- Synapse and Neural Circuit Research Section, National Institute of Neurological Disorders and Stroke, National Institutes of Health, Bethesda, MD, United States
Diverse populations of GABAA receptors (GABAARs) throughout the brain mediate fast inhibitory transmission and are modulated by various endogenous ligands and therapeutic drugs. Deficits in GABAAR signaling underlie the pathophysiology behind neurological and neuropsychiatric disorders such as epilepsy, anxiety, and depression. Pharmacological intervention for these disorders relies on several drug classes that target GABAARs, such as benzodiazepines and more recently neurosteroids. It has been widely demonstrated that subunit composition and receptor stoichiometry impact the biophysical and pharmacological properties of GABAARs. However, current GABAAR-targeting drugs have limited subunit selectivity and produce their therapeutic effects concomitantly with undesired side effects. Therefore, there is still a need to develop more selective GABAAR pharmaceuticals, as well as evaluate the potential for developing next-generation drugs that can target accessory proteins associated with native GABAARs. In this review, we briefly discuss the effects of benzodiazepines and neurosteroids on GABAARs, their use as therapeutics, and some of the pitfalls associated with their adverse side effects. We also discuss recent advances toward understanding the structure, function, and pharmacology of GABAARs with a focus on benzodiazepines and neurosteroids, as well as newly identified transmembrane proteins that modulate GABAARs.
Introduction
Efforts aimed at uncovering mechanisms driving inhibitory transmission have not only contributed to our understanding of nervous system function, but have also led to the development of several drugs used in the treatment of neurological and psychiatric disorders. In the brain, fast inhibitory transmission is predominantly mediated by GABAA receptors (GABAARs), which are pentameric ligand-gated ion channels that conduct Cl– upon activation (Olsen and Sieghart, 2009; Sigel and Steinmann, 2012; Gielen and Corringer, 2018). Thus far, nineteen GABAAR subunits, α(1–6), β(1–3), γ(1–3), δ, ρ(1–3), ε, θ, and π, have been identified in humans (Farrant and Nusser, 2005; Olsen and Sieghart, 2009; Sieghart and Savić, 2018) and the subunit composition, as well as arrangement, of GABAARs contribute to receptor properties such as trafficking, localization, kinetics, and pharmacology (Levitan et al., 1988a; Sigel et al., 1990; Lavoie et al., 1997; Belelli and Lambert, 2005; Herd et al., 2007; Sigel and Steinmann, 2012). Neuronal activity is dynamically regulated by both phasic and tonic inhibition resulting from GABAARs localized at synaptic or extrasynaptic regions, respectively (Mody and Pearce, 2004; Farrant and Nusser, 2005; Jacob et al., 2008; Lorenz-Guertin and Jacob, 2018; Chiu et al., 2019; Tomita, 2019). Furthermore, GABAARs are ubiquitously expressed across the brain, albeit in a region-, circuit- and cell-specific manner (Sieghart and Sperk, 2002; Engin et al., 2018). Deficits in GABAergic signaling are associated with the pathophysiology behind several neurological and psychiatric conditions (Macdonald et al., 2004; Ramamoorthi and Lin, 2011; Ben-Ari et al., 2012; Brickley and Mody, 2012; Hines et al., 2012; MacKenzie and Maguire, 2013; Rudolph and Möhler, 2014; Lorenz-Guertin et al., 2018) and many treatment strategies employ GABAAR-targeting drugs. Several therapeutic drug classes, including barbiturates, benzodiazepines, general anesthetics, and neurosteroids, target GABAARs at distinct allosteric binding sites and are commonly used to treat these disorders (Olsen and Sieghart, 2009; Olsen, 2018). Although they are widely employed for their sedative-hypnotic, anxiolytic, anticonvulsant, and/or muscle relaxant properties (Sieghart and Savić, 2018), adverse consequences such as drug dependence and withdrawal set limitations to their long-term use (Lalive et al., 2011; Tan et al., 2011; Balon and Starcevic, 2020). Thus, elucidating the structural and functional properties of GABAARs, as well as developing more selective GABAAR-targeting drugs with less side effects remain of high importance in modern drug discovery.
Contemporary studies on GABAARs have provided potentially and exciting opportunities for the development of more selective and efficacious drugs that target these receptors. Recent breakthroughs include new structural insights into ligand-bound GABAARs (Laverty et al., 2017, 2019; Miller et al., 2017; Zhu et al., 2018; Masiulis et al., 2019; Kim et al., 2020), the properties of different GABAAR subunit variants (Benkwitz et al., 2004; Boileau et al., 2010; Rajgor et al., 2020), and the recent characterization of transmembrane interacting proteins that modulate GABAAR trafficking and function (Davenport et al., 2017; Martenson et al., 2017; Yamasaki et al., 2017; Ge et al., 2018; Han et al., 2019, 2020). Although only initially characterized, these new observations of GABAAR accessory proteins add another layer of complexity to GABAAR regulation that should importantly be considered in drug screens and future pharmacology studies (Han et al., 2020). Our review will briefly focus on the role of GABAAR dysfunction in epilepsy, anxiety, and postpartum depression (PPD) as GABAAR-based pharmacotherapy is primarily employed as a treatment strategy in these conditions. Due to their widespread use in these disorders, an update on GABAAR function and pharmacology with respect to benzodiazepines and neurosteroids will be given. Additionally, we discuss recent studies on GABAAR structures, GABAAR subunit variants, and the discovery of GABAAR-associated transmembrane proteins. Lastly, we highlight potential opportunities in GABAAR pharmacology development as a result of these advancements.
A Brief History on GABAARs as Prolific Drug Targets and Their Therapeutic Usage
Drugs targeting GABAARs have been in use since the early 1900s (Figure 1), long before the isolation and cloning of receptor subunits in the 1980s (Sigel et al., 1982, 1983; Barnard et al., 1987; Schofield et al., 1987; Seeburg et al., 1990). Barbiturates were first employed for their anticonvulsant and sedative-hypnotic properties (Smart and Stephenson, 2019). However, a decline in their clinical use resulted from high mortality risk due to accidental overdose and the advent of benzodiazepines (López-Muñoz et al., 2005). The initial discovery of chlordiazepoxide in 1955 by Leo Sternbach at Hoffman-La Roche and diazepam (DZ) shortly after in 1959 created excitement for benzodiazepines (Figure 1), allowing them to become one of the most widely marketed and prescribed drugs (Mehdi, 2012). However, it was not accepted until decades later that adverse side effects such as addiction could occur with long-term usage (Lalive et al., 2011; Mehdi, 2012; Votaw et al., 2019). Although their site-of-action had not yet been determined, by the mid-to-late 1970s, it was known that barbiturates and benzodiazepines enhanced inhibition by potentiating the actions of GABA (Smart and Stephenson, 2019). Following the discovery of the various GABAAR subunits, many studies have been devoted toward characterizing the physiological and pharmacological properties of GABAARs with respect to subunit composition (Barnard et al., 1987; Schofield et al., 1987; Levitan et al., 1988b; Pritchett et al., 1989; Seeburg et al., 1990; Farrant and Nusser, 2005; Vithlani et al., 2011; Engin et al., 2018; Sieghart and Savić, 2018). GABAARs harbor several binding sites for barbiturates, benzodiazepines, general anesthetics, alcohol, and neurosteroids (Sieghart, 2015). Depending on the GABAAR subtype, many of these compounds exhibit differences in ligand sensitivity, resulting in different physiological and behavioral responses (Olsen and Sieghart, 2009). Accordingly, substantial work has been devoted toward understanding the binding mode and functional responses of various ligands at different GABAAR subtypes.
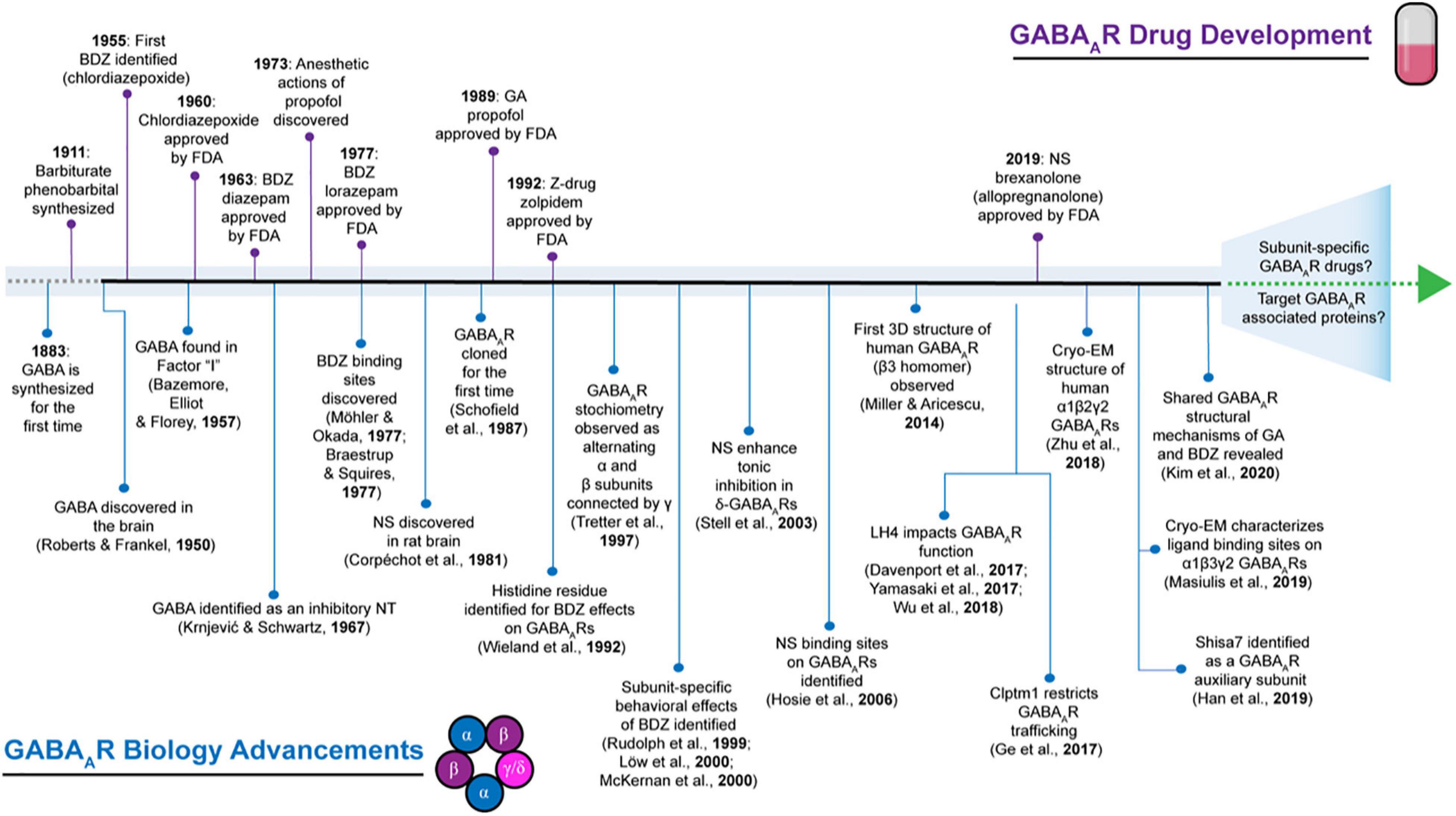
Figure 1. Historical timeline depicting select highlights that have advanced the field of GABAAR biology contrasted to events in GABAAR pharmaceutical development. BDZ, benzodiazepines; EM, electron microscopy; FDA: Food and Drug Administration; GA, general anesthetics; NS, neurosteroids; NT, neurotransmitter.
Given their pivotal role in mediating fast inhibitory neurotransmission, it is not surprising that alterations to GABAAR function are involved in many neurological and psychiatric disorders. GABAAR dysfunction has been observed in a myriad of conditions including seizures, sleep disorders, and anxiety-like disorders (Greenfield, 2013; Nuss, 2015; Engin et al., 2018; Amengual-Gual et al., 2019). Mutations in discrete GABAAR subunits have been shown to impair receptor properties, such as trafficking and ligand sensitivity (Hernandez and Macdonald, 2019; Maljevic et al., 2019). For instance, the first GABAAR subunit mutations associated with epilepsy were discovered in the γ2 subunit (Baulac et al., 2001; Wallace et al., 2001) and since then, a multitude of other mutations have been identified (Hernandez and Macdonald, 2019; Maljevic et al., 2019). Importantly, benzodiazepines remain as frontline drugs in attenuating seizures (Greenfield, 2013; Amengual-Gual et al., 2019) even though a variety of GABAAR-independent pathophysiological alterations can contribute to seizure generation (Stafstrom, 2010). Additionally, anxiety-like behaviors have also been observed concomitantly with dysregulation of inhibitory circuits (Smith and Rudolph, 2012; Smith et al., 2012; Nuss, 2015; Engin et al., 2018) and benzodiazepines continue to stand the test of time as effective anxiolytics (Balon and Starcevic, 2020). Apart from benzodiazepines, the therapeutic potential of neurosteroids have also garnered interest following the recent FDA approval of brexanolone (i.e., allopregnanolone; Mody, 2019) for postpartum depression (PPD) (Figure 1). During pregnancy, increased hormone production has been observed along with enhanced neurosteroid levels, such as allopregnanolone, and transient changes in allopregnanolone concentrations are implicated as a contributing factor to temporary changes in GABA-mediated inhibition, which can result in PPD-associated affective behaviors (Frye et al., 2011; Schüle et al., 2014). The therapeutic utility of neurosteroids in other forms of depression, anxiety, and epilepsies are also currently being explored (Lévesque et al., 2017; Czyk, 2019; Walton and Maguire, 2019; Zorumski et al., 2019; Belelli et al., 2020). We acknowledge that a variety of neurological and neuropsychiatric conditions involve GABA dysregulation (Ramamoorthi and Lin, 2011; Mele et al., 2019), but we have chosen to focus on disorders where GABAAR pharmacology is currently and commonly used: benzodiazepines for epilepsy and anxiety, as well as neurosteroids for PPD. Individuals suffering from these conditions stand the most to gain from the development of novel therapeutic GABAAR-targeting drugs that achieve higher specificity and efficacy, while also mitigating adverse side effects.
Benzodiazepine Actions on GABAARs
GABAARs with high sensitivity to benzodiazepines are typically tri-heteromeric and composed of two α 1-3, 5, two β (1-3), and one γ2 subunit (Farrant and Nusser, 2005). Similar to GABA, subunit composition alters sensitivity to benzodiazepines (Minier and Sigel, 2004). The classical, high-affinity benzodiazepine binding site is present at the extracellular α-γ interface and upon binding, benzodiazepines can increase GABA affinity (Twyman et al., 1989; Lavoie and Twyman, 1996), modulate gating by priming the receptor toward a preactivated step, and affect the rate of desensitization (Twyman et al., 1989; Gielen et al., 2012; Goldschen-Ohm et al., 2014; Jatczak-Śliwa et al., 2018). With respect to single-channel properties, benzodiazepines increase the frequency of channel opening and bursting, with no effect on conductance or channel opening duration (Twyman et al., 1989; Rogers et al., 1994). The actions of benzodiazepines observed at the microscopic level continue to shape our understanding of how inhibitory postsynaptic currents (IPSCs) are modulated. Accordingly, in the presence of benzodiazepines, IPSC decay is prolonged and at some synapses, an increase in amplitude has also been observed (Möhler et al., 2002; Mozrzymas et al., 2007; Karayannis et al., 2010). The efficacy of benzodiazepine potentiation ranges from partial to full allosteric modulation, which is dependent on the binding mode of the benzodiazepine ligand and its effects on GABAAR gating properties (Elgarf, 2018).
Given that the high affinity binding site exists at the α–γ interface, a multitude of side effects can occur following administration due to their non-selective targeting of GABAARs. Classical benzodiazepines interact non-selectively with all GABAARs containing α1-, α2-, α3-, α5-, and γ2 subunits (Moody and Jenkins, 2018). Whereas α1-containing GABAARs have the greatest distribution throughout the brain (Möhler et al., 2002), α2-, α3-, and α5-containing GABAARs are expressed with greater confinement to specific brain regions (Engin et al., 2018). Modulation of α1- and α2-containing GABAARs are associated with the sedative (Rudolph et al., 1999; McKernan et al., 2000; Rowlett et al., 2005) and anxiolytic (Löw et al., 2000) properties of benzodiazepines, respectively. In addition, α3-containing GABAARs have also been implicated with the anxiolytic effects of benzodiazepines (Atack et al., 2005; Dias et al., 2005), but this has also been disputed (Löw et al., 2000; Behlke et al., 2016). Lastly, α5-containing GABAARs may also mediate certain aspects of anxiety behaviors (Botta et al., 2015) and modulation of this subtype can result in anxiolysis (Behlke et al., 2016). As a result of the predominant behavioral effects produced by α1- and α2-containing GABAARs, there have been attempts to develop novel compounds that target these GABAAR subtypes specifically. Most notably, non-benzodiazepines (also known as z-drugs) were developed and are used as sleep aids due to their higher selectivity for α1-containing GABAARs (Atack, 2011; Tan et al., 2011; Cheng et al., 2018; Sieghart and Savić, 2018). However, there are currently no FDA-approved, subtype-selective GABAAR-targeting drugs that function as anxiolytics and are devoid of sedative properties.
One concern associated with long-term usage of benzodiazepines is the development of tolerance based on observations of reduced GABAergic transmission along with altered subunit composition following chronic benzodiazepine treatment (Uusi-Oukari and Korpi, 2010; Jacob et al., 2012; Vinkers and Olivier, 2012; Lorenz-Guertin et al., 2019). The development of benzodiazepine tolerance likely results from decreased GABAAR surface availability due to enhanced inhibition from prolonged drug exposure (Gallager et al., 1984). Interestingly, changes in GABAAR expression appear to be subunit-specific (Uusi-Oukari and Korpi, 2010) and are also dependent on cell-type and brain region (Poisbeau et al., 1997; Jacob et al., 2012; Foitzick et al., 2020). For example, prolonged DZ treatment has been observed to reduce total γ2 expression due to increased lysosomal degradation of this subunit (Lorenz-Guertin et al., 2019). Phosphorylation of GABAARs by specific protein kinase C (PKC) isoforms have been demonstrated to impact benzodiazepine sensitivity in recombinant GABAARs (Leidenheimer et al., 1992; Qi et al., 2007; Nakamura et al., 2015). In addition, calcineurin-dependent dephosphorylation following application of DZ has also been shown to induce endocytosis of GABAARs (Nicholson et al., 2018). Although benzodiazepines non-selectively target α1-3,5/γ2-containing GABAARs throughout the brain, it is possible that variability in benzodiazepine sensitivity among different cell types arises from changes in GABAAR subunit post-translational modifications (PTMs) and/or their interactions with GABAAR-interacting proteins (Jacob et al., 2012). Together, these features could result in variable GABAAR turnover rate in different neuronal populations and may explain why benzodiazepine tolerance for the sedative-hypnotic effects occur more rapidly in comparison to the anticonvulsant/anxiolytic actions (Bateson, 2002; Vinkers and Olivier, 2012), but this supposition requires further clarification.
Furthermore, although acute treatment with benzodiazepines is generally considered safe, their chronic use can result in physical dependence (Soyka, 2017; Silberman et al., 2020) and potentially drug abuse/misuse (Lalive et al., 2011; Tan et al., 2011), which is a major public health concern across the world (Votaw et al., 2019). In general, drugs of abuse “hijack” brain reward circuitry leading to enhanced dopamine (DA) release from the ventral tegmental area (VTA) into the nucleus accumbens (NAc) (Kauer and Malenka, 2007). Mechanistically, benzodiazepines activate α1-containing GABAARs on GABAergic interneurons in the VTA, resulting in disinhibition which promotes DA release (Tan et al., 2010). Additionally, activation of α2-containing GABAARs in the NAc have also been shown to mediate reward learning associated with benzodiazepines (Reynolds et al., 2012; Engin et al., 2014). It is important to note that the abuse liability for benzodiazepines is the highest in individuals who use other drugs of abuse (Silberman et al., 2020). Additionally, the development of physical dependence can occur independently of addiction (Silberman et al., 2020). Lastly, how tolerance develops requires further elucidation, due to observations that the development of tolerance differs based on the usage of the benzodiazepine (Vinkers and Olivier, 2012). One potential reason for disparities observed in tolerance and risk of misuse is due to the overall half-life of the type of benzodiazepine (Vinkers and Olivier, 2012). Thus, targeting GABAARs based on subunit-specificity continues to remain important not only toward understanding the roles of different subtypes in circuits and behaviors, but also for achieving optimal therapeutic efficacy while mitigating adverse effects.
Neurosteroid Actions on GABAARs
In contrast to the historical usage of benzodiazepines as pharmacotherapy, neurosteroids are the newest class of GABAAR-targeting drugs, with brexanolone as the first therapy indicated for the treatment of PPD (Cristea and Naudet, 2019; Mody, 2019). Neurosteroids are robust modulators of both synaptic and extrasynaptic GABAARs (Belelli and Lambert, 2005; Belelli et al., 2020). Thus, their effects on GABAergic transmission are mediated by prolonging phasic inhibition, as well as increasing tonic conductance. Neurosteroid binding sites on GABAARs were initially discovered within the transmembrane domains of the α1β2γ2 between the α and β subunit interface. and key residues regulating their binding are largely conserved among α2-5/β3γ2 and α4β3δ subtypes (Hosie et al., 2006, 2009). Additionally, neurosteroids potentiate GABA-mediated currents with higher efficacy in δ-containing GABAARs compared to γ2-containing GABAARs (Bianchi and Macdonald, 2003; Belelli et al., 2020). Comparable to benzodiazepines, neurosteroids increase the frequency of single channel openings, but also prolong the channel open duration similarly to barbiturates (Twyman and Macdonald, 1992; Belelli et al., 2020). Furthermore, neurosteroids enhance the duration of IPSCs by prolonging the decay time (Lambert et al., 2003). Interestingly, relatively high concentrations (>100 nM) of neurosteroids can directly activate GABAARs in the absence of GABA (Belelli and Lambert, 2005). These observations may potentially be clinically relevant given that altered neurosteroid concentrations in the brain have been observed in neuropsychiatric conditions such as PPD, anxiety, and stress (Purdy et al., 1991; Schumacher et al., 2003; Belelli et al., 2020).
It has been previously demonstrated that knock-in mice with α2-containing GABAARs rendered insensitive to neurosteroid potentiation for this subtype exhibit anxiety-like behaviors without displaying depressive-like phenotypes nor effects on analgesia (Durkin et al., 2018). These observations potentially suggest a specific role for how neurosteroids might be useful as anxiolytics given that α2-containing GABAARs have already been associated with the anxiolytic effects of benzodiazepines (Löw et al., 2000). While the behavioral effects of neurosteroids can be interrogated based on their interaction with distinct GABAAR subtypes (Belelli et al., 2020), achieving subtype-selectivity may prove challenging due to conserved binding sites among synaptic and extrasynaptic GABAARs (Hosie et al., 2007, 2009). Neurosteroid modulation of GABAARs are also regulated by phosphorylation status, which affects receptor expression and/or surface trafficking, as well as neurosteroid sensitivity (Smith et al., 2007; Abramian et al., 2014; Comenencia-Ortiz et al., 2014; Nakamura et al., 2015). Distinct neurons have been observed to exhibit differences in their sensitivity to neurosteroids due to changes in phosphorylation mediated by different kinases, such as protein kinase A (PKA) or PKC (Fáncsik et al., 2000; Hodge et al., 2002; Vicini et al., 2002; Harney et al., 2003; Kia et al., 2011). Together, both phosphorylation of GABAAR subunits and changes to endogenous neurosteroid levels can have profound effects on phasic and tonic inhibition, contributing to the heterogeneity of inhibition across brain regions.
There has been growing interest in the therapeutic development of neurosteroids for potential application in other forms of depression such as major depressive disorder (zuranolone; Sage Therapeutics) and treatment-resistant depression (zuranolone; Sage Therapeutics, ganaxolone; Marinus Pharmaceuticals). Indeed, it has been observed that neurosteroid levels are reduced in depression and treatment with various antidepressants can normalize these concentrations (Lüscher and Möhler, 2019). Given the comorbidity of anxiety and depression, the potential for neurosteriods being employed as effective anxiolytics are also being explored (Schüle et al., 2014; Czyk, 2019; Gunduz-Bruce et al., 2019; Lüscher and Möhler, 2019; Zorumski et al., 2019). Additionally, neurosteroids are also being considered for use in seizure disorders such as refractory status epilepticus and PCDH19-related epilepsy (ganaxolone; Marinus Pharmaceuticals). Polypharmacy involving administration of both benzodiazepines and neurosteroids together are also being considered for mitigating epileptic seizures (Rogawski et al., 2020). Taken together, there is the possibility that further development of neurosteroids as a therapeutic agent will be applicable to other neurological disorders.
Recent Advances in GABAAR Biology
Extensive characterization into the physiological and pharmacological properties of distinct GABAARs continues to provide insight toward their relevance at the cellular, circuit, and behavioral level. The past two decades have seen the development of subtype-selective compounds targeting GABAARs with specific pharmacological and behavioral actions (Rudolph and Knoflach, 2011; Sieghart and Savić, 2018; Chen et al., 2019). Additionally the recent emergence of structural data revealing GABAARs bound with different ligands will certainly help refine and guide drug development (Olsen et al., 2019). Differences in expression profiles and receptor function among GABAAR subtypes, subunit variants due to alternative splicing (Boileau et al., 2003, 2010; Eom et al., 2011; Miller et al., 2018), and mechanisms that preferentially enrich mRNA transcripts of discrete GABAAR subunits at specific subcellular regions (Rajgor et al., 2020), continue to highlight the diversity of GABAergic signaling in neuronal inhibition. Furthermore, the discovery of transmembrane proteins that associate with GABAARs are beginning to shed light on their molecular mechanisms in vivo and may also provide strategies for targeting select GABAAR subtypes and in turn, lead to the development of more effective therapeutics for neurological and psychiatric disorders.
Structural Insights Into GABAARs and Signaling Mechanisms
Molecular modeling of drug-receptor interactions relies on high-resolution structures and aims to characterize not only the spatial architecture of the receptor, but how ligand binding induces changes in receptor conformation and influences channel gating (Olsen et al., 2019). Several structures of AMPA receptors (AMPARs) bound to ligands and/or in complex with auxiliary subunits help exemplify how these interactions are critical for modulating AMPAR function (Herguedas et al., 2019; Nakagawa, 2019; Zhao et al., 2019; Kamalova and Nakagawa, 2020). In contrast, until recently, there has been a lack of structural data regarding GABAARs, which has limited our understanding of how these receptors structurally interact with their ligands. New studies using cryogenic electron microscopy (cryo-EM) to examine full-length, synaptic GABAARs are now available and will be important for bridging the link between receptor architecture and their pharmacological signaling mechanisms (Laverty et al., 2019; Masiulis et al., 2019; Kim et al., 2020). In addition to high affinity benzodiazepine sites at the α-γ interface, other benzodiazepine binding sites on GABAARs have also been inferred (Walters et al., 2000; Baur et al., 2008; Ramerstorfer et al., 2011; Wongsamitkul et al., 2017; Sigel and Ernst, 2018; Lian et al., 2020). Recently, cryo-EM studies confirmed the presence of a low-affinity binding site present within the transmembrane domain of the αβ interface as described in earlier studies (Walters et al., 2000; Ramerstorfer et al., 2011; Sigel and Ernst, 2018; Masiulis et al., 2019; Lian et al., 2020). Moreover cryo-EM studies have identified the presence of other distinct benzodiazepine binding sites, specifically within the β-α and γ-β interfaces (Masiulis et al., 2019; Kim et al., 2020). It has been shown that DZ concentration-response curves exhibit biphasic responses (Walters et al., 2000; Baur et al., 2008; Ramerstorfer et al., 2011; Wongsamitkul et al., 2017). Potentiation of GABA by high concentrations of DZ (>20 μM) is thought to be mediated by a low-affinity binding site and submaximal GABA responses from α1β2 receptors lacking the γ2 subunit have been shown to be potentiated by high concentrations of DZ, which were not blocked by flumazenil (Walters et al., 2000; Baur et al., 2008; Ramerstorfer et al., 2011; Wongsamitkul et al., 2017). Although the amount of DZ used in this particular study far exceeds the concentration needed for their therapeutic effects (<0.3 μM), activation of this low-affinity site may potentially mediate the anesthetic properties of benzodiazepines (Walters et al., 2000; Olsen, 2018; Masiulis et al., 2019; Kim et al., 2020). While this study only assessed the effects of DZ in α1β2 and α1β2γ2 GABAARs (Walters et al., 2000), it should be noted that other benzodiazepine binding sites distinct from the α-γ interface appear to be present in cryo-EM structures of both α1β2γ2 (Kim et al., 2020) and α1β3γ2 GABAARs (Walters et al., 2000; Olsen, 2018; Masiulis et al., 2019). Interestingly, when comparing the binding of partial and full benzodiazepine agonists, partial benzodiazepine agonists appear to sit deeper within the α/γ binding pocket and could explain differences in efficacy between DZ and bretazenil, respectively (Masiulis et al., 2019). Although these studies highlight the discovery of other unique benzodiazepine binding sites, there are currently no studies that have demonstrated that these unique, low-affinity binding sites can mediate the anesthetic effects of benzodiazepines in isolation. In the future, it would be interesting to explore the conditions that facilitate the activity of GABAARs through these low-affinity sites and whether these sites can be selectively targeted. It also is possible that future cryo-EM structures may reveal subtle differences in the benzodiazepine binding pocket among distinct, benzodiazepine-sensitive GABAARs, which could help in the development of more subtype-selective compounds.
Structural data has also highlighted the importance of key residues (Hosie et al., 2006) and the binding modes of distinct neurosteroid ligands on GABAARs (Laverty et al., 2017; Miller et al., 2017; Sugasawa et al., 2020). Previously, it was thought that the allosteric modulation and direct activation of GABAARs by neurosteroids were due to binding on distinct sites, at the α subunit and the αβ interface, respectively (Hosie et al., 2006). However, structures of GABAAR chimeras bound with tetrahydrodeoxycorticosterone (THDOC) later revealed that both actions resulted from binding at the same site (Laverty et al., 2017). It was also shown that neurosteroids, such as pregnanolone sulfate, occupy a separate site within the intra-subunit transmembrane domain of the α subunit distinct from THDOC (Laverty et al., 2017). Accordingly, it has recently been shown that different neurosteroid ligands can promote either the activation or desensitization of α1β3 GABAARs by binding to the intersubunit interface of the β-α subunits or within the intrasubunit on β3, respectively (Laverty et al., 2017; Miller et al., 2017; Sugasawa et al., 2020). Currently, it remains unknown whether neurosteroid binding sites among synaptic and extrasynaptic GABAAR are structurally distinct when ligand bound and if these differences can be exploited for the development of novel neurosteroids with varying efficacy and specificity. However, to date, there are a lack of neurosteroid-based ligands designed for subtype-selectivity (Althaus et al., 2020), such as exclusively targeting α2-containing GABAARs for their anxiolytic properties without modulating α4-containing GABAARs. Similar to benzodiazepines, future cryo-EM studies may also uncover unique differences among neurosteroid binding sites in different GABAAR subtypes that can be therapeutically leveraged.
Spatial Expression Profiles of GABAAR Subtypes and GABAAR Subunit Variants
The circuit and behavioral roles mediated by specific GABAAR subtypes are dependent on their abundance in precisely defined regions. High GABAAR sensitivity to benzodiazepines is conferred by a histidine residue in the N-terminal extracellular region (Wieland et al., 1992) and is specific to GABAARs containing α1-, α2-, α3-, and/or α5 subunits. However, GABAARs containing these subunits can be found across the brain and overall modulation of these GABAARs contributes to both the desired therapeutic effect, but also some of the side effects. Therefore, it is important to understand how brain regions are modulated in a circuit-specific manner and the physiological role of GABAARs subtypes within brain regions.
With respect to the anxiolytic effects of benzodiazepines, the amygdala has received prominent attention due to its role in mediating emotional responses and this structure can be divided into discrete subdivisions based on cell-type, circuit, and physiological role (Ehrlich et al., 2009). Differences in GABAAR subtypes expressed in subdivisions of the amygdala have been observed (Fritschy and Mohler, 1995; Pirker et al., 2000; Kaufmann et al., 2003; Fujimura et al., 2005). Although nearly all GABAARs are expressed throughout the amygdala, the region and cellular localization of these GABAAR subtypes can impact anxiety behaviors (Engin et al., 2018). For example, in the central amygdala (CeA), α5-containing GABAARs are associated with anxiety-like behaviors in a cell-specific manner (Haubensak et al., 2010; Botta et al., 2015). Specifically, knockdown (KD) of α5-containing GABAARs in PKCδ+ neurons results in anxiogenesis, highlighting the importance of subtype- and cell-specific regulation of anxiety-like behaviors (Haubensak et al., 2010; Herman et al., 2013; Botta et al., 2015). Additionally, α1-containing GABAARs are localized on corticosterone releasing factor neurons and have been shown to contribute to anxiety-like phenotypes possibly through the regulation of neuronal excitability (Gafford et al., 2012; Herman et al., 2013). However, the specific role of α1-containing GABAAR activity within the CeA with respect to control over anxiety-like behaviors has yet to fully be determined (Engin et al., 2018). In line with these observations, benzodiazepines have also been shown to impact CeA activity and anxiety-like behaviors (Carvalho et al., 2012; Botta et al., 2015; Griessner et al., 2018). Although this could also be due to a higher degree of α2-containing GABAAR expression (Fritschy and Mohler, 1995; Pirker et al., 2000), the cell-type and circuit-specific role of α2-containing GABAARs in CeA is not completely understood (Engin et al., 2018). Further complications teasing out the effects of benzodiazepines come from the fact that benzodiazepines non-selectively modulate all α1-3, 5-containing GABAARs distributed across the entire amygdala (Fritschy and Mohler, 1995; Pirker et al., 2000; Engin et al., 2018). Taken together, the complexity regarding the microcircuitry governing anxiety and how both region- and cell-specific expression of GABAAR subtypes can impact anxiety behaviors still requires further investigation.
Furthermore, subcellular localization of GABAARs also profoundly determines the type of neuronal inhibition exhibited and how GABAAR-targeting drugs will modulate neuronal activity (Kerti-Szigeti and Nusser, 2016; Nathanson et al., 2019; Kramer et al., 2020). The mobility and diffusion of GABAARs between synaptic and extrasynaptic regions are dependent on subunit composition, specific motifs within the intracellular domain of certain subunits, and the interaction of GABAARs with scaffolding partners such as gephyrin or radixin (Jacob et al., 2005; Thomas et al., 2005; Loebrich et al., 2006; Bannai et al., 2009; Mukherjee et al., 2011; Tyagarajan and Fritschy, 2014; Hausrat et al., 2015; Hannan et al., 2019; Davenport et al., 2020). Recently, there have been reports of differences in synaptic and extrasynaptic GABAAR subunit localization, as well as neuron-specific differences (Schulz et al., 2018; Magnin et al., 2019). For example, it is well-established that in hippocampal pyramidal neurons, the α5 subunit is predominantly expressed at extrasynaptic regions and mediates the majority of tonic inhibition (Crestani et al., 2002; Caraiscos et al., 2004; Farrant and Nusser, 2005; Glykys and Mody, 2007; Glykys et al., 2008). Interestingly, α5-containing GABAARs can also localize synaptically and contribute to phasic inhibition (Brady and Jacob, 2015; Schulz et al., 2018; Davenport et al., 2020). Furthermore, in hippocampal somatostatin interneurons, α5-containing GABAARs appear to be localized synaptically as they co-localize with VGAT and are targeted by vasoactive intestinal polypeptide (VIP)- and calretinin-expressing (Schulz et al., 2018; Magnin et al., 2019), but not parvalbumin interneurons. In addition, α5-GABAARs targeted by VIP interneurons appear to be involved in anxiety-like behaviors (Magnin et al., 2019). More work is needed to understand how distinct GABAARs are targeted by different inhibitory inputs and how differences in compartment localization can dynamically regulate neuronal activity. For example, it is currently unknown whether pharmacological targeting of perisomatic or dendritic inhibition exclusively can result in better therapeutic efficacy. Although currently not feasible, future endeavors examining the specific roles of GABAARs and their contributions to perisynaptic and/or dendritic inhibition will provide a more mechanistic understanding of input-specific inhibition and could perhaps allow these mechanisms to be manipulated pharmacologically.
In addition, GABAAR splice variants with differences in function and pharmacology continue to further enhance the diversity and classification of GABAAR subtypes (Whiting et al., 1990; Boileau et al., 2010; Miller et al., 2018; Smart and Stephenson, 2019; Rajgor et al., 2020). For example, the γ2 subunit can exist as either a long (γ2L) or short (γ2S) variant, with the latter missing eight amino acids in the long intracellular loop (Whiting et al., 1990; Boileau et al., 2010). Functionally, γ2S differs from γ2L in zinc sensitivity and kinetics, and the surface expression of γ2S alone is possible even when co-transfected with α and β subunits (Boileau et al., 2003, 2010). Additionally, γ2L and γ2S expression changes over the course of development and their expression is confined to different brain regions (Wang and Burt, 1991; Gutiérrez et al., 1996). Noteworthily, it has also been observed that in schizophrenia, γ2S is decreased (Huntsman et al., 1998) which elicits the question as to whether γ2L and/or γ2S are differentially involved in other neurological and neuropsychiatric conditions. Lastly, PTMs can influence GABAAR properties and phosphorylation of the serine site S343 which is exclusive to the γ2L variant and not in γ2S (Lorenz-Guertin et al., 2018) has been documented (Nakamura et al., 2015). However, more research is required in order to identify other residues within the γ2L and γ2S variants that are subject to phosphorylation or other PTMs, as well as how these PTMs modulate GABAAR properties. With newer tools that can achieve greater drug targeting specificity (Shields et al., 2017; Atasoy and Sternson, 2018; Rao et al., 2019; Crocetti and Guerrini, 2020) along with rational drug design (Antkowiak and Rammes, 2019; Scott and Aricescu, 2019), it may be possible in the near future for drug development strategies to exploit these differences in order to maximize the therapeutic efficacy of newer compounds while also decreasing the likelihood of unwanted side effects by “sparing” other GABAAR subtypes.
GABAAR-Associated Transmembrane Proteins
Although a majority of compounds have been developed that target the pore-forming subunits of GABAARs, recent advances in the field of GABAAR biology have illuminated that native GABAAR exist as a complex with other accessory proteins rather than in isolation (Khayenko and Maric, 2019). Within the past decade (Figure 1), novel transmembrane proteins have been discovered that associate with native GABAARs and have distinct effects on receptor trafficking, kinetics, and/or pharmacology (see Han et al., 2020 for an in-depth review). Therefore, these proteins present themselves as potentially novel sites for new GABAAR drug development.
Lipoma HMGIC Fusion Partner-Like 4
Lipoma HMGIC fusion partner-like 4 (Lhfpl4, LH4; also referred to as GABAAR Regulatory Lhfpl4 [GARLH4]) is a four-pass transmembrane protein that was recently shown to critically regulate GABAAR anchoring at inhibitory synapses and thus impact the strength of fast inhibitory synaptic transmission. Indeed, both KD (Yamasaki et al., 2017) and knockout (KO) (Davenport et al., 2017; Wu et al., 2018) of LH4 resulted in decreased GABAAR clustering, as well as diminished GABAergic synaptic transmission and GABAergic synapse density (Davenport et al., 2017; Yamasaki et al., 2017; Wu et al., 2018). Additionally, LH4 forms a tripartite complex with GABAARs and neuroligin-2 (NL2) (Davenport et al., 2017; Yamasaki et al., 2017; Wu et al., 2018), a postsynaptic inhibitory cell adhesion molecule (Poulopoulos et al., 2009; Li et al., 2017b; Lu et al., 2017). Interestingly, the δ subunit plays an important role in the regulation of GABAAR assembly within the cerebellum by preventing incorporation of γ2 and LH4 (Martenson et al., 2017). Incorporation of the δ subunit prevented assembly of γ2 into GABAARs, as well as the interaction with LH4 (Martenson et al., 2017). In this manner, δ-containing GABAARs became extrasynaptically localized whereas γ2-containing GABAARs were localized at synapses via their interaction with both LH4 and NL2 (Davenport et al., 2017; Martenson et al., 2017; Yamasaki et al., 2017; Wu et al., 2018). Lastly, although LH4 did not alter sensitivity to endogenous GABA, THIP, or picrotoxin (Yamasaki et al., 2017), LH4-dependent modulation of other GABAAR-targeting compounds still requires further investigation.
Cleft Lip and Palate Transmembrane Protein 1
Abnormal trafficking of GABAARs can involve a variety of mechanisms, leading to a lack of GABAAR availability at the neuronal surface. Specifically, a novel transmembrane protein, cleft lip and palate transmembrane protein 1 (Clptm1) was identified as a negative regulator of GABAAR forward trafficking of both synaptic and extrasynaptic GABAARs through receptor confinement primarily in the ER and reduced the surface availability of GABAARs (Ge et al., 2018). Importantly, this modulation of GABAAR forward trafficking impacted inhibitory transmission bi-directionally; overexpression and KD of Clptm1 resulted in diminished and enhanced postsynaptic inhibitory responses, respectively. Additionally, this bi-directional effect was similarly observed in tonic currents generated from extrasynaptic GABAARs. Mechanistically, these data suggest that Clptm1 is a pan-GABAAR regulator and thus impacts both synaptic and tonic inhibition through restriction of receptor forward trafficking.
Shisa7
Members of the Shisa family of proteins are single-pass transmembrane proteins containing both cysteine and proline rich domain on the N- and C-terminus, respectively (Pei and Grishin, 2012). Specifically, Shisa6-9 are referred to as cystine knot AMPAR membrane proteins (CKAMP) (Farrow et al., 2015) due to the presence of an AMPAR interacting domain in the C-terminus (von Engelhardt, 2019). Notably, Shisa7 (CKAMP59) has emerged as an interesting member of the Shisa family in that unlike other CKAMP counterparts, Shisa7 has a direct role in GABAAR regulation at inhibitory synapses (Han et al., 2019). While other CKAMPs are localized at glutamatergic synapses (von Engelhardt et al., 2010; Klaassen et al., 2016; Peter et al., 2020), we observed that Shisa7 co-localizes specifically with gephyrin and GABAARs in hippocampal neurons (Han et al., 2019) and not at excitatory synapses as reported in an earlier study (Schmitz et al., 2017). Functionally, Shisa7 regulated GABAAR trafficking and inhibitory transmission without affecting excitatory synaptic transmission (Han et al., 2019). Strikingly, Shisa7 also modulates GABAAR kinetics and pharmacological properties. Indeed, in heterologous cells, Shisa7 decreased the deactivation time constants of α1β2γ2 and α2β3γ2 receptors, and conversely Shisa7 KO prolonged the decay time constant of GABAergic transmission in hippocampal neurons (Han et al., 2019). Lastly, Shisa7 increased DZ-induced potentiation of GABAARs in heterologous cells and Shisa7 KO significantly reduced DZ actions in vivo (Han et al., 2019). Taken together, this is the first documentation of a transmembrane auxiliary subunit unique to GABAARs that can influence receptor trafficking, kinetics, and pharmacology.
Targeting Transmembrane Accessory Molecules That Interact With Native GABAARs
Although 19 different GABAARs subunits have been identified, there are a multitude of different possible subunit combinations that can occur. Thus, one potential obstacle to overcome regarding the development of subtype-specific GABAAR-targeting drugs is achieving better therapeutic efficacy and selectivity. One strategy is to “think outside the receptor” and evaluate whether there are “druggable” targets that coexist with native GABAARs independent of the pore-forming subunits. For example, gephyrin is a well-known postsynaptic scaffolding protein that associates with GABAARs at inhibitory synapses and dysregulation of gephyrin possibly contributes to disrupted GABAAR signaling in disease (Tyagarajan and Fritschy, 2014). A recent preliminary study identified that artemisinin, an anti-malarial compound, could bind to gephyrin and subsequently affect GABAAR-mediated signaling in pancreatic cells, suggesting within this context that artemisinins could prove useful in treating diabetes (Li et al., 2017a). Additionally, crystallography studies identified that artemisinin and its derivatives bind to the GABAAR binding pocket in gephyrin and resulted in destabilization of gephyrin, as well as α1- and α2-containing GABAARs (Kasaragod et al., 2019). This exciting piece of evidence suggests that proteins that interact with GABAARs can be targeted and subsequently impact GABAAR signaling, making them ripe candidates for new drug development.
In addition to GABAAR-associated scaffolds and molecular adaptor proteins (Khayenko and Maric, 2019), newly identified transmembrane proteins that interact with GABAARs are additional targets that can potentially be exploited in drug development. In fact, transmembrane AMPAR regulatory proteins (TARPs), which are auxiliary subunits of AMPA receptors (Ziff, 2007; Milstein and Nicoll, 2008), are currently being evaluated for the treatment of epilepsy and pain (Maher et al., 2017). Thus, the discovery of novel GABAAR-associated transmembrane proteins (LH4, Clptm1, and Shisa7) as discussed above provide a potentially exciting opportunity for drug development. For example, Shisa7 can modulate GABAAR kinetics and pharmacology (Han et al., 2019). Thus, it will be interesting to investigate whether there are any compounds that can interact with Shisa7 and/or other transmembrane proteins, or their interfaces with GABAARs, to produce clinically relevant effects. In terms of selectivity, LH4 could potentially offer an opportunity to selectively target γ2-containing GABAARs while “sparring” δ-containing GABAARs. Collectively, these initial characterizations of transmembrane GABAAR regulators have provided the foundation for a new understanding of GABAAR-mediated mechanisms of inhibitory control (Han et al., 2020), and present new potential targets for GABAAR drug screening.
Summary and Future Outlook
Characterization and investigation of the various binding sites on GABAARs have provided invaluable data for the development of pharmaceuticals that are used to treat a wide variety of neurological conditions and psychiatric disorders. However, there are still many challenges and issues to be faced with the current state of available GABAAR-targeting drugs. One of the biggest challenges comes from ubiquitous expression of GABAAR subtypes throughout the brain. This can prove to be problematic when considering off-target drug effects and unforeseen complications from drug administration due to drug binding across many GABAAR subtypes in different brain regions. Considering the nature of current GABAAR pharmaceuticals, the design of these drugs is based on previously characterized binding sites, such as the high affinity benzodiazepine binding site which exists between the α and γ subunits (Moody and Jenkins, 2018). However, there is still a lack of new and clinically relevant subunit-specific GABAAR-targeting drugs despite scientific success in furthering our understanding of GABAAR structure and function (Figure 1). The need for subtype-specific GABAAR-targeting drugs is not solely confined to clinical applications, but is also needed in biomedical research. The expression of specific GABAARs in unique cell populations and the lack of compounds for certain subunits, such as a commercially available δ subunit-selective antagonist, only highlights the importance for the future development of more highly selective compounds and will help address the role of specific GABAARs at the cellular, circuit, and behavioral level.
Although initial studies have characterized the role of transmembrane GABAAR accessory proteins within the hippocampus and cerebellum (Martenson et al., 2017; Yamasaki et al., 2017; Ge et al., 2018; Wu et al., 2018; Han et al., 2019), it has not yet been investigated whether these observations apply to other brain regions. Therefore, the possibility exists that these proteins might differentially impact GABAAR function within discrete brain regions which contributes to their impact on physiology and behavior, as well as the pharmacological effect of drugs. While GABAARs are expressed throughout the brain, the potential region-specific distribution of GABAAR-associated transmembrane proteins could enhance the selectivity for future GABAAR-targeting drugs. Considering this, further investigation requires cell- and circuit-specific interrogation to define how these accessory proteins function in different brain regions. Understanding how these transmembrane proteins associate with GABAAR and their role within defined brain structures could potentially allow for the development of drugs that target these GABAAR-associated transmembrane proteins directly or compounds that work synergistically with other GABAAR-targeting drugs to enhance their therapeutic efficacy and limit unwanted side effects.
To complement structural and binding studies, genetic studies have also proved invaluable in our understanding of GABAARs. For example, knockin mice harboring histidine-to-arginine mutations in α1- α2- α3-, or α5- GABAAR subunits render them insensitive to benzodiazepines (Rudolph et al., 1999; Löw et al., 2000; McKernan et al., 2000; Rudolph and Möhler, 2004) and have provided critical insight and direction toward the development of several subtype-selective ligands (Rudolph and Möhler, 2006; Rudolph and Knoflach, 2011; Richter et al., 2012; Forman and Miller, 2016; Yamaura et al., 2016; Solomon et al., 2019). However, genetic approaches also have limitations and can pose a challenge in studying specific contributions of discrete GABAAR subtypes, as well as modeling alterations to GABAAR function in disease. For example, GABAAR expression and subunit composition change throughout development and there are differences in GABAAR subtypes within various brain regions (Luscher et al., 2011), which creates difficulty in studying the role of GABAAR in KO models. Genetic deletion of subunits can also create complications when studying discrete subunit contributions to GABAAR function. For example, complete KO of the γ2 subunit results in death shortly after birth (Günther et al., 1995), preventing a functional analysis of γ2 subunit in vivo at later time points. Furthermore, genetic deletion of α subunits can result in compensatory effects. Indeed, deletion of the α1 subunit can promote upregulation of other α-containing subtypes in response (Sur et al., 2001; Kralic et al., 2002a, b, 2006). Additionally, deletion of the γ2 subunit can lead to compensation through replacement of synaptic GABAARs with the γ3 subunit (Kerti-Szigeti et al., 2014). Thus, the development of more selective drugs would allow for precise investigation as to the role of subtype-selective GABAARs and further elucidates the role of GABAARs in health and disease.
A major goal in GABAAR drug discovery has been to discover compounds that are more selective and efficacious with less side effects. Although classical benzodiazepines have been successfully used to treat a wide variety of conditions (Chen et al., 2019), their non-selective binding to essentially all γ2-containing GABAARs can account for many of their side effects (Engin et al., 2018; Chen et al., 2019). The recent emergence of several high-resolution structures of GABAARs (Laverty et al., 2017, 2019; Miller et al., 2017; Phulera et al., 2018; Zhu et al., 2018; Masiulis et al., 2019) will be important for precision-based drug design that enhances drug selectivity for discrete receptor subtypes. Excitingly, the discovery of transmembrane GABAAR accessory proteins will likely provide further opportunities to develop compounds that can target GABAARs in complex with other accessory proteins, but not bind and modulate GABAARs in isolation. In summary, there is a need for future interrogation of GABAAR pharmacology which takes into account distinct subunit compositions, discrete brain region localizations, and associated GABAAR proteins that better mimic native receptor complexes when designing future pharmaceuticals.
Author Contributions
All authors listed have made a substantial, direct and intellectual contribution to the work, and approved it for publication.
Funding
This work was supported by the NIH Intramural Research Program to WL. DC is supported by a NINDS Diversity Training Fellowship. RS is supported by a Postdoctoral Research Fellowship from the Center on Compulsive Behaviors (CCB).
Conflict of Interest
The authors declare that the research was conducted in the absence of any commercial or financial relationships that could be construed as a potential conflict of interest.
References
Abramian, A. M., Comenencia-Ortiz, E., Modgil, A., Vien, T. N., Nakamura, Y., Moore, Y. E., et al. (2014). Neurosteroids promote phosphorylation and membrane insertion of extrasynaptic GABAA receptors. Proc. Natl. Acad. Sci. U.S.A. 111, 7132–7137. doi: 10.1073/pnas.1403285111
Althaus, A. L., Ackley, M. A., Belfort, G. M., Gee, S. M., Dai, J., Nguyen, D. P., et al. (2020). Preclinical characterization of zuranolone (SAGE-217), a sele neuroactive steroid GABAA receptor positive allosteric modulator. Neuropharmacology 181:108333. doi: 10.1016/j.neuropharm.2020.108333
Amengual-Gual, M., Sánchez Fernández, I., and Wainwright, M. S. (2019). Novel drugs and early polypharmacotherapy in status epilepticus. Seizure 68, 79–88. doi: 10.1016/j.seizure.2018.08.004
Antkowiak, B., and Rammes, G. (2019). GABA(A) receptor-targeted drug development -New perspectives in perioperative anesthesia. Expert Opin. Drug Discov. 14, 683–699. doi: 10.1080/17460441.2019.1599356
Atack, J. R. (2011). GABAA receptor subtype-selective modulators. I. α2/α3-selective agonists as non-sedating anxiolytics. Curr. Top. Med. Chem. 11, 1176–1202. doi: 10.2174/156802611795371350
Atack, J. R., Hutson, P. H., Collinson, N., Marshall, G., Bentley, G., Moyes, C., et al. (2005). Anxiogenic properties of an inverse agonist selective for α3 subunit-containing GABAA receptors. Br. J. Pharmacol. 144, 357–366. doi: 10.1038/sj.bjp.0706056
Atasoy, D., and Sternson, S. M. (2018). Chemogenetic tools for causal cellular and neuronal biology. Physiol. Rev. 98, 391–418. doi: 10.1152/physrev.00009.2017
Balon, R., and Starcevic, V. (2020). Role of benzodiazepines in anxiety disorders. Adv. Exp. Med. Biol. 1191, 367–388. doi: 10.1007/978-981-32-9705-0_20
Bannai, H., Lévi, S., Schweizer, C., Inoue, T., Launey, T., Racine, V., et al. (2009). Activity-dependent tuning of inhibitory neurotransmission based on GABAAR diffusion dynamics. Neuron 62, 670–682. doi: 10.1016/j.neuron.2009.04.023
Barnard, E. A., Darlison, M. G., and Seeburg, P. (1987). Molecular biology of the GABAA receptor: the receptor/channel superfamily. Trends Neurosci. 10, 502–509. doi: 10.1016/0166-2236(87)90130-5
Bateson, A. N. (2002). Basic pharmacologic mechanisms involved in benzodiazepine tolerance and withdrawal. Curr. Pharm. Des. 8, 5–21. doi: 10.2174/1381612023396681
Baulac, S., Huberfeld, G., Gourfinkel-An, I., Mitropoulou, G., Beranger, A., Prud’homme, J.-F., et al. (2001). First genetic evidence of GABA A receptor dysfunction in epilepsy: a mutation in the γ2-subunit gene. Nat. Genet. 28, 46–48. doi: 10.1038/ng0501-46
Baur, R., Tan, K. R., Lüscher, B. P., Gonthier, A., Goeldner, M., and Sigel, E. (2008). Covalent modification of GABAA receptor isoforms by a diazepam analogue provides evidence for a novel benzodiazepine binding site that prevents modulation by these drugs. J. Neurochem. 106, 2353–2363. doi: 10.1111/j.1471-4159.2008.05574.x
Behlke, L. M., Foster, R. A., Liu, J., Benke, D., Benham, R. S., Nathanson, A. J., et al. (2016). A pharmacogenetic “restriction-of-function” approach reveals evidence for anxiolytic-like actions mediated by α5-containing gabaa receptors in mice. Neuropsychopharmacology 41, 2492–2501. doi: 10.1038/npp.2016.49
Belelli, D., Hogenkamp, D., Gee, K. W., and Lambert, J. J. (2020). Realising the therapeutic potential of neuroactive steroid modulators of the GABAA receptor. Neurobiol. Stress 12:100207. doi: 10.1016/j.ynstr.2019.100207
Belelli, D., and Lambert, J. J. (2005). Neurosteroids: endogenous regulators of the GABA(A) receptor. Nat. Rev. Neurosci. 6, 565–575. doi: 10.1038/nrn1703
Ben-Ari, Y., Khalilov, I., Kahle, K. T., and Cherubini, E. (2012). The GABA excitatory/inhibitory shift in brain maturation and neurological disorders. Neuroscientist 18, 467–486. doi: 10.1177/1073858412438697
Benkwitz, C., Banks, M. I., and Pearce, R. A. (2004). Influence of GABAA receptor γ2 splice variants on receptor kinetics and isoflurane modulation. Anesthesiology 101, 924–936. doi: 10.1097/00000542-200410000-00018
Bianchi, M. T., and Macdonald, R. L. (2003). Neurosteroids shift partial agonist activation of GABAA receptor channels from low- to high-efficacy gating patterns. J. Neurosci. 23, 10934–10943. doi: 10.1523/JNEUROSCI.23-34-10934.2003
Boileau, A. J., Li, T., Benkwitz, C., Czajkowski, C., and Pearce, R. A. (2003). Effects of γ2S subunit incorporation on GABAA receptor macroscopic kinetics. Neuropharmacology 44, 1003–1012. doi: 10.1016/S0028-3908(03)00114-X
Boileau, A. J., Pearce, R. A., and Czajkowski, C. (2010). The short splice variant of the gamma 2 subunit acts as an external modulator of GABA(A) receptor function. J. Neurosci. 30, 4895–4903. doi: 10.1523/JNEUROSCI.5039-09.2010
Botta, P., Demmou, L., Kasugai, Y., Markovic, M., Xu, C., Fadok, J. P., et al. (2015). Regulating anxiety with extrasynaptic inhibition. Nat. Neurosci. 18, 1493–1500. doi: 10.1038/nn.4102
Brady, M. L., and Jacob, T. C. (2015). Synaptic localization of α5 GABA (A) receptors via gephyrin interaction regulates dendritic outgrowth and spine maturation. Dev. Neurobiol. 75, 1241–1251. doi: 10.1002/dneu.22280
Brickley, S. G., and Mody, I. (2012). Extrasynaptic GABAA receptors: their function in the CNS and implications for disease. Neuron 73, 23–34. doi: 10.1016/j.neuron.2011.12.012
Caraiscos, V. B., Elliott, E. M., You-Ten, K. E., Cheng, V. Y., Belelli, D., Glen Newell, J., et al. (2004). Tonic inhibition in mouse hippocampal CA1 pyramidal neurons is mediated by α5 subunit-containing γ-aminobutyric acid type A receptors. Proc. Natl. Acad. Sci. U.S.A. 101, 3662–3667. doi: 10.1073/pnas.0307231101
Carvalho, M. C., Moreira, C. M., Zanoveli, J. M., and Brandão, M. L. (2012). Central, but not basolateral, amygdala involvement in the anxiolytic-like effects of midazolam in rats in the elevated plus maze. J. Psychopharmacol. 26, 543–554. doi: 10.1177/0269881110389209
Chen, X., van Gerven, J., Cohen, A., and Jacobs, G. (2019). Human pharmacology of positive GABA-A subtype-selective receptor modulators for the treatment of anxiety. Acta Pharmacol. Sin. 40, 571–582. doi: 10.1038/s41401-018-0185-5
Cheng, T., Wallace, D. M., Ponteri, B., and Tuli, M. (2018). Valium without dependence? Individual GABAA receptor subtype contribution toward benzodiazepine addiction, tolerance, and therapeutic effects. Neuropsychiatr. Dis. Treat. 14, 1351–1361. doi: 10.2147/NDT.S164307
Chiu, C. Q., Barberis, A., and Higley, M. J. (2019). Preserving the balance: diverse forms of long-term GABAergic synaptic plasticity. Nat. Rev. Neurosci. 20, 272–281. doi: 10.1038/s41583-019-0141-5
Comenencia-Ortiz, E., Moss, S. J., and Davies, P. A. (2014). Phosphorylation of GABAA receptors influences receptor trafficking and neurosteroid actions. Psychopharmacology 231, 3453–3465. doi: 10.1007/s00213-014-3617-z
Crestani, F., Keist, R., Fritschy, J.-M., Benke, D., Vogt, K., Prut, L., et al. (2002). Trace fear conditioning involves hippocampal α5 GABAA receptors. Proc. Natl. Acad. Sci. U.S.A. 99, 8980–8985. doi: 10.1073/pnas.142288699
Cristea, I. A., and Naudet, F. (2019). US Food and Drug Administration approval of esketamine and brexanolone. Lancet Psychiatry 6, 975–977. doi: 10.1016/S2215-0366(19)30292-5
Crocetti, L., and Guerrini, G. (2020). GABAA receptor subtype modulators in medicinal chemistry: an updated patent review (2014-present). Expert Opin. Ther. Patents 30, 409–432. doi: 10.1080/13543776.2020.1746764
Czyk, Z. (2019). Neurosteroids in the Treatment of Anxiety. EDS and Chronic Pain News & Info. Available online at: https://edsinfo.wordpress.com/2019/08/17/neurosteroids-in-the-treatment-of-anxiety/ (accessed June 29, 2020).
Davenport, C. M., Rajappa, R., Katchan, L., Taylor, C. R., Tsai, M.-C., Smith, C. M., et al. (2020). Relocation of an extrasynaptic GABAA receptor to inhibitory synapses freezes excitatory synaptic strength and preserves memory. Neuron [Epub ahead of print]. doi: 10.1016/j.neuron.2020.09.037
Davenport, E. C., Pendolino, V., Kontou, G., McGee, T. P., Sheehan, D. F., López-Doménech, G., et al. (2017). An essential role for the tetraspanin LHFPL4 in the cell-type-specific targeting and clustering of synaptic GABAA receptors. Cell Rep. 21, 70–83. doi: 10.1016/j.celrep.2017.09.025
Dias, R., Sheppard, W. F. A., Fradley, R. L., Garrett, E. M., Stanley, J. L., Tye, S. J., et al. (2005). Evidence for a significant role of α3-containing GABAA receptors in mediating the anxiolytic effects of benzodiazepines. J. Neurosci. 25, 10682–10688. doi: 10.1523/JNEUROSCI.1166-05.2005
Durkin, E. J., Muessig, L., Herlt, T., Lumb, M. J., Patel, R., Thomas, P., et al. (2018). Brain neurosteroids are natural anxiolytics targeting α2 subunit γ-aminobutyric acid type-A receptors. biorxiv [Preprint]. doi: 10.1101/462457
Ehrlich, I., Humeau, Y., Grenier, F., Ciocchi, S., Herry, C., and Lüthi, A. (2009). Amygdala inhibitory circuits and the control of fear memory. Neuron 62, 757–771. doi: 10.1016/j.neuron.2009.05.026
Elgarf, A. A. (2018). Binding behavior of different benzodiazepine ligands implies the use of more than one binding pose in their interaction with GABA(A) receptors. Intrinsic Act. 6:A3.4. doi: 10.25006/ia.6.s1-a3.4
Engin, E., Bakhurin, K. I., Smith, K. S., Hines, R. M., Reynolds, L. M., Tang, W., et al. (2014). Neural basis of benzodiazepine reward: requirement for α2 containing GABAA receptors in the nucleus accumbens. Neuropsychopharmacology 39, 1805–1815. doi: 10.1038/npp.2014.41
Engin, E., Benham, R. S., and Rudolph, U. (2018). An emerging circuit pharmacology of GABAA receptors. Trends Pharmacol. Sci. 39, 710–732. doi: 10.1016/j.tips.2018.04.003
Eom, W., Lee, J. M., Park, J., Choi, K., Jung, S.-J., and Kim, H.-S. (2011). The effects of midazolam and sevoflurane on the GABA(A) receptors with alternatively spliced variants of the γ2 subunit. Korean J. Anesthesiol. 60, 109–118. doi: 10.4097/kjae.2011.60.2.109
Fáncsik, A., Linn, D. M., and Tasker, J. G. (2000). Neurosteroid modulation of GABA IPSCs is phosphorylation dependent. J. Neurosci. 20, 3067–3075. doi: 10.1523/jneurosci.20-09-03067.2000
Farrant, M., and Nusser, Z. (2005). Variations on an inhibitory theme: phasic and tonic activation of GABA(A) receptors. Nat. Rev. Neurosci. 6, 215–229. doi: 10.1038/nrn1625
Farrow, P., Khodosevich, K., Sapir, Y., Schulmann, A., Aslam, M., Stern-Bach, Y., et al. (2015). Auxiliary subunits of the CKAMP family differentially modulate AMPA receptor properties. eLife 4:e09693. doi: 10.7554/eLife.09693
Foitzick, M. F., Medina, N. B., García, L. C. I., and Gravielle, M. C. (2020). Benzodiazepine exposure induces transcriptional down-regulation of GABAA receptor α1 subunit gene via L-type voltage-gated calcium channel activation in rat cerebrocortical neurons. Neurosci. Lett. 721:134801. doi: 10.1016/j.neulet.2020.134801
Forman, S. A., and Miller, K. W. (2016). Mapping general anesthetic sites in heteromeric GABA A receptors reveals a potential for targeting receptor subtypes. Anesth. Analg. 123, 1263–1273. doi: 10.1213/ane.0000000000001368
Fritschy, J.-M., and Mohler, H. (1995). GABAA-receptor heterogeneity in the adult rat brain: differential regional and cellular distribution of seven major subunits. J. Comp. Neurol. 359, 154–194. doi: 10.1002/cne.903590111
Frye, C. A., Hirst, J. J., Brunton, P. J., and Russell, J. A. (2011). Neurosteroids for a successful pregnancy. Stress 14, 1–5. doi: 10.3109/10253890.2010.540050
Fujimura, J., Nagano, M., and Suzuki, H. (2005). Differential expression of GABAA receptor subunits in the distinct nuclei of the rat amygdala. Mol. Brain Res. 138, 17–23. doi: 10.1016/j.molbrainres.2005.03.013
Gafford, G. M., Guo, J.-D., Flandreau, E. I., Hazra, R., Rainnie, D. G., and Ressler, K. J. (2012). Cell-type specific deletion of GABA (A) α1 in corticotropin-releasing factor-containing neurons enhances anxiety and disrupts fear extinction. Proc. Natl. Acad. Sci. U.S.A. 109, 16330–16335. doi: 10.1073/pnas.1119261109
Gallager, D. W., Lakoski, J. M., Gonsalves, S. F., and Rauch, S. L. (1984). Chronic benzodiazepine treatment decreases postsynaptic GABA sensitivity. Nature 308, 74–77. doi: 10.1038/308074a0
Ge, Y., Kang, Y., Cassidy, R. M., Moon, K.-M., Lewis, R., Wong, R. O. L., et al. (2018). Clptm1 limits forward trafficking of GABAA receptors to scale inhibitory synaptic strength. Neuron 97, 596.e8–610.e8. doi: 10.1016/j.neuron.2017.12.038
Gielen, M., and Corringer, P.-J. (2018). The dual-gate model for pentameric ligand-gated ion channels activation and desensitization. J. Physiol. 596, 1873–1902. doi: 10.1113/jp275100
Gielen, M. C., Lumb, M. J., and Smart, T. G. (2012). Benzodiazepines modulate GABAA receptors by regulating the preactivation step after GABA binding. J. Neurosci. 32, 5707–5715. doi: 10.1523/JNEUROSCI.5663-11.2012
Glykys, J., Mann, E. O., and Mody, I. (2008). Which GABAA receptor subunits are necessary for tonic inhibition in the hippocampus? J. Neurosci. 28, 1421–1426. doi: 10.1523/JNEUROSCI.4751-07.2008
Glykys, J., and Mody, I. (2007). The main source of ambient GABA responsible for tonic inhibition in the mouse hippocampus. J. Physiol. 582, 1163–1178. doi: 10.1113/jphysiol.2007.134460
Goldschen-Ohm, M. P., Haroldson, A., Jones, M. V., and Pearce, R. A. (2014). A nonequilibrium binary elements-based kinetic model for benzodiazepine regulation of GABAA receptors. J. Gen. Physiol. 144, 27–39. doi: 10.1085/jgp.201411183
Greenfield, L. J. Jr. (2013). Molecular mechanisms of antiseizure drug activity at GABAA receptors. Seizure 22, 589–600. doi: 10.1016/j.seizure.2013.04.015
Griessner, J., Pasieka, M., Böhm, V., Grössl, F., Kaczanowska, J., Pliota, P., et al. (2018). Central amygdala circuit dynamics underlying the benzodiazepine anxiolytic effect. Mol. Psychiatry [Epub ahead of print]. doi: 10.1038/s41380-018-0310-3
Gunduz-Bruce, H., Silber, C., Rothschild, A., Riesenberg, R., Sankoh, A., Li, H., et al. (2019). SAGE-217 in major depressive disorder: a multicenter, randomized, double-blind, Phase 2 placebo-controlled trial. Eur. Neuropsychopharmacol. 29, S59–S60. doi: 10.1016/j.euroneuro.2018.11.1036
Günther, U., Benson, J., Benke, D., Fritschy, J. M., Reyes, G., Knoflach, F., et al. (1995). Benzodiazepine-insensitive mice generated by targeted disruption of the gamma 2 subunit gene of gamma-aminobutyric acid type A receptors. Proc. Natl. Acad. Sci. U.S.A. 92, 7749–7753. doi: 10.1073/pnas.92.17.7749
Gutiérrez, A., Khan, Z. U., Miralles, C. P., and De Blas, A. L. (1996). Altered expression of γ2L and γ2S GABAA receptor subunits in the aging rat brain. Mol. Brain Res. 35, 91–102. doi: 10.1016/0169-328X(95)00187-W
Han, W., Li, J., Pelkey, K. A., Pandey, S., Chen, X., Wang, Y.-X., et al. (2019). Shisa7 is a GABAA receptor auxiliary subunit controlling benzodiazepine actions. Science 366, 246–250. doi: 10.1126/science.aax5719
Han, W., Shepard, R. D., and Lu, W. (2020). Regulation of GABAARs by transmembrane accessory proteins. Trends Neurosci. [Epub ahead of print]. doi: 10.1016/j.tins.2020.10.011
Hannan, S., Minere, M., Harris, J., Izquierdo, P., Thomas, P., Tench, B., et al. (2019). GABAAR isoform and subunit structural motifs determine synaptic and extrasynaptic receptor localisation. Neuropharmacology 169:107540. doi: 10.1016/j.neuropharm.2019.02.022
Harney, S. C., Frenguelli, B. G., and Lambert, J. J. (2003). Phosphorylation influences neurosteroid modulation of synaptic GABAA receptors in rat CA1 and dentate gyrus neurones. Neuropharmacology 45, 873–883. doi: 10.1016/s0028-3908(03)00251-x
Haubensak, W., Kunwar, P. S., Cai, H., Ciocchi, S., Wall, N. R., Ponnusamy, R., et al. (2010). Genetic dissection of an amygdala microcircuit that gates conditioned fear. Nature 468, 270–276. doi: 10.1038/nature09553
Hausrat, T. J., Muhia, M., Gerrow, K., Thomas, P., Hirdes, W., Tsukita, S., et al. (2015). Radixin regulates synaptic GABAA receptor density and is essential for reversal learning and short-term memory. Nat. Commun. 6:6872. doi: 10.1038/ncomms7872
Herd, M. B., Belelli, D., and Lambert, J. J. (2007). Neurosteroid modulation of synaptic and extrasynaptic GABAA receptors. Pharmacol. Ther. 116, 20–34. doi: 10.1016/j.pharmthera.2007.03.007
Herguedas, B., Watson, J. F., Ho, H., Cais, O., García-Nafría, J., and Greger, I. H. (2019). Architecture of the heteromeric GluA1/2 AMPA receptor in complex with the auxiliary subunit TARP γ8. Science 364:eaav9011. doi: 10.1126/science.aav9011
Herman, M. A., Contet, C., Justice, N. J., Vale, W., and Roberto, M. (2013). Novel subunit-specific tonic GABA currents and differential effects of ethanol in the central amygdala of CRF receptor-1 reporter mice. J. Neurosci. 33, 3284–3298. doi: 10.1523/JNEUROSCI.2490-12.2013
Hernandez, C. C., and Macdonald, R. L. (2019). A structural look at GABAA receptor mutations linked to epilepsy syndromes. Brain Res. 1714, 234–247. doi: 10.1016/j.brainres.2019.03.004
Hines, R. M., Davies, P. A., Moss, S. J., and Maguire, J. (2012). Functional regulation of GABAA receptors in nervous system pathologies. Curr. Opin. Neurobiol. 22, 552–558. doi: 10.1016/j.conb.2011.10.007
Hodge, C. W., Raber, J., McMahon, T., Walter, H., Sanchez-Perez, A. M., Olive, M. F., et al. (2002). Decreased anxiety-like behavior, reduced stress hormones, and neurosteroid supersensitivity in mice lacking protein kinase Cε. J. Clin. Invest. 110, 1003–1010. doi: 10.1172/JCI15903
Hosie, A. M., Clarke, L., da Silva, H., and Smart, T. G. (2009). Conserved site for neurosteroid modulation of GABAA receptors. Neuropharmacology 56, 149–154. doi: 10.1016/j.neuropharm.2008.07.050
Hosie, A. M., Wilkins, M. E., da Silva, H. M. A., and Smart, T. G. (2006). Endogenous neurosteroids regulate GABAA receptors through two discrete transmembrane sites. Nature 444, 486–489. doi: 10.1038/nature05324
Hosie, A. M., Wilkins, M. E., and Smart, T. G. (2007). Neurosteroid binding sites on GABAA receptors. Pharmacol. Ther. 116, 7–19. doi: 10.1016/j.pharmthera.2007.03.011
Huntsman, M. M., Tran, B.-V., Potkin, S. G., Bunney, W. E., and Jones, E. G. (1998). Altered ratios of alternatively spliced long and short 2 subunit mRNAs of the -amino butyrate type A receptor in prefrontal cortex of schizophrenics. Proc. Natl. Acad. Sci. U.S.A. 95, 15066–15071. doi: 10.1073/pnas.95.25.15066
Jacob, T. C., Bogdanov, Y. D., Magnus, C., Saliba, R. S., Kittler, J. T., Haydon, P. G., et al. (2005). Gephyrin regulates the cell surface dynamics of synaptic GABAA receptors. J. Neurosci. 25, 10469–10478. doi: 10.1523/JNEUROSCI.2267-05.2005
Jacob, T. C., Michels, G., Silayeva, L., Haydon, J., Succol, F., and Moss, S. J. (2012). Benzodiazepine treatment induces subtype-specific changes in GABAA receptor trafficking and decreases synaptic inhibition. Proc. Natl. Acad. Sci. U.S.A. 109, 18595–18600. doi: 10.1073/pnas.1204994109
Jacob, T. C., Moss, S. J., and Jurd, R. (2008). GABA(A) receptor trafficking and its role in the dynamic modulation of neuronal inhibition. Nat. Rev. Neurosci. 9, 331–343. doi: 10.1038/nrn2370
Jatczak-Śliwa, M., Terejko, K., Brodzki, M., Michałowski, M. A., Czyzewska, M. M., Nowicka, J. M., et al. (2018). Distinct modulation of spontaneous and GABA-evoked gating by flurazepam shapes cross-talk between agonist-free and liganded GABAA receptor activity. Front. Cell. Neurosci. 12:237. doi: 10.3389/fncel.2018.00237
Kamalova, A., and Nakagawa, T. (2020). AMPA receptor structure and auxiliary subunits. J. Physiol. [Epub ahead of print]. doi: 10.1113/JP278701
Karayannis, T., Elfant, D., Huerta-Ocampo, I., Teki, S., Scott, R. S., Rusakov, D. A., et al. (2010). Slow GABA transient and receptor desensitization shape synaptic responses evoked by hippocampal neurogliaform cells. J. Neurosci. 30, 9898–9909. doi: 10.1523/JNEUROSCI.5883-09.2010
Kasaragod, V. B., Hausrat, T. J., Schaefer, N., Kuhn, M., Christensen, N. R., Tessmer, I., et al. (2019). Elucidating the molecular basis for inhibitory neurotransmission regulation by artemisinins. Neuron 101, 673.e11–689.e11. doi: 10.1016/j.neuron.2019.01.001
Kauer, J. A., and Malenka, R. C. (2007). Synaptic plasticity and addiction. Nat. Rev. Neurosci. 8, 844–858. doi: 10.1038/nrn2234
Kaufmann, W. A., Humpel, C., Alheid, G. F., and Marksteiner, J. (2003). Compartmentation of alpha 1 and alpha 2 GABAA receptor subunits within rat extended amygdala: implications for benzodiazepine action. Brain Res. 964, 91–99. doi: 10.1016/S0006-8993(02)04082-9
Kerti-Szigeti, K., and Nusser, Z. (2016). Similar GABAA receptor subunit composition in somatic and axon initial segment synapses of hippocampal pyramidal cells. eLife 5:e18426. doi: 10.7554/eLife.18426
Kerti-Szigeti, K., Nusser, Z., and Eyre, M. D. (2014). Synaptic GABAA receptor clustering without the γ2 subunit. J. Neurosci. 34, 10219–10233. doi: 10.1523/JNEUROSCI.1721-14.2014
Khayenko, V., and Maric, H. M. (2019). Targeting GABAAR-associated proteins: new modulators, labels and concepts. Front. Mol. Neurosci. 12:162. doi: 10.3389/fnmol.2019.00162
Kia, A., Ribeiro, F., Nelson, R., Gavrilovici, C., Ferguson, S. S. G., and Poulter, M. O. (2011). Kindling alters neurosteroid-induced modulation of phasic and tonic GABAA receptor-mediated currents: role of phosphorylation. J. Neurochem. 116, 1043–1056. doi: 10.1111/j.1471-4159.2010.07156.x
Kim, J. J., Gharpure, A., Teng, J., Zhuang, Y., Howard, R. J., Zhu, S., et al. (2020). Shared structural mechanisms of general anaesthetics and benzodiazepines. Nature 585, 303–308. doi: 10.1038/s41586-020-2654-5
Klaassen, R. V., Stroeder, J., Coussen, F., Hafner, A.-S., Petersen, J. D., Renancio, C., et al. (2016). Shisa6 traps AMPA receptors at postsynaptic sites and prevents their desensitization during synaptic activity. Nat. Commun. 7:10682. doi: 10.1038/ncomms10682
Kralic, J. E., Korpi, E. R., O’Buckley, T. K., Homanics, G. E., and Morrow, A. L. (2002a). Molecular and pharmacological characterization of GABA(A) receptor alpha1 subunit knockout mice. J. Pharmacol. Exp. Ther. 302, 1037–1045. doi: 10.1124/jpet.102.036665
Kralic, J. E., O’Buckley, T. K., Khisti, R. T., Hodge, C. W., Homanics, G. E., and Morrow, A. L. (2002b). GABA(A) receptor alpha-1 subunit deletion alters receptor subtype assembly, pharmacological and behavioral responses to benzodiazepines and zolpidem. Neuropharmacology 43, 685–694. doi: 10.1016/s0028-3908(02)00174-0
Kralic, J. E., Sidler, C., Parpan, F., Homanics, G. E., Morrow, A. L., and Fritschy, J.-M. (2006). Compensatory alteration of inhibitory synaptic circuits in cerebellum and thalamus of gamma-aminobutyric acid type A receptor alpha1 subunit knockout mice. J. Comp. Neurol. 495, 408–421. doi: 10.1002/cne.20866
Kramer, P. F., Twedell, E. L., Shin, J. H., Zhang, R., and Khaliq, Z. M. (2020). Axonal mechanisms mediating γ-aminobutyric acid receptor type A (GABA-A) inhibition of striatal dopamine release. eLife 9:e55729. doi: 10.7554/eLife.55729
Lalive, A. L., Rudolph, U., Lüscher, C., and Tan, K. R. (2011). Is there a way to curb benzodiazepine addiction? Swiss. Med. Wkly. 141:w13277.
Lambert, J. J., Belelli, D., Peden, D. R., Vardy, A. W., and Peters, J. A. (2003). Neurosteroid modulation of GABAA receptors. Prog. Neurobiol. 71, 67–80. doi: 10.1016/j.pneurobio.2003.09.001
Laverty, D., Desai, R., Uchański, T., Masiulis, S., Stec, W. J., Malinauskas, T., et al. (2019). Cryo-EM structure of the human α1β3γ2 GABA A receptor in a lipid bilayer. Nature 565, 516–520. doi: 10.1038/s41586-018-0833-4
Laverty, D., Thomas, P., Field, M., Andersen, O. J., Gold, M. G., Biggin, P. C., et al. (2017). Crystal structures of a GABAA-receptor chimera reveal new endogenous neurosteroid-binding sites. Nat. Struct. Mol. Biol. 24, 977–985. doi: 10.1038/nsmb.3477
Lavoie, A. M., Tingey, J. J., Harrison, N. L., Pritchett, D. B., and Twyman, R. E. (1997). Activation and deactivation rates of recombinant GABA(A) receptor channels are dependent on alpha-subunit isoform. Biophys. J. 73, 2518–2526. doi: 10.1016/S0006-3495(97)78280-8
Lavoie, A. M., and Twyman, R. E. (1996). Direct evidence for diazepam modulation of GABAA receptor microscopic affinity. Neuropharmacology 35, 1383–1392. doi: 10.1016/s0028-3908(96)00077-9
Leidenheimer, N. J., McQuilkin, S. J., Hahner, L. D., Whiting, P., and Harris, R. A. (1992). Activation of protein kinase C selectively inhibits the gamma-aminobutyric acidA receptor: role of desensitization. Mol. Pharmacol. 41, 1116–1123.
Lévesque, M., Herrington, R., Leclerc, L., Rogawski, M. A., and Avoli, M. (2017). Allopregnanolone decreases interictal spiking and fast ripples in an animal model of mesial temporal lobe epilepsy. Neuropharmacology 121, 12–19. doi: 10.1016/j.neuropharm.2017.04.020
Levitan, E. S., Blair, L. A., Dionne, V. E., and Barnard, E. A. (1988a). Biophysical and pharmacological properties of cloned GABAA receptor subunits expressed in Xenopus oocytes. Neuron 1, 773–781. doi: 10.1016/0896-6273(88)90125-0
Levitan, E. S., Schofield, P. R., Burt, D. R., Rhee, L. M., Wisden, W., Köhler, M., et al. (1988b). Structural and functional basis for GABA A receptor heterogeneity. Nature 335, 76–79. doi: 10.1038/335076a0
Li, J., Casteels, T., Frogne, T., Ingvorsen, C., Honoré, C., Courtney, M., et al. (2017a). Artemisinins target GABAA receptor signaling and impair α cell identity. Cell 168, 86.e15–100.e15. doi: 10.1016/j.cell.2016.11.010
Li, J., Han, W., Pelkey, K. A., Duan, J., Mao, X., Wang, Y.-X., et al. (2017b). Molecular dissection of neuroligin 2 and Slitrk3 reveals an essential framework for GABAergic synapse development. Neuron 96, 808.e8–826.e8. doi: 10.1016/j.neuron.2017.10.003
Lian, J.-J., Cao, Y.-Q., Li, Y.-L., Yu, G., and Su, R.-B. (2020). Flumazenil-insensitive benzodiazepine effects in recombinant αβ and neuronal GABAA receptors. Brain Sci. 10:150. doi: 10.3390/brainsci10030150
Loebrich, S., Bähring, R., Katsuno, T., Tsukita, S., and Kneussel, M. (2006). Activated radixin is essential for GABAA receptor alpha5 subunit anchoring at the actin cytoskeleton. EMBO J. 25, 987–999. doi: 10.1038/sj.emboj.7600995
López-Muñoz, F., Ucha-Udabe, R., and Alamo, C. (2005). The history of barbiturates a century after their clinical introduction. Neuropsychiatr. Dis. Treat. 1:329.
Lorenz-Guertin, J. M., Bambino, M. J., Das, S., Weintraub, S. T., and Jacob, T. C. (2019). Diazepam accelerates GABAAR synaptic exchange and alters intracellular trafficking. Front. Cell. Neurosci. 13:163. doi: 10.3389/fncel.2019.00163
Lorenz-Guertin, J. M., Bambino, M. J., and Jacob, T. C. (2018). γ2 GABAAR trafficking and the consequences of human genetic variation. Front. Cell. Neurosci. 12:265. doi: 10.3389/fncel.2018.00265
Lorenz-Guertin, J. M., and Jacob, T. C. (2018). GABA type a receptor trafficking and the architecture of synaptic inhibition. Dev. Neurobiol. 78, 238–270. doi: 10.1002/dneu.22536
Löw, K., Crestani, F., Keist, R., Benke, D., Brünig, I., Benson, J. A., et al. (2000). Molecular and neuronal substrate for the selective attenuation of anxiety. Science 290, 131–134. doi: 10.1126/science.290.5489.131
Lu, W., Bromley-Coolidge, S., and Li, J. (2017). Regulation of GABAergic synapse development by postsynaptic membrane proteins. Brain Res. Bull. 129, 30–42. doi: 10.1016/j.brainresbull.2016.07.004
Luscher, B., Fuchs, T., and Kilpatrick, C. L. (2011). GABAA receptor trafficking-mediated plasticity of inhibitory synapses. Neuron 70, 385–409. doi: 10.1016/j.neuron.2011.03.024
Lüscher, B., and Möhler, H. (2019). Brexanolone, a neurosteroid antidepressant, vindicates the GABAergic deficit hypothesis of depression and may foster resilience. F1000Research 8:F1000 Faculty Rev-751.
Macdonald, R. L., Gallagher, M. J., Feng, H.-J., and Kang, J. (2004). GABAA receptor epilepsy mutations. Biochem. Pharmacol. 68, 1497–1506. doi: 10.1016/j.bcp.2004.07.029
MacKenzie, G., and Maguire, J. (2013). Neurosteroids and GABAergic signaling in health and disease. Biomol. Concepts 4, 29–42. doi: 10.1515/bmc-2012-0033
Magnin, E., Francavilla, R., Amalyan, S., Gervais, E., David, L. S., Luo, X., et al. (2019). Input-specific synaptic location and function of the α5 GABAA receptor subunit in the mouse CA1 hippocampal neurons. J. Neurosci. 39, 788–801. doi: 10.1523/JNEUROSCI.0567-18.2018
Maher, M. P., Matta, J. A., Gu, S., Seierstad, M., and Bredt, D. S. (2017). Getting a handle on neuropharmacology by targeting receptor-associated proteins. Neuron 96, 989–1001. doi: 10.1016/j.neuron.2017.10.001
Maljevic, S., Møller, R. S., Reid, C. A., Pérez-Palma, E., Lal, D., May, P., et al. (2019). Spectrum of GABAA receptor variants in epilepsy. Curr. Opin. Neurol. 32, 183–190. doi: 10.1097/WCO.0000000000000657
Martenson, J. S., Yamasaki, T., Chaudhury, N. H., Albrecht, D., and Tomita, S. (2017). Assembly rules for GABAA receptor complexes in the brain. eLife 6:e27443. doi: 10.7554/eLife.27443
Masiulis, S., Desai, R., Uchański, T., Martin, I. S., Laverty, D., Karia, D., et al. (2019). GABA A receptor signalling mechanisms revealed by structural pharmacology. Nature 565, 454–459. doi: 10.1038/s41586-018-0832-5
McKernan, R. M., Rosahl, T. W., Reynolds, D. S., Sur, C., Wafford, K. A., Atack, J. R., et al. (2000). Sedative but not anxiolytic properties of benzodiazepines are mediated by the GABAA receptor α1 subtype. Nat. Neurosci. 3, 587–592. doi: 10.1038/75761
Mele, M., Costa, R. O., and Duarte, C. B. (2019). Alterations in GABAA-receptor trafficking and synaptic dysfunction in brain disorders. Front. Cell. Neurosci. 13:77. doi: 10.3389/fncel.2019.00077
Miller, P. S., Scott, S., Masiulis, S., De Colibus, L., Pardon, E., Steyaert, J., et al. (2017). Structural basis for GABAA receptor potentiation by neurosteroids. Nat. Struct. Mol. Biol. 24, 986–992. doi: 10.1038/nsmb.3484
Miller, S. M., Pelly, S., Kalanjati, V. P., Lee, A., Colditz, P. B., and Bjorkman, S. T. (2018). Identification and expression of a unique neonatal variant of the GABAA receptor α3 subunit. Brain Struct. Funct. 223, 1025–1033. doi: 10.1007/s00429-017-1597-6
Milstein, A. D., and Nicoll, R. A. (2008). Regulation of AMPA receptor gating and pharmacology by TARP auxiliary subunits. Trends Pharmacol. Sci. 29, 333–339. doi: 10.1016/j.tips.2008.04.004
Minier, F., and Sigel, E. (2004). Positioning of the -subunit isoforms confers a functional signature to -aminobutyric acid type A receptors. Proc. Natl. Acad. Sci. U.S.A. 101, 7769–7774. doi: 10.1073/pnas.0400220101
Mody, I. (2019). GABAAR modulator for postpartum depression. Cell 176:1. doi: 10.1016/j.cell.2018.12.016
Mody, I., and Pearce, R. A. (2004). Diversity of inhibitory neurotransmission through GABAA receptors. Trends Neurosci. 27, 569–575. doi: 10.1016/j.tins.2004.07.002
Möhler, H., Fritschy, J. M., and Rudolph, U. (2002). A new benzodiazepine pharmacology. J. Pharmacol. Exp. Ther. 300, 2–8. doi: 10.1124/jpet.300.1.2
Moody, O. A., and Jenkins, A. (2018). The role of loops B and C in determining the potentiation of GABAA receptors by midazolam. Pharmacol. Res. Perspect. 6:e00433. doi: 10.1002/prp2.433
Mozrzymas, J. W., Wójtowicz, T., Piast, M., Lebida, K., Wyrembek, P., and Mercik, K. (2007). GABA transient sets the susceptibility of mIPSCs to modulation by benzodiazepine receptor agonists in rat hippocampal neurons. J. Physiol. 585, 29–46. doi: 10.1113/jphysiol.2007.143602
Mukherjee, J., Kretschmannova, K., Gouzer, G., Maric, H.-M., Ramsden, S., Tretter, V., et al. (2011). The residence time of GABAARs at inhibitory synapses is determined by direct binding of the receptor α1 subunit to gephyrin. J. Neurosci. 31, 14677–14687. doi: 10.1523/JNEUROSCI.2001-11.2011
Nakagawa, T. (2019). Structures of the AMPA receptor in complex with its auxiliary subunit cornichon. Science 366, 1259–1263. doi: 10.1126/science.aay2783
Nakamura, Y., Darnieder, L. M., Deeb, T. Z., and Moss, S. J. (2015). Regulation of GABAARs by phosphorylation. Adv. Pharmacol. 72, 97–146. doi: 10.1016/bs.apha.2014.11.008
Nathanson, A. J., Davies, P. A., and Moss, S. J. (2019). Inhibitory synapse formation at the axon initial segment. Front. Mol. Neurosci. 12:266. doi: 10.3389/fnmol.2019.00266
Nicholson, M. W., Sweeney, A., Pekle, E., Alam, S., Ali, A. B., Duchen, M., et al. (2018). Diazepam-induced loss of inhibitory synapses mediated by PLCδ/Ca 2+/calcineurin signalling downstream of GABAA receptors. Mol. Psychiatry 23, 1851–1867. doi: 10.1038/s41380-018-0100-y
Nuss, P. (2015). Anxiety disorders and GABA neurotransmission: a disturbance of modulation. Neuropsychiatr. Dis. Treat. 11, 165–175. doi: 10.2147/NDT.S58841
Olsen, R. W. (2018). GABAA receptor: positive and negative allosteric modulators. Neuropharmacology 136, 10–22. doi: 10.1016/j.neuropharm.2018.01.036
Olsen, R. W., Lindemeyer, A. K., Wallner, M., Li, X., Huynh, K. W., and Zhou, Z. H. (2019). Cryo-electron microscopy reveals informative details of GABAA receptor structural pharmacology: implications for drug discovery. Ann. Transl. Med. 7:S144. doi: 10.21037/atm.2019.06.23
Olsen, R. W., and Sieghart, W. (2009). GABAA receptors: subtypes provide diversity of function and pharmacology. Neuropharmacology 56, 141–148. doi: 10.1016/j.neuropharm.2008.07.045
Pei, J., and Grishin, N. V. (2012). Unexpected diversity in Shisa-like proteins suggests the importance of their roles as transmembrane adaptors. Cell. Signal. 24, 758–769. doi: 10.1016/j.cellsig.2011.11.011
Peter, S., Urbanus, B. H. A., Klaassen, R. V., Wu, B., Boele, H.-J., Azizi, S., et al. (2020). AMPAR auxiliary protein SHISA6 facilitates purkinje cell synaptic excitability and procedural memory formation. Cell Rep. 31:107515. doi: 10.1016/j.celrep.2020.03.079
Phulera, S., Zhu, H., Yu, J., Claxton, D. P., Yoder, N., Yoshioka, C., et al. (2018). Cryo-EM structure of the benzodiazepine-sensitive α1β1γ2S tri-heteromeric GABAA receptor in complex with GABA. eLife 7:e39383. doi: 10.7554/eLife.39383
Pirker, S., Schwarzer, C., Wieselthaler, A., Sieghart, W., and Sperk, G. (2000). GABAA receptors: immunocytochemical distribution of 13 subunits in the adult rat brain. Neuroscience 101, 815–850. doi: 10.1016/S0306-4522(00)00442-5
Poisbeau, P., Williams, S. R., and Mody, I. (1997). Silent GABAA synapses during flurazepam withdrawal are region-specific in the hippocampal formation. J. Neurosci. 17, 3467–3475. doi: 10.1523/jneurosci.17-10-03467.1997
Poulopoulos, A., Aramuni, G., Meyer, G., Soykan, T., Hoon, M., Papadopoulos, T., et al. (2009). Neuroligin 2 drives postsynaptic assembly at perisomatic inhibitory synapses through gephyrin and collybistin. Neuron 63, 628–642. doi: 10.1016/j.neuron.2009.08.023
Pritchett, D. B., Sontheimer, H., Shivers, B. D., Ymer, S., Kettenmann, H., Schofield, P. R., et al. (1989). Importance of a novel GABA A receptor subunit for benzodiazepine pharmacology. Nature 338, 582–585. doi: 10.1038/338582a0
Purdy, R. H., Morrow, A. L., Moore, P. H. Jr., and Paul, S. M. (1991). Stress-induced elevations of gamma-aminobutyric acid type A receptor-active steroids in the rat brain. Proc. Natl. Acad. Sci. U.S.A. 88, 4553–4557. doi: 10.1073/pnas.88.10.4553
Qi, Z.-H., Song, M., Wallace, M. J., Wang, D., Newton, P. M., McMahon, T., et al. (2007). Protein kinase Cε regulates γ-aminobutyrate type a receptor sensitivity to ethanol and benzodiazepines through phosphorylation of γ2 subunits. J. Biol. Chem. 282, 33052–33063. doi: 10.1074/jbc.M707233200
Rajgor, D., Purkey, A. M., Sanderson, J. L., Welle, T. M., Garcia, J. D., Dell’Acqua, M. L., et al. (2020). Local miRNA-dependent translational control of GABAAR synthesis during inhibitory long-term potentiation. Cell Rep. 31:107785. doi: 10.1016/j.celrep.2020.107785
Ramamoorthi, K., and Lin, Y. (2011). The contribution of GABAergic dysfunction to neurodevelopmental disorders. Trends Mol. Med. 17, 452–462. doi: 10.1016/j.molmed.2011.03.003
Ramerstorfer, J., Furtmüller, R., Sarto-Jackson, I., Varagic, Z., Sieghart, W., and Ernst, M. (2011). The GABAA receptor α+ β- interface: a novel target for subtype selective drugs. J. Neurosci. 31, 870–877. doi: 10.1523/jneurosci.5012-10.2011
Rao, S., Chen, R., LaRocca, A. A., Christiansen, M. G., Senko, A. W., Shi, C. H., et al. (2019). Remotely controlled chemomagnetic modulation of targeted neural circuits. Nat. Nanotechnol. 14, 967–973. doi: 10.1038/s41565-019-0521-z
Reynolds, L. M., Engin, E., Tantillo, G., Lau, H. M., Muschamp, J. W., Carlezon, W. A., et al. (2012). Differential roles of GABA(A) receptor subtypes in benzodiazepine-induced enhancement of brain-stimulation reward. Neuropsychopharmacology 37, 2531–2540. doi: 10.1038/npp.2012.115
Richter, L., de Graaf, C., Sieghart, W., Varagic, Z., Mörzinger, M., de Esch, I. J. P., et al. (2012). Diazepam-bound GABAA receptor models identify new benzodiazepine binding-site ligands. Nat. Chem. Biol. 8, 455–464. doi: 10.1038/nchembio.917
Rogawski, M. A., Pessah, I. N., Zhengyu, C. A. O., and Lein, P. J. (2020). Mitigation of Epileptic Seizures by Combination Therapy Using Benzodiazepines and Neurosteroids. US Patent. Available online at: https://patentimages.storage.googleapis.com/7d/fb/90/cf7ae69ea589a7/US20200215078A1.pdf (accessed August 13, 2020).
Rogers, C. J., Twyman, R. E., and Macdonald, R. L. (1994). Benzodiazepine and beta-carboline regulation of single GABAA receptor channels of mouse spinal neurones in culture. J. Physiol. 475, 69–82. doi: 10.1113/jphysiol.1994.sp020050
Rowlett, J. K., Platt, D. M., Lelas, S., Atack, J. R., and Dawson, G. R. (2005). Different GABAA receptor subtypes mediate the anxiolytic, abuse-related, and motor effects of benzodiazepine-like drugs in primates. Proc. Natl. Acad. Sci. U.S.A. 102, 915–920. doi: 10.1073/pnas.0405621102
Rudolph, U., Crestani, F., Benke, D., Brünig, I., Benson, J. A., Fritschy, J.-M., et al. (1999). Benzodiazepine actions mediated by specific γ-aminobutyric acidA receptor subtypes. Nature 401, 796–800. doi: 10.1038/44579
Rudolph, U., and Knoflach, F. (2011). Beyond classical benzodiazepines: novel therapeutic potential of GABA A receptor subtypes. Nat. Rev. Drug Discov. 10, 685–697. doi: 10.1038/nrd3502
Rudolph, U., and Möhler, H. (2004). Analysis of GABAA receptor function and dissection of the pharmacology of benzodiazepines and general anesthetics through mouse genetics. Annu. Rev. Pharmacol. Toxicol. 44, 475–498. doi: 10.1146/annurev.pharmtox.44.101802.121429
Rudolph, U., and Möhler, H. (2006). GABA-based therapeutic approaches: GABAA receptor subtype functions. Curr. Opin. Pharmacol. 6, 18–23. doi: 10.1016/j.coph.2005.10.003
Rudolph, U., and Möhler, H. (2014). GABAA receptor subtypes: therapeutic potential in Down syndrome, affective disorders, schizophrenia, and autism. Annu. Rev. Pharmacol. Toxicol. 54, 483–507. doi: 10.1146/annurev-pharmtox-011613-135947
Schmitz, L. J. M., Klaassen, R. V., Ruiperez-Alonso, M., Zamri, A. E., Stroeder, J., Rao-Ruiz, P., et al. (2017). The AMPA receptor-associated protein Shisa7 regulates hippocampal synaptic function and contextual memory. eLife 6:e24192. doi: 10.7554/elife.24192
Schofield, P. R., Darlison, M. G., Fujita, N., Burt, D. R., Stephenson, F. A., Rodriguez, H., et al. (1987). Sequence and functional expression of the GABA A receptor shows a ligand-gated receptor super-family. Nature 328, 221–227. doi: 10.1038/328221a0
Schüle, C., Nothdurfter, C., and Rupprecht, R. (2014). The role of allopregnanolone in depression and anxiety. Prog. Neurobiol. 113, 79–87. doi: 10.1016/j.pneurobio.2013.09.003
Schulz, J. M., Knoflach, F., Hernandez, M.-C., and Bischofberger, J. (2018). Dendrite-targeting interneurons control synaptic NMDA-receptor activation via nonlinear α5-GABA A receptors. Nat. Commun. 9, 1–16.
Schumacher, M., Weill-Engerer, S., Liere, P., Robert, F., Franklin, R. J. M., Garcia-Segura, L. M., et al. (2003). Steroid hormones and neurosteroids in normal and pathological aging of the nervous system. Prog. Neurobiol. 71, 3–29. doi: 10.1016/j.pneurobio.2003.09.004
Scott, S., and Aricescu, A. R. (2019). A structural perspective on GABAA receptor pharmacology. Curr. Opin. Struct. Biol. 54, 189–197. doi: 10.1016/j.sbi.2019.03.023
Seeburg, P. H., Wisden, W., Verdoorn, T. A., Pritchett, D. B., Werner, P., Herb, A., et al. (1990). The GABAA receptor family: molecular and functional diversity. Cold Spring Harb. Symp. Quant. Biol. 55, 29–40. doi: 10.1101/sqb.1990.055.01.006
Shields, B. C., Kahuno, E., Kim, C., Apostolides, P. F., Brown, J., Lindo, S., et al. (2017). Deconstructing behavioral neuropharmacology with cellular specificity. Science 356:eaaj2161. doi: 10.1126/science.aaj2161
Sieghart, W. (2015). Allosteric modulation of GABAA receptors via multiple drug-binding sites. Adv. Pharmacol. 72, 53–96. doi: 10.1016/bs.apha.2014.10.002
Sieghart, W., and Savić, M. M. (2018). International union of basic and clinical pharmacology. cvi: gabaa receptor subtype- and function-selective ligands: key issues in translation to humans. Pharmacol. Rev. 70, 836–878. doi: 10.1124/pr.117.014449
Sieghart, W., and Sperk, G. (2002). Subunit composition, distribution and function of GABA(A) receptor subtypes. Curr. Top. Med. Chem. 2, 795–816. doi: 10.2174/1568026023393507
Sigel, E., Baur, R., Trube, G., Möhler, H., and Malherbe, P. (1990). The effect of subunit composition of rat brain GABAA receptors on channel function. Neuron 5, 703–711. doi: 10.1016/0896-6273(90)90224-4
Sigel, E., and Ernst, M. (2018). The Benzodiazepine Binding Sites of GABAA Receptors. Trends Pharmacol. Sci. 39, 659–671. doi: 10.1016/j.tips.2018.03.006
Sigel, E., Mamalaki, C., and Barnard, E. A. (1982). Isolation of a GABA receptor from bovine brain using a benzodiazepine affinity column. FEBS Lett. 147, 45–48. doi: 10.1016/0014-5793(82)81008-9
Sigel, E., and Steinmann, M. E. (2012). Structure, function, and modulation of GABAA receptors. J. Biol. Chem. 287, 40224–40231. doi: 10.1074/jbc.R112.386664
Sigel, E., Stephenson, F. A., Mamalaki, C., and Barnard, E. A. (1983). A gamma-aminobutyric acid/benzodiazepine receptor complex of bovine cerebral cortex. J. Biol. Chem. 258, 6965–6971.
Silberman, E., Balon, R., Starcevic, V., Shader, R., Cosci, F., Fava, G. A., et al. (2020). Benzodiazepines: it’s time to return to the evidence. Br. J. Psychiatry [Epub ahead of print]. doi: 10.1192/bjp.2020.164
Smart, T. G., and Stephenson, F. A. (2019). A half century of γ-aminobutyric acid. Brain Neurosci. Adv. 3:2398212819858249. doi: 10.1177/2398212819858249
Smith, K. S., Engin, E., Meloni, E. G., and Rudolph, U. (2012). Benzodiazepine-induced anxiolysis and reduction of conditioned fear are mediated by distinct GABAA receptor subtypes in mice. Neuropharmacology 63, 250–258. doi: 10.1016/j.neuropharm.2012.03.001
Smith, K. S., and Rudolph, U. (2012). Anxiety and depression: mouse genetics and pharmacological approaches to the role of GABAA receptor subtypes. Neuropharmacology 62, 54–62. doi: 10.1016/j.neuropharm.2011.07.026
Smith, S. S., Shen, H., Gong, Q. H., and Zhou, X. (2007). Neurosteroid regulation of GABA(A) receptors: focus on the alpha4 and delta subunits. Pharmacol. Ther. 116, 58–76. doi: 10.1016/j.pharmthera.2007.03.008
Solomon, V. R., Tallapragada, V. J., Chebib, M., Johnston, G. A. R., and Hanrahan, J. R. (2019). GABA allosteric modulators: an overview of recent developments in non-benzodiazepine modulators. Eur. J. Med. Chem. 171, 434–461. doi: 10.1016/j.ejmech.2019.03.043
Soyka, M. (2017). Treatment of benzodiazepine dependence. N. Engl. J. Med. 376, 2399–2400. doi: 10.1056/NEJMc1705239
Stafstrom, C. (2010). “Pathophysiological mechanisms of seizures and epilepsy: a primer,” in Epilepsy: Mechanisms, Models, and Translational Perspectives, eds J. M. Rho, C. E. Stafstrom, and R. Sankar (Boca Raton, FL: CRC Press), 3–19. doi: 10.1201/9781420085594-c1
Sugasawa, Y., Cheng, W. W. L., Bracamontes, J. R., Chen, Z.-W., Wang, L., Germann, A. L., et al. (2020). Site-specific effects of neurosteroids on GABAA receptor activation and desensitization. bioRxiv [Preprint]. doi: 10.1101/2020.04.27.063404
Sur, C., Wafford, K. A., Reynolds, D. S., Hadingham, K. L., Bromidge, F., Macaulay, A., et al. (2001). Loss of the major GABAAReceptor subtype in the brain is not lethal in mice. J. Neurosci. 21, 3409–3418. doi: 10.1523/jneurosci.21-10-03409.2001
Tan, K. R., Brown, M., Labouèbe, G., Yvon, C., Creton, C., Fritschy, J.-M., et al. (2010). Neural bases for addictive properties of benzodiazepines. Nature 463, 769–774. doi: 10.1038/nature08758
Tan, K. R., Rudolph, U., and Lüscher, C. (2011). Hooked on benzodiazepines: GABAA receptor subtypes and addiction. Trends Neurosci. 34, 188–197. doi: 10.1016/j.tins.2011.01.004
Thomas, P., Mortensen, M., Hosie, A. M., and Smart, T. G. (2005). Dynamic mobility of functional GABA A receptors at inhibitory synapses. Nat. Neurosci. 8, 889–897. doi: 10.1038/nn1483
Tomita, S. (2019). Molecular constituents and localization of the ionotropic GABA receptor complex in vivo. Curr. Opin. Neurobiol. 57, 81–86. doi: 10.1016/j.conb.2019.01.017
Twyman, R. E., and Macdonald, R. L. (1992). Neurosteroid regulation of GABAA receptor single-channel kinetic properties of mouse spinal cord neurons in culture. J. Physiol. 456, 215–245. doi: 10.1113/jphysiol.1992.sp019334
Twyman, R. E., Rogers, C. J., and Macdonald, R. L. (1989). Differential regulation of gamma-aminobutyric acid receptor channels by diazepam and phenobarbital. Ann. Neurol. 25, 213–220. doi: 10.1002/ana.410250302
Tyagarajan, S. K., and Fritschy, J.-M. (2014). Gephyrin: a master regulator of neuronal function? Nat. Rev. Neurosci. 15, 141–156. doi: 10.1038/nrn3670
Uusi-Oukari, M., and Korpi, E. R. (2010). Regulation of GABAA receptor subunit expression by pharmacological agents. Pharmacol. Rev. 62, 97–135. doi: 10.1124/pr.109.002063
Vicini, S., Losi, G., and Homanics, G. E. (2002). GABA(A) receptor delta subunit deletion prevents neurosteroid modulation of inhibitory synaptic currents in cerebellar neurons. Neuropharmacology 43, 646–650. doi: 10.1016/s0028-3908(02)00126-0
Vinkers, C. H., and Olivier, B. (2012). Mechanisms underlying tolerance after long-term benzodiazepine use: a future for subtype-selective GABAA receptor modulators? Adv. Pharmacol. Sci. 2012:416864.
Vithlani, M., Terunuma, M., and Moss, S. J. (2011). The dynamic modulation of GABA(A) receptor trafficking and its role in regulating the plasticity of inhibitory synapses. Physiol. Rev. 91, 1009–1022. doi: 10.1152/physrev.00015.2010
von Engelhardt, J. (2019). AMPA receptor auxiliary proteins of the CKAMP family. Int. J. Mol. Sci. 20:1460. doi: 10.3390/ijms20061460
von Engelhardt, J., Mack, V., Sprengel, R., Kavenstock, N., Li, K. W., Stern-Bach, Y., et al. (2010). CKAMP44: a brain-specific protein attenuating short-term synaptic plasticity in the dentate gyrus. Science 327, 1518–1522. doi: 10.1126/science.1184178
Votaw, V. R., Geyer, R., Rieselbach, M. M., and McHugh, R. K. (2019). The epidemiology of benzodiazepine misuse: a systematic review. Drug Alcohol Depend. 200, 95–114. doi: 10.1016/j.drugalcdep.2019.02.033
Wallace, R. H., Marini, C., Petrou, S., Harkin, L. A., Bowser, D. N., Panchal, R. G., et al. (2001). Mutant GABA A receptor γ2-subunit in childhood absence epilepsy and febrile seizures. Nat. Genet. 28, 49–52. doi: 10.1038/ng0501-49
Walters, R. J., Hadley, S. H., Morris, K. D., and Amin, J. (2000). Benzodiazepines act on GABAA receptors via two distinct and separable mechanisms. Nat. Neurosci. 3, 1274–1281. doi: 10.1038/81800
Walton, N., and Maguire, J. (2019). Allopregnanolone-based treatments for postpartum depression: Why/how do they work? Neurobiol. Stress 11:100198. doi: 10.1016/j.ynstr.2019.100198
Wang, J. B., and Burt, D. R. (1991). Differential expression of two forms of GABAA receptor gamma 2-subunit in mice. Brain Res. Bull. 27, 731–735. doi: 10.1016/0361-9230(91)90054-n
Whiting, P., McKernan, R. M., and Iversen, L. L. (1990). Another mechanism for creating diversity in gamma-aminobutyrate type A receptors: RNA splicing directs expression of two forms of gamma 2 phosphorylation site. Proc. Natl. Acad. Sci. U.S.A. 87, 9966–9970. doi: 10.1073/pnas.87.24.9966
Wieland, H. A., Lüddens, H., and Seeburg, P. H. (1992). A single histidine in GABAA receptors is essential for benzodiazepine agonist binding. J. Biol. Chem. 267, 1426–1429.
Wongsamitkul, N., Maldifassi, M. C., Simeone, X., Baur, R., Ernst, M., and Sigel, E. (2017). α subunits in GABAA receptors are dispensable for GABA and diazepam action. Sci. Rep. 7:15498. doi: 10.1038/s41598-017-15628-7
Wu, M., Tian, H.-L., Liu, X., Lai, J. H. C., Du, S., and Xia, J. (2018). Impairment of inhibitory synapse formation and motor behavior in mice lacking the NL2 binding partner LHFPL4/GARLH4. Cell Rep. 23, 1691–1705. doi: 10.1016/j.celrep.2018.04.015
Yamasaki, T., Hoyos-Ramirez, E., Martenson, J. S., Morimoto-Tomita, M., and Tomita, S. (2017). GARLH family proteins stabilize GABAA receptors at synapses. Neuron 93, 1138.e6–1152.e6. doi: 10.1016/j.neuron.2017.02.023
Yamaura, K., Kiyonaka, S., Numata, T., Inoue, R., and Hamachi, I. (2016). Discovery of allosteric modulators for GABA A receptors by ligand-directed chemistry. Nat. Chem. Biol. 12, 822–830. doi: 10.1038/nchembio.2150
Zhao, Y., Chen, S., Swensen, A. C., Qian, W.-J., and Gouaux, E. (2019). Architecture and subunit arrangement of native AMPA receptors elucidated by cryo-EM. Science 364, 355–362. doi: 10.1126/science.aaw8250
Zhu, S., Noviello, C. M., Teng, J., Walsh, R. M. Jr., Kim, J. J., and Hibbs, R. E. (2018). Structure of a human synaptic GABAA receptor. Nature 559, 67–72. doi: 10.1038/s41586-018-0255-3
Ziff, E. B. (2007). TARPs and the AMPA receptor trafficking paradox. Neuron 53, 627–633. doi: 10.1016/j.neuron.2007.02.006
Keywords: GABA, GABAAR, benzodiazepines, neurosteroids, pharmacology, LH4, Clptm1, Shisa7
Citation: Castellano D, Shepard RD and Lu W (2021) Looking for Novelty in an “Old” Receptor: Recent Advances Toward Our Understanding of GABAARs and Their Implications in Receptor Pharmacology. Front. Neurosci. 14:616298. doi: 10.3389/fnins.2020.616298
Received: 11 October 2020; Accepted: 14 December 2020;
Published: 14 January 2021.
Edited by:
Cesar Mattei, Université d’Angers, FranceReviewed by:
Miroslav M. Savic, University of Belgrade, SerbiaJosipa Vlainic, Rudjer Boskovic Institute, Croatia
Rochelle Marie Hines, University of Nevada, Las Vegas, United States
Copyright © 2021 Castellano, Shepard and Lu. This is an open-access article distributed under the terms of the Creative Commons Attribution License (CC BY). The use, distribution or reproduction in other forums is permitted, provided the original author(s) and the copyright owner(s) are credited and that the original publication in this journal is cited, in accordance with accepted academic practice. No use, distribution or reproduction is permitted which does not comply with these terms.
*Correspondence: Wei Lu, bHV3NEBtYWlsLm5paC5nb3Y=
†These authors have contributed equally to this work