Macgillivray, T., Pattie, A., Deary, I. J., and Dhillon, B. (2005). Retinal vascular image analysis as a potential screening tool for cerebrovascular disease: a rationale based on homology between cerebral and retinal microvasculatures. J. Anat. 206, 319–348. doi: 10.1111/j.1469-7580.2005.00395.x
Pichi, F., Sarraf, D., Arepalli, S., Lowder, C. Y., Cunningham, E. T. Jr., Neri, P., et al. (2017). The application of optical coherence tomography angiography in uveitis and inflammatory eye diseases. Prog. Retin. Eye Res. 59, 178–201. doi: 10.1016/j.preteyeres.2017.04.005
Planton, M., Raposo, N., Albucher, J. F., and Pariente, J. (2017). Cerebral amyloid angiopathy-related cognitive impairment: the search for a specific neuropsychological pattern. Rev. Neurol. (Paris) 173, 562–565. doi: 10.1016/j.neurol.2017.09.006
Polans, J., Keller, B., Carrasco-Zevallos, O. M., Larocca, F., Cole, E., Whitson, H. E., et al. (2017). Wide-field retinal optical coherence tomography with wavefront sensorless adaptive optics for enhanced imaging of targeted regions. Biomed. Opt. Express 8, 16–37. doi: 10.1364/boe.8.000016
Polo, V., Rodrigo, M. J., Garcia-Martin, E., Otin, S., Larrosa, J. M., Fuertes, M. I., et al. (2017). Visual dysfunction and its correlation with retinal changes in patients with Alzheimer’s disease. Eye (Lond.) 31, 1034–1041. doi: 10.1038/eye.2017.23
Popescu, D. P., Choo-Smith, L. P., Flueraru, C., Mao, Y., Chang, S., Disano, J., et al. (2011). Optical coherence tomography: fundamental principles, instrumental designs and biomedical applications. Biophys. Rev. 3:155. doi: 10.1007/s12551-011-0054-7
Prensa, L. (2014). Alzheimer’s Disease Risk And The Importance Of Brain Health [Online]. Available online at: https://montgomery.pa.networkofcare.org/mh/news-article-detail.aspx?id=55601 (accessed Jun 7, 2021).
Purves, D. E. A. (ed.) (2001). “The Retina, Chapters 11 and 12,” in Neuroscience, 2nd Edn (Sunderland, MA: Sinauer Associates).
Qiu, Y., Jin, T., Mason, E., and Campbell, M. C. W. (2020). Predicting thioflavin fluorescence of retinal amyloid deposits associated with Alzheimer’s Disease from their polarimetric properties. Transl. Vis. Sci. Technol. 9:47. doi: 10.1167/tvst.9.2.47
Rentsendorj, A., Sheyn, J., Fuchs, D. T., Daley, D., Salumbides, B. C., Schubloom, H. E., et al. (2018). A novel role for osteopontin in macrophage-mediated amyloid-beta clearance in Alzheimer’s models. Brain Behav. Immun. 67, 163–180. doi: 10.1016/j.bbi.2017.08.019
Rifai, O. M., Mcgrory, S., Robbins, C. B., Grewal, D. S., Liu, A., Fekrat, S., et al. (2021). The application of optical coherence tomography angiography in Alzheimer’s disease: a systematic review. Alzheimers Demen. (Amst.) 13:e12149.
Risacher, S. L., Wudunn, D., Tallman, E. F., West, J. D., Gao, S., Farlow, M. R., et al. (2020). Visual contrast sensitivity is associated with the presence of cerebral amyloid and tau deposition. Brain Commun. 2:fcaa019.
Roher, A. E., Garami, Z., Tyas, S. L., Maarouf, C. L., Kokjohn, T. A., Belohlavek, M., et al. (2011). Transcranial doppler ultrasound blood flow velocity and pulsatility index as systemic indicators for Alzheimer’s disease. Alzheimers Dement. 7, 445–455. doi: 10.1016/j.jalz.2010.09.002
Ryu, J. K., and McLarnon, J. G. (2009). A leaky blood-brain barrier, fibrinogen infiltration and microglial reactivity in inflamed Alzheimer’s disease brain. J. Cell Mol. Med. 13, 2911–2925. doi: 10.1111/j.1582-4934.2008.00434.x
Sadda, S. R., Borrelli, E., Fan, W., Ebraheem, A., Marion, K. M., Harrington, M., et al. (2019). A pilot study of fluorescence lifetime imaging ophthalmoscopy in preclinical Alzheimer’s disease. Eye (Lond.) 33, 1271–1279. doi: 10.1038/s41433-019-0406-2
Sadun, A. A., and Bassi, C. J. (1990). Optic nerve damage in Alzheimer’s disease. Ophthalmology 97, 9–17. doi: 10.1016/s0161-6420(90)32621-0
Salobrar-Garcia, E., De Hoz, R., Ramirez, A. I., Lopez-Cuenca, I., Rojas, P., Vazirani, R., et al. (2019). Changes in visual function and retinal structure in the progression of Alzheimer’s disease. PLoS One 14:e0220535. doi: 10.1371/journal.pone.0220535
Sathyan, P., Shilpa, S., and Anitha, A. (2012). Optical coherence tomography in glaucoma. J. Curr. Glaucoma Pract. 6, 1–5.
Schallek, J., Geng, Y., Nguyen, H., and Williams, D. R. (2013). Morphology and topography of retinal pericytes in the living mouse retina using in vivo adaptive optics imaging and ex vivo characterization. Invest. Ophthalmol. Vis. Sci. 54, 8237–8250.
Schneider, E. W., and Fowler, S. C. (2018). Optical coherence tomography angiography in the management of age-related macular degeneration. Curr. Opin. Ophthalmol. 29, 217–225.
Schon, C., Hoffmann, N. A., Ochs, S. M., Burgold, S., Filser, S., Steinbach, S., et al. (2012). Long-term in vivo imaging of fibrillar tau in the retina of P301S transgenic mice. PLoS One 7:e53547. doi: 10.1371/journal.pone.0053547
Schultz, N., Byman, E., Netherlands Brain, B., and Wennstrom, M. (2020). Levels of retinal amyloid-beta correlate with levels of retinal IAPP and hippocampal amyloid-beta in neuropathologically evaluated individuals. J. Alzheimers Dis. 73, 1201–1209. doi: 10.3233/jad-190868
Selkoe, D. J., and Hardy, J. (2016). The amyloid hypothesis of Alzheimer’s disease at 25 years. EMBO Mol. Med. 8, 595–608.
Sengillo, J. D., Winkler, E. A., Walker, C. T., Sullivan, J. S., Johnson, M., and Zlokovic, B. V. (2013). Deficiency in mural vascular cells coincides with blood-brain barrier disruption in Alzheimer’s disease. Brain Pathol. 23, 303–310. doi: 10.1111/bpa.12004
Shi, H., Koronyo, Y., Fuchs, D. T., Sheyn, J., Wawrowsky, K., Lahiri, S., et al. (2020a). Retinal capillary degeneration and blood-retinal barrier disruption in murine models of Alzheimer’s disease. Acta Neuropathol. Commun. 8:202.
Shi, H., Koronyo, Y., Rentsendorj, A., Regis, G. C., Sheyn, J., Fuchs, D. T., et al. (2020b). Identification of early pericyte loss and vascular amyloidosis in Alzheimer’s disease retina. Acta Neuropathol. 139, 813–836. doi: 10.1007/s00401-020-02134-w
Shi, Z., Wu, Y., Wang, M., Cao, J., Feng, W., Cheng, Y., et al. (2014). Greater attenuation of retinal nerve fiber layer thickness in Alzheimer’s disease patients. J. Alzheimers Dis. 40, 277–283. doi: 10.3233/jad-131898
Sidiqi, A., Wahl, D., Lee, S., Ma, D., To, E., Cui, J., et al. (2020). In vivo retinal fluorescence imaging with curcumin in an alzheimer mouse model. Front. Neurosci. 14:713. doi: 10.3389/fnins.2020.00713
Slemmon, J. R., Hughes, C. M., Campbell, G. A., and Flood, D. G. (1994). Increased levels of hemoglobin-derived and other peptides in Alzheimer’s disease cerebellum. J. Neurosci. 14, 2225–2235. doi: 10.1523/jneurosci.14-04-02225.1994
Smith, C. D., Andersen, A. H., Kryscio, R. J., Schmitt, F. A., Kindy, M. S., Blonder, L. X., et al. (1999). Altered brain activation in cognitively intact individuals at high risk for Alzheimer’s disease. Neurology 53, 1391–1396. doi: 10.1212/wnl.53.7.1391
Snyder, P. J., Alber, J., Alt, C., Bain, L. J., Bouma, B. E., Bouwman, F. H., et al. (2021). Retinal imaging in Alzheimer’s and neurodegenerative diseases. Alzheimers Demen. 17, 103–111.
Sweeney, M. D., Sagare, A. P., and Zlokovic, B. V. (2018). Blood-brain barrier breakdown in Alzheimer disease and other neurodegenerative disorders. Nat. Rev. Neurol. 14, 133–150. doi: 10.1038/nrneurol.2017.188
Tey, K. Y., Teo, K., Tan, A. C. S., Devarajan, K., Tan, B., Tan, J., et al. (2019). Optical coherence tomography angiography in diabetic retinopathy: a review of current applications. Eye Vis. (Lond.) 6:37.
Tonda-Turo, C., Origlia, N., Mattu, C., Accorroni, A., and Chiono, V. (2018). Current limitations in the treatment of parkinson’s and Alzheimer’s Diseases: state-of-the-art and future perspective of polymeric carriers. Curr. Med. Chem. 25, 5755–5771. doi: 10.2174/0929867325666180221125759
Torres, J. B., Andreozzi, E. M., Dunn, J. T., Siddique, M., Szanda, I., Howlett, D. R., et al. (2016). PET imaging of copper trafficking in a mouse model of Alzheimer Disease. J. Nucl. Med. 57, 109–114. doi: 10.2967/jnumed.115.162370
Trick, G. L., Barris, M. C., and Bickler-Bluth, M. (1989). Abnormal pattern electroretinograms in patients with senile dementia of the Alzheimer type. Ann. Neurol. 26, 226–231. doi: 10.1002/ana.410260208
Tripathy, D., Thirumangalakudi, L., and Grammas, P. (2007). Expression of macrophage inflammatory protein 1-alpha is elevated in Alzheimer’s vessels and is regulated by oxidative stress. J. Alzheimers Dis. 11, 447–455. doi: 10.3233/jad-2007-11405
Tsai, Y., Lu, B., Ljubimov, A. V., Girman, S., Ross-Cisneros, F. N., Sadun, A. A., et al. (2014). Ocular changes in TgF344-AD rat model of Alzheimer’s disease. Invest. Ophthalmol. Vis. Sci. 55, 523–534. doi: 10.1167/iovs.13-12888
van de Haar, H. J., Burgmans, S., Jansen, J. F., Van Osch, M. J., Van Buchem, M. A., Muller, M., et al. (2016a). Blood-brain barrier leakage in patients with early Alzheimer Disease. Radiology 281, 527–535. doi: 10.1148/radiol.2016152244
van de Haar, H. J., Jansen, J. F. A., Van Osch, M. J. P., Van Buchem, M. A., Muller, M., Wong, S. M., et al. (2016b). Neurovascular unit impairment in early Alzheimer’s disease measured with magnetic resonance imaging. Neurobiol. Aging 45, 190–196. doi: 10.1016/j.neurobiolaging.2016.06.006
van der Flier, W. M., Skoog, I., Schneider, J. A., Pantoni, L., Mok, V., Chen, C. L. H., et al. (2018). Vascular cognitive impairment. Nat. Rev. Dis. Primers 4:18003.
Vidal, D., and Mavet, S. (1989). In vitro and in vivo toxicity of T-2 toxin, a fusarium mycotoxin, to mouse peritoneal macrophages. Infect. Immun. 57, 2260–2264. doi: 10.1128/iai.57.7.2260-2264.1989
Vinters, H. V., Secor, D. L., Read, S. L., Frazee, J. G., Tomiyasu, U., Stanley, T. M., et al. (1994). Microvasculature in brain biopsy specimens from patients with Alzheimer’s disease: an immunohistochemical and ultrastructural study. Ultrastruct. Pathol. 18, 333–348. doi: 10.3109/01913129409023202
Viswanathan, A., and Greenberg, S. M. (2011). Cerebral amyloid angiopathy in the elderly. Ann. Neurol. 70, 871–880.
Vit, J. P., Fuchs, D. T., Angel, A., Levy, A., Lamensdorf, I., Black, K. L., et al. (2021). Color and contrast vision in mouse models of aging and Alzheimer’s disease using a novel visual-stimuli four-arm maze. Sci. Rep. 11:1255.
Wang, C., and Holtzman, D. M. (2020). Bidirectional relationship between sleep and Alzheimer’s disease: role of amyloid, tau, and other factors. Neuropsychopharmacology 45, 104–120. doi: 10.1038/s41386-019-0478-5
Wang, X., Lou, N., Eberhardt, A., Yang, Y., Kusk, P., Xu, Q., et al. (2020). An ocular glymphatic clearance system removes β-amyloid from the rodent eye. Sci. Transl. Med. 12:eaaw3210. doi: 10.1126/scitranslmed.aaw3210
Williams, E. A., Mcguone, D., Frosch, M. P., Hyman, B. T., Laver, N., and Stemmer-Rachamimov, A. (2017). Absence of Alzheimer Disease neuropathologic changes in eyes of subjects with alzheimer disease. J. Neuropathol. Exp. Neurol. 76, 376–383. doi: 10.1093/jnen/nlx020
Williams, M. A., Mcgowan, A. J., Cardwell, C. R., Cheung, C. Y., Craig, D., Passmore, P., et al. (2015). Retinal microvascular network attenuation in Alzheimer’s disease. Alzheimers Demen. (Amst.) 1, 229–235. doi: 10.1016/j.dadm.2015.04.001
Williams, P. A., Thirgood, R. A., Oliphant, H., Frizzati, A., Littlewood, E., Votruba, M., et al. (2013). Retinal ganglion cell dendritic degeneration in a mouse model of Alzheimer’s disease. Neurobiol. Aging 34, 1799–1806. doi: 10.1016/j.neurobiolaging.2013.01.006
Williams, S., Chalmers, K., Wilcock, G. K., and Love, S. (2005). Relationship of neurofibrillary pathology to cerebral amyloid angiopathy in Alzheimer’s disease. Neuropathol. Appl. Neurobiol. 31, 414–421. doi: 10.1111/j.1365-2990.2005.00663.x
Wolters, F. J., Zonneveld, H. I., Hofman, A., Van Der Lugt, A., Koudstaal, P. J., Vernooij, M. W., et al. (2017). Cerebral perfusion and the risk of dementia: a population-based study. Circulation 136, 719–728. doi: 10.1161/circulationaha.117.027448
Wu, J., Zhang, X., Azhati, G., Li, T., Xu, G., and Liu, F. (2020). Retinal microvascular attenuation in mental cognitive impairment and Alzheimer’s disease by optical coherence tomography angiography. Acta Ophthalmol. 98, e781–e787.
Xu, Q., Qaum, T., and Adamis, A. P. (2001). Sensitive blood-retinal barrier breakdown quantitation using evans blue. Invest. Ophthalmol. Vis. Sci. 42, 789–794.
Yan, Y., Wu, X., Wang, X., Geng, Z., Wang, L., Xiao, G., et al. (2021). The retinal vessel density can reflect cognitive function in patients with Alzheimer’s disease: evidence from optical coherence tomography angiography. J. Alzheimers Dis. 79, 1307–1316. doi: 10.3233/jad-200971
Yang, A. C., Stevens, M. Y., Chen, M. B., Lee, D. P., Stahli, D., Gate, D., et al. (2020). Physiological blood-brain transport is impaired with age by a shift in transcytosis. Nature 583, 425–430. doi: 10.1038/s41586-020-2453-z
Yang, Y., Shiao, C., Hemingway, J. F., Jorstad, N. L., Shalloway, B. R., Chang, R., et al. (2013). Suppressed retinal degeneration in aged wild type and APPswe/PS1DeltaE9 mice by bone marrow transplantation. PLoS One 8:e64246. doi: 10.1371/journal.pone.0064246
Yarchoan, M., Xie, S. X., Kling, M. A., Toledo, J. B., Wolk, D. A., Lee, E. B., et al. (2012). Cerebrovascular atherosclerosis correlates with Alzheimer pathology in neurodegenerative dementias. Brain 135, 3749–3756.
Yoon, S. P., Grewal, D. S., Thompson, A. C., Polascik, B. W., Dunn, C., Burke, J. R., et al. (2019). Retinal microvascular and neurodegenerative changes in Alzheimer’s disease and mild cognitive impairment compared with control participants. Ophthalmol. Retina 3, 489–499. doi: 10.1016/j.oret.2019.02.002
Zabel, P., Kaluzny, J. J., Wilkosc-Debczynska, M., Gebska-Toloczko, M., Suwala, K., Zabel, K., et al. (2019). Comparison of retinal microvasculature in patients with Alzheimer’s disease and primary open-angle glaucoma by optical coherence tomography angiography. Invest. Ophthalmol. Vis. Sci. 60, 3447–3455. doi: 10.1167/iovs.19-27028
Zenaro, E., Piacentino, G., and Constantin, G. (2017). The blood-brain barrier in Alzheimer’s disease. Neurobiol. Dis. 107, 41–56.
Zhang, Y. S., Zhou, N., Knoll, B. M., Samra, S., Ward, M. R., Weintraub, S., et al. (2019). Parafoveal vessel loss and correlation between peripapillary vessel density and cognitive performance in amnestic mild cognitive impairment and early Alzheimer’s disease on optical coherence tomography angiography. PLoS One 14:e0214685. doi: 10.1371/journal.pone.0214685
Zhao, H., Chang, R., Che, H., Wang, J., Yang, L., Fang, W., et al. (2013). Hyperphosphorylation of tau protein by calpain regulation in retina of Alzheimer’s disease transgenic mouse. Neurosci. Lett. 551, 12–16. doi: 10.1016/j.neulet.2013.06.026
Zhao, Z., Sagare, A. P., Ma, Q., Halliday, M. R., Kong, P., Kisler, K., et al. (2015). Central role for PICALM in amyloid-beta blood-brain barrier transcytosis and clearance. Nat. Neurosci. 18, 978–987. doi: 10.1038/nn.4025
Zipser, B. D., Johanson, C. E., Gonzalez, L., Berzin, T. M., Tavares, R., Hulette, C. M., et al. (2007). Microvascular injury and blood-brain barrier leakage in Alzheimer’s disease. Neurobiol. Aging 28, 977–986.
Keywords: cerebral amyloid angiopathy, vascular amyloidosis, eye, ocular disease, retinal imaging, blood retinal barrier, Alzheimer’s disease, neurodegenerative disease
Citation: Shi H, Koronyo Y, Rentsendorj A, Fuchs D-T, Sheyn J, Black KL, Mirzaei N and Koronyo-Hamaoui M (2021) Retinal Vasculopathy in Alzheimer’s Disease. Front. Neurosci. 15:731614. doi: 10.3389/fnins.2021.731614
Received: 27 June 2021; Accepted: 26 August 2021;
Published: 22 September 2021.
Edited by:
Tim Magnus, University of Hamburg, GermanyReviewed by:
Gareth R. Howell, Jackson Laboratory, United StatesGiovanni Luca Romano, University of Catania, Italy
Copyright © 2021 Shi, Koronyo, Rentsendorj, Fuchs, Sheyn, Black, Mirzaei and Koronyo-Hamaoui. This is an open-access article distributed under the terms of the Creative Commons Attribution License (CC BY). The use, distribution or reproduction in other forums is permitted, provided the original author(s) and the copyright owner(s) are credited and that the original publication in this journal is cited, in accordance with accepted academic practice. No use, distribution or reproduction is permitted which does not comply with these terms.
*Correspondence: Maya Koronyo-Hamaoui, bWF5YS5rb3JvbnlvQGNzbWMuZWR1