- 1Medication Development Program, Molecular Targets and Medication Discovery Branch, National Institute on Drug Abuse, Intramural Research Program, National Institutes of Health, Baltimore, MD, United States
- 2Clinical Psychoneuroendo- crinology and Neuropsychopharmacology Section, Translational Addiction Medicine Branch, National Institute on Drug Abuse Intramural Research Program, National Institutes of Health, Baltimore, MD, United States
- 3National Institute on Alcohol Abuse and Alcoholism, Division of Intramural Clinical and Biological Research, National Institutes of Health, Bethesda, MD, United States
The number of individuals affected by psychostimulant use disorder (PSUD) has increased rapidly over the last few decades resulting in economic, emotional, and physical burdens on our society. Further compounding this issue is the current lack of clinically approved medications to treat this disorder. The dopamine transporter (DAT) is a common target of psychostimulant actions related to their use and dependence, and the recent availability of atypical DAT inhibitors as a potential therapeutic option has garnered popularity in this research field. Modafinil (MOD), which is approved for clinical use for the treatment of narcolepsy and sleep disorders, blocks DAT just like commonly abused psychostimulants. However, preclinical and clinical studies have shown that it lacks the addictive properties (in both behavioral and neurochemical studies) associated with other abused DAT inhibitors. Clinical availability of MOD has facilitated its off-label use for several psychiatric disorders related to alteration of brain dopamine (DA) systems, including PSUD. In this review, we highlight clinical and preclinical research on MOD and its R-enantiomer, R-MOD, as potential medications for PSUD. Given the complexity of PSUD, we have also reported the effects of MOD on psychostimulant-induced appearance of several symptoms that could intensify the severity of the disease (i.e., sleep disorders and impairment of cognitive functions), besides the potential therapeutic effects of MOD on PSUD.
Introduction
Psychostimulant use disorder is a complex disease defined by DSM-5 which includes both former (DSM-IV) diagnoses of abuse and dependence on a psychostimulant, such as cocaine or amphetamines. While illicit drugs have long been a societal concern, drug use rates have been growing in recent years. Globally, stimulants such as cocaine and amphetamines are used by approximately 0.35–0.4% and 0.7–0.77% of the population, respectively (Peacock et al., 2018; Farrell et al., 2019). Of these subpopulations, 16% are dependent on cocaine, while 11% are dependent on amphetamines (Farrell et al., 2019). In the United States, it was estimated that about 5.5 million people age 12 and older used cocaine in 2018 (2% of the United States population) (SAMHSA, 2018) and 1.9 million people age 12 and older used METH in 2018 (0.7% of the United States population) (SAMHSA, 2018). A major issue with substance use disorders is the risk of overdose. Recent data show that between 2012 and 2018, drug overdoses involving cocaine more than tripled, and drug overdoses involving abused psychostimulants increased nearly five-fold (Hedegaard et al., 2020).
Classically, the neurobiology underlying PSUD has focused on the neurotransmitter dopamine (DA) for its role in reward processing (Wise and Rompre, 1989; Wise, 2008; Arias-Carrión et al., 2010; Taber et al., 2012). Indeed, commonly abused stimulants exert effects on brain DA levels through their interactions with the neuronal membrane DAT (Das, 1993; Nestler, 2005). Increased DA levels after psychostimulant administration lead to arousal and euphoria, which facilitate the transition from the initial recreational use to continued excessive use, and parallel the potential clinical development of addiction in patients with the most severe form of the disorder (Compton et al., 2018).
The clinical severity of PSUD can be often worsened by medical and mental health comorbidities, e.g., mood and sleep disorders (Mahfoud et al., 2009; Gould, 2010; Torrens and Rossi, 2015). Furthermore, PSUD may be associated with cognitive impairment, which in turn lead to higher treatment dropout rates (Sofuoglu et al., 2013, 2016; Nuijten et al., 2016). These indicate a potential treatment avenue to ameliorate some of the effects of PSUD, which may contribute to increased abstinence rates overall.
Treatment of PSUD relies primarily on behavioral remedies, which may include 12-step facilitation, contingency management, relapse prevention, motivational enhancement therapy, and CBT (for a review, see: Vocci and Montoya, 2009). However, these approaches are time- and resource-intensive and their effect sizes are sub-optimal: integration with effective pharmacotherapies would be likely to improve outcomes and success rates. However, to date there are no approved pharmacologic treatments for PSUD (Phillips et al., 2014). Medications such as antidepressants, DA agonists/partial agonists, mood stabilizers, neuro-protectives, and agonist-like replacement therapy (de Lima et al., 2003; Elkashef et al., 2005; Diana, 2011; Phillips et al., 2014; Jordan et al., 2019) have all been tested with minimal success. The lack of pharmacological treatments for PSUD is a driving force for research toward the development of novel medications.
Among the potential pharmacotherapeutic options for PSUD is MOD, a clinically available medication that inhibits the uptake of DA by blocking DAT (Mignot et al., 1994; Loland et al., 2012). This pharmacological effect is shared with abused psychostimulants but, in spite of that, MOD shows behavioral and neurochemical actions that suggest limited, if any, potential for misuse (Jasinski, 2000; Deroche-Gamonet et al., 2002; Myrick et al., 2004; Food and Drug Administration, 2007; Vosburg et al., 2010; Mereu et al., 2020). Currently, this agent is prescribed for its wake-promoting effects (Czeisler et al., 2005; Kumar, 2008), consistent with its approval for narcolepsy, shift work sleep disorder, and obstructive sleep apnea/hypopnea syndrome. Off-label, MOD has been used for its pro-cognitive effects, especially in patients with cognitive impairment associated with psychiatric disorders (Peñaloza et al., 2013; Turner et al., 2014). During the last two decades, MOD has been tested as a potential medication to treat some of the primary (dependence) and secondary (cognitive and sleep disorders) symptoms of PSUD, representing a potential additional treatment option for selected populations affected by PSUD.
In this review, we will mainly focus on preclinical studies showing how MOD and R-MOD (its R-enantiomer) interact with DAT, the DAergic system, and the reinforcing actions of abused psychostimulants. We will also review clinical studies where MOD efficacy as a potential treatment for PSUD has been evaluated, including for related symptoms such as alteration of sleep and cognitive dysfunction.
Modafinil Pharmacology Related to PSUD
Modafinil [2-(Diphenylmethyl) sulfinyl acetamide; Alertec, Modavigil, Provigil] and its long-acting, enantiopure form, R-MOD (Nuvigil, Artvigil) (Wong et al., 1999), are clinically available and prescribed as wake-promoting agents for narcolepsy and sleep disorders (Bastoji and Jouvet, 1988; Broughton et al., 1997; US Modafinil in Narcolepsy Multicenter Study Group, 1998, 2000). Early evidence suggested that MOD had a weak, low μM affinity, but relatively good selectivity, for DAT (Mignot et al., 1994), confirmed by more recent studies (Madras et al., 2006; Loland et al., 2012). Thus, the main mechanism of action for MOD appears predominantly driven by actions at neural membrane DATs to stimulate catecholamine neurotransmission (Wisor et al., 2001; Madras et al., 2006). DAT knockout mice were used to confirm the importance of DAT in the mechanism of action of MOD, as studies have found that the pharmacological wake-promoting effects of MOD administration were abolished in those mutant mice (Wisor et al., 2001). Volkow et al. (2009) used PET to show that, after oral administration, MOD (200 to 400 mg) occupies and blocks DAT in the human brain (caudate, NAcc, and putamen). The latter effect was also shown for the enantiomer, R-MOD (Spencer et al., 2010). Further, as a result of the DAT inhibition induced by administration of MOD or R-MOD, increased brain DA levels can be observed in several dopaminergic nerve terminal regions (Ferraro et al., 1996c; Wisor et al., 2001; Volkow et al., 2009; Loland et al., 2012). Further, DAT trafficking could be affected by psychostimulants. Administration of DAT substrates like METH and amphetamine decreases the trafficking of DAT to the cell surface (Saunders et al., 2000; Zahniser and Sorkin, 2009), while DAT inhibitors like cocaine have been shown to increase DAT trafficking to the cell surface (Daws et al., 2002; Little et al., 2002; Zahniser and Sorkin, 2009). Although the effects of MOD administration on DAT trafficking have yet to be fully elucidated, it has been shown that MOD prevents METH-induced decreases in DAT immunoreactivity 6 days after treatment (Raineri et al., 2012).
Beyond DAT, MOD does not show significant affinity for other important pharmacological brain targets. For example, MOD affinity for the NET falls in the 100 μM range (Madras et al., 2006), and it is still unclear if the increases in brain NE levels induced by MOD are the result of its interaction with NET (see for review Mereu et al., 2013). These effects on brain NE levels in PFC and rostro-medial hypothalamus (de Saint Hilaire et al., 2001) could be of interest due to a well-documented role for NE in wakefulness and arousal (reviewed in Mitchell and Weinshenker, 2010). Interestingly, MOD did not show direct activity on trace amine-associated receptor 1 (TAAR1) (Madras et al., 2006), in contrast to amphetamines (Xie and Miller, 2009; Liu et al., 2020). MOD has been shown to have indirect actions on TAAR1 through activation of DAT, which can augment TAAR1 activation (Madras et al., 2006). TAAR1 has been implicated in wakefulness, which represents a predictable effect given the receptor’s ability to modulate the activity of other monoamine systems (Revel et al., 2013; Liu et al., 2020). In a recent report, deletion of TAAR1 receptor in mice did not produce substantial effects on MOD-induced wakefulness as compared to WT mice (Schwartz et al., 2018). In the same report, reductions in MOD-induced gamma-band activity in EEG studies in TAAR1 KO mice were found, and the authors suggest that TAAR1 may regulate neurophysiological factors related cortical and cognitive functions (Schwartz et al., 2018).
Regardless of its affinity for pharmacological targets, MOD has been reported to affect the levels of several neurotransmitters. MOD stimulates brain glutamate levels in the hypothalamus (medial preoptic area and posterior hypothalamus), thalamus (ventromedial and ventrolateral regions), and hippocampus (Ferraro et al., 1997b, 1999), and it has been shown to decrease the levels of GABA in the NAcc, hypothalamus (medial preoptic area and posterior hypothalamus), striatal, and pallidal regions (Ferraro et al., 1996b, 1997a, 1999). MOD induced stimulation in brain serotonin levels in the PFC (Ferraro et al., 2000; de Saint Hilaire et al., 2001), increases in histamine levels and/or activation in the tuberomammillary nucleus and the anterior hypothalamus (Scammell et al., 2000; Ishizuka et al., 2003, 2008), and limited activation of orexin/hypocretin neurons in the perifornical areas and lateral hypothalamus (Chemelli et al., 1999; Scammell et al., 2000; Willie et al., 2005) has also been observed (reviewed in Kumar, 2008; Minzenberg and Carter, 2008; Mereu et al., 2013).
In addition to its effects on neurotransmitter levels, MOD administration affects the induction and inhibition of hepatic cytochrome P450 isoenzymes (Robertson et al., 2000). In vitro, MOD competitively inhibits CYP2C19 and suppresses CYP2C9, as well as moderately induces CYP1A2, CYP3A4, and CYP2B6 (Robertson et al., 2000). Pharmacokinetic studies in vivo with warfarin and ethinylestradiol, which react with CYP2C9 and CYP3A4 respectively, have not shown the same magnitude of effect as in vitro studies (Robertson and Hellriegel, 2003). Through MOD’s induction and inhibition of the P450 isoenzymes, MOD co-administration may decrease or prolong plasma concentrations of other drugs metabolized through these enzymes (Schwartz, 2005). There have been clinical reports of MOD interactions with medications, for example, cyclosporine and clomipramine. Specifically, the immunosuppressive effect of cyclosporine decreased after 200 mg/day MOD, which appeared to be from CYP3A4 induction (for a review, see e.g., Robertson and Hellriegel, 2003). A patient treated with clomipramine was found to lack functional CYP2D6, and the ancillary CYP2C19 pathways inhibited by MOD contributed to increased clomipramine levels in the blood (Robertson and Hellriegel, 2003). MOD also has notable effects as a facilitator of electrotonic coupling in neurons and astroglia through actions at gap junctions (Garcia-Rill et al., 2007; Urbano et al., 2007; Liu et al., 2013; Duchêne et al., 2016; Mereu et al., 2020). In particular, it has been shown that the gap junction inhibitor carbenoxolone blunted the ability of MOD to potentiate self-administration of cocaine in rats (Mereu et al., 2020). These properties are likely important for the agent’s pharmacological actions, as well as interactions with other drugs and biomolecules.
Modafinil, DAT Inhibition, and Potential Abuse Liability
As a result of inhibition of DAT, it is not surprising that MOD activities could overlap with some of those observed after administration of commonly abused psychostimulants. However, as reported in Table 1, some of its actions seem directed to improve specific symptoms observed in patients with a PSUD diagnosis, i.e., impairments in cognition, sleep, cardiovascular function, and mood disturbances, as well as elevated neuroinflammation. Moreover, MOD fails to display the abuse potential (Jasinski, 2000; Deroche-Gamonet et al., 2002; Myrick et al., 2004; Food and Drug Administration, 2007; Vosburg et al., 2010) or the withdrawal symptoms (Hermant et al., 1991; Myrick et al., 2004) observed with typical psychostimulants. Indeed, to our knowledge, only a very few anecdotical reports of MOD abuse and dependence have been reported in the literature (Kate et al., 2012; Ozturk and Deveci, 2014; Krishnan and Chary, 2015) despite the climbing rates of its non-medical use as a cognitive enhancer in schools and at the workplace (Sharif et al., 2021). Further, important behavioral and neurochemical differences between MOD, or R-MOD, and typical abused psychostimulants have been found in preclinical studies, suggesting they have a unique pharmacological, psychostimulant profile. Taken together, these actions highlight the potential for MOD to reduce the harm associated with the complexity of the symptoms in PSUD. In the next sections, we will briefly highlight some of the pharmacological actions of MOD, i.e., increased wakefulness, improved cognition and cardiovascular function, that could play a potential role in its therapeutic activity against PSUD (summarized in Table 1).
Modafinil Interactions With Sleep and Wakefulness Activities
Modafinil was introduced as a wake-promoting agent in the 1990s and approved by the US FDA to treat excessive sleeping (narcolepsy, shift work sleep disorder, obstructive sleep apnea/hypopnea syndrome) (Bastoji and Jouvet, 1988; Broughton et al., 1997; US Modafinil in Narcolepsy Multicenter Study Group, 1998, 2000). In addition to its approved uses, MOD is often used off-label for the treatment of fatigue symptoms in neurological, psychiatric, and excessive fatigue disorders (reviewed in Kumar, 2008). Like amphetamines, the molecular mechanism by which MOD imposes wakefulness has been largely debated. The mechanism is suspected to be linked to the inhibition of monoamine transport by plasma membrane transporters like DAT. In particular, studies have shown that DAT and DA regulation in key areas involved in wakefulness/sleep (i.e., medial preoptic area and posterior hypothalamus) is crucial in the wake-promoting effects of amphetamines and MOD (Jones et al., 1977; Nishino and Mignot, 1997; Nishino et al., 1998; Wisor et al., 2001). Further, researchers have shown that DA activity fluctuates with arousal state (Trulson, 1985). MOD’s effects on other neurotransmitters are also suspected to play a role in the wakefulness-promoting properties of this agent (Boutrel and Koob, 2004). For example, changes in brain NE neurotransmission have been suggested to play a role in wakefulness and arousal (reviewed in Mitchell and Weinshenker, 2010), and MOD stimulation of brain NE levels in PFC and rostro-medial hypothalamus (de Saint Hilaire et al., 2001) could be related to its effect on sleep disturbances. While it is still unclear if NET blockade is the mechanism of action related to MOD stimulation of brain NE levels, some studies have suggested that NET inhibition is less efficacious in the promotion of wakefulness than DAT inhibition (Nishino and Mignot, 1997; Nishino et al., 1998). It is of interest to note that MOD, as highlighted above, interacts with other key neurotransmitters and systems that could play a role in the regulation of sleep, including glutamate, GABA, serotonin, histamine, and orexin/hypocretin (reviewed in Monti, 2013). There is also evidence that MOD increases glutamine synthetase in the rat brain, an enzyme that converts glutamate to glutamine for storage, which may be important for the wakefulness effects of MOD (Touret et al., 1994). The orexin system has a well-established role in sleep-wake regulation (Espana et al., 2001; Sakurai, 2007). MOD administration increases the expression of c-Fos (a marker of neuronal activation) in orexin neurons in the hypothalamus (Chemelli et al., 1999; Scammell et al., 2000). Orexin neuronal projections can activate histamine release in the hypothalamus as well (Huang et al., 2001; Ishizuka et al., 2002). Histamine also has a well-documented role in regulating sleep-wake cycle (Haas et al., 2008). Interestingly, MOD administration produced activation of histaminergic cells (Scammell et al., 2000) but only in the presence of an intact orexin system (Ishizuka et al., 2010). Further, decreases in histamine or loss of histamine neurons blunted MOD-induced increases in locomotion (Ishizuka et al., 2008) as well as the drug’s wake-promoting actions (Yu et al., 2019). On the other hand, orexin-null mice displayed heightened wakefulness following MOD administration compared to wild-type mice (Willie et al., 2005). These findings suggest impaired regulation of the arousal system following removal of orexin, but they also suggest that orexin is not necessarily required for MOD’s wake-promoting actions, or that in those mice possible neuronal adaptations would substitute for removal of orexin.
Modafinil Interactions With Cognitive Functions
Enhancements in cognitive functions have been reported following MOD administration in rodents and humans (Turner et al., 2003; Minzenberg and Carter, 2008; Cope et al., 2017). MOD produces dose-dependent improvements in working memory (Béracochéa et al., 2001; Ward et al., 2004; Piérard et al., 2006), speed of learning (Béracochéa et al., 2002, 2003; Ward et al., 2004), and sustained attention (Morgan et al., 2007) in animals. In humans, MOD produces similar effects, improving memory and attention (reviewed in Minzenberg and Carter, 2008). Importantly, MOD heightens attention independently of its effects on wakefulness/arousal (Cope et al., 2017). MOD administration elicits changes in activation of brain regions associated with cognition, including the hippocampus (Ferraro et al., 1997b; Shuman et al., 2009; Brandt et al., 2014; Yan et al., 2015) and the PFC (Müller et al., 2004; González et al., 2014, 2018). Thus, improvements in cognitive functions associated with MOD’s actions on the dopaminergic system may underlie those specific changes in DA transmission (reviewed in Minzenberg and Carter, 2008; Mereu et al., 2013), for example in the PFC, that have been recognized for their role in working memory (Sawaguchi and Goldman-Rakic, 1991, 1994). Further, DA receptors can be found on glutamatergic pyramidal cells (Tseng and O’Donnell, 2004) and GABAergic neurons (Tseng and O’Donnell, 2007) in the PFC where they can gate glutamatergic and GABAergic transmission linked to cognition (reviewed in Minzenberg and Carter, 2008). MOD’s effects on brain NE may also affect cognition due to NE’s established role in modulation of cognitive function (reviewed in Chamberlain and Robbins, 2013). Stimulation of NE neurotransmission following MOD administration is also implicated in cognition (Minzenberg and Carter, 2008), while MOD actions on the acetylcholine system have been shown to have effects on learning and memory (reviewed in Mereu et al., 2013). It has also been shown that MOD produced increased motivation, likely by activating D1 receptors (Young and Geyer, 2010). Importantly, MOD is an appealing candidate to target cognitive dysfunction associated with ADHD and psychiatric disorders (Ballon and Feifel, 2006), as well as PSUD. Specifically, treatment with MOD has been shown to improve cognition in PSUD patients (Dean et al., 2011) (see also the “Human studies” sections below).
The pro-cognitive effects of MOD have stimulated a debate about an ethical dilemma and potential concern regarding its rapidly increasing off-label, non-medical use in healthy individuals to improve attention, focus, memory, and cognitive functions (Cakic, 2009; Sahakian and Morein-Zamir, 2011; Peñaloza et al., 2013).
Modafinil/DAT Inhibition and Inflammation
Additional potential actions of MOD include the ability to act as an anti-inflammatory agent. Specifically, MOD has been shown to reduce neuroinflammation via suppressing inflammatory cytokines (Han et al., 2018), T-cell differentiation (Brandao et al., 2019), monocyte recruitment/activation (Zager et al., 2018), and activation of glial cells (Raineri et al., 2012). This MOD-induced immune activation may be essential for decreasing the neurotoxic and inflammatory consequences of many diseases including PSUD, an exceptionally important effect given that many stimulants are pro-inflammatory in nature. METH administration is marked by increases in TNF-α, IL-1β, and IL-6 expression, as well as elevated microglial activation (Cadet et al., 1994; Lai et al., 2009; Gonçalves et al., 2010). Cocaine has similarly been associated with increases in TNF-α, IL-6, IL-8, activator protein 1 (AP-1), and nuclear factor kappa B (NFκB) (Zhang et al., 1998; Gan et al., 1999; Lee et al., 2001; Dhillon et al., 2008). Nicotine is marked by increases in TNF-α, IL-18, IL-1β, and chemokines, including CCL2, CCL8, and CXC3CL1 (Bradford et al., 2011). Pro-inflammatory agents, such as stimulants, have also been associated with deterioration of the natural obstacle that protects the brain; the blood brain barrier, further magnifying their neurotoxic effects (Czub et al., 2001; Nath et al., 2002). MOD has been shown to counteract the toxic and neuroinflammatory effects of METH in mice (Raineri et al., 2012), but effects against other drugs of abuse have yet to be reported.
Modafinil administration has also been shown to exert effects on histamine, a common marker of inflammation and neurotransmitter involved in sleep/wakefulness (Haas et al., 2008). Using in vivo microdialysis, an increased histamine release in the anterior hypothalamus was observed following MOD administration (Ishizuka et al., 2003).
Preclinical Studies on MOD as a Pharmacotherapeutic Treatment for PSUD
Neurochemical Studies
In this section, we will review the neurochemistry of MOD as it relates to PSUD. The main pharmacologic activity of MOD is due to its affinity and inhibitory actions at DAT, which result in stimulation of brain extracellular DA levels. DAT and the DA system also play a major role in the abuse liability of psychostimulants. Thus, we will start this section with a brief background about DAT and DA roles in PSUD.
DA and DAT, Their Role in Drug Abuse, Dependence, and as Potential Targets for Pharmacotherapy of PSUD
Dopamine’s role in the brain’s reward circuit has been extensively studied (Wise and Rompre, 1989; Di Chiara et al., 1993a, 1998; Wise, 2008; Arias-Carrión et al., 2010; Taber et al., 2012), however its role in drug abuse and dependence is still not fully clarified (Volkow et al., 2011; Wise and Robble, 2020). Following acute administration of drugs of abuse, including central stimulants and depressants, opiates, cannabinoids, and cholinergic agonists, increased levels of extracellular DA have been reported in the brain regions that are the projection fields of dopaminergic neurons, specifically the NAcc and caudate (Di Chiara and Imperato, 1988; Koob, 1992; Pontieri et al., 1995, 1996; Tanda et al., 1997a; Di Chiara et al., 1999). Acute administration of psychostimulants, in particular, has been shown to increase DA levels in a dose dependent manner in the NAcc shell and core, and in the striatum (Di Chiara et al., 1993b; Pontieri et al., 1995; Tanda et al., 1997b). These effects are likely related to the initial positive experience of drug use that could also lead to acquisition of drug-seeking behaviors and to the desire to repeat behaviors that lead to a pleasurable experience (Pettit and Justice, 1989; Woolverton and Johnson, 1992; Koob et al., 1998), but do not account for all neurological aspects of substance use disorder (Salamone et al., 2003; Robinson and Kolb, 2004; Russo et al., 2009; Golden and Russo, 2012). Repeated drug use has been shown to cause synaptic changes, allowing for the development of a different regulation of neurotransmission and other neuronal activities, which is believed to be the driving force behind drug addiction (Thomas et al., 2008; Luscher and Malenka, 2011). Indeed, addictive drugs consistently elicit neurological changes that are indicative of potential targets for better understanding and treating the development of specific patterns of drug use and dependence.
Regulation of expression and trafficking of presynaptic DATs by synaptic DA levels has been proposed as a pharmacological target involved in the development of PSUD (Zahniser and Sorkin, 2004). Indeed, both acute and chronic cocaine exposure increases DAT density in the NAcc and DS (Zahniser and Sorkin, 2004), while other psychostimulants such as amphetamine and METH decrease DAT expression in the same regions (Saunders et al., 2000; Sandoval et al., 2001; Barr et al., 2006; Kahlig et al., 2006). Despite varying levels of transporter presence, a primary result of psychostimulant use is an increase in synaptic DA levels by inhibiting its presynaptic neuronal reuptake or by interacting with the VMAT2, releasing DA into the cytoplasm and then releasing DA into the synapse by reversing its transport direction through DAT (Sulzer et al., 2005; Xie and Miller, 2009; Calipari et al., 2013). The regulation of DAT expression allows the formation of a feedback loop between DAT abundance and psychostimulant presence in the brain (Verma, 2015). The resulting changes in DAT density after drug use perpetuates a need for consistent amounts of the drug to avoid withdrawal and to maintain significant levels of DA and DAT expression.
DA and DAT as Potential Pharmacologic Target for the Therapeutic Actions of MOD Against PSUD
The use of MOD as a therapeutic agent for PSUD is largely based on its mechanistic actions that, in part, overlap with those of other abused psychostimulants. For instance, abused psychostimulants increase mesolimbic extracellular DA, often by interacting with DAT (Mortensen and Amara, 2003; Zhu and Reith, 2008), and MOD has been shown to stimulate DA levels in the same dopaminergic areas related to psychostimulant actions (Ferraro et al., 1996c; Zolkowska et al., 2009; Loland et al., 2012; Rowley et al., 2014; Bobak et al., 2016; Mereu et al., 2020). Even though the pharmacological actions of MOD have been mainly explained by its affinity for DAT, its unique psychostimulant profile has been shown to differ from that of typical DAT inhibitors, as shown in behavioral, neurochemical and molecular pharmacology studies (Schmitt and Reith, 2011; Loland et al., 2012; Mereu et al., 2013, 2017, 2020). For example, MOD binding to DAT differs from that of other typical, cocaine-like, DAT blockers (Schmitt and Reith, 2011). In contrast to cocaine, MOD prefers to bind to, or stabilize the DAT protein in a more inward-facing occluded conformation (Schmitt and Reith, 2011; Loland et al., 2012) that still inhibits uptake and results in increases in extracellular DA in the NAcc (Ferraro et al., 1996c; Zolkowska et al., 2009), the NAcc shell (NAS) (Loland et al., 2012; Mereu et al., 2020), and the striatum (Rowley et al., 2014). MOD also increases electrically evoked DA in the DS and VS (Bobak et al., 2016) (summarized in Table 2) like abused psychostimulants (Nisell et al., 1994; Pontieri et al., 1996; Munzar et al., 2004; Kohut et al., 2014). However, while acute administration of MOD (Mereu et al., 2017, 2020) or its enantiomers (Loland et al., 2012; Keighron et al., 2019a, b) increases extracellular NAcc DA levels in rodents, these effects, even at very high doses, elicited a limited stimulation of DA in striatal areas compared to the stimulation elicited by abused psychostimulants (Loland et al., 2012; Mereu et al., 2017, 2020). This limited efficacy of MOD to increase DA levels, as compared to abused psychostimulants, also predicts a limited potential for abuse.
Cocaine psychostimulant actions and its abuse liability have been related to its ability to slow DA reuptake by inhibiting DAT and stimulating DA neurotransmission (Wise and Bozarth, 1987; Kuhar et al., 1991). It is interesting to note that administration of MOD (10–32 mg/kg, i.p.) prior to cocaine produced no further increase in extracellular NAS DA levels beyond that produced by cocaine alone (Mereu et al., 2020). This effect varied with the additive effects on DA levels obtained with combinations of cocaine and typical DAT blockers like methylphenidate or WIN 35,428 (Tanda et al., 2009; Mereu et al., 2020), but similar to the effects shown by combinations of cocaine and an atypical DAT blocker like JHW007 (Tanda et al., 2009), suggesting a potential atypical DAT inhibitor effect for MOD in these tests.
Another abused psychostimulant, METH, is transported into DA neurons and its nerve terminals as a DAT substrate, like DA, where it has also been shown to affect the VMAT2 function. As a consequence, decreased vesicular DA concentrations and increased cytoplasmic DA levels result, via reverse transport of DA through DAT (Kahlig and Galli, 2003; Sulzer et al., 2005; Howell and Kimmel, 2008), resulting in dramatic increases in extracellular DA levels and robust stimulation of behavioral activities (Munzar et al., 2004). When administered prior to METH, MOD significantly attenuated the stimulatory effects of METH on extracellular NAcc DA levels (see Table 2) (Zolkowska et al., 2009). This effect suggests the possibility that blockade of DAT by MOD pretreatment could affect the ability of METH to be transported by DAT as its substrate into the DA nerve terminal, thus reducing its ability to enhance extracellular DA levels. Reducing the dopaminergic effects of METH could play a role in the therapeutic effects shown by MOD in some preclinical behavioral reports and in clinical studies on METH dependent subjects.
Nicotine, the key addictive component in tobacco, exerts indirect actions on DAT. Voltammetry studies revealed that nicotine slows DA clearance (Hart and Ksir, 1996), in addition to nicotine’s actions in modulating dopaminergic transmission via activation of nicotinic acetylcholine receptors on DA neurons (Clarke and Pert, 1985; Picciotto et al., 1998; Laviolette and Van Der Kooy, 2004). When administered prior to nicotine, MOD produced a reduction in nicotine-induced stimulation of extracellular NAcc DA levels (see Table 2) (Wang et al., 2015).
These preclinical actions of MOD as an atypical DAT inhibitor suggest a strong potential for its therapeutic use in PSUDs (see Table 2).
Modulation of Brain Glutamate Levels by MOD Plays a Role in Its Therapeutic Actions on PSUD
The excitatory neurotransmitter, glutamate, has long been associated with many brain physiological functions and brain diseases including addiction (Meldrum, 2000; Kalivas, 2009). Interestingly, the effects of MOD administration on glutamate levels varies by brain region (reviewed in Gerrard and Malcolm, 2007; Mereu et al., 2013). It is predicted that this could be due, in part, to corresponding activation/inactivation of the inhibitory neurotransmitter, GABA. MOD produced increases in glutamate in the medial preoptic areas (Ferraro et al., 1996b), posterior hypothalamus (Ferraro et al., 1996b), thalamus (Ferraro et al., 1997a), hippocampus (Ferraro et al., 1997a), and striatum (Ferraro et al., 1996a, 1998). It was only at high does (300 mg/kg MOD) that increases in glutamate were observed in the substantia nigra or the pallidum (Ferraro et al., 1998). MOD also shows agonist activity at some glutamate receptors (group II metabotropic; mGlu2/3) (Tahsili-Fahadan et al., 2010), although this is likely not due to direct receptor activation. Behaviorally, the impaired reinstatement of extinguished CPP for opiates following MOD administration was blunted with an mGlu2/3 antagonist pretreatment (Tahsili-Fahadan et al., 2010). Neurochemically, cystine-glutamate exchange or voltage dependent calcium channel antagonist administration blocked increases in glutamate in the NAcc following MOD, in rats chronically trained to self-administer cocaine (Mahler et al., 2014).
The effects of MOD on glutamate can be directly linked to many of the agent’s biological effects. For example, MOD-produced increases in synaptic plasticity and long-term potentiation of glutamatergic connections to orexin neurons in the lateral hypothalamus is linked to improved wakefulness and cognition (Rao et al., 2007), but it is also linked to drug reinforced behaviors (Boutrel et al., 2013).
Effects of MOD on Behavioral Models of PSUD
Herein, we will review animal preclinical data on behavioral tests, mainly in rodents, used to model specific aspects of human substance use disorders, especially PSUD. Importantly, we will compare results from reports analyzing the effects of psychostimulants alone, MOD alone, and MOD in combination with psychostimulants, as summarized in Table 3.
Locomotion, Stereotypy, and Behavioral Sensitization
Acute administration of psychostimulant drugs of abuse generally produces a dose-dependent stimulation of exploratory behaviors, including locomotion and stereotyped movements in rodents (Sahakian et al., 1975). Repeated administration of psychostimulants might result in behavioral sensitization (Kalivas and Duffy, 1993; Mereu et al., 2015), a phenomena related to neurobiological adaptations (Ghasemzadeh et al., 2009; Bowers et al., 2010), which lead to a heightened behavioral response to a psychostimulant. The potential of novel drugs to cause sensitization can be indicative of their potential neurological long-term effects that could be related to the development of drug dependence (Kauer and Malenka, 2007).
Modafinil administered alone induced dose-dependent changes in locomotion and stereotyped movements in rats (Zolkowska et al., 2009; Chang et al., 2010; Alam and Choudhary, 2018) and mice (Paterson et al., 2010; Wuo-Silva et al., 2011, 2016; Young et al., 2011), with similar results found in response to R-MOD (Zhang et al., 2017). However, a report by Shuman et al. (2012) found no significant change in locomotion in mice treated with both low and high doses of MOD (Shuman et al., 2012). In rhesus monkeys, nighttime locomotion increased, but daytime locomotion had no significant effect (Andersen et al., 2010), calling into question whether the behaviors measured in these assays are due to the same mechanisms as psychostimulant drugs, or if it is a by-product of the primary wake inducing effects of MOD (Chang et al., 2010). In another report, when locomotion was tested relative to time spent awake in rats, the time awake increased, but locomotor activity only increased for the lowest dose administered (30 mg/kg) (Wisor et al., 2006).
The locomotor activating effects of MOD have also been tested in combination with several psychiatric medications and abused psychostimulants that affect brain neurotransmission at different levels. Haloperidol, a DA D2 receptor antagonist and a commonly prescribed antipsychotic medication, decreased MOD induced locomotion in rats (Alam and Choudhary, 2018), indicating a potential interaction between MOD-induced stimulation of DA levels by blockade of DAT, and inhibition of DA transmission due to blockade of DA D2 receptors by haloperidol. Further, these effects suggest the potential interactions of medications for mental disorders and addiction, which are often found comorbidly. A pretreatment with MOD did not produce significant alteration in cocaine-induced locomotion in mice (Shuman et al., 2012), but MOD significantly decreased METH induced locomotion in rats (Zolkowska et al., 2009), indicating a lack of compounding effects on locomotor activities of MOD in the latter report, which could be dependent on differences in the specific mechanisms of action between different stimulants: cocaine is a DAT blocker, while METH is a DAT substrate and a blocker of the vesicular VMAT2 transporter.
It has been reported that repeated MOD exposure in rats (Chang et al., 2010) and mice (Paterson et al., 2010; Wuo-Silva et al., 2011) would induce behavioral sensitization of locomotion and stereotyped movements, which is further enhanced by exposure to stress (Alam and Chaudhary, 2020). Also, clear individual differences in responses of mice to MOD-induced sensitization have been found (da Costa Soeiro et al., 2012), indicating the importance of better understanding how these differences may lead to individualized treatment. Rapid-onset sensitization was decreased by DA antagonists SCH23390 and sulpiride (Wuo-Silva et al., 2019), and behavioral cross-sensitization was induced between MOD and apomorphine, a direct DA agonist (Chang et al., 2010). MOD administered with cocaine (Wuo-Silva et al., 2011, 2016; Shuman et al., 2012) or METH (da Costa Soeiro et al., 2012) also caused bidirectional sensitization in mice, indicating similar neurological effects of these drugs. While these results require further validation, they may indicate possible neuronal plasticity, which for some drugs has been suggested to have a role in their dependence producing actions (Kauer and Malenka, 2007).
Conditioned Place Preference
Drug CPP paradigms consist of classically conditioning an animal to associate a contextually unique location (chamber) with administration of a drug reinforcer, while a different chamber is associated with administration of the reinforcer’s vehicle. After training, animals are given the opportunity to freely explore the distinct locations previously associated with administration of the reinforcer or its vehicle. Assessing the difference in time spent by animals in the two chambers would provide an index of their preference (potentially drug-seeking behavior), indifference, or even aversion toward the chamber associated with the reinforcer (Tzschentke, 2007). Induction of CPP can be obtained by administration of specific doses of drugs of abuse, for example psychostimulants, such as cocaine (Mueller and Stewart, 2000; Itzhak and Martin, 2002) and METH (Itzhak and Martin, 2002), but can also be obtained through illicit drugs (Liu et al., 2008) and other natural reinforcers such as palatable foods (Velázquez-Sánchez et al., 2015). Therefore, CPP is a common preclinical assay that could be used to assess the potential pleasurable effects of a novel medication and to evaluate its potential for abuse.
Modafinil administered alone was unable to induce CPP in rats when administered orally (Deroche-Gamonet et al., 2002), or by intraperitoneal injection (Tahsili-Fahadan et al., 2010; Quisenberry et al., 2013), in contrast with results found in mice (Nguyen et al., 2011; Wuo-Silva et al., 2011; Shuman et al., 2012). These results indicate a minimal, if any, pleasurable effect of MOD, resulting in a low abuse liability for naïve subjects. However, the results in mice indicate a potential species difference, thus further investigation into various model species is required to thoroughly understand the effects of MOD.
The CPP assay can also be applied to understand whether MOD can reinstate seeking of the pleasurable effects of an extinguished behavioral response to a drug of abuse (Napier et al., 2013). Practically, the chamber previously associated with administration of the reinforcer is no longer associated to it, leading the subjects to forget the learned association and return to spending equal amounts of time in both chambers. Reinstatement of CPP occurs quickly following a single administration of the reinforcer. Administration of MOD alone has been shown to reinstate cocaine induced CPP in rats (Bernardi et al., 2009). In contrast to psychostimulants, opioid CPP is not reinstated by MOD treatment (Tahsili-Fahadan et al., 2010). These studies indicate a potential relapse inducing effect of MOD, which may be detrimental to PSUD target subjects. Further investigation is required to determine the varying effects of MOD on reinstatement of drug seeking for different drugs of abuse.
Self-Administration
The abuse potential of a substance can be assessed by using animal models of self-administration behavior, which could also model the transition from sampling or recreational use of a substance to its compulsive intake (Ator and Griffiths, 2003; Edwards and Koob, 2013). Compulsive self-administration behavior in animals has been observed under specific experimental operational conditions when selected doses of psychostimulant drugs of abuse such as cocaine or amphetamines were made available (Deneau et al., 1969). In these models, the rate at which subjects would self-administer a substance could indicate the potential for abuse of a novel medication.
Modafinil (0.28–1.7 mg/kg/inj) (Deroche-Gamonet et al., 2002), alone did not promote intravenous self-administration behavior in naïve rats, indicating a lack of MOD reinforcing effects at the doses tested. Further, MOD alone (0.1–10 mg/kg/inj) did not maintain self-administration behavior in rats previously trained with intravenous doses of cocaine (Mereu et al., 2020), and similarly R-MOD was not self-administered in rats trained with nicotine (Wang et al., 2015). However, administration of MOD increased behavioral response rates for at least one dose for each subject in Rhesus monkeys previously trained to self-administer cocaine (Gold and Balster, 1996). This contradiction may be due to species differences or other procedural variables and requires further investigation.
Acute MOD treatment prior to psychostimulant self-administration sessions might indicate whether MOD would affect the reinforcing effects of those drugs. MOD or R-MOD pretreatment did not affect intravenous cocaine self-administration in rats (Deroche-Gamonet et al., 2002; Zhang et al., 2017). In contrast, a more recent study showed increased cocaine self-administration behavior at low cocaine doses (Mereu et al., 2020). Such effect was surprisingly not accompanied by enhancement of cocaine-induced stimulation of NAS DA levels, but it was reversed by pretreatments with carbenoxolone, an inhibitor of electrotonic coupling (Mereu et al., 2020). In rats, R-MOD has been shown to decrease METH (Tunstall et al., 2018) and nicotine self-administration behavior (Wang et al., 2015). Moreover, when MOD was administered chronically, it decreased cocaine self-administration responding in Rhesus monkeys (Newman et al., 2010).
After animals acquire and maintain self-administration behavior induced by abused psychostimulants, these behaviors can be extinguished by stopping drug-injections or eliminating conditioned stimuli associated with the availability or the injection of the drug. After extinction, it has been shown that non-contingent injections of the training drug or reintroduction of its associated cues can reinstate the operant behavior required to deliver the drug, suggesting a potential for relapse. These procedures could also assess the potential effects of test compounds, administered alone or as a pre-treatment, on the likelihood of relapse. Using these procedures, MOD administered alone, either acutely (Reichel and See, 2010; Holtz et al., 2012) or chronically (Reichel and See, 2012), did not reinstate behavior in rats initially trained to self-administer METH. Similar results were found with administration of R-MOD (Wang et al., 2015). However, in rhesus monkeys previously trained to self-administer cocaine, a high dose (10 mg/kg) induced reinstatement of cocaine responding (Andersen et al., 2010), which may indicate a species difference could be a factor in the obtained results. In METH-primed reinstatement tests, both acute (Reichel and See, 2010) and chronic (Reichel and See, 2012) MOD pretreatments attenuated reinstatement of drug-seeking behavior in both male and female rats (Holtz et al., 2012). MOD pretreatments did not significantly modify likelihood for reinstatement of cocaine self-administration behavior in rats (Deroche-Gamonet et al., 2002), but R-MOD reduced cocaine seeking at high doses (Zhang et al., 2017). Additionally, in rats, R-MOD pretreatment reduced nicotine-induced reinstatement of self-administration behavior (Wang et al., 2015). These results indicate that, in contrast to abused psychostimulants, MOD and R-MOD do not induce self-administration behavior, suggesting limited, if any, abuse liability. Also, they may diminish the potential for abuse of psychostimulants or reduce the drive to obtain them, and, finally, attenuate drug-induced reinstatement of drug seeking behaviors, suggesting a potential therapeutic effect in the prevention of relapse to drug use.
Intracranial Self-Stimulation
Intracranial self-stimulation is another indicator of the potential abuse liability of a substance. In this procedure, electrodes are placed in the medial forebrain bundle, and electrical stimulation is given when the subject performs the required operant task, for example nose-poking or pressing a lever. In comparison to self-administration studies, where the drug itself acts as the reinforcer, the electrical stimulation is the reinforcer in ICSS studies, allowing the assessment of whether the drug causes increased sensitivity to rewarding stimuli by altering the self-stimulation rates (Negus and Miller, 2014). Cocaine, METH, and other monoamine releasers have been found to facilitate ICSS (Bauer et al., 2013; Negus and Miller, 2014) with a correlation between facilitation rates and DA selectivity (Bauer et al., 2013; Negus and Miller, 2014), further implicating DA and DAT in the rewarding effects of these drugs.
Modafinil has been shown to facilitate ICSS responses in rats when administered orally (Lazenka and Negus, 2017) and intraperitoneally (Burrows et al., 2015). R-MOD shows a trend toward ICSS facilitation at high doses (150 mg/kg) in rats, without reaching significance (Burrows et al., 2015). However, when compared with commonly abused psychostimulants, such as methylphenidate or cocaine, MOD shows significant changes in ICSS rates only when administered at very high doses, while abused drugs show effects at significantly lower doses (Burrows et al., 2015; Lazenka and Negus, 2017). These dose differences may indicate that MOD abuse liability, if any, might require specific conditions, including very high doses, as compared to commonly abused psychostimulants. Indeed, MOD shows very low, if any, abuse liability in humans, and the benefits offered by MOD treatment against PSUD seem to outweigh the possibility of dependence.
Drug Discrimination
Administration of drugs, especially those abused by humans, would induce specific interoceptive stimuli that could be perceived and recognized by human subjects as well as animals (Kamien et al., 1993). The ability of subjects to discriminate between interoceptive stimuli elicited by a specific drug and those elicited by the drug’s vehicle could be assessed in drug discrimination procedures (Porter et al., 2018). Indeed, the presence or absence of the drug stimulus could result in different operant responses, for example pressing a lever associated to the drug stimulus or that associated to the drug vehicle. Correct responses are usually rewarded with delivery of food pellets. After training with a specific drug, tests can be performed with administration of, for example, novel compounds. It is important to note that drugs belonging to the same pharmacological class (i.e., opioids, cannabinoids, psychostimulants) usually share a common discriminative stimulus specific for their drug class. Thus, while the drug-discrimination procedure does not measure the reinforcing/rewarding effects of drugs of abuse, similarities between subjective effects of a known abused psychostimulant and novel compounds might suggest their potential for abuse (Katz and Goldberg, 1988; Berquist and Fantegrossi, 2018). Thus, several drug-discrimination studies have tested the possibility that administration of MOD produced subjective effects similar to the discriminative stimulus effects of cocaine.
Modafinil doses below 100 mg/kg produced saline only responses when administered 30 min prior to testing, and higher doses partially substituted for cocaine in rats (Gold and Balster, 1996), but later studies found full cocaine substitution (Paterson et al., 2010). In Rhesus monkeys, MOD dose dependently substituted for cocaine in three of four animals at the highest doses when administered immediately prior to testing (Newman et al., 2010) and in mice, MOD fully substituted for cocaine (Loland et al., 2012; Mereu et al., 2017) when administered 5 or 60 min prior to testing. These results indicate that the subjective effects of MOD are similar to those of cocaine. However, there was a significant difference in potency for those effects, and MOD was found about 10 (Loland et al., 2012; Mereu et al., 2017) to 25 times less potent than cocaine (Gold and Balster, 1996). Further, MOD discrimination responses in rats were lower than that of ephedrine, a common over-the-counter decongestant and bronchodilator (Gold and Balster, 1996). These findings might indicate that high doses of MOD and R-MOD could have abuse potential, but the lower doses which would aid in reducing the likelihood of relapse have little abuse potential, as shown by lack of consistent reinforcing effects in the self-administration studies above.
Behavioral Tests Related to Cognitive Functions
Cognitive impairments, such as memory deficits, decision making abilities, and learning rates are a potential concern as a consequence of persistent psychostimulant use (Block et al., 2002). While acute administration of psychostimulants has been found to positively affect cognitive functioning when given immediately prior to testing (Grilly, 2000; Del Olmo et al., 2007), long-term, repeated exposure to these drugs may produce detrimental cognitive effects. For example, in animal models, impairment of cognitive function has been reported in response to chronic administration of METH (Rogers et al., 2008) and cocaine (García-Pardo et al., 2017), among others (Marston et al., 1999; Dalley et al., 2005). MOD has been found to reverse some of the impairments induced by phencyclidine in rats (Redrobe et al., 2010), and by METH in rats and mice (González et al., 2014; Reichel et al., 2014). However, it has been reported that MOD administration had no effect on the object recognition of animals not pretreated with psychostimulants (Reichel et al., 2014), or spatial memory acquisition in rats not treated with DMSO (Shanmugasundaram et al., 2017), indicating a potential restoration of the cognitive impairments induced by drugs of abuse.
Modafinil has also been shown to improve decision making skills by decreasing impulsive responses in rats (Heyer-Osorno and Juárez, 2020), and both acute and chronic administration increases learning and memory abilities in mice (Béracochéa et al., 2002, 2003; Shuman et al., 2009) and rats (Ward et al., 2004; Morgan et al., 2007; Shuman et al., 2009). However, chronic MOD decreased long-term visuo-spatial memory errors, but increased operant conditioning learning errors, indicating an overall benefit for hippocampus dependent tasks in rats (Burgos et al., 2010). in a different study, an enhanced hippocampus dependent memory performance was reported after low doses of MOD (0.75 mg/kg), but not high doses (75 mg/kg), indicating a bell-shaped response curve (Shuman et al., 2009). Further, no effects on impulsive response rates were reported in healthy rats (Waters et al., 2005), however, these findings were explained later when improvements on a response rate task were only present in subjects previously showing slow or impaired response rates (Eagle et al., 2007). In general, these findings indicate that MOD has the potential to enhance cognitive abilities, especially when treating drug of abuse induced impairments, which may influence treatment engagement and likelihood of relapse in PSUD patients (Sofuoglu et al., 2013; Nuijten et al., 2016).
Human Studies on MOD as a Potential Pharmacotherapy for PSUD
Modafinil has shown therapeutic efficacy for treatment of individuals affected by narcolepsy and sleep disorders (Czeisler et al., 2005; Kumar, 2008), and its off-label uses have shown beneficial effects in improving cognitive function in patients with neuropsychiatric disorders, e.g., Parkinson’s disease, ADHD or PSUD (Peñaloza et al., 2013; Turner et al., 2014). Even though MOD has been suggested as a potential therapeutic agent for the treatment of PSUD (Mereu et al., 2013; Tanda et al., 2021), initial concerns related to its potential abuse liability due to its effects on the central dopaminergic system, akin to those associated with many abused psychostimulants (Jasinski, 2000; Stoops et al., 2005; Volkow et al., 2009). Concerns about its potential for abuse have also been raised by the non-medical use of MOD by healthy individuals to enhance their cognitive function, attention, learning, and memory, in order to improve academic or work-related performance (Fond et al., 2016), leading to a significant debate about potential ethical issues related to a so called “cosmetic neurology” (Cakic, 2009; Sahakian and Morein-Zamir, 2011). However, the increased non-medical use of MOD to potentially improve cognitive performance in school or work settings (Sharif et al., 2021) supports the very low risk, if any, of abuse liability (Kate et al., 2012; Ozturk and Deveci, 2014; Krishnan and Chary, 2015).
Potential Therapeutic Effects of MOD for PSUD
As summarized in Table 4, clinical studies testing MOD as a potential treatment for PSUD have generated different and sometime inconsistent results.
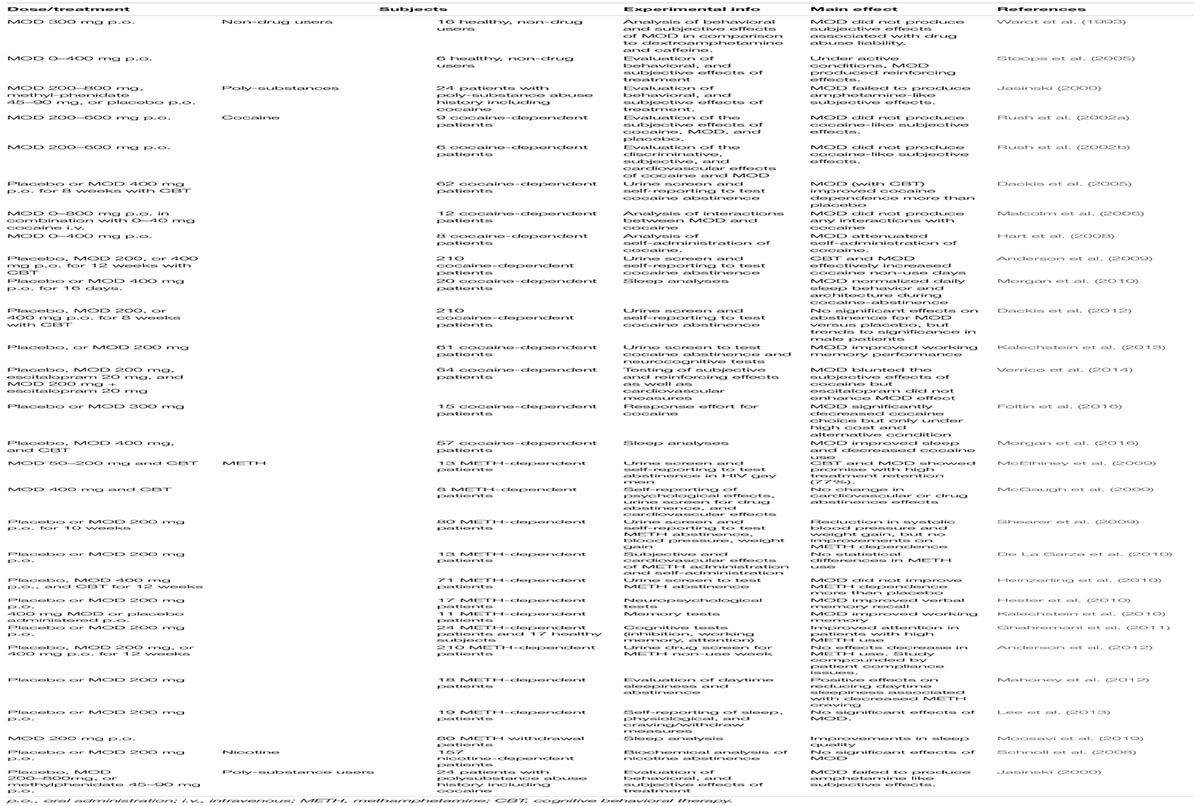
Table 4. Results of clinical studies on MOD as a pharmacological therapy for PSUD, including studies on sleep disorders and cognitive dysfunction in PSUD patients.
In an early double-blind, placebo-controlled 8-week study with 62 cocaine dependent patients, MOD 400 mg daily, combined with CBT, significantly improved BE (benzoylecgonine - a cocaine metabolite) negative urine samples over placebo, and significantly increased abstinence rate (3 or more weeks) (Dackis et al., 2005). That study also indicated the safety of MOD administered to cocaine-dependent individuals (Dackis et al., 2005), a finding consistent with previous experimental safety studies that indicated the safety of the co-administration of MOD and intravenous cocaine (Dackis et al., 2003; Malcolm et al., 2006). More recently, another double-blind, placebo-controlled study with cocaine dependent patients (N = 94), over an 8-week period, showed that patients treated with 300 mg MOD daily, combined with weekly individual therapy, were significantly more likely to be abstinent than those treated with placebo and weekly individual therapy (Kampman et al., 2015). Furthermore, MOD-treated patients reported significantly lower craving levels compared to those treated with placebo (Kampman et al., 2015). Other experimental human laboratory studies have investigated the potential role of MOD in modulating cocaine’s subjective effects, such as self-reported decreases in ‘good effects,’ ‘stimulation’ and ‘high’ (Malcolm et al., 2006; Hart et al., 2008; McGaugh et al., 2009; Verrico et al., 2014). Further, a decrease in cocaine-associated cardiovascular effects was reported after treatments with both 200 and 400 mg MOD doses, showing an objective physical response, as well as decreased self-administration of high cocaine doses (25 and 50 mg) (Hart et al., 2008).
While the safety of MOD treatments has also been observed in METH-dependent individuals (McGaugh et al., 2009), clinical studies on METH-dependent subjects are less promising than those in cocaine-dependents, although METH studies have been conducted in significantly smaller samples. For example, in a small trial of 13 METH-addicted patients treated with 200 mg of MOD, the authors did not find any significant differences versus placebo, although they reported trends of lowering METH choice by 25% in 3 days of treatment (De La Garza et al., 2010). In a different study, MOD, 200 mg daily, was tested over a 7-day inpatient period on 19 METH abstinent subjects, but no differences, compared to placebo, were found for abstinence, reported craving, or sleep measures (Lee et al., 2013). In another study, METH-dependent patients were assigned to placebo or 200 mg of MOD daily for 10 weeks (Shearer et al., 2009), resulting in no difference in retention and medical adherence between placebo and MOD in self-reported use and urine analysis. The study limitations of relying on self-reported measures and small sample size may confound the results and explain some of the variability among clinical studies for the efficacy of MOD in PSUD (Karila et al., 2010).
Efficacy of MOD Treatments in Subpopulations of Patients With Cocaine or METH Use Disorder but Without Comorbid Dependences From Other Substances
With inconsistencies plaguing the results of MOD clinical studies, future research should be focused on the specific patient groups who showed beneficial effects from MOD treatment. While Shearer et al. (2009) didn’t find significant differences between placebo and MOD treatments in the entire subjects sample, a post hoc analysis showed greatest reductions in METH use compared to placebo with MOD treatments in patients with only METH dependence (removing comorbid opioid dependent patients) (Shearer et al., 2009). MOD didn’t increase the number of non-cocaine use days compared to placebo in a trial of cocaine-dependent patients, but post hoc analysis of data found that MOD was superior to placebo in patients without an alcohol codependency (Anderson et al., 2009). Similar outcomes were also reported in a more recent study, 8-week double-blind, placebo-controlled clinical trial in cocaine-dependent subjects without comorbid alcohol dependence, where MOD (300 mg daily) treated patients were more likely to be abstinent from cocaine than patients treated with placebo (Kampman et al., 2015). In another trial on cocaine-dependent patients, no difference was reported between retention or abstinence for MOD treatment compared to placebo, but post hoc analysis revealed a significant gender difference with males taking 400 mg MOD showing a greater estimate of abstinence (Dackis et al., 2012).
In a double-blind, placebo controlled trial, MOD, 400 mg daily over 12 weeks, increased retention, while decreasing METH use, depression symptoms, and cravings in those with high METH use and low CBT attendance (Heinzerling et al., 2010). In a different study, it was found that escitalopram, a selective serotonin reuptake inhibitor and commonly prescribed antidepressant, decreased MOD’s effects, raising concern of MOD’s effect on populations of patients treated for depressive disorders alongside drug addiction (Verrico et al., 2014).
Reduced METH use in patients with an abuse diagnosis that included HIV+ participants was found in a 12-week 200 mg MOD study (McElhiney et al., 2009). In the same study, the patients using METH on average 2.2 days per week reported better MOD effects on fatigue due to withdrawal, as well as maintaining abstinence, than patients who used meth 6 days per week (McElhiney et al., 2009). As clinical research on MOD continues, it is increasingly important to study the groups of individuals that do and do not respond to treatment in order to provide critical information toward precision medicine.
MOD Effects on Sleep Disorders Related to Psychostimulant Use
The relationship between sleep disorders and substance abuse is only loosely understood, but shows some relation with sleep problems reinforcing substance use disorders, as well as substance use leading to sleep disturbances (Angarita et al., 2016). In their review, Angarita et al. (2016) characterized several sleep disturbances produced by alcohol, cocaine, cannabis, and opioid short-term and long-term abstinence, suggesting that substantial research into the effectiveness of sleep agents for addiction treatment is needed. A more recent review links the effects of neurotransmitters on sleep during intoxication and withdrawal from a variety of drugs, but notes the lack of research depth on these neurological interactions and their bearing on drug abuse and dependence (Valentino and Volkow, 2020). Also, gender differences regarding the relationship between drug abstinence and sleep have been described (Coffey et al., 2000; Morgan et al., 2009). A study of short-term METH abstinence found a positive correlation between wanting a nap and craving METH (Mahoney et al., 2012). The study found that a single dose of MOD 200 mg decreased daytime sleepiness, supporting the potential use of MOD as an adjunct treatment for PSUD.
Modafinil has been shown to increase and normalize slow wave sleep to healthy patterns in abstinent cocaine users (Morgan et al., 2010). It was also recently found that while increasing slow wave sleep did not lead to complete, continued abstinence, 400 mg MOD treatment was associated with higher daily rates of abstinence and more consecutive days of abstinence (Morgan et al., 2016). Further, is has been reported that 200 mg MOD improved the sleep quantity and pattern in patients during METH withdrawal (Moosavi et al., 2019).
MOD Effects on Cognitive Impairment Produced by Psychostimulant Use
Addiction brings changes to the brain beyond the reward pathway. Mental processing dysfunction can hamper rehabilitation attempts and, thus, a drug that can attenuate these risks would be beneficial to the addicted population (Gould, 2010). Cocaine-dependent patients in abstinence showed lower activation compared to healthy controls in areas associated with motor and cognitive functions (Kjome et al., 2010). There have been quite a few studies into MOD’s effects on working memory. In a double-blind, placebo controlled study, it was shown that 400 mg MOD improved working memory in 11 METH-dependent subjects, with poor performance at baseline, after 3 days of treatment (Kalechstein et al., 2010). The same group later showed, in a placebo controlled study, that MOD at 200 mg improved visual and working memory in a group of 61 cocaine-dependent patients, as well as attention and impulsivity, with 5 days of treatment (Kalechstein et al., 2013). While promising, these studies also hold some limitations, in particular the short-term period of treatment and the small samples.
Even though not directly related to PSUD, effects of MOD on performance related to cognitive function were shown in a randomized, double-blind, placebo controlled, crossover study, where 200 mg of MOD administered acutely improved cognitive control in alcohol-dependent patients, but not in the healthy control group (Schmaal et al., 2013). Also, the same researchers showed that administration of 200 mg of MOD improved impulsive decision making in alcohol dependent patients compared to healthy controls (Schmaal et al., 2014). The alcohol dependent group had poor baseline performances compared to the healthy group. This difference could imply that MOD normalizes the brain’s engagement to improve cognition to normal levels in lower performing groups, and the authors suggest that there was likely no room for improvement by MOD in the healthy controls (Schmaal et al., 2014). Further, it has been shown that MOD improved response inhibition in alcohol dependent patients whose initial response was poor (Schmaal et al., 2013). Similar effects related to PSUD were shown in METH-dependent patients in a double-blind, placebo controlled, crossover study, where 200 mg of MOD increased poorly cognitive performance in METH-dependent patients to the same level as the healthy control (Ghahremani et al., 2011). Post hoc analysis also revealed that MOD produced larger effects in lower performing participants. Similar findings were also reported in METH-dependent patients where MOD treatments showed larger effects on inhibitory control, processing speed/attention, and motor speed in subject using higher levels of METH compared to those with lower METH usage (Dean et al., 2011). In another study, it was shown that cocaine dependent participants had lower Balloon Analog Risk Task (BART) scores but MOD treated cocaine-dependent participants had higher BART scores, which were comparable to the healthy placebo, showing a normalization of risk taking while on MOD (Canavan et al., 2014).
In a study combining MOD with CBT, it was found that crack cocaine-dependent patients with lower baselines of impulsivity (self-reported) had higher CBT retention and lower crack cocaine use (Nuijten et al., 2016). However, MOD treatment in these patients did not improve CBT retention or outcomes, which is likely as a result of the low MOD adherence during this trial. This study weakness was reported by the same researchers showing that only 10% adherence was reported during a 12 week CBT and MOD trial (Nuijten et al., 2015).
Beyond MOD: Drug Development of MOD Analogs as Pharmacotherapeutics for PSUD
The effectiveness of MOD as a medication for PSUD has been shown to reach significance in sub-populations of patients without comorbid dependencies from other drugs. In recent years, this important limitation of MOD efficacy has stimulated the development of new structural analogs of MOD to extend therapeutic actions to a broader population and, thus, maximize the effects of the parent drug for use in treatment of PSUD. Some of these novel agents showing atypical DAT blocker properties, have been highlighted in recently published reviews (Newman et al., 2021; Tanda et al., 2021). Among them, some have been shown to bind with high affinity to DAT, and those that promote an inward facing conformation of DAT have shown behavioral and neurochemical preclinical activities different from those of typical abused psychostimulants (Cao et al., 2010, 2016; Okunola-Bakare et al., 2014). Such effects suggest an atypical DAT blocker profile (Keighron et al., 2019a; Newman et al., 2019, 2021; Tanda et al., 2021) and their potential as novel agents for use in the treatment of PSUD.
The effects of MOD analogs on DA neurochemistry have shown varying results (see Table 5). One of the tested analogs, JJC8-016, was unable to stimulate extracellular levels of DA after systemic administration (Zhang et al., 2017; Keighron et al., 2019b), in contrast to other MOD analogs, like JJC8-088 that significantly increased DA levels in a cocaine-like manner, or like JJC8-091 that elicited significant, but less efficacious, increases in DA levels. It is worth noting that the varying effects on stimulation of DA levels were not a result of an altered efficacy as DAT blockers. Indeed, all of these compounds were able to block and reduce DA uptake, an effect highly correlated to their affinity to DAT, as demonstrated by voltammetry studies in rats and mice (Keighron et al., 2019b; Newman et al., 2019). Moreover, their varying ability to enhance the stimulation of elicited DA release in voltammetry studies was unrelated to their affinity for DAT (Keighron et al., 2019b; Newman et al., 2019). These effects once more suggest that compounds that prefer or stabilize an inward facing conformation of DAT would produce limited, if any, cocaine-like effects (Keighron et al., 2019a, b; Giancola et al., 2020; Slack et al., 2020). The same MOD analogs have been tested in behavioral activities related to the reinforcing effects of psychostimulants, and those showing very low stimulation of DA output in microdialysis and voltammetry studies were also among those that produced limited stimulation of ambulatory activities (Keighron et al., 2019a, b; Giancola et al., 2020; Slack et al., 2020). Also, while they did not elicit acquisition or maintenance of self-administration behavior, these MOD analogs blunted cocaine or METH reinforcing and drug-seeking behaviors (Zhang et al., 2017; Tunstall et al., 2018; Newman et al., 2019), suggesting once more that their atypical DAT blocker profile and potential therapeutic activity could be useful as PSUD medications.
Conclusion
Modafinil is clinically approved for narcolepsy and other sleep disorders (Bastoji and Jouvet, 1988; Broughton et al., 1997; US Modafinil in Narcolepsy Multicenter Study Group, 1998, 2000), but its off-label use for treatment of several psychiatric disorders has been repeatedly reported (Ballon and Feifel, 2006; Peñaloza et al., 2013; Turner et al., 2014). During the last two decades, there have been several preclinical and clinical studies that suggested potential efficacy of MOD as a treatment for PSUD, but also contrasting results from other studies which limited its progression (Lee et al., 2013; Schmitz et al., 2014). Among the positive results, it is interesting to note that after many years of clinical use, there are only a few reports of abuse in MOD-treated patients (Kate et al., 2012; Ozturk and Deveci, 2014; Krishnan and Chary, 2015), a result in agreement with clinical and preclinical studies showing its limited, if any, potential for abuse (Jasinski, 2000; Deroche-Gamonet et al., 2002; Myrick et al., 2004; Food and Drug Administration, 2007; Vosburg et al., 2010). On the other hand, disappointing results of clinical trials testing MOD as a treatment for PSUD have been obtained in the general population of drug-dependents. However, based on results from several of those reports, positive treatment outcomes have been found when the population sample included only subjects with psychostimulant dependency, without concurrent alcohol or other drug dependencies (Anderson et al., 2009; Shearer et al., 2009; Kampman et al., 2015). These studies underscore the importance of pursing personalized treatment approaches for PSUD, similarly to other medical disorders (Hamburg and Collins, 2010; Schork, 2015). It is clear that the complexity of PSUD, the huge differences in how PSUD develops among the population, and the presence of many other individual, genetic, or environmental variables, suggest it is unlikely that there will ever be a “silver bullet” medication to treat all individuals with PSUD. Thus, personalized medicine approaches, together with behavioral cognitive treatments, might be the most effective path to reduce the harm produced by PSUD. While MOD has been shown to improve several emerging pathological conditions related to psychostimulant use, i.e., dependence, sleep, and cognitive impairments, its overall limited success has triggered medicinal chemistry research toward discovery of structural analogs of MOD, that might hold more robust efficacy in PSUD. In conclusion, while MOD could be an effective pharmacological treatment already available for subpopulations of individuals suffering from PSUD, new pharmacological tools derived from MOD show promising preclinical efficacy and could help to provide more efficacious future treatment opportunities for PSUD.
Author Contributions
All authors contributed to the manuscript and approved the submitted version.
Funding
This work was supported by the Medication Development Program (Z1A-DA000611), National Institute on Drug Abuse, Intramural Research Program, NIH, DHHS.
Conflict of Interest
The authors declare that the research was conducted in the absence of any commercial or financial relationships that could be construed as a potential conflict of interest.
The reviewer TK declared a past co-authorship with one of the authors, AN, to the handling editor.
Acknowledgments
The authors would like to thank Dr. Gail Seabold for her suggestions and comments on an earlier version of this manuscript.
Abbreviations
ADHD, attention deficit hyperactivity disorder; CNS, central nervous system; CPP, conditioned place preference; CBT, cognitive behavioral therapy; DA, dopamine; DAT, dopamine transporter; DS, dorsal striatum; ICSS, intracranial self-stimulation; MOD, modafinil; METH, methamphetamine; NAcc, nucleus accumbens; NAS, nucleus accumbens shell; NE, norepinephrine; NET, norepinephrine transporter; PET, positron emission tomography; PFC, prefrontal cortex; PSUD, psychostimulant use disorder; R-MOD, (R)-enantiomer of modafinil; VMAT2, vesicular monoamine transporter; VS, ventral striatum.
References
Alam, N., and Chaudhary, K. (2020). Repeated restraint stress potentiates methylphenidate and modafinil-induced behavioral sensitization in rats. Naunyn Schmiedebergs Arch. Pharmacol. 393, 785–795. doi: 10.1007/s00210-019-01790-4
Alam, N., and Choudhary, K. (2018). Haloperidol attenuates Methylphenidate and Modafinil induced behavioural sensitization and cognitive enhancement. Metab. Brain Dis. 33, 893–906. doi: 10.1007/s11011-018-0190-x
Andersen, M. L., Kessler, E., Murnane, K. S., Mcclung, J. C., Tufik, S., and Howell, L. L. J. P. (2010). Dopamine transporter-related effects of modafinil in rhesus monkeys. Psychopharmacology 210, 439–448. doi: 10.1007/s00213-010-1839-2
Anderson, A. L., Li, S.-H., Biswas, K., Mcsherry, F., Holmes, T., Iturriaga, E., et al. (2012). Modafinil for the treatment of methamphetamine dependence. J. Drug Alcohol. Depend. 120, 135–141. doi: 10.1016/j.drugalcdep.2011.07.007
Anderson, A. L., Reid, M. S., Li, S.-H., Holmes, T., Shemanski, L., Slee, A., et al. (2009). Modafinil for the treatment of cocaine dependence. Drug Alcohol. Depend. 104, 133–139.
Angarita, G. A., Emadi, N., Hodges, S., and Morgan, P. T. (2016). Sleep abnormalities associated with alcohol, cannabis, cocaine, and opiate use: a comprehensive review. Addict. Sci. Clin. Pract. 11:9.
Arias-Carrión, O., Stamelou, M., Murillo-Rodríguez, E., Menéndez-González, M., and Pöppel, E. (2010). Dopaminergic reward system: a short integrative review. Int. Arch. Med. 3:24. doi: 10.1186/1755-7682-3-24
Ator, N. A., and Griffiths, R. R. (2003). Principles of drug abuse liability assessment in laboratory animals. Drug Alcohol. Depend. 70, S55–S72.
Ballon, J. S., and Feifel, D. (2006). A systematic review of modafinil: potential clinical uses and mechanisms of action. J. Clin. Psychiatry 67, 554–566. doi: 10.4088/jcp.v67n0406
Barr, A. M., Panenka, W. J., Macewan, G. W., Thornton, A. E., Lang, D. J., Honer, W. G., et al. (2006). The need for speed: an update on methamphetamine addiction. J. Psychiatry Neurosci. 31, 301–313.
Bastoji, H., and Jouvet, M. (1988). Successful treatment of idiopathic hypersomnia and narcolepsy with modafinil. Prog. Neuro Psychopharmacol. Biol. Psychiatry 12, 695–700. doi: 10.1016/0278-5846(88)90014-0
Bauer, C., Banks, M., Blough, B., and Negus, S. (2013). Use of intracranial self-stimulation to evaluate abuse-related and abuse-limiting effects of monoamine releasers in rats. Br. J. Pharmacol. 168, 850–862. doi: 10.1111/j.1476-5381.2012.02214.x
Béracochéa, D., Cagnard, B., Célérier, A., Le Merrer, J., Pérès, M., and Piérard, C. (2001). First evidence of a delay-dependent working memory-enhancing effect of modafinil in mice. Neuroreport 12, 375–378. doi: 10.1097/00001756-200102120-00038
Béracochéa, D., Celerier, A., Borde, N., Valleau, M., Peres, M., and Pierard, C. (2002). Improvement of learning processes following chronic systemic administration of modafinil in mice. Pharmacol. Biochem. Behav. 73, 723–728. doi: 10.1016/s0091-3057(02)00877-8
Béracochéa, D., Celerier, A., Peres, M., and Pierard, C. (2003). Enhancement of learning processes following an acute modafinil injection in mice. Pharmacol. Biochem. Behav. 76, 473–479. doi: 10.1016/j.pbb.2003.09.007
Bernardi, R. E., Lewis, J. R., Lattal, K. M., and Berger, S. P. (2009). Modafinil reinstates a cocaine conditioned place preference following extinction in rats. Behav. Brain Res. 204, 250–253. doi: 10.1016/j.bbr.2009.05.028
Berquist, M. D., and Fantegrossi, W. E. (2018). Discriminative stimulus effects of psychostimulants. Curr. Top. Behav. Neurosci. 39, 29–49. doi: 10.1007/7854_2017_5
Beusterien, K. M., Rogers, A. E., Walsleben, J. A., Emsellem, H. A., Reblando, J. A., Wang, L., et al. (1999). Health-related quality of life effects of modafinil for treatment of narcolepsy. Sleep 22, 757–765. doi: 10.1093/sleep/22.6.757
Block, R. I., Erwin, W. J., and Ghoneim, M. M. (2002). Chronic drug use and cognitive impairments. Pharmacol. Biochem. Behav. 73, 491–504. doi: 10.1016/s0091-3057(02)00816-x
Bobak, M. J., Weber, M. W., Doellman, M. A., Schuweiler, D. R., Athens, J. M., Juliano, S. A., et al. (2016). Modafinil activates phasic dopamine signaling in dorsal and ventral striata. J. Pharmacol. Exp. Ther. 359, 460–470. doi: 10.1124/jpet.116.236000
Bolla, K. I., Rothman, R., and Cadet, J. L. (1999). Dose-related neurobehavioral effects of chronic cocaine use. J. Neuropsychiatry Clin. Neurosci. 11, 361–369. doi: 10.1176/jnp.11.3.361
Boutrel, B., and Koob, G. F. (2004). What keeps us awake: the neuropharmacology of stimulants and wakefulness promoting medications. Sleep 27, 1181–1194. doi: 10.1093/sleep/27.6.1181
Boutrel, B., Steiner, N., and Halfon, O. (2013). The hypocretins and the reward function: what have we learned so far? Front. Behav. Neurosci. 7:59.
Bowers, M. S., Chen, B. T., and Bonci, A. (2010). AMPA receptor synaptic plasticity induced by psychostimulants: the past, present, and therapeutic future. Neuron 67, 11–24. doi: 10.1016/j.neuron.2010.06.004
Bradford, S. T., Stamatovic, S. M., Dondeti, R. S., Keep, R. F., and Andjelkovic, A. V. (2011). Nicotine aggravates the brain postischemic inflammatory response. Am. J. Physiol. Heart Circulatory Physiol. 300, H1518–H1529.
Brandao, W. N., Andersen, M. L., Palermo-Neto, J., Peron, J. P., and Zager, A. (2019). Therapeutic treatment with Modafinil decreases the severity of experimental autoimmune encephalomyelitis in mice. Int. Immunopharmacol. 75:105809. doi: 10.1016/j.intimp.2019.105809
Brandt, M. D., Ellwardt, E., and Storch, A. (2014). Short- and long-term treatment with modafinil differentially affects adult Hippocampal neurogenesis. Neuroscience 278, 267–275. doi: 10.1016/j.neuroscience.2014.08.014
Broughton, R., Fleming, J., George, C., Hill, J., Kryger, M., Moldofsky, H., et al. (1997). Randomized, double-blind, placebo-controlled crossover trial of modafinil in the treatment of excessive daytime sleepiness in narcolepsy. Neurology 49, 444–451. doi: 10.1212/wnl.49.2.444
Burgos, H., Castillo, A., Flores, O., Puentes, G., Morgan, C., Gatica, A., et al. (2010). Effect of modafinil on learning performance and neocortical long-term potentiation in rats. Brain Res. Bull. 83, 238–244. doi: 10.1016/j.brainresbull.2010.08.010
Burrows, B. T., Watterson, L. R., Johnson, M. A., and Olive, M. F. (2015). Effects of modafinil and R-modafinil on brain stimulation reward thresholds: implications for their use in the treatment of psychostimulant dependence. J. Drug Alcohol. Res. 4:235958.
Cadet, J. L., Sheng, P., All, S., Rothman, R., Carlson, E., and Epstein, C. (1994). Rapid communication: attenuation of methamphetamine-induced neurotoxicity in Copper/Zinc Superoxide dismutase transgenic mice. J. Neurochem. 62, 380–383. doi: 10.1046/j.1471-4159.1994.62010380.x
Cakic, V. (2009). Smart drugs for cognitive enhancement: ethical and pragmatic considerations in the era of cosmetic neurology. J. Med. Ethics 35, 611–615. doi: 10.1136/jme.2009.030882
Calipari, E. S., Ferris, M. J., Salahpour, A., Caron, M. G., and Jones, S. R. (2013). Methylphenidate amplifies the potency and reinforcing effects of amphetamines by increasing dopamine transporter expression. Nat. Commun. 4:2720.
Canavan, S. V., Forselius, E. L., Bessette, A. J., and Morgan, P. T. (2014). Preliminary evidence for normalization of risk taking by modafinil in chronic cocaine users. Addict. Behav. 39, 1057–1061. doi: 10.1016/j.addbeh.2014.02.015
Cao, J., Prisinzano, T. E., Okunola, O. M., Kopajtic, T., Shook, M., Katz, J. L., et al. (2010). Structure-activity relationships at the monoamine transporters for a novel series of modafinil (2-[(diphenylmethyl)sulfinyl]acetamide) analogues. ACS Med. Chem. Lett. 2, 48–52.
Cao, J., Slack, R. D., Bakare, O. M., Burzynski, C., Rais, R., Slusher, B. S., et al. (2016). Novel and high Affinity 2-[(Diphenylmethyl)sulfinyl]acetamide (Modafinil) analogues as atypical dopamine transporter inhibitors. J. Med. Chem. 59, 10676–10691. doi: 10.1021/acs.jmedchem.6b01373
Chamberlain, S. R., and Robbins, T. W. (2013). Noradrenergic modulation of cognition: therapeutic implications. J. Psychopharmacol. 27, 694–718. doi: 10.1177/0269881113480988
Chang, S.-T., Tung, C.-S., Lin, Y.-L., Chuang, C.-H., Lee, A.-R., and Liu, Y.-P. (2010). Behavioral and cross sensitization after repeated exposure to modafinil and apomorphine in rats. Chin. J. Physiol. 53, 318–327. doi: 10.4077/cjp.2010.amk067
Chemelli, R. M., Willie, J. T., Sinton, C. M., Elmquist, J. K., Scammell, T., Lee, C., et al. (1999). Narcolepsy in orexin knockout mice: molecular genetics of sleep regulation. Cell 98, 437–451.
Clarke, P. B., and Pert, A. (1985). Autoradiographic evidence for nicotine receptors on nigrostriatal and mesolimbic dopaminergic neurons. Brain Res. 348, 355–358. doi: 10.1016/0006-8993(85)90456-1
Coffey, S. F., Dansky, B. S., Carrigan, M. H., and Brady, K. T. (2000). Acute and protracted cocaine abstinence in an outpatient population: a prospective study of mood, sleep and withdrawal symptoms. Drug Alcohol. Depend. 59, 277–286. doi: 10.1016/s0376-8716(99)00126-x
Compton, W. M., Han, B., Blanco, C., Johnson, K., and Jones, C. M. (2018). Prevalence and correlates of prescription stimulant use, misuse, use disorders, and motivations for misuse among adults in the United States. Am. J. Psychiatry 175, 741–755. doi: 10.1176/appi.ajp.2018.17091048
Cope, Z. A., Minassian, A., Kreitner, D., Macqueen, D. A., Milienne-Petiot, M., Geyer, M. A., et al. (2017). Modafinil improves attentional performance in healthy, non-sleep deprived humans at doses not inducing hyperarousal across species. Neuropharmacology 125, 254–262. doi: 10.1016/j.neuropharm.2017.07.031
Czeisler, C. A., Walsh, J. K., Roth, T., Hughes, R. J., Wright, K. P., Kingsbury, L., et al. (2005). Modafinil for excessive sleepiness associated with shift-work sleep disorder. N. Engl. J. Med. 353, 476–486. doi: 10.1056/nejmoa041292
Czub, S., Koutsilieri, E., Sopper, S., Czub, M., Stahl-Hennig, C., Müller, J., et al. (2001). Enhancement of central nervous system pathology in early simian immunodeficiency virus infection by dopaminergic drugs. Acta Neuropathol. 101, 85–91.
da Costa Soeiro, A., Moreira, K. D. M., Abrahao, K. P., Quadros, I. M. H., and Oliveira, M. G. M. (2012). Individual differences are critical in determining modafinil-induced behavioral sensitization and cross-sensitization with methamphetamine in mice. Behav. Brain Res. 233, 367–374. doi: 10.1016/j.bbr.2012.05.023
Dackis, C. A., Kampman, K. M., Lynch, K. G., Pettinati, H. M., and O’brien, C. P. (2005). A double-blind, placebo-controlled trial of modafinil for cocaine dependence. Neuropsychopharmacology 30, 205–211. doi: 10.1038/sj.npp.1300600
Dackis, C. A., Kampman, K. M., Lynch, K. G., Plebani, J. G., Pettinati, H. M., Sparkman, T., et al. (2012). A double-blind, placebo-controlled trial of modafinil for cocaine dependence. J. Subst. Abuse Treat. 43, 303–312.
Dackis, C. A., Lynch, K. G., Yu, E., Samaha, F. F., Kampman, K. M., Cornish, J. W., et al. (2003). Modafinil and cocaine: a double-blind, placebo-controlled drug interaction study. Drug Alcohol. Depend. 70, 29–37. doi: 10.1016/s0376-8716(02)00335-6
Dalley, J. W., Lääne, K., Pena, Y., Theobald, D. E., Everitt, B. J., and Robbins, T. W. (2005). Attentional and motivational deficits in rats withdrawn from intravenous self-administration of cocaine or heroin. Psychopharmacology 182, 579–587. doi: 10.1007/s00213-005-0107-3
Das, G. (1993). Cocaine abuse in North America: a milestone in history. J. Clin. Pharmacol. 33, 296–310. doi: 10.1002/j.1552-4604.1993.tb04661.x
Daws, L. C., Callaghan, P. D., Morón, J. A., Kahlig, K. M., Shippenberg, T. S., Javitch, J. A., et al. (2002). Cocaine increases dopamine uptake and cell surface expression of dopamine transporters. Biochem. Biophys. Res. Commun. 290, 1545–1550. doi: 10.1006/bbrc.2002.6384
De La Garza, R., Zorick, T., London, E. D., and Newton, T. F. (2010). Evaluation of modafinil effects on cardiovascular, subjective, and reinforcing effects of methamphetamine in methamphetamine-dependent volunteers. Drug Alcohol. Depend. 106, 173–180. doi: 10.1016/j.drugalcdep.2009.08.013
de Lima, M. S., Farrell, M., Reisser, A. A. L., and Soares, B. (2003). Antidepressants for cocaine dependence. Cochrane Database Syst. Res. 7:CD002950.
de Saint Hilaire, Z., Orosco, M., Rouch, C., Blanc, G., and Nicolaidis, S. (2001). Variations in extracellular monoamines in the prefrontal cortex and medial hypothalamus after modafinil administration: a microdialysis study in rats. Neuroreport 12, 3533–3537. doi: 10.1097/00001756-200111160-00032
Dean, A. C., Sevak, R. J., Monterosso, J. R., Hellemann, G., Sugar, C. A., and London, E. D. (2011). Acute modafinil effects on attention and inhibitory control in methamphetamine-dependent humans. J. Stud. Alcohol Drugs 72, 943–953. doi: 10.15288/jsad.2011.72.943
Del Olmo, N., Higuera-Matas, A., Miguens, M., Garcia-Lecumberri, C., and Ambrosio, E. (2007). Cocaine self-administration improves performance in a highly demanding water maze task. Psychopharmacology 195, 19–25. doi: 10.1007/s00213-007-0873-1
Deneau, G., Yanagita, T., and Seevers, M. (1969). Self-administration of psychoactive substances by the monkey. Psychopharmacologia 16, 30–48. doi: 10.1007/bf00405254
Deroche-Gamonet, V., Darnaudery, M., Bruins-Slot, L., Piat, F., Le Moal, M., and Piazza, P. (2002). Study of the addictive potential of modafinil in naive and cocaine-experienced rats. Psychopharmacology 161, 387–395. doi: 10.1007/s00213-002-1080-8
Dhillon, N. K., Peng, F., Bokhari, S., Callen, S., Shin, S.-H., Zhu, X., et al. (2008). Cocaine-mediated alteration in tight junction protein expression and modulation of CCL2/CCR2 axis across the blood-brain barrier: implications for HIV-dementia. J. Neuroimmune Pharmacol. 3, 52–56. doi: 10.1007/s11481-007-9091-1
Di Chiara, G., Acquas, E., Tanda, G., and Cadoni, C. (1993a). Drugs of abuse: biochemical surrogates of specific aspects of natural reward? Biochem. Soc. Symp. 59, 65–81.
Di Chiara, G., and Imperato, A. (1988). Drugs abused by humans preferentially increase synaptic dopamine concentrations in the mesolimbic system of freely moving rats. Proc. Natl. Acad. Sci. U.S.A. 85, 5274–5278. doi: 10.1073/pnas.85.14.5274
Di Chiara, G., Tanda, G., Bassareo, V., Pontieri, F., Acquas, E., Fenu, S., et al. (1999). Drug addiction as a disorder of associative learning. Role of nucleus accumbens shell/extended amygdala dopamine. Ann. N. Y. Acad. Sci. 877, 461–485. doi: 10.1111/j.1749-6632.1999.tb09283.x
Di Chiara, G., Tanda, G., Cadoni, C., Acquas, E., Bassareo, V., and Carboni, E. (1998). Homologies and differences in the action of drugs of abuse and a conventional reinforcer (food) on dopamine transmission: an interpretative framework of the mechanism of drug dependence. Adv. Pharmacol. 42, 983–987. doi: 10.1016/s1054-3589(08)60911-4
Di Chiara, G., Tanda, G., Frau, R., and Carboni, E. (1993b). On the preferential release of dopamine in the nucleus accumbens by amphetamine: further evidence obtained by vertically implanted concentric dialysis probes. Psychopharmacology 112, 398–402. doi: 10.1007/bf02244939
Diana, M. (2011). The dopamine hypothesis of drug addiction and its potential therapeutic value. Front. Psychiatry 2:64.
Duchêne, A., Perier, M., Zhao, Y., Liu, X., Thomasson, J., Chauveau, F., et al. (2016). Impact of astroglial connexins on modafinil pharmacological properties. Sleep 39, 1283–1292. doi: 10.5665/sleep.5854
Duflou, J. (2020). Psychostimulant use disorder and the heart. Addiction 115, 175–183. doi: 10.1111/add.14713
Eagle, D. M., Tufft, M. R., Goodchild, H. L., and Robbins, T. W. J. P. (2007). Differential effects of modafinil and methylphenidate on stop-signal reaction time task performance in the rat, and interactions with the dopamine receptor antagonist cis-flupenthixol. Psychopharmacology 192, 193–206. doi: 10.1007/s00213-007-0701-7
Edwards, S., and Koob, G. F. (2013). Escalation of drug self-administration as a hallmark of persistent addiction liability. Behav. Pharmacol. 24, 356–362. doi: 10.1097/fbp.0b013e3283644d15
Elkashef, A., Holmes, T. H., Bloch, D. A., Shoptaw, S., Kampman, K., Reid, M. S., et al. (2005). Retrospective analyses of pooled data from CREST I and CREST II trials for treatment of cocaine dependence. Addiction 100, 91–101. doi: 10.1111/j.1360-0443.2005.00986.x
Espana, R., Baldo, B., Kelley, A., and Berridge, C. (2001). Wake-promoting and sleep-suppressing actions of hypocretin (orexin): basal forebrain sites of action. Neuroscience 106, 699–715. doi: 10.1016/s0306-4522(01)00319-0
Farrell, M., Martin, N. K., Stockings, E., Bórquez, A., Cepeda, J. A., Degenhardt, L., et al. (2019). Responding to global stimulant use: challenges and opportunities. Lancet 394, 1652–1667. doi: 10.1016/s0140-6736(19)32230-5
Ferraro, L., Antonelli, T., O’connor, W. T., Tanganelli, S., Rambert, F. A., and Fuxe, K. (1997a). Modafinil: an antinarcoleptic drug with a different neurochemical profile to d-amphetamine and dopamine uptake blockers. Biol. Psychiatry 42, 1181–1183. doi: 10.1016/s0006-3223(97)00353-3
Ferraro, L., Antonelli, T., O’connor, W. T., Tanganelli, S., Rambert, F., and Fuxe, K. (1997b). The antinarcoleptic drug modafinil increases glutamate release in thalamic areas and hippocampus. Neuroreport 8, 2883–2887. doi: 10.1097/00001756-199709080-00016
Ferraro, L., Antonelli, T., O’connor, W. T., Tanganelli, S., Rambert, F. A., and Fuxe, K. (1998). The effects of modafinil on striatal, pallidal and nigral GABA and glutamate release in the conscious rat: evidence for a preferential inhibition of striato-pallidal GABA transmission. Neurosci. Lett. 253, 135–138.
Ferraro, L., Antonelli, T., Tanganelli, S., O’connor, W. T., De La Mora, M. P., Mendez-Franco, J., et al. (1999). The vigilance promoting drug modafinil increases extracellular glutamate levels in the medial preoptic area and the posterior hypothalamus of the conscious rat: prevention by local GABA A receptor blockade. Neuropsychopharmacology 20, 346–356. doi: 10.1016/s0893-133x(98)00085-2
Ferraro, L., Fuxe, K., Tanganelli, S., Fernandez, M., Rambert, F., and Antonelli, T. (2000). Amplification of cortical serotonin release: a further neurochemical action of the vigilance-promoting drug modafinil. Neuropharmacology 39, 1974–1983. doi: 10.1016/s0028-3908(00)00019-8
Ferraro, L., O’connor, W. T., Li, X. M., Rimondini, R., Beani, L., Ungerstedt, U., et al. (1996a). Evidence for a differential cholecystokinin-B and -A receptor regulation of GABA release in the rat nucleus accumbens mediated via dopaminergic and cholinergic mechanisms. Neuroscience 73, 941–950. doi: 10.1016/0306-4522(96)00098-x
Ferraro, L., Tanganelli, S., O’connor, W. T., Antonelli, T., Rambert, F., and Fuxe, K. (1996b). The vigilance promoting drug modafinil decreases GABA release in the medial preoptic area and in the posterior hypothalamus of the awake rat: possible involvement of the serotonergic 5-HT3 receptor. Neurosci. Lett. 220, 5–8. doi: 10.1016/s0304-3940(96)13212-2
Ferraro, L., Tanganelli, S., O’connor, W. T., Antonelli, T., Rambert, F., and Fuxe, K. (1996c). The vigilance promoting drug modafinil increases dopamine release in the rat nucleus accumbens via the involvement of a local GABAergic mechanism. Eur. J. Pharmacol. 306, 33–39. doi: 10.1016/0014-2999(96)00182-3
Finke, K., Dodds, C. M., Bublak, P., Regenthal, R., Baumann, F., Manly, T., et al. (2010). Effects of modafinil and methylphenidate on visual attention capacity: a TVA-based study. Psychopharmacology 210, 317–329. doi: 10.1007/s00213-010-1823-x
Foltin, R. W., Haney, M., Bedi, G., and Evans, S. M. (2016). Modafinil decreases cocaine choice in human cocaine smokers only when the response requirement and the alternative reinforcer magnitude are large. Pharmacol. Biochem. Behav. 150, 8–13. doi: 10.1016/j.pbb.2016.08.009
Fond, G., Gavaret, M., Vidal, C., Brunel, L., Riveline, J.-P., Micoulaud-Franchi, J.-A., et al. (2016). (Mis) use of prescribed stimulants in the medical student community: motives and behaviors: a population-based cross-sectional study. Medicine 95:e3366. doi: 10.1097/md.0000000000003366
Food and Drug Administration (2007). FDA Approved Labeling, PROVIGIL§(modafinil) Tablets. Silver Spring: Food and Drug Administration.
Frye, M. A., Grunze, H., Suppes, T., Mcelroy, S. L., Keck, P. E., Walden, J., et al. (2007). A placebo-controlled evaluation of adjunctive modafinil in the treatment of bipolar depression. Am. J. Psychiatry 164, 1242–1249. doi: 10.1176/appi.ajp.2007.06060981
Gan, X., Zhang, L., Berger, O., Stins, M. F., Way, D., Taub, D. D., et al. (1999). Cocaine enhances brain endothelial adhesion molecules and leukocyte migration. Clin. Immunol. 91, 68–76. doi: 10.1006/clim.1998.4683
Garcia, V. A., De Freitas, B. S., Busato, S. B., Portal, B. C. D. A., Piazza, F. C., and Schröder, N. J. N. (2013). Differential effects of modafinil on memory in naïve and memory-impaired rats. Neuropharmacology 75, 304–311. doi: 10.1016/j.neuropharm.2013.07.038
García-Pardo, M. P., De La Rubia, Ortí, J. E., and Calpe, M. A. A. (2017). Differential effects of MDMA and cocaine on inhibitory avoidance and object recognition tests in rodents. Neurobiol. Learn. Mem. 146, 1–11. doi: 10.1016/j.nlm.2017.10.013
Garcia-Rill, E., Heister, D. S., Ye, M., Charlesworth, A., and Hayar, A. (2007). Electrical coupling: novel mechanism for sleep-wake control. Sleep 30, 1405–1414. doi: 10.1093/sleep/30.11.1405
Gawin, F. H. (1991). Cocaine addiction: psychology and neurophysiology. Science 251, 1580–1586. doi: 10.1126/science.2011738
Gerrard, P., and Malcolm, R. (2007). Mechanisms of modafinil: a review of current research. Neuropsychiatr. Dis. Treat. 3, 349–364.
Ghahremani, D. G., Tabibnia, G., Monterosso, J., Hellemann, G., Poldrack, R. A., and London, E. D. (2011). Effect of modafinil on learning and task-related brain activity in methamphetamine-dependent and healthy individuals. Neuropsychopharmacology 36, 950–959. doi: 10.1038/npp.2010.233
Ghasemzadeh, M. B., Vasudevan, P., and Mueller, C. (2009). Locomotor sensitization to cocaine is associated with distinct pattern of glutamate receptor trafficking to the postsynaptic density in prefrontal cortex: early versus late withdrawal effects. Pharmacol. Biochem. Behav. 92, 383–392. doi: 10.1016/j.pbb.2008.12.004
Giancola, J. B., Bonifazi, A., Cao, J., Ku, T., Haraczy, A. J., Lam, J., et al. (2020). Structure-activity relationships for a series of (Bis(4-fluorophenyl)methyl)sulfinylethyl-aminopiperidines and -piperidine amines at the dopamine transporter: Bioisosteric replacement of the piperazine improves metabolic stability. Eur. J. Med. Chem. 208:112674. doi: 10.1016/j.ejmech.2020.112674
Gold, L. H., and Balster, R. L. (1996). Evaluation of the cocaine-like discriminative stimulus effects and reinforcing effects of modafinil. Psychopharmacology 126, 286–292. doi: 10.1007/bf02247379
Golden, S. A., and Russo, S. J. (2012). Mechanisms of psychostimulant-induced structural plasticity. Cold Spring Harbor Perspect. Med. 2:a011957. doi: 10.1101/cshperspect.a011957
Gonçalves, J., Baptista, S., Martins, T., Milhazes, N., Borges, F., Ribeiro, C. F., et al. (2010). Methamphetamine-induced neuroinflammation and neuronal dysfunction in the mice hippocampus: preventive effect of indomethacin. Eur. J. Neurosci. 31, 315–326. doi: 10.1111/j.1460-9568.2009.07059.x
González, B., Jayanthi, S., Gomez, N., Torres, O. V., Sosa, M. H., Bernardi, A., et al. (2018). Repeated methamphetamine and modafinil induce differential cognitive effects and specific histone acetylation and DNA methylation profiles in the mouse medial prefrontal cortex. Prog. Neuro Psychopharmacol. Biol. Psychiatry 82, 1–11. doi: 10.1016/j.pnpbp.2017.12.009
González, B., Raineri, M., Cadet, J. L., García-Rill, E., Urbano, F. J., and Bisagno, V. (2014). Modafinil improves methamphetamine-induced object recognition deficits and restores prefrontal cortex ERK signaling in mice. Neuropharmacology 87, 188–197. doi: 10.1016/j.neuropharm.2014.02.002
Grilly, D. M. (2000). A verification of psychostimulant-induced improvement in sustained attention in rats: effects of d-amphetamine, nicotine, and pemoline. Exp. Clin. Psychopharmacol. 8, 14–21. doi: 10.1037/1064-1297.8.1.14
Haas, H. L., Sergeeva, O. A., and Selbach, O. (2008). Histamine in the nervous system. Physiol. Rev. 88, 1183–1241.
Hamburg, M. A., and Collins, F. S. (2010). The path to personalized medicine. N. Engl. J. Med. 363, 301–304.
Han, J., Chen, D., Liu, D., and Zhu, Y. (2018). Modafinil attenuates inflammation via inhibiting Akt/NF-kappaB pathway in apoE-deficient mouse model of atherosclerosis. Inflammopharmacology 26, 385–393. doi: 10.1007/s10787-017-0387-3
Hart, C. L., Haney, M., Vosburg, S. K., Rubin, E., and Foltin, R. W. (2008). Smoked cocaine self-administration is decreased by modafinil. Neuropsychopharmacology 33, 761–768. doi: 10.1038/sj.npp.1301472
Hart, C., and Ksir, C. (1996). Nicotine effects on dopamine clearance in rat nucleus accumbens. J. Neurochem. 66, 216–221. doi: 10.1046/j.1471-4159.1996.66010216.x
Hasler, B. P., Smith, L. J., Cousins, J. C., and Bootzin, R. R. (2012). Circadian rhythms, sleep, and substance abuse. Sleep Med. Rev. 16, 67–81. doi: 10.1016/j.smrv.2011.03.004
Hedegaard, H., Miniño, A. M., and Warner, M. (2020). Drug Overdose Deaths in the United States, 1999–2018: NCHS Data Brief No 356. Hyattsville, MD: National Center for Health Statistics. Available online at: https://www.cdc.gov/nchs/products/databriefs/db356.htm
Heinzerling, K. G., Swanson, A.-N., Kim, S., Cederblom, L., Moe, A., Ling, W., et al. (2010). Randomized, double-blind, placebo-controlled trial of modafinil for the treatment of methamphetamine dependence. Drug Alcohol Depend. 109, 20–29. doi: 10.1016/j.drugalcdep.2009.11.023
Hermant, J.-F., Rambert, F. A., and Duteil, J. (1991). Awakening properties of modafinil: effect on nocturnal activity in monkeys (Macaca mulatta) after acute and repeated administration. Psychopharmacology 103, 28–32. doi: 10.1007/bf02244069
Hester, R., Lee, N., Pennay, A., Nielsen, S., and Ferris, J. (2010). The effects of modafinil treatment on neuropsychological and attentional bias performance during 7-day inpatient withdrawal from methamphetamine dependence. Exp. Clin. Psychopharmacol. 18, 489–497. doi: 10.1037/a0021791
Heyer-Osorno, R., and Juárez, J. (2020). Modafinil reduces choice impulsivity while increasing motor activity in preadolescent rats treated prenatally with alcohol. Pharmacol. Biochem. Behav. 194:172936. doi: 10.1016/j.pbb.2020.172936
Holtz, N. A., Lozama, A., Prisinzano, T. E., and Carroll, M. E. (2012). Reinstatement of methamphetamine seeking in male and female rats treated with modafinil and allopregnanolone. Drug Alcohol Depend. 120, 233–237. doi: 10.1016/j.drugalcdep.2011.07.010
Howell, L. L., and Kimmel, H. L. (2008). Monoamine transporters and psychostimulant addiction. Biochem. Pharmacol. 75, 196–217. doi: 10.1016/j.bcp.2007.08.003
Huang, Z.-L., Qu, W.-M., Li, W.-D., Mochizuki, T., Eguchi, N., Watanabe, T., et al. (2001). Arousal effect of orexin a depends on activation of the histaminergic system. Proc. Natl. Acad. Sci. U.S.A. 98, 9965–9970. doi: 10.1073/pnas.181330998
Ishizuka, T., Murakami, M., and Yamatodani, A. (2008). Involvement of central histaminergic systems in modafinil-induced but not methylphenidate-induced increases in locomotor activity in rats. Eur. J. Pharmacol. 578, 209–215. doi: 10.1016/j.ejphar.2007.09.009
Ishizuka, T., Murotani, T., and Yamatodani, A. (2010). Modanifil activates the histaminergic system through the orexinergic neurons. Neurosci. Lett. 483, 193–196. doi: 10.1016/j.neulet.2010.08.005
Ishizuka, T., Sakamoto, Y., Sakurai, T., and Yamatodani, A. (2003). Modafinil increases histamine release in the anterior hypothalamus of rats. Neurosci. Lett. 339, 143–146. doi: 10.1016/s0304-3940(03)00006-5
Ishizuka, T., Yamamoto, Y., and Yamatodani, A. (2002). The effect of orexin-a and-B on the histamine release in the anterior hypothalamus in rats. Neurosci. Lett. 323, 93–96. doi: 10.1016/s0304-3940(01)02552-6
Itzhak, Y., and Martin, J. L. (2002). Cocaine-induced conditioned place preference in mice: induction, extinction and reinstatement by related psychostimulants. Neuropsychopharmacology 26, 130–134. doi: 10.1016/s0893-133x(01)00303-7
Jasinski, D. R. (2000). An evaluation of the abuse potential of modafinil using methylphenidate as a reference. J. Psychopharmacol. 14, 53–60. doi: 10.1177/026988110001400107
Jones, B. E., Harper, S. T., and Halaris, A. E. (1977). Effects of locus coeruleus lesions upon cerebral monoamine content, sleep-wakefulness states and the response to amphetamine in the cat. Brain Res. 124, 473–496. doi: 10.1016/0006-8993(77)90948-9
Jordan, C. J., Cao, J., Newman, A. H., and Xi, Z. X. (2019). Progress in agonist therapy for substance use disorders: lessons learned from methadone and buprenorphine. Neuropharmacology 158:107609. doi: 10.1016/j.neuropharm.2019.04.015
Kahlig, K. M., and Galli, A. (2003). Regulation of dopamine transporter function and plasma membrane expression by dopamine, amphetamine, and cocaine. Eur. J. Pharmacol. 479, 153–158. doi: 10.1016/j.ejphar.2003.08.065
Kahlig, K. M., Lute, B. J., Wei, Y., Loland, C. J., Gether, U., Javitch, J. A., et al. (2006). Regulation of dopamine transporter trafficking by intracellular amphetamine. Mol. Pharmacol. 70, 542–548. doi: 10.1124/mol.106.023952
Kalechstein, A. D., De La Garza, I., and Richard, and Newton, T. F. (2010). Modafinil administration improves working memory in methamphetamine-dependent individuals who demonstrate baseline impairment. Am. J. Addict. 19, 340–344.
Kalechstein, A., Mahoney, J., Yoon, J., Bennett, R., and De La Garza Ii, R. (2013). Modafinil, but not escitalopram, improves working memory and sustained attention in long-term, high-dose cocaine users. Neuropharmacology 64, 472–478. doi: 10.1016/j.neuropharm.2012.06.064
Kalivas, P. W. (2009). The glutamate homeostasis hypothesis of addiction. Nat. Rev. Neurosci. 10, 561–572. doi: 10.1038/nrn2515
Kalivas, P. W., and Duffy, P. (1993). Time course of extracellular dopamine and behavioral sensitization to cocaine. I. dopamine axon terminals. J. Neurosci.. 13, 266–275. doi: 10.1523/jneurosci.13-01-00266.1993
Kamien, J. B., Bickel, W. K., Hughes, J. R., Higgins, S. T., and Smith, B. J. (1993). Drug discrimination by humans compared to nonhumans: current status and future directions. Psychopharmacology 111, 259–270. doi: 10.1007/bf02244940
Kampman, K. M., Lynch, K. G., Pettinati, H. M., Spratt, K., Wierzbicki, M. R., Dackis, C., et al. (2015). A double blind, placebo controlled trial of modafinil for the treatment of cocaine dependence without co-morbid alcohol dependence. Drug Alcohol Depend. 155, 105–110. doi: 10.1016/j.drugalcdep.2015.08.005
Karila, L., Weinstein, A., Aubin, H. J., Benyamina, A., Reynaud, M., and Batki, S. L. (2010). Pharmacological approaches to methamphetamine dependence: a focused review. Br. J. Clin. Pharmacol. 69, 578–592. doi: 10.1111/j.1365-2125.2010.03639.x
Kate, N., Grover, S., and Ghormode, D. (2012). Dependence on supratherapeutic doses of modafinil: a case report. Prim. Care Companion CNS Disord. 14:CC.11l01333.
Katz, J. L., and Goldberg, S. R. (1988). Preclinical assessment of abuse liability of drugs. Agents Actions 23, 18–26. doi: 10.1007/bf01967174
Kauer, J. A., and Malenka, R. C. (2007). Synaptic plasticity and addiction. Nat. Rev. Neurosci. 8, 844–858.
Kaye, S., Mcketin, R., Duflou, J., and Darke, S. (2007). Methamphetamine and cardiovascular pathology: a review of the evidence. Addiction 102, 1204–1211. doi: 10.1111/j.1360-0443.2007.01874.x
Keighron, J. D., Giancola, J. B., Shaffer, R. J., Demarco, E. M., Coggiano, M. A., Slack, R. D., et al. (2019a). Distinct effects of (R)-modafinil and its (R)-and (S)-fluoro-analogs on mesolimbic extracellular dopamine assessed by voltammetry and microdialysis in rats. Eur. J. Neurosci. 50, 2045–2053. doi: 10.1111/ejn.14256
Keighron, J. D., Quarterman, J. C., Cao, J., Demarco, E. M., Coggiano, M. A., Gleaves, A., et al. (2019b). Effects of (R)-modafinil and modafinil analogues on dopamine dynamics assessed by voltammetry and microdialysis in the mouse nucleus accumbens shell. ACS Chem. Neurosci. 10, 2012–2021. doi: 10.1021/acschemneuro.8b00340
Killgore, W. D., Kahn-Greene, E. T., Grugle, N. L., Killgore, D. B., and Balkin, T. J. (2009). Sustaining executive functions during sleep deprivation: a comparison of caffeine, dextroamphetamine, and modafinil. Sleep 32, 205–216. doi: 10.1093/sleep/32.2.205
Kjome, K. L., Lane, S. D., Schmitz, J. M., Green, C., Ma, L., Prasla, I., et al. (2010). Relationship between impulsivity and decision making in cocaine dependence. Psychiatry Res. 178, 299–304. doi: 10.1016/j.psychres.2009.11.024
Kohut, S. J., Hiranita, T., Hong, S.-K., Ebbs, A. L., Tronci, V., Green, J., et al. (2014). Preference for distinct functional conformations of the dopamine transporter alters the relationship between subjective effects of cocaine and stimulation of mesolimbic dopamine. Biol. Psychiatry 76, 802–809. doi: 10.1016/j.biopsych.2014.03.031
Koob, G. F. (1992). Dopamine, addiction and reward. Semin. Neurosci. 4, 139–148. doi: 10.1016/1044-5765(92)90012-q
Kousik, S. M., Napier, T. C., and Carvey, P. M. (2012). The effects of psychostimulant drugs on blood brain barrier function and neuroinflammation. Front. Pharmacol. 3:121.
Krishnan, R., and Chary, K. V. (2015). A rare case modafinil dependence. J. Pharmacol. Pharmacother. 6, 49–50. doi: 10.4103/0976-500x.149149
Kuhar, M., Ritz, M., and Boja, J. (1991). The dopamine hypothesis of the reinforcing properties of cocaine. Trends Neurosci. 14, 299–302. doi: 10.1016/0166-2236(91)90141-g
Kumar, R. (2008). Approved and investigational uses of modafinil. Drugs 68, 1803–1839. doi: 10.2165/00003495-200868130-00003
Lai, Y.-T., Tsai, Y.-P. N., Cherng, C. G., Ke, J.-J., Ho, M.-C., Tsai, C.-W., et al. (2009). Lipopolysaccharide mitagates methamphetamine-induced striatal dopamine depletion via modulating local TNF-α and dopamine transporter expression. J. Neural. Transm. 116, 405–415. doi: 10.1007/s00702-009-0204-2
Lange, R. A., and Hillis, L. D. (2001). Cardiovascular complications of cocaine use. N. Engl. J. Med. 345, 351–358.
Laviolette, S. R., and Van Der Kooy, D. (2004). The neurobiology of nicotine addiction: bridging the gap from molecules to behaviour. Nat. Rev. Neurosci. 5, 55–65. doi: 10.1038/nrn1298
Lazenka, M. F., and Negus, S. S. (2017). Oral modafinil facilitates intracranial self-stimulation in rats: comparison to methylphenidate. Behav. Pharmacol. 28, 318–322. doi: 10.1097/fbp.0000000000000288
Lee, N., Pennay, A., Hester, R., Mcketin, R., Nielsen, S., and Ferris, J. (2013). A pilot randomised controlled trial of modafinil during acute methamphetamine withdrawal: feasibility, tolerability and clinical outcomes. Drug Alcohol Rev. 32, 88–95. doi: 10.1111/j.1465-3362.2012.00473.x
Lee, Y. W., Hennig, B., Fiala, M., Kim, K. S., and Toborek, M. (2001). Cocaine activates redox-regulated transcription factors and induces TNF-α expression in human brain endothelial cells. Brain Res. 920, 125–133. doi: 10.1016/s0006-8993(01)03047-5
Little, K. Y., Elmer, L. W., Zhong, H., Scheys, J. O., and Zhang, L. (2002). Cocaine induction of dopamine transporter trafficking to the plasma membrane. Mol. Pharmacol. 61, 436–445. doi: 10.1124/mol.61.2.436
Liu, J., Wu, R., and Li, J.-X. (2020). TAAR1 and psychostimulant addiction. Cell Mol. Neurobiol. 40, 229–238. doi: 10.1007/s10571-020-00792-8
Liu, X., Petit, J.-M., Ezan, P., Gyger, J., Magistretti, P., and Giaume, C. (2013). The psychostimulant modafinil enhances gap junctional communication in cortical astrocytes. Neuropharmacology 75, 533–538. doi: 10.1016/j.neuropharm.2013.04.019
Liu, Y., Le Foll, B., Liu, Y., Wang, X., and Lu, L. (2008). Conditioned place preference induced by licit drugs: establishment, extinction, and reinstatement. Sci. World J. 8, 1228–1245. doi: 10.1100/tsw.2008.154
Loland, C. J., Mereu, M., Okunola, O. M., Cao, J., Prisinzano, T. E., Mazier, S., et al. (2012). R-modafinil (armodafinil): a unique dopamine uptake inhibitor and potential medication for psychostimulant abuse. Biol. Psychiatry 72, 405–413. doi: 10.1016/j.biopsych.2012.03.022
Luscher, C., and Malenka, R. C. (2011). Drug-evoked synaptic plasticity in addiction: from molecular changes to circuit remodeling. Neuron 69, 650–663. doi: 10.1016/j.neuron.2011.01.017
Madras, B. K., Xie, Z., Lin, Z., Jassen, A., Panas, H., Lynch, L., et al. (2006). Modafinil occupies dopamine and norepinephrine transporters in vivo and modulates the transporters and trace amine activity in vitro. J. Pharmacol. Exp. Ther. 319, 561–569. doi: 10.1124/jpet.106.106583
Mahfoud, Y., Talih, F., Streem, D., and Budur, K. (2009). Sleep disorders in substance abusers: how common are they? Psychiatry 6, 38–42.
Mahler, S. V., Hensley-Simon, M., Tahsili-Fahadan, P., Lalumiere, R. T., Thomas, C., Fallon, R. V., et al. (2014). Modafinil attenuates reinstatement of cocaine seeking: role for cystine–glutamate exchange and metabotropic glutamate receptors. Addict. Biol. 19, 49–60. doi: 10.1111/j.1369-1600.2012.00506.x
Mahoney, J. J., Jackson, B. J., Kalechstein, A. D., De La Garza, R., Chang, L. C., and Newton, T. F. (2012). Acute modafinil exposure reduces daytime sleepiness in abstinent methamphetamine-dependent volunteers. Int. J. Neuropsychopharmacol. 15, 1241–1249. doi: 10.1017/s1461145711001805
Makris, A. P., Rush, C. R., Frederich, R. C., and Kelly, T. H. (2004). Wake-promoting agents with different mechanisms of action: comparison of effects of modafinil and amphetamine on food intake and cardiovascular activity. Appetite 42, 185–195. doi: 10.1016/j.appet.2003.11.003
Malcolm, R., Swayngim, K., Donovan, J. L., Devane, C. L., Elkashef, A., Chiang, N., et al. (2006). Modafinil and cocaine interactions. Am. J. Drug Alcohol Abuse 32, 577–587. doi: 10.1016/b978-0-12-803750-8.00058-0
Marston, H. M., Reid, M. E., Lawrence, J. A., Olverman, H. J., and Butcher, S. P. (1999). Behavioural analysis of the acute and chronic effects of MDMA treatment in the rat. Psychopharmacology 144, 67–76. doi: 10.1007/s002130050978
McElhiney, M. C., Rabkin, J. G., Rabkin, R., and Nunes, E. V. (2009). Provigil (modafinil) plus cognitive behavioral therapy for methamphetamine use in HIV+ gay men: a pilot study. Am. J. Drug Alcohol Abuse 35, 34–37. doi: 10.1080/00952990802342907
McGaugh, J., Mancino, M. J., Feldman, Z., Chopra, M. P., Gentry, W. B., Cargile, C., et al. (2009). Open label pilot study of modafinil for methamphetamine dependence. J. Clin. Psychopharmacol. 29, 488–491. doi: 10.1097/jcp.0b013e3181b591e0
Meldrum, B. S. (2000). Glutamate as a neurotransmitter in the brain: review of physiology and pathology. J. Nutr. 130, 1007S–1015S.
Mereu, M., Bonci, A., Newman, A. H., and Tanda, G. (2013). The neurobiology of modafinil as an enhancer of cognitive performance and a potential treatment for substance use disorders. Psychopharmacology 229, 415–434. doi: 10.1007/s00213-013-3232-4
Mereu, M., Chun, L. E., Prisinzano, T. E., Newman, A. H., Katz, J. L., and Tanda, G. (2017). The unique psychostimulant profile of (±)-modafinil: investigation of behavioral and neurochemical effects in mice. Eur. J. Neurosci. 45, 167–174. doi: 10.1111/ejn.13376
Mereu, M., Hiranita, T., Jordan, C. J., Chun, L. E., Lopez, J. P., Coggiano, M. A., et al. (2020). Modafinil potentiates cocaine self-administration by a dopamine-independent mechanism: possible involvement of gap junctions. Neuropsychopharmacology 45, 1518–1526. doi: 10.1038/s41386-020-0680-5
Mereu, M., Tronci, V., Chun, L. E., Thomas, A. M., Green, J. L., Katz, J. L., et al. (2015). Cocaine-induced endocannabinoid release modulates behavioral and neurochemical sensitization in mice. Addict. Biol. 20, 91–103. doi: 10.1111/adb.12080
Mignot, E., Nishino, S., Guilleminault, C., and Dement, W. (1994). Modafinil binds to the dopamine uptake carrier site with low affinity. Sleep 17, 436–437. doi: 10.1093/sleep/17.5.436
Minzenberg, M. J., and Carter, C. S. (2008). Modafinil: a review of neurochemical actions and effects on cognition. Neuropsychopharmacology 33, 1477–1502. doi: 10.1038/sj.npp.1301534
Mitchell, H. A., and Weinshenker, D. (2010). Good night and good luck: norepinephrine in sleep pharmacology. Biochem. Pharmacol. 79, 801–809. doi: 10.1016/j.bcp.2009.10.004
Monti, J. M. (2013). The neurotransmitters of sleep and wake, a physiological reviews series. Sleep Med. Rev. 17, 313–315. doi: 10.1016/j.smrv.2013.02.004
Moosavi, S. M., Yazdani-Charati, J., and Amini, F. (2019). Effects of modafinil on sleep pattern during methamphetamine withdrawal: a double-blind randomized controlled trial. Addict. Health 11, 165–172.
Morgan, P. T., Angarita, G. A., Canavan, S., Pittman, B., Oberleitner, L., Malison, R. T., et al. (2016). Modafinil and sleep architecture in an inpatient–outpatient treatment study of cocaine dependence. Drug Alcohol Depend. 160, 49–56. doi: 10.1016/j.drugalcdep.2015.12.004
Morgan, P. T., Pace-Schott, E., Pittman, B., Stickgold, R., and Malison, R. T. (2010). Normalizing effects of modafinil on sleep in chronic cocaine users. Am. J. Psychiatry 167, 331–340. doi: 10.1176/appi.ajp.2009.09050613
Morgan, P. T., Paliwal, P., Malison, R. T., and Sinha, R. (2009). Sex differences in sleep and sleep-dependent learning in abstinent cocaine users. Pharmacol. Biochem. Behav. 93, 54–58. doi: 10.1016/j.pbb.2009.04.006
Morgan, R. E., Crowley, J. M., Smith, R. H., Laroche, R. B., and Dopheide, M. M. (2007). Modafinil improves attention, inhibitory control, and reaction time in healthy, middle-aged rats. Pharmacol. Biochem. Behav. 86, 531–541. doi: 10.1016/j.pbb.2007.01.015
Mortensen, O. V., and Amara, S. G. (2003). Dynamic regulation of the dopamine transporter. Eur. J. Pharmacol 479, 159–170.
Mueller, D., and Stewart, J. (2000). Cocaine-induced conditioned place preference: reinstatement by priming injections of cocaine after extinction. Behav. Brain Res. 115, 39–47. doi: 10.1016/s0166-4328(00)00239-4
Müller, U., Steffenhagen, N., Regenthal, R., and Bublak, P. (2004). Effects of modafinil on working memory processes in humans. Psychopharmacology 177, 161–169. doi: 10.1007/s00213-004-1926-3
Munzar, P., Tanda, G., Justinova, Z., and Goldberg, S. R. (2004). Histamine h3 receptor antagonists potentiate methamphetamine self-administration and methamphetamine-induced accumbal dopamine release. Neuropsychopharmacology 29, 705–717. doi: 10.1038/sj.npp.1300380
Murillo-Rodríguez, E., Haro, R., Palomero-Rivero, M., Millán-Aldaco, D., and Drucker-Colín, R. (2007). Modafinil enhances extracellular levels of dopamine in the nucleus accumbens and increases wakefulness in rats. Behav. Brain Res. 176, 353–357. doi: 10.1016/j.bbr.2006.10.016
Myrick, H., Malcolm, R., Taylor, B., and Larowe, S. (2004). Modafinil: preclinical, clinical, and post-marketing surveillance—a review of abuse liability issues. Ann. Clin. Psychiatry 16, 101–109. doi: 10.1080/10401230490453743
Napier, T. C., Herrold, A. A., and De Wit, H. (2013). Using conditioned place preference to identify relapse prevention medications. Neurosci. Biobehav. Rev. 37, 2081–2086. doi: 10.1016/j.neubiorev.2013.05.002
Nath, A., Hauser, K. F., Wojna, V., Booze, R. M., Maragos, W., Prendergast, M., et al. (2002). Molecular basis for interactions of HIV and drugs of abuse. J. Acquir. Immune Defic. Syndr. 31, S62–S69.
Negus, S. S., and Miller, L. L. (2014). Intracranial self-stimulation to evaluate abuse potential of drugs. Pharmacol. Rev. 66, 869–917. doi: 10.1124/pr.112.007419
Newman, A. H., Cao, J., Keighron, J. D., Jordan, C. J., Bi, G.-H., Liang, Y., et al. (2019). Translating the atypical dopamine uptake inhibitor hypothesis toward therapeutics for treatment of psychostimulant use disorders. Neuropsychopharmacology 44, 1435–1444. doi: 10.1038/s41386-019-0366-z
Newman, A. H., Ku, T., Jordan, C. J., Bonifazi, A., and Xi, Z. X. (2021). New drugs, old targets: tweaking the dopamine system to treat psychostimulant use disorders. Annu. Rev. Pharmacol. Toxicol. 61, 609–628. doi: 10.1146/annurev-pharmtox-030220-124205
Newman, J. L., Negus, S. S., Lozama, A., Prisinzano, T. E., and Mello, N. K. (2010). Behavioral evaluation of modafinil and the abuse-related effects of cocaine in rhesus monkeys. Exp. Clin. Psychopharmacol. 18, 395–408. doi: 10.1037/a0021042
Nguyen, T. L., Tian, Y. H., You, I. J., Lee, S. Y., and Jang, C. G. (2011). Modafinil-induced conditioned place preference via dopaminergic system in mice. Synapse 65, 733–741. doi: 10.1002/syn.20892
Nisell, M., Nomikos, G. G., and Svensson, T. H. (1994). Systemic nicotine-induced dopamine release in the rat nucleus accumbens is regulated by nicotinic receptors in the ventral tegmental area. Synapse 16, 36–44. doi: 10.1002/syn.890160105
Nishino, S., and Mignot, E. (1997). Pharmacological aspects of human and canine narcolepsy. Prog. Neurobiol. 52, 27–78. doi: 10.1016/s0301-0082(96)00070-6
Nishino, S., Mao, J., Sampathkumaran, R., Shelton, J., and Mignot, E. (1998). Increased dopaminergic transmission mediates the wake-promoting effects of CNS stimulants. Sleep Res Online 1, 49–61.
Nordahl, T. E., Salo, R., and Leamon, M. (2003). Neuropsychological effects of chronic methamphetamine use on neurotransmitters and cognition: a review. J. Neuropsychiatry Clin. Neurosci. 15, 317–325. doi: 10.1176/jnp.15.3.317
Nuijten, M., Blanken, P., Van Den Brink, W., and Hendriks, V. (2015). Modafinil in the treatment of crack-cocaine dependence in the Netherlands: results of an open-label randomised controlled feasibility trial. J. Psychopharmacol. 29, 678–687. doi: 10.1177/0269881115582151
Nuijten, M., Blanken, P., Van Den Brink, W., Goudriaan, A. E., and Hendriks, V. M. (2016). Impulsivity and attentional bias as predictors of modafinil treatment outcome for retention and drug use in crack-cocaine dependent patients: results of a randomised controlled trial. J. Psychopharmacol. 30, 616–626. doi: 10.1177/0269881116645268
Okunola-Bakare, O. M., Cao, J., Kopajtic, T., Katz, J. L., Loland, C. J., Shi, L., et al. (2014). Elucidation of structural elements for selectivity across monoamine transporters: novel 2-[(diphenylmethyl)sulfinyl]acetamide (modafinil) analogues. J. Med. Chem. 57, 1000–1013. doi: 10.1021/jm401754x
Ozturk, A., and Deveci, E. (2014). Drug abuse of modafinil by a cannabis user. Bull. Clin. Psychopharmacol. 24, 405–407. doi: 10.5455/bcp.20130624013303
Paterson, N. E., Fedolak, A., Olivier, B., Hanania, T., Ghavami, A., and Caldarone, B. (2010). Psychostimulant-like discriminative stimulus and locomotor sensitization properties of the wake-promoting agent modafinil in rodents. Pharmacol. Biochem. Behav. 95, 449–456. doi: 10.1016/j.pbb.2010.03.006
Peacock, A., Leung, J., Larney, S., Colledge, S., Hickman, M., Rehm, J., et al. (2018). Global statistics on alcohol, tobacco and illicit drug use: 2017 status report. Addiction 113, 1905–1926. doi: 10.1111/add.14234
Peñaloza, R. A., Sarkar, U., Claman, D. M., and Omachi, T. A. (2013). Trends in on-label and off-label modafinil use in a nationally representative sample. JAMA Internal Med. 173, 704–706. doi: 10.1001/jamainternmed.2013.2807
Pettit, H. O., and Justice, J. B. (1989). Dopamine in the nucleus accumbens during cocaine self-administration as studied by in vivo microdialysis. Pharmacol. Biochem. Behav. 34, 899–904. doi: 10.1016/0091-3057(89)90291-8
Phillips, K. A., Epstein, D. H., and Preston, K. L. (2014). Psychostimulant addiction treatment. Neuropharmacology 87, 150–160. doi: 10.1016/j.neuropharm.2014.04.002
Picciotto, M. R., Zoli, M., Rimondini, R., Léna, C., Marubio, L. M., Pich, E. M., et al. (1998). Acetylcholine receptors containing the β2 subunit are involved in the reinforcing properties of nicotine. Nature 391, 173–177. doi: 10.1038/34413
Piérard, C., Liscia, P., Valleau, M., Drouet, I., Chauveau, F., Huart, B., et al. (2006). Modafinil-induced modulation of working memory and plasma corticosterone in chronically-stressed mice. Pharmacol. Biochem. Behav. 83, 1–8. doi: 10.1016/j.pbb.2005.11.018
Pigeau, R., Naitoh, P., Buguet, A., Mccann, C., Baranski, J., Taylor, M., et al. (1995). Modafinil, d-amphetamine and placebo during 64 hours of sustained mental work. I. effects on mood, fatigue, cognitive performance and body temperature. J. Sleep Res. 4, 212–228. doi: 10.1111/j.1365-2869.1995.tb00172.x
Pontieri, F. E., Tanda, G., and Di Chiara, G. (1995). Intravenous cocaine, morphine, and amphetamine preferentially increase extracellular dopamine in the “shell” as compared with the “core” of the rat nucleus accumbens. Proc. Natl. Acad. Sci. U.S.A. 92, 12304–12308. doi: 10.1073/pnas.92.26.12304
Pontieri, F. E., Tanda, G., Orzi, F., and Di Chiara, G. (1996). Effects of nicotine on the nucleus accumbens and similarity to those of addictive drugs. Nature 382, 255–257. doi: 10.1038/382255a0
Porter, J. H., Prus, A. J., and Overton, D. A. (2018). Drug discrimination: historical origins, important concepts, and principles. Curr. Top. Behav. Neurosci. 39, 3–26. doi: 10.1007/7854_2018_40
Quisenberry, A. J., Prisinzano, T. E., and Baker, L. E. (2013). Modafinil alone and in combination with low dose amphetamine does not establish conditioned place preference in male sprague-dawley rats. Exp. Clin. Psychopharmacol. 21, 252–258. doi: 10.1037/a0031832
Raineri, M., Gonzalez, B., Goitia, B., Garcia-Rill, E., Krasnova, I. N., Cadet, J. L., et al. (2012). Modafinil abrogates methamphetamine-induced neuroinflammation and apoptotic effects in the mouse striatum. PLoS One 7:e46599. doi: 10.1371/journal.pone.0046599
Rao, Y., Liu, Z. W., Borok, E., Rabenstein, R. L., Shanabrough, M., Lu, M., et al. (2007). Prolonged wakefulness induces experience-dependent synaptic plasticity in mouse hypocretin/orexin neurons. J. Clin. Invest. 117, 4022–4033. doi: 10.1172/jci32829
Redrobe, J. P., Bull, S., and Plath, N. (2010). Translational aspects of the novel object recognition task in rats abstinent following sub-chronic treatment with phencyclidine (PCP): effects of modafinil and relevance to cognitive deficits in schizophrenia. Front. Psychiatry 1:146.
Reichel, C. M., and See, R. E. (2010). Modafinil effects on reinstatement of methamphetamine seeking in a rat model of relapse. Psychopharmacology 210, 337–346. doi: 10.1007/s00213-010-1828-5
Reichel, C. M., and See, R. E. (2012). Chronic modafinil effects on drug-seeking following methamphetamine self-administration in rats. Int. J. Neuropsychopharmacol. 15, 919–929. doi: 10.1017/s1461145711000988
Reichel, C. M., Gilstrap, M. G., Ramsey, L. A., and See, R. E. (2014). Modafinil restores methamphetamine induced object-in-place memory deficits in rats independent of glutamate N-methyl-d-aspartate receptor expression. Drug Alcohol Depend. 134, 115–122. doi: 10.1016/j.drugalcdep.2013.09.018
Revel, F., Moreau, J., Pouzet, B., Mory, R., Bradaia, A., Buchy, D., et al. (2013). A new perspective for schizophrenia: TAAR1 agonists reveal antipsychotic-and antidepressant-like activity, improve cognition and control body weight. Mol. Psychiatry 18, 543–556. doi: 10.1038/mp.2012.57
Robertson, P., and Hellriegel, E. T. (2003). Clinical pharmacokinetic profile of modafinil. Clin. Pharmacokinet. 42, 123–137. doi: 10.2165/00003088-200342020-00002
Robertson, P., Decory, H. H., Madan, A., and Parkinson, A. (2000). In vitro inhibition and induction of human hepatic cytochrome P450 enzymes by modafinil. Drug Metab. Dispos. 28, 664–671.
Robinson, T. E., and Kolb, B. (2004). Structural plasticity associated with exposure to drugs of abuse. Neuropharmacology 47, 33–46. doi: 10.1016/j.neuropharm.2004.06.025
Rogers, J., De Santis, S., and See, R. (2008). Extended methamphetamine self-administration enhances reinstatement of drug seeking and impairs novel object recognition in rats. Psychopharmacology 199, 615–624. doi: 10.1007/s00213-008-1187-7
Rounsaville, B. J., Anton, S. F., Carroll, K., Budde, D., Prusoff, B. A., and Gawin, F. (1991). Psychiatric diagnoses of treatment-seeking cocaine abusers. Arch. Gen. Psychiatry 48, 43–51. doi: 10.1001/archpsyc.1991.01810250045005
Rowley, H. L., Kulkarni, R. S., Gosden, J., Brammer, R. J., Hackett, D., and Heal, D. J. (2014). Differences in the neurochemical and behavioural profiles of lisdexamfetamine methylphenidate and modafinil revealed by simultaneous dual-probe microdialysis and locomotor activity measurements in freely-moving rats. J. Psychopharmacol. 28, 254–269. doi: 10.1177/0269881113513850
Rush, C. R., Kelly, T. H., Hays, L. R., Baker, R., and Wooten, A. (2002a). Acute behavioral and physiological effects of modafinil in drug abusers. Behav. Pharmacol. 13, 105–115. doi: 10.1097/00008877-200203000-00002
Rush, C. R., Kelly, T. H., Hays, L. R., and Wooten, A. F. (2002b). Discriminative-stimulus effects of modafinil in cocaine-trained humans. Drug Alcohol Depend. 67, 311–322.
Russo, S. J., Mazei-Robison, M. S., Ables, J. L., and Nestler, E. J. (2009). Neurotrophic factors and structural plasticity in addiction. Neuropharmacology 56, 73–82.
Sahakian, B. J., and Morein-Zamir, S. (2011). Neuroethical issues in cognitive enhancement. J. Psychopharmacol. 25, 197–204. doi: 10.1177/0269881109106926
Sahakian, B., Robbins, T., Morgan, M., and Iversen, S. (1975). The effects of psychomotor stimulants on stereotypy and locomotor activity in socially-deprived and control rats. Brain Res. 84, 195–205. doi: 10.1016/0006-8993(75)90975-0
Sakurai, T. (2007). The neural circuit of orexin (hypocretin): maintaining sleep and wakefulness. Nat. Rev. Neurosci. 8, 171–181. doi: 10.1038/nrn2092
Salamone, J. D., Correa, M., Mingote, S., and Weber, S. M. (2003). Nucleus accumbens dopamine and the regulation of effort in food-seeking behavior: implications for studies of natural motivation, psychiatry, and drug abuse. J. Pharmacol. Exp. Ther. 305, 1–8. doi: 10.1124/jpet.102.035063
Salo, R., Flower, K., Kielstein, A., Leamon, M. H., Nordahl, T. E., and Galloway, G. P. (2011). Psychiatric comorbidity in methamphetamine dependence. Psychiatry Res. 186, 356–361. doi: 10.1016/j.psychres.2010.09.014
SAMHSA (2018). Mental Health Indicators in the United States: Results from the 2018 National Survey on Drug Use and Health. Rockville, MD: SAMHSA.
Sandoval, V., Riddle, E. L., Ugarte, Y. V., Hanson, G. R., and Fleckenstein, A. E. (2001). Methamphetamine-induced rapid and reversible changes in dopamine transporter function: an in vitro model. J. Neurosci. 21, 1413–1419. doi: 10.1523/jneurosci.21-04-01413.2001
Sarnyai, Z., Bíró, É, Gardi, J., Vecsernyés, M., Julesz, J., and Telegdy, G. (1995). Brain corticotropin-releasing factor mediates ‘anxiety-like’behavior induced by cocaine withdrawal in rats. Brain Res. 675, 89–97. doi: 10.1016/0006-8993(95)00043-p
Saunders, C., Ferrer, J. V., Shi, L., Chen, J., Merrill, G., Lamb, M. E., et al. (2000). Amphetamine-induced loss of human dopamine transporter activity: an internalization-dependent and cocaine-sensitive mechanism. Proc. Natl. Acad. Sci. U.S.A. 97, 6850–6855. doi: 10.1073/pnas.110035297
Sawaguchi, T., and Goldman-Rakic, P. S. (1991). D1 dopamine receptors in prefrontal cortex: involvement in working memory. Science 251, 947–950. doi: 10.1126/science.1825731
Sawaguchi, T., and Goldman-Rakic, P. S. (1994). The role of D1-dopamine receptor in working memory: local injections of dopamine antagonists into the prefrontal cortex of rhesus monkeys performing an oculomotor delayed-response task. J. Neurophysiol. 71, 515–528. doi: 10.1152/jn.1994.71.2.515
Scammell, T. E., Estabrooke, I. V., Mccarthy, M. T., Chemelli, R. M., Yanagisawa, M., Miller, M. S., et al. (2000). Hypothalamic arousal regions are activated during modafinil-induced wakefulness. J. Neurosci. 20, 8620–8628. doi: 10.1523/jneurosci.20-22-08620.2000
Schierenbeck, T., Riemann, D., Berger, M., and Hornyak, M. (2008). Effect of illicit recreational drugs upon sleep: cocaine, ecstasy and marijuana. Sleep Med. Rev. 12, 381–389. doi: 10.1016/j.smrv.2007.12.004
Schmaal, L., Goudriaan, A., Joos, L., Dom, G., Pattij, T., Van Den Brink, W., et al. (2014). Neural substrates of impulsive decision making modulated by modafinil in alcohol-dependent patients. Psychol. Med. 44, 2787–2798. doi: 10.1017/s0033291714000312
Schmaal, L., Joos, L., Koeleman, M., Veltman, D. J., Van Den Brink, W., and Goudriaan, A. E. (2013). Effects of modafinil on neural correlates of response inhibition in alcohol-dependent patients. Biol. Psychiatry 73, 211–218. doi: 10.1016/j.biopsych.2012.06.032
Schmitt, K. C., and Reith, M. E. (2011). The atypical stimulant and nootropic modafinil interacts with the dopamine transporter in a different manner than classical cocaine-like inhibitors. PLoS One 6:e25790. doi: 10.1371/journal.pone.0025790
Schmitz, J. M., Green, C. E., Stotts, A. L., Lindsay, J. A., Rathnayaka, N. S., Grabowski, J., et al. (2014). A two-phased screening paradigm for evaluating candidate medications for cocaine cessation or relapse prevention: Modafinil, Levodopa–Carbidopa, Naltrexone. Drug Alcohol Depend. 136, 100–107. doi: 10.1016/j.drugalcdep.2013.12.015
Schnoll, R. A., Wileyto, E. P., Pinto, A., Leone, F., Gariti, P., Siegel, S., et al. (2008). A placebo-controlled trial of modafinil for nicotine dependence. Drug Alcohol Depend. 98, 86–93. doi: 10.1016/j.drugalcdep.2008.04.008
Schork, N. J. (2015). Personalized medicine: time for one-person trials. Nature 520, 609–611. doi: 10.1038/520609a
Schwartz, J. R. (2005). Modafinil: new indications for wake promotion. Expert Opin. Pharmacother. 6, 115–129. doi: 10.1517/14656566.6.1.115
Schwartz, M. D., Palmerston, J. B., Lee, D. L., Hoener, M. C., and Kilduff, T. S. (2018). Deletion of trace amine-associated receptor 1 attenuates behavioral responses to caffeine. Front. Pharmacol. 9:35.
Shanmugasundaram, B., Aher, Y. D., Aradska, J., Ilic, M., Daba Feyissa, D., Kalaba, P., et al. (2017). R-Modafinil exerts weak effects on spatial memory acquisition and dentate gyrus synaptic plasticity. PLoS One 12:e0179675. doi: 10.1371/journal.pone.0179675
Sharif, S., Guirguis, A., Fergus, S., and Schifano, F. (2021). The use and impact of cognitive enhancers among university students: a systematic review. Brain Sci. 11:355. doi: 10.3390/brainsci11030355
Shearer, J., Darke, S., Rodgers, C., Slade, T., Van Beek, I., Lewis, J., et al. (2009). A double-blind, placebo-controlled trial of modafinil (200 mg/day) for methamphetamine dependence. Addiction 104, 224–233. doi: 10.1111/j.1360-0443.2008.02437.x
Shuman, T., Cai, D. J., Sage, J. R., and Anagnostaras, S. G. (2012). Interactions between modafinil and cocaine during the induction of conditioned place preference and locomotor sensitization in mice: implications for addiction. Behav. Brain Res. 235, 105–112. doi: 10.1016/j.bbr.2012.07.039
Shuman, T., Wood, S. C., and Anagnostaras, S. G. (2009). Modafinil and memory: effects of modafinil on Morris water maze learning and Pavlovian fear conditioning. Behav. Neurosci. 123, 257–266. doi: 10.1037/a0014366
Slack, R. D., Ku, T. C., Cao, J., Giancola, J. B., Bonifazi, A., Loland, C. J., et al. (2020). Structure-activity relationships for a series of (Bis(4-fluorophenyl)methyl)sulfinyl Alkyl Alicyclic Amines at the dopamine transporter: functionalizing the terminal Nitrogen affects affinity, selectivity, and metabolic stability. J. Med. Chem. 63, 2343–2357. doi: 10.1021/acs.jmedchem.9b01188
Sofuoglu, M., Devito, E. E., Waters, A. J., and Carroll, K. M. (2013). Cognitive enhancement as a treatment for drug addictions. Neuropharmacology 64, 452–463. doi: 10.1016/j.neuropharm.2012.06.021
Sofuoglu, M., Devito, E. E., Waters, A. J., and Carroll, K. M. (2016). Cognitive function as a transdiagnostic treatment target in stimulant use disorders. J. Dual Diagnosis 12, 90–106. doi: 10.1080/15504263.2016.1146383
Spencer, T. J., Madras, B. K., Bonab, A. A., Dougherty, D. D., Clarke, A., Mirto, T., et al. (2010). A positron emission tomography study examining the dopaminergic activity of armodafinil in adults using [11C] altropane and [11C] raclopride. Biol. Psychiatry 68, 964–970. doi: 10.1016/j.biopsych.2010.08.026
Stoops, W. W., Lile, J. A., Fillmore, M. T., Glaser, P. E., and Rush, C. R. (2005). Reinforcing effects of modafinil: influence of dose and behavioral demands following drug administration. Psychopharmacology 182, 186–193. doi: 10.1007/s00213-005-0044-1
Sulzer, D., Sonders, M. S., Poulsen, N. W., and Galli, A. (2005). Mechanisms of neurotransmitter release by amphetamines: a review. Prog. Neurobiol. 75, 406–433. doi: 10.1016/j.pneurobio.2005.04.003
Taber, K. H., Black, D. N., Porrino, L. J., and Hurley, R. A. (2012). Neuroanatomy of dopamine: reward and addiction. J. Neuropsychiatry Clin. Neurosci. 24, 1–4. doi: 10.1176/appi.neuropsych.24.1.1
Tahsili-Fahadan, P., Carr, G. V., Harris, G. C., and Aston-Jones, G. (2010). Modafinil blocks reinstatement of extinguished opiate-seeking in rats: mediation by a glutamate mechanism. Neuropsychopharmacology 35, 2203–2210. doi: 10.1038/npp.2010.94
Tanda, G., Hersey, M., Hempel, B., Xi, Z.-X., and Newman, A. H. (2021). Modafinil and its structural analogs as atypical dopamine uptake inhibitors and potential medications for psychostimulant use disorder. Curr. Opin. Pharmacol. 56, 13–21. doi: 10.1016/j.coph.2020.07.007
Tanda, G., Newman, A. H., Ebbs, A. L., Tronci, V., Green, J. L., Tallarida, R. J., et al. (2009). Combinations of cocaine with other dopamine uptake inhibitors: assessment of additivity. J. Pharmacol. Exp. Ther. 330, 802–809. doi: 10.1124/jpet.109.154302
Tanda, G., Pontieri, F. E., and Di Chiara, G. (1997a). Cannabinoid and heroin activation of mesolimbic dopamine transmission by a common mu1 opioid receptor mechanism. Science 276, 2048–2050. doi: 10.1126/science.276.5321.2048
Tanda, G., Pontieri, F. E., Frau, R., and Di Chiara, G. (1997b). Contribution of blockade of the noradrenaline carrier to the increase of extracellular dopamine in the rat prefrontal cortex by amphetamine and cocaine. Eur. J. Neurosci. 9, 2077–2085. doi: 10.1111/j.1460-9568.1997.tb01375.x
Thomas, M., Kalivas, P., and Shaham, Y. (2008). Neuroplasticity in the mesolimbic dopamine system and cocaine addiction. Br. J. Pharmacol. 154, 327–342. doi: 10.1038/bjp.2008.77
Torrens, M., and Rossi, P. (2015). “Mood disorders and addiction,” in Co-occurring Addictive and Psychiatric Disorders, eds F. Moggi and G. Dom (Berlin: Springer), 103–117. doi: 10.1007/978-3-642-45375-5_8
Touret, M., Sallanon-Moulin, M., Fages, C., Roudier, V., Didier-Bazes, M., Roussel, B., et al. (1994). Effects of modafinil-induced wakefulness on glutamine synthetase regulation in the rat brain. Mol. Brain Res. 26, 123–128. doi: 10.1016/0169-328x(94)90082-5
Trulson, M. E. (1985). Simultaneous recording of substantia nigra neurons and voltammetric release of dopamine in the caudate of behaving cats. Brain Res. Bull. 15, 221–223. doi: 10.1016/0361-9230(85)90140-6
Tseng, K. Y., and O’Donnell, P. (2004). Dopamine–glutamate interactions controlling prefrontal cortical pyramidal cell excitability involve multiple signaling mechanisms. J. Neurosci. 24, 5131–5139. doi: 10.1523/jneurosci.1021-04.2004
Tseng, K. Y., and O’Donnell, P. (2007). D2 dopamine receptors recruit a GABA component for their attenuation of excitatory synaptic transmission in the adult rat prefrontal cortex. Synapse 61, 843–850. doi: 10.1002/syn.20432
Tunstall, B. J., Ho, C. P., Cao, J., Vendruscolo, J. C., Schmeichel, B. E., Slack, R. D., et al. (2018). Atypical dopamine transporter inhibitors attenuate compulsive-like methamphetamine self-administration in rats. Neuropharmacology 131, 96–103. doi: 10.1016/j.neuropharm.2017.12.006
Turner, C., Belyavin, A., and Nicholson, A. (2014). Duration of activity and mode of action of modafinil: Studies on sleep and wakefulness in humans. J. Psychopharmacol. 28, 643–654. doi: 10.1177/0269881113508173
Turner, D. C., Robbins, T. W., Clark, L., Aron, A. R., Dowson, J., and Sahakian, B. J. (2003). Cognitive enhancing effects of modafinil in healthy volunteers. Psychopharmacology 165, 260–269. doi: 10.1007/s00213-002-1250-8
Tzschentke, T. M. (2007). Review on CPP: measuring reward with the conditioned place preference (CPP) paradigm: update of the last decade. Addict. Biol. 12, 227–462. doi: 10.1111/j.1369-1600.2007.00070.x
Urbano, F. J., Leznik, E., and Llinás, R. R. (2007). Modafinil enhances thalamocortical activity by increasing neuronal electrotonic coupling. Proc. Natl. Acad. Sci. U.S.A. 104, 12554–12559. doi: 10.1073/pnas.0705087104
US Modafinil in Narcolepsy Multicenter Study Group (1998). Randomized trial of modafinil for the treatment of pathological somnolence in narcolepsy. Ann. Neurol. 43, 88–97. doi: 10.1002/ana.410430115
US Modafinil in Narcolepsy Multicenter Study Group (2000). Randomized trial of modafinil as a treatment for the excessive daytime somnolence of narcolepsy. Neurology 54, 1166–1175. doi: 10.1212/wnl.54.5.1166
Valentino, R. J., and Volkow, N. D. (2020). Drugs, sleep, and the addicted brain. Neuropsychopharmacology 45, 3–5. doi: 10.1038/s41386-019-0465-x
Velázquez-Sánchez, C., Santos, J. W., Smith, K. L., Ferragud, A., Sabino, V., and Cottone, P. (2015). Seeking behavior, place conditioning, and resistance to conditioned suppression of feeding in rats intermittently exposed to palatable food. Behav. Neurosci. 129, 219–224. doi: 10.1037/bne0000042
Verma, V. (2015). Classic studies on the interaction of cocaine and the dopamine transporter. Clin. Psychopharmacol. Neurosci. 13, 227–238. doi: 10.9758/cpn.2015.13.3.227
Verrico, C. D., Haile, C. N., Mahoney Iii, J. J., Thompson-Lake, D. G., Newton, T. F., and De La Garza, et al. (2014). Treatment with modafinil and escitalopram, alone and in combination, on cocaine-induced effects: a randomized, double blind, placebo-controlled human laboratory study. Drug Alcohol Depend. 141, 72–78. doi: 10.1016/j.drugalcdep.2014.05.008
Vocci, F. J., and Montoya, I. D. (2009). Psychological treatments for stimulant misuse, comparing and contrasting those for amphetamine dependence and those for cocaine dependence. Curr. Opin. Psychiatry 22, 263–268. doi: 10.1097/yco.0b013e32832a3b44
Volkow, N. D., Fowler, J. S., Logan, J., Alexoff, D., Zhu, W., Telang, F., et al. (2009). Effects of modafinil on dopamine and dopamine transporters in the male human brain: clinical implications. JAMA 301, 1148–1154. doi: 10.1001/jama.2009.351
Volkow, N. D., Wang, G.-J., Fowler, J. S., Tomasi, D., and Telang, F. (2011). Addiction: beyond dopamine reward circuitry. Proc. Natl. Acad. Sci. U.S.A. 108, 15037–15042. doi: 10.1073/pnas.1010654108
Vosburg, S. K., Hart, C. L., Haney, M., Rubin, E., and Foltin, R. W. (2010). Modafinil does not serve as a reinforcer in cocaine abusers. Drug Alcohol Depend. 106, 233–236. doi: 10.1016/j.drugalcdep.2009.09.002
Wang, X.-F., Bi, G.-H., He, Y., Yang, H.-J., Gao, J.-T., Okunola-Bakare, O. M., et al. (2015). R-modafinil attenuates nicotine-taking and nicotine-seeking behavior in alcohol-preferring rats. Neuropsychopharmacology 40, 1762–1771. doi: 10.1038/npp.2015.24
Ward, C. P., Harsh, J. R., York, K. M., Stewart, K. L., and Mccoy, J. G. (2004). Modafinil facilitates performance on a delayed nonmatching to position swim task in rats. Pharmacol. Biochem. Behav. 78, 735–741. doi: 10.1016/j.pbb.2004.05.005
Warot, D., Corruble, E., Payan, C., Weil, J., and Puech, A. (1993). Subjective effects of modafinil, a new central adrenergic stimulant in healthy volunteers: a comparison with amphetamine, caffeine and placebo. Eur. Psychiatry 8, 201–208. doi: 10.1017/s0924933800002923
Waters, K. A., Burnham, K. E., O’connor, D., Dawson, G. R., and Dias, R. J. J. O. P. (2005). Assessment of modafinil on attentional processes in a five-choice serial reaction time test in the rat. J. Psychopharmacol. 19, 149–158. doi: 10.1177/0269881105048995
Willie, J., Renthal, W., Chemelli, R., Miller, M., Scammell, T., Yanagisawa, M., et al. (2005). Modafinil more effectively induces wakefulness in orexin-null mice than in wild-type littermates. Neuroscience 130, 983–995. doi: 10.1016/j.neuroscience.2004.10.005
Wise, R. A. (2008). Dopamine and reward: the anhedonia hypothesis 30 years on. Neurotox. Res. 14, 169–183. doi: 10.1007/bf03033808
Wise, R. A., and Bozarth, M. A. (1987). A psychomotor stimulant theory of addiction. Psychol. Rev. 94, 469–492. doi: 10.1037/0033-295x.94.4.469
Wisor, J. P., Dement, W. C., Aimone, L., Williams, M., and Bozyczko-Coyne, D. (2006). Armodafinil, the R-enantiomer of modafinil: wake-promoting effects and pharmacokinetic profile in the rat. Pharmacol. Biochem. Behav. 85, 492–499. doi: 10.1016/j.pbb.2006.09.018
Wisor, J. P., Nishino, S., Sora, I., Uhl, G. H., Mignot, E., and Edgar, D. M. (2001). Dopaminergic role in stimulant-induced wakefulness. J. Neurosci. 21, 1787–1794. doi: 10.1523/jneurosci.21-05-01787.2001
Wong, Y. N., Simcoe, D., Hartman, L. N., Laughton, W. B., King, S. P., Mccormick, G. C., et al. (1999). A double-blind, placebo-controlled, ascending-dose evaluation of the pharmacokinetics and tolerability of modafinil tablets in healthy male volunteers. J. Clin. Pharmacol. 39, 30–40. doi: 10.1177/00912709922007534
Woolverton, W. L., and Johnson, K. M. (1992). Neurobiology of cocaine abuse. Trends Pharmacol. Sci. 13, 193–200. doi: 10.1016/0165-6147(92)90063-c
Wuo-Silva, R., Fukushiro, D. F., Borçoi, A. R., Fernandes, H. A., Procópio-Souza, R., Hollais, A. W., et al. (2011). Addictive potential of modafinil and cross-sensitization with cocaine: a pre-clinical study. Addict. Biol. 16, 565–579. doi: 10.1111/j.1369-1600.2011.00341.x
Wuo-Silva, R., Fukushiro, D. F., Hollais, A. W., Santos-Baldaia, R., Mári-Kawamoto, E., Berro, L. F., et al. (2016). Modafinil induces rapid-onset behavioral sensitization and cross-sensitization with cocaine in mice: implications for the addictive potential of modafinil. Front. Pharmacol. 7:420.
Wuo-Silva, R., Fukushiro-Lopes, D. F., Fialho, B. P., Hollais, A. W., Santos-Baldaia, R., Marinho, E. A., et al. (2019). Participation of dopamine D1 and D2 receptors in the rapid-onset behavioral sensitization to modafinil. Front. Pharmacol. 10:211.
Xie, Z., and Miller, G. M. (2009). A receptor mechanism for methamphetamine action in dopamine transporter regulation in brain. J. Pharmacol. Exp. Ther. 330, 316–325. doi: 10.1124/jpet.109.153775
Yan, W.-W., Yao, L.-H., Chen, C., Wang, H.-X., Li, C.-H., Huang, J.-N., et al. (2015). Effects of modafinil on behavioral learning and hippocampal synaptic transmission in rats. Int. Neurourol. J. 19, 220–227. doi: 10.5213/inj.2015.19.4.220
Young, J. W., and Geyer, M. A. (2010). Action of modafinil—increased motivation via the dopamine transporter inhibition and D1 receptors? Biol. Psychiatry 67, 784–787. doi: 10.1016/j.biopsych.2009.12.015
Young, J. W., Kooistra, K., and Geyer, M. A. (2011). Dopamine receptor mediation of the exploratory/hyperactivity effects of modafinil. Neuropsychopharmacology 36, 1385–1396. doi: 10.1038/npp.2011.23
Yu, X., Ma, Y., Harding, E. C., Yustos, R., Vyssotski, A. L., Franks, N. P., et al. (2019). Genetic lesioning of histamine neurons increases sleep–wake fragmentation and reveals their contribution to modafinil-induced wakefulness. Sleep 42:zsz031.
Zager, A., Brandão, W. N., Margatho, R. O., Peron, J. P., Tufik, S., Andersen, M. L., et al. (2018). The wake-promoting drug Modafinil prevents motor impairment in sickness behavior induced by LPS in mice: role for dopaminergic D1 receptor. Prog. Neuro Psychopharmacol. Biol. Psychiatry 81, 468–476. doi: 10.1016/j.pnpbp.2017.05.003
Zahniser, N. R., and Sorkin, A. (2004). Rapid regulation of the dopamine transporter: role in stimulant addiction? Neuropharmacology 47, 80–91. doi: 10.1016/j.neuropharm.2004.07.010
Zahniser, N. R., and Sorkin, A. (2009). Trafficking of dopamine transporters in psychostimulant actions. Semin. Cell Dev. Biol. 20, 411–417.
Zhang, H.-Y., Bi, G.-H., Yang, H.-J., He, Y., Xue, G., Cao, J., et al. (2017). The novel modafinil analog, JJC8-016, as a potential cocaine abuse pharmacotherapeutic. Neuropsychopharmacology 42, 1871–1883. doi: 10.1038/npp.2017.41
Zhang, L., Looney, D., Taub, D., Chang, S. L., Way, D., Witte, M. H., et al. (1998). Cocaine opens the blood-brain barrier to HIV-1 invasion. J. Neurovirol. 4, 619–626. doi: 10.3109/13550289809114228
Zhu, J., and Reith, M. (2008). Role of the dopamine transporter in the action of psychostimulants, nicotine, and other drugs of abuse. CNS Neurol. Disord. Drug Targets 7, 393–409. doi: 10.2174/187152708786927877
Keywords: cocaine, modafinil, dopamine, dopamine transporter blocker, psychostimulant use disorder, drug abuse and dependence, drug addiction
Citation: Hersey M, Bacon AK, Bailey LG, Coggiano MA, Newman AH, Leggio L and Tanda G (2021) Psychostimulant Use Disorder, an Unmet Therapeutic Goal: Can Modafinil Narrow the Gap? Front. Neurosci. 15:656475. doi: 10.3389/fnins.2021.656475
Received: 20 January 2021; Accepted: 20 April 2021;
Published: 26 May 2021.
Edited by:
Anna R. Carta, University of Cagliari, ItalyReviewed by:
Thomas M. Keck, Rowan University, United StatesMarco Bortolato, The University of Utah, United States
Copyright © 2021 Hersey, Bacon, Bailey, Coggiano, Newman, Leggio and Tanda. This is an open-access article distributed under the terms of the Creative Commons Attribution License (CC BY). The use, distribution or reproduction in other forums is permitted, provided the original author(s) and the copyright owner(s) are credited and that the original publication in this journal is cited, in accordance with accepted academic practice. No use, distribution or reproduction is permitted which does not comply with these terms.
*Correspondence: Gianluigi Tanda, Z3RhbmRhQGludHJhLm5pZGEubmloLmdvdg==
†These authors have contributed equally to this work and share first authorship