- 1Department of Biological and Medical Psychology, University of Bergen, Bergen, Norway
- 2Department of Education, The Arctic University of Norway, Tromsø, Norway
- 3Mohn Medical Imaging and Visualization Centre, Haukeland University Hospital, Bergen, Norway
Auditory repetition suppression and omission activation are opposite neural phenomena and manifestations of principles of predictive processing. Repetition suppression describes the temporal decrease in neural activity when a stimulus is constant or repeated in an expected temporal fashion; omission activity is the transient increase in neural activity when a stimulus is temporarily and unexpectedly absent. The temporal, repetitive nature of musical rhythms is ideal for investigating these phenomena. During an fMRI session, 10 healthy participants underwent scanning while listening to musical rhythms with two levels of metric complexity, and with beat omissions with different positional complexity. Participants first listened to 16-s-long presentations of continuous rhythms, before listening to a longer continuous presentation with beat omissions quasi-randomly introduced. We found deactivation in bilateral superior temporal gyri during the repeated presentation of the normal, unaltered rhythmic stimulus, with more suppression of activity in the left hemisphere. Omission activation of bilateral middle temporal gyri was right lateralized. Persistent activity was found in areas including the supplementary motor area, caudate nucleus, anterior insula, frontal areas, and middle and posterior cingulate cortex, not overlapping with either listening, suppression, or omission activation. This suggests that the areas are perhaps specialized for working memory maintenance. We found no effect of metric complexity for either the normal presentation or omissions, but we found evidence for a small effect of omission position—at an uncorrected threshold—where omissions in the more metrical salient position, i.e., the first position in the bar, showed higher activation in anterior cingulate/medial superior frontal gyrus, compared to omissions in the less salient position, in line with the role of the anterior cingulate cortex for saliency detection. The results are consistent with findings in our previous studies on Parkinson’s disease, but are put into a bigger theoretical frameset.
Introduction
New musical forms and experiments often challenge established structural forms in composition or reshape old ones into new uses, reordering fundamental musical building blocks to challenge the perception of music. The challenge of studying such diverse music in a principled and systematic manner within a context of neuroscience demands first to establish some fundamental and perhaps common mechanisms in music perception, a work that has been flourishing in the last decades. In this article, we focus on rhythm, one of these fundamental building blocks. Within new music, some artists work with rhythmic entropy while others try to dispel rhythm altogether. Insights into some basic mechanisms of the “listening apparatus” in our nervous system as they pertain to perception of musical rhythms might perhaps still be of use to both researchers and artists, either as a starting point for more advanced research or as a starting point of artistic defiance.
In short, auditory repetition suppression and omission activation, which this study address, are opposite neural phenomena and manifestations of principles of predictive processing. Repetition suppression is the reduction of neuronal activity during listening to a repeated sound that is present, omission activation is the increased neuronal activity that occurs when an expected sound is not present. Listening activates bilateral superior temporal cortices, independent of whether we are exposed to tones, words, animal, and instrumental sounds (Specht and Reul, 2003), while prolonged listening to an unchanging sequence of sounds quickly leads to a deactivation of neural activity, a phenomenon known as repetition suppression (Grill-Spector et al., 2006). Auditory repetition suppression is a robust, experience-dependent adjustment of neural functions (Grotheer and Kovacs, 2016), predictability (Costa-Faidella et al., 2011; Cacciaglia et al., 2019), and prior expectation (Summerfield et al., 2008; Todorovic et al., 2011). It is modulated by a range of factors, such as time scales of presentation rates, sequence position and stimuli similarities (Kovács and Schweinberger, 2016), and stimuli-specific characteristics (Linke et al., 2011), and is also task-dependent (Arnott et al., 2005). Repetition suppression also neatly demonstrates principles of predictive coding (Friston, 2005; Baldeweg, 2006; Auksztulewicz and Friston, 2016), where repetition suppression biases the activity in sensory cortices (Sreenivasan et al., 2014). For auditory stimuli, repetition suppression can be seen in the temporal cortices, including Herschel’s gyrus (HG), superior temporal gyrus (STG), and middle temporal gyrus (MTG) (Auksztulewicz and Friston, 2016; Cacciaglia et al., 2019).
Repetition suppression is related to working memory mechanisms, where attenuation of neural activity can be interpreted as a minimization of activity needed in a working memory maintenance stage (Kumar et al., 2016) through the suppression of irrelevant information (Linke et al., 2011; Ahveninen et al., 2017). It is, however, unclear whether working memory is dependent on persistent neural activity (for maintaining the information) (Huang et al., 2016) or on transient reorganization of synaptic weights (D’Esposito and Postle, 2015) in representational states (Myers et al., 2017; Sreenivasan and D’Esposito, 2019) or a combination of the two (Sreenivasan et al., 2014). A potential theoretical (or actual) difference between these two descriptions—working memory as either persistent or transient neural states [or what within a predictive coding framework can be called “representation units” (Clark, 2013)]—could lie in a distinction between auditory sensory memory (shorter low-level sensory cortical retention intervals) and higher-level working memory network organization (Nees, 2016). Different cortical activation for simple sequence processing and more complex, task-specific working-memory maintenance could point to such nuances (Brechmann et al., 2007). Neural correlates for auditory working memory have been shown in temporal cortices, including STG, HG, and planum temporale (PT) (Brechmann et al., 2007; Kumar et al., 2016). Furthermore, distinct neural differences between perceptual processing and active working memory tasks for melody and pitch (Zatorre et al., 1994), separate neural correlates for duration-based and beat-based auditory timing (Teki et al., 2011), and differentiations for melody and rhythm have been shown—with the right inferior frontal gyrus and insula particularly involved (Jerde et al., 2011).
Within a predictive coding framework, auditory prediction errors (Friston, 2005) must depend on working memory or sensory memory mechanisms, since they occur when an unchanging and predictable sequence of sounds suddenly changes, i.e., when predictions and expectations are breached, or when the incoming sensory signal does not match the “representation unit” (Clark, 2013); what we will henceforth call the representational maintenance. The concept of prediction errors [or “surprisal” (Clark, 2013)] draws on findings in EEG/ERP-studies on mismatch negativity (MMN) (Naatanen et al., 1978; Kompus et al., 2015). MMN potentials are measurable neural spikes triggered by deviant and rare stimuli in a chain of standard stimuli, where the difference between the deviant and the standard stimuli are proportional to the deviance [see (Näätänen, 1992) for a review]. This difference is the mismatch or prediction error (Friston, 2005). In fMRI, as in EEG/ERP studies, the size of activation reflects the magnitude of the MMN deviant (Mathiak et al., 2002; Liebenthal et al., 2003; Eichele et al., 2008). Omissions are a particularly interesting type of deviant stimuli, where omission activation (Raij et al., 1997; Wacongne et al., 2011) describes cortical activation as a result of missing stimuli in a predictable sequence of sounds, and can therefore be assumed to be generating internal responses based solely on expectancy or prediction, and not by a change in the deviant characteristics in the stimuli itself (Jongsma et al., 2005). As with repetition suppression, MMNs or prediction error magnitude is modulated by numerous factors (Näätänen, 1992), and as with repetition suppression and auditory working memory, specific parts of the temporal cortices have repeatedly been shown to be involved in the reporting of such prediction errors (Friston, 2005). Imaging and electrophysiological studies have consistently shown that a main source of MMN potentials is located in the intersection of STG, PT, and HG (Recasens et al., 2014), predominantly right lateralized (Tervaniemi et al., 2000; Opitz et al., 2002; Doeller et al., 2003; Rinne et al., 2005), also for omissions (Mustovic et al., 2003; Voisin et al., 2006; SanMiguel et al., 2013a, b), although one study has found omission activation predominantly on the left (Nazimek et al., 2013). Pertaining to our study, these cortical areas are sensitive to beat and pattern deviations, as shown in both EEG/ERP and fMRI studies (Tervaniemi et al., 2000; Opitz et al., 2002; Doeller et al., 2003; Mustovic et al., 2003; Rinne et al., 2005; Voisin et al., 2006; Nazimek et al., 2013; Recasens et al., 2014). Beat omission and positional saliency have been used to investigate rhythm and pattern-related phenomena with several imaging and neurophysiological techniques, indicating different levels of magnitude for salient and less salient metric beat positions, although findings are somewhat ambiguous (Winkler and Schroger, 1995; Jongsma et al., 2003, 2005, 2006; Ladinig et al., 2009; Wacongne et al., 2011; Salisbury, 2012; Bouwer et al., 2014; Damsma and van Rijn, 2016).
In the current study, we wanted to examine repetition suppression, representational maintenance, and omission activation during the perceptual processing of musical rhythms. In short, these three phenomena can be seen as manifestations of key principles in predictive processing frameworks, and musical rhythms are ideal stimuli to operationalize and demonstrate these principles because of their predictive, temporal nature (Vuust and Witek, 2014; Koelsch et al., 2019). Musical rhythms also facilitate operationalization of modulating factors such as contextual characteristics (simple or complex rhythms) and saliency (position of omission).
In addition, the neuronal mechanisms involved in the perception of musical rhythms are partly known, which makes it possible to compare our results with existing literature. Listening to rhythms activates cortical motor areas, such as premotor cortex and supplementary motor area (SMA), the basal ganglia, as well as large-scale networks across the brain (Grahn and Brett, 2007; Chen et al., 2008a, b; Bengtsson et al., 2009; Geiser et al., 2009, 2012; Grahn, 2009; Trost et al., 2014; Large et al., 2015).
We also wanted to examine the effect of pattern complexity on repetition suppression and omission activation and the effect of positional saliency on omission activation. To this end, two musical rhythms, one simple and one complex, were presented several times to the participants during scans. The first part of the stimuli presentation consisted of a short presentation (16 s) of continuous, unperturbed rhythmic repetition to examine repetition suppression, segueing into a longer continuous presentation of the same rhythm. In this second part, an overt target-detection task (with a quasi-randomly distributed deviant tone) was introduced to keep the participant attending to the rhythm, while quasi-randomly distributed beat omissions were used to covertly examine omission activation (see section “Materials and Methods” for more details on the stimuli and the paradigm).
Based on previous literature, we expected to see listening, repetition suppression, and omission activation in largely overlapping areas in the temporal cortices, with omission activation occurring in more posterior areas than suppression and maintenance. We hypothesized that representation maintenance would also occur in the temporal cortices, with additional activation of inferior frontal areas, insula, and premotor cortices. We hypothesized that complexity would differentially affect suppression, as the encoding stage presumably would be affected by a higher cognitive load for the complex rhythm. We also hypothesized that omission activation would be modulated by rhythmic context where the cognitive demand (i.e., higher neural activity in a representational maintenance) of the more complex rhythm would result in smaller omission sizes, and furthermore that positional saliency would modulate omission activation, where a more salient position (beat position number one) would show higher activation than the less salient position (beat position number two). Finally, we hypothesized that there would be an interaction between pattern complexity and beat position for omission activation.
Materials and Methods
Participants
Participants were recruited among Norwegian-speaking students enrolled at the University of Bergen (UiB). Fourteen participants underwent scanning with fMRI, but four were excluded due to head movement in the scanner. Analyses were done on the remaining 10 (6 females, mean age = 24.4). Eight were right-handed by self-report. The number of years participants had played instruments (outside mandatory music lessons in public school) was done by self-report. A participant was labeled as a musician if s/he had 5 or more years of consistent instrument practice, and 5 out of the 10 participants reached this target. Personal data were coded and stored offline and anonymity was assured. All procedures were approved by the Regional Committee for Medical and Health Research Ethics (REK no 2014/1915) and carried out in accordance with the code of Ethics of the World Medical Association, Declaration of Helsinki. Upon enrollment in the study, all participants gave written informed consent to participate in the study and were rewarded 50NOK for their participation.
Stimuli
We used two musical rhythmical stimuli of different rhythmic complexity described elsewhere (Vikene et al., 2018, 2019). The stimuli consisted of deep, multilayered synthesizer bass sounds in two octaves and a sampled bass drum sound, to place the general character of the stimuli in a different frequency range than the Eigenfrequency of the scanner during the echo planar imaging (EPI) sequence. The first 8 bars (16 s) of each stimulus contained an alternating piano chord, at the first position to clearly mark the beginning of the bar. For each rhythm (simple/complex), these chords were composed in one major and one minor mode for listening variation (no tests where planned for the effect of mode). The remaining 44 bars/88 s of each stimuli were constructed with quasi-distributed overt deviant probe tones (consisting of a six-note up-shift of tonality, to keep the participants attending to the musical rhythms), and covert beat omissions. Probe tones were always placed on the first position of the bar, while omissions were placed in equal numbers on first and second positions. Each of the four versions of the stimuli blocks (simple/complex vs. major/minor) had six omissions. The omissions were either at the first or second position of the rhythmic patterns. In two versions of the blocks, three omissions were at the first position and three omissions were at the second position. In one version of the blocks, four omissions were at the first position and two were at the second position. In one version of the blocks, two omissions were at the first position and four were at the second position. The smallest time between two consecutive omissions was 8.5 s; the longest was 17.5 s. Because we were interested in the covert, or passive, detection of omission, and not active detection success, and because target detection of the probe tones involved button-pushing on a hand grip leading to motor area activity, no analysis was planned for the overt probe tone detection. All participants did, however, correctly detect all target tones. Stimuli were created using Steinberg Cubase 7 and presented in the scanner with EPrime 2.0 (Ver 2.3 Professional), which was also used to collect responses to the overt task.
Experimental Design
Participants were given earplugs and were placed comfortably in the scanner. They were given fMRI-compatible headphones with additional physical noise cancelation foamed ear plugs. Participants were also fitted with fMRI-compatible video goggles and a handgrip with buttons to respond to the overt target-detection attentional task. After initial structural scans and a 5-min-long resting-state fMRI scan (not part of this report), goggles were turned on and participants were given instructions for the study. Participants were told to keep eyes open and look at a cross in the middle of the screen and asked to press a button on the hand grip when the probe tone was detected. Instructions were followed by a short test run before scanning started. Before each trial, the same written instructions were repeated in the goggles (4.5 s), followed by a blank screen and silences ranging from 13 to 19 s. When the music stimuli began playing, a cross was presented in the goggles as a focus point to minimize head movement. Each sound file was presented twice during the scan, in randomized order between subjects. Total scan time for the paradigm was 33 min. See Figure 1 for an overview of the paradigm.
Data Acquisition and Pre-processing
fMRI images were acquired using a 3-T scanner (GE Signa Excite 750) with a 32-channel coil. Repetition time (TR) for the EPI sequence was 1.5 s, echo time (TE) was 30 ms, voxel size was 3.44 × 3.44 × 5 with 28 slices interleaved, for 1325 volumes. Pre-processing steps included realignment (0.9 quality, 5 mm smoothing kernel, registered to first image with second-degree B-spline), unwarping (using 12 × 12), resliced to mean image, normalized to ICBM template (with 2mm3 voxel size), and smoothing with Gaussian kernel (5 mm3).
First-Level Analysis
We aligned the onset of stimuli epochs (below) to 13th of the 28 interlaced slices. A high-pass filtering threshold was set at 1/249 Hz cutoff (calculated as the mean between onsets of the stimuli blocks). Single-subject data were analyzed by specifying a general linear model, and for the whole scan, movement-related variance (realignment parameters) was included in the model as six covariates of no interest.
Each block was modeled as follows: 4.5 s of on-screen instructions were labeled as “READ.” Silence periods (randomly assigned between 13 and 19 s) between each block were not segmented and thus served as contrast for all other epochs (“REST”). The whole block was segmented into 2-s bins, i.e., the total length of one whole rhythmic pattern. The first 4 s of each block were labeled “LEARNING”; the next 12 s, “SUPPRESSION.” In the following 88 s of each block, bins containing a probe tone were labeled “PROBE,” bins containing the normal, unperturbed version of the rhythmic pattern were labeled “MAINTENANCE,” while 2-s bins containing an omission were labeled according to the position of the omission (i.e., “OMISSION1” for the first position-omission). The 2-s bin immediately following an omission was labeled “NOT OF INTEREST” to avoid any secondary effects of the omission spilling into the segments labeled “MAINTENANCE.”
All blocks were divided into simple and complex rhythm, and segmentation of different epochs were labeled “SIMPLE” or “COMPLEX.” For example, the “LEARNING” epochs in the simple rhythm were called “SIMPLE_LEARNING”; for the omission in position 2 in the complex rhythm: “COMPLEX_OMISSION2.” First-level analysis produced 10 contrasts for “SIMPLE/COMPLEX” blocks, with “LEARNING/SUPPRESSION/MAINTENANCE/OMISSION (1/2)” epochs, all with REST epochs subtracted for the contrasts.
Second-Level Analysis (Initial)
A full factorial analysis—akin to a repeated measures ANOVA—was conducted, with MUSICIAN (musician/non-musician), RHYTHM (“SIMPLE/COMPLEX”), and TYPE [“LEARNING/SUPPRESSION/MAINTENANCE/OMISSION (1/2)] as dependent factors. The analysis was examined with a threshold of family-wise error (FWE) correction for multiple comparisons at p < 0.05, with a minimal voxel-cluster size of at least 10 voxels. No main effect or interaction effects were found for neither MUSICIAN nor RHYTHM, but as expected, a main effect for TYPE was found. Since we had a clear hypothesis on omission complexity and position, we did, however, probe these comparisons through t-tests, but did not find any significant differences on omission, neither between rhythms nor positions at a FWE-corrected level. For completeness, we would nonetheless mention that at an uncorrected level (p < 0.001, cluster size of 100 voxels), we found higher activation in an area in the anterior cingulate cortex (ACC)/medial prefrontal gyrus (see Supplementary Figure 1 and Supplementary Table 1).
Second-Level Analysis (Reduced Model)
Based on the lack of main and interaction effects for RHYTHM, and lack of significant t-test results on OMISSION, we decided to re-segment the data, dispelling of the division into two rhythms, as well as omission position. Furthermore, based on the lack of main and interaction effects of the categorical MUSICIAN group division, we instead included the number of years playing an instrument as a covariate in the analysis. A new full factorial analysis was therefore conducted using only TYPE as dependent factors, i.e., “LEARNING/LISTENING/MAINTENANCE/OMISSION.” A main effect for TYPE was found [F(1,31) = 18.36, p < 0.001], and we proceed to do t-tests for our planned comparisons.
Results
All results are reported with a FWE-corrected threshold of p < 0.05 and at least 10 voxels per cluster. Figure 2 shows a detailed excerpt of the frontal right hemisphere of the findings listed below. Figure 3 shows a more detailed overview of the findings across the while brain.
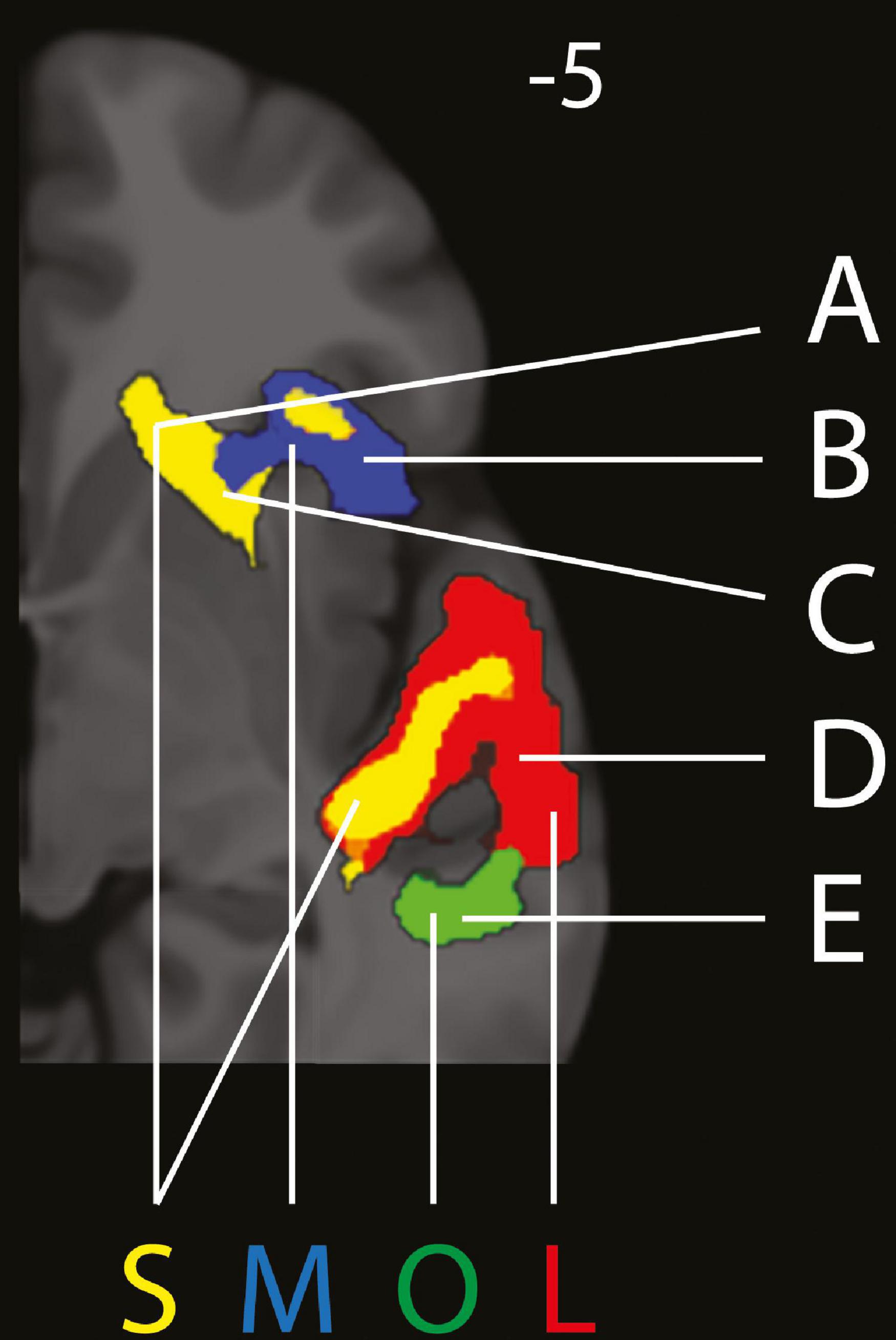
Figure 2. Excerpt from right hemisphere of separation of (in red) LISTENING (L), (in yellow) SUPRESSION (S), (in blue) MAINTENANCE (M) and (in green) OMISSION (O). A = Caudate Nucleus, B = Anterior Insula, C = Putamen, D = Superior Temporal Gyrus, E = Middle Temporal Gyrus. Panel at MNI z = –5 on the axial plane. (Figure made partly with MRIcroGL, with small cluster removal).
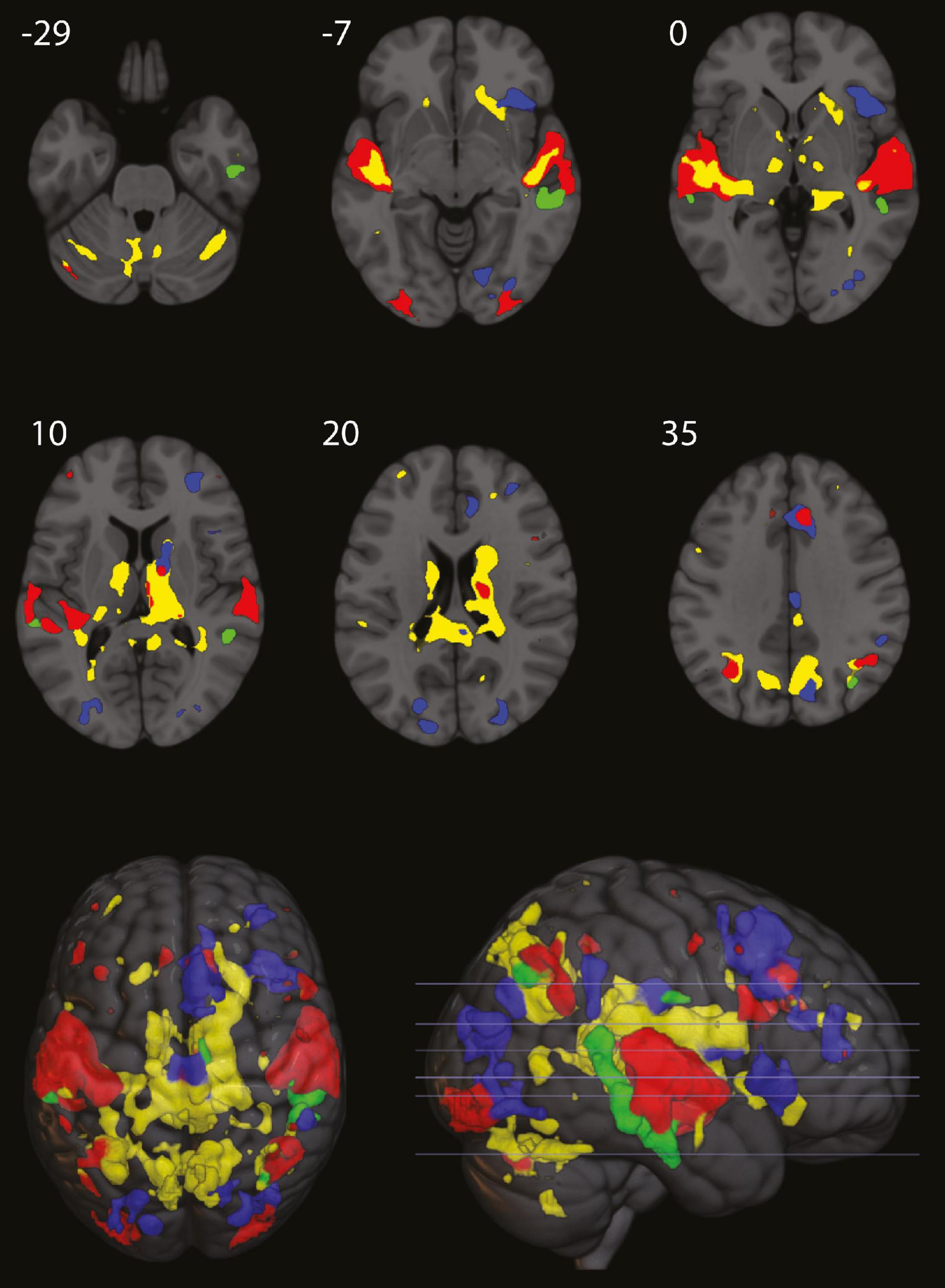
Figure 3. Red areas, overall activity during listening for the first 16 s. Yellow areas, where activity decreased in the last 12 s, compared to the first 4 s of listening. Blue areas, persistent activity for the remaining 88 s of listening (only for epochs of normal presentation of the rhythm). Green areas, beat omission activity in the middle temporal gyrus. Numbers refer to MNI coordinate on the axial plane (figure made partly with MRIcroGL, with small cluster removal).
Overall Listening
For the combined LEARNING, SUPPRESSION, and MAINTENANCE bins, we found activations in large parts of bilateral superior and middle temporal gyri (STG/MTG), as well as bilateral angular gyri, SMA, cerebellum, and posterior areas [fusiform, occipital, posterior cingulate cortex (PCC)] (Table 1).
Learning/Suppression
We contrasted the first 4 s of the introduction of music, LEARNING, by subtracting the following 12 s of SUPPRESSION. This showed more bilateral activations across many areas, including STG, angular gyri, and cerebellum (crus 1 and vermis 6), the basal ganglia (caudate nucleus and putamen), and thalamus, in the first 4 s (Table 2).
Maintenance
We subtracted the last of the 12 s of the initial listening (SUPPRESSION) from MAINTENANCE to examine which brain areas, after the initial listening period, showed more activation during maintenance of the rhythm throughout the remaining 88 s. The rationale behind this contrast was that after the first 4 s (LEARNING), the next 12 s (SUPPRESSION) consolidates the (reduced) neural activation related to the establishment of a predictive model of the rhythmic pattern, while MAINTENANCE represents additional areas needed to keep the rhythmic pattern in working memory pertaining to the overt task. Areas including SMA; caudate nucleus; anterior insula (AIN); superior, middle, and inferior frontal gyrus (SFG/MFG/IFG); and middle and posterior cingulate cortex, as well as parts of the occipital cortex, showed more activation during MAINTENANCE than during SUPPRESSION (Table 3).
Omission
We contrasted OMISSION by subtracting MAINTENANCE to examine which brain areas were activated during beat omissions. The rationale behind this contrast was that MAINTENANCE, as stated above, represents areas needed to keep the rhythmic pattern in working memory, while OMISSION represents the neural activation related to the prediction error triggered by the missing beat.
For OMISSION, more activation was seen, predominantly in the right MTG, extending from the inferior to the superior temporal gyrus. In addition, a smaller activation was seen in the left MTG as well as in the right angular gyrus (Table 4).
Discussion
Listening to musical rhythms predictably activated the bilateral STG as well as bilateral angular gyrus. Areas in the STG attenuated after the first 4 s of repetition of the rhythmic patterns overlapped exclusively with these areas, with the size of deactivation being larger in the left STG. In addition, activity in bilateral cerebellum, and the (predominantly right) basal ganglia, including caudate nucleus, putamen, and thalamus, decreased after the first 4 s. Since processing of music (Zatorre and Zarate, 2012) and particularly rhythm (Thaut et al., 2014) has been found to be right lateralized (Large et al., 2015), this larger deactivation in the left STG might reflect an asymmetric allocation of resources, where—after initial processing in sensory cortices—the processing of musical rhythms is predominantly done in the right STG. The rapid deactivation of the basal ganglia and cerebellar areas points to a role for these areas in initial beat detection (Peretz and Zatorre, 2005; Grahn, 2009).
During the prolonged listening to rhythms after the initial 16 s of encoding, larger activity was found in the SMA and the caudate nucleus, areas well known to be activated by rhythm (Grahn and Brett, 2007; Bengtsson et al., 2009). Areas related to attention, such as the anterior insula and frontal areas (the inferior frontal gyrus in particular, but also middle and superior frontal areas), were also activated. These areas have been directly implemented in rhythm perception (Chapin et al., 2010; Heard and Lee, 2020), in particular as crucial in working memory for rhythm (Jerde et al., 2011). Activity in the precuneus, the posterior part of the cingulate cortex, and middle prefrontal cortex has in addition been found to play a particular role in the maintenance of musical beats with high beat salience (Toiviainen et al., 2020), which the rhythms in the current study must be characterized as. The areas found to be more activated during maintenance (anterior insula, frontal areas, SMA, posterior cingulate, and precuneus), with more activity in the right hemisphere, were clearly distinct compared to the other conditions and could indicate that working memory mechanisms of representational maintenance are allocated in different areas of the brain, separate from primary cortices activated during initial sensory processing.
Omission activation was distinctly more posterior than listening, suppression, and maintenance, located predominantly in the MTG, and, as expected, omission activation was significantly bigger in the right MTG (Raij et al., 1997) (see Figure 3 and Table 4), with coordinates closely matching those found in previous fMRI (Mustovic et al., 2003; Voisin et al., 2006) and EEG (SanMiguel et al., 2013a, b) studies on omissions and silences in healthy controls and in our previous fMRI study on persons with Parkinson’s disease (Vikene et al., 2019).
In addition, at uncorrected levels, the more salient position of the omission showed a higher activation in the ACC, consistent with previous research implicating the ACC in saliency detection (Menon, 2011) (Supplementary Figure 1 and Supplementary Table 1).
On a theoretical level, our findings can be interpreted as manifestations of crucial principles in predictive processing frameworks, where repetition suppression (Friston, 2005; Baldeweg, 2006; Auksztulewicz and Friston, 2016) can be interpreted as model building; maintenance (Brechmann et al., 2007; Kumar et al., 2016) as a “representation unit” (Clark, 2013); and omission activation (Raij et al., 1997; Wacongne et al., 2011)—an internal response based solely on expectancy or prediction (Jongsma et al., 2005)—as a prediction error (Friston, 2005). Despite the limitations in this study, we will claim that it robustly shows results in line with previous findings on repetition suppression and omission activation—and the perception of musical rhythms in general—and that our paradigm, concretely or abstractly, can be used as a starting point for more refined studies of predictive processing mechanisms for the perception of musical rhythms.
Limitations
Due to the small sample of participants (n = 10) taken from a homogenous population of Western Educated Industrialized Rich Democratic (WEIRD) students of psychology, our findings are difficult to generalize. During a continuous scanning paradigm, the scanner is never silent, which makes the study of omissions questionable, although our results are consistent with previous research. Previous studies have also shown differences in omission detection between musicians and non-musicians (Ono et al., 2013, 2015), but we did not find a difference between them in this study. This might be a result of a low number of participants in the study. Using musical stimuli at “ecological” tempo (120 bpm), with 250 ms ISI in the isochronous metric framework, meant that omissions had to be distributed at fairly long intervals (between 8 and 17.5 s), and to amass sufficient instances of the omissions for statistical analysis, the total scan time for the paradigm was long (33 min). The lengthy paradigm could have affected levels of vigilance and attention during the scan and, as a consequence, could have influenced the results. We did try to remedy this by adding an overt target-detection task, which all participants performed correctly. Furthermore, we only used two rhythms, both of which were repeated several times during the scans. The consequence of longer-term habituation and learning effects could therefore also have influenced the results. In addition, the lack of effects on complexity in our study could indicate that the two rhythms chosen for the study did not differ (enough) in their level of complexity to yield such differences. Finally, due to the poor temporal resolution of fMRI, we might not have been able to pick up finer details of the mechanisms we have tried to describe. Future studies should try to limit paradigm length and use more varied and perhaps “real” musical samples, also with more levels of complexity. Factors such as musical aptitude, level of vigilance, and general working memory capacity should also be taken into consideration in future studies.
Conclusion
Our study successfully replicated previous findings for repetition suppression and omission activation and shows that tailored musical stimuli can be used in an fMRI setting to robustly investigate such neural phenomena, even with a limited number of participants (n = 10). Importantly, our findings show a clear separation between repetition suppression and prediction error activation, and additionally indicate that representational maintenance activates areas different from those deactivated during repetition suppression. While listening and subsequent repetition suppression were located mainly in anterior parts of the superior temporal gyrus, prediction errors (omission activation) were clearly separated from these areas and located mainly in posterior parts of the MTG. Representational maintenance activated SMA, caudate nucleus, anterior insula, frontal areas, and middle and posterior cingulate cortex, potentially showing persistent representational maintenance activity in areas separate from initial listening (encoding), repetition suppression (attenuation), and prediction error (omission).
Data Availability Statement
The raw data supporting the conclusions of this article will be made available by the authors, without undue reservation.
Ethics Statement
The studies involving human participants were reviewed and approved by Regional Committee for Medical and Health Research Ethics, REK-VEST (2014/1915). The patients/participants provided their written informed consent to participate in this study.
Author Contributions
UF, KS, and KV contributed in the conception of the research project, did statistical analysis, and contributed to the final version of the manuscript. UF recruited the participants, organized the study, and wrote the first draft. KV designed the paradigm. All authors contributed to the article and approved the submitted version.
Funding
This project was financed through a grant from the Research Council of Norway (Grant Number: 217932/F20) awarded to KS. The salary of UF was covered in part by a master student scholarship at the University of Bergen, and in part by a grant from the Research Council of Norway (Grant Number: 260576) awarded to Stefan Koelsch. KV has received traveling grants from Meltzer’s Fund, University of Bergen, Norwegian Research School of Neuroscience, and Department of Biological and Medical Psychology, University of Bergen, and financial support through GC Rieber Foundations and the Research Council of Norway (Grant Number: 217932/F20).
Conflict of Interest
The authors declare that the research was conducted in the absence of any commercial or financial relationships that could be construed as a potential conflict of interest.
Publisher’s Note
All claims expressed in this article are solely those of the authors and do not necessarily represent those of their affiliated organizations, or those of the publisher, the editors and the reviewers. Any product that may be evaluated in this article, or claim that may be made by its manufacturer, is not guaranteed or endorsed by the publisher.
Acknowledgments
The authors wish to thank the participants who took part in this study. A particular thank you to the staff at the Department of Radiology, University Hospital of Haukeland, Bergen, for their invaluable help with scanning the participants.
Supplementary Material
The Supplementary Material for this article can be found online at: https://www.frontiersin.org/articles/10.3389/fnins.2021.674050/full#supplementary-material
Supplementary Figure 1 | Visualization of findings in Supplementary Table 1.
Supplementary Table 1 | Omission Position 1 > Position 2 across rhythms. Results reported as t-tests uncorrected at p < 0.001, cluster size of 100 voxels. ACC, anterior cingulate cortex; MPFC, medial prefrontal cortex.
References
Ahveninen, J., Seidman, L. J., Chang, W. T., Hamalainen, M., and Huang, S. (2017). Suppression of irrelevant sounds during auditory working memory. Neuroimage 161, 1–8. doi: 10.1016/j.neuroimage.2017.08.040
Arnott, S. R., Grady, C. L., Hevenor, S. J., Graham, S., and Alain, C. (2005). The functional organization of auditory working memory as revealed by fMRI. J. Cogn. Neurosci. 17, 819–831. doi: 10.1162/0898929053747612
Auksztulewicz, R., and Friston, K. (2016). Repetition suppression and its contextual determinants in predictive coding. Cortex 80, 125–140. doi: 10.1016/j.cortex.2015.11.024
Baldeweg, T. (2006). Repetition effects to sounds: evidence for predictive coding in the auditory system. Trends Cogn. Sci. 10, 93–94. doi: 10.1016/j.tics.2006.01.010
Bengtsson, S. L., Ullen, F., Ehrsson, H. H., Hashimoto, T., Kito, T., Naito, E., et al. (2009). Listening to rhythms activates motor and premotor cortices. Cortex 45, 62–71. doi: 10.1016/j.cortex.2008.07.002
Bouwer, F. L., Van Zuijen, T. L., and Honing, H. (2014). Beat processing is pre-attentive for metrically simple rhythms with clear accents: an ERP study. PLoS One 9:e97467. doi: 10.1371/journal.pone.0097467
Brechmann, A., Gaschler-Markefski, B., Sohr, M., Yoneda, K., Kaulisch, T., and Scheich, H. (2007). Working memory–specific activity in auditory cortex: potential correlates of sequential processing and maintenance. Cereb. Cortex 17, 2544–2552. doi: 10.1093/cercor/bhl160
Cacciaglia, R., Costa-Faidella, J., Zarnowiec, K., Grimm, S., and Escera, C. (2019). Auditory predictions shape the neural responses to stimulus repetition and sensory change. Neuroimage 186, 200–210. doi: 10.1016/j.neuroimage.2018.11.007
Chapin, H. L., Zanto, T., Jantzen, K. J., Kelso, S. J., Steinberg, F., and Large, E. W. (2010). Neural responses to complex auditory rhythms: the role of attending. Front. Psychol. 1:224. doi: 10.3389/fpsyg.2010.00224
Chen, J. L., Penhune, V. B., and Zatorre, R. J. (2008b). Moving on time: brain network for auditory-motor synchronization is modulated by rhythm complexity and musical training. J. Cogn. Neurosci. 20, 226–239. doi: 10.1162/jocn.2008.20018
Chen, J. L., Penhune, V. B., and Zatorre, R. J. (2008a). Listening to musical rhythms recruits motor regions of the brain. Cereb. Cortex 18, 2844–2854. doi: 10.1093/cercor/bhn042
Clark, A. (2013). Whatever next? Predictive brains, situated agents, and the future of cognitive science. Behav. Brain Sci. 36, 181–204. doi: 10.1017/s0140525x12000477
Costa-Faidella, J., Baldeweg, T., Grimm, S., and Escera, C. (2011). Interactions between “What” and “When” in the auditory system: temporal predictability enhances repetition suppression. J. Neurosci. 31, 18590–18597. doi: 10.1523/jneurosci.2599-11.2011
Damsma, A., and van Rijn, H. (2016). Pupillary response indexes the metrical hierarchy of unattended rhythmic violations. Brain Cogn. 111, 95–103. doi: 10.1016/j.bandc.2016.10.004
D’Esposito, M., and Postle, B. R. (2015). The cognitive neuroscience of working memory. Annu. Rev. Psychol. 66, 115–142.
Doeller, C. F., Opitz, B., Mecklinger, A., Krick, C., Reith, W., and Schroger, E. (2003). Prefrontal cortex involvement in preattentive auditory deviance detection: neuroimaging and electrophysiological evidence. Neuroimage 20, 1270–1282. doi: 10.1016/s1053-8119(03)00389-6
Eichele, T., Calhoun, V. D., Moosmann, M., Specht, K., Jongsma, M. L., Quiroga, R. Q., et al. (2008). Unmixing concurrent EEG-fMRI with parallel independent component analysis. Int. J. Psychophysiol. 67, 222–234. doi: 10.1016/j.ijpsycho.2007.04.010
Friston, K. (2005). A theory of cortical responses. Philos. Trans. R. Soc. Lond. B Biol. Sci. 360, 815–836.
Geiser, E., Notter, M., and Gabrieli, J. D. (2012). A corticostriatal neural system enhances auditory perception through temporal context processing. J. Neurosci. 32, 6177–6182. doi: 10.1523/jneurosci.5153-11.2012
Geiser, E., Ziegler, E., Jancke, L., and Meyer, M. (2009). Early electrophysiological correlates of meter and rhythm processing in music perception. Cortex 45, 93–102. doi: 10.1016/j.cortex.2007.09.010
Grahn, J. A. (2009). “The role of the basal ganglia in beat perception neuroimaging and neuropsychological investigations,” in Neurosciences and Music Iii: Disorders and Plasticity, eds S. DallaBella, N. Kraus, K. Overy, C. Pantev, J. S. Snyder, M. Tervaniemi, et al. (New York, NY: Annals of the New York Academy of Sciences) 35–45. doi: 10.1111/j.1749-6632.2009.04553.x
Grahn, J. A., and Brett, M. (2007). Rhythm and beat perception in motor areas of the brain. J. Cogn. Neurosci. 19, 893–906. doi: 10.1162/jocn.2007.19.5.893
Grill-Spector, K., Henson, R., and Martin, A. (2006). Repetition and the brain: neural models of stimulus-specific effects. Trends Cogn. Sci. 10, 14–23. doi: 10.1016/j.tics.2005.11.006
Grotheer, M., and Kovacs, G. (2016). Can predictive coding explain repetition suppression? Cortex 80, 113–124. doi: 10.1016/j.cortex.2015.11.027
Heard, M., and Lee, Y. S. (2020). Shared neural resources of rhythm and syntax: an ALE meta-analysis. Neuropsychologia 137:107284. doi: 10.1016/j.neuropsychologia.2019.107284
Huang, Y., Matysiak, A., Heil, P., König, R., and Brosch, M. (2016). Persistent neural activity in auditory cortex is related to auditory working memory in humans and nonhuman primates. eLife 5:e15441.
Jerde, T. A., Childs, S. K., Handy, S. T., Nagode, J. C., and Pardo, J. V. (2011). Dissociable systems of working memory for rhythm and melody. Neuroimage 57, 1572–1579. doi: 10.1016/j.neuroimage.2011.05.061
Jongsma, M. L., Desain, P., Honing, H., and van Rijn, C. M. (2003). Evoked potentials to test rhythm perception theories. Ann. N. Y. Acad. Sci. 999, 180–183. doi: 10.1196/annals.1284.025
Jongsma, M. L., Eichele, T., Quian Quiroga, R., Jenks, K. M., Desain, P., Honing, H., et al. (2005). Expectancy effects on omission evoked potentials in musicians and non-musicians. Psychophysiology 42, 191–201. doi: 10.1111/j.1469-8986.2005.00269.x
Jongsma, M. L., Eichele, T., Van Rijn, C. M., Coenen, A. M., Hugdahl, K., Nordby, H., et al. (2006). Tracking pattern learning with single-trial event-related potentials. Clin. Neurophysiol. 117, 1957–1973. doi: 10.1016/j.clinph.2006.05.012
Koelsch, S., Vuust, P., and Friston, K. (2019). Predictive processes and the peculiar case of music. Trends Cogn. Sci. 23, 63–77. doi: 10.1016/j.tics.2018.10.006
Kompus, K., Westerhausen, R., Craven, A. R., Kreegipuu, K., Põldver, N., Passow, S., et al. (2015). Resting-state glutamatergic neurotransmission is related to the peak latency of the auditory mismatch negativity (MMN) for duration deviants: an 1H-MRS-EEG study. Psychophysiology 52, 1131–1139. doi: 10.1111/psyp.12445
Kovács, G., and Schweinberger, S. R. (2016). Repetition suppression-An integrative view. Cortex 80, 1–4. doi: 10.1016/j.cortex.2016.04.022
Kumar, S., Joseph, S., Gander, P. E., Barascud, N., Halpern, A. R., Griffiths, T. D., et al. (2016). A brain system for auditory working memory. J. Neurosci. 36, 4492–4505.
Ladinig, O., Honing, H., Haden, G., and Winkler, I. (2009). Probing attentive and preattentive emergent meter in adult listeners without extensive music training. Music Percept. 26, 377–386. doi: 10.1525/mp.2009.26.4.377
Large, E. W., Herrera, J. A., and Velasco, M. J. (2015). Neural networks for beat perception in musical rhythm. Front. Syst. Neurosci. 9:159. doi: 10.3389/fnsys.2015.00159
Liebenthal, E., Ellingson, M. L., Spanaki, M. V., Prieto, T. E., Ropella, K. M., and Binder, J. R. (2003). Simultaneous ERP and fMRI of the auditory cortex in a passive oddball paradigm. Neuroimage 19, 1395–1404. doi: 10.1016/s1053-8119(03)00228-3
Linke, A. C., Vicente-Grabovetsky, A., and Cusack, R. (2011). Stimulus-specific suppression preserves information in auditory short-term memory. Proc. Natl. Acad. Sci. U.S.A. 108, 12961–12966. doi: 10.1073/pnas.1102118108
Mathiak, K., Rapp, A., Kircher, T. T. J., Grodd, W., Hertrich, I., Weiskopf, N., et al. (2002). Mismatch responses to randomized gradient switching noise as reflected by fMRI and whole-head magnetoencephalography. Hum. Brain Mapp. 16, 190–195. doi: 10.1002/hbm.10041
Menon, V. (2011). Large-scale brain networks and psychopathology: a unifying triple network model. Trends Cogn. Sci. 15, 483–506. doi: 10.1016/j.tics.2011.08.003
Mustovic, H., Scheffler, K., Di Salle, F., Esposito, F., Neuhoff, J. G., Hennig, J., et al. (2003). Temporal integration of sequential auditory events: silent period in sound pattern activates human planum temporale. Neuroimage 20, 429–434. doi: 10.1016/s1053-8119(03)00293-3
Myers, N. E., Stokes, M. G., and Nobre, A. C. (2017). Prioritizing information during working memory: beyond sustained internal attention. Trends Cogn. Sci. 21, 449–461. doi: 10.1016/j.tics.2017.03.010
Naatanen, R., Gaillard, A. W., and Mantysalo, S. (1978). Early selective-attention effect on evoked potential reinterpreted. Acta Psychol. (Amst.) 42, 313–329. doi: 10.1016/0001-6918(78)90006-9
Nazimek, J. M., Hunter, M. D., Hoskin, R., Wilkinson, I., and Woodruff, P. W. (2013). Neural basis of auditory expectation within temporal cortex. Neuropsychologia 51, 2245–2250. doi: 10.1016/j.neuropsychologia.2013.07.019
Nees, M. A. (2016). Have we forgotten auditory sensory memory? Retention intervals in studies of nonverbal auditory working memory. Front. Psychol. 7:1892. doi: 10.3389/fpsyg.2016.01892
Ono, K., Altmann, C. F., Matsuhashi, M., Mima, T., and Fukuyama, H. (2015). Neural correlates of perceptual grouping effects in the processing of sound omission by musicians and nonmusicians. Hear. Res. 319, 25–31. doi: 10.1016/j.heares.2014.10.013
Ono, K., Matsuhashi, M., Mima, T., Fukuyama, H., and Altmann, C. F. (2013). Effects of regularity on the processing of sound omission in a tone sequence in musicians and non-musicians. Eur. J. Neurosci. 38, 2786–2792. doi: 10.1111/ejn.12254
Opitz, B., Rinne, T., Mecklinger, A., von Cramon, D. Y., and Schroger, E. (2002). Differential contribution of frontal and temporal cortices to auditory change detection: fMRI and ERP results. Neuroimage 15, 167–174. doi: 10.1006/nimg.2001.0970
Peretz, I., and Zatorre, R. J. (2005). Brain organization for music processing. Annu. Rev. Psychol. 56, 89–114. doi: 10.1146/annurev.psych.56.091103.070225
Raij, T., McEvoy, L., Mäkelä, J. P., and Hari, R. (1997). Human auditory cortex is activated by omissions of auditory stimuli. Brain Res. 745, 134–143. doi: 10.1016/s0006-8993(96)01140-7
Recasens, M., Grimm, S., Capilla, A., Nowak, R., and Escera, C. (2014). Two sequential processes of change detection in hierarchically ordered areas of the human auditory cortex. Cereb. Cortex 24, 143–153. doi: 10.1093/cercor/bhs295
Rinne, T., Degerman, A., and Alho, K. (2005). Superior temporal and inferior frontal cortices are activated by infrequent sound duration decrements: an fMRI study. Neuroimage 26, 66–72. doi: 10.1016/j.neuroimage.2005.01.017
Salisbury, D. F. (2012). Finding the missing stimulus mismatch negativity (MMN): emitted MMN to violations of an auditory gestalt. Psychophysiology 49, 544–548. doi: 10.1111/j.1469-8986.2011.01336.x
SanMiguel, I., Saupe, K., and Schroger, E. (2013a). I know what is missing here: electrophysiological prediction error signals elicited by omissions of predicted “what” but not “when”. Front. Hum. Neurosci. 7:403. doi: 10.3389/fnhum.2013.00407
SanMiguel, I., Widmann, A., Bendixen, A., Trujillo-Barreto, N., and Schröger, E. (2013b). Hearing silences: human auditory processing relies on preactivation of sound-specific brain activity patterns. J. Neurosci. 33, 8633–8639. doi: 10.1523/jneurosci.5821-12.2013
Specht, K., and Reul, J. (2003). Functional segregation of the temporal lobes into highly differentiated subsystems for auditory perception: an auditory rapid event-related fMRI-task. Neuroimage 20, 1944–1954. doi: 10.1016/j.neuroimage.2003.07.034
Sreenivasan, K. K., Curtis, C. E., and D’Esposito, M. (2014). Revisiting the role of persistent neural activity during working memory. Trends Cogn. Sci. 18, 82–89. doi: 10.1016/j.tics.2013.12.001
Sreenivasan, K. K., and D’Esposito, M. (2019). The what, where and how of delay activity. Nat. Rev. Neurosci. 20, 466–481. doi: 10.1038/s41583-019-0176-7
Summerfield, C., Trittschuh, E. H., Monti, J. M., Mesulam, M. M., and Egner, T. (2008). Neural repetition suppression reflects fulfilled perceptual expectations. Nat. Neurosci. 11, 1004–1006. doi: 10.1038/nn.2163
Teki, S., Grube, M., Kumar, S., and Griffiths, T. D. (2011). Distinct neural substrates of duration-based and beat-based auditory timing. J. Neurosci. 31, 3805–3812. doi: 10.1523/jneurosci.5561-10.2011
Tervaniemi, M., Schroger, E., Saher, M., and Naatanen, R. (2000). Effects of spectral complexity and sound duration on automatic complex-sound pitch processing in humans - a mismatch negativity study. Neurosci. Lett. 290, 66–70. doi: 10.1016/s0304-3940(00)01290-8
Thaut, M. H., Trimarchi, P. D., and Parsons, L. M. (2014). Human brain basis of musical rhythm perception: common and distinct neural substrates for meter, tempo, and pattern. Brain Sci. 4, 428–452. doi: 10.3390/brainsci4020428
Todorovic, A., van Ede, F., Maris, E., and de Lange, F. P. (2011). Prior expectation mediates neural adaptation to repeated sounds in the auditory cortex: an MEG study. J. Neurosci. 31, 9118–9123. doi: 10.1523/jneurosci.1425-11.2011
Toiviainen, P., Burunat, I., Brattico, E., Vuust, P., and Alluri, V. (2020). The chronnectome of musical beat. Neuroimage 216:116191. doi: 10.1016/j.neuroimage.2019.116191
Trost, W., Fruhholz, S., Schon, D., Labbe, C., Pichon, S., Grandjean, D., et al. (2014). Getting the beat: entrainment of brain activity by musical rhythm and pleasantness. Neuroimage 103, 55–64. doi: 10.1016/j.neuroimage.2014.09.009
Vikene, K., Skeie, G. O., and Specht, K. (2018). Abnormal phasic activity in saliency network, motor areas, and basal ganglia in Parkinson’s disease during rhythm perception. Hum. Brain Mapp. 40, 916–927. doi: 10.1002/hbm.24421
Vikene, K., Skeie, G. O., and Specht, K. (2019). Compensatory task-specific hypersensitivity in bilateral planum temporale and right superior temporal gyrus during auditory rhythm and omission processing in Parkinson’s disease. Sci. Rep. 9:12623.
Voisin, J., Bidet-Caulet, A., Bertrand, O., and Fonlupt, P. (2006). Listening in silence activates auditory areas: a functional magnetic resonance imaging study. J. Neurosci. 26, 273–278. doi: 10.1523/jneurosci.2967-05.2006
Vuust, P., and Witek, M. A. (2014). Rhythmic complexity and predictive coding: a novel approach to modeling rhythm and meter perception in music. Front. Psychol. 5:1111. doi: 10.3389/fpsyg.2014.01111
Wacongne, C., Labyt, E., van Wassenhove, V., Bekinschtein, T., Naccache, L., and Dehaene, S. (2011). Evidence for a hierarchy of predictions and prediction errors in human cortex. Proc. Natl. Acad. Sci. U.S.A. 108, 20754–20759. doi: 10.1073/pnas.1117807108
Winkler, I., and Schroger, E. (1995). Neural representation for the temporal structure of sound patterns. Neuroreport 6, 690–694. doi: 10.1097/00001756-199503000-00026
Zatorre, R. J., Evans, A. C., and Meyer, E. (1994). Neural mechanisms underlying melodic perception and memory for pitch. J. Neurosci. 14, 1908–1919. doi: 10.1523/jneurosci.14-04-01908.1994
Keywords: musical rhythm and beat processing, temporal cortex, predicitve maintenance, supression, surprisal
Citation: Færøvik U, Specht K and Vikene K (2021) Suppression, Maintenance, and Surprise: Neuronal Correlates of Predictive Processing Specialization for Musical Rhythm. Front. Neurosci. 15:674050. doi: 10.3389/fnins.2021.674050
Received: 28 February 2021; Accepted: 17 June 2021;
Published: 27 August 2021.
Edited by:
Thomas James Lundy, Cuttlefish Arts, United StatesReviewed by:
Daya Shankar Gupta, Camden County College, United StatesLu Zhang, Georgia Institute of Technology, United States
Copyright © 2021 Færøvik, Specht and Vikene. This is an open-access article distributed under the terms of the Creative Commons Attribution License (CC BY). The use, distribution or reproduction in other forums is permitted, provided the original author(s) and the copyright owner(s) are credited and that the original publication in this journal is cited, in accordance with accepted academic practice. No use, distribution or reproduction is permitted which does not comply with these terms.
*Correspondence: Kjetil Vikene, a2pldGlsLnZpa2VuZUB1aWIubm8=