- 1Ph.D. Program in Biological and Health Sciences, Universidad Autónoma Metropolitana, Mexico City, Mexico
- 2Division of Biotechnology-Bioterio and Experimental Surgery, Instituto Nacional de Rehabilitación-Luis Guillermo Ibarra Ibarra, Mexico City, Mexico
- 3Division of Neurosciences, Instituto Nacional de Rehabilitación-Luis Guillermo Ibarra Ibarra, Mexico City, Mexico
- 4Neurophysiology, Behavior and Animal Welfare Assessment, DPAA, Universidad Autónoma Metropolitana, Mexico City, Mexico
Disabilities are estimated to occur in approximately 2% of survivors of traumatic brain injury (TBI) worldwide, and disability may persist even decades after brain injury. Facilitation or modulation of functional recovery is an important goal of rehabilitation in all patients who survive severe TBI. However, this recovery tends to vary among patients because it is affected by the biological and physical characteristics of the patients; the types, doses, and application regimens of the drugs used; and clinical indications. In clinical practice, diverse dopaminergic drugs with various dosing and application procedures are used for TBI. Previous studies have shown that dopamine (DA) neurotransmission is disrupted following moderate to severe TBI and have reported beneficial effects of drugs that affect the dopaminergic system. However, the mechanisms of action of dopaminergic drugs have not been completely clarified, partly because dopaminergic receptor activation can lead to restoration of the pathway of the corticobasal ganglia after injury in brain structures with high densities of these receptors. This review aims to provide an overview of the functionality of the dopaminergic system in the striatum and its roles in functional recovery or rehabilitation after TBI.
Introduction
Traumatic brain injury (TBI) is a main cause of disability; approximately 2% of people worldwide suffer from a TBI-related disability (Bales et al., 2009). Currently, TBI is defined as “an alteration in brain function, or other evidence of brain pathology, caused by an external force” (Menon et al., 2010). Anatomical damage, neurological deficits and mental status are common indices used to categorize TBI as mild, moderate or severe (Corrigan et al., 2010). One of the structures that is typically injured in TBI is the cerebral cortex, which is anatomically connected with other brain regions, such as the striatum, thalamus, pons and cerebellum, by afferent and efferent axons in pathways such as the corticobasal ganglia-thalamocortical and cerebellothalamocortical pathways (Daskalakis et al., 2004; Bostan and Strick, 2010; Mendoza and Merchant, 2014).
The striatum is the main input nucleus of the basal ganglia and receives glutamatergic afferents, such as motor, oculomotor, executive/associative and emotion-/motivation-related afferents, from diverse cortical areas (Reiner et al., 2010; Mathai and Smith, 2011; Kandel et al., 2013). Corticostriatal glutamatergic inputs are bilateral but have an ipsilateral predominance in the striatum (Reep et al., 2003; Lei et al., 2004; Wu et al., 2009; Reiner et al., 2010) and are critically modulated by dopamine (DA) produced by the substantia nigra pars compacta (SNc) (Bjorklund and Dunnett, 2007). TBI is associated with effects on dopaminergic transmission at the level of the striatum (Bales et al., 2009; Karelina et al., 2017; Jenkins et al., 2018; Plummer et al., 2018), such as reductions in the synthesis and release of striatal DA (Wagner et al., 2005b; Shin et al., 2011).
The literature supports the beneficial effects of dopaminergic drugs in patients with TBI, confirming that they accelerate functional recovery and rehabilitation after brain injury (Harmon and Boyeson, 1997; Bales et al., 2009; Frenette et al., 2011, 2012; Osier and Dixon, 2016a; Carrillo-Mora et al., 2017; Munakomi et al., 2017; Duong et al., 2018; Plummer et al., 2018; Loggini et al., 2020). The mechanisms of action of dopaminergic drugs administered to patients with TBI include blockade of the DA transporter (DAT), inhibition of DA reuptake and facilitation of DA synthesis, and the drugs most highly recommended by the Neurotrauma Foundation are methylphenidate (MPD), amantadine, and bromocriptine (Bales et al., 2009). However, administration of these drugs to patients with TBI is recommended only for symptomatic therapy to enhance attentional function, processing speed, and executive function (Bales et al., 2009).
The mechanisms of action of dopaminergic drugs have not been completely clarified, partly because dopaminergic receptor activation leads to restoration of neuronal circuits after injury to brain structures with high densities of these receptors. This review aims to provide an overview of the functionality of the dopaminergic system in the dorsal striatum and its role in functional recovery or rehabilitation after TBI.
Organization of the Corticostriatal Pathway
The corticostriatal pathway originates mainly in the motor and premotor cortices of the brain from pyramidal neurons located in layers V and III (Mathai and Smith, 2011). Glutamatergic cortical afferents target dendritic spines of medium spiny neurons (MSNs) in the striatum (Bolam et al., 2000; Surmeier et al., 2007; Kreitzer and Malenka, 2008; Silberberg and Bolam, 2015). In addition, the striatum receives glutamatergic afferent inputs from the thalamus, subthalamic nucleus, pedunculopontine nucleus, hippocampus, and amygdala (Smith and Parent, 1986; Doig et al., 2010; Lodge and Grace, 2011; Mathai and Smith, 2011; Koshimizu et al., 2013; Haber, 2016; Wouterlood et al., 2018; Assous et al., 2019). The main glutamatergic inputs to the MSNs are derived from the brain cortex and thalamus (Haber, 2016). Cortical inputs to the striatum are dominant with respect to thalamic inputs in terms of axonal density and synaptic interactions with MSNs (Lei et al., 2004; Smith et al., 2004; Reiner et al., 2010; Silberberg and Bolam, 2015; Haber, 2016). These corticostriatal inputs are bilateral but have an ipsilateral predominance (Reep et al., 2003; Lei et al., 2004; Wu et al., 2009; Reiner et al., 2010).
In rodents, the striatum receives corticostriatal inputs from intratelencephalic (IT) neurons and pyramidal tract (PT) neurons (Mathai and Smith, 2011; Shepherd, 2013). IT afferents are principally derived from neurons located in layer III and in upper layer V of the brain cortex (Lei et al., 2004; Mathai and Smith, 2011). In the striatum, afferent inputs from IT and PT cortical neurons target both direct and indirect pathways (Doig et al., 2010; Mathai and Smith, 2011; Silberberg and Bolam, 2015; Haber, 2016). The striatal dorsolateral area receives cortical input from the sensory-motor region, the striatal central and dorsomedial areas receive inputs from the associative cortical region, and the striatal ventromedial area receives input from the limbic region (Haber, 2016; Kupferschmidt et al., 2017).
Distribution and Function of DA Receptors
The DA receptor family includes D1-like (D1 and D5) and D2-like (D2, D3, and D4) subtypes (Bunzow et al., 1988; Sunahara et al., 1991; Van Tol et al., 1991; Missale et al., 1998; Vallone et al., 2000). In the cerebral cortex, autoradiographic localization studies have shown that both rodent and primate cortices contain D1 receptors (D1Rs) and D2 receptors (D2Rs) (Camps et al., 1990), but the density of D1Rs is 10–20-fold higher than that of D2Rs (Lidow et al., 1991). In addition, the highest ratio of mRNA expression of D1Rs compared with D2Rs is found in the prefrontal cortex (Santana and Artigas, 2017), where both D1Rs and D2Rs are expressed in glutamatergic and GABAergic neurons (Figure 1A; Santana and Artigas, 2017). As previously reported (Abekawa et al., 2000), D1R activation reduces extracellular glutamate and GABA levels in the medial prefrontal cortex. In one study, D1R activation of interneurons led to decreased activity of the corticostriatal pathway (Muly et al., 1998). Other studies have documented the localization of D1Rs in corticostriatal pyramidal neurons, while some authors reported that the effects of D2R activation are confined to type I corticopontine neurons of layer V (Gaspar et al., 1995; Leyrer-Jackson and Thomas, 2017). Similar to the case in the striatum, D2Rs in the prefrontal cortex are restricted to specific neural populations (Gee et al., 2012). In addition, D2Rs are localized in the axons of dopaminergic afferents (Bjorklund and Dunnett, 2007).
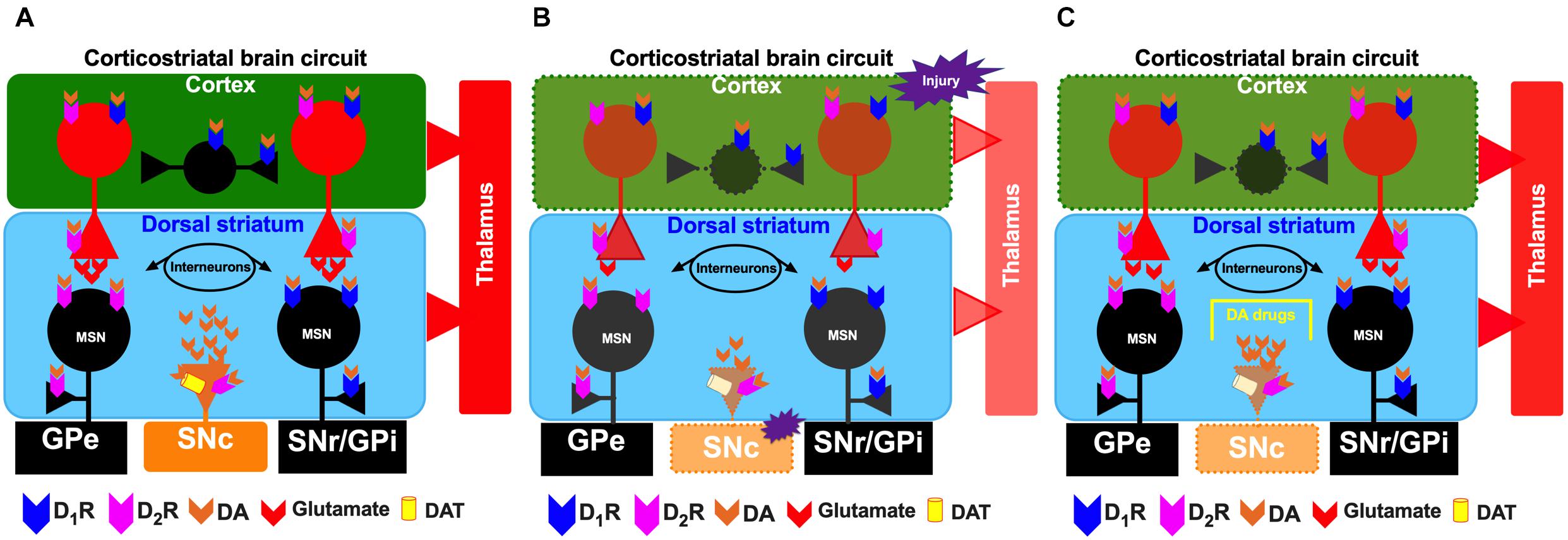
Figure 1. Schematic representation of the corticostriatal pathway under normal conditions (A), under conditions of cortical injury (B), and during the injury recovery process/the proposed effects of administration of dopaminergic drugs (C). For the circles, triangles and lines, red indicates excitatory projections, whereas black indicates inhibitory projections. The color orange represents the substantia nigra pars compacta (SNc). The dotted lines indicate depletion by injury in the cerebral cortex and SNc. SNr, substantia nigra pars reticulata; GPi, internal segment of the globus pallidus; GPe, external segment of the globus pallidus; STN, subthalamic nucleus.
In the striatum, a subpopulation of MSNs known as striatonigral neurons express D1-like receptors, predominantly D1Rs, at high levels (Bergson et al., 1995; Le Moine and Bloch, 1995; Surmeier et al., 1996); however, D2Rs are selectively expressed in a second subpopulation, the striatopallidal MSNs (Le Moine and Bloch, 1995; Valjent et al., 2009). Activation of pre- and postsynaptic D1Rs and D2Rs is associated with modulation of corticostriatal glutamatergic transmission and synaptic plasticity in corticostriatal synapses (Kerkerian et al., 1987; Hsu et al., 1995; López De Maturana and Sánchez-Pernaute, 2010). Functionally, striatal D1Rs and D2Rs, but not extrastriatal DA receptors, are responsible for dopaminergic motor stimulation (Wang and Zhou, 2017). As shown in our recent studies, D1R activation maintains motor coordination and balance in both normal and injured rats (Avila-Luna et al., 2018a, b).
Neuronal Death and Axonal Injury in Neural Groups After TBI
Primary injury is characterized locally by destruction of brain tissue, including neuronal and glial destruction originating from intracranial hemorrhage, epidural and subdural hematomas, brain contusions and direct mechanical injury (Eakin et al., 2013; Loane and Kumar, 2016; Lu et al., 2019; Shi et al., 2019). Primary brain injury leads to secondary events, including hypoxia, excitotoxicity, free radical generation, vascular dysfunction, apoptotic cell death, the inflammatory response and brain edema (Eakin et al., 2013; Chandran et al., 2018; Lu et al., 2019; Ng and Lee, 2019; Shi et al., 2019). As consequences of this damage, the concentrations of neurotransmitters, including monoamines (DA, norepinephrine, and serotonin) (Massucci et al., 2004; Bueno-Nava et al., 2008, 2010b; Huang et al., 2014a; Chen et al., 2015b, 2017b) and amino acids (GABA and glutamate) (Krobert et al., 1994; Bueno-Nava et al., 2008, 2010a; Shin et al., 2011; Guerriero et al., 2015), change in other brain structures. Glutamate release is associated with excitotoxicity following TBI, which leads to neuronal death (Guerriero et al., 2015), and elevated glutamate levels that persist over time (up to 4 days) are associated with a high mortality rate (23.6%) or with poor functional recovery in patients with injury (Chamoun et al., 2010).
Other consequences of brain injury are alterations in adjacent or distant neural groups with respect to the brain cortex, including structures that are anatomically related to the injured site, such as the striatum, cerebellum, pons, and hippocampus (Dunn-Meynell and Levin, 1997; Reep and Corwin, 1999; Van Vleet et al., 2003; Park et al., 2007; Bueno-Nava et al., 2008, 2010b; Doig et al., 2010; Kernie and Parent, 2010; Wiley et al., 2016; Shipp, 2017; Gálvez-Rosas et al., 2019). Therefore, brain injury results in disruption of the connectivity of the corticostriatal, corticohypothalamic, corticopontocerebellar, and cerebellothalamocortical pathways (Leergaard, 2003; Daskalakis et al., 2004; Shepherd, 2013). Other neural groups, such as the dopaminergic, noradrenergic, histaminergic and serotonergic systems, are altered by local axonal injury in brain contusions and direct mechanical injury (Bueno-Nava et al., 2008, 2010b; Eakin et al., 2013; Jenkins et al., 2016, 2018; Avila-Luna et al., 2019; Liao et al., 2019; Dougherty et al., 2020).
Striatal Dopaminergic Disruption After TBI
Primary cortical injury also involves local destruction of dopaminergic axons, a critical factor in the disruption of striatal dopaminergic signaling after TBI (Chen et al., 2017a; Karelina et al., 2017; Jenkins et al., 2018; Lu et al., 2019; Dougherty et al., 2020). As mentioned above, several events occur during secondary injury, but one event of interest in our review is the disruption of the nigrostriatal dopaminergic system at the striatal level (Figure 1B).
In the dorsal striatum, TBI is associated with effects on dopaminergic transmission on the side ipsilateral to the injury, such as reductions in the synthesis and release of striatal DA (Figure 1B; Wagner et al., 2005b; Shin et al., 2011; Van Bregt et al., 2012; Huang et al., 2014a; Chen et al., 2015b), subacute and chronic deficits in tyrosine hydroxylase activity (Wagner et al., 2005b; Hutson et al., 2011; Shin et al., 2011) and decreases in DAT levels in the striatum ipsilateral and/or contralateral to the injury (Donnemiller et al., 2000; Wagner et al., 2005a, b, 2014; Karelina et al., 2017). All previously mentioned studies indicate injury to dopaminergic neuronal cell bodies in the SNc (Jenkins et al., 2018). Patients with moderate-severe TBI reduced striatal DAT levels in the caudate nucleus (Figure 1B; Jenkins et al., 2018).
In striatonigral MSNs, D1R activation increases the phosphorylation of the cAMP-regulated protein of 32 kDa (DARPP-32) at Thr34 (p-DARPP-32-Thr34) (Langley et al., 1997; Greengard et al., 1999; Calabresi et al., 2000; Undieh, 2010; Rangel-Barajas et al., 2015). Bales et al. (2011) showed that TBI decreases p-DARPP-32-Thr34 levels on both sides of the striatum and that the decrease in Thr34 phosphorylation is due to increased activity of protein phosphatase-1 (PP-1). DARPP-32 is a phosphoprotein regulated by DA, and one explanation for the decrease in DARPP-32 phosphorylation at Thr34 is a decrease in DA signaling, while the increased activity of PP-1 is associated with an alteration in PKA (Bales et al., 2011). Molecular studies in our laboratory on rats that had recovered from motor deficits at 192 h after cortical injury showed that D1R mRNA expression was decreased in the striatum ipsilateral to the injury site. This reduction in D1R mRNA expression was reversed by systemic administration of the D1R agonist SKF-38393 (2 mg/kg), an effect that was blocked by the D1R antagonist SCH-23390 (Gálvez-Rosas et al., 2019). In another study, we evaluated the effect of SKF-38393 on spontaneous motor activity in normal rats and found that it increased both the distance traveled and horizontal counts (Figure 2; Avila-Luna et al., 2018b). This increased locomotion was prevented by coadministration of SCH-23390; however, administration of SCH-23390 alone decreased both the distance traveled and the horizontal counts (Figure 2; Avila-Luna et al., 2018b). In this same study, we showed that D1Rs mediated the SCH-23390-induced deficits in motor coordination and spontaneous motor activity and that the effect was reversed upon subsequent administration of the full D1R agonist SKF-82958 (Avila-Luna et al., 2018a, b). However, the use of a D1R agonist did not accelerate motor recovery, although an intact striatum may be necessary to achieve recovery at 192 h postinjury (Avila-Luna et al., 2018a). Few studies have investigated neuronal injury in the striatum in humans and animals after TBI (Primus et al., 1997; Huang et al., 2018); more information on this type of injury is needed to better interpret the changes in D1R expression and function that occur in the striatum after TBI.
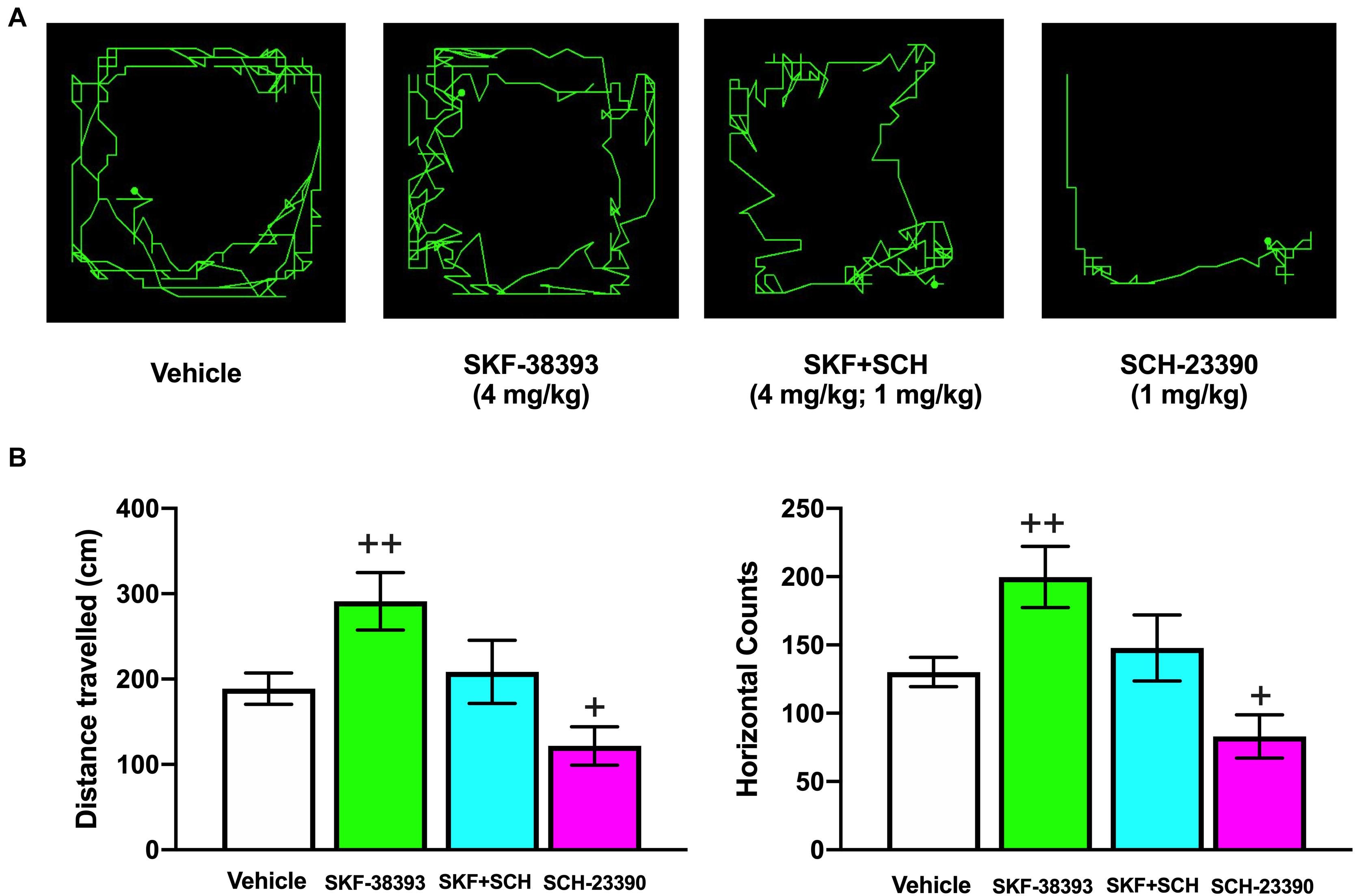
Figure 2. Effects of systemic administration of SKF-38393 alone, coadministration of SKF-38393 + SCH-23390 and administration of SCH-23390 alone on horizontal movement (distance traveled) and vertical movement (rearing behavior) in the spontaneous motor activity test. Representative maps for the distance traveled in each treatment group obtained at 20 or 40 min after drug administration (A). Analysis of the groups (B). The values are reported as the means ± SEMs. The statistical analyses were performed using one-way ANOVA followed by Dunnett’s test. +P < 0.05 and ++P < 0.02 compared with the vehicle group. The results shown have been reported previously (Avila-Luna et al., 2018b).
Another subpopulation of striatopallidal MSNs expresses D2-like receptors (D2Rs), which were recently reported to be altered in humans after TBI (Jolly et al., 2019) and have been studied and verified in animal models (Karelina et al., 2017). Immunohistochemistry of mouse tissues has indicated that D2R expression is decreased in the striatum ipsilateral to the injury site (Karelina et al., 2017), but such a decrease can be reversed by exercise on a motorized treadmill in rats that are exercised after injury, as reported by Ko et al. (2019). Two binding studies using single-photon or positron emission tomography (PET) have shown that striatal D2R binding is reduced in human patients with TBI (Donnemiller et al., 2000; Jolly et al., 2019). Another study has shown that caudate and putamen binding is reduced in patients with TBI and a length polymorphism near the D2R gene (Wagner et al., 2014). Low binding may be associated with nigrostriatal fiber loss (Donnemiller et al., 2000; Wagner et al., 2014).
The abovementioned evidence suggests that the dopaminergic system in the striatum is altered after TBI and that functional recovery is modulated by a D1R-dependent mechanism (Davis et al., 2007). Nevertheless, further studies on the striatum are needed to determine D2R function after TBI.
Mechanisms of Action of Dopaminergic Drugs and Their Beneficial Effects on Brain Injury
Due to the high levels of D1R and D2R expression in the striatum and the strong connectivity of cortical glutamatergic afferents with both MSNs and dopaminergic afferents, alterations in this neuronal population may contribute to selective neuronal dysfunction and degeneration after brain injury (Jackson and Westlind-Danielsson, 1994; Le Moine and Bloch, 1995; Surmeier et al., 1996, 2007; Gerfen and Surmeier, 2011; Guerriero et al., 2015). Previous studies have shown that drugs that modulate dopaminergic transmission elicit favorable results during rehabilitation of patients with TBI, such as enhanced cognitive recovery, executive function, attentional function, processing speed and memory (Figure 1C; Harmon and Boyeson, 1997; Bales et al., 2009; Frenette et al., 2011, 2012; Osier and Dixon, 2016a; Plummer et al., 2018). Dopaminergic drugs administered to patients with TBI have various mechanisms of action, such as blockade of DAT, inhibition of DA reuptake and facilitation of DA synthesis. MPD, amantadine and bromocriptine are among the drugs most highly recommended by the Neurotrauma Foundation (Bales et al., 2009).
Effects on DA Reuptake and the DAT
Catecholamine levels in the brain are increased in the first few hours after brain injury but decrease thereafter (Huger and Patrick, 1979; Dunn-Meynell et al., 1994; Levin et al., 1995; Osier and Dixon, 2016a). Striatal DA levels are altered in this way (Chen et al., 2015b, 2017b), and the alterations are accompanied by decreases in local and striatal DAT expression from the first to fourth weeks after cortical injury (Wagner et al., 2005a, b). The dorsal striatum is innervated by dopaminergic afferents from the SNc, which form synaptic contacts with dendritic spine necks on MSNs (Freund et al., 1984), and DAT is localized in the cell membrane of dopaminergic axons and terminals (Hersch et al., 1997). Focal cortical injury leads to loss of MSN spines and to decreases in synaptic contacts (Kemp et al., 1971; Cheng et al., 1997; Neely et al., 2007). In this context, imaging results in humans (Donnemiller et al., 2000) have been consistent with western blot findings (Wagner et al., 2005a, b), indicating that striatal DAT levels are decreased after brain injury (Wagner et al., 2005b). However, DAT inhibitors including amphetamine (Feeney et al., 1982; Hovda et al., 1987; Rau et al., 2016; Duong et al., 2018), MPD (Kline et al., 1994, Kaelin et al., 1996, Kline et al., 2000; Arciniegas et al., 2002; Weber and Lütschg, 2002; Demarchi et al., 2005; Wagner et al., 2007; Wortzel and Arciniegas, 2012; Ekinci et al., 2017), and amantadine (Arciniegas et al., 2002; Demarchi et al., 2005; Wu and Garmel, 2005; Wortzel and Arciniegas, 2012; Bleimeister et al., 2019; Okigbo et al., 2019; Loggini et al., 2020) have been used in animals and humans to evaluate the neuroprotective and symptomatic effects associated with improved recovery after brain injury. MPD and amantadine are clinically relevant treatments for TBI during the acute and chronic phases, including during the rehabilitative period (Arciniegas et al., 2002; Demarchi et al., 2005; Warden et al., 2006; Bales et al., 2009). In patients with TBI, administration of these drugs ameliorates neurocognitive impairments in attention, memory and executive function (Arciniegas et al., 2002; Rau et al., 2016). One explanation for the clinical use of DAT inhibitors despite the abnormally low DAT levels, is associated with changes in catecholamines after TBI (Osier and Dixon, 2016a), particularly decreases in catecholamine levels (Levin et al., 1995; Chen et al., 2015a, 2017b; Osier and Dixon, 2016a).
DA Synthesis and Release
Functional neuroimaging results have shown that the substantia nigra is altered after cortical injury in TBI patients (Jenkins et al., 2018; Lu et al., 2019) and that this alteration can promote progressive degeneration of nigrostriatal dopaminergic neurons (Figure 1B; Hutson et al., 2011; Van Bregt et al., 2012). Subsequently, it leads to alterations in dopaminergic signaling in the striatum, including reductions in the synthesis and release of striatal DA (Wagner et al., 2005b; Shin et al., 2011; Van Bregt et al., 2012; Huang et al., 2014a; Chen et al., 2015b) and subacute and chronic deficits in tyrosine hydroxylase activity (Wagner et al., 2005b; Hutson et al., 2011; Shin et al., 2011). Levodopa is a DA precursor drug that is not only used to treat Parkinson’s disease but also administered to patients with stroke, as it exerts beneficial effects on motor recovery and cognitive function (Liepert, 2008; Seniów et al., 2009). In patients with TBI, levodopa is used to treat cognitive impairment (Arciniegas et al., 2002) and altered-consciousness states (Krimchansky et al., 2004; Matsuda et al., 2005). Clinical studies administering levodopa alone have been scarce and have included a limited number of patients (Demarchi et al., 2005); however, in some of these studies, administration of levodopa has improved the cognitive function and behaviors of all patients (Lal et al., 1988). Other studies have reported some beneficial effects of levodopa in clinical cases (Demarchi et al., 2005). For example, levodopa can be combined with other drugs, such as pramipexole, ropinirole, amantadine and bromocriptine, to improve apraxia (Jang et al., 2018; Choi et al., 2020). To exert its effects, levodopa increases striatal DA levels, which can lead to changes in signaling at DA receptors, including both D1Rs and D2Rs (De La Fuente-Fernandez et al., 2004; Aubert et al., 2005; Guigoni et al., 2005).
D2Rs are localized on dopaminergic axons (Bjorklund and Dunnett, 2007), in striatopallidal MSNs, in cholinergic interneurons and on corticostriatal terminals in the striatum (Le Moine and Bloch, 1995; Kreitzer and Malenka, 2008; Valjent et al., 2009). Bromocriptine is an ergot alkaloid and D2R-selective agonist that is known to enhance cognitive function in the chronic recovery period after brain injury (Ozga et al., 2018). In the traditional mechanism, DA D2 autoreceptor activation by agonist drugs at striatal presynaptic sites inhibits DA release (Brannan et al., 1993; L’hirondel et al., 1998; Schmitz et al., 2002, 2003) and synthesis (Schmitz et al., 2003; Yoshida et al., 2006). However, postsynaptic D2R activation modulates the functions of GABAergic MSNs and interneurons (Surmeier et al., 2007; Keeler et al., 2014). Notably, low (2.5 mg/kg) and medium (5 mg/kg) doses of bromocriptine increase DA release, an effect associated with the action of bromocriptine as a partial antagonist (Brannan et al., 1993). Compared with healthy control patients, who exhibit improved working memory, patients with mild TBI have altered responsivity to DA after administration of 1.25 mg of bromocriptine at 1 month after brain injury (Mcallister et al., 2011). In one study, administration of doses of 1.25 or 2.5 mg of bromocriptine 2 times daily resulted in enhanced functional recovery in patients with TBI who were in a vegetative state; however, the authors recognized the small sample size analyzed as a limitation of the study (Passler and Riggs, 2001). As mentioned above, bromocriptine is a D2R agonist but may also act as a partial antagonist (Lieberman and Goldstein, 1985), and some studies have shown a protective effect of bromocriptine against oxidative stress (Kline et al., 2004; Bales et al., 2009; Osier and Dixon, 2016a).
Dopaminergic Mechanism of Action in Functional Recovery After Brain Injury
The mechanisms of action of the dopaminergic drugs used to treat TBI have not been completely clarified, primarily because dopaminergic receptor activation may lead to restoration of neuronal circuits after injury in brain structures with high densities of these receptors. Dopaminergic abnormalities following TBI are clearly associated with functional disability in animals and humans (Bales et al., 2009; Osier and Dixon, 2016a, b; Jenkins et al., 2018; Traeger et al., 2020; Tsuda et al., 2020), and drugs with dopaminergic actions are beneficial for functional recovery after brain injury (Meythaler et al., 2002; Matsuda et al., 2005; Frenette et al., 2012; Huang et al., 2014b; Duong et al., 2018; Lan et al., 2019; Okigbo et al., 2019; Loggini et al., 2020). In the striatum, activation of D1Rs and D2Rs on MSNs is associated with increased and decreased excitability, respectively, in response to corticostriatal glutamatergic inputs (Wickens and Wilson, 1998; Surmeier et al., 2007; Gerfen and Surmeier, 2011). The classic model of basal ganglia function (Figure 1A) suggests that glutamatergic corticostriatal projections are critical for the activity of striatonigral and striatopallidal MSNs that form the direct and indirect pathways in the basal ganglia, respectively (Albin et al., 1989). Alterations in dopaminergic function in the striatum are associated with motor disability, such as that observed in individuals with Parkinson’s disease and levodopa-induced dyskinesia (Calabresi et al., 2014; Bastide et al., 2015; Fundament et al., 2016; Schonfeld et al., 2017; Avila-Luna et al., 2019). Therefore, some components of TBI pathophysiology likely have a basis in striatal dopaminergic dysfunction based on clinical and experimental evidence obtained from the basal ganglia, particularly the dorsal striatum (Figure 1B; Donnemiller et al., 2000; Costa et al., 2006; Yan et al., 2007; Bales et al., 2009, 2011; Van Bregt et al., 2012; Osier and Dixon, 2016a; Karelina et al., 2017; Treble-Barna et al., 2017; Jenkins et al., 2018; Jolly et al., 2019). Similar to Parkinson’s disease, TBI leads to a hypodopaminergic state in the long term (Wagner et al., 2014; Jenkins et al., 2016; Karelina et al., 2017; Fridman et al., 2019). Various drugs have been used to counteract the adverse effects of the hypodopaminergic state, including DA agonists such as bromocriptine and amantadine, dopaminergic drugs used for Parkinson’s disease; stimulants; and reuptake inhibitors (Figure 1C and Table 1; Osier and Dixon, 2016a; Fridman et al., 2019).
Functional Interactions Between DA and Other Neurotransmission Systems in the Striatum
In the striatum, MSN heteroreceptors are localized in the cell membrane, including DA receptors (D1Rs and D2Rs), histamine receptors (H3Rs), muscarinic receptors (M1 and M4), adenosine receptors (A1Rs and A2ARs), cannabinoid receptors (CB1Rs), metabotropic glutamate receptors (mGlu1/5Rs), and ionotropic glutamate receptors [N-methyl-D-aspartic acid (NMDA) receptors, NMDARs] (Jones et al., 2001; Meschler and Howlett, 2001; Kreitzer and Malenka, 2008; Bolam and Ellender, 2016; Rapanelli, 2017). As mentioned above with regard to the role of DA after TBI and during functional recovery, studies have shown that functional interactions exist between DA receptors and other heteroreceptors localized in MSNs of the striatum, such as D1R/A1R (Fuxe et al., 2008, 2010), D1R/D3R/A1R (Fuxe et al., 2008), D1R/D3R (Fuxe et al., 2008; Marcellino et al., 2008), D1R/H3R (Garcia et al., 1997; Ferrada et al., 2009; Moreno et al., 2011; Avila-Luna et al., 2019), D2R/A2AR (Fuxe et al., 2008, 2010; Ferré et al., 2016; Ferré and Ciruela, 2019), D2R/H3R (Humbert-Claude et al., 2007; Ferrada et al., 2008; Rapanelli et al., 2016; Rapanelli, 2017), D2R/A2AR/mGlu5R (Fuxe et al., 2008, 2010), D2R/NMDA (Fuxe et al., 2008), D2R/CB1R (Fuxe et al., 2008), and D2R/CB1R/A2AR (Meschler and Howlett, 2001; Fuxe et al., 2008, 2010) interactions (see Figure 3). Most of these functional interactions regulate neuronal activity via the adenylyl cyclase-mediated response in MSNs from both direct and indirect pathways of the basal ganglia (see Figure 3), but other interactions, such as D2R/NMDAR interactions, are associated with modulation of transmembrane ion currents (Fuxe et al., 2008). One explanation for the relevance of functional interactions between receptors to TBI-mediated effects involves the D1R/H3R functional interaction in MSNs, as it has been reported that H3R activation by the agonist immepip selectively inhibits the component of depolarization-evoked GABA release that depends on concomitant D1R stimulation in rat striatum and substantia nigra pars reticulata (SNr) slices (Garcia et al., 1997; Arias-Montaño et al., 2001). At the postsynaptic level, H3R activation inhibits D1R-induced cAMP formation in rat striatal slices (Sanchez-Lemus and Arias-Montano, 2004), and D1Rs play a permissive role in H3R-mediated activation of mitogen-activated protein kinases (MAPKs) (Moreno et al., 2011). At the motor behavior level, chronic H3R activation reduces dyskinesias induced by L-Dopa (L-3,4-dihydroxyphenylalanine) in rats with 6-hydroxydopamine-induced lesions (Avila-Luna et al., 2019). All of the functional interactions mentioned above may have important implications for future research on TBI and its treatment.
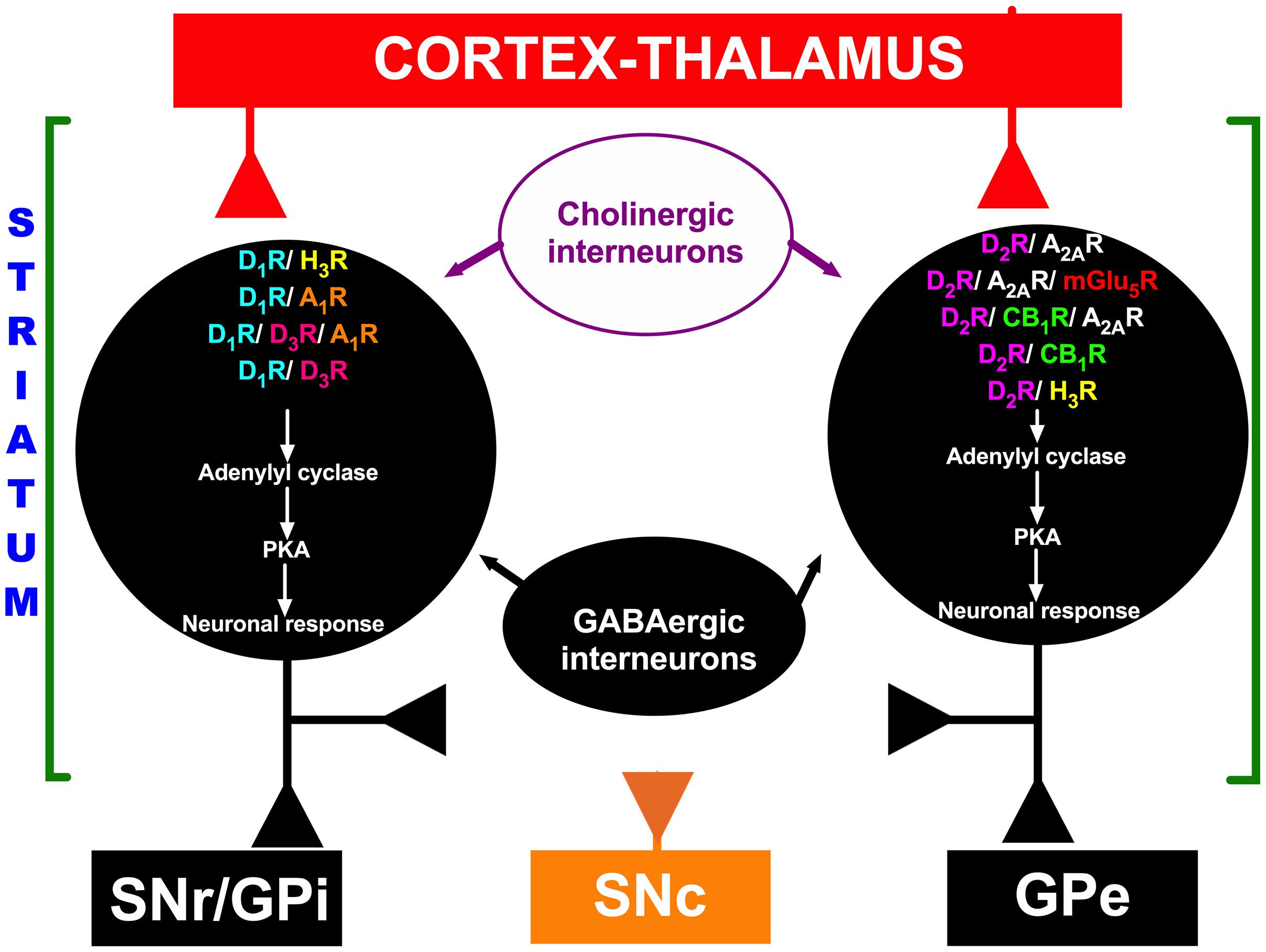
Figure 3. Schematic representation of functional interactions between DA receptors and other heteroreceptors localized in medium spiny neurons (MSNs). Red indicates excitatory projections, and black indicates inhibitory projections. In the striatum, GABAergic MSNs communicate with neurons in the substantia nigra pars reticulata (SNr) or internal segment of the globus pallidus (GPi) through a direct pathway and to the external segment of the globus pallidus (GPe); the GPe in turn projects to the subthalamic nucleus (STN), which projects to the SNr, forming the indirect pathway. The orange color represents the substantia nigra pars compacta (SNc). D1R, D1 receptor; D2R, D2 receptor; A1R, A1 receptor; A2R, A2 receptor; H3R, H3 receptor; D3R, D3 receptor; mGlu5R, mGlu5 receptor; CB1R, CB1 receptor; PKA, protein kinase A.
Clinical Functional Recovery Associated With the Dopaminergic System
By ∼10 or ∼20 years after injury, most patients with moderate and severe TBI show good recovery or moderate disability (Andelic et al., 2018). Facilitation or modulation of functional recovery is an important goal of rehabilitation in all patients with severe TBI, but the degree of recovery varies among patients due to factors associated with the injury and to the patients’ biological and physical characteristics. One common limitation in the published studies is a small sample size. In this context, we considered illustrative examples from studies included in this review that provide evidence for an association between functional recovery and the dopaminergic system.
Pharmacological and physical rehabilitation is commonly utilized to treat patients with TBI (Horn et al., 2015; Bhatnagar et al., 2016; Ng and Lee, 2019; Traeger et al., 2020). As mentioned previously, dopaminergic drugs are often administered after brain injury. The evidence for an association of the dopaminergic system with functional recovery is described below. In the dopaminergic system, the nigrostriatal pathway is implicated in spatial learning/memory, reward processing and cognitive function, whereas the mesocorticolimbic pathway is associated with memory consolidation, motivation and addiction (Osier and Dixon, 2016a). A review by Osier and Dixon has suggested that therapies targeting the catecholaminergic system may attenuate functional limitations after moderate and severe TBI (Osier and Dixon, 2016a) and functional disability that may persist even decades after brain injury (Andelic et al., 2018).
Evidence indicates that DA neurotransmission is disrupted following moderate and severe TBI, and benefits associated with dopaminergic system-affecting drugs have been reported (Passler and Riggs, 2001; Meythaler et al., 2002; Sawyer et al., 2008; Bales et al., 2009; Jenkins et al., 2016, 2018; Osier and Dixon, 2016a; Lan et al., 2019). For example, administration of amantadine at 100–200 mg (twice a day) to patients with TBI appears to be beneficial in promoting intermediate-term cognitive recovery (Loggini et al., 2020). Administration of methamphetamine, which is highly addictive and is of limited usefulness due to its potential for abuse and dependence, to patients with TBI improves Glasgow Coma Scale (GCS) and Glasgow Outcome Scale (GOS) scores, whereas in injured rats, it improves motor and cognitive performance in a dose-dependent manner (Duong et al., 2018). Bromocriptine enhances arousal in patients with TBI who are in a minimally conscious state and improves neurological sequelae associated with hemiparesis (56% of cases), aphasia (80%), memory (50%), and cognitive impairment (67%) (Munakomi et al., 2017). Administration of MPD at a dose of 0.3 mg/kg (∼2.5 mg) exerts positive effects on attention deficits after TBI (Whyte et al., 2004).
Clinical Evidence Associated With the Dopaminergic System
Clinically, a variety of drugs with dopaminergic effects are used for TBI. These drugs have diverse dosing and application regimens and clinical indications. A prudent approach would be to first describe two main categories of drugs that differ on the basis of their clinical use. The first category includes drugs that are used as adjuvant stimulant treatments during the rehabilitation or recovery period in patients with TBI. The expected (at least theoretically) effects of these drugs in modulating neuroplasticity processes are cumulative and long-term; such effects result in improved functional recovery of patients during follow-up (Ghalaenovi et al., 2018). The second category includes drugs that are administered to treat any symptoms or complications that patients are currently experiencing, for example, behavioral disturbances (aggressiveness and irritability), mood or motivational disorders (depression, apathy, etc.) and cognitive complaints (attention, posttraumatic amnesia, etc.) (Ter Mors et al., 2019).
The drugs that have been most commonly used in the clinic include amantadine, levodopa, amphetamine, MPD, bromocriptine and pergolide, among others. These drugs differ widely in their pharmacokinetic properties, mechanisms of action and selectivity for the dopaminergic system (Table 1; Lan et al., 2019). On the other hand, drugs with dopaminergic action have been used to treat various clinical conditions; for example, stimulants have been used in patients with disorders of consciousness, including patients with unresponsive wakefulness syndrome and patients in a minimally conscious state (Gao et al., 2020); for the treatment of some cognitive complaints such as attention disorders, posttraumatic amnesia, and executive or working memory dysfunction (Whyte et al., 2008); for management of behavior or mood alterations, such as aggressiveness, apathy, and agitation (Hammond et al., 2017); for stimulation of motor recovery; and even for management of autonomic dysfunctions secondary to TBI (Ghalaenovi et al., 2018). These drugs have mainly been administered to patients with severe TBI sequelae; however, reports have also described their use in patients with mild TBI (Iaccarino et al., 2020). The evolution of the injury is also highly variable, namely, the evolution from the acute or subacute stage to the chronic stage, which undoubtedly influences the clinical results obtained (Hammond et al., 2017). Additionally, the duration of treatment and the doses used have varied widely among different studies. For some drugs, such as amantadine, systematic reviews or meta-analyses have been published because numerous studies are available (Loggini et al., 2020); in contrast, for other drugs, only anecdotal reports of their use in case reports or case series are available. Finally, although multiple studies have shown positive effects of dopaminergic drugs, studies have also reported negative results; thus, the evidence is not entirely consistent in this regard (Hammond et al., 2017). A detailed description of each of the dopaminergic drugs and their clinical effects on TBI is beyond the scope of this review, but some recent publications on this topic are available (Carrillo-Mora et al., 2017; Bradley and Damiano, 2019). This variability among studies increases the difficulty of reaching categorical conclusions on the usefulness of dopaminergic drugs for TBI treatment in the clinical setting; however, the currently available evidence appears to suggest that they improve some disorders in patients with TBI, such as attention and alertness disorders, aggressiveness, and other behavioral symptoms. However, more clinical studies are needed to provide stronger support for their positive effects.
It is very important to emphasize that in the clinical management of TBI patients, in addition to using dopamine agonists for different purposes, clinicians frequently use dopamine antagonists (antipsychotics; either typical or atypical, which have different selectivities for D1Rs and D2Rs) to control some acute symptoms/complications, such as agitation, delirium, hallucinations and psychosis (Polich et al., 2019; Williamson et al., 2019). However, also importantly, there is experimental and clinical evidence that some of these dopaminergic blockers may have negative effects on neuroplasticity processes, affecting motor or cognitive recovery in patients with brain damage (Phelps et al., 2015; Folweiler et al., 2017). In this sense, previous studies have suggested that atypical antipsychotics have better safety profiles than typical antipsychotics (Phelps et al., 2017). Interestingly, this evidence regarding the negative effects of dopamine antagonists on motor or cognitive recovery from brain damage also indirectly supports the hypothesis that dopamine stimulation is a good therapeutic strategy for stimulation of motor and cognitive recovery after TBI; however, more studies are needed to confirm or rule out these negative effects of antipsychotic drugs.
Conclusion
Facilitation or modulation of functional recovery is an important goal of rehabilitation in all patients after severe TBI, but the degree of recovery varies among patients due to factors associated with the biological and physical characteristics of the patients, the variety of the drugs and of the dosing and application regimens used, and clinical indications. A limitation of most studies is a small sample size. In this context, we considered illustrative examples from studies that were included in this review and provided some evidence for functional recovery associated with the dopaminergic system. Importantly, the dopaminergic nigrostriatal pathway is implicated in spatial learning/memory, reward processing, cognitive function and motor function, whereas the mesocorticolimbic pathway is associated with memory consolidation, motivation and addiction. In this review, we have described experimental and clinical evidence indicating that DA neurotransmission is disrupted following TBI and discussed the benefits associated with administration of dopaminergic system-affecting drugs. Ultimately, our review shows that TBI-induced disability may be partially associated with alterations in nigrostriatal dopaminergic signaling in the striatum that are localized in the basal ganglia and establish reciprocal interconnections with the corticostriatal pathway.
Author Contributions
AB-N, AV-M, PC-M, and AA-L: drafting and refining of the manuscript. DM-R, AG-R, and AO-H: critical reading of the manuscript. All authors: read and approved the manuscript.
Funding
This work was supported by INR-LGII (research project numbers: 91/17 and 06/18) and CONACyT (grants: CB-2016-287614 to AB-N and CB-2016-288512 to AA-L).
Conflict of Interest
The authors declare that the research was conducted in the absence of any commercial or financial relationships that could be construed as a potential conflict of interest.
References
Abekawa, T., Ohmori, T., Ito, K., and Koyama, T. (2000). D1 dopamine receptor activation reduces extracellular glutamate and GABA concentrations in the medial prefrontal cortex. Brain Res. 867, 250–254. doi: 10.1016/s0006-8993(00)02298-8
Al-Adawi, S., Al-Naamani, A., Jaju, S., Al-Farsi, Y. M., Dorvlo, A. S. S., Al-Maashani, A., et al. (2020). Methylphenidate improves executive functions in patients with traumatic brain injuries: a feasibility trial via the idiographic approach. BMC Neurol. 20:103.
Albin, R. L., Young, A. B., and Penney, J. B. (1989). The functional-anatomy of basal ganglia disorders. Trends Neurosci. 12, 366–375.
Andelic, N., Howe, E. I., Hellstrom, T., Sanchez, M. F., Lu, J., Lovstad, M., et al. (2018). Disability and quality of life 20 years after traumatic brain injury. Brain Behav. 2018:8.
Arciniegas, D. B., Held, K., and Wagner, P. (2002). Cognitive impairment following traumatic brain injury. Curr. Treat. Option. Neurol. 4, 43–57.
Arias-Montaño, J. A., Floran, B., Garcia, M., Aceves, J., and Young, J. M. (2001). Histamine H(3) receptor-mediated inhibition of depolarization-induced, dopamine D(1) receptor-dependent release of (3)H-gamma-aminobutryic acid from rat striatal slices. Br. J. Pharm. 133, 165–171. doi: 10.1038/sj.bjp.0704053
Assous, M., Dautan, D., Tepper, J. M., and Mena-Segovia, J. (2019). Pedunculopontine glutamatergic neurons provide a novel source of feedforward inhibition in the striatum by selectively targeting interneurons. J. Neurosci. 39, 4727. doi: 10.1523/jneurosci.2913-18.2019
Aubert, I., Guigoni, C., Hakansson, K., Li, Q., Dovero, S., Barthe, N., et al. (2005). Increased D-1 dopamine receptor signaling in levodopa-induced dyskinesia. Ann. Neurol. 57, 17–26. doi: 10.1002/ana.20296
Auffret, M., Drapier, S., and Vérin, M. (2019). New tricks for an old dog: A repurposing approach of apomorphine. Eur. J. Pharmacol. 843, 66–79. doi: 10.1016/j.ejphar.2018.10.052
Avila-Luna, A., Galvez-Rosas, A., Alfaro-Rodriguez, A., Reyes-Legorreta, C., Garza-Montano, P., Gonzalez-Pina, R., et al. (2018a). Dopamine D1 receptor activation maintains motor coordination in injured rats but does not accelerate the recovery of the motor coordination deficit. Behav. Brain Res. 336, 145–150. doi: 10.1016/j.bbr.2017.08.026
Avila-Luna, A., Galvez-Rosas, A., Durand-Rivera, A., Ramos-Languren, L. E., Rios, C., Arias-Montano, J. A., et al. (2018b). Dopamine D1 receptor activation maintains motor coordination and balance in rats. Metab. Brain Dis. 33, 99–105. doi: 10.1007/s11011-017-0126-x
Avila-Luna, A., Ríos, C., Gálvez-Rosas, A., Montes, S., Arias-Montano, J.-A., and Bueno-Nava, A. (2019). Chronic administration of the histamine H3 receptor agonist immepip decreases l-Dopa-induced dyskinesias in 6-hydroxydopamine-lesioned rats. Psychopharmacology 236, 1937–1948. doi: 10.1007/s00213-019-5182-y
Bales, J. W., Wagner, A. K., Kline, A. E., and Dixon, C. E. (2009). Persistent cognitive dysfunction after traumatic brain injury: A dopamine hypothesis. Neurosci. Biobehav. Rev. 33, 981–1003. doi: 10.1016/j.neubiorev.2009.03.011
Bales, J. W., Yan, H. Q., Ma, X., Li, Y., Samarasinghe, R., and Dixon, C. E. (2011). The dopamine and cAMP regulated phosphoprotein, 32 kDa (DARPP-32) signaling pathway: A novel therapeutic target in traumatic brain injury. Exp. Neurol. 229, 300–307. doi: 10.1016/j.expneurol.2011.02.013
Barnett, M., and Reid, L. (2020). The effectiveness of methylphenidate in improving cognition after brain injury in adults: a systematic review. Brain Injury 34, 1–10. doi: 10.1080/02699052.2019.1667538
Bastide, M. F., Meissner, W. G., Picconi, B., Fasano, S., Fernagut, P. O., Feyder, M., et al. (2015). Pathophysiology of L-dopa-induced motor and non-motor complications in Parkinson’s disease. Prog. Neurobiol. 132, 96–168.
Bergson, C., Mrzljak, L., Smiley, J. F., Pappy, M., Levenson, R., and Goldman-Rakic, P. S. (1995). Regional, cellular, and subcellular variations in the distribution of D1 and D5 dopamine receptors in primate brain. J. Neurosci. 15, 7821–7836. doi: 10.1523/jneurosci.15-12-07821.1995
Bhatnagar, S., Iaccarino, M. A., and Zafonte, R. (2016). Pharmacotherapy in rehabilitation of post-acute traumatic brain injury. Brain Res. 1640, 164–179. doi: 10.1016/j.brainres.2016.01.021
Bjorklund, A., and Dunnett, S. B. (2007). Dopamine neuron systems in the brain: an update. Trends Neurosci. 30, 194–202. doi: 10.1016/j.tins.2007.03.006
Bleimeister, I. H., Wolff, M., Lam, T. R., Brooks, D. M., Patel, R., Cheng, J. P., et al. (2019). Environmental enrichment and amantadine confer individual but nonadditive enhancements in motor and spatial learning after controlled cortical impact injury. Brain Res. 1714, 227–233. doi: 10.1016/j.brainres.2019.03.007
Bolam, J. P., and Ellender, T. J. (2016). Histamine and the striatum. Neuropharmacology 106, 74–84. doi: 10.1016/j.neuropharm.2015.08.013
Bolam, J. P., Hanley, J. J., Booth, P. A., and Bevan, M. D. (2000). Synaptic organisation of the basal ganglia. J. Anat. 196(Pt 4), 527–542. doi: 10.1046/j.1469-7580.2000.19640527.x
Borghol, A., Aucoin, M., Onor, I., Jamero, D., and Hawawini, F. (2018). Modafinil for the Improvement of Patient Outcomes Following Traumatic Brain Injury. Innov. Clin. Neurosci. 15, 17–23.
Bostan, A. C., and Strick, P. L. (2010). The Cerebellum and Basal Ganglia are Interconnected. Neuropsychol. Rev. 20, 261–270. doi: 10.1007/s11065-010-9143-9
Bradley, C. L., and Damiano, D. L. (2019). Effects of Dopamine on Motor Recovery and Training in Adults and Children With Nonprogressive Neurological Injuries: A Systematic Review. Neurorehabilit. Neural. Repair 33, 331–344. doi: 10.1177/1545968319837289
Brannan, T., Martínez-Tica, J., Di Rocco, A., and Yahr, M. D. (1993). Low and high dose bromocriptine have different effects on striatal dopamine release: an in vivo study. J. Neural. Transm. Park Dis. Dement Sect. 6, 81–87. doi: 10.1007/bf02261001
Bueno-Nava, A., Gonzalez-Pina, R., and Alfaro-Rodriguez, A. (2010a). Iron-dextran injection into the substantia nigra in rats decreases striatal dopamine content ipsilateral to the injury site and impairs motor function. Metab. Brain Dis. 25, 235–239. doi: 10.1007/s11011-010-9200-3
Bueno-Nava, A., Gonzalez-Pina, R., Alfaro-Rodriguez, A., Nekrassov-Protasova, V., Durand-Rivera, A., Montes, S., et al. (2010b). Recovery of Motor Deficit, Cerebellar Serotonin and Lipid Peroxidation Levels in the Cortex of Injured Rats. Neurochem. Res. 35, 1538–1545. doi: 10.1007/s11064-010-0213-4
Bueno-Nava, A., Montes, S., Delagarza-Montano, P., Alfaro-Rodriguez, A., Ortiz, A., and Gonzalez-Pina, R. (2008). Reversal of noradrenergic depletion and lipid peroxidation in the pons after brain injury correlates with motor function recovery in rats. Neurosci. Lett. 443, 32–36. doi: 10.1016/j.neulet.2008.07.046
Bunzow, J. R., Van Tol, H. H., Grandy, D. K., Albert, P., Salon, J., Christie, M., et al. (1988). Cloning and expression of a rat D2 dopamine receptor cDNA. Nature 336, 783–787. doi: 10.1038/336783a0
Calabresi, P., Gubellini, P., Centonze, D., Picconi, B., Bernardi, G., Chergui, K., et al. (2000). Dopamine and cAMP-Regulated Phosphoprotein 32 kDa Controls Both Striatal Long-Term Depression and Long-Term Potentiation, Opposing Forms of Synaptic Plasticity. J. Neurosci. 20:8443. doi: 10.1523/jneurosci.20-22-08443.2000
Calabresi, P., Picconi, B., Tozzi, A., Ghiglieri, V., and Di Filippo, M. (2014). Direct and indirect pathways of basal ganglia: a critical reappraisal. Nat. Neurosci. 17, 1022–1030. doi: 10.1038/nn.3743
Camps, M., Kelly, P. H., and Palacios, J. M. (1990). Autoradiographic localization of dopamine D1 and D2 receptors in the brain of several mammalian species. J. Neural Trans. Gen. Sect. 80, 105–127. doi: 10.1007/bf01257077
Carabenciov, I. D., Bureau, B. L., Cutrer, M., and Savica, R. (2019). Amantadine Use for Postconcussion Syndrome. Mayo Clin. Proc. 94, 275–277. doi: 10.1016/j.mayocp.2018.10.021
Carrillo-Mora, P., Alcantar-Shramm, J. M., Almaguer-Benavides, K. M., Macías-Gallardo, J. J., Fuentes-Bello, A., and Rodríguez-Barragán, M. A. (2017). Pharmacological Stimulation of Neuronal Plasticity in Acquired Brain Injury. Clin. Neuropharmacol. 40, 131–139. doi: 10.1097/wnf.0000000000000217
Celik, J. B., Duman, A., Arun, O., Onal, I. O., Ilban, O., and Sonmez, A. E. (2015). Dopaminergic Challenge With Bromocriptine in Patients With Severe Brain Injury. Intens. Care Med. Exp. 3:A485.
Chamoun, R., Suki, D., Gopinath, S. P., Goodman, J. C., and Robertson, C. (2010). Role of extracellular glutamate measured by cerebral microdialysis in severe traumatic brain injury. J. Neurosurg. 113, 564–570. doi: 10.3171/2009.12.jns09689
Chandran, R., Kim, T., Mehta, S. L., Udho, E., Chanana, V., Cengiz, P., et al. (2018). A combination antioxidant therapy to inhibit NOX2 and activate Nrf2 decreases secondary brain damage and improves functional recovery after traumatic brain injury. J. Cereb. Blood Flow Metab. 38, 1818–1827. doi: 10.1177/0271678x17738701
Chen, Y.-H., Huang, E. Y.-K., Kuo, T.-T., Miller, J., Chiang, Y.-H., and Hoffer, B. J. (2017a). Impact of Traumatic Brain Injury on Dopaminergic Transmission. Cell Transplant. 26, 1156–1168. doi: 10.1177/0963689717714105
Chen, Y. H., Huang, E. Y., Kuo, T. T., Ma, H. I., Hoffer, B. J., Tsui, P. F., et al. (2015a). Dopamine Release Impairment in Striatum After Different Levels of Cerebral Cortical Fluid Percussion Injury. Cell Transplant. 24, 2113–2128. doi: 10.3727/096368914x683584
Chen, Y. H., Huang, E. Y. K., Kuo, T. T., Hoffer, B. J., Miller, J., Chou, Y. C., et al. (2017b). Dopamine release in the nucleus accumbens is altered following traumatic brain injury. Neuroscience 348, 180–190. doi: 10.1016/j.neuroscience.2017.02.001
Chen, Y. H., Huang, E. Y. K., Kuo, T. T., Ma, H. I., Hoffer, B. J., Tsui, P. F., et al. (2015b). Dopamine Release Impairment in Striatum After Different Levels of Cerebral Cortical Fluid Percussion Injury. Cell Transplant. 24, 2113–2128. doi: 10.3727/096368914x683584
Cheng, H.-W., Rafols, J. A., Goshgarian, H. G., Anavi, Y., Tong, J., and Mcneill, T. H. (1997). Differential Spine Loss and Regrowth of Striatal Neurons Following Multiple Forms of Deafferentation: A Golgi Study. Exp. Neurol. 147, 287–298. doi: 10.1006/exnr.1997.6618
Chien, Y.-J., Chien, Y.-C., Liu, C.-T., Wu, H.-C., Chang, C.-Y., and Wu, M.-Y. (2019). Effects of Methylphenidate on Cognitive Function in Adults with Traumatic Brain Injury: A Meta-Analysis. Brain Sci. 9:291. doi: 10.3390/brainsci9110291
Choi, E. B., Kim, J. Y., and Jang, S. H. (2020). Motor recovery of hemiparetic leg by improvement of limb-kinetic apraxia in a chronic patient with traumatic brain injury: A case report. Medicine 2020:99.
Corrigan, J. D., Selassie, A. W., and Orman, J. A. (2010). The Epidemiology of Traumatic Brain Injury. J. Head Trauma Rehabilit. 25, 72–80.
Corsi, F. M., Gerace, C., and Ricci, M. (2020). Rotigotine effect in prolonged disturbance of consciousness. Brief Report of two cases. Acta Biomed. 91, 132–133.
Costa, R. M., Lin, S.-C., Sotnikova, T. D., Cyr, M., Gainetdinov, R. R., Caron, M. G., et al. (2006). Rapid alterations in corticostriatal ensemble coordination during acute dopamine-dependent motor dysfunction. Neuron 52, 359–369. doi: 10.1016/j.neuron.2006.07.030
Daskalakis, Z. J., Paradiso, G. O., Christensen, B. K., Fitzgerald, P. B., Gunraj, C., and Chen, R. (2004). Exploring the connectivity between the cerebellum and motor cortex in humans. J. Physiol. 557, 689–700. doi: 10.1113/jphysiol.2003.059808
Davis, E. J. H., Coyne, C., and Mcneill, T. H. (2007). Intrastriatal dopamine D1 antagonism dampens neural plasticity in response to motor cortex lesion. Neuroscience 146, 784–791. doi: 10.1016/j.neuroscience.2007.01.039
De La Fuente-Fernandez, R., Sossi, V., Huang, Z. G., Furtado, S., Lu, J. Q., Calne, D. B., et al. (2004). Levodopa-induced changes in synaptic dopamine levels increase with progression of Parkinson’s disease: implications for dyskinesias. Brain 127, 2747–2754. doi: 10.1093/brain/awh290
Demarchi, R., Bansal, V., Hung, A., Wroblewski, K., Dua, H., Sockalingam, S., et al. (2005). Review of Awakening Agents. Can. J. Neurol. Sci. 32, 4–17.
Dhamapurkar, S. K., Wilson, B. A., Rose, A., Watson, P., and Shiel, A. (2017). Does Modafinil improve the level of consciousness for people with a prolonged disorder of consciousness? a retrospective pilot study. Disabil. Rehabil. 39, 2633–2639. doi: 10.1080/09638288.2016.1236414
Doig, N. M., Moss, J., and Bolam, J. P. (2010). Cortical and Thalamic Innervation of Direct and Indirect Pathway Medium-Sized Spiny Neurons in Mouse Striatum. J. Neurosci. 30:14610. doi: 10.1523/jneurosci.1623-10.2010
Donnemiller, E., Brenneis, C., Wissel, J., Scherfler, C., Poewe, W., Riccabona, G., et al. (2000). Impaired dopaminergic neurotransmission in patients with traumatic brain injury: a SPET study using 123I-β-CIT and 123I-IBZM. Eur. J. Nuclear Med. 27, 1410–1414. doi: 10.1007/s002590000308
Dorer, C. L., Manktelow, A. E., Allanson, J., Sahakian, B. J., Pickard, J. D., Bateman, A., et al. (2018). Methylphenidate-mediated motor control network enhancement in patients with traumatic brain injury. Brain Injury 32, 1040–1049. doi: 10.1080/02699052.2018.1469166
Dougherty, S. E., Kajstura, T. J., Jin, Y., Chan-Cortés, M. H., Kota, A., and Linden, D. J. (2020). Catecholaminergic axons in the neocortex of adult mice regrow following brain injury. Exp. Neurol. 323:113089. doi: 10.1016/j.expneurol.2019.113089
Dunn-Meynell, A., Shijun, P., and Levin, B. E. (1994). Focal traumatic brain injury causes widespread reductions in rat brain norepinephrine turnover from 6 to 24 h. Brain Res. 660, 88–95. doi: 10.1016/0006-8993(94)90842-7
Dunn-Meynell, A. A., and Levin, B. E. (1997). Histological markers of neuronal, axonal and astrocytic changes after lateral rigid impact traumatic brain injury. Brain Res. 761, 25–41. doi: 10.1016/s0006-8993(97)00210-2
Duong, J., Elia, C., Takayanagi, A., Lanzilotta, T., Ananda, A., and Miulli, D. (2018). The impact of methamphetamines in patients with traumatic brain injury, a retrospective review. Clin. Neurol. Neurosurg. 170, 99–101. doi: 10.1016/j.clineuro.2018.04.030
Eakin, K., Hoffer, B., and Miller, J. (2013). Substantia nigra vulnerability after a single moderate diffuse brain injury in the rat. Exp. Neurol. 240, 103–107. doi: 10.1016/j.expneurol.2012.11.005
Ekinci, O., Direk, M. Ç, Gunes, S., Teke, H., Ekinci, N., and Yıldırım, F. (2017). Short-term efficacy and tolerability of methylphenidate in children with traumatic brain injury and attention problems. Brain Dev. 39, 327–336. doi: 10.1016/j.braindev.2016.11.005
Ennis, J. D., Harvey, D., Ho, E., Chari, V., Graham, A., and Nesathurai, S. (2013). Levodopa/carbidopa to improve motor function subsequent to brain tumor excision. Am. J. Phys. Med. Rehabil. 92, 307–311. doi: 10.1097/phm.0b013e318278dc20
Espadas, M., Insa, I., Chamorro, M., and Alda-Diez, J. A. (2018). Efectos secundarios del metilfenidato en poblacion infantil y juvenil [Side effects of methylphenidate in children and the young]. Rev. Neurol. 66, 157–162. doi: 10.33588/rn.6605.2017480
Feeney, D. M., Gonzalez, A., and Law, W. A. (1982). Amphetamine, haloperidol, and experience interact to affect rate of recovery after motor cortex injury. Science 217, 855–857. doi: 10.1126/science.7100929
Ferrada, C., Ferre, S., Casado, V., Cortes, A., Justinova, Z., Barnes, C., et al. (2008). Interactions between histamine H-3 and dopamine D-2 receptors and the implications for striatal function. Neuropharmacology 55, 190–197. doi: 10.1016/j.neuropharm.2008.05.008
Ferrada, C., Moreno, E., Casado, V., Bongers, G., Cortes, A., Mallol, J., et al. (2009). Marked changes in signal transduction upon heteromerization of dopamine D-1 and histamine H-3 receptors. Br. J. Pharm. 157, 64–75. doi: 10.1111/j.1476-5381.2009.00152.x
Ferré, S., Bonaventura, J., Tomasi, D., Navarro, G., Moreno, E., Cortés, A., et al. (2016). Allosteric mechanisms within the adenosine A2A–dopamine D2 receptor heterotetramer. Neuropharmacology 104, 154–160. doi: 10.1016/j.neuropharm.2015.05.028
Ferré, S., and Ciruela, F. (2019). Functional and Neuroprotective Role of Striatal Adenosine A(2A) Receptor Heterotetramers. J. Caffein. Adenos. Res. 9, 89–97. doi: 10.1089/caff.2019.0008
Flashman, L. A., Mcdonald, B. C., Ford, J. C., Kenny, R. M., Andrews, K. D., Saykin, A. J., et al. (2021). Differential Effects of Pergolide and Bromocriptine on Working Memory Performance and Brain Activation after Mild Traumatic Brain Injury. J. Neurotrauma. 38, 225–234.
Folweiler, K. A., Bondi, C. O., Ogunsanya, E. A., Laporte, M. J., Leary, J. B., Radabaugh, H. L., et al. (2017). Combining the Antipsychotic Drug Haloperidol and Environmental Enrichment after Traumatic Brain Injury Is a Double-Edged Sword. J. Neurotrauma. 34, 451–458. doi: 10.1089/neu.2016.4417
Frenette, A. J., Kanji, S., Rees, L., Williamson, D. R., Perreault, M. M., Turgeon, A. F., et al. (2011). Efficacy and Safety of Dopamine Agonists in Traumatic Brain Injury: A Systematic Review of Randomized Controlled Trials. J. Neurotrauma. 29, 1–18. doi: 10.1089/neu.2011.1812
Frenette, A. J., Kanji, S., Rees, L., Williamson, D. R., Perreault, M. M., Turgeon, A. F., et al. (2012). Efficacy and Safety of Dopamine Agonists in Traumatic Brain Injury: A Systematic Review of Randomized Controlled Trials. J. Neurotrauma. 29, 1–18. doi: 10.1089/neu.2011.1812
Freund, T. F., Powell, J. F., and Smith, A. D. (1984). Tyrosine hydroxylase-immunoreactive boutons in synaptic contact with identified striatonigral neurons, with particular reference to dendritic spines. Neuroscience 13, 1189–1215. doi: 10.1016/0306-4522(84)90294-x
Fridman, E. A., Krimchansky, B. Z., Bonetto, M., Galperin, T., Gamzu, E. R., Leiguarda, R. C., et al. (2010). Continuous subcutaneous apomorphine for severe disorders of consciousness after traumatic brain injury. Brain Inj. 24, 636–641. doi: 10.3109/02699051003610433
Fridman, E. A., Osborne, J. R., Mozley, P. D., Victor, J. D., and Schiff, N. D. (2019). Presynaptic dopamine deficit in minimally conscious state patients following traumatic brain injury. Brain 142, 1887–1893. doi: 10.1093/brain/awz118
Fundament, T., Eldridge, P. R., Green, A. L., Whone, A. L., Taylor, R. S., Williams, A. C., et al. (2016). Deep Brain Stimulation for Parkinson’s Disease with Early Motor Complications: A UK Cost-Effectiveness Analysis. PLoS One 2016:11.
Fuxe, K., Marcellino, D., Borroto-Escuela, D. O., Guescini, M., Fernández-Dueñas, V., Tanganelli, S., et al. (2010). Adenosine–Dopamine Interactions in the Pathophysiology and Treatment of CNS Disorders. CNS Neurosci. Therapeut. 16, e18–e42.
Fuxe, K., Marcellino, D., Rivera, A., Diaz-Cabiale, Z., Filip, M., Gago, B., et al. (2008). Receptor–receptor interactions within receptor mosaics. Impact on neuropsychopharmacology. Brain Res. Rev. 58, 415–452. doi: 10.1016/j.brainresrev.2007.11.007
Gálvez-Rosas, A., Avila-Luna, A., Valdés-Flores, M., Montes, S., and Bueno-Nava, A. (2019). GABAergic imbalance is normalized by dopamine D1 receptor activation in the striatum contralateral to the cortical injury in motor deficit-recovered rats. Psychopharmacology 236:5215.
Gao, Y., Ma, L., Liang, F., Zhang, Y., Yang, L., Liu, X., et al. (2020). The use of amantadine in patients with unresponsive wakefulness syndrome after severe cerebral hemorrhage. Brain Inj. 34, 1084–1088. doi: 10.1080/02699052.2020.1780315
Garcia, M., Floran, B., Arias-Montano, J. A., Young, J. M., and Aceves, J. (1997). Histamine H3 receptor activation selectively inhibits dopamine D1 receptor-dependent [3H]GABA release from depolarization-stimulated slices of rat substantia nigra pars reticulata. Neuroscience 80, 241–249. doi: 10.1016/s0306-4522(97)00100-0
Gaspar, P., Bloch, B., and Le Moine, C. (1995). D1 and D2 Receptor Gene Expression in the Rat Frontal Cortex: Cellular Localization in Different Classes of Efferent Neurons. Eur. J. Neurosci. 7, 1050–1063. doi: 10.1111/j.1460-9568.1995.tb01092.x
Gee, S., Ellwood, I., Patel, T., Luongo, F., Deisseroth, K., and Sohal, V. S. (2012). Synaptic activity unmasks dopamine D2 receptor modulation of a specific class of layer V pyramidal neurons in prefrontal cortex. J. Neurosci. 32, 4959–4971. doi: 10.1523/jneurosci.5835-11.2012
Gerfen, C. R., and Surmeier, D. J. (2011). Modulation of Striatal Projection Systems by Dopamine. Ann. Rev. Neurosci. 34, 441–466. doi: 10.1146/annurev-neuro-061010-113641
Ghalaenovi, H., Fattahi, A., Koohpayehzadeh, J., Khodadost, M., Fatahi, N., Taheri, M., et al. (2018). The effects of amantadine on traumatic brain injury outcome: a double-blind, randomized, controlled, clinical trial. Brain Inj. 32, 1050–1055. doi: 10.1080/02699052.2018.1476733
Ghate, P. S., Bhanage, A., Sarkar, H., and Katkar, A. (2018). Efficacy of Amantadine in Improving Cognitive Dysfunction in Adults with Severe Traumatic Brain Injury in Indian Population: A Pilot Study. Asian J. Neurosurg. 13, 647–650.
Giacino, J. T., Whyte, J., Bagiella, E., Kalmar, K., Childs, N., Khademi, A., et al. (2012). Placebo-Controlled Trial of Amantadine for Severe Traumatic Brain Injury. N. Engl. J. Med. 366, 819–826.
Gramish, J. A., Kopp, B. J., and Patanwala, A. E. (2017). Effect of Amantadine on Agitation in Critically Ill Patients With Traumatic Brain Injury. Clin. Neuropharm. 2017:40.
Greengard, P., Allen, P. B., and Nairn, A. C. (1999). Beyond the Dopamine Receptor: the DARPP-32/Protein Phosphatase-1 Cascade. Neuron 23, 435–447.
Guerriero, R. M., Giza, C. C., and Rotenberg, A. (2015). Glutamate and GABA Imbalance Following Traumatic Brain Injury. Curr. Neurol. Neurosci. Rep. 2015:15.
Guigoni, C., Aubert, I., Li, Q., Gurevich, V. V., Benovic, J. L., Ferry, S., et al. (2005). Pathogenesis of levodopa-induced dyskinesia: focus on D1 and D3 dopamine receptors. Parkinson. Relat. Dis. 11, S25–S29.
Haber, S. N. (2016). Corticostriatal circuitry. Dial. Clin. Neurosci. 18, 7–21. doi: 10.31887/dcns.2016.18.1/shaber
Hadgu, R. M., Borghol, A., Gillard, C., Wilson, C., Elqess Mossa, S., Mckay, M., et al. (2020). Evaluation of Outcomes in Patients Receiving Amantadine to Improve Alertness After Traumatic Brain Injury. Hospital Pharm. 0:0018578720920803.
Hammond, F. M., Malec, J. F., Zafonte, R. D., Sherer, M., Bogner, J., Dikmen, S., et al. (2017). Potential Impact of Amantadine on Aggression in Chronic Traumatic Brain Injury. J. Head Traum. Rehabilit. 32, 308–318. doi: 10.1097/htr.0000000000000342
Harmon, R. L., and Boyeson, M. G. (1997). Clinical Neuropharmacology of Behavioral Recovery Following Brain Injury. Phys. Med. Rehabilit. Clin. North Am. 8, 651–665. doi: 10.1016/s1047-9651(18)30295-x
Hersch, S. M., Yi, H., Heilman, C. J., Edwards, R. H., and Levey, A. I. (1997). Subcellular localization and molecular topology of the dopamine transporter in the striatum and substantia nigra. J. Comp. Neurol. 388, 211–227. doi: 10.1002/(sici)1096-9861(19971117)388:2<211::aid-cne3>3.0.co;2-4
Horn, S. D., Corrigan, J. D., and Dijkers, M. P. (2015). Traumatic Brain Injury Rehabilitation Comparative Effectiveness Research: Introduction to the Traumatic Brain-Practice Based Evidence Archives Supplement. Archiv. Phys. Med. Rehabilit. 96, S173–S177.
Hovda, D. A., Sutton, R. L., and Feeney, D. M. (1987). Recovery of tactile placing after visual cortex ablation in cat: A behavioral and metabolic study of diaschisis. Exp. Neurol. 97, 391–402. doi: 10.1016/0014-4886(87)90099-9
Hsu, K. S., Huang, C. C., Yang, C. H., and Gean, P. W. (1995). Presynaptic D-2 dopaminergic receptors mediate inhibition of excitatory synaptic transmission in rat neostriatum. Brain Res. 690, 264–268. doi: 10.1016/0006-8993(95)00734-8
Huang, E. Y.-K., Tsai, T.-H., Kuo, T.-T., Tsai, J. Jr., Tsui, P.-F., Chou, Y.-C., et al. (2014a). Remote effects on the striatal dopamine system after fluid percussion injury. Behav. Brain Res. 267, 156–172. doi: 10.1016/j.bbr.2014.03.033
Huang, E. Y.-K., Tsui, P.-F., Kuo, T.-T., Tsai, J. Jr., Chou, Y.-C., Ma, H.-I., et al. (2014b). Amantadine Ameliorates Dopamine-Releasing Deficits and Behavioral Deficits in Rats after Fluid Percussion Injury. PLoS One 9:e86354. doi: 10.1371/journal.pone.0086354
Huang, Y.-N., Yang, L.-Y., Greig, N. H., Wang, Y.-C., Lai, C.-C., and Wang, J.-Y. (2018). Neuroprotective effects of pifithrin-α against traumatic brain injury in the striatum through suppression of neuroinflammation, oxidative stress, autophagy, and apoptosis. Sci. Rep. 8, 2368–2368.
Huger, F., and Patrick, G. (1979). EFFECT OF CONCUSSIVE HEAD INJURY ON CENTRAL CATECHOLAMINE LEVELS AND SYNTHESIS RATES IN RAT BRAIN REGIONS 1. J. Neurochem. 33, 89–95. doi: 10.1111/j.1471-4159.1979.tb11710.x
Humbert-Claude, M., Morisset, S., Gbahou, F., and Arrang, J.-M. (2007). Histamine H3 and dopamine D2 receptor-mediated [35S]GTPγ[S] binding in rat striatum: Evidence for additive effects but lack of interactions. Biochem. Pharm. 73, 1172–1181. doi: 10.1016/j.bcp.2007.01.006
Hutson, C. B., Lazo, C. R., Mortazavi, F., Giza, C. C., Hovda, D., and Chesselet, M.-F. (2011). Traumatic brain injury in adult rats causes progressive nigrostriatal dopaminergic cell loss and enhanced vulnerability to the pesticide paraquat. J. Neurot. 28, 1783–1801. doi: 10.1089/neu.2010.1723
Iaccarino, M. A., Philpotts, L. L., Zafonte, R., and Biederman, J. (2020). Stimulant Use in the Management of Mild Traumatic Brain Injury: A Qualitative Literature Review. J. Attent. Dis. 24, 309–317. doi: 10.1177/1087054718759752
Jackson, D. M., and Westlind-Danielsson, A. (1994). Dopamine receptors: molecular biology, biochemistry and behavioural aspects. Pharm. Therap. 64, 291–370. doi: 10.1016/0163-7258(94)90041-8
Jang, S. H., Kim, S. H., and Seo, J. P. (2018). Recovery of an injured corticofugal tract from the supplementary motor area in a patient with traumatic brain injury: A case report. Medicine 97, e9063–e9063.
Jenkins, P. O., De Simoni, S., Bourke, N. J., Fleminger, J., Scott, G., Towey, D. J., et al. (2018). Dopaminergic abnormalities following traumatic brain injury. Brain 141, 797–810.
Jenkins, P. O., Mehta, M. A., and Sharp, D. J. (2016). Catecholamines and cognition after traumatic brain injury. Brain 139, 2345–2371. doi: 10.1093/brain/aww128
Johansson, B., Wentzel, A.-P., Andréll, P., Rönnbäck, L., and Mannheimer, C. (2017). Long-term treatment with methylphenidate for fatigue after traumatic brain injury. Acta Neurol. Scand. 135, 100–107. doi: 10.1111/ane.12587
Johansson, B., Wentzel, A. P., Andréll, P., Mannheimer, C., and Rönnbäck, L. (2015). Methylphenidate reduces mental fatigue and improves processing speed in persons suffered a traumatic brain injury. Brain Inj. 29, 758–765. doi: 10.3109/02699052.2015.1004747
Jolly, A. E., Raymont, V., Cole, J. H., Whittington, A., Scott, G., De Simoni, S., et al. (2019). Dopamine D2/D3 receptor abnormalities after traumatic brain injury and their relationship to post-traumatic depression. NeuroImage Clin. 24:101950. doi: 10.1016/j.nicl.2019.101950
Jones, E. A., Wang, J. Q., and Mcginty, J. F. (2001). Intrastriatal GABAA receptor blockade does not alter dopamine D1/D2 receptor interactions in the intact rat striatum. Neuroscience 102, 381–389. doi: 10.1016/s0306-4522(00)00451-6
Kaelin, D. L., Cifu, D. X., and Matthies, B. (1996). Methylphenidate effect on attention deficit in the acutely brain-injured adult. Archiv. Phys. Med. Rehabilit. 77, 6–9. doi: 10.1016/s0003-9993(96)90211-7
Kaiser, P. R., Valko, P. O., Werth, E., Thomann, J., Meier, J., Stocker, R., et al. (2010). Modafinil ameliorates excessive daytime sleepiness after traumatic brain injury. Neurology 75, 1780–1785. doi: 10.1212/wnl.0b013e3181fd62a2
Kandel, E. R., Schwartz, J. H., Jessell, T. M., Siegelbaum, S. A., and Hudspeth, A. J. (2013). Principles of Neural Science. Chennai: McGraw Hill Education.
Karelina, K., Gaier, K. R., and Weil, Z. M. (2017). Traumatic brain injuries during development disrupt dopaminergic signaling. Exp. Neurol. 297, 110–117. doi: 10.1016/j.expneurol.2017.08.003
Keeler, J. F., Pretsell, D. O., and Robbins, T. W. (2014). Functional implications of dopamine D1 vs. D2 receptors: a ‘prepare and select’ model of the striatal direct vs. Indirect pathways. Neuroscience 282, 156–175. doi: 10.1016/j.neuroscience.2014.07.021
Kemp, J. M., Powell, T. P. S., and Harris, G. W. (1971). The termination of fibres from the cerebral cortex and thalamus upon dendritic spines in the caudate nucleus: a study with the Golgi method. Philosop. Transac. R. Soc. Lond. B Biol. Sci. 262, 429–439. doi: 10.1098/rstb.1971.0105
Kerkerian, L., Dusticier, N., and Nieoullon, A. (1987). Modulatory effect of dopamine on high-affinity glutamate uptake in the rat striatum. J. Neurochem. 48, 1301–1306. doi: 10.1111/j.1471-4159.1987.tb05661.x
Kernie, S. G., and Parent, J. M. (2010). Forebrain neurogenesis after focal Ischemic and traumatic brain injury. Neurob. Dis. 37, 267–274. doi: 10.1016/j.nbd.2009.11.002
Kline, A. E., Chen, M. J., Tso-Olivas, D. Y., and Feeney, D. M. (1994). Methylphenidate treatment following ablation-induced hemiplegia in rat: Experience during drug action alters effects on recovery of function. Pharm. Biochem. Behav. 48, 773–779. doi: 10.1016/0091-3057(94)90345-x
Kline, A. E., Massucci, J. L., Ma, X., Zafonte, R. D., and Edward Dixon, C. (2004). Bromocriptine Reduces Lipid Peroxidation and Enhances Spatial Learning and Hippocampal Neuron Survival in a Rodent Model of Focal Brain Trauma. J. Neurot. 21, 1712–1722. doi: 10.1089/neu.2004.21.1712
Kline, A. E., Yan, H. Q., Bao, J., Marion, D. W., and Dixon, C. E. (2000). Chronic methylphenidate treatment enhances water maze performance following traumatic brain injury in rats. Neurosci. Lett. 280, 163–166. doi: 10.1016/s0304-3940(00)00797-7
Ko, I.-G., Kim, C.-J., and Kim, H. (2019). Treadmill exercise improves memory by up-regulating dopamine and down-regulating D(2) dopamine receptor in traumatic brain injury rats. J. Exer. Rehabilit. 15, 504–511. doi: 10.12965/jer.1938316.158
Koshimizu, Y., Fujiyama, F., Nakamura, K. C., Furuta, T., and Kaneko, T. (2013). Quantitative analysis of axon bouton distribution of subthalamic nucleus neurons in the rat by single neuron visualization with a viral vector. J. Comp. Neurol. 521, 2125–2146. doi: 10.1002/cne.23277
Kreitzer, A. C., and Malenka, R. C. (2008). Striatal Plasticity and Basal Ganglia Circuit Function. Neuron 60, 543–554. doi: 10.1016/j.neuron.2008.11.005
Krimchansky, B.-Z., Keren, O., Sazbon, L., and Groswasser, Z. (2004). Differential time and related appearance of signs, indicating improvement in the state of consciousness in vegetative state traumatic brain injury (VS-TBI) patients after initiation of dopamine treatment. Brain Inj. 18, 1099–1105. doi: 10.1080/02699050310001646206
Krobert, K. A., Sutton, R. L., and Feeney, D. M. (1994). Spontaneous and amphetamine-evoked release of cerebellar noradrenaline after sensorimotor cortex contusion: an in vivo microdialysis study in the awake rat. J. Neurochem. 62, 2233–2240. doi: 10.1046/j.1471-4159.1994.62062233.x
Kupferschmidt, D. A., Juczewski, K., Cui, G., Johnson, K. A., and Lovinger, D. M. (2017). Parallel, but Dissociable, Processing in Discrete Corticostriatal Inputs Encodes Skill Learning. Neuron 96, 476.e–489.e.
L’hirondel, M., Chéramy, A., Godeheu, G., Artaud, F., Saiardi, A., Borrelli, E., et al. (1998). Lack of autoreceptor-mediated inhibitory control of dopamine release in striatal synaptosomes of D2 receptor-deficient mice. Brain Res. 792, 253–262. doi: 10.1016/s0006-8993(98)00146-2
Lal, S., Merbtiz, C. P., and Grip, J. C. (1988). Modification of function in head-injured patients with Sinemet. Brain Inj. 2, 225–233. doi: 10.3109/02699058809150946
Lan, Y. L., Li, S., Lou, J. C., Ma, X. C., and Zhang, B. (2019). The potential roles of dopamine in traumatic brain injury: a preclinical and clinical update. Am. J. Transl. Res. 11, 2616–2631.
Langley, K. C., Bergson, C., Greengard, P., and Ouimet, C. C. (1997). Co-localization of the D1 dopamine receptor in a subset of DARPP-32-containing neurons in rat caudate–putamen. Neuroscience 78, 977–983. doi: 10.1016/s0306-4522(96)00583-0
Le Moine, C., and Bloch, B. (1995). D1 and D2 dopamine receptor gene expression in the rat striatum: sensitive cRNA probes demonstrate prominent segregation of D1 and D2 mRNAs in distinct neuronal populations of the dorsal and ventral striatum. J. Comparat. Neurol. 355, 418–426. doi: 10.1002/cne.903550308
Leergaard, T. B. (2003). Clustered and laminar topographic patterns in rat cerebro-pontine pathways. Anat. Embryol. 206, 149–162. doi: 10.1007/s00429-002-0272-7
Lei, W. L., Jiao, Y., Del Mar, N., and Reiner, A. (2004). Evidence for differential cortical input to direct pathway versus indirect pathway striatal projection neurons in rats. J. Neurosci. 24, 8289–8299. doi: 10.1523/jneurosci.1990-04.2004
Levin, B. E., Brown, K. L., Pawar, G., and Dunn-Meynell, A. (1995). Widespread and lateralized effects of acute traumatic brain injury on norepinephrine turnover in the rat brain. Brain Res. 674, 307–313. doi: 10.1016/0006-8993(95)00032-l
Leyrer-Jackson, J. M., and Thomas, M. P. (2017). Subtype-specific effects of dopaminergic D2 receptor activation on synaptic trains in layer V pyramidal neurons in the mouse prefrontal cortex. Physiolog. Rep. 5:e13499. doi: 10.14814/phy2.13499
Liao, R., Chen, Y., Cheng, L., Fan, L., Chen, H., Wan, Y., et al. (2019). Histamine H1 Receptors in Neural Stem Cells Are Required for the Promotion of Neurogenesis Conferred by H3 Receptor Antagonism following Traumatic Brain Injury. Stem Cell Rep. 12, 532–544. doi: 10.1016/j.stemcr.2019.01.004
Lidow, M. S., Goldmanrakic, P. S., Gallager, D. W., and Rakic, P. (1991). Distribution of dopaminergic receptors in the primate cerebral-cortex - quantitative autoradiographic analysis using 3H raclopride, 3H spiperone and 3H SCH23390. Neuroscience 40, 657–671. doi: 10.1016/0306-4522(91)90003-7
Lieberman, A. N., and Goldstein, M. (1985). Bromocriptine in Parkinson disease. Pharm. Rev. 37, 217.
Loane, D. J., and Kumar, A. (2016). Microglia in the TBI brain: The good, the bad, and the dysregulated. Exp. Neurol. 275, 316–327. doi: 10.1016/j.expneurol.2015.08.018
Lodge, D. J., and Grace, A. A. (2011). Hippocampal dysregulation of dopamine system function and the pathophysiology of schizophrenia. Trends Pharm. Sci. 32, 507–513. doi: 10.1016/j.tips.2011.05.001
Loggini, A., Tangonan, R., El Ammar, F., Mansour, A., Goldenberg, F. D., Kramer, C. L., et al. (2020). The role of amantadine in cognitive recovery early after traumatic brain injury: A systematic review. Clin. Neurol. Neurosurg. 194:105815. doi: 10.1016/j.clineuro.2020.105815
López De Maturana, R., and Sánchez-Pernaute, R. (2010). Regulation of Corticostriatal Synaptic Plasticity by G Protein-Coupled Receptors. CNS Neurol. Dis. Drug Targ. 9, 601–615. doi: 10.2174/187152710793361531
Lu, L., Li, F., Ma, Y., Chen, H., Wang, P., Peng, M., et al. (2019). Functional connectivity disruption of the substantia nigra associated with cognitive impairment in acute mild traumatic brain injury. Eur. J. Radiol. 114, 69–75. doi: 10.1016/j.ejrad.2019.03.002
Ma, H. M., and Zafonte, R. D. (2020). Amantadine and memantine: a comprehensive review for acquired brain injury. Brain Inj. 34, 299–315. doi: 10.1080/02699052.2020.1723697
Manktelow, A. E., Menon, D. K., Sahakian, B. J., and Stamatakis, E. A. (2017). Working Memory after Traumatic Brain Injury: The Neural Basis of Improved Performance with Methylphenidate. Front. Behav. Neurosci. 2017:11.
Marcellino, D., Ferré, S., Casadó, V., Cortés, A., Le Foll, B., Mazzola, C., et al. (2008). Identification of Dopamine D1–D3 Receptor Heteromers: indications for a role of synergistic d1–d3 receptor interactions in the striatum∗. J. Biol. Chem. 283, 26016–26025.
Massucci, J. L., Kline, A. E., Ma, X., Zafonte, R. D., and Dixon, C. E. (2004). Time dependent alterations in dopamine tissue levels and metabolism after experimental traumatic brain injury in rats. Neurosci. Lett. 372, 127–131. doi: 10.1016/j.neulet.2004.09.026
Mathai, A., and Smith, Y. (2011). The Corticostriatal and Corticosubthalamic Pathways: Two Entries, One Target. So What? Front. Syst. Neurosci. 5, 1–10.
Matsuda, W., Komatsu, Y., Yanaka, K., and Matsumura, A. (2005). Levodopa treatment for patients in persistent vegetative or minimally conscious states. Neuropsychol. Rehabilit. 15, 414–427. doi: 10.1080/09602010443000588
Mcallister, T. W., Mcdonald, B. C., Ferrell, R. B., Tosteson, T. D., Yanofsky, N. N., Grove, M. R., et al. (2011). Dopaminergic challenge with bromocriptine one month after mild traumatic brain injury: altered working memory and BOLD response. J. Neuropsychiat. Clin. Neurosci. 23, 277–286. doi: 10.1176/jnp.23.3.jnp277
Mendoza, G., and Merchant, H. (2014). Motor system evolution and the emergence of high cognitive functions. Prog. Neurobiol. 122, 73–93. doi: 10.1016/j.pneurobio.2014.09.001
Menon, D. K., Schwab, K., Wright, D. W., Maas, A. I., and Int Interagency Initiative, C. (2010). Position Statement: Definition of Traumatic Brain Injury. Archiv. Phys. Med. Rehabilit. 91, 1637–1640. doi: 10.1016/j.apmr.2010.05.017
Meschler, J. P., and Howlett, A. C. (2001). Signal transduction interactions between CB1 cannabinoid and dopamine receptors in the rat and monkey striatum. Neuropharmacology 40, 918–926. doi: 10.1016/s0028-3908(01)00012-0
Meythaler, J. M., Brunner, R. C., Johnson, A., and Novack, T. A. (2002). Amantadine to Improve Neurorecovery in Traumatic Brain Injury–Associated Diffuse Axonal Injury: A Pilot Double-blind Randomized Trial. J. Head Trauma Rehabilit. 17, 300–313. doi: 10.1097/00001199-200208000-00004
Missale, C., Nash, S. R., Robinson, S. W., Jaber, M., and Caron, M. G. (1998). Dopamine receptors: from structure to function. Physiolog. Rev. 78, 189–225.
Moreno, E., Hoffmann, H., Gonzalez-Sepulveda, M., Navarro, G., Casado, V., Cortes, A., et al. (2011). Dopamine D-1-histamine H-3 Receptor Heteromers Provide a Selective Link to MAPK Signaling in GABAergic Neurons of the Direct Striatal Pathway. J. Biolog. Chem. 286, 5846–5854. doi: 10.1074/jbc.m110.161489
Muly, E. C., Szigeti, K., and Goldman-Rakic, P. S. (1998). D1 receptor in interneurons of macaque prefrontal cortex: Distribution and subcellular localization. J. Neurosci. 18, 10553–10565. doi: 10.1523/jneurosci.18-24-10553.1998
Munakomi, S., Bhattarai, B., and Mohan Kumar, B. (2017). Role of bromocriptine in multi-spectral manifestations of traumatic brain injury. Chin. J. Traumatol. 20, 84–86. doi: 10.1016/j.cjtee.2016.04.009
Neely, M. D., Schmidt, D. E., and Deutch, A. Y. (2007). Cortical regulation of dopamine depletion-induced dendritic spine loss in striatal medium spiny neurons. Neuroscience 149, 457–464. doi: 10.1016/j.neuroscience.2007.06.044
Ng, S. Y., and Lee, A. Y. W. (2019). Traumatic Brain Injuries: Pathophysiology and Potential Therapeutic Targets. Front. Cell. Neurosci. 2019:13.
Okigbo, A. A., Helkowski, M. S., Royes, B. J., Bleimeister, I. H., Lam, T. R., Bao, G. C., et al. (2019). Dose-dependent neurorestorative effects of amantadine after cortical impact injury. Neurosci. Lett. 694, 69–73. doi: 10.1016/j.neulet.2018.11.030
Osier, N. D., and Dixon, C. E. (2016a). Catecholaminergic based therapies for functional recovery after TBI. Brain Res. 1640, 15–35. doi: 10.1016/j.brainres.2015.12.026
Osier, N. D., and Dixon, C. E. (2016b). The Controlled Cortical Impact Model: Applications, Considerations for Researchers, and Future Directions. Front. Neurol. 2016:7.
Otero Villaverde, S., Martin Mourelle, R., Crespo Lopez, C., Cabrera Sarmiento, J. J., Ruiz Castillo, A., and Canosa Hermida, E. (2019). Uso de bromocriptina en pacientes con alteración de conciencia secundaria a traumatismo craneoencefálico [Bromocriptine in disorders of consciouness due to traumatic brain injury]. Rehabilitacion 53, 155–161. doi: 10.1016/j.rh.2019.03.001
Ozga, J. E., Povroznik, J. M., Engler-Chiurazzi, E. B., and Vonder Haar, C. (2018). Executive (dys)function after traumatic brain injury: special considerations for behavioral pharmacology. Behav. Pharm. 29, 617–637. doi: 10.1097/fbp.0000000000000430
Park, E., Ai, J., and Baker, A. J. (2007). “Cerebellar injury: clinical relevance and potential in traumatic brain injury research,” in Progress in Brain Research, eds J. T. Weber and A. I. R. Maas (Amsterdam: Elsevier), 327–338. doi: 10.1016/s0079-6123(06)61023-6
Passler, M. A., and Riggs, R. V. (2001). Positive outcomes in traumatic brain injury–vegetative state: Patients treated with bromocriptine. Archiv. Phys. Med. Rehabilit. 82, 311–315. doi: 10.1053/apmr.2001.20831
Phelps, T. I., Bondi, C. O., Ahmed, R. H., Olugbade, Y. T., and Kline, A. E. (2015). Divergent long-term consequences of chronic treatment with haloperidol, risperidone, and bromocriptine on traumatic brain injury-induced cognitive deficits. J. Neurot. 32, 590–597. doi: 10.1089/neu.2014.3711
Phelps, T. I., Bondi, C. O., Mattiola, V. V., and Kline, A. E. (2017). Relative to Typical Antipsychotic Drugs, Aripiprazole Is a Safer Alternative for Alleviating Behavioral Disturbances After Experimental Brain Trauma. Neurorehabil. Neural. Repair. 31, 25–33. doi: 10.1177/1545968316650281
Plummer, N. R., Tam, A. W. F., Mulvaney, C. A., Preston, N. J., and Laha, S. K. (2018). Dopamine agonists for traumatic brain injury. Cochrane Database Syst. Rev. 2018:CD013062.
Polich, G., Iaccarino, M. A., and Zafonte, R. (2019). Psychopharmacology of traumatic brain injury. Handb. Clin. Neurol. 165, 253–267. doi: 10.1016/b978-0-444-64012-3.00015-0
Primus, E. A., Bigler, E. D., Anderson, C. V., Johnson, S. C., Mueller, R. M., and Blatter, D. (1997). Corpus striatum and traumatic brain injury. Brain Inj. 11, 577–586. doi: 10.1080/026990597123278
Rangel-Barajas, C., Coronel, I., and Floran, B. (2015). Dopamine Receptors and Neurodegeneration. Aging and Disease 6, 349–368. doi: 10.14336/ad.2015.0330
Rapanelli, M. (2017). The magnificent two: histamine and the H3 receptor as key modulators of striatal circuitry. Prog. Neuro-Psychopharm. Biol. Psychiatr. 73, 36–40. doi: 10.1016/j.pnpbp.2016.10.002
Rapanelli, M., Frick, L. R., Horn, K. D., Schwarcz, R. C., Pogorelov, V., Nairn, A. C., et al. (2016). The Histamine H3 Receptor Differentially Modulates Mitogen-activated Protein Kinase (MAPK) and Akt Signaling in Striatonigral and Striatopallidal Neurons. J. Biol. Chem. 291, 21042–21052. doi: 10.1074/jbc.m116.731406
Rau, T., Ziemniak, J., and Poulsen, D. (2016). The neuroprotective potential of low-dose methamphetamine in preclinical models of stroke and traumatic brain injury. Prog. Neuro-Psychopharm. Biol. Psychiatr. 64, 231–236. doi: 10.1016/j.pnpbp.2015.02.013
Reddy, C. C., Collins, M., Lovell, M., and Kontos, A. P. (2013). Efficacy of Amantadine Treatment on Symptoms and Neurocognitive Performance Among Adolescents Following Sports-Related Concussion. J. Head Trauma Rehabilitat. 2013:28.
Reep, R. L., Cheatwood, J. L., and Corwin, J. V. (2003). The associative striatum: Organization of cortical projections to the dorsocentral striatum in rats. J. Comparat. Neurol. 467, 271–292. doi: 10.1002/cne.10868
Reep, R. L., and Corwin, J. V. (1999). Topographic organization of the striatal and thalamic connections of rat medial agranular cortex. Brain Res. 841, 43–52. doi: 10.1016/s0006-8993(99)01779-5
Reiner, A., Hart, N. M., Lei, W. L., and Deng, Y. P. (2010). Corticostriatal projection neurons - dichotomous types and dichotomous functions. Front. Neuroanat. 4, 1–15. doi: 10.1007/978-3-7643-8732-7_1
Repantis, D., Bovy, L., Ohla, K., Kühn, S., and Dresler, M. (2021). Cognitive enhancement effects of stimulants: a randomized controlled trial testing methylphenidate, modafinil, and caffeine. Psychopharmacology 238, 441–451. doi: 10.1007/s00213-020-05691-w
Sanchez-Lemus, E., and Arias-Montano, J. A. (2004). Histamine H-3 receptor activation inhibits dopamine D-1 receptor-induced cAMP accumulation in rat striatal slices. Neurosci. Lett. 364, 179–184. doi: 10.1016/j.neulet.2004.04.045
Santana, N., and Artigas, F. (2017). Laminar and Cellular Distribution of Monoamine Receptors in Rat Medial Prefrontal Cortex. Front. Neuroanat. 11:87–87.
Sawyer, E., Maura, L. S., and Ohlinger, M. J. (2008). Amantadine Enhancement of Arousal and Cognition After Traumatic Brain Injury. Ann. Pharmacother. 42, 247–252. doi: 10.1345/aph.1k284
Schmitz, Y., Benoit-Marand, M., Gonon, F., and Sulzer, D. (2003). Presynaptic regulation of dopaminergic neurotransmission. J. Neurochem. 87, 273–289. doi: 10.1046/j.1471-4159.2003.02050.x
Schmitz, Y., Schmauss, C., and Sulzer, D. (2002). Altered dopamine release and uptake kinetics in mice lacking D2 receptors. J. Neurosci. 22, 8002–8009. doi: 10.1523/jneurosci.22-18-08002.2002
Schonfeld, L. M., Dooley, D., Jahanshahi, A., Temel, Y., and Hendrix, S. (2017). Evaluating rodent motor functions: Which tests to choose? Neurosci. Biobehav. Rev. 83, 298–312. doi: 10.1016/j.neubiorev.2017.10.021
Seniów, J., Litwin, M., Litwin, T., Leśniak, M., and Członkowska, A. (2009). New approach to the rehabilitation of post-stroke focal cognitive syndrome: Effect of levodopa combined with speech and language therapy on functional recovery from aphasia. J. Neurolog. Sci. 283, 214–218. doi: 10.1016/j.jns.2009.02.336
Shepherd, G. M. G. (2013). Corticostriatal connectivity and its role in disease. Nat. Rev. Neurosci. 14, 278. doi: 10.1038/nrn3469
Shi, K., Zhang, J., Dong, J.-F., and Shi, F.-D. (2019). Dissemination of brain inflammation in traumatic brain injury. Cell. Mole. Immunol. 16:1.
Shin, S. S., Bray, E. R., Zhang, C. Q., and Dixon, C. E. (2011). Traumatic brain injury reduces striatal tyrosine hydroxylase activity and potassium-evoked dopamine release in rats. Brain Res. 1369, 208–215. doi: 10.1016/j.brainres.2010.10.096
Shipp, S. (2017). The functional logic of corticostriatal connections. Brain Struct. Funct. 222, 669–706. doi: 10.1007/s00429-016-1250-9
Silberberg, G., and Bolam, J. P. (2015). Local and afferent synaptic pathways in the striatal microcircuitry. Curr. Opin. Neurobiol. 33, 182–187. doi: 10.1016/j.conb.2015.05.002
Smith, Y., and Parent, A. (1986). Differential connections of caudate nucleus and putamen in the squirrel monkey (Saimiri sciureus). Neuroscience 18, 347–371. doi: 10.1016/0306-4522(86)90159-4
Smith, Y., Raju, D. V., Pare, J. F., and Sidibe, M. (2004). The thalamostriatal system: a highly specific network of the basal ganglia circuitry. Trends Neurosci. 27, 520–527. doi: 10.1016/j.tins.2004.07.004
Spritzer, S. D., Kinney, C. L., Condie, J., Wellik, K. E., Hoffman-Snyder, C. R., Wingerchuk, D. M., et al. (2015). Amantadine for Patients With Severe Traumatic Brain Injury: A Critically Appraised Topic. Neurologist 19, 61–64. doi: 10.1097/nrl.0000000000000001
Sunahara, R. K., Guan, H. C., O’dowd, B. F., Seeman, P., Laurier, L. G., Ng, G., et al. (1991). Cloning of the gene for a human dopamine D5 receptor with higher affinity for dopamine than D1. Nature 350, 614–619. doi: 10.1038/350614a0
Surmeier, D. J., Ding, J., Day, M., Wang, Z., and Shen, W. (2007). D1 and D2 dopamine-receptor modulation of striatal glutamatergic signaling in striatal medium spiny neurons. Trends Neurosci. 30, 228–235. doi: 10.1016/j.tins.2007.03.008
Surmeier, D. J., Song, W. J., and Yan, Z. (1996). Coordinated expression of dopamine receptors in neostriatal medium spiny neurons. J. Neurosci. 16, 6579–6591. doi: 10.1523/jneurosci.16-20-06579.1996
Ter Mors, B. J., Backx, A. P. M., Spauwen, P., Ponds, R. W. H. M., Van Harten, P. N., and Van Heugten, C. M. (2019). Efficacy of amantadine on behavioural problems due to acquired brain injury: A systematic review. Brain Inj. 33, 1137–1150. doi: 10.1080/02699052.2019.1631482
Traeger, J., Hoffman, B., Misencik, J., Hoffer, A., and Makii, J. (2020). Pharmacologic Treatment of Neurobehavioral Sequelae Following Traumatic Brain Injury. Crit. Care Nurs. Q. 2020:43.
Treble-Barna, A., Wade, S. L., Martin, L. J., Pilipenko, V., Yeates, K. O., Taylor, H. G., et al. (2017). Influence of Dopamine-Related Genes on Neurobehavioral Recovery after Traumatic Brain Injury during Early Childhood. J. Neurotr. 34, 1919–1931. doi: 10.1089/neu.2016.4840
Tsuda, S., Golam, M., Hou, J., Nelson, R., Bernavil, P., Richardson, K., et al. (2020). Altered monoaminergic levels, spasticity, and balance disability following repetitive blast-induced traumatic brain injury in rats. Brain Res. 1747, 147060. doi: 10.1016/j.brainres.2020.147060
Undieh, A. S. (2010). Pharmacology of signaling induced by dopamine D-1-like receptor activation. Pharm. Ther. 128, 37–60. doi: 10.1016/j.pharmthera.2010.05.003
Valjent, E., Bertran-Gonzalez, J., Herve, D., Fisone, G., and Girault, J.-A. (2009). Looking BAC at striatal signaling: cell-specific analysis in new transgenic mice. Trends Neurosci. 32, 538–547. doi: 10.1016/j.tins.2009.06.005
Vallone, D., Picetti, R., and Borrelli, E. (2000). Structure and function of dopamine receptors. Neurosci. Biobehav. Rev. 24, 125–132.
Van Bregt, D. R., Thomas, T. C., Hinzman, J. M., Cao, T., Liu, M., Bing, G., et al. (2012). Substantia nigra vulnerability after a single moderate diffuse brain injury in the rat. Exp. Neurol. 234, 8–19. doi: 10.1016/j.expneurol.2011.12.003
Van Tol, H. H., Bunzow, J. R., Guan, H. C., Sunahara, R. K., Seeman, P., Niznik, H. B., et al. (1991). Cloning of the gene for a human dopamine D4 receptor with high affinity for the antipsychotic clozapine. Nature 350, 610–614. doi: 10.1038/350610a0
Van Vleet, T. M., Heldt, S. A., Pyter, B., Corwin, J. V., and Reep, R. L. (2003). Effects of light deprivation on recovery from neglect and extinction induced by unilateral lesions of the medial agranular cortex and dorsocentral striatum. Behav. Brain Res. 138, 165–178. doi: 10.1016/s0166-4328(02)00246-2
Wagner, A. K., Chen, X. B., Kline, A. E., Li, Y. M., Zafonte, R. D., and Dixon, C. E. (2005a). Gender and environmental enrichment impact dopamine transporter expression after experimental traumatic brain injury. Exp. Neurol. 195, 475–483. doi: 10.1016/j.expneurol.2005.06.009
Wagner, A. K., Kline, A. E., Ren, D., Willard, L. A., Wenger, M. K., Zafonte, R. D., et al. (2007). Gender associations with chronic methylphenidate treatment and behavioral performance following experimental traumatic brain injury. Behav. Brain Res. 181, 200–209. doi: 10.1016/j.bbr.2007.04.006
Wagner, A. K., Scanlon, J. M., Becker, C. R., Ritter, A. C., Niyonkuru, C., Dixon, C. E., et al. (2014). The influence of genetic variants on striatal dopamine transporter and D2 receptor binding after TBI. J. Cereb. Blood flow Metab. 34, 1328–1339. doi: 10.1038/jcbfm.2014.87
Wagner, A. K., Sokoloski, J. E., Ren, D., Chen, X., Khan, A. S., Zafonte, R. D., et al. (2005b). Controlled cortical impact injury affects dopaminergic transmission in the rat striatum. J. Neurochem. 95, 457–465. doi: 10.1111/j.1471-4159.2005.03382.x
Wang, Y. H., and Zhou, F. M. (2017). Striatal But Not Extrastriatal Dopamine Receptors Are Critical to Dopaminergic Motor Stimulation. Front. Pharm. 8, 1–13.
Warden, D. L., Gordon, B., Mcallister, T. W., Silver, J. M., Barth, J. T., Bruns, J., et al. (2006). Guidelines for the Pharmacologic Treatment of Neurobehavioral Sequelae of Traumatic Brain Injury. J. Neurot. 23, 1468–1501.
Weber, P., and Lütschg, J. (2002). Methylphenidate treatment. Pediatr. Neurol. 26, 261–266. doi: 10.1016/s0887-8994(01)00408-8
Whyte, J., Hart, T., Vaccaro, M., Grieb-Neff, P., Risser, A., Polansky, M., et al. (2004). Effects of Methylphenidate on Attention Deficits After Traumatic Brain Injury: A Multidimensional, Randomized, Controlled Trial. Am. J. Phys. Med. Rehabilit. 2004:83.
Whyte, J., Vaccaro, M., Grieb-Neff, P., Hart, T., Polansky, M., and Coslett, H. B. (2008). The Effects of Bromocriptine on Attention Deficits After Traumatic Brain Injury: A Placebo-Controlled Pilot Study. Am. J. Phys. Med. Rehabilit. 87, 85–99. doi: 10.1097/phm.0b013e3181619609
Wickens, J. R., and Wilson, C. J. (1998). Regulation of action-potential firing in spiny neurons of the rat neostriatum in vivo. J. Neurophys. 79, 2358–2364. doi: 10.1152/jn.1998.79.5.2358
Wiley, C. A., Bissel, S. J., Lesniak, A., Dixon, C. E., Franks, J., Stolz, D. B., et al. (2016). Ultrastructure of Diaschisis Lesions after Traumatic Brain Injury. J. Neurotr. 33, 1866–1882. doi: 10.1089/neu.2015.4272
Williamson, D., Frenette, A. J., Burry, L. D., Perreault, M., Charbonney, E., Lamontagne, F., et al. (2019). Pharmacological interventions for agitated behaviours in patients with traumatic brain injury: a systematic review. BMJ Open 9, e029604. doi: 10.1136/bmjopen-2019-029604
Wortzel, H. S., and Arciniegas, D. B. (2012). Treatment of Post-Traumatic Cognitive Impairments. Curr. Treat. Option. Neurol. 14, 493–508. doi: 10.1007/s11940-012-0193-6
Wouterlood, F. G., Van Oort, S., Bloemhard, L., Flierman, N. A., Spijkerman, J., and Wright, C. I (2018). Neurochemical fingerprinting of amygdalostriatal and intra-amygdaloid projections: a tracing–immunofluorescence study in the rat. J. Chem. Neuroanat. 94, 154–172. doi: 10.1016/j.jchemneu.2018.11.001
Wu, J. H., Corwin, J. V., and Reep, R. L. (2009). Organization of the corticostriatal projection from rat medial agranular cortex to far dorsolateral striatum. Brain Res. 1280, 69–76. doi: 10.1016/j.brainres.2009.05.044
Wu, T. S., and Garmel, G. M. (2005). Improved neurological function after Amantadine treatment in two patients with brain injury. J. Emer. Med. 28, 289–292. doi: 10.1016/j.jemermed.2004.11.016
Yan, H. Q., Ma, X., Chen, X., Li, Y., Shao, L., and Dixon, C. E. (2007). Delayed increase of tyrosine hydroxylase expression in rat nigrostriatal system after traumatic brain injury. Brain Res. 1134, 171–179. doi: 10.1016/j.brainres.2006.11.087
Yoshida, S. T., Baella, S. A., Stuebner, N. M., Crawford, C. A., and Mcdougall, S. A. (2006). Effects of a partial D2-like receptor agonist on striatal dopamine autoreceptor functioning in preweanling rats. Brain Res. 1073-1074, 269–275. doi: 10.1016/j.brainres.2005.12.054
Zakhary, T., and Sabry, A. (2017). Bromocriptine in Central Hyperthermia after Severe Traumatic Brain Injury. Open J. Emer. Med. 5, 102–110. doi: 10.4236/ojem.2017.53010
Keywords: dopamine, corticostriatal pathway, functional recovery, traumatic brain injury, dopamine receptors
Citation: Verduzco-Mendoza A, Carrillo-Mora P, Avila-Luna A, Gálvez-Rosas A, Olmos-Hernández A, Mota-Rojas D and Bueno-Nava A (2021) Role of the Dopaminergic System in the Striatum and Its Association With Functional Recovery or Rehabilitation After Brain Injury. Front. Neurosci. 15:693404. doi: 10.3389/fnins.2021.693404
Received: 10 April 2021; Accepted: 03 June 2021;
Published: 24 June 2021.
Edited by:
Santiago Perez-Lloret, Consejo Nacional de Investigaciones Científicas y Técnicas (CONICET), ArgentinaReviewed by:
Yoland Smith, Emory University, United StatesHarumasa Takano, National Center of Neurology and Psychiatry, Japan
Copyright © 2021 Verduzco-Mendoza, Carrillo-Mora, Avila-Luna, Gálvez-Rosas, Olmos-Hernández, Mota-Rojas and Bueno-Nava. This is an open-access article distributed under the terms of the Creative Commons Attribution License (CC BY). The use, distribution or reproduction in other forums is permitted, provided the original author(s) and the copyright owner(s) are credited and that the original publication in this journal is cited, in accordance with accepted academic practice. No use, distribution or reproduction is permitted which does not comply with these terms.
*Correspondence: Antonio Bueno-Nava, YWJ1ZW5vQGluci5nb2IubXg=; dG9uNjMxQGhvdG1haWwuY29t