- Department of Psychiatry and Behavioral Neurobiology, University of Alabama at Birmingham, Birmingham, AL, United States
Testosterone can induce impulsivity, a behavioral impairment associated with various psychiatric illnesses. The molecular mechanisms associated with testosterone-induced impulsivity are unclear. Our earlier studies showed that supraphysiological doses of testosterone to rats induced impulsive behavior, impacted hypothalamic-pituitary-adrenal axis (HPA) and hypothalamic-pituitary-gonadal axis interactions, and altered α2A adrenergic receptors in prefrontal cortex (PFC). Owing to the importance of GABAergic system in impulsivity and memory, the present study examines whether testosterone-mediated impulsivity is associated with changes in the expression of Gamma-Aminobutyric Acid (GABA) A and B receptor subunit transcripts (Gabra1, Gabra2, Gabra2 transcript variant 2, Gabra3, Gabra4, Gabra5, Gabra6, Gabrb1, Gabrb2, Gabrb3, Gabrg1, Gabrg2, Gabrg3, Gabbr1, Gabbr2) in rat PFC, and whether testosterone influences GABAA receptor subunit organization. We studied GABA receptor functions by examining GABA receptor-mediated calcium/calmodulin-dependent kinase signaling genes (Calm1, Calm2, Calm3, Camk2a, Camk2b, Camk2g, Camk2d, Camk4) in the testosterone-induced impulsivity model. Rats were left untreated as controls (C), gonadectomized (GDX), or GDX and injected with supraphysiological doses of testosterone (T). Impulsive behavior was examined using the go/no-go paradigm. Gene expression was studied using qRT-PCR and GABAA subunit reorganization using cross correlation. Our findings show that expressions of select GABAA receptor subunits (Gabra3, Gabra5, Gabra6) were significantly upregulated in PFC of T group compared to GDX or C groups. GABAA receptor subunit organization was different in C, T, and GDX groups. Additionally, Camk4 expression was significantly downregulated in T compared to C group. Our findings suggest that specific GABAA receptor subunit expression, their reorganization, and Camk4-mediated functions may be associated with testosterone-mediated impulsivity.
Introduction
Impulsivity is described as decision-making or acting without regard to prior thinking. Being a non-unitary construct, impulsivity is often defined as a multidimensional concept related to maladaptive personality traits critical to many neuropsychiatric conditions. Attention-deficit hyperactivity disorder, mood disorders, addiction-related disorders, impulse-control disorders, non-suicidal self-injury, and suicidal behavior are some of the neuropsychiatric conditions that are significantly associated with impulsivity (Bakhshani, 2014; Liu et al., 2017). Despite its significance in neuropsychiatric illnesses, the underlying mechanisms associated with impulsivity are not clearly understood. Recent evidence suggests that increased levels of testosterone and impulsivity are highly correlated in humans (Wu et al., 2019). It has been shown that there is a significant correlation between increased testosterone level and suicide attempts in men (Stefansson et al., 2016). Past studies also suggest an association of increased use of androgen-enhancing drugs, known as anabolic-androgenic steroids, with psychopathologies, such as a blunted hypothalamic-pituitary-adrenal axis (HPA) response and increased risk of suicide attempts (Thiblin et al., 1999; Trenton and Currier, 2005; Melhem et al., 2016). In fact, we have earlier shown that testosterone not only increases impulsivity in rats, but also heightens the interaction of HPA and hypothalamic-pituitary-gonadal axis (HPG) axes in the brains of these rats (Ludwig et al., 2019). In addition, we found that the α2A adrenergic signaling pathway is significantly impacted in the prefrontal cortex (PFC) of rats given supraphysiological doses of testosterone, which was correlated with impulsivity behavior (Agrawal et al., 2019). Our study indicates that neurochemical changes may be central to testosterone-mediated impulsivity.
Gamma-Aminobutyric Acid (GABA) is the main inhibitory neurotransmitter in the mammalian central nervous system. In conjunction with excitatory glutamate, GABA is involved in balancing excitatory and inhibitory response, critical in proper brain functioning (Petroff, 2002). GABA binds to GABA receptors to mediate its functions. There are two main types of GABA receptors: (1) GABAA receptor subtypes, which are ligand-gated ion channels and (2) GABAB receptor subtype, which are G-protein-coupled receptors (Hayes et al., 2014). GABAA is the primary GABA receptor in the brain, which, due to its chloride ion channel activity, quickly hyperpolarizes the post-synaptic neurons, causing an inhibitory effect. GABAA receptors are pentameric, composed of α, β, γ, δ, and ρ subunits. They have been found to be localized in post-synaptic and extrasynaptic locations (Shrivastava et al., 2011; Hammond, 2015). Based on transcription patterns, GABAA subunits α1 and β2 are co-regulated (Goetz et al., 2007). GABA binds between the α and β subunits, and β subunits regulate ion selectivity of the GABAA receptor (Goetz et al., 2007). The γ2 subunit plays a role in anchoring the receptor to the synapse. Subunit composition determines the GABAA receptors’ conductance and deactivation rate (Goetz et al., 2007). On the other hand, GABAB receptors are composed of GABAB1 and GABAB2 subunits, forming a heterodimer. GABAB receptors localize pre-synaptically, post-synaptically, and potentially extrasynaptically. GABAB receptors have a slower response than GABAA receptors and play a role in regulating neurotransmission. GABAB1 and GABAB2 receptor subunits must be co-expressed to render a functional GABAB receptor (Mott, 2015).
Recent studies have shown that GABA may be involved in impulsive behavior. For example, it has been shown that impulsive choice or impulsive action can be associated with expression changes in GABA receptor subunits in rat orbitofrontal cortex (Ucha et al., 2019). Also, GABA level may predict individual differences in rash impulsivity (Boy et al., 2011). A past study examined gonadectomy (GDX) and testosterone treatment on GABA receptors in rats. An alteration in the GABAA/benzodiazepine receptors was reported in testosterone-treated animals (Svensson et al., 2000). 36Cl– uptake in synaptoneurosomes was increased in the testosterone-treated compared to control rats, suggesting that testosterone may increase GABAA receptor function in this group (Svensson et al., 2003). However, this study did not observe receptor subunit differences between the groups. Furthermore, whether impulsivity induced by testosterone is associated with GABA receptor subunits and their functions has not been studied.
In the present study, we hypothesize that testosterone will disrupt the GABA receptor signaling by increasing their expression in testosterone-treated (T) group compared to control (C) and gonadectomized (GDX) groups. GABAA α, β, and γ subunits were chosen for this study because they comprise the majority of GABAA receptors. Both subunits of GABAB receptors were studied (Shrivastava et al., 2011). We also tested whether testosterone influences GABAA subunit organization by correlating various GABAA receptor subunits in individual groups of rats, as subunit correlations have been found to be disrupted in the brains of subjects with psychiatric disorders such as depression and depressed individuals who died by suicide (Merali et al., 2004). Additionally, we examined the expression of calmodulin (Cal) and calcium/calmodulin-dependent (CaM) protein kinase 2 (CaMk2) as they are considered to be active downstream transducers of GABA receptor functions (Churn and DeLorenzo, 1998; Churn et al., 2002). Furthermore, CaM kinase 4 (CaMk4) gene was studied for its role in GABA receptor expression changes. These studies were performed under the supraphysiological influence of testosterone in PFC in testosterone-induced impulsivity rat model. The PFC was chosen because of its well-studied role in motor control (Robbins and Dalley, 2017; Sholler et al., 2020) decision making (Hiser and Koenigs, 2018; Saberi Moghadam et al., 2019), and emotional processing and regulation (Dixon et al., 2017). Our previous study also indicated that PFC plays a significant role in testosterone-mediated impulsivity (Agrawal et al., 2019).
Materials and Methods
Experimental Group Design, Behavioral Testing, and Tissue Collection
Rodent Impulsivity Model Preparation
A total of 30 Male Long Evans rats were received from Envigo Laboratories and maintained as described in our previous studies (Agrawal et al., 2019; Ludwig et al., 2019). They were individually housed in a reversed 14 h of light: 10 h of dark photoperiod. They were weighed daily and given food ad libitum. The rats were acclimatized for 5 days before starting the experiment. They were divided into a control (C) group and two experimental groups, both of which received gonadectomies to eliminate testosterone. The age of rats was ∼6 weeks at the time of gonadectomy. One of the experimental groups was given daily injections of excessive testosterone and is known as the testosterone (T) group. The group with the gonadectomy only is known as the gonadectomized (GDX) group. Experimental procedures were approved by the IACUC of the University of Alabama at Birmingham and all procedures were conducted in strict adherence to the National Institutes of Health (NIH) Guide for the Care and Use of Laboratory Animals.
Rats receiving a GDX or testosterone injections were subjected to the same protocol used previously in this lab (Agrawal et al., 2019; Ludwig et al., 2019). To summarize, rats received isoflurane as an anesthetic (5% induction, 1–3% maintenance), and their vitals were checked regularly to minimize the risk of cardiorespiratory failure. Rats also received carprofen (5 mg/kg, subcutaneous) and buprenorphine (0.1 mg/kg, subcutaneous) before incision. For the incision, the aseptic technique was followed. Rats’ abdomen were shaved, and betadine was used to cleanse. Then, a single transverse incision in the caudal abdomen was made, and using blunt forceps, the testicular fat pad on one side was pulled through the incision. Both testes and epididymis were removed using a hemostat and ligature for control. Monocryl sutures were used to close the incisions. During and after surgery, rats’ body temperatures were monitored using a heating pad. 24 h post-surgery, rats received a second subcutaneous injection of carprofen (5 mg/kg).
A schematic diagram showing the experimental protocol is mentioned in Figure 1. One day after surgery, GDX rats were randomly assigned to receive testosterone injections and form the testosterone (T) group. They were subcutaneously injected with testosterone propionate (7.5 mg/kg, dissolved in 0.1 mL corn oil). This dose is equivalent to a heavy steroid dose in humans, and has been used in previous studies (Wood et al., 2013; Cooper et al., 2014). Injections were given daily for a 24-week period. GDX rats, not receiving testosterone, received corn oil injections of equal volume and for the same duration. Rats were weighed every 2 weeks, and the daily dose of testosterone was adjusted for their weight.
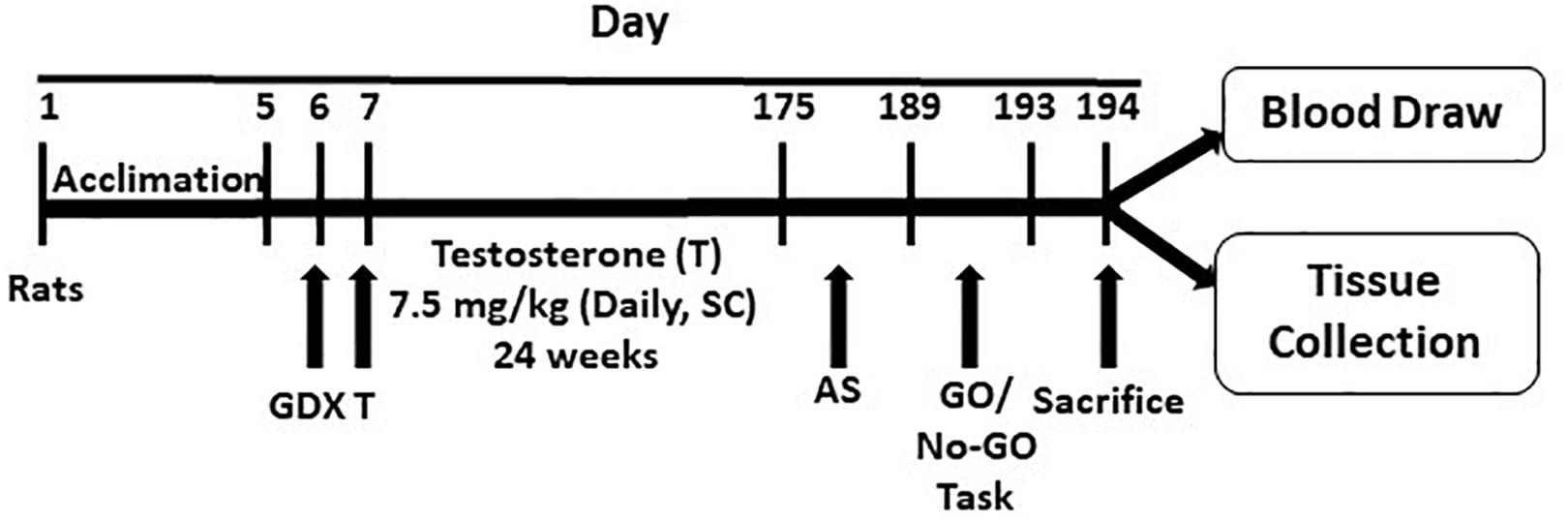
Figure 1. Schematic diagram showing the experimental protocol. The protocol is detailed in the text. GDX, gonadectomy; T, testosterone; AS, autoshaping; SC, subcutaneous.
Behavioral Testing
After 24 weeks of testosterone administration, rats were subjected to the go/no-go task paradigm to test their impulsive behavior. This paradigm is described in our previous study (Agrawal et al., 2019). Rats placed in sound-attenuated and ventilated chambers were autoshaped for 15 days to learn that pressing the lever in the chamber would result in the dispensing of 45 mg sucrose pellets. Rats who successfully learned (defined as pressing the lever at least 20 times during 30 min of the “go” phase, when the light was on) continued testing, and those who did not were removed from testing.
Rats that continued testing moved to the “go/no go” task phase of the experiment. Rats were to press the lever to dispense sucrose pellets during the “go” phase, which was signaled by a bright white light and continued with a house light. They were not to press the lever during the “no-go” phase, which was signaled by a red light and continued with no light. Each phase was 10 min, with 10 s timeouts between phases. In total, there were 4 “go” and 4 “no-go” phases which alternated for a total of an 80 m session. Rats underwent 3 sessions that were included in results, and they completed one warm-up session of this paradigm that was not included in results. The sessions were controlled by Med-PC IV® software ran on a computer. Impulsive behavior was defined as pressing the lever during the “no-go” phase. Impulsive behavior was indexed by the go/no-go ratio, which was the number of times the lever was pressed during the “go” phase compared to the number of times it was pressed during the “no-go” phase. A high ratio indicated low impulsive behavior, while a low ratio indicated high impulsive behavior. If variance in this ratio between the 3 sessions was <10% for any individual rat, results were recorded. Individual responses were averaged based on experimental group (C, T, and GDX).
Twenty-four hours after the last session, rats were decapitated, trunk blood was collected, and brains were flash frozen and stored at −80°C. The PFC was dissected out from 300 μm sections prepared from the frozen brain on a cryostat (Leica CM1950, Leica, Germany) and PFC was carved out precisely by scalpel using the Rat Brain Atlas and stored at −80°C until further analyses.
Testosterone Levels
Serum isolated from trunk blood was tested for testosterone levels using an ELISA kit (Abcam, MA, United States). One-way ANOVA and Student’s t-tests were performed between groups to determine variance and statistical significance. Data are presented as testosterone concentration values in ng/mL.
Expression Levels of GABA Receptor Subunits
Primer Design
Primers were designed using Rat Genomic Database (RGD) and NCBI BLAST search tool. Primer sequences were designed to target the 3′ end of the gene and accounted for all the transcript variants for each gene. Primers were designed to have low self-complementarity, low self-3′ complementarity, low GC%, and melt temperatures within 2°C of each other. A list of all primers used in the gene expression assay can be found in Table 1.
RNA Isolation and qPCR-Based Gene Expression Analysis of GABA Receptor Subunits and Signaling Genes in Rat PFC
RNA was isolated following TRIzol® (Life Technologies, United States) method as described earlier (Roy et al., 2017). RNA purity was determined by measuring the optical density with an absorbance ratio of 260/280 (NanoDrop 2000c, Thermo-Scientific, Waltham, MA, United States), and integrity was tested following denaturing agarose gel electrophoresis. All samples had 260/280 ratio >1.8 and demonstrated 28S:18S rRNA ratio of 2:1 on agarose gel.
The single-stranded cDNA was prepared following the previously described method (Timberlake et al., 2018). Following the preparation of mRNA specific first strand cDNA, relative transcript abundance of coding genes was measured with quantitative real-time PCR (Stratagene MxPro3005, La Jolla, CA, United States) method. With the help of EvaGreen chemistry (Applied Biological Material Inc., Canada), qPCR amplification for the specific gene was performed using gene-specific forward and reverse primers as mentioned in Table 1. Primers were designed for the following genes: GABAA receptor subunits α1–6 (Gabra1, Gabra2, Gabra2-transcript variant 2, Gabra3, Gabra4, Gabra5, Gabra6), GABAA receptor subunits β1–3 (Gabrb1, Gabrb2, Gabrb3), GABAA receptor subunits γ1–3 (Gabrg1, Gabrg2, Gabrg3), GABAB receptor subunits 1–2 (Gabbr1, Gabbr2), calmodulin genes 1–3 (Calm1, Calm2, Calm3), calcium/calmodulin-dependent protein kinase type II subunits α, β, γ, and δ (Camk2a, Camk2b, Camk2g, Camk2d), and calcium/calmodulin-dependent protein kinase type IV (Camk4). The possibility of primer dimer formation and secondary product amplification was ruled out by running template-free samples. Gapdh normalized gene expression values were used to determine the relative gene expression levels of individual transcripts following Livak’s ΔΔCt calculation method (Livak and Schmittgen, 2001).
GABAA Receptor Subunit Transcript Reorganization
GABAA receptor subunit mRNA reorganization was determined by correlating expression levels of various GABAA receptor subunits (Gabra1, Gabra2, Gabra2-transcript variant 2, Gabra3, Gabra4, Gabra5, Gabra6, Gabrb1, Gabrb2, Gabrb3, Gabrg1, Gabrg2, and Gabrg3) in C, T, and GDX groups independently using Pearson Product Moment Analysis.
Results
Serum Testosterone Levels
As can be seen in our prior study (Agrawal et al., 2019), average testosterone concentration for the testosterone (T) group (n = 5) was 11.6 ng/mL (SEM = 4.67), compared to the average concentration for the control (C) group (n = 6) of 1.63 ng/mL (SEM = 0.4), and the average concentration for the gonadectomized (GDX) group (n = 6) of 0 ng/mL (SEM = 0.0061). T group concentration was significantly higher than both C group (p = 0.04) and GDX group (p = 0.02). GDX group concentration was significantly lower than C group (p = 0.001).
Impulsive Behavior Testing Results
As detailed in our previous study, T group rats had significantly lower go/no-go ratios than those in the GDX and C groups (Agrawal et al., 2019). T group (n = 5) had a ratio of 0.54 (SEM = 0.035), C group (n = 6) had a ratio of 0.87 (SEM = 0.073), and GDX group (n = 6) had a ratio of 0.76 (SEM = 0.075). T group’s ratio was significantly lower than the ratio for C group (p = 0.004) and GDX group (p = 0.03). There was no significant difference between the ratios of C group and GDX group (p = 0.32).
GABA Receptor Gene Expression Analysis
Gene expression analysis was done in rat PFC to determine the effect of a supraphysiological dose of testosterone (T) on GABA receptor subunit genes and genes associated with calcium-dependent calmodulin kinase signaling pathway compared to rats with normal levels of testosterone (C) and rats with no testosterone due to gonadectomy (GDX).
Expression level changes of all GABAA and GABAB receptor subunit transcripts in PFC are presented in Figure 2. Fold changes between groups were standardized with C group as the reference (fold change = 1). Gabra3 fold change was 1.29 (SEM = 0.058) for T group and 1.10 (SEM = 0.035) for GDX group, compared to 1 (SEM = 0.28) for C group. T group’s Gabra3 expression was significantly upregulated compared to GDX (p = 0.008), but not significant compared to C group (p = 0.244). Gabra5 fold change was 1.71 (SEM = 0.18) for T group and 1.39 (SEM = 0.15) for GDX group, compared to 1 (SEM = 0.22) for C group. T group’s Gabra5 expression was significantly upregulated compared to C (p = 0.038), but not significant compared to GDX group (p = 0.24). Gabra6 fold change was 2.14 (SEM = 0.17) for T group and 1.10 (SEM = 0.19) for GDX group, compared to 1 (SEM = 0.40) for C group. T group’s Gabra6 expression was significantly upregulated compared to both GDX (p = 0.009) and C (p = 0.047).
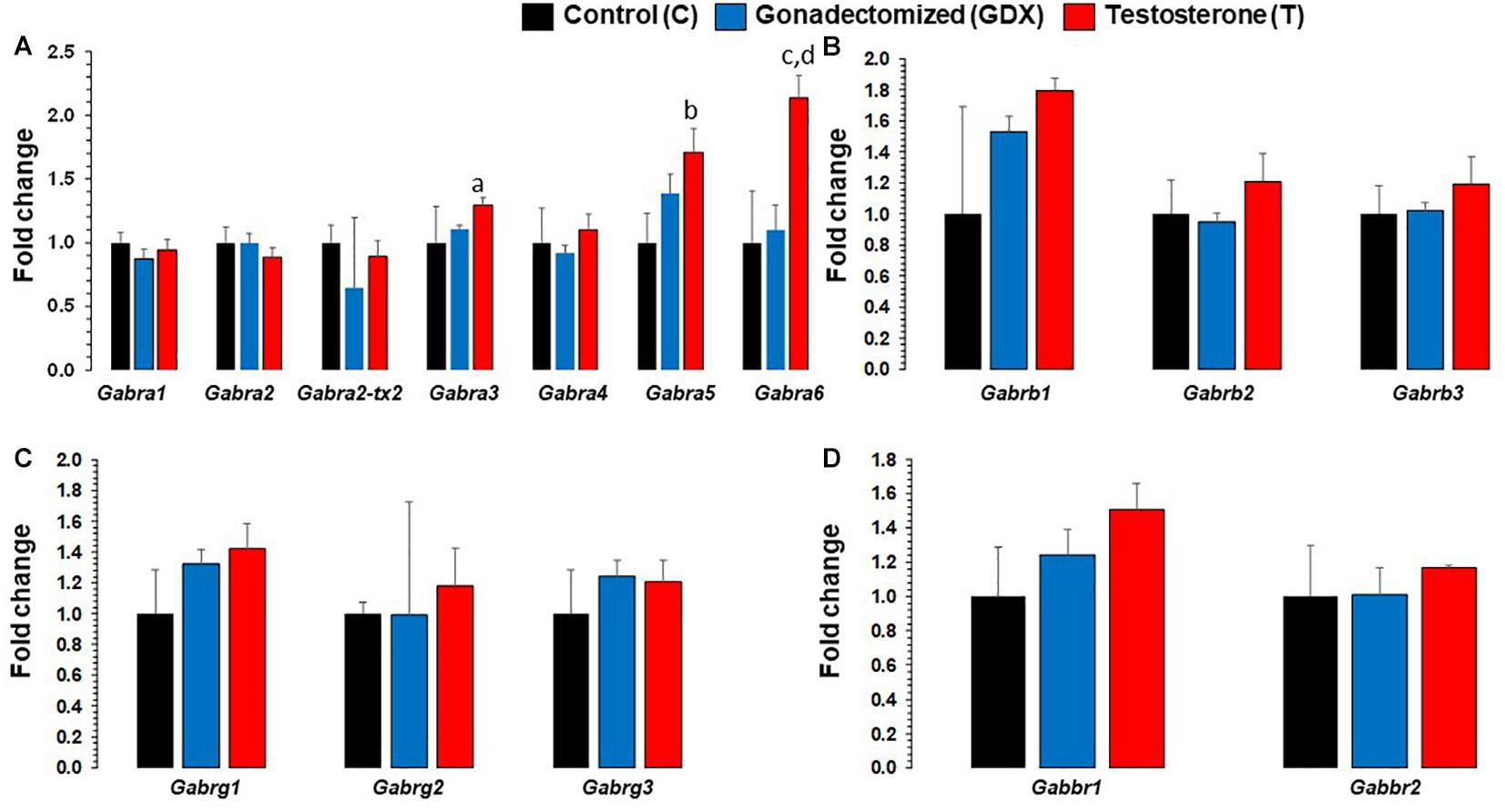
Figure 2. Bar diagram showing gene expression results for GABAA and GABAB receptor subunits in prefrontal cortex across control (C, n = 4), gonadectomized (GDX, n = 5), and testosterone (T, n = 4) groups. Data are the mean ± SEM. (A) Expression changes in GABAA α receptor subunits across T and GDX groups. The T rats displayed significantly upregulated Gabra3 mRNA transcript levels (ap = 0.0087) compared with the GDX group. Similar significant expression upregulation was seen for Gabra6 gene in T rats as compared to GDX rats (dp = 0.009). The Gabra5 (bp = 0.037) and Gabra6 (cp =0.047) were all found to be upregulated in the T group compared to the C group. No significant changes in Gabra1, Gabra2, Gabra2-tx2, Gabra4 were noticed when comparing T group of rats with GDX rats, nor when comparing T rats with C rats. (B) Expression levels of GABAA β receptor subunits across C, T, and GDX groups. No significant changes in Gabrb1, Gabrb2, and Gabrb3 were noted when comparing T group of rats with GDX rats, or T rats with C rats. (C) Expression levels of GABAA γ receptor subunits across C, T, and GDX groups. No significant differences in Gabrg1, Gabrg2, and Gabrg3 were noted when comparing T group of rats with GDX rats, or T rats with C rats. (D) Expression levels of GABAB receptor subunits across C, T, and GDX groups. No significant changes in Gabbr1 and Gabbr2 were noted when comparing T group of rats with GDX rats, or T rats with C rats.
Other GABAA receptor subunits (Gabra1, Gabra2, Gabra2-transcript variant 2, Gabra4, Gabrb1, Gabrb2, Gabrb3, Gabrg1, Gabrg2, and Gabrg3) were not significantly altered between groups. Neither of the GABAB receptor subunits (Gabbr1 and Gabbr2) were significantly different between groups.
Calmodulin and CaM Kinase Gene Expression Analysis
mRNA expression changes of all calmodulin and CaMK subunit genes in PFC are presented in Figure 3. Based on ANOVA, significant expression differences were found across groups in Camk4. Camk4 fold change was 0.55 (SEM = 0.222) for T group and 0.55 (SEM = 0.227) for GDX group, compared to 1 (SEM = 0.173) for C group. Camk4 expression was significantly downregulated in T group compared to C group (p = 0.02). Camk4 expression was also significantly downregulated in GDX group compared to C group (p = 0.03). No significant differences were found in Camk4 expression between T and GDX groups. Additionally, Calm3 expression was nearly significantly downregulated in T group compared to C group (p = 0.069), but not to GDX (p = 0.233). No significant differences were found in the expression levels of Calm1, Calm2, Camk2a, Camk2b, Camk2g, and Camk2d.
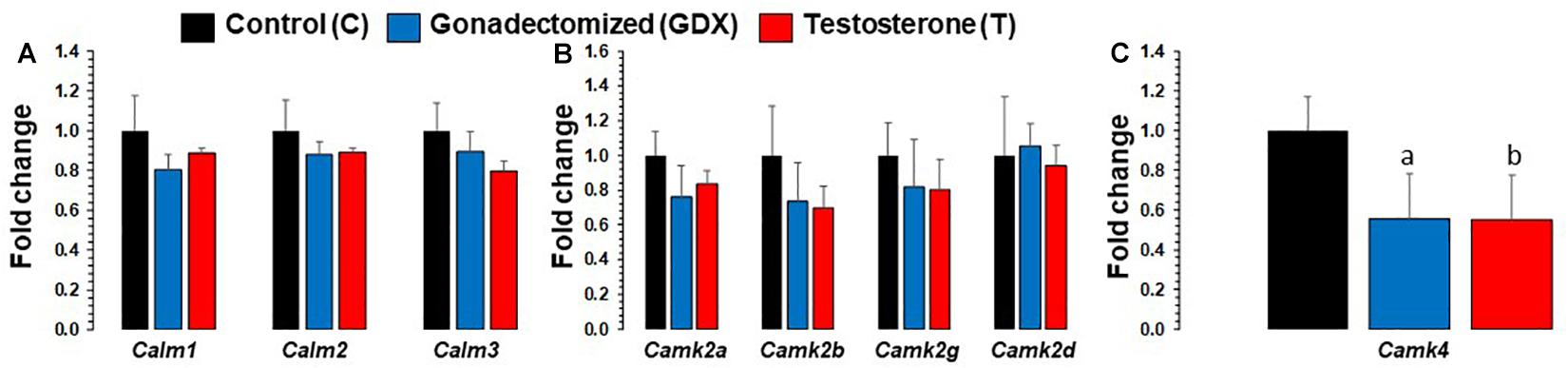
Figure 3. Bar diagram showing gene expression results for calmodulin and Calcium/calmodulin-dependent protein kinase subunits in prefrontal cortex across control (C, n = 4), gonadectomized (GDX, n = 5), and testosterone (T, n = 4) groups. Data are the mean ± SEM. (A) Expression levels of Calm genes across C, T, and GDX groups. No significant differences were noted in Calm1 and Calm2 genes, however, calm3 was nearly significant (p = 0.06) when comparing T rats to C rats. (B) Expression levels of Camk2 genes across C, T, and GDX groups. No significant differences were found in the following genes: Camk2a, Camk2b, Camk2g, and Camk2d when comparing T to GDX, T to C, or GDX to C. (C) Expression changes in Camk4 across T and GDX groups. One-way ANOVA found significant differences across groups in the Camk4 gene. The T rats displayed significantly downregulated Camk4 mRNA transcript levels (bp = 0.02) compared with the C group. Similar significant expression downregulation was seen for Camk4 in GDX rats as compared to C rats (ap = 0.03).
GABAA Receptor Subunit Transcript Organization
Figure 4 shows Pearson correlations for GABAA receptor subunit gene expression in the control group. Significant correlations were found in 17 different subunit combinations: Gabra3 and Gabra4, Gabra3 and Gabra5, Gabra4 and Gabra5, Gabra5 and Gabrb1, Gabra3 and Gabrb2, Gabra4 and Gabrb2, Gabra5 and Gabrb2, Gabrb1 and Gabrb2, Gabra3 and Gabrb3, Gabra4 and Gabrb3, Gabra2 and Gabrg1, Gabra2 and Gabrg2, Gabrg1 and Gabrg2, Gabra2 and Gabrg3, Gabra3 and Gabrg3, Gabrg1 and Gabrg3, and Gabrg2 and Gabrg3.
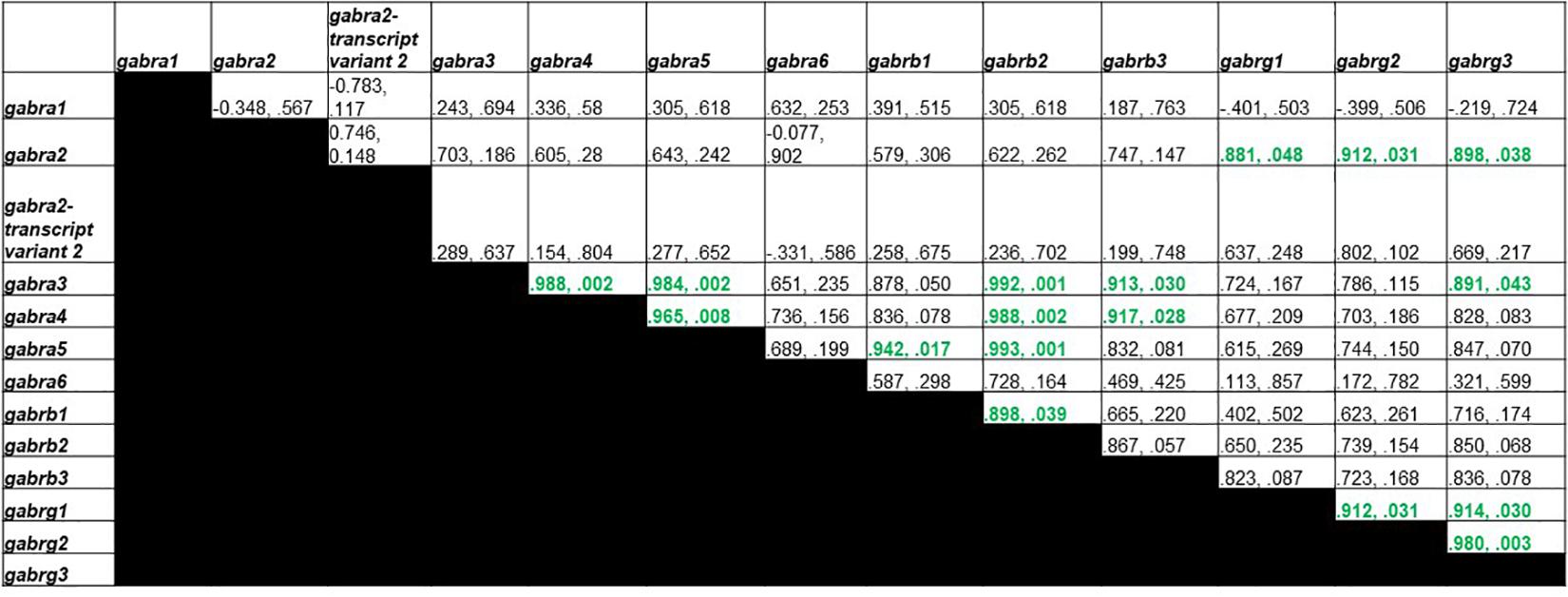
Figure 4. Pearson Correlations for C group GABAA receptor subunit gene expression. Data are presented as R and p. R is the correlation coefficient, and p is the p-value from student’s t-test. 17 significant correlations were found between Gabra3 and Gabra4, Gabra3 and Gabra5, Gabra4 and Gabra5, Gabra5 and Gabrb1, Gabra3 and Gabrb2, Gabra4 and Gabrb2, Gabra5 and Gabrb2, Gabrb1 and Gabrb2, Gabra3 and Gabrb3, Gabra4 and Gabrb3, Gabra2 and Gabrg1, Gabra2 and Gabrg2, Gabrg1 and Gabrg2, Gabra2 and Gabrg3, Gabra3 and Gabrg3, Gabrg1 and Gabrg3, and Gabrg2 and Gabrg3.
Figure 5 shows Pearson correlations for the T group GABAA receptor subunit gene expression. Significant correlations were found in 9 different subunit combinations: Gabra1 and Gabra4, Gabra2-transcript variant 2 and Gabra6, Gabra2-transcript variant 2 and Gabrb3, Gabra6 and Gabrb3, Gabra2-transcript variant 2 and Gabrg1, Gabra6 and Gabrg1, Gabrb2 and Gabrg2, Gabra6 and Gabrg3, and Gabrg1 and Gabrg3.
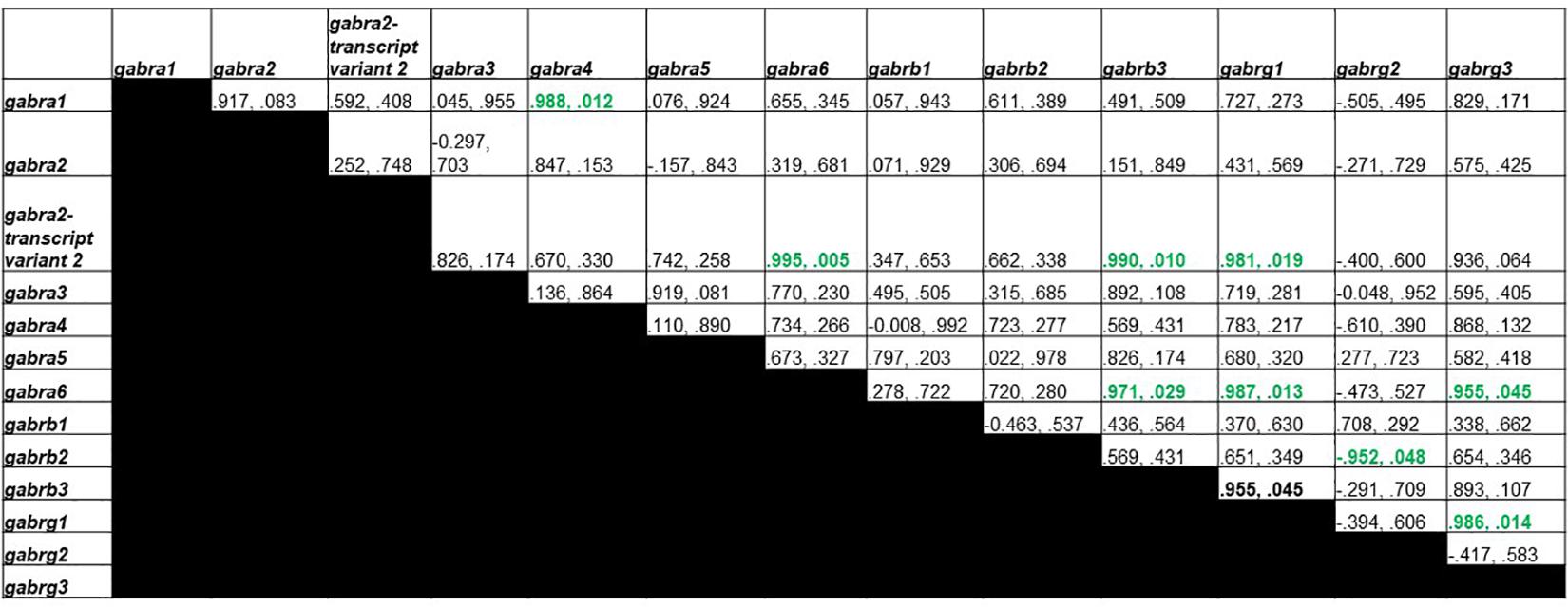
Figure 5. Pearson Correlations for T group GABAA receptor subunit gene expression. Data are presented as R and p. R is the correlation coefficient, and p is the p-value from student’s t-test. 9 significant correlations were found between Gabra1 and Gabra4, Gabra2-transcript variant 2 and Gabra6, Gabra2-transcript variant 2 and Gabrb3, Gabra6 and Gabrb3, Gabra2-transcript variant 2 and Gabrg1, Gabra6 and Gabrg1, Gabrb2 and Gabrg2, Gabra6 and Gabrg3, and Gabrg1 and Gabrg3.
Figure 6 shows Pearson correlations for GDX group GABAA receptor subunit gene expression. Significant correlations were found in 5 different subunit combinations: Gabra3 and Gabra4, Gabra4 and Gabra6, Gabra3 and Gabrb3, Gabra2 and Gabrg1, and Gabrb1 and Gabrg2.
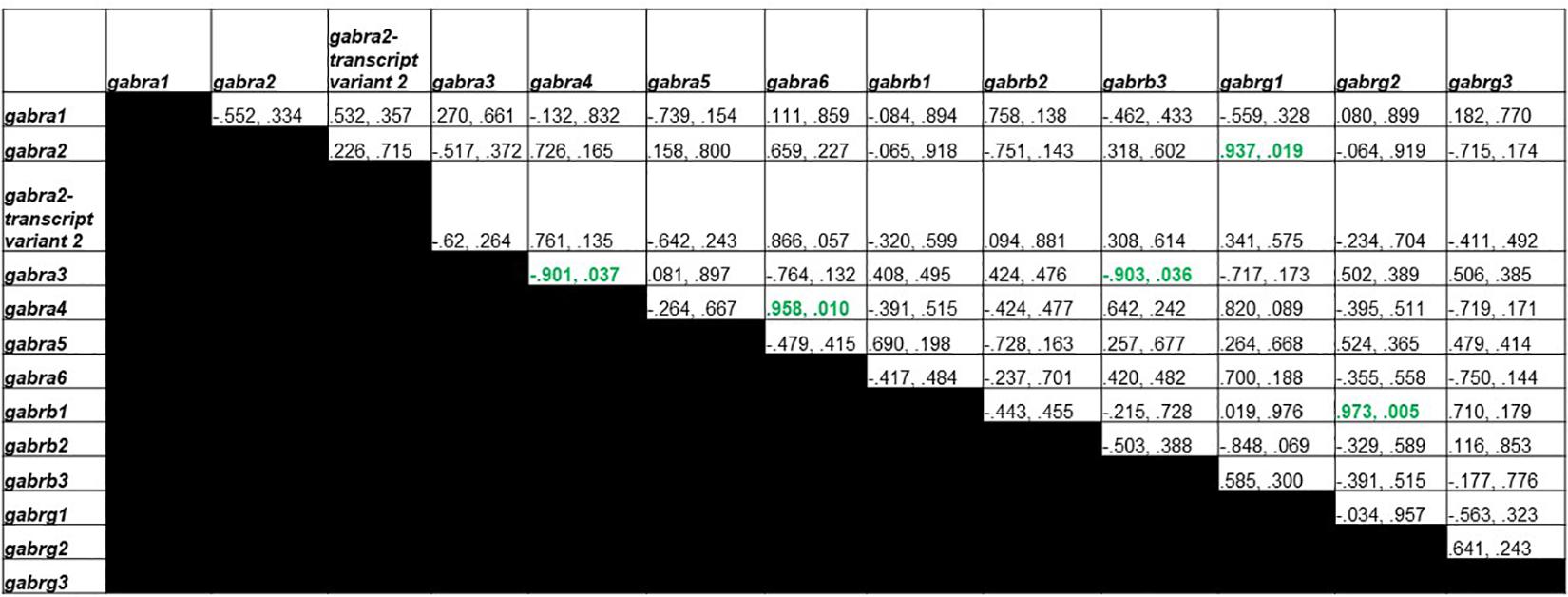
Figure 6. Pearson Correlations for GDX group GABAA receptor subunit gene expression. Data are presented as R and p. R is the correlation coefficient, and p is the p-value from student’s t-test. 5 significant correlations were found between Gabra3 and Gabra4, Gabra4 and Gabra6, Gabra3 and Gabrb3, Gabra2 and Gabrg1, and Gabrb1 and Gabrg2.
Discussion
In this study, we analyzed the effects of testosterone-induced impulsivity on GABAA and GABAB receptor subunit mRNA levels in rat PFC. As indicated in the results section, we found that only GABAA, but not GABAB receptor transcript levels, showed changes. Within GABAA receptors, testosterone had a subunit-specific effect on Gabra3, Gabra5, and Gabra6 transcript levels. We predict that changes in only GABAA but not in GABAB could be due to the underlying differences in receptor composition between these two receptors and their distinct mechanisms of interaction with specific ligands and consequent downstream functions (Hammond, 2015; Mott, 2015). GABAB receptors are inhibitory G-protein coupled receptors and are found in both pre- and post-synaptic locations (Wu and Sun, 2015). GABAA receptors are ionotropic, fast hyperpolarizing, and expressed throughout the brain. Of various GABAA receptor subunits, GABAA 4, 5, and 6 subunits are extrasynaptic (Wu and Sun, 2015). These receptors have a high GABA binding affinity and are persistently activated even at a low concentration of GABA, resulting in tonic inhibition (Farrant and Nusser, 2005). This tonic inhibition could be a compensatory mechanism shown in testosterone-induced impulsivity. Because testosterone is a hydrophobic steroid hormone, it is able to cross the Blood-Brain Barrier and target GABA receptors This effect could be localized to several brain regions by signaling molecules directing testosterone’s activity, or by increased binding affinity for location-specific receptors. Testosterone may target GABA receptors that are not present at the synapse itself but are still along the neuronal cell surface. Increased GABAA receptor activity in impulsivity is consistent with previous findings in rodent models. For example, a study found that increased GABAA receptor activity in the infralimbic PFC, rather than the prelimbic PFC, increased impulsive responding in rats (Murphy et al., 2012). Another study examined GABA receptors in gambling disorder and found that individuals with gambling disorder had higher levels of GABAA receptors in the right hippocampus compared to healthy controls (Mick et al., 2017). While this study focused on testosterone’s effect on the PFC due to the PFC’s well-studied role in impulsivity and our previous findings showing a PFC-specific alteration in noradrenergic signaling, it is important to note that other brain regions may also be involved in impulsivity. For instance, a study recently found a neural circuit connecting the ventral hippocampus to the PFC in food impulsivity inhibition (Hsu et al., 2018). Because our previous study indicated the localized effect that testosterone had on the PFC and not on the hippocampus or amygdala, we chose to continue focusing on the PFC in this study for testosterone-mediated alterations of other neurotransmitter receptor signaling pathways (Agrawal et al., 2019).
We observed that increased testosterone level was associated with altered expression of specific GABAA subunit mRNA expression. The reasons for the altered expression of specific subunit genes of GABAA receptors are not clear at the present time; however, several transcription factors have been found to be associated with promoter regions of these genes (Steiger and Russek, 2004). These include early growth response factors 1 and 3, Myc associated growth factor, and zinc binding protein 9 and 89. Whether these transcription factors influence these subunits needs to be further studied. In a recent study, we observed that testosterone interacts with the HPA axis through molecules that influence stress response (Ludwig et al., 2019). For example, corticotropin-releasing hormone (Crh) and FK506 binding protein 5 (Fkbp5) genes were significantly upregulated in GDX rats. Interestingly, there is a significant interaction between CRH and GABA receptor systems. It has been reported that pharmacological agents that influence GABA can profoundly impact CRH systems (Cullinan and Wolfe, 2000; Skelton et al., 2000; Stout et al., 2001; Gilmor et al., 2003). Also, CRH is uniquely expressed in glutamic acid decarboxylase (GAD)-positive neurons (Cullinan and Wolfe, 2000). GAD is an enzyme responsible for the conversion of glutamate to GABA. Several GABAA receptor subunits are altered within CRH neurons (Cullinan and Wolfe, 2000). Thus, the possibility of HPA axis-responsive genes and changes in specific subunits of GABA by testosterone cannot be ruled out. Whereas an association between CRH-GABA has been established under stressful conditions (Yan et al., 1998), whether this interaction plays a role in impulsivity needs to be tested.
Our study also assessed cross-correlations between GABAA receptor subunit mRNA levels in control (C), testosterone (T), and gonadectomized (GDX) groups. We found that while C group rats had 17 significant correlations, T rats had 9, and GDX had only 5. These differences in subunit composition indicate a loss of co-regulated genes in GDX group and testosterone partially restored this loss. Interestingly, studies have examined the organization of various GABA receptor subunits and found that in certain psychiatric conditions, such as depression and suicide, not only are mRNA expression altered in specific GABA receptor subunits, but inter-relations between various subunits differ (Brambilla et al., 2003; Merali et al., 2004; Poulter et al., 2010; Yin et al., 2016). Although these studies did not correlate GABA subunit organization with impulsivity, it is quite possible that altered stoichiometric organization could influence neuronal firing patterns and their timing, which may be consequential in impulsivity.
Protein phosphorylation is one of the main mechanisms of regulating GABA receptor functions. It has been shown that CamKII-dependent phosphorylation can increase GABA receptor binding, and thus modulate GABA receptor-mediated chloride ion channel activity (Churn and DeLorenzo, 1998). It has also been reported that CaMKII activation can lead to an increase in specific GABA receptor subunits (Churn et al., 2002). Calmodulin genes Calm1, Calm2, and Calm3 have been studied to produce identical calmodulin proteins. Calmodulin is a calcium-binding protein that plays a role in memory formation. The calcium/calmodulin dependent kinase IIa (Camk2a) has been found to play a role in integrating Ca2+ signals in dendritic spines (Chang et al., 2019), and increasing expression after long-term potentiation (Thomas et al., 1994). Camk2b is found to be more prevalent in sympathetic neurons (Ma et al., 2015), and to play a role in tethering the Camk2 protein complex to dendritic spines (Shen et al., 1998). While Camk2a and Camk2b are primarily found in the nervous system, Camk2g and Camk2d are found throughout the body (Gray and Heller Brown, 2014). Camk2g functions to transport calmodulin from the cell surface to the nucleus (Malik et al., 2014). Camk2d is predominantly found in cardiac tissue, and its expression alters during cardiomyocyte differentiation, heart failure, and ischemia (Gray and Heller Brown, 2014). Also, both calmodulin and a Camk2 inhibitor can block the potentiating effect of Ca2+ on Cl– current gated by GABAA receptors (Aguayo et al., 1998). Moreover, glutamatergic synaptic activity is controlled by GABAA receptors by inhibiting glutamate release via Ca2+/calmodulin-dependent signaling (Long et al., 2009). Although the relationship of GABAA receptors and CaMKIV is not well established, a histochemical study shows CaMKIV is expressed in a subgroup of GABAergic neurons in all layers of cortical interneurons of adult monkey area V1 in which parvalbumin was present (Lalonde et al., 2004). In this study, we examined the expression of calmodulin genes and various CaM kinases: Calm1, Calm2, Calm3, Camk2a, Camk2b, Camk2g, Camk2d, and Camk4. It was observed that Camk4 gene expression was significantly lower when comparing T rats to C rats and GDX rats to C rats. Additionally, Calm3 was lower when comparing T rats to C rats, but it could not reach significance level. It appears that Camk4 might be involved in differential regulation of Gabra3, Gabra5, and Gabra6 receptors. However, changes in Camk4 are rather surprising given that this kinase is not very well linked to GABAA receptor functioning (Houston et al., 2009). The possibility of GABA-independent functions of Camk4 cannot be ruled out.
Conclusion and Limitations
Altogether, this is the first GABAA and GABAB subunit gene expression analysis in an animal model of testosterone-induced impulsivity. We show that the impulsivity response may be GABAA subunit-specific when involving supraphysiological concentrations of testosterone. Also, the organization of GABAA receptor subunits is quite different between control, testosterone, and GDX rats. Further, this study shows that subunit-specific effects of testosterone may be associated with calmodulin/calcium-dependent kinases. Our study thus provides an interplay between testosterone, impulsivity and GABAergic functions. From a clinical perspective, GABAA receptors are more frequently used as therapeutic targets when treating disorders such as anxiety and epilepsy (Arin et al., 2018). In the future, it will be interesting to translate this study to a human level to examine if GABA receptor functions are altered during impulsivity, particularly in suicidal people where this interaction has been shown to play a critical role in attempted and completed suicide (Sher et al., 2017; Lenz et al., 2019). There were a few limitations to our study. This study did not have a group with a normal amount of testosterone (created by GDX + normal amount of testosterone). However, in the results section, testosterone level analyses from serum confirmed the following 3 groups: a testosterone-depleted GDX group, a group with supraphysiological levels of testosterone (T+) and another group with physiological levels of testosterone (C). Thus, the C rats were used for normal level of testosterone. One caveat of the study is that the results were derived from the transcript (mRNA) levels of GABAA receptor subunits and CaM kinases and not from protein levels. Thus, there is a possibility that changes in transcription may not necessarily reflect changes in protein. Similarly, CaM kinase activity was not studied. However, as mentioned above, based on mRNA expression levels, Poulter et al. (2010) showed that GABAA receptor organization was altered in the brain of depressed subjects. These investigators convincingly argued that the relative mRNA abundance of GABAA receptor subunits would be a mechanism that ensured proportional abundance of protein. In addition, Brooks-Kayal et al. (1999) demonstrated that subunit mRNA levels correlated closely with receptor pharmacology within individual dentate granule cells, which could be similar to those predicted by studies of recombinant receptors. Nevertheless, our present study needs to be confirmed at the protein level to reach a definite conclusion. In addition, though the most common GABAA receptors were chosen to study, other subunits such as δ and ρ could also be studied in the future.
Data Availability Statement
The raw data supporting the conclusions of this article will be made available by the authors, without undue reservation.
Ethics Statement
The experimental procedures were approved by the IACUC of the University of Alabama at Birmingham.
Author Contributions
JA performed the experiments and analyzed the data. YD conceptualized the idea. JA and YD co-wrote the manuscript. Both authors contributed to the article and approved the submitted version.
Funding
The research was supported by grants from the National Institute of Mental Health (R01MH082802, R01MH101890, R01MH107183, and R01MH118884) to YD. The sponsoring agency had no role in the study design, collection, analysis, interpretation of data, and in the writing of the manuscript. Funds received for open access publication.
Conflict of Interest
The authors declare that the research was conducted in the absence of any commercial or financial relationships that could be construed as a potential conflict of interest.
Acknowledgments
We would like to acknowledge Dr. Yoshino Yuta for his help in the PCR experiments and Dr. Birgit Ludwig for preparing the animals and behavioral analysis.
References
Agrawal, J., Ludwig, B., Roy, B., and Dwivedi, Y. (2019). Chronic testosterone increases impulsivity and influences the transcriptional activity of the Alpha-2A adrenergic receptor signaling pathway in rat brain. Mol. Neurobiol. 56, 4061–4071. doi: 10.1007/s12035-018-1350-z
Aguayo, L. G., Espinoza, F., Kunos, G., and Satin, L. S. (1998). Effects of intracellular calcium on GABAA receptors in mouse cortical neurons. Pflügers Archiv. 435, 382–387. doi: 10.1007/s004240050527
Arin, B., Martin, W., Anne Kerstin, L., and Richard, W. O. (2018). GABAA Receptor Physiology and Pharmacology. Oxford: Oxford University Press.
Bakhshani, N.-M. (2014). Impulsivity: a predisposition toward risky behaviors. Int. J. High Risk Behav. Addict. 3:e20428. doi: 10.5812/ijhrba.20428
Boy, F., Evans, C. J., Edden, R. A., Lawrence, A. D., Singh, K. D., Husain, M., et al. (2011). Dorsolateral prefrontal gamma-aminobutyric acid in men predicts individual differences in rash impulsivity. Biol. Psychiatry 70, 866–872. doi: 10.1016/j.biopsych.2011.05.030
Brambilla, P., Perez, J., Barale, F., Schettini, G., and Soares, J. C. (2003). GABAergic dysfunction in mood disorders. Mol. Psychiatry 8, 721–737. doi: 10.1038/sj.mp.4001362
Brooks-Kayal, A. R., Schumate, M. D., Jin, H., Lin, D. D., Rikhter, T. Y., Hollway, K. L., et al. (1999). Human neuronal γ-aminobutyric acidA receptors: coordinated subunit mRNA expression and functional correlates in individual dentate granule cells. J. Neurosci. 19, 8312–8318. doi: 10.1523/JNEUROSCI.19-19-08312
Chang, J.-Y., Nakahata, Y., Hayano, Y., and Yasuda, R. (2019). Mechanisms of Ca2+/calmodulin-dependent kinase II activation in single dendritic spines. Nat. Commun. 10:2784. doi: 10.1038/s41467-019-10694-z
Churn, S. B., and DeLorenzo, R. J. (1998). Modulation of GABAergic receptor binding by activation of calcium and calmodulin-dependent kinase II membrane phosphorylation. Brain Res. 809, 68–76. doi: 10.1016/S0006-8993(98)00834-8
Churn, S. B., Rana, A., Lee, K., Parsons, J. T., De Blas, A., and Delorenzo, R. J. (2002). Calcium/calmodulin-dependent kinase II phosphorylation of the GABAA receptor α1 subunit modulates benzodiazepine binding. J. Neurochem. 82, 1065–1076. doi: 10.1046/j.1471-4159.2002.01032.x
Cooper, S. E., Goings, S. P., Kim, J. Y., and Wood, R. I. (2014). Testosterone enhances risk tolerance without altering motor impulsivity in male rats. Psychoneuroendocrinology 40, 201–212. doi: 10.1016/j.psyneuen.2013.11.017
Cullinan, W. E., and Wolfe, T. J. (2000). Chronic stress regulates levels of mRNA transcripts encoding β subunits of the GABAA receptor in the rat stress axis. Brain Res. 887, 118–124. doi: 10.1016/S0006-8993(00)03000-6
Dixon, M. L., Thiruchselvam, R., Todd, R., and Christoff, K. (2017). Emotion and the prefrontal cortex: an integrative review. Psychol. Bull. 143, 1033–1081. doi: 10.1037/bul0000096
Farrant, M., and Nusser, Z. (2005). Variations on an inhibitory theme: phasic and tonic activation of GABAA receptors. Nat. Rev. Neurosci. 6, 215–229. doi: 10.1038/nrn1625
Gilmor, M. L., Skelton, K. H., Nemeroff, C. B., and Owens, M. J. (2003). The effects of chronic treatment with the mood stabilizers valproic acid and lithium on corticotropin-releasing factor neuronal systems. J. Pharmacol. Exp. Therap. 305, 434–439. doi: 10.1124/jpet.102.045419
Goetz, T., Arslan, A., Wisden, W., and Wulff, P. (2007). GABA(A) receptors: structure and function in the basal ganglia. Prog. Brain Res. 160, 21–41. doi: 10.1016/S0079-6123(06)60003-4
Gray, C. B. B., and Heller Brown, J. (2014). CaMKIIdelta subtypes: localization and function. Front. Pharmacol. 5:15. doi: 10.3389/fphar.2014.00015
Hammond, C. (2015). “Chapter 9 – The ionotropic GABAA receptor,” in Cellular and Molecular Neurophysiology (Fourth Edition), ed. C. Hammond (Boston, MA: Academic Press), 199–219.
Hayes, D. J., Jupp, B., Sawiak, S. J., Merlo, E., Caprioli, D., and Dalley, J. W. (2014). Brain γ-aminobutyric acid: a neglected role in impulsivity. Eur. J. Neurosci. 39, 1921–1932. doi: 10.1111/ejn.12485
Hiser, J., and Koenigs, M. (2018). The multifaceted role of the ventromedial prefrontal cortex in emotion, decision making, social cognition, and psychopathology. Biol. Psychiatry 83, 638–647. doi: 10.1016/j.biopsych.2017.10.030
Houston, C. M., He, Q., and Smart, T. G. (2009). CaMKII phosphorylation of the GABAA receptor: receptor subtype- and synapse-specific modulation. J. Physiol. 587, 2115–2125. doi: 10.1113/jphysiol.2009.171603
Hsu, T. M., Noble, E. E., Liu, C. M., Cortella, A. M., Konanur, V. R., Suarez, A. N., et al. (2018). A hippocampus to prefrontal cortex neural pathway inhibits food motivation through glucagon-like peptide-1 signaling. Mol. Psychiatry 23, 1555–1565. doi: 10.1038/mp.2017.91
Lalonde, J., Lachance, P. E. D., and Chaudhuri, A. (2004). Monocular enucleation induces nuclear localization of calcium/calmodulin-dependent protein Kinase IV in cortical interneurons of adult monkey area V1. J. Neurosci. 24, 554–564. doi: 10.1523/jneurosci.1668-03.2004
Lenz, B., Röther, M., Bouna-Pyrrou, P., Mühle, C., Tektas, O. Y., and Kornhuber, J. (2019). The androgen model of suicide completion. Prog. Neurobiol. 172, 84–103. doi: 10.1016/j.pneurobio.2018.06.003
Liu, R. T., Trout, Z. M., Hernandez, E. M., Cheek, S. M., and Gerlus, N. (2017). A behavioral and cognitive neuroscience perspective on impulsivity, suicide, and non-suicidal self-injury: meta-analysis and recommendations for future research. Neurosci. Biobehav. Rev. 83, 440–450. doi: 10.1016/j.neubiorev.2017.09.019
Livak, K. J., and Schmittgen, T. D. (2001). Analysis of relative gene expression data using real-time quantitative PCR and the 2(-Delta Delta C(T)) Method. Methods 25, 402–408. doi: 10.1006/meth.2001.1262
Long, P., Mercer, A., Begum, R., Stephens, G. J., Sihra, T. S., and Jovanovic, J. N. (2009). Nerve terminal GABAA receptors activate Ca2+/calmodulin-dependent signaling to inhibit voltage-gated Ca2+ influx and glutamate release. J. Biol. Chem. 284, 8726–8737. doi: 10.1074/jbc.M805322200
Ludwig, B., Roy, B., and Dwivedi, Y. (2019). Role of HPA and the HPG axis interaction in testosterone-mediated learned helpless behavior. Mol. Neurobiol. 56, 394–405. doi: 10.1007/s12035-018-1085-x
Ma, H., Li, B., and Tsien, R. W. (2015). Distinct roles of multiple isoforms of CaMKII in signaling to the nucleus. Biochim. Biophys. Acta 1853, 1953–1957. doi: 10.1016/j.bbamcr.2015.02.008
Malik, Z. A., Stein, I. S., Navedo, M. F., and Hell, J. W. (2014). Mission CaMKIIγ: shuttle calmodulin from membrane to nucleus. Cell 159, 235–237. doi: 10.1016/j.cell.2014.09.023
Melhem, N. M., Keilp, J. G., Porta, G., Oquendo, M. A., Burke, A., Stanley, B., et al. (2016). Blunted HPA axis activity in suicide attempters compared to those at high risk for suicidal behavior. Neuropsychopharmacology 41, 1447–1456. doi: 10.1038/npp.2015.309
Merali, Z., Du, L., Hrdina, P., Palkovits, M., Faludi, G., Poulter, M. O., et al. (2004). Dysregulation in the suicide brain: mRNA expression of corticotropin-releasing hormone receptors and GABA(A) receptor subunits in frontal cortical brain region. J. Neurosci. 24, 1478–1485. doi: 10.1523/JNEUROSCI.4734-03.2004
Mick, I., Ramos, A. C., Myers, J., Stokes, P. R., Chandrasekera, S., Erritzoe, D., et al. (2017). Evidence for GABA-A receptor dysregulation in gambling disorder: correlation with impulsivity. Addict. Biol. 22, 1601–1609. doi: 10.1111/adb.12457
Mott, D. (2015). “Chapter 11 – The metabotropic GABAB receptors,” in Cellular and Molecular Neurophysiology (Fourth Edition), ed. C. Hammond (Boston, MA: Academic Press), 245–267.
Murphy, E. R., Fernando, A. B. P., Urcelay, G. P., Robinson, E. S. J., Mar, A. C., Theobald, D. E. H., et al. (2012). Impulsive behaviour induced by both NMDA receptor antagonism and GABAA receptor activation in rat ventromedial prefrontal cortex. Psychopharmacology 219, 401–410. doi: 10.1007/s00213-011-2572-1
Petroff, O. A. C. (2002). Book review: GABA and glutamate in the human brain. Neuroscientist 8, 562–573. doi: 10.1177/1073858402238515
Poulter, M., Du, L., Zhurov, V., Palkovits, M., Faludi, G., Merali, Z., et al. (2010). Altered organization of GABAA receptor mRNA expression in the depressed suicide brain. Front. Mol. Neurosci. 3:3. doi: 10.3389/neuro.02.003.2010
Robbins, T. W., and Dalley, J. W. (2017). “Chapter 7 – Impulsivity, risky choice, and impulse control disorders: animal models,” in Decision Neuroscience, eds J.-C. Dreher and L. Tremblay (San Diego, CA: Academic Press), 81–93.
Roy, B., Dunbar, M., Shelton, R. C., and Dwivedi, Y. (2017). Identification of MicroRNA-124-3p as a putative epigenetic signature of major depressive disorder. Neuropsychopharmacology 42, 864–875. doi: 10.1038/npp.2016.175
Saberi Moghadam, S., Samsami Khodadad, F., and Khazaeinezhad, V. (2019). An algorithmic model of decision making in the human brain. Basic Clin. Neurosci. 10, 443–449. doi: 10.32598/bcn.9.10.395
Shen, K., Teruel, M. N., Subramanian, K., and Meyer, T. (1998). CaMKIIbeta functions as an F-actin targeting module that localizes CaMKIIalpha/beta heterooligomers to dendritic spines. Neuron 21, 593–606. doi: 10.1016/s0896-6273(00)80569-3
Sher, L., Grunebaum, M. F., Burke, A. K., Chaudhury, S., Mann, J. J., and Oquendo, M. A. (2017). Depressed multiple-suicide attempters—a high-risk phenotype. Crisis 38, 367–375. doi: 10.1027/0227-5910/a000475
Sholler, D. J., Merritt, C. R., Davis-Reyes, B. D., Golovko, G., Anastasio, N. C., and Cunningham, K. A. (2020). Inherent motor impulsivity associates with specific gene targets in the rat medial prefrontal cortex. Neuroscience 435, 161–173. doi: 10.1016/j.neuroscience.2020.03.045
Shrivastava, A. N., Triller, A., and Sieghart, W. (2011). GABA(A) receptors: post-synaptic co-localization and cross-talk with other receptors. Front. Cell. Neurosci. 5:7. doi: 10.3389/fncel.2011.00007
Skelton, K. H., Nemeroff, C. B., Knight, D. L., and Owens, M. J. (2000). Chronic administration of the triazolobenzodiazepine alprazolam produces opposite effects on corticotropin-releasing factor and urocortin neuronal systems. J. Neurosci. 20, 1240–1248.
Stefansson, J., Chatzittofis, A., Nordstrom, P., Arver, S., Asberg, M., and Jokinen, J. (2016). CSF and plasma testosterone in attempted suicide. Psychoneuroendocrinology 74, 1–6. doi: 10.1016/j.psyneuen.2016.08.009
Steiger, J. L., and Russek, S. J. (2004). GABAA receptors: building the bridge between subunit mRNAs, their promoters, and cognate transcription factors. Pharmacol. Therap. 101, 259–281. doi: 10.1016/j.pharmthera.2003.12.002
Stout, S. C., Owens, M. J., Lindsey, K. P., Knight, D. L., and Nemeroff, C. B. (2001). Effects of sodium valproate on corticotropin-releasing factor systems in rat brain. Neuropsychopharmacology 24, 624–631. doi: 10.1016/S0893-133X(00)00243-8
Svensson, A. I., Åkesson, P., Engel, J. A., and Söderpalm, B. (2003). Testosterone treatment induces behavioral disinhibition in adult male rats. Pharmacol. Biochem. Behav. 75, 481–490. doi: 10.1016/S0091-3057(03)00137-0
Svensson, A. I., Berntsson, A., Engel, J. A., and Söderpalm, B. (2000). Disinhibitory behavior and GABAA receptor function in serotonin-depleted adult male rats are reduced by gonadectomy. Pharmacol. Biochem. Behav. 67, 613–620. doi: 10.1016/S0091-3057(00)00403-2
Thiblin, I., Runeson, B., and Rajs, J. (1999). Anabolic androgenic steroids and suicide. Ann. Clin. Psychiatry 11, 223–231. doi: 10.1023/a:1022313529794
Thomas, K. L., Laroche, S., Errington, M. L., Bliss, T. V. P., and Hunt, S. P. (1994). Spatial and temporal changes in signal transduction pathways during LTP. Neuron 13, 737–745. doi: 10.1016/0896-6273(94)90040-X
Timberlake, M. II, Prall, K., Roy, B., and Dwivedi, Y. (2018). Unfolded protein response and associated alterations in toll-like receptor expression and interaction in the hippocampus of restraint rats. Psychoneuroendocrinology 89, 185–193. doi: 10.1016/j.psyneuen.2018.01.017
Trenton, A. J., and Currier, G. W. (2005). Behavioural manifestations of anabolic steroid use. CNS Drugs 19, 571–595. doi: 10.2165/00023210-200519070-00002
Ucha, M., Roura-Martinez, D., Contreras, A., Pinto-Rivero, S., Orihuel, J., Ambrosio, E., et al. (2019). Impulsive action and impulsive choice are differentially associated with gene expression variations of the GABAA Receptor Alfa 1 Subunit and the CB1 receptor in the lateral and medial orbitofrontal cortices. Front. Behav. Neurosci. 13:22. doi: 10.3389/fnbeh.2019.00022
Wood, R. I., Armstrong, A., Fridkin, V., Shah, V., Najafi, A., and Jakowec, M. (2013). ‘Roid rage in rats? Testosterone effects on aggressive motivation, impulsivity and tyrosine hydroxylase. Physiol. Behav. 11, 6–12. doi: 10.1016/j.physbeh.2012.12.005
Wu, C., and Sun, D. (2015). GABA receptors in brain development, function, and injury. Metab. Brain Dis. 30, 367–379. doi: 10.1007/s11011-014-9560-1
Wu, Y., Shen, B., Liao, J., Li, Y., Zilioli, S., and Li, H. (2019). Single dose testosterone administration increases impulsivity in the intertemporal choice task among healthy males. Horm. Behav. 118:104634. doi: 10.1016/j.yhbeh.2019.104634
Yan, X. X., Baram, T. Z., Gerth, A., Schultz, L., and Ribak, C. E. (1998). Co-localization of corticotropin-releasing hormone with glutamate decarboxylase and calcium-binding proteins in infant rat neocortical interneurons. Exp. Brain Res. 123, 334–340. doi: 10.1007/s002210050576
Yin, H., Pantazatos, S. P., Galfalvy, H., Huang, Y.-Y., Rosoklija, G. B., Dwork, A. J., et al. (2016). A pilot integrative genomics study of GABA and glutamate neurotransmitter systems in suicide, suicidal behavior, and major depressive disorder. Am. J. Med. Genet. Part B Neuropsychiatr. Genet. 171, 414–426. doi: 10.1002/ajmg.b.32423
Keywords: impulsivity, testosterone, GABAA receptor, rodent model, transcript level
Citation: Agrawal J and Dwivedi Y (2020) GABAA Receptor Subunit Transcriptional Regulation, Expression Organization, and Mediated Calmodulin Signaling in Prefrontal Cortex of Rats Showing Testosterone-Mediated Impulsive Behavior. Front. Neurosci. 14:600099. doi: 10.3389/fnins.2020.600099
Received: 28 August 2020; Accepted: 14 October 2020;
Published: 06 November 2020.
Edited by:
Ashok Kumar, University of Florida, United StatesReviewed by:
Anilkumar Pillai, Augusta University, United StatesJosipa Vlainic, Rudjer Boskovic Institute, Croatia
Copyright © 2020 Agrawal and Dwivedi. This is an open-access article distributed under the terms of the Creative Commons Attribution License (CC BY). The use, distribution or reproduction in other forums is permitted, provided the original author(s) and the copyright owner(s) are credited and that the original publication in this journal is cited, in accordance with accepted academic practice. No use, distribution or reproduction is permitted which does not comply with these terms.
*Correspondence: Yogesh Dwivedi, ydwivedi@uab.edu