- 1Institute for Human Movement and Medical Sciences, Niigata University of Health and Welfare, Niigata, Japan
- 2Department of Physical Therapy, Niigata University of Health and Welfare, Niigata, Japan
Changes in α-band cortical oscillatory activity (8–13 Hz) affect perception; however, how these changes in the left posterior parietal cortex (PPC) and primary somatosensory cortex (S1), which play different roles in determining the two-point discrimination (TPD) threshold, affect TPD threshold remains unelucidated. Therefore, to determine TPD threshold, we aimed to investigate the function of the left PPC and S1 by applying α-band transcranial alternating current stimulation (α-tACS; 10 Hz). TPD threshold was examined at the pad of the right index finger, contralateral to the stimulation site, in 17 healthy adults using a custom-made, computer-controlled, two-point tactile stimulation device, with random application of either active or sham α-tACS over the left PPC (Experiment 1) and left S1 (Experiment 2). Then, 50% TPD threshold was obtained in the active and sham conditions via logistic regression analysis. Afterward, we compared the difference between the active and sham conditions at 50% TPD threshold in each region and found that α-tACS reduced TPD threshold when applied over the left PPC (P = 0.010); however, its effect was insignificant when applied over the left S1 (P = 0.74). Moreover, a comparison of the change in 50% TPD threshold among the regions revealed that α-tACS applied over the left PPC significantly reduced TPD threshold compared with that applied over the left S1 (P = 0.003). Although we did not reveal the actual changes in cortical activity induced by α-tACS, this is the first empirical evidence that α-tACS applied over the left PPC and left S1 exerts region-specific effects on determining TPD threshold assessed in the contralateral index finger pad by stimulation.
Introduction
Haptic information from the outside world is input to areas 3b and 1 of the primary somatosensory cortex (S1) by ascending afferent fibers, such as the slowly adapting fibers and rapidly adapting fibers, and is then sent to the secondary somatosensory cortex (S2) and higher-order areas, such as the posterior parietal cortex (PPC) (Inui et al., 2004). Two-point discrimination (TPD), a type of tactile function, has been extensively used in neurophysiological research and clinical practice since it was proposed in 1834 by Weber as a measurement of higher-order perceptual functions. Moreover, TPD threshold increases with aging and certain clinical conditions, such as central or peripheral nerve involvement and non-specific low back pain (Bassetti et al., 1993; Stevens and Cruz, 1996; Heriseanu et al., 2005; Catley et al., 2014a; Harvie et al., 2018). Conversely, it has been reported that TPD threshold decreases by tactile or electrical stimulation to the measurement site (Godde et al., 2000; Ragert et al., 2003, 2008; Tegenthoff et al., 2005; Dinse et al., 2006) and that improvements in TPD positively correlate with the enlarged area of the blood-oxygen-level-dependent signal (Pleger et al., 2003) and gray matter volume in the S1 (Schmidt-Wilcke et al., 2018). Furthermore, the inferior parietal lobule within the left PPC, a major region involved in attentional control (Corbetta et al., 2000; Yantis et al., 2002), is reportedly activated when performing a TPD task rather than during a simple sensory detection task (Sripati et al., 2006; Akatsuka et al., 2007, 2008; Pleger et al., 2016). Therefore, both S1 and PPC play important roles in the TPD process.
Furthermore, alpha (α)-band oscillatory activity (8–13 Hz) in the cortex reportedly affects the process of sensory perception in the brain. Perceptual performance negatively correlates with the power of α-band activity in the visual (Van Dijk et al., 2008; Iemi et al., 2017) and somatosensory (Haegens et al., 2011b; Lange et al., 2012; Baumgarten et al., 2016; Craddock et al., 2017) domains. The underlying mechanisms of these phenomena comprise α-band activity exerting a gating effect that suppresses signal transduction by increasing its power in areas that are not involved in the task at hand (Jensen and Mazaheri, 2010; Foxe and Snyder, 2011; Jensen et al., 2014; Van Diepen et al., 2019). This modulation of α-band activity is considered to be controlled by top–down attention modulation in both the visual (Thut et al., 2006; Gould et al., 2011; Jensen et al., 2014) and the somatosensory (Jones et al., 2010; Zhang and Ding, 2010; Haegens et al., 2011a) domains. Therefore, in the somatosensory cortex, α-band activity is considered to have an inhibitory role.
Conversely, increased α-band activity is reportedly associated with improved performance requiring sustained attention, such as in a working-memory task, where higher-order functions are involved (Makeig and Inlow, 1993; Dockree et al., 2007; Braboszcz and Delorme, 2011). For example, Dockree et al. (2007) reported that a higher α-band power predicts good sustained attention performance and that the oscillatory α-band activity correlated to it is generated in the parietal and occipital lobes. That is, while the α-band activity in the S1 has an inhibitory role, it may have a facilitative role in the PPC, which is involved in attentional control.
However, recent studies have suggested that modulation of α-band activity affects decision confidence and awareness of sensory experiences rather than sensitivity during discrimination tasks in the visual domain (Samaha et al., 2017, 2020; Iemi and Busch, 2018). In addition, as previously mentioned, the role of α-band activity varied among the studies because it plays different roles depending on the requirements during information processing. Although the S1 and PPC are important regions for somatosensory information processing (Inui et al., 2004; Jones et al., 2009; Haegens et al., 2010; Gundlach et al., 2017) and have been proposed as generators of α-band activity (Dockree et al., 2007; Jones et al., 2009; Halgren et al., 2019), the manner in which they affect TPD, a higher-order somatosensory perception, remains unclear. Owing to the hierarchal requirement of information processing in the S1 and PPC, the role of α-band activity might be different depending on the brain regions; therefore, modulation of α-band activity in these regions may have different effects on TPD performance.
Transcranial alternating current stimulation (tACS) has attracted considerable attention as a non-invasive method to modulate cortical oscillatory activity (Helfrich et al., 2014; Vossen et al., 2015). This type of stimulation can only synchronize oscillatory neural activity in the cortical area beneath the stimulus and artificially enhance cortical oscillation in that frequency range when applied over the occipital (Zaehle et al., 2010; Helfrich et al., 2014; Vossen et al., 2015) and somatosensory (Berger et al., 2018) cortices. However, the effects of α-tACS on somatosensory perception have also been reported to remain unchanged (Sliva et al., 2018) or to decrease after stimulation (Gundlach et al., 2017); therefore, there is no unified view yet. Furthermore, a recent study of tACS on the motor system suggested that the reported effect of tACS is not dominated by transcranial stimulation to the brain but by transcutaneous stimulation of the peripheral nerves (Asamoah et al., 2019); studies on rats and cadavers have reported that high-intensity currents of >4 mA are required to directly phase-entrain brain rhythms (Vöröslakos et al., 2018). Because we have not measured actual brain oscillatory activity during α-tACS and have not conducted an electrical field simulation, there were many limitations to our design to strongly address in our initial hypothesis. Although the exact mechanism of tACS is still under investigation, a previous study reported that α-tACS increases somatosensory α-band activity immediately after stimulation (Berger et al., 2018). Further, another study reported that tACS may at least impact circuit dynamics (Sliva et al., 2018); therefore, we conducted the present experiments based on the hypothesis that α-tACS may increase α-band activity.
Considering these findings, although the change in the actual oscillatory brain activity caused by α-tACS remains unknown in the present study, we hypothesized that α-tACS may decrease TPD threshold when applied over the left PPC because increased α-band activities are reported to facilitate sustained attention (Dockree et al., 2007; Clayton et al., 2018). Conversely, α-tACS’ application to the left S1 may increase TPD threshold because it inhibits information processing to the higher-order regions (Jensen and Mazaheri, 2010; Foxe and Snyder, 2011; Jensen et al., 2014; Van Diepen et al., 2019). We tested our hypothesis by measuring TPD threshold, a higher-order somatosensory function that requires sustained attention, after applying 10-Hz α-tACS to the left PPC (Experiment 1) and S1 (Experiment 2), wherein these regions were believed to play different roles in information processing (Corbetta et al., 2000; Yantis et al., 2002; Akatsuka et al., 2007, 2008). Furthermore, by comparing the differences in TPD thresholds in the two regions based on the results of Experiments 1 and 2, we found the cortical region that effectively improved TPD following α-tACS of either the left PPC or S1.
Materials and Methods
Participants
Overall, 21 healthy college students (12 men and nine women; age, 20.5 ± 0.8 years) with no history of neurological, orthopedic, or psychiatric disorders and who were not receiving any medication during the experimental period were included in this study. All participants underwent the experiments via the application of α-tACS to the left PPC (Experiment 1) and left S1 (Experiment 2). In the TPD task, four participants who answered two points to a one-point stimulus were excluded from the analysis to maintain the consistency of the task; as a result, final analysis included 17 participants. This experiment was conducted in accordance with the Declaration of Helsinki and was approved by the Ethics Committee of Niigata University of Health and Welfare (18264-19092). Full written informed consent was obtained from all participants.
tACS
Transcranial alternating current stimulation was delivered using a battery-driven, constant current stimulator (Eldith, neuroConn GmbH, Ilmenau, Germany) through a pair of saline-soaked surface sponge electrodes (5 × 5 cm, 25 cm2). One of the electrodes was placed over the left PPC (P3 according to the international 10–20 method; Experiment 1) or left S1 (2 cm posterior to C3; Experiment 2), whereas the other electrode was placed on the lateral side of the contralateral shoulder (Figures 1A,B). The reason for placing the reference electrode on the lateral side of the shoulder is that phosphenes reportedly occur during low-frequency tACS at low frequencies, which may affect the results of the measurements (Raco et al., 2014). Specifically, it is possible that the presence of phosphenes may alert the participants about the application of tACS or sham stimulation during the experiment; as a result, the correct answer rate may be affected by the input of sensory information unrelated to the task during the TPD task. Previous studies have solved this issue by placing a reference electrode on the lateral side of the shoulder; therefore, we adopted the same method in this experiment (Mehta et al., 2015; Miyaguchi et al., 2018). Although Mehta et al. (2015) reported that the extracephalic reference resulted in increased stimulation effects of the subcortical brain regions, it has also been reported that the reference electrode placed over the contralateral shoulder to the transcranial direct current stimulation (tDCS) for the dorsolateral prefrontal cortex (DLPFC) improves attentional bias compared with that placed over the left DLPFC (Shahbabaie et al., 2018). Moreover, the current density by tDCS remained the same regardless whether the position of the reference electrode was on the right cheek or right shoulder (Im et al., 2012). Therefore, we assumed that similar results were obtained with tACS and decided to use the extracephalic reference electrode. tACS was applied at 10 Hz with a sinusoidal wave with a constant current intensity of 1.0 mA (peak-to-peak). The impedance was maintained at <10 kΩ during the stimulation in accordance with the latest tACS guidelines (Antal et al., 2017).
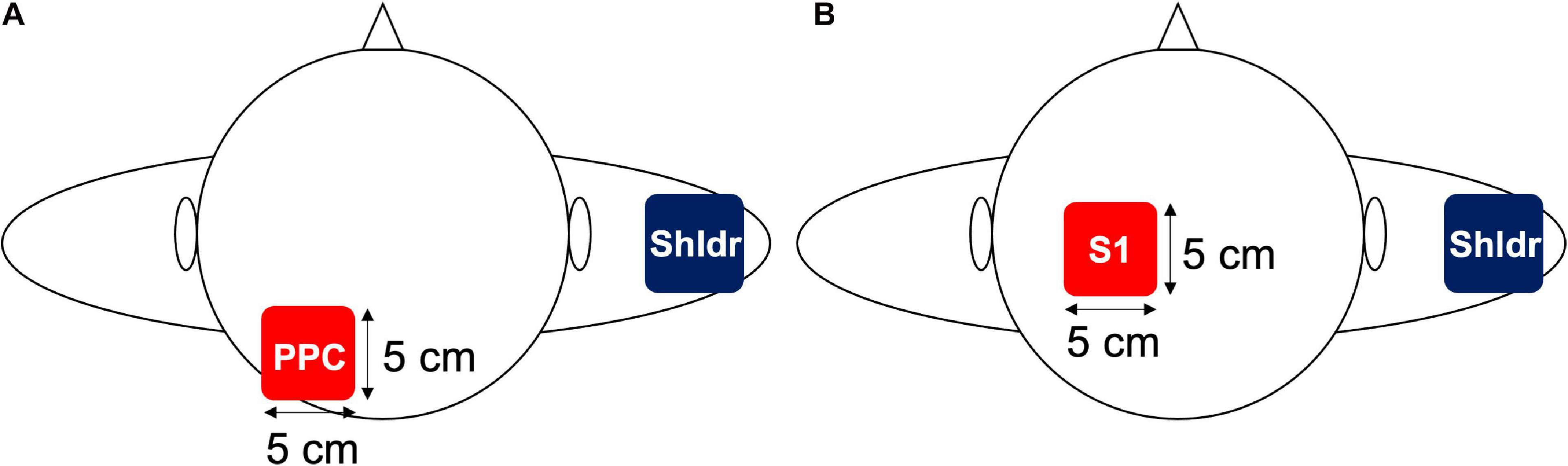
Figure 1. Electrode placement in Experiments 1 and 2. (A) In Experiment 1, an electrode was placed over the left PPC, which is located at P3 in the international 10–20 system, whereas the reference electrode was placed over the contralateral shoulder. (B) In Experiment 2, an electrode was placed over the left S1, which is located 2 cm posterior to the C3 in the international 10–20 system, whereas the reference electrode was placed over the contralateral shoulder.
TPD Threshold
For TPD measurements, the participants were seated in a resting position on a chair with a backrest and the right shoulder and elbow joints were placed in a slightly flexed position, whereas the forearm was positioned in a pronated position (Figure 2A). A custom-made, two-point tactile stimulator (Takei; Niigata, Japan) that can control stimulus conditions at a given value using a computer was used to randomly present a total of 10 stimuli to the right index finger pad, which is the contralateral side of the stimulated hemisphere, including one point (0 mm between the stimulus pins) or two points (nine stimuli ranging from 1 to 5.0 mm at 0.5-mm intervals; Figure 2B). We explained the instructions to the participants that two points should only be considered if they could be clearly identified as two points, whereas other vague stimuli and one point should be defined as one point. The participants were instructed to respond as quickly as possible when they recognized the stimulus by pushing a button held by the left hand. The stimuli were controlled by a computer under the following conditions: stimulus speed, 10.0 mm/s; stimulus penetration depth, 1.0 mm; and stimulus presentation time, 1.0 s, which were the optimal measurement conditions of TPD, as obtained in our previous study (Yokota et al., 2020) (Figure 2C). Moreover, it was reported that the α-band activity decreased in the hemisphere opposite to the one in which visual or tactile attention was directed; however, in this study, we attempted to increase the α-band activity in the left hemisphere, which perceives information from the right index finger (Van Dijk et al., 2008; Haegens et al., 2010; Jensen and Mazaheri, 2010). Furthermore, TPD threshold reportedly decreased by gazing at the measurement site during the measurement (Kennett et al., 2001; Moseley and Wiech, 2009; Catley et al., 2014b); therefore, we considered that these conditions could affect the measurement results. To minimize these effects, the participants were instructed to relax and look at a fixed point set at the height of each participant’s eyes, at a distance of ∼1.5 m, at all times during the experiment.
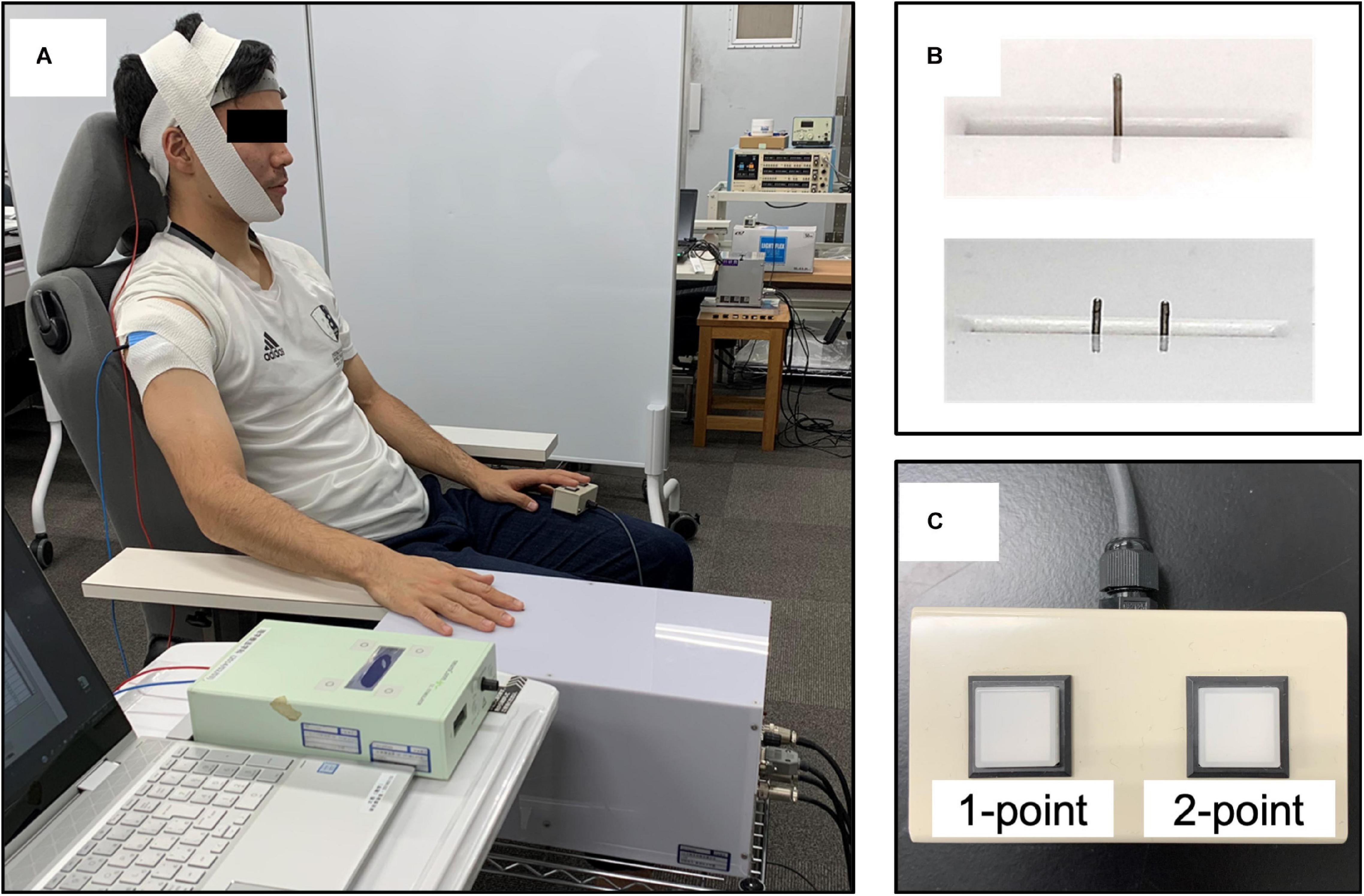
Figure 2. Participants’ position and the two-point tactile stimulation device. (A) The participants were seated on a fixed chair with a backrest, with the shoulder and elbow joints slightly flexed and the forearm in a pronated position. (B) The stimulus conditions (stimulus speed, 10.0 mm/s; stimulus penetration depth, 1.0 mm; stimulus presentation time, 1.0 s; and stimulus interval, 5 s) were fixed, and pin spacing was randomly assigned (one point, 0 mm and two points, nine different places, ranging from 1.0 to 5.0 mm at 0.5-mm intervals) using a custom-made computer-controlled two-point tactile stimulation device. (C) Answer button: participants were asked to respond about whether they perceived two points only when they were confident that there were two points, and an uncertain stimulus or one point was classified as one point.
Experimental Procedures
All participants first participated in Experiment 1 and then participated in Experiment 2 at least 1 week after the completion of Experiment 1. First, the participants performed 10 practice trials to understand how the stimulus would be presented and how they should respond to the stimulus during the TPD task. After the practice period, TPD was measured in four blocks: two blocks for each of the active and sham conditions, in which 10 different stimulus intervals were presented eight times in each block to obtain 160 measurements for each condition. In the tACS condition of Experiments 1 and 2, tACS was initiated 1 min before TPD measurements, and the TPD task was performed while receiving consistent stimulation during the block and was stopped immediately after the end of TPD measurements (all participants completed TPD measurements within 8 min). In the sham condition, stimulation was initiated 1 min before the onset of the TPD task but was only applied for 20 s (10 s of fade in and 10 s of fade out) to avoid stimulation of the cortex during the TPD task. The order of the blocks was randomly assigned for each participant and a 5-min break was introduced between blocks to avoid any carry-over effects from the previous block (Figure 3).
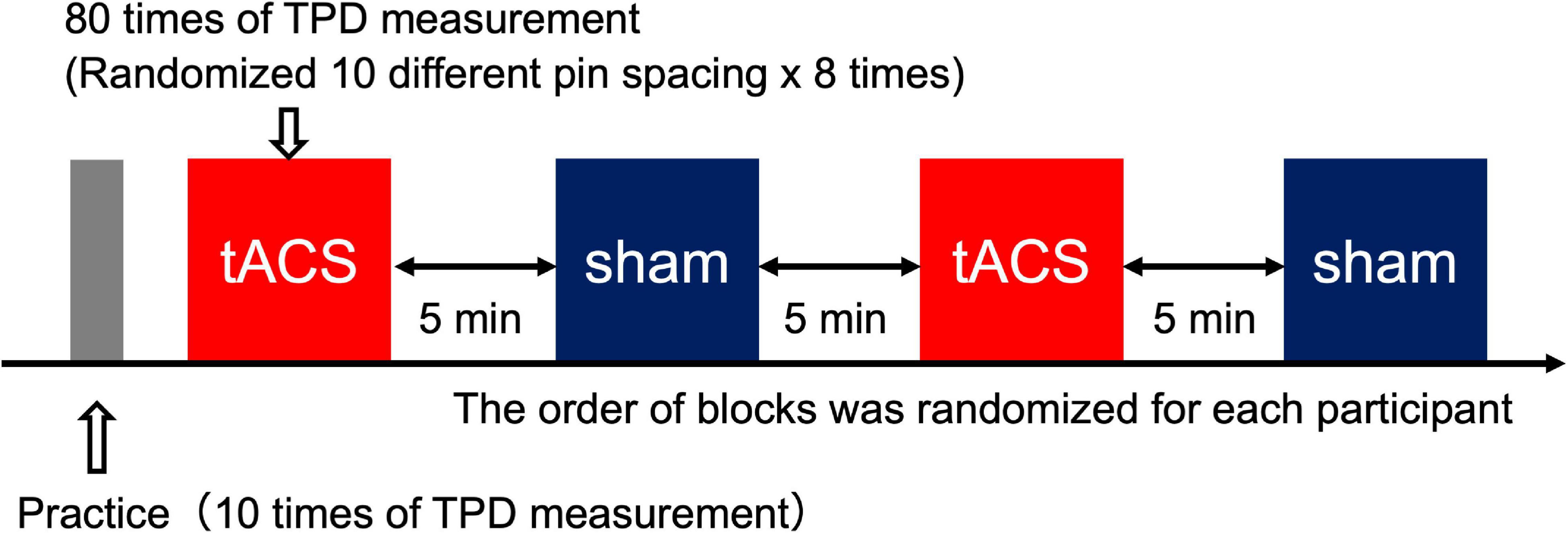
Figure 3. Experimental procedure. Each block included 80 trials (five trials × 18 intervals). There were four blocks in total (two blocks for the tACS condition and two blocks for the sham condition). The order of the blocks was randomized for each participant to minimize order effects. In total, 160 trials (80 trials × two blocks) from each condition were used for subsequent analysis.
Data and Statistical Analyses
To analyze the data, the distance between the pins and the correct answer rate were plotted on the X-axis and Y-axis, respectively, following which we fitted a binomial logistic regression model [“glmfit” function on MATLAB (MathWorks Inc.) with “binomial” and “logit” settings] to the data to draw a psychometric function. Thresholds at the correct rates of 25, 50, and 75% were calculated for each stimulus condition (tACS or sham) based on the psychophysical curve via logistic regression analysis. Moreover, 50% threshold was defined as TPD threshold and 75% threshold minus 25% threshold was defined as just the noticeable difference (JND), indicating discrimination sensitivity, as previously described (Otsuru et al., 2019). Moreover, the differences in 50% threshold between the tACS and sham conditions were calculated for the PPC and S1 by subtracting the 50% threshold calculated in the tACS condition from that calculated in the sham condition. The findings were compared to identify the cortical region that effectively improved TPD after α-tACS of either the left PPC or left S1.
For statistical analysis, we performed the Shapiro–Wilk test to verify the normal distribution of each stimulus region for 25, 50, and 75% thresholds, followed by the two-tailed paired t-test with correspondence between stimulus conditions (tACS or sham). Moreover, we analyzed discrimination sensitivity using statistical software (SPSS; IBM) in both Experiments 1 and 2. Furthermore, the differences in the 50% threshold recorded in the left PPC and left S1 were compared using the two-tailed paired t-test. To directly compare the possible regions and to demonstrate the reliability of the TPD measures used in our experiments, we used the two-tailed paired t-test to compare the means of the 50% threshold data obtained from the sham conditions in Experiments 1 and 2. The significance level was set at 5% for all statistical analyses. Data are expressed as mean ± standard error (SE).
Results
Experiment 1. Effects of tACS Over the PPC on the TPD Threshold
Figures 4A,B show the psychophysical curve fitted via logistic regression analysis obtained in Experiment 1 as well as 50% TPD threshold, which was calculated from the curve. The 50% TPD threshold (mean ± SE) was 2.45 ± 0.78 mm in the tACS condition and 2.65 ± 0.74 mm in the sham condition. Moreover, TPD threshold decreased in 13 (76.5%) of the 17 participants during the α-tACS condition compared with that during the sham condition. The two-tailed paired t-test between the two groups revealed a significant decrease in the tACS condition compared with the sham condition [t(16) = 2.54, p = 0.01, r = 0.54] (Figures 4A,B). JND was 0.68 ± 0.17 and 0.81 ± 0.25 in the tACS and sham conditions, respectively, with no significant differences between them.
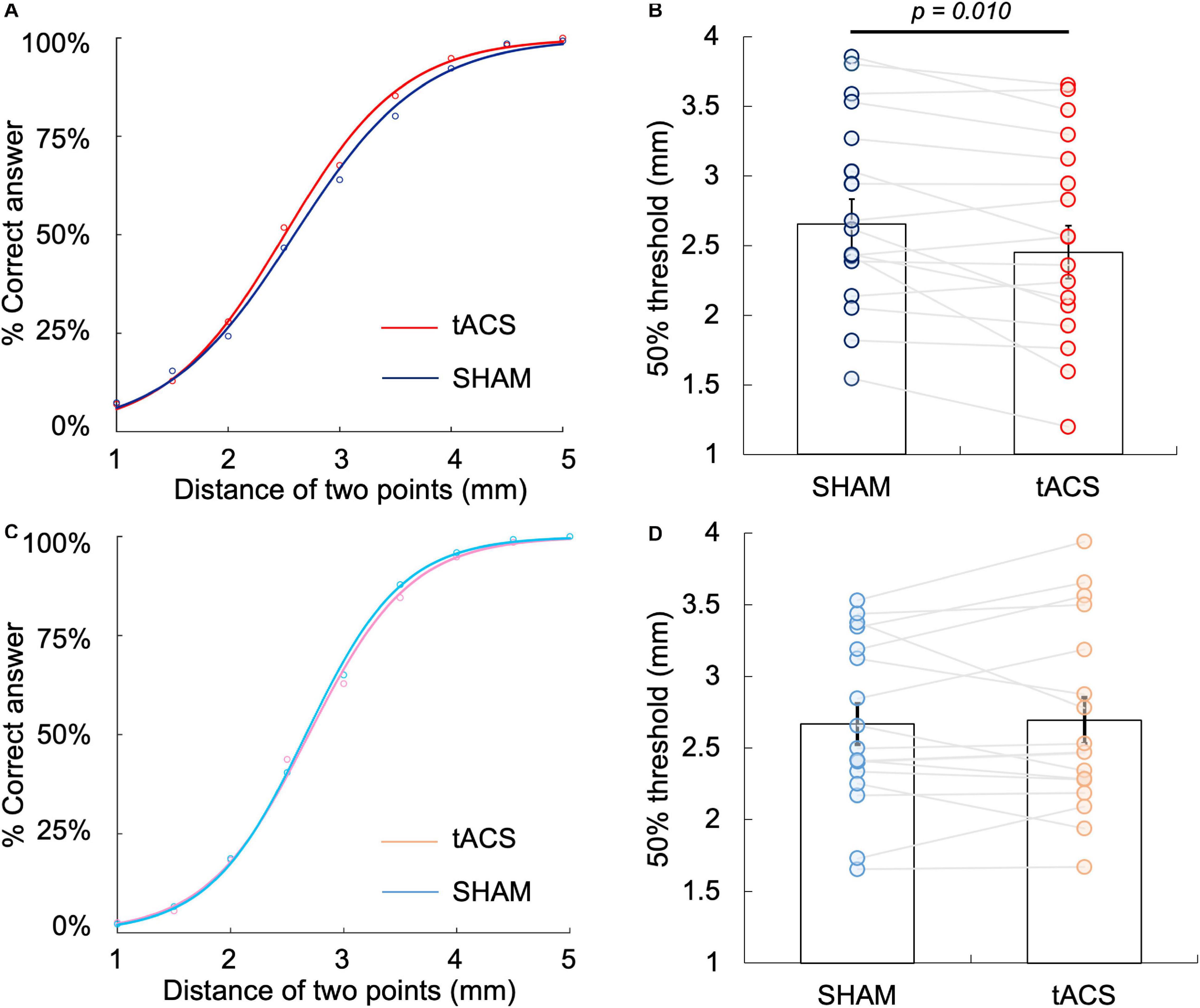
Figure 4. Differences in 50% TPD threshold between the tACS and sham conditions at the left PPC and left S1. (A) Psychophysical curve of the tACS and sham conditions at the left PPC in Experiment 1. (B) Comparison of 50% TPD threshold between the tACS and sham conditions in Experiment 1, where the X-axis indicates TPD threshold and Y-axis indicates the percentage of correct answers. The 50% TPD threshold in the tACS condition was significantly lower than that in the sham condition (P = 0.010). (C) Psychophysical curve of the tACS and sham conditions at the left S1 in Experiment 2. (D) Comparison of 50% TPD threshold between the tACS and sham conditions in Experiment 2, where the X-axis indicates TPD threshold and the Y-axis indicates the percentage of correct answers. There was no significant difference in 50% threshold between the tACS and sham conditions (P > 0.05).
Experiment 2. Effects of α-tACS Over the S1 on the TPD Threshold
Figures 4C,D show the psychophysical curve fitted via logistic regression analysis obtained in Experiment 2 as well as 50% TPD threshold, which was calculated from the curve. The 50% TPD threshold (mean ± SE) was 2.69 ± 0.66 mm in the tACS condition and 2.67 ± 0.59 mm in the sham condition. Although compared with the sham condition, TPD threshold increased in 11 (64.7%) of the 17 participants during α-tACS application, there was no significant difference between the conditions, according to the two-tailed paired t-test [t(16) = –0.34, p = 0.74, r = 0.08] (Figures 4C,D). JND was 0.56 ± 0.21 and 0.58 ± 0.25 in the tACS and sham conditions, respectively, with no significant differences between the two conditions.
Comparison of the Differences in α-tACS-Induced Threshold Between the Left PPC and Left S1
Based on the results obtained in Experiments 1 and 2, we compared the differences in α-tACS-induced threshold (50% threshold in the sham condition – 50% threshold in the tACS condition) in the left PPC and left S1. The differences in α-tACS-induced threshold in the left PPC and S1 were 0.2 ± 0.28 and –0.02 ± 0.27 mm, respectively, with the changes being significantly larger in the left PPC than in the left S1 [t(16) = 3.507, p = 0.003, r = 0.66, two-tailed] (Figure 5A). However, there was no significant difference in the sham conditions between the regions [t(16) = 0.014, p = 0.93, r = 0.00, two-tailed] (Figure 5B). Therefore, α-tACS over the left PPC effectively lowered the TPD threshold compared with that over the left S1. Moreover, a comparison of the mean of 50% TPD threshold in sham conditions between Experiments 1 and 2 revealed no statistical difference, which helped ameliorate the reliability of the TPD measures used in our experiments.
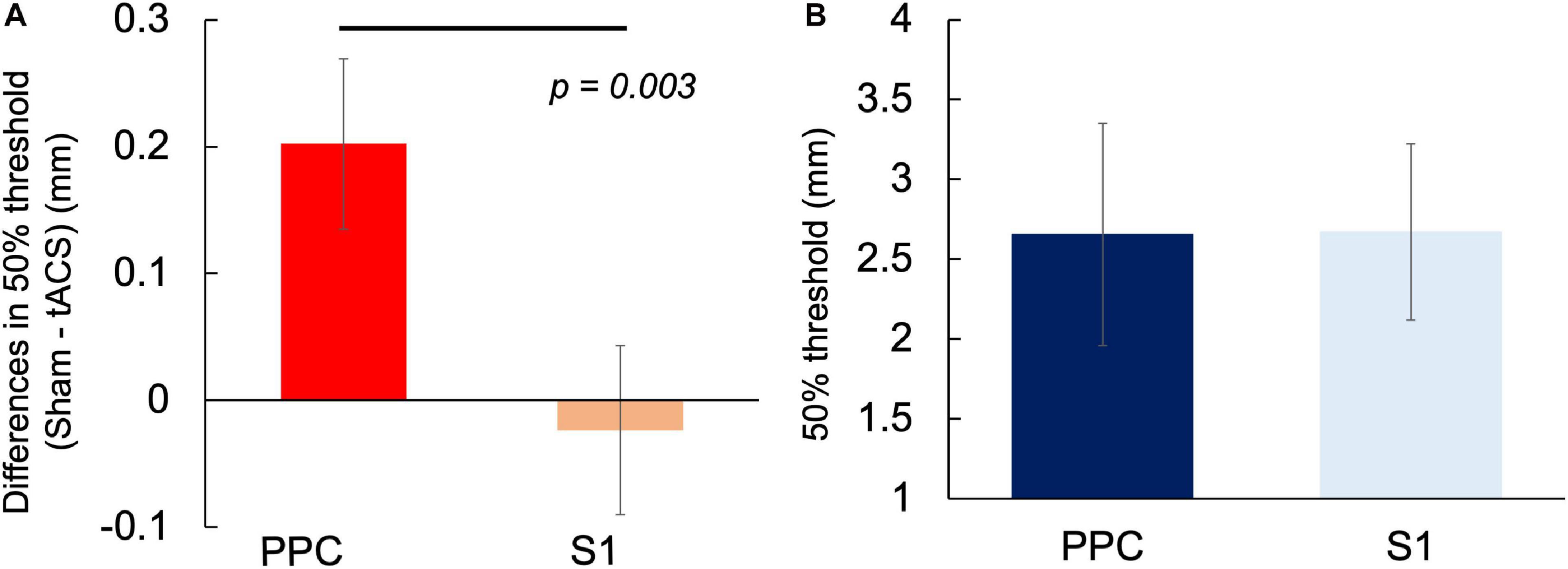
Figure 5. Comparison of the differences in α-tACS-induced threshold change and 50% TPD threshold in the sham condition between the left PPC and left S1. (A) Significant differences in threshold differences (50% threshold in the sham condition – 50% threshold in the tACS condition) were observed between the left PPC and the left S1 (P = 0.003). (B) No difference was observed in 50% threshold between the sham condition (P > 0.05).
Discussion
The present study aimed to clarify the effects of non-invasive brain stimulation over the left PPC (Experiment 1) and left S1 (Experiment 2), which were suggested to be related to TPD, on TPD threshold in the right index finger pad using 10-Hz α-tACS. The results of Experiment 1 illustrated that 10-Hz α-tACS of the left PPC, which is the area that plays a vital role in the TPD process and is responsible for attentional control, decreased TPD threshold. In contrast, in Experiment 2, similar stimulation with α-tACS over the left S1, which is suggested to be involved in tactile signal transfer to the higher-order regions of TPD, yielded no changes in TPD threshold. Furthermore, a comparison of the effects of α-tACS on the left PPC and left S1 based on the differences in 50% TPD threshold revealed that α-tACS applied over the left PPC significantly decreases TPD threshold compared with that applied over the left S1.
A possible explanation for the improvement of TPD following 10-Hz α-tACS over the left PPC is the increased α-band activity caused by α-tACS. Previous studies have shown that α-tACS enhances α-band activity at the stimulated region (Zaehle et al., 2010; Helfrich et al., 2014; Vossen et al., 2015; Berger et al., 2018); therefore, α-band activity at the left PPC might have increased under the tACS condition in the present study; this suggests that this increase in α-band activity improves TPD. This result is consistent with that of previous studies related to the effect of α-band activity on performance during cognitive tasks (Dockree et al., 2007; Braboszcz and Delorme, 2011). Dockree et al. (2007) reported that individuals who performed well on a working-memory task requiring sustained attention for an average of 24 blocks of 4.7 min exhibited a significant increase in α-band activity in the occipitoparietal region during the task. Moreover, in tasks requiring sustained attention for a long period, such as a 28 min × 2 session auditory discrimination task and a 20 min × 3 block respiratory-rate counting task, the α power decreased when participants provided incorrect answers during the task on the simultaneously measured EEG in the same individuals (Makeig and Inlow, 1993; Dockree et al., 2007; Braboszcz and Delorme, 2011). Furthermore, in a temporal order judgment task using electrical stimulation to the left and right index fingers, a 10-Hz α-tACS applied over the PPC, similar to the present study, reportedly improved the discrimination ability, suggesting the involvement of an increased α-band activity in the PPC in the cognitive task (Otsuru et al., 2019). As the TPD task used in this study is a cognitive task requiring prolonged and sustained attention (for approximately 10 min in four blocks, with a 5-min rest period between blocks), the α-tACS-induced increase in α-band activity in the left PPC might have promoted sustained attention to the task and improved task performance. In other words, because α-band activity is considered to exert a gating effect that suppresses signal transduction by increasing its power (Jensen and Mazaheri, 2010; Foxe and Snyder, 2011; Jensen et al., 2014; Van Diepen et al., 2019), increased α-band activity can be deemed to inhibit the left PPC and prevent switching attention away from the task to distractors (Yantis et al., 2002).
Unlike Experiment 1, α-tACS of the left S1 in Experiment 2 did not yield any change in TPD. Similar to visual attention, previous studies have shown that when an electrical stimulus is administered to the index finger, the α-band activity in the S1 on the opposite side of the stimulus decreases (Haegens et al., 2010; Gould et al., 2011). This is important because γ-band activity in the high-frequency region (30–150 Hz), which is important for information transmission, is periodically suppressed by α-band activity in a phase-dependent manner. This is referred to as cross-frequency phase–amplitude coupling (Klimesch et al., 2007; Osipova et al., 2008; Haegens et al., 2011b; Roux et al., 2013; Jensen et al., 2014). Therefore, in the region involved in the task, it is considered that information transfer is facilitated by a burst of γ-band activity that occurs during the trough of α-band activity (Jensen and Mazaheri, 2010; Jensen et al., 2014; Bonnefond and Jensen, 2015; Gips et al., 2016). Moreover, increased α-band activity in the S1 is reportedly correlated with the decreased frequency of neural firing in macaque monkeys and decreased excitability in human MEG and fMRI studies (Osipova et al., 2008; Haegens et al., 2011b; Scheeringa et al., 2011). These data suggest that α-band activity in the S1, which is a relay point of haptic information, needs to decrease physiologically to increase neural firing, thereby facilitating information transfer and improving TPD. In contrast, if the α-band activity in the S1 was decreased during the application of α-tACS, similar to the previously reported decrease in α-band activity after α-tACS application (Gundlach et al., 2017), it may facilitate information transfer and decrease TPD threshold. However, in this study, no significant difference in TPD threshold was observed when α-tACS was applied over the S1. Therefore, our results did not support our initial hypothesis that α-tACS increases α-band activity during stimulation, similar to the previous findings in the visual and parietal cortexes (Helfrich et al., 2014; Vossen et al., 2015; Berger et al., 2018), and thus, disturbed information transfer. That is, the α-tACS over the S1 used in this experiment may not have an effect on α-band activity. However, recent studies have suggested that discrimination sensitivity is not affected even though α-band activity increases in the visual domain (Samaha et al., 2017; Iemi and Busch, 2018; Samaha et al., 2020). Therefore, further studies are warranted to clarify the causal relationship between α-tACS and α-band activity in the S1.
Furthermore, on comparing the differences in TPD threshold because of α-tACS (50% threshold in the sham condition – 50% threshold in the tACS condition) applied over the left PPC and left S1 in Experiments 1 and 2, respectively, the amount of change in the left PPC was significantly larger than that in the left S1. Akatsuka et al. (2008) demonstrated that the inferior parietal lobule in the left PPC selectively showed significantly higher activity during the TPD task in a study that compared cortical activity during the TPD task with the task of discriminating the intensity of stimuli using fMRI. Because increases in α-band activity are critical for cognitive tasks requiring sustained attention (Makeig and Inlow, 1993; Dockree et al., 2007; Braboszcz and Delorme, 2011; Otsuru et al., 2019) and considering that the PPC plays a pivotal role in attentional control (Corbetta et al., 2000; Yantis et al., 2002), our result supports previous results that the left PPC plays an important role in the TPD process (Akatsuka et al., 2008). Moreover, the S1 is important in the process of information processing of TPD and decreased α-band activity must be further decreased to increase neural activity in the S1 such that it can facilitate signal transfer to higher-order regions, such as the PPC. Although this appeared to be paradoxical, the hierarchical mechanism of increases and decreases in α-band activities is controlled by a top–down regulation (Sadaghiani and Kleinschmidt, 2016; Van Diepen et al., 2019); this appears to be crucial for the optimal function of TPD. Therefore, although we did not measure the actual α-band activity during α-tACS application and the focal increment in α-band activity in each cortical region was not supported, it might have been separately modulated by α-tACS to some extent in our experiment, as proposed in previous studies (Zaehle et al., 2010; Helfrich et al., 2014; Vossen et al., 2015; Berger et al., 2018); it might have affected TPD processing, thereby differently shifting TPD threshold depending on the role of α-band activity in each region in the TPD process.
The reliability of the TPD measurement method was a major issue in the present study, as previous studies questioned the reliability of TPD as a measure of perceptual function (Catley et al., 2013; Tong et al., 2013). Slowly adapting I fiber and rapidly adapting I fiber are the primary afferents involved in TPD (Johansson and Vallbo, 1979; Darian-Smith and Kenins, 1980; Craig and Lyle, 2001). Moreover, the frequency of firing of these afferents, including their receptors, changes according to stimulus intensity (Vega-Bermudez and Johnson, 1999a, b). Therefore, the manner in which the stimulus pins are applied during measurements may affect the test results. To overcome this limitation, we used a custom-made two-point tactile stimulator that can control the stimulus speed and stimulus penetration depth of the stimulation pins at the time of measurement to arbitrary values on the computer, thereby providing a comprehensive measurement of TPD threshold. Accordingly, we reported that the threshold value was the lowest at a stimulus speed of 10.0 mm/s and a stimulus penetration depth of 1.0 mm, which represents a reliable measurement method for studying the effects of these interventions (Yokota et al., 2020). There was no significant difference in the mean 50% threshold in the sham conditions between Experiments 1 and 2. This indicates that the TPD measurement method used in this experiment was indeed reliable. Therefore, the TPDs used in this experiment clearly demonstrate the region-specific effects of α-tACS on the left PPC and left S1 because they may accurately reflect the changes in higher-order perceptual functions caused by α-tACS. In the future, the potential clinical applications of these results, such as whether similar results can be obtained in patients with impaired TPD, should be explored.
This study had some limitations in its experimental method. Previous studies have reported changes in cortical oscillatory activity induced by α-tACS (Zaehle et al., 2010; Helfrich et al., 2014; Berger et al., 2018); therefore, we attempted to increase α-band activity in the left PPC and S1 by administering 10-Hz α-tACS. However, we did not record the actual brain oscillatory activity in the cortical region. Therefore, another recently reported mechanism of α-tACS-induced improvement of the TPD, i.e., the entraining of the cortical neurons by the rhythmic activity from the skin’s peripheral nerves under the electrode input via the sensory pathway (Asamoah et al., 2019), should be considered. It is also reported that in rats and cadavers, high-intensity currents exceeding 4 mA are needed to directly phase-entrain brain rhythms (Vöröslakos et al., 2018). In this manner, the entrainment of the cortical activity caused by α-tACS in the present study might not only be focal under the electrode because the rhythmic activity was transmitted via the sensory pathway. Moreover, we have not measured actual brain activity during α-tACS or simulated electrical field distribution; therefore, it is not possible to explain exactly how α-tACS affects cortical oscillatory activity in the left PPC and left S1. Furthermore, the placement of the reference electrode should be considered. Although we placed the reference electrode over the shoulder contralateral to the stimulating electrode to minimize the occurrence of phosphenes, according to previous studies (Mehta et al., 2015; Miyaguchi et al., 2018), the placement of the distant electrode reportedly impacts not only the area under the electrode but also the surrounding area (Faria et al., 2011; Karabanov et al., 2019). The extracephalic reference also increases the stimulation effects on subcortical brain regions (Mehta et al., 2015). Furthermore, the current flow and electrical field distribution by the α-tACS montage used in this experiment were not simulated; therefore, the effects of α-tACS might not be less focal than expected. Therefore, elucidating whether the effects of α-tACS were focal to the left PPC and impossible on the left S1 and that there may be some overlap between the S1 and PPC is essential. However, α-tACS was found to exert different effects over the left PPC and left S1; therefore, the specific reactions of each region could be demonstrated to some extent. Detailed studies, such as those using a device that can simultaneously measure brain oscillatory activity during α-tACS and perform a simulation of the electrical field distribution, are warranted to confirm these results in the future.
Conclusion
The present study reveals that the use of 10-Hz α-tACS over the left PPC effectively reduces TPD threshold; however, a similar stimulation over the left S1 does not yield any significant changes. The effect of 10-Hz α-tACS on the determination of TPD threshold was region-specific in each cortical region involved in TPD. However, our results are speculative because actual cortical activity was not measured; therefore, additional studies are warranted to confirm the causal relationship between α-tACS in the left PPC and sustained attention or α-tACS in the S1, and information processing during the determination of TPD threshold using a more precise experimental paradigm, such as the concurrent measurement of cortical activity, during 10-Hz α-tACS.
Data Availability Statement
The raw data supporting the conclusions of this article will be made available by the authors, without undue reservation.
Ethics Statement
The studies involving human participants were reviewed and approved by the Niigata University of Health and Welfare. The patients/participants provided their written informed consent to participate in this study.
Author Contributions
HY and HO conceived the study and designed the experiments. KS and SK performed the experiments. NO interpreted the data. SM, YI, and KN helped draft the manuscript. All authors approved the final version of the submitted manuscript.
Funding
This work was supported by a Grant-in-Aid for Young Scientists 20K19429 and a Grant-in-Aid for Scientific Research (A) 19H01090 from the Japan Society for the Promotion of Science.
Conflict of Interest
The authors declare that the research was conducted in the absence of any commercial or financial relationships that could be construed as a potential conflict of interest.
Acknowledgments
The authors would like to thank Enago (www.enago.jp) for the English language review.
References
Akatsuka, K., Noguchi, Y., Harada, T., Sadato, N., and Kakigi, R. (2008). Neural codes for somatosensory two-point discrimination in inferior parietal lobule: an fMRI study. Neuroimage 40, 852–858. doi: 10.1016/j.neuroimage.2007.12.013
Akatsuka, K., Wasaka, T., Nakata, H., Kida, T., Hoshiyama, M., Tamura, Y., et al. (2007). Objective examination for two-point stimulation using a somatosensory oddball paradigm: an MEG study. Clin. Neurophysiol. 118, 403–411. doi: 10.1016/j.clinph.2006.09.030
Antal, A., Alekseichuk, I., Bikson, M., Brockmoller, J., Brunoni, A. R., Chen, R., et al. (2017). Low intensity transcranial electric stimulation: safety, ethical, legal regulatory and application guidelines. Clin. Neurophysiol. 128, 1774–1809. doi: 10.1016/j.clinph.2017.06.001
Asamoah, B., Khatoun, A., and Mc Laughlin, M. (2019). tACS motor system effects can be caused by transcutaneous stimulation of peripheral nerves. Nat. Commun. 10, 266.
Bassetti, C., Bogousslavsky, J., and Regli, F. (1993). Sensory syndromes in parietal stroke. Neurology 43, 1942–1949. doi: 10.1212/wnl.43.10.1942
Baumgarten, T. J., Schnitzler, A., and Lange, J. (2016). Prestimulus alpha power influences tactile temporal perceptual discrimination and confidence in decisions. Cereb. Cortex 26, 891–903. doi: 10.1093/cercor/bhu247
Berger, A., Pixa, N. H., Steinberg, F., and Doppelmayr, M. (2018). Brain oscillatory and hemodynamic activity in a bimanual coordination task following transcranial alternating current stimulation (tACS): a combined EEG-fNIRS study. Front. Behav. Neurosci. 12:67. doi: 10.3389/fnbeh.2018.00067
Bonnefond, M., and Jensen, O. (2015). Gamma activity coupled to alpha phase as a mechanism for top-down controlled gating. PLoS One 10:e0128667. doi: 10.1371/journal.pone.0128667
Braboszcz, C., and Delorme, A. (2011). Lost in thoughts: neural markers of low alertness during mind wandering. Neuroimage 54, 3040–3047. doi: 10.1016/j.neuroimage.2010.10.008
Catley, M. J., O’connell, N. E., Berryman, C., Ayhan, F. F., and Moseley, G. L. (2014a). Is tactile acuity altered in people with chronic pain? a systematic review and meta-analysis. J. Pain 15, 985–1000. doi: 10.1016/j.jpain.2014.06.009
Catley, M. J., Tabor, A., Miegel, R. G., Wand, B. M., Spence, C., and Moseley, G. L. (2014b). Show me the skin! Does seeing the back enhance tactile acuity at the back? Man Ther. 19, 461–466. doi: 10.1016/j.math.2014.04.015
Catley, M. J., Tabor, A., Wand, B. M., and Moseley, G. L. (2013). Assessing tactile acuity in rheumatology and musculoskeletal medicine–how reliable are two-point discrimination tests at the neck, hand, back and foot? Rheumatology (Oxford) 52, 1454–1461. doi: 10.1093/rheumatology/ket140
Clayton, M. S., Yeung, N., and Cohen Kadosh, R. (2018). The many characters of visual alpha oscillations. Eur. J. Neurosci. 48, 2498–2508. doi: 10.1111/ejn.13747
Corbetta, M., Kincade, J. M., Ollinger, J. M., Mcavoy, M. P., and Shulman, G. L. (2000). Voluntary orienting is dissociated from target detection in human posterior parietal cortex. Nat. Neurosci. 3, 292–297. doi: 10.1038/73009
Craddock, M., Poliakoff, E., El-Deredy, W., Klepousniotou, E., and Lloyd, D. M. (2017). Pre-stimulus alpha oscillations over somatosensory cortex predict tactile misperceptions. Neuropsychologia 96, 9–18. doi: 10.1016/j.neuropsychologia.2016.12.030
Craig, J. C., and Lyle, K. B. (2001). A comparison of tactile spatial sensitivity on the palm and fingerpad. Percept. Psychophys. 63, 337–347. doi: 10.3758/bf03194474
Darian-Smith, I., and Kenins, P. (1980). Innervation density of mechanoreceptive fibres supplying glabrous skin of the monkey’s index finger. J. Physiol. 309, 147–155. doi: 10.1113/jphysiol.1980.sp013500
Dinse, H. R., Kleibel, N., Kalisch, T., Ragert, P., Wilimzig, C., and Tegenthoff, M. (2006). Tactile coactivation resets age-related decline of human tactile discrimination. Ann. Neurol. 60, 88–94. doi: 10.1002/ana.20862
Dockree, P. M., Kelly, S. P., Foxe, J. J., Reilly, R. B., and Robertson, I. H. (2007). Optimal sustained attention is linked to the spectral content of background EEG activity: greater ongoing tonic alpha (approximately 10 Hz) power supports successful phasic goal activation. Eur. J. Neurosci. 25, 900–907. doi: 10.1111/j.1460-9568.2007.05324.x
Faria, P., Hallett, M., and Miranda, P. C. (2011). A finite element analysis of the effect of electrode area and inter-electrode distance on the spatial distribution of the current density in tDCS. J. Neural Eng. 8, 066017. doi: 10.1088/1741-2560/8/6/066017
Foxe, J. J., and Snyder, A. C. (2011). The role of alpha-band brain oscillations as a sensory suppression mechanism during selective attention. Front. Psychol. 2:154. doi: 10.3389/fpsyg.2011.00154
Gips, B., Van Der Eerden, J. P., and Jensen, O. (2016). A biologically plausible mechanism for neuronal coding organized by the phase of alpha oscillations. Eur. J. Neurosci. 44, 2147–2161. doi: 10.1111/ejn.13318
Godde, B., Stauffenberg, B., Spengler, F., and Dinse, H. R. (2000). Tactile coactivation-induced changes in spatial discrimination performance. J. Neurosci. 20, 1597–1604. doi: 10.1523/jneurosci.20-04-01597.2000
Gould, I. C., Rushworth, M. F., and Nobre, A. C. (2011). Indexing the graded allocation of visuospatial attention using anticipatory alpha oscillations. J. Neurophysiol. 105, 1318–1326. doi: 10.1152/jn.00653.2010
Gundlach, C., Muller, M. M., Nierhaus, T., Villringer, A., and Sehm, B. (2017). Modulation of somatosensory alpha rhythm by transcranial alternating current stimulation at mu-frequency. Front. Hum. Neurosci. 11:432. doi: 10.3389/fnhum.2017.00432
Haegens, S., Händel, B. F., and Jensen, O. (2011a). Top-down controlled alpha band activity in somatosensory areas determines behavioral performance in a discrimination task. J. Neurosci. 31, 5197–5204. doi: 10.1523/jneurosci.5199-10.2011
Haegens, S., Nacher, V., Luna, R., Romo, R., and Jensen, O. (2011b). Alpha-Oscillations in the monkey sensorimotor network influence discrimination performance by rhythmical inhibition of neuronal spiking. Proc. Natl. Acad. Sci. U.S.A. 108, 19377–19382. doi: 10.1073/pnas.1117190108
Haegens, S., Osipova, D., Oostenveld, R., and Jensen, O. (2010). Somatosensory working memory performance in humans depends on both engagement and disengagement of regions in a distributed network. Hum. Brain Mapp. 31, 26–35.
Halgren, M., Ulbert, I., Bastuji, H., Fabó, D., Erõss, L., Rey, M., et al. (2019). The generation and propagation of the human alpha rhythm. Proc. Natl. Acad. Sci. U.S.A. 116, 23772–23782.
Harvie, D. S., Edmond-Hank, G., and Smith, A. D. (2018). Tactile acuity is reduced in people with chronic neck pain. Musculoskelet. Sci. Pract. 33, 61–66. doi: 10.1016/j.msksp.2017.11.009
Helfrich, R. F., Schneider, T. R., Rach, S., Trautmann-Lengsfeld, S. A., Engel, A. K., and Herrmann, C. S. (2014). Entrainment of brain oscillations by transcranial alternating current stimulation. Curr. Biol. 24, 333–339. doi: 10.1016/j.cub.2013.12.041
Heriseanu, R., Baguley, I. J., and Slewa-Younan, S. (2005). Two-point discrimination following traumatic brain injury. J. Clin. Neurosci. 12, 156–160. doi: 10.1016/j.jocn.2004.03.029
Iemi, L., and Busch, N. A. (2018). Moment-to-moment fluctuations in neuronal excitability bias subjective perception rather than strategic decision-making. eNeuro 5:ENEURO.0430-17.2018. doi: 10.1523/ENEURO.0430-17.2018
Iemi, L., Chaumon, M., Crouzet, S. M., and Busch, N. A. (2017). Spontaneous neural oscillations bias perception by modulating baseline excitability. J. Neurosci. 37, 807–819. doi: 10.1523/jneurosci.1432-16.2016
Im, C. H., Park, J. H., Shim, M., Chang, W. H., and Kim, Y. H. (2012). Evaluation of local electric fields generated by transcranial direct current stimulation with an extracephalic reference electrode based on realistic 3D body modeling. Phys. Med. Biol. 57, 2137–2150. doi: 10.1088/0031-9155/57/8/2137
Inui, K., Wang, X., Tamura, Y., Kaneoke, Y., and Kakigi, R. (2004). Serial processing in the human somatosensory system. Cereb. Cortex 14, 851–857. doi: 10.1093/cercor/bhh043
Jensen, O., Gips, B., Bergmann, T. O., and Bonnefond, M. (2014). Temporal coding organized by coupled alpha and gamma oscillations prioritize visual processing. Trends Neurosci. 37, 357–369. doi: 10.1016/j.tins.2014.04.001
Jensen, O., and Mazaheri, A. (2010). Shaping functional architecture by oscillatory alpha activity: gating by inhibition. Front Hum Neurosci 4:186. doi: 10.3389/fnhum.2010.00186
Johansson, R. S., and Vallbo, A. B. (1979). Tactile sensibility in the human hand: relative and absolute densities of four types of mechanoreceptive units in glabrous skin. J. Physiol. 286, 283–300. doi: 10.1113/jphysiol.1979.sp012619
Jones, S. R., Kerr, C. E., Wan, Q., Pritchett, D. L., Hämäläinen, M., and Moore, C. I. (2010). Cued spatial attention drives functionally relevant modulation of the mu rhythm in primary somatosensory cortex. J. Neurosci. 30, 13760–13765. doi: 10.1523/jneurosci.2969-10.2010
Jones, S. R., Pritchett, D. L., Sikora, M. A., Stufflebeam, S. M., Hämäläinen, M., and Moore, C. I. (2009). Quantitative analysis and biophysically realistic neural modeling of the MEG mu rhythm: rhythmogenesis and modulation of sensory-evoked responses. J. Neurophysiol. 102, 3554–3572. doi: 10.1152/jn.00535.2009
Karabanov, A. N., Saturnino, G. B., Thielscher, A., and Siebner, H. R. (2019). Can transcranial electrical stimulation localize brain function? Front. Psychol. 10:213. doi: 10.3389/fpsyg.2019.00213
Kennett, S., Taylor-Clarke, M., and Haggard, P. (2001). Noninformative vision improves the spatial resolution of touch in humans. Curr. Biol. 11, 1188–1191. doi: 10.1016/s0960-9822(01)00327-x
Klimesch, W., Sauseng, P., and Hanslmayr, S. (2007). EEG alpha oscillations: the inhibition-timing hypothesis. Brain Res. Rev. 53, 63–88. doi: 10.1016/j.brainresrev.2006.06.003
Lange, J., Halacz, J., Van Dijk, H., Kahlbrock, N., and Schnitzler, A. (2012). Fluctuations of prestimulus oscillatory power predict subjective perception of tactile simultaneity. Cereb. Cortex 22, 2564–2574. doi: 10.1093/cercor/bhr329
Makeig, S., and Inlow, M. (1993). Lapses in alertness: coherence of fluctuations in performance and EEG spectrum. Electroencephalogr. Clin. Neurophysiol. 86, 23–35. doi: 10.1016/0013-4694(93)90064-3
Mehta, A. R., Pogosyan, A., Brown, P., and Brittain, J. S. (2015). Montage matters: the influence of transcranial alternating current stimulation on human physiological tremor. Brain Stimul. 8, 260–268. doi: 10.1016/j.brs.2014.11.003
Miyaguchi, S., Otsuru, N., Kojima, S., Saito, K., Inukai, Y., Masaki, M., et al. (2018). Transcranial alternating current stimulation with gamma oscillations over the primary motor cortex and cerebellar hemisphere improved visuomotor performance. Front. Behav. Neurosci. 12:132. doi: 10.3389/fnbeh.2018.00132
Moseley, G. L., and Wiech, K. (2009). The effect of tactile discrimination training is enhanced when patients watch the reflected image of their unaffected limb during training. Pain 144, 314–319. doi: 10.1016/j.pain.2009.04.030
Osipova, D., Hermes, D., and Jensen, O. (2008). Gamma power is phase-locked to posterior alpha activity. PLoS One 3:e3990. doi: 10.1371/journal.pone.0003990
Otsuru, N., Kamijo, K., Otsuki, T., Kojima, S., Miyaguchi, S., Saito, K., et al. (2019). 10 Hz transcranial alternating current stimulation over posterior parietal cortex facilitates tactile temporal order judgment. Behav. Brain Res. 368:111899. doi: 10.1016/j.bbr.2019.111899
Pleger, B., Foerster, A. F., Ragert, P., Dinse, H. R., Schwenkreis, P., Malin, J. P., et al. (2003). Functional imaging of perceptual learning in human primary and secondary somatosensory cortex. Neuron 40, 643–653. doi: 10.1016/s0896-6273(03)00677-9
Pleger, B., Wilimzig, C., Nicolas, V., Kalisch, T., Ragert, P., Tegenthoff, M., et al. (2016). A complementary role of intracortical inhibition in age-related tactile degradation and its remodelling in humans. Sci. Rep. 6:27388.
Raco, V., Bauer, R., Olenik, M., Brkic, D., and Gharabaghi, A. (2014). Neurosensory effects of transcranial alternating current stimulation. Brain Stimul. 7, 823–831. doi: 10.1016/j.brs.2014.08.005
Ragert, P., Dinse, H. R., Pleger, B., Wilimzig, C., Frombach, E., Schwenkreis, P., et al. (2003). Combination of 5 Hz repetitive transcranial magnetic stimulation (rTMS) and tactile coactivation boosts tactile discrimination in humans. Neurosci. Lett. 348, 105–108. doi: 10.1016/s0304-3940(03)00745-6
Ragert, P., Kalisch, T., Bliem, B., Franzkowiak, S., and Dinse, H. R. (2008). Differential effects of tactile high- and low-frequency stimulation on tactile discrimination in human subjects. BMC Neurosci. 9:9. doi: 10.1186/1471-2202-9-9
Roux, F., Wibral, M., Singer, W., Aru, J., and Uhlhaas, P. J. (2013). The phase of thalamic alpha activity modulates cortical gamma-band activity: evidence from resting-state MEG recordings. J. Neurosci. 33, 17827–17835. doi: 10.1523/jneurosci.5778-12.2013
Sadaghiani, S., and Kleinschmidt, A. (2016). Brain networks and alpha-oscillations: structural and functional foundations of cognitive control. Trends Cogn. Sci. 20, 805–817. doi: 10.1016/j.tics.2016.09.004
Samaha, J., Iemi, L., Haegens, S., and Busch, N. A. (2020). Spontaneous brain oscillations and perceptual decision-making. Trends Cogn. Sci. 24, 639–653. doi: 10.1016/j.tics.2020.05.004
Samaha, J., Iemi, L., and Postle, B. R. (2017). Prestimulus alpha-band power biases visual discrimination confidence, but not accuracy. Conscious Cogn. 54, 47–55. doi: 10.1016/j.concog.2017.02.005
Scheeringa, R., Mazaheri, A., Bojak, I., Norris, D. G., and Kleinschmidt, A. (2011). Modulation of visually evoked cortical FMRI responses by phase of ongoing occipital alpha oscillations. J. Neurosci. 31, 3813–3820. doi: 10.1523/jneurosci.4697-10.2011
Schmidt-Wilcke, T., Wulms, N., Heba, S., Pleger, B., Puts, N. A., Glaubitz, B., et al. (2018). Structural changes in brain morphology induced by brief periods of repetitive sensory stimulation. Neuroimage 165, 148–157. doi: 10.1016/j.neuroimage.2017.10.016
Shahbabaie, A., Hatami, J., Farhoudian, A., Ekhtiari, H., Khatibi, A., and Nitsche, M. A. (2018). Optimizing electrode montages of transcranial direct current stimulation for attentional bias modification in early abstinent methamphetamine users. Front. Pharmacol. 9:907. doi: 10.3389/fphar.2018.00907
Sliva, D. D., Black, C. J., Bowary, P., Agrawal, U., Santoyo, J. F., Philip, N. S., et al. (2018). A prospective study of the impact of transcranial alternating current stimulation on EEG correlates of somatosensory perception. Front. Psychol. 9:2117. doi: 10.3389/fpsyg.2018.02117
Sripati, A. P., Yoshioka, T., Denchev, P., Hsiao, S. S., and Johnson, K. O. (2006). Spatiotemporal receptive fields of peripheral afferents and cortical area 3b and 1 neurons in the primate somatosensory system. J. Neurosci. 26, 2101–2114. doi: 10.1523/jneurosci.3720-05.2006
Stevens, J. C., and Cruz, L. A. (1996). Spatial acuity of touch: ubiquitous decline with aging revealed by repeated threshold testing. Somatosens. Mot. Res. 13, 1–10. doi: 10.3109/08990229609028907
Tegenthoff, M., Ragert, P., Pleger, B., Schwenkreis, P., Forster, A. F., Nicolas, V., et al. (2005). Improvement of tactile discrimination performance and enlargement of cortical somatosensory maps after 5 Hz rTMS. PLoS Biol. 3:e362. doi: 10.1371/journal.pbio.0030362
Thut, G., Nietzel, A., Brandt, S. A., and Pascual-Leone, A. (2006). Alpha-band electroencephalographic activity over occipital cortex indexes visuospatial attention bias and predicts visual target detection. J. Neurosci. 26, 9494–9502. doi: 10.1523/jneurosci.0875-06.2006
Tong, J., Mao, O., and Goldreich, D. (2013). Two-point orientation discrimination versus the traditional two-point test for tactile spatial acuity assessment. Front. Hum. Neurosci. 7:579. doi: 10.3389/fnhum.2013.00579
Van Diepen, R. M., Foxe, J. J., and Mazaheri, A. (2019). The functional role of alpha-band activity in attentional processing: the current zeitgeist and future outlook. Curr. Opin. Psychol. 29, 229–238. doi: 10.1016/j.copsyc.2019.03.015
Van Dijk, H., Schoffelen, J. M., Oostenveld, R., and Jensen, O. (2008). Prestimulus oscillatory activity in the alpha band predicts visual discrimination ability. J. Neurosci. 28, 1816–1823. doi: 10.1523/jneurosci.1853-07.2008
Vega-Bermudez, F., and Johnson, K. O. (1999a). SA1 and RA receptive fields, response variability, and population responses mapped with a probe array. J. Neurophysiol. 81, 2701–2710. doi: 10.1152/jn.1999.81.6.2701
Vega-Bermudez, F., and Johnson, K. O. (1999b). Surround suppression in the responses of primate SA1 and RA mechanoreceptive afferents mapped with a probe array. J. Neurophysiol. 81, 2711–2719. doi: 10.1152/jn.1999.81.6.2711
Vöröslakos, M., Takeuchi, Y., Brinyiczki, K., Zombori, T., Oliva, A., Fernández-Ruiz, A., et al. (2018). Direct effects of transcranial electric stimulation on brain circuits in rats and humans. Nat. Commun. 9:483.
Vossen, A., Gross, J., and Thut, G. (2015). Alpha power increase after transcranial alternating current stimulation at alpha frequency (alpha-tACS) reflects plastic changes rather than entrainment. Brain Stimul. 8, 499–508. doi: 10.1016/j.brs.2014.12.004
Yantis, S., Schwarzbach, J., Serences, J. T., Carlson, R. L., Steinmetz, M. A., Pekar, J. J., et al. (2002). Transient neural activity in human parietal cortex during spatial attention shifts. Nat. Neurosci. 5, 995–1002. doi: 10.1038/nn921
Yokota, H., Otsuru, N., Kikuchi, R., Suzuki, R., Kojima, S., Saito, K., et al. (2020). Establishment of optimal two-point discrimination test method and consideration of reproducibility. Neurosci. Lett. 714:134525. doi: 10.1016/j.neulet.2019.134525
Zaehle, T., Rach, S., and Herrmann, C. S. (2010). Transcranial alternating current stimulation enhances individual alpha activity in human EEG. PLoS One 5:e13766. doi: 10.1371/journal.pone.0013766
Keywords: transcranial alternating current stimulation, α-band activity, two-point discrimination, posterior parietal cortex, primary somatosensory cortex
Citation: Yokota H, Otsuru N, Saito K, Kojima S, Miyaguchi S, Inukai Y, Nagasaka K and Onishi H (2021) Region-Specific Effects of 10-Hz Transcranial Alternate Current Stimulation Over the Left Posterior Parietal Cortex and Primary Somatosensory Area on Tactile Two-Point Discrimination Threshold. Front. Neurosci. 15:576526. doi: 10.3389/fnins.2021.576526
Received: 26 June 2020; Accepted: 20 January 2021;
Published: 18 February 2021.
Edited by:
Gregor Thut, University of Glasgow, United KingdomReviewed by:
Christopher Gundlach, Leipzig University, GermanyDanielle D. Sliva, Brown University, United States
Copyright © 2021 Yokota, Otsuru, Saito, Kojima, Miyaguchi, Inukai, Nagasaka and Onishi. This is an open-access article distributed under the terms of the Creative Commons Attribution License (CC BY). The use, distribution or reproduction in other forums is permitted, provided the original author(s) and the copyright owner(s) are credited and that the original publication in this journal is cited, in accordance with accepted academic practice. No use, distribution or reproduction is permitted which does not comply with these terms.
*Correspondence: Hirotake Yokota, eW9rb3RhQG51aHcuYWMuanA=